- 1Neuroprotection and Neurogenesis in Brain Repair Group, Center for Neuroscience and Cell Biology, University of Coimbra, Coimbra, Portugal
- 2Faculty of Medicine, Institute of Biomedical Imaging and Life Sciences, University of Coimbra, Coimbra, Portugal
- 3Institute for Interdisciplinary Research, University of Coimbra, Coimbra, Portugal
Microglial Origin and Function
Microglial cells originate from precursor cells located in the yolk sac that migrate into the developing central nervous system (CNS) around E8.5-10 in mice. Considering their origin, microglial cells could be regarded as invaders of the CNS in charge of debris clearance, just active during brain injury, infection or degeneration. In adulthood and under certain conditions, monocytes may penetrate the blood brain barrier, reach the CNS and become non-resident brain macrophages. CNS infiltrating-macrophages are difficult to distinguish from resident microglial cells and it is not clear the grade of functional similarity between these two types of cells (Prinz and Mildner, 2011; Gomez Perdiguero et al., 2013). Interestingly, microglial cells are able to self-renew independently of circulating monocytes or bone marrow hematopoietic stem cells (Ajami et al., 2007; Elmore et al., 2014), and show a specific molecular signature (Butovsky et al., 2014). Moreover, during the last decade microglial cells have been demonstrated to be involved in normal brain development and function while maintaining a, previously assumed, “resting” state (Pont-Lezica et al., 2011; Tremblay et al., 2011; Valero et al., 2012). Thus, microglial cells cannot be considered just as macrophages.
The Actively “Resting” Microglia and their Interaction with Neurons
The healthy brain is continuously under the active surveillance of a dynamic network of microglial processes. These processes have been shown to permanently protrude and retract, and to physically interact with neuronal synapses to modulate synaptic plasticity. Microglia phagocytic control of synaptic pruning is mediated by the complement pathway (Schafer et al., 2012) and fractalkine signaling (Paolicelli et al., 2011). Furthermore, microglia also regulate synaptic plasticity by the release of diffusible molecules like the brain-derived neurotrophic factor (Parkhurst et al., 2013) or the coactivator of synaptic NMDA receptors D-serine (Scianni et al., 2013). Therefore, microglial cells are able to modulate neuronal function in several ways. Importantly, this is not a unidirectional path of communication, but a dialog between microglia and neurons (Saijo and Glass, 2011; Eyo and Wu, 2013). As a clear example, the chemokine fractalkine (constitutively expressed by neurons), and its receptor (CX3CR1, mainly present in microglial cells), participate in the modulation of hippocampal synaptic pruning and function (Paolicelli et al., 2011; Scianni et al., 2013; Zhan et al., 2014). Fractalkine/CX3CR1 axis is better known to be involved in keeping microglia in their “resting” surveillance state (Sheridan and Murphy, 2013; Wolf et al., 2013). Thus, fractlakine/CX3CR1 system represents a clear example of mutual functional regulation between neurons and microglia. Moreover, there are other pairs of neuronal ligands and microglial receptors which mediate communication between these two elements of the CNS: the neuronal surface proteins CD200, CD47, and CD22 that bind to the microglial receptors CD200R, CD172, and CD45, respectively (Biber et al., 2007).
Microglial cells show regional differences in the brain in terms of density, molecular characteristics, morphology, and responsiveness (Olah et al., 2011; Butovsky et al., 2014). All microglial cells express the colony-stimulating factor receptor (CSF1R), which is required for their development. Interleukine-34, a ligand of CSF1R, is mainly expressed by neurons in specific regions of the brain (cortex, hippocampus, striatum, and anterior olfactory nucleus) where it promotes microglial survival (Greter et al., 2012; Wang et al., 2012). Similarly, high levels of fractalkine expression have been found in neurons of the amygdala, striatum, globus pallidus, thalamus, olfactory bulb, hippocampus, and cerebral cortex (Tarozzo et al., 2003; Kim et al., 2011). Fractalkine is also expressed by astrocytes at lower levels (Hulshof et al., 2003; Sunnemark et al., 2005). Interestingly, the specific molecular signature of adult microglia is dependent on the presence of the transforming growth factor-β (TGF-β) which is expressed at low levels by neurons and glial cells (Butovsky et al., 2014). Therefore, neurons, by producing different levels of the aforementioned molecules, may be responsible of the regional characteristics and heterogeneity of microglial cells.
Microglial Cells in the Absence of Neurons
Taking into account previous data, it seems clear that neurons are active modulators of microglial functional state and could be responsible for microglial regional differences. Considering that, under normal conditions, neurons shape microglial cells and that the main role of microglia is the functional modulation and maintenance of neurons, an important question emerges: does it make sense to talk about microglia in the absence of neurons? Indeed, this question can be re-formulated as: do microglial cells retain their identity in the absence of neurons? If we consider that the interaction with neurons is crucial for the definition of microglia, the answer seems to be clear. Our opinion paper aims to provoke reflection and is in no way intended to despise the value of prior studies that, using isolated microglial cells or cell lines, have contributed to the current knowledge of microglial cell biology.
It is important to note that isolated microglia do not display, in cell cultures, the highly ramified structure that is typically observed in the normal, healthy CNS. Ex vivo microglial cells analyzed immediately upon isolation resemble reactive amoeboid microglia, probably due to the isolation process itself. Nevertheless, this activation seems to wane with time and cell passages, reaching an approximation to the typical “resting” morphology. Immortalized microglial cell lines are an alternative to the use of primary microglial cell cultures, which are costly and time consuming. Several microglial cell lines derived from rat (HAPI), mouse (BV2, N9, EOC), and also human (HMO6), have been used. These cell lines exhibit characteristic microglial/macrophage cell markers and behaviors (cytokine release, migration, and phagocytosis) in the presence of endotoxins such as the gram-negative bacteria lipopolysaccharide (LPS, Figure 1). However, the immortalization renders these cells different from primary microglia; in terms of molecular expression (Butovsky et al., 2014), morphology, proliferation, and adhesion (reviewed in Stansley et al., 2012).
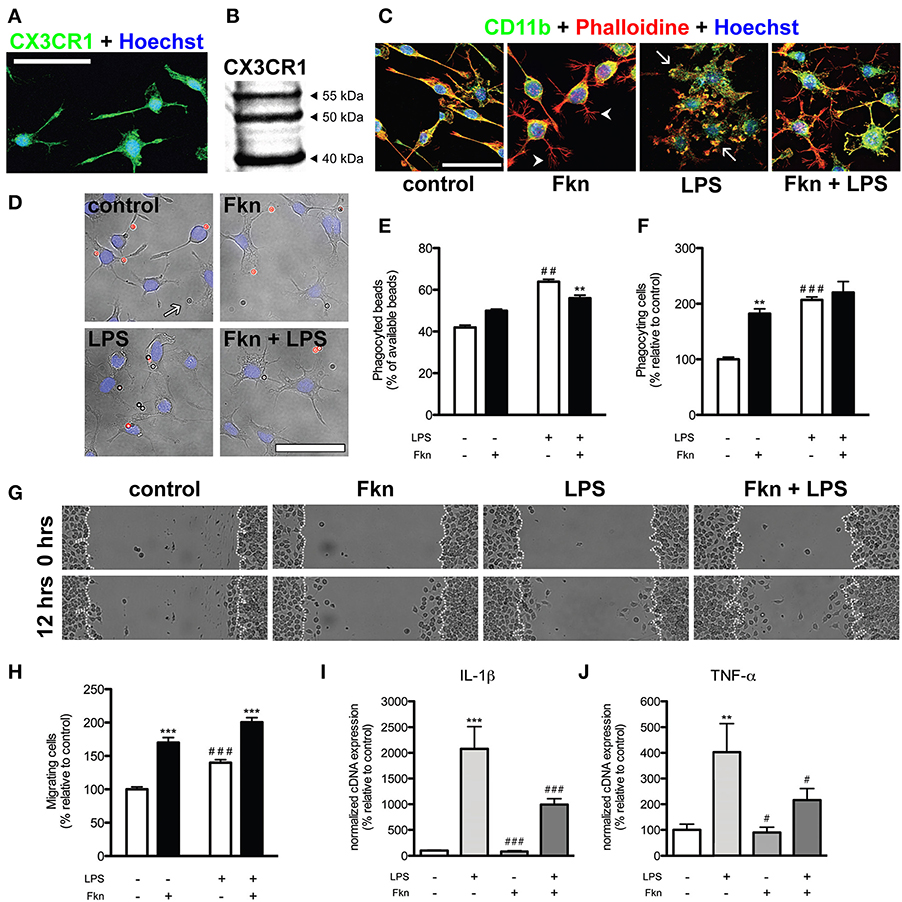
Figure 1. Soluble fractalkine changes morphology and behavior of N9 microglial cells. (A) Confocal microscopy image of N9 microglial cells expressing CX3CR1 (green, rabbit anti-CX3CR1, eBioscience). Cell nuclei were stained with Hoechst 33342 (blue, Life Technologies). Almost all N9 cells in basal culture conditions showed expression of CX3CR1. (B) Western blotting of 50 μ g whole cell extracts from N9 cells showing the previously described pattern (Yang et al., 2007). (C) Representative confocal images of N9 microglial cells stained for CD11b (green, rat anti-CD11b, AbD Serotec), filamentous actin (red, phalloidin-Alexa Fluor® 594 conjugate, Life Technologies) and Hoechst 33342 (blue). In basal (control) conditions N9 microglial cells showed different morphologies (from bipolar to ramified). Treatment of N9 cells with fractalkine chemokine domain (Fkn, 6 h, 200 ng/ml, Sigma-Aldrich) induced the adoption of a ramified morphology by N9 cells characterized by thin filopodia-like structures (arrowheads). As expected, lipopolysaccharide treatment (LPS, 6 h, 100 ng/ml, from Escherichia coli, Sigma-Aldrich) induced swelling, loss of ramified morphology and the appearance of thick membrane protrusions (“ruffles,” arrows) in N9 microglia. In the presence of both LPS and Fkn, N9 cells displayed an intermediate morphology showing “ruffles” and filopodia-like structures. (D) Representative epifluorescence/phase contrast images of N9 cells (cell nuclei stained with Hoechst 33342, blue) in the presence of beads (2 × 106 beads/well, Sigma-Aldrich) coated with IgG from rabbit serum (Sigma-Aldrich). Due to the protocol used (for details check: Ferreira et al., 2011) phagocyted beads were not stained (arrows) while non-phagocyted beads showed red fluorescence (Alexa Fluor® 594 donkey anti-rabbit IgG, Life Technologies). (E) LPS treatment (6 h) led to an increase in the total number of phagocyted beads. Incubation with Fkn (6 h) just slightly reduced the percentage of beads phagocyted by LPS treated N9 cells. (F) LPS and Fkn treatments (6 h) increased the proportion of phagocyting cells. (G,H) A scratch wound assay was carried out to analyse N9 migratory activity (for details see: Ferreira et al., 2012) based on the mean number of cells that moved into the wound after 12 h. (G) Phase-contrast representative images of the scratch wound assay. (H) Co-incubation of N9 cells with LPS and Fkn resulted in a greater migratory induction than that elicited by LPS treatment alone. (I) IL-1β and (J) TNF-α mRNA expression in N9 cells was increased in the presence of LPS (4 h). Importantly Fkn treatment downregulated the increase in IL-1β and TNF-α mRNAs induced by LPS. All scale bars = 50 μm. Data are presented as mean ± s.e.m. (E,F,H): **p < 0.01 and ***p < 0.001 vs. respective condition without Fkn, ##p < 0.01 and ###p < 0.001 vs. control condition (2-Way ANOVA and Bonferroni's post-hoc test). (I,J): **p < 0.01 and ***p < 0.001 vs. control condition, #p < 0.05 and ###p < 0.001 vs. LPS (Pair Wise Fixed Reallocation Randomization Test©, Pfaffl et al., 2002).
Filling the Gap Between in vitro and in vivo Microglia
It still remains to be elucidated whether isolated microglial cells of in vitro systems behave as proper microglia. The importance of this question probably depends on the parameters to be evaluated, e.g., in the absence of direct contact with neurons these cells will fail to develop some specific characteristics of microglial cells. The obvious solution for the aforementioned problem is the use of co-culture systems in which microglial cells and neurons co-exist. Again, the regional origin of microglial and neuronal cells may influence their interaction and should be taking into account when inferring general mechanisms. Nevertheless, some studies require the use of isolated microglia. As previously mentioned, neurons release several factors that regulate microglial state. The use of the adequate combination of these factors in cell culture could contribute to the reduction of the gap between in vivo and in vitro systems, even in the absence of neurons. On this respect, we have investigated the effects of treating N9 microglial cells with soluble fractalkine in basal conditions or after activation with LPS. We observed that N9 cells expressed CX3CR1 mRNA and protein using quantitative real time PCR (not shown), immunofluorescence (Figure 1A) and western blotting (Figure 1B). Interestingly, in basal and LPS conditions fractalkine induced N9 cells to acquire a more ramified morphology (Figure 1C). Fractalkine treatment also increased the number of phagocyting (Figures 1D–F) and migrating N9 cells (Figures 1G,H). Furthermore, fractalkine was also able to reduce the expression of interleukin-1β (IL-1β) and tumor necrosis factor-α (TNF-α) mRNAs induced by LPS treatment (Figures 1I,J) while maintaining elevated levels of phagocytic and migratory activity in N9 cells. Fractalkine has a clear role in shaping microglial cells, evident at the morphological, functional and molecular levels. This role could be carried out by the membrane bound or even the constitutively cleaved fractalkine in vivo, keeping the cells in an alerted but relatively latent state (Sheridan and Murphy, 2013; Wolf et al., 2013). Thus, the lack of fractalkine in isolated microglial cultures is a possible reason for the lack of “surveying”-like microglial phenotype observed in these systems. Our data suggest that supplementation of microglial culture media with fractalkine may serve to shorten the gap between the typical morphology and behavior of microglia that is observed in vivo versus displayed in most in vitro models. Nevertheless, the addition of fractalkine to cell cultures is far from being a definitive solution. Therefore, this idea could be extended to the use of the adequate combination of microglial modulating factors released by neurons and/or macroglial cells. Butovsky et al. (2014) observed that adult microglia cultured in the presence of MCSF (macrophage colony-stimulating factor) and TGF-β 1 showed a molecular expression pattern similar to freshly sorted adult microglia. Nevertheless, treatment of N9 and BV2 cells with these factors did not induce the expression of such microglial molecular pattern (Butovsky et al., 2014), indicating the limitations of microglial cell lines. Thus, further research should be done to identify and define the individual contribution and combined effect of different factors to maintain the functional and molecular characteristics of microglial cells.
We must assume that the perfect in vitro system is just a utopia. By definition, in vitro microglia systems will never reach the complexity of in vivo ones, but in turn will allow the study of some aspects of microglial biology that are masked by surrounding factors (e.g. by the presence of neurons and/or macroglial cells). Thus, some intrinsic characteristics of microglia can be easier analyzed in vitro, like the molecular mechanisms involved in phagocytosis, cell migration, transcriptional control, and metabolic functioning. In the other way around, the analysis of complex systems can mask particular aspects of its individual components (microglial cells), but lead to the discovery of new mechanisms and functionalities that emerge from the interaction of the parts (e.g., microglial regulation of synaptogenesis). However, even in vivo experimental designs, due to the need of controlling as many variables as possible and to the use of experimental manipulations, are normally far from the complexity of natural systems. Thus, we should be cautious when trying to predict, from our particular experimental systems, the features and behavior of microglial cells in their natural milieu (a CNS integrated into a full alive organism, which is also influenced by its surrounding environment).
Conclusions
Our initial question could be seen just as a mere language issue related to how we define “microglia”: (1) based on their intrinsic properties or (2) based on the specific characteristics that emerge through their interaction with other elements of the CNS. We consider that microglia are defined by these two aspects of their biology and that one influences the other. As an example, the constitutive expression of CX3CR1 by microglial cells, an intrinsic property shared with other macrophages, allows them to be shaped by neuronal fractalkine. As previously mentioned, the binding of fractalkine to its receptor controls microglial surveillance state but also mediates their role on the modulation of synaptic function, a specific characteristic that emerges through their interaction with neurons. Therefore, the study of intrinsic features (maybe shared with other cell types) and specific characteristics of microglia that emerge through their interaction with other components of the CNS are equally important to understand the nature of these cells. Obviously, these two ways of studying microglia will benefit in different grades from distinct experimental approaches, ranging from the examination of isolated cells in vitro to their analysis in their natural environment in vivo. Again, we will just need to be careful when generalizing or directly translating our observations from one to another level of biological complexity. These considerations will be specially relevant for the development of strategies aimed to use microglial cells as cell therapeutic agents.
Author Contributions
Conceived and designed the experiments: Ismael Neiva, João O. Malva, and Jorge Valero. Performed the experiments: Ismael Neiva, Analyzed the data: Ismael Neiva. Prepared figures: Ismael Neiva and Jorge Valero. Wrote the paper: Ismael Neiva, João O. Malva, and Jorge Valero.
Conflict of Interest Statement
The authors declare that the research was conducted in the absence of any commercial or financial relationships that could be construed as a potential conflict of interest.
Acknowledgments
This work was supported by FEDER funds through COMPETE program and FCT (“Fundação para a Ciência e a Tecnologia”) national funds: PEst-C/SAU/LA0001/2013-2014. Jorge Valero salary was supported by a FCT postdoctoral grant (SFRH/BPD/68950/2010).
References
Ajami, B., Bennett, J. L., Krieger, C., Tetzlaff, W., and Rossi, F. M. V. (2007). Local self-renewal can sustain CNS microglia maintenance and function throughout adult life. Nat. Neurosci. 10, 1538–1543. doi: 10.1038/nn2014
Biber, K., Neumann, H., Inoue, K., and Boddeke, H. W. G. M. (2007). Neuronal “On” and “Off” signals control microglia. Trends Neurosci. 30, 596–602. doi: 10.1016/j.tins.2007.08.007
Butovsky, O., Jedrychowski, M. P., Moore, C. S., Cialic, R., Lanser, A. J., Gabriely, G., et al. (2014). Identification of a unique TGF-β-dependent molecular and functional signature in microglia. Nat. Neurosci. 17, 131–143. doi: 10.1038/nn.3599
Elmore, M. R. P., Najafi, A. R., Koike, M. A., Dagher, N. N., Spangenberg, E. E., Rice, R. A., et al. (2014). Colony-stimulating factor 1 receptor signaling is necessary for microglia viability, unmasking a microglia progenitor cell in the adult brain. Neuron 82, 380–397. doi: 10.1016/j.neuron.2014.02.040
Eyo, U. B., and Wu, L.-J. (2013). Bidirectional microglia-neuron communication in the healthy brain. Neural Plast. 2013. doi: 10.1155/2013/456857
Ferreira, R., Santos, T., Cortes, L., Cochaud, S., Agasse, F., Silva, A. P., et al. (2012). Neuropeptide Y inhibits interleukin-1 beta-induced microglia motility. J. Neurochem. 120, 93–105. doi: 10.1111/j.1471-4159.2011.07541.x
Ferreira, R., Santos, T., Viegas, M., Cortes, L., Bernardino, L., Vieira, O. V., et al. (2011). Neuropeptide Y inhibits interleukin-1β-induced phagocytosis by microglial cells. J. Neuroinflammation 8:169. doi: 10.1186/1742-2094-8-169
Gomez Perdiguero, E., Schulz, C., and Geissmann, F. (2013). Development and homeostasis of “resident” myeloid cells: the case of the microglia. Glia 61, 112–120. doi: 10.1002/glia.22393
Greter, M., Lelios, I., Pelczar, P., Hoeffel, G., Price, J., Leboeuf, M., et al. (2012). Stroma-derived interleukin-34 controls the development and maintenance of langerhans cells and the maintenance of microglia. Immunity 37, 1050–1060. doi: 10.1016/j.immuni.2012.11.001
Hulshof, S., van Haastert, E. S., Kuipers, H. F., van den Elsen, P. J., De Groot, C. J., van der Valk, P., et al. (2003). CX3CL1 and CX3CR1 expression in human brain tissue: noninflammatory control versus multiple sclerosis. J. Neuropathol. Exp. Neurol. 62, 899–907.
Kim, K.-W., Vallon-Eberhard, A., Zigmond, E., Farache, J., Shezen, E., Shakhar, G., et al. (2011). In vivo structure/function and expression analysis of the CX3C chemokine fractalkine. Blood 118, e156–e167. doi: 10.1182/blood-2011-04-348946
Olah, M., Biber, K., Vinet, J., and Boddeke, H. W. (2011). Microglia phenotype diversity. CNS Neurol. Disord. Drug Targets 10, 108–118. doi: 10.2174/187152711794488575
Paolicelli, R. C., Bolasco, G., Pagani, F., Maggi, L., Scianni, M., Panzanelli, P., et al. (2011). Synaptic pruning by microglia is necessary for normal brain development. Science 333, 1456–1458. doi: 10.1126/science.1202529
Parkhurst, C. N., Yang, G., Ninan, I., Savas, J. N., Yates, J. R., Lafaille, J. J., et al. (2013). Microglia promote learning-dependent synapse formation through BDNF. Cell 155, 1596–1609. doi: 10.1016/j.cell.2013.11.030
Pfaffl, M. W., Horgan, G. W., and Dempfle, L. (2002). Relative expression software tool (REST©) for group-wise comparison and statistical analysis of relative expression results in real-time PCR. Nucleic Acids Res. 30, e36–e36. doi: 10.1093/nar/30.9.e36
Pont-Lezica, L., Béchade, C., Belarif-Cantaut, Y., Pascual, O., and Bessis, A. (2011). Physiological roles of microglia during development. J. Neurochem. 119, 901–908. doi: 10.1111/j.1471-4159.2011.07504.x
Prinz, M., and Mildner, A. (2011). Microglia in the CNS: immigrants from another world. Glia 59, 177–187. doi: 10.1002/glia.21104
Saijo, K., and Glass, C. K. (2011). Microglial cell origin and phenotypes in health and disease. Nat. Rev. Immunol. 11, 775–787. doi: 10.1038/nri3086
Schafer, D. P., Lehrman, E. K., Kautzman, A. G., Koyama, R., Mardinly, A. R., Yamasaki, R., et al. (2012). Microglia sculpt postnatal neural circuits in an activity and complement-dependent manner. Neuron 74, 691–705. doi: 10.1016/j.neuron.2012.03.026
Scianni, M., Antonilli, L., Chece, G., Cristalli, G., Castro, M. A. D., Limatola, C., et al. (2013). Fractalkine (CX3CL1) enhances hippocampal N-methyl-d-aspartate receptor (NMDAR) function via d-serine and adenosine receptor type A2 (A2AR) activity. J. Neuroinflammation 10:108. doi: 10.1186/1742-2094-10-108
Sheridan, G. K., and Murphy, K. J. (2013). Neuron–glia crosstalk in health and disease: fractalkine and CX3CR1 take centre stage. Open Biol. 3:130181. doi: 10.1098/rsob.130181
Stansley, B., Post, J., and Hensley, K. (2012). A comparative review of cell culture systems for the study of microglial biology in Alzheimer's disease. J. Neuroinflammation 9:115. doi: 10.1186/1742-2094-9-115
Sunnemark, D., Eltayeb, S., Nilsson, M., Wallström, E., Lassmann, H., Olsson, T., et al. (2005). CX3CL1 (fractalkine) and CX3CR1 expression in myelin oligodendrocyte glycoprotein-induced experimental autoimmune encephalomyelitis: kinetics and cellular origin. J. Neuroinflammation 2:17. doi: 10.1186/1742-2094-2-17
Tarozzo, G., Bortolazzi, S., Crochemore, C., Chen, S.-C., Lira, A. S., Abrams, J., et al. (2003). Fractalkine protein localization and gene expression in mouse brain. J. Neurosci. Res. 73, 81–88. doi: 10.1002/jnr.10645
Tremblay, M.-È., Stevens, B., Sierra, A., Wake, H., Bessis, A., and Nimmerjahn, A. (2011). The role of microglia in the healthy brain. J. Neurosci. 31, 16064–16069. doi: 10.1523/JNEUROSCI.4158-11.2011
Valero, J., Eiriz, M. F., Santos, T., Neiva, I., Ferreira, R., and Malva, J. O. (2012). “Microglia: the bodyguard and the hunter of the adult neurogenic niche,” in Advances in Stem Cell Research Stem Cell Biology and Regenerative Medicine, eds H. Baharvand and N. Aghdami (Humana Press), 245–279. Available online at: http://link.springer.com/chapter/10.1007/978-1-61779-940-2_14 (Accessed May 12, 2013).
Wang, Y., Szretter, K. J., Vermi, W., Gilfillan, S., Rossini, C., Cella, M., et al. (2012). IL-34 is a tissue-restricted ligand of CSF1R required for the development of Langerhans cells and microglia. Nat. Immunol. 13, 753–760. doi: 10.1038/ni.2360
Wolf, Y., Yona, S., Kim, K.-W., and Jung, S. (2013). Microglia, seen from the CX3CR1 angle. Front. Cell. Neurosci. 7:26. doi: 10.3389/fncel.2013.00026
Yang, X. P., Mattagajasingh, S., Su, S., Chen, G., Cai, Z., Fox-Talbot, K., et al. (2007). Fractalkine upregulates intercellular adhesion molecule-1 in endothelial cells through CX3CR1 and the JAK–STAT5 pathway. Circ. Res. 101, 1001–1008. doi: 10.1161/CIRCRESAHA.107.160812
Keywords: cell culture, CX3CR1, fractalkine, microglia, N9 cells
Citation: Neiva I, Malva JO and Valero J (2014) Can we talk about microglia without neurons? A discussion of microglial cell autonomous properties in culture. Front. Cell. Neurosci. 8:202. doi: 10.3389/fncel.2014.00202
Received: 05 May 2014; Accepted: 04 July 2014;
Published online: 24 July 2014.
Edited by:
Raquel Ferreira, University of Southern California, USAReviewed by:
Uwe-Karsten Hanisch, University of Göttingen, GermanyMarie-Eve Tremblay, Université Laval, Canada
Copyright © 2014 Neiva, Malva and Valero. This is an open-access article distributed under the terms of the Creative Commons Attribution License (CC BY). The use, distribution or reproduction in other forums is permitted, provided the original author(s) or licensor are credited and that the original publication in this journal is cited, in accordance with accepted academic practice. No use, distribution or reproduction is permitted which does not comply with these terms.
*Correspondence:am9yZ2UudmFsZXJvQGNuYy51Yy5wdA==