- 1Center for Networked Biomedical Research in Neurodegenerative Diseases, Madrid, Spain
- 2Laboratory of Neurobiology and Experimental Neurology, Department of Basic Medical Sciences, Physiology, Faculty of Medicine, University of La Laguna, San Cristóbal de La Laguna, Spain
Although basal ganglia (BG) are involved in the motor disorders of aged people, the effect of aging on the functional interaction of BG is not well-known. This work was aimed at studying the influence of aging on the functional connectivity of the motor circuit of BG (BGmC). Thirty healthy volunteers were studied (young-group 26.4 ± 5.7 years old; aged-group 63.1 ± 5.8 years old) with a procedure planned to prevent the spurious functional connectivity induced by the closed-loop arrangement of the BGmC. BG showed different functional interactions during the inter-task intervals and when subjects did not perform any voluntary task. Aging induced marked changes in the functional connectivity of the BGmC during these inter-task intervals. The finger movements changed the functional connectivity of the BG, these modifications were also different in the aged-group. Taken together, these data show a marked effect of aging on the functional connectivity of the BGmC, and these effects may be at the basis of the motor handicaps of aged people during the execution of motor-tasks and when they are not performing any voluntary motor task.
Highlights
- The effect of aging on the basal ganglia interactions was studied with neuroimaging methods.
- Aging deteriorates the functional connectivity of basal ganglia.
- Basal ganglia are involved in the motor disorders of aged people.
Introduction
The decline of motor abilities associated with aging normally occurs parallel to changes in different cortical and subcortical motor centers (Fjell and Walhovd, 2010; Seidler et al., 2010). Although changes in the volume (Seidler et al., 2010; Walhovd et al., 2011) and structural connectivity (Bhagat and Beaulieu, 2004; Wang et al., 2010; De Groot et al., 2015; Cox et al., 2016; Behler et al., 2021) of basal ganglia (BG) may be involved in age-associated motor deterioration, the actual role of these changes has not been clearly established. A circumstance that limits the association of the motor handicaps and the BG changes induced by aging is that although motor deterioration is better known (Sun et al., 2012; Ferreira and Busatto, 2013; Mathys et al., 2014; Sala-Llonch et al., 2015; Xiao et al., 2018), the effect of aging on the functional connectivity of BG is less clear. The study of BG activity with magnetic resonance imaging (MRI), and particularly with functional connectivity MRI (fcMRI), has reported inconsistent results indicating an increase (Marchand et al., 2011), a decrease (Taniwaki et al., 2007) or no changes (Baudrexel et al., 2011) in the functional connectivity of BG with aging. This low consistency of fcMRI studies may be associated with age-related changes in the neuro-vascular coupling (Riecker et al., 2003), the small size of some BG (which hampers the grouping of data obtained in different subjects), and the closed-loop wiring of BG (the interaction between two centers may reflect the circulation of information across the BG closed-loop circuit more than their direct interaction).
This work was planned to study the influence of aging on the functional connectivity of the BG regions directly involved in the execution of movements, the BG nuclei included in the BG motor circuit (BGmC). BGmC is a closed-loop circuit composed of projections from the primary motor cortex (M1) to the posterior regions of the putamen (Put), and from this center to the external globus pallidum (GPe), subthalamic nucleus (STN), internal globus pallidum (GPi), substantia nigra (SN), and the motor thalamus (MTal), a thalamic region that sends projections back to the M1 and completes the closed-loop circuit of BG (Alexander et al., 1986; Hoover and Strick, 1993; Delong and Wichmann, 2009). In order to prevent the fcMRI computed between two centers from being “contaminated” by their common interactions with other BG, data used to study the interaction between each two BG were “regressed” with data recorded in all the other BG (partial correlation) (Zhang et al., 2008, 2010). In order to prevent the “contamination” of the blood-oxygen-level-dependent (BOLD) data of the smallest BG (e.g., STN) by those of surrounding structures, the data used to represent the activity of each center was computed by averaging voxels included in a volume-of-interest (VOI) which was located inside each BG of each subject according to previously reported procedures (Rodriguez-Sabate et al., 2015, 2017b). The partial correlation coefficient (CC) was used to estimate the “magnitude” of the functional interaction of BG (Fox and Raichle, 2007), and a block-task paradigm with interleaved “no-motion”/“motion” (hand movements) intervals was used to study the influence of motion on the BG interaction (Fair et al., 2007).
Methods
Subjects
Thirty healthy volunteers 20–67 years of age (15 men and 15 women; 45.1 ± 12.6 years old; mean ± standard deviation) showing: (1) no acute or chronic illness, (2) no history of neurological diseases (they showed a normal neurological examination and no evidence of motor disorders according to the Hoehn and Yahr, the Schwab and England scales), (3) no history of psychiatric diseases (including no evidence of dementia and normal values in the Montreal Cognitive Assessment and the Mini Mental State examinations), (4) normal values in basic laboratory tests, and (5) Normal MRI scans. Written informed consent was provided by all participants, and all procedures were in accordance with the ethical standards of the Declaration of Helsinki. The study was approved by an institutional review board (Human Studies Committee-La Laguna University). Subjects were divided into two groups, the young-group (26.4 ± 5.7 years old; 8 men with an average age of 25.4 and 7 women with an average age of 27.3) and the aged-group (63.1 ± 5.8 years old; 7 men with an average age of 61.7 and 8 women with an average age of 64.5).
Data Collection
The basic experimental procedures were similar to those previously reported (Rodriguez-Sabate et al., 2015, 2017a). Briefly, the BOLD-fluctuation of BG was used to study the functional connectivity of the BGmC in subjects who performed a motor-task or remained at rest. A block-task paradigm with interleaved “no-motion”/“motion” intervals was used. During the motor-task block, subjects performed a repetitive sequence of finger extensions/flexions with the right-hand (from the little finger to the thumb and back to the little finger). During the no-motion block, subjects did not perform any planned task. The transitions between the no-motion and motion time intervals were orally announced by a single word, “MOVE” to start motion and “STOP” to finish motion. One hundred volumes were recorded in each of the four task-blocks. In order to prevent the effects of the transitions between tasks (the change of the BOLD signal baseline may need seconds) the frames 1–10 of each block were not included in the data analysis.
BOLD-contrast images (64 × 64 sampling matrix with voxels of 4 × 4 × 4 mm) were acquired (GE; 3.0 T) in a coronal plane (250 × 250 mm field of view) with gradient-echo (echo-planar imaging; repetition-time 1,600 ms; echo-time 21.6 ms; flip-angle 90°). fMRI data were co-registered with 3D anatomical images (repetition-time 7.6 ms; echo-time 1.6 ms; flip-angle 12°; 250 × 250 mm field of view; 256 × 256 sampling matrix; voxels of 1 × 1 × 1 mm). Functional and anatomical studies were obtained in a single session and with the head fixed in the same position. Different structural markers were jointly used to decide where to place each ROI in the brain of each subject (Rodriguez-Sabate et al., 2017b). Briefly, ROIs were positioned in each center of each subject by using the Talairach coordinates, the shape of the nucleus, and the anatomical relationship of the nucleus with other structures (external cues) as the main indicators (working with normalized 3D-anatomical images). All centers were identified in coronal slices located 4–27 mm posterior to the anterior commissure. The optic tract, internal capsule, and medial forebrain bundle were used as external cues to identify the putamen, GPe, GPi and MTal. GPi was initially identified ≈6 mm posterior to anterior commissure and just over the optic tract. The ROI for the putamen was located at post-commissural level because the somato-sensorimotor regions primarily project to the posterior putamen. The post-commissural putamen was then identified ≈5 mm posterior to anterior commissure. GPe was located ≈3 mm posterior to the anterior commissure. MTal was located ≈11 mm posterior to anterior commissure (5 mm posterior to the GPi). Special care was taken to identify the STN. Three external cues were used to identify the STN, the oculomotor nerve, cerebral peduncle, and pons. Coronal images were initially moved backwards and forwards (between 10 and 18 mm posterior to anterior commissure) to identify the slice where the oculomotor nerve was trapped in the most medial region of the contact between the pons and cerebral peduncle. The backward-forward movements of the slices were also used to identify the slice where the oculomotor nerve was trapped between the pons and cerebral peduncle. The STN was identified, in this slice, as the center located 10 mm medial to the optical tract, just above a horizontal line crossing this tract and near the medial boundary of the cerebral peduncle. The STN, in this position, was mainly surrounded by tracts and located anterior to the SN. So as not to mix data on specific centers with those of the surrounding centers, the ROIs were generally small and clearly located within each nucleus. This was not the case of the SN. In humans, SN pars compacta is intermixed with SN pars reticulata and both portions of the SN cannot be clearly segregated in MRI images. Thus, the ROI in this case included the whole SN, and was located between the red nucleus and posterior commissure in slices −22 to −26 mm posterior to the anterior commissure. The M1 representation of the hand was located in the precentral gyrus, just posterior to the junction of the superior frontal sulcus with the precentral sulcus. Depending on the slice level, the hand representation in M1 has a “zig-zag” or “step-like” shape or a “hook” or “Ω” shape. This distribution was generally observed in a position anterior to the protrusion (“knob”) of the precentral gyrus toward the central sulcus. The fMRI response was also used to verify the location of M1 (10 voxels showing the maximum BOLD response to finger movements) because the comparison of the BOLD signal between the no-motion and the motion intervals clearly showed an activation in hand representation in M1. This is a time consuming method, but it prevents the mixture of different brain regions when BOLD-data are integrated in an experimental group. All data sets were normalized to the Talairach space.
Data Pre-processing
Data were preprocessed (BrainVoyager software) with a slice scan-time correction, a 3D-motion correction, and a temporal filtering (0.009 Hz high-pass GLM-Fourier filter). No spatial smoothing was performed, and studies with a brain-translation >0.5 mm or a brain-rotation >0.5 degrees were rejected. Residual motion artifacts and physiological signals (respiration, cardiac activity) were diminished by regressing the BOLD-signals with the mean average of the BOLD-signals recorded in white matter and brain ventricles (Power et al., 2014).
Correlation Methods
fcMRI was computed with the mean BOLD-signal of voxels included in each VOI, and values of right and left brain centers were grouped together (Gopinath et al., 2011). The Pearson correlation coefficient (r; p < 0.001 two-tailed) was used to estimate the “strength” of the functional connectivity of the BG centers (Statistica-Statsoft, Tulsa). Partial correlations were used to eliminate the collateral influence of the other BG (used as “regressors”) on the functional connectivity between two particular centers, a method which is particularly useful in closed-loop networks where the activity of any center may have time-relationships with the activity of all the other centers of the network (Zhang et al., 2008, 2010).
The motor-task effect and the aging effect on the interaction between two centers were considered to be significant when the change of the partial correlation computed for their BOLD-signals reached statistical significance. Differences between two correlation coefficients were identified by using the r-to-Fisher-z transformation (r′ = 0.5*(ln(1 + r) – ln(1-r); r′ being the Fisher-z transformed r) and a two-sided t comparison (mean and standard error of each sample evaluated against the t distribution with df = n1 + n2 – 2 degrees of freedom; n1 and n2 being the sample sizes) adjusted for multiple comparisons (Greicius et al., 2003).
Results
Table 1 shows the position and size (no. of voxels) of VOIs used to characterize the BOLD activity of BG. No statistical difference was found between the VOI sizes in the young and aged groups.
Functional Connectivity of BG During the No-Motion Intervals
Figure 1 shows the functional connectivity during the no-motion intervals (indicated by the partial correlation coefficient CC) in the young (left) and aged (right) groups. In the young-group, the M1 BOLD-activity showed a significant positive CC with the Put and GPe, and a negative correlation with the SN (Figure 1A). Positive correlations were also found between Put-STN (Figure 1C), GPe-SN (Figure 1E), GPe-GPi (Figure 1E), and STN-GPi (Figure 1G). The GPi, SN, and MTal did not showed any significant correlation between them (Figure 1G).
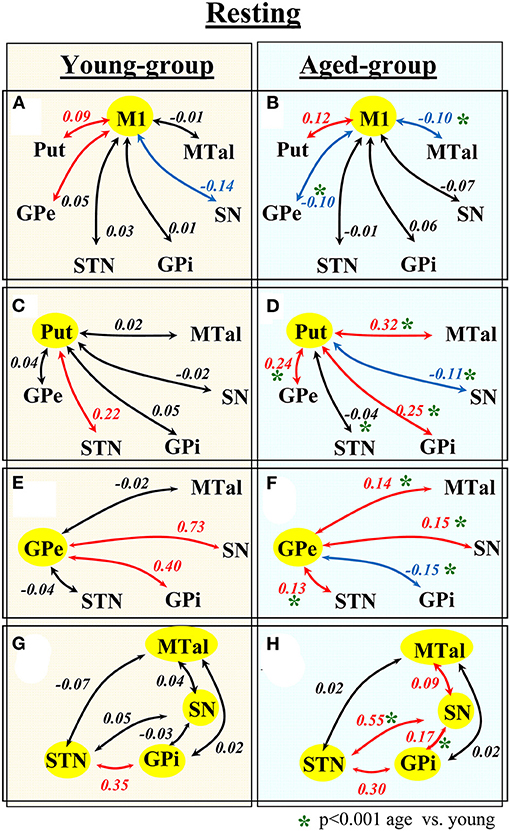
Figure 1. Functional connectivity of BG during task-resting in the young- and aged-groups. Diagrams show the partial correlation coefficient in the young-group (left-hand side) and aged-group (right-hand side). Numbers represent the mean correlation coefficient, in red positive correlations, in blue negative correlations and in black no statistical correlations. Statistical differences between the correlation computed in the young- and aged-groups are shown by green asterisks. M1, primary motor cortex; Put, post-commissural putamen; GPe, external globus pallidum; STN, subthalamic nucleus; GPi, internal globus pallidum; SN, substantia nigral; and MTal, motor thalamus.
The functional connectivity in the aged-group was clearly different to that observed in the young-group. In the aged-group, the M1 showed a significant positive correlation with the Put, and a negative correlation with the GPe and MTal (Figure 1B). The Put showed a positive correlation with the GPe, GPi, and MTal and a negative correlation with the SN (Figure 1D). The GPe showed a positive correlation with the STN, SN and MTal, and a negative correlation with the GPi (Figure 1F). The STN showed a positive correlation with the GPi and SN (Figure 1H). The SN showed a positive correlation with the GPi and MTal (Figure 1H). When compared with the young-group, the aged-group showed a significant increase of positive correlations between Put-GPe, Put-GPi, and Put-MTal (Figures 1C,D), GPe-MTal and GPe-STN (Figures 1E,F), STN-SN, SN-GPi and SN-MTal (Figures 1G,H), and a significant increase of negative correlations between M1-MTal, M1-GPe (Figures 1A,B) and Put-SN (Figures 1C,D). Some positive correlations found in the young-group vanished (Put-STN; Figures 1C,D) or were replaced by negative correlations (GPe-GPi in Figures 1E,F) in the aged-group.
Thus, aging induced a marked reconfiguration of BG activity during the no-motion intervals which in many cases increased the synchronicity of BG (positive correlations), but which in some cases increased their anti-synchronic activity (negative correlations) or replaced their synchronic behavior by anti-synchronic behavior. A summary of these changes is shown in Figure 2, where the significant synchronicity of two nuclei is shown with red lines, and their anti-synchronicity with blue lines. Three chains of BG connections were found in the young-group during the no-motion intervals (Figure 2 left), a M1 ↔ Put ↔ STN ↔ GPi synchronic connection (green area), a M1 ↔ GPe ↔ GPi/SN synchronic connection (blue area), and an M1 ↔ SN anti-synchronic connection (orange area). In the aged-group (Figure 2 right): (1) M1 ↔ Put ↔ STN ↔ GPi synchronicity was replaced by M1 ↔ Put ↔ GPe ↔ STN ↔ GPi synchronicity, (2) M1 ↔ GPe ↔ GPi/SN synchronicity showed a marked decrease of GPe ↔ SN synchronicity and M1 ↔ GPe and GPe ↔ GPi synchronicity were replaced by anti-synchronic activities, and (3) M1-SN anti-synchronicity vanished. These changes were accompanied by a synchronic co-activation of Put-GPi, Put-MTal, GPe-MTal, GPi-SN, SN-MTal, and STN-SN and by an anti-synchronic activation of the Put-SN and MTal-M1.
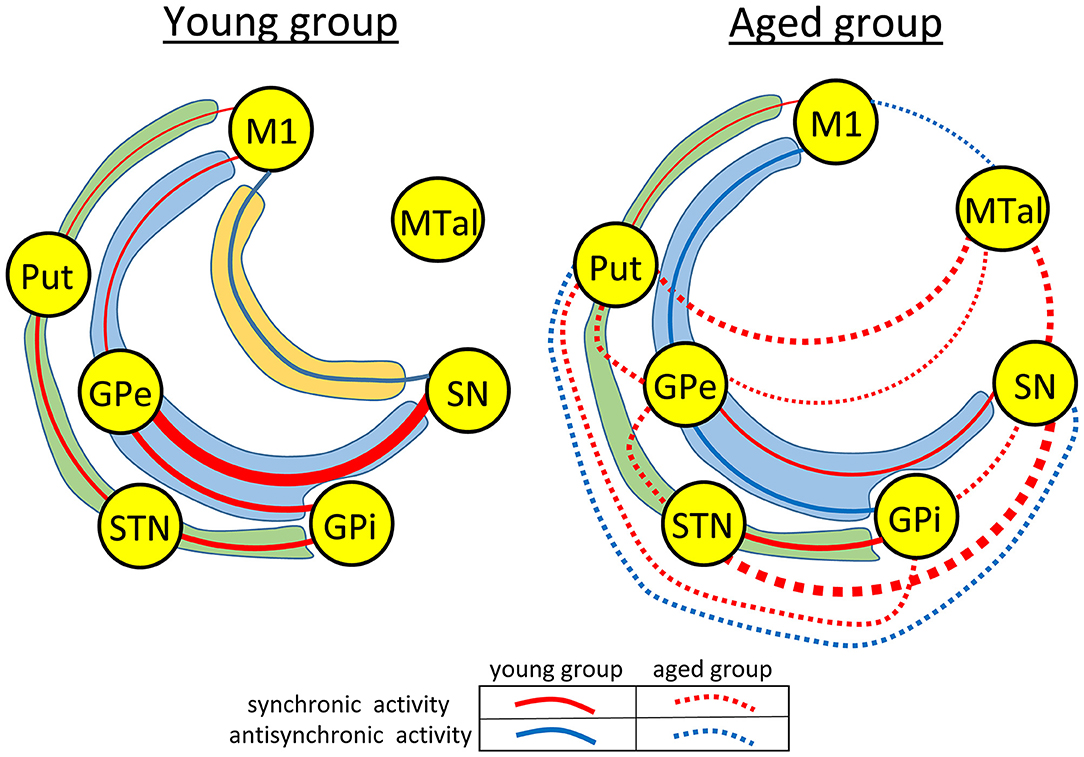
Figure 2. Diagrams showing a summary of the main functional networks of the basal ganglia motor circuit in the young- and aged-groups during the no-motion intervals. Functional relationships with statistical significance are shown with red (synchronic activity) or blue (anti-synchronic activity) lines. The amplitude of correlations is represented by the thickness of the lines connecting the different basal ganglia. In the aged-group (right-side hand), correlations found in both the aged and young groups are shown with continuous lines, and those found in the aged-group but not in the young-group are shown with discontinuous lines. The main functional networks found during the no-motion intervals are grouped within areas of different colors, the M1 ↔ Put ↔ STN ↔ GPi network in green areas, the M1 ↔ GPe ↔ GPi/SN network in blue areas, and the M1-SN network in an orange area. M1, primary motor cortex; Put, post-commissural putamen; GPe, external globus pallidum; STN, subthalamic nucleus; GPi, internal globus pallidum; SN, substantia nigral; and MTal, motor thalamus.
Functional Connectivity of BG During the Motor-Task
The influence of motion on the functional connectivity of BG was tested by comparing the CC computed during the motion and no-motion intervals. Figure 3 shows the CC values in the young-group (Figure 3 left) and aged-group (Figure 3 right), with the CC values computed for the no-motion and motion intervals shown at the top and bottom of each square, respectively. Red numbers show significant positive correlations, blue numbers significant negative correlations and black numbers non-significant correlations. Significant differences in CC computed between the no-motion and motion intervals (asterisk) indicate the effect of motion on the BG functional connectivity. Only the functional relationships which changed with the motor activity are shown in this figure. Red arrows indicate significant changes of positive CC and blue arrows significant changes of negative CC.
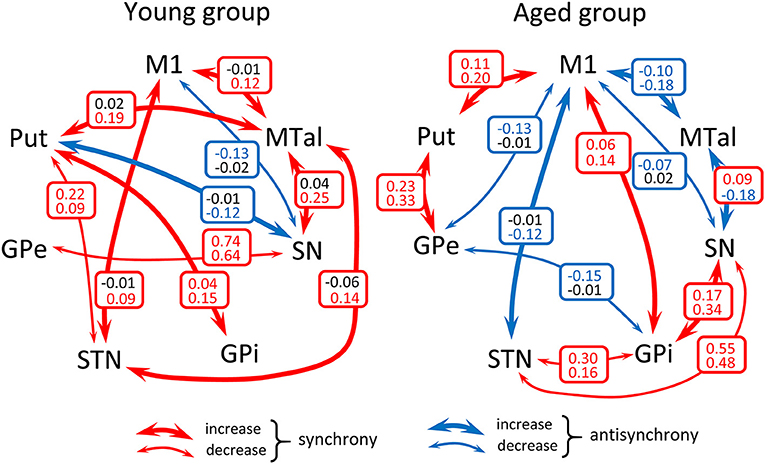
Figure 3. Diagrams showing a summary of the action of motor activity on the functional connectivity of BG in the young- and aged-groups. Numbers show the mean correlation coefficient for each group computed during the no-motion (top of squares) and the motion (bottom of squares) time-intervals in the young-group (left-side hand) and aged-group (right-side hand). Red numbers show significant positive correlations, blue numbers significant negative correlations and black numbers non-significant correlations. Significant differences of the correlation coefficient computed during task-resting and the motor-task are shown by asterisks. Only the functional relationships which changed with the motor activity are shown in this figure (all comparison reached a p < 0.001 statistical significance). Red arrows indicate significant changes of positive correlations and blue arrows significant changes of negative correlations. M1, primary motor cortex; Put, post-commissural putamen; GPe, external globus pallidum; STN, subthalamic nucleus; GPi, internal globus pallidum; SN, substantia nigral; and MTal, motor thalamus.
In the young-group, finger-movements increased the M1-STN, M1-MTal, Put-GPi, Put-MTal, STN-MTal, and SN-MTal synchronicity, increased the Put-SN anti-synchronicity, decreased the Put-STN and GPe-SN synchronicity, and decreased the M1-SN anti-synchronicity. The effect of motion was different in the aged-group, which showed an increase of M1-Put, M1-GPi, Put-GPe, and GPi-SN synchronicity, an increase of the M1-STN, M1-MTal, and SN-MTal anti-synchronicity, a decrease of STN-GPi and STN-SN synchronicity and a decrease of M1-GPe, M1-SN, and GPe-GPi anti-synchronicity.
Discussion
The use of BOLD-data of VOIs located in the main nuclei of the BGmC and of the partial correlation method proved useful to study the effect of aging on the functional interaction of BG. BG showed marked changes in aged people during both the no-motion and motion intervals, suggesting that a different functional connectivity of BGmC nuclei may be at the basis of the motor deterioration induced by aging.
Functional Connectivity of the BGmC in Young People
The classical BG-model is based on excitatory/inhibitory relationships between their nuclei (Alexander et al., 1986; Hoover and Strick, 1993; Delong and Wichmann, 2009). fcMRI does not provide information about the structural connectivity between centers or about the mechanisms involved in their functional interactions. Positive and negative BOLD-correlations do not indicate the existence of excitatory or inhibitory connections, and a high BOLD-correlation between two centers does not necessarily imply their “direct” interaction (the functional connectivity between two centers can be facilitated by other “crossing centers” able to transmit the information between each other). Despite these methodological constraints, fcMRI may reveal some aspects of the functional dynamic of BG that can go unnoticed for the tracing techniques that identify structural connections between brain centers and for the single-unit recordings that identify excitatory-inhibitory interactions between individual neurons of two brain centers. Motor tasks often need seconds to be executed, which allows the identification of task-positive motor networks with fcMRI methods (1.6 s is the time-resolution here). Previous studies have identified functional networks by detecting brain centers with synchronous BOLD-signal fluctuations and a positive correlation between the BOLD-signals of their centers (Biswal et al., 1995; Uddin et al., 2009; Tomasi and Volkow, 2010; Van Dijk et al., 2010; Tomasi et al., 2016). From this point of view, two centers showing no significant correlations do not belong to the same functional network (and could work in parallel without disturbing each other), and two centers showing a negative correlation (anti-synchronous BOLD-fluctuation) could belong to different networks with incompatible activities (Fox et al., 2005; Fair et al., 2007; Uddin et al., 2009; Hampson et al., 2010). In addition, motor networks may maintain their activity during the no-motion intervals, also working when subjects are not performing any voluntary motor task. Therefore, fcMRI is more suitable for analyzing the behavior of neuronal networks than for studying its “wiring” or the mechanisms involved in the interaction of their components. fcMRI data are more suitable to identify functional networks that to identify structural networks or to study the excitatory-inhibitory interactions between the centers of structural networks.
The young-group data suggest that the indirect pathway may work following two different functional arrangement, one involving a M1 ↔ Put ↔ STN ↔ GPi functional connectivity and the other involving a M1 ↔ GPe ↔ SN/GPi functional connectivity. M1 showed an anti-synchronous relationship with SN which suggests that the SN activation is followed by an M1 inactivation, a possibility which agrees with both the classical BG model (the GABAergic projections of SN to MTal inhibits the glutamatergic excitatory action of MTal on M1) (Alexander et al., 1986; Delong, 1990; Parent and Hazrati, 1995; Delong and Wichmann, 2009; Yin, 2017), and the fact that the inhibition of the SN activity (e.g., by inhibiting or lesioning the STN), and the fact that the inhibition of the SN activity (e.g., by inhibiting the STN) increases the M1 activity in Parkinson's disease (Obeso et al., 2000, 2017; Delong and Wichmann, 2009; Rodriguez-Rojas et al., 2018). The functional networks observed here during the no-motion intervals may be involved in the modulation of muscle tone, in the stabilization of body posture or in any function performed by BG when subjects are not executing voluntary motor patterns. The functional interaction of BG changed with the execution of the motor task. The synchronicity between most BG increased with voluntary movements (left-side Figure 3), showing that some nuclei of the BGmC are also involved in task-positive motor networks.
Functional Connectivity of the BGmC in Aged People
The aging processes induced a substantial restructuration of the BG activity during the no-motion intervals. In the M1 ↔ Put ↔ STN ↔ GPi network, the Put ↔ STN synchronicity was replaced by the Put ↔ GPe ↔ STN synchronicity. In the M1 ↔ GPe ↔ SN/GPi network, M1 ↔ GPe ↔ GPi synchronicity was replaced by anti-synchronicity, and GPe ↔ SN synchronicity decreased. The SN-M1 anti-synchronicity observed in the young-group vanished in the aged-group. Additional interactions were observed in the aged-group, including a synchronicity between Put-MTal, Put-GPi, STN-SN, GPe-MTal, GPi-SN and SN-MTal, and anti-synchronicity between Put-SN and MTal-M1 (right-side Figure 3). The BGmC is involved in the stabilization of body posture (Takakusaki, 2017) and the modulation of muscle tone (Takakusaki et al., 2004), physiological functions that could be performed when subjects are not performing voluntary motor tasks. In this case, these motor problems of aged people (Woodhull-Mcneal, 1992; Agyapong-Badu et al., 2016) could be caused by a deficient functional connectivity of the no-motion networks of the BGmC.
The effect of motion on the BGmC was also different in the young and aged groups. BG synchronicities activated by motion in the young groups vanished in the aged group, with a number of anti-synchronicities and some new synchronicities not observed in the young group emerging in the aged-group (Figure 3). The BGmC is involved in the execution of unsupervised automatic motor patterns (Lehericy et al., 2005) which also decline with aging (Hellmers et al., 2018). The deficient functional connectivity of the task-positive motor networks of the BGmC observed here in the age-group could be at the basis of this behavioral problem.
The aged-group had an average age of 63 years old which was enough to find age-related differences with the young-group. These differences could be greater for older people but some misleading variables (e.g., instability of the motor task during the MRI study) could hamper the analysis of results, and therefore no person with more than 70 years of age was included in the study. The young and aged groups had similar compositions of men and women (8 men and 7 women in the young-group and 7 men and 8 women in the aged-group), and differences observed between the young and aged groups cannot be attributed to effects associated with gender. This does not mean that the effect of aging on BGmC is the same in both sexes, a possibility that would require a specific study. Different aged-related neurodegenerative diseases present muscle tone and motor behavior disorders. This is the case of Parkinson's disease, which normally shows slowness movements and muscle rigidity. It has been suggested that Parkinson's disease is produced by an accelerated aging of the brain, and particularly of the dopaminergic cells which control the basal ganglia motor circuit studied here (Rodriguez et al., 2014, 2015). Thus, it is possible that an increase of the aging effects on the basal ganglia motor circuit found here may be at the basis of some of the motor disorders of Parkinson disease.
In summary, present data provide evidence that the partial correlation of fcMRI data may be used to study the interactions of human BG when subjects are not performing voluntary movements, and to identify the modification of these interactions during the execution of particular tasks. This experimental approach even proved to be suitable to study complex closed-loop networks which, as occurs with the BGmC, present multiple structural and functional interactions between their components. The BGmC showed a generalized change of the functional connectivity of its center with aging, an effect observed during both the motion and the no-motion time-intervals. These changes may be at the basis of the movement and posture deterioration observed in age-related neurodegenerative disorders such as Parkinson's disease. New studies in aged people with particular motor handicaps are necessary to understand which BG interaction is involved in each of these problems. A similar study could be performed in Parkinson's patients with slowness movements (bradykinesia) or with a deterioration of the automatic activities which are necessary to maintain body posture.
Data Availability Statement
The original contributions presented in the study are included in the article/supplementary material, further inquiries can be directed to the corresponding author/s.
Ethics Statement
The studies involving human participants were reviewed and approved by an Institutional Review Board (Human Studies Committee-La Laguna University). The patients/participants provided their written informed consent to participate in this study.
Author Contributions
CR-S was involved in the planning and execution of the study, in the recording of data, and in the manuscript review. IM was involved in the execution of the study. MR was involved in the planning of the study, analysis and interpretation of data, and in the manuscript review. All authors contributed to the article and approved the submitted version.
Funding
This work was supported by the Center for Networked Biomedical Research in Neurodegenerative Diseases (CIBERNED; 2021/02), Madrid, Spain, and the Foundation Curemos el Parkinson, La Coruña, Spain.
Conflict of Interest
The authors declare that the research was conducted in the absence of any commercial or financial relationships that could be construed as a potential conflict of interest.
Publisher's Note
All claims expressed in this article are solely those of the authors and do not necessarily represent those of their affiliated organizations, or those of the publisher, the editors and the reviewers. Any product that may be evaluated in this article, or claim that may be made by its manufacturer, is not guaranteed or endorsed by the publisher.
References
Agyapong-Badu, S., Warner, M., Samuel, D., and Stokes, M. (2016). Measurement of ageing effects on muscle tone and mechanical properties of rectus femoris and biceps brachii in healthy males and females using a novel hand-held myometric device. Arch. Gerontol. Geriatr. 62, 59–67. doi: 10.1016/j.archger.2015.09.011
Alexander, G. E., Delong, M. R., and Strick, P. L. (1986). Parallel organization of functionally segregated circuits linking basal ganglia and cortex. Annu. Rev. Neurosci. 9, 357–381. doi: 10.1146/annurev.ne.09.030186.002041
Baudrexel, S., Witte, T., Seifried, C., Von Wegner, F., Beissner, F., Klein, J. C., et al. (2011). Resting state fMRI reveals increased subthalamic nucleus-motor cortex connectivity in Parkinson's disease. Neuroimage 55, 1728–1738. doi: 10.1016/j.neuroimage.2011.01.017
Behler, A., Kassubek, J., and Muller, H. P. (2021). Age-related alterations in DTI metrics in the human brain-consequences for age correction. Front. Aging Neurosci. 13:682109. doi: 10.3389/fnagi.2021.682109
Bhagat, Y. A., and Beaulieu, C. (2004). Diffusion anisotropy in subcortical white matter and cortical gray matter: changes with aging and the role of CSF-suppression. J. Magn. Reson. Imaging 20, 216–227. doi: 10.1002/jmri.20102
Biswal, B., Yetkin, F. Z., Haughton, V. M., and Hyde, J. S. (1995). Functional connectivity in the motor cortex of resting human brain using echo-planar MRI. Magn. Reson. Med. 34, 537–541. doi: 10.1002/mrm.1910340409
Cox, S. R., Ritchie, S. J., Tucker-Drob, E. M., Liewald, D. C., Hagenaars, S. P., Davies, G., et al. (2016). Ageing and brain white matter structure in 3,513 UK Biobank participants. Nat. Commun. 7:13629. doi: 10.1038/ncomms13629
De Groot, M., Ikram, M. A., Akoudad, S., Krestin, G. P., Hofman, A., Van Der Lugt, A., et al. (2015). Tract-specific white matter degeneration in aging: the Rotterdam Study. Alzheimers Dement 11, 321–330. doi: 10.1016/j.jalz.2014.06.011
Delong, M., and Wichmann, T. (2009). Update on models of basal ganglia function and dysfunction. Parkinsonism Relat. Disord. 15(Suppl. 3), S237–240. doi: 10.1016/S1353-8020(09)70822-3
Delong, M. R. (1990). Primate models of movement disorders of basal ganglia origin. Trends Neurosci. 13, 281–285. doi: 10.1016/0166-2236(90)90110-V
Fair, D. A., Schlaggar, B. L., Cohen, A. L., Miezin, F. M., Dosenbach, N. U., Wenger, K. K., et al. (2007). A method for using blocked and event-related fMRI data to study “resting state” functional connectivity. Neuroimage 35, 396–405. doi: 10.1016/j.neuroimage.2006.11.051
Ferreira, L. K., and Busatto, G. F. (2013). Resting-state functional connectivity in normal brain aging. Neurosci. Biobehav. Rev. 37, 384–400. doi: 10.1016/j.neubiorev.2013.01.017
Fjell, A. M., and Walhovd, K. B. (2010). Structural brain changes in aging: courses, causes and cognitive consequences. Rev. Neurosci. 21, 187–221. doi: 10.1515/REVNEURO.2010.21.3.187
Fox, M. D., and Raichle, M. E. (2007). Spontaneous fluctuations in brain activity observed with functional magnetic resonance imaging. Nat. Rev. Neurosci. 8, 700–711. doi: 10.1038/nrn2201
Fox, M. D., Snyder, A. Z., Vincent, J. L., Corbetta, M., Van Essen, D. C., and Raichle, M. E. (2005). The human brain is intrinsically organized into dynamic, anticorrelated functional networks. Proc. Natl. Acad. Sci. USA. 102, 9673–9678. doi: 10.1073/pnas.0504136102
Gopinath, K., Ringe, W., Goyal, A., Carter, K., Dinse, H. R., Haley, R., et al. (2011). Striatal functional connectivity networks are modulated by fMRI resting state conditions. Neuroimage 54, 380–388. doi: 10.1016/j.neuroimage.2010.07.021
Greicius, M. D., Krasnow, B., Reiss, A. L., and Menon, V. (2003). Functional connectivity in the resting brain: a network analysis of the default mode hypothesis. Proc. Natl. Acad. Sci. USA. 100, 253–258. doi: 10.1073/pnas.0135058100
Hampson, M., Driesen, N., Roth, J. K., Gore, J. C., and Constable, R. T. (2010). Functional connectivity between task-positive and task-negative brain areas and its relation to working memory performance. Magn. Reson. Imaging. 28, 1051–1057. doi: 10.1016/j.mri.2010.03.021
Hellmers, S., Izadpanah, B., Dasenbrock, L., Diekmann, R., Bauer, J. M., Hein, A., et al. (2018). Towards an automated unsupervised mobility assessment for older people based on inertial TUG measurements. Sensors (Basel) 18:3310. doi: 10.3390/s18103310
Hoover, J. E., and Strick, P. L. (1993). Multiple output channels in the basal ganglia. Science 259, 819–821. doi: 10.1126/science.7679223
Lehericy, S., Benali, H., Van De Moortele, P. F., Pelegrini-Issac, M., Waechter, T., Ugurbil, K., et al. (2005). Distinct basal ganglia territories are engaged in early and advanced motor sequence learning. Proc. Natl. Acad. Sci. USA. 102, 12566–12571. doi: 10.1073/pnas.0502762102
Marchand, W. R., Lee, J. N., Suchy, Y., Garn, C., Johnson, S., Wood, N., et al. (2011). Age-related changes of the functional architecture of the cortico-basal ganglia circuitry during motor task execution. Neuroimage 55, 194–203. doi: 10.1016/j.neuroimage.2010.12.030
Mathys, C., Hoffstaedter, F., Caspers, J., Caspers, S., Sudmeyer, M., Grefkes, C., et al. (2014). An age-related shift of resting-state functional connectivity of the subthalamic nucleus: a potential mechanism for compensating motor performance decline in older adults. Front. Aging Neurosci. 6, 178. doi: 10.3389/fnagi.2014.00178
Obeso, I., Casabona, E., Rodriguez-Rojas, R., Bringas, M. L., Macias, R., Pavon, N., et al. (2017). Unilateral subthalamotomy in Parkinson's disease: cognitive, psychiatric and neuroimaging changes. Cortex 94, 39–48. doi: 10.1016/j.cortex.2017.06.006
Obeso, J. A., Rodriguez-Oroz, M. C., Rodriguez, M., Macias, R., Alvarez, L., Guridi, J., et al. (2000). Pathophysiologic basis of surgery for Parkinson's disease. Neurology 55, S7–12.
Parent, A., and Hazrati, L. N. (1995). Functional anatomy of the basal ganglia. I. The cortico-basal ganglia-thalamo-cortical loop. Brain Res. Brain Res. Rev. 20, 91–127. doi: 10.1016/0165-0173(94)00007-C
Power, J. D., Mitra, A., Laumann, T. O., Snyder, A. Z., Schlaggar, B. L., and Petersen, S. E. (2014). Methods to detect, characterize, and remove motion artifact in resting state fMRI. Neuroimage 84, 320–341. doi: 10.1016/j.neuroimage.2013.08.048
Riecker, A., Grodd, W., Klose, U., Schulz, J. B., Groschel, K., Erb, M., et al. (2003). Relation between regional functional MRI activation and vascular reactivity to carbon dioxide during normal aging. J. Cereb Blood Flow. Metab. 23, 565–573. doi: 10.1097/01.WCB.0000056063.25434.04
Rodriguez, M., Morales, I., Rodriguez-Sabate, C., Sanchez, A., Castro, R., Brito, J. M., et al. (2014). The degeneration and replacement of dopamine cells in Parkinson's disease: the role of aging. Front. Neuroanat. 8:80. doi: 10.3389/fnana.2014.00080
Rodriguez, M., Rodriguez-Sabate, C., Morales, I., Sanchez, A., and Sabate, M. (2015). Parkinson's disease as a result of aging. Aging. Cell. 14, 293–308. doi: 10.1111/acel.12312
Rodriguez-Rojas, R., Carballo-Barreda, M., Alvarez, L., Guridi, J., Pavon, N., Garcia-Maeso, I., et al. (2018). Subthalamotomy for Parkinson's disease: clinical outcome and topography of lesions. J. Neurol. Neurosurg. Psychiatry 89, 572–578. doi: 10.1136/jnnp-2017-316241
Rodriguez-Sabate, C., Llanos, C., Morales, I., Garcia-Alvarez, R., Sabate, M., and Rodriguez, M. (2015). The functional connectivity of intralaminar thalamic nuclei in the human basal ganglia. Hum. Brain. Mapp. 36, 1335–1347. doi: 10.1002/hbm.22705
Rodriguez-Sabate, C., Morales, I., Sanchez, A., and Rodriguez, M. (2017a). The multiple correspondence analysis method and brain functional connectivity: its application to the study of the non-linear relationships of motor cortex and Basal Ganglia. Front. Neurosci. 11:345. doi: 10.3389/fnins.2017.00345
Rodriguez-Sabate, C., Sabate, M., Llanos, C., Morales, I., Sanchez, A., and Rodriguez, M. (2017b). The functional connectivity in the motor loop of human basal ganglia. Brain Imaging Behav. 11, 417–429. doi: 10.1007/s11682-016-9512-y
Sala-Llonch, R., Bartres-Faz, D., and Junque, C. (2015). Reorganization of brain networks in aging: a review of functional connectivity studies. Front. Psychol. 6:663. doi: 10.3389/fpsyg.2015.00663
Seidler, R. D., Bernard, J. A., Burutolu, T. B., Fling, B. W., Gordon, M. T., Gwin, J. T., et al. (2010). Motor control and aging: links to age-related brain structural, functional, and biochemical effects. Neurosci. Biobehav. Rev. 34, 721–733. doi: 10.1016/j.neubiorev.2009.10.005
Sun, J., Tong, S., and Yang, G. Y. (2012). Reorganization of brain networks in aging and age-related diseases. Aging. Dis. 3, 181–193.
Takakusaki, K. (2017). Functional neuroanatomy for posture and gait control. J. Mov. Disord. 10, 1–17. doi: 10.14802/jmd.16062
Takakusaki, K., Oohinata-Sugimoto, J., Saitoh, K., and Habaguchi, T. (2004). Role of basal ganglia-brainstem systems in the control of postural muscle tone and locomotion. Prog. Brain Res. 143, 231–237. doi: 10.1016/S0079-6123(03)43023-9
Taniwaki, T., Okayama, A., Yoshiura, T., Togao, O., Nakamura, Y., Yamasaki, T., et al. (2007). Age-related alterations of the functional interactions within the basal ganglia and cerebellar motor loops in vivo. Neuroimage 36, 1263–1276. doi: 10.1016/j.neuroimage.2007.04.027
Tomasi, D., Shokri-Kojori, E., and Volkow, N. D. (2016). High-resolution functional connectivity density: hub locations, sensitivity, specificity, reproducibility, and reliability. Cereb Cortex 26, 3249–3259. doi: 10.1093/cercor/bhv171
Tomasi, D., and Volkow, N. D. (2010). Functional connectivity density mapping. Proc. Natl. Acad. Sci. USA. 107, 9885–9890. doi: 10.1073/pnas.1001414107
Uddin, L. Q., Kelly, A. M., Biswal, B. B., Xavier Castellanos, F., and Milham, M. P. (2009). Functional connectivity of default mode network components: correlation, anticorrelation, and causality. Hum. Brain. Mapp. 30, 625–637. doi: 10.1002/hbm.20531
Van Dijk, K. R., Hedden, T., Venkataraman, A., Evans, K. C., Lazar, S. W., and Buckner, R. L. (2010). Intrinsic functional connectivity as a tool for human connectomics: theory, properties, and optimization. J. Neurophysiol. 103, 297–321. doi: 10.1152/jn.00783.2009
Walhovd, K. B., Westlye, L. T., Amlien, I., Espeseth, T., Reinvang, I., Raz, N., et al. (2011). Consistent neuroanatomical age-related volume differences across multiple samples. Neurobiol. Aging. 32, 916–932. doi: 10.1016/j.neurobiolaging.2009.05.013
Wang, Q., Xu, X., and Zhang, M. (2010). Normal aging in the basal ganglia evaluated by eigenvalues of diffusion tensor imaging. Am. J. Neuroradiol. 31, 516–520. doi: 10.3174/ajnr.A1862
Woodhull-Mcneal, A. P. (1992). Changes in posture and balance with age. Aging (Milano) 4, 219–225. doi: 10.1007/BF03324095
Xiao, T., Zhang, S., Lee, L. E., Chao, H. H., Van Dyck, C., and Li, C. R. (2018). Exploring age-related changes in resting state functional connectivity of the amygdala: from young to middle adulthood. Front. Aging Neurosci. 10:209. doi: 10.3389/fnagi.2018.00209
Yin, H. H. (2017). The basal Ganglia in action. Neuroscientist. 23:299–313. doi: 10.1177/1073858416654115
Zhang, D., Snyder, A. Z., Fox, M. D., Sansbury, M. W., Shimony, J. S., and Raichle, M. E. (2008). Intrinsic functional relations between human cerebral cortex and thalamus. J. Neurophysiol. 100, 1740–1748. doi: 10.1152/jn.90463.2008
Keywords: aging, basal ganglia, functional connectivity, hand motion, resting state
Citation: Rodriguez-Sabate C, Morales I and Rodriguez M (2022) The Influence of Aging on the Functional Connectivity of the Human Basal Ganglia. Front. Aging Neurosci. 13:785666. doi: 10.3389/fnagi.2021.785666
Received: 29 September 2021; Accepted: 14 December 2021;
Published: 12 January 2022.
Edited by:
Henning Müller, University of Applied Sciences and Arts of Western Switzerland, SwitzerlandReviewed by:
Jan Kassubek, University of Ulm, GermanyKaundinya S. Gopinath, Emory University, United States
Copyright © 2022 Rodriguez-Sabate, Morales and Rodriguez. This is an open-access article distributed under the terms of the Creative Commons Attribution License (CC BY). The use, distribution or reproduction in other forums is permitted, provided the original author(s) and the copyright owner(s) are credited and that the original publication in this journal is cited, in accordance with accepted academic practice. No use, distribution or reproduction is permitted which does not comply with these terms.
*Correspondence: Manuel Rodriguez, bXJkaWF6QHVsbC5lZHUuZXM=