- 1Neurophysiologie de la Synapse Auditive, INSERM UMRS 1120, Bordeaux Neurocampus, Université de Bordeaux, Bordeaux, France
- 2Institut de l'Audition, Centre Institut Pasteur/Inserm, Paris, France
- 3INCIA, UMR 5287, CNRS, University of Bordeaux, Bat B2, Pessac, France
Age-related hidden hearing loss is often described as a cochlear synaptopathy that results from a progressive degeneration of the inner hair cell (IHC) ribbon synapses. The functional changes occurring at these synapses during aging are not fully understood. Here, we characterized this aging process in IHCs of C57BL/6J mice, a strain which is known to carry a cadherin-23 mutation and experiences early hearing loss with age. These mice, while displaying a large increase in auditory brainstem thresholds due to 50% loss of IHC synaptic ribbons at middle age (postnatal day 365), paradoxically showed enhanced acoustic startle reflex suggesting a hyperacusis-like response. The auditory defect was associated with a large shrinkage of the IHCs' cell body and a drastic enlargement of their remaining presynaptic ribbons which were facing enlarged postsynaptic AMPAR clusters. Presynaptic Ca2+ microdomains and the capacity of IHCs to sustain high rates of exocytosis were largely increased, while on the contrary the expression of the fast-repolarizing BK channels, known to negatively control transmitter release, was decreased. This age-related synaptic plasticity in IHCs suggested a functional potentiation of synaptic transmission at the surviving synapses, a process that could partially compensate the decrease in synapse number and underlie hyperacusis.
Introduction
Age-related hearing loss (ARHL or presbycusis) is the most prevalent form of sensory disability in human populations and adversely affects the quality of life of many elderly individuals. Simultaneously with ARHL, chronic tinnitus and hyperacusis often develop due to abnormal neural activity in the central auditory system whose origin may arise from cochlear pathologies (for review see Knipper et al., 2013). The development of ARHL has been generally considered to be associated with primary degeneration of cochlear hair cells, with secondary deafferentation associating degeneration of spiral ganglion neurons and ribbon synapses. However, recent studies have resulted in a paradigm shift in the understanding of the early cause of ARHL (Kujawa and Liberman, 2015, 2019). During aging, hearing thresholds in the elderly often remain normal but speech intelligibility becomes hampered, especially in noisy environment. This pathology in the elderly is referred as hidden hearing loss and has been proposed to be caused in mice by an early loss of ribbon synapses contacting the inner hair cells (IHCs), well before any major loss of hair cells and spiral ganglion neurons (Stamataki et al., 2006). While glutamate excitotoxicity, resulting in nerve terminal swelling, has been proposed to play a role in the setting of noise-induced hearing loss (Pujol and Puel, 1999; Hu et al., 2020), the molecular mechanisms underlying the degeneration of the cochlear ribbon synapses during aging remain to be elucidated. Oxidative damage due to an increase in ROS levels seems to play a crucial role in ARHL (Fu et al., 2018) but how the ribbon synapses are functionally affected remain unknown.
The C57BL/6J inbred strain of mice has been extensively used as model for ARHL (Johnson et al., 1997; Spongr et al., 1997; Hequembourg and Liberman, 2001; Bartolome et al., 2002; Henry, 2002). This mouse strain carries a cadherin-23 mutation (Cdh23753A, also known as Ahl), which affects inner ear structures, characterized by progressive degeneration of ribbon synapses and spiral ganglion neurons, and results in age-related hearing loss. Ahl is a recessive single nucleotide mutation at 753 (G = >A) on the Cdh23 gene on mouse chromosome 10 (Noben-Trauth et al., 2003). Cdh23 encodes cadherin 23, a protein necessary for inner ear development and maintenance of the sensory hair cell structures, in particular the tip and lateral links of the stereocilia at the hair bundle (Siemens et al., 2004; Sollner et al., 2004; Lagziel et al., 2005; Michel et al., 2005; Kazmierczak et al., 2007), but growing anatomical evidence also suggests that synaptic rearrangements on sensory hair cells also contribute to auditory functional decline in C57BL/6J mice (Stamataki et al., 2006; Jiang et al., 2015; Zachary and Fuchs, 2015; Jeng et al., 2021). Surprisingly, aging C57BL/6J mice, although showing cochlear ribbon synapse degeneration and hearing loss, display increased acoustic startle reflex amplitudes (Ison and Allen, 2003; Ouagazzal et al., 2006; Ison et al., 2007) and a time increase in recovery from ABR short-term adaptation (Walton et al., 1995). Whether this exaggerated startle reflex can be explained by functional changes of cochlear hair cell ribbon synapses remain to be explored. Our present study will show that aged C57BL/6J mice, while losing nearly up to 50% of their IHC ribbon synapses, have IHCs with reduced BK channel clusters, larger remaining presynaptic ribbons (with increased Ca2+ microdomains and larger sustained exocytotic responses) facing postsynaptic afferent boutons with larger AMPAR clusters. All these features indicated that synaptic release potentiation occurs at auditory IHC ribbon synapses during aging. This age-related synaptic plasticity could be viewed as compensatory mechanisms of the decrease IHC synapse number and may explain the hyperacusis-like startle reflex observed in aged C57BL/6J mice.
Results
Increased ABR Thresholds and Hyperacusis-Like Effect on ASR
As previously described (Zheng et al., 1999; Zhou et al., 2006), CBA/J mice, used here as a reference, showed no sign of rapid degradation of their hearing function with aging, as attested by constant click ABR thresholds near 15 dB SPL up to 1 year of age (Figure 1A). On the contrary, C57BL/6J mice, carrying the Cdh23753A mutation, displayed an early progressive increase in ABR thresholds with aging (Figure 1A). Hearing thresholds started to increase significantly at postnatal day P180 to reach a mean 50 dB increase at P365 (click ABR thresholds above 65 dB SPL) as compared to young mature P30 mice. Distortion product otoacoustic emissions (DPOAEs) in P365 C57BL/6J mice were still present but significantly reduced, indicating that the electromechanical activity of the outer hair cells (OHCs) was also affected (Figure 1D). However, counting hair cells on surface preparations of organs of Corti from these P365 mice, in the mid-cochlea region (8–16 kHz), indicated only a moderate loss of OHCs and IHCs (statistically not significant; Figure 2E), in good agreement with the study of Zachary and Fuchs (Zachary and Fuchs, 2015). This moderate loss of OHCs suggested that the profound hearing loss occurring at P365 in C57BL/6J mice arose from additional factors than a simple alteration of the cochlear amplifier. We then compared the amplitude on the ABR wave-I as a function of sound intensity in young and old mice. The wave-I is known to result from the synchronous activity of the auditory nerve fibers and can provide an objective measure of ribbon synapses loss when measured at high sound intensity above 70 dB SPL. In P365 old mice, ABR wave-I amplitudes were largely reduced as compared to young mature P30 mice, even at high sound intensity when IHCs are directly stimulated and bypass the amplification process of the OHCs (Figure 1B). The large decrease in wave-I amplitude indicated a decreased number of auditory nerve fibers activated by sound and/or a decrease in their firing rate or synchrony. The latencies of the ABR wave-I were also significantly increased suggesting again that the IHC ribbon synapses are affected (Figure 1C).
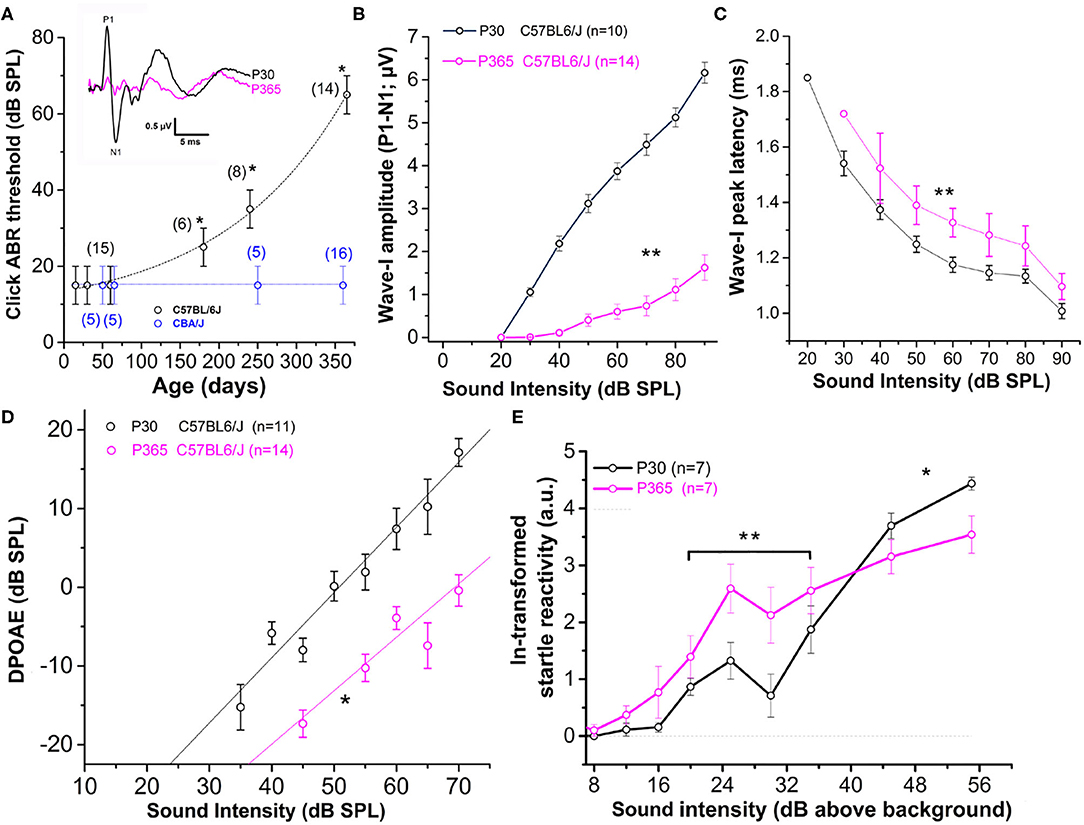
Figure 1. Sensorineural hearing loss with Aging in C57BL/6J mice. (A) Click-ABR thresholds as a function of age in CBA/J mice (blue; n = 5–16) and C57BL/6J mice (black, n = 6–15). For each strain, asterisks indicate significant difference with p < 0.05 as compared to P30 mice (two-way ANOVA). Inset show comparative mean ABR waves at 70 dB SPL in P30 and P365 C57BL/6J mice. (B) Wave-I amplitude (P1–N1) as a function of sound intensity in young P30 (black) and old P365 (pink) C57BL/6J mice. Asterisks indicate significant difference with p < 0.05 (two-way ANOVA; p = 0.001). (C) Latency to reach peak amplitude of wave-I as a function of sound intensity. Significantly different with p = 0.003 between 80 and 50 dB SPL (two-way ANOVA; n is indicated in graph B). (D) Distortion products otoacoustic emissions (DPOAEs; 2f 1–f 2 with f 1 = 12.73 kHz and f 2 = 15.26 kHz) as a function of sound intensity in young P30 (black) and old P360 (pink) C57BL/6J mice. Asterisk indicated significant difference with p < 0.05 (two-way ANOVA, p = 0.01). (E) Startle reactivity to acoustic stimulation. The intensity of startle reaction (in mV) was ln-transformed and obtained through two conditions of white-noise bursts of 20 and 40 ms. Note that below 35 dB above background the startle reaction is increased at P360 whereas at 45 and 55 dB above background the startle reaction is decreased compared to P30 mice. Asterisks indicated significant difference with p < 0.05 (two-way ANOVA, p = 0.007 between 20 and 35 dB and p = 0.005 between 45 and 55 dB).
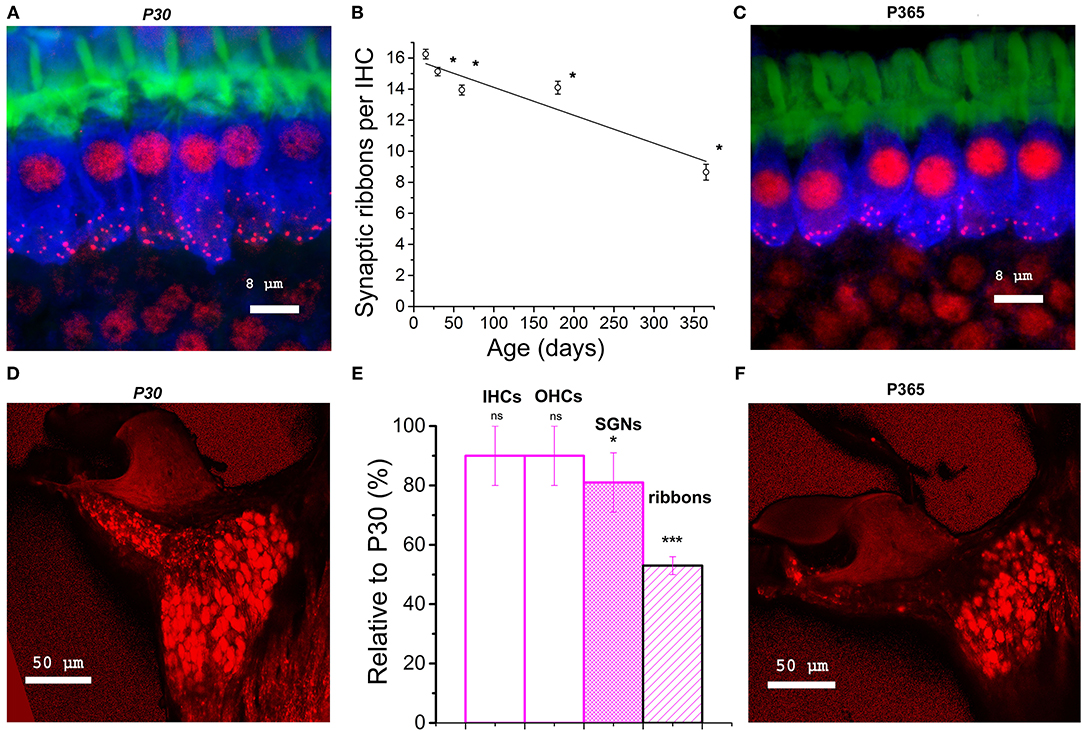
Figure 2. Synaptic ribbon loss in aging IHCs and comparative density of spiral ganglion neurons. (A–C) Confocal immunofluorescence imaging of IHCs from P30 and P360 C57BL/6J mice. Image resulted from stack reconstructions of 22 slices of 0.3 μm thickness, showing F-actin (green, labeling the hair bundles), otoferlin (blue; a synaptic Ca2+ sensor labeling the entire IHC body) and synaptic ribbons (Ribeye/CtBP2, red dots). The white bars indicated 8 μm. (B) Plot of synaptic ribbon counts per IHC as a function of age in C57BL/6J mice (black) in the mid-frequency cochlear region (8–16 kHz). Asterisks indicate significant difference with p < 0.05 (comparison with P15 C57BL/6J mice, one-way ANOVA post-hoc Tukey test). 203, 306, 306, 306, and 295 ribbons were, respectively, analyzed at P15, P30, P60, P180, and P365 in 3–5 mice at each age. (D–F) Confocal immunofluorescence imaging of cochlear transversal sections from P30 and P360 mice (8–16 kHz area) where neurons and their afferent dendrites are labeled with dextran amines 3,000. (E) Analyzed in the mid-cochlea (8–16 kHz), comparative histogram of hair cells counts, SGNs and synaptic ribbons (three mice at each age relative to young mature P30 C57BL/6J mice). The asterisks indicated statistical difference with p < 0.05 (from left to right p = 0.37, 0.14, and 0.020 for SGNs and p = 1.01E−14 for ribbons, using unpaired t-test). Note the reduction in SGNs and the drastic decrease of the fluorescent labeling of the afferent nerve fibers projecting to the organ of Corti.
Surprisingly, at lower sound intensities tested (between 20 and 35 dB above background noise level), the strength of the acoustic startle reflex (ASR) was found larger in old P365 C57BL/6J mice as compared to young P60, indicating hyperacusis reactivity (Figure 1E). The origin of this acoustic hypereactivity at low sound intensities could arise from central neural reorganization (Willott, 1996) and be consecutive to functional defect of the IHC ribbon synapses in aging C57BL/6J mice that we are going to characterize in this study. At loud sound intensities, 45 dB above background noise, the amplitudes of the ASR responses were however diminished in old mice, likely reflecting the increase in ABR thresholds due to a drastic decrease in the number of ribbon synapses per IHCs.
Drastic Loss of IHC Synaptic Ribbons With Aging
Confocal immunofluorescence imaging of the synaptic ribbons in IHCs from C57BL/6J mice, in the cochlear region encoding between 8 and 16 kHz, showed a progressive and large decrease in the number of synaptic ribbons with aging, from 16.3 ± 0.3 ribbons/IHC at P15 to 8.7 ± 0.5 ribbons/IHC at P365 (Figures 2A–C). This nearly 50% loss of synaptic ribbons in IHCs of C57BL/6J mice at P365 was in good agreement with previous studies (Zachary and Fuchs, 2015; Jeng et al., 2020). Counting the spiral ganglion neurons in the mid-frequency region of the cochlea (8–16 kHz) showed a mean 20% loss of neurons in P365 mice as compared to P30 mice (Figures 2D–F). Remarkably, the age-related loss of neurons, averaging 20%, was much less pronounced than the loss of IHC synaptic ribbons (50%), suggesting that there was a large number of neurons surviving without dendritic/synaptic projection toward the organ of Corti as previously shown by Stamataki et al. (2006).
Enlarged Presynaptic Ribbons Associated With Larger Postsynaptic AMPAR Clusters
When analyzing the 3-D imaging reconstruction of the IHC synaptic ribbons from P365 C57BL/6J IHCs from high resolution confocal STED immunofluorescent sections, we found that these structures displayed a progressive increase in their mean size (volume) as compared to young P15 mice (Figure 3A). The mean volume of the synaptic ribbons increased nearly three-fold between P15 and P365 (Figure 3B). Interestingly, the increase in size of the ribbons started very early after P30, an age at which their number also started to decrease (Figure 2B) while hearing thresholds were still normal (Figure 1A).
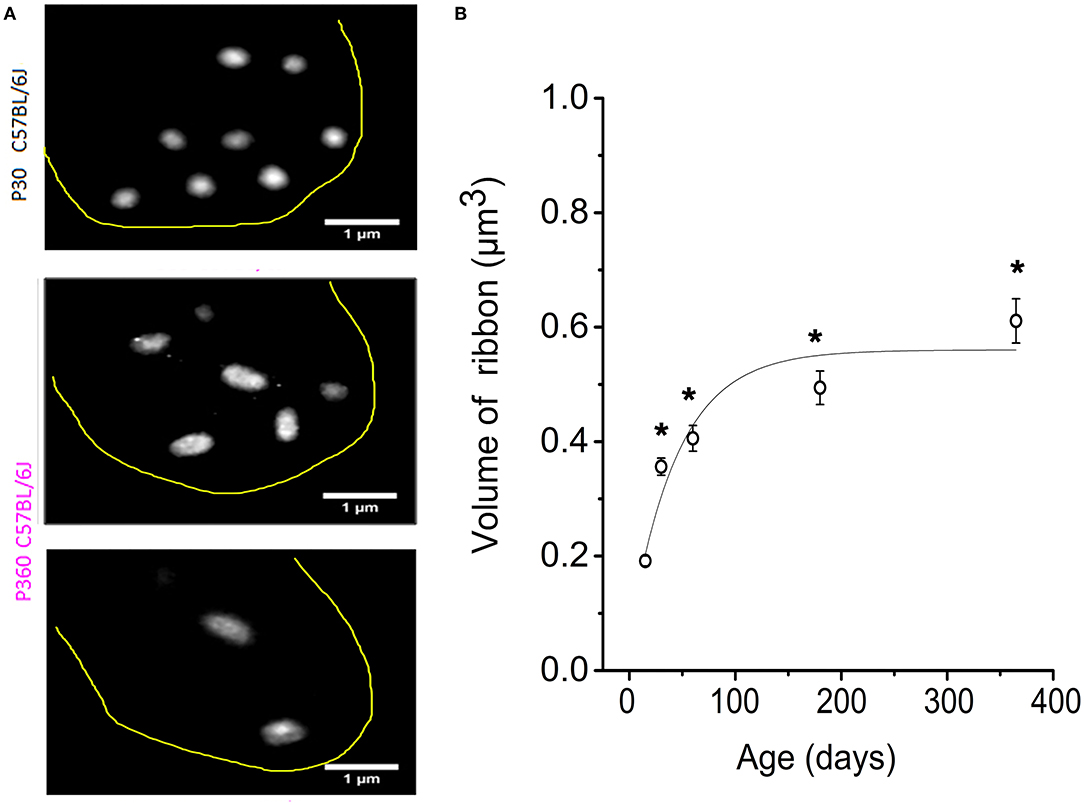
Figure 3. IHC synaptic ribbons progressively increased in size with aging. (A) Example of high magnification confocal STED images (stack reconstruction of five 0.25 μm slices). The yellow line delineates the IHC contour. Ribbons were less numerous and larger in size in IHCs from P365 C57BL/6J mice. (B) The mean volume of the IHC synaptic ribbons was calculated in the mid-cochlear region (encoding 8–16 kHz), using the plugin 3D-Object counter of ImageJ, and was plotted as a function of age: P15 (203 ribbons–40 IHCs–3 mice), P30 (306 ribbons–98 IHCs–5 mice), P60 (306 ribbons–95 IHCs–3 mice), P180 (306 ribbons–67 IHCs–3 mice), P365 (295 ribbons–43 IHCs–3 mice). As indicated by the asterisks, when comparing with P15, the mean volume of the ribbons started significantly to increase significantly from P30 (p < 0.05; one-way ANOVA).
We next investigated the changes occurring with aging in the spatial distribution of the synaptic ribbons at the basolateral membrane of the IHCs. It is worth recalling that larger presynaptic ribbons associated to low-spontaneous-rate afferent fibers are usually found at the modiolar side of the IHCs (i.e., the baso-lateral curved side of the IHCs directed toward the spiral ganglion neurons) while smaller ribbons associated with high-spontaneous-rate afferent fibers predominates at the pillar side (Liberman, 1982; Liberman et al., 2011). We found that, while a larger proportion of synaptic ribbons distributed at the modiolar side in young P30 IHCs (~60%), the surviving ribbons in old P365 IHCs were evenly distributed at the pillar and modiolar side (Figures 4A,B). The loss of synaptic ribbons with aging was more pronounced at the modiolar side, i.e., at the side where most of the low-spontaneous (high threshold) fibers make contact to IHCs (Figure 4C). An increase in volume was noted both in pillar and modiolar ribbons (Figure 4D). Unlike young P30 IHCs, old P365 IHCs had modiolar and pillar ribbons with similar volume (0.7 ± 0.1 μm3 and 0.57 ± 0.07 μm3, respectively; not significantly different, unpaired t-test p = 0.28, Figure 4D). Similar preferential loss of synaptic modiolar ribbons associated with an increase in ribbon volume was also reported after noise-induced hearing loss (Liberman and Kujawa, 2017; Hickman et al., 2020).
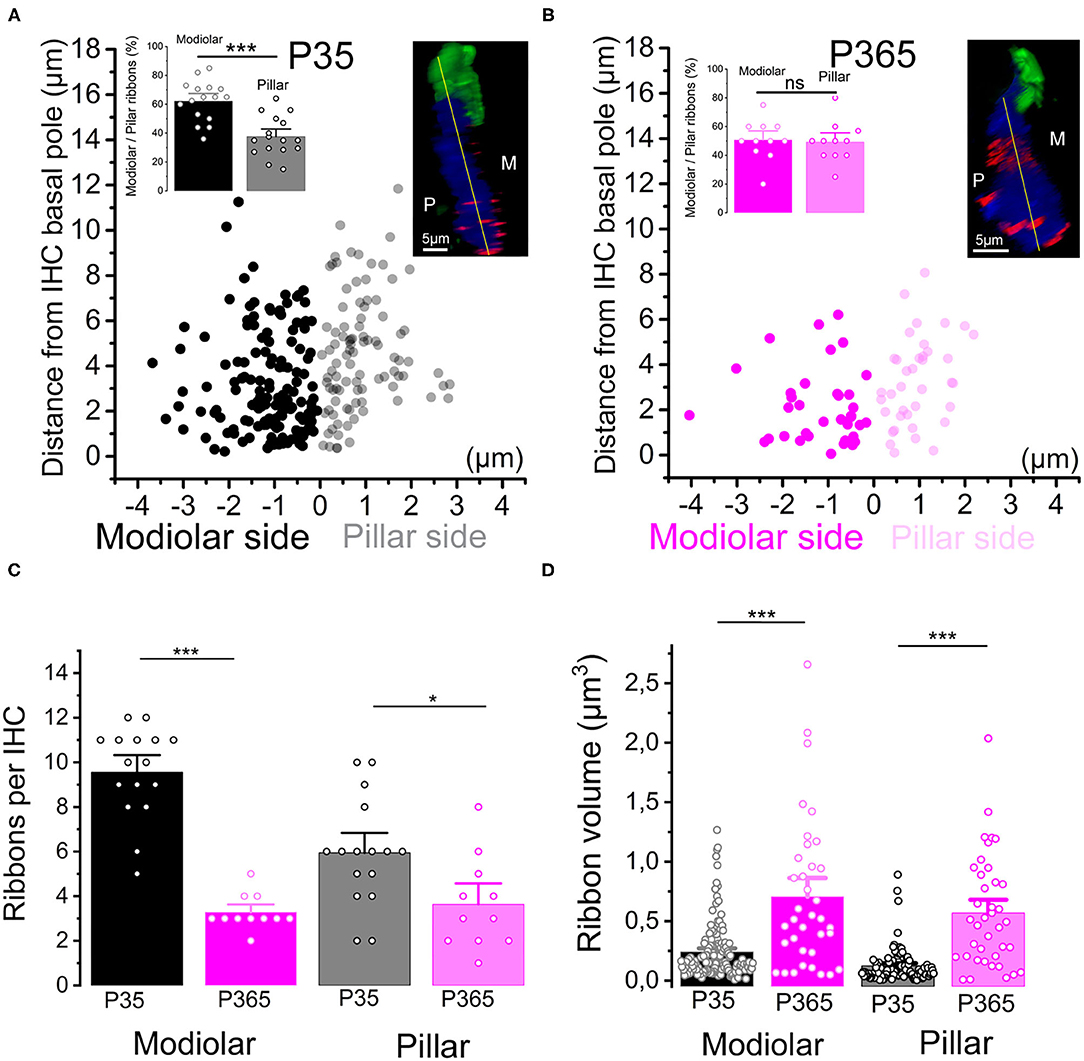
Figure 4. Modiolar vs. pillar distribution of the synaptic ribbons with aging. (A,B) The distribution of IHC synaptic ribbons was splitted in two cell areas: P (pillar side) and M (Modiolar side). The two regions were determined using the K-means clustering method for each cell (see section Methods). The yellow lines in the inset confocal images was drawn to arbitrarily delineate the frontier between pillar and modiolar ribbons (green labeling F-actin, blue Otoferlin and red the synaptic ribbons). Note that in young P35 IHCs (n = 16), synaptic ribbons are preferentially localized in the modiolar region (62.3 ± 3.5% vs. 37.7 ± 3.5; unpaired t-test p = 2.07 E−5). In old P365 IHCs (n = 11), the remaining ribbons equally distributed in the modiolar and pillar side (50.7 ± 4.0% vs. 49.3 ± 4.0%, unpaired t-test; p = 0.81). (C) Synaptic ribbons were significantly loss both at the modiolar side and pillar side. However, the loss was much more pronounced in the IHCs' modiolar side (9.56 ± 0.5 ribbons (n = 16 IHCs) vs. 3.27 ± 0.24 ribbons (n = 11 IHCs); unpaired t-test p = 5.57 E−10) and pillar (5.9 ± 0.6 ribbons vs. 3.6 ± 0.6 ribbons, unpaired t-test p = 0.016). (D) The increase in volume affected both modiolar [P35 = 0.24 ± 0.02 μm3 (153 ribbons) vs. P365 = 0.7 ± 0.1 μm3 (36 ribbons) unpaired t-test, p = 3.7 E−11] and pillar [P35 = 0.12 ± 0.01 μm3 (95 ribbons) vs. P365= 0.57 ± 0.07 μm3 (40 ribbons), unpaired t-test p = 7.5 E−14] synaptic ribbons. This comparative analysis was performed in IHCs of the mid-cochlear region (encoding 8–16 kHz). Asterisks indicate statistical difference with *p < 0.05 and ***p < 0.001.
Postsynaptic afferent endings to ribbon IHCs express AMPA-type ionotropic glutamate [glutamate receptor A2 (GluA2)]-containing AMPA receptors (Matsubara et al., 1996; Hu et al., 2020). To determine whether the organization of postsynaptic AMPARs facing the IHCs ribbon was modified in old P365 mice we performed GluA2 immunostaining and confocal fluorescence imaging (Figure 5). As compared to young ribbon synapses, old synapses had larger ribbons associated with larger AMPAR clusters, suggesting that the changes occurring with aging are both at the pre and postsynaptic side in good agreement with the EM morphological analysis of Stamataki et al. (2006).
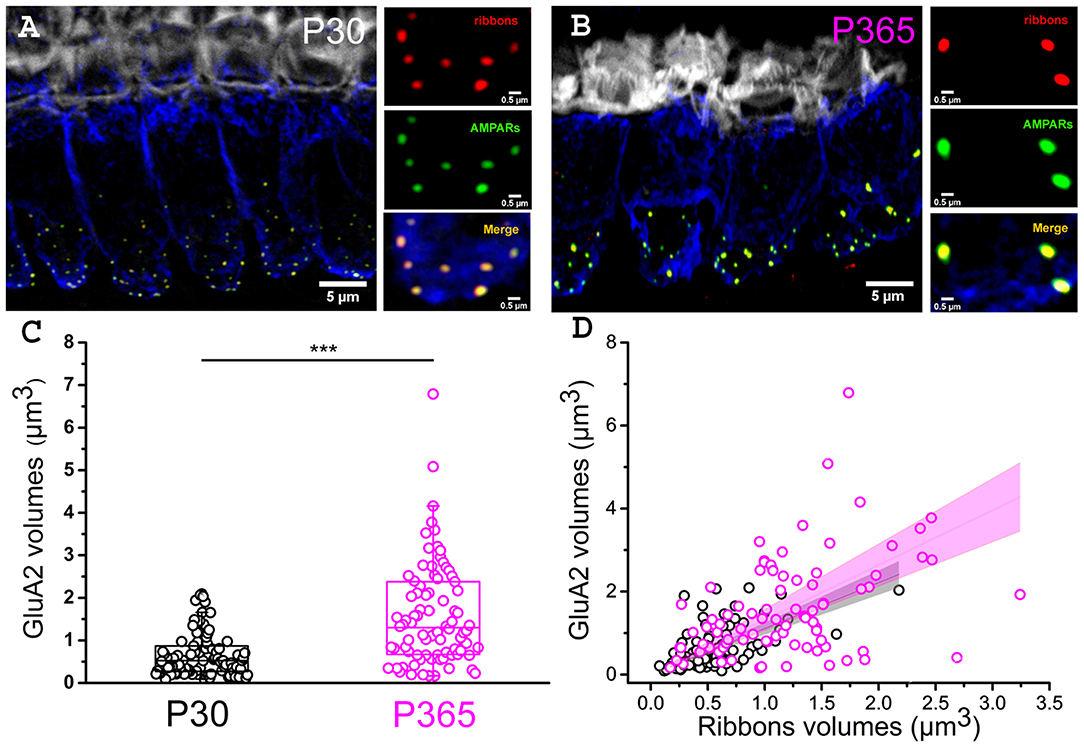
Figure 5. Larger postsynaptic patches of GluA2 receptors in old IHCs. (A,B) Immuno-confocal imaging of IHCs (stack reconstructions of 22 slices of 0.3 μm thickness) showing otoferlin (blue), the synaptic ribbons (Ribeye/CtBP2, red dots) and the post-synaptic AMPA receptors (GluA2, green dots). Fluorescent-phaloidin (gray) labeled F-actin in the strereocilia. The white bars indicate 5 μm in (A,B), then 0.5 μm in the 3 small panels. (C) Comparative distribution of the volume of postsynaptic patches of AMPA GluA2 receptors. Note that the GluA2 receptor volume significantly increased with aging in C57Bl/6J mice (p < 0.001; unpaired-t test); (n = 86 synapses; from three mice at P30 (16 IHCs) and P365 (29 IHCs); mid-cochlear region encoding 8–16 kHz). (D) Comparative volume distribution of postsynaptic GluA2 and corresponding postsynaptic ribbon in P30 and P365 IHCs. Note a good linear correlation between GluA2 and ribbon volumes (Pearson's coefficient r = 0.93 and 0.86, respectively, for P30 and P360).
Drastic IHC Cell Size Reduction With Aging
To determine whether the loss of synaptic ribbons was associated with a cell size reduction in old P365 IHCs, we labeled the cytosol of IHCs with an antibody against myo-7a, an unconventional myosin essential for the hair bundle functional integrity and found in the apical sterocilia as well as the cytoplasm of the hair cells (Hasson et al., 1995). We found a drastic reduction of the basal infra-nuclear synaptic zone of the IHCs from an average of 15.7 ± 0.1 μm (n = 34) in P21 IHCs to 12.4 ± 0.3 μm (n = 50) in old P365 IHCs (unpaired-t test, p = 1.1 E−13, Figures 6A–C). The cell size reduction of old IHCs was confirmed by another method using whole-cell patch clamp measurement of the IHC resting membrane capacitance in organs of Corti preparation ex-vivo (Figures 6D,E). P365 IHCs displayed a significant smaller resting capacitance, measured at −70 mV, of 7.9 ± 0.4 pF (n = 22) as compared to P21 IHCs (10. 8 ± 0.1 pF, n = 44, unpaired t-test, p = 3.7 E−10).
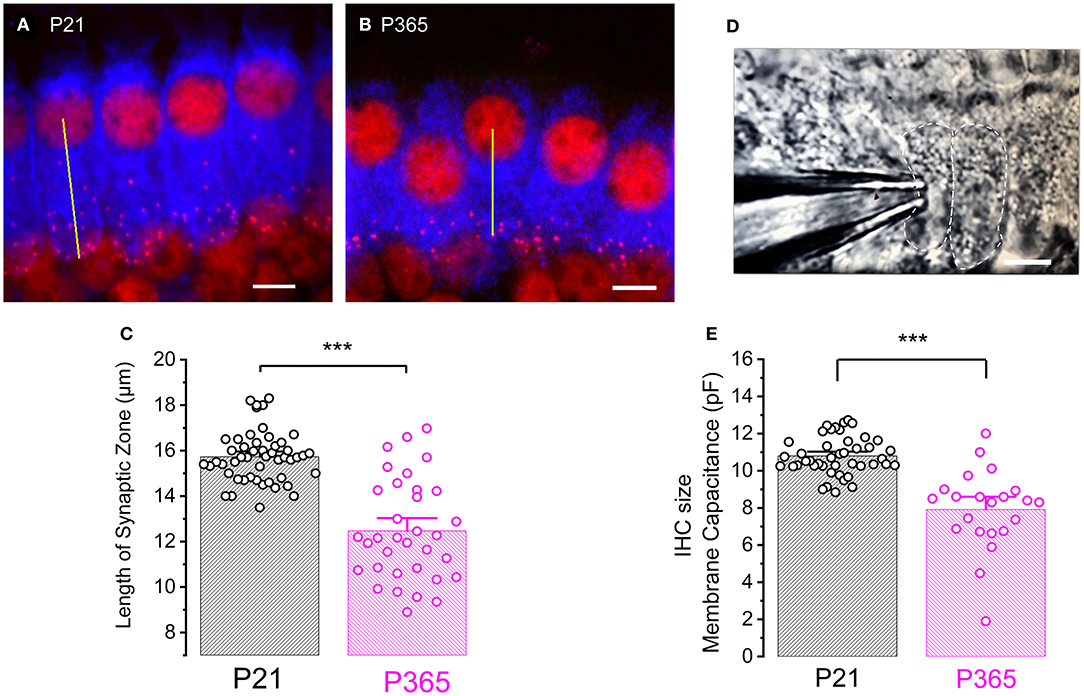
Figure 6. Cell size reduction of IHCs with aging. (A,B) Immuno-confocal imaging of IHCs labeled for myo-7a (blue) and CtBP2 (labeling the ribbons, red) in P21 (A) and P365 (B) mice (reconstructed stack images of 22 slices of 0.3 μm thick). The length of the infra-nuclear synaptic zone was measured from the center of the nucleus to the bottom of the cell as indicated by the yellow line. The scale bar indicated 5 μm. (C) Comparative histogram of the length of the infra-nuclear synaptic zone. Asterisks indicated statistical significance with p < 0.0001, unpaired t-test, n = 50 IHCs for P21 and 34 IHCs for P365. Measurements were made from IHCs of the mid-cochlear region (8–16 kHz). (D) Photomicrograph showing a row of IHCs in an ex-vivo organ of Corti explant and a patch-clamp recording electrode allowing measurements of capacitive and ionic currents. (E) Comparative mean IHC resting membrane capacitance, measured at −70 mV, in whole-cell patch-clamp configuration. Asterisks indicated values with significant difference with p < 0.0001, unpaired-t test, n = 44 IHCs for P21 and n = 22 for P365.
Higher Ca2+ Current Density and Exocytosis in Old IHCs
Although the loss of IHC ribbon synapses in C57BL/6J mice with aging is well-documented in the literature (Wan and Corfas, 2015), the functional changes of the remaining “old” IHC ribbon synapses has not yet been explored in detail at the cellular level. We investigated here the changes in the calcium-evoked exocytotic responses of aging IHCs from ex-vivo explants of the organ of Corti from aging C57BL/6J mice. We recall that Ca2+ is a key messenger for normal hearing since it tunes the transduction of the analogic sound signal into nerve impulses. This transduction occurs at the IHC synaptic active zone through the voltage-activation of Cav1.3 Ca2+ channels (Brandt et al., 2003; Vincent et al., 2017) and the action of the Ca2+ sensor otoferlin (Roux et al., 2006; Beurg et al., 2010; Michalski et al., 2017). Increase in the density of L-type Ca2+ channels have long been recognized as a characteristic of aging central neurons (Campbell et al., 1996; Thibault and Landfield, 1996) but possible altered function in Ca2+ signaling has not been investigated in aging IHCs.
In the present study, whole cell patch-clamp recordings from old P365 IHCs showed a significantly increase in Ca2+ current density (Ca2+ current amplitude normalized to cell size) as compared to young mature P30 IHCs, rising from a mean peak value of 15.8 ± 1.75 pA/pF to 20.1 ± 1.9 pA/pF at −10 mV (Figure 7A; unpaired t-test, p < 0.05). The voltage-activation curve of the Ca2+ currents in old P365 IHCs remained similar to the young mature P30 IHCs, with a mean half-voltage activation (V1/2) of −29.3 ± 1.1 and −26.7 ± 2.8 mV, respectively (unpaired t-test, p = 0.06).
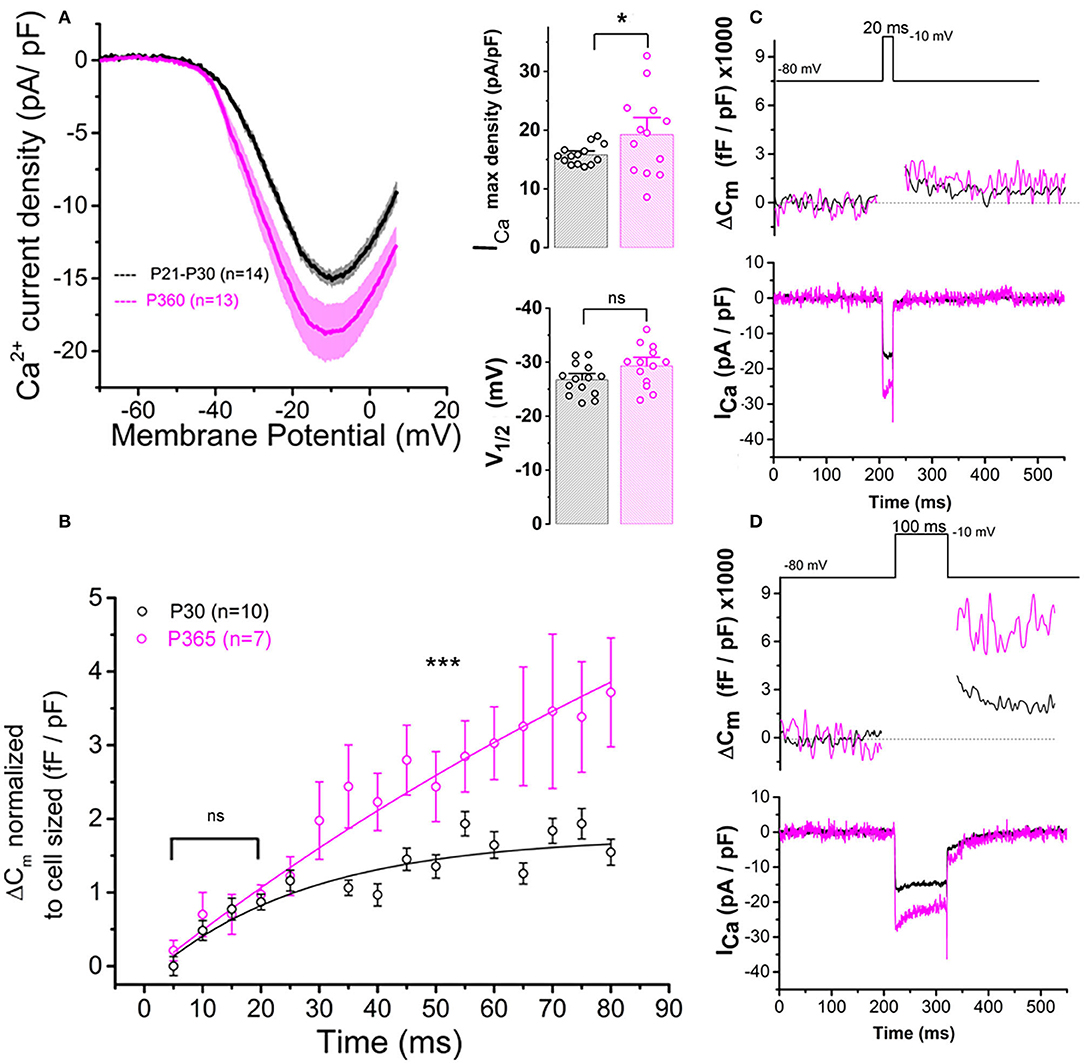
Figure 7. Ca2+ currents and exocytosis are exacerbated in IHCs from aged C57BL6/J mice. (A) Ca2+ current density of old P365 IHCs (pink) was larger as compared to young mature P21 IHCs (black). IHCs were subjected to a voltage ramp stimulation from −90 to +30 mV in 120 ms, giving a slope change in voltage of 1 mV/ms. Comparative ICa maximum density measured at −10 mV indicated a significant increase in P360 IHCs (unpaired two-sample t-test, p = 0.03) while the half-max voltage activation (V1/2) of the Ca2+ currents remained unchanged (unpaired t-test, p = 0.06). (B) Kinetics of exocytosis were evoked by a voltage-steps from −80 to −10 mV with increasing duration from 5 to 80 ms. Note that brief stimulations below 25 ms, a time-frame addressing the release of the Readily Releasable Pool of vesicles, the exocytotic response (normalized to cell size) was similar in P30 and P360 IHCs (p = 0.2; two-way ANOVA). Longer stimulations, above 30 ms, which probed the activation of the secondarily releasable pool of vesicles, showed a significant increase in exocytosis (two-way ANOVA; p = 2.3 E−8), indicating that old IHCs were more likely to sustain high rates of vesicular release. (C,D) Representative examples of ΔCm and ICa recordings from P30 and P365 IHCs during a 20 ms and a 100 ms voltage-step stimulation from −80 to −10 mV. The number of IHCs, indicated in graph, were obtained from five mice at P365 and six mice at P30. Asterisks indicate statistical difference with *p < 0.05 and ***p < 0.001.
Patch-clamp measurement of membrane capacitance upon depolarizing voltage-steps from −80 to −10 mV with increasing time duration allowed to compare the kinetics of vesicle exocytosis in young and old IHCs. For brief stimulations below 25 ms, a time-frame addressing the release of the Readily Releasable Pool (RRP) of vesicles, the exocytosis response (normalized to cell size) was similar in P30 and P365 IHCs (Figures 7B,C). However, longer depolarization above 25 ms revealed a more sustained exocytotic response in old IHCs, suggesting the mobilization of a more efficient secondarily releasable pool (SRP), i.e., a more efficient vesicle recruitment in these cells (Figures 7B,D). To investigate whether this increased SRP exocytosis was due to higher Ca2+ microdomains at the ribbon active zones, we used the high affinity Ca2+-indicator Rhod-2 (Kd = 570 nM) which is known to allow a good monitoring of low Ca2+ concentrations ranging from 20 nM to 20 μM (Del Nido et al., 1998). We choose to use Rhod-2 rather than a low affinity Ca2+ dye such as OGB-5N with a Kd at 20 μM (Vincent et al., 2014) because we wanted here to primarily probe the amplitude of the Ca2+ microdomains, where the changes in Ca2+ concentrations are due to Ca2+ diffusion and are lower than at the nanodomain range near the Ca2+ channels. Ca2+ microdomains allow vesicle replenishment, a process occurring at the μm scale away from the ribbon and their associated Ca2+ channels (Spassova et al., 2004; Johnson et al., 2005; Graydon et al., 2011). We found that, upon 5 consecutive long 100 ms voltage-step stimulations (from −80 to −10 mV), IHCs displayed brighter transient Ca2+ hot spots (measured in 1 μm2 areas) at their basal synaptic area in P365 mice as compared to young P30 IHCs (Figures 8A,B). This indicated the expression of synaptic Ca2+ microdomains with larger amplitude in old P365 IHCs, a response likely due to a larger Ca2+ channel density at their ribbon active zones (Figure 7A). Simultaneously, in the same cells, the application of the 5 consecutive 100 ms stimulations, generated a facilitated vesicular recruitment in old P365 IHCs (Figure 8D). Also, as a consequence of increased Ca2+ microdomains in old IHCs, the simultaneous recording of Ca2+ currents showed an enhanced time-inactivation, indicative of an increased Ca2+-dependent inactivation due to higher intracellular Ca2+ concentration (Figure 8C).
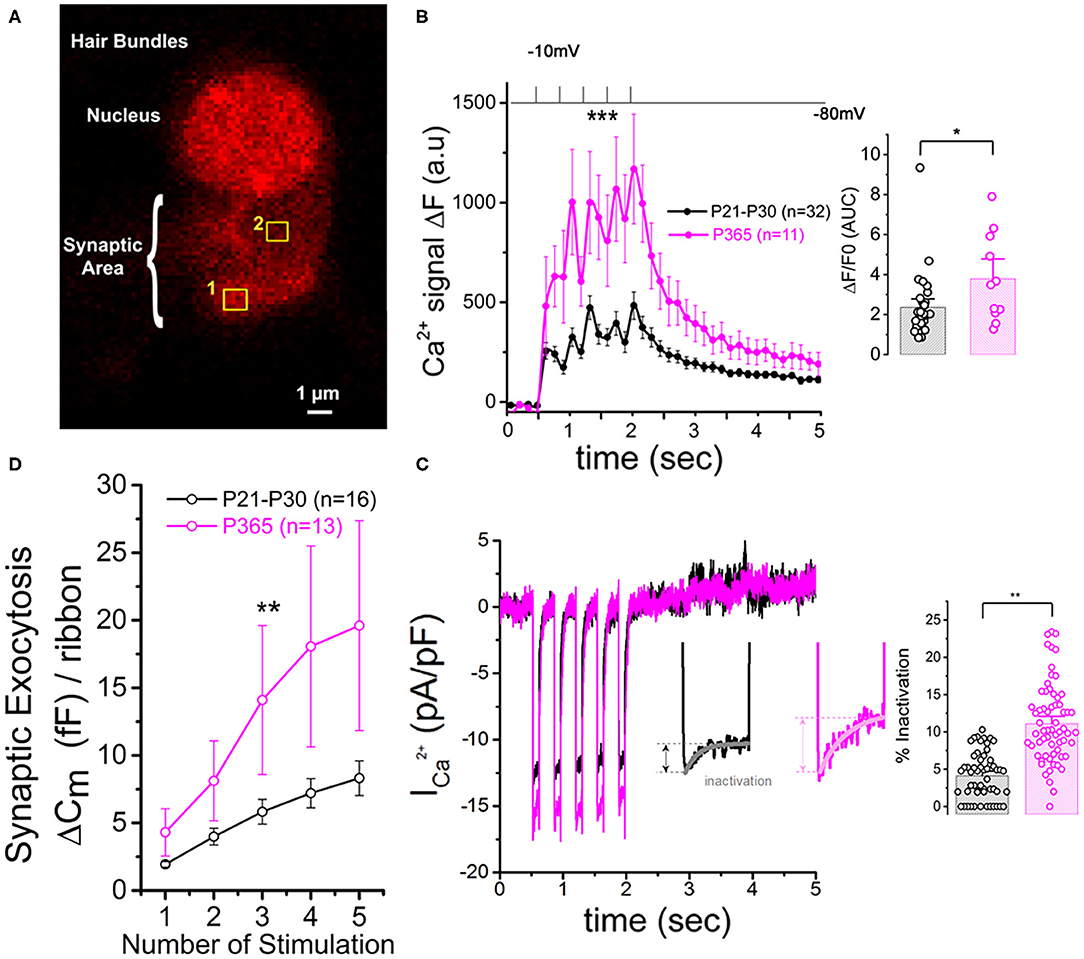
Figure 8. Larger synaptic Ca2+ signaling and increased sustained exocytosis in aged IHCs. (A) Example of confocal Ca2+ imaging, using the intracellular fluorescent Ca2+ indicator Rhod2, in an IHC. The cell was voltage-clamped at −80 mV and stimulated by 5 (100 ms) consecutive voltage-step to −10 mV. The photograph shows two examples of square zones (1 μm2) below the cell nucleus where voltage-induced calcium increase was measured. (B) Comparative mean amplitude of transient fluorescent hot spots showed higher Ca2+ responses at the presynaptic active zones of old P365 IHCs (pink) as compared to young mature P30 IHCs (black). Hair cells were depolarized by 5 five consecutive 100 ms depolarizing steps from −80 to −10 mV. The values n in the graph indicated the number of active zones analyzed for young (six IHCs from six mice) and old mice (five IHCs from five mice), respectively. The peak of the 5 calcium responses (ΔF = F–F0 with F0 as the fluorescent base line value averaged 0.5 s before stimulation) were significantly larger in old IHCs [two-way ANOVA (p = 8.8 E−5)]. The histogram compared the overall fluorescent response ΔF/F0 occurring during the 5 stimulations (0.5–2.5 s). The integral of the Ca2+ response (Area Under the Curve, AUC) was significantly larger in old P365 IHCs (unpaired t-test, p = 0.03). (C) Comparative mean of calcium current recorded at the same time during the five stimulations shown in (B). Note that the inactivating part of the calcium current is increased in P365 IHCs. Asterisk indicate statistical analysis with unpaired t-test. (D) The concomitant exocytosis was also measured during the five stimulations shown in (B), Exocytosis was normalized for each IHC to their ribbon number or cell size; both normalizations giving similar results. The exocytotic responses were larger in old P365 IHCs. The asterisks indicated statistically significant difference with p < 0.05 (two-way ANOVA; p = 0.0015).
Aging Promoted Autophagy in IHCs Through the Recruitment of LC3B
The size reduction of the old IHCs (Figure 6) suggested that the degenerative process of the ribbon synapses was associated with a concomitant reduction of the cell surface membrane of IHCs during aging, suggesting a largely augmented uptake of plasma membrane through endocytosis. Like other type of cells, such as neurons or retinal cells, auditory hair cells utilize autophagic pathways to maintain cell homeostasis during excessive ROS production (Fu et al., 2018; Defourny et al., 2019). Autophagy is a degradation process where cytoplasmic and plasma membrane components are engulfed by vesicles called autophagosomes. To determine whether this process could explain the IHC size reduction with aging, we investigated the recruitment of LC3B, a structural protein of autophagosomal membranes. We assessed and compared the autophagosome numbers and surface area in young P30 and old P365 IHCs by quantifying LC3B puncta numbers/cell by using confocal immunofluoresence. We found that the number and overall surface area of LC3-positive structures in IHCs largely increased with aging (Figure 9), indicating an increased autophagosome formation. Similar investigations in P365 CBA/J mice, a strain which does not display early age-related hearing loss (Figure 1), showed a weak expression of LC3B in IHCs (Figure 9).
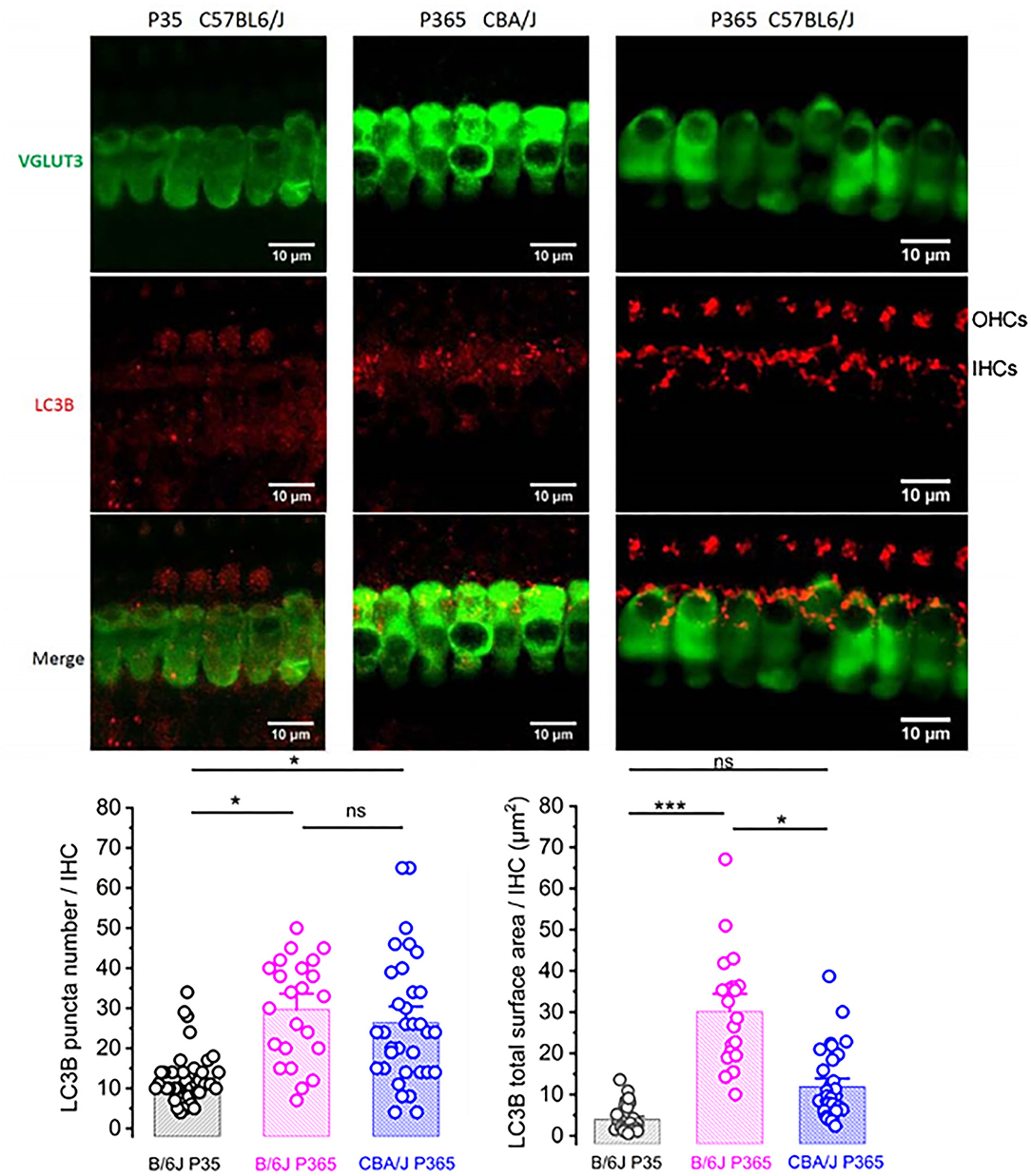
Figure 9. LC3-positive structures are prominent in aging IHCs. Representative confocal immunofluorescence images showing LC3B staining in IHCs from young P30 C57BL/6J (left), old P365 CBA/J (middle), and old P365 C57BL/6J (right) mice. The histograms below show the quantification of the LC3B puncta average number and average mean surface area per IHC. Images were obtained from processing confocal stack images (22 of 0.3 μm thickness) using ImageJ quantification tool (3D-object counter). Quantification was performed from 23 to 38 IHCs for each condition in the mid-cochlea (encoding region 8–16 kHz; 3 mice). Asterisks indicate statistical significance p < 0.05 (unpaired t-test).
Clustering and Voltage-Dependent Activation of BK Channels Are Affected in Aging IHCs
There is increasing evidence that oxydative damage due to an increase in ROS levels plays a crucial role in age-related degeneration of the synaptic ribbon synapses (Fu et al., 2018; Rousset et al., 2020). BK (KCa1.1) channels are known to be highly sensitive to oxydative stress and their activity is a good indicator of intracellular ROS production (Sahoo et al., 2014; Hermann et al., 2015). Interestingly, a decrease in BK channel expression in IHCs has been associated to noise-related ROS and peroxysome pathology (Delmaghani et al., 2015) but never yet described in IHCs during age-relate hearing loss. It is worth recalling that BK channels allows fast repolarization of IHCs and are essential for phase-locking of the auditory afferent neurons (Kros et al., 1998; Skinner et al., 2003; Oliver et al., 2006; Kurt et al., 2012). We here investigated, using immunofluorescent confocal microscopy, the comparative level expression of BK channels in IHCs from young (P30) and old P365 C57BL/6J mice. In young mature IHCs, BK channels are known to be organized in a dozen of clusters at the neck of the IHCs below the cuticular plate (Pyott et al., 2004; Hafidi et al., 2005). We used 3-D reconstructions from stacks of confocal images of immunostained organs of Corti (mid-cochlear frequency region 8–16 kHz) to determine the mean total surface area of the BK clusters per IHCs (Figures 10A–C). The results indicated that the membrane surface covered by BK channel clusters was reduced almost three-fold in P365 IHCs as compared to P30 IHCs.
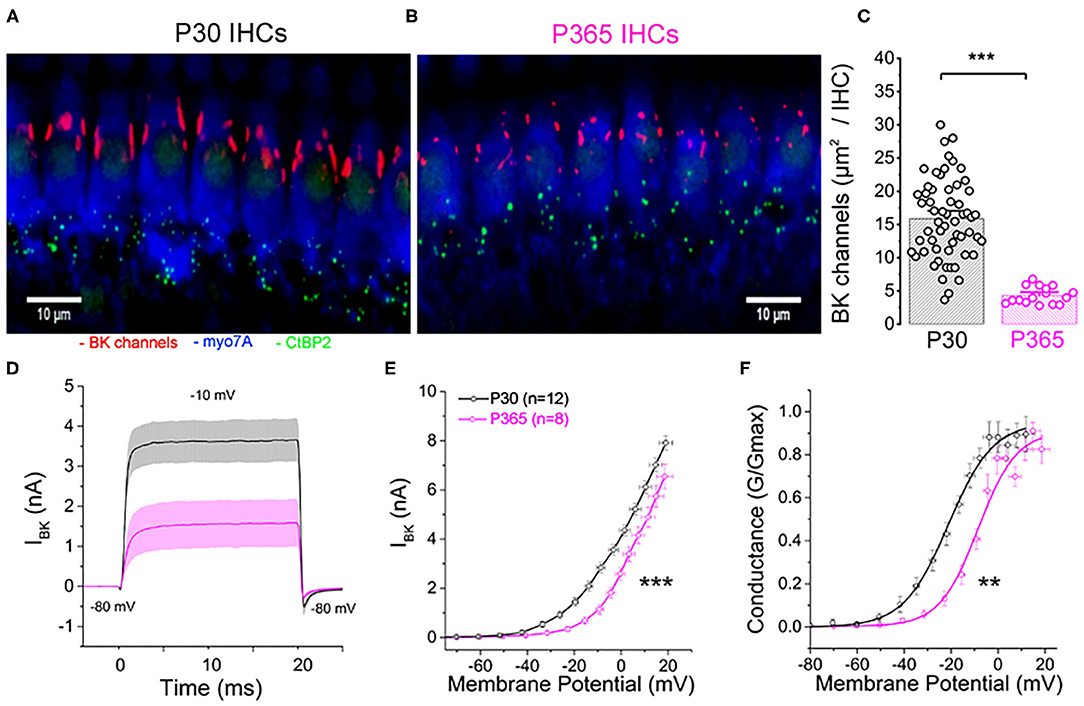
Figure 10. Immunofluorescent confocal imaging of BK channels in aging IHCs. (A) Numerous large BK channel clusters (red) are localized extrasynaptically below the cuticular plate of IHCs in young mature P30 C57BL6/J mice. (B) Note the strong reduction in size of the BK channels clusters in IHCs of old P365 C57BL6/J mice. (C) Histogram comparing the total surface area covered by the BK channel clusters per IHC in the mid-turn cochlea (region encoding 8–16 kHz). The total number of IHCs analyzed was 58 and 16 from P30 (n = 3) and P365 (n = 3) mice, respectively. Asterisks indicate statistical significance with p < 0.05 (p = 1.12 E−7; unpaired t-test). (D) Comparative fast outward potassium currents (IKf), carried by BK channels, recorded from P30 (black, n = 12) and P360 (pink, n = 8) IHCs when using a brief depolarization step of 20 ms from −80 to −10 mV. (E) Comparative BK current–voltage curves (n = 12 and 8 IHCs, respectively, in P30 and P360 mice, from three mice at each age). Significantly different two-way ANOVA, p = 2.7 E−8. IKf was measured 2.5 ms after the onset of the voltage-step stimulation. (F) Comparative BK conductance fitted with a Boltzman function indicated a V1/2 of −20.5 ± 5.7 mV in P30 IHCs and of −13.7 ± 1.4 mV in P365 IHCs, respectively (unpaired t-test, p = 0.009). Note that voltage error across series resistance (Rs = 5 ± 2 MΩ) and leak current were compensated for each IHC. All values are expressed as means ± SEM.
To further characterize the changes in BK channel function with aging, we performed whole cell patch-clamp recordings in IHCs from P30 and P365 mice. Since BK currents in IHCs have the peculiar property to be extremely fast activating currents (also termed IKf; Kros et al., 1998), these currents were recorded during brief 20 ms depolarizing steps and measured 2.5 ms after their onset (Figures 10D–F). BK currents in IHCs from young mature have also the particularity to activate at extremely negative membrane potential (near −60 mV) (Lingle et al., 2019). While the whole-cell conductance of BK channels from P365 IHCs was not significantly different from P30 IHCs (0.26 ± 0.04 nS and 0.23 ±.0.03 nS, respectively), their voltage-dependant activation showed a large positive shift of 7 mV (Figure 10F). Interestingly, the decrease in size of the BK patches and the positive voltage-activation shift in old IHCs somewhat resembled the phenotype observed in mice KO for LRRC52, a γ BK regulatory subunits (Lang et al., 2019; Lingle et al., 2019), suggesting that the BK complex formed with this γ subunit could be one the target of the degenerative oxydative process in aging C57BL56/J mice IHCs.
Discussion
Larger Synaptic Ribbons Associated With Increased Ca2+ Signals
Ribbons are presynaptic organelles tethering glutamate-filled vesicles at the hair cell active zone (AZ, Figure 11). These intracellular structures, composed of the protein RIBEYE, are anchored by the scaffold protein bassoon to the AZ plasma membrane, adjacent to Ca2+ CaV1.3 channels (Brandt et al., 2003; Graydon et al., 2011; Jing et al., 2013; Vincent et al., 2017). Ribbons are essential for high rate of transmitter release and for high temporal precision of neuronal encoding of sound at the auditory afferent nerve fibers (Becker et al., 2018; Jean et al., 2018). Each auditory IHC have 10 to 20 synaptic ribbons depending of their cochlear frequency location (Meyer et al., 2009). Ribbons are heterogeneous in size and each ribbon makes a single synaptic contact with one afferent nerve fiber. Remarkably, fibers associated to large ribbons display low spontaneous rate and high-threshold have the tendency to be distributed at the modiolar side of IHCs (Liberman et al., 2011). Nerve fibers associated with smaller ribbons display high spontaneous rate and low-threshold activation, are mainly located toward the pillar side of IHCs. Interestingly, we found here that old C57BL6/J IHCs preferentially loose modiolar ribbons (known to be associated with high threshold low spontaneous fibers), therefore mimicking what is happening during noise-induced hearing loss (Fernandez et al., 2015; Liberman and Kujawa, 2017; Hickman et al., 2020). Heterogeneity in the voltage-dependence of Ca2+ channels and release site coupling has been recently proposed to contribute to the firing fiber specificity, with modiolar ribbons being activated at more negative potentials (Ozcete and Moser, 2021). In our study, we did not find evidence for a voltage-shift in the voltage-dependence of Ca2+ channels with aging but we did observe an increase in the density of Ca2+ currents. The increase in Ca2+ entry was further confirmed by the stronger time-inactivation of the Ca2+ currents (Grant and Fuchs, 2008; Vincent et al., 2017).
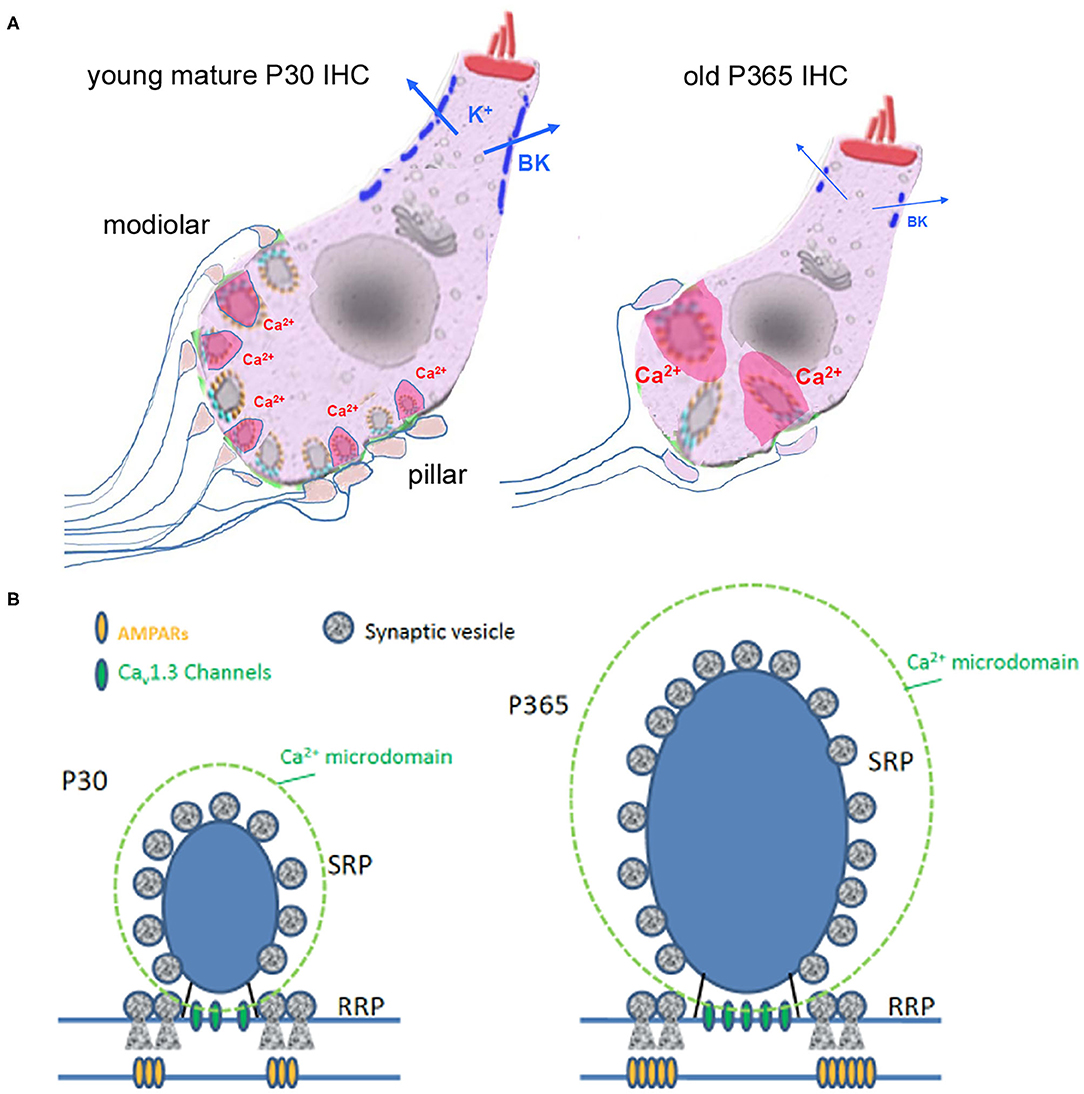
Figure 11. Synaptic IHC degeneration was associated with a strong potentiation of the remaining ribbon synapses in aging C57BL/6J mice. (A) Age-related hearing loss in P360 mice was associated with IHC shrinkage (cell size reduction), an important loss of afferent ribbon synapses (>50%), a drastic enlargement of the surviving synaptic ribbons (both at pillar and modiolar side), an increase in Ca2+ signaling (larger Ca2+ microdomain amplitude as indicated by the larger pink domains around the ribbons) and a large decrease in the fast-repolarizing BK channel clusters (blue bars). (B) Hypothetical view of a young and old IHC ribbon synapse.
One of the most striking observations was that the IHC synaptic ribbons underwent a nearly three-fold increase in size with aging, confirming a certain degree of plasticity of these synaptic structures. The enlargement of the presynaptic ribbons was in good agreement with a previous ultrastructural study in aging C57BL/6J mice by Stamataki et al. (2006) and the recent study of Jeng et al. (2020). Interestingly, the size of the young mature IHC synaptic ribbons is known to correlate positively with the AZ Ca2+ microdomain amplitude, as well as the number and gating of the Cav1.3 Ca2+ channels (Frank et al., 2009; Neef et al., 2018). Also, hair cells of transgenic zebrafish with enlarged ribbons display larger ribbon-localized Ca2+ currents (Sheets et al., 2017). In agreement with these previous studies, we found here, in IHCs from aging C57BL/6J mice, that the remaining larger ribbons were associated with larger Ca2+ microdomain amplitude, and larger density of calcium currents, reinforcing the idea that Ca2+ ions regulate the size of the synaptic ribbons. In this context, It is worth recalling that mitochondrial-Ca2+ uptake has been proposed to lower cellular NAD+/NADH redox and regulates ribbon size, possibly by acting through the NAD+ binding site of the B domain of RIBEYE (Wong et al., 2019). In vitro work has shown that NAD+ and NADH can promote interactions between ribeye domains (Magupalli et al., 2008) and the size of the ribbons would be then sensitive to the subcellular synaptic NAD+/NADH redox level (Wong et al., 2019), suggesting that the increase in size of the IHCs ribbons from aged C57BL/6J mice also results from NAD+/NADH homeostasis disruption.
Increased Capacity for Sustained IHC Synaptic Exocytosis: Implication for the Discharge Pattern of the Auditory Nerve Firing
As a consequence of larger Ca2+ microdomains, we found that IHCs from old C57BL/6J mice had larger and more sustained exocytotic responses. These larger release responses could also well be explained by a higher synaptic vesicle density at the active zone of the IHC ribbon synapses as suggested by the EM study of Stamataki et al. (2006). Surprisingly, the recent study of Jeng et al. (2020), which did not perform Ca2+ imaging, concluded that the size and kinetics of Ca2+-dependent exocytosis in IHCs were unaffected in aging mice but this study did not normalize the IHC exocytotic responses to their cell size or to their ribbon synapse number, and therefore may have overlooked the differential phenotype.
What would be the consequences on the discharge pattern of the remaining afferent fibers of a more sustained release capacity in old ribbon synapses? Fast adaptation in the discharge rate of the afferent auditory nerve fibers is thought to be one of their essential property for emphasizing the timing information of sound stimuli. This adaption takes the form of a fast increase in spike rate at stimulus onset that rapidly declines in a double exponential decay to a lower steady-state rate after a few tens of milliseconds (Taberner and Liberman, 2005). The origin of this fast adaptation has been proposed to arise first from H+ release block of Ca2+ channels and second to rapid depletion of synaptic vesicle release (Vincent et al., 2017). We predict that the increased capacity to sustain SRP exocytosis in old IHCs with larger ribbons would have the tendency to decrease the level of firing adaptation of the auditory fibers and therefore explain the altered recovery from short-term adaptation in old C57BL/6J mice (Walton et al., 1995).
Furthermore, we confirmed here the hyperacusis-like effect of the startle responses in old C57BL/6J mice (Ison et al., 2007). This increased response at low sound intensity could be explained by a release potentiation at the IHC ribbon synapses due to the presynaptic higher Ca2+ channel density associated with a larger ribbon coupled with a larger vesicular SRP, and increased expression of post-synaptic AMPARs. All these features would make the auditory ribbon synapses hypersensitive and underlie the hyperacusis-like reflex in old C57BL/6J mice.
IHC Shrinkage and Disruption of BK Channel Clusters
Another novel observation of our study was the drastic reduction in cell size of IHCs from aging C57BL/6J mice. Interestingly, tuberous sclerosis rapamycin-sensitive complex 1 (mTORC1) has been shown to be overactivated and promote oxidative damages to synapse ribbon loss in aging C57BL/6 mice (Fu et al., 2018). We found that aged P365 IHCs had an increase expression of the autophagy marker LC3B. The activation of the mTOR pathway with aging could therefore trigger the cell size reduction of IHCs, by activating the autophagy pathway, as proposed in many other cell types (Fingar et al., 2002; Fumarola et al., 2005). In addition, our study described another novel remarkable feature of aging IHCs, a drastic disruption of the BK channel clusters, suggesting that these potassium channels are also a good indicator of the redox state and autophagy in IHCs. The expression and distribution of BK channels could be regulated through the rapamycin insensitive mTOR complex 2 (mTORC2) as shown in the kidney glomerulus podocytes (Wang et al., 2019). Interestingly, the disruption of the BK channel clusters in aging IHCs was associated with a large positive shift in their voltage-activation curve, suggesting that the fast-repolarizing property mediated by BK channels which likely contributes to decrease transmitter release (Skinner et al., 2003), would be largely hampered during sound stimuli in aging mice, an additional factor that may also contribute to hyperacusis.
A Role of Cadherin-23 in Ribbon Synapse Maintenance and Function?
In the CNS, cadherins are considered as essential Ca2+-binding transmembrane cell adhesion molecules that regulate synapse formation and function (Bamji, 2005). In the murine retina, cadherin-23 is highly abundant at the ribbon synapse of rod photoreceptor cells (Bolz et al., 2002). The presence of cadherin-23 at IHC ribbon synapses remain unknown. The results observed by our study and others in C57BL/6J mice suggest that this molecule plays a crucial role in hair cell ribbon synapse maintenance and function. It is possible that the Cdh23753A mutation weakens the mechanical organization of the hair cell synaptic contacts leading with aging to their progressive disorganization. The exacerbated release of glutamate in aging ribbon synapses could then lead to excitotoxicity and progressive synapse degeneration.
Conclusions
In conclusion, our study unraveled several novel functional and structural features of aging in IHC ribbon synapses from C57BL/6J mice (Figure 11): (1) a large reduction in IHC cell size, (2) a drastic enlargement of the presynaptic ribbons and postsynaptic AMPAR clusters, (3) an increased presynaptic Ca2+ signaling associated with a stronger sustained exocytotic response, and (4) a disruption of BK channel clusters and a drastic shift in their voltage-dependence decreasing their potential negative feedback on neurotransmission. Overall, these results suggested that aging IHCs ribbon synapses can undergo important structural and functional plasticity that led to synaptic potentiation that could explain the paradoxical hyperacusis-like exaggeration of the acoustic startle reflex observed in C57BL/6J mice.
Materials and Methods
Mice
Inbred C57BL/6J mice of either sex (Souris JAX™ C57BL/6J, Jackson Laboratory) which are homozygous for the recessive cadherin-23 mutation Cdh23753A (also known as Ah1) were used in the present study. Hearing thresholds and IHC ribbon counts in mice of either sex were performed at postnatal ages: P15, P30, P60, P180, and P365. CBA/J mice of either sex (from Charles River) were also tested as controls at P50, P250, and P365. Animal care and all procedures were approved by the institutional care and use committee of the University of Bordeaux and the French Ministry of Agriculture (agreement C33-063-075).
Assessment of Hearing Thresholds (ABRs)
To record ABRs (Auditory Brainstem Responses, which represent the sound-evoked synchronous firing of the auditory cochlear nerve fibers and the activation of the subsequent central auditory relays), mice were anesthetized with intraperitoneal injection of xylazine (6 mg/ml, Rompun Bayer) and ketamine (80 mg/ml, Virbac) mixture diluted in physiological saline. The mouse was placed in a closed acoustic chamber and its body temperature was kept constant at 37°C during ABRs recording. For sound stimulus generation and data acquisition, we used a TDT RZ6/BioSigRZ system (Tucker-Davis Technologies). Click-based ABR signals were averaged after the presentation of a series of 512 stimulations. Thresholds were defined as the lowest stimulus for recognizable wave-I and II. The amplitude of ABR wave-I was estimated by measuring the voltage difference between the positive (P1) and negative (N1) peak of wave-I. Sound intensities of 10–90 dB SPL in 10 dB step, were tested.
Distortion product otoacoustic emissions (DPOAEs), which originate from the electromechanical activity of the outer hair cells (OHCs), were tested by using two simultaneous continuous pure tones with frequency ratio of 1.2 (f 1 = 12.73 kHz and f 2 = 15.26 kHz). DPOAEs were collected with the TDT RZ6/BioSigRZ system designed to measure the level of the “cubic difference tone” 2f 1–f 2.
Acoustic Startle Reflex Measurements
Testing was conducted in four acoustic startle chambers for mice (SR-LAB, San Diego Instruments, San Diego, CA). Each chamber consisted of a nonrestrictive cylindrical enclosure attached horizontally on a mobile platform, which was in turn resting on a solid base inside a sound-attenuated isolation cubicle. A high-frequency loudspeaker mounted directly above the animal enclosure inside each cubicle produced a continuous background noise of 65 dBA and various acoustic stimuli in the form of white noise. Mechanical vibrations caused by the startle response of the mouse were converted into analog signals by a piezoelectric accelerometer attached to the platform. A total of 130 readings were taken at 0.5-ms intervals (i.e., spanning across 65 ms), starting at the onset of the startle stimulus. The average amplitude over the 65 ms was used to determine the stimulus reactivity. The sensitivity of the stabilimeter was routinely calibrated to ensure consistency between chambers and across sessions.
Acoustic startle reflex was assessed during a session lasting for ~30 min, in which the mice were presented with a series of discrete pulse-alone trials of different intensities and durations. Ten pulse intensities were used: 69, 73, 77, 81, 85, 90, 95, 100, 110, and 120 dBA lasting for either 20 or 40 ms (background noise level: 65 dBA). A session began when the animals were placed into the Plexiglas enclosure. The mice were acclimatized to the apparatus for 2 min before the first trial began. The first six trials consisted of six pulse-alone trials of 120 dBA, comprising three trials of each duration. These trials served to stabilize the animals' startle response, and were analyzed separately. Subsequently, the animals were presented with five blocks of discrete test trials. Each block consisted of 20 pulse alone trials, one for each intensity and duration. All trials were presented in a pseudorandom order with an inter-trials interval of 14 s.
Tissue Preparation and Immunocytochemistry
Mice were deeply anesthetized with isofluorane (Vetflurane, Virbac). The ramps of the cochlear apparatus were dissected and prepared as previously described by Vincent et al. (2014). Inner ears (cochleae) were fixed by incubation in 4% paraformaldehyde in phosphate-buffered saline (PBS), pH 7.4, at 4°C overnight and washed with cold phosphate buffered saline (PBS). They were then incubated for 2 days in PBS solution containing 10% EDTA pH 7.4 at 4°C. The middle part of the organ of Corti (area encoding between 8 and 16 kHz) was then dissected and the tectorial membrane removed. The tissue was first incubated with PBS containing 30% normal horse serum and triton X100 0.5 % for 1 h at room temperature. Synaptic ribbons were labeled with anti-CtBP2 (1/200 Goat polyclonal, Santa Cruz, USA; cat # SC-5966 or 1/200 Mouse IgG1 monoclonal antibody BD Biosciences, cat # 612044). Otoferlin was labeled with a mouse monoclonal antibody (1/200 AbCam, cat # ab53233). Autophagy was detected by labeling LC3B protein with anti-Rabbit monoclonal antibody [1/200 LC3B(D11) XP, Cell Signaling Technology, cat # 3868]. BK channels were labeled with a Rabbit polyclonal antibody (1/200, Alomone, Israel, cat #APC-021). Explants of Organ of Corti were incubated overnight at 4°C with primary antibodies. The following fluorescent secondary antibodies 1/500 were then used: anti-Goat Donkey polyclonal Fluoprobes 547H (Interchim, cat# FP-SB2110), anti-Mouse Donkey polyclonal Fluoprobes 647H (Interchim, cat# FP-SC4110), anti-Mouse Donkey polyclonal Alexa Fluor 488 (AbCam, cat# ab150109), anti-Mouse Goat polyclonal Alexa Fluor 546 (Molecular Probes, cat# A-11003), anti-Mouse Goat polyclonal Alexa Fluor 488 (Molecular Probes, cat# A-10680), anti-Rabbit Donkey polyclonal Fluoprobes 594 (Interchim, cat# FP-SD5110). Actin-F was also used to visualize hair cells (1/100, Phalloidin Fluoprobe 405, Interchim, Montlucon, France; cat # FP-CA9870). For Image Acquisition, organ of Corti samples were analyzed using a confocal laser scanning microscope Leica SP8 with a 63X oil immersion objective (NA = 1.4) and white light laser (470–670 nm) (Bordeaux Imaging Center). Phalloidin was imaged by using a diode laser at 405 nm also mounted on the microscope. For complete 3D-stack reconstruction of IHCs, 25–30 images (0.3 μm thickness) were acquired as previously described (Vincent et al., 2014). Ribbon volumes were calculated using the 3D-Objects Counter Plugin of ImageJ. Image resolution was 0.038 μm/pixel. As previously described by Hickman et al. (2020) and Jeng et al. (2020), the x,y,z coordinates of ribbons in each z-stack were transformed into a coordinate system based on the modiolar-pillar polarity of the IHCs. We determined the pillar vs. modiolar distribution of the synaptic ribbons by using a k-means clustering method in the z-stack position of each ribbon, with arbitrarily choosing the number of clusters as 2 (OriginPro 9.1software, OriginLab, Northampton, USA). From these two clusters, we determine arbitrarily the border between the modiolar and pillar side in each IHC by taking the half-distance between the two nearest ribbon of each cluster.
High Resolution Imaging of Synaptic Ribbons With Confocal STED Microscopy
For high-resolution imaging of IHC ribbons, we used a Leica DMI6000 TCS SP8 STED microscope with 93X glycerol immersion objective (NA 1.3) (Bordeaux Imaging Center). Mouse sensory organs were fixed and incubated with primary anti CtBP2 (1/100) antibodies in conditions similar to those described above for confocal microscopy. Secondary antibodies Goat anti- IgG1 Mouse polyclonal Alexa Fluor 647 (Jackson, cat# 115-605-205) were used to label CtBP2 primary antibodies. The pulsed STED depletion laser (pulsed laser IR, Spectra Physics Mai Tai) was set at 775 nm. Stack images were acquired with the following parameters: scan rate 200 Hz, 775 nm depletion laser power between 8 and 15%, scans frames accumulated per X–Y section 8 times, z-step size 0.25 μm, number of plans between 8 and 10, pixel size 20 nm giving an X–Y image size of 21 × 5 μm (1,024 × 256 pixels).
Counting Spiral Ganglion Neurons
The afferent nerve fibers of the auditory nerve were fluorescently labeled using a retrograde track tracing technique with dextran amines (Fritzsch et al., 2016). Cochleae were extracted from temporal bone of freshly euthanized mice and rapidly immerged intact into ice-cold artificial perilymph (NaCl 135; KCl 5.8; CaCl2 1.3; MgCl2 0.9; NaH2PO4 0.7; Glucose 5.6; Na pyruvate 2; HEPES 10, pH 7.4, 305 mOsm). A small crystal of tetramethyl rhodamine dextran amines 3000 (Life technologies, NY) was placed for 15–20 min at the edge of the auditory nerve coming out at the internal auditory canal of the intact cochlea and rinsed with artificial perilymph. The intracochlear sensory tissues were then fixed by gentle perfusion of a 4% PFA solution for 30 min and rinsed with PBS. They were then decalcified for 2 days in PBS solution containing 10% EDTA pH 7.4 at 4°C. The cochleae were then sliced with a razor blade along the longitudinal cochlear axes and the slices were then mounted on a glass-slide for fluorescent confocal microscopy observation. The quantification of the spiral ganglion neurons per surface area of the neural ganglion were made in the middle part of the cochlea in a region encoding 8–16 kHz, by using the 3D object counter application of ImageJ software.
Ca2+ Currents and Whole-Cell Membrane Capacitance Measurement in IHCs
Measurements of resting membrane capacitance of IHCs were performed at P30 and P365 mice in the 20–40% normalized distance from the apex, an area coding for frequencies ranging from 8 to 16 kHz, by using an EPC10 amplifier controlled by Patchmaster pulse software (HEKA Elektronik, Germany), as previously described in detail (Vincent et al., 2014, 2015). The organ of Corti was incubated in an extracellular perilymph-like solution containing NaCl 135; KCl 5.8; CaCl2 5; MgCl2 0.9; NaH2PO4 0.7; Glucose 5.6; Na pyruvate 2; HEPES 10, pH 7.4, 305 mOsm. This extracellular solution was complemented with 0.25 μM of apamin (Latoxan; cat # L8407) and 1 μM of XE-991 (Tocris Bioscience; cat # 2000) to block SK channels and KCNQ4 channels, respectively. The external Ca2+ concentration was increased from 1.3 to 5 mM to enhance the amplitude of Ca2+ currents to levels nearby body temperature. All experiments were performed at room temperature (22–24°C).
Patch pipettes were pulled with a micropipette Puller P-97 Flaming/Brown (Sutter Instrument, Novato, CA, USA) and fire-polished with a Micro forge MF-830 (Narishige, Japan) to obtain a resistance range from 3 to 5 MΩ. Patch pipettes were filled with a cesium-based intracellular solution containing (in mM): CsCl 145; MgCl2 1; HEPES 5; EGTA 1; TEA 20, ATP 2, GTP 0.3, pH 7.2, 300 mOsm. Measurements of the resting membrane capacitance (cell size) of IHCs were obtained in whole-cell voltage-clamp configuration at −70 mV and after 2 min equilibrium of the internal patch-pipette recording solution with the IHC cytosol.
BK Currents
Whole-cell recordings of BK currents were recorded in P30 and P365 IHCs from ex-vivo whole-mount preparation (Skinner et al., 2003) as described above for Ca2+ currents. Recording pipettes were filled with a KCl-based intracellular solution containing in mM: 158 KCl, 2 MgCl2, 1.1 EGTA, 5 HEPES, and 3.05 KOH, pH 7.20.
Intracellular Ca2+ Imaging
Fluorescent Ca2+ signals were measured with a C2 confocal system and NIS-element imaging software (Nikon) coupled to the FN1 upright microscope as previously described (Vincent et al., 2014, 2017). For Ca2+ imaging in IHCs, in which membrane capacitance and Ca2+ currents were simultaneously recorded, patch pipettes were filled with the same cesium-base solution described above supplemented with 50 μM of the red fluorescent indicator Rhod2 (R14220, InvitrogenTM, Thermofisher Scientific). The Ca2+ dye was excited with a 543 nm Helium Neon Laser system (Melles Griot 05-LGR-193-381) coupled to a Nikon C2-confocal FN1- upright microscope and emission fluorescence at 552–617 nm was recorded at 18 images/s (resolution 0.4 μm/pixel). Using the Nikon imaging system NIS-Elements, IHC active zones were first individually identified by their fluorescent transient responses, following a 100 ms stimulation (from −80 to −10 mV) and then the focal plane (with pinhole 1.1 μm) was adjusted in the z dimension to give the brightest value for each active zone. Then, after a 1 min rest, each active zone was stimulated by a train of five consecutive 100 ms stimulation, each separated by 250 ms. Fluorescence emission in each active zone was subsequently measured with ImageJ software by drawing a region of interest of 1 μm2 at the synaptic zone (Figure 8A). Emission fluorescent signals were analyzed and normalized by the ratio ΔF/F0 ratio, where F0 was the fluorescence level before stimulation.
Statistical Analysis
Results were analyzed with OriginPro 9.1software (OriginLab, Northampton, USA). Data were tested for normal distribution using the Shapiro–Wilk normality test, and parametric or nonparametric tests were applied accordingly. For statistical analyses with two data sets, two-tailed unpaired t-tests or two-sided Mann–Whitney tests were used. For comparisons of more than two data sets, one-way ANOVA or two-way ANOVA followed by Tukey multiple comparison test were used when data was normally distributed. If a data set was not normally distributed Kruskal–Wallis tests followed by Dunn multiple comparison tests, respectively, was used. When more than two data sets were compared and significant differences were found, reported p-values correspond to the post-hoc multiple comparison test. Values of p < 0.05 were considered significant. Results are expressed as mean ± SEM.
Data Availability Statement
The raw data supporting the conclusions of this article will be made available by the authors, without undue reservation.
Ethics Statement
The animal study was reviewed and approved by Ethic Committee University of Bordeaux CEEA50.
Author Contributions
DD designed research and wrote the paper. SB, TP, YB, and DD performed research and analyzed the data. SP performed the Acoustic Startle Experiments. All authors contributed to the article and approved the submitted version.
Funding
This work was supported by a grant from the Fondation Pour l'Audition to DD (Starting Grant FPA IDA09 2019-2024, CLINICAL TRANSLA–010703E), and from the French Society of Audioprosthesis professionals, Entendre SAS (Saint Cyr l'Ecole 78210 France). Part of the content of the manuscript has previously appeared as a preprint online at bioRxiv https://doi.org/10.1101/2020.05.15.097550.
Conflict of Interest
The authors declare that the research was conducted in the absence of any commercial or financial relationships that could be construed as a potential conflict of interest.
Publisher's Note
All claims expressed in this article are solely those of the authors and do not necessarily represent those of their affiliated organizations, or those of the publisher, the editors and the reviewers. Any product that may be evaluated in this article, or claim that may be made by its manufacturer, is not guaranteed or endorsed by the publisher.
Abbreviations
ABR, Auditory brainstem responses; AHRL, Age-related hearing loss; ASR, Acoustic startle reflex; AZ, active zone; dB(SPL), decibel Sound Pressure Level; DPOAE, Distorsion product otoacoustic emission; IHC, Inner Hair Cell; OHC, Outer Hair Cell; ROS, reactive oxygen species; RRP, readily releasable pool; SRP, secondarily releasable pool.
References
Bamji, S. X. (2005). Cadherins: actin with the cytoskeleton to form synapses. Neuron 47, 175–8. doi: 10.1016/j.neuron.2005.06.024
Bartolome, M. V., del, C. E., Lopez, L. M., Carricondo, F., Poch-Broto, J., and Gil-Loyzaga, P. (2002). Effects of aging on C57BL/6J mice: an electrophysiological and morphological study. Adv. Otorhinolaryngol. 59, 106–111. doi: 10.1159/000059247
Becker, L., Schnee, M. E., Niwa, M., Sun, W., Maxeiner, S., Talaei, S., et al. (2018). The presynaptic ribbon maintains vesicle populations at the hair cell afferent fiber synapse. Elife 7:e30241. doi: 10.7554/eLife.30241
Beurg, M., Michalski, N., Safieddine, S., Bouleau, Y., Schneggenburger, R., Chapman, E. R., et al. (2010). Control of exocytosis by synaptotagmins and otoferlin in auditory hair cells. J. Neurosci. 30, 13281–13290. doi: 10.1523/JNEUROSCI.2528-10.2010
Bolz, H., Reiners, J., Wolfrum, U., and Gal, A. (2002). “The role of cadherins in Ca2+-mediated cell adhesion and inherited photoreceptor degeneration,” in Photoreceptors and Calcium, Vol. 514, Advances in Experimental Medicine and Biology, eds. W. Baehr and K. Palczewski (Boston, MA: Springer), 399–410.
Brandt, A., Striessnig, J., and Moser, T. (2003). CaV1.3 channels are essential for development and presynaptic activity of cochlear inner hair cells. J. Neurosci. 23, 10832–10840. doi: 10.1523/JNEUROSCI.23-34-10832.2003
Campbell, L. W., Hao, S. Y., Thibault, O., Blalock, E. M., and Landfield, P. W. (1996). Aging changes in voltage-gated calcium currents in hippocampal CA1 neurons. J. Neurosci. 16, 6286–6295. doi: 10.1523/JNEUROSCI.16-19-06286.1996
Defourny, J., Aghaie, A., Perfettini, I., Avan, P., Delmaghani, S., and Petit, C. (2019). Pejvakin-mediated pexophagy protects auditory hair cells against noise-induced damage. Proc. Natl. Acad. Sci. U. S. A. 116, 8010–8017. doi: 10.1073/pnas.1821844116
Del Nido, P. J., Glynn, P., Buenaventura, P., Salama, G., and Koretsky, A. P. (1998). Fluorescence measurement of calcium transients in perfused rabbit heart using rhod 2. Am. J. Physiol. 274, H728–H741. doi: 10.1152/ajpheart.1998.274.2.H728
Delmaghani, S., Defourny, J., Aghaie, A., Beurg, M., Dulon, D., Thelen, N., et al. (2015). Hypervulnerability to sound exposure through impaired adaptive proliferation of peroxisomes. Cell 163, 894–906. doi: 10.1016/j.cell.2015.10.023
Fernandez, K. A., Jeffers, P. W., Lall, K., Liberman, M. C., and Kujawa, S. G. (2015). Aging after noise exposure: acceleration of cochlear synaptopathy in “recovered” ears. J. Neurosci. 35, 7509–7520. doi: 10.1523/JNEUROSCI.5138-14.2015
Fingar, D. C., Salama, S., Tsou, C., Harlow, E., and Blenis, J. (2002). Mammalian cell size is controlled by mTOR and its downstream targets S6K1 and 4EBP1/eIF4E. Genes Dev. 16, 1472–1487. doi: 10.1101/gad.995802
Frank, T., Khimich, D., Neef, A., and Moser, T. (2009). Mechanisms contributing to synaptic Ca2+ signals and their heterogeneity in hair cells. Proc. Natl. Acad. Sci. U. S. A. 106, 4483–4488. doi: 10.1073/pnas.0813213106
Fritzsch, B., Duncan, J. S., Kersigo, J., Gray, B., and Elliott, K. L. (2016). Neuroanatomical tracing techniques in the ear: history, state of the art, and future developments. Methods Mol. Biol. 1427, 243–262. doi: 10.1007/978-1-4939-3615-1_14
Fu, X., Sun, X., Zhang, L., Jin, Y., Chai, R., Yang, L., et al. (2018). Tuberous sclerosis complex-mediated mTORC1 overactivation promotes age-related hearing loss. J. Clin. Invest. 128, 4938–4955. doi: 10.1172/JCI98058
Fumarola, C., La Monica, S., Alfieri, R. R., Borra, E., and Guidotti, G. G. (2005). Cell size reduction induced by inhibition of the mTOR/S6K-signaling pathway protects Jurkat cells from apoptosis. Cell Death Differ. 12, 1344–1357. doi: 10.1038/sj.cdd.4401660
Grant, L., and Fuchs, P. (2008). Calcium- and calmodulin-dependent inactivation of calcium channels in inner hair cells of the rat cochlea. J. Neurophysiol. 99, 2183–2193. doi: 10.1152/jn.01174.2007
Graydon, C. W., Cho, S., Li, G. L., Kachar, B., and von Gersdorff, H. (2011). Sharp Ca(2)(+) nanodomains beneath the ribbon promote highly synchronous multivesicular release at hair cell synapses. J. Neurosci. 31, 16637–16650. doi: 10.1523/JNEUROSCI.1866-11.2011
Hafidi, A., Beurg, M., and Dulon, D. (2005). Localization and developmental expression of BK channels in mammalian cochlear hair cells. Neuroscience 130, 475–484. doi: 10.1016/j.neuroscience.2004.09.038
Hasson, T., Heintzelman, M. B., Santos-Sacchi, J., Corey, D. P., and Mooseker, M. S. (1995). Expression in cochlea and retina of myosin VIIa, the gene product defective in Usher syndrome type 1B. Proc. Natl. Acad. Sci. U. S. A. 92, 9815–9819. doi: 10.1073/pnas.92.21.9815
Henry, K. R. (2002). Sex- and age-related elevation of cochlear nerve envelope response (CNER) and auditory brainstem response (ABR) thresholds in C57BL/6 mice. Hear. Res. 170, 107–115. doi: 10.1016/S0378-5955(02)00391-X
Hequembourg, S., and Liberman, M. C. (2001). Spiral ligament pathology: a major aspect of age-related cochlear degeneration in C57BL/6 mice. J. Assoc. Res. Otolaryngol. 2, 118–129. doi: 10.1007/s101620010075
Hermann, A., Sitdikova, G. F., and Weiger, T. M. (2015). Oxidative stress and maxi calcium-activated potassium (BK) channels. Biomolecules 5, 1870–1911. doi: 10.3390/biom5031870
Hickman, T. T., Hashimoto, K., Liberman, L. D., and Liberman, M. C. (2020). Synaptic migration and reorganization after noise exposure suggests regeneration in a mature mammalian cochlea. Sci. Rep. 10:19945. doi: 10.1038/s41598-020-76553-w
Hu, N., Rutherford, M. A., and Green, S. H. (2020). Protection of cochlear synapses from noise-induced excitotoxic trauma by blockade of Ca(2+)-permeable AMPA receptors. Proc. Natl. Acad. Sci. U. S. A. 117, 3828–3838. doi: 10.1073/pnas.1914247117
Ison, J. R., and Allen, P. D. (2003). Low-frequency tone pips elicit exaggerated startle reflexes in C57BL/6J mice with hearing loss. J. Assoc. Res. Otolaryngol. 4, 495–504. doi: 10.1007/s10162-002-3046-2
Ison, J. R., Allen, P. D., and O'Neill, W. E. (2007). Age-related hearing loss in C57BL/6J mice has both frequency-specific and non-frequency-specific components that produce a hyperacusis-like exaggeration of the acoustic startle reflex. J. Assoc. Res. Otolaryngol. 8, 539–550. doi: 10.1007/s10162-007-0098-3
Jean, P., Lopez de la Morena, D., Michanski, S., Jaime Tobon, L. M., Chakrabarti, R., Picher, M. M., et al. (2018). The synaptic ribbon is critical for sound encoding at high rates and with temporal precision. Elife 7:e29275. doi: 10.7554/eLife.29275
Jeng, J. Y., Carlton, A. J., Johnson, S. L., Brown, S. D. M., Holley, M. C., Bowl, M. R., et al. (2021). Biophysical and morphological changes in inner hair cells and their efferent innervation in the ageing mouse cochlea. J. Physiol. 599, 269–287. doi: 10.1113/JP280256
Jeng, J. Y., Ceriani, F., Olt, J., Brown, S. D. M., Holley, M. C., Bowl, M. R., et al. (2020). Pathophysiological changes in inner hair cell ribbon synapses in the ageing mammalian cochlea. J. Physiol. 598, 4339–4355. doi: 10.1113/JP280018
Jiang, X. W., Li, X. R., and Zhang, Y. P. (2015). Changes of ribbon synapses number of cochlear hair cells in C57BL/6J mice with age(Delta). Int. J. Clin. Exp. Med. 8, 19058–19064.
Jing, Z., Rutherford, M. A., Takago, H., Frank, T., Fejtova, A., Khimich, D., et al. (2013). Disruption of the presynaptic cytomatrix protein bassoon degrades ribbon anchorage, multiquantal release, and sound encoding at the hair cell afferent synapse. J. Neurosci. 33, 4456–4467. doi: 10.1523/JNEUROSCI.3491-12.2013
Johnson, K. R., Erway, L. C., Cook, S. A., Willott, J. F., and Zheng, Q. Y. (1997). A major gene affecting age-related hearing loss in C57BL/6J mice. Hear. Res. 114, 83–92. doi: 10.1016/S0378-5955(97)00155-X
Johnson, S. L., Marcotti, W., and Kros, C. J. (2005). Increase in efficiency and reduction in Ca2+ dependence of exocytosis during development of mouse inner hair cells. J. Physiol. 563(Pt 1), 177–191. doi: 10.1113/jphysiol.2004.074740
Kazmierczak, P., Sakaguchi, H., Tokita, J., Wilson-Kubalek, E. M., Milligan, R. A., Muller, U., et al. (2007). Cadherin 23 and protocadherin 15 interact to form tip-link filaments in sensory hair cells. Nature 449, 87–91. doi: 10.1038/nature06091
Knipper, M., Van Dijk, P., Nunes, I., Ruttiger, L., and Zimmermann, U. (2013). Advances in the neurobiology of hearing disorders: recent developments regarding the basis of tinnitus and hyperacusis. Prog. Neurobiol. 111, 17–33. doi: 10.1016/j.pneurobio.2013.08.002
Kros, C. J., Ruppersberg, J. P., and Rusch, A. (1998). Expression of a potassium current in inner hair cells during development of hearing in mice. Nature 394, 281–284. doi: 10.1038/28401
Kujawa, S. G., and Liberman, M. C. (2015). Synaptopathy in the noise-exposed and aging cochlea: primary neural degeneration in acquired sensorineural hearing loss. Hear. Res. 330(Pt B), 191–199. doi: 10.1016/j.heares.2015.02.009
Kujawa, S. G., and Liberman, M. C. (2019). Translating animal models to human therapeutics in noise-induced and age-related hearing loss. Hear. Res. 377, 44–52. doi: 10.1016/j.heares.2019.03.003
Kurt, S., Sausbier, M., Ruttiger, L., Brandt, N., Moeller, C. K., Kindler, J., et al. (2012). Critical role for cochlear hair cell BK channels for coding the temporal structure and dynamic range of auditory information for central auditory processing. FASEB J. 26, 3834–3843. doi: 10.1096/fj.11-200535
Lagziel, A., Ahmed, Z. M., Schultz, J. M., Morell, R. J., Belyantseva, I. A., and Friedman, T. B. (2005). Spatiotemporal pattern and isoforms of cadherin 23 in wild type and waltzer mice during inner ear hair cell development. Dev. Biol. 280, 295–306. doi: 10.1016/j.ydbio.2005.01.015
Lang, I., Jung, M., Niemeyer, B. A., Ruth, P., and Engel, J. (2019). Expression of the LRRC52 gamma subunit (gamma2) may provide Ca(2+)-independent activation of BK currents in mouse inner hair cells. FASEB J. 33, 11721–11734. doi: 10.1096/fj.201900701RR
Liberman, L. D., Wang, H., and Liberman, M. C. (2011). Opposing gradients of ribbon size and AMPA receptor expression underlie sensitivity differences among cochlear-nerve/hair-cell synapses. J. Neurosci. 31, 801–808. doi: 10.1523/JNEUROSCI.3389-10.2011
Liberman, M. C. (1982). Single-neuron labeling in the cat auditory nerve. Science 216, 1239–1241. doi: 10.1126/science.7079757
Liberman, M. C., and Kujawa, S. G. (2017). Cochlear synaptopathy in acquired sensorineural hearing loss: manifestations and mechanisms. Hear. Res. 349, 138–147. doi: 10.1016/j.heares.2017.01.003
Lingle, C. J., Martinez-Espinosa, P. L., Yang-Hood, A., Boero, L. E., Payne, S., Persic, D., et al. (2019). LRRC52 regulates BK channel function and localization in mouse cochlear inner hair cells. Proc. Natl. Acad. Sci. U. S. A. 116, 18397–18403. doi: 10.1073/pnas.1907065116
Magupalli, V. G., Schwarz, K., Alpadi, K., Natarajan, S., Seigel, G. M., and Schmitz, F. (2008). Multiple RIBEYE-RIBEYE interactions create a dynamic scaffold for the formation of synaptic ribbons. J. Neurosci. 28, 7954–7967. doi: 10.1523/JNEUROSCI.1964-08.2008
Matsubara, A., Laake, J. H., Davanger, S., Usami, S., and Ottersen, O. P. (1996). Organization of AMPA receptor subunits at a glutamate synapse: a quantitative immunogold analysis of hair cell synapses in the rat organ of Corti. J. Neurosci. 16, 4457–4467. doi: 10.1523/JNEUROSCI.16-14-04457.1996
Meyer, A. C., Frank, T., Khimich, D., Hoch, G., Riedel, D., Chapochnikov, N. M., et al. (2009). Tuning of synapse number, structure and function in the cochlea. Nat. Neurosci. 12, 444–453. doi: 10.1038/nn.2293
Michalski, N., Goutman, J. D., Auclair, S. M., Boutet de Monvel, J., Tertrais, M., Emptoz, A., et al. (2017). Otoferlin acts as a Ca(2+) sensor for vesicle fusion and vesicle pool replenishment at auditory hair cell ribbon synapses. Elife 6:e31013. doi: 10.7554/eLife.31013.024
Michel, V., Goodyear, R. J., Weil, D., Marcotti, W., Perfettini, I., Wolfrum, U., et al. (2005). Cadherin 23 is a component of the transient lateral links in the developing hair bundles of cochlear sensory cells. Dev. Biol. 280, 281–294. doi: 10.1016/j.ydbio.2005.01.014
Neef, J., Urban, N. T., Ohn, T. L., Frank, T., Jean, P., Hell, S. W., et al. (2018). Quantitative optical nanophysiology of Ca(2+) signaling at inner hair cell active zones. Nat. Commun. 9:290. doi: 10.1038/s41467-017-02612-y
Noben-Trauth, K., Zheng, Q. Y., and Johnson, K. R. (2003). Association of cadherin 23 with polygenic inheritance and genetic modification of sensorineural hearing loss. Nat. Genet. 35, 21–23. doi: 10.1038/ng1226
Oliver, D., Taberner, A. M., Thurm, H., Sausbier, M., Arntz, C., Ruth, P., et al. (2006). The role of BKCa channels in electrical signal encoding in the mammalian auditory periphery. J. Neurosci. 26, 6181–6189. doi: 10.1523/JNEUROSCI.1047-06.2006
Ouagazzal, A. M., Reiss, D., and Romand, R. (2006). Effects of age-related hearing loss on startle reflex and prepulse inhibition in mice on pure and mixed C57BL and 129 genetic background. Behav. Brain Res. 172, 307–315. doi: 10.1016/j.bbr.2006.05.018
Ozcete, O. D., and Moser, T. (2021). A sensory cell diversifies its output by varying Ca(2+) influx-release coupling among active zones. EMBO J. 40:e106010. doi: 10.15252/embj.2020106010
Pujol, R., and Puel, J. L. (1999). Excitotoxicity, synaptic repair, and functional recovery in the mammalian cochlea: a review of recent findings. Ann. N. Y. Acad. Sci. 884, 249–254. doi: 10.1111/j.1749-6632.1999.tb08646.x
Pyott, S. J., Glowatzki, E., Trimmer, J. S., and Aldrich, R. W. (2004). Extrasynaptic localization of inactivating calcium-activated potassium channels in mouse inner hair cells. J. Neurosci. 24, 9469–9474. doi: 10.1523/JNEUROSCI.3162-04.2004
Rousset, F., Nacher-Soler, G., Coelho, M., Ilmjarv, S., Kokje, V. B. C., Marteyn, A., et al. (2020). Redox activation of excitatory pathways in auditory neurons as mechanism of age-related hearing loss. Redox Biol. 30:101434. doi: 10.1016/j.redox.2020.101434
Roux, I., Safieddine, S., Nouvian, R., Grati, M., Simmler, M. C., Bahloul, A., et al. (2006). Otoferlin, defective in a human deafness form, is essential for exocytosis at the auditory ribbon synapse. Cell 127, 277–289. doi: 10.1016/j.cell.2006.08.040
Sahoo, N., Hoshi, T., and Heinemann, S. H. (2014). Oxidative modulation of voltage-gated potassium channels. Antioxid. Redox Signal. 21, 933–952. doi: 10.1089/ars.2013.5614
Sheets, L., He, X. J., Olt, J., Schreck, M., Petralia, R. S., Wang, Y. X., et al. (2017). Enlargement of ribbons in zebrafish hair cells increases calcium currents but disrupts afferent spontaneous activity and timing of stimulus onset. J. Neurosci. 37, 6299–6313. doi: 10.1523/JNEUROSCI.2878-16.2017
Siemens, J., Lillo, C., Dumont, R. A., Reynolds, A., Williams, D. S., Gillespie, P. G., et al. (2004). Cadherin 23 is a component of the tip link in hair-cell stereocilia. Nature 428, 950–955. doi: 10.1038/nature02483
Skinner, L. J., Enee, V., Beurg, M., Jung, H. H., Ryan, A. F., Hafidi, A., et al. (2003). Contribution of BK Ca2+-activated K+ channels to auditory neurotransmission in the Guinea pig cochlea. J. Neurophysiol. 90, 320–332. doi: 10.1152/jn.01155.2002
Sollner, C., Rauch, G. J., Siemens, J., Geisler, R., Schuster, S. C., Muller, U., et al. (2004). Mutations in cadherin 23 affect tip links in zebrafish sensory hair cells. Nature 428, 955–959. doi: 10.1038/nature02484
Spassova, M. A., Soboloff, J., He, L. P., Hewavitharana, T., Xu, W., Venkatachalam, K., et al. (2004). Calcium entry mediated by SOCs and TRP channels: variations and enigma. Biochim. Biophys. Acta 1742, 9–20. doi: 10.1016/j.bbamcr.2004.09.001
Spongr, V. P., Flood, D. G., Frisina, R. D., and Salvi, R. J. (1997). Quantitative measures of hair cell loss in CBA and C57BL/6 mice throughout their life spans. J. Acoust. Soc. Am. 101, 3546–3553. doi: 10.1121/1.418315
Stamataki, S., Francis, H. W., Lehar, M., May, B. J., and Ryugo, D. K. (2006). Synaptic alterations at inner hair cells precede spiral ganglion cell loss in aging C57BL/6J mice. Hear. Res. 221, 104–118. doi: 10.1016/j.heares.2006.07.014
Taberner, A. M., and Liberman, M. C. (2005). Response properties of single auditory nerve fibers in the mouse. J. Neurophysiol. 93, 557–569. doi: 10.1152/jn.00574.2004
Thibault, O., and Landfield, P. W. (1996). Increase in single L-type calcium channels in hippocampal neurons during aging. Science. 272, 1017–1020. doi: 10.1126/science.272.5264.1017
Vincent, P. F., Bouleau, Y., Charpentier, G., Emptoz, A., Safieddine, S., Petit, C., et al. (2017). Different CaV1.3 channel isoforms control distinct components of the synaptic vesicle cycle in auditory inner hair cells. J. Neurosci. 37, 2960–2975. doi: 10.1523/JNEUROSCI.2374-16.2017
Vincent, P. F., Bouleau, Y., Petit, C., and Dulon, D. (2015). A synaptic F-actin network controls otoferlin-dependent exocytosis in auditory inner hair cells. Elife 4:10988. doi: 10.7554/eLife.10988.008
Vincent, P. F., Bouleau, Y., Safieddine, S., Petit, C., and Dulon, D. (2014). Exocytotic machineries of vestibular type I and cochlear ribbon synapses display similar intrinsic otoferlin-dependent Ca2+ sensitivity but a different coupling to Ca2+ channels. J. Neurosci. 34, 10853–10869. doi: 10.1523/JNEUROSCI.0947-14.2014
Walton, J. P., Frisina, R. D., and Meierhans, L. R. (1995). Sensorineural hearing loss alters recovery from short-term adaptation in the C57BL/6 mouse. Hear. Res. 88, 19–26. doi: 10.1016/0378-5955(95)00093-J
Wan, G., and Corfas, G. (2015). No longer falling on deaf ears: mechanisms of degeneration and regeneration of cochlear ribbon synapses. Hear. Res. 329, 1–10. doi: 10.1016/j.heares.2015.04.008
Wang, Y., Tao, J., Wang, M., Yang, L., Ning, F., Xin, H., et al. (2019). Mechanism of regulation of big-conductance Ca(2+)-activated K(+) channels by mTOR complex 2 in podocytes. Front. Physiol. 10:167. doi: 10.3389/fphys.2019.00167
Willott, J. F. (1996). Anatomic and physiologic aging: a behavioral neuroscience perspective. J. Am. Acad. Audiol. 7, 141–151.
Wong, H. C., Zhang, Q., Beirl, A. J., Petralia, R. S., Wang, Y. X., and Kindt, K. (2019). Synaptic mitochondria regulate hair-cell synapse size and function. Elife 8:e48914. doi: 10.7554/eLife.48914.sa2
Zachary, S. P., and Fuchs, P. A. (2015). Re-emergent inhibition of cochlear inner hair cells in a mouse model of hearing loss. J. Neurosci. 35, 9701–9706. doi: 10.1523/JNEUROSCI.0879-15.2015
Zheng, Q. Y., Johnson, K. R., and Erway, L. C. (1999). Assessment of hearing in 80 inbred strains of mice by ABR threshold analyses. Hear. Res. 130, 94–107. doi: 10.1016/S0378-5955(99)00003-9
Keywords: auditory hair cells, synaptic ribbons, synaptopathy, hyperacusis, Ca2+ channels, exocytosis
Citation: Peineau T, Belleudy S, Pietropaolo S, Bouleau Y and Dulon D (2021) Synaptic Release Potentiation at Aging Auditory Ribbon Synapses. Front. Aging Neurosci. 13:756449. doi: 10.3389/fnagi.2021.756449
Received: 10 August 2021; Accepted: 21 September 2021;
Published: 18 October 2021.
Edited by:
Francesco Panza, University of Bari Aldo Moro, ItalyReviewed by:
Qiaojie Xiong, Stony Brook University, United StatesJun Li, Baylor College of Medicine, United States
Copyright © 2021 Peineau, Belleudy, Pietropaolo, Bouleau and Dulon. This is an open-access article distributed under the terms of the Creative Commons Attribution License (CC BY). The use, distribution or reproduction in other forums is permitted, provided the original author(s) and the copyright owner(s) are credited and that the original publication in this journal is cited, in accordance with accepted academic practice. No use, distribution or reproduction is permitted which does not comply with these terms.
*Correspondence: Didier Dulon, ZGlkaWVyLmR1bG9uQGluc2VybS5mcg==
†These authors have contributed equally to this work and share first authorship