Altered Cortical Cholinergic Network in Parkinson’s Disease at Different Stage: A Resting-State fMRI Study
- 1Department of Radiology, The First Affiliated Hospital of Wenzhou Medical University, Wenzhou, China
- 2Department of Radiology, The Second Affiliated Hospital, Zhejiang University School of Medicine, Hangzhou, China
- 3Department of Neurology, The Second Affiliated Hospital, Zhejiang University School of Medicine, Hangzhou, China
The cholinergic system is critical in Parkinson’s disease (PD) pathology, which accounts for various clinical symptoms in PD patients. The substantia innominata (SI) provides the main source of cortical cholinergic innervation. Previous studies revealed cholinergic-related dysfunction in PD pathology at early stage. Since PD is a progressive disorder, alterations of cholinergic system function along with the PD progression have yet to be elucidated. Seventy-nine PD patients, including thirty-five early-stage PD patients (PD-E) and forty-four middle-to-late stage PD patients (PD-M), and sixty-four healthy controls (HC) underwent brain magnetic resonance imaging and clinical assessments. We employed seed-based resting-state functional connectivity analysis to explore the cholinergic-related functional alterations. Correlation analysis was used to investigate the relationship between altered functional connectivity and the severity of motor symptoms in PD patients. Results showed that both PD-E and PD-M groups exhibited decreased functional connectivity between left SI and left frontal inferior opercularis areas and increased functional connectivity between left SI and left cingulum middle area as well as right primary motor and sensory areas when comparing with HC. At advanced stages of PD, functional connectivity in the right primary motor and sensory areas was further increased. These altered functional connectivity were also significantly correlated with the Unified Parkinson’s Disease Rating Scale motor scores. In conclusion, this study illustrated that altered cholinergic function plays an important role in the motor disruptions in PD patients both in early stage as well as during the progression of the disease.
Introduction
Parkinson’s disease (PD), a chronic and progressive movement disorder, has been recognized as a heterogeneous syndrome (Zhang et al., 2005; Reich and Savitt, 2019). The pathological and neurotransmitter basis of PD is not all dopaminergic, other non-dopaminergic neurotransmitter systems are involved (Sethi, 2008), which leads to the heterogeneous clinical manifestations, not only including classic motor symptoms but involving differed extents of non-motor symptoms. Over the past decades, a major pathological emphasis has been placed on the disruption of dopaminergic system; however, it was found that the damage of non-dopaminergic system precedes the development of dopaminergic pathology (Braak et al., 2003), and has a profound influence on disease progression (Langston, 2006). Therefore, the investigation of the non-dopaminergic neurotransmitter system could provide a better understanding of the mechanism of PD.
Cholinergic system is a kind of major non-dopaminergic neurotransmitter system. Previous studies revealed that the abnormal function of cholinergic system is critical in the PD pathology (Mallet et al., 2019), which could account for various clinical symptoms in PD, including both motor symptoms (e.g., gait impairment, balance dysfunction and falls) (Bohnen et al., 2009; Bohnen and Albin, 2011; Lord et al., 2011; Yarnall et al., 2011; Rochester et al., 2012; Dalrymple et al., 2021) and non-motor symptoms (e.g., cognition impairment and visual hallucinations) (Yarnall et al., 2011; Kim et al., 2017; Lee et al., 2018). The substantia innominata (SI) in the basal forebrain is the major sources of cholinergic projections in the brain (Mesulam and Geula, 1988; Li et al., 2014). The loss of SI neurons represents cortical cholinergic deficits (Perry et al., 1985). According to the classic PD pathology model, basal forebrain is a main target of the α-synuclein accumulation (Braak et al., 2003), and the α-synuclein accumulation in which area simultaneously occurs with the accumulation in the substantia nigra at the early stage of PD (Lee et al., 2018). A positron emission tomography (PET) study of cerebral acetylcholinesterase demonstrated that cholinergic dysfunction occurs in the early course of PD (Hughes et al., 1992; Latt et al., 2009). This evidence suggests that the pathology of early PD involves the cholinergic-related dysfunction. Given that PD is a progressive disorder, patients at advanced stages seem to have a significantly faster disease progression (Zhao et al., 2010) and show a high incidence of balance dysfunction (Latt et al., 2009). These heterogeneous clinical symptoms indicate that the degenerative mechanisms of the cholinergic system may be different during PD progression, which have yet to be elucidated.
Advanced magnetic resonance imaging (MRI) technology provides an avenue to explore the cholinergic function in PD patients. Currently, MRI studies have found a piece of evidence indicating the abnormal cholinergic function in PD patients. A whole brain voxel-based morphometry study has found that the gray matter density of SI was reduced in PD patients and it was associated with gait impairment (specially reduced gait speed) as well as balance dysfunction (Dalrymple et al., 2021). Other studies using resting-state functional MRI (rs-fMRI) revealed that in PD patients, altered cholinergic network of SI was significantly correlated with cognitive performance (Kim et al., 2017; Lee et al., 2018). However, these studies mainly focused on the specific PD population, such as PD patients at early stage or patients before the surgical stage. Researchers argue that functional alterations likely precede structural atrophy and examination of cerebral functional connectivity may be essential to understanding the etiologies of many neuropsychiatric disease (Liang et al., 2011; Guan et al., 2019). Low-frequency fluctuations of resting-state blood oxygenation level-dependent (BOLD) signal reflect connectivity between functionally related brain regions (Fox and Raichle, 2007). So resting-state functional connectivity (rsFC) can be used to evaluate altered relationships between the SI and particular areas of the whole brain thus defining brain regions related to the severity of motor symptoms. Earlier studies of rsFC have mainly focused on the role of cholinergic function in cognition (Kim et al., 2017; Lee et al., 2018). Taken together, considering the progressive characteristics of PD as we mentioned above, exploring the cholinergic function of PD patients at different stages would help us understand the pathophysiological mechanism with PD progression better.
On the basis of a previous study (George et al., 2011), we firstly segmented bilateral SI in the individual high-resolution structural images and defined them as seeds in the following rsFC calculation. We aimed to explore the altered cholinergic function in different stages of PD patients via the measure of functional connectivity of SI and investigate the relationships between aberrant SI connectivity and the disease severity. We hypothesized that with the progression of disease, PD patients’ SI-FC would be disrupted and associated with the disease severity.
Materials and Methods
Participants
All participants were recruited from the Department of Neurology, Second Affiliated Hospital of Zhejiang University and this study was approved by the Medical Ethics Committee of The Second Affiliated Hospital of Zhejiang University School of Medicine and the ethical approval number was (2017) Ethical Approval Study No. 008. Written informed consent was obtained from all participants before enrollment in the study. We excluded participants with a history of anticholinergic drugs, a history of neurologic or psychiatric disorders, brain trauma, or general exclusion criteria for MR scanning and analyzing. Specifically, seven normal controls and eight patients with PD were excluded for the following reasons: (1) with significant motion artifact during scanning, n = 6; (2) with severe brain atrophy or enlarged ventricles, n = 4; (3) with poor coregistration results, n = 2; (4) with incomplete demographic information, n = 3. After exclusion, 79 patients with PD and 64 healthy controls (HC) were included in this study. PD was diagnosed by a senior neurologist (BZ) according to the United Kingdom PD Society Brain Bank criteria (Hughes et al., 1992). For PD patients who were under antiparkinsonian treatment, MRI scanning and clinical assessments were performed in the morning after withdrawing all antiparkinsonian drugs overnight (at least 12 h) (on “drug-off status”). Basic demographic information, including age, gender, education duration, drug state and neurologic, and psychiatric scales including Hoehn-Yahr stage, Unified Parkinson’s Disease Rating Scale (UPDRS) and Montreal Cognitive Assessment (MoCA) score were obtained from all PD patients. UPDRS motor scores were divided into subscores of axial symptoms (items 27–30). According to Hoehn-Yahr stage, PD patients were divided into two groups: 35 patients with Hoehn-Yahr stage ≤ 1.5 were grouped into early-stage PD group (PD-E) and 44 patients with Hoehn-Yahr stage ≥ 2 were grouped into middle-to-late stage PD group (PD-M) (Li et al., 2020). For HC, basic demographic information and MoCA score were recorded.
MRI Data Acquisition
All participants were scanned on a 3.0-Tesla MRI scanner (GE Discovery 750) equipped with an 8-channel head coil. During MRI scanning, the head was stabilized using restraining foam pads, and earplugs were provided to reduce the noise during scanning. Structural T1 images were acquired using a fast spoiled gradient recalled sequence: repetition time = 7.336 ms; echo time = 3.036 ms; inversion time = 450 ms; flip angle = 11°; field of view = 260 × 260 mm2; matrix = 256 × 256; slice thickness = 1.2 mm; 196 continuous sagittal slices. Rs-fMRI images were acquired using gradient recalled echo-echo planar imaging sequence: repetition time = 2,000 ms; echo time = 30 ms; flip angle = 77°; field of view = 240 × 240 mm2; matrix = 64 × 64; slice thickness = 4 mm; slice gap = 0 mm; 38 interleaved axial slices. During MRI scanning, subjects were instructed to remain awake with their eyes closed and not to move or focus on a specific thought.
Seed Definition and Normalization
The bilateral SI were manually drawn on the coronal T1-weighted MRI images by a radiologist who was blinded to the participants’ identity according to the method provided by George et al. (2011). Specifically, the SI was drawn at three consecutive gapless 1.2 mm-thick slices on T1-weighted coronal images reformatted to be perpendicular to the anterior commissure (AC)-posterior commissure (PC) line. The three consecutive sections analyzed were located at the level of the crossing of the AC, the level where the AC might be uncrossed, and the level of the emergence of the AC from the temporal lobe. The boundaries of the SI were as follows: the dorsal border was the ventral aspect of the globus pallidus, the ventral border was the base of the brain containing the anterior perforated space, the medial border was operationally defined by a vertical line extending from the ventrolateral border of the bed nucleus of the stria terminalis to the base of the brain, and the lateral border extended to the medial aspect of the putamen. The SI delineation of each section was shown in Figure 1.
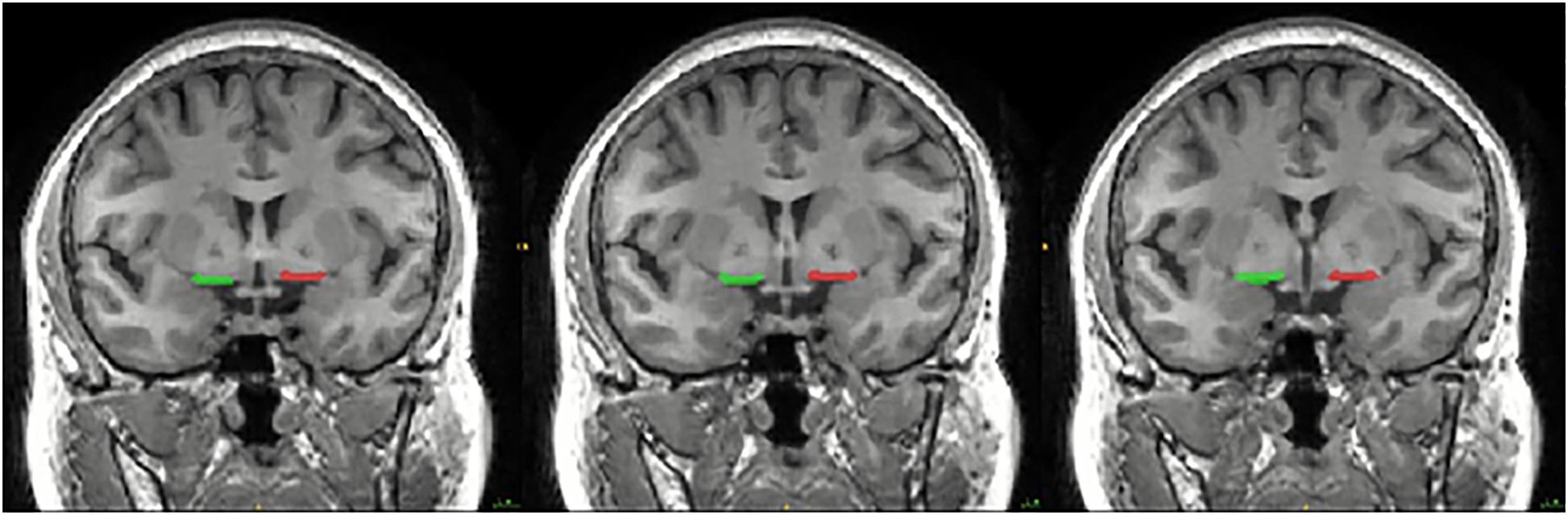
Figure 1. Coronal slice of the MRI image showing the location of the substantia innominate (SI) used in the seed-based resting-state functional connectivity (rsFC) analysis. The SI was drawn at three consecutive gapless 1.2 mm-thick slices on T1-weighted coronal images reformatted to be perpendicular to the anterior commissure (AC)-posterior commissure (PC) line. The three consecutive sections analyzed were located at the level of the crossing of the AC, the level where the AC might be uncrossed, and the level of the emergence of the AC from the temporal lobe. The boundaries of the SI were as follows: the dorsal border was the ventral aspect of the globus pallidus, the ventral border was the base of the brain containing the anterior perforated space, the medial border was operationally defined by a vertical line extending from the ventrolateral border of the bed nucleus of the stria terminalis to the base of the brain, and the lateral border extended to the medial aspect of the putamen.
The bilateral SI normalization was conducted using the VBM8 toolbox1 implemented in SPM8.2 All native T1-weighted images were normalized to the standard Montreal Neurological Institute (MNI) space. The corresponding normalization parameters were then applied to the bilateral SI, and therefore native SI was transformed into standard MNI space. After the SI normalization, a group-based probability map of bilateral SI was generated, and a threshold of 0.4 was used to obtain the binary SI mask (de Flores et al., 2017).
MRI Data Preprocessing and Functional Connectivity Analysis
The rs-fMRI data preprocessing was performed using the standard pipeline in the Data Processing and Analysis for (Resting-State) Brain Imaging suite (see text footnote 2) (Yan et al., 2016), which included first 10 volumes removing, slice timing, realignment, the nuisance covariates regression (Friston 24 head motion parameters, white matter, and cerebrospinal fluid signal), spatial normalization through structure images, smoothing with a gaussian kernel of 6 × 6 × 6 mm3 full width at half maximum, temporal band-pass filtering (0.01–0.1 Hz), linear detrending, and scrubbing. No subject showed apparent head motion over 2 mm (transformation) and/or 2°(rotation). The bilateral SI mask in MNI space was used as seed, respectively, and a seed-based functional connectivity in whole brain was performed. Fisher’s r-to-z transformation was applied to improve data’s normality for parametric statistical analysis.
Statistical Analysis
Statistical analyses of demographic and clinical data were performed using SPSS 20.0 statistical software. Categorical variables were assessed using chi-square tests. The one-sample Kolmogorov-Smirnov test was used to check the data’s normality. Normally distributed continuous variables were assessed using independent sample t test or analysis of variance (ANOVA). Non-parametric data were assessed using Wilcoxon rank-sum test and Kruskal-Wallis test. LSD correction was performed for the multiple comparisons in post hoc analyses. Two-tailed p < 0.05 was regarded as significant.
To locate the brain regions with significant difference in functional connectivity with SI among the three groups, one-way analysis of covariance (ANCOVA) was performed by using the statistical analysis tool of DPABI (Yan et al., 2016). Age, sex, and education duration were incorporated as covariates. Gaussian Random Field (GRF) correction (voxel p = 0.001, cluster p = 0.05, two-tailed) was used for multivoxel comparisons. Then, the mean FC of each individual in these significantly differed regions was extracted to perform the group comparisons as well as post hoc analyses. Least-Significant Difference (LSD) correction was used to correct the multiple comparisons in post hoc analyses. Two-tailed p < 0.05 was regarded as significant.
To test whether functional connectivity in brain regions showing significant group difference was correlated with the disease severity indicated by motor symptoms, partial correlation analysis was then conducted to evaluate the relationship between the FC and raw scores of UPDRS III, controlling age, gender and education duration as covariates. Bonferroni correction was performed for the multiple comparisons in post hoc analyses. Statistical significance was set at p < 0.05.
Results
Demographic and Clinical Characteristics
Demographic and clinical characteristics of all participants were shown in Table 1. No significant differences in gender (p = 0.140), education duration (p = 0.359) and MoCA score (p = 0.555) were observed between PD-E, PD-M and HC. No significant differences in disease duration (p = 0.275) and drug state (p = 0.813) were observed between PD-E and PD-M. And the details of drug state of each PD patient were shown in Supplementary Table 1, none of them have a history of anticholinergic drugs. The age (p = 0.027) of PD-M is older than PD-E. Hoehn-Yahr stage (p < 0.001) and UPDRS III scores (p < 0.001) of PD-M were significantly higher than PD-E.
Comparative Analysis of Functional Connectivity Between SI and the Rest of Brain Regions
Brain regions showing significant difference of functional connectivity between left SI and the rest of brain among three groups were shown in Figure 2A, and the comparisons of functional connectivity between groups were shown in Figure 2B. Anatomical location and post hoc comparison results of altered functional connectivity in significant brain regions were shown in Table 2. Compared with HC, both PD-E and PD-M groups showed decreased functional connectivity in left frontal inferior opercularis areas, partly extending to the left insula (cluster 1), and increased functional connectivity in the left cingulum middle area (cluster 2) and right primary motor and sensory areas (cluster 3). Interestingly, functional connectivity in the right primary motor and sensory areas (cluster 3) continued to increase in PD-M group when compared with PD-E group. These results indicated that even in the early stages of PD, cortical cholinergic denervation has occurred in left frontal inferior opercularis areas (cluster 1), and cholinergic hyperactivity has occurred in the left cingulum middle area (cluster 2) and right primary motor and sensory areas (cluster 3). And at advanced stages of PD, cholinergic hyperactivity in the right primary motor and sensory areas would develop further. There was no difference of functional connectivity between right SI and the rest of brain among three groups.
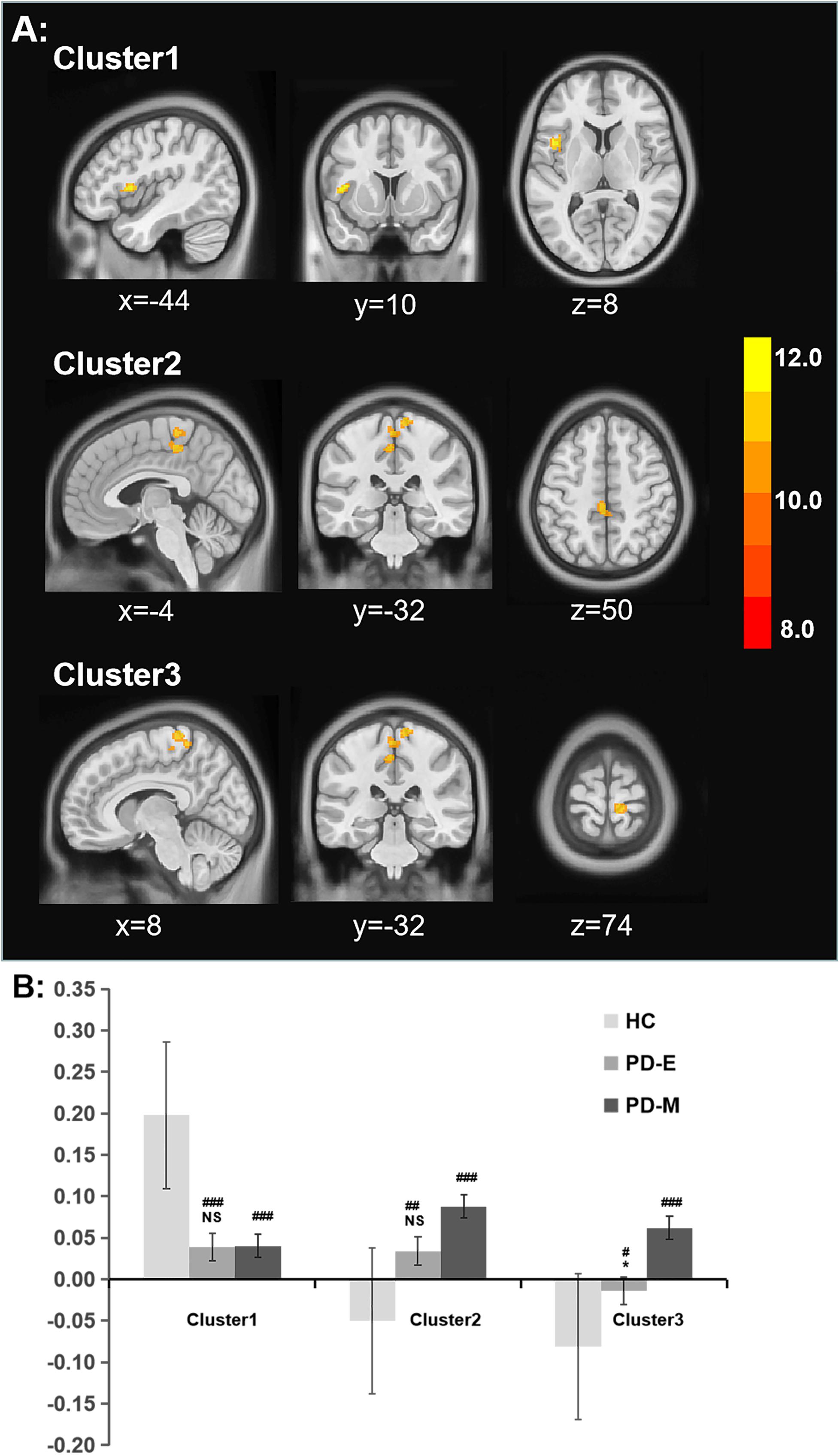
Figure 2. (A) Significant alterations of rsFC between the left SI and whole brain among all groups. (B) The bar plot of comparisons of functional connectivity between groups. *p < 0.05 vs the PD-M group, #p < 0.05; ##p < 0.01; and ###p < 0.001 vs the HC group. rsFC, resting-state functional connectivity; SI, substantia innominate.
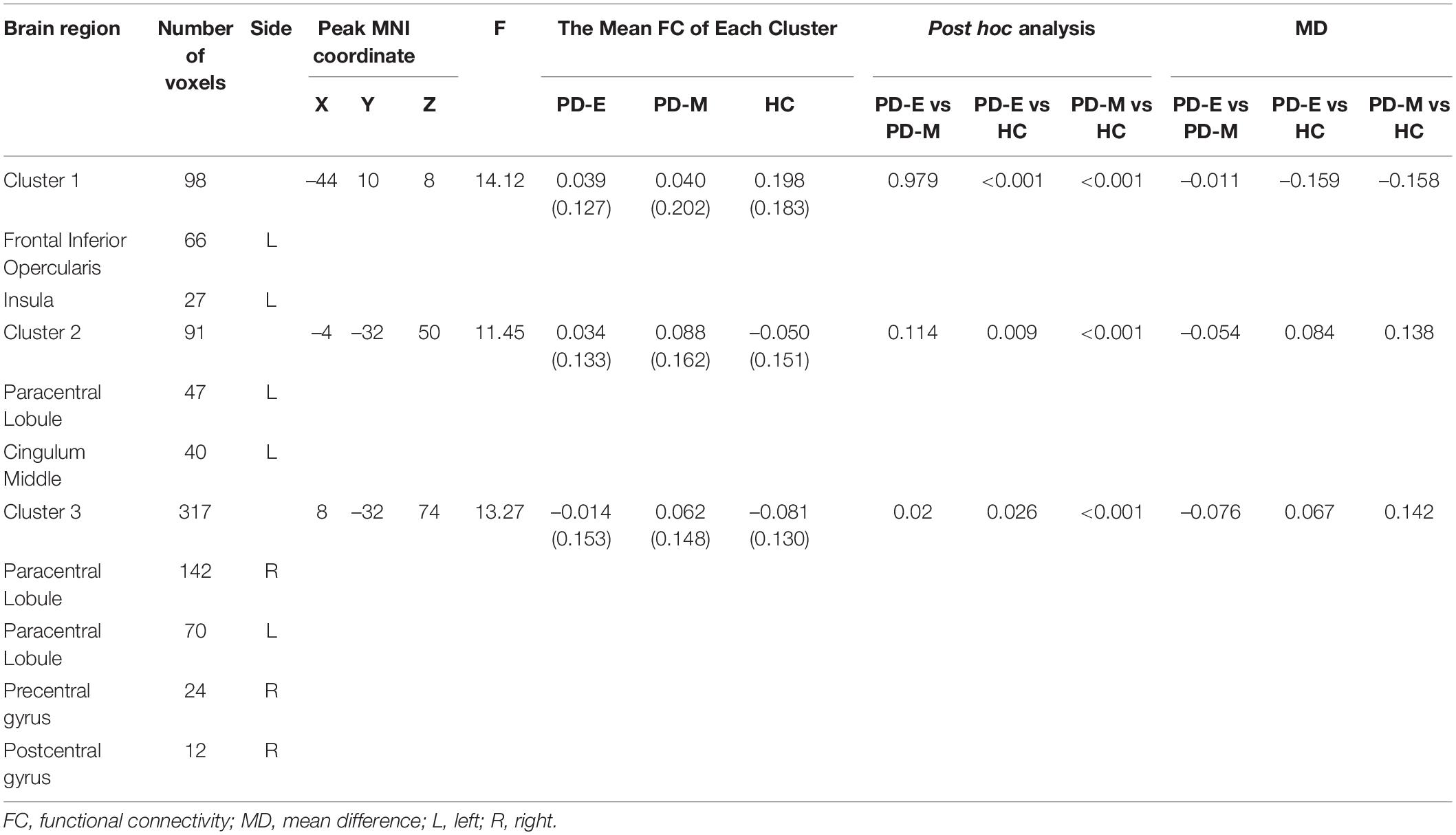
Table 2. Anatomical location and post hoc comparison results of altered functional connectivity in significant brain regions.
Correlation Analysis of UPDRS III Scores and Resting State Functional Connectivity
The results of correlation analyses were shown in Figure 3. Partial correlation analysis showed that decreased functional connectivity in left frontal inferior opercularis and insula area (cluster 1) was negatively correlated with UPDRS III scores (r = –0.336, p < 0.001) and subscores of axial symptoms (r = –0.261, p = 0.002). Increased functional connectivity in left cingulum middle area (cluster 2) and right primary motor and sensory areas (cluster 3) was positively correlated with UPDRS III scores (r = 0.315, p < 0.001, and r = 0.325, p < 0.001, respectively) and subscores of axial symptoms (r = 0.342, p < 0.001, and r = 0.355, p < 0.001, respectively).
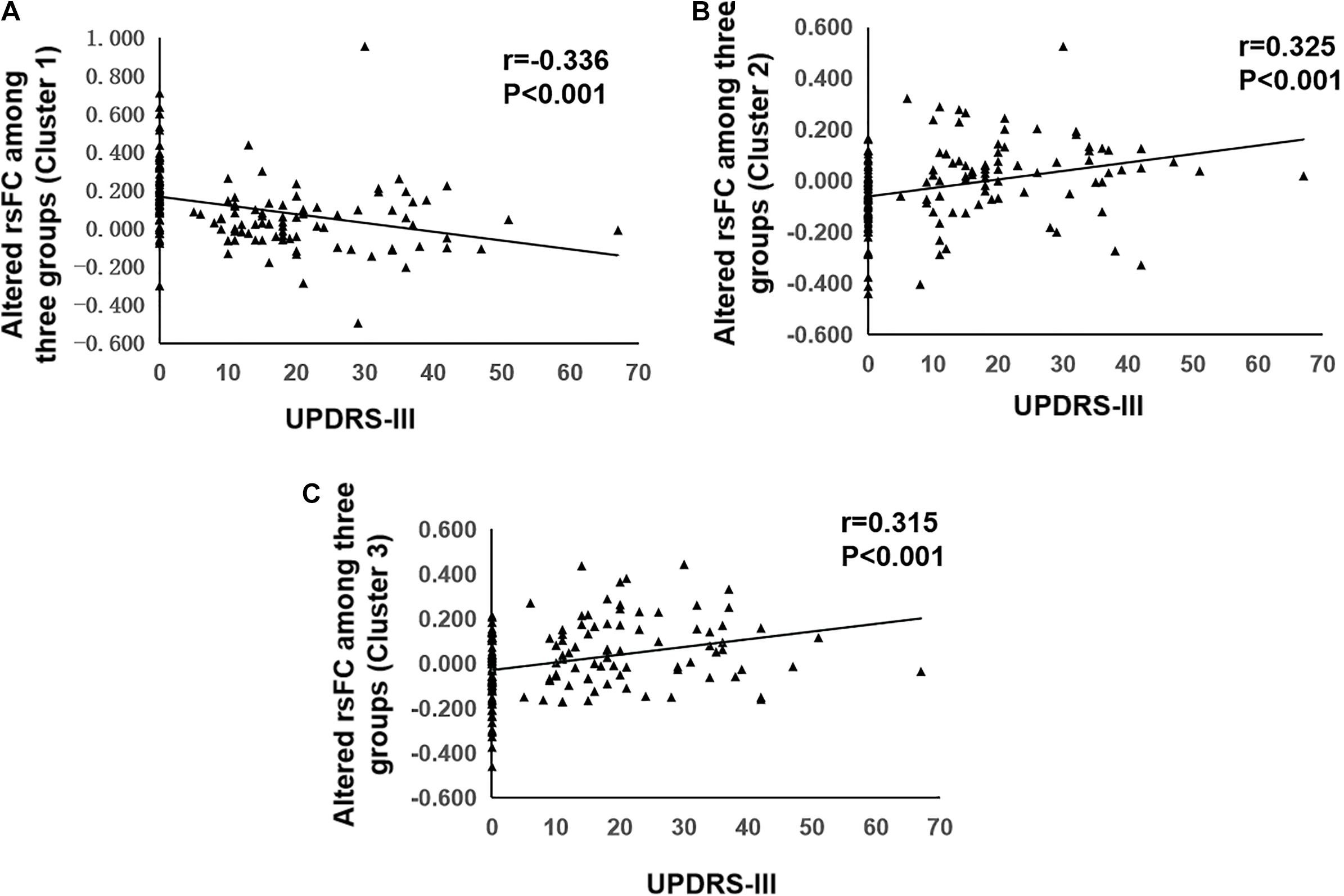
Figure 3. Correlation analysis of UPDRS-III and resting state functional connectivity. (A) Correlation analysis of UPDRS-III and altered rsFC of Cluster 1. (B) Correlation analysis of UPDRS-III and altered rsFC of Cluster 2. (C) Correlation analysis of UPDRS-III and altered rsFC of Cluster 3. rsFC, resting-state functional connectivity; UPDRS-III, part III of the Unified Parkinson’s Disease Rating Scale.
Discussion
The main findings of the present study were as follows: (1) The decreased functional connectivity in left frontal inferior opercularis areas, partly extending to the left insula (cluster 1), and increased functional connectivity in the left cingulum middle area (cluster 2) and right primary motor and sensory areas (cluster 3) were both shown in PD-E and PD-M groups when compared with HC; (2) at advanced stages of PD, functional connectivity in the right primary motor and sensory areas (cluster 3) was further increased; (3) decreased functional connectivity in left frontal inferior opercularis and insula area (cluster 1) was negatively correlated with UPDRS III scores and subscores of axial symptoms. And increased functional connectivity in left cingulum middle area (cluster 2) and right primary motor and sensory areas (cluster 3) was positively correlated with UPDRS III scores and subscores of axial symptoms.
Compared with the HC, we found that PD patients showed decreased functional connectivity between left SI and left frontal inferior opercularis, indicating the cholinergic denervation in the frontal inferior opercularis. A PET study found the neuroinflammation of SI in patients with REM sleep behavior disorder, which would lead to cortical cholinergic dysfunction in the frontal inferior opercularis (Staer et al., 2020). Frontal inferior opercularis plays a key role in postural and gait control, and an impairment of this area might lead to abnormal postural and gait control. Previous studies showed that the disruption of the projection from the SI to frontal inferior opercularis may result to the deficiency in the information processing from the temporoparietal cortex to the frontal cortex, which may cause errors in anticipatory postural adjustment and gait difficulties (Takakusaki, 2017; Vastik et al., 2017). And a MRI study showed that the decreased cortical thickness in frontal inferior opercularis was associated with motor symptoms (e.g., gait impairment) in PD (Herman et al., 2014; Vastik et al., 2017). This evidence indicated that the disrupted function of frontal inferior opercularis driving by the cholinergic degeneration was related to the motor difficulties. In this study, we found that the cortical cholinergic denervation in left frontal inferior opercularis was negatively associated with UPDRS motor scores and subscores of axial symptoms, which further supported that the cholinergic dysfunction in frontal inferior opercularis was associated with the severity of motor symptoms in PD patients.
In this study, we found increased functional connectivity between SI and cingulum middle areas both in PD-E and PD-M patients, which indicated the cholinergic hyperactivity in cingulum middle areas in PD patients. The cingulate gyrus is an important component of the limbic system which has rich distribution and intensity of acetylcholinesterase containing fiber (Mesulam et al., 1984). Previous study found the functional connectivity increases in the cingulate gyrus in PD patients with mild cognitive impairment (Zhan et al., 2018), which was in line with our findings. Former studies revealed that the generation and release of acetylcholine (ACh) and dopamine (DA) are both reduced in PD, however, overall acetylcholine was in a dominant position, resulting to a relatively cholinergic hyperactivity; further, the preponderance of ACh over DA contributes to the motor deficit (McKinley et al., 2019). An animal study showed that the parkinsonian motor dysfunction could be relieved by locally injecting the botulinum neurotoxin A in order to reduce the release of ACh (Wree et al., 2011). These studies indicated that cholinergic hyperactivity was related to the motor deficits in PD. In this study, we found the cholinergic functional connectivity in cingulum middle areas was positively correlated with UPDRS III scores and subscores of axial symptoms, suggesting that the more cholinergic hyperconnectivity in cingulum middle areas, the more severe of motor symptoms, which were similar to the previous studies. Taken together, we proposed that cholinergic hyperactivity in cingulum middle area may cause severe motor symptoms in PD patients.
An interesting finding in this study was the progressively increment of the cholinergic functional connectivity in primary motor and sensory areas at advanced stages of PD. A previous PET study found that specific populations (e.g., patients with movement disorders) exhibited decreased acetylcholinesterase activity in paracentral lobule, precentral gyrus, and postcentral gyrus (Hirano et al., 2010), pointing to the increased activity of cholinergic function in these brain regions. Intriguingly, some studies found the functional connectivity in primary motor and sensory areas was increased (Onu et al., 2015) and a greater improvement in UPDRS-III scores following L-dopa administration was characterized by lower functional connectivity in primary motor and sensory area, which were in agreement with our findings (Akram et al., 2017). Additionally, we found that the hyperactivity in primary motor and sensory areas was positively correlated with UPDRS III score and subscores of axial symptoms, suggesting a disease severity relevant role of cholinergic hyperactivity of these regions. Considering the progressive characteristic of PD evolution, we supposed that the cholinergic hyperconnectivity in primary motor and sensory areas may be a crucial mechanism for the disease progression.
There are some limitations of this study. First, selecting seed of seed-based rsFC analysis must be based on previous literature, which is subjective and cannot fully explore altered functional connectivity of the whole brain. Second, the sample size of this study was moderate. Third, in this study, we did not find any correlation between general cognition function (MoCa) and cholinergic network. Because most of the patients did not have multiple-domain cognition assessment, current finding should be cautiously translated to other relevant studies. And future studies are warranted to further disclose this interesting topic. Finally, this study is retrospective and cross-sectional. Further prospective and longitudinal studies with a larger sample size are expected to validate these finds and, importantly, to explore the longitudinal alterations of cholinergic-related functional connectivity along the disease progression, which could provide greater insight into the cholinergic neuromechanism of PD progression.
In conclusion, this study revealed altered cholinergic functional connectivity in PD patients, which were associated with the severity of motor symptoms. Specifically, cholinergic functional connectivity in primary motor and sensory cortex was progressively increased at advanced stages of PD. These findings illustrated that altered cholinergic function plays an important role in the motor disruptions in PD patients both in early stage as well as during the progression of the disease.
Data Availability Statement
The original contributions presented in the study are included in the article/Supplementary Material, further inquiries can be directed to the corresponding authors.
Ethics Statement
The studies involving human participants were reviewed and approved by the Medical Ethics Committee of The Second Affiliated Hospital of Zhejiang University School of Medicine. The patients/participants provided their written informed consent to participate in this study.
Author Contributions
XX, XG, YY, and MZ were responsible for the study concept and design. WS wrote the main manuscript text. TGu revised the main manuscript text. WS, TGa, CZ, JW, TGu, JP, and BZ contributed to the acquisition of imaging data. WS, TGu, CZ, and JW performed data analysis and interpreted the findings. All authors contributed to the article and approved the submitted version.
Funding
This work was supported by the 13th Five-year Plan for National Key Research and Development Program of China (Grant No. 2016YFC1306600); the National Natural Science Foundation of China (Grant Nos. 82001767, 81971577, 82001767, 81701647, and 81771820); the Zhejiang Provincial Natural Science Foundation (Nos. LQ21H180008 and LQ20H180012); China Postdoctoral Science Foundation (Grant Nos. 2021T140599 and 2019M662082); the Health Foundation for Creative Talents in Zhejiang Province, China (Grant No. 2016), and Scientific Research Incubation Project of The First Affiliated Hospital of Wenzhou Medical University (FHY2019091).
Conflict of Interest
The authors declare that the research was conducted in the absence of any commercial or financial relationships that could be construed as a potential conflict of interest.
Publisher’s Note
All claims expressed in this article are solely those of the authors and do not necessarily represent those of their affiliated organizations, or those of the publisher, the editors and the reviewers. Any product that may be evaluated in this article, or claim that may be made by its manufacturer, is not guaranteed or endorsed by the publisher.
Acknowledgments
The authors sincerely thank all the volunteers and PD patients recruited in this project.
Supplementary Material
The Supplementary Material for this article can be found online at: https://www.frontiersin.org/articles/10.3389/fnagi.2021.723948/full#supplementary-material
Footnotes
References
Akram, H., Wu, C., Hyam, J., Foltynie, T., Limousin, P., De Vita, E., et al. (2017). l-Dopa responsiveness is associated with distinctive connectivity patterns in advanced Parkinson’s disease. Mov. Disord. 32, 874–883. doi: 10.1002/mds.27017
Bohnen, N. I., and Albin, R. L. (2011). The cholinergic system and Parkinson disease. Behav. Brain Res. 221, 564–573. doi: 10.1016/j.bbr.2009.12.048
Bohnen, N. I., Müller, M. L., Koeppe, R. A., Studenski, S. A., Kilbourn, M. A., Frey, K. A., et al. (2009). History of falls in Parkinson disease is associated with reduced cholinergic activity. Neurology 73, 1670–1676. doi: 10.1212/WNL.0b013e3181c1ded6
Braak, H., Del Tredici, K., Rüb, U., de Vos, R. A., Jansen Steur, E. N., and Braak, E. (2003). Staging of brain pathology related to sporadic Parkinson’s disease. Neurobiol. Aging 24, 197–211. doi: 10.1016/s0197-4580(02)00065-9
Dalrymple, W. A., Huss, D. S., Blair, J., Flanigan, J. L., Patrie, J., Sperling, S. A., et al. (2021). Cholinergic nucleus 4 atrophy and gait impairment in Parkinson’s disease. J. Neurol. 268, 95–101. doi: 10.1007/s00415-020-10111-2
de Flores, R., Mutlu, J., Bejanin, A., Gonneaud, J., Landeau, B., Tomadesso, C., et al. (2017). Intrinsic connectivity of hippocampal subfields in normal elderly and mild cognitive impairment patients. Hum. Brain Mapp. 38, 4922–4932. doi: 10.1002/hbm.23704
Fox, M. D., and Raichle, M. E. (2007). Spontaneous fluctuations in brain activity observed with functional magnetic resonance imaging. Nat. Rev. Neurosci. 8, 700–711. doi: 10.1038/nrn2201
George, S., Mufson, E. J., Leurgans, S., Shah, R. C., Ferrari, C., and deToledo-Morrell, L. (2011). MRI-based volumetric measurement of the substantia innominata in amnestic MCI and mild AD. Neurobiol. Aging 32, 1756–1764. doi: 10.1016/j.neurobiolaging.2009.11.006
Guan, X., Zhang, Y., Wei, H., Guo, T., Zeng, Q., Zhou, C., et al. (2019). Iron-related nigral degeneration influences functional topology mediated by striatal dysfunction in Parkinson’s disease. Neurobiol. Aging 75, 83–97. doi: 10.1016/j.neurobiolaging.2018.11.013
Herman, T., Rosenberg-Katz, K., Jacob, Y., Giladi, N., and Hausdorff, J. M. (2014). Gray matter atrophy and freezing of gait in Parkinson’s disease: is the evidence black-on-white? Mov. Disord. 29, 134–139. doi: 10.1002/mds.25697
Hirano, S., Shinotoh, H., Shimada, H., Aotsuka, A., Tanaka, N., Ota, T., et al. (2010). Cholinergic imaging in corticobasal syndrome, progressive supranuclear palsy and frontotemporal dementia. Brain 133, 2058–2068. doi: 10.1093/brain/awq120
Hughes, A. J., Daniel, S. E., Kilford, L., and Lees, A. J. (1992). Accuracy of clinical diagnosis of idiopathic Parkinson’s disease: a clinico-pathological study of 100 cases. J. Neurol. Neurosurg. Psychiatry 55, 181–184. doi: 10.1136/jnnp.55.3.181
Kim, I., Shin, N. Y., Yunjin, B., Hyu Lee, P., Lee, S. K., and Mee Lim, S. (2017). Early-onset mild cognitive impairment in Parkinson’s disease: altered corticopetal cholinergic network. Sci. Rep. 7:2381. doi: 10.1038/s41598-017-02420-w
Langston, J. W. (2006). The Parkinson’s complex: parkinsonism is just the tip of the iceberg. Ann. Neurol. 59, 591–596. doi: 10.1002/ana.20834
Latt, M. D., Lord, S. R., Morris, J. G., and Fung, V. S. (2009). Clinical and physiological assessments for elucidating falls risk in Parkinson’s disease. Mov. Disord. 24, 1280–1289. doi: 10.1002/mds.22561
Lee, Y., Ham, J. H., Cha, J., Park, Y. H., Lee, J. J., Sunwoo, M. K., et al. (2018). The cholinergic contribution to the resting-state functional network in non-demented Parkinson’s disease. Sci. Rep. 8:7683. doi: 10.1038/s41598-018-26075-3
Li, C. S., Ide, J. S., Zhang, S., Hu, S., Chao, H. H., and Zaborszky, L. (2014). Resting state functional connectivity of the basal nucleus of Meynert in humans: in comparison to the ventral striatum and the effects of age. Neuroimage 97, 321–332. doi: 10.1016/j.neuroimage.2014.04.019
Li, Y., Huang, P., Guo, T., Guan, X., Gao, T., Sheng, W., et al. (2020). Brain structural correlates of depressive symptoms in Parkinson’s disease patients at different disease stage. Psychiatry Res. Neuroimaging 296:111029. doi: 10.1016/j.pscychresns.2019.111029
Liang, P., Wang, Z., Yang, Y., Jia, X., and Li, K. (2011). Functional disconnection and compensation in mild cognitive impairment: evidence from DLPFC connectivity using resting-state fMRI. PLoS One 6:e22153. doi: 10.1371/journal.pone.0022153
Lord, S., Baker, K., Nieuwboer, A., Burn, D., and Rochester, L. (2011). Gait variability in Parkinson’s disease: an indicator of non-dopaminergic contributors to gait dysfunction? J. Neurol. 258, 566–572. doi: 10.1007/s00415-010-5789-8
Mallet, N., Leblois, A., Maurice, N., Beurrier, C., et al. (2019). Striatal cholinergic interneurons: how to elucidate their function in health and disease. Front. Pharmacol. 10:1488. doi: 10.3389/fphar.2019.01488
McKinley, J. W., Shi, Z., Kawikova, I., Hur, M., Bamford, I. J., Sudarsana Devi, S. P., et al. (2019). Dopamine deficiency reduces striatal cholinergic interneuron function in models of parkinson’s disease. Neuron 103, 1056–1072.e6. doi: 10.1016/j.neuron.2019.06.013
Mesulam, M. M., and Geula, C. (1988). Nucleus basalis (Ch4) and cortical cholinergic innervation in the human brain: observations based on the distribution of acetylcholinesterase and choline acetyltransferase. J. Comp. Neurol. 275, 216–240. doi: 10.1002/cne.902750205
Mesulam, M. M., Rosen, A. D., and Mufson, E. J. (1984). Regional variations in cortical cholinergic innervation: chemoarchitectonics of acetylcholinesterase-containing fibers in the macaque brain. Brain Res. 311, 245–258. doi: 10.1016/0006-8993(84)90087-8
Onu, M., Badea, L., Roceanu, A., Tivarus, M., and Bajenaru, O. (2015). Increased connectivity between sensorimotor and attentional areas in Parkinson’s disease. Neuroradiology 57, 957–968. doi: 10.1007/s00234-015-1556-y
Perry, E. K., Curtis, M., Dick, D. J., Candy, J. M., Atack, J. R., Bloxham, C. A., et al. (1985). Cholinergic correlates of cognitive impairment in Parkinson’s disease: comparisons with Alzheimer’s disease. J. Neurol. Neurosurg. Psychiatry 48, 413–421. doi: 10.1136/jnnp.48.5.413
Reich, S. G., and Savitt, J. M. (2019). Parkinson’s Disease. Med. Clin. North Am. 103, 337–350. doi: 10.1016/j.mcna.2018.10.014
Rochester, L., Yarnall, A. J., Baker, M. R., David, R. V., Lord, S., Galna, B., et al. (2012). Cholinergic dysfunction contributes to gait disturbance in early Parkinson’s disease. Brain 135, 2779–2788. doi: 10.1093/brain/aws207
Sethi, K. (2008). Levodopa unresponsive symptoms in Parkinson disease. Mov. Disord. 23, S521–S533. doi: 10.1002/mds.22049
Staer, K., Iranzo, A., Stokholm, M. G., Østergaard, K., Serradell, M., Otto, M., et al. (2020). Cortical cholinergic dysfunction correlates with microglial activation in the substantia innominata in REM sleep behavior disorder. Parkinsonism Relat. Disord. 81, 89–93. doi: 10.1016/j.parkreldis.2020.10.014
Takakusaki, K. (2017). Functional neuroanatomy for posture and gait control. J. Mov. Disord. 10, 1–17. doi: 10.14802/jmd.16062
Vastik, M., Hok, P., Valosek, J., Hlustik, P., Mensikova, K., and Kanovsky, P. (2017). Freezing of gait is associated with cortical thinning in mesial frontal cortex. Biomed. Pap. Med. Fac. Univ. Palacky Olomouc Czech Repub. 161, 389–396. doi: 10.5507/bp.2017.035
Wree, A., Mix, E., Hawlitschka, A., Antipova, V., Witt, M., Schmitt, O., et al. (2011). Intrastriatal botulinum toxin abolishes pathologic rotational behaviour and induces axonal varicosities in the 6-OHDA rat model of Parkinson’s disease. Neurobiol. Dis. 41, 291–298. doi: 10.1016/j.nbd.2010.09.017
Yan, C. G., Wang, X. D., Zuo, X. N., and Zang, Y. F. (2016). DPABI: Data Processing & Analysis for (Resting-State) Brain Imaging. Neuroinformatics 14, 339–351. doi: 10.1007/s12021-016-9299-4
Yarnall, A., Rochester, L., and Burn, D. J. (2011). The interplay of cholinergic function, attention, and falls in Parkinson’s disease. Mov. Disord. 26, 2496–2503. doi: 10.1002/mds.23932
Zhan, Z. W., Lin, L. Z., Yu, E. H., Xin, J. W., Lin, L., Lin, H. L., et al. (2018). Abnormal resting-state functional connectivity in posterior cingulate cortex of Parkinson’s disease with mild cognitive impairment and dementia. CNS Neurosci. Ther. 24, 897–905. doi: 10.1111/cns.12838
Zhang, Z. X., Roman, G. C., Hong, Z., Wu, C. B., Qu, Q. M., Huang, J. B., et al. (2005). Parkinson’s disease in China: prevalence in Beijing, Xian, and Shanghai. Lancet 365, 595–597. doi: 10.1016/s0140-6736(05)17909-4
Keywords: Parkinson’s disease, substantia innominata, cholinergic network, motor, functional connectivity
Citation: Sheng W, Guo T, Zhou C, Wu J, Gao T, Pu J, Zhang B, Zhang M, Yang Y, Guan X and Xu X (2021) Altered Cortical Cholinergic Network in Parkinson’s Disease at Different Stage: A Resting-State fMRI Study. Front. Aging Neurosci. 13:723948. doi: 10.3389/fnagi.2021.723948
Received: 11 June 2021; Accepted: 09 August 2021;
Published: 10 September 2021.
Edited by:
Wei Cheng, Fudan University, ChinaReviewed by:
Zaixu Cui, University of Pennsylvania, United StatesFeng-Tao Liu, Fudan University, China
Zhang Jinbo, Chinese Institute for Brain Research, Beijing, China, in collaboration with reviewer ZC
Copyright © 2021 Sheng, Guo, Zhou, Wu, Gao, Pu, Zhang, Zhang, Yang, Guan and Xu. This is an open-access article distributed under the terms of the Creative Commons Attribution License (CC BY). The use, distribution or reproduction in other forums is permitted, provided the original author(s) and the copyright owner(s) are credited and that the original publication in this journal is cited, in accordance with accepted academic practice. No use, distribution or reproduction is permitted which does not comply with these terms.
*Correspondence: Minming Zhang, zhangminming@zju.edu.cn; Yunjun Yang, yyjunjim@163.com; Xiaojun Guan, xiaojunguan1102@zju.edu.cn; Xiaojun Xu, xxjmailbox@zju.edu.cn
†These authors have contributed equally to this work and share first authorship