- 1Key Laboratory of Adolescent Health Assessment and Exercise Intervention of Ministry of Education, East China Normal University, Shanghai, China
- 2College of Physical Education and Health, East China Normal University, Shanghai, China
Alzheimer’s disease (AD) is an age-related neurodegenerative disorder, characterized by the accumulation of proteinaceous aggregates and neurofibrillary lesions composed of β-amyloid (Aβ) peptide and hyperphosphorylated microtubule-associated protein tau, respectively. It has long been known that dysregulation of cholinergic and monoaminergic (i.e., dopaminergic, serotoninergic, and noradrenergic) systems is involved in the pathogenesis of AD. Abnormalities in neuronal activity, neurotransmitter signaling input, and receptor function exaggerate Aβ deposition and tau hyperphosphorylation. Maintenance of normal neurotransmission is essential to halt AD progression. Most neurotransmitters and neurotransmitter-related drugs modulate the pathology of AD and improve cognitive function through G protein-coupled receptors (GPCRs). Exercise therapies provide an important alternative or adjunctive intervention for AD. Cumulative evidence indicates that exercise can prevent multiple pathological features found in AD and improve cognitive function through delaying the degeneration of cholinergic and monoaminergic neurons; increasing levels of acetylcholine, norepinephrine, serotonin, and dopamine; and modulating the activity of certain neurotransmitter-related GPCRs. Emerging insights into the mechanistic links among exercise, the neurotransmitter system, and AD highlight the potential of this intervention as a therapeutic approach for AD.
Introduction
With a rapidly aging global population, the number of individuals living with dementia has more than doubled from 1990 to 2016 (Collaborators, 2019). Currently, dementia affects more than 50 million people worldwide, and this number is projected to reach 152 million by 2050 (Patterson, 2020). Alzheimer’s disease (AD) is the most common cause of dementia No authors listed (2021). The earliest and most prominent symptom of AD is memory decline; however, as the disease progresses, it can also cause a large number of psychological and behavioral changes, which create immense distress for patients and care givers (Geda et al., 2013; Ismail et al., 2022). Although AD represents a growing burden for families and society, its complexity and multifactorial etiology pose unique challenges in the study of its pathogenesis and the development therapies.
Based on previous studies, extracellular senile plaques composed of deposits of β-amyloid (Aβ) peptide and neurofibrillary tangles (NFTs) of hyperphosphorylated tau protein are widely recognized as pathological hallmarks of AD (Jack et al., 2013; Chong et al., 2021). However, despite great expectations, only a small number of antibodies targeting Aβ or tau have been selected for investigation in clinical trials (Long and Holtzman, 2019). In June 2021, aducanumab (aducanumab-avwa; Aduhelm™), a human immunoglobulin gamma 1 monoclonal antibody directed against aggregated soluble and insoluble forms of Aβ, was approved by the Food and Drug Administration (FDA) as the first immunotherapy for AD (Dhillon, 2021). As aducanumab is a new drug, its efficacy, durability, and side effects still need to be further evaluated (Day et al., 2022). The neurotransmitter system is among the earliest affected and most strongly affected systems during the development of AD. Accumulating evidence shows that aberrant neurotransmission, especially in the cholinergic system, is a major pathological factor in AD (Hampel et al., 2018; Richter et al., 2018). In addition to aducanumab and memantine, another three drugs for AD treatment have been approved by the FDA, namely donepezil, galantamine, and rivastigmine. These are all inhibitors of the enzyme acetylcholinesterase (AChE), which can effectively increase acetylcholine (ACh) levels and offer some symptomatic benefit for patients with AD (Joe and Ringman, 2019). Furthermore, AD is closely associated with impaired monoaminergic neurotransmission, mainly involving the dopaminergic, serotoninergic, and noradrenergic systems (Simic et al., 2017; Morgese and Trabace, 2019). In addition to AD, defects in the cholinergic or/and monoaminergic neurotransmitter systems have been shown to be associated with pathological development and clinical manifestations of primary tauopathies, including frontotemporal dementia, progressive supranuclear palsy, and corticobasal syndrome (Huey et al., 2006; Murley and Rowe, 2018), as well as tauopathies with environmental exposure such as chronic traumatic encephalopathy (Mufson et al., 2021) and Parkinsonism-dementia complex of Guam (Nakano and Hirano, 1983; Yamamoto and Hirano, 1985; Goto et al., 1990). Thus, enzymes and proteins involved in the anabolism and catabolism of neurotransmitters and their receptors are potential therapeutic targets for multiple tauopathies including AD.
A wide variety of molecular structures have been found to act as neurotransmitter receptors, the most numerous of which are ligand-gated channels and G protein-coupled receptors (GPCRs) (Nicoll et al., 1990). GPCRs, a large superfamily of receptors with seven transmembrane segments, are the targets of approximately 34% of all drugs approved by the FDA for the treatment of various diseases (Hauser et al., 2017). GPCRs detect and translate extracellular events such as changes in neurotransmitter concentration into intracellular responses by activating signaling effector proteins, i.e., heterotrimeric G proteins, GPCR kinases (GRKs), and arrestins (Wang W. J. et al., 2018). Heterotrimeric G proteins are key transducers of GPCRs and have alpha (α), beta (β), and gamma (γ) subunits. The β and γ subunits remain associated throughout the signaling cycle and form the Gβγ dimer. Gα proteins can be divided into four main classes according to their Gα sequence: Gαs, Gαi/o, Gαq/11, and Gα12/13 (Simon et al., 1991). A large body of evidence indicates that G protein-mediated signaling pathways have important regulatory roles in Aβ deposition and tau hyperphosphorylation (see Figure 1 for details). Moreover, the roles of GRKs including GRK5 (Zhao J. et al., 2019) and arrestins such as β1-arrestin (Liu et al., 2013) and β2-arrestin (Thathiah et al., 2013), among others, should not be ignored.
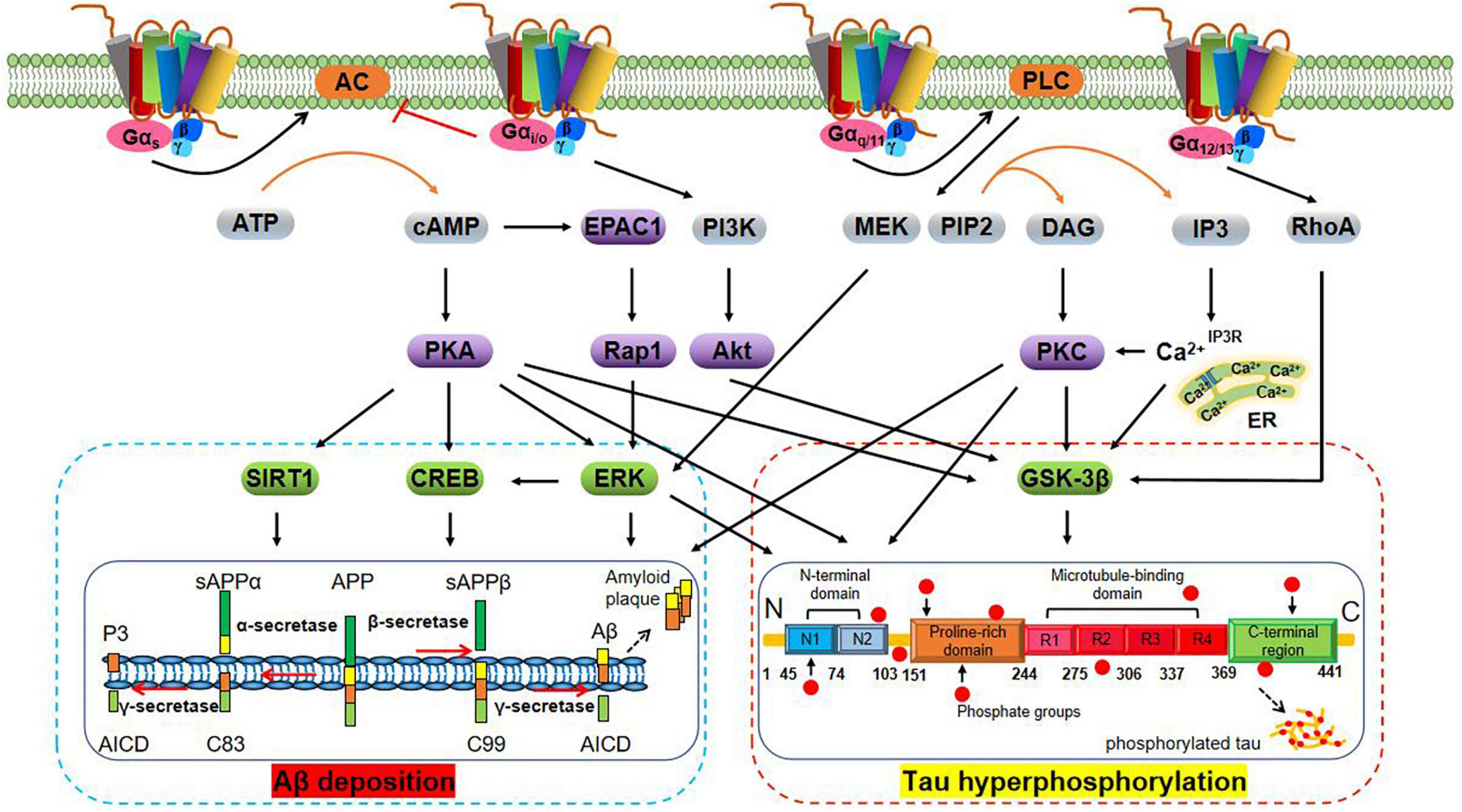
Figure 1. Schematic representation of G protein regulation of Aβ deposition and tau hyperphosphorylation-related signaling pathways. Aβ is derived from the amyloidogenic cleavage of the transmembrane amyloid precursor protein (APP) mediated by α-, β-, and γ-secretases. In the amyloidogenic pathway, APP is first cleaved by the β-secretase (BACE-1), which generates soluble amyloid precursor protein β (sAPPβ) and the β-C-terminal fragment (β-CTF, also termed C99). The latter is cleaved by γ-secretase to generate the Aβ peptide and the amyloid intracellular domain (AICD). The Aβ peptide aggregates to form Aβ oligomers (oAβ) and extracellular amyloid plaques (Hamm et al., 2017). In the non-amyloidogenic pathway, cleavage of APP by α-secretases [especially A disintegrin and metalloprotease 9 (ADAM9), ADAM10, and ADAM17] generates sAPPα and carboxy-terminal fragment termed C83. Subsequent cleavage of C83 by the γ-secretase complex yields the AICD and a short fragment termed P3 (De Strooper, 2010). Tau is an axonal protein expressed in mature neurons that promotes the self-assembly of tubulin into microtubules and its stabilization. The physiological function of tau depends on its phosphorylation status and is regulated by tau protein kinase and phosphatase. In AD brains, tau hyperphosphorylation under the abnormal regulation of protein kinases [e.g., glycogen synthase kinase-3β (GSK-3β)] results in the formation of NFTs (Congdon and Sigurdsson, 2018). Activation of Gαs protein activates adenylyl cyclase (AC) and promotes cyclic adenosine monophosphate (cAMP) generation. cAMP regulates Aβ deposition and tau hyperphosphorylation via activation of the extracellular regulated protein kinase (ERK) (Angulo et al., 2003), cAMP-response element binding protein (CREB) (Wang Z. et al., 2018), silent mating type information regulation 2 homolog 1 (SIRT1), and GSK3β interaction protein/GSK3 (Ko et al., 2019; Zhang Z. et al., 2020) signaling pathways in a protein kinase A (PKA)-dependent manner (Lebel et al., 2009), and exchange protein directly activated by cAMP 1 (EPAC1)/Rap1 in a PKA-independent manner (Maillet et al., 2003). By contrast, activation of Gαi/o protein inhibits the cAMP/PKA pathway. Activation of Gβγ protein may regulate tau phosphorylation through phosphatidylinositol 3-kinase (PI3K)/protein kinase B (PKB, also termed Akt)/GSK-3β pathway (Wang et al., 2016). Activation of Gαq/11 protein activates phospholipase C (PLC) to produce inositol trisphosphate (IP3) and diacylglycerol (DAG), which in turn increases concentrations of intracellular calcium (Ca2+) and activates PKC, and leads to blocking of tau hyperphosphorylation and inactivation of GSK-3β (Medeiros et al., 2011; Garwain et al., 2020). Furthermore, the Gαq/11/PLC pathway can regulate Aβ generation through the MEK/ERK/CREB pathway, among others (Wang Z. et al., 2018). Activation of Gα12/13 protein activates GSK-3β in a manner dependent on Ras homolog gene family, member A (RhoA) (Sayas et al., 2002).
Extensive studies have investigated the effects of physical activity or exercise on individuals with AD. Prior cross-sectional studies confirmed that levels of physical activity or exercise in autosomal dominant AD mutation carriers were associated with levels of AD biomarkers within the central nervous system and with cognitive performance (Brown et al., 2017; Muller et al., 2018). Furthermore, longitudinal studies demonstrated that low levels of physical activity were associated with a higher risk of dementia in older individuals (Tan et al., 2017), whereas regular physical activity could reduce the risk or delay the onset of dementia and AD, especially among genetically susceptible individuals (Rovio et al., 2005). Nowadays, physical activity and exercise have been widely acknowledged as effective strategies for improving AD pathology and AD-associated cognitive impairment (Northey et al., 2018; Jia et al., 2019; de Farias et al., 2021). From a mechanistic perspective, macroscopically, regular exercise has been shown to alleviate some abnormalities of brain structure and function and to increase cerebral blood flow in subjects with mild cognitive impairment (MCI) and AD (Broadhouse et al., 2020; Tomoto et al., 2021; Yu et al., 2021); microscopically, exercise training not only increases levels of exerkines (e.g., irisin, Lourenco et al., 2019; Islam et al., 2021) and metabolic factors (e.g., lactate, El Hayek et al., 2019) in the peripheral circulation, which act on the AD brain indirectly, but also exert direct neuroprotective effects by increasing levels of brain-derived neurotrophic factor (BDNF) (Wang and Holsinger, 2018) and promoting adult hippocampal neurogenesis (Choi et al., 2018), enhancing synaptic plasticity (Mu et al., 2022), reducing neuroinflammation and oxidative stress (Zhang et al., 2019), and ameliorating Aβ deposition and tau hyperphosphorylation (Brown et al., 2019). Strikingly, the activity of central neurotransmitter systems seems to be strongly modulated by exercise. Changes in physiological levels of neurotransmitters and activity of GPCRs may represent important pathways by which exercise improves AD. This article summarizes the correlations between abnormalities in the cholinergic and monoaminergic systems and the development of AD neuropathology, as well as the underlying mechanisms by which exercise affects these processes.
Cholinergic System
Cholinergic Disturbances in Alzheimer’s Disease
The basal forebrain complex comprising the medial septum, horizontal and vertical diagonal band (VDB) of Broca and nucleus basalis of Meynert (NBM), is an important structural basis for cholinergic projections (Schliebs and Arendt, 2011). Aging leads to moderate degenerative changes in basal forebrain cholinergic neurons (BFCNs) located in this complex, accompanied by loss of the neurotransmitter ACh, resulting in reduction of cholinergic projections in the cerebral cortex and hippocampus (Schliebs and Arendt, 2011; Ruan et al., 2018). Considerable advances have been made in our understanding of the roles of the cholinergic system in the development of AD since the 1970s and 1980s (Bowen et al., 1976; Davies and Maloney, 1976; Whitehouse et al., 1982; Coyle et al., 1983). Abnormal cholinergic activity and function have been extensively observed in both AD animal models (Zhu et al., 2017; Xhima et al., 2020) and human patients (Fernandez-Cabello et al., 2020; Machado et al., 2020). Notably, the loss of cholinergic neurotransmission in AD is mainly due to the dysfunction of BFCNs and a decrease in physiological levels of ACh at the cholinergic synapse. The degeneration of BFCNs in the NBM and VDB induced by Aβ deposition and tau hyperphosphorylation is an important pathological mechanism underlying cognitive deficits in AD patients (Vana et al., 2011; Baker-Nigh et al., 2015; Gonzalez et al., 2021). Thus, there is an urgent need for therapeutics and delivery methods that slow or reverse the degeneration of cholinergic neurons in AD.
ACh, an important neurotransmitter in cholinergic transmission, participates in a range of cognitive activities including attention, learning, and memory (Hasselmo, 2006; Haam and Yakel, 2017). The cholinergic hypothesis of AD has inspired research into the roles of ACh in this disease, resulting in a widely held view that restoring levels of ACh may be useful in treating AD. ACh can promote the soluble Aβ peptide conformation rather than the aggregation-prone β-sheet conformation (Grimaldi et al., 2016; Polverino et al., 2018), and regulate tau phosphorylation (Rubio et al., 2006), to combat Aβ and tau pathology. Of concern, the synthesis, transport, release, and metabolism of ACh are multi-step processes that need to be finely modulated by choline, acetyl coenzyme A, choline acetyltransferase (ChAT), vesicular acetylcholine transporter, AChE, and choline transporters, among others (Ferreira-Vieira et al., 2016). However, the progressive dysfunction of certain key components during aging results in decreased ACh levels and subsequent memory deficits (Bartus et al., 1982). Currently, much attention is paid to changes in the activity of ACh-synthesizing enzyme ChAT and ACh-degrading enzyme AChE in AD. Biochemical examinations of brain tissue samples obtained from AD animal models and human patients have revealed reduced ChAT activity and increased AChE activity in multiple brain areas (Bailey and Lahiri, 2012; Atukeren et al., 2017; Zheng et al., 2018). Supplementation with exogenous ChAT and/or AChE inhibitors (AChEIs) therefore represents a potential therapeutic strategy against AD.
The role of cholinergic receptors, i.e., nicotinic and muscarinic receptors in cholinergic neurotransmission, is not negligible. Muscarinic acetylcholine receptors (mAChRs) belong to the GPCR family and comprise the M1 and M2 subfamilies with a total of five subtypes. M1 receptors (M1, M3, and M5 mAChRs) are located in the postsynaptic membrane and couple to the Gαq/11 protein (Berizzi et al., 2018), whereas M2 receptors (M2 and M4 mAChRs) act as auto-receptors of the presynaptic membrane to inhibit ACh synthesis and release and couple to the Gαi/o protein (Santiago and Abrol, 2019). M1 mAChR is highly expressed in the frontal cortex and hippocampus, and reduction of its levels exacerbates AD-like pathology and cognitive decline (Shiozaki et al., 2001; Tsang et al., 2006). These findings have more recently been confirmed in animal studies. Data from experiments in 3 × Tg-AD and Tg-SwDI mice indicated that ablating M1 mAChR promoted tau hyperphosphorylation and amyloidogenic processing, which were attributed to changes in PKC and GSK-3β activities, as well as increasing the astrocytic and microglial response associated with Aβ plaques (Medeiros et al., 2011). Consistent with this, the loss of M1 mAChR also resulted in increased levels of brain Aβ and greater accumulation of amyloid plaques in APP/PS1 transgenic mice (Davis et al., 2010). By contrast, activation of M1 mAChR was found to regulate Aβ neurotoxicity and tau pathology and reverse cognitive deficits through activation of PKC and inactivation of GSK-3β in a Gαq/11-dependent manner (Farias et al., 2004; Caccamo et al., 2006). Recently, based on knowledge of M1 mAChR, a variety of agonists (e.g., HTL9936, Brown et al., 2021) and positive allosteric modulators (e.g., VU0486846, Abd-Elrahman et al., 2021) have been designed and synthesized to alleviate the pathology of AD and improve cognitive function (Table 1).
Similar to the results obtained for M1 mAChR, data from experimental in vitro studies indicated that M3 mAChR might be involved in coupling with PLC-mediated hydrolysis of phosphatidylinositol-4, 5-bisphosphate and PKC activation to stimulate the release of sAPP and reduce levels of Aβ (Buxbaum et al., 1992; Nitsch et al., 1992). This suggested that the activity of M3 mAChR was associated with amyloidogenic processing. Furthermore, M3 mAChR may be involved in improving learning and memory in a manner dependent on receptor phosphorylation/arrestin rather than via G protein signaling (Poulin et al., 2010). Abnormalities of M2 auto-receptors in AD brains have been mainly found in the cortex and hippocampus (Mulugeta et al., 2003; Bellucci et al., 2006). Notably, ACh levels can be increased by blockade of presynaptic M2 receptors that regulate ACh release; treatment with an M2 receptor antagonist, i.e., SCH-72788, can increase ACh contents (Lachowicz et al., 2001). The role of M2 receptors in AD has been partially established using a GRK5-deletion animal model. GRK5 is a serine/threonine kinase whose dysfunction selectively impairs desensitization of presynaptic M2 receptors, causes M2 receptors hyperactivity, and inhibits ACh release, resulting in cognitive impairment and AD-like pathology (Liu et al., 2009). In APP transgenic mice, inhibition of hyperactivity of presynaptic M2 receptors by antagonist methoctramine has been shown to be an effective therapy for eliminating the increase in Aβ and tau pathology induced by GRK5 deficiency and promoting ACh release (Cheng et al., 2010; Zhang et al., 2014; Table 1). Collectively, these findings provide evidence that activation of M1 receptors and inhibition of M2 receptors have potential benefits in ameliorating neuropathological features resembling those of AD.
Physical Exercise, Cholinergic System, and Alzheimer’s Disease
Exercise increases cholinergic input in the cortex and hippocampus. In the cerebral cortex, significantly increased ACh contents were observed in rats following walking for as little as 5 min (Kurosawa et al., 1993). This suggests that ACh is released in response to exercise stimulation, even if the exercise volume is not large. Teglas et al. (2019) and Xu et al. (2019) found that chronic exercise programs, i.e., months of treadmill running [40 min/session, 3 sessions/week at 60% maximal oxygen intake (VO2max)] or voluntary wheel running (1 h/session, 5 sessions/week), attenuated age-related reduction of cholinergic fibers, reduced malformations of cholinergic forebrain innervation, and prevented the loss of cholinergic inputs in the hippocampus. Concomitant with these alterations, improvements in cognitive and motor behaviors was recorded. Similarly, Itou et al. (2011) reported that voluntary wheel running could reverse age-associated impairments of cholinergic innervation in the hippocampus of nestin promoter-GFP transgenic mice. In addition, Hall and Savage (2016) and Hall J. M. et al. (2018) observed re-emergence of the cholinergic/nestin neuronal phenotype (i.e., ChAT+/nestin+ neurons) within the medial septum/diagonal band (MS-DB) following exercise training; this improvement may in part have been mediated by nerve growth factor (NGF). These findings represent possible mechanisms by which the cholinergic system could promote cognitive function in response to exercise.
Several studies have reported regulation of the cholinergic system by exercise in AD animal models. In aged APP/PS1 transgenic mice, 4 weeks of continuous non-shock treadmill running (20–60 min/session, 5 sessions/week) improved learning and memory in association with an increased number of cholinergic neurons in the MS-DB (Ke et al., 2011). In THY-Tau22 mice, long-term voluntary wheel running for 9 months reversed pathological reduction of ChAT+ neurons in the MS-DB; this was accompanied by improvements in tau pathology and neuroinflammation within the hippocampus (Belarbi et al., 2011). Moreover, where comparisons were possible, the effect size for exercise was generally comparable with that of donepezil (Pisani et al., 2021). In Aβ25–35-induced rats, both chronic aerobic and resistance exercise have similar effects to those of AChEIs, reducing AChE activity and reversing recognition memory deficits (Farzi et al., 2019). However, conflicting results have been obtained regarding changes in AChE activity after exercise intervention; some investigators found that chronic exercise could increase AChE activity altered by Aβ neurotoxicity to maintain cholinergic system activity (Schimidt et al., 2019, 2021; Dare et al., 2020). Such contradictory results clearly warrant further study.
Several researchers have described the effects of combined interventions on the cholinergic system in AD. Donepezil hydrochloride combined with swimming exercise (7 sessions/week for 4 weeks with no weight bearing) improved learning and memory in Aβ1–40-induced rats, and the mechanism was related to increased ChAT activity and decreased AChE activity in the cortex and hippocampus (Jiangbo and Liyun, 2018). Probiotics can modulate the inflammatory process, counteract oxidative stress, and modify gut microbiota and are considered to be among the best preventive measures against cognitive decline in AD (Naomi et al., 2021). A study found that mono or combined progressive treadmill running (5 sessions/week for 8 weeks) and probiotic (e.g., Bifidobacterium bifidum and Lactobacillus plantarum) treatment significantly increased levels of ACh in the brains of Aβ1–42-induced rats and reversed spatial learning impairment (Shamsipour et al., 2021). Moreover, combined interventions could modulate the activity of M1 mAChR in the brain. Grape seed proanthocyanidin extract (GSPE) has been shown to have a strong antioxidant effect, can protect the central nervous system from oxidative stress damage, and may have a role in alleviating AD-related cognitive impairment (Sun et al., 2019; Gao et al., 2020). A study demonstrated that administration of GSPE and swimming training (2 h/session, 5 sessions/week for 14 weeks with 3% weight bearing) either individually or in combination led to improvements in learning and memory with reduced AChE activity in the medial prefrontal cortex and hippocampus of adult and middle-aged rats. Moreover, both mRNA and protein levels of M1 mAChR were increased in the cortex and hippocampus, and activation of the ERK/CREB/BDNF pathway was observed following swimming training with GSPE treatment (Abhijit et al., 2017). Although other mechanisms may be involved, these findings in individual models of AD indicate the importance of regulation of the cholinergic system (especially cholinergic neurons, ChAT, AChE, ACh, and M1 mAChR) by physical exercise.
Noradrenergic System
Noradrenergic Disturbances in Alzheimer’s Disease
The locus coeruleus (LC) is the norepinephrine (NE)-containing nucleus in the brainstem and innervates into widespread brain regions. It is composed of noradrenergic neurons that project to different brain regions and supplies NE to the cortex, hippocampus, striatum, amygdala, cerebellum, and basal forebrain, among others (Schwarz and Luo, 2015). The integrity of the LC-NE system is critical for attention, arousal, and specific aspects of learning and memory, and its activation across the lifespan is a crucial determinant of later-life cognitive reserve (Sara, 2009; Wilson et al., 2013; Mather and Harley, 2016). However, the LC-NE system is especially vulnerable to toxins and infection (Mather and Harley, 2016). During aging, decline of the LC-NE system is associated with reduced cognitive abilities relating to episodic memory and reduced cognitive reserve (Betts et al., 2019). Cumulative evidence suggests that aberrant tau accumulation in the LC and noradrenergic dysfunction are critical early events in the progression of AD (Mravec et al., 2016; Rorabaugh et al., 2017; Matchett et al., 2021). Aβ aggregation can also cause axonal degeneration in LC neurons (Sakakibara et al., 2021). Ablation of the LC-NE system, in turn, further exacerbates Aβ and tau pathology and the resulting cognitive deficits (Chalermpalanupap et al., 2018; Jacobs et al., 2021) and neuroinflammation (Jardanhazi-Kurutz et al., 2011; Cao et al., 2021), setting up a vicious cycle. Consequently, targeting the LC-NE system may have significant therapeutic potential in AD.
Notably, NE is widely regarded as a mediator of cognitive regulation in multiple neurodegenerative diseases, including AD (Holland et al., 2021). Animal studies suggest a progressive reduction of NE levels within the hippocampus, cortex, and cerebellum during the development of AD (Francis et al., 2012; Rorabaugh et al., 2017); these changes have been found to coincide with altered expression of BDNF and to precede the onset of cognitive and behavioral impairments (Francis et al., 2012). NE also has a central role in regulating Aβ production and Aβ-related pathologies. At the molecular level, it can interact with Aβ and inhibit Aβ generation (Liu et al., 2017; Zou et al., 2019). Furthermore, NE can reduce Aβ-induced neurotoxicity via activating tyrosine kinase receptor B (TrkB) (Liu et al., 2015), alleviate neuroinflammation by downregulating the expression of inducible nitric oxide synthase and interleukin 1β (IL-1β) (Heneka et al., 2002), and attenuate oxidative stress through limiting production of reactive oxygen species (ROS) (Jhang et al., 2014). By contrast, deficiency of NE increases the Aβ burden and activation of microglia and astroglia, and decreases expression and activity of the Aβ degrading-enzyme neprilysin (Kalinin et al., 2007). Thus, NE may improve a wide range of physiological and pathophysiological processes in AD.
To exert its neuroprotective effect, NE binds to adrenergic receptors (ARs), which can be classified into three main categories (i.e., α1, α2, and β) and all belong to the GPCR family. In general, the α1-AR subtypes (i.e., α1A, α1B, and α1D) couple to Gαq/11 (Chen and Minneman, 2005) and Gα12/13 (Maruyama et al., 2002) proteins, whereas the α2-AR subtypes (i.e., α2A, α2B, α2C, and α2D) couple to the Gαi/o protein (Reynolds et al., 2005). Several studies have suggested that inhibition of α1-AR and/or α2-AR activity may represent a new strategy for anti-Aβ therapy. Previous studies showed that treatment with an α1-AR antagonist, i.e., prazosin or doxazosin, could reduce the generation of Aβ in N2a cells, alleviate neuroinflammation, and prevent memory deficits in APP23 transgenic mice (Katsouri et al., 2013), as well as protecting hippocampal slices from Aβ neurotoxicity through prevention of GSK-3β activation and tau hyperphosphorylation in an in vitro model of AD (Coelho et al., 2019). Similar to α1-AR, treatment with α2-AR antagonists including fluparoxan (Scullion et al., 2011), dexefaroxan (Francis et al., 2012), and mesedin (Melkonyan et al., 2017) was found to be beneficial for improving AD-like pathological mechanisms (Table 2). In addition, increased activity of α2A-AR was observed in AD patients and mouse models (Zhang F. et al., 2020). APP enhances the surface retention of α2A-AR and the intensity and duration of its signaling (Zhang et al., 2017); then, α2A-AR reduces Golgi localization of APP and concurrently promotes APP distribution in endosomes and cleavage by BACE1 (Chen et al., 2014). It follows that α2A-AR could serve as a key biological target for Aβ generation. Moreover, α2A-AR may represent a bridge between Aβ and tau pathology. One study demonstrated that oAβ bind to an allosteric site on α2A-AR to redirect NE-elicited signaling and thus to increase tau hyperphosphorylation, which depends on GSK-3β signaling (Zhang F. et al., 2020). The aforementioned results indicate that activation of α2A-AR may aggravate the pathological development of AD. It is necessary to further verify whether its genetic or pharmacological blockade could reduce Aβ production and Aβ-related neuropathology.
β-ARs are crucial targets for increasing synaptic plasticity and maintaining learning and memory (Goodman et al., 2021); however, the total number of β-ARs is significantly reduced in AD brains (Kalaria et al., 1989). NE may exert pleiotropic neuroprotective effects via its action on β-AR signaling in various cell types. Activation of β-AR signaling may be responsible for the activation of CREB and the induction of NGF and BDNF to protect against Aβ neurotoxicity in neurons (Counts and Mufson, 2010). In microglia-like cells, NE suppresses Aβ-induced toxicity and production of monocytic chemotactic protein-1, a pro-inflammatory chemokine, through activating β-AR signaling, accompanied by activation of the cAMP/PKA/CREB pathway (Yang et al., 2012). Furthermore, as shown in Table 2, accumulating evidence from pharmacological intervention studies confirms that β-ARs mediate distinct functions related to different aspects of AD pathology. Some previous studies, for instance, have reported that application of isoproterenol, a non-selective β-AR agonist, worsened Aβ and tau pathology; however, other studies have demonstrated the opposite effect. Hence, the molecular mechanism of the differential effect of the same β-AR agonist in different models of AD needs to be studied. The regulatory roles of different β-AR subtypes in AD pathology should also be considered.
There are three β-AR subtypes (β1, β2, and β3) in the brain that couple to the Gαs protein (Liu et al., 2019; Lee et al., 2020; Su et al., 2020). Recently, the contribution of the β1- and β3-ARs to AD pathology and cognitive function has been a subject of interest. Animal studies have revealed that selective activation of β1-AR activates the cAMP/PKA/CREB pathway to rescue social memory deficit in APP mice (Coutellier et al., 2014), as well as inhibiting the expression of neuroinflammatory markers (e.g., ionized calcium binding adapter molecule 1) and reducing Aβ and tau pathology in 5 × FAD mice (Ardestani et al., 2017). Moreover, treatment with CL-316243, a β3-AR agonist, could reduce Aβ pathology and reverse memory loss (Gibbs et al., 2010; Tournissac et al., 2021; Table 2). Therefore, the current consensus is that pharmacological activation of β1- and β3-ARs is beneficial in AD. However, prior research on β2-AR in AD resulted in seemingly contradictory findings. Some studies showed that activation of β2-AR accelerated amyloid plaque formation, and that this beneficial effect could be reversed by antagonist ICI-118551 (Ni et al., 2006; Yu et al., 2010). Conversely, other studies have demonstrated that treatment with highly selective β2-AR agonists such as clenbuterol can reduce Aβ levels, promote hippocampal neurogenesis, enhance synaptic plasticity, and improve neuronal death and microglial inflammation (Chai et al., 2016, 2017), whereas application of ICI-118551 exacerbates Aβ and tau neuropathology and cognitive deficits (Branca et al., 2014; Wu et al., 2017). Further studies are warranted to confirm and explain these contradictory observations.
Physical Exercise, Noradrenergic System, and Alzheimer’s Disease
Previous studies in animals have shown that central noradrenergic neurons are activated in response to exercise training and participate in thermoregulation during exercise (Ohiwa et al., 2006; Rodovalho et al., 2020). Acute running increases noradrenergic activity, and the longer the running time, the longer the duration of central activation in the recovery period (Pagliari and Peyrin, 1995a,b). In the course of chronic exercise intervention, rodents with long-term exercise training experience showed significant increases (approximately 26%) in levels of NE in the brain (Ostman and Nyback, 1976). Consistent with this, progressive treadmill running for 8 weeks in rodents was accompanied by brain noradrenergic adaptations and increases in NE levels in the areas of NE cell bodies and the spinal cord (Dunn et al., 1996). Based on this information, a single bout of exercise appears to temporarily increase central noradrenergic activity, and the cumulative effect of long-term regular exercise leads to a significant increase in the levels of NE.
Salivary α-amylase (sAA), a non-invasive biomarker of central noradrenergic activity, is a promising avenue for characterizing the arousal-mediated effects of exercise on cognition (Weiss et al., 2019). A study demonstrated that 6 min of stationary bicycle exercise at 70% VO2max significantly enhanced memory consolidation in both patients with amnestic MCI and cognitively normal individuals through activating the noradrenergic system (as determined by measuring sAA) (Segal et al., 2012). In another study, patients with MCI who underwent a chronic mind-body exercise program [60 min/session, 3 sessions/week for 24 weeks at 55–75% heart rate reserve (HRR)] showed a significant increase in intrinsic functional connectivity in the LC-NE system and improvements in cognitive performance, as measured by magnetic resonance imaging (MRI) scans and the Montreal Cognitive Assessment (Liu et al., 2021). These findings suggest that activation of the noradrenergic system by exercise improves cognitive performance of individuals in the prodromal stage of AD.
Studies in animal models found that exercise could also effectively reduce the activity of α2-AR and increase the activity of β-ARs. Spontaneously-Running-Tokushima-Shikoku rats, an animal model for high levels of wheel-running activity, showed decreased hippocampal monoamine oxidase A levels and increased extracellular NE levels, and the elevation of NE levels caused downregulation of α2-AR (Morishima et al., 2006). Furthermore, the affinity of this receptor in the nucleus tractus solitarius was reduced in trained rats compared with sedentary animals (De Souza et al., 2001). These results indicate that long-term exercise may lead to reduced affinity of α2-AR in multiple brain regions. Considering the anti-AD effect produced by inhibiting α2-AR, the potential of α2-AR-mediated exercise to improve AD deserves future exploration. In addition to the findings for α2-AR, Ebrahimi et al. (2010) confirmed that exercise enhanced learning and memory through β-AR-dependent pathways by administering propranolol to mice. Indeed, previous studies have proposed that an intact noradrenergic system, especially activation of β-ARs by NE, serves as a vital link in the upregulation of BDNF expression by exercise (Garcia et al., 2003; Ma, 2008). BDNF can induce the expression of thioredoxin-1 (TRX-1) via the TrkB/Akt/CREB pathway (Bai et al., 2019). TRX-1 is a disulfide-reducin-system low-molecular-weight protein with redox properties, levels of which are significantly reduced in AD brains (Akterin et al., 2006). Increased TRX-1 levels can alleviate endoplasmic reticulum stress, oxidative stress, and apoptosis in AD (Guo et al., 2021). Recently, an experimental study showed that a treadmill running program (60 min/session, 6 sessions/week for 3 weeks) increased the content of TRX-1 in the hippocampus of mice and activated the ERK1/2/β-catenin/T-cell factor pathway, which in turn promoted hippocampal cell proliferation and neurogenesis (Kim and Leem, 2019). Notably, Kim and Leem (2019) proposed a hypothesis regarding the signaling that links exercise, β2-AR, BDNF, and TRX-1; that is, exercise may promote the expression and interaction of BDNF and TRX-1 through activating the β2-AR/cAMP/PKA pathway. A study further to test this hypothesis subjected an animal model of cognitive impairment induced by a high-fat diet to a treadmill running program (30 min/session, 5 sessions/week for 23 weeks at 40–50% VO2peak); activation of the β2-AR/cAMP/PKA pathway, increased expression of TRX-1 and BDNF, inhibition of microglial activation, decreased expression of inflammatory markers, and reduction of oxidative stress markers in the dentate gyrus of the hippocampus were observed (Han et al., 2019). These results suggest that exercise alleviates neuroinflammation and oxidative stress potentially through a signaling cascade involving β2-AR, BDNF, and TRX-1. However, the relationship among the these three factors needs to be validated in AD animal models and patients.
Serotonergic System
Serotonergic Disturbances in Alzheimer’s Disease
Serotonergic neurotransmission is dependent on the synthesis and release of the neurotransmitter serotonin [5-hydroxytryptamine (5-HT)], and the serotonergic projections from the dorsal raphe nucleus (DRN) have widespread ramifications throughout the brain, including the frontal cortex, temporal cortex, and hippocampus (Vertes, 1991; Vertes et al., 1999). Aging exerts complex effects on the central serotoninergic system. Impaired serotonergic neurotransmission and altered expression of 5-HT transporter (5-HTT) and 5-HT receptors (5-HTRs) have been observed in multiple brain regions, although the number of serotonergic neurons did not change significantly (Rodriguez et al., 2012). Several studies have demonstrated that dysfunction of the serotonergic system is linked to the development of AD pathology (Rodriguez et al., 2012; Tajeddinn et al., 2016b; Jankowska et al., 2018). The number of serotonergic neurons in the DRN (Aletrino et al., 1992; Lyness et al., 2003) and the contents of 5-HT, 5-HTT, and its metabolite 5-hydroxyindoleacetic acid (5-HIAA) in the cortex and hippocampus have been shown to be significantly reduced in AD brains (Palmer et al., 1987; Thomas et al., 2006; Vermeiren et al., 2014). Furthermore, lower concentrations of 5-HT in cerebrospinal fluid (Lanctot et al., 2002) and platelets (Prokselj et al., 2014; Tajeddinn et al., 2016a) have been observed in patients with AD compared with controls. Treatment studies aim to increase serotonergic tone; selective serotonin reuptake inhibitors including escitalopram (Ehrhardt et al., 2019), citalopram (Porsteinsson et al., 2014), and fluoxetine (Xie et al., 2019) have been found to have beneficial effects on psychiatric symptoms and cognitive impairment in patients with AD.
Increasing attention has been paid to the functions of 5-HTRs and their impact on the pathophysiology of AD. In general, 5-HTRs constitutes seven subfamilies, which can be split into a total of 14 subtypes (i.e., 5-HT1A–1FR, 5-HT2A–2cR, 5-HT3R, 5-HT4R, 5-HT5A–5BR, 5-HT6R, and 5-HT7R). Except for 5-HT3R, these receptors belong to the GPCR family (Sharp and Barnes, 2020). The 5-HT1R and 5-HT5R subfamilies couple to the Gαi/o protein and mainly inhibit PKA signaling (Francken et al., 2000; Rojas et al., 2017). 5-HT1R members are expressed in large quantities in the hippocampus and have a significant role in the regulation of memory processes (Ogren et al., 2008). 5-HT1AR is a well-studied member of this subfamily and shows overexpression under Aβ stimulation (Verdurand et al., 2011, 2016). Treatment with 5-HT1AR antagonists (e.g., NAD-299 and WAY-100635) has been shown to reduce amyloid plaque deposition, increase levels of hippocampal BDNF, alleviate neuroinflammation and oxidative stress, and improve cognitive deficits in individual animal models of AD (Afshar et al., 2018, 2019; Wang et al., 2020; Table 3). These results imply that 5-HT1AR, in response to specific ligands, is involved in the regulation of AD pathology through multiple pathways.
Unlike the above two subfamilies, members of the 5-HT2R subfamily couple to the Gαq/11 protein (Odagaki et al., 2017) and are strongly associated with Aβ pathology, especially 5-HT2AR and 5-HT2CR. An imaging study has revealed prominent reductions in neocortical 5-HT2R in patients with AD (Blin et al., 1993). The binding of 5-HT2AR is significantly decreased in the brains of AD animal models (Holm et al., 2010) and human patients (Lai et al., 2005; Marner et al., 2012). Molecular biochemistry studies have disclosed that 5-HT could induce the release of sAPP through activation of 5-HT2AR and 5-HT2CR (Nitsch et al., 1996). Although there is evidence to suggest that the 5-HT2AR and 5-HT2CR modulate sAPP secretion in vitro and in vivo (Table 3), further studies are required to determine whether this effect is mediated by a change in α- or β-secretase activity and whether this effect correlates with a change in Aβ generation. Furthermore, studies have found that administration of 5-HT2AR-selective ligands stimulated the autophagy process of microglia, enhanced the phagocytosis of Aβ (Lu et al., 2021), and reduced amyloid plaques (Afshar et al., 2018). Hence, 5-HT2AR may also be a key target for regulating Aβ clearance and degradation.
The remaining subfamilies (5-HT4R, 5-HT6R, and 5-HT7R) couple to the Gαs protein and activate PKA signaling (Fisher J. R. et al., 2016). 5-HT4R and 5-HT6R are mainly located in brain regions involved in cognitive processes (King et al., 2008). Neurochemical and behavioral studies have demonstrated that activation of 5-HT4R or blockade of 5-HT6R improved cognitive performance (Quiedeville et al., 2015). Recently, 5-HT4R agonists and 5-HT6R antagonists have attracted interest with respect to AD treatment and have been widely investigated from a drug discovery perspective. Studies in vitro and in vivo suggest that activation of 5-HT4R by agonists (e.g., ML10302, prucalopride, and RS-67333) increases levels of neuroprotective sAPPα, reduces amyloid plaque deposition, and rescues cognitive deficits through the classical cAMP/PKA pathway and non-classical pathway. In addition, inhibition of 5-HT6R by antagonists (e.g., SB-258585, SB-399885, and SB-271046) can inhibit Aβ generation, and protect neurons from Aβ-induced neurotoxicity, neuroinflammation, oxidative stress, and apoptosis (Table 3). Preclinical and early clinical studies of 5-HT4R agonism and 5-HT6R antagonism are being conducted to investigate the ability of these approaches to alleviate cognitive deficits associated with AD (Ivachtchenko et al., 2016; Nirogi et al., 2021). 5-HT7R, the most recently discovered receptor of 5-HT, has been shown to regulate individual cognition (Gasbarri and Pompili, 2014). Chronic treatment with AS-19, a selective 5-HT7R agonist, could prevent cognitive deficits by alleviating Aβ plaque accumulation and neuronal apoptosis and improving neuronal plasticity (Hashemi-Firouzi et al., 2017; Shahidi et al., 2018). Furthermore, a recent study revealed that 5-HT7R is an important target for the treatment of tauopathy (Labus et al., 2021). Collectively, 5-HT4R, 5-HT6R, and 5-HT7R may represent novel therapeutic targets for the treatment and prevention of AD.
Physical Exercise, Serotonergic System, and Alzheimer’s Disease
The positive effects of physical exercise on emotional and cognitive performance through activation of the serotonergic system can be summarized as follows. First, exercise enhances the function of the serotonergic system via increases in numbers and activity of serotonergic neurons in the DRN. Treadmill running exercise, whether performed acutely for 30 min or chronically for 3 or 8 weeks, increased the number and activity of serotonergic neurons in the DRN of rodent models (Hong et al., 2015; Otsuka et al., 2016; Ge and Dai, 2020). In particular, 4 weeks of progressive treadmill running (20–60 min/session, 5 sessions/week) selectively improved spatial learning and memory in association with an increase in numbers of serotonergic neurons in the DRN of aged APP/PS1 transgenic mice (Ke et al., 2011); this exercise paradigm also reduced Aβ levels and abnormal microglia activation, but not enough to reduce the plaque loading in the hippocampus.
Second, exercise increases the availability of 5-HT precursor tryptophan (TRP) and TRP hydroxylase (TPH), and increases levels of 5-HT and 5-HTAA. Studies in humans suggest that a bout of exercise, i.e., 35 min of graded exercise or 60 min of treadmill exercise, can increase serum TRP and 5-HT levels and exert pro-cognitive and antidepressant effects (Melancon et al., 2012; Zimmer et al., 2016). Additional studies found that 5-HT released during yoga and meditative practices activated an alternate cleavage of APP to produce a fragment with known neurotrophic effects, giving it the unique ability to inhibit the oAβ production cycle in an in vitro AD model (Hassan et al., 2018). Data from animal studies indicate that exercise significantly increases the synthesis and metabolism of central 5-HT (Dey et al., 1992), and is required for the exercise-induced neurogenic response, especially adult hippocampal neurogenesis (Klempin et al., 2013, 2018). Furthermore, saffron combined with endurance exercise increased levels of hippocampal 5-HIAA, and this change was associated with improved short-term memory (Akbari-Fakhrabadi et al., 2021). Regulation of central 5-HT synthesis and metabolism by exercise may represent a therapeutic opportunity in depression and age-related cognitive decline. Isolation stress accelerates the onset of AD (Huang et al., 2015; Peterman et al., 2020), reducing TPH and 5-HT expression in the DRN and promoting apoptosis in the hippocampus, leading to anxiety and memory decline during old age, whereas a swimming exercise program (30 min/session, 5 sessions/week for 4 weeks) reversed these changes (Park et al., 2020). Moreover, three different forms of exercise (i.e., treadmill exercise, involuntary exercise and voluntary exercise, 5 sessions/week for 4 weeks) could all improve cognitive and behavioral functions by increasing levels of hippocampal 5-HT in a vascular dementia rat model (Zhang L. et al., 2020).
Third, exercise can regulate the expression of the serotonergic receptor and the activity of its downstream signaling pathway to improve impaired cognitive ability and abnormal emotion. Notably, as the hippocampal neurons mainly express type II AC, which is not regulated by the Gαi/o subunit but is activated by the Gβγ subunit, the cAMP/PKA pathway is activated by 5-HT1AR (Albert and Vahid-Ansari, 2019). Exercise improves cognitive function by increasing the expression of 5-HT1AR in hippocampal neurons, which is potentially associated with BDNF-related signaling. Results from several animal studies are consistent with this notion. For instance, Kim et al. (2015) reported that 4 weeks of treadmill running (30 min/session, 7 sessions/week) enhanced CREB phosphorylation and increased the expression of BDNF and TrkB via activation of 5-HT1AR in rat hippocampus neurons. Consistent with this, the improvements in learning and memory after rats underwent a chronic and progressive treadmill running program (30–60 min/session, 3 sessions/week for 14 weeks at 60–70% VO2max) were found to be due to increased expression of 5-HT, 5-HTT, 5-HT1AR, and BDNF in the hippocampal CA1 area (Pietrelli et al., 2018). In addition, treadmill exercise training (45 min/session, 3 sessions/week for 32 weeks) could ameliorate anxious/depressive-like behavior and attenuate fear-avoidance behavior deficits in TgF344-AD rats in the early stage of Alzheimer’s pathogenesis by increasing the expression of 5-HT and 5-HT6R in the cortex and hippocampus (Wu et al., 2020). Existing studies on the regulation of individual cognition by exercise through the regulation of serotonergic receptor function are still lacking, and more detailed evidence is necessary.
Dopaminergic System
Dopaminergic Disturbances in Alzheimer’s Disease
Dopamine (DA) is a major catecholamine neurotransmitter that projects dopaminergic signaling to the prefrontal cortex, hippocampus, striatum, nucleus accumbens, amygdala, and other areas from the ventral tegmental nucleus area (VTA) of the midbrain; it is mainly involved in emotion, behavior, and cognition, and in regulation of synaptic plasticity (Jay, 2003; Bjorklund and Dunnett, 2007). Aging is associated with a loss of dopaminergic function, which may originate from defects on multiple components, including loss of dopamine-producing neurons, atrophy of projection brain regions, and reduced density of dopamine receptors. These alterations result in the efficiency of dopaminergic projecting systems declines slowly during physiological aging (Ciampa et al., 2021; Gasiorowska et al., 2021). Cumulative evidence suggests that impaired dopaminergic neurotransmission is also involved in the pathological development of a variety of neurological disorders, including AD (Martorana and Koch, 2014; D’Amelio et al., 2018). In particular, loss of dopaminergic neurons in the VTA and/or substantia nigra pars compacta (SNpc) (Mann et al., 1987; Nobili et al., 2017) and significantly decreased levels and availability of DA in the cortex and hippocampus have been observed in AD animal models and human patients, where they led to severe synaptic dysfunction and cognitive deficits (Reinikainen et al., 1988; Trabace et al., 2007). In a recent animal study, Aβ decreased cortical DA levels and caused profound impairment of both long-term potentiation (LTP) and long-term depression, as well as recognition memory (Moreno-Castilla et al., 2016). In the Tg2576 mouse model of AD, dopaminergic neuron loss was shown to begin before Aβ plaque formation, resulting in reduced hippocampal DA outflow, which decreased neuronal synaptic plasticity and excitability and contributed to memory and reward dysfunction; nevertheless, these defects could be partially reversed with DA precursor levodopa (L-DOPA) supplementation (Nobili et al., 2017; Cordella et al., 2018). Thus, increasing DA levels moderately may ameliorate synaptic dysfunction and cognitive decline in AD.
In addition to improvements in neuroplasticity, the effects of DA on AD neuropathology include disruption of Aβ protofibril and inhibition of Aβ aggregation, as well as partial alleviation of neuroinflammation, and oxidative stress. On the one hand, DA can inhibit Aβ aggregation and disrupt Aβ fibrils in a dose-dependent manner (Huong et al., 2010; Liu et al., 2016). Recently, Chen et al. (2021) reported that DA disrupted Aβ protofibrils and prevented Aβ dimerization at the molecular level mostly through π-π stacking interactions with residues F4, H6, and H13; hydrogen-bonding interactions with negatively charged residues D7, E11, E22, and D23; and cation-π interactions with residue R5. This may be an important mechanism by which DA interferes with Aβ generation. On the other hand, DA and its derivatives significantly diminish neuroinflammation and oxidative stress triggered by lipopolysaccharides (LPS) and Aβ through decreasing levels of inflammatory mediators and upregulating expression of heme oxygenase-1, the enzyme responsible for production of antioxidants (Nam et al., 2018). Further research should determine the optimal dose-effect relationship for DA regulation of AD-like pathology.
Dopaminergic receptors, which are widely distributed in various brain regions, belong to the GPCR family and can be divided into D1-like receptors (i.e., DA1R and DA5R) and D2-like receptors (i.e., DA2R, DA3R, and DA4R) according to their biological and pharmacological properties (Beaulieu and Gainetdinov, 2011). D1-like receptors are widely distributed in the brain, couple to Gαs and Gαq/11 proteins (Himmelreich et al., 2017), and regulate PKA and PLC signaling, whereas D2-like receptors couple to the Gαi/o protein (Conley and Watts, 2013). Interestingly, a recent systematic review and network meta-analysis indicated that DA1R and DA2R levels were decreased in patients with AD compared with controls; the dopaminergic receptors were ranked as follows according to their correlation with AD from highest to lowest: DA2R, DA3R, DA4R, DA5R, and DA1R (Pan et al., 2019). As shown in Table 4, application of D1-like receptor agonists (e.g., L-stepholidine, L-theanine, and SKF-38393) reduced Aβ and tau pathology and significantly improved synaptic dysfunction and cognition. These results are largely consistent with the activation of PKA signaling. Furthermore, in vitro and in vivo experiments confirmed that application of selective DA1R agonist A-68930 significantly ameliorated neuroinflammation and mitochondrial dysfunction through adenine monophosphate activated protein kinase (AMPK)-related signaling pathways (Cheng et al., 2020, 2021). Together, these results indicate that activation of D1-like receptors represents an important strategy for prevention and treatment of AD-like pathology.
Accumulating evidence over the past decade suggests that D2-like receptor agonists can prevent multiple pathological features found in AD. For instance, rotigotine, a DA2/3R agonist, could increase cortical excitability and restore central cholinergic transmission in patients with AD (Martorana et al., 2013) and reduce symptoms associated with frontal lobe cognitive dysfunction, thereby delaying impairment of activities of daily living (Koch et al., 2020). However, distinct ligands may allow D2-like receptors to exert a dual role in pathobiological activity of Aβ and tau. In vitro, both L-DOPA and piribedil promoted the generation of Aβ and increased the activity of γ-secretase, mediated by the activation of the DA2R and β2-arrestin signaling pathway in neuronal cells (Lu et al., 2017), whereas pretreatment with higher concentrations of DA2R agonist quinpirole protected neurons from Aβ toxicity (Melief et al., 2015). In addition, Koppel et al. (2016) reported that a tau mouse model of AD treated with DA2R antagonist haloperidol showed a significant reduction in tau phosphorylation associated with an inactivation of the tau kinase AMPK, whereas a study by Ziu et al. (2020) suggested an effective role of DA2/3R agonists in inhibiting tau aggregation. The underlying mechanism of this dual effect requires further verification.
Physical Exercise, Dopaminergic System, and Alzheimer’s Disease
The effects of exercise on the dopaminergic system in PD patients and animal models has been widely reported (Hou et al., 2017; Sacheli et al., 2019). The dopaminergic system is also known to be involved in the effects of exercise on AD. Studies have shown that exercise can increase the contents of DA in the hippocampus. Using a microdialysis technique, Goekint et al. (2012) found that a bout of treadmill running for 60 min induced a twofold increase in hippocampal DA release in rats. In agreement with this, 30 min of treadmill running at 60–70% VO2max rather than strength exercise ameliorated Aβ neurotoxicity by increasing hippocampal DA levels and promoted recognition learning in Aβ-induced rats (Rossi Dare et al., 2020). A chronic exercise intervention program consisting of 4 weeks of treadmill running (60 min/session, 5 sessions/week) before intraperitoneal LPS injection prevented LPS-induced loss of dopaminergic neurons in the SNpc, reduction in DA levels, and dysfunction of motor coordination (Wu et al., 2011). Furthermore, after swimming exercise for 4 weeks (30 min/session, 7 sessions/week), increases in the DA contents of the brain were found to be associated with improvements in learning and memory in AD rats induced by LPS, with the best effects for combined vitamin D and exercise treatment (Medhat et al., 2020). Thus, exercise appears to markedly improve LPS-induced cognitive and motor deficits by rescuing dysfunction of the dopaminergic system.
D1-like receptors are important participants in the improvements in cognition that occur under exercise stimuli. One study reported promotion of the persistence of object recognition memory and induction of the release of DA in the hippocampus as a result of 30 min of treadmill running at an intensity of 60–70% VO2max, whereas this effect was blocked by treatment with D1-like receptor antagonist SCH-23390 (Vargas et al., 2020). A recent study by Ramires Lima et al. (2021) identified specific mechanisms and found that a similar protocol activated D1-like receptors in rat hippocampus and improved memory persistence; however, the administration of SCH-23390 or inhibition of PKA but not PKC impaired the effect of acute aerobic exercise on memory persistence. In another study, 1 month of voluntary wheel running was shown to activate the DA1R/cAMP/PKA pathway, induce differentiation of hippocampal neurons, and enhance neurogenesis via the AMPK/CREB pathway in mice (Zang et al., 2017). Thus, signaling events mediated by PKA are critical for exercise to improve cognition. However, the effects of exercise on cognition mediated by D1-like receptors need further validation in models of AD in the future.
In AD brains, expression of D2-like receptors has been shown to be reduced in the cortex, striatal, and hippocampus regions (Pizzolato et al., 1996; Kemppainen et al., 2003; Kumar and Patel, 2007). Although research has proven that regular exercise can increase DA2R levels and improve dopaminergic signaling (Bauer et al., 2020), the relationships among exercise, DA, D2-like receptors, and cognition are not understood in sufficient detail. Several human studies offer some insight. In a cross-sectional study, the intensity of habitual physical activity of elderly individuals was found to be positively correlated with episodic memory and the availability of DA2/3R in the striatum, but the frequency of physical activity was not related to the availability of DA2/3R (Kohncke et al., 2018). In another study, elderly participants underwent an aerobic exercise intervention for 6 months, which led to significant increases in DA contents and improvements in working memory, compared with an active control; unexpectedly, DA2R levels decreased with exercise, and there was no relationship between DA2R and working memory at baseline or following exercise (Jonasson et al., 2019). Thus, the regulation of cognitive function by exercise through D2-like receptors is complicated, and it is still difficult to determine whether D2-like receptors are involved in the improvements in cognitive function of AD patients that are associated with exercise.
Conclusion and Future Perspectives
Adverse changes in the cholinergic and monoaminergic systems of AD brains are mainly reflected in degeneration of cholinergic neurons and monoaminergic neurons; reductions in levels of neurotransmitters ACh, NE, 5-HT, and DA; and abnormalities of the activity of cholinergic receptors and monoaminergic receptors. Treatment with enzymes and proteins involved in the anabolism and catabolism of neurotransmitters and agents to target GPCRs can at least partially prevent multiple pathological features found in AD, including Aβ, tau, neurotoxicity, neuroinflammation, oxidative stress, synaptic dysfunction, and neuronal apoptosis. Further discussion of the relationship between neurotransmitters and distinct neurologic disorders including tauopathies would be very valuable to broaden the physiological functions of neurotransmitters and to explore therapeutic strategies. Traditional pharmacological therapies have failed to show long-term efficacy, and the specificity and possible side-effects of a pharmacological agent are always a concern. Fortunately, exercise therapy has significant promise as a highly efficacious, low-toxicity, and cost-effective therapy that can replace drugs to improve the function of the cholinergic and monoaminergic systems and enhance the cognitive performance of AD patients (Table 5 and Figure 2). Moreover, the effects of exercise combined with drug interventions are better than those of exercise interventions alone (Abhijit et al., 2017; Medhat et al., 2020; Shamsipour et al., 2021). However, in many exercise intervention studies, rodent models were selected as research subjects rather than humans, and the results reported in animals may not reflect what occurs in humans. In human intervention studies, combining biochemical and neuroimaging methods such as MRI, magnetic resonance spectroscopy, or positron emission tomography could provide fruitful avenues for research. Notably, the application of physical exercise-based interventions in humans has several limitations. Although such interventions are beneficial, patients with moderate-to-advanced disease usually experience limitations in their capacity for physical activity. For these patients, safety is a primary concern during the course of the exercise intervention; the elements of the intervention, including exercise type, intensity, frequency, and duration, need to be strictly controlled, which may require the supervision and guidance of a substantial number of specialized health care workers.
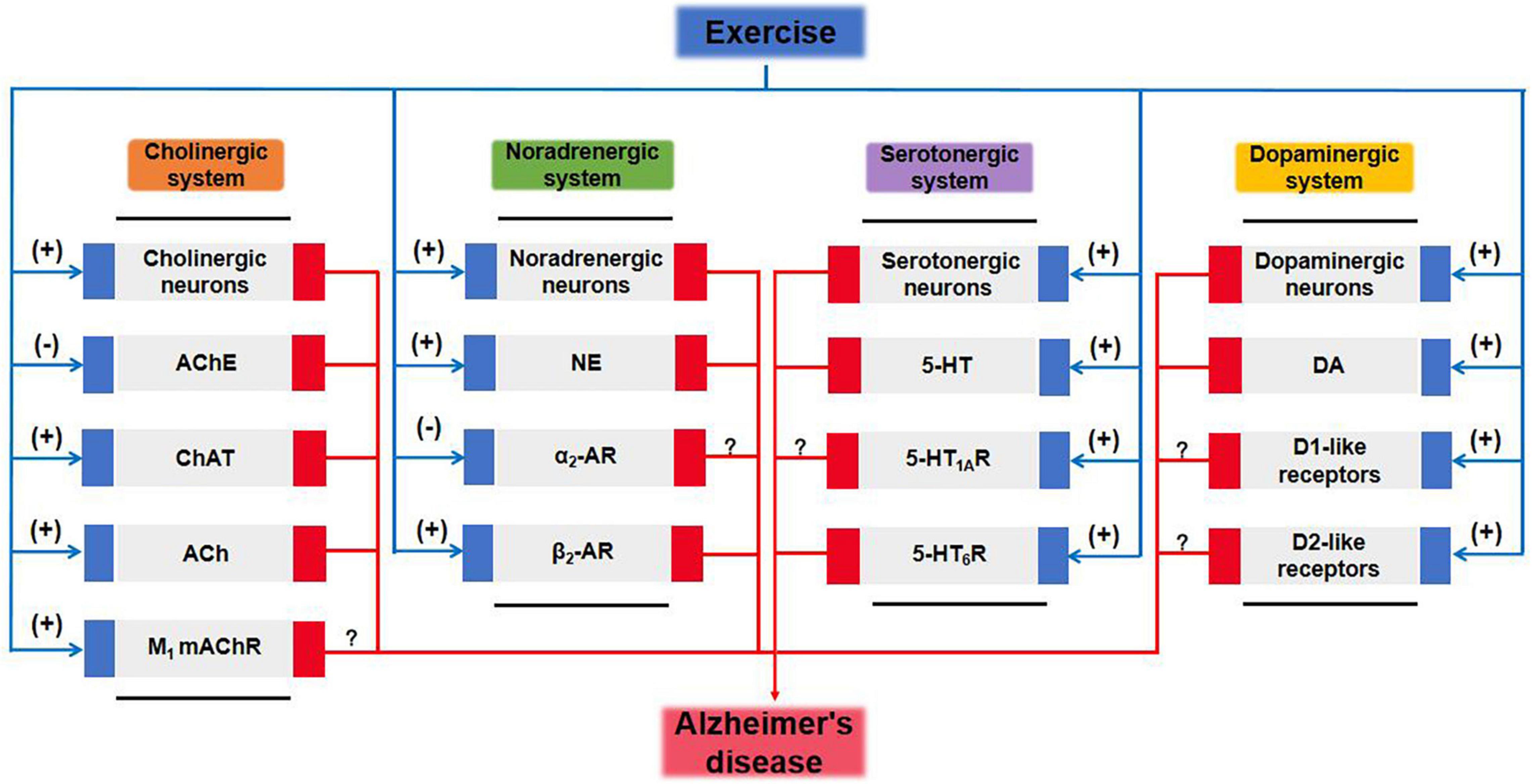
Figure 2. Cholinergic and monoaminergic systems mediate potential pathways for exercise amelioration in Alzheimer’s disease. 5-HT, 5-hydroxytryptamine; 5-HTR, 5-hydroxytryptamine receptor; ACh, acetylcholine; AChE, acetylcholinesterase; AR, adrenergic receptor; ChAT, choline acetyltransferase; DA, dopamine; mAChR, muscarinic acetylcholine receptor; MAOA, monoamine oxidase A; NE, norepinephrine.
Author Contributions
BZ prepared the first draft and final version of the manuscript. FY designed the diagrams and wrote the draft. XZ and WZ involved in literature searching. LL, SL, and PS critically edited and revised the manuscript. All authors contributed to the article and approved the submitted version.
Conflict of Interest
The authors declare that the research was conducted in the absence of any commercial or financial relationships that could be construed as a potential conflict of interest.
Publisher’s Note
All claims expressed in this article are solely those of the authors and do not necessarily represent those of their affiliated organizations, or those of the publisher, the editors and the reviewers. Any product that may be evaluated in this article, or claim that may be made by its manufacturer, is not guaranteed or endorsed by the publisher.
References
Abdel Rasheed, N. O., El Sayed, N. S., and El-Khatib, A. S. (2018). Targeting central beta2 receptors ameliorates streptozotocin-induced neuroinflammation via inhibition of glycogen synthase kinase3 pathway in mice. Prog. Neuropsychopharmacol. Biol. Psychiatry 86, 65–75. doi: 10.1016/j.pnpbp.2018.05.010
Abd-Elrahman, K. S., Sarasija, S., Colson, T. L., and Ferguson, S. S. G. (2021). A M1 muscarinic acetylcholine receptor positive allosteric modulator improves pathology and cognitive deficits in female APPswe/PSEN1DeltaE9 mice. Br. J. Pharmacol. 179, 1769–1783. doi: 10.1111/bph.15750
Abhijit, S., Subramanyam, M. V. V., and Devi, S. A. (2017). Grape Seed Proanthocyanidin and Swimming Exercise Protects Against Cognitive Decline: A Study on M1 Acetylcholine Receptors in Aging Male Rat Brain. Neurochem. Res. 42, 3573–3586. doi: 10.1007/s11064-017-2406-6
Afshar, S., Shahidi, S., Rohani, A. H., Komaki, A., and Asl, S. S. (2018). The effect of NAD-299 and TCB-2 on learning and memory, hippocampal BDNF levels and amyloid plaques in Streptozotocin-induced memory deficits in male rats. Psychopharmacology 235, 2809–2822. doi: 10.1007/s00213-018-4973-x
Afshar, S., Shahidi, S., Rohani, A. H., Soleimani Asl, S., and Komaki, A. (2019). Protective effects of 5-HT1A receptor antagonist and 5-HT2A receptor agonist on the biochemical and histological features in a rat model of Alzheimer’s disease. J. Chem. Neuroanat. 96, 140–147. doi: 10.1016/j.jchemneu.2019.01.008
Akbari-Fakhrabadi, M., Najafi, M., Mortazavian, S., Memari, A. H., Shidfar, F., Shahbazi, A., et al. (2021). Saffron (Crocus Sativus L.), Combined with Endurance Exercise, Synergistically Enhances BDNF, Serotonin, and NT-3 in Wistar Rats. Rep. Biochem. Mol. Biol. 9, 426–434. doi: 10.52547/rbmb.9.4.426
Akterin, S., Cowburn, R. F., Miranda-Vizuete, A., Jimenez, A., Bogdanovic, N., Winblad, B., et al. (2006). Involvement of glutaredoxin-1 and thioredoxin-1 in beta-amyloid toxicity and Alzheimer’s disease. Cell Death Differ. 13, 1454–1465. doi: 10.1038/sj.cdd.4401818
Albert, P. R., and Vahid-Ansari, F. (2019). The 5-HT1A receptor: Signaling to behavior. Biochimie 161, 34–45. doi: 10.1016/j.biochi.2018.10.015
Aletrino, M. A., Vogels, O. J., Van Domburg, P. H., and Ten Donkelaar, H. J. (1992). Cell loss in the nucleus raphes dorsalis in Alzheimer’s disease. Neurobiol. Aging 13, 461–468. doi: 10.1016/0197-458090073-7
Angulo, E., Casado, V., Mallol, J., Canela, E. I., Vinals, F., Ferrer, I., et al. (2003). A1 adenosine receptors accumulate in neurodegenerative structures in Alzheimer disease and mediate both amyloid precursor protein processing and tau phosphorylation and translocation. Brain Pathol. 13, 440–451. doi: 10.1111/j.1750-3639.2003.tb00475.x
Ardestani, P. M., Evans, A. K., Yi, B., Nguyen, T., Coutellier, L., and Shamloo, M. (2017). Modulation of neuroinflammation and pathology in the 5XFAD mouse model of Alzheimer’s disease using a biased and selective beta-1 adrenergic receptor partial agonist. Neuropharmacology 116, 371–386. doi: 10.1016/j.neuropharm.2017.01.010
Arjona, A. A., Pooler, A. M., Lee, R. K., and Wurtman, R. J. (2002). Effect of a 5-HT(2C) serotonin agonist, dexnorfenfluramine, on amyloid precursor protein metabolism in guinea pigs. Brain Res. 951, 135–140. doi: 10.1016/s0006-899303153-0
Atukeren, P., Cengiz, M., Yavuzer, H., Gelisgen, R., Altunoglu, E., Oner, S., et al. (2017). The efficacy of donepezil administration on acetylcholinesterase activity and altered redox homeostasis in Alzheimer’s disease. Biomed. Pharmacother. 90, 786–795. doi: 10.1016/j.biopha.2017.03.101
Bai, L., Zhang, S., Zhou, X., Li, Y., and Bai, J. (2019). Brain-derived neurotrophic factor induces thioredoxin-1 expression through TrkB/Akt/CREB pathway in SH-SY5Y cells. Biochimie 160, 55–60. doi: 10.1016/j.biochi.2019.02.011
Bailey, J. A., and Lahiri, D. K. (2012). Chromatographic separation of reaction products from the choline acetyltransferase and carnitine acetyltransferase assay: differential ChAT and CrAT activity in brain extracts from Alzheimer’s disease versus controls. J. Neurochem. 122, 672–680. doi: 10.1111/j.1471-4159.2012.07793.x
Baker-Nigh, A., Vahedi, S., Davis, E. G., Weintraub, S., Bigio, E. H., Klein, W. L., et al. (2015). Neuronal amyloid-beta accumulation within cholinergic basal forebrain in ageing and Alzheimer’s disease. Brain 138, 1722–1737. doi: 10.1093/brain/awv024
Baranger, K., Giannoni, P., Girard, S. D., Girot, S., Gaven, F., Stephan, D., et al. (2017). Chronic treatments with a 5-HT4 receptor agonist decrease amyloid pathology in the entorhinal cortex and learning and memory deficits in the 5xFAD mouse model of Alzheimer’s disease. Neuropharmacology 126, 128–141. doi: 10.1016/j.neuropharm.2017.08.031
Bartus, R. T., Dean, R. L. III, Beer, B., and Lippa, A. S. (1982). The cholinergic hypothesis of geriatric memory dysfunction. Science 217, 408–414. doi: 10.1126/science.7046051
Bauer, E. E., Buhr, T. J., Reed, C. H., and Clark, P. J. (2020). Exercise-Induced Adaptations to the Mouse Striatal Adenosine System. Neural. Plast. 2020:5859098. doi: 10.1155/2020/5859098
Beaulieu, J. M., and Gainetdinov, R. R. (2011). The physiology, signaling, and pharmacology of dopamine receptors. Pharmacol. Rev. 63, 182–217. doi: 10.1124/pr.110.002642
Belarbi, K., Burnouf, S., Fernandez-Gomez, F. J., Laurent, C., Lestavel, S., Figeac, M., et al. (2011). Beneficial effects of exercise in a transgenic mouse model of Alzheimer’s disease-like Tau pathology. Neurobiol. Dis. 43, 486–494. doi: 10.1016/j.nbd.2011.04.022
Bellucci, A., Luccarini, I., Scali, C., Prosperi, C., Giovannini, M. G., Pepeu, G., et al. (2006). Cholinergic dysfunction, neuronal damage and axonal loss in TgCRND8 mice. Neurobiol. Dis. 23, 260–272. doi: 10.1016/j.nbd.2006.03.012
Berizzi, A. E., Bender, A. M., Lindsley, C. W., Conn, P. J., Sexton, P. M., Langmead, C. J., et al. (2018). Structure-Activity Relationships of Pan-Galphaq/11 Coupled Muscarinic Acetylcholine Receptor Positive Allosteric Modulators. ACS Chem. Neurosci. 9, 1818–1828. doi: 10.1021/acschemneuro.8b00136
Betts, M. J., Kirilina, E., Otaduy, M. C. G., Ivanov, D., Acosta-Cabronero, J., Callaghan, M. F., et al. (2019). Locus coeruleus imaging as a biomarker for noradrenergic dysfunction in neurodegenerative diseases. Brain 142, 2558–2571. doi: 10.1093/brain/awz193
Bjorklund, A., and Dunnett, S. B. (2007). Dopamine neuron systems in the brain: an update. Trends Neurosci. 30, 194–202. doi: 10.1016/j.tins.2007.03.006
Blin, J., Baron, J. C., Dubois, B., Crouzel, C., Fiorelli, M., Attar-Levy, D., et al. (1993). Loss of brain 5-HT2 receptors in Alzheimer’s disease. In vivo assessment with positron emission tomography and [18F]setoperone. Brain 116, 497–510. doi: 10.1093/brain/116.3.497
Bokare, A. M., Praveenkumar, A. K., Bhonde, M., Nayak, Y., Pal, R., and Goel, R. (2017). 5-HT6 Receptor Agonist and Antagonist Against beta-Amyloid-Peptide-Induced Neurotoxicity in PC-12 Cells. Neurochem. Res. 42, 1571–1579. doi: 10.1007/s11064-017-2217-9
Bowen, D. M., Smith, C. B., White, P., and Davison, A. N. (1976). Neurotransmitter-related enzymes and indices of hypoxia in senile dementia and other abiotrophies. Brain 99, 459–496. doi: 10.1093/brain/99.3.459
Branca, C., Wisely, E. V., Hartman, L. K., Caccamo, A., and Oddo, S. (2014). Administration of a selective beta2 adrenergic receptor antagonist exacerbates neuropathology and cognitive deficits in a mouse model of Alzheimer’s disease. Neurobio.l Aging 35, 2726–2735. doi: 10.1016/j.neurobiolaging.2014.06.011
Broadhouse, K. M., Singh, M. F., Suo, C., Gates, N., Wen, W., and Brodaty, H. (2020). Hippocampal plasticity underpins long-term cognitive gains from resistance exercise in MCI. Neuroimage. Clin. 25:102182. doi: 10.1016/j.nicl.2020.102182
Brown, A. J. H., Bradley, S. J., Marshall, F. H., Brown, G. A., Bennett, K. A., Brown, J., et al. (2021). From structure to clinic: Design of a muscarinic M1 receptor agonist with potential to treatment of Alzheimer’s disease. Cell 184, 5886–5901.e22. doi: 10.1016/j.cell.2021.11.001
Brown, B. M., Peiffer, J., and Rainey-Smith, S. R. (2019). Exploring the relationship between physical activity, beta-amyloid and tau: A narrative review. Ageing Res. Rev. 50, 9–18. doi: 10.1016/j.arr.2019.01.003
Brown, B. M., Sohrabi, H. R., Taddei, K., Gardener, S. L., Rainey-Smith, S. R., Peiffer, J. J., et al. (2017). Habitual exercise levels are associated with cerebral amyloid load in presymptomatic autosomal dominant Alzheimer’s disease. Alzheimers Dement. 13, 1197–1206. doi: 10.1016/j.jalz.2017.03.008
Buxbaum, J. D., Oishi, M., Chen, H. I., Pinkas-Kramarski, R., Jaffe, E. A., Gandy, S. E., et al. (1992). Cholinergic agonists and interleukin 1 regulate processing and secretion of the Alzheimer beta/A4 amyloid protein precursor. Proc. Natl. Acad. Sci. U S A. 89, 10075–10078. doi: 10.1073/pnas.89.21.10075
Caccamo, A., Oddo, S., Billings, L. M., Green, K. N., Martinez-Coria, H., Fisher, A., et al. (2006). M1 receptors play a central role in modulating AD-like pathology in transgenic mice. Neuron 49, 671–682. doi: 10.1016/j.neuron.2006.01.020
Cachard-Chastel, M., Lezoualc’h, F., Dewachter, I., Delomenie, C., Croes, S., Devijver, H., et al. (2007). 5-HT4 receptor agonists increase sAPPalpha levels in the cortex and hippocampus of male C57BL/6j mice. Br. J. Pharmacol. 150, 883–892. doi: 10.1038/sj.bjp.0707178
Cao, S., Fisher, D. W., Rodriguez, G., Yu, T., and Dong, H. (2021). Comparisons of neuroinflammation, microglial activation, and degeneration of the locus coeruleus-norepinephrine system in APP/PS1 and aging mice. J. Neuroinflammation 18:10. doi: 10.1186/s12974-020-02054-2
Chai, G. S., Wang, Y. Y., Yasheng, A., and Zhao, P. (2016). Beta 2-adrenergic receptor activation enhances neurogenesis in Alzheimer’s disease mice. Neural. Regen. Res. 11, 1617–1624. doi: 10.4103/1673-5374.193241
Chai, G. S., Wang, Y. Y., Zhu, D., Yasheng, A., and Zhao, P. (2017). Activation of beta2-adrenergic receptor promotes dendrite ramification and spine generation in APP/PS1 mice. Neurosci. Lett. 636, 158–164. doi: 10.1016/j.neulet.2016.11.022
Chalermpalanupap, T., Schroeder, J. P., Rorabaugh, J. M., Liles, L. C., Lah, J. J., Levey, A. I., et al. (2018). Locus Coeruleus Ablation Exacerbates Cognitive Deficits, Neuropathology, and Lethality in P301S Tau Transgenic Mice. J. Neurosci. 38, 74–92. doi: 10.1523/JNEUROSCI.1483-17.2017
Chen, Y. J., Li, X. H., Zhan, C. D., Lao, Z. H., Li, F. Y., Dong, X. W., et al. (2021). A Comprehensive Insight into the Mechanisms of Dopamine in Disrupting A beta Protofibrils and Inhibiting A beta Aggregation. ACS Chem. Neurosci. 12, 4007–4019. doi: 10.1021/acschemneuro.1c00306
Chen, Y. J., Peng, Y., Che, P. L., Gannon, M., Liu, Y., Li, L., et al. (2014). alpha(2A) adrenergic receptor promotes amyloidogenesis through disrupting APP-SorLA interaction. Proc. Natl. Acad. Sci. U S A. 111, 17296–17301. doi: 10.1073/pnas.1409513111
Chen, Z. J., and Minneman, K. P. (2005). Recent progress in alpha1-adrenergic receptor research. Acta Pharmacol. Sin. 26, 1281–1287. doi: 10.1111/j.1745-7254.2005.00224.x
Cheng, S., Li, L., He, S., Liu, J., Sun, Y., He, M., et al. (2010). GRK5 deficiency accelerates {beta}-amyloid accumulation in Tg2576 mice via impaired cholinergic activity. J. Biol. Chem. 285, 41541–41548. doi: 10.1074/jbc.M110.170894
Cheng, Z. Y., Hu, Y. H., Xia, Q. P., Wang, C., and He, L. (2021). DRD1 agonist A-68930 improves mitochondrial dysfunction and cognitive deficits in a streptozotocin-induced mouse model. Brain Res. Bull. 175, 136–149. doi: 10.1016/j.brainresbull.2021.07.015
Cheng, Z. Y., Xia, Q. P., Hu, Y. H., Wang, C., and He, L. (2020). Dopamine D1 receptor agonist A-68930 ameliorates Abeta1-42-induced cognitive impairment and neuroinflammation in mice. Int. Immunopharmacol. 88:106963. doi: 10.1016/j.intimp.2020.106963
Cho, S., and Hu, Y. (2007). Activation of 5-HT4 receptors inhibits secretion of beta-amyloid peptides and increases neuronal survival. Exp. Neurol. 203, 274–278. doi: 10.1016/j.expneurol.2006.07.021
Choi, S. H., Bylykbashi, E., Chatila, Z. K., Lee, S. W., Pulli, B., Clemenson, G. D., et al. (2018). Combined adult neurogenesis and BDNF mimic exercise effects on cognition in an Alzheimer’s mouse model. Science 361:eaan8821. doi: 10.1126/science.aan8821
Chong, J. R., Ashton, N. J., Karikari, T. K., Tanaka, T., Scholl, M., Zetterberg, H., et al. (2021). Blood-based high sensitivity measurements of beta-amyloid and phosphorylated tau as biomarkers of Alzheimer’s disease: a focused review on recent advances. J. Neurol. Neurosurg. Psychiatry 92, 1231–1241. doi: 10.1136/jnnp-2021-327370
Ciampa, C. J., Parent, J. H., Lapoint, M. R., Swinnerton, K. N., Taylor, M. M., Tennant, V. R., et al. (2021). Elevated Dopamine Synthesis as a Mechanism of Cognitive Resilience in Aging. Cerebral Cortex doi: 10.1093/cercor/bhab379 [Epub ahead of print].
Cisse, M., Braun, U., Leitges, M., Fisher, A., Pages, G., Checler, F., et al. (2011). ERK1-independent alpha-secretase cut of beta-amyloid precursor protein via M1 muscarinic receptors and PKCalpha/epsilon. Mol. Cell Neurosci. 47, 223–232. doi: 10.1016/j.mcn.2011.04.008
Coelho, B. P., Gaelzer, M. M., Dos Santos Petry, F., Hoppe, J. B., Trindade, V. M. T., Salbego, C. G., et al. (2019). Dual Effect of Doxazosin: Anticancer Activity on SH-SY5Y Neuroblastoma Cells and Neuroprotection on an In Vitro Model of Alzheimer’s Disease. Neuroscience 404, 314–325. doi: 10.1016/j.neuroscience.2019.02.005
Collaborators, G. D. (2019). Global, regional, and national burden of Alzheimer’s disease and other dementias, 1990-2016: a systematic analysis for the Global Burden of Disease Study 2016. Lancet Neurol. 18, 88–106. doi: 10.1016/S1474-442230403-4
Congdon, E. E., and Sigurdsson, E. M. (2018). Tau-targeting therapies for Alzheimer disease. Nat. Rev. Neurol. 14, 399–415. doi: 10.1038/s41582-018-0013-z
Conley, J. M., and Watts, V. J. (2013). Differential effects of AGS3 expression on D(2L) dopamine receptor-mediated adenylyl cyclase signaling. Cell Mol. Neurobiol. 33, 551–558. doi: 10.1007/s10571-013-9925-8
Cordella, A., Krashia, P., Nobili, A., Pignataro, A., La Barbera, L., Viscomi, M. T., et al. (2018). Dopamine loss alters the hippocampus-nucleus accumbens synaptic transmission in the Tg2576 mouse model of Alzheimer’s disease. Neurobiol. Dis. 116, 142–154. doi: 10.1016/j.nbd.2018.05.006
Counts, S. E., and Mufson, E. J. (2010). Noradrenaline activation of neurotrophic pathways protects against neuronal amyloid toxicity. J. Neurochem. 113, 649–660. doi: 10.1111/j.1471-4159.2010.06622.x
Coutellier, L., Ardestani, P. M., and Shamloo, M. (2014). beta1-adrenergic receptor activation enhances memory in Alzheimer’s disease model. Ann. Clin. Transl. Neurol. 1, 348–360. doi: 10.1002/acn3.57
Coyle, J. T., Price, D. L., and DeLong, M. R. (1983). Alzheimer’s disease: a disorder of cortical cholinergic innervation. Science 219, 1184–1190. doi: 10.1126/science.6338589
D’Amelio, M., Puglisi-Allegra, S., and Mercuri, N. (2018). The role of dopaminergic midbrain in Alzheimer’s disease: Translating basic science into clinical practice. Pharmacol. Res. 130, 414–419. doi: 10.1016/j.phrs.2018.01.016
Dare, L. R., Garcia, A., Soares, C. B., Lopes, L., Neves, B. S., Dias, D. V., et al. (2020). The Reversal of Memory Deficits in an Alzheimer’s Disease Model Using Physical and Cognitive Exercise. Front. Behav. Neurosci. 14:152. doi: 10.3389/fnbeh.2020.00152
Davies, P., and Maloney, A. J. (1976). Selective loss of central cholinergic neurons in Alzheimer’s disease. Lancet 2, 1403. doi: 10.1016/s0140-673691936-x
Davis, A. A., Fritz, J. J., Wess, J., Lah, J. J., and Levey, A. I. (2010). Deletion of M1 muscarinic acetylcholine receptors increases amyloid pathology in vitro and in vivo. J. Neurosci. 30, 4190–4196. doi: 10.1523/JNEUROSCI.6393-09.2010
Day, G. S., Scarmeas, N., Dubinsky, R., Coerver, K., Mostacero, A., West, B., et al. (2022). Aducanumab Use in Symptomatic Alzheimer Disease Evidence in Focus: Report of the AAN Guidelines Subcommittee. Neurology doi: 10.1212/wnl.0000000000200176 [Epub ahead of print].
de Farias, J. M., Dos Santos Tramontin, N., Pereira, E. V., de Moraes, G. L., Furtado, B. G., Tietbohl, L. T. W., et al. (2021). Physical Exercise Training Improves Judgment and Problem-Solving and Modulates Serum Biomarkers in Patients with Alzheimer’s Disease. Mol. Neurobiol. 58, 4217–4225. doi: 10.1007/s12035-021-02411-z
De Souza, C. G., Michelini, L. C., and Fior-Chadi, D. R. (2001). Receptor changes in the nucleus tractus solitarii of the rat after exercise training. Med. Sci. Sports Exerc. 33, 1471–1476. doi: 10.1097/00005768-200109000-00008
De Strooper, B. (2010). Proteases and proteolysis in Alzheimer disease: a multifactorial view on the disease process. Physiol. Rev. 90, 465–494. doi: 10.1152/physrev.00023.2009
Dey, S., Singh, R. H., and Dey, P. K. (1992). Exercise training: significance of regional alterations in serotonin metabolism of rat brain in relation to antidepressant effect of exercise. Physiol. Behav. 52, 1095–1099. doi: 10.1016/0031-938490465-e
Dhillon, S. (2021). Aducanumab: First Approval. Drugs 81, 1437–1443. doi: 10.1007/s40265-021-01569-z
Dobarro, M., Orejana, L., Aguirre, N., and Ramirez, M. J. (2013a). Propranolol reduces cognitive deficits, amyloid beta levels, tau phosphorylation and insulin resistance in response to chronic corticosterone administration. Int. J. Neuropsychopharmacol. 16, 1351–1360. doi: 10.1017/S1461145712001393
Dobarro, M., Orejana, L., Aguirre, N., and Ramirez, M. J. (2013b). Propranolol restores cognitive deficits and improves amyloid and Tau pathologies in a senescence-accelerated mouse model. Neuropharmacology 64, 137–144. doi: 10.1016/j.neuropharm.2012.06.047
Dunn, A. L., Reigle, T. G., Youngstedt, S. D., Armstrong, R. B., and Dishman, R. K. (1996). Brain norepinephrine and metabolites after treadmill training and wheel running in rats. Med. Sci. Sports Exerc. 28, 204–209. doi: 10.1097/00005768-199602000-00008
Ebrahimi, S., Rashidy-Pour, A., Vafaei, A. A., and Akhavan, M. M. (2010). Central beta-adrenergic receptors play an important role in the enhancing effect of voluntary exercise on learning and memory in rat. Behav. Brain Res. 208, 189–193. doi: 10.1016/j.bbr.2009.11.032
Ehrhardt, S., Porsteinsson, A. P., Munro, C. A., Rosenberg, P. B., Pollock, B. G., Devanand, D. P., et al. (2019). Escitalopram for agitation in Alzheimer’s disease (S-CitAD): Methods and design of an investigator-initiated, randomized, controlled, multicenter clinical trial. Alzheimers Dement. 15, 1427–1436. doi: 10.1016/j.jalz.2019.06.4946
El Hayek, L., Khalifeh, M., Zibara, V., Assaad, R. A., Emmanuel, N., Karnib, N., et al. (2019). Lactate Mediates the Effects of Exercise on Learning and Memory through SIRT1-Dependent Activation of Hippocampal Brain-Derived Neurotrophic Factor (BDNF). J. Neurosci. 39, 2369–2382. doi: 10.1523/Jneurosci.1661-18.2019
Farias, G. G., Godoy, J. A., Hernandez, F., Avila, J., Fisher, A., and Inestrosa, N. C. (2004). M1 muscarinic receptor activation protects neurons from beta-amyloid toxicity. A role for Wnt signaling pathway. Neurobiol. Dis. 17, 337–348. doi: 10.1016/j.nbd.2004.07.016
Farzi, M. A., Sadigh-Eteghad, S., Ebrahimi, K., and Talebi, M. (2019). Exercise Improves Recognition Memory and Acetylcholinesterase Activity in the Beta Amyloid-Induced Rat Model of Alzheimer’s Disease. Ann. Neurosci. 25, 121–125. doi: 10.1159/000488580
Fernandez-Cabello, S., Kronbichler, M., Van Dijk, K. R. A., Goodman, J. A., Spreng, R. N., Schmitz, T. W., et al. (2020). Basal forebrain volume reliably predicts the cortical spread of Alzheimer’s degeneration. Brain 143, 993–1009. doi: 10.1093/brain/awaa012
Ferreira-Vieira, T. H., Guimaraes, I. M., Silva, F. R., and Ribeiro, F. M. (2016). Alzheimer’s disease: Targeting the Cholinergic System. Curr. Neuropharmacol. 14, 101–115. doi: 10.2174/1570159x13666150716165726
Fisher, A., Bezprozvanny, I., Wu, L., Ryskamp, D. A., Bar-Ner, N., Natan, N., et al. (2016). AF710B, a Novel M1/sigma1 Agonist with Therapeutic Efficacy in Animal Models of Alzheimer’s Disease. Neurodegener. Dis. 16, 95–110. doi: 10.1159/000440864
Fisher, A., Brandeis, R., Karton, I., Pittel, Z., Gurwitz, D., Haring, R., et al. (1991). (+-)-cis-2-methyl-spiro(1,3-oxathiolane-5,3’)quinuclidine, an M1 selective cholinergic agonist, attenuates cognitive dysfunctions in an animal model of Alzheimer’s disease. J. Pharmacol. Exp. Ther. 257, 392–403.
Fisher, J. R., Wallace, C. E., Tripoli, D. L., Sheline, Y. I., and Cirrito, J. R. (2016). Redundant Gs-coupled serotonin receptors regulate amyloid-beta metabolism in vivo. Mol. Neurodegener. 11:45. doi: 10.1186/s13024-016-0112-5
Forlenza, O. V., Spink, J. M., Dayanandan, R., Anderton, B. H., Olesen, O. F., and Lovestone, S. (2000). Muscarinic agonists reduce tau phosphorylation in non-neuronal cells via GSK-3beta inhibition and in neurons. J. Neural. Transm. 107, 1201–1212. doi: 10.1007/s007020070034
Francis, B. M., Yang, J., Hajderi, E., Brown, M. E., Michalski, B., McLaurin, J., et al. (2012). Reduced tissue levels of noradrenaline are associated with behavioral phenotypes of the TgCRND8 mouse model of Alzheimer’s disease. Neuropsychopharmacology 37, 1934–1944. doi: 10.1038/npp.2012.40
Francken, B. J., Josson, K., Lijnen, P., Jurzak, M., Luyten, W. H., and Leysen, J. E. (2000). Human 5-hydroxytryptamine(5A) receptors activate coexpressed G(i) and G(o) proteins in Spodoptera frugiperda 9 cells. Mol. Pharmacol. 57, 1034–1044.
Gao, W. L., Li, X. H., Dun, X. P., Jing, X. K., Yang, K., and Li, Y. K. (2020). Grape Seed Proanthocyanidin Extract Ameliorates Streptozotocin-induced Cognitive and Synaptic Plasticity Deficits by Inhibiting Oxidative Stress and Preserving AKT and ERK Activities. Curr. Med. Sci. 40, 434–443. doi: 10.1007/s11596-020-2197-x
Garcia, C., Chen, M. J., Garza, A. A., Cotman, C. W., and Russo-Neustadt, A. (2003). The influence of specific noradrenergic and serotonergic lesions on the expression of hippocampal brain-derived neurotrophic factor transcripts following voluntary physical activity. Neuroscience 119, 721–732. doi: 10.1016/s0306-452200192-1
Garwain, O., Yerramilli, V. S., Romero, K., and Scarlata, S. (2020). The Galphaq/phospholipase Cbeta signaling system represses tau aggregation. Cell Signal. 71:109620. doi: 10.1016/j.cellsig.2020.109620
Gasbarri, A., and Pompili, A. (2014). Serotonergic 5-HT7 receptors and cognition. Rev. Neurosci. 25, 311–323. doi: 10.1515/revneuro-2013-0066
Gasiorowska, A., Wydrych, M., Drapich, P., Zadrozny, M., Steczkowska, M., Niewiadomski, W., et al. (2021). The Biology and Pathobiology of Glutamatergic, Cholinergic, and Dopaminergic Signaling in the Aging Brain. Front. Aging Neurosci. 13:654931. doi: 10.3389/fnagi.2021.654931
Ge, R., and Dai, Y. (2020). Three-Week Treadmill Exercise Enhances Persistent Inward Currents, Facilitates Dendritic Plasticity, and Upregulates the Excitability of Dorsal Raphe Serotonin Neurons in ePet-EYFP Mice. Front. Cell Neurosci. 14:575626. doi: 10.3389/fncel.2020.575626
Geda, Y. E., Schneider, L. S., Gitlin, L. N., Miller, D. S., Smith, G. S., Bell, J., et al. (2013). Neuropsychiatric symptoms in Alzheimer’s disease: past progress and anticipation of the future. Alzheimers Dement. 9, 602–608. doi: 10.1016/j.jalz.2012.12.001
Genis, I., Fisher, A., and Michaelson, D. M. (1999). Site-specific dephosphorylation of tau of apolipoprotein E-deficient and control mice by M1 muscarinic agonist treatment. J. Neurochem. 72, 206–213. doi: 10.1046/j.1471-4159.1999.0720206.x
Giannoni, P., Gaven, F., de Bundel, D., Baranger, K., Marchetti-Gauthier, E., Roman, F. S., et al. (2013). Early administration of RS 67333, a specific 5-HT4 receptor agonist, prevents amyloidogenesis and behavioral deficits in the 5XFAD mouse model of Alzheimer’s disease. Front. Aging Neurosci. 5:96. doi: 10.3389/fnagi.2013.00096
Gibbs, M. E., Maksel, D., Gibbs, Z., Hou, X., Summers, R. J., and Small, D. H. (2010). Memory loss caused by beta-amyloid protein is rescued by a beta-adrenoceptor agonist. Neurobiol. Aging 31, 614–624. doi: 10.1016/j.neurobiolaging.2008.05.018
Goekint, M., Bos, I., Heyman, E., Meeusen, R., Michotte, Y., and Sarre, S. (2012). Acute running stimulates hippocampal dopaminergic neurotransmission in rats, but has no influence on brain-derived neurotrophic factor. J. Appl. Physiol. 112, 535–541. doi: 10.1152/japplphysiol.00306.2011
Gonzalez, S., McHugh, T. L. M., Yang, T., Syriani, W., Massa, S. M., Longo, F. M., et al. (2021). Small molecule modulation of TrkB and TrkC neurotrophin receptors prevents cholinergic neuron atrophy in an Alzheimer’s disease mouse model at an advanced pathological stage. Neurobiol. Dis. 162:105563. doi: 10.1016/j.nbd.2021.105563
Goodman, A. M., Langner, B. M., Jackson, N., Alex, C., and McMahon, L. L. (2021). Heightened Hippocampal beta-Adrenergic Receptor Function Drives Synaptic Potentiation and Supports Learning and Memory in the TgF344-AD Rat Model during Prodromal Alzheimer’s Disease. J. Neurosci. 41, 5747–5761. doi: 10.1523/JNEUROSCI.0119-21.2021
Goto, S., Hirano, A., and Matsumoto, S. (1990). Immunohistochemical study of the striatal efferents and nigral dopaminergic neurons in parkinsonism-dementia complex on Guam in comparison with those in Parkinson’s and Alzheimer’s diseases. Ann. Neurol. 27, 520–527. doi: 10.1002/ana.410270511
Grimaldi, M., Marino, S. D., Florenzano, F., Ciotta, M. T., Nori, S. L., Rodriquez, M., et al. (2016). beta-Amyloid-acetylcholine molecular interaction: new role of cholinergic mediators in anti-Alzheimer therapy? Future Med. Chem. 8, 1179–1189. doi: 10.4155/fmc-2016-0006
Guo, Y., Zhang, C., Wang, C., Huang, Y., Liu, J., Chu, H., et al. (2021). Thioredoxin-1 Is a Target to Attenuate Alzheimer-Like Pathology in Diabetic Encephalopathy by Alleviating Endoplasmic Reticulum Stress and Oxidative Stress. Front. Physiol. 12:651105. doi: 10.3389/fphys.2021.651105
Haam, J., and Yakel, J. L. (2017). Cholinergic modulation of the hippocampal region and memory function. J. Neurochem. 142, 111–121. doi: 10.1111/jnc.14052
Hall, H., Iulita, M. F., Gubert, P., Flores Aguilar, L., Ducatenzeiler, A., Fisher, A., et al. (2018). AF710B, an M1/sigma-1 receptor agonist with long-lasting disease-modifying properties in a transgenic rat model of Alzheimer’s disease. Alzheimers Dement. 14, 811–823. doi: 10.1016/j.jalz.2017.11.009
Hall, J. M., and Savage, L. M. (2016). Exercise leads to the re-emergence of the cholinergic/nestin neuronal phenotype within the medial septum/diagonal band and subsequent rescue of both hippocampal ACh efflux and spatial behavior. Exp. Neurol. 278, 62–75. doi: 10.1016/j.expneurol.2016.01.018
Hall, J. M., Gomez-Pinilla, F., and Savage, L. M. (2018). Nerve Growth Factor Is Responsible for Exercise-Induced Recovery of Septohippocampal Cholinergic Structure and Function. Front. Neurosci. 12:773. doi: 10.3389/fnins.2018.00773
Hamm, V., Heraud, C., Bott, J. B., Herbeaux, K., Strittmatter, C., Mathis, C., et al. (2017). Differential contribution of APP metabolites to early cognitive deficits in a TgCRND8 mouse model of Alzheimer’s disease. Sci. Adv. 3:e1601068. doi: 10.1126/sciadv.1601068
Hampel, H., Mesulam, M. M., Cuello, A. C., Farlow, M. R., Giacobini, E., Grossberg, G. T., et al. (2018). The cholinergic system in the pathophysiology and treatment of Alzheimer’s disease. Brain 141, 1917–1933. doi: 10.1093/brain/awy132
Han, T. K., Leem, Y. H., and Kim, H. S. (2019). Treadmill exercise restores high fat diet-induced disturbance of hippocampal neurogenesis through beta2-adrenergic receptor-dependent induction of thioredoxin-1 and brain-derived neurotrophic factor. Brain Res. 1707, 154–163. doi: 10.1016/j.brainres.2018.11.035
Hao, J. R., Sun, N., Lei, L., Li, X. Y., Yao, B., Sun, K., et al. (2015). L-Stepholidine rescues memory deficit and synaptic plasticity in models of Alzheimer’s disease via activating dopamine D1 receptor/PKA signaling pathway. Cell Death Dis. 6:e1965. doi: 10.1038/cddis.2015.315
Hashemi-Firouzi, N., Komaki, A., Soleimani Asl, S., and Shahidi, S. (2017). The effects of the 5-HT7 receptor on hippocampal long-term potentiation and apoptosis in a rat model of Alzheimer’s disease. Brain Res. Bull. 135, 85–91. doi: 10.1016/j.brainresbull.2017.10.004
Hashemi-Firouzi, N., Shahidi, S., and Soleimani Asl, S. (2021). Chronic stimulation of the serotonergic 5-HT4 receptor modulates amyloid-beta-related impairments in synaptic plasticity and memory deficits in male rats. Brain Res. 1773, 147701. doi: 10.1016/j.brainres.2021.147701
Hashemi-Firouzi, N., Shahidi, S., Soleimani-Asl, S., and Komaki, A. (2018). 5-Hydroxytryptamine receptor 6 antagonist. Metab. Brain Dis. 33, 1243–1253. doi: 10.1007/s11011-018-0228-0
Hashimoto, G., Sakurai, M., Teich, A. F., Saeed, F., Aziz, F., and Arancio, O. (2012). 5-HT(4) receptor stimulation leads to soluble AbetaPPalpha production through MMP-9 upregulation. J. Alzheimers Dis. 32, 437–445. doi: 10.3233/JAD-2012-111235
Hassan, A., Robinson, M., and Willerth, S. M. (2018). Modeling the Effects of Yoga on the Progression of Alzheimer’s Disease in a Dish. Cells Tissues. Organs. 206, 263–271. doi: 10.1159/000499503
Hasselmo, M. E. (2006). The role of acetylcholine in learning and memory. Curr. Opin. Neurobiol. 16, 710–715. doi: 10.1016/j.conb.2006.09.002
Hauser, A. S., Attwood, M. M., Rask-Andersen, M., Schioth, H. B., and Gloriam, D. E. (2017). Trends in GPCR drug discovery: new agents, targets and indications. Nat. Rev. Drug. Discov. 16, 829–842. doi: 10.1038/nrd.2017.178
Heneka, M. T., Galea, E., Gavriluyk, V., Dumitrescu-Ozimek, L., Daeschner, J., O’Banion, M. K., et al. (2002). Noradrenergic depletion potentiates beta - amyloid-induced cortical inflammation: implications for Alzheimer’s disease. J. Neurosci. 22, 2434–2442.
Himmelreich, S., Masuho, I., Berry, J. A., MacMullen, C., Skamangas, N. K., Martemyanov, K. A., et al. (2017). Dopamine Receptor DAMB Signals via Gq to Mediate Forgetting in Drosophila. Cell Rep. 21, 2074–2081. doi: 10.1016/j.celrep.2017.10.108
Hock, C., Maddalena, A., Raschig, A., Muller-Spahn, F., Eschweiler, G., Hager, K., et al. (2003). Treatment with the selective muscarinic m1 agonist talsaclidine decreases cerebrospinal fluid levels of A beta 42 in patients with Alzheimer’s disease. Amyloid 10, 285–91. doi: 10.3109/13506120308995249
Holland, N., Robbins, T. W., and Rowe, J. B. (2021). The role of noradrenaline in cognition and cognitive disorders. Brain 144, 2243–2256. doi: 10.1093/brain/awab111
Holm, P., Ettrup, A., Klein, A. B., Santini, M. A., El-Sayed, M., Elvang, A. B., et al. (2010). Plaque deposition dependent decrease in 5-HT2A serotonin receptor in AbetaPPswe/PS1dE9 amyloid overexpressing mice. J. Alzheimers Dis. 20, 1201–1213. doi: 10.3233/JAD-2010-100117
Hong, Y. P., Lee, H. C., and Kim, H. T. (2015). Treadmill exercise after social isolation increases the levels of NGF, BDNF, and synapsin I to induce survival of neurons in the hippocampus, and improves depression-like behavior. J. Exerc. Nutr. Biochem. 19, 11–18. doi: 10.5717/jenb.2015.19.1.11
Hou, L., Chen, W., Liu, X., Qiao, D., and Zhou, F. M. (2017). Exercise-Induced Neuroprotection of the Nigrostriatal Dopamine System in Parkinson’s Disease. Front. Aging. Neurosci. 9:358. doi: 10.3389/fnagi.2017.00358
Hu, L., Wang, B., and Zhang, Y. (2017). Serotonin 5-HT6 receptors affect cognition in a mouse model of Alzheimer’s disease by regulating cilia function. Alzheimers Res. Ther. 9:76. doi: 10.1186/s13195-017-0304-4
Huang, H., Wang, L., Cao, M., Marshall, C., Gao, J., Xiao, N., et al. (2015). Isolation Housing Exacerbates Alzheimer’s Disease-Like Pathophysiology in Aged APP/PS1 Mice. Int. J. Neuropsychopharmacol. 18:pyu116. doi: 10.1093/ijnp/pyu116
Huey, E. D., Putnam, K. T., and Grafman, J. (2006). A systematic review of neurotransmitter deficits and treatments in frontotemporal dementia. Neurology 66, 17–22. doi: 10.1212/01.wnl.0000191304.55196.4d
Hung, A. Y., Haass, C., Nitsch, R. M., Qiu, W. Q., Citron, M., Wurtman, R. J., et al. (1993). Activation of protein kinase C inhibits cellular production of the amyloid beta-protein. J. Biol. Chem. 268, 22959–22962.
Huong, V. T., Shimanouchi, T., Shimauchi, N., Yagi, H., Umakoshi, H., Goto, Y., et al. (2010). Catechol derivatives inhibit the fibril formation of amyloid-beta peptides. J. Biosci. Bioeng. 109, 629–634. doi: 10.1016/j.jbiosc.2009.11.010
Islam, M. R., Valaris, S., Young, M. F., Haley, E. B., Luo, R. H., Bond, S. F., et al. (2021). Exercise hormone irisin is a critical regulator of cognitive function (vol 3, pg 1058, 2021). Nat. Metab. 3, 1432–1432. doi: 10.1038/s42255-021-00476-7
Ismail, Z., Creese, B., Aarsland, D., Kales, H. C., Lyketsos, C. G., Sweet, R. A., et al. (2022). Psychosis in Alzheimer disease - mechanisms, genetics and therapeutic opportunities. Nat. Rev. Neurol. 18, 131–144. doi: 10.1038/s41582-021-00597-3
Itou, Y., Nochi, R., Kuribayashi, H., Saito, Y., and Hisatsune, T. (2011). Cholinergic activation of hippocampal neural stem cells in aged dentate gyrus. Hippocampus 21, 446–459. doi: 10.1002/hipo.20761
Ivachtchenko, A. V., Lavrovsky, Y., and Ivanenkov, Y. A. (2016). AVN-211, Novel and Highly Selective 5-HT6 Receptor Small Molecule Antagonist, for the Treatment of Alzheimer’s Disease. Mol. Pharm. 13, 945–963. doi: 10.1021/acs.molpharmaceut.5b00830
Jack, C. R. Jr., Knopman, D. S., Jagust, W. J., Petersen, R. C., Weiner, M. W., Aisen, P. S., et al. (2013). Tracking pathophysiological processes in Alzheimer’s disease: an updated hypothetical model of dynamic biomarkers. Lancet Neurol. 12, 207–216. doi: 10.1016/S1474-4422(12)70291-0
Jacobs, H. I. L., Riphagen, J. M., Ramakers, I., and Verhey, F. R. J. (2021). Alzheimer’s disease pathology: pathways between central norepinephrine activity, memory, and neuropsychiatric symptoms. Mol. Psychiatry 26, 897–906. doi: 10.1038/s41380-019-0437-x
Jankowska, A., Wesolowska, A., Pawlowski, M., and Chlon-Rzepa, G. (2018). Multi-Target-Directed Ligands Affecting Serotonergic Neurotransmission for Alzheimer’s Disease Therapy: Advances in Chemical and Biological Research. Curr. Med. Chem. 25, 2045–2067. doi: 10.2174/0929867324666170529122802
Jardanhazi-Kurutz, D., Kummer, M. P., Terwel, D., Vogel, K., Thiele, A., and Heneka, M. T. (2011). Distinct adrenergic system changes and neuroinflammation in response to induced locus ceruleus degeneration in APP/PS1 transgenic mice. Neuroscience 176, 396–407. doi: 10.1016/j.neuroscience.2010.11.052
Jay, T. M. (2003). Dopamine: a potential substrate for synaptic plasticity and memory mechanisms. Prog. Neurobiol. 69, 375–390. doi: 10.1016/s0301-0082(03)00085-6
Jhang, K. A., Lee, E. O., Kim, H. S., and Chong, Y. H. (2014). Norepinephrine provides short-term neuroprotection against Abeta1-42 by reducing oxidative stress independent of Nrf2 activation. Neurobiol. Aging 35, 2465–2473. doi: 10.1016/j.neurobiolaging.2014.05.020
Jia, R. X., Liang, J. H., Xu, Y., and Wang, Y. Q. (2019). Effects of physical activity and exercise on the cognitive function of patients with Alzheimer disease: a meta-analysis. BMC Geriatr. 19:181. doi: 10.1186/s12877-019-1175-2
Jiangbo, N., and Liyun, Z. (2018). Effect of donepezil hydrochloride & aerobic exercise training on learning and memory and its mechanism of action in an Alzheimer’s disease rat model. Pak. J. Pharm. Sci. 31, 2897–2901.
Joe, E., and Ringman, J. M. (2019). Cognitive symptoms of Alzheimer’s disease: clinical management and prevention. BMJ 367:l6217. doi: 10.1136/bmj.l6217
Jonasson, L. S., Nyberg, L., Axelsson, J., Kramer, A. F., Riklund, K., and Boraxbekk, C. J. (2019). Higher striatal D2-receptor availability in aerobically fit older adults but non-selective intervention effects after aerobic versus resistance training. Neuroimage 202:116044. doi: 10.1016/j.neuroimage.2019.116044
Jones, C. K., Brady, A. E., Davis, A. A., Xiang, Z., Bubser, M., Tantawy, M. N., et al. (2008). Novel selective allosteric activator of the M1 muscarinic acetylcholine receptor regulates amyloid processing and produces antipsychotic-like activity in rats. J Neurosci. 28, 10422–10433. doi: 10.1523/JNEUROSCI.1850-08.2008
Jurgensen, S., Antonio, L. L., Mussi, G. E., Brito-Moreira, J., Bomfim, T. R., De Felice, F. G., et al. (2011). Activation of D1/D5 dopamine receptors protects neurons from synapse dysfunction induced by amyloid-beta oligomers. J. Biol. Chem. 286, 3270–3276. doi: 10.1074/jbc.M110.177790
Kalaria, R. N., Andorn, A. C., Tabaton, M., Whitehouse, P. J., Harik, S. I., and Unnerstall, J. R. (1989). Adrenergic receptors in aging and Alzheimer’s disease: increased beta 2-receptors in prefrontal cortex and hippocampus. J. Neurochem. 53, 1772–1781. doi: 10.1111/j.1471-4159.1989.tb09242.x
Kalinin, S., Gavrilyuk, V., Polak, P. E., Vasser, R., Zhao, J., Heneka, M. T., et al. (2007). Noradrenaline deficiency in brain increases beta-amyloid plaque burden in an animal model of Alzheimer’s disease. Neurobiol. Aging 28, 1206–1214. doi: 10.1016/j.neurobiolaging.2006.06.003
Katsouri, L., Vizcaychipi, M. P., McArthur, S., Harrison, I., Suarez-Calvet, M., Lleo, A., et al. (2013). Prazosin, an alpha(1)-adrenoceptor antagonist, prevents memory deterioration in the APP23 transgenic mouse model of Alzheimer’s disease. Neurobiol. Aging 34, 1105–1115. doi: 10.1016/j.neurobiolaging.2012.09.010
Ke, H. C., Huang, H. J., Liang, K. C., and Hsieh-Li, H. M. (2011). Selective improvement of cognitive function in adult and aged APP/PS1 transgenic mice by continuous non-shock treadmill exercise. Brain Res. 1403, 1–11. doi: 10.1016/j.brainres.2011.05.056
Kemppainen, N., Laine, M., Laakso, M. P., Kaasinen, V., Nagren, K., Vahlberg, T., et al. (2003). Hippocampal dopamine D2 receptors correlate with memory functions in Alzheimer’s disease. Eur. J. Neurosci. 18, 149–154. doi: 10.1046/j.1460-9568.2003.02716.x
Kim, M. H., and Leem, Y. H. (2019). Neurogenic effect of exercise via the thioredoxin-1/extracellular regulated kinase/beta-catenin signaling pathway mediated by beta2-adrenergic receptors in chronically stressed dentate gyrus. J. Exerc. Nutr. Biochem. 23, 13–21. doi: 10.20463/jenb.2019.0018
Kim, T. W., Lim, B. V., Kim, K., Seo, J. H., and Kim, C. J. (2015). Treadmill exercise alleviates stress-induced impairment of social interaction through 5-hydroxytryptamine 1A receptor activation in rats. J. Exerc. Rehabil. 11, 192–197. doi: 10.12965/jer.150225
King, M. V., Marsden, C. A., and Fone, K. C. (2008). A role for the 5-HT(1A), 5-HT4 and 5-HT6 receptors in learning and memory. Trends Pharmacol. Sci. 29, 482–492. doi: 10.1016/j.tips.2008.07.001
Klempin, F., Beis, D., Mosienko, V., Kempermann, G., Bader, M., and Alenina, N. (2013). Serotonin is required for exercise-induced adult hippocampal neurogenesis. J. Neurosci. 33, 8270–8275. doi: 10.1523/JNEUROSCI.5855-12.2013
Klempin, F., Mosienko, V., Matthes, S., Villela, D. C., Todiras, M., Penninger, J. M., et al. (2018). Depletion of angiotensin-converting enzyme 2 reduces brain serotonin and impairs the running-induced neurogenic response. Cell Mol. Life. Sci. 75, 3625–3634. doi: 10.1007/s00018-018-2815-y
Ko, H. J., Chiou, S. J., Wong, Y. H., Wang, Y. H., Lai, Y., Chou, C. H., et al. (2019). GSKIP-Mediated Anchoring Increases Phosphorylation of Tau by PKA but Not by GSK3beta via cAMP/PKA/GSKIP/GSK3/Tau Axis Signaling in Cerebrospinal Fluid and iPS Cells in Alzheimer Disease. J. Clin. Med. 8:1751. doi: 10.3390/jcm8101751
Koch, G., Motta, C., Bonni, S., Pellicciari, M. C., Picazio, S., Casula, E. P., et al. (2020). Effect of Rotigotine vs Placebo on Cognitive Functions Among Patients With Mild to Moderate Alzheimer Disease: A Randomized Clinical Trial. JAMA Netw. Open 3:e2010372. doi: 10.1001/jamanetworkopen.2020.10372
Kohncke, Y., Papenberg, G., Jonasson, L., Karalija, N., Wahlin, A., Salami, A., et al. (2018). Self-rated intensity of habitual physical activities is positively associated with dopamine D2/3 receptor availability and cognition. Neuroimage 181, 605–616. doi: 10.1016/j.neuroimage.2018.07.036
Kong, Y., Ruan, L., Qian, L., Liu, X., and Le, Y. (2010). Norepinephrine promotes microglia to uptake and degrade amyloid beta peptide through upregulation of mouse formyl peptide receptor 2 and induction of insulin-degrading enzyme. J. Neurosci. 30, 11848–11857. doi: 10.1523/JNEUROSCI.2985-10.2010
Koppel, J., Jimenez, H., Adrien, L., Greenwald, B. S., Marambaud, P., Cinamon, E., et al. (2016). Haloperidol inactivates AMPK and reduces tau phosphorylation in a tau mouse model of Alzheimer’s disease. Alzheimers Dement. 2, 121–130. doi: 10.1016/j.trci.2016.05.003
Kumar, A., Prakash, A., and Dogra, S. (2011). Neuroprotective effect of carvedilol against aluminium induced toxicity: possible behavioral and biochemical alterations in rats. Pharmacol. Rep. 63, 915–923. doi: 10.1016/s1734-1140(11)70607-7
Kumar, U., and Patel, S. C. (2007). Immunohistochemical localization of dopamine receptor subtypes (D1R-D5R) in Alzheimer’s disease brain. Brain Res. 1131, 187–196. doi: 10.1016/j.brainres.2006.10.049
Kurosawa, M., Okada, K., Sato, A., and Uchida, S. (1993). Extracellular release of acetylcholine, noradrenaline and serotonin increases in the cerebral cortex during walking in conscious rats. Neurosci. Lett. 161, 73–76. doi: 10.1016/0304-3940(93)90143-9
Labus, J., Rohrs, K. F., Ackmann, J., Varbanov, H., Muller, F. E., Jia, S., et al. (2021). Amelioration of Tau pathology and memory deficits by targeting 5-HT7 receptor. Prog. Neurobiol. 197:101900. doi: 10.1016/j.pneurobio.2020.101900
Lachowicz, J. E., Duffy, R. A., Ruperto, V., Kozlowski, J., Zhou, G., Clader, J., et al. (2001). Facilitation of acetylcholine release and improvement in cognition by a selective M2 muscarinic antagonist, SCH 72788. Life Sci. 68, 2585–2592. doi: 10.1016/s0024-3205(01)01056-6
Lai, M. K., Tsang, S. W., Alder, J. T., Keene, J., Hope, T., Esiri, M. M., et al. (2005). Loss of serotonin 5-HT2A receptors in the postmortem temporal cortex correlates with rate of cognitive decline in Alzheimer’s disease. Psychopharmacology 179, 673–677. doi: 10.1007/s00213-004-2077-2
Lanctot, K. L., Herrmann, N., Eryavec, G., van Reekum, R., Reed, K., and Naranjo, C. A. (2002). Central serotonergic activity is related to the aggressive behaviors of Alzheimer’s disease. Neuropsychopharmacology 27, 646–654. doi: 10.1016/s0893-133x(02)00339-1
Lebel, M., Patenaude, C., Allyson, J., Massicotte, G., and Cyr, M. (2009). Dopamine D1 receptor activation induces tau phosphorylation via cdk5 and GSK3 signaling pathways. Neuropharmacology 57, 392–402. doi: 10.1016/j.neuropharm.2009.06.041
Lebois, E. P., Schroeder, J. P., Esparza, T. J., Bridges, T. M., Lindsley, C. W., Conn, P. J., et al. (2017). Disease-Modifying Effects of M1 Muscarinic Acetylcholine Receptor Activation in an Alzheimer’s Disease Mouse Model. ACS Chem. Neurosci. 8, 1177–1187. doi: 10.1021/acschemneuro.6b00278
Lee, Y., Warne, T., Nehme, R., Pandey, S., Dwivedi-Agnihotri, H., Chaturvedi, M., et al. (2020). Molecular basis of beta-arrestin coupling to formoterol-bound beta(1)-adrenoceptor. Nature 583:862. doi: 10.1038/s41586-020-2419-1
Li, X., Wang, Q., Hu, T., Wang, Y., Zhao, J., Lu, J., et al. (2017). A tricyclic antidepressant, amoxapine, reduces amyloid-beta generation through multiple serotonin receptor 6-mediated targets. Sci. Rep. 7:4983. doi: 10.1038/s41598-017-04144-3
Li, Z., Jia, K., Duan, Y., Wang, D., Zhou, Z., and Dong, S. (2017). Xanomeline derivative EUK1001 attenuates Alzheimer’s disease pathology in a triple transgenic mouse model. Mol. Med. Rep. 16, 7835–7840. doi: 10.3892/mmr.2017.7502
Liu, J., and Wang, M. (2018). Carvedilol protection against endogenous Abeta-induced neurotoxicity in N2a cells. Cell Stress Chaperones 23, 695–702. doi: 10.1007/s12192-018-0881-6
Liu, J., Rasul, I., Sun, Y., Wu, G., Li, L., Premont, R. T., et al. (2009). GRK5 deficiency leads to reduced hippocampal acetylcholine level via impaired presynaptic M2/M4 autoreceptor desensitization. J. Biol. Chem. 284, 19564–19571. doi: 10.1074/jbc.M109.005959
Liu, J., Tao, J., Xia, R., Li, M., Huang, M., Li, S., et al. (2021). Mind-Body Exercise Modulates Locus Coeruleus and Ventral Tegmental Area Functional Connectivity in Individuals With Mild Cognitive Impairment. Front. Aging Neurosci. 13:646807. doi: 10.3389/fnagi.2021.646807
Liu, M., Kou, L., Bin, Y., Wan, L., and Xiang, J. (2016). Complicated function of dopamine in Abeta-related neurotoxicity: Dual interactions with Tyr(10) and SNK(26-28) of Abeta. J. Inorg. Biochem. 164, 119–128. doi: 10.1016/j.jinorgbio.2016.09.007
Liu, M., Wan, L., Bin, Y., and Xiang, J. (2017). Role of norepinephrine in Abeta-related neurotoxicity: dual interactions with Tyr10 and SNK(26-28) of Abeta. Acta Biochim. Biophys. Sin. 49, 170–178. doi: 10.1093/abbs/gmw126
Liu, X., Xu, X., Hilger, D., Aschauer, P., Tiemann, J. K. S., Du, Y., et al. (2019). Structural Insights into the Process of GPCR-G Protein Complex Formation. Cell 177, 1243–1251e1212. doi: 10.1016/j.cell.2019.04.021
Liu, X., Ye, K., and Weinshenker, D. (2015). Norepinephrine Protects against Amyloid-beta Toxicity via TrkB. J. Alzheimers. Dis. 44, 251–260. doi: 10.3233/JAD-141062
Liu, X., Zhao, X., Zeng, X., Bossers, K., Swaab, D. F., Zhao, J., et al. (2013). beta-arrestin1 regulates gamma-secretase complex assembly and modulates amyloid-beta pathology. Cell Res. 23, 351–365. doi: 10.1038/cr.2012.167
Long, J. M., and Holtzman, D. M. (2019). Alzheimer Disease: An Update on Pathobiology and Treatment Strategies. Cell 179, 312–339. doi: 10.1016/j.cell.2019.09.001
Lourenco, M. V., Frozza, R. L., de Freitas, G. B., Zhang, H., Kincheski, G. C., Ribeiro, F. C., et al. (2019). Exercise-linked FNDC5/irisin rescues synaptic plasticity and memory defects in Alzheimer’s models. Nat. Med. 25, 165–175. doi: 10.1038/s41591-018-0275-4
Lu, J., Li, X., Wang, Q., and Pei, G. (2017). Dopamine D2 receptor and beta-arrestin 2 mediate Amyloid-beta elevation induced by anti-parkinson’s disease drugs, levodopa and piribedil, in neuronal cells. PLoS One 12:e0173240. doi: 10.1371/journal.pone.0173240
Lu, J., Zhang, C., Lv, J., Zhu, X., Jiang, X., Lu, W., et al. (2021). Antiallergic drug desloratadine as a selective antagonist of 5HT2A receptor ameliorates pathology of Alzheimer’s disease model mice by improving microglial dysfunction. Aging Cell 20:e13286. doi: 10.1111/acel.13286
Lyness, S. A., Zarow, C., and Chui, H. C. (2003). Neuron loss in key cholinergic and aminergic nuclei in Alzheimer disease: a meta-analysis. Neurobiol. Aging 24, 1–23. doi: 10.1016/s0197-4580(02)00057-x
Ma, Q. (2008). Beneficial effects of moderate voluntary physical exercise and its biological mechanisms on brain health. Neurosci. Bull. 24, 265–270. doi: 10.1007/s12264-008-0402-1
Machado, A., Ferreira, D., Grothe, M. J., Eyjolfsdottir, H., Almqvist, P. M., Cavallin, L., et al. (2020). The cholinergic system in subtypes of Alzheimer’s disease: an in vivo longitudinal MRI study. Alzheimers Res. Ther. 12:51. doi: 10.1186/s13195-020-00620-7
Maillet, M., Robert, S. J., Cacquevel, M., Gastineau, M., Vivien, D., Bertoglio, J., et al. (2003). Crosstalk between Rap1 and Rac regulates secretion of sAPPalpha. Nat. Cell Biol. 5, 633–639. doi: 10.1038/ncb1007
Mann, D. M., Yates, P. O., and Marcyniuk, B. (1987). Dopaminergic neurotransmitter systems in Alzheimer’s disease and in Down’s syndrome at middle age. J. Neurol. Neurosurg. Psychiatry 50, 341–344. doi: 10.1136/jnnp.50.3.341
Marner, L., Frokjaer, V. G., Kalbitzer, J., Lehel, S., Madsen, K., Baare, W. F., et al. (2012). Loss of serotonin 2A receptors exceeds loss of serotonergic projections in early Alzheimer’s disease: a combined [11C]DASB and [18F]altanserin-PET study. Neurobiol. Aging 33, 479–487. doi: 10.1016/j.neurobiolaging.2010.03.023
Martorana, A., and Koch, G. (2014). Is dopamine involved in Alzheimer’s disease? Front. Aging Neurosci. 6:252. doi: 10.3389/fnagi.2014.00252
Martorana, A., Di Lorenzo, F., Esposito, Z., Lo Giudice, T., Bernardi, G., Caltagirone, C., et al. (2013). Dopamine D(2)-agonist rotigotine effects on cortical excitability and central cholinergic transmission in Alzheimer’s disease patients. Neuropharmacology 64, 108–113. doi: 10.1016/j.neuropharm.2012.07.015
Maruyama, Y., Nishida, M., Sugimoto, Y., Tanabe, S., Turner, J. H., Kozasa, T., et al. (2002). Galpha(12/13) mediates alpha(1)-adrenergic receptor-induced cardiac hypertrophy. Circ. Res. 91, 961–969. doi: 10.1161/01.res.0000043282.39776.7c
Matchett, B. J., Grinberg, L. T., Theofilas, P., and Murray, M. E. (2021). The mechanistic link between selective vulnerability of the locus coeruleus and neurodegeneration in Alzheimer’s disease. Acta Neuropathol. 141, 631–650. doi: 10.1007/s00401-020-02248-1
Mather, M., and Harley, C. W. (2016). The Locus Coeruleus: Essential for Maintaining Cognitive Function and the Aging Brain. Trends Cogn. Sci. 20, 214–226. doi: 10.1016/j.tics.2016.01.001
Medeiros, R., Kitazawa, M., Caccamo, A., Baglietto-Vargas, D., Estrada-Hernandez, T., Cribbs, D. H., et al. (2011). Loss of muscarinic M1 receptor exacerbates Alzheimer’s disease-like pathology and cognitive decline. Am. J. Pathol. 179, 980–991. doi: 10.1016/j.ajpath.2011.04.041
Medhat, E., Rashed, L., Abdelgwad, M., Aboulhoda, B. E., Khalifa, M. M., and El-Din, S. S. (2020). Exercise enhances the effectiveness of vitamin D therapy in rats with Alzheimer’s disease: emphasis on oxidative stress and inflammation. Metab. Brain Dis. 35, 111–120. doi: 10.1007/s11011-019-00504-2
Melancon, M. O., Lorrain, D., and Dionne, I. J. (2012). Exercise increases tryptophan availability to the brain in older men age 57-70 years. Med. Sci. Sports Exerc. 44, 881–887. doi: 10.1249/MSS.0b013e31823ede8e
Melief, E. J., Cudaback, E., Jorstad, N. L., Sherfield, E., Postupna, N., Wilson, A., et al. (2015). Partial depletion of striatal dopamine enhances penetrance of cognitive deficits in a transgenic mouse model of Alzheimer’s disease. J. Neurosci. Res. 93, 1413–1422. doi: 10.1002/jnr.23592
Melkonyan, M. M., Hunanyan, L., Lourhmati, A., Layer, N., Beer-Hammer, S., Yenkoyan, K., et al. (2017). Neuroprotective, Neurogenic, and Amyloid Beta Reducing Effect of a Novel Alpha 2-Adrenoblocker, Mesedin, on Astroglia and Neuronal Progenitors upon Hypoxia and Glutamate Exposure. Int. J. Mol. Sci. 19:9. doi: 10.3390/ijms19010009
Moreno-Castilla, P., Rodriguez-Duran, L. F., Guzman-Ramos, K., Barcenas-Femat, A., Escobar, M. L., and Bermudez-Rattoni, F. (2016). Dopaminergic neurotransmission dysfunction induced by amyloid-beta transforms cortical long-term potentiation into long-term depression and produces memory impairment. Neurobiol. Aging 41, 187–199. doi: 10.1016/j.neurobiolaging.2016.02.021
Morgese, M. G., and Trabace, L. (2019). Monoaminergic System Modulation in Depression and Alzheimer’s Disease: A New Standpoint? Front. Pharmacol. 10:483. doi: 10.3389/fphar.2019.00483
Morishima, M., Harada, N., Hara, S., Sano, A., Seno, H., Takahashi, A., et al. (2006). Monoamine oxidase A activity and norepinephrine level in hippocampus determine hyperwheel running in SPORTS rats. Neuropsychopharmacology 31, 2627–2638. doi: 10.1038/sj.npp.1301028
Mravec, B., Lejavova, K., Vargovic, P., Ondicova, K., Horvathova, L., Novak, P., et al. (2016). Tauopathy in transgenic (SHR72) rats impairs function of central noradrenergic system and promotes neuroinflammation. J. Neuroinflammation 13:15. doi: 10.1186/s12974-016-0482-1
Mu, L., Cai, J., Gu, B., Yu, L., Li, C., Liu, Q. S., et al. (2022). Treadmill Exercise Prevents Decline in Spatial Learning and Memory in 3xTg-AD Mice through Enhancement of Structural Synaptic Plasticity of the Hippocampus and Prefrontal Cortex. Cells 11:244. doi: 10.3390/cells11020244
Mufson, E. J., Kelley, C., and Perez, S. E. (2021). Chronic traumatic encephalopathy and the nucleus basalis of Meynert. Handb. Clin. Neurol. 182, 9–29. doi: 10.1016/B978-0-12-819973-2.00002-2
Muller, S., Preische, O., Sohrabi, H. R., Graber, S., Jucker, M., Ringman, J. M., et al. (2018). Relationship between physical activity, cognition, and Alzheimer pathology in autosomal dominant Alzheimer’s disease. Alzheimers Dement. 14, 1427–1437. doi: 10.1016/j.jalz.2018.06.3059
Mulugeta, E., Karlsson, E., Islam, A., Kalaria, R., Mangat, H., Winblad, B., et al. (2003). Loss of muscarinic M4 receptors in hippocampus of Alzheimer patients. Brain Res. 960, 259–262. doi: 10.1016/s0006-8993(02)03542-4
Murley, A. G., and Rowe, J. B. (2018). Neurotransmitter deficits from frontotemporal lobar degeneration. Brain 141, 1263–1285. doi: 10.1093/brain/awx327
Nakano, I., and Hirano, A. (1983). Neuron loss in the nucleus basalis of Meynert in parkinsonism-dementia complex of Guam. Ann. Neurol. 13, 87–91. doi: 10.1002/ana.410130118
Nam, E., Derrick, J. S., Lee, S., Kang, J., Han, J., Lee, S. J. C., et al. (2018). Regulatory Activities of Dopamine and Its Derivatives toward Metal-Free and Metal-Induced Amyloid-beta Aggregation, Oxidative Stress, and Inflammation in Alzheimer’s Disease. ACS Chem. Neurosci. 9, 2655–2666. doi: 10.1021/acschemneuro.8b00122
Naomi, R., Embong, H., Othman, F., Ghazi, H. F., Maruthey, N., and Bahari, H. (2021). Probiotics for Alzheimer’s Disease: A Systematic Review. Nutrients 14:20. doi: 10.3390/nu14010020
Ni, Y. X., Zhao, X. H., Bao, G. B., Zou, L., Teng, L., Wang, Z., et al. (2006). Activation of beta(2)-adrenergic receptor stimulates gamma-secretase activity and accelerates amyloid plaque formation. Nat. Med. 12, 1390–1396. doi: 10.1038/nm1485
Nicoll, R. A., Malenka, R. C., and Kauer, J. A. (1990). Functional comparison of neurotransmitter receptor subtypes in mammalian central nervous system. Physiol. Rev. 70, 513–565. doi: 10.1152/physrev.1990.70.2.513
Nirogi, R., Mohammed, A. R., Shinde, A. K., Gagginapally, S. R., Kancharla, D. M., Ravella, S. R., et al. (2021). Discovery and Preclinical Characterization of Usmarapride (SUVN-D4010): A Potent. J. Med. Chem. 64, 10641–10665. doi: 10.1021/acs.jmedchem.1c00703
Nitsch, R. M., Deng, M., Growdon, J. H., and Wurtman, R. J. (1996). Serotonin 5-HT2a and 5-HT2c receptors stimulate amyloid precursor protein ectodomain secretion. J. Biol. Chem. 271, 4188–4194. doi: 10.1074/jbc.271.8.4188
Nitsch, R. M., Deng, M., Tennis, M., Schoenfeld, D., and Growdon, J. H. (2000). The selective muscarinic M1 agonist AF102B decreases levels of total Abeta in cerebrospinal fluid of patients with Alzheimer’s disease. Ann. Neurol. 48, 913–918.
Nitsch, R. M., Slack, B. E., Wurtman, R. J., and Growdon, J. H. (1992). Release of Alzheimer amyloid precursor derivatives stimulated by activation of muscarinic acetylcholine receptors. Science 258, 304–307. doi: 10.1126/science.1411529
No authors listed (2021). 2021 Alzheimer’s disease facts and figures. Alzheimers Dement. 17, 327–406. doi: 10.1002/alz.12328
Nobili, A., Latagliata, E. C., Viscomi, M. T., Cavallucci, V., Cutuli, D., Giacovazzo, G., et al. (2017). Dopamine neuronal loss contributes to memory and reward dysfunction in a model of Alzheimer’s disease. Nat. Commun. 8:14727. doi: 10.1038/ncomms14727
Northey, J. M., Cherbuin, N., Pumpa, K. L., Smee, D. J., and Rattray, B. (2018). Exercise interventions for cognitive function in adults older than 50: a systematic review with meta-analysis. Br. J. Sports Med. 52, 154–160. doi: 10.1136/bjsports-2016-096587
Odagaki, Y., Kinoshita, M., Ota, T., Javier Meana, J., Callado, L. F., and Garcia-Sevilla, J. A. (2017). Functional activation of Galphaq coupled to 5-HT2A receptor and M1 muscarinic acetylcholine receptor in postmortem human cortical membranes. J. Neural. Transm. 124, 1123–1133. doi: 10.1007/s00702-017-1749-0
Ogren, S. O., Eriksson, T. M., Elvander-Tottie, E., D’Addario, C., Ekstrom, J. C., Svenningsson, P., et al. (2008). The role of 5-HT(1A) receptors in learning and memory. Behav. Brain. Res. 195, 54–77. doi: 10.1016/j.bbr.2008.02.023
Ohiwa, N., Saito, T., Chang, H., Omori, T., Fujikawa, T., Asada, T., et al. (2006). Activation of A1 and A2 noradrenergic neurons in response to running in the rat. Neurosci. Lett. 395, 46–50. doi: 10.1016/j.neulet.2005.10.053
Ostman, I., and Nyback, H. (1976). Adaptive changes in central and peripheral noradrenergic neurons in rats following chronic exercise. Neuroscience 1, 41–47. doi: 10.1016/0306-4522(76)90046-4
Otsuka, T., Nishii, A., Amemiya, S., Kubota, N., Nishijima, T., and Kita, I. (2016). Effects of acute treadmill running at different intensities on activities of serotonin and corticotropin-releasing factor neurons, and anxiety- and depressive-like behaviors in rats. Behav. Brain Res. 298, 44–51. doi: 10.1016/j.bbr.2015.10.055
Pagliari, R., and Peyrin, L. (1995a). Norepinephrine release in the rat frontal cortex under treadmill exercise: a study with microdialysis. J. Appl. Physiol. 78, 2121–2130. doi: 10.1152/jappl.1995.78.6.2121
Pagliari, R., and Peyrin, L. (1995b). Physical conditioning in rats influences the central and peripheral catecholamine responses to sustained exercise. Eur. J. Appl. Physiol. Occup. Physiol. 71, 41–52. doi: 10.1007/BF00511231
Palmer, A. M., Francis, P. T., Benton, J. S., Sims, N. R., Mann, D. M., Neary, D., et al. (1987). Presynaptic serotonergic dysfunction in patients with Alzheimer’s disease. J. Neurochem. 48, 8–15. doi: 10.1111/j.1471-4159.1987.tb13120.x
Pan, X., Kaminga, A. C., Wen, S. W., Wu, X., Acheampong, K., and Liu, A. (2019). Dopamine and Dopamine Receptors in Alzheimer’s Disease: A Systematic Review and Network Meta-Analysis. Front. Aging Neurosci. 11:175. doi: 10.3389/fnagi.2019.00175
Park, S. S., Park, H. S., Kim, T. W., and Lee, S. J. (2020). Effects of swimming exercise on social isolation-induced memory impairment and apoptosis in old rats. J. Exerc. Rehabil. 16, 234–241. doi: 10.12965/jer.2040366.183
Patterson, C. (2020). “World Alzheimer report 2018. The state of the art of dementia research: new frontiers,” in An Analysis of Prevalence, Incidence, Cost and Trends, ed. C. Patterson (London: Alzheimer’s Disease International), 1–48.
Peterman, J. L., White, J. D., Calcagno, A., Hagen, C., Quiring, M., Paulhus, K., et al. (2020). Prolonged isolation stress accelerates the onset of Alzheimer’s disease-related pathology in 5xFAD mice despite running wheels and environmental enrichment. Behav. Brain Res. 379:112366. doi: 10.1016/j.bbr.2019.112366
Pietrelli, A., Matkovic, L., Vacotto, M., Lopez-Costa, J. J., Basso, N., and Brusco, A. (2018). Aerobic exercise upregulates the BDNF-Serotonin systems and improves the cognitive function in rats. Neurobiol. Learn. Mem. 155, 528–542. doi: 10.1016/j.nlm.2018.05.007
Pisani, S., Mueller, C., Huntley, J., Aarsland, D., and Kempton, M. J. (2021). A meta-analysis of randomised controlled trials of physical activity in people with Alzheimer’s disease and mild cognitive impairment with a comparison to donepezil. Int. J. Geriatr. Psychiatry 36, 1471–1487. doi: 10.1002/gps.5581
Pizzolato, G., Chierichetti, F., Fabbri, M., Cagnin, A., Dam, M., Ferlin, G., et al. (1996). Reduced striatal dopamine receptors in Alzheimer’s disease: single photon emission tomography study with the D2 tracer [123I]-IBZM. Neurology 47, 1065–1068. doi: 10.1212/wnl.47.4.1065
Polverino, A., Grimaldi, M., Sorrentino, P., Jacini, F., D’Ursi, A. M., and Sorrentino, G. (2018). Effects of Acetylcholine on beta-Amyloid-Induced cPLA2 Activation in the TB Neuroectodermal Cell Line: Implications for the Pathogenesis of Alzheimer’s Disease. Cell Mol. Neurobiol. 38, 817–826. doi: 10.1007/s10571-017-0555-4
Porsteinsson, A. P., Drye, L. T., Pollock, B. G., Devanand, D. P., Frangakis, C., Ismail, Z., et al. (2014). Effect of citalopram on agitation in Alzheimer disease: the CitAD randomized clinical trial. JAMA 311, 682–691. doi: 10.1001/jama.2014.93
Poulin, B., Butcher, A., McWilliams, P., Bourgognon, J. M., Pawlak, R., Kong, K. C., et al. (2010). The M3-muscarinic receptor regulates learning and memory in a receptor phosphorylation/arrestin-dependent manner. Proc. Natl. Acad. Sci. U S A. 107, 9440–9445. doi: 10.1073/pnas.0914801107
Prokselj, T., Jerin, A., Muck-Seler, D., and Kogoj, A. (2014). Decreased platelet serotonin concentration in Alzheimer’s disease with involuntary emotional expression disorder. Neurosci. Lett. 578, 71–74. doi: 10.1016/j.neulet.2014.06.034
Puri, V., Wang, X., Vardigan, J. D., Kuduk, S. D., and Uslaner, J. M. (2015). The selective positive allosteric M1 muscarinic receptor modulator PQCA attenuates learning and memory deficits in the Tg2576 Alzheimer’s disease mouse model. Behav. Brain Res. 287, 96–99. doi: 10.1016/j.bbr.2015.03.029
Qiu, Y., Wu, X. J., and Chen, H. Z. (2003). Simultaneous changes in secretory amyloid precursor protein and beta-amyloid peptide release from rat hippocampus by activation of muscarinic receptors. Neurosci. Lett. 352, 41–44. doi: 10.1016/j.neulet.2003.08.022
Quiedeville, A., Boulouard, M., Hamidouche, K., Da Silva Costa-Aze, V., Nee, G., Rochais, C., et al. (2015). Chronic activation of 5-HT4 receptors or blockade of 5-HT6 receptors improve memory performances. Behav. Brain Res. 293, 10–17. doi: 10.1016/j.bbr.2015.07.020
Ramires Lima, K., de Souza da Rosa, A. C., Severo Picua, S., Souza, E. S. S., Marks Soares, N., and Billig Mello-Carpes, P. (2021). One single physical exercise session improves memory persistence by hippocampal activation of D1 dopamine receptors and PKA signaling in rats. Brain Res. 1762:147439. doi: 10.1016/j.brainres.2021.147439
Ramis, M. R., Sarubbo, F., Moranta, D., Tejada, S., Llado, J., Miralles, A., et al. (2021). Neurochemical and Cognitive Beneficial Effects of Moderate Physical Activity and Catechin in Aged Rats. Antioxidants 10:621. doi: 10.3390/antiox10040621
Reinikainen, K. J., Paljarvi, L., Halonen, T., Malminen, O., Kosma, V. M., Laakso, M., et al. (1988). Dopaminergic system and monoamine oxidase-B activity in Alzheimer’s disease. Neurobiol. Aging 9, 245–252. doi: 10.1016/s0197-4580(88)80061-7
Reynolds, J. L., Ignatowski, T. A., and Spengler, R. N. (2005). Effect of tumor necrosis factor-alpha on the reciprocal G-protein-induced regulation of norepinephrine release by the alpha2-adrenergic receptor. J Neurosci. Res. 79, 779–787. doi: 10.1002/jnr.20407
Richter, N., Beckers, N., Onur, O. A., Dietlein, M., Tittgemeyer, M., Kracht, L., et al. (2018). Effect of cholinergic treatment depends on cholinergic integrity in early Alzheimer’s disease. Brain 141, 903–915. doi: 10.1093/brain/awx356
Robert, S. J., Zugaza, J. L., Fischmeister, R., Gardier, A. M., and Lezoualc’h, F. (2001). The human serotonin 5-HT4 receptor regulates secretion of non-amyloidogenic precursor protein. J. Biol. Chem. 276, 44881–44888. doi: 10.1074/jbc.M109008200
Rodovalho, G. V., Drummond, L. R., and Coimbra, C. C. (2020). Involvement of brainstem noradrenergic system in cutaneous heat loss during exercise. Brain Res. Bull. 164, 372–379. doi: 10.1016/j.brainresbull.2020.08.029
Rodriguez, J. J., Noristani, H. N., and Verkhratsky, A. (2012). The serotonergic system in ageing and Alzheimer’s disease. Prog. Neurobiol. 99, 15–41. doi: 10.1016/j.pneurobio.2012.06.010
Rojas, P. S., Aguayo, F., Neira, D., Tejos, M., Aliaga, E., Munoz, J. P., et al. (2017). Dual effect of serotonin on the dendritic growth of cultured hippocampal neurons: Involvement of 5-HT1A and 5-HT7 receptors. Mol. Cell Neurosci. 85, 148–161. doi: 10.1016/j.mcn.2017.09.009
Rorabaugh, J. M., Chalermpalanupap, T., Botz-Zapp, C. A., Fu, V. M., Lembeck, N. A., Cohen, R. M., et al. (2017). Chemogenetic locus coeruleus activation restores reversal learning in a rat model of Alzheimer’s disease. Brain 140, 3023–3038. doi: 10.1093/brain/awx232
Rossi Dare, L., Garcia, A., Neves, B. H., and Mello-Carpes, P. B. (2020). One physical exercise session promotes recognition learning in rats with cognitive deficits related to amyloid beta neurotoxicity. Brain Res. 1744:146918. doi: 10.1016/j.brainres.2020.146918
Rovio, S., Kåreholt, I., Helkala, E.-L., Viitanen, M., Winblad, B., Tuomilehto, J., et al. (2005). Leisure-time physical activity at midlife and the risk of dementia and Alzheimer’s disease. Lancet Neurol. 4, 705–711.
Ruan, Q., Yu, Z., Zhang, W., Ruan, J., Liu, C., and Zhang, R. (2018). Cholinergic Hypofunction in Presbycusis-Related Tinnitus With Cognitive Function Impairment: Emerging Hypotheses. Front. Aging Neurosci. 10:98. doi: 10.3389/fnagi.2018.00098
Rubio, A., Avila, J., and Perez, M. (2006). Effect of acetylcholine on tau phosphorylation in human neuroblastoma cells. J. Mol. Neurosci. 30, 185–188. doi: 10.1385/JMN:30:1:185
Russo, O., Cachard-Chastel, M., Riviere, C., Giner, M., Soulier, J. L., Berthouze, M., et al. (2009). Design, synthesis, and biological evaluation of new 5-HT4 receptor agonists: application as amyloid cascade modulators and potential therapeutic utility in Alzheimer’s disease. J. Med. Chem. 52, 2214–2225. doi: 10.1021/jm801327q
Sacheli, M. A., Neva, J. L., Lakhani, B., Murray, D. K., Vafai, N., Shahinfard, E., et al. (2019). Exercise increases caudate dopamine release and ventral striatal activation in Parkinson’s disease. Mov. Disord. 34, 1891–1900. doi: 10.1002/mds.27865
Sadot, E., Gurwitz, D., Barg, J., Behar, L., Ginzburg, I., and Fisher, A. (1996). Activation of m1 muscarinic acetylcholine receptor regulates tau phosphorylation in transfected PC12 cells. J. Neurochem. 66, 877–880. doi: 10.1046/j.1471-4159.1996.66020877.x
Sakakibara, Y., Hirota, Y., Ibaraki, K., Takei, K., Chikamatsu, S., Tsubokawa, Y., et al. (2021). Widespread Reduced Density of Noradrenergic Locus Coeruleus Axons in the App Knock-In Mouse Model of Amyloid-beta Amyloidosis. J. Alzheimers Dis. 82, 1513–1530. doi: 10.3233/JAD-210385
Santiago, L. J., and Abrol, R. (2019). Understanding G Protein Selectivity of Muscarinic Acetylcholine Receptors Using Computational Methods. Int. J. Mol. Sci. 20:5290. doi: 10.3390/ijms20215290
Sara, S. J. (2009). The locus coeruleus and noradrenergic modulation of cognition. Nat. Rev. Neurosci. 10, 211–223. doi: 10.1038/nrn2573
Sayas, C. L., Avila, J., and Wandosell, F. (2002). Glycogen synthase kinase-3 is activated in neuronal cells by Galpha12 and Galpha13 by Rho-independent and Rho-dependent mechanisms. J. Neurosci. 22, 6863–6875. doi: 10.1523/JNEUROSCI.22-16-06863.2002
Schimidt, H. L., Carrazoni, G. S., Garcia, A., Izquierdo, I., Mello-Carpes, P. B., and Carpes, F. P. (2021). Strength training or green tea prevent memory deficits in a beta-amyloid peptide-mediated Alzheimer’s disease model. Exp. Gerontol. 143:111186. doi: 10.1016/j.exger.2020.111186
Schimidt, H. L., Garcia, A., Izquierdo, I., Mello-Carpes, P. B., and Carpes, F. P. (2019). Strength training and running elicit different neuroprotective outcomes in a beta-amyloid peptide-mediated Alzheimer’s disease model. Physiol. Behav. 206, 206–212. doi: 10.1016/j.physbeh.2019.04.012
Schliebs, R., and Arendt, T. (2011). The cholinergic system in aging and neuronal degeneration. Behav. Brain Res. 221, 555–563. doi: 10.1016/j.bbr.2010.11.058
Schwarz, L. A., and Luo, L. (2015). Organization of the locus coeruleus-norepinephrine system. Curr. Biol. 25, R1051–R1056. doi: 10.1016/j.cub.2015.09.039
Scullion, G. A., Kendall, D. A., Marsden, C. A., Sunter, D., and Pardon, M. C. (2011). Chronic treatment with the alpha2-adrenoceptor antagonist fluparoxan prevents age-related deficits in spatial working memory in APPxPS1 transgenic mice without altering beta-amyloid plaque load or astrocytosis. Neuropharmacology 60, 223–234. doi: 10.1016/j.neuropharm.2010.09.002
Segal, S. K., Cotman, C. W., and Cahill, L. F. (2012). Exercise-induced noradrenergic activation enhances memory consolidation in both normal aging and patients with amnestic mild cognitive impairment. J. Alzheimers Dis. 32, 1011–1018. doi: 10.3233/JAD-2012-121078
Shahidi, S., Asl, S. S., Komaki, A., and Hashemi-Firouzi, N. (2018). The effect of chronic stimulation of serotonin receptor type 7 on recognition, passive avoidance memory, hippocampal long-term potentiation, and neuronal apoptosis in the amyloid beta protein treated rat. Psychopharmacology 235, 1513–1525. doi: 10.1007/s00213-018-4862-3
Shahidi, S., Hashemi-Firouzi, N., Afshar, S., Asl, S. S., and Komaki, A. (2019a). Protective Effects of 5-HT1A Receptor Inhibition and 5-HT2A Receptor Stimulation Against Streptozotocin-Induced Apoptosis in the Hippocampus. Malays. J. Med. Sci. 26, 40–51. doi: 10.21315/mjms2019.26.2.5
Shahidi, S., Hashemi-Firouzi, N., Asl, S. S., and Komaki, A. (2019b). Serotonin type 6 receptor antagonist attenuates the impairment of long-term potentiation and memory induced by Abeta. Behav. Brain Res. 364, 205–212. doi: 10.1016/j.bbr.2019.02.004
Shamsipour, S., Sharifi, G., and Taghian, F. (2021). An 8-Week Administration of Bifidobacterium bifidum and Lactobacillus plantarum Combined with Exercise Training Alleviates Neurotoxicity of Abeta and Spatial Learning via Acetylcholine in Alzheimer Rat Model. J. Mol. Neurosci. 71, 1495–1505. doi: 10.1007/s12031-021-01812-y
Sharp, T., and Barnes, N. M. (2020). Central 5-HT receptors and their function; present and future. Neuropharmacology 177:108155. doi: 10.1016/j.neuropharm.2020.108155
Shen, L., Yan, M., and He, L. (2016). D5 receptor agonist 027075 promotes cognitive function recovery and neurogenesis in a Abeta1-42-induced mouse model. Neuropharmacology 105, 72–83. doi: 10.1016/j.neuropharm.2016.01.008
Shiozaki, K., Iseki, E., Hino, H., and Kosaka, K. (2001). Distribution of m1 muscarinic acetylcholine receptors in the hippocampus of patients with Alzheimer’s disease and dementia with Lewy bodies-an immunohistochemical study. J. Neurol. Sci. 193, 23–28. doi: 10.1016/s0022-510x(01)00638-4
Shirey, J. K., Brady, A. E., Jones, P. J., Davis, A. A., Bridges, T. M., Kennedy, J. P., et al. (2009). A selective allosteric potentiator of the M1 muscarinic acetylcholine receptor increases activity of medial prefrontal cortical neurons and restores impairments in reversal learning. J. Neurosci. 29, 14271–14286. doi: 10.1523/JNEUROSCI.3930-09.2009
Simic, G., Babic Leko, M., Wray, S., Harrington, C. R., Delalle, I., Jovanov-Milosevic, N., et al. (2017). Monoaminergic neuropathology in Alzheimer’s disease. Prog. Neurobiol. 151, 101–138. doi: 10.1016/j.pneurobio.2016.04.001
Simon, M. I., Strathmann, M. P., and Gautam, N. (1991). Diversity of G proteins in signal transduction. Science 252, 802–808. doi: 10.1126/science.1902986
Soeda, Y., Yoshikawa, M., Almeida, O. F., Sumioka, A., Maeda, S., Osada, H., et al. (2015). Toxic tau oligomer formation blocked by capping of cysteine residues with 1,2-dihydroxybenzene groups. Nat. Commun. 6:10216. doi: 10.1038/ncomms10216
Su, M., Zhu, L., Zhang, Y., Paknejad, N., Dey, R., Huang, J., et al. (2020). Structural Basis of the Activation of Heterotrimeric Gs-Protein by Isoproterenol-Bound beta1-Adrenergic Receptor. Mol. Cell. 80:e54. doi: 10.1016/j.molcel.2020.08.001
Sun, L., Wang, X., Liu, S., Wang, Q., Wang, J., Bennecib, M., et al. (2005). Bilateral injection of isoproterenol into hippocampus induces Alzheimer-like hyperphosphorylation of tau and spatial memory deficit in rat. FEBS Lett. 579, 251–258. doi: 10.1016/j.febslet.2004.11.083
Sun, Q., Jia, N., Li, X., Yang, J., and Chen, G. (2019). Grape seed proanthocyanidins ameliorate neuronal oxidative damage by inhibiting GSK-3beta-dependent mitochondrial permeability transition pore opening in an experimental model of sporadic Alzheimer’s disease. Aging 11, 4107–4124. doi: 10.18632/aging.102041
Tajeddinn, W., Fereshtehnejad, S. M., Seed Ahmed, M., Yoshitake, T., Kehr, J., Shahnaz, T., et al. (2016a). Association of Platelet Serotonin Levels in Alzheimer’s Disease with Clinical and Cerebrospinal Fluid Markers. J. Alzheimers Dis. 53, 621–630. doi: 10.3233/JAD-160022
Tajeddinn, W., Persson, T., Calvo-Garrido, J., Seed Ahmed, M., Maioli, S., Vijayaraghavan, S., et al. (2016b). Pharmacological Modulations of the Serotonergic System in a Cell-Model of Familial Alzheimer’s Disease. J. Alzheimers Dis. 53, 349–361. doi: 10.3233/JAD-160046
Tan, Z. S., Spartano, N. L., Beiser, A. S., DeCarli, C., Auerbach, S. H., Vasan, R. S., et al. (2017). Physical Activity, Brain Volume, and Dementia Risk: The Framingham Study. J. Geronto Biol. Sci. Med. Sci. 72, 789–795. doi: 10.1093/gerona/glw130
Teglas, T., Nemeth, Z., Koller, A., Van der Zee, E. A., Luiten, P. G. M., and Nyakas, C. (2019). Effects of Long-Term Moderate Intensity Exercise on Cognitive Behaviors and Cholinergic Forebrain in the Aging Rat. Neuroscience 411, 65–75. doi: 10.1016/j.neuroscience.2019.05.037
Tesseur, I., Pimenova, A. A., Lo, A. C., Ciesielska, M., Lichtenthaler, S. F., De Maeyer, J. H., et al. (2013). Chronic 5-HT4 receptor activation decreases Abeta production and deposition in hAPP/PS1 mice. Neurobiol. Aging 34, 1779–1789. doi: 10.1016/j.neurobiolaging.2013.01.020
Thathiah, A., Horre, K., Snellinx, A., Vandewyer, E., Huang, Y., Ciesielska, M., et al. (2013). beta-arrestin 2 regulates Abeta generation and gamma-secretase activity in Alzheimer’s disease. Nat. Med. 19, 43–49. doi: 10.1038/nm.3023
Thomas, A. J., Hendriksen, M., Piggott, M., Ferrier, I. N., Perry, E., Ince, P., et al. (2006). A study of the serotonin transporter in the prefrontal cortex in late-life depression and Alzheimer’s disease with and without depression. Neuropathol. Appl. Neurobiol. 32, 296–303. doi: 10.1111/j.1365-2990.2006.00728.x
Tian, X. L., Yu, L. H., Li, W. Q., Hu, Y., Yin, M., and Wang, Z. J. (2015). Activation of 5-HT(2C) receptor promotes the expression of neprilysin in U251 human glioma cells. Cell Mol. Neurobiol. 35, 425–432. doi: 10.1007/s10571-014-0138-6
Tomoto, T., Liu, J., Tseng, B. Y., Pasha, E. P., Cardim, D., Tarumi, T., et al. (2021). One-Year Aerobic Exercise Reduced Carotid Arterial Stiffness and Increased Cerebral Blood Flow in Amnestic Mild Cognitive Impairment. J. Alzheimers Dis. 80, 841–853. doi: 10.3233/JAD-201456
Tournissac, M., Vu, T. M., Vrabic, N., Hozer, C., Tremblay, C., Melancon, K., et al. (2021). Repurposing beta-3 adrenergic receptor agonists for Alzheimer’s disease: beneficial effects in a mouse model. Alzheimers Res. Ther. 13:103. doi: 10.1186/s13195-021-00842-3
Trabace, L., Kendrick, K. M., Castrignano, S., Colaianna, M., De Giorgi, A., Schiavone, S., et al. (2007). Soluble amyloid beta1-42 reduces dopamine levels in rat prefrontal cortex: relationship to nitric oxide. Neuroscience 147, 652–663. doi: 10.1016/j.neuroscience.2007.04.056
Tsang, S. W., Lai, M. K., Kirvell, S., Francis, P. T., Esiri, M. M., Hope, T., et al. (2006). Impaired coupling of muscarinic M1 receptors to G-proteins in the neocortex is associated with severity of dementia in Alzheimer’s disease. Neurobiol. Aging 27, 1216–1223. doi: 10.1016/j.neurobiolaging.2005.07.010
Vana, L., Kanaan, N. M., Ugwu, I. C., Wuu, J., Mufson, E. J., and Binder, L. I. (2011). Progression of tau pathology in cholinergic Basal forebrain neurons in mild cognitive impairment and Alzheimer’s disease. Am. J. Pathol. 179, 2533–2550. doi: 10.1016/j.ajpath.2011.07.044
Vargas, L. S., Ramires Lima, K., Piaia Ramborger, B., Roehrs, R., Izquierdo, I., and Mello-Carpes, P. B. (2020). Catecholaminergic hippocampal activation is necessary for object recognition memory persistence induced by one-single physical exercise session. Behav. Brain Res. 379:112356. doi: 10.1016/j.bbr.2019.112356
Verdurand, M., Berod, A., Le Bars, D., and Zimmer, L. (2011). Effects of amyloid-beta peptides on the serotoninergic 5-HT1A receptors in the rat hippocampus. Neurobiol. Aging 32, 103–114. doi: 10.1016/j.neurobiolaging.2009.01.008
Verdurand, M., Chauveau, F., Daoust, A., Morel, A. L., Bonnefoi, F., Liger, F., et al. (2016). Differential effects of amyloid-beta 1-40 and 1-42 fibrils on 5-HT1A serotonin receptors in rat brain. Neurobiol. Aging 40, 11–21. doi: 10.1016/j.neurobiolaging.2015.12.008
Vermeiren, Y., Van Dam, D., Aerts, T., Engelborghs, S., and De Deyn, P. P. (2014). Monoaminergic neurotransmitter alterations in postmortem brain regions of depressed and aggressive patients with Alzheimer’s disease. Neurobiol. Aging 35, 2691–2700. doi: 10.1016/j.neurobiolaging.2014.05.031
Vertes, R. P. (1991). A PHA-L analysis of ascending projections of the dorsal raphe nucleus in the rat. J. Comp. Neurol. 313, 643–668. doi: 10.1002/cne.903130409
Vertes, R. P., Fortin, W. J., and Crane, A. M. (1999). Projections of the median raphe nucleus in the rat. J. Comp. Neurol. 407, 555–582.
Wang, D. L., Ling, Z. Q., Cao, F. Y., Zhu, L. Q., and Wang, J. Z. (2004). Melatonin attenuates isoproterenol-induced protein kinase A overactivation and tau hyperphosphorylation in rat brain. J. Pineal. Res. 37, 11–16. doi: 10.1111/j.1600-079X.2004.00130.x
Wang, D., Yang, L., Su, J., Niu, Y., Lei, X., Xiong, J., et al. (2011). Attenuation of neurodegenerative phenotypes in Alzheimer-like presenilin 1/presenilin 2 conditional double knockout mice by EUK1001, a promising derivative of xanomeline. Biochem. Biophys. Res. Commun. 410, 229–234. doi: 10.1016/j.bbrc.2011.05.120
Wang, J., Ono, K., Dickstein, D. L., Arrieta-Cruz, I., Zhao, W., Qian, X., et al. (2011). Carvedilol as a potential novel agent for the treatment of Alzheimer’s disease. Neurobiol. Aging 32, 2321.e1–12. doi: 10.1016/j.neurobiolaging.2010.05.004
Wang, M., Zong, H. F., Chang, K. W., Han, H., Yasir Rizvi, M., Iffat Neha, S., et al. (2020). 5-HT1AR alleviates Abeta-induced cognitive decline and neuroinflammation through crosstalk with NF-kappaB pathway in mice. Int. Immunopharmacol. 82:106354. doi: 10.1016/j.intimp.2020.106354
Wang, Q. W., Rowan, M. J., and Anwyl, R. (2009). Inhibition of LTP by beta-amyloid is prevented by activation of beta2 adrenoceptors and stimulation of the cAMP/PKA signalling pathway. Neurobiol. Aging 30, 1608–1613. doi: 10.1016/j.neurobiolaging.2007.12.004
Wang, R., and Holsinger, R. M. D. (2018). Exercise-induced brain-derived neurotrophic factor expression: Therapeutic implications for Alzheimer’s dementia. Ageing Res. Rev. 48, 109–121. doi: 10.1016/j.arr.2018.10.002
Wang, W. J., Qiao, Y. H., and Li, Z. J. (2018). New Insights into Modes of GPCR Activation. Trends Pharmacol. Sci. 39, 367–386. doi: 10.1016/j.tips.2018.01.001
Wang, Y. J., Ren, Q. G., Gong, W. G., Wu, D., Tang, X., Li, X. L., et al. (2016). Escitalopram attenuates beta-amyloid-induced tau hyperphosphorylation in primary hippocampal neurons through the 5-HT1A receptor mediated Akt/GSK-3beta pathway. Oncotarget 7, 13328–13339. doi: 10.18632/oncotarget.7798
Wang, Z., Zhang, Y. H., Zhang, W., Gao, H. L., Zhong, M. L., Huang, T. T., et al. (2018). Copper chelators promote nonamyloidogenic processing of AbetaPP via MT1/2/CREB-dependent signaling pathways in AbetaPP/PS1 transgenic mice. J. Pineal. Res. 65:e12502. doi: 10.1111/jpi.12502
Weiss, L. R., Venezia, A. C., and Smith, J. C. (2019). A single bout of hard RPE-based cycling exercise increases salivary alpha-amylase. Physiol. Behav. 208:112555. doi: 10.1016/j.physbeh.2019.05.016
Welt, T., Kulic, L., Hoey, S. E., McAfoose, J., Spani, C., Chadha, A. S., et al. (2015). Acute Effects of Muscarinic M1 Receptor Modulation on AbetaPP Metabolism and Amyloid-beta Levels in vivo: A Microdialysis Study. J. Alzheimers Dis. 46, 971–982. doi: 10.3233/JAD-150152
Whitehouse, P. J., Price, D. L., Struble, R. G., Clark, A. W., Coyle, J. T., and Delon, M. R. (1982). Alzheimer’s disease and senile dementia: loss of neurons in the basal forebrain. Science 215, 1237–1239. doi: 10.1126/science.7058341
Wilson, R. S., Nag, S., Boyle, P. A., Hizel, L. P., Yu, L., Buchman, A. S., et al. (2013). Neural reserve, neuronal density in the locus ceruleus, and cognitive decline. Neurology 80, 1202–1208. doi: 10.1212/WNL.0b013e3182897103
Wu, C., Yang, L., Li, Y., Dong, Y., Yang, B., Tucker, L. D., et al. (2020). Effects of Exercise Training on Anxious-Depressive-like Behavior in Alzheimer Rat. Med. Sci. Sports Exerc. 52, 1456–1469. doi: 10.1249/MSS.0000000000002294
Wu, Q., Sun, J. X., Song, X. H., Wang, J., Xiong, C. Q., Teng, F. X., et al. (2017). Blocking beta 2-adrenergic receptor inhibits dendrite ramification in a mouse model of Alzheimer’s disease. Neural. Regen. Res. 12, 1499–1506. doi: 10.4103/1673-5374.215261
Wu, S. Y., Wang, T. F., Yu, L., Jen, C. J., Chuang, J. I., Wu, F. S., et al. (2011). Running exercise protects the substantia nigra dopaminergic neurons against inflammation-induced degeneration via the activation of BDNF signaling pathway. Brain Behav. Immun. 25, 135–146. doi: 10.1016/j.bbi.2010.09.006
Xhima, K., Markham-Coultes, K., Nedev, H., Heinen, S., Saragovi, H. U., Hynynen, K., et al. (2020). Focused ultrasound delivery of a selective TrkA agonist rescues cholinergic function in a mouse model of Alzheimer’s disease. Sci. Adv. 6:eaax6646. doi: 10.1126/sciadv.aax6646
Xie, Y., Liu, P. P., Lian, Y. J., Liu, H. B., and Kang, J. S. (2019). The effect of selective serotonin reuptake inhibitors on cognitive function in patients with Alzheimer’s disease and vascular dementia: focusing on fluoxetine with long follow-up periods. Signal Transduct Target Ther. 4:30. doi: 10.1038/s41392-019-0064-7
Xu, H., Rajsombath, M. M., Weikop, P., and Selkoe, D. J. (2018). Enriched environment enhances beta-adrenergic signaling to prevent microglia inflammation by amyloid-beta. EMBO Mol. Med. 10:e8931. doi: 10.15252/emmm.201808931
Xu, L., Long, J., Su, Z., Xu, B., Lin, M., Chen, Y., et al. (2019). Restored presynaptic synaptophysin and cholinergic inputs contribute to the protective effects of physical running on spatial memory in aged mice. Neurobiol. Dis. 132:104586. doi: 10.1016/j.nbd.2019.104586
Yamamoto, T., and Hirano, A. (1985). Nucleus raphe dorsalis in parkinsonism-dementia complex of Guam. Acta Neuropathol. 67, 296–299. doi: 10.1007/BF00687815
Yang, J. H., Lee, E. O., Kim, S. E., Suh, Y. H., and Chong, Y. H. (2012). Norepinephrine differentially modulates the innate inflammatory response provoked by amyloid-beta peptide via action at beta-adrenoceptors and activation of cAMP/PKA pathway in human THP-1 macrophages. Exp. Neurol. 236, 199–206. doi: 10.1016/j.expneurol.2012.05.008
Yang, Y., Zhang, L., Yu, J., Ma, Z., Li, M., Wang, J., et al. (2021). A Novel 5-HT1B Receptor Agonist of Herbal Compounds and One of the Therapeutic Uses for Alzheimer’s Disease. Front. Pharmacol. 12:735876. doi: 10.3389/fphar.2021.735876
Yu, F., Mathiason, M. A., Han, S., Gunter, J. L., Jones, D., Botha, H., et al. (2021). Mechanistic Effects of Aerobic Exercise in Alzheimer’s Disease: Imaging Findings From the Pilot FIT-AD Trial. Front. Aging Neurosci. 13:703691. doi: 10.3389/fnagi.2021.703691
Yu, N. N., Wang, X. X., Yu, J. T., Wang, N. D., Lu, R. C., Miao, D., et al. (2010). Blocking beta2-adrenergic receptor attenuates acute stress-induced amyloid beta peptides production. Brain Res. 1317, 305–310. doi: 10.1016/j.brainres.2009.12.087
Yuan Xiang, P., Janc, O., Grochowska, K. M., Kreutz, M. R., and Reymann, K. G. (2016). Dopamine agonists rescue Abeta-induced LTP impairment by Src-family tyrosine kinases. Neurobiol. Aging 40, 98–102. doi: 10.1016/j.neurobiolaging.2016.01.008
Yuede, C. M., Wallace, C. E., Davis, T. A., Gardiner, W. D., Hettinger, J. C., Edwards, H. M., et al. (2021). Pimavanserin, a 5HT2A receptor inverse agonist, rapidly suppresses Abeta production and related pathology in a mouse model of Alzheimer’s disease. J. Neurochem. 156, 658–673. doi: 10.1111/jnc.15260
Yun, H. M., Park, K. R., Kim, E. C., Kim, S., and Hong, J. T. (2015). Serotonin 6 receptor controls Alzheimer’s disease and depression. Oncotarget 6, 26716–26728. doi: 10.18632/oncotarget.5777
Zang, J., Liu, Y., Li, W., Xiao, D., Zhang, Y., Luo, Y., et al. (2017). Voluntary exercise increases adult hippocampal neurogenesis by increasing GSK-3beta activity in mice. Neuroscience 354, 122–135. doi: 10.1016/j.neuroscience.2017.04.024
Zang, X., Cheng, Z. Y., Sun, Y., Hua, N., Zhu, L. H., and He, L. (2018). The ameliorative effects and underlying mechanisms of dopamine D1-like receptor agonist SKF38393 on Abeta1-42-induced cognitive impairment. Prog. Neuropsychopharmacol. Biol. Psychiatry 81, 250–261. doi: 10.1016/j.pnpbp.2017.09.017
Zhang, F., Gannon, M., Chen, Y., Yan, S., Zhang, S., Feng, W., et al. (2020). beta-amyloid redirects norepinephrine signaling to activate the pathogenic GSK3beta/tau cascade. Sci. Transl. Med. 12:eaay6931. doi: 10.1126/scitranslmed.aay6931
Zhang, F., Gannon, M., Chen, Y., Zhou, L., Jiao, K., and Wang, Q. (2017). The amyloid precursor protein modulates alpha2A-adrenergic receptor endocytosis and signaling through disrupting arrestin 3 recruitment. FASEB J. 31, 4434–4446. doi: 10.1096/fj.201700346R
Zhang, L., Fan, Y., Kong, X., and Hao, W. (2020). Neuroprotective effect of different physical exercises on cognition and behavior function by dopamine and 5-HT level in rats of vascular dementia. Behav. Brain Res. 388:112648. doi: 10.1016/j.bbr.2020.112648
Zhang, X., He, Q., Huang, T., Zhao, N., Liang, F., Xu, B., et al. (2019). Treadmill Exercise Decreases Abeta Deposition and Counteracts Cognitive Decline in APP/PS1 Mice, Possibly via Hippocampal Microglia Modifications. Front. Aging Neurosci. 11:78. doi: 10.3389/fnagi.2019.00078
Zhang, Y., Chen, L., Shen, G., Zhao, Q., Shangguan, L., and He, M. (2014). GRK5 dysfunction accelerates tau hyperphosphorylation in APP (swe) mice through impaired cholinergic activity. Neuroreport 25, 542–547. doi: 10.1097/WNR.0000000000000142
Zhang, Z., Shen, Q., Wu, X., Zhang, D., and Xing, D. (2020). Activation of PKA/SIRT1 signaling pathway by photobiomodulation therapy reduces Abeta levels in Alzheimer’s disease models. Aging Cell 19:e13054. doi: 10.1111/acel.13054
Zhao, J., Li, X., Chen, X., Cai, Y., Wang, Y., Sun, W., et al. (2019). GRK5 influences the phosphorylation of tau via GSK3beta and contributes to Alzheimer’s disease. J. Cell Physiol. 234, 10411–10420. doi: 10.1002/jcp.27709
Zhao, L. X., Chen, M. W., Qian, Y., Yang, Q. H., Ge, Y. H., Chen, H. Z., et al. (2019). M1 Muscarinic Receptor Activation Rescues beta-Amyloid-Induced Cognitive Impairment through AMPA Receptor GluA1 Subunit. Neuroscience 408, 239–247. doi: 10.1016/j.neuroscience.2019.04.007
Zheng, H., Niu, S., Zhao, H., Li, S., and Jiao, J. (2018). Donepezil improves the cognitive impairment in a tree shrew model of Alzheimer’s disease induced by amyloid-beta1-40 via activating the BDNF/TrkB signal pathway. Metab. Brain Dis. 33, 1961–1974. doi: 10.1007/s11011-018-0303-6
Zhu, G., Yang, S., Xie, Z., and Wan, X. (2018). Synaptic modification by L-theanine, a natural constituent in green tea, rescues the impairment of hippocampal long-term potentiation and memory in AD mice. Neuropharmacology 138, 331–340. doi: 10.1016/j.neuropharm.2018.06.030
Zhu, H., Yan, H., Tang, N., Li, X., Pang, P., Li, H., et al. (2017). Impairments of spatial memory in an Alzheimer’s disease model via degeneration of hippocampal cholinergic synapses. Nat. Commun. 8:1676. doi: 10.1038/s41467-017-01943-0
Zimmer, P., Stritt, C., Bloch, W., Schmidt, F. P., Hubner, S. T., Binnebossel, S., et al. (2016). The effects of different aerobic exercise intensities on serum serotonin concentrations and their association with Stroop task performance: a randomized controlled trial. Eur. J. Appl. Physiol. 116, 2025–2034. doi: 10.1007/s00421-016-3456-1
Ziu, I., Rettig, I., Luo, D., Dutta, A., McCormick, T. M., Wu, C., et al. (2020). The multifunctional dopamine D2/D3 receptor agonists also possess inhibitory activity against the full-length tau441 protein aggregation. Bioorg. Med. Chem. 28:115667. doi: 10.1016/j.bmc.2020.115667
Keywords: Alzheimer’s disease, exercise, G protein-coupled receptor, acetylcholine, norepinephrine, serotonin, dopamine
Citation: Zong B, Yu F, Zhang X, Zhao W, Sun P, Li S and Li L (2022) Understanding How Physical Exercise Improves Alzheimer’s Disease: Cholinergic and Monoaminergic Systems. Front. Aging Neurosci. 14:869507. doi: 10.3389/fnagi.2022.869507
Received: 04 February 2022; Accepted: 14 April 2022;
Published: 18 May 2022.
Edited by:
Bo Su, Shandong University, ChinaReviewed by:
Dandan Chu, Nantong University, ChinaTim Huang, Sanford Burnham Prebys Medical Discovery Institute, United States
Copyright © 2022 Zong, Yu, Zhang, Zhao, Sun, Li and Li. This is an open-access article distributed under the terms of the Creative Commons Attribution License (CC BY). The use, distribution or reproduction in other forums is permitted, provided the original author(s) and the copyright owner(s) are credited and that the original publication in this journal is cited, in accordance with accepted academic practice. No use, distribution or reproduction is permitted which does not comply with these terms.
*Correspondence: Lin Li, bGlsaW4ueHR0QDE2My5jb20=