- 1Department of Neurophysiology, Hamamatsu University School of Medicine, Hamamatsu, Shizuoka, Japan
- 2Department of Neurophysiology, Hirosaki University Graduate School of Medicine, Hirosaki, Aomori, Japan
- 3Department of Chemistry, Hamamatsu University School of Medicine, Hamamatsu, Shizuoka, Japan
- 4Department of Genetic and Behavioral Neuroscience, Gunma University Graduate School of Medicine, Maebashi, Gunma, Japan
γ-Aminobutyric acid (GABA) depolarizes embryonic cerebrocortical neurons and continuous activation of the GABAA receptor (GABAAR) contributes to their tonic depolarization. Although multiple reports have demonstrated a role of GABAAR activation in neocortical development, including in migration, most of these studies have used pharmacological blockers. Herein, we performed in utero electroporation in GABA synthesis-lacking homozygous GAD67-GFP knock-in mice (GAD67GFP/GFP) to label neurons born in the ventricular zone. Three days after electroporation, there were no differences in the distribution of labeled cells between the genotypes. The dose–response properties of labeled cells to GABA were equivalent among genotypes. However, continuous blockade of GABAAR with the GABAAR antagonist SR95531 accelerated radial migration. This effect of GABAAR blockade in GAD67GFP/GFP mice suggested a role for alternative endogenous GABAAR agonists. Thus, we tested the role of taurine, which is derived from maternal blood but is abundant in the fetal brain. The taurine-evoked currents in labeled cells were mediated by GABAAR. Taurine uptake was blocked by a taurine transporter inhibitor, 2-(guanidino)ethanesulfonic acid (GES), and taurine release was blocked by a volume-sensitive anion channel blocker, 4-(2-butyl-6,7-dichlor-2-cyclopentylindan-1-on-5-yl) oxobutyric acid, as examined through high-performance liquid chromatography. GES increased the extracellular taurine concentration and induced an inward shift of the holding current, which was reversed by SR95531. In a taurine-deficient mouse model, the GABAAR-mediated tonic currents were greatly reduced, and radial migration was accelerated. As the tonic currents were equivalent among the genotypes of GAD67-GFP knock-in mice, taurine, rather than GABA, might play a major role as an endogenous agonist of embryonic tonic GABAAR conductance, regulating the radial migration of neurons in the developing neocortex.
Introduction
During the development of the nervous system, cortical neurons are generated in the ventricular zone (VZ; Sidman and Rakic, 1973; Caviness and Rakic, 1978). After terminal mitosis, neurons derived from the VZ migrate radially through the cell-sparse intermediate zone (IZ) into the cortical plate (CP) to give rise to glutamatergic neurons of the neocortex, such as pyramidal and stellate neurons (Tan et al., 1998; Andjelic et al., 2009). During the early developmental stage of cortical neurons, functional GABAA receptors (GABAAR) are present on both proliferative and early postmitotic cells (LoTurco et al., 1995; Owens et al., 1996). Furthermore, GABAAR activation can regulate the migration of neuronal precursors (Komuro and Rakic, 1998; Nadarajah and Parnavelas, 2002; Marin and Rubenstein, 2003). In the developing cerebral cortex, activation of GABAAR modulates radial migration as a stop signal (Behar et al., 1998; Heck et al., 2007). GABAARs on neural precursor cells in the postnatal subventricular zone (SVZ) are activated tonically, which reduces the migration speed of the cells (Bolteus and Bordey, 2004; Bolteus et al., 2005). Tonic activation of GABAARs is also evident in embryonic neurons before synapse formation has occurred (Valeyev et al., 1993; LoTurco et al., 1995; Owens et al., 1999; Demarque et al., 2002). The effects of GABAAR activation on neuronal migration have been described using GABAAR antagonists in cultured brain slices and dissociated cells (Behar et al., 1996, 1998, 2000; Bolteus and Bordey, 2004). However, most reports have only focused on the role of GABAAR in cortical cell migration assuming that under natural conditions, these receptors are activated by GABA. However, this hypothesis has never been directly tested, and the roles of other endogenous GABAAR agonists have not been addressed.
GABA is one of the earliest neurotransmitters detectable in the developing central nervous system (CNS; Lauder et al., 1986; Behar et al., 1993; Ma and Barker, 1995) and is synthesized from glutamic acid by glutamic acid decarboxylase (GAD). The GAD67 isoform of GAD is expressed in GABAergic interneurons. GAD67-expressing cells originating from ganglionic eminences migrate tangentially and become distributed in the developing cerebral cortex (Tamamaki et al., 2003). It is generally thought that GABA from GAD67-expressing cells activates GABAARs and plays an important role in radial migration. However, the gross structure of the brain appears normal in new-born GAD67 knock-out mice (Asada et al., 1997; Ji et al., 1999), which is inconsistent with a role of GABA in radial migration. Thus, it is also possible that the GABAARs of radially migrating cells may be activated by endogenous ligands other than GABA.
In the CNS, taurine (2-aminoethanesulfonic acid) is the most abundant amino acid (Kontro et al., 1984; Huxtable, 1989; Benitez-Diaz et al., 2003). Taurine is an endogenous partial agonist of the GABAAR and induces chloride currents in neurons (Linne et al., 1996; Ye et al., 1997). Although the capacity to synthesize taurine is low during fetal life (Hayes and Sturman, 1981; Kuo and Stipanuk, 1984; Ghisolfi, 1987), taurine levels are higher in the immature brain than in the adult brain (Kaczmarek, 1976; Benitez-Diaz et al., 2003). In mammals, sufficient taurine for embryonic development is received from the mother via the placenta, while neonates obtain taurine from their mothers’ milk (Sturman et al., 1977; Sturman, 1981). Taurine exhibits physiological actions as a trophic factor and as a neuromodulator during the development of the CNS (Sturman, 1993; Oja and Saransaari, 1996; Hernandez-Benitez et al., 2012). In taurine-deficient kittens, the migration of granule cells from the external granule cell layer to the inner layers of the cerebellum is delayed (Sturman et al., 1985). Although the physiological properties of taurine in the developing brain have been described, it is not yet known whether endogenous taurine activates GABAARs and modulates radial migration in the developing cerebral cortex.
In the present study, we hypothesized that taurine activates GABAAR tonically and affects radial migration of neurons in the developing cerebral cortex. Similar to GAD67 knock-out mice, GABA levels are decreased in heterozygous (GAD67+/GFP) and homozygous (GAD67GFP/GFP) GAD67-GFP knock-in mice (Tamamaki et al., 2003; Morishima et al., 2010). Furthermore, taurine synthesis can be suppressed via the intraperitoneal injection of D-cysteinesulfinic acid (D-CSA; Weinstein et al., 1988), and it has been reported that a low maternal taurine level results in low taurine levels in the fetus (Aerts and Van Assche, 2002). Thus, using GAD67-GFP knock-in mice and D-CSA, we examined the effects of the taurine activation of GABAAR on radial migration in the developing mouse cerebral cortex. Our results provide evidence of taurine-mediated tonic activation of GABAARs and reveal that taurine retards radial migration in the developing mouse cerebral cortex.
Materials and Methods
Ethical Approval
All experiments conformed to the guidelines issued by the Hamamatsu University School of Medicine for the ethical use of animals for experimentation, and all efforts were made to minimize the number of animals used and their suffering.
Animals
The generation of GAD67-GFP (Δneo) mice has been described previously (Tamamaki et al., 2003). In the present study, female heterozygous (GAD67+/GFP) mice were placed overnight with male (>9 weeks) GAD67+/GFP mice in a cage under a 12-h light–dark cycle (lights off from 19:00 to 07:00). The day on which a virginal plug was observed in the morning was designated as embryonic day 0.5 (E0.5). Polymerase chain reaction (PCR) amplification was performed to discriminate the genotypes of individuals. For some experiments, we used also Wistar rats purchased from Japan SLC (Hamamatsu, Japan).
In Utero Electroporation
Cells were transfected in vivo through in utero electroporation, as described previously (Inoue et al., 2012). Briefly, plasmids carrying monomeric red fluorescent protein (mRFP) downstream of a CAG promoter (Addgene, MA, USA) were prepared using the EndoFree Plasmid Kit (Qiagen, Hilden, Germany). Pregnant mice and rats were anesthetized with sodium pentobarbital (50 mg/kg intraperitoneally) at E14.5 and E15.5, respectively, and their uterine horns were exposed. Plasmid DNA was dissolved in phosphate buffered saline (PBS) at a final concentration of 0.5 μg/μl with Fast Green (final concentration 0.05% [v/v]). Plasmids were injected into the lateral ventricle using a glass micropipette and a controlled pipette system (IM-30, Narishige, Tokyo, Japan). The micropipettes were generated from glass capillaries (outer diameter 1.0 mm; Harvard Apparatus, South Natick, MA, USA) that were pulled using a P-97 micropipette puller (Sutter Instrument Co., Novato, CA, USA). Electric pulses were produced by an electroporator (CUY21EDIT; NepaGene, Ichikawa, Japan) and delivered by a round plate forceps-type electrode with a 5-mm diameter (CUY650P5; NepaGene). Electric pulses (43 V, 50 ms) were applied five times at intervals of 950 ms. The uterine horns were then returned to the abdomen.
Implantation of PLGA for Sustained Drug Administration In Vivo
Copoly(d,l-lactic/glycolic acid) (PLGA) exhibits high bio-affinity and biodegradability and is therefore a useful material for achieving sustained drug release (Miller et al., 1977). PLGA (20 mg; Wako, Osaka, Japan) was dissolved in 100 μl of N-methyl-2-pyrrolidone (Wako; Kranz et al., 2001), and 10 μl of 10 mM SR95531 or water (Control) was added. Following in utero electroporation, the PLGA solution or SR95531-adsorbed PLGA (0.5 μl) was injected into the lateral ventricles of fetuses.
Analysis of Radial Migration Based on Distribution Patterns of Neurons
Fetuses were killed at E17.5 and decapitated, and their brains were dissected out. The brains were fixed in 4% paraformaldehyde for 3 h at 4°C and then transferred to 30% sucrose phosphate buffer (0.1 M, pH 7.4) and left immersed for 3 days. The brains were then sectioned coronally at a thickness of 30 μm using a cryostat and counterstained with 4′,6-diamidino-2-phenylindole (DAPI) to indicate proliferative zones, after which the sections were transferred to slides and coverslipped. Images were subsequently captured using a cooled charge-coupled device (CCD) camera (Orca ER-G; Hamamatsu photonics, Hamamatsu, Japan) attached to an epifluorescence microscope (BX-51; Olympus, Tokyo, Japan). The E17.5 neocortex is laminated into the marginal zone (MZ), CP, subplate (SP), IZ, and SVZ/VZ (Shinozaki et al., 2007). Based on the cytoarchitecture revealed by DAPI counterstaining, the regions with abundant cells were considered the SVZ/VZ and CP. The IZ and SP were defined as the regions between these areas (Caric et al., 1997; Inoue et al., 2012). The boundary between the IZ and SP was assessed based on the DAPI signal density, which was higher in the IZ than the SP. To determine the distribution pattern of migrating neurons, all of the red fluorescent protein (RFP)-positive cells in the cortex of each section were counted. The area in which GFP-positive cells were counted was approximately 300 μm wide and included the full thickness of the cortex. The numbers of RFP- or GFP-positive cells in the MZ, CP, SP, IZ, and SVZ/VZ were counted using ImageJ software (National Institutes of Health, Bethesda, MD, USA). The RFP- or GFP-positive cells in each area were counted and are presented as the ratio to the number of counted cells in a slice.
Histology and Immunohistochemistry
Taurine localization was observed immunohistochemically. The fetal neocortex of E17.5 mice was fixed via perfusion with 4% paraformaldehyde/0.5% glutaraldehyde in 0.1 M Tris buffer (TB). The brains were removed and immersed in the same fixative for 3 h at 4°C. After post-fixing, the brains were transferred to 15% sucrose TB for 1 h and 30% sucrose TB for 3 days. Tissues were cut coronally with a cryostat into sections with a thickness of 30 μm and rinsed in Tris-buffered saline (TBS). The sections were first treated with 0.5% NaBH4, then incubated for 0.5 h in 0.3% H2O2 in TBS and subsequently with an antibody against taurine (TT100, polyclonal rabbit IgG; Signature Immunologics, Salt Lake City, UT, USA) for 36 h at 4°C. After rinsing in TBS, the sections were incubated for 1 h with a secondary antibody against rabbit IgG (anti-rabbit biotinylated, BA1000; Vector Laboratories, Burlingame, CA, USA). The primary and secondary antibodies were diluted in TBS containing 2% horse serum albumin and 0.1% Triton X-100. Visualization was performed using the Biotin/Avidin system (Vector Laboratories). Sections were treated in diaminobenzidine (DAB). After rinsing, the sections were mounted on slides and coverslipped and finally observed under a microscope (AX-80; Olympus) equipped with a CCD camera (EP-70; Olympus).
Electron Microscopy
After deeply anesthetizing the animals via hypothermia and perfusing them transcardially with 4% paraformaldehyde/0.5% glutaraldehyde in 0.1 M 4-(2-hydroxyethyl)-1-piperazineethanesulfonic acid (HEPES) buffer, their brains were removed and post-fixed for 10 min. Coronal sections (50 μm) were cut with a vibratome (DTK-1500; Dosaka, Kyoto, Japan) in the same fixative solution. The sections were incubated in 0.1 M HEPES buffer for 1 day at 4°C. After rinsing in PBST (0.1% Triton X-100/0.1 M PBS) three times, the sections were incubated for 1 h in blocking buffer containing 2% horse serum albumin and 0.1% Triton X-100, then incubated for 36 h at 4°C with a polyclonal antibody against taurine (Signature Immunologics). The sections were subsequently washed with PBS three times, incubated in blocking buffer for 1 h at room temperature, and then treated with an anti-rabbit secondary antibody coupled with 1.4-nm diameter gold particles (Nanogold; Nanoprobes, Yaphank, NY, USA) overnight at room temperature. After this step, an immunoelectron microscopic analysis was performed by Tokai Electron Microscopy Inc. (Nagoya, Japan). After the sections were washed with PBS and fixed in 2% glutaraldehyde in 0.1 M sodium cacodylate buffer (pH 7.4) for 3 h at room temperature, the gold particles were enlarged for microscopic examination with the HQ-Silver Enhancement Kit (Nanoprobes) and routinely processed for electron microscopic examination. The embedded samples were sectioned (thickness 80 nm) with an ultra-microtome (LKB2088; LKB, Bromma, Sweden) and stained with uranyl acetate and lead citrate. The sections were then carbon-coated in a vacuum and observed with a JEOL transmission electron microscope (JEM 2000EX; JEOL, Tokyo, Japan) at 100 kV.
Acute Slice Preparation
Coronal cortical slices were obtained from mouse fetuses at E17.5 (or rat fetuses at E18.5). The maternal mice or rats were deeply anesthetized via inhalation of halothane, and the fetal brains were rapidly removed and placed in cold (4°C), oxygenated, modified artificial cerebrospinal fluid (ACSF). The solution contained the following components (in mM): 220 sucrose, 2.5 KCl, 1.25 NaH2PO4, 10.0 MgSO4, 0.5 CaCl2.2H2O, 26.0 NaHCO3, and 30.0 glucose (330–340 mOsm). Coronal slices (400 μm) were cut in the modified ACSF using a vibratome (VT-1000; Leica, Wetzlar, Germany). The slices were maintained in oxygenated standard ACSF consisting of (in mM) 126 NaCl, 2.5 KCl, 1.25 NaH2PO4, 2.0 MgSO4, 2.0 CaCl2, 26.0 NaHCO3, and 20.0 glucose gassed with 95% O2–5% CO2 at room temperature prior to recording. Individual slices were transferred to a recording chamber, perfused (2 ml/min) with pre-gassed standard ACSF, and maintained at 30°C.
Electrophysiology
In all of the electrophysiological experiments, D-(-)-2-amino-5-phosphonopentanoic acid (D-AP5, 50 μM), 6-cyano-7-nitroquinoxaline-2,3-dione (CNQX, 10 μM), CGP55845 (3 μM), and tetrodotoxin (TTX, 0.5 μM) were added to the ACSF. Patch electrodes were fabricated from 1.5-mm diameter borosilicate capillary tubing (GD-1.5; Narishige) using a P-97 horizontal puller (Sutter Instruments). The electrode resistance ranged from 7 to 10 MΩ when the electrode was filled with a solution containing (in mM) 110 CsCl, 6 MgCl2, 10 ethylene glycol tetraacetic acid (EGTA), 10 HEPES, 4 K2-ATP, 20 K2-phosphocreatine, and 50 U creatine phosphokinase. The pH was adjusted to 7.3 with CsOH. Under these experimental conditions, GABAergic IPSCs (inhibitory postsynaptic currents) reversed at approximately 0 mV (the ECl predicted by the Nernst equation). With the cells voltage-clamped at -60 mV, the GABAergic IPSC appeared as an inward current. The recorded currents were filtered at 2 kHz and digitized at 1–10 kHz using DigiData1322A and pCLAMP9 software (Molecular Devices, Sunnyvale, CA, USA). GABA, taurine, and glycine were added through another pipette to the recorded cell soma using a puffer application system (IM-300; Narishige). The data were analyzed using Clampfit9 (Molecular Devices). Dose–response relationships for GABA were fitted to the Hill equation: where I is the peak current measured at a given concentration of GABA; Imax is the current at the maximal GABA concentration; the EC50 is the half-maximal effective GABA concentration; and n is the Hill coefficient. Ligand applications were separated by 3 min intervals to allow recovery from desensitization when present.
Measurement of Ambient GABA and Taurine
Ambient GABA and taurine levels were measured using high-performance liquid chromatography (HPLC) methods. Following the preparation of tissue slices, the cerebral cortex was cut from two coronal slices. The cortices were equilibrated for 1 h in a holding chamber filled with continuously oxygenated ACSF. In taurine loading experiments, 1 or 10 mM taurine was dissolved in the ACSF. After 1 h of incubation with or without taurine, the cortices were rinsed thoroughly and placed in a submerged-type small chamber filled with ACSF that was oxygenated and filtered (Minisart; Sartorius, Göttingen, Germany) and incubated for 1 h at 30°C. Hypotonic medium consisting of (in mM) 31 NaCl, 2.5 KCl, 1.25 NaH2PO4, 2.0 MgSO4, 2.0 CaCl2, 26.0 NaHCO3, and 20.0 glucose gassed with 95% O2–5% CO2, was used for hypotonic stimulation. In some experiments, drugs were dissolved in ACSF in the small chamber to inhibit taurine transporter or anion channel. The supernatant (120 μl) was subsequently collected and stored at -80°C until HPLC analysis. After the samples were derivatized with o-phthalaldehyde, the concentrations of amino acid neurotransmitters were determined via reverse-phase HPLC with electrochemical detection. The HPLC system (BAC-300 system; EICOM, Kyoto, Japan) consisted of an EP-300 pump, an ECD-300 electrode and a PowerChrom system (ADInstruments, Sydney, Australia). Separation of amino acid derivatives was achieved using an Eicompack MA-5ODS column (150 mm × 3 mm, EICOM) kept at 30°C. The detection potential was set at +700 mV against an Ag/AgCl reference electrode. The flow rate was 0.5 ml/min, and the sensitivity was set at 64 nA/V full-scale. The mobile phase consisted of (in mM) 86.2 NaH2PO4.2H2O, 13.8 Na2HPO4.12H2O, 0.015 EDTA.2Na, and 30% (v/v) methanol at pH 6.0 and was membrane filtered (0.45 μm) and degassed (DG-300; EICOM) at a flow rate of 0.5 ml/min (EP-300). The amino acids and the derivatives were mixed and allowed to react for exactly 2 min before injection. The amino acid derivatives were then detected electrochemically. Sample concentrations were determined based on comparison of peak areas with that of an external standard.
D-Cysteinesulfinic Acid Administration
Taurine is formed via the oxidation of hypotaurine, an intermediate generated by L-type cysteinesulfinic acid (L-CSA) decarboxylation. L-CSA is formed by the oxidation of cysteine and is the main taurine precursor in rodents. D-CSA is used as a metabolism-resistant, potent and specific inhibitor of L-CSA decarboxylase (Weinstein et al., 1988; Dominy et al., 2004). After electroporation, D-CSA (15 mmol/kg) was administered every 12 h via maternal intraperitoneal injection. As a control group, saline was administered to other mice at the same interval. D-CSA was custom synthesized from D-cysteine using D-cysteine thiosulfonate according to a previously reported procedure (Emiliozzi and Pichat, 1959).
Drugs
The following drugs were used: SR95531, bicuculline methiodide, and strychnine (Sigma-Aldrich, St. Louis, MO, USA); CNQX, D-AP5, picrotoxin, bumetanide, and 2-(guanidino)ethanesulfonic acid (GES), CGP55845 (Tocris Bioscience, Bristol, UK); PLGA and TTX (Wako); 4-(2-butyl-6,7-dichlor-2-cyclopentylindan-1-on-5-yl) oxobutyric acid (DCPIB; Santa Cruz Biotechnology, Santa Cruz, CA, USA); 4,4′-diisothiocyanatostilbene-2,2′-disulfonic acid (DIDS; Invitrogen, Carlsbad, CA, USA); and D-cystine (Acros Organics, Fair Lawn, NJ, USA).
Statistics
Unless otherwise indicated, all numerical data are presented as the mean ± SEM. Differences between two groups were assessed using Student’s t-test for absolute values and the Mann–Whitney U (MWU)-test for normalized values. Comparisons among several groups were performed via one-way ANOVA. The distribution patterns of radially migrating cells were compared using chi-square tests (χ2-tests). Differences were considered statistically significant at P < 0.05.
Results
The Distribution of Radially Migrating Cortical Plate Cells does not Differ between Wild-Type and GAD67-GFP Knock-in Mice
We previously demonstrated that the ambient levels of GABA in the cerebral cortices of heterozygous (GAD67+/GFP) and homozygous (GAD67GFP/GFP) GAD67-GFP knock-in fetuses were 67.8 and 12.7%, respectively, of the level in wild-type (GAD67+/+) fetuses (Morishima et al., 2010). Thus, GAD67-GFP knock-in mice are suitable for investigating the influence of decreased ambient GABA levels on the radial migration of neurons during neocortical development. We performed in utero electroporation to label radially migrating neurons with RFP at E14.5. Three days after electroporation (E17.5), we analyzed the distribution of RFP- and GFP-positive cells across the proliferative and the migratory zones of the neocortex in cortical slices. The boundaries of individual zones were determined based on differences in cell density revealed by DAPI counterstaining (Figure 1C). RFP-positive cells were mainly distributed in the CP, SP, and IZ in all three genotypes of fetuses (Figure 1A). In GAD67+/GFP and GAD67GFP/GFP fetuses, GFP-positive cells were also distributed in the MZ (Figure 1B). There were no cells expressing both RFP and GFP, confirming that the radially migrating neurons were different from the GABAergic neurons. The RFP- and GFP-positive cells in each region were counted and are presented as the percentages of total RFP- and GFP-positive cells in the neocortex, respectively (Figures 1D,E). There were no significant differences in the distribution patterns of RFP- and GFP-positive cells between the genotypes (P = 0.925 for RFP-positive cells, Figure 1D; P = 0.833 for GFP-positive cells, Figure 1E; χ2-test). Thus, these data suggest that the radial migration of neurons in the neocortex was not affected by decrements in ambient GABA levels.
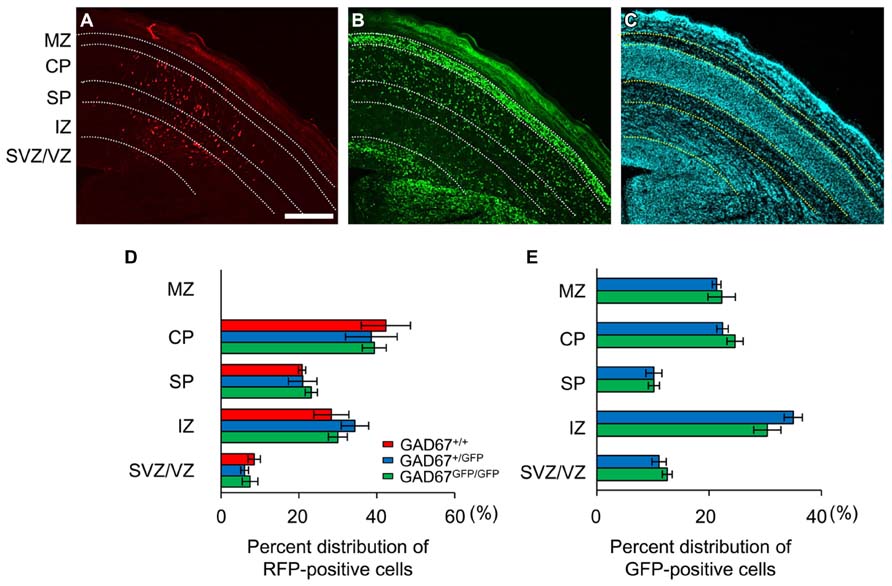
FIGURE 1. Distribution patterns of radially migrating neurons and tangentially migrating GABAergic neurons in the cerebral cortex at E17.5. (A) Distribution of radially migrating neurons expressing mRFP (red) in a coronal section of the neocortex of a GAD67+/GFP fetus. (B) Distribution of GABAergic neurons expressing GFP (green) in the same section. (C) The section was counterstained with DAPI. The dotted lines in (A–C) indicate the boundaries of the proliferative and migratory zones. The scale bar represents 200 μm. MZ, marginal zone; CP, cortical plate; SP, subplate; IZ, intermediate zone; SVZ, subventricular zone; VZ, ventricular zone. (D) Percent distributions of RFP-positive cells in the CP, SP, IZ, and SVZ/VZ averaged over five GAD67+/+ fetuses, four GAD67+/GFP fetuses, and five GAD67GFP/GFP fetuses. (E) Percent distributions of GFP-positive cells in the MZ, CP/SP, IZ, and SVZ/VZ (GAD67+/+: n = 4; GAD67+/GFP: n = 5). Error bars indicate the mean ± SEM.
The Sensitivity of GABAARs in Radially Migrating Neurons does not Differ between Wild-Type and GAD67-GFP Knock-in Mice
Previous studies have shown that functional GABAARs are present in developing neocortical cells (LoTurco et al., 1995; Owens et al., 1996). To examine the sensitivity of GABAARs to GABA in wild-type and GAD67-GFP knock-in fetuses, we examined the currents elicited via puff application of GABA in RFP-positive, radially migrating neurons in all three genotypes. Under whole-cell voltage-clamp conditions at -60 mV, the application of GABA (3–300 μM) produced inward currents in all of the tested RFP-positive neurons (Figure 2A). The dose dependence of the peak amplitude of the current on GABA was fitted with a Hill equation (see Materials and Methods), and the dependence was examined in each plate/zone of the neocortex. The EC50s and Hill coefficients of the current responses were approximately 18 μM and 1.3, respectively, in all GAD67+/+, GAD67+/GFP, and GAD67GFP/GFP fetuses, and there were no significant differences in these values between the genotypes or between the plates and zones (Figure 2B). Thus, these data indicate that the sensitivity of GABAARs between the genotypes does not differ at any of the migration stages of radially migrating neurons.
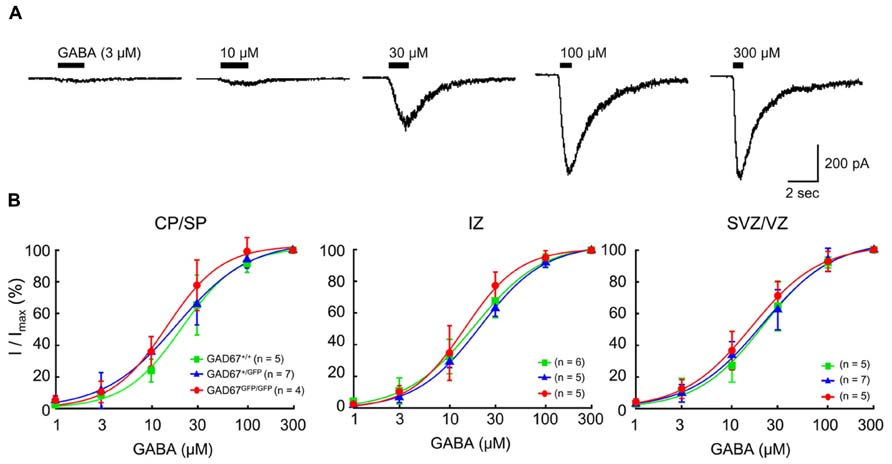
FIGURE 2. The sensitivity of GABAARs to GABA in RFP-positive cells in the E17.5 cerebral cortex does not differ between wild-type and GAD67-GFP knock-in mice. (A) Representative GABA-evoked currents recorded in an RFP-positive cell from a GAD67+/+ fetus. The holding potential (VH) was -60 mV. The puff application of GABA was stopped immediately after desensitization was initiated. (B) Dose–response curves of GABA currents. The EC50s of the current responses in GAD67+/+, GAD67+/GFP, and GAD67GFP/GFP fetuses were 16.23 ± 1.75, 17.46 ± 2.11, and 19.22 ± 2.43 μM, respectively, in the CP/SP (P = 0.657 by ANOVA); 16.98 ± 1.80, 20.28 ± 1.13, and 18.53 ± 2.64 μM, respectively, in the IZ (P = 0.512); and 16.24 ± 2.61, 18.79 ± 1.66, and 20.39 ± 2.12 μM, respectively, in the SVZ/VZ (P = 0.422). The Hill coefficients were 1.36 ± 0.13, 1.26 ± 0.08, and 1.34 ± 0.07, respectively, in the CP/SP (P = 0.696); 1.49 ± 0.11, 1.28 ± 0.10, and 1.28 ± 0.08, respectively, in the IZ (P = 0.295); and 1.33 ± 0.11, 1.28 ± 0.10, and 1.23 ± 0.16, respectively, in the SVZ/VZ (P = 0.891). Error bars: mean ± SEM.
Blockade of GABAARs In Vivo Increases the Radial Migration Speed in GAD67GFP/GFP Mice
We showed that the distribution pattern and GABAAR sensitivity of radially migrating neurons were normal, even in GABA-deficient GAD67GFP/GFP mice (Figures 1 and 2). These results suggest the possibility that GABAARs might not be involved in radial migration. To test this hypothesis, we continuously administered the selective GABAAR antagonist SR95531 to GAD67GFP/GFP fetuses via intra-ventricular injection. SR95531-adsorbed PLGA was injected into the lateral ventricles of GAD67GFP/GFP fetuses immediately after in utero electroporation at E14.5. Three days after PLGA injection, the distribution pattern of RFP-positive cells was analyzed. SR95531 administration significantly altered the distribution pattern of RFP-positive cells compared with the Control injected with drug-free PLGA (P = 0.049 by χ2-test, n = 6 in Control, n = 6 in SR95531; Figure 3). The numbers of RFP-positive cells were significantly increased in the CP and decreased in the SP and IZ following SR95531 administration (P = 0.001 in CP, P = 0.014 in SP, P = 0.002 in IZ by MWU-test, n = 6 in Control, n = 6 in SR95531; Figure 3B). This indicates an acceleration of radial migration induced by SR95531 in GAD67GFP/GFP fetuses, suggesting that under normal conditions, GABAARs are activated and play a role in slowing the radial migration speed, even in GAD67GFP/GFP fetuses, where the ambient GABA level in the neocortex is very low. These data suggest that another endogenous GABAAR agonist might activate the GABAARs of radially migrating cells.
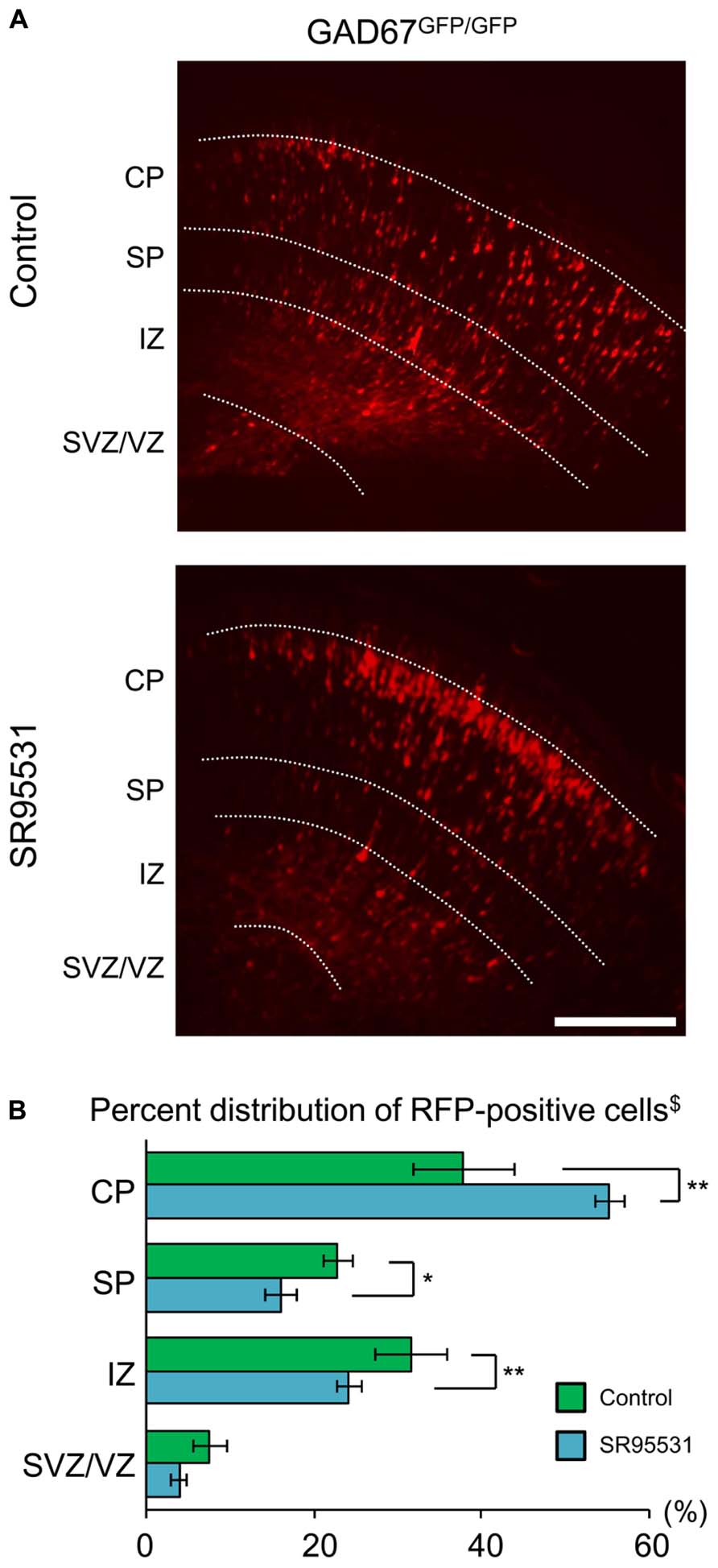
FIGURE 3. Sustained administration of a GABAAR antagonist facilitates radial migration in GAD67GFP/GFP fetuses. (A) Photomicrographs showing the distribution of RFP-positive cells in Control (upper) and SR95531-treated (lower) GAD67GFP/GFP fetuses at E17.5. PLGA with or without SR95531 was injected into the lateral ventricles of GAD67GFP/GFP fetuses at E14.5, immediately after the electroporation of RFP vectors. (B) Proportions of radially migrating cells in the CP, SP, IZ, and SVZ/VZ. The distribution pattern of RFP-positive cells in SR95531-treated GAD67GFP/GFP fetuses was significantly different from that in Control, untreated fetuses (χ2-test, $P < 0.05; Control: n = 6, SR95531-treated: n = 6). The percentage of RFP-positive cells was increased in the CP and decreased in the SP and IZ by SR95531 administration (*P < 0.05, **P < 0.01). Error bars: mean ± SEM. Scale bar: 200 μm.
Localizations of Taurine and Taurine Transporters in The MZ and SP of the Developing Cerebral Cortex at E17.5
Next, we examined the hypothesis that the GABAAR agonist taurine might play a role in regulating radial migration in GAD67GFP/GFP fetuses. To investigate taurine localization in the developing cerebral cortex at E17.5, we performed immunohistochemical analyses in GAD67+/+ mice. Immunoreactivity for taurine was prominent in the MZ and SP (Figures 4A–C). To further identify the precise location of taurine in the cells, we next performed immunoelectron microscopic observations using a taurine antibody. Taurine immunoreactivity was present intracellularly and was diffusely located in the cytosol (Figure 4D). In the examined specimens, neither presynaptic structures such as synaptic vesicles nor postsynaptic densities were observed.
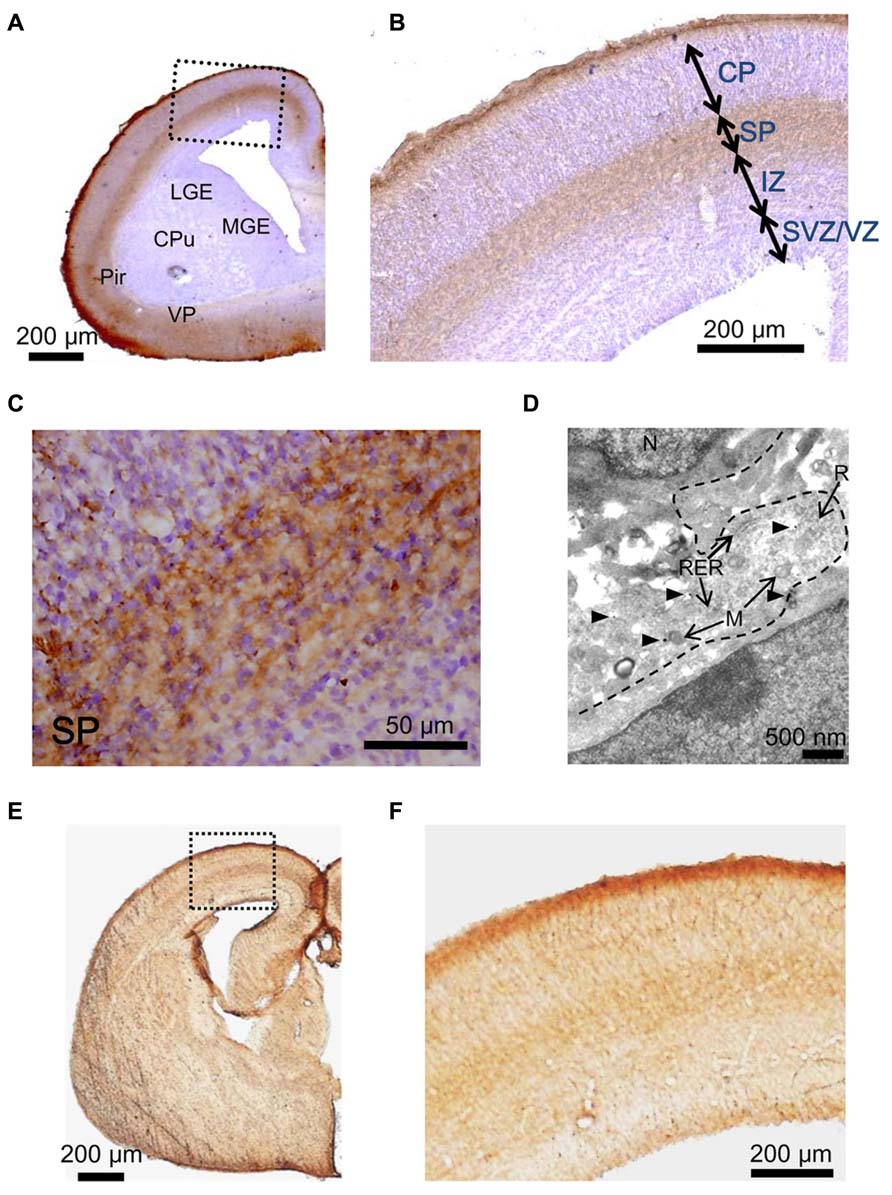
FIGURE 4. Immunostaining to detect taurine and the taurine transporter in the cerebral cortices of GAD67+/+ fetuses at E17.5. (A) Photomicrograph showing the distribution of taurine in a coronal section of the cortex. The section was counterstained with hematoxylin and eosin (blue). CPu, caudate putamen; LGE, lateral ganglionic eminence; MGE, medial ganglionic eminence; VP, ventral pallidum; Pir, piriform cortex. (B) Enlarged view of the boxed area shown in (A). (C) Higher magnification view of the SP area shown in (B). (D) Electron micrograph of a taurine-immunolabeled section. Arrowheads indicate gold particles. M, mitochondria; N, nucleus; R, ribosome; RER, rough endoplasmic reticulum. (E) Photomicrograph of the distribution of the taurine transporter in a coronal section of the cortex. (F) Enlarged view of the boxed area shown in (E).
Taurine is transported into cells by taurine transporters, which have been reported to be expressed in the developing brain (Smith et al., 1992). The localization of taurine transporters in the cerebral cortex was also examined in GAD67+/+ fetuses at E17.5. Immunoreactivity for taurine transporters was observed in the MZ and SP, which are the same regions where taurine immunoreactivity was found (Figures 4E,F). These data suggest that taurine accumulates in cells in the MZ and SP, where it may be released to activate GABAARs on radially migrating cells.
Taurine Evokes GABAAR-Mediated Currents in Radially Migrating Cells
Taurine is known to act as an agonist of glycine receptors (GlyRs). GlyRs have been reported to be activated non-synaptically during early neocortical development in rat fetuses (Flint et al., 1998). In addition, taurine may act as a full agonist or a partial agonist of GABAARs (Dominguez-Perrot et al., 1996). To test whether taurine activates GlyRs or GABAARs in radially migrating neurons, we compared the currents evoked by the application of GABA, taurine, and glycine in RFP-positive cells located in the SP of the cortices of GAD67+/+ fetuses at E17.5 and examined the effects of blockers of GABAARs and GlyRs on these currents. The peak amplitude of GABA (10 μM)-evoked inward currents was 46.20 ± 7.78 pA/pF (n = 10; Figures 5A,D, left), and these currents were not blocked by the selective GlyR antagonist strychnine (10 μM: 91.29 ± 6.01%, P = 0.161 by paired t-test, n = 10; Figures 5A,D,E, left), whereas they were blocked by SR95531 (10 μM: 7.54 ± 1.41%, P < 0.001, n = 9; Figures 5A,D,E, left). Indeed, taurine (10 mM) evoked inward currents (13.13 ± 1.57 pA/pF, n = 10; Figures 5B,D, left), which were also not blocked by strychnine (94.44 ± 7.50%, P = 0.455, n = 10; Figures 5B,D,E, left) but were blocked by SR95531 (17.42 ± 2.32%, P < 0.001, n = 9; Figures 5B,D,E, left). The application of glycine (3 mM) did not produce any currents in RFP-positive cells (1.45 ± 0.18 pA/pF, n = 10; Figures 5C,D, left). Thus, radially migrating neurons in fetal mouse cortices do not exhibit functional GlyRs, and taurine activates GABAARs in these neurons.
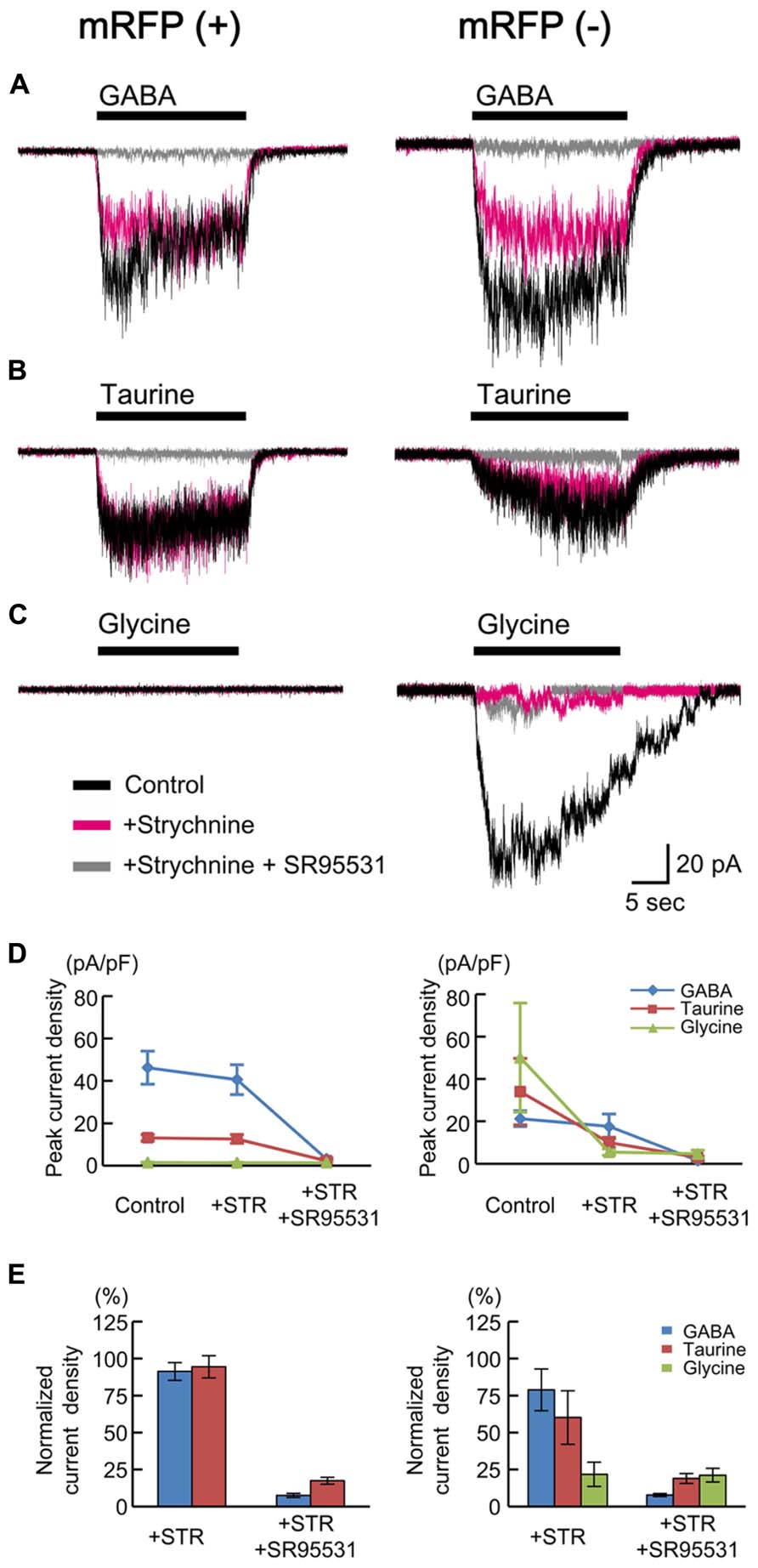
FIGURE 5. GABA-, taurine-, and glycine-evoked currents in RFP-positive and -negative cells in GAD67+/+ fetuses. Typical traces of the currents evoked by the applications of 10 μM GABA (A), 10 mM taurine (B), and 3 mM glycine (C) in RFP-positive (left panels) and RFP-negative (right panels) cells are shown. Black traces indicate the currents in the absence of blockers; red traces are the currents obtained after the application of 10 μM strychnine through bath perfusion in the same cells; and gray traces are the currents recorded after the further addition of 10 μM SR95531 to the bath solution. VH was -60 mV. (D) The means ± SEMs of the peak current densities of the agonist-evoked currents in RFP-positive (left panel) and RFP-negative (right panel) cells in the absence and presence of strychnine (STR) and after the addition of SR95531 are plotted. (E) Effects of blockers on agonist-evoked currents. The peak current densities in the absence of blockers were normalized to 100%.
We also examined the currents in RFP-negative cells for comparison and found that glycine could evoke strychnine-sensitive inward currents in six out of seven recorded cells (50.08 ± 25.71 pA/pF, 21.78 ± 8.20% in the presence of strychnine, n = 6; Figures 5C–E, right). Thus, some RFP-negative cells express functional GlyRs. GABA and taurine were able to elicit SR95531-sensitive inward currents in the RFP-negative neurons responsive to glycine, but the taurine-evoked currents were also blocked weakly by strychnine in these neurons (60.14 ± 18.06%, P = 0.205, n = 6; Figures 5B,D,E, right), and the GABA-evoked currents were blocked more weakly (78.84 ± 14.10%, P = 0.372, n = 6; Figures 5A,D,E, right). This indicates that the RFP-negative cells possessing GlyRs also respond to taurine.
The insensitivity of RFP-positive cells to glycine (Figures 5C,D) appears to be inconsistent with findings in rat fetuses (Flint et al., 1998; Nimmervoll et al., 2011). Therefore, we also examined the currents in RFP-positive cells in cortical slices obtained from rat fetuses at E18.5. The peak amplitudes of GABA (10 μM)-evoked inward currents recorded in RFP-positive cells located in the CP of rat cortices were 19.66 ± 3.22 pA/pF (n = 6; Figures 6A,D), and these currents were not blocked by strychnine (10 μM: 74.92 ± 7.84%, P = 0.062 by paired t-test, n = 6; Figures 6A,D,E) but were blocked by SR95531 (10 μM: 14.25 ± 3.07%, P < 0.001, n = 6; Figures 6A,D,E). Taurine (10 mM) also evoked inward currents (22.94 ± 2.87 pA/pF, n = 6; Figures 6B,D), but these currents were found to be significantly blocked by strychnine (29.09 ± 2.63%, P < 0.001, n = 6; Figures 6B,D,E). Additionally, the application of glycine (3 mM) indeed produced strychnine-sensitive inward currents in RFP-positive cells in rat cortices (30.32 ± 4.14 pA/pF, n = 6; blocked by strychnine to 14.13 ± 2.99%, P < 0.001, n = 6; Figures 6C,D,E). Thus, in the rat fetal neocortex, radially migrating neurons express GlyRs (Nimmervoll et al., 2011), and the taurine-evoked inward currents are mainly mediated by these GlyRs.
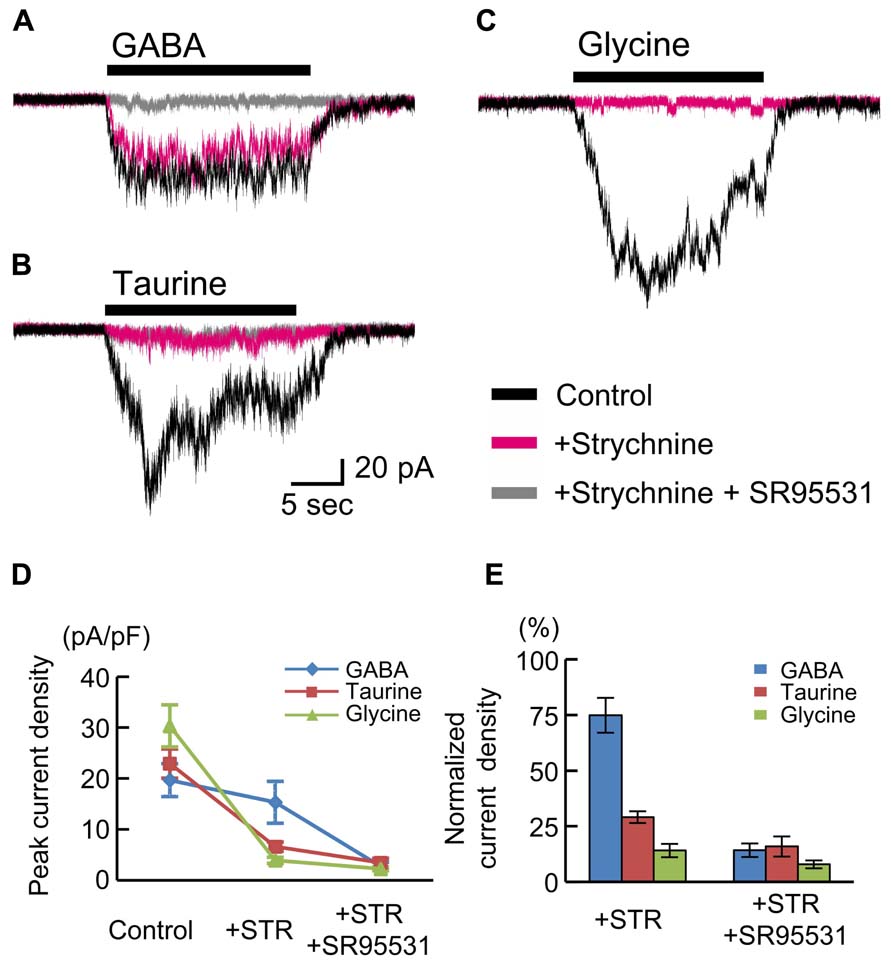
FIGURE 6. GABA-, taurine-, and glycine-evoked currents in RFP-positive cells in rat fetuses at E18.5. Typical traces of the currents evoked by the application of 10 μM GABA (A), 10 mM taurine (B), and 3 mM glycine (C) in RFP-positive cells are shown. Black traces indicate the currents in the absence of blockers; red traces are the currents obtained after the application of 10 μM strychnine through bath perfusion in the same cells; and gray traces are the currents recorded after the further addition of 10 μM SR95531 to the bath solution. VH was -60 mV. (D) The means ± SEMs of the peak current densities in the absence and presence of strychnine (STR) and after the addition of SR95531 are plotted. (E) Effects of blockers on agonist-evoked currents. The peak current densities in the absence of blockers were normalized to 100%.
Endogenous Taurine Activates GABAARs, Producing Tonic Currents in Radially Migrating Neurons
We next examined whether endogenous taurine activates GABAARs in radially migrating cells in the fetal mouse neocortex. GABAAR-mediated tonic currents were recorded under whole-cell voltage-clamp conditions at -60 mV in RFP-positive cells located in the SP region of the developing cortex at E17.5, and the currents were compared between GAD67+/+ and GAD67GFP/GFP fetuses. The GABAAR-mediated components were revealed by the application of 10 μM SR95531 (Figure 7A). There was no difference in the GABAAR-mediated component observed under normal conditions between GAD67+/+ (n = 5) and GAD67GFP/GFP (n = 5) fetuses (P = 0.609 by Student’s t-test; Figure 7C, left bars). The membrane capacitance of the recorded cells was similar between the two types of fetuses (GAD67+/+: 11.29 ± 0.97 pF, GADGFP/GFP: 8.57 ± 2.15 pF, P = 0.283 by Student’s t-test). Application of the taurine transporter inhibitor GES (300 μM) induced an inward shift of the holding currents, which was reversed by the application of SR95531 (Figure 7B). The baseline noise of the currents [root mean square (rms) noise level; GAD67+/+: 1.42 ± 0.09 pA; GADGFP/GFP: 1.80 ± 0.09 pA] was also enhanced by GES treatment (GAD67+/+: 4.58 ± 0.99 pA, P = 0.030 by paired t-test; GADGFP/GFP: 4.07 ± 0.22 pA, P < 0.001 by paired t-test) and suppressed by SR95531 (GAD67+/+: 1.83 ± 0.30 pA, P = 0.025 by paired t-test; GADGFP/GFP: 2.08 ± 0.15 pA, P = 0.001 by paired t-test). Thus, GABAAR activity was increased in the presence of GES, causing an increase in holding currents, while the currents recorded in GAD67+/+ and GAD67GFP/GFP mice showed no significant difference in magnitude (P = 0.303, n = 5; Figure 7C, right bars).
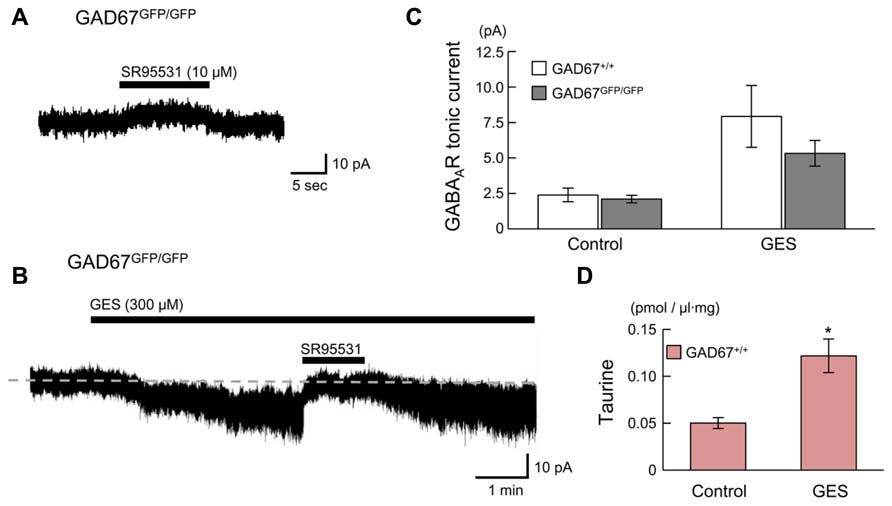
FIGURE 7. GABAARs in radially migrating neurons are activated by endogenous taurine. (A) Typical trace of a GABAAR-mediated tonic current in an RFP-positive cell in the SP under normal conditions. VH was -60 mV. (B) SR95531-sensitive inward current induced by the application of GES. The baseline currents immediately before (for 5 s) the initiation and the cessation of SR95531 application were averaged, to determine the tonic currents based on their difference. (C) Averaged tonic current amplitudes before and after GES application. There were no significant differences between the GAD67+/+ (n = 5) and GAD67GFP/GFP (n = 5) mice. (D) HPLC measurements of the taurine concentrations in the cortical slice incubation medium either without (Control, n = 5) or with GES (300 μM, n = 4). Error bar: mean ± SEM. *P < 0.01.
GES has been reported to activate GABAARs in cerebellar granule cells (Mellor et al., 2000). To confirm whether GES acts on GABAARs or taurine transporters in radially migrating neurons, we measured ambient taurine levels in cortical slices incubated in medium with or without GES via HPLC. The taurine levels in the cortices of GAD67+/+ fetuses were significantly enhanced in the presence of GES (P = 0.004 by Student’s t-test; Figure 7D). Thus, GES blocked taurine transporters in the cortices, thereby blocking the uptake of extracellular taurine into the cells and increasing ambient taurine levels. These data suggest that endogenous taurine can activate GABAARs to produce tonic currents in the developing cerebral cortices of both GAD67+/+ and in GAD67GFP/GFP fetuses. Furthermore, we confirmed that the differences in the expression level of GAD67 between the genotypes did not affect the ambient taurine levels (GAD67+/+: 50.21 ± 5.79 fmol/μl·mg, n = 5; GAD67+/GFP: 46.87 ± 3.85 fmol/μl·mg, n = 6; GAD67GFP/GFP: 45.74 ± 5.16 fmol/μl·mg, n = 4; P = 0.811 by ANOVA). Thus, the presence of GABAAR-mediated tonic currents in GAD67GFP/GFP fetuses under normal conditions with amplitudes similar to those found in GAD67+/+ fetuses (Figure 7C, left bars) suggests that taurine, rather than GABA, contributes to the generation of the tonic currents in GAD67GFP/GFP fetuses.
Maternal Administration of D-CSA Decreases Ambient Taurine Levels and GABAAR-Mediated Tonic Currents in both Wild-Type and GAD67 Knock-in Fetuses
If taurine is the major endogenous agonist of GABAARs in GAD67GFP/GFP fetuses, inhibition of taurine production in the mother would have an effect on radial migration in the fetuses, similar to the effect of blockade of GABAARs; i.e., facilitation of migration (Figure 3). To investigate this hypothesis, we intraperitoneally injected a competitive inhibitor of taurine production, D-CSA, into pregnant mice every 12 h beginning at E14.5, after in utero electroporation for cell labeling and examined its effects on ambient taurine levels, GABAAR activation and the radial migration of neurons in the fetal neocortices at E17.5.
First, we determined the ambient taurine levels in cortical slices from GAD67+/+ fetuses via HPLC. The taurine levels were significantly decreased by maternal administration of D-CSA (Control: 50.21 ± 5.79 fmol/μl·mg, n = 5; D-CSA: 29.42 ± 2.69 fmol/μl·mg, n = 5; P = 0.012 by Student’s t-test; Figure 8A). Next, we examined the effects of decreased taurine levels on GABAAR-mediated tonic currents in RFP-positive, radially migrating neurons in the SP and CP regions of the cortical slices. In both the SP and CP regions, maternal D-CSA administration significantly reduced the amplitudes of the tonic currents, not only in GADGFP/GFP fetuses (Control SP: 2.10 ± 0.27 pA, n = 5; D-CSA SP: 0.61 ± 0.13 pA, n = 4; P = 0.002 by Student’s t-test; Figures 8B,C, third bar group from the left; Control CP: 1.03 ± 0.16 pA, n = 5; D-CSA CP: 0.55 ± 0.11 pA, n = 5; P = 0.042 by Student’s t-test; Figure 8C, rightmost bar group) but also in GAD67+/+ fetuses (Control SP: 2.39 ± 0.48 pA, n = 5; D-CSA SP: 0.47 ± 0.11 pA, n = 5; P = 0.004 by Student’s t-test; Figure 8C, leftmost bar group; Control CP: 1.58 ± 0.26 pA, n = 6; D-CSA CP: 0.91 ± 0.14 pA, n = 6; P = 0.046 by Student’s t-test; Figure 8C, second bar group from the left). There was no difference in the membrane capacitance of the recorded cells observed between Control and maternal D-CSA-treated fetuses (GAD67+/+; 11.29 ± 0.97 pF in Control SP, 12.59 ± 3.19 pF in D-CSA SP, P = 0.708 by Student’s t-test, 8.58 ± 0.91 pF in Control CP, 7.02 ± 1.22 pF in D-CSA CP, P = 0.334 by Student’s t-test; GADGFP/GFP; 8.57 ± 2.15 pF in Control SP, 8.50 ± 1.22 pF in D-CSA SP, P = 0.979, by Student’s t-test, 8.63 ± 1.53 pF in Control CP, 8.86 ± 1.29 pF in D-CSA CP, P = 0.913, by Student’s t-test). The significant suppression of tonic currents observed in even GABA-rich GAD67+/+ fetuses suggests that taurine plays a major role as an endogenous agonist of GABAARs, producing tonic currents irrespective of the genotype involved, at least in the SP and CP regions of the fetal cerebral cortex at E17.5.
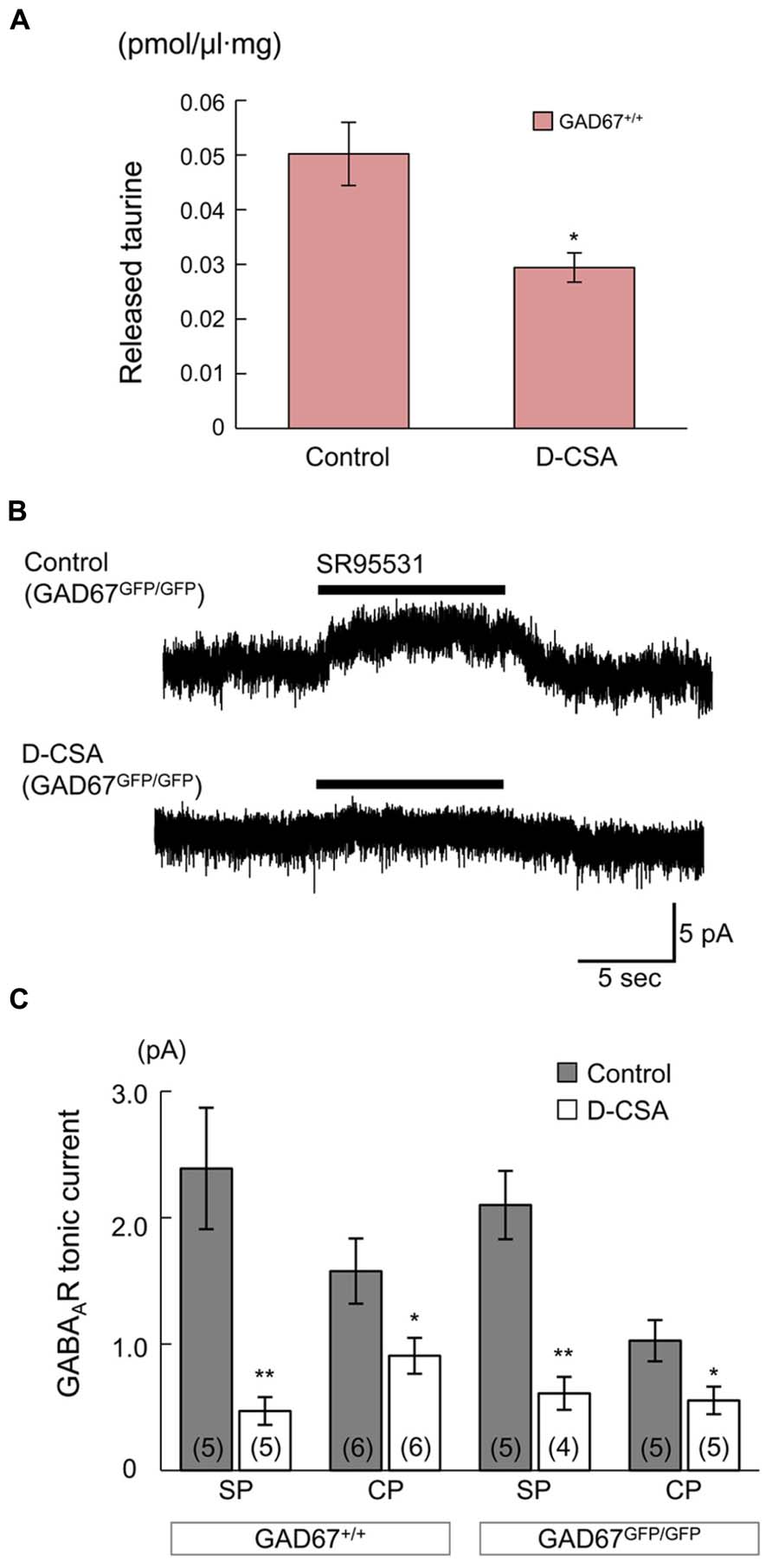
FIGURE 8. Effects of maternal D-CSA administration on ambient taurine levels and GABAAR-mediated tonic currents in the fetal neocortex. (A) Ambient taurine levels in fetal cortical slices determined via HPLC. E17.5 GAD67+/+ fetuses were obtained from pregnant mice injected with either saline (Control, n = 5) or D-CSA (n = 5). (B) Typical traces of GABAAR-mediated tonic currents recorded in RFP-positive neurons in the SP regions of GAD67GFP/GFP fetal cortical slices. The fetuces were obtained from saline- (upper trace) and D-CSA-treated (lower trace) pregnant GAD67+/GFP mice. (C) Averaged tonic current amplitudes in the SP and the CP regions of cortical slices obtained from GAD67+/+ (left bar group) and GAD67GFP/GFP (right bar group) fetuses either without (gray bars) or with (white bars) maternal D-CSA injection. The numbers in parentheses indicate the number of samples. *P < 0.05, **P < 0.01. Error bar: mean ± SEM.
Radial Migration is Accelerated by Reduced Ambient Taurine levels in GAD67-GFP Knock-in Mice
Next, we examined the effects of maternal D-CSA administration on the radial migration of neurons in the fetal neocortex. The distribution patterns of RFP-positive cells with and without D-CSA treatment were compared in GAD67+/+, GAD67+/GFP, and GAD67GFP/GFP fetuses. In the GAD67+/GFP and GAD67GFP/GFP fetuses, the distribution pattern of RFP-labeled cells was altered significantly by D-CSA administration (P = 0.046 in GAD67+/GFP, Figure 9C, middle; P = 0.015 in GAD67GFP/GFP; Figures 9A–C, right; χ2-test). In GAD67+/GFP and GAD67GFP/GFP fetal cortices, the number of RFP-positive cells was increased in the CP (P = 0.023 in GAD67+/GFP; P = 0.012 in GAD67GFP/GFP; MWU-test) and decreased in the SP (P = 0.040 in GAD67+/GFP; GAD67GFP/GFP; P = 0.012) by D-CSA administration. In GAD67GFP/GFP fetuses, the number of RFP-positive cells in the IZ was also significantly reduced (P = 0.022). Thus, radial migration in GAD67+/GFP and GAD67GFP/GFP fetuses was accelerated by the depletion of taurine. Although the change in the pattern was not significant in GAD67+/+ fetuses (P = 0.169; Figure 9C, left), a similar trend was apparent. In addition, the distribution patterns of RFP-positive cells with and without D-CSA treatment were compared in GAD67GFP/GFP fetuses at E16.5 and E18.5 and were not found to be significantly altered by D-CSA administration (E16.5: P = 0.488, Figure 9D, left, χ2-test; E18.5, P = 0.854, Figure 9D, right, χ2-test). Thus, the speed of radial migration during the period between E16.5 and E18.5 was increased by D-CSA, as early exit from the SP was apparent at E17.5, although the final distribution of E14.5-born neurons at E18.5 was not disturbed by D-CSA (Figure 9D, right). These data suggest that in GAD67-GFP knock-in fetuses, taurine, rather than GABA, regulates the radial migration of neurons by activating GABAARs and slowing the speed of migration.
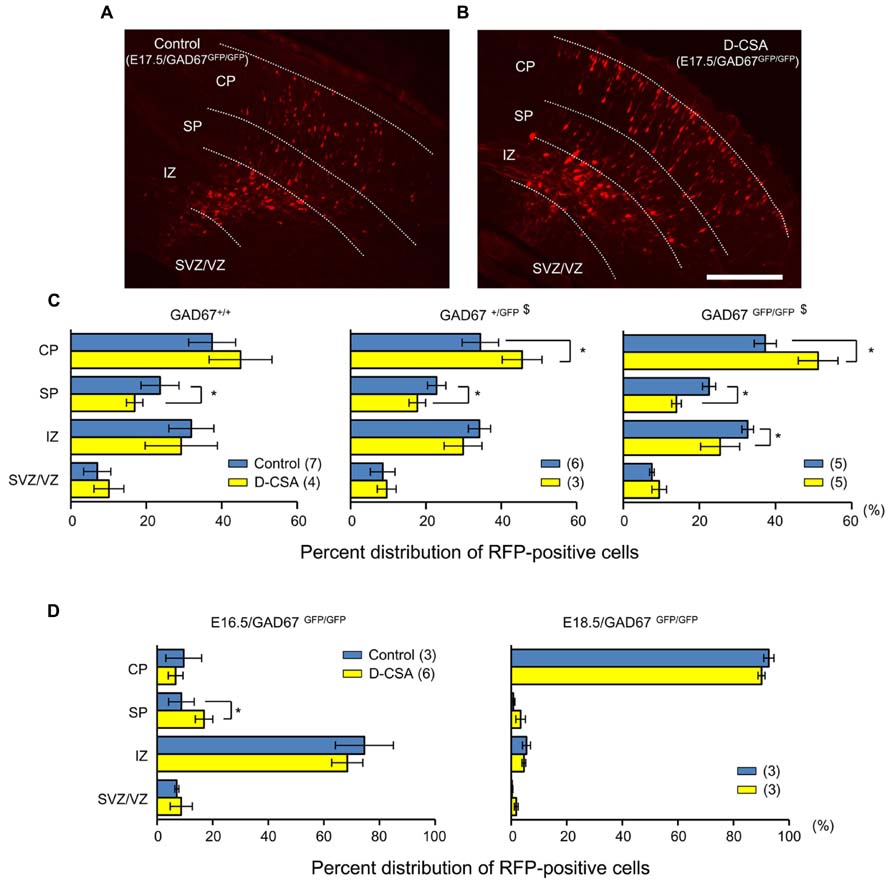
FIGURE 9. Effects of maternal D-CSA administration on the distribution of radially migrating neurons in the fetal neocortex. (A,B) Fluorescent micrographs showing the distribution of RFP-labeled radially migrating cells in cortical sections from GAD67GFP/GFP fetuses at E17.5. Saline (Control) (A) or D-CSA (B) was administered to the mothers every 12 h for 3 days (E14–17). (C) The averaged proportions of radially migrating cells in the CP, SP, IZ, and SVZ/VZ regions of cortical slices obtained from GAD67+/+ (leftmost), GAD67+/GFP (middle), and GAD67GFP/GFP (rightmost) fetuses at E17.5 with (yellow bars) and without (blue bars) maternal D-CSA administration. (D) The averaged proportions of radially migrating cells in cortical slices obtained from GAD67GFP/GFP fetuses at E16.5 (left) and E18.5 (right) either with (yellow bars) or without (blue bars) maternal D-CSA administration. The numbers in parentheses indicate the number of fetuses examined. $P < 0.05 by χ2-test. *P < 0.05 by MWU-test. Error bar: mean ± SEM. Scale bar: 200 μm.
Taurine Activates a Fraction of GABAARs in Radially Migrating Neurons
The affinity of GABAARs for taurine was recently reported to depend on the subunit composition of GABAARs (Kletke et al., 2013). To examine how large a fraction of GABAARs are activated by taurine in radially migrating neurons, we compared the currents evoked by GABA and taurine in the same radially migrating neuron in the SP region of GAD67+/+ fetal cortical slices. For this purpose, we first recorded the currents evoked by the topical application of 10 μM GABA, followed by bath application of taurine at 10 mM. Taurine perfusion induced inward currents that were much weaker than those evoked by GABA, while taurine did not induce significant current desensitization during its perfusion (Figure 10Aa). When GABA was again applied in the presence of taurine, the amplitudes of the GABA-evoked inward currents were 49.97 ± 5.83% (n = 10) of those prior to taurine perfusion (Figures 10Ab,B, right). The peak levels of the GABA-evoked currents observed during GABA application were unchanged by taurine perfusion (Figures 10Ab,B, left; P = 0.867 by paired t-test, n = 10). Thus, taurine activates only a fraction (~49.97%) of GABAARs, with a much lower affinity than GABA in radially migrating neurons.
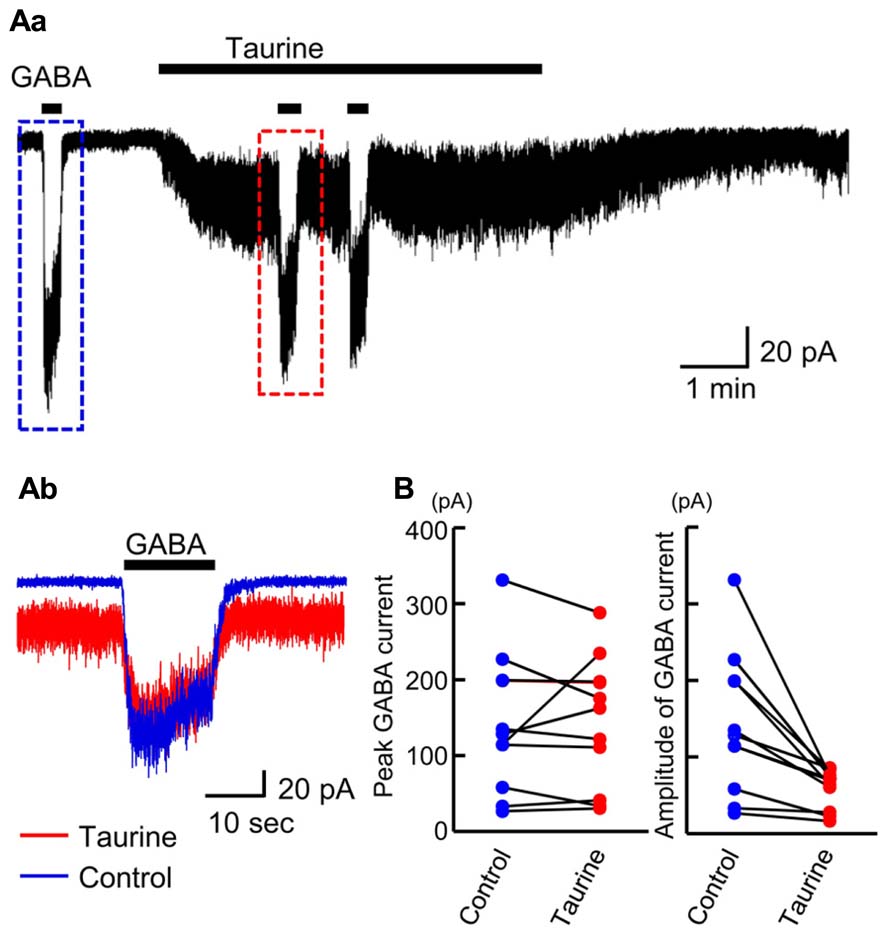
FIGURE 10. Comparison of GABA-evoked currents before and during taurine application in radially migrating neurons in the SP region of the fetal neocortex. (Aa) Typical trace of the currents evoked via the puff application of 10 μM GABA before and during bath perfusion of 10 mM taurine in a single RFP-positive neuron. The GABA-evoked currents boxed with dashed colored lines were superimposed in (Ab). VH was -60 mV. (B) The peak levels of GABA-evoked currents before (Control) and during taurine application (Taurine) are plotted and compared (left, n = 10). The levels were not significantly altered by taurine application (P = 0.867 by paired t-test). The amplitudes of GABA-evoked currents before (Control) and during taurine application (Taurine) are plotted and compared (right). The amplitudes were significantly altered by taurine application (P = 0.004 by paired t-test).
Taurine is Released Through Volume-Sensitive Anion Channels in the Developing Cerebral Cortex
Finally, we examined how taurine is released in the developing cerebral cortex. Some studies have reported that the taurine release induced by hypotonic shock in adult brain tissues can be blocked by anion channel blockers (Schousboe et al., 1991; Oja and Saransaari, 1992; Haskew-Layton et al., 2008). Thus, volume-sensitive anion channels are likely to be responsible for this taurine release (Haskew-Layton et al., 2008). To determine whether such anion channels are involved in taurine release in the fetal cerebral cortex, we examined the effects of anion channel blockers and a hypotonic medium on the release of taurine from acute cortical slices obtained from GAD67+/+ fetuses at E17.5.
To observe these effects clearly, first we preloaded the slices with 10 mM taurine by preincubating them in taurine-containing medium for 1 h. This was expected to enhance the intracellular taurine content, thereby increasing the taurine release flux from the cells. Indeed, the ambient taurine level measured via HPLC after preloading was greatly enhanced (Figure 11, fourth bar from the left). This enhancement was suppressed by adding the taurine transporter blocker GES (300 μM) to the preincubation medium (Figure 11, fifth bar from the left). Preloading with 1 mM taurine had no detectable effect (Figure 11, third bar from the left), suggesting that the rate of taurine uptake by taurine transporters under these loading conditions was balanced by the rate of taurine release from the cells. In addition to preloading the cortical slices with 10 mM taurine, we added GES during the period of taurine level measurement to isolate the taurine release flux. However, GES did not further enhance the measured taurine level (Figure 11, fifth bar from the right).
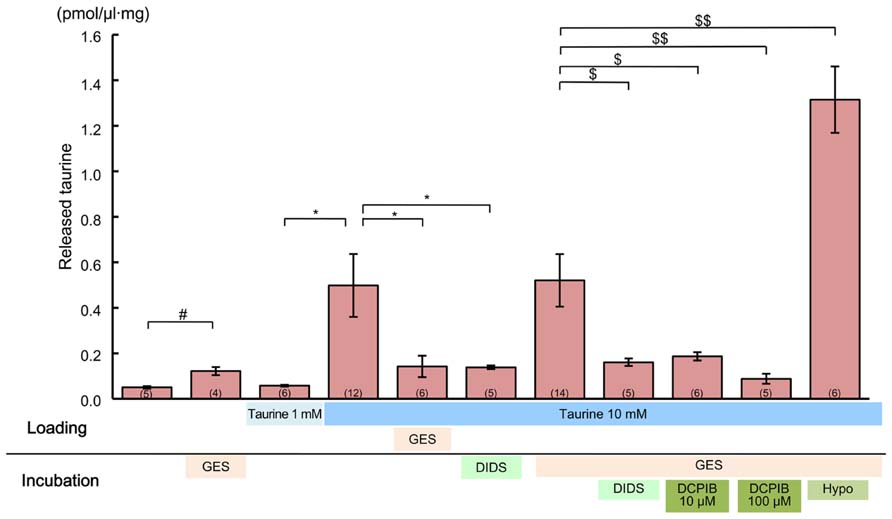
FIGURE 11. Effects of anion channel blockers on taurine release in the developing neocortex. Each bar represents the taurine concentration determined via HPLC in the measurement medium containing cortical slices from GAD67+/+ fetuses at E17.5, either without or with the inhibitors indicated below the bar (“incubation”). The loading conditions, either without or with taurine (1 or 10 mM) and/or GES (300 μM), before the measurements were performed are also indicated just beneath the bar (“loading”). DIDS (1 mM) and DCPIB (10 or 100 μM) were dissolved in incubation media. The numbers in parentheses at the bottom of the bars indicate the number of samples. One sample was obtained from one fetus. #*$P < 0.05, $$P < 0.01. Error bar: mean ± SEM.
Application of a global inhibitor of anion channels, DIDS (1 mM), to the measurement medium markedly reduced taurine release (Figure 11, fourth bar from the right). This reduction was still clearly observable, even in the absence of GES treatment (Figure 11, sixth bar from the right). Treatment with a more specific inhibitor of volume-sensitive anion channels, DCPIB (10 and 100 μM), also markedly suppressed taurine release in a dose-dependent manner (Figure 11, second and third bars from the right). Moreover, the taurine release was markedly enhanced in the hypotonic medium, which is the most effective activator of volume-sensitive anion channels (Figure 11, rightmost bar). Thus, taurine must be released through volume-sensitive anion channels in the developing cerebral cortex.
Discussion
In the present study, we showed that radial migration in the developing mouse cerebral cortex was modulated by GABAAR activation in ambient GABA-deficient GAD67-GFP knock-in mice. Ambient taurine, which abundantly expressed in the SP and MZ, acts as an endogenous agonist of GABAAR to tonically activate GABAARs and attenuate the speed of radial migration.
GABAARs have been reported to regulate neuronal migration in several cell types and are known to act as a stop signal for radially migrating neurons in the developing cortex (Owens and Kriegstein, 2002; Heck et al., 2007; Manent and Represa, 2007; Wang et al., 2013; for a review, see Egawa and Fukuda, 2013). However, the effect of GABAAR activation on neuronal migration has mostly been investigated in vitro using GABAAR antagonists (Behar et al., 1996, 1998, 2000; Bolteus and Bordey, 2004). In this study, radially migrating cells, labeled via in utero electroporation at E14.5, were identified in the cerebral cortex at E17.5. We found that the distribution pattern of the radially migrating cells was normal, even in GABA-deficient GAD67GFP/GFP fetuses. In addition, electrophysiological recordings demonstrated that the GABAAR sensitivities of radially migrating cells did not differ among the different genotypes of GAD67-GFP knock-in fetuses. These results are consistent with a previous report showing that GAD65/67 double knock-out embryonic mouse brains do not show any histological abnormality (Ji et al., 1999). Furthermore, we demonstrated that sustained blockade of GABAARs via SR95531 administration accelerated radial migration in the cerebral cortex of GABA-deficient GAD67GFP/GFP fetuses. These findings imply that activation of GABAAR indeed affects radial migration, but that GABAARs are not necessarily activated by endogenous GABA.
Functional Expression of GABAAR, but not GlyR, in Radially Migrating Cells in the Fetal Mouse Neocortex
GABA-induced inward currents were observed in all recordings obtained from RFP-labeled cells, which is consistent with previous studies demonstrating the presence of functional GABAARs in these cells (LoTurco et al., 1995; Owens et al., 1996). RFP-positive, radially migrating cells showed GABAAR-mediated currents in response to taurine application. Interestingly, none of the RFP-positive cells in which recordings were made showed glycine-induced currents. These results suggest that the GABAARs, but not GlyRs, on radially migrating cells were activated by taurine in the fetal mouse neocortex. Functional expression of GlyRs has been reported in the fetal and neonatal rat cortex (Malosio et al., 1991; Flint et al., 1998; Nimmervoll et al., 2011). Accordingly, in E18.5 rat fetuses, we found that radially migrating cells labeled via in utero electroporation 3 days before recordings were performed exhibited glycine-induced inward currents (Figure 6). In addition, in mouse fetuses, some of RFP-negative cells that were presumably generated earlier than the RFP-positive cells responded to the application of glycine by producing large currents. Considering these results, the expression of functional GlyRs might differ between rats and mice. In mice, GlyRs may be expressed after the migratory period of corticogenesis, in contrast to those in rats (Nimmervoll et al., 2011).
The functional properties of GABAARs and the affinity of taurine are subunit dependent. Previous studies have found that taurine acts on a specific subtype of GABAAR containing the β2 subunit (Bureau and Olsen, 1991; Kash et al., 2004). Kletke et al. (2013) also reported that the efficacy of taurine is independent of the α subunit type and is instead determined by the β subunit type based on recombinant studies. Furthermore, δ-containing receptors are more potently and efficiently gated by taurine than γ-containing receptors (Jia et al., 2008; Kletke et al., 2013). Considering these findings, taurine is likely to potently activate αxβ1/2δ subunit-containing GABAARs. GABAAR subunits α2–α5, β1–β3, γ1–γ2, and δ are expressed in immature cortical cells, and these subunits are developmentally regulated (Gambarana et al., 1991; Araki et al., 1992; Laurie et al., 1992; Cheng et al., 2006; Peden et al., 2008; Cuzon Carlson and Yeh, 2010). Although the expression of GABAAR subunits in radially migrating cells remains unclear, it is possible that they may express the components that mediate the actions of taurine.
Ambient Taurine Tonically Activates GABAAR and Modulates Radial Migration in the Fetal Cerebral Cortex
Previous studies have shown that tonic activation of GABAAR is evident in certain embryonic neurons before synapse formation has taken place (Valeyev et al., 1993; LoTurco et al., 1995; Owens et al., 1999; Farrant and Nusser, 2005; Cancedda et al., 2007). While we also demonstrated that GABAARs were tonically activated in the fetal cerebral cortex, GABAAR-mediated tonic currents were detected even in GABA-deficient GAD67GFP/GFP fetuses. We found that taurine and its transporter are expressed at particularly high levels in the SP and MZ of the fetal cerebral cortex and that depletion of taurine through maternal D-CSA administration greatly reduces the recorded tonic currents, not only in the SP but also in the CP, in both GAD67+/+ and GAD67GFP/GFP fetuses. Moreover, although it did not reach statistical significance, there was a trend of smaller tonic currents observed in the CP compared to the SP, even under control (D-CSA-untreated) conditions (Figure 8C). This might reflect the differences in ambient taurine levels between the SP and CP sensed by GABAARs. These findings suggest that in the cerebral cortex of fetal mice, taurine might play a major role as an endogenous agonist of GABAARs and that the GABAARs in radially migrating cells are activated tonically by ambient taurine, rather than GABA. However, our results do not fully exclude the possibility that some component of GABAARs is activated by endogenous GABA. The affinity of GABAARs for GABA is more than three orders of magnitude higher than for taurine (Kletke et al., 2013), which was also indicated by our results (Figure 10). Therefore, even though the applied D-CSA treatment decreased the basal taurine level in the fetal cerebral cortex by only 40% (Figure 8A), it is possible that the component of tonic GABAAR currents that remains after D-CSA treatment (Figure 8C) might be due to the action of endogenous GABA to some extent.
The amplitude of tonic GABAAR currents in the SP, which were found to be mostly caused by taurine action, was approximately 2.5 pA in both GAD67+/+ and GAD67GFP/GFP fetuses (Figures 7C and 8C). However, these currents were recorded in the presence of a high intracellular Cl- concentration (122 mM), introduced through whole-cell patch pipettes. As the intracellular Cl- level under physiological conditions must be lower than that in our experiments, the tonic currents that occur under physiological conditions must be smaller than those we measured because of the weaker driving force for Cl- movement. In previous reports, the resting membrane potential and the reversal potential for GABAAR-mediated currents measured by gramicidin-perforated patch-clamp recordings were reported to be -65 and -43 mV, respectively, in rat CP cells at E19 or E18.5 (Owens et al., 1996; Inoue et al., 2012). Assuming conditions similar to these previous reports, the amplitude of tonic currents we measured at the holding potential of -60 mV with an Cl- equilibrium potential of -2 mV would correspond to ~0.9 pA. The membrane resistance of the SP cells estimated in the present study, based on the holding current level at -60 mV (10.08 ± 0.79 pA, n = 5), was approximately 6 GΩ. Thus, the 0.9 pA tonic currents may depolarize the cells by 5.4 mV. Nevertheless, because Cs+ salts were used in our pipette solution to block K+ channels, the 6 GΩ membrane resistance must be an overestimated value, and the degree of depolarization elicited by tonic currents in SP cells under physiological conditions would therefore be no greater than 5 mV.
When the taurine-mediated tonic GABAAR currents were reduced via maternal D-CSA administration, the distribution pattern of RFP-positive cells was significantly changed, especially in GAD67GFP/GFP fetuses, indicating an increase in the fraction of RFP-positive cells in the CP and a reciprocal decrease in the SP and IZ at E17.5 (Figures 9A–C). This pattern mimicked the change in distribution caused by GABAAR blockade (Figure 3). These results clearly indicate that the reduction of GABAAR activity in the SP caused by taurine depletion accelerates the exit of radially migrating neurons from the SP. Thus, taurine slows the speed of radially migrating neurons across the SP through activating GABAARs in these neurons. In the cortices of GAD67+/GFP fetuses, in which the ambient GABA level is reduced by 32% (Morishima et al., 2010), a similar change in distribution was elicited by taurine depletion, while the change observed in GAD67+/+ fetuses was subtle (Figure 9C). As such, the ambient GABA levels could also regulate radial migration, presumably through activating the GABAARs in regions other than the SP.
The mechanism underlying the regulation of the speed of migrating neurons via taurine-mediated GABAAR activation has not yet been resolved. It has been demonstrated in immature cortical neurons that GABAAR activation is required to maintain intracellular oscillatory Ca2+ signaling (Behar et al., 1996; Owens et al., 1996; Garaschuk et al., 2000; Heck et al., 2007). Thus, the stop signal for migration generated by taurine is also expected to be mediated by the Ca2+ signaling. By contrast, in the developing cerebellum, the migration of granule neurons has been reported to be stopped by the loss of intracellular Ca2+ signaling (Kumada and Komuro, 2004). Hence, the role of intracellular Ca2+ signaling in neuronal migration seems to differ between the cortex and the cerebellum. Nevertheless, the notion that the action of taurine in the cerebellum also maintains Ca2+ signaling in granule cells through activating GABAARs would be consistent with a previous report indicating a delay in granule cell migration in taurine-deficient kittens (Sturman et al., 1985).
Even when the radial migration of neurons in the developing cortex was accelerated by taurine depletion at E17.5, the destination of the migrating neurons at E18.5 was not affected by taurine depletion (Figure 9D). This suggests that the effect of taurine-mediated GABAAR activation on radial migration is temporary, and a different process might be involved in the final maturation of the cortex. Nevertheless, it is possible that the early exit of migrating neurons from the SP caused by taurine depletion may affect final circuit formation and/or the function of the neurons after birth in some manner. To examine this possibility, it will be necessary to follow the effect of taurine depletion on the maturation of the cortex in GAD67+/GFP mice in detail even after birth because the GAD67GFP/GFP genotype is lethal immediately after birth due to severe cleft palate, which has been well established in GAD67 knock-out mice (Asada et al., 1997).
Ambient Taurine is Taken up by Taurine Transporters and Released Via Volume-Sensitive Anion Channels
Taurine transporters are highly expressed in the neocortex (Smith et al., 1992). These transporters belong to a family of Na+ and Cl--dependent neurotransmitter transporters that mediate the uptake of taurine into cells under resting conditions (Molchanova et al., 2004; Tappaz, 2004). Because taurine uptake is driven by the membrane gradient of Na+ and Cl-, taurine transporter activity can be reversed by stimuli that alter this gradient (Oja et al., 1985; Oja and Kontro, 1987; Takuma et al., 1996). In the present study, the ambient taurine level in fetal cortical slices was increased via the application of GES. In addition, taurine release from 10 mM taurine-loaded cortical slices was decreased by GES application during the taurine loading period. Furthermore, GABAAR-mediated tonic currents of SP cells were enhanced by GES application. These results are compatible with the normal taurine transporter function of extracellular taurine uptake. Thus, ambient taurine is taken up by taurine transporters in the mouse fetal neocortex.
In the present study, we observed ambient taurine levels in the fetal cerebral cortex via HPLC. The released taurine concentration was 45.7–50.2 fmol/μl·mg, while no released GABA was detectable. As our HPLC system is able to detect GABA at the 0.05 fmol/μl·mg, level (Morishima et al., 2010), the taurine levels in our study were more than 1,000 times higher than those of GABA in the cerebral cortex of E17.5 mice. Furthermore, in taurine loading experiments, the released taurine concentration was increased by loading with 10 mM taurine but was not changed by 1 mM taurine loading. These data suggest that the ambient taurine concentration in the fetal cerebral cortex may be in the millimolar range.
We showed that taurine was expressed in the SP and MZ of the cerebral cortex at E17.5 through immunohistochemical analysis. Immunoelectron microscopic analysis demonstrated that taurine was located within immature neurons, whereas it was not detectable in presynaptic structures. These results imply that the release of taurine may be controlled in a non-vesicular manner, which is in accord with previous studies suggesting that taurine can be released as an osmolyte through non-vesicular systems in neurons and glial cells (Calvert and Shennan, 1998; Flint et al., 1998; Mongin et al., 1999; Mulligan and MacVicar, 2006). Previous studies have also shown that taurine can be released via volume-sensitive anion channels (Fugelli and Thoroed, 1986; Jackson and Strange, 1993; Hall, 1995; Shennan, 1999; Haskew-Layton et al., 2008). However, there are limited data on the release of taurine in the fetal nervous system, despite its important role in functional development (Sturman et al., 1985; Palackal et al., 1986); non-synaptic and hypo-osmotic taurine release has been observed in the immature cortex of rats (Flint et al., 1998; Kilb et al., 2008). Our data showed that administration of DIDS, a broad Cl- channel blocker, and DCPIB, a specific volume-sensitive anion channel blocker, blocked taurine release, while a hypotonic medium stimulated taurine release. These results provide evidence that taurine release in the fetal cerebral cortex is mediated by volume-sensitive anion channels (Jackson and Strange, 1993; Shennan, 1999; Molchanova et al., 2004; Haskew-Layton et al., 2008).
The involvement of volume-sensitive anion channels in the radial migration of neurons is an interesting issue. Volume-sensitive anion channels are typically activated in response to cell swelling (Okada, 1997), but they may also be activated without swelling when certain types of chemical mediator or transmitter act on the cell (Liu et al., 2009; Okada et al., 2009). Moreover, it has recently been demonstrated in mouse astrocytes that the transmitter-induced activation of volume-sensitive anion channels is locally controlled via regions of high concentrations of intracellular Ca2+ in the immediate vicinity of Ca2+-permeable ion channel pores, or so-called “Ca2+ nanodomains” (Akita and Okada, 2011; Akita et al., 2011). Therefore, if the volume-sensitive anion channels located on the leading edges of migrating neurons are activated in response to taurine-induced intracellular Ca2+ signaling in a similar manner to that observed in astrocytes, a net efflux of Cl- and K+ should ensue according to their electrochemical gradients, which should accompany an obligatory water efflux. This may facilitate shrinkage of the leading edge and slow the migrating neurons (Schwab et al., 2012). Furthermore, taurine release through the activated channels may send synchronizing signals to adjacent neurons. Exploring the mechanism of the activation of the volume-sensitive anion channels and their roles in the fetal neocortex in detail would therefore shed light on the mystery of fetal brain development, which needs to be addressed in future studies.
Conclusion
We provide new evidence that ambient taurine in the mouse fetal cerebral cortex modulates radial migration. Ambient taurine exhibits a relatively wide distribution with relatively high levels in the SP and is released via volume-sensitive anion channels to tonically activate GABAARs and slow radial migration in the developing cerebral cortex.
Conflict of Interest Statement
The authors declare that the research was conducted in the absence of any commercial or financial relationships that could be construed as a potential conflict of interest.
Acknowledgments
This work was supported by Grants-in-Aid for Scientific Research on Priority Areas (#19045012, #21026013) and Innovative Areas (#23115506) from the Ministry of Education, Culture, Sports, Science and Technology of Japan (to Atsuo Fukuda) and Grants-in-Aid for Scientific Research (B) #19390058, #22390041, #25293052 and for Challenging Exploratory Research #23659535, #24659508 from the Japan Society for the Promotion of Science (to Atsuo Fukuda).
References
Aerts, L., and Van Assche, F. A. (2002). Taurine and taurine-deficiency in the perinatal period. J. Perinat. Med. 30, 281–286. doi: 10.1515/JPM.2002.040
Akita, T., Fedorovich, S. V., and Okada, Y. (2011). Ca2+ nanodomain-mediated component of swelling-induced volume-sensitive outwardly rectifying anion current triggered by autocrine action of ATP in mouse astrocytes. Cell. Physiol. Biochem. 28, 1181–1190. doi: 10.1159/000335867
Akita, T., and Okada, Y. (2011). Regulation of bradykinin-induced activation of volume-sensitive outwardly rectifying anion channels by Ca2+ nanodomains in mouse astrocytes. J. Physiol. 589, 3909–3927. doi: 10.1113/jphysiol.2011.208173
Andjelic, S., Gallopin, T., Cauli, B., Hill, E. L., Roux, L., Badr, S., et al. (2009). Glutamatergic nonpyramidal neurons from neocortical layer VI and their comparison with pyramidal and spiny stellate neurons. J. Neurophysiol. 101, 641–654. doi: 10.1152/jn.91094.2008
Araki, T., Kiyama, H., and Tohyama, M. (1992). GABAA receptor subunit messenger RNAs show differential expression during cortical development in the rat brain. Neuroscience 51, 583–591. doi: 10.1016/0306-4522(92)90298-G
Asada, H., Kawamura, Y., Maruyama, K., Kume, H., Ding, R. G., Kanbara, N., et al. (1997). Cleft palate and decreased brain gamma-aminobutyric acid in mice lacking the 67-kDa isoform of glutamic acid decarboxylase. Proc. Natl. Acad. Sci. U.S.A. 94, 6496–6499. doi: 10.1073/pnas.94.12.6496
Behar, T., Schaffner, A., Laing, P., Hudson, L., Komoly, S., and Barker, J. (1993). Many spinal cord cells transiently express low molecular weight forms of glutamic acid decarboxylase during embryonic development. Brain Res. Dev. Brain Res. 72, 203–218. doi: 10.1016/0165-3806(93)90185-D
Behar, T. N., Li, Y. X., Tran, H. T., Ma, W., Dunlap, V., Scott, C., et al. (1996). GABA stimulates chemotaxis and chemokinesis of embryonic cortical neurons via calcium-dependent mechanisms. J. Neurosci. 16, 1808–1818.
Behar, T. N., Schaffner, A. E., Scott, C. A., Greene, C. L., and Barker, J. L. (2000). GABA receptor antagonists modulate postmitotic cell migration in slice cultures of embryonic rat cortex. Cereb. Cortex 10, 899–909. doi: 10.1093/cercor/10.9.899
Behar, T. N., Schaffner, A. E., Scott, C. A., O’Connell, C., and Barker, J. L. (1998). Differential response of cortical plate and ventricular zone cells to GABA as a migration stimulus. J. Neurosci. 18, 6378–6387.
Benitez-Diaz, P., Miranda-Contreras, L., Mendoza-Briceno, R. V., Pena-Contreras, Z., and Palacios-Pru, E. (2003). Prenatal and postnatal contents of amino acid neurotransmitters in mouse parietal cortex. Dev. Neurosci. 25, 366–374. doi: 10.1159/000073514
Bolteus, A. J., and Bordey, A. (2004). GABA release and uptake regulate neuronal precursor migration in the postnatal subventricular zone. J. Neurosci. 24, 7623–7631. doi: 10.1523/JNEUROSCI.1999-04.2004
Bolteus, A. J., Garganta, C., and Bordey, A. (2005). Assays for measuring extracellular GABA levels and cell migration rate in acute slices. Brain Res. Brain Res. Protoc. 14, 126–134. doi: 10.1016/j.brainresprot.2004.12.005
Bureau, M. H., and Olsen, R. W. (1991). Taurine acts on a subclass of GABAA receptors in mammalian brain in vitro. Eur. J. Pharmacol. 207, 9–16. doi: 10.1016/S0922-4106(05)80031-8
Calvert, D. T., and Shennan, D. B. (1998). Volume-activated taurine efflux from the in situ perfused lactating rat mammary gland. Acta Physiol. Scand. 162, 97–105. doi: 10.1046/j.1365-201X.1998.0267f.x
Cancedda, L., Fiumelli, H., Chen, K., and Poo, M. M. (2007). Excitatory GABA action is essential for morphological maturation of cortical neurons in vivo. J. Neurosci. 27, 5224–5235. doi: 10.1523/JNEUROSCI.5169-06.2007
Caric, D., Gooday, D., Hill, R. E., McConnell, S. K., and Price, D. J. (1997). Determination of the migratory capacity of embryonic cortical cells lacking the transcription factor Pax-6. Development 124, 5087–5096.
Caviness, V. S. Jr., and Rakic, P. (1978). Mechanisms of cortical development: a view from mutations in mice. Annu. Rev. Neurosci. 1, 297–326. doi: 10.1146/annurev.ne.01.030178.001501
Cheng, Q., Yeh, P. W., and Yeh, H. H. (2006). Cajal–Retzius cells switch from expressing gamma-less to gamma-containing GABA receptors during corticogenesis. Eur. J. Neurosci. 24, 2145–2151. doi: 10.1111/j.1460-9568.2006.05122.x
Cuzon Carlson, V. C., and Yeh, H. H. (2010). GABAA receptor subunit profiles of tangentially migrating neurons derived from the medial ganglionic eminence. Cereb. Cortex 21, 1792–1802. doi: 10.1093/cercor/bhq247
Demarque, M., Represa, A., Becq, H., Khalilov, I., Ben-Ari, Y., and Aniksztejn, L. (2002). Paracrine intercellular communication by a Ca2+- and SNARE-independent release of GABA and glutamate prior to synapse formation. Neuron 36, 1051–1061. doi: 10.1016/S0896-6273(02)01053-X
Dominguez-Perrot, C., Feltz, P., and Poulter, M. O. (1996). Recombinant GABAA receptor desensitization: the role of the gamma 2 subunit and its physiological significance. J. Physiol. 497, 145–159.
Dominy, J., Eller, S., and Dawson, R. (2004). Building biosynthetic schools: reviewing compartmentation of CNS taurine synthesis. Neurochem. Res. 29, 97–103. doi: 10.1023/B:NERE.0000010437.81860.d5
Egawa, K., and Fukuda, A. (2013). Pathophysiological power of improper tonic GABAA conductances in mature and immature models. Front. Neural Circuits 7:170. doi: 10.3389/fncir.2013.00170
Emiliozzi, R., and Pichat, L. (1959). A simple method for the preparation of cysteinesulfinic acid. Bull. Soc. Chim. Fr. 11–12, 1887–1888.
Farrant, M., and Nusser, Z. (2005). Variations on an inhibitory theme: phasic and tonic activation of GABAA receptors. Nat. Rev. Neurosci. 6, 215–229. doi: 10.1038/nrn1625
Flint, A. C., Liu, X., and Kriegstein, A. R. (1998). Nonsynaptic glycine receptor activation during early neocortical development. Neuron 20, 43–53. doi: 10.1016/S0896-6273(00)80433-X
Fugelli, K., and Thoroed, S. M. (1986). Taurine transport associated with cell volume regulation in flounder erythrocytes under anisosmotic conditions. J. Physiol. 374, 245–261.
Gambarana, C., Beattie, C. E., Rodriguez, Z. R., and Siegel, R. E. (1991). Region-specific expression of messenger RNAs encoding GABAA receptor subunits in the developing rat brain. Neuroscience 45, 423–432. doi: 10.1016/0306-4522(91)90238-J
Garaschuk, O., Linn, J., Eilers, J., and Konnerth, A. (2000). Large-scale oscillatory calcium waves in the immature cortex. Nat. Neurosci. 3, 452–459. doi: 10.1038/74823
Hall, A. C. (1995). Volume-sensitive taurine transport in bovine articular chondrocytes. J. Physiol. 484, 755–766.
Haskew-Layton, R. E., Rudkouskaya, A., Jin, Y., Feustel, P. J., Kimelberg, H. K., and Mongin, A. A. (2008). Two distinct modes of hypoosmotic medium-induced release of excitatory amino acids and taurine in the rat brain in vivo. PLoS ONE 3:e3543. doi: 10.1371/journal.pone.0003543
Hayes, K. C., and Sturman, J. A. (1981). Taurine in metabolism. Annu. Rev. Nutr. 1, 401–425. doi: 10.1146/annurev.nu.01.070181.002153
Heck, N., Kilb, W., Reiprich, P., Kubota, H., Furukawa, T., Fukuda, A., et al. (2007). GABA-A receptors regulate neocortical neuronal migration in vitro and in vivo. Cereb. Cortex 17, 138–148. doi: 10.1093/cercor/bhj135
Hernandez-Benitez, R., Ramos-Mandujano, G., and Pasantes-Morales, H. (2012). Taurine stimulates proliferation and promotes neurogenesis of mouse adult cultured neural stem/progenitor cells. Stem Cell Res. 9, 24–34. doi: 10.1016/j.scr.2012.02.004
Huxtable, R. J. (1989). Taurine in the central nervous system and the mammalian actions of taurine. Prog. Neurobiol. 32, 471–533. doi: 10.1016/0301-0082(89)90019-1
Inoue, K., Furukawa, T., Kumada, T., Yamada, J., Wang, T., Inoue, R., et al. (2012). Taurine inhibits K+–Cl- cotransporter KCC2 to regulate embryonic Cl- homeostasis via with-no-lysine (WNK) protein kinase signaling pathway. J. Biol. Chem. 287, 20839–20850. doi: 10.1074/jbc.M111.319418
Jackson, P. S., and Strange, K. (1993). Volume-sensitive anion channels mediate swelling-activated inositol and taurine efflux. Am. J. Physiol. 265, 1489–1500.
Ji, F., Kanbara, N., and Obata, K. (1999). GABA and histogenesis in fetal and neonatal mouse brain lacking both the isoforms of glutamic acid decarboxylase. Neurosci. Res. 33, 187–194. doi: 10.1016/S0168-0102(99)00011-5
Jia, F., Yue, M., Chandra, D., Keramidas, A., Goldstein, P. A., Homanics, G. E., et al. (2008). Taurine is a potent activator of extrasynaptic GABAA receptors in the thalamus. J. Neurosci. 28, 106–115. doi: 10.1523/JNEUROSCI.3996-07.2008
Kaczmarek, L. (1976). “A comparison of the evidence for taurine and GABA as neurotransmitters,” in Taurine, eds R. Huxtable and A. Barbeau (New York: Raven Press), 283–292.
Kash, T. L., Dizon, M. J., Trudell, J. R., and Harrison, N. L. (2004). Charged residues in the beta2 subunit involved in GABAA receptor activation. J. Biol. Chem. 279, 4887–4893. doi: 10.1074/jbc.M311441200
Kilb, W., Hanganu, I. L., Okabe, A., Shimizu-Okabe, C., Fukuda, A., and Luhmann, H. J. (2008). Glycine receptors mediate excitation of subplate neurons in neonatal rat cerebral cortex. J. Neurophysiol. 100, 698–707. doi: 10.1152/jn.00657.2007
Kletke, O., Gisselmann, G., May, A., Hatt, H., and O, A. S. (2013). Partial agonism of taurine at gamma-containing native and recombinant GABAA receptors. PLoS ONE 8:e61733. doi: 10.1371/journal.pone.0061733
Komuro, H., and Rakic, P. (1998). Orchestration of neuronal migration by activity of ion channels, neurotransmitter receptors, and intracellular Ca2+ fluctuations. J. Neurobiol. 37, 110–130. doi: 10.1002/(SICI)1097-4695(199810)37:1<110::AID-NEU9>3.0.CO;2-C
Kontro, P., Marnela, K. M., and Oja, S. S. (1984). GABA, taurine and hypotaurine in developing mouse brain. Acta Physiol. Scand. Suppl. 537, 71–74.
Kranz, H., Brazeau, G. A., Napaporn, J., Martin, R. L., Millard, W., and Bodmeier, R. (2001). Myotoxicity studies of injectable biodegradable in-situ forming drug delivery systems. Int. J. Pharm. 212, 11–18. doi: 10.1016/S0378-5173(00)00568-8
Kumada, T., and Komuro, H. (2004). Completion of neuronal migration regulated by loss of Ca2+ transients. Proc. Natl. Acad. Sci. U.S.A. 101, 8479–8484. doi: 10.1073/pnas.0401000101
Kuo, S. M., and Stipanuk, M. H. (1984). Changes in cysteine dioxygenase and cysteinesulfinate decarboxylase activities and taurine levels in tissues of pregnant or lactating rat dams and their fetuses or pups. Biol. Neonate 46, 237–248. doi: 10.1159/000242071
Lauder, J. M., Han, V. K., Henderson, P., Verdoorn, T., and Towle, A. C. (1986). Prenatal ontogeny of the GABAergic system in the rat brain: an immunocytochemical study. Neuroscience 19, 465–493. doi: 10.1016/0306-4522(86)90275-7
Laurie, D. J., Wisden, W., and Seeburg, P. H. (1992). The distribution of thirteen GABAA receptor subunit mRNAs in the rat brain. III. Embryonic and postnatal development. J. Neurosci. 12, 4151–4172.
Linne, M. L., Jalonen, T. O., Saransaari, P., and Oja, S. S. (1996). Taurine-induced single-channel currents in cultured rat cerebellar granule cells. Adv. Exp. Med. Biol. 403, 455–462. doi: 10.1007/978-1-4899-0182-8_49
Liu, H. T., Akita, T., Shimizu, T., Sabirov, R. Z., and Okada, Y. (2009). Bradykinin-induced astrocyte-neuron signalling: glutamate release is mediated by ROS-activated volume-sensitive outwardly rectifying anion channels. J. Physiol. 587, 2197–2209. doi: 10.1113/jphysiol.2008.165084
LoTurco, J. J., Owens, D. F., Heath, M. J., Davis, M. B., and Kriegstein, A. R. (1995). GABA and glutamate depolarize cortical progenitor cells and inhibit DNA synthesis. Neuron 15, 1287–1298. doi: 10.1016/0896-6273(95)90008-X
Ma, W., and Barker, J. L. (1995). Complementary expressions of transcripts encoding GAD67 and GABAA receptor alpha 4, beta 1, and gamma 1 subunits in the proliferative zone of the embryonic rat central nervous system. J. Neurosci. 15, 2547–2560.
Malosio, M. L., Marqueze-Pouey, B., Kuhse, J., and Betz, H. (1991). Widespread expression of glycine receptor subunit mRNAs in the adult and developing rat brain. EMBO J. 10, 2401–2409.
Manent, J. B., and Represa, A. (2007). Neurotransmitters and brain maturation: early paracrine actions of GABA and glutamate modulate neuronal migration. Neuroscientist 13, 268–279. doi: 10.1177/1073858406298918
Marin, O., and Rubenstein, J. L. (2003). Cell migration in the forebrain. Annu. Rev. Neurosci. 26, 441–483. doi: 10.1146/annurev.neuro.26.041002.131058
Mellor, J. R., Gunthorpe, M. J., and Randall, A. D. (2000). The taurine uptake inhibitor guanidinoethyl sulphonate is an agonist at gamma-aminobutyric acidA receptors in cultured murine cerebellar granule cells. Neurosci. Lett. 286, 25–28. doi: 10.1016/S0304-3940(00)01082-X
Miller, R. A., Brady, J. M., and Cutright, D. E. (1977). Degradation rates of oral resorbable implants (polylactates and polyglycolates): rate modification with changes in PLA/PGA copolymer ratios. J. Biomed. Mater. Res. 11, 711–719. doi: 10.1002/jbm.820110507
Molchanova, S., Oja, S. S., and Saransaari, P. (2004). Characteristics of basal taurine release in the rat striatum measured by microdialysis. Amino Acids 27, 261–268. doi: 10.1007/s00726-004-0139-8
Mongin, A. A., Reddi, J. M., Charniga, C., and Kimelberg, H. K. (1999). [3H]taurine and D-[3H]aspartate release from astrocyte cultures are differently regulated by tyrosine kinases. Am. J. Physiol. 276, 1226–1230.
Morishima, T., Uematsu, M., Furukawa, T., Yanagawa, Y., Fukuda, A., and Yoshida, S. (2010). GABA imaging in brain slices using immobilized enzyme-linked photoanalysis. Neurosci. Res. 67, 347–353. doi: 10.1016/j.neures.2010.04.005
Mulligan, S. J., and MacVicar, B. A. (2006). VRACs CARVe a path for novel mechanisms of communication in the CNS. Sci. STKE 357, 42.
Nadarajah, B., and Parnavelas, J. G. (2002). Modes of neuronal migration in the developing cerebral cortex. Nat. Rev. Neurosci. 3, 423–432. doi: 10.1038/nrn845
Nimmervoll, B., Denter, D. G., Sava, I., Kilb, W., and Luhmann, H. J. (2011). Glycine receptors influence radial migration in the embryonic mouse neocortex. Neuroreport 22, 509–513. doi: 10.1097/WNR.0b013e328348aafe
Oja, S. S., and Kontro, P. (1987). Cation effects on taurine release from brain slices: comparison to GABA. J. Neurosci. Res. 17, 302–311. doi: 10.1002/jnr.490170316
Oja, S. S., Korpi, E. R., Holopainen, I., and Kontro, P. (1985). Mechanisms of stimulated taurine release from nervous tissue. Prog. Clin. Biol. Res. 179, 237–247.
Oja, S. S., and Saransaari, P. (1992). Taurine release and swelling of cerebral cortex slices from adult and developing mice in media of different ionic compositions. J. Neurosci. Res. 32, 551–561. doi: 10.1002/jnr.490320410
Oja, S. S., and Saransaari, P. (1996). Taurine as osmoregulator and neuromodulator in the brain. Metab. Brain Dis. 11, 153–164. doi: 10.1007/BF02069502
Okada, Y. (1997). Volume expansion-sensing outward-rectifier Cl- channel: fresh start to the molecular identity and volume sensor. Am. J. Physiol. 273, C755–C789.
Okada, Y., Sato, K., and Numata, T. (2009). Pathophysiology and puzzles of the volume-sensitive outwardly rectifying anion channel. J. Physiol. 587, 2141–2149. doi: 10.1113/jphysiol.2008.165076
Owens, D. F., Boyce, L. H., Davis, M. B., and Kriegstein, A. R. (1996). Excitatory GABA responses in embryonic and neonatal cortical slices demonstrated by gramicidin perforated-patch recordings and calcium imaging. J. Neurosci. 16, 6414–6423.
Owens, D. F., and Kriegstein, A. R. (2002). Is there more to GABA than synaptic inhibition? Nat. Rev. Neurosci. 3, 715–727. doi: 10.1038/nrn919
Owens, D. F., Liu, X., and Kriegstein, A. R. (1999). Changing properties of GABAA receptor-mediated signaling during early neocortical development. J. Neurophysiol. 82, 570–583.
Palackal, T., Moretz, R., Wisniewski, H., and Sturman, J. (1986). Abnormal visual cortex development in the kitten associated with maternal dietary taurine deprivation. J. Neurosci. Res. 15, 223–239. doi: 10.1002/jnr.490150212
Peden, D. R., Petitjean, C. M., Herd, M. B., Durakoglugil, M. S., Rosahl, T. W., Wafford, K., et al. (2008). Developmental maturation of synaptic and extrasynaptic GABAA receptors in mouse thalamic ventrobasal neurones. J. Physiol. 586, 965–987. doi: 10.1113/jphysiol.2007.145375
Schousboe, A., Sanchez Olea, R., Moran, J., and Pasantes-Morales, H. (1991). Hyposmolarity-induced taurine release in cerebellar granule cells is associated with diffusion and not with high-affinity transport. J. Neurosci. Res. 30, 661–665. doi: 10.1002/jnr.490300409
Schwab, A., Fabian, A., Hanley, P. J., and Stock, C. (2012). Role of ion channels and transporters in cell migration. Physiol. Rev. 92, 1865–1913. doi: 10.1152/physrev.00018.2011
Shennan, D. B. (1999). Properties of volume-activated taurine and iodide efflux from term human placental tissue. Placenta 20, 485–491. doi: 10.1053/plac.1999.0391
Shinozaki, J., Hanakawa, T., and Fukuyama, H. (2007). Heterospecific and conspecific social cognition in the anterior cingulate cortex. Neuroreport 18, 993–997. doi: 10.1097/WNR.0b013e3281ac2161
Sidman, R. L., and Rakic, P. (1973). Neuronal migration, with special reference to developing human brain: a review. Brain Res. 62, 1–35. doi: 10.1016/0006-8993(73)90617-3
Smith, K. E., Borden, L. A., Wang, C. H., Hartig, P. R., Branchek, T. A., and Weinshank, R. L. (1992). Cloning and expression of a high affinity taurine transporter from rat brain. Mol. Pharmacol. 42, 563–569.
Sturman, J. A. (1981). Origin of taurine in developing rat brain. Brain Res. 254, 111–128. doi: 10.1016/0165-3806(81)90063-8
Sturman, J. A., Moretz, R. C., French, J. H., and Wisniewski, H. M. (1985). Taurine deficiency in the developing cat: persistence of the cerebellar external granule cell layer. J. Neurosci. Res. 13, 405–416. doi: 10.1002/jnr.490130307
Sturman, J. A., Rassin, D. K., and Gaull, G. E. (1977). Taurine in developing rat brain: transfer of [35S] taurine to pups via the milk. Pediatr. Res. 11, 28–33. doi: 10.1203/00006450-197711010-00008
Takuma, K., Matsuda, T., Kishida, Y., Asano, S., Azuma, J., and Baba, A. (1996). Ca2+ depletion facilitates taurine release in cultured rat astrocytes. Jpn. J. Pharmacol. 72, 75–78. doi: 10.1254/jjp.72.75
Tamamaki, N., Yanagawa, Y., Tomioka, R., Miyazaki, J., Obata, K., and Kaneko, T. (2003). Green fluorescent protein expression and colocalization with calretinin, parvalbumin, and somatostatin in the GAD67-GFP knock-in mouse. J. Comp. Neurol. 467, 60–79. doi: 10.1002/cne.10905
Tan, S. S., Kalloniatis, M., Sturm, K., Tam, P. P., Reese, B. E., and Faulkner-Jones, B. (1998). Separate progenitors for radial and tangential cell dispersion during development of the cerebral neocortex. Neuron 21, 295–304. doi: 10.1016/S0896-6273(00)80539-5
Tappaz, M. L. (2004). Taurine biosynthetic enzymes and taurine transporter: molecular identification and regulations. Neurochem. Res. 29, 83–96. doi: 10.1023/B:NERE.0000010436.44223.f8
Valeyev, A. Y., Cruciani, R. A., Lange, G. D., Smallwood, V. S., and Barker, J. L. (1993). Cl- channels are randomly activated by continuous GABA secretion in cultured embryonic rat hippocampal neurons. Neurosci. Lett. 155, 199–203. doi: 10.1016/0304-3940(93)90707-R
Wang, T., Kumada, T., Morishima, T., Iwata, S., Kaneko, T., Yanagawa, Y., et al. (2013). Accumulation of GABAergic neurons, causing a focal ambient GABA gradient, and downregulation of KCC2 are induced during microgyrus formation in a mouse model of polymicrogyria. Cereb. Cortex 4, 1088–1101. doi: 10.1093/cercor/bhs375
Weinstein, C. L., Haschemeyer, R. H., and Griffith, O. W. (1988). In vivo studies of cysteine metabolism. Use of D-cysteinesulfinate, a novel cysteinesulfinate decarboxylase inhibitor, to probe taurine and pyruvate synthesis. J. Biol. Chem. 263, 16568–16579.
Keywords: taurine, GABAA receptor, tonic current, radial migration, non-vesicular release, volume-sensitive anion channel, GAD67, taurine transporter
Citation: Furukawa T, Yamada J, Akita T, Matsushima Y, Yanagawa Y and Fukuda A (2014) Roles of taurine-mediated tonic GABAA receptor activation in the radial migration of neurons in the fetal mouse cerebral cortex. Front. Cell. Neurosci. 8:88. doi: 10.3389/fncel.2014.00088
Received: 12 December 2013; Accepted: 10 March 2014;
Published online: 28 March 2014.
Edited by:
Laura Cancedda, Istituto Italiano di Tecnologia, ItalyReviewed by:
Rustem Khazipov, Institut National de la Santé et de la Recherche Médicale, FranceArnold Kriegstein, University of California at San Francisco, USA
Copyright © 2014 Furukawa, Yamada, Akita, Matsushima, Yanagawa and Fukuda. This is an open-access article distributed under the terms of the Creative Commons Attribution License (CC BY). The use, distribution or reproduction in other forums is permitted, provided the original author(s) or licensor are credited and that the original publication in this journal is cited, in accordance with accepted academic practice. No use, distribution or reproduction is permitted which does not comply with these terms.
*Correspondence: Atsuo Fukuda, Department of Neurophysiology, Hamamatsu University School of Medicine, 20-1 Handayama 1-chome, Higashi-ku, Hamamatsu, Shizuoka 431-3192, Japan e-mail:YXhmdWt1ZGFAaGFtYS1tZWQuYWMuanA=