- Department of Neuroscience and Experimental Therapeutics, College of Medicine, Texas A&M University Health Science Center, Bryan, TX, USA
This article describes the emerging evidence of hormonal influence on epileptogenesis, which is a process whereby a brain becomes progressively epileptic due to an initial precipitating event of diverse origin such as brain injury, stroke, infection, or prolonged seizures. The molecular mechanisms underlying the development of epilepsy are poorly understood. Neuroinflammation and neurodegeneration appear to trigger epileptogenesis. There is an intense search for drugs that truly prevent the development of epilepsy in people at risk. Hormones play an important role in children and adults with epilepsy. Corticosteroids, progesterone, estrogens, and neurosteroids have been shown to affect seizure activity in animal models and in clinical studies. However, the impact of hormones on epileptogenesis has not been investigated widely. There is emerging new evidence that progesterone, neurosteroids, and endogenous hormones may play a role in regulating the epileptogenesis. Corticosterone has excitatory effects and triggers epileptogenesis in animal models. Progesterone has disease-modifying activity in epileptogenic models. The antiepileptogenic effect of progesterone has been attributed to its conversion to neurosteroids, which binds to GABA-A receptors and enhances phasic and tonic inhibition in the brain. Neurosteroids are robust anticonvulsants. There is pilot evidence that neurosteroids may have antiepileptogenic properties. Future studies may generate new insight on the disease-modifying potential of hormonal agents and neurosteroids in epileptogenesis.
Introduction
Epilepsy, one of the most common serious neurological disorders, is characterized by the unpredictable occurrence of seizures. A seizure is an abnormal electrical discharge in the brain that causes an alteration in consciousness, sensations, and behaviors. The symptoms that occur depend on the parts of the brain affected during the seizure. Common signs of seizures include staring, unusual feelings, twitching, unconsciousness, and jerking in the arms or legs. Epilepsy affects an estimated 3 million Americans and about 65 million people worldwide in a variety of ways (Jacobs et al., 2009; Hesdorffer et al., 2013). About 150,000 new cases of epilepsy are diagnosed in the United States annually (Hesdorffer et al., 2013). Children and adults are the fastest-growing segments of the population with new cases of epilepsy.
Epilepsy is a collective designation for a group of brain disorders consisting of a complex spectrum of different seizure types and syndromes. Epileptic seizures are classified into partial (simple and complex partial seizures) and generalized seizures (absence, tonic–clonic, myoclonic, and atonic seizures). Accurate diagnosis of seizure type and epileptic syndrome is critical for determining appropriate drug therapy and prognosis. The International League Against Epilepsy (ILAE) provided a definition of “seizure” and “epilepsy” (Fisher et al., 2005). A seizure is defined as “a transient occurrence of signs and/or symptoms due to abnormal synchronous neuronal activity in the brain.” Epilepsy is defined as “a disorder of the brain characterized by an enduring predisposition to generate epileptic seizures.” A single seizure, therefore, does not constitute epilepsy. The diagnosis of epilepsy requires the occurrence of recurrent (two or more) epileptic seizures separated by at least 24 h, unprovoked by any immediate identified cause. Antiepileptic drugs (AEDs) are the mainstay for controlling seizures (Table 1). Current drug therapy is symptomatic in that available drugs inhibit seizures, but neither effective prophylaxis nor cure are available. The goal of the therapy is to eliminate seizures without interfering with normal function (Glauser et al., 2006, 2013). Despite many advances in epilepsy research, presently an estimated 30% of people with epilepsy have “intractable seizures” that do not respond to even the best available medication. There is renewed focus on the pathophysiology of epileptogenesis, the process whereby a brain becomes progressively epileptic due to an initial precipitating event.
This article describes the emerging evidence of hormonal influence on epileptogenesis and the potential mechanisms underlying their actions on neuronal excitability and seizure activity. It also describes recent studies on neurosteroid agents that prevent or delay the development of epilepsy. The main focus of the review is on steroid hormones and neurosteroids with seizure-modulating activity. Neuropeptides and other hormones such as oxytocin, neuropeptide-Y, and galanin, which may affect neuronal excitability, are not discussed here because such description is beyond the scope of this article. The seizure-modulating effects of neuropeptides are discussed elsewhere (Robertson et al., 2011).
Overview of Epileptogenesis and Intervention Strategies
Epilepsy is a chronic condition with many possible causes. Epilepsy may develop because of an abnormality in neural connectivity, an imbalance in inhibitory and excitatory neurotransmitters, or some combination of these factors. Primary epilepsy (50%) is idiopathic (“unknown cause”). In secondary epilepsy (50%), seizures may result from a variety of conditions including trauma, anoxia, metabolic imbalances, tumors, encephalitis, drug withdrawal, and neurotoxicity (Engel et al., 2007). The molecular mechanisms underlying the development of acquired epilepsy are not very well understood. The term “epileptogenesis” is used to describe the complex plastic changes in the brain that, following a precipitating event, convert a normal brain into a brain debilitated by recurrent seizures (Pitkänen et al., 2009; Pitkänen and Lukasiuk, 2011). Although specific types of epilepsy may have unique pathophysiological mechanisms, a broad hypothesis in this field is that convergent neuronal mechanisms are common in different forms of acquired epilepsy.
The current hypothesis about the pathogenesis of epilepsy (epileptogenesis) involves three stages: (1) the initial precipitating event; (2) the latent period (no seizures); and (3) the chronic period with spontaneous seizures (Figure 1). Acquired epilepsy typically develops due to an initial precipitating event such as traumatic brain injury (TBI), stroke, brain infections, or prolonged seizures. The other possible precipitating triggers for epileptogenesis include febrile seizures, metabolic dysfunction, alcohol withdrawal, and status epilepticus, an emergency condition characterized by continuous seizures or repeated seizures without regaining consciousness for 30 min or more (McClelland et al., 2011). Exposure to organophosphorous pesticides and chemical warfare nerve agents, such as soman, can cause epilepsy as a result of cholinergic neurotoxicity and status epilepticus (de Araujo Furtado et al., 2012).
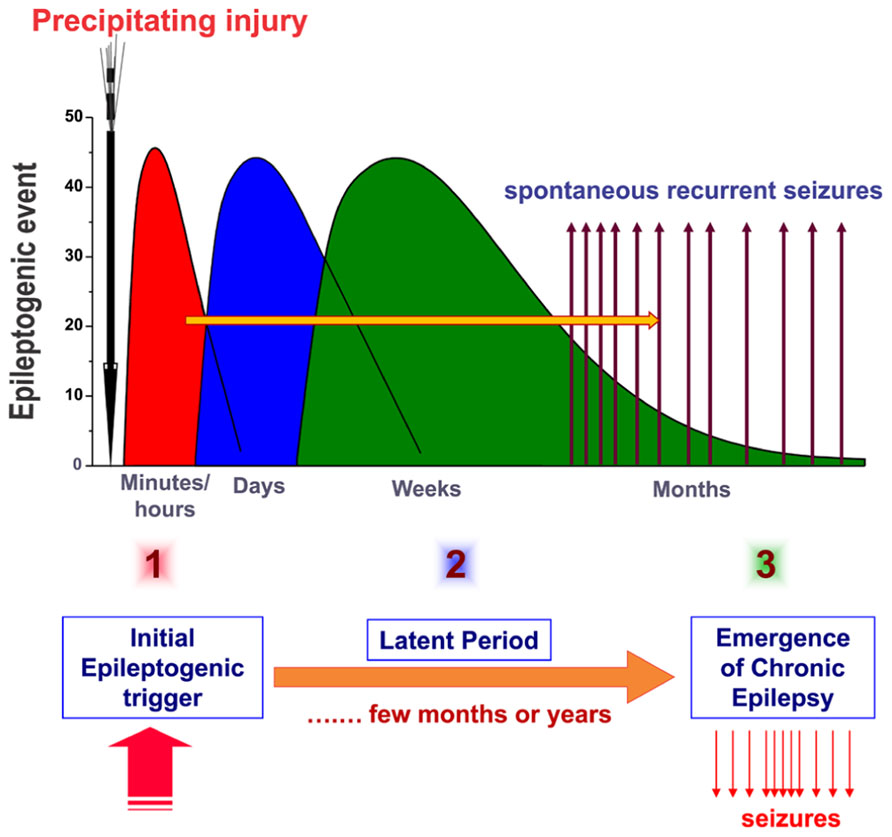
FIGURE 1. Pathophysiology of epileptogenesis. Epileptogenesis is the process whereby a normal brain becomes progressively epileptic because of precipitating injury or risk factors such as TBI, stroke, brain infections, or prolonged seizures. Epilepsy development can be described in three stages: (1) the initial injury (epileptogenic event); (2) the latent period (silent period with no seizure activity); and (3) chronic period with spontaneous recurrent seizures. Although the precise mechanisms underlying spatial and temporal events remain unclear, epileptogenesis may involve an interaction of acute and delayed anatomic, molecular, and physiological events that are both complex and multifaceted. The initial precipitating factor activates diverse signaling events, such as inflammation, oxidation, apoptosis, neurogenesis, and synaptic plasticity, which eventually lead to structural and functional changes in neurons. These changes are eventually manifested as abnormal hyperexcitability and spontaneous seizures.
The development of epileptogenesis is thought to be a step-function of time after the brain injury, with a latent period present between the brain injury and the first unprovoked seizure. There are some alternative hypotheses to this notion that view epileptogenesis as a continuous process that extends past the first spontaneous seizure (Dudek and Staley, 2011). Temporal lobe epilepsy (TLE) is one of the common forms of chronic epilepsies (Wieser and ILAE Commission on Neurosurgery of Epilepsy, 2004). TLE is characterized by the progressive expansion of spontaneous seizures originating from the limbic system regions, especially the hippocampus, most often due to neuronal injury. The hippocampal sclerosis, which is characterized by aberrant mossy fiber sprouting and widespread neuronal loss in the dentate hilus and CA1 and CA3 subfields, is the hallmark of epilepsy pathology (Sutula et al., 1989; Buckmaster et al., 2002; Nadler, 2003; Morimoto et al., 2004). However, there is an ongoing debate about whether the hippocampal sclerosis is the basis or the outcome of recurring seizures. There is emerging evidence from refractory models that epilepsy involves “progressive epileptogenesis” much beyond the latent period and the onset of the first seizure (Williams et al., 2009). Several mechanisms have been described including loss of interneurons (van Vliet et al., 2004; Sloviter and Bumanglag, 2013) and neuroinflammation (Vezzani et al., 2011). There is great variation in the onset of spontaneous seizures following a precipitating factor (Rao et al., 2006; Norwood et al., 2010). Thus, the critical window for effective “antiepileptogenic” interventions remain poorly defined for curing epilepsy in people at risk.
Studies in animal models have provided improved understanding of neurophysiological basis of epileptic seizures (O’Dell et al., 2012; Simonato et al., 2012). Spontaneous seizures arise from hyperexcitable and hypersynchronous neuronal networks and involve both cortical and several key subcortical structures. The general cellular pathway underlying occurrence of epileptic seizures is apparent in three phases: (i) focal epileptogenicity (initiation); (ii) synchronization of the surrounding neurons (sync); and (iii) propagation of the seizure discharge to other areas of the brain (spread). Experimental field and intracellular recordings in isolated brain sections provide a detailed description of neurophysiological abnormalities underlying epileptic regions. Paroxysmal depolarization shift (PDS) is identified as a hallmark of epileptic neurons. It is characteristic of neurons in epileptic cortical zones and consists of an abnormally prolonged depolarization with repetitive spiking reflected as interictal discharges in the electroencephalogram (EEG). High-frequency oscillations, termed ripples (80–200 Hz) and fast ripples (250–600 Hz), are recorded in the EEG of epileptic patients and in animal epilepsy models (Köhling and Staley, 2011; Lévesque et al., 2011). Fast ripples are thought to reflect pathological activity and seizure onset zones.
Gowers (1881) proposed that seizures beget seizures. The kindling model has provided a conceptual framework for this idea and for developing new molecular targets for preventing epilepsy (Goddard et al., 1969; McNamara et al., 1992). Post-status epilepticus paradigms are widely used for modeling the epileptogenesis in which a single episode of prolonged seizures (by pilocarpine, kainate, or electrical stimulation) triggers progressive development of seizure activity (Buckmaster and Dudek, 1997; Hellier et al., 1999; Glien et al., 2001; Rao et al., 2006; Löscher, 2012). These chronic models share many features of human limbic epilepsy (Löscher, 2002; Stables et al., 2002). Pilocarpine, kainate, or perforant path stimulation induce acute SE and neuronal injury and follow a pattern of latent period similar to that observed in limbic epilepsy. Like TLE, mossy fiber sprouting, neurodegeneration, and ectopic granule cell proliferation are evident after the latent period (Löscher, 2002; Rao et al., 2006).
Despite decades of research, currently there is no single Food and Drug Administration (FDA)-approved drug that truly prevents the development of epilepsy in people at risk. A variety of intervention approaches have been tested in animal models of epileptogenesis (Acharya et al., 2008; Pitkänen and Lukasiuk, 2011). A number of clinical trials show a lack of antiepileptogenic efficacy of AEDs, including phenytoin and carbamazepine, in patients at high risk for developing epilepsy (Temkin, 2001; Mani et al., 2011). There is a desperate need for drugs that truly prevent the development of epilepsy (“antiepileptogenic agents”) or alter its natural course to delay the appearance or severity of epileptic seizures (“disease-modifying agents”).
In 2000, National Institute of Neurological Disorders and Stroke (NINDS) and epilepsy research and advocacy groups organized the first “Curing Epilepsy” conference, which marked a turning point for shifting and expanding the focus of epilepsy research toward cures for epilepsy and the prevention of epilepsy in those at risk (Jacobs et al., 2001). During the past decade, there has been increasing research emphasis on the prevention of epileptogenesis and translation of lead discoveries in this field into therapies for curing epilepsy (Jacobs et al., 2009; Simonato et al., 2012). The Institute of Medicine (IOM) released a consensus report in 2012 on public health dimensions of the epilepsies focusing on promoting health and understanding epilepsy (Austin et al., 2012; Hesdorffer et al., 2013). The IOM report, Epilepsy Across the Spectrum: Promoting Health and Understanding, provided 13 recommendations for future work in the field of epilepsy. The report contains research priorities which include one key recommendation on prevention of epilepsy.
Role of Steroid Hormones in Epileptogenesis
Steroid hormones play a key role in the neuroendocrine control of neuronal excitability and seizure susceptibility (Table 2; Herzog, 2002; Reddy, 2003a, 2010; Verrotti et al., 2007). Steroid hormones are synthesized and secreted from ovarian, gonadal, and adrenal sources. In men, the main circulating steroids are androgenic steroids (testosterone and dihydrotestosterone) and adrenal corticosteroids (cortisol and aldosterone). Deoxycorticosterone (DOC) is also released from adrenal cortex in response to stress. In women, the primary reproductive steroid hormones are estrogens and progesterone, which are released during the menstrual cycle. The early follicular phase is associated with low levels of estrogens and progesterone. The synthesis and secretion of estrogens and progesterone from the ovaries are controlled primarily by hypothalamic gonadotropin releasing hormone (GnRH) and the pituitary gonadotropins, follicle stimulating hormone (FSH) and luteinizing hormone (LH). As ovulation approaches, the level of estrogen rises and triggers a large surge of LH leading to ovulation. Following ovulation, the ruptured follicle luteinizes and forms a corpus luteum that secretes progesterone and estrogen. Estradiol is secreted in the second half of the follicular phase and increases to a peak at midcycle, while progesterone is elevated during the luteal phase and declines before menstruation begins.
The cyclical changes of estrogens and progesterone are now widely believed to be important in the pathogenesis of catamenial epilepsy, a menstrual cycle-related seizure disorder in women with epilepsy (Reddy, 2009a, 2013). Catamenial epilepsy is a multifaceted neuroendocrine condition in which seizures are clustered around specific points in the menstrual cycle, most often around perimenstrual or periovulatory period. Generally, estrogens are found to be excitatory or proconvulsant, while progesterone has powerful antiseizure effect and reduces seizures, and thus they play a central role in the pathophysiology of epilepsy in women (Reddy, 2009a, 2013). Progesterone is an intermediate precursor for the synthesis of neurosteroids, which are increased in parallel during the ovarian cycle. There is emerging evidence that endogenous neurosteroids influence seizure susceptibility and epileptogenesis (Reddy, 2011; Reddy and Rogawski, 2012).
Progesterone
Progesterone is an endogenous anticonvulsant hormone with substantial impact on seizure susceptibility. The potential molecular pathways for the progesterone modulation of seizure activity are illustrated in Figure 2. Progesterone is an appealing hormone for prophylactic interventions on epilepsy development, due to its multifunctional modulatory actions in the brain. Progesterone has long been known to have antiseizure activity in animal models (Selye, 1942; Craig, 1966; Kokate et al., 1999a; Frye and Scalise, 2000; Reddy et al., 2004), and in clinical studies (Bäckström et al., 1984; Herzog, 1995, 1999). Women with epilepsy are prone to seizures in response to decreased levels of progesterone during perimenstrual periods (Herzog et al., 1997; Reddy, 2009a). Indeed, the incidence of epilepsy is generally lower in women than in men (Hauser et al., 1993; Christensen et al., 2005; McHugh and Delanty, 2008). This gender difference could be caused by ovarian hormones such as progesterone. Although progesterone is known to inhibit stimulation-evoked seizures in kindling models (Holmes and Weber, 1984; Mohammad et al., 1998; Lonsdale and Burnham, 2003), it has not been investigated widely for potential disease-modifying effect in epileptogenic models. In the kindling model, progesterone has been shown to impair or retard epileptogenesis (Holmes and Weber, 1984; Edwards et al., 2001; Reddy et al., 2010).
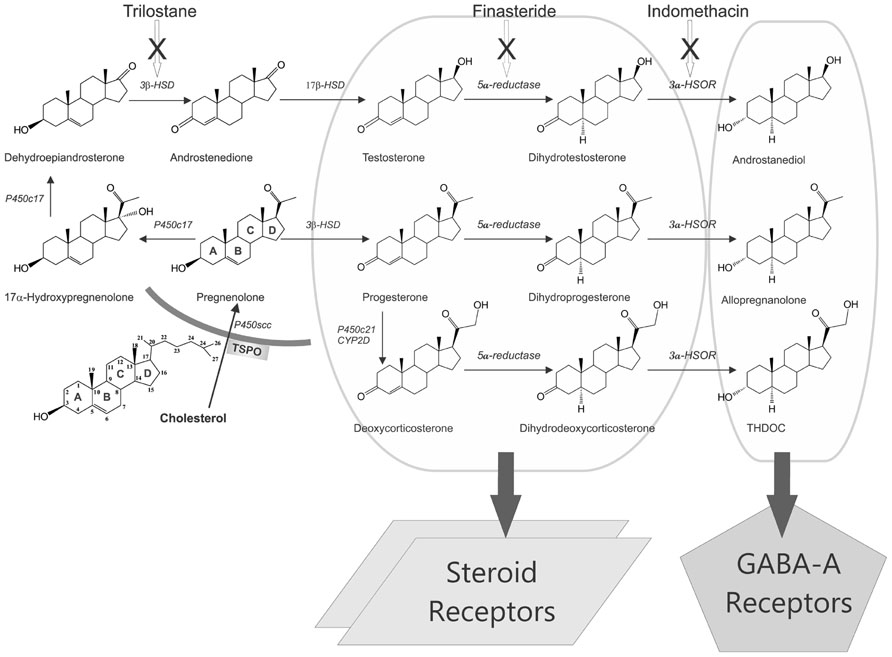
FIGURE 2. Biosynthesis and targets of steroid hormones and neurosteroids in the brain. Enzymatic pathways for the production of three prototype neurosteroids allopregnanolone, THDOC, and androstanediol are illustrated from cholesterol. Steroid hormones progesterone, deoxycorticosterone, and testosterone undergo two sequential A-ring reduction steps catalyzed by 5α-reductase and 3α-HSOR to form the 5α,3α-reduced neurosteroids. The conversion of intermediate precursor steroids into neurosteroids occurs in the hippocampus and several other regions within the brain, where they can affect neuronal function. As evident from the pathways, many modifications are made by the same enzymes, which can be blocked by specific inhibitors (trilostane, finasteride, and indomethacin). There are two mechanisms by which steroid hormones affects neuronal function: (i) binding to steroid receptors (PRs, ARs, or MRs; left panel) and (ii) conversion to GABA-A receptor-modulating neurosteroids (right panel). Progesterone, deoxycorticosterone, or testosterone binding to their cognate steroid receptors could lead to activation of gene expression in the brain. Neurosteroids rapidly modulate neuronal excitability by direct interaction with inhibitory GABA-A receptors in the brain.
Recently progesterone has been evaluated in an National Institutes of Health (NIH)-sponsored, multicenter clinical trial as a treatment for epilepsy in women (Herzog et al., 2012). This randomized, double-blind, placebo-controlled, phase III, multicenter, clinical trial compared the efficacy and safety of adjunctive cyclic natural progesterone therapy versus placebo treatment of intractable seizures in 294 subjects randomized 2:1 to progesterone or placebo, stratified by catamenial and non-catamenial status. The results indicate lack of significant difference in proportions of responders between progesterone and placebo groups. However, a more restricted analysis on a subset of the data found a significantly higher responder rate in women with perimenstrual seizure exacerbation. These findings suggest that progesterone may provide a clinically important benefit for a subset of women with perimenstrual catamenial epilepsy. The dramatic response to progesterone, which is a neurosteroid precursor, in women with perimenstrual catamenial epilepsy is attributable to the unique neurosteroid sensitivity of perimenstrual catamenial seizures (Reddy, 2009a).
Previous studies have shown that progesterone supports the normal development of neurons, and that it reduces the extent of brain damage after TBI (Roof et al., 1994; Stein, 2013). It has been observed in animal models that females have reduced susceptibility to TBI and this protective effect has been hypothesized to be caused by increased circulating levels of progesterone in females (Roof and Hall, 2000; Meffre et al., 2007). A number of additional studies have confirmed that progesterone has neuroprotective effects (Gibson et al., 2008; Singh and Su, 2013). Promising results have also been reported in human clinical trials. Recently, two clinical studies have evaluated progesterone as a treatment for moderate to severe TBI (Wright et al., 2007; Xiao et al., 2008). These studies demonstrated the efficacy of progesterone as a neuroprotective agent in TBI. Progesterone is highly efficacious in reducing disability and death in TBI. Progesterone has neuroprotective properties in acute models of ischemic injury, stroke, and astroglial dysfunction (Koenig et al., 1995; Jiang et al., 1996; He et al., 2004), suggesting its beneficial effects in brain injury.
Progesterone targets multiple molecular and cellular mechanisms relevant to epileptogenesis, and may therefore be a natural disease-modifying agent. Progesterone’s cellular actions are mediated by the progesterone receptors (PRs), which are expressed in the hypothalamus, neocortex, hippocampus, and limbic areas (Brinton et al., 2008). Progesterone is an intermediate precursor for the synthesis of neurosteroids (see Role of Neurosteroids in Epileptogenesis). Progesterone’s antiseizure activity is mediated mainly by its conversion to allopregnanolone, a neurosteroid and positive modulator of GABA-A receptors with broad-spectrum antiseizure properties (Belelli et al., 1989; Kokate et al., 1999a; Frye et al., 2002; Kaminski et al., 2004; Reddy et al., 2004). Moreover, neurosteroids can modulate PRs via intracellular metabolism to analogs that bind to PRs (Rupprecht et al., 1993). Progesterone may modulate signaling cascades of inflammation, apoptosis, neurogenesis, and synaptic plasticity (Patel, 2004; Vezzani, 2005; Stein and Sayeed, 2010), and therefore, progesterone may directly exert disease-modifying effects on epileptogenesis.
Recently, progesterone has been tested in rodent models of hippocampus epileptogenesis (Reddy et al., 2010; Reddy and Mohan, 2011; Reddy and Ramanathan, 2012). At low, non-sedative doses, progesterone treatment for 2 weeks significantly suppressed the rate of development of kindled seizure activity evoked by daily hippocampus stimulation in mice, indicating a disease-modifying effect of progesterone on limbic epileptogenesis. There was a significant increase in the rate of “rebound or withdrawal” kindling during drug-free stimulation sessions following abrupt discontinuation of progesterone treatment. A washout period after termination of progesterone treatment prevented such acceleration in kindling. The molecular mechanisms underlying progesterone’s attenuating effect on kindling development remains unknown. There are several potential mechanisms by which progesterone could inhibit epileptogenesis, including activation of PRs, synthesis of neurosteroids, modulation of oxidative cascades, and promoting neuroprotection. The effect of progesterone on the early kindling progression is reduced in mice lacking PRs, which provide evidence that PRs may be partly involved in progesterone’s disease-modifying effects (Reddy et al., 2010). These findings are consistent with the role of PRs in progesterone inhibition of epileptiform activity in the hippocampus (Edwards et al., 2000). Despite the early attenuation, late stage kindling progressed normally in the PRKO mice, suggesting that PRs are not involved in the later part of the kindling progression. However, the extent to which PRs mediate the progesterone’s disease-modifying effect remains unclear. Collectively, the disease-modifying effect of progesterone may occur through a complex mechanism partly involving PR-dependent and PR-independent pathways.
Progesterone is rapidly metabolized into neurosteroids pregnanolone and allopregnanolone, which could mediate progesterone’s attenuating effects on kindling epileptogenesis. This possibility is supported by emerging evidence that neurosteroids can retard the development of spontaneous seizures in post-SE models of epileptogenesis (Biagini et al., 2006, 2009). It is suggested that 5α-reductase converts progesterone to allopregnanolone and related neurosteroids that retard epileptogenesis. To further test this hypothesis, we utilized the mouse hippocampus kindling model of epileptogenesis and investigated the effect of finasteride, a 5α-reductase and neurosteroid synthesis inhibitor (Reddy and Ramanathan, 2012). In a kindling model in adult mice, pre-treatment with finasteride significantly blocked progesterone’s inhibition of epileptogenesis (Reddy and Mohan, 2011), and led to complete inhibition of the progesterone-induced retardation of limbic epileptogenesis in mice (Reddy and Ramanathan, 2012). Therefore, neurosteroids such as allopregnanolone may mediate the disease-modifying effect of progesterone in the kindling model (see Role of Neurosteroids in Epileptogenesis).
In addition, progesterone’s modulation of inflammation is suggested as an appealing mechanism because pro-inflammatory molecules and oxidative signaling has been found to be activated in animal models of epilepsy (Patel, 2004; Vezzani, 2005). Progesterone has pleiotropic effects on inflammation and cell growth/survival (He et al., 2004; Stein and Sayeed, 2010) that may contribute to its attenuating effects on epileptogenesis. Progesterone inhibits secreted phospholipase A2 enzyme, a very high level target in the inflammatory cascade that has been shown to induce neurodegeneration through glutamate release (DeCoster et al., 2002; Yagami et al., 2002). Progesterone has been shown in numerous preclinical models to be neuroprotective after injury (Roof et al., 1994; Koenig et al., 1995; Jiang et al., 1996; Cutler et al., 2007). Recently, two clinical studies have evaluated progesterone as a treatment for moderate to severe TBI (Wright et al., 2007; Xiao et al., 2008). The progesterone was administered over a period of 3 or 5 days beginning within 8 or 11 h of the injury. In both studies, the groups receiving progesterone had significantly fewer deaths than those receiving placebo. In addition, there was evidence of improved functional outcomes in the progesterone-treated groups, suggesting that progesterone is highly efficacious in reducing morbidity and mortality in TBI, which is a leading cause of epilepsy in adults and military persons.
Testosterone
Testosterone has marked impact on seizure susceptibility. The potential biosynthetic pathways of testosterone metabolism are illustrated in Figure 2. Testosterone is known to produce both proconvulsant and anticonvulsant effects depending on the animal model and the seizure type (Reddy, 2008). Both animal and clinical studies show that testosterone enhances seizure activity by metabolism to estrogens (Isojarvi et al., 1988; Thomas and Yang, 1991; Herzog et al., 1998; Edwards et al., 1999; El-Khayat et al., 2003). Epidemiological data indicate that the occurrence of focal and tonic–clonic epileptic seizures is ~50% higher in intact than in castrated dogs (VMDB Report, 2003). On the contrary, testosterone and related androgens have protective effects against seizures induced by pentylenetetrazol and kainic acid (Schwartz-Giblin et al., 1989; Frye and Reed, 1998; Frye et al., 2001a,b; Reddy, 2004b). Moreover, studies in orchidectomized or castrated animals have shown that decreased testosterone is associated with higher incidence of seizures and replacement with testosterone attenuates seizures (Grigorian and Khudaverkian, 1970; Thomas and McLean, 1991; Pericić et al., 1996; Pesce et al., 2000). It is demonstrated that testosterone modulation of seizure susceptibility occurs through its conversion to neurosteroids with “anticonvulsant” and “proconvulsant” actions, and hence the net effect of testosterone on neural excitability and seizure activity depends on the levels of distinct testosterone metabolites within the brain (Reddy, 2004a,b). Unlike estradiol, which generally facilitates seizures (Bäckström, 1976; Hom and Buterbaugh, 1986; Buterbaugh, 1989; Woolley, 2000), androstanediol has been shown to produce powerful antiseizure effects (Reddy, 2004b; Kaminski et al., 2005). Testosterone might have a biphasic effect on seizures: proconvulsant at higher doses, anticonvulsant at lower doses. However, testosterone itself has not been reported to improve seizures clinically (Herzog et al., 1998). Reductions of seizures were observed only when testosterone was given together with an estrogen synthesis inhibitor (Herzog et al., 1998), suggesting the estradiol modulation of seizure activity.
In many men with epilepsy, testosterone deficiency is an unusually common clinical observation (Macphee et al., 1988; Herzog, 1991; El-Khayat et al., 2003). TLE surgery has been shown to reduce seizure occurrence and normalize serum androgen concentrations in men with epilepsy (Bauer et al., 2000). Alterations in testosterone levels, therefore, may possibly contribute to exacerbation of seizures. The introduction of finasteride (Propecia), which inhibits dihydrotestosterone and androstanediol synthesis, for the treatment of male pattern baldness led to recurrent seizures, which then subsided once the drug was discontinued. Finasteride-induced seizure exacerbation has also been reported recently (Herzog and Frye, 2003). There is a new case report implicating endogenous neurosteroids in TLE (Pugnaghi et al., 2013). Two-week phenytoin treatment has been shown to affect the hippocampal levels of testosterone, cytochrome P450 (CYP) isoforms, and androgen receptor (AR) expression (Meyer et al., 2006). The increased metabolism of testosterone leading to augmented androgen metabolite formation most likely led to enhanced expression of CYP19 and AR in hippocampus, which is a critical area for limbic epileptogenesis.
Aromatase is the key enzyme for the conversion of testosterone to estradiol, a neuroactive steroid that promotes seizures. Aromatase is expressed in discrete areas in the brain such as hippocampus and neocortex that are involved in epileptogenesis. Aromatase inhibitors could decrease brain excitability by decreasing local estradiol levels and therefore, could be beneficial for the treatment of epilepsy (MacLusky et al., 1994). Consequently, aromatase inhibitors have been proposed as a suitable approach to seizure therapy in some men with epilepsy. Some aromatase inhibitors have been tested in men with epilepsy: testolactone, letrozole, and anastrozole. Herzog et al. (1998) tested the efficacy of testosterone and testolactone in men with intractable complex partial seizures. Improvement in seizure control was reportedly achieved with testosterone therapy when testosterone was used along with testolactone. In a case report, letrozole has been shown to improve seizure control in a 61-year-old man with epilepsy (Harden and MacLusky, 2004, 2005). In a pilot study, the safety and efficacy of add-on anastrozole therapy was tested in men with intractable epilepsy. Men with the greatest seizure reduction showed unexpectedly elevated levels in FSH, a pituitary-derived gonadotropin. Hence, the outcome of trials with three distinct aromatase inhibitors – testolactone, letrozole, and anastrozole – suggests a beneficial treatment modality for men with epilepsy (Harden and MacLusky, 2005). Therefore, it is likely that aromatase inhibitors could be potential agents for interruption of proepileptogenic estrogens in the brain.
Estrogens
Although estrogens can affect seizure susceptibility, the role of various estrogens in epileptogenesis is poorly understood. In general, estrogens have proconvulsant and epileptogenic properties in animals and humans (Scharfman and MacLusky, 2006). There are limited studies that support protective effects of estrogens, but it may act as a anticonvulsant under some conditions (Velíšková, 2006). Estradiol has been widely investigated in animal epilepsy models. The effect of estrogens on seizure susceptibility is highly variable and depends on factors such as treatment duration, dosage, hormonal status, and the seizure model (Velíšková, J., and Velísek, 2007). Early studies of estradiol administration to ovariectomized rats revealed proconvulsant effects (Reddy, 2009a). The effect of estrogens on hippocampus seizure susceptibility is controversial (Scharfman and MacLusky, 2006). While estradiol has been shown to be proconvulsant in several studies, there is also evidence that support lack of effect or protective effect of estrogens (Reibel et al., 2000; Velíškováx et al., 2000; Velíšková, J., and Velísek 2007). The effect of circulating estrogens has been studied in female rats with epilepsy (Scharfman et al., 2008, 2009). Epileptic female rats show cyclic increases in epileptiform activity in EEG recordings that coincide with their ovarian cycle, mostly attributable to estrogens.
Estradiol has been known to play a role in the exacerbation of seizures in women with epilepsy (Logothetis et al., 1959; Bäckström, 1976; Jacono and Robinson, 1987). Plasma estradiol levels are found to increase during both the follicular and luteal phase of the normal menstrual cycle. Bäckström (1976) was the first investigator to characterize the relationship between seizures and steroid hormones. In women with epilepsy, a positive correlation between seizure susceptibility and the estrogen-to-progesterone ratio was observed, peaking in the premenstrual and preovulatory periods and declining during the midluteal phase. Logothetis et al. (1959) have demonstrated that intravenous infusions of estrogen were associated with rapid interictal epileptiform activity in women with epilepsy, and seizures were exacerbated when estrogen was given premenstrually. Therefore, it is hypothesized that estrogens may facilitate some forms of catamenial seizures observed during these phases. The periovulatory catamenial exacerbation has been attributed to the midcycle surge of estrogen that is relatively unopposed by progesterone until early luteal phase (Logothetis et al., 1959). An increase in the ratio of estrogen-to-progesterone levels during perimenstrual period might at least partly contribute to the development of perimenstrual catamenial epilepsy (Bonuccelli et al., 1989; Herzog et al., 1997). A recent report from the Nurses’ health study in 114,847 nurses identified key factors associated with seizures in women with epilepsy (Dworetzky et al., 2012). Menstrual irregularity at ages 18–22 years was specifically associated with an increased risk of epilepsy. Menstrual irregularity during follow-up and early age at menarche increased the risk of isolated seizures. Oral contraceptive uses are not associated with isolated seizure or epilepsy.
Glucocorticoids
Pituitary–adrenal hormones have long been known to affect epileptogenesis (Aird and Gordan, 1951; Rose et al., 1979; Weiss et al., 1993; Joëls, 2009; Borekci et al., 2010). Acute stress raises seizure threshold in animals, but chronic stress is known to be a clear risk factor for precipitating seizures in patients with epilepsies. Stress increases plasma and brain concentrations of corticosteroids and neurosteroids. Acute physical or psychological stress causes increased production of hypothalamic corticotrophin releasing hormone (CRH), which is transported via hypophyseal portal system to the pituitary, where it increases both adrenocorticotropic hormone (ACTH) synthesis and secretion. Major physiological effects result from ACTH’s action on adrenal cortex to increase the circulating levels of corticosteroids, principally the glucocorticoid cortisol, and the mineralocorticoid DOC. Cortisol is a major corticosteroid secreted from the adrenal cortex. Cortisol is an excitatory steroid. It elicits proconvulsant and epileptogenic effects (Roberts and Keith, 1995; Joëls, 1997). DOC elicits inhibitory effects and protects against seizures (Reddy, 2003b). An imbalance in cortisol and DOC and other corticosteroids may contribute to susceptibility or resistance to epileptogenesis.
Stress enhances epileptogenesis. In general, chronic or repeated stress has been shown to enhance vulnerability to epileptogenesis in animal models (Joëls, 2009). Corticosterone, the major adrenal steroid in rodents, has been tested extensively in animal models (Weiss et al., 1993; Kumar et al., 2007, 2011; Joëls, 2009; Borekci et al., 2010; Desgent et al., 2012). Prolonged exposure to elevated corticosterone, used as a model of chronic stress, accelerates limbic epileptogenesis (Kumar et al., 2007). Exposure to repeated experimental stress accelerates the development of limbic epileptogenesis, an effect which may be related to elevated corticosterone levels (Jones et al., 2013). Chronic low-dose corticosterone supplementation is shown to enhance epileptogenesis in the rat amygdala kindling model (Kumar et al., 2007). Episodic corticosterone treatment elicits a striking acceleration in kindling epileptogenesis and triggers long-term changes in hippocampal CA1 neurons (Karst et al., 1999). Overall, corticosterone – with other stress hormones – rapidly enhances CA1/CA3 hippocampal activity shortly after stress and could imposes a risk for neuronal injury, such as during epileptic activity. In the hippocampus, stress-induced elevations in neurosteroids promote inhibitory tone mediated through GABA-A receptors. Under conditions of repetitive stress, hormonal influences on the inhibitory tone might diminish and instead, increased excitation become more apparent. In agreement, perinatal stress and elevated corticosteroid levels accelerate epileptogenesis and lower seizure threshold in rodent epilepsy models (Salzberg et al., 2007; Lai et al., 2009; Desgent et al., 2012). Therefore, exposure to stressful events during a critical phase in epileptogenesis could impose lasting deleterious effects on the course of epilepsy.
Deoxycorticosterone, a mineralocorticoid precursor with anesthetic and antiseizure properties, is also produced in the adrenal zona fasciculate. Although the antiseizure properties of DOC in human were first described in 1944 (Aird, 1944; Aird and Gordan, 1951), the mechanisms underlying the brain actions of DOC were only recently identified. The antiseizure activity of DOC requires its enzymatic conversion to 3α,21-dihydroxy-5α-pregnan-20-one (THDOC), a neurosteroid that is a powerful positive allosteric modulator of GABA-A receptors (Reddy, 2003b; Figure 2). THDOC is released during physiological stress nearly exclusively from adrenal sources (Purdy et al., 1990; Reddy, 2003b). Plasma and brain levels of THDOC rise rapidly following acute stress (Purdy et al., 1991; Concas et al., 1998; Reddy and Rogawski, 2002). Acute stressors such as swimming, foot shock, or carbon dioxide exposure elicit an increase in allopregnanolone and THDOC concentrations in plasma and in brain (Barbaccia et al., 1996, 1997; Vallée et al., 2000). Stress-induced THDOC and neurosteroids have been demonstrated to elevate seizure threshold (Reddy and Rogawski, 2002) and contributes to neuroprotection (Reddy, 2003b, 2006).
11-Deoxycortisol (pregn-4-ene-17,21-diol-3,20-dione; DC) is an immediate precursor of cortisol. DC acts as a competitive antagonist of glucocorticoid receptor in vitro, but is ineffective as a glucocorticoid antagonist in vivo due to adrenal 11-hydroxylation (Cutler et al., 1979). Nearly 50 years ago, Heuser and Eidelberg (1961) observed that systemic administration of large doses of DC succinate induces long-lasting seizure activity in rats and cats. The mechanism underlying pro-epileptic properties of DC has been studied recently (Kaminski et al., 2011). DC is capable of inducing long-lasting status epilepticus in rodents that is refractory to several anticonvulsant drugs. In electrophysiological studies, DC is shown to accelerate the decay time of the inhibitory post-synaptic currents mediated by GABA-A receptors in brain slices, indicating that it significantly impedes GABAergic inhibition which may lead to paroxysmal epileptiform network activity and convulsive seizures. Because DC is an endogenous substance, it is suggested to contribute to an increased seizure propensity in some clinical situations. However, the specific role of DC to the pathophysiology of epileptogenesis is remains unclear.
Physical activity has been suggested as a positive disease-modifying factor for preventing or delaying the development of epilepsy. Exercise has beneficial effects on epileptogenesis (Arida et al., 1998, 2007, 2010; Silva de Lacerda et al., 2007). Exercise treatment reduced brain susceptibility in the kindling or the pilocarpine model of epilepsy. Behavioral analysis showed a reduced frequency of seizures during physical exercise program. Metabolic, electrophysiological, and immunohistochemical studies have confirmed the positive influence of exercise on epilepsy (Arida et al., 1999, 2007). Although a variety of factors can contribute to such favorable responses, the mechanisms remain poorly understood. Dendritic plasticity, increased neurogenesis, induction of trophic factors and release of neurosteroids are some factors underlying the inhibitory effects of exercise on epileptogenesis. Enrichment of environment has been shown to delay kindling epileptogenesis in rats (Auvergne et al., 2002). It is likely that endogenous neurosteroids may be involved in the neuroprotective effects of exercise and enriched environment. Thus, prevention of loss of interneurons, reduced GABA-A receptor plasticity, and decrease in axonal sprouting could contribute to the disease-modifying effect of exercise and enriched environment.
Despite acute stress-induced seizure protection in animals (Pericić et al., 2000; Reddy and Rogawski, 2002), patients, and clinicians are not likely to recognize a reduction in seizure frequency associated with stress. It is well known that emotional factors can affect seizure control (Temkin and Davis, 1984). In general, stressful events are associated with more frequent epileptiform spikes and seizures (Frucht et al., 2000). Indeed, stress has been reported to trigger seizure activity in persons with epilepsy (Temkin and Davis, 1984; Frucht et al., 2000). During stressful episodes adrenal hormone levels are expected to fluctuate, possibly affecting epileptogenic events. In agreement with this hypothesis, perinatal stress and elevated steroid levels have been shown accelerate epileptogenesis and lower seizure threshold in various animal models for epilepsy (Joëls, 2009).
Acute stress has anticonvulsant-like effects, while chronic stress is known to induce epileptic seizures. How can such contradictory observations be reconciled? Although the exact pathophysiology of possible seizure facilitation by stress is unknown, there are certainly many neural and endocrine pathways through which stress can alter neuronal excitability and thereby affect seizure susceptibility. The extent of seizure susceptibility during stress might therefore represent a balance between anticonvulsant (e.g., neurosteroids) and proconvulsant factors (e.g., glucocorticoids and CRH). Stress-induced seizures would thus occur when the balance is shifted to favor the proconvulsant factors, surpassing the anticonvulsant action of endogenous neurosteroids (Reddy, 2006). Although little is known regarding proconvulsant factors, stress can increase brain levels of “proconvulsant” sulfated neurosteroids such as pregnenolone sulfate (PS) and dehydroepiandrosterone sulfate (DHEAS; see Role of Neurosteroids in Epileptogenesis). Additionally, repeated episodes of stress and neurosteroid release might lead to a sort of neurosteroid withdrawal-induced hyperexcitable state (Reddy et al., 2001, 2012) and could predispose patients to stress-induced seizures. Nevertheless, alleviating the effects of stress by pharmacological interventions may help reduce the epileptogenicity in people with risk factors for epilepsy.
Role of Neurosteroids in Epileptogenesis
Neurosteroids are steroids synthesized within the brain with unconventional rapid effects on neuronal excitability. It is well known that steroid hormones such as progesterone and DOC can exert anticonvulsant actions (Selye, 1941; Clarke et al., 1973). The anticonvulsant properties of progesterone and DOC are predominantly due to their conversion in the brain to neurosteroids allopregnanolone (3α-hydroxy-5α-pregnane-20-one, AP) and allotetrahydrodeoxycorticosterone (THDOC), respectively (Reddy, 2003a; Reddy et al., 2004; Figure 2). A variety of neurosteroids are known to be synthesized in the brain (Baulieu, 1981; Kulkarni and Reddy, 1995). The most widely studied are AP, THDOC, and androstanediol. These neurosteroids are produced via sequential A-ring reduction of the steroid hormones by 5α-reductase and 3α-hydroxysteroid-oxidoreductase (3α-HSOR) isoenzymes (Reddy, 2009a). The androgenic neurosteroid androstanediol (5α-androstan-3α,17β-diol; Figure 2) is synthesized from testosterone (Reddy, 2004a,b).
In the periphery, the steroid precursors are mainly synthesized in the gonads, adrenal gland, and feto-placental unit, but synthesis of these neurosteroids likely occurs in the brain from cholesterol or from peripherally derived intermediates. Since neurosteroids are highly lipophilic and can readily cross the blood–brain barrier, neurosteroids synthesized in peripheral tissues accumulate in the brain (Reddy and Rogawski, 2010). Recent evidence indicates that neurosteroids are present mainly in principal neurons in many brain regions that are relevant to focal epilepsies, including the hippocampus and neocortex (Agís-Balboa et al., 2006; Saalmann et al., 2007; Do Rego et al., 2009). The biosynthesis of neurosteroids is controlled by the translocator protein (18 kDa; TSPO), formerly called peripheral or mitochondrial benzodiazepine receptor (Rupprecht et al., 2009, 2010). Activation of TSPO by endogenous signals and ligands facilitates the intramitochondrial flux of cholesterol and thereby promotes neurosteroid synthesis. It is suggested that TSPO ligands might be an alternative approach for neurosteroid therapeutics (Nothdurfter et al., 2011). Currently, synthetic analogs of endogenous neurosteroids are under clinical trial for treatment of epilepsy (Reddy and Rogawski, 2010, 2012).
Potentiation of Phasic and Tonic Inhibition
Neurosteroids rapidly alter neuronal excitability through direct interaction with GABA-A receptors (Harrison and Simmonds, 1984; Majewska et al., 1986; Harrison et al., 1987; Gee et al., 1988; Purdy et al., 1990; Hosie et al., 2007, 2009), which are the major receptors for the inhibitory neurotransmitter GABA. Activation of the GABA-A receptor by various ligands leads to an influx of chloride ions and to a hyperpolarization of the membrane that dampens the excitability. Allopregnanolone and other structurally related neurosteroids act as positive allosteric modulators and direct activators of GABA-A receptors (Figure 3). At low concentrations, neurosteroids potentiate GABA-A receptor currents, whereas at higher concentrations, they directly activate the receptor (Harrison et al., 1987; Reddy and Rogawski, 2002). Like barbiturates, neurosteroid enhancement of GABA-A receptors occurs through increases in both the channel open frequency and channel open duration (Twyman and Macdonald, 1992; Lambert et al., 2009; Ramakrishnan and Hess, 2010). The GABA-A receptor is a pentamer consisting of five subunits that form a chloride channel. Sixteen subunits (α1-6, β1-3, γ1-3, δ, ε, θ, and π subunits) have been identified so far. The GABA site is located at the interface between α and β subunits. Benzodiazepines bind at the interface between α and γ subunits and they interact with subunit combinations α1,2,3,5β2γ2.
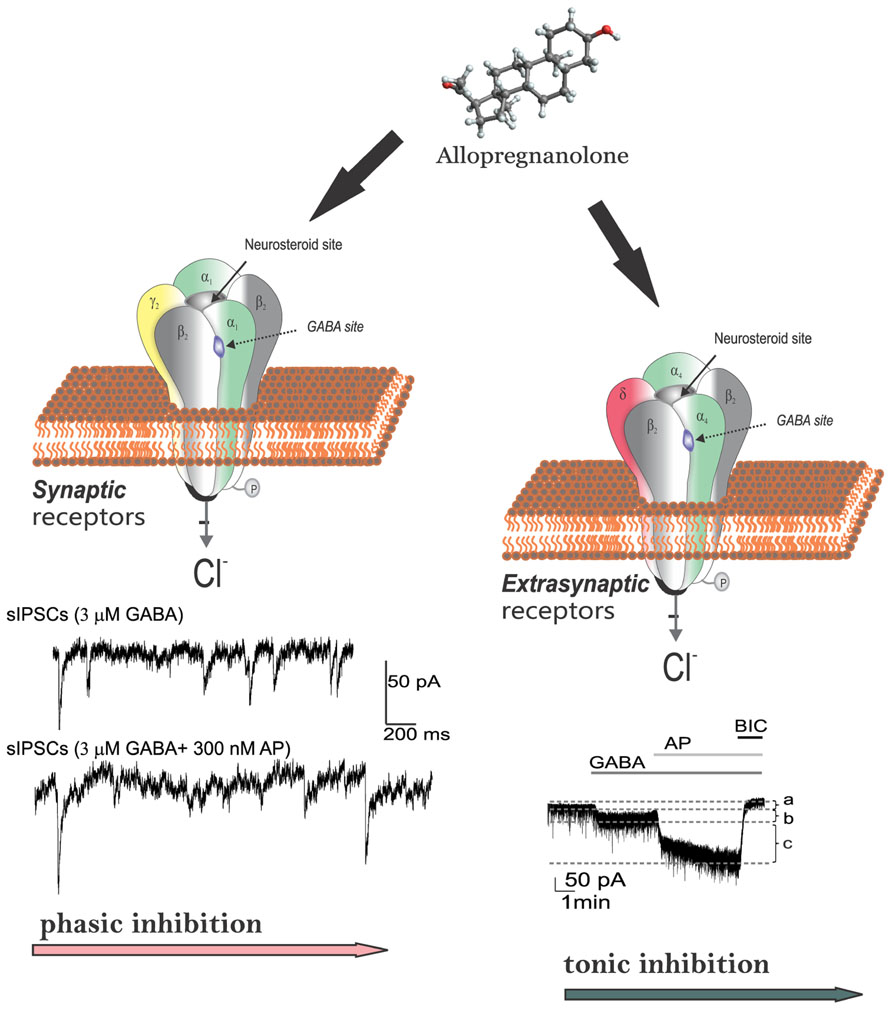
FIGURE 3. Neurosteroid modulation of GABA-A receptors in the brain. Allopregnanolone and related neurosteroids binds and enhances GABA-A receptor-mediated inhibition in the brain. GABA-A receptors are pentameric with five protein subunits that form the chloride ion channel pore. Neurosteroids directly binds to the “neurosteroid binding sites” and potentiate the GABA-gated chloride currents. The neurosteroid binding sites are distinct from sites for GABA, benzodiazepines, and barbiturates. There are two types of GABA-A receptors with different functions. Post-synaptic GABA-A receptors, which are pentameric chloride channels composed of 2α2βγ subunits, mediate the phasic portion of GABAergic inhibition, while extrasynaptic GABA-A receptors, pentamers composed of 2α2βδ subunits, primarily contribute to tonic inhibition in the hippocampus. Neurosteroids activate both synaptic and extrasynaptic receptors and enhance the phasic and tonic inhibition, and thereby promote maximal network inhibition in the brain.
Activation of GABA-A receptors produces two forms of inhibition: phasic inhibition generated by the rapid, transient activation of synaptic GABA-A receptors by presynaptic GABA release, and tonic inhibition generated by the persistent activation of extrasynaptic GABA-A receptors, which can detect extracellular GABA (Figure 3). There are major differences between synaptic and extrasynaptic GABA-A receptors (Table 3). The extrasynaptic GABA-A receptors are distributed within the hippocampus (α4βδ, α5βδ, or α1βδ), neocortex (α4βδ, α5βδ), thalamus (α4βδ), striatum (α4βδ), hypothalamus (α4βδ), and cerebellum (α6βδ). Although GABA activates synaptic (γ2-containing) GABA-A receptors with high-efficacy, GABA activation of the extrasynaptic (δ-containing) GABA-A receptors are limited to low-efficacy activity characterized by minimal desensitization and brief openings. Such tonic currents are particularly evident in dentate granule cells, which play a major role in hippocampus excitability. The high sensitivity of δ-containing receptor channels to neurosteroid modulation may be dependent on the δ-subunit or the low-efficacy channel function that it confers. There is evidence that neurosteroids preferentially enhance low-efficacy GABA-A receptor activity independent of subunit composition (Bianchi and Macdonald, 2003).
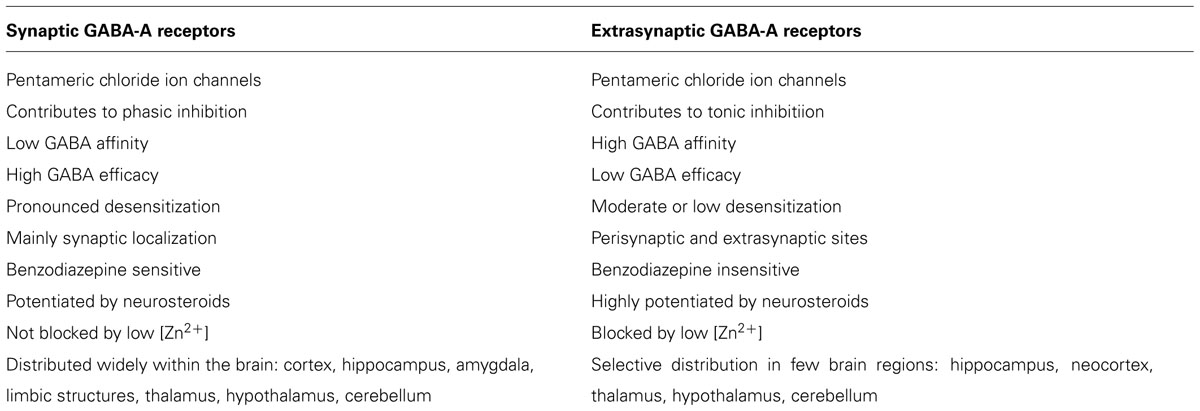
TABLE 3. An overview of synaptic (αβγ2-containing) and extrasynaptic (αβδ-containing) GABA-A receptors in the brain.
The effect of neurosteroids on GABA-A receptors occurs by binding to discrete sites on the receptor–channel complex that are located within the transmembrane domains of the α- and β-subunits (Hosie et al., 2006, 2007), which they access by lateral membrane diffusion (Chisari et al., 2009, 2010; Figure 3). The binding sites for neurosteroids are distinct from the recognition sites for GABA, benzodiazepines, and barbiturates (Hosie et al., 2009). Androgenic neurosteroids such as androstanediol may interact with these sites, and a recent study indicates that this agent is a positive allosteric modulator of GABA-A receptors (Reddy and Jian, 2010). Although neurosteroids act on all GABA-A-receptor isoforms, they have large effects on extrasynaptic δ-subunit containing GABA-A receptors that mediate tonic currents (Belelli et al., 2002; Wohlfarth et al., 2002). The potentiation of δ-subunit-containing receptors by THDOC and other neurosteroids is selective for channels with low-efficacy gating characteristics marked by brief bursts and channel openings in conditions of both low and high GABA concentrations, and neurosteroids can thereby preferentially increase the efficacy of these receptors based on pharmacokinetics which are not yet fully understood (Bianchi and Macdonald, 2003). Neurosteroids therefore markedly enhance the current generated by δ-subunit-containing receptors even in the presence of saturating GABA concentrations. Consequently, GABA-A receptors that contain the δ-subunit are highly sensitive to neurosteroid potentiation and mice lacking δ-subunits show drastically reduced sensitivity to neurosteroids (Mihalek et al., 1999; Spigelman et al., 2002). Tonic current causes a steady inhibition of neurons and reduces their excitability. Neurosteroids therefore could play a role in setting the level of excitability by potentiation of tonic inhibition during seizures when ambient GABA rises (Stell et al., 2003).
The pharmacological profile of major neurosteroids is outlined in Table 4. Allopregnanolone-like neurosteroids are powerful antiseizure agents. Exogenously administered neurosteroids exhibit broad-spectrum anticonvulsant effects in diverse rodent seizure models (Reddy, 2010). Neurosteroids protect against seizures induced by GABA-A receptor antagonists, including pentylenetetrazol and bicuculline, and are effective against pilocarpine-induced limbic seizures and seizures in kindled animals (Belelli et al., 1989; Kokate et al., 1994; Frye, 1995; Wieland et al., 1995; Reddy and Rogawski, 2001, 2010; Kaminski et al., 2004, 2005; Reddy et al., 2004). Like other GABAergic agents, they may exacerbate generalized absence seizures (Snead, 1998; Citraro et al., 2006). The potencies of neurosteroids in models where they confer seizure protection vary largely in accordance with their activities as positive allosteric modulators of GABA-A receptors (Reddy, 2004a,b; Kaminski et al., 2005). Like other GABAergic agents, neurosteroids are inactive or only weakly active against seizures elicited by maximal electroshock. Neurosteroids are highly active in the 6-Hz model, a better paradigm in which limbic-like seizures are induced by electrical stimulation of lower frequency and longer duration than in the maximal electroshock test (Kaminski et al., 2004). Androstanediol, but not its 3β-epimer, produced a dose-dependent suppression of behavioral and electrographic seizures in the mouse hippocampus kindling (Reddy and Jian, 2010). In addition, neurosteroids are also highly effective in suppressing seizures due to withdrawal of GABA-A receptor modulators including neurosteroids and benzodiazepines, as well as other types of agents such as ethanol and cocaine (Devaud et al., 1996; Tsuda et al., 1997; Reddy and Rogawski, 2001; Gangisetty and Reddy, 2010). In contrast to benzodiazepines, where utility in the chronic treatment of epilepsy is limited by tolerance, anticonvulsant tolerance is not evident with neurosteroids (Kokate et al., 1998; Reddy and Rogawski, 2000), which indicate that neurosteroids are more effective than benzodiazepines for long-term treatment. Novel therapeutic approaches are being developed based on the emerging information on neurosteroid interaction with GABA-A receptors (Reddy and Rogawski, 2009; Murashima and Yoshii, 2010).
Antiepileptogenic Activity
Neurosteroids may play a role in chronic epilepsy. Neurosteroid modulation of tonic activation of extrasynaptic GABA-A receptors can regulate excitability during epileptogenicity. Given the complex plasticity in GABA-A receptors in epilepsy, it is difficult to predict the functional outcome of altered subunit compositions. A consistent finding from studies that have used various models of chronic epilepsy is that tonic conductances are largely preserved in epileptic brain around the time when synaptic inhibition is reduced (Mtchedlishvili et al., 2001; Sun et al., 2007; Zhang et al., 2007). Studies in a status epilepticus model of TLE have shown a striking reduction in δ-subunit containing GABA-A receptors in the dentate gyrus (Peng et al., 2004; Zhang et al., 2007), suggesting that neurosteroid effects on non-synaptic GABA-A receptors may be reduced. There was a compensatory increase in γ2-subunit, so that tonic inhibition is preserved though the efficacy of THDOC in modulating tonic current is decreased. In addition, neurosteroid modulation of synaptic currents is diminished in dentate gyrus granule cells and α4-subunit-containing receptors are expressed at synaptic sites (Sun et al., 2007). All of these changes may exacerbate seizures in epileptic animals and reduce the potency but not efficacy of endogenous neurosteroids. The expression of neurosteroidogenic enzymes such as P450scc and 3α-HSOR appears to be elevated in the hippocampus in animals and human subjects affected by TLE (Stoffel-Wagner et al., 2000; VMDB Report, 2003; Biagini et al., 2009). If local neurosteroidogenesis is enhanced, this may in part counteract the epileptogenesis-induced changes.
There is emerging evidence that endogenous neurosteroids play a role in regulating epileptogenesis (Edwards et al., 2001; Biagini et al., 2006, 2009, 2010; Reddy et al., 2010; Figure 4). Using the kindling model, we demonstrated that the development and persistence of limbic epileptogenesis are impaired in mice lacking PRs (Reddy and Mohan, 2011). To explore mechanisms underlying the observed seizure resistance, we investigated the role of neurosteroids using finasteride, a 5α-reductase inhibitor that blocks the synthesis of progesterone-derived neurosteroids. We determined the rate of rapid kindling in both control animals and those which had received injections of progesterone with or without concurrent finasteride treatment (Reddy and Mohan, 2011). Progesterone produced a significant delay in the rate of kindling and pretreatment with finasteride blocked progesterone’s inhibition of kindling epileptogenesis (Reddy and Ramanathan, 2012). These findings are consistent with a contributory role of neurosteroids in limbic epileptogenesis. Thus, it is possible that inhibition of neurosteroids could incite mechanisms that may promote epileptogenesis.
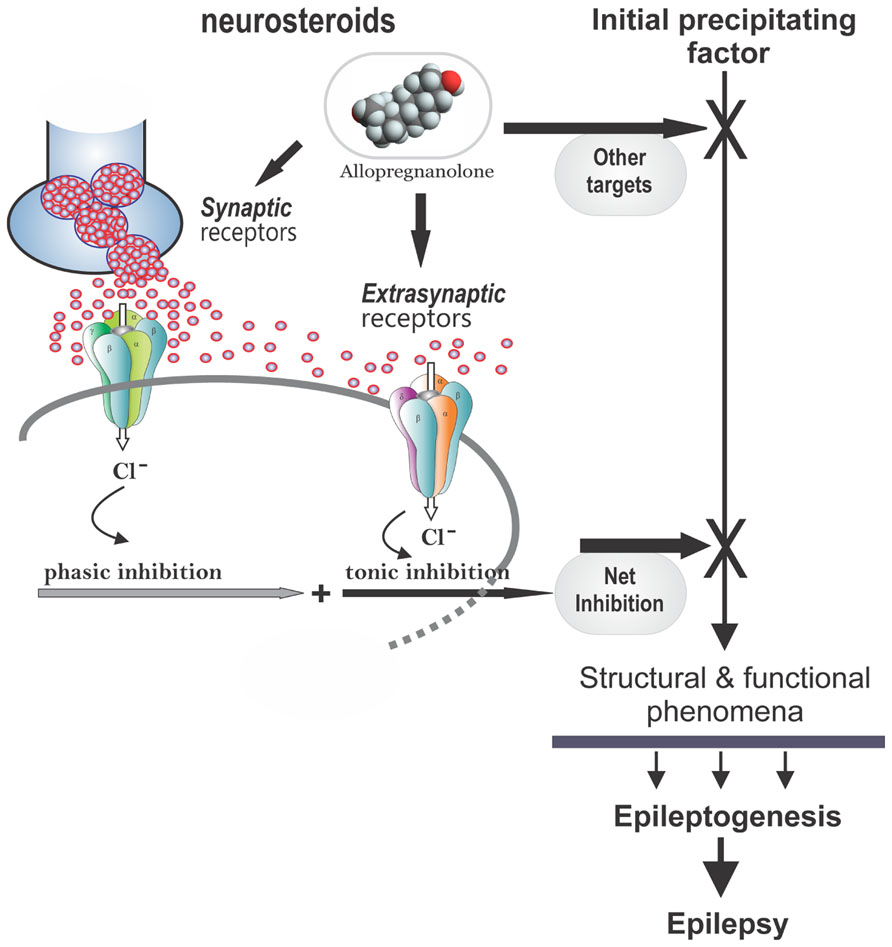
FIGURE 4. Potential molecular mechanisms of neurosteroid interruption of epileptogenesis. In the brain, allopregnanolone and related neurosteroids may retard epileptogenesis by the interruption of one or more of the pathways leading to development of epilepsy, which generally occurs following an initial precipitating event. Neurosteroids such as allopregnanolone binds to synaptic and extrasynaptic GABA-A receptors and enhances phasic and tonic inhibition within the brain, and thereby may affect epileptogenesis. Other potential mechanisms include modulation of neuroinflammation and neurogenesis in the brain.
The P450sc is a critical enzyme for the biosynthesis of neurosteroids (Figure 2). It is present in neurons, oligodendrocytes, astrocytes, and activated microglia. Following pilocarpine-induced status epilepticus in the rat, the neurosteroidogenic enzyme P450scc is upregulated for several weeks, suggesting that it may be associated with promotion of neurosteroidogenesis (Biagini et al., 2009). Ordinarily, rats develop spontaneous recurrent seizures following a latent period of similar duration to the period during which P450scc is elevated. The role of neurosteroids in delaying seizure onset in the pilocarpine model is confirmed using finasteride, which can exacerbate seizures by inhibition of neurosteroid synthesis. Inhibiting neurosteroid synthesis with finasteride accelerated the onset of spontaneous recurrent seizures (Biagini et al., 2006), suggesting that endogenous neurosteroids play a role in restraining epileptogenesis, or at least act to inhibit the expression of seizures.
The development of epilepsy is linked to complex alterations in neuroplastic mechanisms. Dysregulation of neurosteroid synthesis may also play a role. This premise is being tested in various epileptogenic models (Reddy and Mohan, 2011). We investigated the role of the prototype endogenous neurosteroid allopregnanolone in controlling limbic epileptogenesis. Treatment with finasteride, a neurosteroid synthesis inhibitor, resulted in a significant increase in epileptogenesis in the hippocampus kindling model (Ramanathan and Reddy, 2011). Exogenous administration of allopregnanolone, at doses that produce levels similar to gonadotropins, markedly inhibited epileptogenesis. In female epilepsy rats, finasteride treatment exacerbates seizure frequency (Lawrence et al., 2010). Neurosteroid-mediated increase in tonic inhibition in the hippocampus could inhibit the spread of the seizure discharge from the hippocampal focus and thereby suppress the rate of development of behavioral kindled seizure activity without affecting the focal electrographic discharges (Figure 4). The exact mechanisms are unclear. Increased tonic inhibition by allopregnanolone is shown to impair the NMDA (N-methyl-D-aspartate) receptor-mediated excitability in the hippocampus (Shen et al., 2010). It is likely that such a mechanism may underlie the progesterone’s disease-modifying effects in the kindling model. Based on these pilot studies, it is suggested that augmentation of neurosteroid synthesis may represent a unique strategy for preventing or retarding epileptogenesis.
Proconvulsant and Epileptogenic Activity
Neurosteroids that are sulfated at C3 have inhibitory actions on GABA-A receptors (Park-Chung et al., 1999). PS and DHEAS block GABA-A receptors at low micromolar concentrations (Majewska, 1992). These “sulfated steroids” act as non-competitive antagonists of the GABA-A receptor by interacting with a site that is distinct from that of neurosteroids such as allopregnanolone and THDOC (Majewska and Schwartz, 1987; Majewska et al., 1990; Park-Chung et al., 1999). The steroid negative modulatory action on GABA-A receptors occurs through a reduction in channel opening frequency, although the precise mechanism of block is not well understood (Mienville and Vicini, 1989; Akk et al., 2001). PS has been shown to possess agonistic actions on NMDA receptors (Wu et al., 1991) and presynaptic sites (Mtchedlishvili and Kapur, 2003). Given their abundance in brain, it seems reasonable that PS and DHEAS could function as endogenous neuromodulators. In contrast to allopregnanolone, sulfated neurosteroids PS and DHEAS exert proconvulsant or convulsant actions (Reddy and Kulkarni, 1998; Kokate et al., 1999b). Direct injection of PS to rodent brain elicits seizures which increase in severity and frequency with time and eventually progress to status epilepticus, tonic hindlimb extension, and death of the animals (Kokate et al., 1999b; Williamson et al., 2004). However, systemic administration of PS does not induce overt seizure activity (Kokate et al., 1999b). The pharmacology of PS seizures is poorly understood, but both clonazepam and allopregnanolone can effectively protect against PS-induced seizure activity (Kokate et al., 1999b). The proconvulsant effect of DHEAS is reduced by progesterone, an intermediate precursor of neurosteroid synthesis. Moreover, exogenous application or endogenous stimulation of DHEAS modulates hippocampal GABA inhibition possibly by entraining hippocampal neurons to theta rhythm (Steffensen, 1995), suggesting a potential physiological relevance of the proconvulsant effects of DHEAS in animals.
Gender Differences in Epilepsy and Neurosteroids
Epilepsy shows sex differences in incidence, progression, and severity, as well as in responsiveness to therapy. The incidence of epilepsy is generally higher in males than in females. More women than men are diagnosed with idiopathic generalized epilepsy, but localization-related symptomatic epilepsies are more frequent in men, and cryptogenic localization-related epilepsies are more frequent in women (Hauser, 1997; Christensen et al., 2005). Sex differences have been described in patients with TLE, with respect to distinct regional distribution of brain dysfunction during interictal periods as well as to the extent of neuronal damage. Women tend to have less structural atrophy than men, regardless of the seizure rate. During brain development, sex hormones have organizational effects leading to permanent differences between males and females in distinct brain regions (Velíšková, 2009). However, the precise mechanisms underlying the sex-dependent differentiation of the specific neuronal circuits, particularly brain regions involved in seizure control, are not clear. Many factors are involved in determining sex differences in seizure susceptibility, including the presence of sexual dimorphism in brain structures involved in seizure generation and control, in regional connectivity, sensitivity of neurotransmitter systems, receptor distribution, and dependence on hormonal milieu and on changes in sex hormone levels during the life span.
Neurosteroids exhibit a strong gender differences in their pharmacological effects with more potency in females than males (Reddy, 2009b). Steroid hormones such as progesterone and testosterone play a key role in the gender-related differences in susceptibility to seizures. However, the precise mechanism underlying such sexual dimorphism is obscure. Many of the biological actions of steroid hormones are mediated through intracellular receptors. Studies have suggested that these sex differences in seizure sensitivity are due to gender-specific distribution of steroid hormones or other sexually dimorphic characteristics in specific brain areas relevant to epilepsy. For example, estradiol reduces seizure-induced hippocampal injury in ovariectomized female but not in male rats, suggesting that the effects of estradiol on seizure threshold and damage may be determined by sex-related differences in the hormonal environment. Neurosteroids may play a key role in gender-related differences in seizure susceptibility (Reddy, 2009b). Both progesterone and allopregnanolone protect against experimental seizures in both male and female mice lacking PRs (Reddy et al., 2004). However, female mice exhibit significantly enhanced sensitivity to the protective activity of allopregnanolone as compared to males. In the pilocarpine seizure test, androstanediol has similar increased potency in female mice, which is not related to differences in pharmacokinetics of this neurosteroid. These results underscore the possible role of endogenous neurosteroids in gender-related differences in seizure susceptibility and protection.
Potential Side Effects of Neurosteroids
Steroid hormones such as progesterone and DOC have long been known to have sedative, anesthetic, and antiseizure properties (Aird, 1944; Gyermek et al., 1967; Green et al., 1978). Studies during the past three decades have uncovered that neurosteroids mediate such rapid effects of steroid hormones in the brain (Reddy et al., 2004, 2005). Unlike steroid hormones, the acute effects of neurosteroids are not related to interactions with classical steroid hormone receptors that regulate gene transcription. Moreover, neurosteroids are not themselves active at intracellular steroid receptors. They modulate brain excitability primarily by interaction with neuronal membrane receptors and ion channels, principally GABA-A receptors. Therefore, like other GABAergic agents, neurosteroids have sedative and anxiolytic properties (Reddy, 2003a). At high doses, neurosteroids cause anesthetic effects. This feature is exemplified with alphaxolone, which was introduced as intravenous anesthetic in 1970s. It was withdrawn later from the market due to toxicity of the solvent used for formulation of this synthetic neurosteroid. Although natural neurosteroids can be used for therapeutic purpose in patients with epilepsy, certain obstacles prevent the clinical use of endogenous neurosteroids. First, natural neurosteroids such as allopregnanolone have low bioavailability because they are rapidly inactivated and eliminated by glucuronide or sulfate conjugation at the 3α-hydroxyl group. Secondly, the 3α-hydroxyl group of allopregnanolone may undergo oxidation to the ketone, restoring activity at steroid hormone receptors (Rupprecht et al., 1993). Synthetic neurosteroids, which are devoid of such hormonal actions, could provide a rational alternative approach to therapy (Reddy and Kulkarni, 2000). Recently, a number of synthetic analogs of allopregnanolone and other neurosteroids are tested in animals and human trials. Ganaxolone, the 3β-methyl analog of allopregnanolone, is a synthetic neurosteroid analog that overcomes these limitations (Carter et al., 1997). Results from the clinical trials of ganaxolone demonstrated a benign side effect profile (Reddy and Woodward, 2004). More than 900 subjects (adults and children) have received this neurosteroid in Phase 1 and 2 trials. Overall, the drug is safe and well tolerated. The most common side effect is reversible dose-related sedation. Other adverse events reported by few subjects include dizziness, fatigue, and somnolence, which indicate its GABAergic effects.
Conclusion
The major part of the pathophysiology of epilepsy is epileptogenesis, whereby a normal brain becomes progressively epileptic because of injury factors. Despite increased scientific awareness, there is a large gap in our understanding of epileptogenesis and many questions remain unanswered regarding the cellular and molecular mechanisms underlying the “irreversible conversion” of a normal brain into epileptic brain predisposed to recurrent seizures. Such gaps in our knowledge about epilepsy’s risk factors, comorbidities, and outcomes limit the ability of clinical programs to prevent epilepsy and its consequences. Since the Curing Epilepsy conference in 2000, there has been more focused research on optimizing approaches in preventing epilepsy and there is emphasis on several translation models. Reduction in neuroinflammation and neurodegeneration is a widely targeted approach for curing epilepsy. Despite intense search for drugs that interrupt epileptogenesis, presently there is no FDA-approved drug available for prevention of epilepsy development in patients at risk. It is essential to find a lead target, such as a receptor or signaling pathway that is crucial for the progression of epileptogenesis that can be disrupted by pharmacological agents to prevent or retard epilepsy.
A variety of pharmacological agents have been tested in animal models of epileptogenesis and in clinical trials in patients with a risk factor for epilepsy. However, the outcomes are not highly promising. The pipeline of new drugs for clinical development is very limited. A summary of agents that are currently in development for epilepsy is listed in Table 5. Steroid hormones, especially corticosteroids, estrogen, and progesterone play an important role in epilepsy. Repetitive stress and corticosteroids increases the risk of epilepsy. There is emerging evidence that neurosteroids may play a role in limbic epileptogenesis. Neurosteroids that enhance the GABAergic inhibition within the brain are potent anticonvulsants and they regulate neural excitability networks by enhancing the phasic and tonic inhibition in the hippocampus, a critical region involved in the TLE. Tonic inhibition is therefore an attractive target for antiepileptogenic drugs (Figure 4). The effects of stress and hormonal changes on neuronal excitability are most likely mediated by neurosteroids. Therefore, menstrual, gonadal, and stress-related fluctuations in neurosteroids or dysfunction in their synthesis can alter epileptogenesis in people at risk for epilepsy. As pleotropic agents, steroid hormones can modify, interrupt or reverse the epileptogenic process, such as cell loss, neuroinflammation, neurogenesis, astrogliosis, and axonal sprouting.
Conflict of Interest Statement
The author declares that the research was conducted in the absence of any commercial or financial relationships that could be construed as a potential conflict of interest.
Acknowledgments
The original research described in this article was supported in part by the NIH grants NS051398, NS052158, NS076426, and NS071597 (to Doodipala Samba Reddy) and the seed grant of TAMHSC Women’s Health in Neuroscience (WHIN) program.
References
Acharya, M. M., Hattiangady, B., and Shetty, A. K. (2008). Progress in neuroprotective strategies for preventing epilepsy. Prog. Neurobiol. 84, 363–404. doi: 10.1016/j.pneurobio.2007.10.010
Agís-Balboa, R. C., Pinna, G., Zhubi, A., Maloku, E., Veldic, M., Costa, E., et al. (2006). Characterization of brain neurons that express enzymes mediating neurosteroid biosynthesis. Proc. Natl. Acad. Sci. U.S.A. 103, 14602– 14607. doi: 10.1073/pnas.0606544103
Aird, R. B. (1944). The effect of desoxycorticosterone in epilepsy. J. Nerv. Ment. Dis. 99, 501–510. doi: 10.1097/00005053-194405000-00005
Aird, R. B., and Gordan, G. S. (1951). Anticonvulsive properties of desoxycorticosterone. J. Am. Med. Assn. 145, 715–719. doi: 10.1001/jama.1951.02920280027006
Akk, G., Bracamontes, J., and Steinbach, J. H. (2001). Pregnenolone sulfate block of GABAA receptors: mechanism and involvement of a residue in the M2 region of the subunit. J. Physiol. 532, 673–684. doi: 10.1111/j.1469-7793.2001.0673e.x
Arida, R. M., de Jesus Vieira, A., and Cavalheiro, E. A. (1998). Effect of physical exercise on kindling development. Epilepsy Res. 30, 127–132. doi: 10.1016/S0920-1211(97)00102-2
Arida, R. M., Scorza, F. A., and Cavalheiro, E. A. (2010). Favorable effects of physical activity for recovery in temporal lobe epilepsy. Epilepsia 51(Suppl. 3), 76–79. doi: 10.1111/j.1528-1167.2010.02615.x
Arida, R. M., Scorza, F. A., de Lacerda, A. F., Gomes da Silva, S., and Cavalheiro, E. A. (2007). Physical training in developing rats does not influence the kindling development in the adult life. Physiol. Behav. 90, 629–633. doi: 10.1016/j.physbeh.2006.11.016
Arida, R. M., Scorza, F. A., dos Santos, N. F., Peres, C. A., and Cavalheiro, E. A. (1999). Effect of physical exercise on seizure occurrence in a model of temporal lobe epilepsy in rats. Epilepsy Res. 37, 45–52. doi: 10.1016/S0920-1211(99)00032-7
Austin, J. K., Hesdorffer, D. C., Liverman, C. T., and Schultz, A. M., and Testimony Group (2012). Testimonies submitted for the Institute of Medicine report: epilepsy across the spectrum: promoting health and understanding. Epilepsy Behav. 25, 634–661. doi: 10.1016/j.yebeh.2012.10.003
Auvergne, R., Leré, C., El Bahh, B., Arthaud, S., Lespinet, V., Rougier, A., et al. (2002). Delayed kindling epileptogenesis and increased neurogenesis in adult rats housed in an enriched environment. Brain Res. 954, 277–285.
Bäckström, T. (1976). Epileptic seizures in women related to plasma estrogen and progesterone during the menstrual cycle. Acta Neurol. Scand. 54, 321–347.
Bäckström, T., Zetterlund, B., Blom, S., and Romano, M. (1984). Effect of intravenous progesterone infusions on the epileptic discharge frequency in women with partial epilepsy. Acta Neurol. Scand. 69, 240–248.
Barbaccia, M. L., Roscetti, G., Trabucchi, M., Mostallino, M. C., Concas, A., Purdy, R. H., et al. (1996). Time-dependent changes in rat brain neuroactive steroid concentrations and GABA-A receptor function after acute stress. Neuroendocrinology 63, 166–172. doi: 10.1159/000126953
Barbaccia, M. L., Roscetti, G., Trabucchi, M., Purdy, R. H., Mostallino, M. C., Concas, A., et al. (1997). The effects of inhibitors of GABAergic transmission and stress on brain and plasma allopregnanolone concentrations. Br. J. Pharmacol. 120, 1582–1588. doi: 10.1038/sj.bjp.0701046
Bauer, J., Stoffel-Wagner, B., Flügel, D., Kluge, M., Schramm, J., Bidlingmaier, F., et al. (2000). Serum androgens return to normal after temporal lobe epilepsy surgery in men. Neurology 55, 820–824.
Baulieu, E. E. (1981). “Steroid hormones in the brain: several mechanisms,” in Steroid Hormone Regulation of the Brain, eds F. Fuxe, J. A. Gustafsson, and L. Wetterberg (Oxford: Pergamon Press), 3–14.
Belelli, D., Bolger, M. B., and Gee, K. W. (1989). Anticonvulsant profile of the progesterone metabolite 5 α-pregnan-3 α-ol-20-one. Eur. J. Pharmacol. 166, 325–329. doi: 10.1016/0014-2999(89)90077-0
Belelli, D., Casula, A., Ling, A., and Lambert, J. J. (2002). The influence of subunit composition on the interaction of neurosteroids with GABAA receptors. Neuropharmacology 43, 651–661. doi: 10.1016/S0028-3908(02)00172-7
Biagini, G., Baldelli, E., Longo, D., Pradelli, L., Zini, I., Rogawski, M. A., et al. (2006). Endogenous neurosteroids modulate epileptogenesis in a model of temporal lobe epilepsy. Exp. Neurol. 201, 519–524. doi: 10.1016/j.expneurol.2006.04.029
Biagini, G., Longo, D., Baldelli, E., Zoli, M., Rogawski, M. A., Bertazzoni, G., et al. (2009). Neurosteroids and epileptogenesis in the pilocarpine model: evidence for a relationship between P450scc induction and length of the latent period. Epilepsia 50(Suppl. 1), 53–58. doi: 10.1111/j.1528-1167.2008.01971.x
Biagini, G., Panuccio, G., and Avoli, M. (2010). Neurosteroids and epilepsy. Curr. Opin. Neurol. 23, 170–176. doi: 10.1097/WCO.0b013e32833735cf
Bianchi, M. T., and Macdonald, R. L. (2003). Neurosteroids shift partial agonist activation of GABA-A receptor channels from low- to high-efficacy gating patterns. J. Neurosci. 23, 10934–10943.
Bonuccelli, U., Melis, G. B., Paoletti, A. M., Fioretti, P., Murri, L., and Muratorio, A. (1989). Unbalanced progesterone and estradiol secretion in catamenial epilepsy. Epilepsy Res. 3, 100–106. doi: 10.1016/0920-1211(89)90037-5
Borekci, B., Ingec, M., Yilmaz, M., Kukula, O., Karaca, M., Hacimuftuoglu, A., et al. (2010). Effects of female sex hormones on caffeine-induced epileptiform activity in rats. Gynecol. Endocrinol. 26, 366–371. doi: 10.3109/09513590903511513
Brinton, R. D., Thompson, R. F., Foy, M. R., Baudry, M., Wang, J., Finch, C. E., et al. (2008). Progesterone receptors: form and function in brain. Front. Neuroendocrinol. 29:313–339. doi: 10.1016/j.yfrne.2008.02.001
Buckmaster, P. S., and Dudek, F. E. (1997). Neuron loss, granule cell axon reorganization, and functional changes in the dentate gyrus of epileptic kainate-treated rats. J. Comp. Neurol. 385, 385–404. doi: 10.1002/(SICI)1096-9861(19970901)385:3
Buckmaster, P. S., Zhang, G. F., and Yamawaki, R. (2002). Axon sprouting in a model of temporal lobe epilepsy creates a predominantly excitatory feedback circuit. J. Neurosci. 22, 6650–6658.
Buterbaugh, G. G. (1989). Estradiol replacement facilitates the acquisition of seizures kindled from the anterior neocortex in female rats. Epilepsy Res. 4, 207–215. doi: 10.1016/0920-1211(89)90005-3
Carter, R. B., Wood, P. L., Wieland, S., Hawkinson, J. E., Belelli, D., Lambert, J. J., et al. (1997). Characterization of the anticonvulsant properties of ganaxolone (CCD 1042; 3α-hydroxy-3β-methyl-5α-pregnan-20-one), a selective, high-affinity, steroid modulator of the γ-aminobutyric acid(A) receptor. J. Pharmacol. Exp. Ther. 280, 1284–1295.
Chisari, M., Eisenman, L. N., Covey, D. F., Mennerick, S., and Zorumski, C. F. (2010). The sticky issue of neurosteroids and GABAA receptors. Trends Neurosci. 33, 299–306. doi: 10.1016/j.tins.2010.03.005
Chisari, M., Eisenman, L. N., Krishnan, K., Bandyopadhyaya, A. K., Wang, C., Taylor, A., et al. (2009). The influence of neuroactive steroid lipophilicity on GABAA receptor modulation: evidence for a low-affinity interaction. J. Neurophysiol. 102, 1254–1264. doi: 10.1152/jn.00346.2009
Christensen, J., Kjeldsen, M. J., Anderson, H., Friis, M. L., and Sidenious, P. (2005). Gender differences in epilepsy. Epilepsia 46, 956–960. doi: 10.1111/j.1528-1167.2005. 51204.x
Citraro, R., Russo, E., Di Paola, E. D., Ibbadu, G. F., Gratteri, S., Marra, R., et al. (2006). Effects of some neurosteroids injected into some brain areas of WAG/Rij rats, an animal model of generalized absence epilepsy. Neuropharmacology 50, 1059–1071. doi: 10.1016/j.neuropharm.2006.02.011
Clarke, R. S., Dundee, J. W., and Carson, I. W. (1973). Proceedings: a new steroid anaesthetic-althesin. Proc. R. Soc. Med. 66, 1027–1030.
Concas, A., Mostallino, M. C., Porcu, P., Follesa, P., Barbaccia, M. L., Trabucchi, M., et al. (1998). Role of brain allopregnanolone in the plasticity of gamma-aminobutyric acid type A receptor in rat brain during pregnancy and after delivery. Proc. Natl. Acad. Sci. U.S.A. 95, 13284–13289. doi: 10.1073/pnas.95.22.13284
Craig, C. R. (1966). Anticonvulsant activity of steroids: separability of anticonvulsant from hormonal effects. J. Pharmacol. Exp. Ther. 153, 337–343.
Cutler, G. B. Jr., Barnes, K. M., Sauer, M. A., and Loriaux, D. L. (1979). 11-Deoxycortisol: a glucocorticoid antagonist in vivo. Endocrinology 104, 1839–1844. doi: 10.1210/endo-104-6-1839
Cutler, S. M., Cekic, M., Miller, D. M., Wali, B., VanLandingham, J. W., and Stein, D. G. (2007). Progesterone improves acute recovery after traumatic brain injury in the aged rat. J. Neurotrauma 24, 1475–1486. doi: 10.1089/neu.2007.0294
Cutler, S. M., Pettus, E. H., Hoffman, S. W., and Stein, D. G. (2005). Tapered progesterone withdrawal enhances behavioral and molecular recovery after traumatic brain injury. Exp. Neurol. 195, 423–429. doi: 10.1016/j.expneurol.2005.06.003
de Araujo Furtado, M., Rossetti, F., Chanda, S., and Yourick, D. (2012). Exposure to nerve agents: from status epilepticus to neuroinflammation, brain damage, neurogenesis and epilepsy. Neurotoxicology 33, 1476–1490. doi: 10.1016/j.neuro.2012.09.001
DeCoster, M., Lambeau, G., Lazdunski, M., and Bazan, N. G. (2002). Secreted phospholiase A2 potentiates glutamate-induced calcium increase and cell death in primary neuronal cultures. J. Neurosci. Res. 67, 634–645. doi: 10.1002/jnr.10131
Desgent, S., Duss, S., Sanon, N. T., Lema, P., Lévesque, M., Hébert, D., et al. (2012). Early-life stress is associated with gender-based vulnerability to epileptogenesis in rat pups. PLoS ONE 7:e42622. doi: 10.1371/journal.pone.0042622
Devaud, L. L., Purdy, R. H., Finn, D. A., and Morrow, A. L. (1996). Sensitization of γ-aminobutyric acidA receptors to neuroactive steroids in rats during ethanol withdrawal. J. Pharmacol. Exp. Ther. 278, 510–517.
Do Rego, J. L., Seong, J. Y., Burel, D., Leprince, J., Luu-The, V., Tsutsui, K., et al. (2009). Neurosteroid biosynthesis: enzymatic pathways and neuroendocrine regulation by neurotransmitters and neuropeptides. Front. Neuroendocrinol. 30:259–301. doi: 10.1016/j.yfrne.2009.05.006
Dudek, F. E., and Staley, K. J. (2011). The time course of acquired epilepsy: implications for therapeutic intervention to suppress epileptogenesis. Neurosci. Lett. 497, 240–246. doi: 10.1016/j.neulet.2011.03.071
Dworetzky, B. A., Townsend, M. K., Pennell, P. B., and Kang, J. H. (2012). Female reproductive factors and risk of seizure or epilepsy: data from the Nurses’ Health Study II. Epilepsia 53, 1–4. doi: 10.1111/j.1528-1167.2011.03308.x
Edwards, H. E., Burnham, W. M., and MacLusky, N. J. (1999). Testosterone and its metabolites affect afterdischarge thresholds and the development of amygdala kindled seizures. Brain Res. 838, 151–157. doi: 10.1016/S0006-8993(99)01620-0
Edwards, H. E., Epps, T., Carlen, P. L. J., and MacLusky, N. (2000). Progestin receptors mediate progesterone suppression of epileptiform activity in tetanized hippocampal slices in vitro. Neuroscience 101, 895–906. doi: 10.1016/S0306-4522(00)00439-5
Edwards, H. E., Mo, V., Burnham, W. M., and MacLusky, N. J. (2001). Gonadectomy unmasks an inhibitory effect of progesterone on amygdala kindling in male rats. Brain Res. 889, 260–263. doi: 10.1016/S0006-8993(00)03147-4
El-Khayat, H. A., Shatla, H. M., Ali, G. K., Abdulgani, M. O., Tomoum, H. Y., and Attya, H. A. (2003). Physical and hormonal profile of male sexual development in epilepsy. Epilepsia 44, 447–452. doi: 10.1046/j.1528-1157.2003.26502.x
Engel, J., Pedley, T. A., Aicardy, J., and Dichter, M. A. (2007). Epilepsy: A Comprehensive Textbook, 2nd Edn. Philadelphia: Lippincott Williams & Wilkins.
Fisher, R. S., van Emde Boas, W., Blume, W., Elger, C., Genton, P., Lee, P., et al. (2005). Epileptic seizures and epilepsy: definitions proposed by the international league against epilepsy (ILAE) and the international bureau for epilepsy (IBE). Epilepsia 46, 470–472. doi: 10.1111/j.0013-9580.2005.66104.x
Frucht, M. M., Quigg, M., Schwaner, C., and Fountain, N. B. (2000). Distribution of seizure precipitants among epilepsy syndromes. Epilepsia 41, 1534–1539. doi: 10.1111/j.1499-1654.2000.001534.x
Frye, C. A. (1995). The neuroactive steroid 3 α, 5 α-THP has anti-seizure and possible neuroprotective effects in an animal model of epilepsy. Brain Res. 696, 113–120. doi: 10.1016/0006-8993(95)00793-P
Frye, C. A., and Reed, T. A. (1998). Androgenic neurosteroids: anti-seizure effects in an animal model of epilepsy. Psychoneuroendocrinology 23, 385–399. doi: 10.1016/S0306-4530(98)00009-2
Frye, C. A., Rhodes, M. E., Walf, A. A., and Harney, J. P. (2001a). Testosterone reduces pentylenetetrazole-induced ictal activity of wildtype mice but not those deficient in type I 5α-reductase. Brain Res. 918, 182–186. doi: 10.1016/S0006-8993(01)02967-5
Frye, C. A., Park, D., Tanaka, M., Rosellini, R., and Svare, B. (2001b). The testosterone metabolite and neurosteroid 3α-androstanediol may mediate the effects of testosterone on conditioned place preference. Psychoneuroendocrinology 26, 731–750. doi: 10.1016/S0306-4530(01)00027-0
Frye, C. A., Rhodes, M. E., Walf, A., and Harney, J. (2002). Progesterone reduces pentylenetetrazol-induced ictal activity of wild-type mice but not those deficient in type I 5α -reductase. Epilepsia 43(Suppl. 5), 14–17. doi: 10.1046/j.1528-1157.43.s.5.19.x
Frye, C. A., and Scalise, T. J. (2000). Anti-seizure effects of progesterone and 3α ,5α -THP in kainic acid and perforant pathway models of epilepsy. Psychoneuroendocrinology 25, 407–420. doi: 10.1016/S0306-4530(99)00068-2
Gangisetty, O., and Reddy, D. S. (2010). Neurosteroid withdrawal regulates GABA-A receptor α 4-subunit expression and seizure susceptibility by activation of progesterone receptor-independent early growth response factor-3 pathway. Neuroscience 170, 865–880. doi: 10.1016/j.neuroscience.2010.07.037
Gee, K. W., Bolger, M. B., Brinton, R. E., Coirini, H., and McEwen, B. S. (1988). Steroid modulation of the chloride ionophore in rat brain: structure-activity requirements, regional dependence and mechanism of action. J. Pharmacol. Exp. Ther. 246, 803–812.
Gibson, C. L., Gray, L. J., Bath, P. M., and Murphy, S. P. (2008). Progesterone for the treatment of experimental brain injury; a systematic review. Brain 131, 318–328. doi: 10.1093/brain/awm183
Glauser, T., Ben-Menachem, E., Bourgeois, B., Cnaan, A., Chadwick, D., Guerreiro, C., et al. (2006). ILAE treatment guidelines: evidence-based analysis of antiepileptic drug efficacy and effectiveness as initial monotherapy for epileptic seizures and syndromes. Epilepsia 47, 1094–1120. doi: 10.1111/j.1528-1167.2006.00585.x
Glauser, T., Ben-Menachem, E., Bourgeois, B., Cnaan, A., Guerreiro, C., Kälviäinen, R., et al. (2013). Updated ILAE evidence review of antiepileptic drug efficacy and effectiveness as initial monotherapy for epileptic seizures and syndromes. Epilepsia 54, 551–563.
Glien, M., Brandt, C., Potschka, H., Voigt, H., Ebert, U., and Löscher, W. (2001). Repeated low-dose treatment of rats with pilocarpine: low mortality but high proportion of rats developing epilepsy. Epilepsy Res. 46, 11–119.
Goddard, G. V., McIntyre, D. C., and Leech, C. K. (1969). A permanent change in brain function resulting from daily electrical stimulation. Exp. Neurol. 25, 295–330. doi: 10.1016/0014-4886(69)90128-9
Green, C. J., Halsey, M. J., Precious, S., and Wardley-Smith, B. (1978). Alphaxolone-alphadolone anesthesia in laboratory animals. Lab. Anim. 12, 85–89.
Grigorian, V. Z., and Khudaverkian, D. N. (1970). Effect of castration and subsequent administration of testosterone propionate on susceptibility to convulsions in animals. Zh. Eksp. Klin. Med. 10, 11–17.
Gyermek, L., Genther, G., and Fleming, N. (1967). Some effects of progesterone and related steroids on the central nervous system. Int. J. Neuropharmacol. 6, 191–198. doi: 10.1016/0028-3908(67)90005-6
Harden, C., and MacLusky, N. J. (2004). Aromatase inhibition, testosterone, and seizures. Epilepsy Behav. 5, 260–263. doi: 10.1016/j.yebeh.2003.12.001
Harden, C., and MacLusky, N. J. (2005). Aromatase inhibitors as add-on treatment for men with epilepsy. Expert Rev. Neurother. 5, 123–127. doi: 10.1586/14737175.5.1.123
Harrison, N. L., Majewska, M. D., Harrington, J. W., and Barker, J. L. (1987). Structure-activity relationships for steroid interactions with the γ-aminobutyric acidA receptor complex. J. Pharmacol. Exp. Ther. 241, 346–353.
Harrison, N. L., and Simmonds, M. A. (1984). Modulation of the GABA receptor complex by a steroid anaesthetic. Brain Res. 323, 287–292. doi: 10.1016/0006-8993(84)90299-3
Hauser, W. A. (1997). “Incidence and prevalence,” in Epilepsy, A Comprehensive Textbook, eds J. Engel Jr. and T. A. Pedley (Philadelphia: Lippincott-Raven Publishers), 47–57.
Hauser, W. A., Annegers, J. F., and Kurland, L. T. (1993). Incidence of epilepsy and unprovoked seizures in Rochester Minnesota during 1935–1984. Epilepsia 34, 453–468. doi: 10.1111/j.1528-1157.1993.tb02586.x
He, X.-P., Kotloski, R., Nef, S., Luikart, B. W., Parada, L. F., and McNamara, J. O. (2004). Conditional deletion of TrkB but not BDNF prevents epileptogenesis in the kindling model. Neuron 43, 31–42. doi: 10.1016/j.neuron.2004.06.019
Hellier, J. L., Patrylo, P. R., Dou, P., Nett, M., Rose, G. M., and Dudek, F. E. (1999). Assessment of inhibition and epileptiform activity in the septal dentate gyrus of freely behaving rats during the first week after kainate treatment. J. Neurosci. 19, 10053–10064.
Herzog, A. G. (1991). Reproductive endocrine considerations and hormonal therapy for men with epilepsy. Epilepsia 32(Suppl. 6), S34–S37. doi: 10.1111/j.1528-1157.1991.tb05890.x
Herzog, A. G. (1995). Progesterone therapy in women with complex partial and secondary generalized seizures. Neurology 45, 1600–1662. doi: 10.1212/WNL.45.9.1660
Herzog, A. G. (1999). Progesterone therapy in women with epilepsy: a 3-year follow-up. Neurology 52, 1917–1918. doi: 10.1212/WNL.52.9.1917-a
Herzog, A. G. (2002). Altered reproductive endocrine regulation in men with epilepsy: implications for reproductive function and seizures. Ann. Neurol. 51, 539–542. doi: 10.1002/ana.10230
Herzog, A. G., Fowler, K. M., Smithson, S. D., Kalayjian, L. A., Heck, C. N., Sperling, M. R., et al. (2012). Progesterone vs placebo therapy for women with epilepsy: a randomized clinical trial. Neurology 78, 1959–1966. doi: 10.1212/WNL.0b013e318259e1f9
Herzog, A. G., and Frye, C. A. (2003). Seizure exacerbation associated with inhibition of progesterone metabolism. Ann. Neurol. 53, 390–391. doi: 10.1002/ana.10508
Herzog, A. G., Klein, P., and Jacobs, A. R. (1998). Testosterone versus testosterone and testolactone in treating reproductive and sexual dysfunction in men with epilepsy and hypogonadism. Neurology 50, 782–784. doi: 10.1212/WNL.50.3.782
Herzog, A. G., Klein, P., and Ransil, B. J. (1997). Three patterns of catamenial epilepsy. Epilepsia 38, 1082–1088. doi: 10.1111/j.1528-1157.1997.tb01197.x
Hesdorffer, D. C., Beck, V., Begley, C. E., Bishop, M. L., Cushner-Weinstein, S., Holmes, G. L., et al. (2013). Research implications of the Institute of Medicine Report, Epilepsy Across the Spectrum: promoting health and understanding. Epilepsia 54, 207–216. doi: 10.1111/epi.12056
Heuser, G., and Eidelberg, E. (1961). Steroid-induced convulsions in experimental animals. Endocrinology 69, 915–924. doi: 10.1210/endo-69-5-915
Holmes, G. L., and Weber, D. A. (1984). The effect of progesterone on kindling: a developmental study. Brain Res. 318, 45–53.
Hom, A. C., and Buterbaugh, G. G. (1986). Estrogen alters the acquisition of seizures kindled by repeated amygdala stimulation or pentylenetetrazol administration in ovariectomized female rats. Epilepsia 27, 103–108. doi: 10.1111/j.1528-1157.1986.tb03510.x
Hosie, A. D., Wilkins, M. E., da Silva, H. M. A., and Smart, T. G. (2006). Endogenous neurosteroids regulate GABAA receptors through two discrete transmembrane sites. Nature 444, 486–489. doi: 10.1038/nature05324
Hosie, A. M., Clarke, L., da Silva, H., and Smart, T. G. (2009). Conserved site for neurosteroid modulation of GABAA receptors. Neuropharmacology 56, 149–154. doi: 10.1016/j.neuropharm.2008.07.050
Hosie, A. M., Wilkins, M. E., and Smart, T. G. (2007). Neurosteroid binding sites on GABA-A receptors. Pharmacol. Ther. 116, 7–19. doi: 10.1016/j.pharmthera.2007.03.011
Isojarvi, J. I., Pakarinen, A. J., and Myllyla, V. V. (1988). Effects of carbamazepine therapy on serum sex hormone levels in male patients with epilepsy. Epilepsia 29, 781–786. doi: 10.1111/j.1528-1157.1988.tb04235.x
Jacobs, M. P., Fischbach, G. D., Davis, M. R., Dichter, M. A., Dingledine, R., Lowenstein, D. H., et al. (2001). Future directions for epilepsy research. Neurology 57, 1536–1542. doi: 10.1212/WNL.57.9.1536
Jacobs, M. P., Leblanc, G. G., Brooks-Kayal, A., Jensen, F. E., Lowenstein, D. H., Noebels, J. L., et al. (2009). Curing epilepsy: progress and future directions. Epilepsy Behav. 14, 438–445. doi: 10.1016/j.yebeh.2009.02.036
Jacono, J. J., and Robinson, J. (1987). The effects of estrogen, progesterone, and ionized calcium on seizures during the menstrual cycle in epileptic women. Epilepsia 28, 571–577. doi: 10.1111/j.1528-1157.1987.tb03690.x
Jiang, N., Chopp, M., Stein, D., and Feit, H. (1996). Progesterone is neuroprotective after transient middle cerebral artery occlusion in male rats. Brain Res. 735, 101–107. doi: 10.1016/0006-8993(96)00605-1
Joëls, M. (1997). Steroid hormones and excitability in the mammalian brain. Front. Neuroendocrinol. 18:2–48. doi: 10.1006/frne.1996.0144
Jones, N. C., Lee, H. E., Yang, M., Rees, S. M., Morris, M. J., O’Brien, T. J., et al. (2013). Repeatedly stressed rats have enhanced vulnerability to amygdala kindling epileptogenesis. Psychoneuroendocrinology 38, 263–270. doi: 10.1016/j.psyneuen.2012.06.005
Kaminski, R. M., Fu, Z., Venkatesan, K., Mazzuferi, M., Leclercq, K., Seutin, V., et al. (2011). 11-Deoxycortisol impedes GABAergic neurotransmission and induces drug-resistant status epilepticus in mice. Neuropharmacology 60, 1098–1108. doi: 10.1016/j.neuropharm.2010.09.021
Kaminski, R. M., Livingood, M. R., and Rogawski, M. A. (2004). Allopregnanolone analogs that positively modulate GABA receptors protect against partial seizures induced by 6-Hz electrical stimulation in mice. Epilepsia 45, 864–877. doi: 10.1111/j.0013-9580.2004.04504.x
Kaminski, R. M., Marini, H., Kim, W. J., and Rogawski, M. A. (2005). Anticonvulsant activity of androsterone and etiocholanolone. Epilepsia 46, 819–827. doi: 10.1111/j.1528-1167.2005.00705.x
Karst, H., de Kloet, E. R., and Joëls, M. (1999). Episodic corticosterone treatment accelerates kindling epileptogenesis and triggers long-term changes in hippocampal CA1 cells, in the fully kindled state. Eur. J. Neurosci. 11, 889–898.
Koenig, H., Schumacher, M., Ferzaz, B., Do Thi, A. N., Ressouches, A., Guennoun, R., et al. (1995). Progesterone synthesis and myelin formation by Schwann cells. Science 268, 1500–1503. doi: 10.1126/science.7770777
Köhling, R., and Staley, K. (2011). Network mechanisms for fast ripple activity in epileptic tissue. Epilepsy Res. 97, 318–323.
Kokate, T. G., Banks, M. K., Magee, T., Yamaguchi, S., and Rogawski, M. A. (1999a). Finasteride, a 5α -reductase inhibitor, blocks the anticonvulsant activity of progesterone in mice. J. Pharmacol. Exp. Ther. 288, 679–684.
Kokate, T. G., Juhng, K. N., Kirkby, R. D., Llamas, J., Yamaguchi, S., and Rogawski, M. A. (1999b). Convulsant actions of the neurosteroid pregnenolone sulfate in mice. Brain Res. 831, 119–124. doi: 10.1016/S0006-8993(99)01287-1
Kokate, T. G., Svensson, B. E., and Rogawski, M. A. (1994). Anticonvulsant activity of neuroactive steroids: correlation with γ-aminobutyric acid-evoked chloride current potentiation. J. Pharmacol. Exp. Ther. 270, 1223–1229.
Kokate, T. G., Yamaguchi, S., Pannell, L. K., Rajamani, U., Carroll, D. M., Grossman, A. B., et al. (1998). Lack of anticonvulsant tolerance to the neuroactive steroid pregnanolone in mice. J. Pharmacol. Exp. Ther. 287, 553–558.
Kulkarni, S. K., and Reddy, D. S. (1995). Neurosteroids: a new class of neuromodulators. Drugs Today. 31, 433–455.
Kumar, G., Couper, A., O’Brien, T. J., Salzberg, M. R., Jones, N. C., Rees, S. M., et al. (2007). The acceleration of amygdala kindling epileptogenesis by chronic low-dose corticosterone involves both mineralocorticoid and glucocorticoid receptors. Psychoneuroendocrinology 32, 834–842. doi: 10.1016/j.psyneuen.2007.05.011
Kumar, G., Jones, N. C., Morris, M. J., Rees, S., O’Brien, T. J., and Salzberg, M. R. (2011). Early life stress enhancement of limbic epileptogenesis in adult rats: mechanistic insights. PLoS ONE 6:e24033. doi: 10.1371/journal.pone.0024033
Lai, M. C., Lui, C. C., Yang, S. N., Wang, J. Y., and Huang, L. T. (2009). Epileptogenesis is increased in rats with neonatal isolation and early-life seizure and ameliorated by MK-801: a long-term MRI and histological study. Pediatr. Res. 66, 441–447. doi: 10.1203/PDR.0b013e3181b337d2
Lambert, J. J., Cooper, M. A., Simmons, R. D., Weir, C. J., and Belelli, D. (2009). Neurosteroids: endogenous allosteric modulators of GABA-A receptors. Psychoneuroendocrinology 34(Suppl. 1), S48–58. doi: 10.1016/j.psyneuen.2009.08.009
Lawrence, C., Martin, B. S., Sun, C., Williamson, J., and Kapur, J. (2010). Endogenous neurosteroid synthesis modulates seizure frequency. Ann. Neurol. 67, 689–693.
Lévesque, M., Bortel, A., Gotman, J., and Avoli, M. (2011). High-frequency (80-500 Hz) oscillations and epileptogenesis in temporal lobe epilepsy. Neurobiol. Dis. 42, 231–241.
Logothetis, J., Harner, R., and Morrel, F. (1959). The role of estrogens in catamenial exacerbation of epilepsy. Neurology 9, 352–360. doi: 10.1212/WNL.9.5.352
Lonsdale, D., and Burnham, W. M. (2003). The anticonvulsant effects of progesterone and 5α-dihydroprogesterone on amygdala-kindled seizures in rats. Epilepsia 44, 1494–1499. doi: 10.1111/j.0013-9580.2003.59402.x
Löscher, W. (2002). Animal models of epilepsy for the development of antiepileptogenic and disease-modifying drugs. A comparison of the pharmacology of kindling and post-status epilepticus models of temporal lobe epilepsy. Epilepsy Res. 50, 105–123.
Löscher, W. (2012). “Strategies for antiepileptogenesis: antiepileptic drugs versus novel approaches evaluated in post-status epilepticus models of temporal lobe epilepsy,” in Jasper’s Basic Mechanisms of the Epilepsies [Internet], 4th Edn, eds J. L. Noebels, M. Avoli, M. A. Rogawski, R. W. Olsen, and A. V. Delgado-Escueta (Bethesda, MD: NCBI).
MacLusky, N. J., Walters, M. J., Clark, A. S., and Toran-Allerand, C. D. (1994). Aromatase in the cerebral cortex, hippocampus, and mid-brain: ontogeny and developmental implications. Mol. Cell. Neurosci. 5, 691–698. doi: 10.1006/mcne.1994.1083
Macphee, G. J., Larkin, J. G., Butler, E., Beastall, G. H., and Brodie, M. J. (1988). Circulating hormones and pituitary responsiveness in young epileptic men receiving long-term antiepileptic medication. Epilepsia 29, 468–475. doi: 10.1111/j.1528-1157.1988.tb03747.x
Majewska, M. D. (1992). Neuroactive steroids: endogenous bimodal modulators of the GABA-A receptor. Mechanism of action and physiological significance. Progr. Neurobiol. 38, 379–395. doi: 10.1016/0301-0082(92)90025-A
Majewska, M. D., Demirgoren, S., and Spivak, C. E., and London, E. D. (1990). The neuroactive steroid dehydroepiandrosterone sulfate is an allosteric antagonist of the GABA-A receptor. Brain Res. 526, 143–146. doi: 10.1016/0006-8993(90)90 261-9
Majewska, M. D., Harrison, N. L., Schwartz, R. D., Barker, J. L., and Paul, S. M. (1986). Steroid hormone metabolites are barbiturate-like modulators of the GABA receptor. Science 232, 1004–1007. doi: 10.1126/science.2422758
Majewska, M. D., and Schwartz, R. D. (1987). Pregnenolone sulfate: an endogenous antagonist of the γ-aminobutyric acid receptor complex in brain? Brain Res. 404, 355–360. doi: 10.1016/0006-8993(87)91394-1
Mani, R., Pollard, J., and Dichter, M. A. (2011). Human clinical trails in antiepileptogenesis. Neurosci. Lett. 497, 251–256. doi: 10.1016/j.neulet.2011.03.010
McClelland, S., Dubé, C. M., Yang, J., and Baram, T. Z. (2011). Epileptogenesis after prolonged febrile seizures: mechanisms, biomarkers and therapeutic opportunities. Neurosci. Lett. 497, 155–162.
McHugh, J. C., and Delanty, N. (2008). Epidemiology and classification of epilepsy, gender comparisons. Int. Rev. Neurobiol. 83, 11–26. doi: 10.1016/S0074-7742(08)00002-0
McNamara, J. O., Morrisett, R., and Nadler, J. V. (1992). Recent advances in understanding mechanisms of the kindling model. Adv. Neurol. 57, 555–560.
Meffre, D., Pianos, A., Liere, P., Eychenne, B., Cambourg, A., Schumacher, M., et al. (2007). Steroid profiling in brain and plasma of male and pseudopregnant female rats after traumatic brain injury: analysis by gas chromatography/mass spectrometry. Endocrinology 148, 2505–2517.
Meyer, R. P., Hagemeyer, C. E., Knoth, R., Kaufmann, M. R., and Volk, B. (2006). Anti-epileptic drug phenytoin enhances androgen metabolism and androgen receptor expression in murine hippocampus. J. Neurochem. 96, 460–472. doi: 10.1111/j.1471-4159.2005.03555.x
Mienville, J. M., and Vicini, S. (1989). Pregnenolone sulfate antagonizes GABA-A receptor-mediated currents via a reduction of channel opening frequency. Brain Res. 489, 190–194. doi: 10.1016/0006-8993(89)90024-3
Mihalek, R. M., Banerjee, P. K., Korpi, E. R., Quinlan, J. J., Firestone, L. L., and Mi, Z. P., et al. (1999). Attenuated sensitivity to neuroactive steroids in γ-aminobutyrate type A receptor δ subunit knockout mice. Proc. Natl. Acad. Sci. U.S.A. 96, 12905–12910. doi: 10.1073/pnas.96.22.12905
Mohammad, S., Abolhassan, A., and Pourgholami, M. H. (1998). Evaluation of the anticonvulsant profile of progesterone in male amygdala-kindled rats. Epilepsy Res. 30, 195–202. doi: 10.1016/S0920-1211(98)00004-7
Morimoto, K., Fahnestock, M., and Racine, R. J. (2004). Kindling and status epilepticus models of epilepsy: rewiring the brain. Prog. Neurobiol. 73, 1–60. doi: 10.1016/j.pneurobio.2004.03.009
Mtchedlishvili, Z., Bertram, E. H., and Kapur, J. (2001). Diminished allopregnanolone enhancement of GABA-A receptor currents in a rat model of chronic temporal lobe epilepsy. J. Physiol. 537(Pt 2), 453–465. doi: 10.1111/j.1469-7793.2001.00453.x
Mtchedlishvili, Z., and Kapur, J. (2003). A presynaptic action of the neurosteroid pregnenolone sulfate on GABAergic synaptic transmission. Mol. Pharmacol. 64, 857–864. doi: 10.1124/mol.64.4.857
Murashima, Y. L., and Yoshii, M. (2010). New therapeutic approaches for epilepsies, focusing on reorganization of the GABA-A receptor subunits by neurosteroids. Epilepsia 51(Suppl 3), 131–134. doi: 10.1111/j.1528-1167.2010.02627.x
Nadler, J. V. (2003). The recurrent mossy fiber pathway of the epileptic brain. Neurochem. Res. 28, 1649–1658. doi: 10.1023/A:1026004904199
Norwood, B. A., Bumanglag, A. V., Osculati, F., Sbarbati, A., Marzola, P., Nicolato, E., et al. (2010). Classic hippocampal sclerosis and hippocampal-onset epilepsy produced by a single “cryptic” episode of focal hippocampal excitation in awake rats. J. Comp. Neurol. 518, 3381–3407. doi: 10.1002/cne.22406
Nothdurfter, C., Rammes, G., Baghai, T. C., Schüle, C., Schumacher, M., Papadopoulos, V., et al. (2011). TSPO (18 kDa) as a target for novel anxiolytics with a favourable side-effect profile. J. Neuroendocrinol. 24, 82–92.
O’Dell, C. M., Das, A., Wallace, G. IV, Ray, S. K., and Banik, N. L. (2012). Understanding the basic mechanisms underlying seizures in mesial temporal lobe epilepsy and possible therapeutic targets, a review. J. Neurosci. Res. 90, 913–924. doi: 10.1002/jnr.22829
Park-Chung, M., Malayev, A., Purdy, R. H., Gibbs, T. T., and Farb, D. H. (1999). Sulfated and unsulfated steroids modulate γ-aminobutyric acidA receptor function through distinct sites. Brain Res. 830, 72–87. doi: 10.1016/S0006-8993(99)01381-5
Patel, M. (2004). Mitochondrial dysfunction and oxidative stress, cause and consequences of epileptic seizures. Free Radic. Biol. Med. 37, 1951–1962. doi: 10.1016/j.freeradbiomed.2004.08.021
Peng, Z., Huang, C. S., Stell, B. M., Mody, I., and Houser, C. R. (2004). Altered expression of the δ subunit of the GABAA receptor in a mouse model of temporal lobe epilepsy. J. Neurosci. 24, 8629–8639. doi: 10.1523/JNEUROSCI.2877-04.2004
Pericić, D., Svob, D., Jazvinsćak, M., and Mirković, K. (2000). Anticonvulsive effect of swim stress in mice. Pharmacol. Biochem. Behav. 66, 879–886. doi: 10.1016/S0091-3057(00)00267-7
Pericic, D., Manev, H., and Bujas, M. (1996). Gonadal hormones and picrotoxin-induced convulsions in male and female rats. Brain Res. 736, 174–179. doi: 10.1016/0006-8993(96)00677-4
Pesce, M. E., Acevedo, X., Bustamante, D., Miranda, H. E., and Pinardi, G. (2000). Progesterone and testosterone modulate the convulsant actions of pentylenetetrazol and strychnine in mice. Pharmacol. Toxicol. 87, 116–119. doi: 10.1111/j.0901-9928.2000.870303.x
Pitkänen, A., Immonen, R. J., Gröhn, O. H., and Kharatishvili, I. (2009). From traumatic brain injury to posttraumatic epilepsy, what animal models tell us about the process and treatment options. Epilepsia 50(Suppl. 2), 1–29.
Pitkänen, A., and Lukasiuk, K. (2011). Mechanisms of epileptogenesis and potential treatment targets. Lancet Neurol. 10, 173–186.
Pugnaghi, M., Monti, G., Biagini, G., and Meletti, S. (2013). Temporal lobe epilepsy exacerbation during pharmacological inhibition of endogenous neurosteroid synthesis. BMJ Case Rep. 2013. pii: bBR2012008204.
Purdy, R. H., Morrow, A. L., Blinn, J. R., and Paul, S. M. (1990). Synthesis, metabolism, and pharmacological activity of 3α-hydroxy steroids which potentiate GABA-receptor-mediated chloride ion uptake in rat cerebral cortical synaptoneurosomes. J. Med. Chem. 33, 1572–1581. doi: 10.1021/jm00168a008
Purdy, R. H., Morrow, A. L., Moore, P. H. Jr., and Paul, S. M. (1991). Stress-induced elevations of γ-aminobutyric acid type A receptor-active steroids in the rat brain. Proc. Natl. Acad. Sci. U.S.A. 88, 4553–4557. doi: 10.1073/pnas.88.10.4553
Ramakrishnan, L., and Hess, G. P. (2010). Mechanism of potentiation of a dysfunctional epilepsy-linked mutated GABA(A) receptor by a neurosteroid (3α,21-dihydroxy-5α-pregnan-20-one), transient kinetic investigations. Biochemistry 49, 7892–7901. doi: 10.1021/bi901241g
Ramanathan, G., and Reddy, D. S. (2011). Inhibition of endogenous neurosteroids accelerates limbic epileptogenesis. Epilepsy Curr. 2(Suppl. 1), Abst. 1.022.
Rao, M. S., Hattiangady, B., Reddy, D. S., and Shetty, A. K. (2006). Hippocampal neurodegeneration, spontaneous seizures, and mossy fiber sprouting in the F344 rat model of temporal lobe epilepsy. J. Neurosci. Res. 83, 1088–1105. doi: 10.1002/jnr. 20802
Reddy, D. S. (2003a). Pharmacology of endogenous neuroactive steroids. Crit. Rev. Neurobiology 15, 197–234. doi: 10.1615/CritRevNeurobiol.v15.i34.20
Reddy, D. S. (2003b). Is there a physiological role for the neurosteroid THDOC in stress-sensitive conditions? Trends Pharmacol. Sci. 24, 103–106. doi: 10.1016/S0165-6147(03)00023-3
Reddy, D. S. (2004a). Anticonvulsant activity of the testosterone-derived neurosteroid 3alpha-androstanediol. Neuroreport 15, 515–518. doi: 10.1097/00001756-200403010-00026
Reddy, D. S. (2004b). Testosterone modulation of seizure susceptibility is mediated by neurosteroids 3α-androstanediol and 17β-estradiol. Neuroscience 129, 195–207. doi: 10.1016/j.neuroscience.2004.08.002
Reddy, D. S. (2006). Physiological role of adrenal deoxycorticosterone-derived neuroactive steroids in stress-sensitive conditions. Neuroscience 138, 911–920. doi: 10.1016/j.neuroscience.2005.10.016
Reddy, D. S. (2008). Mass spectrometric quantification and physiological-pharmacological activity of androgenic neurosteroids. Neurochem. Int. 52, 541–553. doi: 10.1016/j.neuint.2007.05.019
Reddy, D. S. (2009a). The role of neurosteroids in the pathophysiology and treatment of catamenial epilepsy. Epilepsy Res. 85, 1–30. doi: 10.1016/j.eplepsyres.2009.02.017
Reddy, D. S. (2009b). “Steroid hormones and sex differences in seizure susceptibility,” in Encyclopedia of Basic Epilepsy Research, Vol. 1, ed. Philip Schwartzkroin (Oxford: Academic Press), 526–533. doi: 10.1016/B978-012373961-2.00157-0
Reddy, D. S. (2010). Neurosteroids, Endogenous role in the human brain and therapeutic potentials. Prog. Brain Res. 186, 113–137. doi: 10.1016/B978-0-444-53630-3.00008-7
Reddy, D. S. (2011). Role of anticonvulsant and antiepileptogenic neurosteroids in the pathophysiology and treatment of epilepsy. Front. Endocrinol. 2:38. doi: 10.3389/fendo.2011.00038
Reddy, D. S. (2013). Neuroendocrine aspects of catamenial epilepsy. Horm. Behav. 63, 254–266. doi: 10.1016/j.yhbeh.2012.04.016
Reddy, D. S., Castaneda, D. C., O’Malley, B. W., and Rogawski, M. A. (2004). Anticonvulsant activity of progesterone and neurosteroids in progesterone receptor knockout mice. J. Pharmacol. Exp. Ther. 310, 230–239. doi: 10.1124/jpet.104.065268
Reddy, D. S., Gangisetty, O., and Briyal, S. (2010). Disease-modifying activity of progesterone in the hippocampus kindling model of epileptogenesis. Neuropharmacology 59, 573–581. doi: 10.1016/j.neuropharm.2010.08.017
Reddy, D. S., Gould, J., and Gangisetty, O. (2012). A mouse kindling model of perimenstrual catamenial epilepsy. J. Pharmacol. Exp. Ther. 341, 784–793. doi: 10.1124/jpet.112.192377
Reddy, D. S., and Jian, K. (2010). The testosterone-derived neurosteroid androstanediol is a positive allosteric modulator of GABA-A receptors. J. Pharmacol. Exp. Ther. 334, 1031–1041. doi: 10.1124/jpet.110.169854
Reddy, D. S., Kim, H. Y., and Rogawski, M. A. (2001). Neurosteroid withdrawal model of perimenstrual catamenial epilepsy. Epilepsia 42, 328–336. doi: 10.1046/j.1528-1157.2001.10100.x
Reddy, D. S., and Kulkarni, S. K. (1998). Proconvulsant effects of neurosteroids pregnenolone sulfate and dehydroepiandrosterone sulfate in mice. Eur. J. Pharmacol. 345, 55–59. doi: 10.1016/S0014-2999(98)0034-X
Reddy, D. S., and Kulkarni, S. K. (2000). Development of neuroactive steroid-based novel psychotropic drugs. Prog. Med. Chem. 37, 135–175. doi: 10.1016/S0079-6468(08)70059-6
Reddy, D. S., and Mohan, A. (2011). Development and persistence of limbic epileptogenesis are impaired in mice lacking progesterone receptors. J. Neurosci. 31, 650–658. doi: 10.1523/JNEUROSCI.4488-10.2011
Reddy, D. S., O’Malley, B. W., and Rogawski, M. A. (2005). Anxiolytic activity of progesterone in progesterone receptor knockout mice. Neuropharmacology 48, 14–24. doi: 10.1016/j.neuropharm.2004.09.002
Reddy, D. S., and Ramanathan, G. (2012). Finasteride inhibits the disease-modifying activity of progesterone in the hippocampus kindling model of epileptogenesis. Epilepsy Behav. 25, 92–97. doi: 10.1016/j.yebeh.2012.05.024
Reddy, D. S., and Rogawski, M. A. (2000). Chronic treatment with the neuroactive steroid ganaxolone in the rat induces anticonvulsant tolerance to diazepam but not to itself. J. Pharmacol. Exp. Ther. 295, 1241–1248.
Reddy, D. S., and Rogawski, M. A. (2001). Enhanced anticonvulsant activity of neuroactive steroids in a rat model of catamenial epilepsy. Epilepsia 42, 303–310.
Reddy, D. S., and Rogawski, M. A. (2002). Stress-induced deoxycorticosterone-derived neuroactive steroids modulates GABA(A) receptor function and seizure susceptibility. J. Neurosci. 42, 3795–3805.
Reddy, D. S., and Rogawski, M. A. (2009). Neurosteroid replacement therapy for catamenial epilepsy. Neurotherapeutics. 6, 392–401. doi: 10.1016/j.nurt.2009.01.006
Reddy, D. S., and Rogawski, M. A. (2010). Ganaxolone suppression of behavioral and electrographic seizures in the mouse amygdala kindling model. Epilepsy Res. 89, 254–260. doi: 10.1016/j.eplepsyres.2010.01.009
Reddy, D. S., and Rogawski, M. A. (2012). “Neurosteroids – endogenous regulators of seizure susceptibility and role in the treatment of epilepsy (Chapter 77),” in Jasper’s Basic Mechanisms of the Epilepsies, 4th Edn, eds J. L. Noebels, M. Avoli, M. A. Rogawski, R. W. Olsen, and A. V. Delgado-Escueta (New York, NY: Oxford University Press), 982–1000.
Reddy, D. S., and Woodward, R. (2004). Ganaxolone: a prospective overview. Drugs Future 29, 227–242. doi: 10.1358/dof.2004.029.03.793135
Reibel, S., André, V., Chassagnon, S., André, G., Marescaux, C., Nehlig, A., et al. (2000). Neuroprotective effects of chronic estradiol benzoate treatment on hippocampal cell loss induced by status epilepticus in the female rat. Neurosci. Lett. 281, 79–82.
Roberts, A. J., and Keith, L. D. (1995). Corticosteroids enhance convulsion susceptibility via central mineralocorticoid receptors. Psychoneuroendocrinology 20, 891–902. doi: 10.1016/0306-4530(95)00016-X
Robertson, C. R., Flynn, S. P., White, H. S., and Bulaj, G. (2011). Anticonvulsant neuropeptides as drug leads for neurological diseases. Nat. Prod. Rep. 28, 741–762. doi: 10.1039/c0np00048e
Roof, R. L., Duvdevani, R., Braswell, L., and Stein, D. G. (1994). Progesterone facilitates cognitive recovery and reduces secondary neuronal loss caused by cortical contusion injury in male rats. Exp. Neurol. 129, 64–69. doi: 10.1006/exnr.1994.1147
Roof, R. L., and Hall, E. D. (2000). Gender differences in acute CNS trauma and stroke, neuroprotective effects of estrogen and progesterone. J. Neurotrauma. 17, 367–388. doi: 10.1089/neu.2000.17.367
Rose, R. P., Morell, F., and Hoeppner, T. J. (1979). Influences of pituitary–adrenal hormones on kindling. Brain Res. 169, 303–315. doi: 10.1016/0006-8993(79)91032-1
Rupprecht, R., Papadopoulos, V., Rammes, G., Baghai, T. C., Fan, J., Akula, N., et al. (2010). Translocator protein (18 kDa) (TSPO) as a therapeutic target for neurological and psychiatric disorders. Nat. Rev. Drug Discov. 9, 971–988. doi: 10.1038/nrd3295
Rupprecht, R., Rammes, G., Eser, D., Baghai, T. C., Schüle, C., Nothdurfter, C., et al. (2009). Translocator protein (18 kD) as target for anxiolytics without benzodiazepine-like side effects. Science 325, 490–493.
Rupprecht, R., Reul, J. M., Trapp, T., van Steensel, B., Wetzel, C., Damm, K., et al. (1993). Progesterone receptor-mediated effects of neuroactive steroids. Neuron 11, 523–530. doi: 10.1016/0896-6273(93)90156-L
Saalmann, Y. B., Kirkcaldie, M. T., Waldron, S., and Calford, M. B. (2007). Cellular distribution of the GABA-A receptor-modulating 3α -hydroxy, 5α -reduced pregnane steroids in the adult rat brain. J. Neuroendocrinol. 19, 272–284. doi: 10.1111/j.1365-2826.2006.01527.x
Salzberg, M., Kumar, G., Supit, L., Jones, N. C., Morris, M. J., Rees, S., and O’Brien, T. J. (2007). Early postnatal stress confers enduring vulnerability to limbic epileptogenesis. Epilepsia 48, 2079–2085. doi: 10.1111/j.1528-1167.2007.01246.x
Scharfman, H. E., Kim, M., Hintz, T. M., and MacLusky, N. J. (2008). Seizures and reproductive function, insights from female rats with epilepsy. Ann. Neurol. 64, 687–697. doi: 10.1002/ana.21518
Scharfman, H. E., and MacLusky, N. J. (2006). The influence of gonadal hormones on neuronal excitability, seizures, and epilepsy in the female. Epilepsia 47, 1423–1440. doi: 10.1111/j.1528-1167.2006.00672.x
Scharfman, H. E., Malthankar-Phatak, G. H., Friedman, D., Pearce, P., McCloskey, D. P., Harden, C. L., et al. (2009). A rat model of epilepsy in women: a tool to study physiological interactions between endocrine systems and seizures. Endocrinology 150, 4437–4442. doi: 10.1210/en.2009-0135
Schwartz-Giblin, S., Korotzer, A., and Pfaff, D. W. (1989). Steroid hormone effects on picrotoxin-induced seizures in female and male rats. Brain Res. 476, 240–247. doi: 10.1016/0006-8993(89)91244-4
Selye, H. (1941). Anesthetics of steroid hormones. Proc. Soc. Exp. Biol. Med. 46, 116–121. doi: 10.3181/00379727-46-11907
Selye, H. (1942). The antagonism between anesthetic steroid hormones and pentamethylenetetrazol (metrazol). J. Lab. Clin. Med. 27, 1051–1053.
Shen, H., Sabaliauskas, N., Sherpa, A., Fenton, A. A., Stelzer, A., Aoki, C., et al. (2010). A critical role for α4βδ GABA-A receptors in shaping learning deficits at puberty in mice. Science 327, 1515–1518. doi: 10.1126/science.1184245
Silva de Lacerda, A. F., Janjoppi, L., Scorza, F. A., Lima, E., Amado, D., Cavalheiro, E. A., et al. (2007). Physical exercise program reverts the effects of pinealectomy on the amygdala kindling development. Brain Res. Bull. 74, 16–220. doi: 10.1016/j.brainresbull.2007.06.011
Simonato, M., Löscher, W., Cole, A. J., Dudek, F. E., Engel, J. Jr., Kaminski, R. M., et al. (2012). Finding a better drug for epilepsy: preclinical screening strategies and experimental trial design. Epilepsia 53, 1860–1867.
Singh, M., and Su, C. (2013). Progesterone and neuroprotection. Horm. Behav. 63, 284–290. doi: 10.1016/j.yhbeh.2012.06.003
Sloviter, R. S., and Bumanglag, A. V. (2013). Defining “epileptogenesis” and identifying “antiepileptogenic targets” in animal models of acquired temporal lobe epilepsy is not as simple as it might seem. Neuropharmacology 69, 3–15. doi: 10.1016/j.neuropharm.2012.01.022
Snead, O. C. III (1998). Ganaxolone, a selective, high-affinity steroid modulator of the γ-aminobutyric acid-A receptor, exacerbates seizures in animal models of absence. Ann. Neurol. 44, 688–691. doi: 10.1002/ana.410440417
Spigelman, I., Li, Z., Banerjee, P. K., Mihalek, R. M., Homanics, G. E., and Olsen, R. W. (2002). Behavior and physiology of mice lacking the GABA-A receptor δ subunit. Epilepsia. 43(Suppl. 5), 3–8. doi: 10.1046/j.1528-1157.43.s.5.8.x
Stables, J. P., Bertram, E. H., White, H. S., Coulter, D. A., Dichter, M. A., Jacobs, M. P., et al. (2002). Models for epilepsy and epileptogenesis: report from the NIH workshop, Bethesda, Maryland. Epilepsia 43, 1410–1420. doi: 10.1046/j.1528-1157.2002.06702.x
Steffensen, S. C. (1995). Dehydroepiandrosterone sulfate suppresses hippocampal recurrent inhibition and synchronizes neuronal activity to theta rhythm. Hippocampus 5, 320–328. doi: 10.1002/hipo.450050405
Stein, D. G. (2013). A clinical/translational perspective: can a developmental hormone play a role in the treatment of traumatic brain injury? Horm. Behav. 63, 291–300. doi: 10.1016/j.yhbeh.2012.05.004
Stein, D. G., and Sayeed, I. (2010). Is progesterone worth consideration as a treatment for brain injury. Am. J. Roentgenol. 194, 20–22. doi: 10.2214/AJR.09.3407
Stell, B. M., Brickley, S. G., Tang, C. Y., Farrant, M., and Mody, I. (2003). Neuroactive steroids reduce neuronal excitability by selectively enhancing tonic inhibition mediated by δ subunit-containing GABA-A receptors. Proc. Natl. Acad. Sci. U.S.A. 100, 14439–14444. doi: 10.1073/pnas.2435457100
Stoffel-Wagner, B., Beyenburg, S., Watzka, M. S., Blumcke, I., Bauer, J., Schramm, J., et al. (2000). Expression of 5α-reductase and 3α-hydroxysteroid oxidoreductase in the hippocampus of patients with chronic temporal lobe epilepsy. Epilepsia 41, 140–147. doi: 10.1111/j.1528-1157.2000.tb00133.x
Stoffel-Wagner, B., Watzka, M., Steckelbroeck, S., Ludwig, M., Clusmann, H., Bidlingmaier, F., et al. (2003). Allopregnanolone serum levels and expression of 5α-reductase and 3α-hydroxysteroid dehydrogenase isoforms in hippocampal and temporal cortex of patients with epilepsy. Epilepsy Res. 54, 11–19. doi: 10.1016/S0920-1211(03)00036-6
Sun, C., Mtchedlishvili, Z., Erisir, A., and Kapur, J. (2007). Diminished neurosteroid sensitivity of synaptic inhibition and altered location of the α4-subunit of GABA-A receptors in an animal model of epilepsy. J. Neurosci. 27, 12641–12650. doi: 10.1523/JNEUROSCI.4141-07.2007
Sutula, T., Cascino, G., Cavazos, J., Parada, I., and Ramirez, L. (1989). Mossy fiber synaptic reorganization in the epileptic human temporal lobe. Ann. Neurol. 26, 321–330. doi: 10.1002/ana.410260303
Temkin, N. R. (2001). Antiepileptogenesis and seizure prevention trials with antiepileptic drugs, meta-analysis of controlled trials. Epilepsia 42, 515–524. doi: 10.1046/j.1528-1157.2001.28900.x
Temkin, N. R., and Davis, G. R. (1984). Stress as a risk factor for seizures among adults with epilepsy. Epilepsia 25, 450–456. doi: 10.1111/j.1528-1157.1984.tb03442.x
Thomas, J., and McLean, J. H. (1991). Castration alters susceptibility of male rats to specific seizures. Physiol. Behav. 49, 1177–1179. doi: 10.1016/0031-9384(91)90347-Q
Thomas, J., and Yang, Y. C. (1991). Allylglycine-induced seizures in male and female rats. Physiol. Behav. 49, 1181–1183. doi: 10.1016/0031-9384(91)90348-R
Tsuda, M., Suzuki, T., and Misawa, M. (1997). Modulation of the decrease in the seizure threshold of pentylenetetrazole in diazepam-withdrawn mice by the neuroactive steroid 5α-pregnan-3α,21-diol-20-one (alloTHDOC). Addict. Biol. 2, 455–460. doi: 10.1080/13556219772516
Twyman, R. E., and Macdonald, R. L. (1992). Neuroactive steroid regulation of GABA-A receptor single-channel kinetic properties of mouse spinal cord neurons in culture. J. Physiol. 456, 215–245.
Vallée, M., Rivera, J. D., Koob, G. F., Purdy, R. H., and Fitzgerald, R. L. (2000). Quantification of neuroactive steroids in rat plasma and brain following swim stress and allopregnanolone administration using negative chemical ionization gas chromatography/mass spectrometry. Anal. Biochem. 287, 153–166.
van Vliet, E. A., Aronica, E., Tolner, E. A., Lopes da Silva, F. H., and Gorter, J. A. (2004). Progression of temporal lobe epilepsy in the rat is associated with immunocytochemical changes in inhibitory interneurons in specific regions of the hippocampal formation. Exp. Neurol. 187, 367–379. doi: 10.1016/j.expneurol.2004.01.016
Velíšková, J. (2006). The role of estrogens in seizures and epilepsy: the bad guys or the good guys? Neuroscience 138, 837–844.
Velíšková, J. (2009). “Sex differences in seizure susceptibility,” in Encyclopedia of Basic Epilepsy Research, ed. Philip Schwartzkroin (Oxford: Academic Press), 1–4.
Velíšková, J., and Velísek, L. (2007). Beta-estradiol increases dentate gyrus inhibition in female rats via augmentation of hilar neuropeptide-Y. J. Neurosci. 27, 6054–6063.
Velíšková, J., Velisek, L., Galanopoulou, A. S., and Sperber, E. F. (2000). Neuroprotective effects of estrogens on hippocampal cells in adult female rats after status epilepticus. Epilepsia 41(Suppl. 6), S30–S35.
Verrotti, A., Latini, G., Manco, R., De Simone, M., and Chiarelli, F. (2007). Influence of sex hormones on brain excitability and epilepsy. J. Endocrinol. Invest. 30, 97–803.
Vezzani, A. (2005). Inflammation and epilepsy. Epilepsy Curr. 5, 1–6. doi: 10.1111/j.1535-7597.2005.05101.x
Vezzani, A., French, J., Bartfai, T., and Baram, T. Z. (2011). The role of inflammation in epilepsy. Nat. Rev. Neurol. 7, 31–40. doi: 10.1038/nrneurol.2010.178
VMDB Report (2003). Search terms “epilepsy” in intact versus castrated dogs. The Veterinary Medical Database. Available at: http://www.vmdb.org/
Weiss, G. K., Castillo, N., and Fernandez, M. (1993). Amygdala kindling rate is altered in rats with a deficit in the responsiveness of the hypothalamo-pituitary-adrenal axis. Neurosci. Lett. 157, 91–94. doi: 10.1016/0304-3940(93)90650-A
Wieland, S., Belluzzi, J. D., Stein, L., and Lan, N. C. (1995). Comparative behavioral characterization of the neuroactive steroids 3α-OH,5α-pregnan-20-one and 3α-OH,5β-pregnan-20-one in rodents. Psychopharmacology. 118, 65–71. doi: 10.1007/BF02245251
Wieser, H. G., and ILAE Commission on Neurosurgery of Epilepsy (2004). ILAE Commission Report. Mesial temporal lobe epilepsy with hippocampal sclerosis. Epilepsia 45, 695–714. doi: 10.1111/j.0013-9580.2004.09004.x
Williams, P. A., White, A. M., Clark, S., Ferraro, D. J., Swiercz, W., Staley, K. J., et al. (2009). Development of spontaneous recurrent seizures after kainate-induced status epilepticus. J. Neurosci. 29, 2103–2112. doi: 10.1523/JNEUROSCI.0980-08.2009
Williamson, J., Mtchedlishvili, Z., and Kapur, J. (2004). Characterization of the convulsant action of pregnenolone sulfate. Neuropharmacology 46, 56–864. doi: 10.1016/j.neuropharm.2003.11.029
Wohlfarth, K. M., Bianchi, M. T., and Macdonald, R. L. (2002). Enhanced neurosteroid potentiation of ternary GABAA receptors containing the δ subunit. J. Neurosci. 22, 1541–1549.
Woolley, C. S. (2000). Estradiol facilitates kainic acid-induced, but not flurothyl-induced, behavioral seizure activity in adult female rats. Epilepsia 41,510–515. doi: 10.1111/j.1528-1157.2000.tb00203.x
Wright, D. W., Kellermann, A. L., Hertzberg, V. S., Clark, P. L., Frankel, M., Goldstein, F. C., et al. (2007). ProTECT: a randomized clinical trial of progesterone for acute traumatic brain injury. Ann. Emerg. Med. 49, 391–402. doi: 10.1016/j.annemergmed.2006.07.932
Wu, F. S., Gibbs, T. T., and Farb, D. H. (1991). Pregnenolone sulfate: a positive allosteric modulator at the N-methyl-D-aspartate receptor. Mol. Pharmacol. 40, 333–336.
Xiao, G., Wei, J., Yan, W., Wang, W., and Lu, Z. (2008). Improved outcomes from the administration of progesterone for patients with acute severe traumatic brain injury: a randomized controlled trial. Crit. Care 12, R61. doi: 10.1186/cc6887
Yagami, T., Ueda, K., Asakura, K., Hayasaki-Kajiwara, Y., Nakazato, H., Sakaeda, T., et al. (2002). Group IB secretory phospholipase A2 induces neuronal cell death via apoptosis. J. Neurochem. 81, 449–461. doi: 10.1046/j.1471-4159.2002.00800.x
Keywords: epilepsy, epileptogenesis, neurosteroid, estrogen, progesterone, kindling
Citation: Reddy DS (2013) Role of hormones and neurosteroids in epileptogenesis. Front. Cell. Neurosci. 7:115. doi: 10.3389/fncel.2013.00115
Received: 18 April 2013; Accepted: 01 July 2013;
Published online: 31 July 2013.
Edited by:
Roberto Di Maio, University of Pittsburgh, USAReviewed by:
Corette J. Wierenga, Utrecht University, NetherlandsJafri Malin Abdullah, Universiti Sains Malaysia, Malaysia
Copyright: © 2013 Reddy. This is an open-access article distributed under the terms of the Creative Commons Attribution License (CC BY). The use, distribution or reproduction in other forums is permitted, provided the original author(s) or licensor are credited and that the original publication in this journal is cited, in accordance with accepted academic practice. No use, distribution or reproduction is permitted which does not comply with these terms.
*Correspondence: D. Samba Reddy, Department of Neuroscience and Experimental Therapeutics, College of Medicine, Texas A&M University Health Science Center, 8447 State Highway 47, MREB Building, Bryan, TX 77807, USA e-mail:cmVkZHlAbWVkaWNpbmUudGFtaHNjLmVkdQ==