Inhibition of phosphodiesterase: A novel therapeutic target for the treatment of mild cognitive impairment and Alzheimer’s disease
- 1Department of Gastroenterology, The People’s Hospital of Yichun City, Yichun, China
- 2Department of Neuroscience, City University of Hong Kong, Kowloon Tong, Hong Kong SAR, China
- 3Department of Biochemistry, Davangere University, Davangere, India
Alzheimer’s disease (AD) is the most common form of dementia and is ranked as the 6th leading cause of death in the US. The prevalence of AD and dementia is steadily increasing and expected cases in USA is 14.8 million by 2050. Neuroinflammation and gradual neurodegeneration occurs in Alzheimer’s disease. However, existing medications has limitation to completely abolish, delay, or prevent disease progression. Phosphodiesterases (PDEs) are large family of enzymes to hydrolyze the 3’-phosphodiester links in cyclic adenosine monophosphate (cAMP) and cyclic guanosine monophosphate (cGMP) in signal-transduction pathways for generation of 5’-cyclic nucleotides. It plays vital role to orchestrate several pharmacological activities for proper cell functioning and regulating the levels of cAMP and cGMP. Several evidence has suggested that abnormal cAMP signaling is linked to cognitive problems in neurodegenerative disorders like AD. Therefore, the PDE family has become a widely accepted and multipotential therapeutic target for neurodegenerative diseases. Notably, modulation of cAMP/cGMP by phytonutrients has a huge potential for the management of AD. Natural compounds have been known to inhibit phosphodiesterase by targeting key enzymes of cGMP synthesis pathway, however, the mechanism of action and their therapeutic efficacy has not been explored extensively. Currently, few PDE inhibitors such as Vinpocetine and Nicergoline have been used for treatment of central nervous system (CNS) disorders. Considering the role of flavonoids to inhibit PDE, this review discussed the therapeutic potential of natural compounds with PDE inhibitory activity for the treatment of AD and related dementia.
Introduction
Alzheimer’s disease (AD) is a neurodegenerative cognitive disorder affecting around 36 million individuals around the world. By 2050, this number is expected to quadruple (Ribaudo et al., 2020). AD is characterized by memory loss, inability to learn, prohibiting their normal communication, prevent daily activity over the time and significantly reduces the patients’ quality of life (Pratap et al., 2017). Cholinergic transmission and glycation dysfunction, amyloid formation, and oxidative stress have been implicated for disease’s onset and progression (Ribaudo et al., 2021). Cholinesterase inhibitors have been approved by Food and Drug Administration (FDA, USA) for the treatment of mild to moderate AD. Memantine, an N-methyl-D-aspartate receptor non-competitive antagonist, is another treatment option for moderate to severe AD (Geerts and Grossberg, 2006). “Disease-modifying” drugs prevents or at least effectively modify the course of AD, are still under investigations (Munoz-Torrero, 2008). These drugs interfere with the pathogenesis factors responsible for clinical symptoms such as production of extracellular amyloid plaques, intracellular neurofibrillary tangles, inflammation, oxidative damage, cholesterol metabolism and prevent the disease progression (Parihar and Hemnani, 2004). Currently available drugs do not cure AD; however, symptoms are alleviated for a brief time. Therefore, it is imperative to explore the novel targets and therapeutics agents from natural compounds for treatment of AD and dementia (Yiannopoulou and Papageorgiou, 2013).
Phosphodiesterases (PDEs) are intracellular enzymes and recently gained attention as potential therapeutic targets for the treatment of cognitive loss in aging and AD (Heckman et al., 2017). The PDE family has been reported as multipotential target in several neurological disorders (Nabavi et al., 2019). PDEs degrades cyclic nucleotides, e.g., cyclic-AMP (cAMP) and cyclic-GMP (cGMP). The role of PDEs in cognitive enhancement has recently been discovered in a transgenic fly model of learning defects (Tibbo et al., 2019). Abnormal cAMP and/or cGMP signaling are associated with cognitive impairment in neurodegenerative disorders (Di Benedetto et al., 2021). Dysfunction of signal transduction in disease condition are caused by abnormal PDE function, resulting uncoordinated cAMP and cGMP in memory related brain areas, enhanced Aβ production and disrupt the memory formation (Nabavi et al., 2019). Memory loss tends to occur before nerve cell death in AD, suggesting that neuronal dysfunction as key pathological factor. Therefore, PDE inhibitors could be postulated to improve AD symptoms by restoring synaptic function due to restoration of cyclic AMP response element-binding protein (CREB) signaling pathway (García-Osta et al., 2012).
PDEs have been emerging as interesting targets for the treatment of neurodegenerative disease such as AD. Some small molecule compounds targeting PDE and its isoforms could be an effective anti-Alzheimer’s agents (Heckman et al., 2017; Ribaudo et al., 2021). In this review, we discussed the mechanisms of PDE inhibitors (PDE-Is) of natural and synthetic compounds based on the currently available literature with preclinical and clinical data.
Methodology
Online literature was retrieved by using well-known scientific search engines such as Google Scholar, PubMed, Elsevier journal, EMBASE, Science Direct, Book chapters, Springer Link, Elsevier, Taylor and Francis, ACS, Wiley publishers and scientific literature, as well as reports and documentation from government organizations, were assessed. The results were cross-referenced, resulting in a total of 144 references listed in this review, spanning the years 1996–2022. The current review discussed the therapeutic potential of PDE inhibitor in mild cognitive impairment (MCI) and AD. Tables 1–3 shows the natural and synthetic compounds and mode of action in the inhibition of PDE.
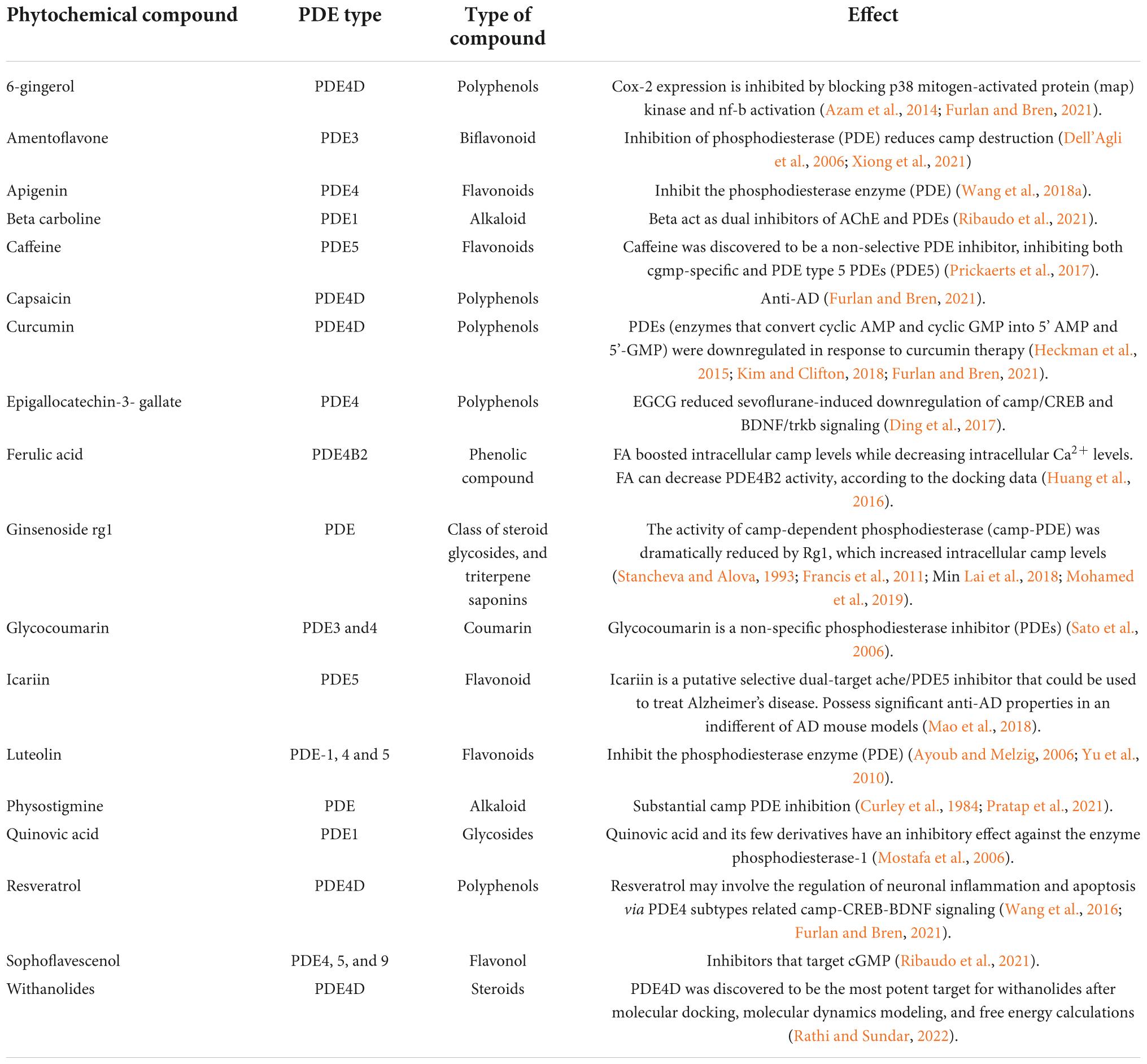
Table 3. List of natural products for PDE inhibitors and their impact on cognitive enhancement in clinical research, with a focus on Alzheimer’s disease.
Phosphodiesterases and their inhibitors
PDEs (cyclic nucleotide phosphodiesterases) are enzymes to catalyze the hydrolysis of cAMP and cGMP and terminate cyclic nucleotide signaling (Zuo et al., 2019). PDEs are classified into 11 subtypes based on their structural and functional characteristics (PDE1-PDE11) (Amin et al., 2018). Inhibition of PDEs, activation of AC/cAMP/PKA, or NO/cGMP/PKG (Francis et al., 2010) signaling pathways increases cAMP and cGMP levels in the brain by increasing the level of the CREB, improving the synaptic transmission, and lower cognitive deficits (Bollen et al., 2014). Preclinical models and clinical studies of AD have reported that PDE inhibitors such as PDE1, PDE2, PDE4, PDE5, PDE9, and PDE10 improve the cognitive deficits (Zhang et al., 2018). Several PDE subfamilies are abundantly expressed in the human brain, suggesting the association of PDE inhibition in neurodegenerative processes through regulation of cAMP and/or cGMP levels. PDEs are currently considered to be attractive targets for the treatment of Alzheimer’s disease, as several PDE inhibitors have been proven to improve cognitive function (Wu et al., 2018). PDE antagonists has also been suggested as multi-target medications for neurodegenerative disorders (González et al., 2019). It has potential to cross the blood–brain barrier and attain optimum inhibitory concentrations in the central nervous system (CNS) to exert therapeutic effects. Several molecules and available medications, such as Sildenafil, Vardenafil, and Tadalafil have been exploited as PDE inhibitors in AD treatment (Ribaudo et al., 2020).
PDEs are categorized into three class: PDEI, PDEII, and PDEIII. Class I PDEs in mammals have half HD domain in the C-terminal and shows a strong affinity for cAMP and/or cGMP (Omori and Kotera, 2007). Half of N-terminal region of the protein regulates PDE enzymatic activity and subcellular localization. Protein kinases targeting phosphorylation sites and lipid modification sites are present in some PDEs (Omori and Kotera, 2007; Lorigo et al., 2022).
In humans, rats, and mice, twenty-one different class I PDE genes have been discovered. They are divided into 11 groups (Table 1) based on structural similarities like sequence homology, protein domains, and enzymatic features including sensitivity to endogenous regulators and inhibitors (Puzzo et al., 2008). The C-terminal catalytic region contains around 270 amino acids are conserved among PDE families, with 35–50% identical sequence (Fisher et al., 1998). Some PDE families are made up of two to four subfamily genes that share more than 70% sequence and have the same protein domain organization (Degerman et al., 1997; Epstein, 2017; Gu et al., 2022).
Characteristics of phosphodiesterases gene families
PDEs are important regulators of intracellular cAMP and cGMP concentrations to modulate their signaling pathways and physiologic effects (Lugnier, 2006; Peiró et al., 2011). There are more than 20 genes in mammals to encode over 50 distinct PDE proteins that are presumably produced in mammalian cells, with each family including one to four genes (Bender and Beavo, 2006; Lugnier, 2006). PDE1 to PDE6 isoforms were first well-characterized due to their prevalence in many tissues and cells, their unique contribution to tissue function and regulation in pathophysiology such as inflammation, neurodegeneration, and cancer (Lugnier, 2006). However, the role of newly identified PDE7 to PDE11 families have not been determined (Pdes et al., 2014). The potential roles of PDE1-11 in signaling pathways and their targets have been discussed below.
Phosphodiesterase 1: It resembles PDEs encoded by PDE1A to -C and known to hydrolyze cAMP and cGMP in the presence of Ca2+ /CaM. PDE1A has a strong affinity for cGMP in humans (Loughney et al., 1996; Omori and Kotera, 2007).
Phosphodiesterase 2: phosphodiesterase 2 (PDE2A) hydrolyzes both cGMP and cAMP at same rates (Pavlaki and Nikolaev, 2018). cGMP binding to PDE2A’s GAF domain allosterically stimulates for mutual control of cAMP and cGMP signaling (Omori and Kotera, 2007).
Phosphodiesterase 3: PDE3A and PDE3B are subfamily genes of PDE3, a protein that binds to both cAMP and cGMP with a high affinity (Heikaus et al., 2009). PDE3s are known as cGMP-inhibited cAMP PDEs (Degerman et al., 1997). The PDE3 family is distinguished by the presence of a 44-amino acids inserted in the catalytic domain. The presence of N-terminal hydrophobic membrane attachment regions (NHRs) is another distinguishing feature (Omori and Kotera, 2007).
Phosphodiesterase 4: PDE4A to-D are four closely related subfamily genes that encode cAMP-specific Rolipram-sensitive PDEs (Table 1). Splice variants in the PDE4 family are divided into three N-terminal variant groups depending on the presence or absence of N-terminal Upstream Conserved Regions (UCR) domains (Omori and Kotera, 2007).
Phosphodiesterase 5: PDE5A has two GAF domains in its half N-terminal and hydrolyzes cGMP selectively. This enzyme’s allosteric binding to cGMP domain suggests PDE5A as a cGMP-binding cGMP-specific PDE (Heikaus et al., 2009). The activation of PDE5A enzyme is linked to PKG and PKA-dependent phosphorylation site in the N-terminal region. cGMP binding to PDE5A/GAFA phosphorylation is promoted by a domain, which enhances the catalytic function along with increased the cGMP binding affinities (Omori and Kotera, 2007; Ahmed et al., 2021; Gu et al., 2022).
Phosphodiesterase 6: The level of cGMP, a second messenger in visual signal transduction, is closely controlled in retinal rod and cone cells by three PDE6 subfamily genes that govern cGMP hydrolysis. PDE6 activity is regulated by inhibitory subunits, which is a unique feature of this PDE family (Cote, 2006). Sildenafil, and vardenafil, but not tadalafil, have lower affinity for inhibiting PDE6 than PDE5A (Omori and Kotera, 2007; Wilkins et al., 2008).
Phosphodiesterase 7: PDE7A and PDE7B are Rolipram insensitive, high-affinity cAMP-specific PDEs. PKA pseudo substrate site exists at the N terminus of the PDE7A subfamily. Dipyridamole has a non-selective inhibitory effect on PDE7 activity (Ahlström, 2001; Omori and Kotera, 2007).
Phosphodiesterase 8: PDE8 is divided into two subfamilies: PDE8A and PDE8B. PDE8s are cAMP-specific PDEs with N-terminal REC (cheY-homologous receiver) and PAS (per-arnt-sim) domains that are resistant to Rolipram and 3-isobutyl-1-methylxanthine (IBMX) (Omori and Kotera, 2007).
Phosphodiesterase 9: PDE9A has a high affinity for cGMP hydrolysis. However, there are no evidence of PDE9A activity regulation or the presence of endogenous PDE9A activity in tissue or cell extracts (Omori and Kotera, 2007).
Phosphodiesterase 10: PDE10A hydrolyzes both cAMP and cGMP and has two GAF domains at the N-terminus (Soderling et al., 1999). cAMP stimulates the enzymatic activity of a chimeric PDE10A GAF domain and cyanobacterial adenylyl cyclase, suggesting that cAMP may acts as an allosteric modulator of PDE10A activity (Omori and Kotera, 2007; Schultz and Natarajan, 2013).
Phosphodiesterase 11: PDE11A4 is a full-length version with two GAF domains and a catalytic domain. In tissue or cell extracts, PDE11A activity has yet to be confirmed. Tadalafil has been proven to suppress PDE11A activity and with less potency than PDE5A (Omori and Kotera, 2007; Schultz and Natarajan, 2013).
Role of phosphodiesterases and their inhibitors in Alzheimer’s disease
Phosphodiesterases (PDEs) are important regulators of cyclic nucleotide-mediated signaling levels (Barnes, 1995). cAMP and cGMP signaling have been linked to neuroplasticity and protection (Figure 1). Usage of PDEs inhibitors to manipulate cAMP and cGMP levels in the cell has become a popular strategy for treatment of various neurodegenerative disorders such as AD (Bollen and Prickaerts, 2012; Hesse et al., 2017).
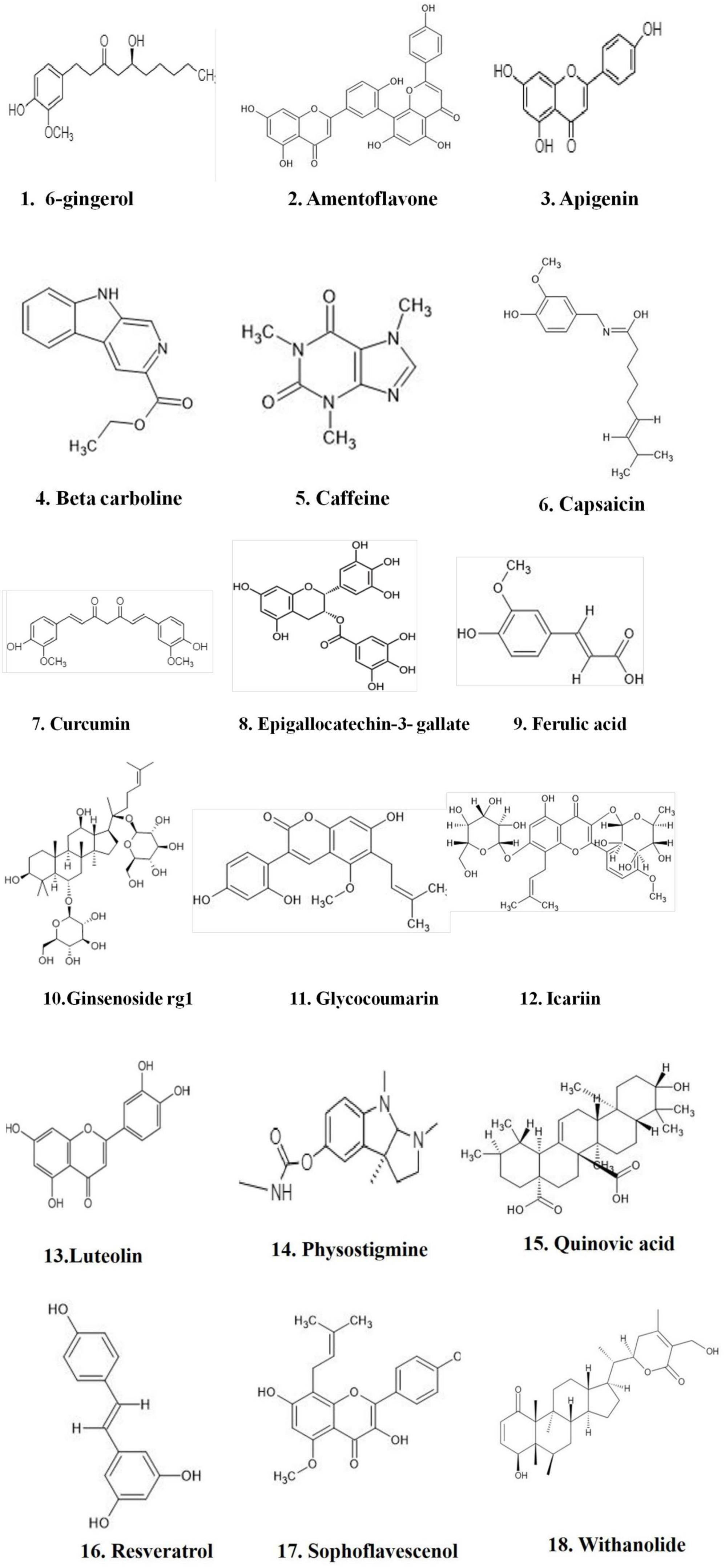
Figure 1. Natural compounds used for PDE inhibitors for the treatment of AD. The figure is original and generated by Bio-render software.
Cognitive impairment has been associated with synthesis, execution, and/or degradation of cyclic nucleotides. In terms of cognition, cyclic nucleotides (cAMP and cGMP) play an important role in gene transcription, neurogenesis, neural circuitry, synaptic plasticity, and neuronal survival (Cardinale and Fusco, 2018) and animal studies have shown the plasticity of synapses and cognition deficit. Animal studies also reports that increasing cGMP-PKG signaling increases long-term memory and required cAMP-PKA (Bollen et al., 2014) signaling. Impairments in these basic processes are underlying cause of cognitive impairment observed in AD patients, implying that cAMP/cGMP signaling plays a critical role in AD patients (Sharma et al., 2020). For example, the AC/cAMP/PKA signaling pathway is downregulated in AD patients. Phosphorylation of tau by cAMP and its main target protein kinase, PKA, is hypothesized to play a role in the genesis of neurofibrillary tangles (Sanders and Rajagopal, 2020). Hence, downregulation of AC/cAMP/PKA signaling pathway, which is also a major activator of CREB, can explain loss of synaptic plasticity and memory impairment in AD (Bollen and Prickaerts, 2012; Argyrousi et al., 2020). Some studies have reported the changes in the expression of cAMP-specific PDE mRNAs in AD brains (Pérez-Torres et al., 2003). In the early stages of AD, increased expression of PDE4A, PDE4B, and PDE7A has also been found (Bollen and Prickaerts, 2012).
Mechanism of action of phosphodiesterase inhibitors
A cyclic nucleotide is a type of nucleotide that has phosphodiesterases (PDEs), which are crucial enzymes in the intracellular signal transduction cascade that occurs when membrane-bound receptors are activated (Ahmad et al., 2015; Preedy, 2020). Second messengers such as cyclic nucleotide (cAMP/cGMP) have been shown to have an important part in the control of cellular functions like signal transduction and synaptic transmission of numerous neurotransmitters in the brain (Gorshkov and Zhang, 2014). Protein kinase A (PKA) and Protein kinase G (PKG) are two target enzymes for cyclic nucleotides such as cAMP/cGMP (Kumar et al., 2015). Cyclic nucleotide mediated transactivation of CREB and brain-derived neurotrophic factor (BDNF) has been reported to have a key impact on cognitive processes (Nair and Vaidya, 2006). CREB is an activity-inducible transcription factor that is activated by numerous kinases binding to the Serine-133 (Ser-133) region. Synaptic plasticity, neuronal growth, and development are enhanced by CREB-mediated transcriptional activity (Nair and Vaidya, 2006). In the conventional model, adenylyl cyclase (AC) or guanylyl cyclase (GC) generate cAMP or cGMP at the plasma membrane in response to external signal, diffuse throughout the cell, where they interact with specific effector proteins to govern a variety of cellular activities (Bolger, 2021).
The changes in the expression of PDE1, PDE4, PDE9, and PDE10 in brain tissues are linked to AD (Kumar et al., 2015; Figure 2). PDE4, a cAMP-specific PDE (García-Osta et al., 2012; Li et al., 2018), PDE9, a cGMP-specific PDE, and the dual cAMP and cGMP specific enzymes PDE1 and PDE10 (Reyes-Irisarri et al., 2007) have been reported to significantly expressed in the AD brain. The PDEs regulate spatial and temporal features of cyclic nucleotide signaling by inactivating cAMP and cGMP through metabolic in activation (Liu et al., 2008). Thus, targeting PDEs to increase synaptic function, or synaptic resilience, could be an effective strategy to treat AD. Furthermore, data from several pre-clinical investigations in AD experimental models have shown that inhibiting PDEs is beneficial (Kumar et al., 2015; Saxena, 2016; Mondal et al., 2017).
PDEs play a crucial role to exerts pharmacological activities, including cell function, by regulating the levels of cAMP and cGMP. The PDE family has been a popular multipotential target to explore a variety of disease pathologies (Nabavi et al., 2019). It has been reported that abnormal cAMP signaling is linked to cognitive impairment in neurodegenerative disorders like Alzheimer’s (Di Benedetto et al., 2021). Disease-related signal transduction dysregulation are caused by abnormal PDE function caused by uncoordinated cAMP responses enhances the Aβ production in brain regions and disrupts the memory formation and (Figure 2; Tibbo et al., 2019). Memory loss tends to occur before nerve cell death in AD, suggesting that neuronal dysfunction as root cause of disease’s pathology (Tian et al., 2014).
Phosphodiesterase inhibitors blocks the phosphodiesterase enzymes PDE-3, PDE-4, and PDE-5 and prevent the degradation of cGMP or cAMP, increases their levels in smooth muscle cells, and induces relaxation and vasodilation in target cells (Padda and Tripp, 2021). Chronic obstructive pulmonary disease (COPD), benign prostatic hyperplasia (BPH), acute decompensated heart failure, psoriasis, psoriatic arthritis (PA), Alzheimer’s disease, atopic dermatitis, and newborn apnea are treated by phosphodiesterase inhibitors (Mannhold et al., 2014). Smooth muscle relaxation, vasodilation, and bronchodilation by PDE inhibitor prevents cAMP and/or cGMP breakdown (Padda and Tripp, 2021).
Specific phosphodiesterase (PDE) inhibitors have been shown to improve memory function in a variety of animal models of AD. PDE inhibitors stimulate gene transcription by activating the CREB (Fiorito et al., 2018; Figure 3). Long-term memory formation and persistent long-term potentiation (LTP) measuring the synaptic plasticity and strength, are driven by CREB-dependent gene expression (Davis et al., 2000). It occurs in the hippocampus through the development of new synaptic connections. Memory loss appears to occur before nerve cell death in AD, suggesting that neuronal dysfunction could be responsible for the pathophysiology of early-stage AD (Abraham, 2019).
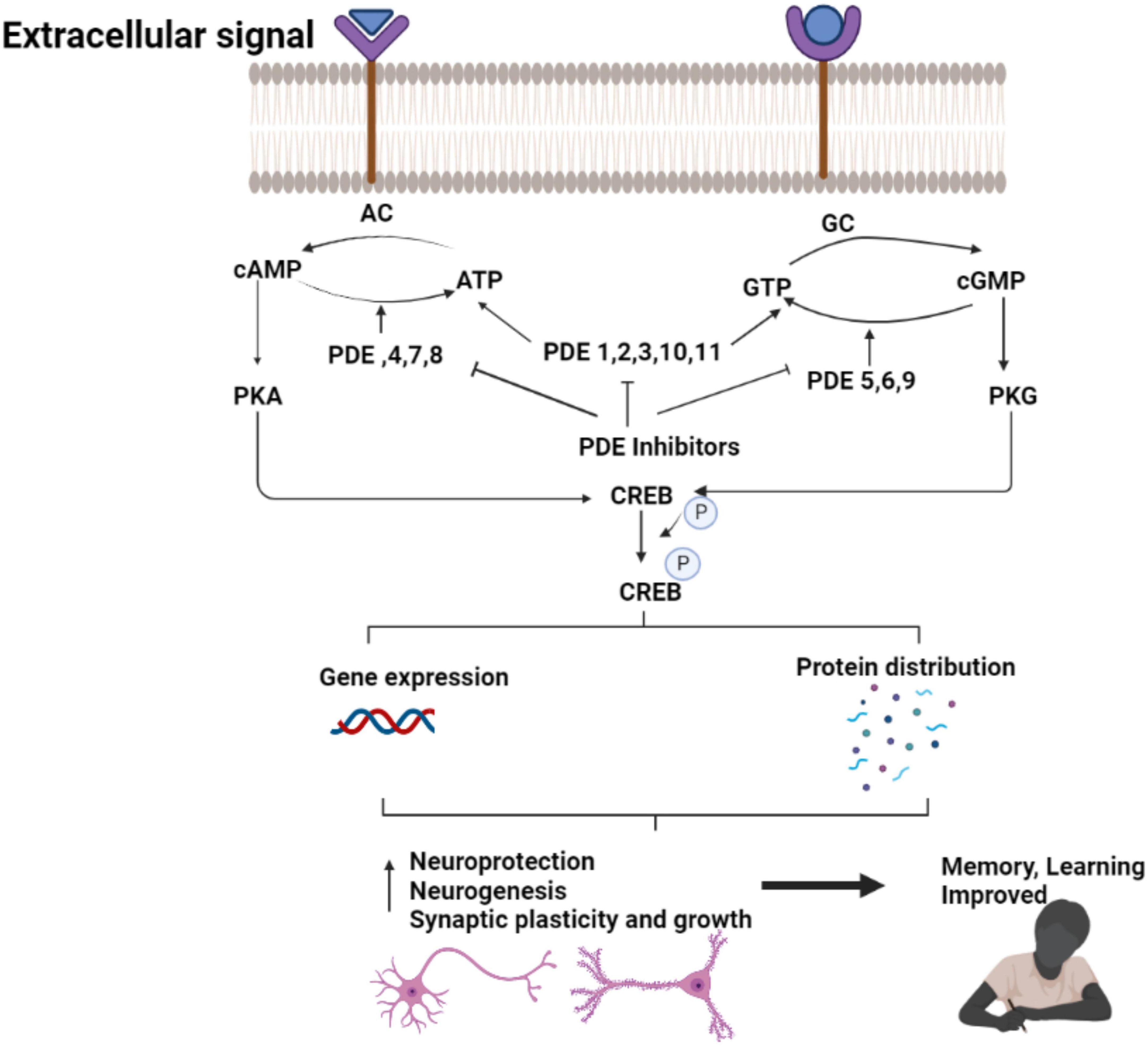
Figure 3. Therapeutic effect of PDE inhibitors in AD. The figure is original and generated by Bio-render software.
The CREB pathway is one of the signaling pathways associated with neuronal plasticity. The brain’s CREB pathway reacts to increased calcium due to neuronal activity. Protein kinase A (PKA), protein kinase C (PKC), the calcium/calmodulin-dependent protein kinases CaMKII and CaMKIV, the extracellular signal-regulated kinase (ERK)-activated kinases mitogen- and stress-activated protein kinase phosphorylates CREB directly (MSK) (García-Osta et al., 2012; Wang et al., 2018a).
The enhancements in memory and learning in FAD mice has been shown after increased CREB signaling, while impaired CREB signaling cause AD symptoms and cognitive deficits. Phosphodiesterases (PDE), a class of enzymes that hydrolyze cAMP (PDE4, PDE7, PDE8), cGMP (PDE5, PDE6, PDE9), or both cAMP and cGMP (PDE1, PDE2, PDE3, PDE10, PDE11) maintain the normal CREB signaling. The strategy to pharmacologically boost CREB signaling could be a potential treatment for cognitive dysfunction in AD by decreasing the expression or activity of PDEs (Bartolotti and Lazarov, 2019).
PDE inhibitors alleviates AD symptoms by restoring synaptic function via stimulation of the CREB signaling pathway (Wang et al., 2018b). Furthermore, other CREB-independent pathways appear to work in tandem to repair cognitive impairment in AD.
Cyclic nucleotide signaling and neuroinflammation
Increased activation of microglia and astrocytes, activated complement proteins, cytokines, and reactive oxygen, nitrogen, and carbonyl species have been shown to linked with AD inflammatory response. Chronic neuroinflammation has been recognized as one of the primary causes of AD (Millington et al., 2014). TNF and IL-1 like inflammatory cytokines increases the production of adhesion molecules on endothelial cells, which bind to leukocyte ligands and allow activated leukocytes to enter the CNS (Strang et al., 2020). Endothelial cells excrete chemokines in response to inflammation, which attract leukocytes to the CNS. Immune cell trafficking across the BBB may start or contribute to a “vicious circle” in the pathological process, resulting in progressive synaptic and neuronal dysfunction and neuronal death in diseases like AD (Peixoto et al., 2015). cGMP is a key mediator of the activity of nitric oxide (NO) and natriuretic peptides in the CNS (Schlossmann and Hofmann, 2005). NO is a gaseous free radical produced by intracellular isoforms of the nitric oxide synthase enzyme and serves as a crucial marker of intra- and extracellular activities. The expression of inflammatory mediators is orchestrated by inflammatory response and regulates the physiological process (Akiyama et al., 2000). Inhibition of IκB degradation due to restriction of IKK activity by cAMP/PKA or increased quantities of resynthesized IB, cAMP interferes with the function of the proinflammatory transcription factor, Nuclear Factor-kappa B (NF-κB). NF-κB activates gene expression for a variety of inflammatory and immune mediators (Peixoto et al., 2017). Stimuli like proinflammatory cytokines, B- and T-cell activators, pathogen-associated molecular patterns (PAMPs), and oxidative stress, cyclic AMP modulates NF-κB. It also affected by less common stimuli like amyloidogenic peptide, thrombin, and high glucose levels (Akiyama et al., 2000; Peixoto et al., 2015; Sanders and Rajagopal, 2020). The NO/cGMP/PKG system play critical role to inhibit the activation of a proapoptotic pathway, allowing brain cells to survive. This neuroprotective process is relevant during ischemia, inflammation, or trauma to the brain. The CREB, a transcription factor involved with regulation of neurotransmitters, growth factors, and other signaling molecules are essential for long-lasting changes in synaptic plasticity, mediating the conversion of short-term memory to long-term memory and neuronal survival in retinal neuroglial progenitor cells, activates the NO/cGMP/PKG antiapoptotic cascade in retinal neuroglial progenitor cells (Akiyama et al., 2000; Peixoto et al., 2015, 2017; Yougbare, 2021).
Current status of phosphodiesterases inhibitors for the treatment of Alzheimer’s disease
PDEs expression in the brain are the focus of current research due to its critical role on regulation of neuroinflammation as discussed above. Based on the genetic variations in patients with neurodevelopmental disorders, animal model phenotypes (Gawel et al., 2020), current research is focused on the pharmacological effects of PDE inhibitors, a medication class that is rapidly evolving and becoming more widely used for the treatment of brain disorders. Pfizer laboratories synthesized UK-92,480 (Sildenafil citrate) compound in 1989, indicating its efficacy as a PDE5 inhibitor. However, multiple studies on healthy volunteers were investigates to study the pharmacokinetics, pharmacodynamics, and tolerance of UK-92,480 revealed side effects including flushing, muscle aches, indigestion, and headaches (Ghofrani et al., 2006).
After a few modifications, sildenafil was approved as the first PDE5 inhibitor for the treatment of erectile dysfunction (ED) in 1998. Vardenafil was approved in 2003, giving patients another option (Huang and Lie, 2013). In comparison to other PDE5 inhibitors, Avanafil was approved by the US Food and Drug Administration in 2012 and the European Medicines Agency in 2013. It was marketed under the brand name Stendra or Spedra and had a short half-life and a rapid onset of action (Bourin, 2018). The NO/cGMP pathway is involved in numerous physiological activities such as the urogenital, pulmonary, and gastrointestinal systems, as well as the central nervous system, Alzheimer’s disease, and the pathophysiology of a wide range of disorders. Despite of typical side effects shown by current drugs like sildenafil, Vardenafil, and Tadalafil (Tables 2, 4), these are most effective oral therapies for treating erectile dysfunction and AD (Ayoub and Melzig, 2006; Prickaerts et al., 2017). However, serious side effects such as non-arteritic hearing loss, dyspepsia, migraines, and priapism have also been recorded (Yafi et al., 2018). As a result, alternate sources of PDE inhibitors are required to eliminate or decrease the side effects of synthetic PDE inhibitors (Anand Ganapathy et al., 2021). Natural or traditional herbs are considered as most desirable alternative sources for the development of new active pharmacophores since they have demonstrated bioactivities with potential utility for health improvement as well as less side effects than synthetic medications (Pratap et al., 2017; Pratap and Shantaram, 2020).
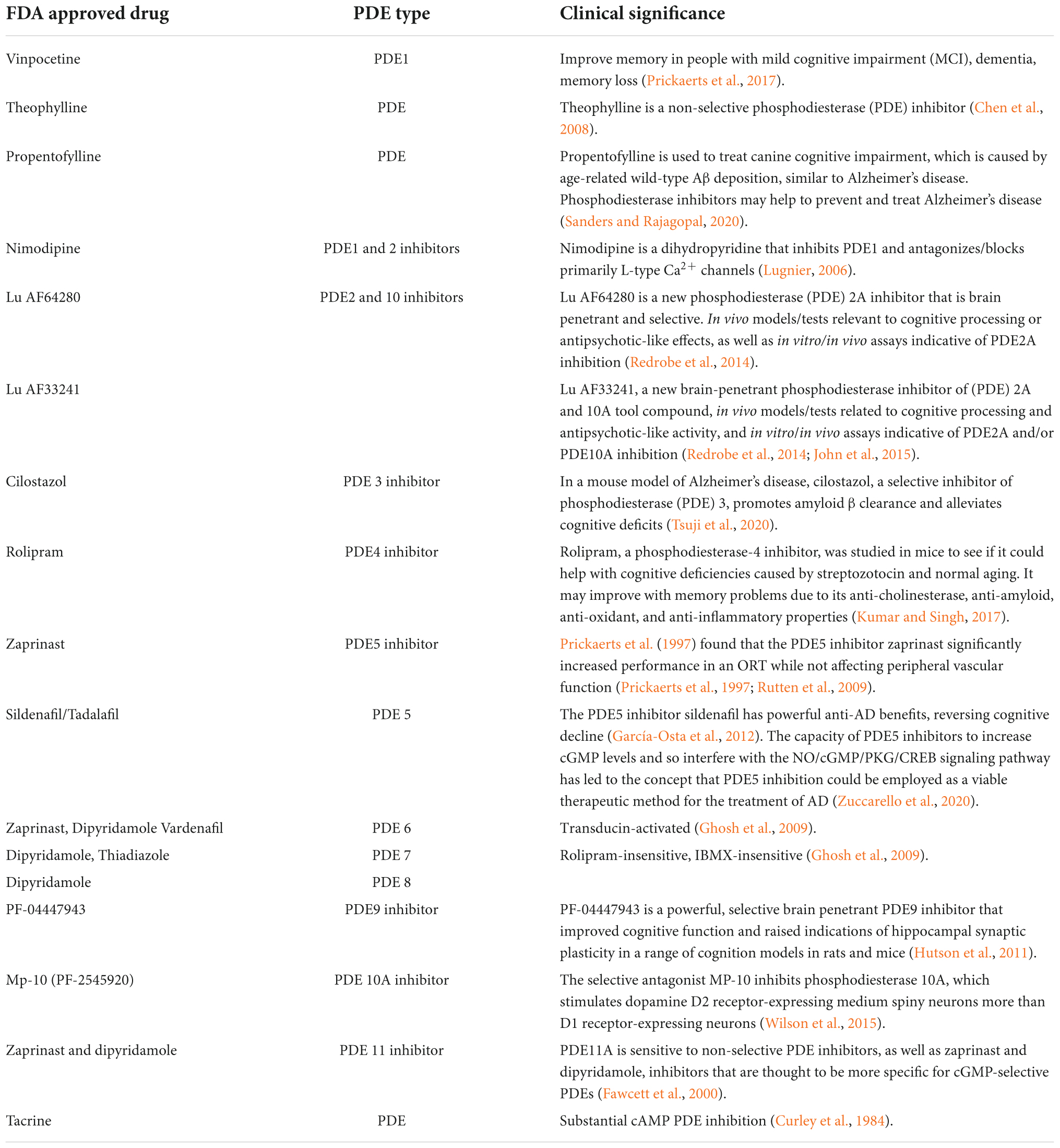
Table 4. List of synthetic drugs for PDE inhibitors and their impact on clinical significance with a focus on Alzheimer’s disease.
Phytochemicals for the inhibition of phosphodiesterases
The growing number of Alzheimer’s patients, along with the aging population, necessitates the development of novel treatment strategy and management (Sarma et al., 2016). The amyloid (Aβ) hypothesis has primarily guided the search for effective AD management, with the primary goal of lowering the number of senile plaques, however, with limited success to date (García-Osta et al., 2012). There is a growing consensus that existing AD therapy options start far too late to substantially decrease the disease progression or delay the emergence of the most severe symptoms (Yiannopoulou and Papageorgiou, 2013). Specific phosphodiesterase (PDE) inhibitors (Table 2) have been demonstrated to improve memory function in a variety of animal models of AD (Xia et al., 2009; Bliss and Cooke, 2011; Heckman et al., 2015; Yan et al., 2016).
Several molecules penetrate the blood-brain barrier and reach concentrations high enough to inhibit PDEs in neurons and glial cells, suggesting that their peripheral activity may have an impact on the CNS (Sallustio and Studer, 2016). Tadalafil may be present in low micromolar quantities in the brain at levels often used in animal models of AD, sufficient to inhibit both PDE5 and PDE11 (Heckman et al., 2015). Increased levels of cAMP and/or cGMP in CNS cells are most likely the major mechanism of action of PDE inhibitors. This result leads to persistent and/or increased activation of signaling pathways that affect neuroprotection/neurodegeneration processes, in addition to replenishing the low levels of cyclic nucleotides present in the elderly brain (García-Osta et al., 2012; Berridge, 2014).
Phytochemicals that inhibit phosphodiesterase
Natural compounds, or small molecules, that operate as dual phosphodiesterase inhibitors would be useful to develop novel anti-neurodegenerative and neuroprotective drugs in the context of the multi-target directed ligand method (Ribaudo et al., 2020, 2021; Table 3). Compounds such as xanthines, alkaloids, flavonoids, coumarins, and polyphenolic acids (Figure 1), are attractive scaffolds for future optimization (Kumar et al., 2015; Mohamed et al., 2019).
Cyclic nucleotide has been shown to play an important role in cognition and motility. Changes in the levels of cyclic nucleotides, such as cAMP and cGMP, have been shown to occur in a variety of neurological disorders, including Alzheimer’s disease (Francis et al., 2011). Phosphodiesterase inhibitors are useful in the treatment of erectile dysfunction and have been proposed as a potential target site for the therapy of a variety of peripheral and neurological illnesses, including asthma, COPD, and CVS (Chronic Villus Sampling) (Maurice et al., 2014; Padda and Tripp, 2021). Rolipram, a selective PDE4 inhibitor, and its synthetic equivalents have been shown be effective for Alzheimer’s patients (Wang et al., 2020).
Various synthetic PDE inhibitors have been used in clinical trials for a variety of illnesses and have been recommended as potential treatment options for neurological disorders (Yang et al., 2019; Table 4). However, the synthetic PDE inhibitors adverse effects have limited their usage in clinical practice. Natural products ingredients are widely accepted and regarded harmless (Pratap and Shantaram, 2020; Pratap et al., 2021). Several plant derived components have been investigated pre-clinically for PDE inhibitory action throughout the last decade (Temkitthawon et al., 2011), and they were proved to be as effective as synthetic drugs in inhibiting PDEs (Figure 4). Inhibition of specific PDEs and accumulation of cGMP may inhibit neuroinflammation and improve synaptic plasticity and memory. The PI3K/Akt pathway is enhanced by an increased cGMP levels through the suppression of PDE activity. By activating PI3K/Akt, the NO/cGMP/PKG/CREB/BDNF pathway plays a crucial part in neurogenesis and synaptic plasticity (Peixoto et al., 2015). We discuss potential plant derived compounds with PDE inhibitory activity, as well as their possible relevance in Alzheimer’s disease.
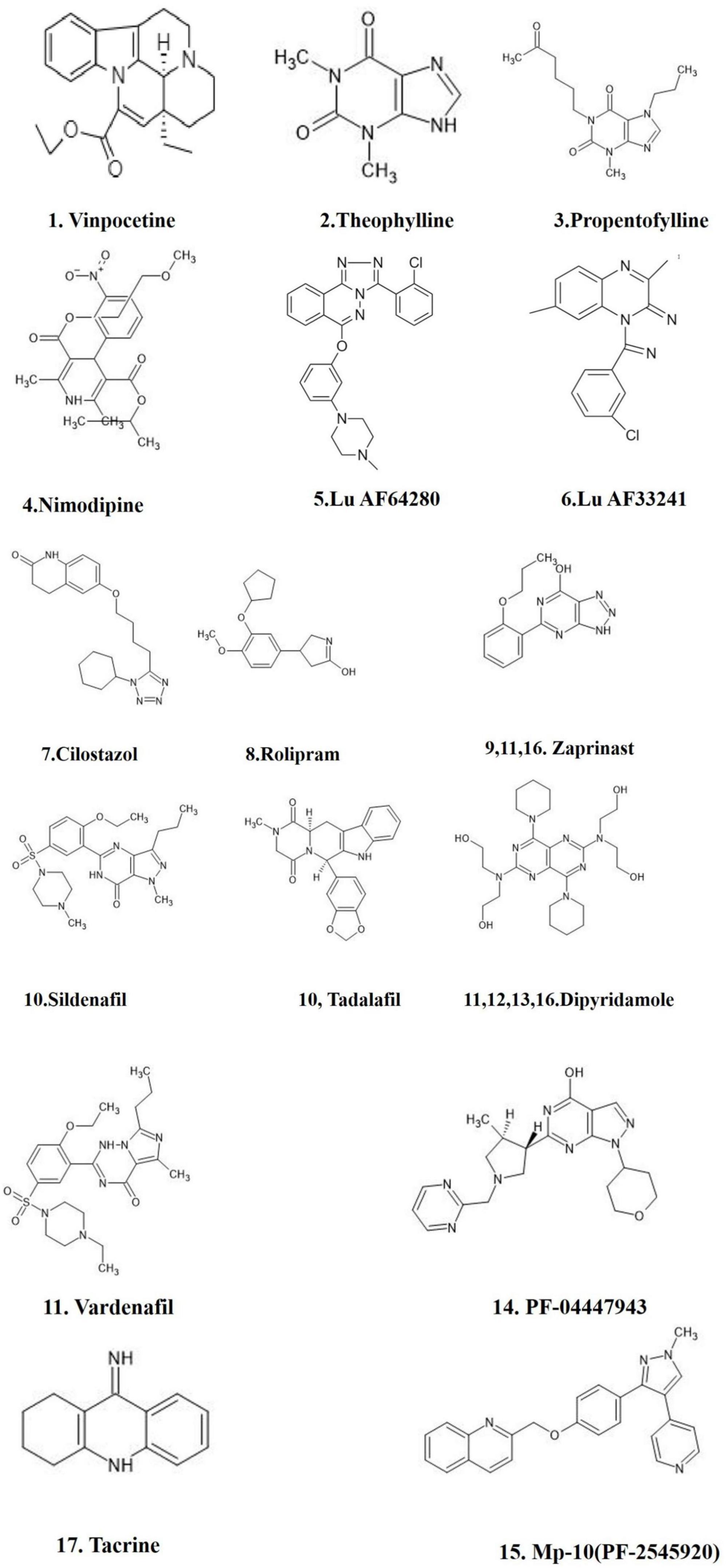
Figure 4. FDA approved PDE inhibitors for the treatment of AD. The figure is original and generated by Bio-render software.
Role of phytochemicals in the pathology of Alzheimer’s disease
Although some therapeutic medications failed at various phases of clinical testing, the increase of remedial approaches for mitigating AD progression has been encouraging (Pratap and Shantaram, 2020). The majority of these therapeutic medications inhibit only one of the numerous pathways involved in AD, which explains their lack of efficacy (Wen et al., 2015). AD is caused by the impairment of several diverse and important biological pathways. Potential AD-treating drugs must acts on multiple disease pathways to improve the efficacy (Guo et al., 2020). Natural products, small compounds, and peptidomimetics have been indicated to inhibit PDEs, prevent Aβ, and tau aggregation (Nalini et al., 2021) and expected to improve the clinical efficacy by enhancing PDE inhibitory activity in the brain, increasing the β- and α-secretase, and kinase activity (Guzior et al., 2015).
Targeting several aspects of the network causing AD could offer better benefit as compared with monotherapies (Zimmermann et al., 2007). Recent studies suggests a novel therapeutic method to treat AD, which targets two independent but synergistic pathways connected to different parts of the disease for the first time (Salloway et al., 2020). Combining HDAC (Histone Deacetylase) inhibitor with the inhibition of phosphodiesterase-5 (PDE5), an enzyme that targets another intracellular pathway has been proposed to be implicated in memory formation and other AD-related symptoms (Cuadrado-Tejedor et al., 2015). The combination of cholinesterase inhibitors with Memantine is well tolerated and safe, and is effective for individuals with moderate to severe Alzheimer’s disease (Epperly et al., 2017). Combinations of diverse disease-modifying medications with different mechanisms, on the other hand, may have potential synergic effects and boost cognition, behavior, and daily living function, except adjuvant therapies of conventional drugs (Fan and Chiu, 2014).
A 3-week chronic therapy with sub-effective levels of the cAMP-specific PDE4-I Roflumilast (0.01 mg/kg) and the cGMP-specific PDE5-I Vardenafil (0.1 mg/kg) improved recognition, spatial, and contextual fear recall, as another combination therapy (Gulisano et al., 2018). Hu et al. used the benzyl piperidine moiety of Donepezil in combination with the pyrazolopyrimidinone structure of a previously known PDE9A inhibitor to study the effects of dual PDE9A/AChE inhibitors (Maramai et al., 2022). Although several amidic or (cyclic)amine chains were investigated as linkers for the two pharmacophores, the greatest results were obtained with 4-member ethereal or carbon tethers, which resulted in compounds with submicromolar inhibitory action against PDE9A and AChE (Huang et al., 2015). Hybridization of the Pyrazolopyrimidinone skeleton with Rivastigmine yielded another series of potential PDE9A inhibitors using a similar strategy (Maramai et al., 2022).
Cuadrado-tejedor et al. (2018) investigated the effect of a simultaneous inhibitor of HDAC6 and PDE5, specifically compound CM-414, on HDACs and other AD-related proteins. The combination of two medications that target these two enzymes (Vorinostat and Tadalafil) has shown in vivo favorable benefits, relieving cognitive deficits in AD animals and reducing hippocampus neuron density (Cuadrado-tejedor et al., 2018; Maramai et al., 2022).
Hu et al. (2019) provided a good example of PDE inhibitors with multifunctional effectiveness against Aβ-induced toxicity and metal-chelating/antioxidant characteristics. They developed hybrid molecules that combine chloroquine’s metal ion chelating framework with critical binding site fragments from the known PDE4 inhibitors Rolipram and Roflumilast, both of which have been studied in preclinical AD models (Maramai et al., 2022).
Summary and future prospects
Extensive experimental and clinical studies suggest that pharmacological targets involved in inhibition of PDEs could be potential therapeutic targets for cognitive impairments and dementia. PDE inhibitors modulate the cyclic purine nucleotide levels and potentially prevent or cure AD, MCI, and dementia. Natural products have been shown to improve dementia, cognitive decline, and AD, symptoms suggesting that PDE inhibitors could help prevent the disease. Dysregulation of several cellular processes, including the immune system, transduction and transcription signaling pathways, and the inflammatory response, may result from abnormality in PDEs’ physiological activity, which play important roles in neurodegenerative disorder. Various PDE inhibitors, including synthetic, natural, and multifactorial modifying and combination therapy have been discussed in this review article. We described that several neuro-psycho-pharmacological pathways are implicated in the therapeutic impact of PDEI in AD. Protective effects of such medications, which are directly related to PDE inhibition and other pharmacological effects of the pharmaceuticals, such as neurotransmitter receptor activity or certain downstream effects, may also be striking (vasodilation) have been discussed. PDEs are emerging as viable targets for the development of new pharmacotherapeutic drugs to treat AD. A well-designed clinical trials are needed to assess the efficacy and safety of PDE inhibitors in Alzheimer’s patients. Researchers can gain insights on the growth of multifunctional drugs for AD patients. With such types of drugs, AD patients would have balanced Aβ and tau balance in the brain, normal PDE and kinase activity levels. A wide range of natural compounds has been used for different diseases like cancer, AD, neuroprotective study, anti-diabetic etc. but very few plant-based compounds are used for PDEI study. Some flavonoids and Phenolic compounds have also been proven to inhibit PDEs, as evidenced by red grape extract’s in vitro suppression of cGMP-specific PDEs. Synthetic drugs show several side effects including flush, nausea, dizziness, dry mouth, transitory hypo- and hypertension, headache, and heartburn as compared to natural compounds. These natural compounds could be a potential candidate for developing as an alternative strategy for PDEs inhibition in AD.
Author contributions
HF conceived the idea of the review. JS, SZ, LW, YL, and PG collected the literature, analyzed, outlined, and drafted the manuscript. HF and GK revised and finalized the manuscript. All authors participated in the study and read and approved the final manuscript.
Conflict of interest
The authors declare that the research was conducted in the absence of any commercial or financial relationships that could be construed as a potential conflict of interest.
Publisher’s note
All claims expressed in this article are solely those of the authors and do not necessarily represent those of their affiliated organizations, or those of the publisher, the editors and the reviewers. Any product that may be evaluated in this article, or claim that may be made by its manufacturer, is not guaranteed or endorsed by the publisher.
Abbreviations
AD, Alzheimer’s Diseases; cAMP, Cyclic Adenosine Monophosphate; cGMP, cyclic guanosine monophosphate; COPD, Chronic Obstructive Pulmonary Disease; CREB, cyclic AMP response element-binding protein; CVS, Chronic Villus Sampling; HDAC, Histone Deacetylase; MCI, Mild Cognitive Impairment; PDE-I, phosphodiesterase inhibitors; PDEs, Phosphodiesterases; PKA, Protein kinase A; PKG, Protein kinase G.
References
Abraham, W. C. (2019). Is plasticity of synapses the mechanism of long-term memory storage ? NPJ Sci. Learn. 9, 1–10. doi: 10.1038/s41539-019-0048-y
Ahlström, M. E. B. (2001). Cyclic nucleotide inactivation in osteoblasts and osteosarcoma cell lines. Academic dissertation Thesis. 1–76. Available online at: https://core.ac.uk/download/pdf/14917723 (accessed July 4, 2022).
Ahmad, F., Murata, T., Shimizu, K., Degerman, E., Maurice, D., and Manganiello, V. (2015). Cyclic nucleotide phosphodiesterases: Important signaling modulators and therapeutic targets. Oral Dis. 21, e25–e50. doi: 10.1111/odi.12275
Ahmed, W. S., Geethakumari, A. M., and Biswas, K. H. (2021). Phosphodiesterase 5 (PDE5): Structure-function regulation and therapeutic applications of inhibitors. Biomed. Pharmacother. 134:111128. doi: 10.1016/j.biopha.2020.111128
Akiyama, H., Barger, S., Barnum, S., Bradt, B., Bauer, J., Cole, G., et al. (2000). Inflammation and Alzheimer’s disease. Neurobiol. Aging 21, 383–421.
Amin, S. A., Bhargava, S., Adhikari, N., Gayen, S., and Jha, T. (2018). Exploring pyrazolo[3,4-d]pyrimidine phosphodiesterase 1 (PDE1) inhibitors: A predictive approach combining comparative validated multiple molecular modelling techniques. J. Biomol. Struct. Dyn. 36, 590–608. doi: 10.1080/07391102.2017.1288659
Anand Ganapathy, A., Hari Priya, V. M., and Kumaran, A. (2021). Medicinal plants as a potential source of Phosphodiesterase-5 inhibitors: A review. J. Ethnopharmacol. 267:113536. doi: 10.1016/j.jep.2020.113536
Argyrousi, E. K., Heckman, P. R. A., and Prickaerts, J. (2020). Role of cyclic nucleotides and their downstream signaling cascades in memory function: Being at the right time at the right spot. Neurosci. Biobehav. Rev. 113, 12–38. doi: 10.1016/j.neubiorev.2020.02.004
Ayoub, S., and Melzig, M. F. (2006). Degradation of Amyloid β-peptide (Aβ) by NEP-induction is increased by selected natural products. Planta Med. 72:217. doi: 10.1016/j.urols.2016.04.003
Azam, F., Amer, A. M., Rabulifa, A., and Elzwawi, M. M. (2014). Ginger components as new leads for the design and development of novel multi-targeted anti-Alzheimer’s drugs: A computational investigation. Drug Design. Dev. Ther. 8, 2045–2059. doi: 10.2147/DDDT.S67778
Barnes, P. J. (1995). Cyclic nucleotides and phosphodiesterases and airway function. Eur. Respir. J. 8, 457–462. doi: 10.1183/09031936.95.08030457
Bartolotti, N., and Lazarov, O. (2019). CREB signals as PBMC-based biomarkers of cognitive dysfunction: A novel perspective of the brain-immune axis. Brain Behav. Immun. 78, 9–20. doi: 10.1016/j.bbi.2019.01.004
Bender, A. T., and Beavo, J. A. (2006). Cyclic nucleotide phosphodiesterases: Molecular regulation to clinical use. Pharmacol. Rev. 58, 488–520.
Berridge, M. J. (2014). Module 12: Signalling defects and disease. Cell Signal. Biol. 6:csb0001012. doi: 10.1042/csb0001012
Bliss, T. V. P., and Cooke, S. F. (2011). Long-term potentiation and long-term depression: A clinical perspective. Clinics 66, 3–17. doi: 10.1590/S1807-59322011001300002
Bolger, G. B. (2021). The PDE-Opathies: Diverse phenotypes produced by a functionally related multigene family. Trends Genet. 37, 669–681. doi: 10.1016/j.tig.2021.03.002
Bollen, E., and Prickaerts, J. (2012). Phosphodiesterases in neurodegenerative disorders. IUBMB Life 64, 965–970. doi: 10.1002/iub.1104
Bollen, E., Puzzo, D., Rutten, K., Privitera, L., De Vry, J., Vanmierlo, T., et al. (2014). Improved long-term memory via enhancing cGMP-PKG signaling requires cAMP-PKA signaling. Neuropsychopharmacology 39, 2497–2505. doi: 10.1038/npp.2014.106
Bourin, M. (2018). Clinical pharmacology of phosphodiesterase 5 inhibitors in erectile dysfunction. SOA Arch. Pharm. Pharmacol. 1, 1–6.
Cardinale, A., and Fusco, F. R. (2018). Inhibition of phosphodiesterases as a strategy to achieve neuroprotection in Huntington’s disease. CNS Neurosci. Ther. 24, 319–328. doi: 10.1111/cns.12834
Chen, Y.-H., Yao, W.-Z., Ding, Y.-L., et al. (2008). Effect of theophylline on endogenous hydrogen sulfide production in patients with COPD. Pulm. Pharmacol. Ther. 21, 40–46. doi: 10.1016/j.pupt.2006.11.002
Cote, R. H. (2006). “Photoreceptor phosphodiesterase (PDE6): a G-protein-activated PDE regulating visual excitation in rod and cone photoreceptor cells,” in Cyclic nucleotide phosphodiesterases in health and disease, eds J. A. Beavo, S. H. Francis, and M. D. Houslay (Boca Raton, FL: CRC Press).
Cuadrado-tejedor, M., Garcia-barroso, C., Sánchez-arias, J. A., and Rabal, O. (2018). A first-in-class small-molecule that acts as a dual inhibitor of HDAC and PDE5 and that rescues hippocampal synaptic impairment in Alzheimer’s Disease mice. Neuropsychopharmacology 42, 524–539. doi: 10.1038/npp.2016.163
Cuadrado-Tejedor, M., Garcia-Barroso, C., Sanzhez-Arias, J., Mederos, S., Rabal, O., Ugarte, A., et al. (2015). Concomitant histone deacetylase and phosphodiesterase 5 inhibition synergistically prevents the disruption in synaptic plasticity and it reverses cognitive impairment in a mouse model of Alzheimer’s disease. Clin. Epigenet. 7:108. doi: 10.1186/s13148-015-0142-9
Curley, W. H., Standaert, F. G., and Dretchen, K. L. (1984). Physostigmine inhibition of 3’, 5’-cyclic AMP phosphodiesterase from cat sciatic nerve. J. Pharmacol. Exp. Ther. 228, 656–661.
Davis, S., Vanhoutte, P., Pages, C., Caboche, J., and Laroche, S. (2000). The MAPK/ERK cascade targets both Elk-1 and cAMP response element-binding protein to control long-term potentiation-dependent gene expression in the dentate gyrus in vivo. J. Neurosci. 20, 4563–4572. doi: 10.1523/JNEUROSCI.20-12-04563.2000
Degerman, E., Belfrage, P., and Manganiello, V. C. (1997). Regulation of cGMP-inhibited Phosphodiesterase (PDE3). J. Biol. Chem. 272, 6823–6827.
Dell’Agli, M., Galli, G. V., and Bosisio, E. (2006). Inhibition of cGMP-phosphodiesterase-5 by biflavones of Ginkgo biloba. Planta Med. 72, 468–470. doi: 10.1055/s-2005-916236
Di Benedetto, G., Iannucci, L. F., Surdo, N. C., Zanin, S., Conca, F., Grisan, F., et al. (2021). Compartmentalized signaling in aging and neurodegeneration. Cells 10, 1–31. doi: 10.3390/cells10020464
Ding, M.-L., Ma, H., Man, Y.-G., and Hong-yan, L. V. (2017). Protective effects of green tea polyphenol, epigallocatechin-3-gallate against sevoflurane-induced neuronal apoptosis involves regulation of CREB – BDNF- Trk-B and PI3K / Akt / mTOR signalling pathways in neonatal mice. Can. J. Physiol. Pharmacol. 95, 1396–1405. doi: 10.1139/cjpp-2016-0333
Dubey, A., Kumar, N., Mishra, A., Singh, Y., and Tiwari, M. (2020). Review on Vinpocetine. Int. J. Pharm. Life Sci. 11, 6590–6597.
Epperly, T., Dunay, M. A., and Boice, J. L. (2017). Alzheimer disease: Pharmacologic and nonpharmacologic therapies for cognitive and functional symptoms. Am. Fam. Physician 95, 771–778.
Epstein, P. M. (2017). Different phosphodiesterases (PDEs) regulate distinct phosphoproteomes during cAMP signaling. Proc. Natl. Acad. Sci. U.S.A. 114, 7741–7743. doi: 10.1073/pnas.1709073114
Fan, L. Y., and Chiu, M. J. (2014). Combotherapy and current concepts as well as future strategies for the treatment of Alzheimer’s disease. Neuropsychiatr. Dis. Treat. 10, 439–451. doi: 10.2147/NDT.S45143
Fawcett, L., Baxendale, R., Stacey, P., McGrouther, C., Harrow, I., Soderling, S., et al. (2000). Molecular cloning and characterization of a distinct human phosphodiesterase gene family: PDE11A. Proc. Natl. Acad. Sci. U.S.A. 97, 3702–3707. doi: 10.1073/pnas.97.7.3702
Fiorito, J., Deng, S.-X., and Landry, D. W. (2018). “Targeting the NO / cGMP / CREB phosphorylation signaling pathway in Alzheimer’s Disease,” in Neurochem basis of brain function and dysfunction, eds T. Heinbockel and A. B. Csoka (Rijeka: Intechopen), 1–24.
Fisher, D. A., Smith, J. F., Pillar, J. S., Denis, S. H. S., and Cheng, J. B. (1998). Isolation and characterization of PDE9A, a novel human cGMP-specific phosphodiesterase. J. Biol. Chem. 273, 15559–15564. doi: 10.1074/jbc.273.25.15559
Francis, S. H., Blount, M. A., and Corbin, J. D. (2011). Mammalian cyclic nucleotide phosphodiesterases: Molecular mechanisms and physiological functions. Physiol. Rev. 91, 651–690. doi: 10.1152/physrev.00030.2010
Francis, S. H., Busch, J. L., and Corbin, J. D. (2010). cGMP-dependent protein kinases and cGMP phosphodiesterases in nitric oxide and cGMP action. Pharmacol. Rev. 62, 525–563. doi: 10.1124/pr.110.002907
Furlan, V., and Bren, U. (2021). Insight into inhibitory mechanism of pde4d by dietary polyphenols using molecular dynamics simulations and free energy calculations. Biomolecules 11, 1–22. doi: 10.3390/biom11030479
García-Osta, A., Cuadrado-Tejedor, M., García-Barroso, C., Oyarzábal, J., and Franco, R. (2012). Phosphodiesterases as therapeutic targets for Alzheimer’s disease. ACS Chem. Neurosci. 3, 832–844. doi: 10.1021/cn3000907
Gawel, K., Langlois, M., Martins, T., van der Ent, W., Tiraboschi, E., Jacmin, M., et al. (2020). Seizing the moment: Zebrafish epilepsy models. Neurosci. Biobehav. Rev. 116, 1–20. doi: 10.1016/j.neubiorev.2020.06.010
Geerts, H., and Grossberg, G. T. (2006). Pharmacology of acetylcholinesterase inhibitors and N-methyl-D-aspartate receptors for combination therapy in the treatment of Alzheimer’s disease. J. Clin. Pharmacol. 46, 8S–16S. doi: 10.1177/0091270006288734
Ghofrani, H. A., Osterloh, I. H., and Grimminger, F. (2006). Sildenafil: From angina to erectile dysfunction to pulmonary hypertension and beyond. Nat. Rev. Drug Discov. 5, 689–702. doi: 10.1038/nrd2030
Ghosh, R., Sawant, O., Ganpathy, P., Pitre, S., and Kadam, V. J. (2009). Phosphodiesterase inhibitors: Their role and implications. Int. J. PharmTech Res. 1, 1148–1160.
González, J. F., Alcántara, A. R., Doadrio, A. L., and Sánchez-Montero, J. M. (2019). Developments with multi-target drugs for Alzheimer’s disease: An overview of the current discovery approaches. Expert Opin Drug Discov. 14, 879–891.
Gorshkov, K., and Zhang, J. (2014). Visualization of cyclic nucleotide dynamics in neurons. Front. Cell. Neurosci. 8:395. doi: 10.3389/fncel.2014.00395
Gu, M., Zhou, X., Zhu, L., Gao, Y., Gao, L., Bai, C., et al. (2022). Myostatin mutation promotes glycolysis by increasing phosphorylation of phosphofructokinase via activation of PDE5A-cGMP-PKG in cattle heart. Front. Cell Dev. Biol. 9:774185. doi: 10.3389/fcell.2021.774185
Gulisano, W., Tropea, M. R., Arancio, O., Palmeri, A., and Puzzo, D. (2018). Sub-efficacious doses of phosphodiesterase 4 and 5 inhibitors improve memory in a mouse model of Alzheimer’s disease. Neuropharmacology 138, 151–159. doi: 10.1016/j.neuropharm.2018.06.002
Guo, T., Zhang, D., Zeng, Y., Huang, T. Y., Xu, H., and Zhao, Y. (2020). Molecular and cellular mechanisms underlying the pathogenesis of Alzheimer’s disease. Mol. Neurodegener. 15, 1–37.
Guzior, N., Wi, A., Panek, D., and Malawska, B. (2015). Recent development of multifunctional agents as potential drug candidates for the treatment of Alzheimer’s Disease. Curr. Med. Chem. 22, 373–404.
Heckman, P. R. A., Blokland, A., and Prickaerts, J. (2017). From age-related cognitive decline to Alzheimer’s disease: A translational overview of the potential role for phosphodiesterases. Adv. Neurobiol. 17, 135–168. doi: 10.1007/978-3-319-58811-7_6
Heckman, P. R. A., Blokland, A., Ramaekers, J., and Prickaerts, J. (2015). PDE and cognitive processing: Beyond the memory domain. Neurobiol. Learn. Mem. 119, 108–122. doi: 10.1016/j.nlm.2014.10.011
Heikaus, C. C., Pandit, J., and Klevit, R. E. (2009). Cyclic Nucleotide Binding GAF Domains from Phosphodiesterases – Structural and Mechanistic Insights. Structure 17, 1551–1557. doi: 10.1016/j.str.2009.07.019.Cyclic
Hesse, R., Lausser, L., Gummert, P., Schmid, F., Wahler, A., and Schnack, C. (2017). Reduced cGMP levels in CSF of AD patients correlate with severity of dementia and current depression. Alzheimers Res. Ther. 9:17. doi: 10.1186/s13195-017-0245-y
Hu, J., Pan, T., An, B., Li, Z., Li, X., and Huang, L. (2019). Synthesis and evaluation of clioquinol-rolipram/roflumilast hybrids as multitargetdirected ligands for the treatment of Alzheimer’s disease. Eur. J. Med. Chem. 163, 512–526. doi: 10.1016/j.ejmech.2018.12.013
Huang, H., Hong, Q., Tan, H.-L., Xiao, C.-R., and Gao, Y. (2016). Ferulic acid prevents LPS-induced up-regulation of PDE4B and stimulates the cAMP / CREB signaling pathway in PC12 cells. Acta Pharmacol Sin. 37, 1543–1554. doi: 10.1038/aps.2016.88
Huang, M., Shao, Y., Hou, J., Cui, W., Liang, B., Huang, Y., et al. (2015). Structural Asymmetry of Phosphodiesterase-9A and a Unique Pocket for Selective Binding of a Potent Enantiomeric Inhibitor Structural Asymmetry of Phosphodiesterase-9A and a Unique Pocket for Selective Binding of a Potent Enantiomeric Inhibitor. Mol. Pharmacol. 88, 836–842. doi: 10.1124/mol.115.099747
Huang, S. A., and Lie, J. D. (2013). Phosphodiesterase-5 (PDE5) inhibitors in the management of erectile dysfunction. Pharm. Ther. 38, 407–4019.
Hutson, P. H., Finger, E. N., Magliaro, B. C., Smith, S. M., Converso, A., Sanderson, P. E., et al. (2011). The selective phosphodiesterase 9 (PDE9) inhibitor PF-04447943 (6-[(3S,4S)-4-methyl-1-(pyrimidin-2-ylmethyl)pyrrolidin-3-yl] -1-(tetrahydro-2H-pyran-4-yl)-1,5-dihydro-4H-pyrazolo[3,4-d]pyrimidin-4-one) enhances synaptic plasticity and cognitive function i. Neuropharmacology 61, 665–676. doi: 10.1016/j.neuropharm.2011.05.009
John, P., Redrobe, L. K. R., Christoffersen, C. T., Bundgaard, C., and Jørgensen, M. (2015). Characterisation of Lu AF33241: A novel, brain-penetrant, dual inhibitor of phosphodiesterase (PDE) 2A and PDE10A. Eur. J. Pharmacol. 15, 79–85. doi: 10.1016/j.physio.2015.03.175
Kim, Y., and Clifton, P. (2018). Curcumin, cardiometabolic health and dementia. Int. J. Environ. Res. Public Health 15, 1–34. doi: 10.3390/ijerph15102093
Kumar, A., and Singh, N. (2017). Inhibitor of Phosphodiestearse-4 improves memory deficits, oxidative stress, neuroinflammation and neuropathological alterations in mouse models of dementia of Alzheimer’s Type. Biomed. Pharmacother. 88, 698–707. doi: 10.1016/j.biopha.2017.01.059
Kumar, A., Sharma, V., Singh, V. P., Kaundal, M., Gupta, M. K., Bariwal, J., et al. (2015). Herbs to curb cyclic nucleotide phosphodiesterase and their potential role in Alzheimer’s disease. Mech. Ageing Dev. 149, 75–87. doi: 10.1016/j.mad.2015.05.009
Lai, M., Zhang, H.-J., Wang, F., Shao, Y.-L., Yang, M.-W., Hong, F.-F., et al. (2018). Anti-aging effects of ginseng and ginsenosides on the Nervous SystemÓ. Int. J. Pharmacol. 14, 1188–1197. doi: 10.21037/atm-22-501
Li, H., Zuo, J., and Tang, W. (2018). Phosphodiesterase-4 inhibitors for the treatment of inflammatory diseases. Front. Pharmacol. 9:1048. doi: 10.3389/fphar.2018.01048
Liu, S., Mansour, M. N., Dillman, K. S., Perez, J. R., Danley, D. E., Aeed, P. A., et al. (2008). Structural basis for the catalytic mechanism of human phosphodiesterase 9. Proc. Natl. Acad. Sci. U.S.A. 105, 13309–13314. doi: 10.1073/pnas.0708850105
Lorigo, M., Oliveira, N., and Cairrao, E. (2022). PDE-mediated cyclic nucleotide compartmentation in vascular smooth muscle cells: From basic to a clinical perspective. J. Cardiovasc. Dev. Dis. 9:4. doi: 10.3390/jcdd9010004
Loughney, K., Martins, T. J., Harris, E. A. S., Sadhu, K., Hicks, J. B., Sonnenburg, W. K., et al. (1996). Isolation and characterization of cDNAs corresponding to two human calcium, calmodulin-regulated, 3’,5’-cyclic nucleotide phosphodiesterase. J. Biol. Chem. 271, 796–806. doi: 10.1074/jbc.271.2.796
Lugnier, C. (2006). Cyclic nucleotide phosphodiesterase (PDE) superfamily : A new target for the development of specific therapeutic agents. Pharmacol. Ther. 109, 366–398. doi: 10.1016/j.pharmthera.2005.07.003
Mannhold, R., Kubinyi, H., and Folkers, G. (2014). Phosphodiesterases and Their Inhibitors, Vol. 61. Hoboken, NJ: John Wiley & Sons.
Mao, F., Wang, H., Ni, W., Zheng, X., Wang, M., Bao, K., et al. (2018). Design, Synthesis, and Biological Evaluation of Orally Available First-Generation Dual-Target Selective Inhibitors of Acetylcholinesterase (AChE) and Phosphodiesterase 5 (PDE5) for the Treatment of Alzheimer’s Disease. ACS Chem. Neurosci. 9, 328–345. doi: 10.1021/acschemneuro.7b00345
Maramai, S., Benchekroun, M., and Gabr, M. T. (2022). Multitarget therapeutic strategies for Alzheimer’s disease : Review on emerging target combinations. BioMed Res. Int. 2020:5120230.
Maurice, D. H., Ke, H., Ahmad, F., Wang, Y., Chung, J., and Manganiello, V. C. (2014). Advances in targeting cyclic nucleotide phosphodiesterases. Nat. Rev. Drug Discov. 13, 290–314. doi: 10.1038/nrd4228
Millington, C., Sonego, S., Karunaweera, N., Rangel, A., Aldrich-Wright, J. R., Campbell, I., et al. (2014). Chronic neuroinflammation in Alzheimer’s disease: New perspectives on animal models and promising candidate drugs. BioMed Res. Int. 2014:309129. doi: 10.1155/2014/309129
Mohamed, Z. A., Eliaser, E. M., Mazzon, E., Rollin, P., Cheng Lian Ee, G., and Abdull Razis, A. F. (2019). Neuroprotective potential of secondary metabolites. Molecules 24, 1–21.
Mondal, S., Hsiao, K., and Goueli, S. A. (2017). Utility of adenosine monophosphate detection system for monitoring the activities of diverse enzyme reactions. ASSAY Drug Dev. Technol. 15, 330–341. doi: 10.1089/adt.2017.815
Mostafa, M., Nahar, N., and Mosihuzzaman, M. (2006). Natural product research : Formerly natural product letters phosphodiesterase-I inhibitor quinovic acid glycosides from Bridelia ndellensis. Nat. Prod. Lett. 20, 37–41. doi: 10.1080/14786410600661658
Munoz-Torrero, D. (2008). Acetylcholinesterase inhibitors as disease-modifying therapies for Alzheimer’s disease. Curr. Med. Chem. 15, 2433–2455.
Nabavi, S. M., Talarek, S., Listos, J., Nabavi, S. F., Devi, K. P., Roberto de Oliveira, M., et al. (2019). Phosphodiesterase inhibitors say NO to Alzheimer’s disease. Food Chem. Toxicol. 134:110822. doi: 10.1016/j.fct.2019.110822
Nair, A., and Vaidya, V. A. (2006). Cyclic AMP response element binding protein and brain-derived neurotrophic factor: Molecules that modulate our mood? J. Biosci. 31, 423–434. doi: 10.1007/BF02704114
Nalini, V., Gorantla, N. V., Sunny, L. P., Rajasekhar, K., Nagaraju, P. G., Cg, P. P., Govindaraju, T., Chinnathambi, S., et al. (2021). Amyloid- β -derived peptidomimetics inhibits tau aggregation. ACS Omega 6, 11131–11138. doi: 10.1021/acsomega.9b03497
Omori, K., and Kotera, J. (2007). Overview of PDEs and their regulation. Circ. Res. 100, 309–327. doi: 10.1161/01.RES.0000256354.95791.f1
Padda, I. S., and Tripp, P. J. (2021). Phosphodiesterase Inhibitors. (Treasure Island, FL: StatPearls), 1–6.
Parihar, M. S., and Hemnani, T. (2004). Alzheimer’s disease pathogenesis and therapeutic interventions. J. Clin. Neurosci. 11, 456–467.
Pavlaki, N., and Nikolaev, V. O. (2018). Imaging of PDE2- and PDE3-mediated cGMP-to-cAMP cross-talk in cardiomyocytes. J. Cardiovasc. Dev. Dis. 5, 1–18. doi: 10.3390/jcdd5010004
Pdes, P., Azevedo, M. F., Faucz, F. R., Bimpaki, E., Horvath, A., Levy, I., et al. (2014). Clinical and Molecular Genetics of the Phosphodiesterases (PDEs). Endocr. Rev. 35, 195–233. doi: 10.1210/er.2013-1053
Peiró, A. M., Tang, C.-M., Murray, F., Zhang, L., Brown, L. M., Chou, D., et al. (2011). Genetic variation in phosphodiesterase (PDE) 7B in chronic lymphocytic leukemia : Overview of genetic variants of cyclic nucleotide PDEs in human disease. J. Hum. Genet. 59, 676–681. doi: 10.1038/jhg.2011.80
Peixoto, C. A., Nunes, A. K. S., and Garcia-Osta, A. (2015). Phosphodiesterase-5 inhibitors: action on the signaling pathways of neuroinflammation, neurodegeneration, and cognition. Mediat. Inflammation 2015:940207. doi: 10.1155/2015/940207
Peixoto, C. A., Nunes, A. K. S., and Rapôso, C. (2017). The role of NO/cGMP signaling on neuroinflammation: A new therapeutic opportunity. Mech. Neuroinflammation 167–208.
Pérez-Torres, S., Cortés, R., Tolnay, M., Probst, A., Palacios, J. M., and Mengod, G. (2003). Alterations on phosphodiesterase type 7 and 8 isozyme mRNA expression in Alzheimer’s disease brains examined by in situ hybridization. Exp. Neurol. 182, 322–334. doi: 10.1016/S0014-4886(03)00042-6
Pratap, G. K., Ananda, D., Joshi, C. G., and Shantaram, M. (2021). Ameliorative activity of medicinal plant fraction for neuroprotection against acrylamide-induced neurotoxicity in Drosophila melanogaster—a comparative study. J. Basic Appl. Zool. 82, 1–10.
Pratap, G. K., and Shantaram, M. (2020). Phytomedicine and Alzheimer’s disease. (Boca Raton, FL: CRC Press), 15–40.
Pratap, G. K., Ashwini, S., and Manjula, S. (2017). Alzheimer’s disease: A challenge in managing with certain medicinal plants-A review. Int. J. Pharm. Sci. Res. 12, 4960–4972.
Preedy, M. E. J. (2020). Cardiac cyclic nucleotide phosphodiesterases: Roles and therapeutic potential in heart failure. Cardiovasc. Drugs Ther. 34, 401–417.
Prickaerts, J., Heckman, P. R. A., and Blokland, A. (2017). Investigational phosphodiesterase inhibitors in phase I and phase II clinical trials for Alzheimer’s disease. Expert Opin. Invest. Drugs 26, 1033–1048. doi: 10.1080/13543784.2017.1364360
Prickaerts, J., Steinbusch, H. W. M., Smits, J. F. M., and Vente, J. D. (1997). Possible role of nitric oxide-cyclic GMP pathway in object recognition memory : Effects of 7-nitroindazole and zaprinast. Eur. J. Pharmacol. 337, 125–136. doi: 10.1016/s0014-2999(97)01301-0
Puzzo, D., Sapienza, S., Arancio, O., and Palmeri, A. (2008). Role of phosphodiesterase 5 in synaptic plasticity and memory. Neuropsychiatr. Dis. Treat. 4, 371–387. doi: 10.2147/ndt.s2447
Rathi, A. K. V., and Sundar, D. (2022). Insights into the potential of withanolides as Phosphodiesterase-4 (PDE4D) inhibitors. J. Biomol. Struct. Dyn. [Epub ahead of print]. doi: 10.1080/07391102.2022.2028679
Redrobe, J. P., Jørgensen, M., and Christoffersen, C. T. (2014). In vitro and in vivo characterisation of Lu AF64280, a novel, brain penetrant phosphodiesterase (PDE) 2A inhibitor : Potential relevance to cognitive deficits in schizophrenia. Psychopharmacology 231, 3151–3167. doi: 10.1007/s00213-014-3492-7
Reyes-Irisarri, E., Markerink-Van Ittersum, M., Mengod, G., and De Vente, J. (2007). Expression of the cGMP-specific phosphodiesterases 2 and 9 in normal and Alzheimer’s disease human brains. Eur. J. Neurosci. 25, 3332–3338. doi: 10.1111/j.1460-9568.2007.05589.x
Ribaudo, G., Memo, M., and Gianoncelli, A. (2021). Multi-target natural and nature-inspired compounds against neurodegeneration: A focus on dual cholinesterase and phosphodiesterase inhibitors. Appl. Sci. 11:5044. doi: 10.3390/app11115044
Ribaudo, G., Ongaro, A., Zagotto, G., Memo, M., and Gianoncelli, A. (2020). Therapeutic potential of phosphodiesterase inhibitors against neurodegeneration: The perspective of the medicinal chemist. ACS Chem. Neurosci. 11, 1726–1739. doi: 10.1021/acschemneuro.0c00244
Rutten, K., Van Donkelaar, E. L., Ferrington, L., Blokland, A., Bollen, E., Steinbusch, H. W., et al. (2009). Phosphodiesterase inhibitors enhance object memory independent of cerebral blood flow and glucose utilization in rats. Neuropsychopharmacology 34, 1914–1925. doi: 10.1038/npp.2009.24
Salloway, S. P., Demattos, R. B., Weber, C. J., Carrillo, M. C., Sevingy, J., Budur, K., et al. (2020). Advancing combination therapy for Alzheimer’s disease. Alzheimers Dement. 6:e12073. doi: 10.1002/trc2.12073
Sallustio, F., and Studer, V. (2016). Targeting new pharmacological approaches for Alzheimer’s disease: Potential for statins and phosphodiesterase inhibitors. CNS Neurol. Disord. Drug Targets 15, 647–659. doi: 10.2174/1871527315666160518123727
Sanders, O., and Rajagopal, L. (2020). Phosphodiesterase inhibitors for Alzheimer’s disease : A systematic review of clinical trials and epidemiology with a mechanistic rationale. J. Alzheimers Dis. Rep. 4, 185–215. doi: 10.3233/ADR-200191
Sarma, A. K., Khandker, N., Kurczewski, L., and Brophy, G. M. (2016). Medical management of epileptic seizures: Challenges and solutions. Neuropsychiatr. Dis. Treat. 12, 467–485.
Sato, Y., Akao, T., He, J. X., Nojima, H., Kuraishi, Y., Morota, T., et al. (2006). Glycycoumarin from Glycyrrhizae Radix acts as a potent antispasmodic through inhibition of phosphodiesterase 3. J. Ethnopharmacol. 105, 409–414. doi: 10.1016/j.jep.2005.11.017
Schlossmann, J., and Hofmann, F. (2005). cGMP-dependent protein kinases in drug discovery. Drug Discov. Today 10, 627–634.
Schultz, J. E., and Natarajan, J. (2013). Regulated unfolding: A basic principle of intraprotein signaling in modular proteins. Trends Biochem. Sci. 38, 538–545. doi: 10.1016/j.tibs.2013.08.005
Sharma, V. K., Singh, T. G., and Singh, S. (2020). Cyclic nucleotides signaling and phosphodiesterase inhibition: Defying Alzheimer’s disease. Curr. Drug Targets 21, 1371–1384. doi: 10.2174/1389450121666200727104728
Soderling, S. H., Bayuga, S. J., and Beavo, J. A. (1999). Isolation and characterization of a dual-substrate phosphodiesterase gene family: PDE10A. Proc. Natl. Acad. Sci. U.S.A. 96, 7071–7076. doi: 10.1073/pnas.96.12.7071
Stancheva, S. L., and Alova, L. G. (1993). Ginsenoside Rg1 inhibits the brain cAMP phosphodiesterase activity in young and aged rats. Gen. Pharmacol. 24, 1459–1462. doi: 10.1016/0306-3623(93)90435-z
Strang, H., Kaul, A., Parikh, U., Masri, L., Saravanan, S., Li, H., et al. (2020). “Role of cytokines and chemokines in wound healing,” in Wound healing, tissue repair, and regeneration in diabetes, eds D. Bagchi, A. Das, and S. Roy (Amsterdam: Elsevier), 197–235.
Temkitthawon, P., Hinds, T. R., Beavo, J. A., Viyoch, J., Suwanborirux, K., Pongamornkul, W., et al. (2011). Kaempferia parviflora, a plant used in traditional medicine to enhance sexual performance contains large amounts of low affinity PDE5 inhibitors. J. Ethnopharmacol. 137, 1437–1441. doi: 10.1016/j.jep.2011.08.025
Tian, Y., Cui, W., Huang, M., Robinson, H., Wan, Y., Wang, Y., et al. (2014). Dual Speci fi city and Novel Structural Folding of Yeast Phosphodiesterase - 1 for Hydrolysis of Second Messengers Cyclic Adenosine and Guanosine 3 ‘,5’-Monophosphate. Biochemistry 53, 4938–4945. doi: 10.1021/bi500406h
Tibbo, A. J., Tejeda, G. S., and Baillie, G. S. (2019). Understanding PDE4’s function in Alzheimer’s disease; A target for novel therapeutic approaches. Biochem. Soc. Trans. 47, 1557–1565. doi: 10.1042/BST20190763
Tsuji, M., Ohshima, M., Yamamoto, Y., Saito, S., Hattori, Y., Tanaka, E., et al. (2020). Cilostazol, a phosphodiesterase 3 inhibitor, moderately attenuates behaviors depending on sex in the Ts65Dn mouse model of Down syndrome. Front. Aging Neurosci. 12:106. doi: 10.3389/fnagi.2020.00106
Wang, G., Chen, L., Pan, X., Chen, J., Wang, L., Wang, W., et al. (2016). The effect of resveratrol on beta amyloid-induced memory impairment involves inhibition of phosphodiesterase-4 related signaling. Oncotarget 7, 17380–17392. doi: 10.18632/oncotarget.8041
Wang, H., Xu, J., Lazarovici, P., Quirion, R., and Zheng, W. (2018a). cAMP response element-binding protein (CREB): A possible signaling molecule link in the pathophysiology of schizophrenia. Front. Mol. Neurosci. 11:255. doi: 10.3389/fnmol.2018.00255
Wang, H., Gaur, U., Xiao, J., Xu, B., Xu, J., and Zheng, W. (2018b). Targeting phosphodiesterase 4 as a potential therapeutic strategy for enhancing neuroplasticity following ischemic stroke. Int. J. Biol. Sci. 14, 1745–1754. doi: 10.7150/ijbs.26230
Wang, H., Zhang, F. F., Xu, Y., Fu, H. R., Wang, X., Wang, L., et al. (2020). The Phosphodiesterase-4 inhibitor roflumilast, a potential treatment for the comorbidity of memory loss and depression in Alzheimer’s disease: A preclinical study in APP/PS1 transgenic mice. Int. J. Neuropsychopharmacol. 23, 700–711. doi: 10.1093/ijnp/pyaa048
Weintraub, W. S. (2006). The vascular effects of cilostazol. Can. J. Cardiol. 22, 56B–60B. doi: 10.1016/s0828-282x(06)70987-4
Wen, H., Jung, H., and Li, X. (2015). Drug delivery approaches in addressing clinical pharmacology-related issues : Opportunities and challenges. AAPS J. 17, 1327–1340. doi: 10.1208/s12248-015-9814-9
Wilkins, M. R., Wharton, J., Grimminger, F., and Ghofrani, H. A. (2008). Phosphodiesterase inhibitors for the treatment of pulmonary hypertension. Eur. Respir. J. 32, 198–209. doi: 10.1183/09031936.00124007
Wilson, J. M., Ogden, A. M. L., Loomis, S., Gilmour, G., Baucum, A. J., Belecky-Adams, T. L., et al. (2015). Phosphodiesterase 10A inhibitor, MP-10 (PF-2545920), produces greater induction of c-Fos in dopamine D2 neurons than in D1 neurons in the neostriatum. Neuropharmacology 99, 379–386. doi: 10.1016/j.neuropharm.2015.08.008
Wu, Y., Li, Z., Huang, Y.-Y., Wu, D., and Luo, H.-B. (2018). Novel phosphodiesterase inhibitors for cognitive improvement in Alzheimer’s Disease: Miniperspective. J. Med. Chem. 61, 5467–5483. doi: 10.1021/acs.jmedchem.7b01370
Xia, M., Huang, R., Guo, V., Southall, N., Cho, M. H., Inglese, J., et al. (2009). Identification of compounds that potentiate CREB signaling as possible enhancers of long-term memory. Proc. Natl. Acad. Sci. U.S.A. 106, 2412–2417. doi: 10.1073/pnas.0813020106
Xiong, X., Tang, N., Lai, X., Zhang, J., Wen, W., Li, X., et al. (2021). Insights into amentoflavone: A natural multifunctional biflavonoid. Front. Pharmacol. 12:768708. doi: 10.3389/fphar.2021.768708
Yafi, F. A., Sharlip, I. D., and Becher, E. F. (2018). Update on the safety of phosphodiesterase type 5 inhibitors for the treatment of erectile dysfunction. Sex. Med. Rev. 6, 242–252. doi: 10.1016/j.sxmr.2017.08.001
Yan, K., Gao, L. N., Cui, Y. L., Zhang, Y., and Zhou, X. (2016). The cyclic AMP signaling pathway: Exploring targets for successful drug discovery (review). Mol. Med. Rep. 13, 3715–3723. doi: 10.3892/mmr.2016.5005
Yang, H., Wang, J., Zhang, X., Zhang, Y., Qin, Z. L., Wang, H., et al. (2019). Application of topical phosphodiesterase 4 inhibitors in mild to moderate atopic dermatitis: A systematic review and meta-analysis. JAMA Dermatol. 155, 585–593. doi: 10.1001/jamadermatol.2019.0008
Yiannopoulou, K. G., and Papageorgiou, S. G. (2013). Current and future treatments for Alzheimer’s disease. Ther. Adv. Neurol. Disord. 6, 19–33. doi: 10.1177/1756285612461679
Yougbare, I. (2021). Alterations of cAMP / cGMP signaling pathways in lupus nephritis. J. Nephrol. Sci. 3, 8–12.
Yu, M. C., Chen, J. H., Lai, C. Y., Han, C. Y., and Ko, W. C. (2010). Luteolin, a non-selective competitive inhibitor of phosphodiesterases 1-5, displaced [3H]-rolipram from high-affinity rolipram binding sites and reversed xylazine/ketamine-induced anesthesia. Eur. J. Pharmacol. 627, 269–275. doi: 10.1016/j.ejphar.2009.10.031
Zhang, C., Zhou, Q., Wu, X. N., Huang, Y. S., Zhou, J., Lai, Z., et al. (2018). Discovery of novel PDE9A inhibitors with antioxidant activities for treatment of Alzheimer’s disease. J. Enzyme Inhib. Med. Chem. 33, 260–270. doi: 10.1080/14756366.2017.1412315
Zimmermann, G. R., Lehar, J., and Keith, C. T. (2007). Multi-target therapeutics: When the whole is greater than the sum of the parts. Drug Discov. Today 12, 34–42. doi: 10.1016/j.drudis.2006.11.008
Zuccarello, E., Acquarone, E., Calcagno, E., Argyrousi, E. K., Deng, S.-X., Landry, D. W., et al. (2020). Development of novel phosphodiesterase 5 inhibitors for the therapy of Alzheimer’s disease. Biochem. Pharmacol. 176:113818.
Keywords: natural products, phosphodiesterase, Alzheimer’s disease, cyclic-AMP, Vinpocetine
Citation: Sheng J, Zhang S, Wu L, Kumar G, Liao Y, GK P and Fan H (2022) Inhibition of phosphodiesterase: A novel therapeutic target for the treatment of mild cognitive impairment and Alzheimer’s disease. Front. Aging Neurosci. 14:1019187. doi: 10.3389/fnagi.2022.1019187
Received: 14 August 2022; Accepted: 05 September 2022;
Published: 04 October 2022.
Edited by:
Ying Xu, University at Buffalo, United StatesReviewed by:
Jinfang Ge, Anhui Medical University, ChinaYunfeng Li, Academy of Military Medical Sciences (AMMS), China
Copyright © 2022 Sheng, Zhang, Wu, Kumar, Liao, GK and Fan. This is an open-access article distributed under the terms of the Creative Commons Attribution License (CC BY). The use, distribution or reproduction in other forums is permitted, provided the original author(s) and the copyright owner(s) are credited and that the original publication in this journal is cited, in accordance with accepted academic practice. No use, distribution or reproduction is permitted which does not comply with these terms.
*Correspondence: Huizhen Fan, fhz9205@163.com