- 1SUNY Eye Research Consortium, Buffalo, NY, USA
- 2Program in Neuroscience, SUNY – Stony Brook, Stony Brook, NY, USA
- 3Medical Scientist Training Program, SUNY – Stony Brook, Stony Brook, NY, USA
- 4Department of Neurobiology and Behavior, SUNY – Stony Brook, Stony Brook, NY, USA
The mammalian neocortex is composed of a variety of cell types organized in a highly interconnected circuit. GABAergic neurons account for only about 20% of cortical neurons. However, they show widespread connectivity and a high degree of diversity in morphology, location, electrophysiological properties and gene expression. In addition, distinct populations of inhibitory neurons have different sensory response properties, capacities for plasticity and sensitivities to changes in sensory experience. In this review we summarize experimental evidence regarding the properties of GABAergic neurons in primary sensory cortex. We will discuss how distinct GABAergic neurons and different forms of GABAergic inhibitory plasticity may contribute to shaping sensory cortical circuit activity and function.
Introduction
The many varieties of synaptic plasticity provide a high degree of flexibility to neural circuits, allowing organisms to adapt to changing environments and learn from their experiences. In primary sensory cortex different forms of synaptic plasticity can be induced by manipulations of sensory drive, some of which are limited to specific developmental windows (Hubel and Wiesel, 1970; Carmignoto and Vicini, 1992; Finnerty et al., 1999; Morales et al., 2002; Hensch, 2004; Maffei and Turrigiano, 2008a; Espinosa and Stryker, 2012; Levelt and Hübener, 2012). While most studies of sensory cortical plasticity have focused on the role of glutamatergic synapses, it is now clear that GABAergic inputs and their plasticity play a fundamental role in cortical circuit refinement throughout life.
GABAergic neurons comprise a variety of cell types with distinct physiological characteristics, anatomical locations and capacities for plasticity (Markram et al., 2004; Ascoli et al., 2008; Caspary et al., 2008; Maffei, 2011; Méndez and Bacci, 2011; Rudy et al., 2011; Vitalis and Rossier, 2011; Taniguchi, 2014). This diversity may be one of the most important features of cortical circuits, as it confers a wide range of possibilities for stabilizing, refining the activity of and setting the state of other neurons and neural circuits (Földy et al., 2004; Santhakumar and Soltesz, 2004). Tools to facilitate the direct investigation of these diverse neurons have only recently become available; therefore, our understanding of the role of GABAergic neurons in cortical circuit function is just beginning.
In this review we will discuss recent findings on the role of GABAergic synaptic transmission and plasticity in sensory cortex. We will focus on the role of local inhibitory circuits; however, it should be noted that long range projecting inhibitory neurons have been identified in neocortex (Germroth et al., 1989). These neurons have only recently begun to be functionally characterized (Melzer et al., 2012), suggesting that much work still needs to be done to fully understand the diversity of connectivity and action of GABAergic neurons in the brain. GABAergic interneurons are involved in sharpening sensory responses and regulating circuit excitability, and they are a sine qua non of experience-dependent circuit refinement (Ben-Ari et al., 2004; Heimel et al., 2011; Isaacson and Scanziani, 2011; Maffei, 2011; Levelt and Hübener, 2012; Le Magueresse and Monyer, 2013). We will provide evidence for these functions and discuss possible mechanisms involved, as well as common and unique features of GABAergic synaptic plasticity in different sensory cortices. We will conclude with an attempt to reconcile seemingly discrepant experimental results and suggest issues that in our opinion need to be addressed to push this field forward.
GABAergic Inhibition and Computation in Sensory Cortex
The contribution of inhibition to neural network computation goes beyond that of only a regulator of circuit excitability. Inhibitory neurons form highly interconnected networks of electrically and synaptically coupled neurons, and they have a wide range of anatomical and physiological properties ideally suited for driving broad network synchronization (Kawaguchi and Kubota, 1997; Tamás et al., 1998; Galarreta and Hestrin, 1999; Gibson et al., 1999; Amitai, 2001; Galarreta and Hestrin, 2002; Pfeffer et al., 2013; Taniguchi, 2014). Many individual GABAergic neurons connect broadly to local excitatory neurons and specifically to local inhibitory neurons, allowing them to exert their influence over large portions of neural circuits (Hestrin and Galarreta, 2005; Oswald et al., 2009; Packer and Yuste, 2011; Fino et al., 2013; Pfeffer et al., 2013). GABAergic inhibitory neurons are, therefore, ideally situated to contribute to the generation of activity associated with wakefulness and cognitive processing (Tamás et al., 2000; Whittington et al., 2000; Oswald et al., 2009).
Inhibitory and excitatory inputs interact dynamically to maintain neural networks in a balanced state that favors neural computations (McCormick, 2002; Haider and McCormick, 2009). They are dynamically coordinated and co-activated during both spontaneous and sensory evoked activity both in acute slice preparations (Adesnik and Scanziani, 2010; Graupner and Reyes, 2013) and in vivo (Okun and Lampl, 2008; Adesnik and Scanziani, 2010). The coordination of excitatory and inhibitory inputs is believed to underlie dynamic modifications of functional cortical connectivity, and these rapid changes in functional connectivity may be necessary for sculpting sensory responses (Haider et al., 2007; Haider and McCormick, 2009). According to computational models, coordinated and balanced excitation and inhibition can promote decorrelated network activity, which would favor efficient information processing (Renart et al., 2010).
Diversity of Inhibitory Neurons and their Connectivity
Computational models and theories of cortical function often treat inhibitory neurons as a single functional class. However, cortical circuits contain groups of GABAergic neurons that can be distinguished according to their functional properties (Markram et al., 2004; Rudy et al., 2011; Taniguchi, 2014). The heterogeneity of GABAergic neuron subtypes has long hindered our understanding of inhibitory circuits and their specific roles in different aspects of circuit function; therefore, principles for identifying and naming the distinct populations of inhibitory neurons across species are being discussed to facilitate comparisons of results obtained from different species and experimental approaches (Ascoli et al., 2008; DeFelipe et al., 2013). One of the most important features of interneurons is their axonal morphology, which can be used to determine the primary subcellular compartment (axons, dendrites, or soma) that they target for inhibition (Somogyi et al., 1998).
In addition, differential gene expression has become a useful tool for identifying populations of interneurons. Nearly all sensory cortical GABAergic neurons are thought to detectably express one, and only one, of three proteins: the calcium binding protein parvalbumin (PV), the peptide hormone somatostatin (SOM), or the ionotropic serotonin receptor 5HT3aR (Kawaguchi and Kubota, 1997; Lee et al., 2010, 2013; Rudy et al., 2011). Expression of the peptide hormone vasoactive intestinal peptide (VIP) delineates a specific subpopulation of 5HT3aR positive interneurons (Lee et al., 2010; Rudy et al., 2011). Because these markers identify distinct populations and account for nearly all GABAergic neurons, they have provided a useful starting point for the functional investigation of groups of inhibitory neurons in sensory cortex (Rudy et al., 2011); however, there is some overlap in the expression of PV and SOM mRNA, and many more markers have been identified both immunohistochemically and through genetic screens that may help identify important functional subclasses (Cauli et al., 1997, 2000; Markram et al., 2004; Freund and Katona, 2007; Lee et al., 2010; DeFelipe et al., 2013; Taniguchi, 2014).
Interneurons have long been classified by their distinct morphological phenotypes and biophysical properties, and these correspond reasonably well with genetic markers. However, the evidence upon which these classifications are drawn relies on observations made across several species, brain regions and ages. These classifications represent the most common features of interneurons marked by these genes that have been observed, but the heterogeneity within these groups has not been systematically studied, especially across development, cortical layers and cortical regions. Most PV expressing neurons show fast spiking (FS) patterns when depolarized and can be classified morphologically as either basket cells or chandelier cells (Kawaguchi and Kubota, 1993; Wang et al., 2002; Markram et al., 2004; Xu and Callaway, 2009; Rudy et al., 2011). Basket cells have axons that synapse predominantly onto the soma and the perisomatic regions of cortical pyramidal neurons, wrapping around the soma like a basket (Kawaguchi and Kubota, 1997; Tamás et al., 2000). Chandelier cells, with axonal boutons resembling candlesticks or “cartridges” (Szentágothai and Arbib, 1974), are a population of interneurons whose axons predominately contact axon initial segments (Somogyi, 1977). Chandelier cells were originally thought to comprise a homogeneous subpopulation of FS PV positive neurons; however, recent evidence that many chandelier cells do not express PV suggests that two types of functionally distinct chandelier cells could exist (Taniguchi et al., 2013; Taniguchi, 2014). While most PV neurons are FS, depending upon the recording and classification method considered, not all PV neurons are FS and not all FS neurons are PV positive (Gray and McCormick, 1996; Cauli et al., 1997; Markram et al., 2004; Ma et al., 2006; Moore and Wehr, 2013; Taniguchi et al., 2013; but see Rudy et al., 2011).
The largest subgroup of SOM expressing neurons is Martinotti cells, which have regular spiking (RS) action potential widths when depolarized, and vertical axons that preferentially target superficial dendritic tufts (Kawaguchi and Kubota, 1997; Thomson et al., 2002; Wang et al., 2004; Ali and Thomson, 2008). However, SOM expressing synapses have also been observed on soma (Gonchar et al., 2002). In addition, a subgroup of SOM positive neurons has spike widths characteristic of FS cells and extensive axonal arborization within layer 4 (Ma et al., 2006; Miyoshi et al., 2007; Xu et al., 2013). The morphology and subcellular targeting of these neurons has not been fully characterized.
VIP is expressed in cells with morphologies consistent with both dendrite and soma targeting interneurons (Lorén et al., 1979; Morrison et al., 1984; Kawaguchi and Kubota, 1996, 1997; Lee et al., 2010; Miyoshi et al., 2010). 5HTR3aR/VIP- neurons comprise the majority of layer 1 neurons and neurogliaform cells (Lee et al., 2010). Neurogliaform cells form gap junction connections with many other classes of cortical interneurons (Simon et al., 2005). They have been proposed to use primarily volume rather than synaptic transmission of GABA (Oláh et al., 2009); however, they have the capacity to regulate specific portions of neural circuits (Chittajallu et al., 2013).
Beyond preference for targeting specific subcellular compartments, patterns of connectivity within and between interneuron subtypes and pyramidal neurons are beginning to emerge. For example, while PV and SOM positive neurons frequently contact pyramidal neurons, VIP positive neurons rarely inhibit pyramidal neurons (Pfeffer et al., 2013). Instead, VIP neurons predominately inhibit SOM neurons (Lee et al., 2013; Pfeffer et al., 2013; Pi et al., 2013), which in turn target VIP and PV neurons (Pfeffer et al., 2013). This connectivity scheme places VIP neurons in an ideal position to mediate disinhibition of excitatory neurons (Lee et al., 2013; Pi et al., 2013). In the primary input layer of sensory cortex, layer 4, basket cells receive direct thalamic input (Beierlein et al., 2003; Cruikshank et al., 2007) and can therefore shape cortical circuit activation through feedforward control over excitatory neuron activity (Gabernet et al., 2005). In addition to inhibiting excitatory neurons, many PV positive neurons target other PV positive neurons (Galarreta and Hestrin, 1999, 2002; Pfeffer et al., 2013).
The ability to perform experiments ex vivo in rodent models has allowed for high resolution analysis of biophysical properties and local connectivity of different populations of inhibitory neurons. For example, it was recently shown that even within the PV positive population of inhibitory neurons in primary visual cortex (V1), distinct biophysical properties (Helm et al., 2013) correlate with the level of expression of the SAP97 scaffold protein (Akgul and Wollmuth, 2013). Since inhibitory neuron populations with distinct biophysical properties, molecular markers, birth dates and birth regions have been identified, it has become possible to label specific subpopulations using genetic approaches to facilitate identification and recording from these groups of inhibitory neurons both ex vivo and in vivo (Taniguchi et al., 2011; Taniguchi, 2014).
While genetic approaches to marking neurons in intact animals are extremely powerful and promising, some of the strategies to genetically label interneuron populations may interfere with GABAergic transmission (Tamamaki et al., 2003; Wang et al., 2009; see discussion of the GAD67-GFP (Δneo) mouse line below) or mislabel neurons, potentially leading to confounds in the interpretation of the data, or producing contrasting findings (Hu et al., 2013). For example, neurons expressing Cre-dependent genes via the somatostatin promoter (Taniguchi et al., 2011) include a population (up to 5–10% of labeled neurons) of FS PV neurons (Hu et al., 2013; Pfeffer et al., 2013), while this degree of overlap has not been observed using immunohistochemical staining (Lee et al., 2010). In addition, the level of expression of neuronal markers used to identify inhibitory neurons can change during development (Wahle, 1993; Felmy and Schneggenburger, 2004) and/or in an activity-dependent fashion (Gomes da Silva et al., 2010; Martin del Campo et al., 2012; Hou and Yu, 2013), possibly affecting the classification of inhibitory neurons. Similarly, the subcellular compartments targeted by inhibitory synapses are under genetic control and can be modified in an activity dependent manner (Bloodgood et al., 2013). Finally, some functionally distinct population of inhibitory neurons may be distinguishable only by graded levels of gene expression, making them difficult to manipulate with genetically encoded tools alone. Comparing the properties, connectivity and function of inhibitory neurons in different circuits, species and developmental stages will be necessary to distinguish general properties of inhibitory neuron function from specific properties that depend on interneuron subtype, species, region or experimental manipulation. However, the heterogeneity within marked populations and potential plasticity of markers and subcellular targets must be taken into account.
Manipulating and Measuring the Activity of GABAergic Neurons in vivo
Several approaches have been employed to study the activity of GABAergic neurons in vivo (Table 1). The characteristic narrow spike waveform of FS inhibitory neurons allows them to be identified and separated from RS neurons, which are mostly pyramidal cells, in extracellular and juxtacellular recordings. Because identification of waveforms extracellularly is prone to error (Henze et al., 2000; González-Burgos et al., 2005; Gold et al., 2006), channelrhodopsins (ChR) can be expressed in subsets of interneurons and photoactivated to rapidly induce spikes and more precisely identify units recorded extracellularly (Cardin, 2012; Moore and Wehr, 2013). Multielectrode recording arrays are useful for recording extracellularly from several neurons simultaneously, but many are limited in their ability to record from superficial layers. Whole cell recording with post-hoc morphological reconstruction allows for more precise laminar identification than is possible with extracellular recordings, and depolarization induced firing patterns and membrane potential fluctuations can be measured. However, due to the size of interneurons relative to pyramidal neurons and their relative scarcity, this technique has low yield. To improve yield and specificity, recordings can be performed in transgenic animals expressing fluorescent markers in populations of GABAergic neurons. Using two-photon microscopy, patch pipettes can be targeted to neurons either expressing or lacking a specific marker (Liu et al., 2009; Gentet et al., 2010). Florescent labeling of GABAergic neurons can also be combined with calcium imaging, which greatly increases the number of neurons that can be simultaneously recorded from at the expense of resolving precise numbers of action potentials (Sohya et al., 2007; Kerlin et al., 2010). Unfortunately, techniques relying on transgenic animals and two photon microscopy are largely limited to studying the superficial layers of mouse cortex at present.
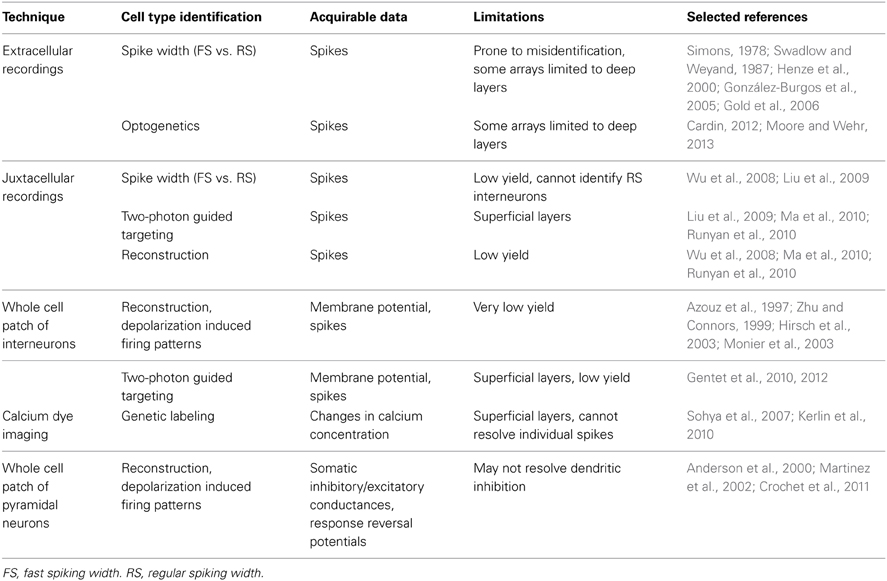
Table 1. Approaches for recording the activity of inhibitory neurons and inhibitory postsynaptic responses in vivo.
Whole cell recordings can also be used to measure inhibitory drive postsynaptically. Somatic inhibitory and excitatory currents/conductances can be measured to investigate how inhibition contributes to shaping sensory responses (Anderson et al., 2000). The dynamic reversal potential of a sensory response can also be calculated, allowing inferences to be made about the contribution of excitatory and inhibitory receptors to the response (Crochet et al., 2011; Sachidhanandam et al., 2013). These techniques are limited, however, in that they may fail to fully resolve inputs to distal dendrites. Distal inhibitory inputs may effectively shunt excitatory inputs that would have led to regenerative activity (Gidon and Segev, 2012) without themselves being fully resolved. Functional GABA mediated inhibition has been observed that requires regenerative dendritic activity to be measurable (Palmer et al., 2012). Measuring inhibitory currents/conductances requires minimizing the contribution of voltage gated channels and would obscure their inhibition. Further, the analysis of inhibitory responses with this approach does not allow experimenters to selectively identify the contribution of distinct inhibitory neuron subgroups to inhibitory synaptic potentials (or currents) evoked by sensory stimuli.
Pharmacology, genetics, optogenetics and alterations in sensory experience have all been used to manipulate GABAergic neurotransmission in vivo (Table 2). Application of the potent GABAA receptor agonist muscimol has been used to increase inhibition (Reiter and Stryker, 1988); however, the ubiquity of GABAA receptors makes it difficult to interpret the results of these experiments as being due specifically to increased synaptic and extrasynaptic inhibition versus the secondary effect of dampening all cortical activity. Benzodiazepines allosterically enhance GABAergic neurotransmission at specific GABAA receptors with benzodiazepine binding sites (Benke and Möhler, 2006; Gielen et al., 2012) and are therefore a more selective tool for probing inhibitory circuit function. Conversely, GABAergic transmission can be disrupted either by administering GABA receptor antagonists or by impairing GABA synthetic pathways. For example, chronic administration of the GAD antagonist 3-mercaptopropionic acid (3-MPA) lowers cortical GABA (Harauzov et al., 2010). Transgenic mice that have altered expression of proteins related to GABAergic neuron function can be used to study the effects of either developmental or induced changes in GABAergic function (Hanover et al., 1999; Huang et al., 1999; Tamamaki et al., 2003; Fagiolini et al., 2004; Wang et al., 2009; Palmer et al., 2012; Nahmani and Turrigiano, 2014). To study the roles of interneuron groups in sensory processes, the light gated ion channel/pumps ChR and halorhodopsin/archaerhodopsin (HaR/ArchR) can be specifically expressed to excite or inhibit a class of neurons upon photoactivation (Cardin, 2012). While results using photoactivation are certainly interesting, it is unclear how artificially evoked (Cardin, 2012), broad patterns of interneuron activation relate to their physiological activation by sensory stimuli. A bold approach to increasing the specificity of photoactivation is to use single cell electroporation to limit expression to a small number of cells (Cottam et al., 2013).
Alterations in sensory experience that induce changes in GABAergic circuitry can be studied in vivo to allow inferences to be made about the effects of the circuit change on sensory responses. These inferences are, of course, limited by the plethora of changes that may be induced in cortical and subcortical circuits: excitatory, inhibitory and neuromodulatory. In this review, we will consider changes in sensory experience in three categories. Sensory deprivation will refer to manipulations that lead to an overall reduction of sensory drive (e.g., binaural hearing loss, dark rearing, or trimming all whiskers on a vibrissal pad). This type of manipulation is typically initiated shortly after birth. Deprivation of one sensory modality can alter cortical circuit activity and responsiveness to sensory stimuli even in sensory areas that are not directly driven by the sensory organ that has been manipulated (Zheng et al., 2014). Environmental enrichment will refer adding objects to an animal's cage that provide multisensory stimulation (Sale et al., 2009). Sensory alteration will refer to manipulations that reduce, or fundamentally alter, a specific sensory input, usually asymmetrically, without eliminating it entirely (e.g., monocular lid suture, surgical strabismus, selective hearing ablation and selective whisker removal). When these manipulations are induced during sensitive periods in postnatal development, they often lead to diminished sensory function if later reversed (Hubel and Wiesel, 1970; Carmignoto and Vicini, 1992; Finnerty et al., 1999; Morales et al., 2002; Hensch, 2004; Maffei and Turrigiano, 2008a; Espinosa and Stryker, 2012; Levelt and Hübener, 2012).
GABAergic Neuron Sensory Response Properties
The diversity of genetic, morphological and electrophysiological properties, as well as patterns of connectivity of GABAergic neurons, suggests that different cell types may have distinct functional properties. Studies of GABAergic neurons' receptive field properties have confirmed this hypothesis, yet sometimes with contrasting results. This is not too surprising, given that important functional differences exist between cortical layers and neuronal subtypes, and different recording techniques selectively favor certain layers and interneuron subtypes over others (Table 1). Additionally, the laminar distribution and cortical organization of neurons with different response properties differs strikingly between species. Cats have ocular dominance and orientation columns, while rodents and rabbits do not (Hubel and Wiesel, 1963; Tiao and Blakemore, 1976; Murphy and Berman, 1979; Grinvald et al., 1986; Métin et al., 1988; Girman et al., 1999; Schuett et al., 2002; Van Hooser et al., 2005). Cats, rats, and mice all have different laminar distributions of neurons with simple and complex visual receptive fields (Hubel and Wiesel, 1962; Kelly and Van Essen, 1974; Gilbert, 1977; Gilbert and Wiesel, 1979; Lin et al., 1979; Parnavelas et al., 1983; Niell and Stryker, 2008). These interspecies differences in the gross organization of neuronal response properties raise the possibility that some features of inhibitory neuron response properties may also be species specific.
In cat V1 there is evidence in favor of FS inhibitory neurons being both broadly and narrowly tuned to sensory stimuli (Azouz et al., 1997; Hirsch et al., 2003; Cardin et al., 2007; Nowak et al., 2008). Tuning appears to vary by cortical layer, as FS neurons in the primary thalamorecipient layer, layer 4, are either broadly tuned or untuned (Hirsch et al., 2003; Cardin et al., 2007; Nowak et al., 2008). FS neurons outside layer 4 show tuning similar to RS neurons (Cardin et al., 2007), but are more binocularly driven (Aton et al., 2013). This suggests that inhibition may modulate sensory response properties in a lamina-specific fashion. In awake rabbits, putative layer 4 FS neurons have broader orientation, direction and frequency tuning, are more binocular and are more sensitive to contrast than putative RS neurons (Swadlow and Weyand, 1987; Swadlow, 1988; Zhuang et al., 2013).
In the anesthetized mouse, populations of interneurons identified as either PV positive or FS are more broadly tuned for orientation than both excitatory neurons (Niell and Stryker, 2008; Kuhlman et al., 2011; Zariwala et al., 2011; Atallah et al., 2012; Li et al., 2012b; Cottam et al., 2013; Runyan and Sur, 2013; but see Runyan et al., 2010) and SOM interneurons (Ma et al., 2010; Cottam et al., 2013). FS neurons are also more binocularly responsive than RS neurons (Yazaki-Sugiyama et al., 2009; Kameyama et al., 2010). Within the PV population, however, there is great tuning diversity, with some PV neurons being well tuned (Runyan et al., 2010; Zariwala et al., 2011; Runyan and Sur, 2013). Interestingly, PV neurons with longer dendrites have broader orientation selectivity than those with shorter dendrites (Runyan and Sur, 2013). Some of the heterogeneous aspects of PV neuron tuning may be due to the diversity of morphological groups of neurons expressing PV: basket cells (small and large) and a subgroup of chandelier cells. While both basket and chandelier cells exert powerful control over pyramidal neuron excitability and firing, they receive distinct sets of inputs, at least in somatosensory cortex (Xu and Callaway, 2009). SOM neurons exhibit weaker responses to visual stimuli than either excitatory or PV neurons (Ma et al., 2010) and exhibit sharper population orientation tuning than PV neurons (Ma et al., 2010; Cottam et al., 2013). Using optogenetic photoactivation of a small number of SOM neurons, it was shown that during visual stimulation, SOM neurons are able to more strongly inhibit responses of PV neurons than excitatory neurons. Interestingly, SOM neuron photoactivation sharpened PV neuron tuning (Cottam et al., 2013).
In layer 2/3 of GAD67-GFP (Δneo) mice, GABAergic neurons are only weakly orientation selective (Sohya et al., 2007; Bonin et al., 2011), and have similar binocularity to glutamatergic neurons (Gandhi et al., 2008). In these mice no difference in selectivity exists between FS and RS GABAergic neurons (Liu et al., 2009) or between SOM, PV, or VIP positive neurons (Kerlin et al., 2010). However, GAD67-GFP (Δneo) mice have reduced orientation selectivity of both GABAergic (Runyan et al., 2010) and glutamatergic neurons compared with control strains (Hagihara and Ohki, 2013; but see Liu et al., 2009). GAD67-GFP (Δneo) mice have decreased expression of GAD67 (Wang et al., 2009). Furthermore, their levels of GABA are reduced neonatally and in the adult (Tamamaki et al., 2003). These caveats should be carefully considered when interpreting data obtained using this strain.
In primary auditory cortex (A1) of anesthetized cats, putative FS inhibitory neurons have short response latencies and broad spectral tuning (Atencio and Schreiner, 2008). Similarly, rat layer 4 FS neurons have faster response latencies and broader tuning than layer 4 pyramidal neurons (Wu et al., 2008). In mice, units identified extracellularly as FS have faster response latencies and broader tuning than RS units (Moore and Wehr, 2013). Once subdivided into PV positive and PV negative units, however, PV positive units did not differ in frequency tuning or response latency from PV negative units, but did have higher spontaneous and evoked firing rates. They also had shallower response gain and were less well tuned for stimulus intensity than PV negative units. Very narrowly tuned PV positive neurons were found, but very rarely. These findings were interpreted as evidence for a role of PV neurons in modulating gain and intensity tuning (Moore and Wehr, 2013). Differently, in superficial layers PV neurons were found to be more broadly tuned than either excitatory or SOM positive neurons, while SOM neurons showed delayed responses compared to PV and excitatory neurons (Li et al., 2014b). VIP neurons are also tone responsive; however, their tuning properties have not been characterized (Pi et al., 2013).
In the barrel portion of primary somatosensory cortex (S1) in rats (paralyzed, anesthetized or sedated) and awake rabbits, putative FS inhibitory neurons show broader sensitivity to the direction of whisker deflection and/or to the number of whiskers stimulated than RS units (Simons, 1978; Simons and Carvell, 1989; Swadlow, 1989; Bruno and Simons, 2002; Gabernet et al., 2005; but see Zhu and Connors, 1999). In addition, they show faster and more reliable responses to stimulation than putative excitatory neurons, especially in layer 4 (Simons, 1978; Swadlow, 1989; Armstrong-James et al., 1993; Bruno and Simons, 2002; Swadlow and Gusev, 2002), where both excitatory and inhibitory neurons are driven monosynaptically from the thalamus (Beierlein et al., 2003; Cruikshank et al., 2007). Within rat layer 2/3, chandelier cells have broader receptive fields than other FS interneurons (Zhu et al., 2004). Within layer 1, deep-projecting GABAergic neurons are well tuned and respond to stimuli rapidly, whereas local interneurons respond slowly and are poorly tuned (Zhu and Zhu, 2004). In the hindpaw region of S1, a sizable portion of inhibitory neurons respond to ipsilateral stimulation (Palmer et al., 2012). Differently from rats and rabbits, putative FS inhibitory neurons in layer 4 of mouse barrel cortex show similar angular tuning to RS units (Kwegyir-Afful et al., 2013). In contrast to other cell types in mouse layer 2/3, SOM neurons are active during quite wakefulness and inhibited during sensation (Gentet et al., 2012). The inhibition of SOM neurons in S1 during whisking is dependent upon VIP neurons, which are driven to increase their activity during whisking by primary motor cortex (Lee et al., 2013).
Few studies have examined the tuning of GABAergic inhibitory interneurons in primary chemosensory cortices. In the primary gustatory cortex (GC) of anesthetized rats, putative FS inhibitory neurons are primarily tuned to one or two (of four) tastants, almost always including NaCl; while RS units are more broadly tuned and may respond several tastants (Yokota et al., 2011). In the primary olfactory cortex (Pir) of anesthetized rats, layer 1 GABAergic neurons are broadly tuned to odors compared to layer 2/3 pyramidal neurons (Poo and Isaacson, 2009).
Finally, recent studies have identified putative inhibitory interneuron involvement in multisensory integration and learning. Inhibitory neurons in layer 2/3 of a mouse visuotactile area between visual and somatosensory cortices exhibit less multisensory enhancement of evoked responses than pyramidal neurons, and their activation suppresses multisensory enhancement of activity in pyramidal neurons (Olcese et al., 2013). In mouse V1, a local inhibitory circuit can be driven by an auditory stimulus (Iurilli et al., 2012), and in GC of awake rats, a few putative FS inhibitory neurons were found that respond to auditory cues that triggered expectation of taste delivery (Samuelsen et al., 2012). Mouse A1 layer 2/3 VIP neurons and layer 1 interneurons inhibit other GABAergic neurons during auditory fear conditioning (Letzkus et al., 2011) and discrimination tasks (Pi et al., 2013), respectively.
While it is clear that there are lamina and species specific interneuron response properties, some common features exist within cell types (Table 3). FS and PV neurons, which predominantly provide somatic, perisomatic and axonal inhibition, tend to be broadly and bilaterally tuned, especially within layer 4. Differently, SOM neurons, which predominantly provide dendritic inhibition, tend to be less strongly responsive to, or inhibited by, stimuli, and those that are responsive to stimuli are tuned intermediately compared to PV and pyramidal neurons. It is unclear how FS SOM neurons and RS PV neurons fit into this scheme. Little is known about the tuning properties of 5HT3aR neurons, whether VIP positive or negative; however, the VIP positive subgroup has been implicated in mediating disinhibition during associative learning and active sensation (Lee et al., 2013; Pi et al., 2013). When comparisons have been made between subgroups of these populations, differences in response properties tied to morphology have been observed. Full understanding of the roles of GABAergic neurons, therefore, will require more detailed investigations of these subclasses, as well as further investigation into the function of genetically identified interneuron classes in subgranular layers.
GABAergic Inhibition and Sensory Responses in Excitatory Cells
The varied response properties of GABAergic neurons make them ideal candidates to modulate the activity of excitatory neurons during sensory processing. In vivo pharmacological manipulation of GABAergic transmission alters receptive fields properties in a number of different species and cortical regions, suggesting a role for cortical inhibition in controlling the stimulus preference and receptive field structure of excitatory neurons (Sillito, 1975, 1979; Sillito et al., 1980; Kyriazi et al., 1996; Tremere et al., 2001; Li et al., 2002; Foeller et al., 2005; Jeong et al., 2009; Razak and Fuzessery, 2009; Isaacson and Scanziani, 2011; Katzner et al., 2011). However, the results of grossly disrupting or augmenting inhibition in cortex do not provide evidence that GABAergic neurons dynamically shape receptive fields, and their contribution to shaping the receptive fields of glutamatergic neurons is hotly debated (Isaacson and Scanziani, 2011; Liu et al., 2011; Wu et al., 2011; Priebe and Ferster, 2012). Depending on the location and specificity of inhibitory inputs onto a pyramidal cell, GABAergic neurons with a range of tuning properties could contribute inhibition tuned similar to, more tightly than or more broadly than excitatory inputs to that neuron, or tonic, stimulus invariant inhibition.
There is general agreement that an inhibitory mechanism contributes to pyramidal neurons having narrower spiking receptive fields than subthreshold receptive fields (Priebe and Ferster, 2008; Isaacson and Scanziani, 2011; Tan et al., 2011; Wu et al., 2011). This sharpening effect of inhibition occurs, at least in part, through an “iceberg” effect, where inhibition contributes to the spike threshold, below which changes in membrane potential do not alter firing. This effect can occur independently of the relative tuning widths of inhibitory and excitatory inputs (see Isaacson and Scanziani, 2011). Static changes in the level of inhibition, independent of tuning, could alter the sharpness of the membrane potential dynamics in response to spiking input/output transformation, effecting a change in gain. Inhibitory inputs with different tuning than excitatory inputs, if they exist, would provide additional sharpening (Wu et al., 2008; Liu et al., 2011).
In V1, lateral inhibition could account for many receptive field properties, including orientation tuning, spatial segregation of light and dark receptive subfields, direction selectivity, contrast invariance and suppression. However, it has also been proposed that most of these response properties can be accounted for by a model based on the properties of feedforward, excitatory thalamic inputs that have been observed in vivo, coupled with a spike threshold determined in part by inhibitory inputs (Priebe and Ferster, 2012). However, the ability to account for most receptive field properties without lateral inhibition does not rule out a role for lateral inhibition. We will focus our discussion on the evidence for and against lateral inhibition being observed and playing a role in shaping receptive fields.
Inhibitory and excitatory light and dark receptive subfields are largely spatially segregated in the visual cortex of cats (Ferster, 1988; Hirsch et al., 1998; Priebe and Ferster, 2005). The spatial opposition of inhibition and excitation was proposed to allow for linear summation of inputs: at a given location within a receptive field, dark and light stimuli evoke opposite responses (Priebe and Ferster, 2005). Conversely, voltage clamp recordings from simple cells in mice have revealed spatial overlapping of receptive subfields, with inhibitory light and dark subfields similarly centered and lying between slightly segregated excitatory light and dark subfields. This suggests a role for inhibition in the segregation of light and dark receptive fields in mice (Liu et al., 2010).
Orientation tuning of excitatory and inhibitory inputs to pyramidal neurons has been observed to be either similarly tuned (Anderson et al., 2000; Tan et al., 2011) or to have a variety of tuning schemes (Martinez et al., 2002; Monier et al., 2003) in cat V1. Orientation tunings of inhibition and excitation were found to be similar in layers 2–4 but different in layer 5 (Martinez et al., 2002). In mice, similar tuning (Tan et al., 2011) and tuning of inhibition just broader than excitation (Liu et al., 2011; Li et al., 2012b) have both been reported. To address whether specific subclasses of inhibitory neurons have the capacity to alter orientation tuning, optogenetic stimulation has been used to control the activation of GABAergic neurons during visual stimulation while recording responses in superficial excitatory neurons. Photoactivation of PV neurons preferentially inhibited responses to optimal orientation without changing orientation selectivity (Atallah et al., 2012; Wilson et al., 2012), while photoactivation of SOM neurons inhibited responses at all orientations equally, sharpening orientation selectivity (Wilson et al., 2012). However, in a contrasting study PV neuron photoactivation sharpened orientation selectivity with minimal effects at optimal orientations, while SOM and VIP positive neurons' photoactivation suppressed firing but did not alter orientation tuning (Lee et al., 2012). Further, performance in an orientation discrimination task was improved by selective photoactivation of PV positive neurons (Lee et al., 2012). The differences in results may be due to the stimulation parameters used (Lee et al., 2012). As discussed above, it is unclear how these results relate to physiological roles of inhibitory neurons.
Experiments examining the role of inhibition in sharpening tuning width in sensory areas other than V1 have yielded similarly contrasting results. In rodent A1, frequency tuning of inhibitory inputs has been found to be approximately similar to (Wehr and Zador, 2003; Zhang et al., 2003; Tan et al., 2004; Froemke et al., 2007; Tan and Wehr, 2009; Dorrn et al., 2010; Li et al., 2014a) or slightly broader than (Wu et al., 2008; Sun et al., 2010) excitatory inputs. In addition, intensity tuning of excitatory and inhibitory inputs to non-monotonically tuned neurons has been observed to be similarly (Wehr and Zador, 2003) and differentially tuned (Wu et al., 2006; Tan et al., 2007). In rat Pir, inhibitory inputs to layer 2/3 pyramidal neurons are broadly tuned to odors (Poo and Isaacson, 2009).
Experimental evidence in favor of a role for lateral inhibition in sharpening the selectivity of tuning curves has been found, but it has not been observed consistently either within species or cortical regions (Table 4; Priebe and Ferster, 2012). Possible reasons for these inconsistencies include anesthetic choices, stimulus parameters, age of animals, recording layers and experimental approaches. Recent data from layer 2/3 of mouse V1 demonstrated that in awake mice the ratio of inhibition to excitation observed in response to visual stimuli is much greater than under anesthesia (Haider et al., 2013). Further, strong inhibition could be evoked by stimulation in the receptive field surround only during wakefulness (Haider et al., 2013). Thus, the state of a neuron or network can profoundly affect receptive field properties and alter the function of inhibition within a circuit. In layer 4 of mouse V1, increasing the contrast of a grating stimulus broadens inhibitory tuning, leading to contrast-dependent sharpening of orientation selectivity (Li et al., 2012a). Age-dependent sharpening (Dorrn et al., 2010) and widening (Sun et al., 2010) of inhibitory tunings have been reported in rat A1. Developmental broadening of inhibition, both in the tuning of PV neurons and in inhibitory inputs to pyramidal cells has been observed in mouse V1 (Kuhlman et al., 2011; Li et al., 2012b). Therefore, there may be specific conditions and developmental windows in which lateral inhibition contributes substantially to sharpening neuronal receptive fields. A neural network computational model has been developed that can operate in both co-tuned and lateral inhibition modes, depending upon the input received (Levy and Reyes, 2011), and another set of theoretical models suggests that the diversity of receptive fields seen in vivo may be accounted for by the existence of multiple network architectures existing simultaneously (de la Rocha et al., 2008; Piëch et al., 2013). These results, coupled with the limitations of recording inhibition somatically, make definitive conclusions against a role for lateral inhibition in sharpening response selectivity tenuous.
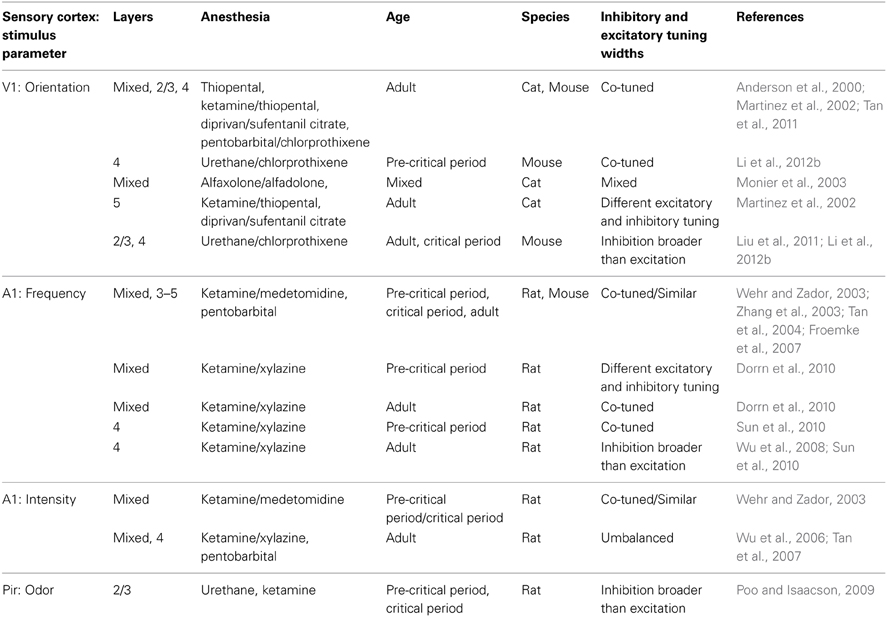
Table 4. Relative tuning of excitatory and inhibitory somatic conductances/currents in excitatory neurons.
The relative timing of excitatory and inhibitory inputs to a neuron can influence its ability to integrate inputs. Inhibition that follows excitation with a brief delay can create a narrow temporal window within which spiking can occur (Wehr and Zador, 2003; Wilent and Contreras, 2004; Higley and Contreras, 2006). If the delay before the onset of inhibition varies with the stimulus, this delay could participate in coding. In rat S1, excitatory and inhibitory inputs to layer 3 and 4 neurons are poorly tuned for the direction of a whisker deflection; however, the temporal gap before the onset of inhibition is greatest for the preferred direction (Wilent and Contreras, 2005). In layer 6 of rat A1, a majority of pyramidal neurons receive strong tone-evoked synaptic inhibition prior to excitation and are thus inhibited by tonal stimulation (Zhou et al., 2010). Interestingly, the time course of excitatory and inhibitory responses to oriented gratings in V1 differs between species: inhibition and excitation wax and wane in opposition in cats (Hirsch et al., 1998; Anderson et al., 2000; Monier et al., 2003; Priebe and Ferster, 2005; Tan et al., 2011), while they are modulated synchronously in the mouse (Tan et al., 2011). Species-specific differences in light and dark receptive subfields have been proposed to underlie this discrepancy (Tan et al., 2011).
In addition to possibly mediating spike timing and tuning curves, inhibition may play a role in response suppression and in maintaining sparseness of spiking responses. Response suppression occurs when a stimulus that would not by itself alter firing of a neuron inhibits the response of that neuron to another stimulus. While GABAergic neurons mediate some forms of response suppression, they likely do not mediate all response suppression. For example, when two tones of the same frequency are played in rapid succession, the cortical response to the second tone is suppressed. As both the onset and offset of stimuli can evoke neuronal responses with transient inhibitory and excitatory components, one hypothesis explaining this effect involves the first tone's offset evoking inhibition that suppresses the response to the second tone (Borg-Graham et al., 1998; Wehr and Zador, 2005; Scholl et al., 2010). However, Scholl et al. found that in rat A1 there is different frequency tuning of inputs for tone onset and offset (Scholl et al., 2010). This differential tuning allows the onset and offset of a given tone to evoke different excitatory and inhibitory current ratios, albeit transiently. Therefore, “forward” suppression of a second tone of the same frequency can only be mediated through feedforward inhibition generated by the first tone's onset if the second tone follows within the brief time window of the first tone's onset inhibitory current (Scholl et al., 2010).
In V1, a stimulus placed adjacent to a neuron's spiking receptive field can alter its responses to stimuli within the spiking receptive field, a phenomenon referred to as surround suppression. Surround suppression has been shown to be mediated, at least in part, through intracortical inhibition (Ozeki et al., 2009; Haider et al., 2010; Adesnik et al., 2012). However, there may be multiple mechanisms through which surround suppression occurs, depending on the nature of the stimuli. For example, in cats, surround suppression by optimally oriented gratings decreases the total excitatory and inhibitory inputs onto a cell: a transient increase in inhibition can be evoked by surround stimuli, which ultimately leads to suppression of both cortical excitatory and inhibitory inputs (Ozeki et al., 2009). For “naturalistic” stimuli, movies of wildlife, adding stimuli to the receptive field surround increases spiking reliability and firing sparseness (Haider et al., 2010). This effect is mediated through an increase in evoked inhibitory potentials throughout the stimulus duration and corresponds with an increase in FS interneuron firing (Haider et al., 2010). Perhaps the discrepancies between these studies could be accounted for by the ever changing nature of the “naturalistic” stimuli, with the many moving edges evoking successive bouts of inhibition that blend together (Haider et al., 2010). In layer 2/3 of awake mice, inhibition through SOM positive interneurons accounts for approximately 30% of surround suppression by optimally oriented gratings (Adesnik et al., 2012).
In layer 5 of S1, stimulation of the ipsilateral paw just prior to stimulating the contralateral paw suppresses the neuronal response to the contralateral paw. This suppression is mediated by layer 1 interneurons driven by colossal projections that provide dendritic inhibition via GABAB receptor activation (Pérez-Garci et al., 2006; Palmer et al., 2012). These findings suggest that multiple intracortical mechanisms exist to generate suppression, with different mechanisms likely contributing to the suppression of different types of stimuli.
Inhibition is ideally suited to contribute to the maintenance of response sparseness. Interestingly, while S1/barrel cortex layer 2/3 pyramidal neurons uniformly show membrane potential responses to whisker stimulation, only ~10% spike during active touch (Crochet et al., 2011). This has been attributed to whisker responses having reversal potentials below spike threshold (Crochet et al., 2011; Sachidhanandam et al., 2013). These responses have reversal potentials well below those of excitatory receptors, suggesting that GABA receptor mediated currents may contribute to preventing these neurons from reaching spike threshold (Sachidhanandam et al., 2013). In favor of this interpretation, there is experimental evidence that whisker stimulation activates inhibitory neurons (Simons, 1978; Simons and Carvell, 1989; Swadlow, 1989; Armstrong-James et al., 1993; Zhu and Connors, 1999; Bruno and Simons, 2002; Swadlow and Gusev, 2002; Beierlein et al., 2003; Zhu et al., 2004; Zhu and Zhu, 2004; Gabernet et al., 2005; Cruikshank et al., 2007; Kwegyir-Afful et al., 2013; Lee et al., 2013; Sachidhanandam et al., 2013) and increases inhibitory drive onto excitatory neurons (Wilent and Contreras, 2005). Optogenetic photoactivation of PV neurons during a detection task both masked subthreshold responses and prevented behavioral responses (Sachidhanandam et al., 2013). These results suggest that inhibition can be precisely tuned to allow sparse firing in layer 2/3, keeping most neurons just below spike threshold after stimulation.
Additional circuit precision can be gained through subcellular compartment specific inhibition. While S1 layer 5 pyramidal neurons are rarely connected to each other directly, they are more commonly coupled disynaptically through layer 5 Martinotti cells (Silberberg and Markram, 2007). When activated, these Martinotti cells can prevent the generation of calcium spikes in the dendritic tufts of layer 5 pyramidal neurons (Murayama et al., 2009). Modeling of this circuit suggests that Martinotti cells are responsible for maintaining the dynamic range of dendritic calcium responses to paw shock that are observed in vivo (Murayama et al., 2009).
GABAergic neurons do not only contact excitatory neurons, but form networks of electrically and synaptically coupled interneurons, often comprised of the same cell type, that can be activated synchronously (Hestrin and Galarreta, 2005; Oswald et al., 2009; Pfeffer et al., 2013). This connectivity is especially well suited to allow for synchronization of local circuits and is thought to contribute to the generation of oscillatory network behaviors (Tamás et al., 2000; Whittington et al., 2000; Oswald et al., 2009). The synchronicity of membrane potential oscillations between neurons can vary depending on stimuli. Neurons with similar receptive fields increase their membrane potential cross-correlation in response to optimal stimuli and decrease it in response to non-optimal stimuli (Lampl et al., 1999). Regardless of relative tuning, coherence in the gamma band between neurons increases for a wide variety of visual stimulus conditions (Yu and Ferster, 2010). Fast rhythmic bursting cells can fire bursts of action potentials in the gamma range (20–80 Hz) in response to visual stimuli, possibly contributing to visually evoked gamma oscillations (Cardin et al., 2005). This evoked gamma activity is dependent upon synaptic drive and is not generated only by the membrane properties of the chattering cells (Cardin et al., 2005). Gamma frequency oscillations measured in local field potentials can be evoked in vivo by optogenetic stimulation of PV positive neurons, suggesting that FS neurons may drive stimulus-evoked activity in the gamma band (Cardin et al., 2009). Photoactivation of these neurons to drive gamma oscillations during whisker deflection increases the precision of excitatory neuron responses and alters the amplitude and timing of responses in an oscillation phase dependent manner (Cardin et al., 2009).
Future work will be necessary to unravel how specific interneuron subtypes contribute to modulating sensory responses, fully understand the roles they play in higher order processing, and address whether there are specific conditions under which lateral inhibition sharpens receptive fields. One recent study in auditory cortex has begun to examine the cell type specific contributions of inhibition to modulating pyramidal cell activity during learning: VIP neurons were found to respond preferentially to reinforcement (Pi et al., 2013). SOM neurons were rapidly inhibited by VIP neurons, which caused an increase in firing of a subset of pyramidal neurons. This finding was interpreted to mean that activation of VIP neurons by reinforcement cues alters the gain of pyramidal neurons via disinhibition of SOM neurons (Pi et al., 2013). Together, the results presented in this section raise the intriguing possibility that the diversity in morphology and biophysical properties of GABAergic neurons is matched by their many functions.
Development of GABAergic Neurons and Synapses in Sensory Cortex
GABAergic neurons and synapses undergo a process of postnatal maturation which extends for quite some time after birth and co-occurs with the development of many functional properties of excitatory neurons (Espinosa and Stryker, 2012). Here, we will focus on the developmental changes that occur after inhibitory neurons have reached their target location within the cortical mantle and after GABAergic synaptic transmission has transitioned from excitatory to inhibitory. Exhaustive readings of earlier developmental processes can be found elsewhere (see Cherubini et al., 1991; Xu et al., 2003; Li and Xu, 2008; Gelman and Marín, 2010; Rudy et al., 2011; Ben-Ari et al., 2012; Ciceri et al., 2013; Taniguchi, 2014).
The intrinsic properties of inhibitory neurons change during postnatal development. In rodent V1, PV FS neurons show a progressive decrease in membrane time constant, cell capacitance and action potential width and an increase in intrinsic excitability during the first 2 weeks after eye opening (Goldberg et al., 2011; Lazarus and Huang, 2011). Conversely, SOM positive neurons show a progressive increase in membrane time constant, input resistance and spike frequency adaptation (Lazarus and Huang, 2011). The properties of inhibitory synapses also change during this developmental time window (Morales et al., 2002; Heinen et al., 2004; Maffei and Turrigiano, 2008a; Pinto et al., 2010). During the postnatal weeks following eye opening there is a progressive increase in a1 containing GABAA receptors and a progressive decrease in a3 and a5 containing GABAA receptors. Significant changes also occur in the expression of γ, δ and ε subunits (Heinen et al., 2004). Changes in receptor subunit composition underlie changes in conductance, clustering and susceptibility to allosteric modulators: all factors that play fundamental roles in determining the amplitude and time course of postsynaptic events (Bosman et al., 2002; Heinen et al., 2004; Möhler, 2006; Eyre et al., 2012). In mature brains GABAA receptors containing specific α subunits are primarily located in specific subcellular compartments: α1 and α3 containing receptors are enriched at synapses on the soma and perisomatic regions of pyramidal neurons (Klausberger et al., 2002), α2 containing receptors are preferentially located at axo-axonal synapses (Nusser et al., 1996), α5 containing receptors are preferentially located at dendritic synapses (Ali and Thomson, 2008), and α4 and α6 containing receptors predominantly mediate extrasynaptic inhibition (Wisden et al., 2002; Chandra et al., 2006; Wu et al., 2012). Whether GABAA receptor subunits are similarly localized during development is not known; however, the observed changes in levels of expression suggest that changes in the subcellular targeting of GABAergic synapses may occur.
Comparable processes of protracted maturation of inhibitory synapses have been reported in A1 and S1. In both regions there is a progressive increase in spontaneous IPSC amplitude (Kobayashi et al., 2008; Zhang et al., 2011; Takesian et al., 2012), and in A1 there are many developmentally regulated changes in inhibitory neuron intrinsic properties and in evoked synaptic strength (Takesian et al., 2010, 2012, 2013; Oswald and Reyes, 2011; Kinnischtzke et al., 2012).
Developmental changes in inhibitory circuit function go beyond subunit composition, strength and kinetic properties. Inhibitory drive tends to increase during postnatal development (Blue and Parnavelas, 1983; Morales et al., 2002; Chattopadhyaya et al., 2004; Maffei and Turrigiano, 2008a; Kuhlman et al., 2011; Li et al., 2012b). Changes in the strength of inhibitory inputs have been shown to decrease the ability of excitatory neurons to fire action potentials (Saraga et al., 2008; Pouille et al., 2013). Thus, developmental regulation of inhibitory synaptic strength may have significant effects on the activity of excitatory neurons. Precise patterns of neural activity are required for the induction of different forms of plasticity; therefore, changes in circuit activity may alter how stimuli drive changes at synapses. Patterns of activity or sensory experiences that facilitate the induction of long term potentiation (LTPi) or depression (LTDi) at inhibitory synapses could gate the induction of specific forms of plasticity at glutamatergic synapses in cortical circuits (Levelt and Hübener, 2012). Gating of excitatory plasticity could occur by altering activity patterns of glutamatergic neurons (Aton et al., 2013), by activating neuromodulatory signals (Huang et al., 2013) and/or through the activation of intracellular signaling cascades (Hayama et al., 2013; Wang and Maffei, 2014).
The capacity for plasticity at inhibitory synapses in V1 changes sharply during postnatal development at a time point corresponding to the transition between the pre-critical and the critical periods for visual cortical plasticity (Lefort et al., 2013). In response to the same pattern of activity, monosynaptic connections from FS neurons onto layer 4 pyramidal neurons show LTDi in the pre-critical period and LTPi in the critical period (Lefort et al., 2013). This shift in capacity for plasticity parallels that reported for layer 4 recurrent excitatory connections (Wang et al., 2012). Surprisingly, the switch in sign of plasticity at excitatory synapses can be reversed by inducing inhibitory plasticity in a connection specific fashion, indicating that inhibitory inputs may contribute to selective rewiring of local circuits despite their widespread connectivity (Wang and Maffei, 2014). This interaction between inhibitory and excitatory plasticity is mediated by G-protein signaling; therefore, it may affect the pyramidal neuron long after its induction (Wang and Maffei, 2014). Such a signaling crosstalk between mechanisms for excitatory and inhibitory plasticity could provide a memory trace that promotes experience-dependent rewiring of local microcircuits with a high degree of specificity and contributes to developmental circuit refinement.
During the developmental window in which inhibitory synapses mature, there are also substantial changes in glutamatergic receptor subunit composition, subcellular localization and function (Carmignoto and Vicini, 1992; Nase et al., 1999; Hensch, 2004; Corlew et al., 2007; Yashiro and Philpot, 2008), voltage signal propagation through the cortical circuit (Barkat et al., 2011; Griffen et al., 2013) and receptive field properties (Fagiolini et al., 1994; Katz and Shatz, 1996; Huang et al., 1999; Zhang et al., 2001; Inan and Crair, 2007; Wang et al., 2010; Espinosa and Stryker, 2012; Chen et al., 2014; Li et al., 2014a). Whether and how GABAergic neurotransmission contributes to these developmental processes is not fully understood. Evidence is beginning to emerge that changes in the receptive fields of the inhibitory drive onto excitatory neurons may at least partially underlie developmental receptive field sharpening (Dorrn et al., 2010; Sun et al., 2010; Li et al., 2012b). However, the underpinning synaptic and circuit mechanisms of this process remain elusive.
Sensory Deprivation Impairs the Development of GABAergic Inhibition
The maturation of inhibitory synapses is strongly influenced by sensory experience. Visual deprivation induced by raising animals in the dark from birth or shortly thereafter disrupts visual response properties (Benevento et al., 1992; Fagiolini et al., 1994; Gianfranceschi et al., 2003; but see Rochefort et al., 2011) and delays the formation of mature innervation onto excitatory neurons; whereas dark rearing started after eye opening does not affect inhibitory drive (Morales et al., 2002; Maffei et al., 2010). Dark rearing decreases the level of expression of GAD65 in layer 2/3 inhibitory neurons (Kreczko et al., 2009) and prevents postnatal increases in IPSC amplitude onto pyramidal neurons from occurring, consistent with a delay in GABAergic input maturation (Morales et al., 2002). Interestingly, dark rearing mice blocks the developmental broadening of the orientation tuning of PV neurons, but it does not fully prevent the developmental sharpening of the orientation tuning of pyramidal neurons in layers 2–4 (Kuhlman et al., 2011; Rochefort et al., 2011; Li et al., 2012b), or of the inhibitory inputs to layer 4 pyramidal neurons (Li et al., 2012b). Dark rearing also delays the maturational increase in the coincidence of binocular inputs to pyramidal neurons in layers 2–4 (Chen et al., 2014). Pharmacologically enhancing inhibition with benzodiazepines early in development disrupts binocular matching of orientation preference in complex cells (Wang et al., 2010, 2013a), widens the spacing of orientation columns and disrupts direction selectivity (Hensch and Stryker, 2004). Together with sensory deprivation experiments, these results suggest that the normal development of GABAergic inhibition is essential for the proper maturation of visual cortex.
Auditory experience also plays a major role in the development of inhibitory inputs in A1, as early hearing loss, either conductive or sensorineural, alters GABAergic neurotransmission (Kotak et al., 2005, 2008; Takesian et al., 2010, 2012). Early conductive hearing loss delays the developmental decrease in IPSC decay time constant (Takesian et al., 2012), and produces a significant, long lasting decrease in spontaneous IPSC amplitude, suggesting the induction of long term depression of inhibitory drive (Takesian et al., 2012). Auditory experience is not only necessary for the maturation of inhibition in A1, but also regulates the capacity for plasticity at inhibitory synapses (Xu et al., 2010) and the feedforward thalamocortical drive onto different classes of inhibitory neurons (Takesian et al., 2013).
While whisker plucking prevents the maturation of feedforward inhibition onto excitatory neurons in the barrel cortex (Chittajallu and Isaac, 2010), the mechanisms engaged in driving GABAergic synapse maturation in S1 are unknown. Across cortices, early sensory deprivation delays the maturation of inhibitory synapses, but how experience-dependent, developmentally regulated cellular and synaptic changes in inhibition directly influence sensory responses is unclear.
GABAergic Maturation and Critical Period Plasticity
Experiments with early sensory deprivation have shown that sensory experience is necessary for the maturation of inhibitory neurons and their synapses. However, if sensory manipulations are started later in postnatal development, for example during identified sensitive periods whose onset has been shown to correlate with the time course of the maturation of inhibition, sensory cortex is highly sensitive to even brief alterations in sensory drive (Hubel and Wiesel, 1970; Carmignoto and Vicini, 1992; Finnerty et al., 1999; Morales et al., 2002; Hensch, 2004; Maffei and Turrigiano, 2008a; Espinosa and Stryker, 2012; Levelt and Hübener, 2012). For example, monocular lid suture decreases the ratio of V1 neuronal spiking responses to the stimulation of the closed eye compared with the open eye, a phenomenon known as ocular dominance plasticity (Wiesel and Hubel, 1963). Ocular dominance plasticity is effectively induced by lid suture during a limited time window in postnatal development known as a critical period (Hubel and Wiesel, 1970; Fagiolini et al., 1994; Gordon and Stryker, 1996).
The maturation of inhibitory synapses has been proposed as a critical step for the modulation of the onset and duration of critical periods (Hensch et al., 1998). Manipulations of inhibition in vivo during early postnatal development shift the onset of the critical period for visual cortical plasticity in V1. In GAD65 knockout mice, which have low levels of GABA throughout life, the critical period never opens (Fagiolini and Hensch, 2000). Increasing intracortical inhibitory drive with benzodiazepines or zolpidem in early development, or at any time in GAD65 knockouts, leads to the onset of a window for ocular dominance plasticity (Fagiolini and Hensch, 2000; Fagiolini et al., 2004; Wang et al., 2013a; Chen et al., 2014). This effect is mediated by GABAA receptors containing α1 subunits, which are preferentially located at FS to pyramidal neuron somatic synapses (Fagiolini and Hensch, 2000; Klausberger et al., 2002; Iwai et al., 2003; Fagiolini et al., 2004; Katagiri et al., 2007). Either overexpressing BDNF or removing polysialic acid accelerates the development of inhibitory synapses and causes a precocious critical period (Hanover et al., 1999; Huang et al., 1999; Di Cristo et al., 2007). Similarly, sensory deprivation by dark rearing prolongs the onset of sensitivity to ocular dominance plasticity until the animal is introduced to light (Cynader and Mitchell, 1980; Mower and Christen, 1985; Mower, 1991; Fagiolini et al., 1994).
Conversely, pharmacologically decreasing inhibition in adult animals can reopen a window for ocular dominance plasticity (Harauzov et al., 2010; Maya-Vetencourt et al., 2012). This can be accomplished by reducing cortical GABA with GAD inhibitors, with the peptide hormone IGF-1, or by blocking GABAA receptors (Harauzov et al., 2010; Maya-Vetencourt et al., 2012). Environmental enrichment, which also decreases intracortical inhibition, can restore the capacity for ocular dominance plasticity in adult rodents in an IGF-1 dependent manner (Sale et al., 2007; Baroncelli et al., 2010; Maya-Vetencourt et al., 2012). Direct evidence that the age of inhibitory neurons, rather than overall inhibitory tone, plays a major role in critical period regulation comes from experiments where implants of GABAergic neural progenitors from embryonic mice were made into V1 of older mice. These implants later opened a window for ocular dominance plasticity that matched the age of the implanted neurons (Southwell et al., 2010). Substantial evidence exists that the maturation of inhibition regulates the timing of critical periods. Uniformly, manipulations that prevent that maturation of inhibition prevent the onset of critical periods, while those that enhance inhibition early in development cause a precocious critical period. Once inhibition has matured, reducing inhibition acutely can restore the capacity for ocular dominance plasticity. Manipulations that delay or accelerate the onset of the critical period for visual cortical plasticity, dark rearing and benzodiazepine application, also disrupt receptive field properties (see previous section), suggesting that inhibitory neurons play a fundamental role in the development of sensory cortex under normal conditions.
Sensory Alteration Induced Plasticity at Inhibitory Synapses
Alterations in sensory experience are not only dependent upon cortical inhibitory tone to induce plasticity; they also induce specific plastic changes at inhibitory synapses. Altering sensory drive engages inhibitory plasticity differently depending on the age of the animal, the nature of the change in sensory drive, and the specific synapses examined. For example, monocular lid suture induces rapid changes in inhibitory synaptic strength and drive, whether the lid is sutured before or after eye opening (Maffei et al., 2004, 2006, 2010; Wang et al., 2011; Kuhlman et al., 2013; Wang and Maffei, 2014). However, brief monocular lid suture before eye opening decreases drive from FS neurons onto pyramidal neurons in layer 4 of V1 (Maffei et al., 2004), whereas lid suture of the same duration started during the critical period potentiates FS to pyramidal neuron synapses in layer 4, both pre- and post-synaptically (Maffei et al., 2006; Nahmani and Turrigiano, 2014).
Removing a single row of whiskers during the first 2–3 postnatal weeks leads to an early potentiation of whisker responses in layer 2/3 of S1 after 3 days of deprivation followed by a loss of responses after 5–10 days (Drew and Feldman, 2009; Li et al., 2014a). Responses in layer 4 are not changed by this manipulation (Drew and Feldman, 2009; Li et al., 2014a). Different from lid suture, removing a single row of whiskers induces potentiation of FS to pyramidal neuron synapses in layer 2/3, where sensory plasticity is expressed (House et al., 2011).
In adult animals one of the main effects of sensory manipulation is the induction of structural changes in inhibitory neurons. A reduced number of dendritic spines has been reported in a subpopulation of inhibitory neurons following adult monocular retinal lesioning (Keck et al., 2011), suggesting that they may become less effectively activated by sensory stimuli. In addition, monocular lid suture in adult animals leads to a decrease in the number of inhibitory inputs onto layer 2/3 pyramidal neurons (van Versendaal et al., 2012), and the fraction of dynamic inhibitory synapses onto layer 2/3 pyramidal neuron spines and shafts increases (Chen et al., 2012). Thus, in adult V1 unilateral manipulation of visual drive induces a rapid restructuring of inhibitory inputs, possibly leading to an overall decreased inhibitory drive onto layer 2/3 pyramidal neurons.
While pharmacologically manipulating inhibition can change the potential for experience-dependent plasticity, global changes in circuit excitability may not be the ideal mechanism for fine-scale refinement of cortical circuit connectivity. Fine scale circuit refinement may require local, possibly synapse specific, regulation of inhibition. The age, layer and cell type specific changes in inhibitory synapses observed following monocular lid suture could provide highly specific modulation of inhibition. The layer 4 FS to pyramidal neuron synapses that are potentiated rapidly following monocular lid suture are sensitive to benzodiazepines, suggesting that they could play a role in critical period regulation (Maffei et al., 2006; Katagiri et al., 2007; Wang and Maffei, 2014). Induction of LTPi at these synapses, either through lid suture or acutely, switches the sign of plasticity induced at pyramidal neuron recurrent synapses by slow wave stimulation from long term potentiation of excitation (LTPe) to long term depression of excitation (LTDe) (Wang et al., 2012; Wang and Maffei, 2014). Acute depotentiation of the FS to pyramidal neuron synapses following lid suture restores the capacity for induction of LTPe, demonstrating that the control of inhibitory plasticity over the capacity for plasticity at excitatory synapses is modulated by experience (Wang and Maffei, 2014). While synapse specific, experience-dependent inhibitory control over excitatory plasticity has only been shown to exist in one microcircuit, this finding raises the intriguing possibility that plasticity at inhibitory synapses may control the expression of other forms of plasticity throughout cortex.
Experience-dependent reductions in inhibitory drive have been proposed to have a homeostatic role in contributing to the maintenance of neuronal activity in the face of altered sensory drive (Maffei et al., 2004; Maffei and Turrigiano, 2008b; Yang et al., 2011; Shao et al., 2013). Lid suture prior to the critical period decreases inhibitory synaptic strength onto excitatory neurons in layer 4 (Maffei et al., 2004). This homeostatic plasticity could be detected as a decrease in the spontaneous inhibitory drive and an increase in the spontaneous excitatory drive onto pyramidal neurons (Maffei et al., 2004). The induction of homeostatic inhibitory plasticity by monocular lid suture is developmentally regulated and shows layer specificity (Maffei et al., 2004; Maffei and Turrigiano, 2008b).
High decibel, high frequency sounds can lead to selective high frequency hearing loss, reorganization of the frequency map in A1 and tinnitus (Yang et al., 2011). Selective hearing loss leads to decreases in spontaneous inhibitory events and tonic inhibition thought to compensate for decreased sensory drive (Yang et al., 2011). Pharmacologically enhancing inhibition successfully eliminates tinnitus, while directly reducing excitation does not (Yang et al., 2011).
Whisker removal during a critical period for S1 plasticity reduces feedforward excitatory drive from layer 4 onto both layer 2/3 FS and pyramidal neurons, likely decreasing the activation of layer 2/3 by sensory input (House et al., 2011; Li et al., 2014a). This reduction in feedforward drive is compensated for by a robust decrease in the ability of layer 2/3 pyramidal neurons to recruit recurrent inhibition (Shao et al., 2013). These results coupled with the finding of FS to pyramidal neuron synaptic potentiation in layer 2/3 (House et al., 2011) suggest that a combination of homeostatic and Hebbian plasticity mechanisms contribute to rewiring the superficial layers of the barrel cortex.
Different forms of inhibitory synaptic plasticity can be engaged to refine or control local circuits. Despite widespread connectivity (Fino and Yuste, 2011; Packer and Yuste, 2011), the diversity of inhibitory neurons, and the selective sensitivity of distinct inhibitory synapses make them well suited to contribute to the maintenance of network excitability, large scale regulation of sensory cortical circuits and fine scale experience-dependent refinement of local microcircuits.
Inhibition and Changes in Responses Following Sensory Alterations
Understanding how changes in GABAergic neurons and their synapses contribute to changing sensory responses and maintaining homeostasis of firing rates in the face of altered sensory drive will be essential to pushing the field of experience-dependent plasticity forward. Recent work has shown that a brief unilateral reduction in visual drive strongly modulates the binocularity of FS inhibitory neurons. While FS inhibitory neurons are normally more binocular than pyramidal neurons (Swadlow, 1988; Yazaki-Sugiyama et al., 2009; Aton et al., 2013), soon after the onset of monocular lid suture or eye patching, layer 2/3 FS neurons are more strongly driven by the closed eye (Yazaki-Sugiyama et al., 2009; Aton et al., 2013; but see Kuhlman et al., 2013), although their spontaneous firing frequency is reduced (Yazaki-Sugiyama et al., 2009; Aton et al., 2013; Hengen et al., 2013). This increase in FS responsiveness to the deprived eye could contribute to reducing excitatory neuron responsiveness to that eye (Yazaki-Sugiyama et al., 2009). Interestingly, if the unilateral deprivation is maintained for several days both changes in ocular dominance of layer 2/3 FS neurons (Yazaki-Sugiyama et al., 2009; Aton et al., 2013; Kuhlman et al., 2013) and their spontaneous firing frequency are reversed (Aton et al., 2013; Hengen et al., 2013; but see Yazaki-Sugiyama et al., 2009). In layer 4 pyramidal neurons of mice, brief monocular lid suture similarly decreases both peak evoked excitatory and inhibitory conductances, and longer deprivation selectively reduces inhibition driven by the open eye (Ma et al., 2013). Differently, in rats longer monocular lid suture reduces excitation and inhibition driven by the deprived eye equally (Iurilli et al., 2013). Together, these results suggest that other mechanisms, such as changes in thalamocortical drive (Shatz and Stryker, 1978; LeVay et al., 1980; Khibnik et al., 2010; Wang et al., 2013b) may cooperate with altered intracortical inhibition to mediate the functional effects of sensory deprivation.
At first glance, studies showing that monocular lid suture does not affect the ratio of excitatory and inhibitory inputs to layer 4 pyramidal neurons (Iurilli et al., 2013; Ma et al., 2013) appear contradictory to the hypothesis that potentiation of inhibition directly suppresses visual responses (Maffei et al., 2006). However, many questions still exist. Visual stimuli used in these experiments were either moving bars or drifting gratings presented over seconds, or noise stimuli presented for several hundred milliseconds. Stimuli were presented only to one eye at a time. Additionally, only peak responses obtained during stimuli presented at peak orientation were examined (Iurilli et al., 2013; Ma et al., 2013). However, FS neurons in visual cortex have response properties that make them ideally suited to modulate extremely rapid visual responses to stimuli presented binocularly (Swadlow and Weyand, 1987; Swadlow, 1988; Yazaki-Sugiyama et al., 2009; Kameyama et al., 2010; Cardin, 2012; Aton et al., 2013; Zhuang et al., 2013). Additionally, their broad tuning raises the possibility that they could contribute to changes in orientation tuning or response suppression following lid suture. Alternatively, changes in patterns of FS neuron activity and synaptic strength could gate activity at other neurons' synapses (Levelt and Hübener, 2012; Wang and Maffei, 2014). Finally, recordings of somatic inhibitory conductances are unable to resolve differential contributions from interneuron subgroup: changes in input from one group may be offset by opposite changes in input from other groups. A recent study examining changes in excitatory and inhibitory conductances following whisker removal underscores the difficulty in interpreting results using this technique (Li et al., 2014a). Short and long durations of whisker trimming reduce evoked excitatory and inhibitory drive, but both decrease inhibition more than excitation. Interestingly, brief whisker trimming increases evoked spiking responses, while longer removal decreases responses (Li et al., 2014a). Thus, additional circuit mechanisms beyond evoked somatic excitatory and inhibitory balance must contribute to this change in responsiveness.
Two particularly exciting studies have shed light on possible roles of inhibitory plasticity in modulating sensory responses. In addition to reducing inhibition in layer 2/3, whisker trimming significantly reduces gamma oscillations in the circuit driven by the deprived whisker (Shao et al., 2013), suggesting that sensory changes in inhibitory drive may modulate network state. Whether reducing evoked gamma oscillations in vivo favors the induction of plasticity is not known. Like lid suture, long term monocular strabismus disrupts ocular dominance (Hubel and Wiesel, 1965), and strabismus leads to binocular suppression, whereby monocular stimuli elicit stronger responses than binocular stimuli (Sengpiel and Blakemore, 1994; Sengpiel et al., 1994). Strabismus was shown to induce binocular suppression through a selective increase in inhibitory drive during binocular stimulation (Scholl et al., 2013). These results suggest that changes in cortical inhibition induced by sensory experience may alter sensory processing in more subtle and complex ways than simply repressing unilateral deprived organ (whisker or eye) responses to optimal stimuli.
Changes in inhibitory drive are certainly not the sole mechanism at play in the experience-dependent rewiring of sensory cortex, as trophic factors, excitatory synapses, neuron intrinsic properties and extracellular matrix interactions have all been shown to contribute to the altered visual response properties and ocular dominance (Berardi et al., 2003; Levelt and Hübener, 2012). Determining how inhibitory neurons and their plasticity contribute to cortical function will require a more comprehensive understanding of how specific subclasses of inhibitory neurons contribute to sensory responses. Techniques that isolate the specific contribution of interneuron groups will need to be combined with detailed analyses of circuit and cellular properties. Many different mechanisms of GABAergic plasticity have been identified (see Castillo et al., 2011; Maffei, 2011). To manipulate these specific forms of inhibitory plasticity, it will be necessary to first identify the parameter space for their induction and expression. Specific mechanisms can then be manipulated to assess their roles in modulating neurons' response properties.
Conclusions
The maturation and plasticity of GABAergic neurons play fundamental roles in the development of sensory cortex. Inhibitory synaptic transmission and plasticity may affect critical period timing, specific receptive field properties, spike timing and other temporal aspects of coding, gain, neural synchrony, spontaneous and evoked firing rates, the capacity for plasticity at other synapses, and contribute to the homeostasis of circuit excitability. The role of all the distinct population of inhibitory neurons in the experience-dependent reorganization of cortical circuits has not been fully explored, but there is sufficient evidence to show that different inhibitory neuron types may be engaged to modulate different aspects of neuron response properties. The challenge ahead lies in identifying the distinct roles of inhibitory neurons in cortical circuits and in determining how their plasticity contributes to modulating other neurons' response properties, connectivity, and capacity for plasticity. Development of new tools and experimental approaches to selectively manipulate specific mechanisms of inhibitory plasticity will be instrumental for determining their role in sensory cortex development and function.
Conflict of Interest Statement
The authors declare that the research was conducted in the absence of any commercial or financial relationships that could be construed as a potential conflict of interest.
Acknowledgments
Support: NIH/R01 EY019885 to Arianna Maffei. Trevor C Griffen was supported by NIHGM MSTP Training award T32-GM008444. We thank Melissa Haley for insightful comments on the manuscript.
References
Adesnik, H., Bruns, W., Taniguchi, H., Huang, Z. J., and Scanziani, M. (2012). A neural circuit for spatial summation in visual cortex. Nature 490, 226–231. doi: 10.1038/nature11526
Adesnik, H., and Scanziani, M. (2010). Lateral competition for cortical space by layer-specific horizontal circuits. Nature 464, 1155–1160. doi: 10.1038/nature08935
Akgul, G., and Wollmuth, L. P. (2013). Synapse-associated protein 97 regulates the membrane properties of fast-spiking parvalbumin interneurons in the visual cortex. J. Neurosci. 33, 12739–12750. doi: 10.1523/JNEUROSCI.0040-13.2013
Ali, A. B., and Thomson, A. M. (2008). Synaptic alpha 5 subunit-containing GABAA receptors mediate IPSPs elicited by dendrite-preferring cells in rat neocortex. Cereb. Cortex 18, 1260–1271. doi: 10.1093/cercor/bhm160
Amitai, Y. (2001). Thalamocortical synaptic connections: efficacy, modulation, inhibition and plasticity. Rev. Neurosci. 12, 159–173. doi: 10.1515/REVNEURO.2001.12.2.159
Anderson, J. S., Carandini, M., and Ferster, D. (2000). Orientation tuning of input conductance, excitation, and inhibition in cat primary visual cortex. J. Neurophysiol. 84, 909–926.
Armstrong-James, M., Welker, E., and Callahan, C. A. (1993). The contribution of NMDA and non-NMDA receptors to fast and slow transmission of sensory information in the rat SI barrel cortex. J. Neurosci. 13, 2149–2160.
Ascoli, G. A., Alonso-Nanclares, L., Anderson, S. A., Barrionuevo, G., Benavides-Piccione, R., Burkhalter, A., et al. (2008). Petilla terminology: nomenclature of features of GABAergic interneurons of the cerebral cortex. Nat. Rev. Neurosci. 9, 557–568. doi: 10.1038/nrn2402
Atallah, B. V., Bruns, W., Carandini, M., and Scanziani, M. (2012). Parvalbumin-expressing interneurons linearly transform cortical responses to visual stimuli. Neuron 73, 159–170. doi: 10.1016/j.neuron.2011.12.013
Atencio, C. A., and Schreiner, C. E. (2008). Spectrotemporal processing differences between auditory cortical fast-spiking and regular-spiking neurons. J. Neurosci. 28, 3897–3910. doi: 10.1523/JNEUROSCI.5366-07.2008
Aton, S. J., Broussard, C., Dumoulin, M., Seibt, J., Watson, A., Coleman, T., et al. (2013). Visual experience and subsequent sleep induce sequential plastic changes in putative inhibitory and excitatory cortical neurons. Proc. Natl. Acad. Sci. U.S.A. 110, 3101–3106. doi: 10.1073/pnas.1208093110
Azouz, R., Gray, C. M., Nowak, L. G., and McCormick, D. A. (1997). Physiological properties of inhibitory interneurons in cat striate cortex. Cereb. Cortex 7, 534–545. doi: 10.1093/cercor/7.6.534
Barkat, T. R., Polley, D. B., and Hensch, T. K. (2011). A critical period for auditory thalamocortical connectivity. Nat. Neurosci. 14, 1189–1194. doi: 10.1038/nn.2882
Baroncelli, L., Sale, A., Viegi, A., Maya Vetencourt, J. F., De Pasquale, R., Baldini, S., et al. (2010). Experience-dependent reactivation of ocular dominance plasticity in the adult visual cortex. Exp. Neurol. 226, 100–109. doi: 10.1016/j.expneurol.2010.08.009
Beierlein, M., Gibson, J. R., and Connors, B. W. (2003). Two dynamically distinct inhibitory networks in layer 4 of the neocortex. J. Neurophysiol. 90, 2987–3000. doi: 10.1152/jn.00283.2003
Ben-Ari, Y., Khalilov, I., Kahle, K. T., and Cherubini, E. (2012). The GABA excitatory/inhibitory shift in brain maturation and neurological disorders. Neuroscientist 18, 467–486. doi: 10.1177/1073858412438697
Ben-Ari, Y., Khalilov, I., Represa, A., and Gozlan, H. (2004). Interneurons set the tune of developing networks. Trends Neurosci. 27, 422–427. doi: 10.1016/j.tins.2004.05.002
Benevento, L. A., Bakkum, B. W., Port, J. D., and Cohen, R. S. (1992). The effects of dark-rearing on the electrophysiology of the rat visual cortex. Brain Res. 572, 198–207. doi: 10.1016/0006-8993(92)90470-T
Benke, D., and Möhler, H. (2006). Benzodiazepine binding to GABA(A) receptors. Curr. Protoc. Pharmacol. Chapter 1: Unit 1.16. doi: 10.1002/0471141755.ph0116s35
Berardi, N., Pizzorusso, T., Ratto, G. M., and Maffei, L. (2003). Molecular basis of plasticity in the visual cortex. Trends Neurosci. 26, 369–378. doi: 10.1016/S0166-2236(03)00168-1
Bloodgood, B. L., Sharma, N., Browne, H. A., Trepman, A. Z., and Greenberg, M. E. (2013). The activity-dependent transcription factor NPAS4 regulates domain-specific inhibition. Nature 503, 121–125. doi: 10.1038/nature12743
Blue, M. E., and Parnavelas, J. G. (1983). The formation and maturation of synapses in the visual cortex of the rat. II. Quantitative analysis. J. Neurocytol. 12, 697–712. doi: 10.1007/BF01181531
Bonin, V., Histed, M. H., Yurgenson, S., and Reid, R. C. (2011). Local diversity and fine-scale organization of receptive fields in mouse visual cortex. J. Neurosci. 31, 18506–18521. doi: 10.1523/JNEUROSCI.2974-11.2011
Borg-Graham, L. J., Monier, C., and Frégnac, Y. (1998). Visual input evokes transient and strong shunting inhibition in visual cortical neurons. Nature 393, 369–373. doi: 10.1038/30735
Bosman, L. W., Rosahl, T. W., and Brussaard, A. B. (2002). Neonatal development of the rat visual cortex: synaptic function of GABAA receptor alpha subunits. J. Physiol. 545, 169–181. doi: 10.1113/jphysiol.2002.026534
Bruno, R. M., and Simons, D. J. (2002). Feedforward mechanisms of excitatory and inhibitory cortical receptive fields. J. Neurosci. 22, 10966–10975.
Cardin, J. A. (2012). Dissecting local circuits in vivo: integrated optogenetic and electrophysiology approaches for exploring inhibitory regulation of cortical activity. J. Physiol. Paris 106, 104–111. doi: 10.1016/j.jphysparis.2011.09.005
Cardin, J. A., Carlén, M., Meletis, K., Knoblich, U., Zhang, F., Deisseroth, K., et al. (2009). Driving fast-spiking cells induces gamma rhythm and controls sensory responses. Nature 459, 663–667. doi: 10.1038/nature08002
Cardin, J. A., Palmer, L. A., and Contreras, D. (2005). Stimulus-dependent gamma (30-50 Hz) oscillations in simple and complex fast rhythmic bursting cells in primary visual cortex. J. Neurosci. 25, 5339–5350. doi: 10.1523/JNEUROSCI.0374-05.2005
Cardin, J. A., Palmer, L. A., and Contreras, D. (2007). Stimulus feature selectivity in excitatory and inhibitory neurons in primary visual cortex. J. Neurosci. 27, 10333–10344. doi: 10.1523/JNEUROSCI.1692-07.2007
Carmignoto, G., and Vicini, S. (1992). Activity-dependent decrease in NMDA receptor responses during development of the visual cortex. Science 258, 1007–1011. doi: 10.1126/science.1279803
Caspary, D. M., Ling, L., Turner, J. G., and Hughes, L. F. (2008). Inhibitory neurotransmission, plasticity and aging in the mammalian central auditory system. J. Exp. Biol. 211, 1781–1791. doi: 10.1242/jeb.013581
Castillo, P. E., Chiu, C. Q., and Carroll, R. C. (2011). Long-term plasticity at inhibitory synapses. Curr. Opin. Neurobiol. 21, 328–338. doi: 10.1016/j.conb.2011.01.006
Cauli, B., Audinat, E., Lambolez, B., Angulo, M. C., Ropert, N., Tsuzuki, K., et al. (1997). Molecular and physiological diversity of cortical nonpyramidal cells. J. Neurosci. 17, 3894–3906.
Cauli, B., Porter, J. T., Tsuzuki, K., Lambolez, B., Rossier, J., Quenet, B., et al. (2000). Classification of fusiform neocortical interneurons based on unsupervised clustering. Proc. Natl. Acad. Sci. U.S.A. 97, 6144–6149. doi: 10.1073/pnas.97.11.6144
Chandra, D., Jia, F., Liang, J., Peng, Z., Suryanarayanan, A., Werner, D. F., et al. (2006). GABAA receptor alpha 4 subunits mediate extrasynaptic inhibition in thalamus and dentate gyrus and the action of gaboxadol. Proc. Natl. Acad. Sci. U.S.A. 103, 15230–15235. doi: 10.1073/pnas.0604304103
Chattopadhyaya, B., Di Cristo, G., Higashiyama, H., Knott, G. W., Kuhlman, S. J., Welker, E., et al. (2004). Experience and activity-dependent maturation of perisomatic GABAergic innervation in primary visual cortex during a postnatal critical period. J. Neurosci. 24, 9598–9611. doi: 10.1523/JNEUROSCI.1851-04.2004
Chen, J. L., Villa, K. L., Cha, J. W., So, P. T., Kubota, Y., and Nedivi, E. (2012). Clustered dynamics of inhibitory synapses and dendritic spines in the adult neocortex. Neuron 74, 361–373. doi: 10.1016/j.neuron.2012.02.030
Chen, X. J., Rasch, M. J., Chen, G., Ye, C. Q., Wu, S., and Zhang, X. H. (2014). Binocular input coincidence mediates critical period plasticity in the mouse primary visual cortex. J. Neurosci. 34, 2940–2955. doi: 10.1523/JNEUROSCI.2640-13.2014
Cherubini, E., Gaiarsa, J. L., and Ben-Ari, Y. (1991). GABA: an excitatory transmitter in early postnatal life. Trends Neurosci. 14, 515–519. doi: 10.1016/0166-2236(91)90003-D
Chittajallu, R., and Isaac, J. T. (2010). Emergence of cortical inhibition by coordinated sensory-driven plasticity at distinct synaptic loci. Nat. Neurosci. 13, 1240–1248. doi: 10.1038/nn.2639
Chittajallu, R., Pelkey, K. A., and Mcbain, C. J. (2013). Neurogliaform cells dynamically regulate somatosensory integration via synapse-specific modulation. Nat. Neurosci. 16, 13–15. doi: 10.1038/nn.3284
Ciceri, G., Dehorter, N., Sols, I., Huang, Z. J., Maravall, M., and Marín, O. (2013). Lineage-specific laminar organization of cortical GABAergic interneurons. Nat. Neurosci. 16, 1199–1210. doi: 10.1038/nn.3485
Corlew, R., Wang, Y., Ghermazien, H., Erisir, A., and Philpot, B. D. (2007). Developmental switch in the contribution of presynaptic and postsynaptic NMDA receptors to long-term depression. J. Neurosci. 27, 9835–9845. doi: 10.1523/JNEUROSCI.5494-06.2007
Cottam, J. C., Smith, S. L., and Häusser, M. (2013). Target-specific effects of somatostatin-expressing interneurons on neocortical visual processing. J. Neurosci. 33, 19567–19578. doi: 10.1523/JNEUROSCI.2624-13.2013
Crochet, S., Poulet, J. F., Kremer, Y., and Petersen, C. C. (2011). Synaptic mechanisms underlying sparse coding of active touch. Neuron 69, 1160–1175. doi: 10.1016/j.neuron.2011.02.022
Cruikshank, S. J., Lewis, T. J., and Connors, B. W. (2007). Synaptic basis for intense thalamocortical activation of feedforward inhibitory cells in neocortex. Nat. Neurosci. 10, 462–468.
Cynader, M., and Mitchell, D. E. (1980). Prolonged sensitivity to monocular deprivation in dark-reared cats. J. Neurophysiol. 43, 1026–1040.
DeFelipe, J., López-Cruz, P. L., Benavides-Piccione, R., Bielza, C., Larrañaga, P., Anderson, S., et al. (2013). New insights into the classification and nomenclature of cortical GABAergic interneurons. Nat. Rev. Neurosci. 14, 202–216. doi: 10.1038/nrn3444
de la Rocha, J., Marchetti, C., Schiff, M., and Reyes, A. D. (2008). Linking the response properties of cells in auditory cortex with network architecture: cotuning versus lateral inhibition. J. Neurosci. 28, 9151–9163. doi: 10.1523/JNEUROSCI.1789-08.2008
Di Cristo, G., Chattopadhyaya, B., Kuhlman, S. J., Fu, Y., Belanger, M. C., Wu, C. Z., et al. (2007). Activity-dependent PSA expression regulates inhibitory maturation and onset of critical period plasticity. Nat. Neurosci. 10, 1569–1577. doi: 10.1038/nn2008
Dorrn, A. L., Yuan, K., Barker, A. J., Schreiner, C. E., and Froemke, R. C. (2010). Developmental sensory experience balances cortical excitation and inhibition. Nature 465, 932–936. doi: 10.1038/nature09119
Drew, P. J., and Feldman, D. E. (2009). Intrinsic signal imaging of deprivation-induced contraction of whisker representations in rat somatosensory cortex. Cereb. Cortex 19, 331–348. doi: 10.1093/cercor/bhn085
Espinosa, J. S., and Stryker, M. P. (2012). Development and plasticity of the primary visual cortex. Neuron 75, 230–249. doi: 10.1016/j.neuron.2012.06.009
Eyre, M. D., Renzi, M., Farrant, M., and Nusser, Z. (2012). Setting the time course of inhibitory synaptic currents by mixing multiple GABA(A) receptor alpha subunit isoforms. J. Neurosci. 32, 5853–5867. doi: 10.1523/JNEUROSCI.6495-11.2012
Fagiolini, M., Fritschy, J. M., Löw, K., Möhler, H., Rudolph, U., and Hensch, T. K. (2004). Specific GABAA circuits for visual cortical plasticity. Science 303, 1681–1683. doi: 10.1126/science.1091032
Fagiolini, M., and Hensch, T. K. (2000). Inhibitory threshold for critical-period activation in primary visual cortex. Nature 404, 183–186. doi: 10.1038/35004582
Fagiolini, M., Pizzorusso, T., Berardi, N., Domenici, L., and Maffei, L. (1994). Functional postnatal development of the rat primary visual cortex and the role of visual experience: dark rearing and monocular deprivation. Vision Res. 34, 709–720. doi: 10.1016/0042-6989(94)90210-0
Felmy, F., and Schneggenburger, R. (2004). Developmental expression of the Ca2+-binding proteins calretinin and parvalbumin at the calyx of held of rats and mice. Eur. J. Neurosci. 20, 1473–1482. doi: 10.1111/j.1460-9568.2004.03604.x
Ferster, D. (1988). Spatially opponent excitation and inhibition in simple cells of the cat visual cortex. J. Neurosci. 8, 1172–1180.
Finnerty, G. T., Roberts, L. S., and Connors, B. W. (1999). Sensory experience modifies the short-term dynamics of neocortical synapses. Nature 400, 367–371. doi: 10.1038/22553
Fino, E., Packer, A. M., and Yuste, R. (2013). The logic of inhibitory connectivity in the neocortex. Neuroscientist 19, 228–237. doi: 10.1177/1073858412456743
Fino, E., and Yuste, R. (2011). Dense inhibitory connectivity in neocortex. Neuron 69, 1188–1203. doi: 10.1016/j.neuron.2011.02.025
Foeller, E., Celikel, T., and Feldman, D. E. (2005). Inhibitory sharpening of receptive fields contributes to whisker map plasticity in rat somatosensory cortex. J. Neurophysiol. 94, 4387–4400. doi: 10.1152/jn.00553.2005
Földy, C., Aradi, I., Howard, A., and Soltesz, I. (2004). Diversity beyond variance: modulation of firing rates and network coherence by GABAergic subpopulations. Eur. J. Neurosci. 19, 119–130. doi: 10.1046/j.1460-9568.2003.03096.x
Freund, T. F., and Katona, I. (2007). Perisomatic inhibition. Neuron 56, 33–42. doi: 10.1016/j.neuron.2007.09.012
Froemke, R. C., Merzenich, M. M., and Schreiner, C. E. (2007). A synaptic memory trace for cortical receptive field plasticity. Nature 450, 425–429. doi: 10.1038/nature06289
Gabernet, L., Jadhav, S. P., Feldman, D. E., Carandini, M., and Scanziani, M. (2005). Somatosensory integration controlled by dynamic thalamocortical feed-forward inhibition. Neuron 48, 315–327. doi: 10.1016/j.neuron.2005.09.022
Galarreta, M., and Hestrin, S. (1999). A network of fast-spiking cells in the neocortex connected by electrical synapses. Nature 402, 72–75. doi: 10.1038/47029
Galarreta, M., and Hestrin, S. (2002). Electrical and chemical synapses among parvalbumin fast-spiking GABAergic interneurons in adult mouse neocortex. Proc. Natl. Acad. Sci. U.S.A. 99, 12438–12443. doi: 10.1073/pnas.192159599
Gandhi, S. P., Yanagawa, Y., and Stryker, M. P. (2008). Delayed plasticity of inhibitory neurons in developing visual cortex. Proc. Natl. Acad. Sci. U.S.A. 105, 16797–16802. doi: 10.1073/pnas.0806159105
Gelman, D. M., and Marín, O. (2010). Generation of interneuron diversity in the mouse cerebral cortex. Eur. J. Neurosci. 31, 2136–2141. doi: 10.1111/j.1460-9568.2010.07267.x
Gentet, L. J., Avermann, M., Matyas, F., Staiger, J. F., and Petersen, C. C. (2010). Membrane potential dynamics of GABAergic neurons in the barrel cortex of behaving mice. Neuron 65, 422–435. doi: 10.1016/j.neuron.2010.01.006
Gentet, L. J., Kremer, Y., Taniguchi, H., Huang, Z. J., Staiger, J. F., and Petersen, C. C. (2012). Unique functional properties of somatostatin-expressing GABAergic neurons in mouse barrel cortex. Nat. Neurosci. 15, 607–612. doi: 10.1038/nn.3051
Germroth, P., Schwerdtfeger, W. K., and Buhl, E. H. (1989). GABAergic neurons in the entorhinal cortex project to the hippocampus. Brain Res. 494, 187–192. doi: 10.1016/0006-8993(89)90162-5
Gianfranceschi, L., Siciliano, R., Walls, J., Morales, B., Kirkwood, A., Huang, Z. J., et al. (2003). Visual cortex is rescued from the effects of dark rearing by overexpression of BDNF. Proc. Natl. Acad. Sci. U.S.A. 100, 12486–12491. doi: 10.1073/pnas.1934836100
Gibson, J. R., Beierlein, M., and Connors, B. W. (1999). Two networks of electrically coupled inhibitory neurons in neocortex. Nature 402, 75–79. doi: 10.1038/47035
Gidon, A., and Segev, I. (2012). Principles governing the operation of synaptic inhibition in dendrites. Neuron 75, 330–341. doi: 10.1016/j.neuron.2012.05.015
Gielen, M. C., Lumb, M. J., and Smart, T. G. (2012). Benzodiazepines modulate GABAA receptors by regulating the preactivation step after GABA binding. J. Neurosci. 32, 5707–5715. doi: 10.1523/JNEUROSCI.5663-11.2012
Gilbert, C. D. (1977). Laminar differences in receptive field properties of cells in cat primary visual cortex. J. Physiol. 268, 391–421.
Gilbert, C. D., and Wiesel, T. N. (1979). Morphology and intracortical projections of functionally characterised neurones in the cat visual cortex. Nature 280, 120–125. doi: 10.1038/280120a0
Girman, S. V., Sauvé, Y., and Lund, R. D. (1999). Receptive field properties of single neurons in rat primary visual cortex. J. Neurophysiol. 82, 301–311.
Gold, C., Henze, D. A., Koch, C., and Buzsáki, G. (2006). On the origin of the extracellular action potential waveform: a modeling study. J. Neurophysiol. 95, 3113–3128. doi: 10.1152/jn.00979.2005
Goldberg, E. M., Jeong, H. Y., Kruglikov, I., Tremblay, R., Lazarenko, R. M., and Rudy, B. (2011). Rapid developmental maturation of neocortical FS cell intrinsic excitability. Cereb. Cortex 21, 666–682. doi: 10.1093/cercor/bhq138
Gomes da Silva, S., Doná, F., da Silva Fernandes, M. J., Scorza, F. A., Cavalheiro, E. A., and Arida, R. M. (2010). Physical exercise during the adolescent period of life increases hippocampal parvalbumin expression. Brain Dev. 32, 137–142. doi: 10.1016/j.braindev.2008.12.012
Gonchar, Y., Turney, S., Price, J. L., and Burkhalter, A. (2002). Axo-axonic synapses formed by somatostatin-expressing GABAergic neurons in rat and monkey visual cortex. J. Comp. Neurol. 443, 1–14. doi: 10.1002/cne.1425
González-Burgos, G., Krimer, L. S., Povysheva, N. V., Barrionuevo, G., and Lewis, D. A. (2005). Functional properties of fast spiking interneurons and their synaptic connections with pyramidal cells in primate dorsolateral prefrontal cortex. J. Neurophysiol. 93, 942–953. doi: 10.1152/jn.00787.2004
Gordon, J. A., and Stryker, M. P. (1996). Experience-dependent plasticity of binocular responses in the primary visual cortex of the mouse. J. Neurosci. 16, 3274–3286.
Graupner, M., and Reyes, A. D. (2013). Synaptic input correlations leading to membrane potential decorrelation of spontaneous activity in cortex. J. Neurosci. 33, 15075–15085. doi: 10.1523/JNEUROSCI.0347-13.2013
Gray, C. M., and McCormick, D. A. (1996). Chattering cells: superficial pyramidal neurons contributing to the generation of synchronous oscillations in the visual cortex. Science 274, 109–113. doi: 10.1126/science.274.5284.109
Griffen, T. C., Wang, L., Fontanini, A., and Maffei, A. (2013). Developmental regulation of spatio-temporal patterns of cortical circuit activation. Front. Cell. Neurosci. 6:65. doi: 10.3389/fncel.2012.00065
Grinvald, A., Lieke, E., Frostig, R. D., Gilbert, C. D., and Wiesel, T. N. (1986). Functional architecture of cortex revealed by optical imaging of intrinsic signals. Nature 324, 361–364. doi: 10.1038/324361a0
Hagihara, K. M., and Ohki, K. (2013). Long-term down-regulation of GABA decreases orientation selectivity without affecting direction selectivity in mouse primary visual cortex. Front. Neural Circuits 7:28. doi: 10.3389/fncir.2013.00028
Haider, B., Duque, A., Hasenstaub, A. R., Yu, Y., and McCormick, D. A. (2007). Enhancement of visual responsiveness by spontaneous local network activity in vivo. J. Neurophysiol. 97, 4186–4202. doi: 10.1152/jn.01114.2006
Haider, B., Hausser, M., and Carandini, M. (2013). Inhibition dominates sensory responses in the awake cortex. Nature 493, 97–100. doi: 10.1038/nature11665
Haider, B., Krause, M. R., Duque, A., Yu, Y., Touryan, J., Mazer, J. A., et al. (2010). Synaptic and network mechanisms of sparse and reliable visual cortical activity during nonclassical receptive field stimulation. Neuron 65, 107–121. doi: 10.1016/j.neuron.2009.12.005
Haider, B., and McCormick, D. A. (2009). Rapid neocortical dynamics: cellular and network mechanisms. Neuron 62, 171–189. doi: 10.1016/j.neuron.2009.04.008
Hanover, J. L., Huang, Z. J., Tonegawa, S., and Stryker, M. P. (1999). Brain-derived neurotrophic factor overexpression induces precocious critical period in mouse visual cortex. J Neurosci 19:RC40.
Harauzov, A., Spolidoro, M., DiCristo, G., De Pasquale, R., Cancedda, L., Pizzorusso, T., et al. (2010). Reducing intracortical inhibition in the adult visual cortex promotes ocular dominance plasticity. J. Neurosci. 30, 361–371. doi: 10.1523/JNEUROSCI.2233-09.2010
Hayama, T., Noguchi, J., Watanabe, S., Takahashi, N., Hayashi-Takagi, A., Ellis-Davies, G. C., et al. (2013). GABA promotes the competitive selection of dendritic spines by controlling local Ca2+ signaling. Nat. Neurosci. 16, 1409–1416. doi: 10.1038/nn.3496
Heimel, J. A., van Versendaal, D., and Levelt, C. N. (2011). The role of GABAergic inhibition in ocular dominance plasticity. Neural Plast. 2011:391763. doi: 10.1155/2011/391763
Heinen, K., Bosman, L. W., Spijker, S., van Pelt, J., Smit, A. B., Voorn, P., et al. (2004). GABAA receptor maturation in relation to eye opening in the rat visual cortex. Neuroscience 124, 161–171. doi: 10.1016/j.neuroscience.2003.11.004
Helm, J., Akgul, G., and Wollmuth, L. P. (2013). Subgroups of parvalbumin-expressing interneurons in layers 2/3 of the visual cortex. J. Neurophysiol. 109, 1600–1613. doi: 10.1152/jn.00782.2012
Hengen, K. B., Lambo, M. E., Van Hooser, S. D., Katz, D. B., and Turrigiano, G. G. (2013). Firing rate homeostasis in visual cortex of freely behaving rodents. Neuron 80, 335–342. doi: 10.1016/j.neuron.2013.08.038
Hensch, T. K. (2004). Critical period regulation. Annu. Rev. Neurosci. 27, 549–579. doi: 10.1146/annurev.neuro.27.070203.144327
Hensch, T. K., Fagiolini, M., Mataga, N., Stryker, M. P., Baekkeskov, S., and Kash, S. F. (1998). Local GABA circuit control of experience-dependent plasticity in developing visual cortex. Science 282, 1504–1508. doi: 10.1126/science.282.5393.1504
Hensch, T. K., and Stryker, M. P. (2004). Columnar architecture sculpted by GABA circuits in developing cat visual cortex. Science 303, 1678–1681. doi: 10.1126/science.1091031
Henze, D. A., Borhegyi, Z., Csicsvari, J., Mamiya, A., Harris, K. D., and Buzsáki, G. (2000). Intracellular features predicted by extracellular recordings in the hippocampus in vivo. J. Neurophysiol. 84, 390–400.
Hestrin, S., and Galarreta, M. (2005). Electrical synapses define networks of neocortical GABAergic neurons. Trends Neurosci. 28, 304–309. doi: 10.1016/j.tins.2005.04.001
Higley, M. J., and Contreras, D. (2006). Balanced excitation and inhibition determine spike timing during frequency adaptation. J. Neurosci. 26, 448–457. doi: 10.1523/JNEUROSCI.3506-05.2006
Hirsch, J. A., Alonso, J. M., Reid, R. C., and Martinez, L. M. (1998). Synaptic integration in striate cortical simple cells. J. Neurosci. 18, 9517–9528.
Hirsch, J. A., Martinez, L. M., Pillai, C., Alonso, J. M., Wang, Q., and Sommer, F. T. (2003). Functionally distinct inhibitory neurons at the first stage of visual cortical processing. Nat. Neurosci. 6, 1300–1308. doi: 10.1038/nn1152
Hou, Z. H., and Yu, X. (2013). Activity-regulated somatostatin expression reduces dendritic spine density and lowers excitatory synaptic transmission via postsynaptic somatostatin receptor 4. J. Biol. Chem. 288, 2501–2509. doi: 10.1074/jbc.M112.419051
House, D. R., Elstrott, J., Koh, E., Chung, J., and Feldman, D. E. (2011). Parallel regulation of feedforward inhibition and excitation during whisker map plasticity. Neuron 72, 819–831. doi: 10.1016/j.neuron.2011.09.008
Hu, H., Cavendish, J. Z., and Agmon, A. (2013). Not all that glitters is gold: off-target recombination in the somatostatin-IRES-Cre mouse line labels a subset of fast-spiking interneurons. Front. Neural Circuits 7:195. doi: 10.3389/fncir.2013.00195
Huang, S., Huganir, R. L., and Kirkwood, A. (2013). Adrenergic gating of Hebbian spike-timing-dependent plasticity in cortical interneurons. J. Neurosci. 33, 13171–13178. doi: 10.1523/JNEUROSCI.5741-12.2013
Huang, Z. J., Kirkwood, A., Pizzorusso, T., Porciatti, V., Morales, B., Bear, M. F., et al. (1999). BDNF regulates the maturation of inhibition and the critical period of plasticity in mouse visual cortex. Cell 98, 739–755. doi: 10.1016/S0092-8674(00)81509-3
Hubel, D. H., and Wiesel, T. N. (1962). Receptive fields, binocular interaction and functional architecture in the cat's visual cortex. J. Physiol. 160, 106–154.
Hubel, D. H., and Wiesel, T. N. (1963). Shape and arrangement of columns in cat's striate cortex. J. Physiol. 165, 559–568.
Hubel, D. H., and Wiesel, T. N. (1965). Binocular interaction in striate cortex of kittens reared with artificial squint. J. Neurophysiol. 28, 1041–1059.
Hubel, D. H., and Wiesel, T. N. (1970). The period of susceptibility to the physiological effects of unilateral eye closure in kittens. J. Physiol. 206, 419–436.
Inan, M., and Crair, M. C. (2007). Development of cortical maps: perspectives from the barrel cortex. Neuroscientist 13, 49–61. doi: 10.1177/1073858406296257
Isaacson, J. S., and Scanziani, M. (2011). How inhibition shapes cortical activity. Neuron 72, 231–243. doi: 10.1016/j.neuron.2011.09.027
Iurilli, G., Ghezzi, D., Olcese, U., Lassi, G., Nazzaro, C., Tonini, R., et al. (2012). Sound-driven synaptic inhibition in primary visual cortex. Neuron 73, 814–828. doi: 10.1016/j.neuron.2011.12.026
Iurilli, G., Olcese, U., and Medini, P. (2013). Preserved excitatory-inhibitory balance of cortical synaptic inputs following deprived eye stimulation after a saturating period of monocular deprivation in rats. PLoS ONE 8:e82044. doi: 10.1371/journal.pone.0082044
Iwai, Y., Fagiolini, M., Obata, K., and Hensch, T. K. (2003). Rapid critical period induction by tonic inhibition in visual cortex. J. Neurosci. 23, 6695–6702.
Jeong, J. K., Tremere, L. A., Ryave, M. J., Vuong, V. C., and Pinaud, R. (2009). Anatomical and functional organization of inhibitory circuits in the songbird auditory forebrain. J. Exp. Neurosci. 2, 43–53.
Kameyama, K., Sohya, K., Ebina, T., Fukuda, A., Yanagawa, Y., and Tsumoto, T. (2010). Difference in binocularity and ocular dominance plasticity between GABAergic and excitatory cortical neurons. J. Neurosci. 30, 1551–1559. doi: 10.1523/JNEUROSCI.5025-09.2010
Katagiri, H., Fagiolini, M., and Hensch, T. K. (2007). Optimization of somatic inhibition at critical period onset in mouse visual cortex. Neuron 53, 805–812. doi: 10.1016/j.neuron.2007.02.026
Katz, L. C., and Shatz, C. J. (1996). Synaptic activity and the construction of cortical circuits. Science 274, 1133–1138. doi: 10.1126/science.274.5290.1133
Katzner, S., Busse, L., and Carandini, M. (2011). GABAA inhibition controls response gain in visual cortex. J. Neurosci. 31, 5931–5941. doi: 10.1523/JNEUROSCI.5753-10.2011
Kawaguchi, Y., and Kubota, Y. (1993). Correlation of physiological subgroupings of nonpyramidal cells with parvalbumin- and calbindinD28k-immunoreactive neurons in layer V of rat frontal cortex. J. Neurophysiol. 70, 387–396.
Kawaguchi, Y., and Kubota, Y. (1996). Physiological and morphological identification of somatostatin- or vasoactive intestinal polypeptide-containing cells among GABAergic cell subtypes in rat frontal cortex. J. Neurosci. 16, 2701–2715.
Kawaguchi, Y., and Kubota, Y. (1997). GABAergic cell subtypes and their synaptic connections in rat frontal cortex. Cereb. Cortex 7, 476–486. doi: 10.1093/cercor/7.6.476
Keck, T., Scheuss, V., Jacobsen, R. I., Wierenga, C. J., Eysel, U. T., Bonhoeffer, T., et al. (2011). Loss of sensory input causes rapid structural changes of inhibitory neurons in adult mouse visual cortex. Neuron 71, 869–882. doi: 10.1016/j.neuron.2011.06.034
Kelly, J. P., and Van Essen, D. C. (1974). Cell structure and function in the visual cortex of the cat. J. Physiol. 238, 515–547.
Kerlin, A. M., Andermann, M. L., Berezovskii, V. K., and Reid, R. C. (2010). Broadly tuned response properties of diverse inhibitory neuron subtypes in mouse visual cortex. Neuron 67, 858–871. doi: 10.1016/j.neuron.2010.08.002
Khibnik, L. A., Cho, K. K., and Bear, M. F. (2010). Relative contribution of feedforward excitatory connections to expression of ocular dominance plasticity in layer 4 of visual cortex. Neuron 66, 493–500. doi: 10.1016/j.neuron.2010.04.012
Kinnischtzke, A. K., Sewall, A. M., Berkepile, J. M., and Fanselow, E. E. (2012). Postnatal maturation of somatostatin-expressing inhibitory cells in the somatosensory cortex of GIN mice. Front. Neural Circuits 6:33. doi: 10.3389/fncir.2012.00033
Klausberger, T., Roberts, J. D., and Somogyi, P. (2002). Cell type- and input-specific differences in the number and subtypes of synaptic GABA(A) receptors in the hippocampus. J. Neurosci. 22, 2513–2521.
Kobayashi, M., Hamada, T., Kogo, M., Yanagawa, Y., Obata, K., and Kang, Y. (2008). Developmental profile of GABAA-mediated synaptic transmission in pyramidal cells of the somatosensory cortex. Eur. J. Neurosci. 28, 849–861. doi: 10.1111/j.1460-9568.2008.06401.x
Kotak, V. C., Fujisawa, S., Lee, F. A., Karthikeyan, O., Aoki, C., and Sanes, D. H. (2005). Hearing loss raises excitability in the auditory cortex. J. Neurosci. 25, 3908–3918. doi: 10.1523/JNEUROSCI.5169-04.2005
Kotak, V. C., Takesian, A. E., and Sanes, D. H. (2008). Hearing loss prevents the maturation of GABAergic transmission in the auditory cortex. Cereb. Cortex 18, 2098–2108. doi: 10.1093/cercor/bhm233
Kreczko, A., Goel, A., Song, L., and Lee, H. K. (2009). Visual deprivation decreases somatic GAD65 puncta number on layer 2/3 pyramidal neurons in mouse visual cortex. Neural Plast. 2009:415135. doi: 10.1155/2009/415135
Kuhlman, S. J., Olivas, N. D., Tring, E., Ikrar, T., Xu, X., and Trachtenberg, J. T. (2013). A disinhibitory microcircuit initiates critical-period plasticity in the visual cortex. Nature 501, 543–546. doi: 10.1038/nature12485
Kuhlman, S. J., Tring, E., and Trachtenberg, J. T. (2011). Fast-spiking interneurons have an initial orientation bias that is lost with vision. Nat. Neurosci. 14, 1121–1123. doi: 10.1038/nn.2890
Kwegyir-Afful, E. E., Kyriazi, H. T., and Simons, D. J. (2013). Weaker feedforward inhibition accounts for less pronounced thalamocortical response transformation in mouse vs. rat barrels. J. Neurophysiol. 110, 2378–2392. doi: 10.1152/jn.00574.2012
Kyriazi, H. T., Carvell, G. E., Brumberg, J. C., and Simons, D. J. (1996). Quantitative effects of GABA and bicuculline methiodide on receptive field properties of neurons in real and simulated whisker barrels. J. Neurophysiol. 75, 547–560.
Lampl, I., Reichova, I., and Ferster, D. (1999). Synchronous membrane potential fluctuations in neurons of the cat visual cortex. Neuron 22, 361–374. doi: 10.1016/S0896-6273(00)81096-X
Lazarus, M. S., and Huang, Z. J. (2011). Distinct maturation profiles of perisomatic and dendritic targeting GABAergic interneurons in the mouse primary visual cortex during the critical period of ocular dominance plasticity. J. Neurophysiol. 106, 775–787. doi: 10.1152/jn.00729.2010
Lee, S., Hjerling-Leffler, J., Zagha, E., Fishell, G., and Rudy, B. (2010). The largest group of superficial neocortical GABAergic interneurons expresses ionotropic serotonin receptors. J. Neurosci. 30, 16796–16808. doi: 10.1523/JNEUROSCI.1869-10.2010
Lee, S. H., Kwan, A. C., Zhang, S., Phoumthipphavong, V., Flannery, J. G., Masmanidis, S. C., et al. (2012). Activation of specific interneurons improves V1 feature selectivity and visual perception. Nature 488, 379–383. doi: 10.1038/nature11312
Lee, S., Kruglikov, I., Huang, Z. J., Fishell, G., and Rudy, B. (2013). A disinhibitory circuit mediates motor integration in the somatosensory cortex. Nat. Neurosci. 16, 1662–1670. doi: 10.1038/nn.3544
Lefort, S., Gray, A. C., and Turrigiano, G. G. (2013). Long-term inhibitory plasticity in visual cortical layer 4 switches sign at the opening of the critical period. Proc. Natl. Acad. Sci. U.S.A. 110, E4540–E4547. doi: 10.1073/pnas.1319571110
Le Magueresse, C., and Monyer, H. (2013). GABAergic interneurons shape the functional maturation of the cortex. Neuron 77, 388–405. doi: 10.1016/j.neuron.2013.01.011
Letzkus, J. J., Wolff, S. B., Meyer, E. M., Tovote, P., Courtin, J., Herry, C., et al. (2011). A disinhibitory microcircuit for associative fear learning in the auditory cortex. Nature 480, 331–335. doi: 10.1038/nature10674
LeVay, S., Wiesel, T. N., and Hubel, D. H. (1980). The development of ocular dominance columns in normal and visually deprived monkeys. J. Comp. Neurol. 191, 1–51. doi: 10.1002/cne.901910102
Levelt, C. N., and Hübener, M. (2012). Critical-period plasticity in the visual cortex. Annu. Rev. Neurosci. 35, 309–330. doi: 10.1146/annurev-neuro-061010-113813
Levy, R. B., and Reyes, A. D. (2011). Coexistence of lateral and co-tuned inhibitory configurations in cortical networks. PLoS Comput. Biol. 7:e1002161. doi: 10.1371/journal.pcbi.1002161
Li, C. X., Callaway, J. C., and Waters, R. S. (2002). Removal of GABAergic inhibition alters subthreshold input in neurons in forepaw barrel subfield (FBS) in rat first somatosensory cortex (SI) after digit stimulation. Exp. Brain Res. 145, 411–428. doi: 10.1007/s00221-002-1124-7
Li, K., and Xu, E. (2008). The role and the mechanism of gamma-aminobutyric acid during central nervous system development. Neurosci. Bull. 24, 195–200. doi: 10.1007/s12264-008-0109-3
Li, L., Gainey, M. A., Goldbeck, J. E., and Feldman, D. E. (2014a). Rapid homeostasis by disinhibition during whisker map plasticity. Proc. Natl. Acad. Sci. U.S.A. 111, 1616–1621. doi: 10.1073/pnas.1312455111
Li, L. Y., Xiong, X. R., Ibrahim, L. A., Yuan, W., Tao, H. W., and Zhang, L. I. (2014b). Differential receptive field properties of parvalbumin and somatostain inhibitory neurons in mouse auditory cortex. Cereb. Cortex. doi: 10.1093/cercor/bht417. [Epub ahead of print].
Li, Y. T., Ma, W. P., Li, L. Y., Ibrahim, L. A., Wang, S. Z., and Tao, H. W. (2012a). Broadening of inhibitory tuning underlies contrast-dependent sharpening of orientation selectivity in mouse visual cortex. J. Neurosci. 32, 16466–16477. doi: 10.1523/JNEUROSCI.3221-12.2012
Li, Y. T., Ma, W. P., Pan, C. J., Zhang, L. I., and Tao, H. W. (2012b). Broadening of cortical inhibition mediates developmental sharpening of orientation selectivity. J. Neurosci. 32, 3981–3991. doi: 10.1523/JNEUROSCI.5514-11.2012
Lin, C. S., Friedlander, M. J., and Sherman, S. M. (1979). Morphology of physiologically identified neurons in the visual cortex of the cat. Brain Res. 172, 344–348. doi: 10.1016/0006-8993(79)90544-4
Liu, B. H., Li, P., Li, Y. T., Sun, Y. J., Yanagawa, Y., Obata, K., et al. (2009). Visual receptive field structure of cortical inhibitory neurons revealed by two-photon imaging guided recording. J. Neurosci. 29, 10520–10532. doi: 10.1523/JNEUROSCI.1915-09.2009
Liu, B. H., Li, P., Sun, Y. J., Li, Y. T., Zhang, L. I., and Tao, H. W. (2010). Intervening inhibition underlies simple-cell receptive field structure in visual cortex. Nat. Neurosci. 13, 89–96. doi: 10.1038/nn.2443
Liu, B. H., Li, Y. T., Ma, W. P., Pan, C. J., Zhang, L. I., and Tao, H. W. (2011). Broad inhibition sharpens orientation selectivity by expanding input dynamic range in mouse simple cells. Neuron 71, 542–554. doi: 10.1016/j.neuron.2011.06.017
Lorén, I., Emson, P. C., Fahrenkrug, J., Björklund, A., Alumets, J., Håkanson, R., et al. (1979). Distribution of vasoactive intestinal polypeptide in the rat and mouse brain. Neuroscience 4, 1953–1976. doi: 10.1016/0306-4522(79)90068-X
Ma, W. P., Liu, B. H., Li, Y. T., Huang, Z. J., Zhang, L. I., and Tao, H. W. (2010). Visual representations by cortical somatostatin inhibitory neurons—selective but with weak and delayed responses. J. Neurosci. 30, 14371–14379. doi: 10.1523/JNEUROSCI.3248-10.2010
Ma, W. P., Li, Y. T., and Tao, H. W. (2013). Downregulation of cortical inhibition mediates ocular dominance plasticity during the critical period. J. Neurosci. 33, 11276–11280. doi: 10.1523/JNEUROSCI.5598-12.2013
Ma, Y., Hu, H., Berrebi, A. S., Mathers, P. H., and Agmon, A. (2006). Distinct subtypes of somatostatin-containing neocortical interneurons revealed in transgenic mice. J. Neurosci. 26, 5069–5082. doi: 10.1523/JNEUROSCI.0661-06.2006
Maffei, A. (2011). The many forms and functions of long term plasticity at GABAergic synapses. Neural Plast. 2011:254724. doi: 10.1155/2011/254724
Maffei, A., Lambo, M. E., and Turrigiano, G. G. (2010). Critical period for inhibitory plasticity in rodent binocular V1. J. Neurosci. 30, 3304–3309. doi: 10.1523/JNEUROSCI.5340-09.2010
Maffei, A., Nataraj, K., Nelson, S. B., and Turrigiano, G. G. (2006). Potentiation of cortical inhibition by visual deprivation. Nature 443, 81–84. doi: 10.1038/nature05079
Maffei, A., Nelson, S. B., and Turrigiano, G. G. (2004). Selective reconfiguration of layer 4 visual cortical circuitry by visual deprivation. Nat. Neurosci. 7, 1353–1359. doi: 10.1038/nn1351
Maffei, A., and Turrigiano, G. (2008a). The age of plasticity: developmental regulation of synaptic plasticity in neocortical microcircuits. Prog. Brain Res. 169, 211–223. doi: 10.1016/S0079-6123(07)00012-X
Maffei, A., and Turrigiano, G. G. (2008b). Multiple modes of network homeostasis in visual cortical layer 2/3. J. Neurosci. 28, 4377–4384. doi: 10.1523/JNEUROSCI.5298-07.2008
Markram, H., Toledo-Rodriguez, M., Wang, Y., Gupta, A., Silberberg, G., and Wu, C. (2004). Interneurons of the neocortical inhibitory system. Nat. Rev. Neurosci. 5, 793–807. doi: 10.1038/nrn1519
Martin del Campo, H. N., Measor, K. R., and Razak, K. A. (2012). Parvalbumin immunoreactivity in the auditory cortex of a mouse model of presbycusis. Hear. Res. 294, 31–39. doi: 10.1016/j.heares.2012.08.017
Martinez, L. M., Alonso, J. M., Reid, R. C., and Hirsch, J. A. (2002). Laminar processing of stimulus orientation in cat visual cortex. J. Physiol. 540, 321–333. doi: 10.1113/jphysiol.2001.012776
Maya-Vetencourt, J. F., Baroncelli, L., Viegi, A., Tiraboschi, E., Castren, E., Cattaneo, A., et al. (2012). IGF-1 restores visual cortex plasticity in adult life by reducing local GABA levels. Neural Plast. 2012:250421. doi: 10.1155/2012/250421
McCormick, D. A. (2002). Cortical and subcortical generators of normal and abnormal rhythmicity. Int. Rev. Neurobiol. 49, 99–114. doi: 10.1016/S0074-7742(02)49009-5
Melzer, S., Michael, M., Caputi, A., Eliava, M., Fuchs, E. C., Whittington, M. A., et al. (2012). Long-range-projecting GABAergic neurons modulate inhibition in hippocampus and entorhinal cortex. Science 335, 1506–1510. doi: 10.1126/science.1217139
Méndez, P., and Bacci, A. (2011). Assortment of GABAergic plasticity in the cortical interneuron melting pot. Neural Plast. 2011:976856. doi: 10.1155/2011/976856
Métin, C., Godement, P., and Imbert, M. (1988). The primary visual cortex in the mouse: receptive field properties and functional organization. Exp. Brain Res. 69, 594–612. doi: 10.1007/BF00247312
Miyoshi, G., Butt, S. J., Takebayashi, H., and Fishell, G. (2007). Physiologically distinct temporal cohorts of cortical interneurons arise from telencephalic Olig2-expressing precursors. J. Neurosci. 27, 7786–7798. doi: 10.1523/JNEUROSCI.1807-07.2007
Miyoshi, G., Hjerling-Leffler, J., Karayannis, T., Sousa, V. H., Butt, S. J., Battiste, J., et al. (2010). Genetic fate mapping reveals that the caudal ganglionic eminence produces a large and diverse population of superficial cortical interneurons. J. Neurosci. 30, 1582–1594. doi: 10.1523/JNEUROSCI.4515-09.2010
Möhler, H. (2006). GABA(A) receptor diversity and pharmacology. Cell Tissue Res. 326, 505–516. doi: 10.1007/s00441-006-0284-3
Monier, C., Chavane, F., Baudot, P., Graham, L. J., and Frégnac, Y. (2003). Orientation and direction selectivity of synaptic inputs in visual cortical neurons: a diversity of combinations produces spike tuning. Neuron 37, 663–680. doi: 10.1016/S0896-6273(03)00064-3
Moore, A. K., and Wehr, M. (2013). Parvalbumin-expressing inhibitory interneurons in auditory cortex are well-tuned for frequency. J. Neurosci. 33, 13713–13723. doi: 10.1523/JNEUROSCI.0663-13.2013
Morales, B., Choi, S. Y., and Kirkwood, A. (2002). Dark rearing alters the development of GABAergic transmission in visual cortex. J. Neurosci. 22, 8084–8090.
Morrison, J. H., Magistretti, P. J., Benoit, R., and Bloom, F. E. (1984). The distribution and morphological characteristics of the intracortical VIP-positive cell: an immunohistochemical analysis. Brain Res. 292, 269–282. doi: 10.1016/0006-8993(84)90763-7
Mower, G. D. (1991). The effect of dark rearing on the time course of the critical period in cat visual cortex. Brain Res. Dev. Brain Res. 58, 151–158. doi: 10.1016/0165-3806(91)90001-Y
Mower, G. D., and Christen, W. G. (1985). Role of visual experience in activating critical period in cat visual cortex. J. Neurophysiol. 53, 572–589.
Murayama, M., Pérez-Garci, E., Nevian, T., Bock, T., Senn, W., and Larkum, M. E. (2009). Dendritic encoding of sensory stimuli controlled by deep cortical interneurons. Nature 457, 1137–1141. doi: 10.1038/nature07663
Murphy, E. H., and Berman, N. (1979). The rabbit and the cat: a comparison of some features of response properties of single cells in the primary visual cortex. J. Comp. Neurol. 188, 401–427. doi: 10.1002/cne.901880305
Nahmani, M., and Turrigiano, G. G. (2014). Deprivation-induced strengthening of presynaptic and postsynaptic inhibitory transmission in layer 4 of visual cortex during the critical period. J. Neurosci. 34, 2571–2582. doi: 10.1523/JNEUROSCI.4600-13.2014
Nase, G., Weishaupt, J., Stern, P., Singer, W., and Monyer, H. (1999). Genetic and epigenetic regulation of NMDA receptor expression in the rat visual cortex. Eur. J. Neurosci. 11, 4320–4326. doi: 10.1046/j.1460-9568.1999.00859.x
Niell, C. M., and Stryker, M. P. (2008). Highly selective receptive fields in mouse visual cortex. J. Neurosci. 28, 7520–7536. doi: 10.1523/JNEUROSCI.0623-08.2008
Nowak, L. G., Sanchez-Vives, M. V., and McCormick, D. A. (2008). Lack of orientation and direction selectivity in a subgroup of fast-spiking inhibitory interneurons: cellular and synaptic mechanisms and comparison with other electrophysiological cell types. Cereb. Cortex 18, 1058–1078. doi: 10.1093/cercor/bhm137
Nusser, Z., Sieghart, W., Benke, D., Fritschy, J. M., and Somogyi, P. (1996). Differential synaptic localization of two major gamma-aminobutyric acid type A receptor alpha subunits on hippocampal pyramidal cells. Proc. Natl. Acad. Sci. U.S.A. 93, 11939–11944. doi: 10.1073/pnas.93.21.11939
Okun, M., and Lampl, I. (2008). Instantaneous correlation of excitation and inhibition during ongoing and sensory-evoked activities. Nat. Neurosci. 11, 535–537. doi: 10.1038/nn.2105
Oláh, S., Füle, M., Komlósi, G., Varga, C., Báldi, R., Barzó, P., et al. (2009). Regulation of cortical microcircuits by unitary GABA-mediated volume transmission. Nature 461, 1278–1281. doi: 10.1038/nature08503
Olcese, U., Iurilli, G., and Medini, P. (2013). Cellular and synaptic architecture of multisensory integration in the mouse neocortex. Neuron 79, 579–593. doi: 10.1016/j.neuron.2013.06.010
Oswald, A. M., Doiron, B., Rinzel, J., and Reyes, A. D. (2009). Spatial profile and differential recruitment of GABAB modulate oscillatory activity in auditory cortex. J. Neurosci. 29, 10321–10334. doi: 10.1523/JNEUROSCI.1703-09.2009
Oswald, A. M., and Reyes, A. D. (2011). Development of inhibitory timescales in auditory cortex. Cereb. Cortex 21, 1351–1361. doi: 10.1093/cercor/bhq214
Ozeki, H., Finn, I. M., Schaffer, E. S., Miller, K. D., and Ferster, D. (2009). Inhibitory stabilization of the cortical network underlies visual surround suppression. Neuron 62, 578–592. doi: 10.1016/j.neuron.2009.03.028
Packer, A. M., and Yuste, R. (2011). Dense, unspecific connectivity of neocortical parvalbumin-positive interneurons: a canonical microcircuit for inhibition? J. Neurosci. 31, 13260–13271. doi: 10.1523/JNEUROSCI.3131-11.2011
Palmer, L. M., Schulz, J. M., Murphy, S. C., Ledergerber, D., Murayama, M., and Larkum, M. E. (2012). The cellular basis of GABA(B)-mediated interhemispheric inhibition. Science 335, 989–993. doi: 10.1126/science.1217276
Parnavelas, J. G., Burne, R. A., and Lin, C. S. (1983). Distribution and morphology of functionally identified neurons in the visual cortex of the rat. Brain Res. 261, 21–29. doi: 10.1016/0006-8993(83)91279-9
Pérez-Garci, E., Gassmann, M., Bettler, B., and Larkum, M. E. (2006). The GABAB1b isoform mediates long-lasting inhibition of dendritic Ca2+ spikes in layer 5 somatosensory pyramidal neurons. Neuron 50, 603–616. doi: 10.1016/j.neuron.2006.04.019
Pfeffer, C. K., Xue, M., He, M., Huang, Z. J., and Scanziani, M. (2013). Inhibition of inhibition in visual cortex: the logic of connections between molecularly distinct interneurons. Nat. Neurosci. 16, 1068–1076. doi: 10.1038/nn.3446
Pi, H. J., Hangya, B., Kvitsiani, D., Sanders, J. I., Huang, Z. J., and Kepecs, A. (2013). Cortical interneurons that specialize in disinhibitory control. Nature 503, 521–524. doi: 10.1038/nature12676
Piëch, V., Li, W., Reeke, G. N., and Gilbert, C. D. (2013). Network model of top-down influences on local gain and contextual interactions in visual cortex. Proc. Natl. Acad. Sci. U.S.A. 110, E4108–E4117. doi: 10.1073/pnas.1317019110
Pinto, J. G., Hornby, K. R., Jones, D. G., and Murphy, K. M. (2010). Developmental changes in GABAergic mechanisms in human visual cortex across the lifespan. Front. Cell. Neurosci. 4:16.
Poo, C., and Isaacson, J. S. (2009). Odor representations in olfactory cortex: “sparse” coding, global inhibition, and oscillations. Neuron 62, 850–861. doi: 10.1016/j.neuron.2009.05.022
Pouille, F., Watkinson, O., Scanziani, M., and Trevelyan, A. J. (2013). The contribution of synaptic location to inhibitory gain control in pyramidal cells. Physiol. Rep. 1:e00067. doi: 10.1002/phy2.67
Priebe, N. J., and Ferster, D. (2005). Direction selectivity of excitation and inhibition in simple cells of the cat primary visual cortex. Neuron 45, 133–145. doi: 10.1016/j.neuron.2004.12.024
Priebe, N. J., and Ferster, D. (2008). Inhibition, spike threshold, and stimulus selectivity in primary visual cortex. Neuron 57, 482–497. doi: 10.1016/j.neuron.2008.02.005
Priebe, N. J., and Ferster, D. (2012). Mechanisms of neuronal computation in mammalian visual cortex. Neuron 75, 194–208. doi: 10.1016/j.neuron.2012.06.011
Razak, K. A., and Fuzessery, Z. M. (2009). GABA shapes selectivity for the rate and direction of frequency-modulated sweeps in the auditory cortex. J. Neurophysiol. 102, 1366–1378. doi: 10.1152/jn.00334.2009
Reiter, H. O., and Stryker, M. P. (1988). Neural plasticity without postsynaptic action potentials: less-active inputs become dominant when kitten visual cortical cells are pharmacologically inhibited. Proc. Natl. Acad. Sci. U.S.A. 85, 3623–3627. doi: 10.1073/pnas.85.10.3623
Renart, A., de la Rocha, J., Bartho, P., Hollender, L., Parga, N., Reyes, A., et al. (2010). The asynchronous state in cortical circuits. Science 327, 587–590. doi: 10.1126/science.1179850
Rochefort, N. L., Narushima, M., Grienberger, C., Marandi, N., Hill, D. N., and Konnerth, A. (2011). Development of direction selectivity in mouse cortical neurons. Neuron 71, 425–432. doi: 10.1016/j.neuron.2011.06.013
Rudy, B., Fishell, G., Lee, S., and Hjerling-Leffler, J. (2011). Three groups of interneurons account for nearly 100% of neocortical GABAergic neurons. Dev. Neurobiol. 71, 45–61. doi: 10.1002/dneu.20853
Runyan, C. A., Schummers, J., Van Wart, A., Kuhlman, S. J., Wilson, N. R., Huang, Z. J., et al. (2010). Response features of parvalbumin-expressing interneurons suggest precise roles for subtypes of inhibition in visual cortex. Neuron 67, 847–857. doi: 10.1016/j.neuron.2010.08.006
Runyan, C. A., and Sur, M. (2013). Response selectivity is correlated to dendritic structure in parvalbumin-expressing inhibitory neurons in visual cortex. J. Neurosci. 33, 11724–11733. doi: 10.1523/JNEUROSCI.2196-12.2013
Sachidhanandam, S., Sreenivasan, V., Kyriakatos, A., Kremer, Y., and Petersen, C. C. (2013). Membrane potential correlates of sensory perception in mouse barrel cortex. Nat. Neurosci. 16, 1671–1677. doi: 10.1038/nn.3532
Sale, A., Berardi, N., and Maffei, L. (2009). Enrich the environment to empower the brain. Trends Neurosci. 32, 233–239. doi: 10.1016/j.tins.2008.12.004
Sale, A., Maya Vetencourt, J. F., Medini, P., Cenni, M. C., Baroncelli, L., De Pasquale, R., et al. (2007). Environmental enrichment in adulthood promotes amblyopia recovery through a reduction of intracortical inhibition. Nat. Neurosci. 10, 679–681. doi: 10.1038/nn1899
Samuelsen, C. L., Gardner, M. P., and Fontanini, A. (2012). Effects of cue-triggered expectation on cortical processing of taste. Neuron 74, 410–422. doi: 10.1016/j.neuron.2012.02.031
Santhakumar, V., and Soltesz, I. (2004). Plasticity of interneuronal species diversity and parameter variance in neurological diseases. Trends Neurosci. 27, 504–510. doi: 10.1016/j.tins.2004.06.002
Saraga, F., Balena, T., Wolansky, T., Dickson, C. T., and Woodin, M. A. (2008). Inhibitory synaptic plasticity regulates pyramidal neuron spiking in the rodent hippocampus. Neuroscience 155, 64–75. doi: 10.1016/j.neuroscience.2008.05.009
Scholl, B., Gao, X., and Wehr, M. (2010). Nonoverlapping sets of synapses drive on responses and off responses in auditory cortex. Neuron 65, 412–421. doi: 10.1016/j.neuron.2010.01.020
Scholl, B., Tan, A. Y., and Priebe, N. J. (2013). Strabismus disrupts binocular synaptic integration in primary visual cortex. J. Neurosci. 33, 17108–17122. doi: 10.1523/JNEUROSCI.1831-13.2013
Schuett, S., Bonhoeffer, T., and Hübener, M. (2002). Mapping retinotopic structure in mouse visual cortex with optical imaging. J. Neurosci. 22, 6549–6559.
Sengpiel, F., and Blakemore, C. (1994). Interocular control of neuronal responsiveness in cat visual cortex. Nature 368, 847–850. doi: 10.1038/368847a0
Sengpiel, F., Blakemore, C., Kind, P. C., and Harrad, R. (1994). Interocular suppression in the visual cortex of strabismic cats. J. Neurosci. 14, 6855–6871.
Shao, Y. R., Isett, B. R., Miyashita, T., Chung, J., Pourzia, O., Gasperini, R. J., et al. (2013). Plasticity of recurrent l2/3 inhibition and gamma oscillations by whisker experience. Neuron 80, 210–222. doi: 10.1016/j.neuron.2013.07.026
Shatz, C. J., and Stryker, M. P. (1978). Ocular dominance in layer IV of the cat's visual cortex and the effects of monocular deprivation. J. Physiol. 281, 267–283.
Silberberg, G., and Markram, H. (2007). Disynaptic inhibition between neocortical pyramidal cells mediated by Martinotti cells. Neuron 53, 735–746. doi: 10.1016/j.neuron.2007.02.012
Sillito, A. M. (1975). The contribution of inhibitory mechanisms to the receptive field properties of neurones in the striate cortex of the cat. J. Physiol. 250, 305–329.
Sillito, A. M. (1979). Inhibitory mechanisms influencing complex cell orientation selectivity and their modification at high resting discharge levels. J. Physiol. 289, 33–53.
Sillito, A. M., Kemp, J. A., and Patel, H. (1980). Inhibitory interactions contributing to the ocular dominance of monocularly dominated cells in the normal cat striate cortex. Exp. Brain Res. 41, 1–10. doi: 10.1007/BF00236673
Simon, A., Oláh, S., Molnár, G., Szabadics, J., and Tamás, G. (2005). Gap-junctional coupling between neurogliaform cells and various interneuron types in the neocortex. J. Neurosci. 25, 6278–6285. doi: 10.1523/JNEUROSCI.1431-05.2005
Simons, D. J. (1978). Response properties of vibrissa units in rat SI somatosensory neocortex. J. Neurophysiol. 41, 798–820.
Simons, D. J., and Carvell, G. E. (1989). Thalamocortical response transformation in the rat vibrissa/barrel system. J. Neurophysiol. 61, 311–330.
Sohya, K., Kameyama, K., Yanagawa, Y., Obata, K., and Tsumoto, T. (2007). GABAergic neurons are less selective to stimulus orientation than excitatory neurons in layer II/III of visual cortex, as revealed by in vivo functional Ca2+ imaging in transgenic mice. J. Neurosci. 27, 2145–2149. doi: 10.1523/JNEUROSCI.4641-06.2007
Somogyi, P. (1977). A specific ‘axo-axonal’ interneuron in the visual cortex of the rat. Brain Res. 136, 345–350. doi: 10.1016/0006-8993(77)90808-3
Somogyi, P., Tamás, G., Lujan, R., and Buhl, E. H. (1998). Salient features of synaptic organisation in the cerebral cortex. Brain Res. Brain Res. Rev. 26, 113–135. doi: 10.1016/S0165-0173(97)00061-1
Southwell, D. G., Froemke, R. C., Alvarez-Buylla, A., Stryker, M. P., and Gandhi, S. P. (2010). Cortical plasticity induced by inhibitory neuron transplantation. Science 327, 1145–1148. doi: 10.1126/science.1183962
Sun, Y. J., Wu, G. K., Liu, B. H., Li, P., Zhou, M., Xiao, Z., et al. (2010). Fine-tuning of pre-balanced excitation and inhibition during auditory cortical development. Nature 465, 927–931. doi: 10.1038/nature09079
Swadlow, H. A. (1988). Efferent neurons and suspected interneurons in binocular visual cortex of the awake rabbit: receptive fields and binocular properties. J. Neurophysiol. 59, 1162–1187.
Swadlow, H. A. (1989). Efferent neurons and suspected interneurons in S-1 vibrissa cortex of the awake rabbit: receptive fields and axonal properties. J. Neurophysiol. 62, 288–308.
Swadlow, H. A., and Gusev, A. G. (2002). Receptive-field construction in cortical inhibitory interneurons. Nat. Neurosci. 5, 403–404. doi: 10.1038/nn847
Swadlow, H. A., and Weyand, T. G. (1987). Corticogeniculate neurons, corticotectal neurons, and suspected interneurons in visual cortex of awake rabbits: receptive-field properties, axonal properties, and effects of EEG arousal. J. Neurophysiol. 57, 977–1001.
Szentágothai, J., and Arbib, M. A. (1974). Conceptual models of neural organization. Neurosci. Res. Program. Bull. 12, 305–510.
Takesian, A. E., Kotak, V. C., and Sanes, D. H. (2010). Presynaptic GABA(B) receptors regulate experience-dependent development of inhibitory short-term plasticity. J. Neurosci. 30, 2716–2727. doi: 10.1523/JNEUROSCI.3903-09.2010
Takesian, A. E., Kotak, V. C., and Sanes, D. H. (2012). Age-dependent effect of hearing loss on cortical inhibitory synapse function. J. Neurophysiol. 107, 937–947. doi: 10.1152/jn.00515.2011
Takesian, A. E., Kotak, V. C., Sharma, N., and Sanes, D. H. (2013). Hearing loss differentially affects thalamic drive to two cortical interneuron subtypes. J. Neurophysiol. 110, 999–1008. doi: 10.1152/jn.00182.2013
Tamamaki, N., Yanagawa, Y., Tomioka, R., Miyazaki, J., Obata, K., and Kaneko, T. (2003). Green fluorescent protein expression and colocalization with calretinin, parvalbumin, and somatostatin in the GAD67-GFP knock-in mouse. J. Comp. Neurol. 467, 60–79. doi: 10.1002/cne.10905
Tamás, G., Buhl, E. H., Lörincz, A., and Somogyi, P. (2000). Proximally targeted GABAergic synapses and gap junctions synchronize cortical interneurons. Nat. Neurosci. 3, 366–371. doi: 10.1038/73936
Tamás, G., Somogyi, P., and Buhl, E. H. (1998). Differentially interconnected networks of GABAergic interneurons in the visual cortex of the cat. J. Neurosci. 18, 4255–4270.
Tan, A. Y., Atencio, C. A., Polley, D. B., Merzenich, M. M., and Schreiner, C. E. (2007). Unbalanced synaptic inhibition can create intensity-tuned auditory cortex neurons. Neuroscience 146, 449–462. doi: 10.1016/j.neuroscience.2007.01.019
Tan, A. Y., Brown, B. D., Scholl, B., Mohanty, D., and Priebe, N. J. (2011). Orientation selectivity of synaptic input to neurons in mouse and cat primary visual cortex. J. Neurosci. 31, 12339–12350. doi: 10.1523/JNEUROSCI.2039-11.2011
Tan, A. Y., and Wehr, M. (2009). Balanced tone-evoked synaptic excitation and inhibition in mouse auditory cortex. Neuroscience 163, 1302–1315. doi: 10.1016/j.neuroscience.2009.07.032
Tan, A. Y., Zhang, L. I., Merzenich, M. M., and Schreiner, C. E. (2004). Tone-evoked excitatory and inhibitory synaptic conductances of primary auditory cortex neurons. J. Neurophysiol. 92, 630–643. doi: 10.1152/jn.01020.2003
Taniguchi, H. (2014). Genetic dissection of GABAergic neural circuits in mouse neocortex. Front. Cell. Neurosci. 8:8. doi: 10.3389/fncel.2014.00008
Taniguchi, H., He, M., Wu, P., Kim, S., Paik, R., Sugino, K., et al. (2011). A resource of Cre driver lines for genetic targeting of GABAergic neurons in cerebral cortex. Neuron 71, 995–1013. doi: 10.1016/j.neuron.2011.07.026
Taniguchi, H., Lu, J., and Huang, Z. J. (2013). The spatial and temporal origin of chandelier cells in mouse neocortex. Science 339, 70–74. doi: 10.1126/science.1227622
Thomson, A. M., West, D. C., Wang, Y., and Bannister, A. P. (2002). Synaptic connections and small circuits involving excitatory and inhibitory neurons in layers 2-5 of adult rat and cat neocortex: triple intracellular recordings and biocytin labelling in vitro. Cereb. Cortex 12, 936–953. doi: 10.1093/cercor/12.9.936
Tiao, Y. C., and Blakemore, C. (1976). Functional organization in the visual cortex of the golden hamster. J. Comp. Neurol. 168, 459–481. doi: 10.1002/cne.901680403
Tremere, L., Hicks, T. P., and Rasmusson, D. D. (2001). Expansion of receptive fields in raccoon somatosensory cortex in vivo by GABA(A) receptor antagonism: implications for cortical reorganization. Exp. Brain Res. 136, 447–455. doi: 10.1007/s002210000612
Van Hooser, S. D., Heimel, J. A., Chung, S., Nelson, S. B., and Toth, L. J. (2005). Orientation selectivity without orientation maps in visual cortex of a highly visual mammal. J. Neurosci. 25, 19–28. doi: 10.1523/JNEUROSCI.4042-04.2005
van Versendaal, D., Rajendran, R., Saiepour, M. H., Klooster, J., Smit-Rigter, L., Sommeijer, J. P., et al. (2012). Elimination of inhibitory synapses is a major component of adult ocular dominance plasticity. Neuron 74, 374–383. doi: 10.1016/j.neuron.2012.03.015
Vitalis, T., and Rossier, J. (2011). New insights into cortical interneurons development and classification: contribution of developmental studies. Dev. Neurobiol. 71, 34–44. doi: 10.1002/dneu.20810
Wahle, P. (1993). Differential regulation of substance P and somatostatin in Martinotti cells of the developing cat visual cortex. J. Comp. Neurol. 329, 519–538. doi: 10.1002/cne.903290408
Wang, B. S., Feng, L., Liu, M., Liu, X., and Cang, J. (2013a). Environmental enrichment rescues binocular matching of orientation preference in mice that have a precocious critical period. Neuron 80, 198–209. doi: 10.1016/j.neuron.2013.07.023
Wang, B. S., Sarnaik, R., and Cang, J. (2010). Critical period plasticity matches binocular orientation preference in the visual cortex. Neuron 65, 246–256. doi: 10.1016/j.neuron.2010.01.002
Wang, L., Fontanini, A., and Maffei, A. (2011). Visual experience modulates spatio-temporal dynamics of circuit activation. Front. Cell. Neurosci. 5:12. doi: 10.3389/fncel.2011.00012
Wang, L., Fontanini, A., and Maffei, A. (2012). Experience-dependent switch in sign and mechanisms for plasticity in layer 4 of primary visual cortex. J. Neurosci. 32, 10562–10573. doi: 10.1523/JNEUROSCI.0622-12.2012
Wang, L., Kloc, M., Gu, Y., Ge, S., and Maffei, A. (2013b). Layer-specific experience-dependent rewiring of thalamocortical circuits. J. Neurosci. 33, 4181–4191. doi: 10.1523/JNEUROSCI.4423-12.2013
Wang, L., and Maffei, A. (2014). Inhibitory plasticity dictates the sign of plasticity at excitatory synapses. J. Neurosci. 34, 1083–1093. doi: 10.1523/JNEUROSCI.4711-13.2014
Wang, Y., Gupta, A., Toledo-Rodriguez, M., Wu, C. Z., and Markram, H. (2002). Anatomical, physiological, molecular and circuit properties of nest basket cells in the developing somatosensory cortex. Cereb. Cortex 12, 395–410. doi: 10.1093/cercor/12.4.395
Wang, Y., Kakizaki, T., Sakagami, H., Saito, K., Ebihara, S., Kato, M., et al. (2009). Fluorescent labeling of both GABAergic and glycinergic neurons in vesicular GABA transporter (VGAT)-venus transgenic mouse. Neuroscience 164, 1031–1043. doi: 10.1016/j.neuroscience.2009.09.010
Wang, Y., Toledo-Rodriguez, M., Gupta, A., Wu, C., Silberberg, G., Luo, J., et al. (2004). Anatomical, physiological and molecular properties of Martinotti cells in the somatosensory cortex of the juvenile rat. J. Physiol. 561, 65–90. doi: 10.1113/jphysiol.2004.073353
Wehr, M., and Zador, A. M. (2003). Balanced inhibition underlies tuning and sharpens spike timing in auditory cortex. Nature 426, 442–446. doi: 10.1038/nature02116
Wehr, M., and Zador, A. M. (2005). Synaptic mechanisms of forward suppression in rat auditory cortex. Neuron 47, 437–445. doi: 10.1016/j.neuron.2005.06.009
Whittington, M. A., Traub, R. D., Kopell, N., Ermentrout, B., and Buhl, E. H. (2000). Inhibition-based rhythms: experimental and mathematical observations on network dynamics. Int. J. Psychophysiol. 38, 315–336. doi: 10.1016/S0167-8760(00)00173-2
Wiesel, T. N., and Hubel, D. H. (1963). Single-cell responses in striate cortex of kittens deprived of vision in one eye. J. Neurophysiol. 26, 1003–1017.
Wilent, W. B., and Contreras, D. (2004). Synaptic responses to whisker deflections in rat barrel cortex as a function of cortical layer and stimulus intensity. J. Neurosci. 24, 3985–3998. doi: 10.1523/JNEUROSCI.5782-03.2004
Wilent, W. B., and Contreras, D. (2005). Dynamics of excitation and inhibition underlying stimulus selectivity in rat somatosensory cortex. Nat. Neurosci. 8, 1364–1370. doi: 10.1038/nn1545
Wilson, N. R., Runyan, C. A., Wang, F. L., and Sur, M. (2012). Division and subtraction by distinct cortical inhibitory networks in vivo. Nature 488, 343–348. doi: 10.1038/nature11347
Wisden, W., Cope, D., Klausberger, T., Hauer, B., Sinkkonen, S. T., Tretter, V., et al. (2002). Ectopic expression of the GABA(A) receptor alpha6 subunit in hippocampal pyramidal neurons produces extrasynaptic receptors and an increased tonic inhibition. Neuropharmacology 43, 530–549. doi: 10.1016/S0028-3908(02)00151-X
Wu, G. K., Arbuckle, R., Liu, B. H., Tao, H. W., and Zhang, L. I. (2008). Lateral sharpening of cortical frequency tuning by approximately balanced inhibition. Neuron 58, 132–143. doi: 10.1016/j.neuron.2008.01.035
Wu, G. K., Li, P., Tao, H. W., and Zhang, L. I. (2006). Nonmonotonic synaptic excitation and imbalanced inhibition underlying cortical intensity tuning. Neuron 52, 705–715. doi: 10.1016/j.neuron.2006.10.009
Wu, G. K., Tao, H. W., and Zhang, L. I. (2011). From elementary synaptic circuits to information processing in primary auditory cortex. Neurosci. Biobehav. Rev. 35, 2094–2104. doi: 10.1016/j.neubiorev.2011.05.004
Wu, X., Wu, Z., Ning, G., Guo, Y., Ali, R., Macdonald, R. L., et al. (2012). gamma-Aminobutyric acid type A (GABAA) receptor alpha subunits play a direct role in synaptic versus extrasynaptic targeting. J. Biol. Chem. 287, 27417–27430. doi: 10.1074/jbc.M112.360461
Xu, H., Jeong, H. Y., Tremblay, R., and Rudy, B. (2013). Neocortical somatostatin-expressing GABAergic interneurons disinhibit the thalamorecipient layer 4. Neuron 77, 155–167. doi: 10.1016/j.neuron.2012.11.004
Xu, H., Kotak, V. C., and Sanes, D. H. (2010). Normal hearing is required for the emergence of long-lasting inhibitory potentiation in cortex. J. Neurosci. 30, 331–341. doi: 10.1523/JNEUROSCI.4554-09.2010
Xu, Q., de la Cruz, E., and Anderson, S. A. (2003). Cortical interneuron fate determination: diverse sources for distinct subtypes? Cereb. Cortex 13, 670–676. doi: 10.1093/cercor/13.6.670
Xu, X., and Callaway, E. M. (2009). Laminar specificity of functional input to distinct types of inhibitory cortical neurons. J. Neurosci. 29, 70–85. doi: 10.1523/JNEUROSCI.4104-08.2009
Yang, S., Weiner, B. D., Zhang, L. S., Cho, S. J., and Bao, S. (2011). Homeostatic plasticity drives tinnitus perception in an animal model. Proc. Natl. Acad. Sci. U.S.A. 108, 14974–14979. doi: 10.1073/pnas.1107998108
Yashiro, K., and Philpot, B. D. (2008). Regulation of NMDA receptor subunit expression and its implications for LTD, LTP, and metaplasticity. Neuropharmacology 55, 1081–1094. doi: 10.1016/j.neuropharm.2008.07.046
Yazaki-Sugiyama, Y., Kang, S., Câteau, H., Fukai, T., and Hensch, T. K. (2009). Bidirectional plasticity in fast-spiking GABA circuits by visual experience. Nature 462, 218–221. doi: 10.1038/nature08485
Yokota, T., Eguchi, K., and Hiraba, K. (2011). Functional properties of putative pyramidal neurons and inhibitory interneurons in the rat gustatory cortex. Cereb. Cortex 21, 597–606. doi: 10.1093/cercor/bhq126
Yu, J., and Ferster, D. (2010). Membrane potential synchrony in primary visual cortex during sensory stimulation. Neuron 68, 1187–1201. doi: 10.1016/j.neuron.2010.11.027
Zariwala, H. A., Madisen, L., Ahrens, K. F., Bernard, A., Lein, E. S., Jones, A. R., et al. (2011). Visual tuning properties of genetically identified layer 2/3 neuronal types in the primary visual cortex of cre-transgenic mice. Front. Syst. Neurosci. 4:162. doi: 10.3389/fnsys.2010.00162
Zhang, L. I., Bao, S., and Merzenich, M. M. (2001). Persistent and specific influences of early acoustic environments on primary auditory cortex. Nat. Neurosci. 4, 1123–1130. doi: 10.1038/nn745
Zhang, L. I., Tan, A. Y., Schreiner, C. E., and Merzenich, M. M. (2003). Topography and synaptic shaping of direction selectivity in primary auditory cortex. Nature 424, 201–205. doi: 10.1038/nature01796
Zhang, Z., Jiao, Y. Y., and Sun, Q. Q. (2011). Developmental maturation of excitation and inhibition balance in principal neurons across four layers of somatosensory cortex. Neuroscience 174, 10–25. doi: 10.1016/j.neuroscience.2010.11.045
Zheng, J. J., Li, S. J., Zhang, X. D., Miao, W. Y., Zhang, D., Yao, H., et al. (2014). Oxytocin mediates early experience-dependent cross-modal plasticity in the sensory cortices. Nat. Neurosci. 17, 391–399. doi: 10.1038/nn.3634
Zhou, Y., Liu, B. H., Wu, G. K., Kim, Y. J., Xiao, Z., Tao, H. W., et al. (2010). Preceding inhibition silences layer 6 neurons in auditory cortex. Neuron 65, 706–717. doi: 10.1016/j.neuron.2010.02.021
Zhuang, J., Stoelzel, C. R., Bereshpolova, Y., Huff, J. M., Hei, X., Alonso, J. M., et al. (2013). Layer 4 in primary visual cortex of the awake rabbit: contrasting properties of simple cells and putative feedforward inhibitory interneurons. J. Neurosci. 33, 11372–11389. doi: 10.1523/JNEUROSCI.0863-13.2013
Zhu, J. J., and Connors, B. W. (1999). Intrinsic firing patterns and whisker-evoked synaptic responses of neurons in the rat barrel cortex. J. Neurophysiol. 81, 1171–1183.
Zhu, Y., Stornetta, R. L., and Zhu, J. J. (2004). Chandelier cells control excessive cortical excitation: characteristics of whisker-evoked synaptic responses of layer 2/3 nonpyramidal and pyramidal neurons. J. Neurosci. 24, 5101–5108. doi: 10.1523/JNEUROSCI.0544-04.2004
Keywords: synaptic plasticity, GABA, inhibitory neurons, sensory cortex, inhibition
Citation: Griffen TC and Maffei A (2014) GABAergic synapses: their plasticity and role in sensory cortex. Front. Cell. Neurosci. 8:91. doi: 10.3389/fncel.2014.00091
Received: 20 January 2014; Accepted: 12 March 2014;
Published online: 26 March 2014.
Edited by:
Andrea Barberis, Fondazione Istituto Italiano di Tecnologia, ItalyReviewed by:
Enrico Cherubini, International School for Advanced Studies, ItalyPaolo Medini, Umeå Universitet, Sweden
Copyright © 2014 Griffen and Maffei. This is an open-access article distributed under the terms of the Creative Commons Attribution License (CC BY). The use, distribution or reproduction in other forums is permitted, provided the original author(s) or licensor are credited and that the original publication in this journal is cited, in accordance with accepted academic practice. No use, distribution or reproduction is permitted which does not comply with these terms.
*Correspondence: Trevor C. Griffen and Arianna Maffei, Department of Neurobiology and Behavior, Life Science Building Rm. 548, SUNY-Stony Brook, Stony Brook, NY 11794, USA e-mail:dHJldm9yLmdyaWZmZW5Ac3Rvbnlicm9vay5lZHU=;YXJpYW5uYS5tYWZmZWlAc3Rvbnlicm9vay5lZHU=