- 1Department of Biological Sciences, Tata Institute of Fundamental Research, Mumbai, India
- 2InStem, Bangalore, India
Microtubules form important cytoskeletal structures that play a role in establishing and maintaining neuronal polarity, regulating neuronal morphology, transporting cargo, and scaffolding signaling molecules to form signaling hubs. Within a neuronal cell, microtubules are found to have variable lengths and can be both stable and dynamic. Microtubule associated proteins, post-translational modifications of tubulin subunits, microtubule severing enzymes, and signaling molecules are all known to influence both stable and dynamic pools of microtubules. Microtubule dynamics, the process of interconversion between stable and dynamic pools, and the proportions of these two pools have the potential to influence a wide variety of cellular processes. Reduced microtubule stability has been observed in several neurodegenerative diseases such as Alzheimer's disease (AD), Parkinson's disease (PD), Amyotrophic Lateral Sclerosis (ALS), and tauopathies like Progressive Supranuclear Palsy. Hyperstable microtubules, as seen in Hereditary Spastic Paraplegia (HSP), also lead to neurodegeneration. Therefore, the ratio of stable and dynamic microtubules is likely to be important for neuronal function and perturbation in microtubule dynamics might contribute to disease progression.
Introduction
Neurons receive information and relay it along axons, to other neurons or muscles, through structures known as synapses. Neurodegeneration refers to the progressive loss of structure and/or function of neurons, often beginning at the synaptic distal ends of axons, a phenomenon termed as “dying-back neuropathy” (Goto et al., 2009; Dadon-Nachum et al., 2010). Neurodegeneration in humans can cause a variety of symptoms depending on the class of neurons affected and can lead to fatal outcomes. Some widely studied neurodegenerative diseases include Alzheimer's disease (AD), Parkinson's disease (PD), Huntington's disease (HD), and Amyotrophic Lateral Sclerosis (ALS). These, and other less well studied neurodegenerative diseases, exhibit a broad range of clinical symptoms, which nonetheless share several common pathological features (Skovronsky et al., 2006; Glass et al., 2010; Arnold et al., 2013; Baird and Bennett, 2013). One prominent cellular feature is the toxic aggregation of proteins that inhibit the protein quality control and the ubiquitin-proteasome machinery of the neuron (Skovronsky et al., 2006; Arnold et al., 2013; Takalo et al., 2013). Other common characteristics include inflammatory responses (Glass et al., 2010), impaired ER calcium homeostasis (Paschen and Mengesdorf, 2005), increased oxidative stress (Ischiropoulos and Beckman, 2003), and microtubule defects (Baird and Bennett, 2013). It is essential to recognize common underlying features that permit degeneration of neurons to understand the most frequent mechanisms contributing to disease progression. Such an understanding can potentially lead to better therapeutic agents that can retard progression of the most debilitating symptoms associated with neurodegeneration.
Microtubules have often been thought to participate in neurodegenerative diseases through their well-established role in long-distance cargo transport. Examples include neurodegenerative diseases such as AD, HD, and several tauopathies that show compromised microtubule-dependent axonal transport in disease models (extensively reviewed in Garcia and Cleveland, 2001; Goedert and Jakes, 2005; De Vos et al., 2008; Baird and Bennett, 2013; Franker and Hoogenraad, 2013; Hinckelmann et al., 2013; Millecamps and Julien, 2013; Beharry et al., 2014; Encalada and Goldstein, 2014). However, these polymers play important roles in many aspects of neuronal cell biology that include establishing and conserving neuronal polarity (Baas et al., 1988, 1989; Craig and Banker, 1994; Witte et al., 2008), maintaining neuronal morphology (Jacobs and Stevens, 1986; Jaworski et al., 2009; Liu and Dwyer, 2014) and modulating signaling events (Janmey, 1998; Wittmann and Waterman-Storer, 2001; Bounoutas et al., 2011; Dent and Baas, 2014). In several neurodegenerative diseases, that include sporadic rather than familial cases, we know little about the causes of disease onset. We propose that disruption of microtubule dynamics may be a key mechanism contributing to neurodegeneration, since an alteration in dynamics can affect a number of the roles mentioned above. Therefore, modulating microtubule dynamics might help in retarding the progress of neurodegenerative diseases.
Microtubules Contribute to Neuronal Polarity
A critical aspect of neuronal function depends on establishing the polarity between the axonal and somatodendritic domains of the neuron (Craig and Banker, 1994). Microtubules are inherently polarized structures, with the α-tubulin subunits present at the slow growing “minus end” and the β-tubulin subunits at the fast growing “plus end” of the tube (Allen and Borisy, 1974) (Figure 1). Microtubules in the axon of a neuron display uniform polarity, with their plus ends oriented toward synapses, whereas those in the dendrites display mixed polarity (Burton and Paige, 1981; Baas et al., 1988, 1989; Dombeck et al., 2003; Stepanova et al., 2003, 2010). Microtubules contribute to the overall polarity by being involved in axon specification, an important early event in establishing neuronal polarity (Burton and Paige, 1981; Heidemann et al., 1981; Witte et al., 2008; Hoogenraad and Bradke, 2009). During this process, microtubules in a single neurite reorganize their mixed polarity orientation to exclusively plus-end distal microtubules, thus breaking cellular symmetry and marking distinct axonal and dendritic domains (Baas et al., 1989; Horton and Ehlers, 2003). This intrinsic polarity of microtubules plays a critical role in the precise trafficking of a variety of cargo within a neuron (Zheng et al., 2008; Maday et al., 2014). Disorganization of microtubule polarity can result in incorrect localization of cargo. For example, in Drosophila, loss of the Dynein motor or microtubule associated LIS1 (both implicated in lissencephaly), results in axons containing both plus- and minus end-distal microtubules, leading to mis-trafficking of dendritic proteins into axons (Liu et al., 2000; Vallee and Tsai, 2006; Zheng et al., 2008; Reiner, 2013). Similarly, in C. elegans, disruption of microtubule polarity causes mislocalization of an axonal Kinesin-3 motor, synaptic vesicles (Maniar et al., 2012), and dense core vesicles (Goodwin et al., 2012) to dendrites, leading to non-specific targeting of axonal proteins. Recovery from complete axon loss after axotomy, in cultured neurons, requires microtubule reorganization and polarity reversal in one of the remaining neurites to re-specify the axon (Gomis-Rüth et al., 2008). An in vivo study using Drosophila shows that this change in microtubule polarity, and consequent re-specification of the axon, is preceded by an increase in the polymerization of microtubules (Stone et al., 2010). In contrast, axon specification is preceded by an increase in stability of microtubules in a single neurite (Witte et al., 2008). Additionally, increasing microtubule stability by Taxol treatment can result in the formation of multiple neuronal processes that exhibit characteristics of a typical axon, viz. localization of axonal markers Tau and synapsin-1 (Witte et al., 2008). These studies show that microtubule dynamics contribute to changes in microtubule orientation and consequently some aspects of neuronal polarity. Altered protein distribution arising from the changes in polarity can be detrimental for the neuron, thereby contributing to disease symptoms.
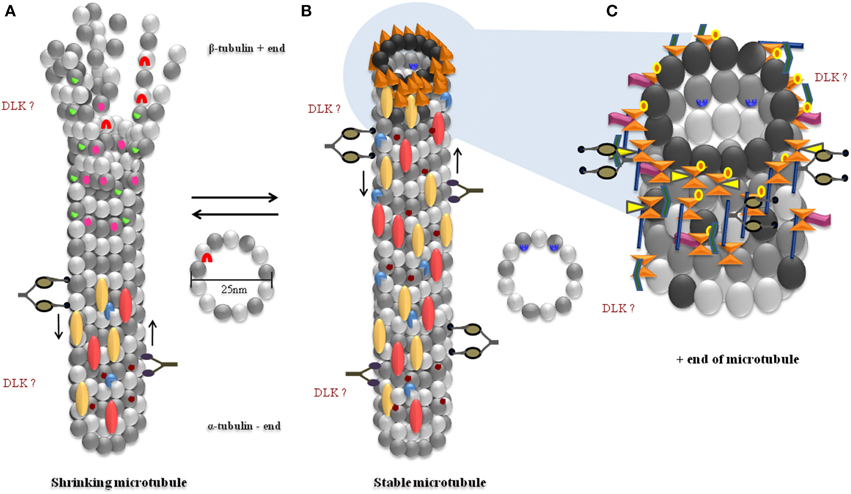
Figure 1. Proteins and modifications associated with unstable and stable microtubules. (A) Shrinking microtubules disassemble from their plus ends, lose their MAPs, are not acetylated but are tyrosinated. LRRK2 binds to the luminal side of β-Tubulin and prevents acetylation of microtubules. (B) Stable microtubules have a large complement of proteins associated with them, are not tyrosinated, are acetylated and have GTP-capped ends with multiple proteins. (C) Plus-ends of microtubules have several +TIP proteins. Several bind to the plus end binding EB proteins and GTP bound β-Tubulin. The precise location of DLK binding on microtubules is unknown. α-Tubulin (), β-Tubulin (
), GTP bound β-Tubulin (
), α/β heterodimer (
), Kinesin motor (
), Dynein motor (
). Present on less stable microtubules: Tyrosination (
), Gαs (
), LRRK2 (
). Present on stable microtubules: MAP1 (axon and dendrites) (
), TAU (axon) (
), MAP2 (
), Acetylation (
), Gβγ (
), +end binding proteins (
). Proteins present on the +end or fast growing end of microtubules (+TIPs): EBP1/2/3 (
), CLIP170 (
), CLASPS (
), APC (
), RHO GEF2 (
), MACF (
).
Microtubule Dynamics Maintain Neuronal Morphology
Neuronal morphology and formation of specific connections to both its pre-and post-synaptic partner cells are critical for neuron function (Morales et al., 2002; Chen et al., 2006; Lewcock et al., 2007). Several studies also show that the morphology of the neuron can be influenced by microtubules. For instance, specific mutations in the C. elegans mec-7 β-tubulin lead to ectopic neurite outgrowth that is suppressed upon treatment with the microtubule-destabilizing drug colchicine (Kirszenblat et al., 2013). Hyperactivation of the Notch signaling pathway is also able to regulate axonal morphology, resulting in thicker neurites, fewer branches, and loss of synaptic varicosity, thought to arise from the observed hyperstabilization of microtubules (Ferrari-Toninelli et al., 2008; Bonini et al., 2013). Such hyperstabilization likely arises from the Notch-induced increase in acetylation and polyglutamylation of α-tubulins, both of which are markers of stable microtubules (Ferrari-Toninelli et al., 2008). Notch is also thought to increase microtubule stability by reducing the expression of the microtubule severing enzyme Spastin (Ferrari-Toninelli et al., 2008). The downstream effectors that mediate such effects of Notch signaling have not been identified. However, a downstream kinase Abl known to interact with microtubules (Miller et al., 2004) and required for axon growth (Giniger, 1998), is a potential candidate that could mediate Notch dependent microtubule effects. These studies suggest that hyper-stable microtubules might be detrimental to neuronal morphology.
Likewise, unstable microtubules also influence neuronal morphology. Unstable microtubules and microtubule-actin interactions are essential for formation of neuronal branches (Dent and Kalil, 2001). Plus-end-binding proteins (+TIPs), such as CLASPs, APC, and MACF that bind to polymerizing microtubules and stabilize it, are required for the microtubule-actin interactions that promote axon elongation or branching (Leung et al., 1999; Zhou et al., 2004; Kornack and Giger, 2005; Watanabe et al., 2009). Therefore, circumstances that elevate dynamic microtubule pools promote axon branching. For example, inhibition of microtubule stabilizing proteins such as Tau result in increased axonal branching (Yu et al., 2008). In addition, the microtubule-associated ubiquitin ligase Phr1 mutants, in mouse, and zebrafish, have axons with sharp kinks, abnormally bent growth cones and mistargeting of motor neurons to inappropriate tissues (Lewcock et al., 2007; Hendricks and Jesuthasan, 2009). One of these studies shows that these axonal abnormalities were greatly reduced by taxol-induced stabilization of microtubules, suggesting that axonal morphology itself might depend on microtubule stability (Lewcock et al., 2007). This hypothesis is also supported by the observed change in dendrite morphology from spine-like to more filopodia-like structures upon destabilization of microtubules in hippocampal CA1 neurons using nocadazole (Jaworski et al., 2009).
All of the above studies also describe cellular processes that are disrupted when microtubule stability is altered; such as axon path-finding and innervation (Lewcock et al., 2007), synaptic plasticity (Jaworski et al., 2009) and regenerative capacity after injury (Kirszenblat et al., 2013). These studies suggest that disproportionate levels of hyper-stable or very unstable microtubules are both detrimental to neuronal morphology.
Microtubules are Highways for Cargo Transport
Microtubules serve as the sub-cellular roads for long-distance transport where they act as “tracks” on which motor proteins such as kinesin and dynein transport multiple organelles and macromolecules (Gunawardena and Goldstein, 2004; Hirokawa and Noda, 2008). The Kinesin family of motors move toward the plus ends of microtubules while Dynein motors walk toward the minus end (Vale et al., 1985a,b; Paschal and Vallee, 1987; Wade, 2009) (Figure 1). Microtubule-mediated fast axonal transport occurs at a speed of ~50–200 mm/day and delivers axonal cargo such as Amyloid Precursor Protein (APP), synaptic proteins, synaptic vesicles, and dense core vesicles (Maday et al., 2014). Microtubule-dependent slow axonal transport occurs at a speed of ~0.2–10 mm/day and carries cytoskeletal proteins such as Tubulin and cytosolic proteins such as Synapsin (Maday et al., 2014; Roy, 2014). Lack of fast axonal transport is known to cause developmental and functional defects in the motor neurons of Drosophila (Hurd and Saxton, 1996), in PLL axons of zebrafish (Lyons et al., 2008), in the spinal nerves and peripheral axons in mice (Warita et al., 1999), and in multiple neurons of C. elegans (Hall and Hedgecock, 1991).
Stable microtubules are an integral part of maintaining axonal transport. When cultured neurons are treated with Parkinsonism-inducing neurotoxin 1-methyl-4-phenylpiridinium (MPP+), there is reduction in microtubule dynamics, perhaps accounting, in part, for the observed mitochondrial accumulation along axons (Cartelli et al., 2010). Another study showed that hyper-dynamic microtubules occur very early, before observable impairment of axonal transport, in a mouse disease model of ALS (Fanara et al., 2007). Further, drug-induced stabilization of microtubules was able to restore normal axonal transport, suggesting that axonal transport defects in this model are a consequence of increased microtubule dynamics (Fanara et al., 2007) (Figures 2A,B). These examples suggest that altered microtubule dynamics are detrimental to axonal transport. This raises the intriguing possibility that several of the observed transport defects, in neurodegenerative diseases (De Vos et al., 2008; Millecamps and Julien, 2013), might arise at least in some instances from altered microtubule dynamics.
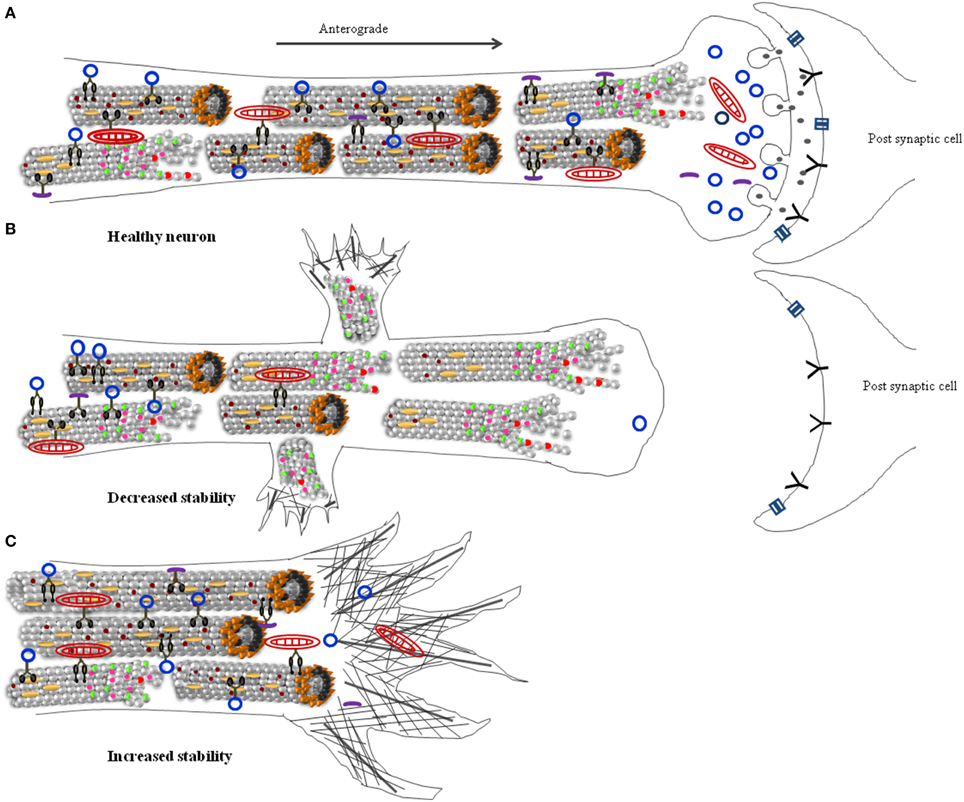
Figure 2. Ratios of stable and dynamic microtubule alter neuronal structure and function. (A) Healthy neurons have short and long, stable, and dynamic microtubules (B) Increased numbers of dynamic microtubules lead to increased neuronal branching, synapse retraction, and reduced axonal transport. This eventually can lead to dying-back neuropathy. (C) Hyperstable microtubules increase the diameter of the neuron, inhibit neurite outgrowth, and inhibit neuronal branching. Stable microtubule (), depolymerzing microtubule (
), microtubule associated proteins (
), microtubule plus end binding proteins (
), mitochondria (
), membranous cargo (
), non-membranous cargo (
), Kinesin motor (
), Dynein motor (
), Actin filaments (
), Actin bundles (
), neurotransmitters (
), channels (
), neurotransmitter receptors (
).
Microtubules as Regulators of Signaling and Gene Expression
It has been suggested recently that neuronal microtubules, through their interactions with G-proteins (Wittmann and Waterman-Storer, 2001), plus-end-tracking proteins (+TIPs) (Akhmanova and Steinmetz, 2008) and minus-end-targeting proteins (-TIPs) (Akhmanova and Hoogenraad, 2015), might act as carriers of information (Dent and Baas, 2014) (Figure 1). For example, disruption of microtubules leads to activation of microtubule-bound RHGF-1, a PDZ Rho GEF, and causes retraction of a collateral neuronal branch in C. elegans (Chen et al., 2014). This RHGF-1 dependent remodeling of neuronal morphology was shown to depend on the MAPKKK, DLK-1 (Chen et al., 2014). This finding is consistent with localization of DLK at the tips of microtubules in cultured cortical neurons (Hirai et al., 2002). DLK activity is also necessary to initiate repair processes after axonal injury (Yan et al., 2009; Ghosh-Roy et al., 2010; Xiong et al., 2010; Chen and Chisholm, 2011), and it has been suggested that cytoskeletal damage alone is potentially sufficient to trigger DLK activity, perhaps following DLK's release from microtubules (Valakh et al., 2013, 2015). Depolymerization of microtubules by chemical treatment, as well as decrease in microtubule number through mutations in mec-12 α-tubulin and mec-7 β-tubulin, cause reduction in expression of proteins through the DLK/p38 MAPK pathway in the touch receptor neurons of C. elegans (Bounoutas et al., 2011). Thus, microtubule numbers and dynamics appear to regulate DLK-dependent signaling in neurons.
Other signaling molecules, such as the heterotrimeric Gsα, strongly interact with α-tubulin (Layden et al., 2008). Gsα activates tubulin GTPase, leading to destabilization of microtubules (Roychowdhury et al., 1999; Schappi et al., 2014). Likewise, binding of Leucine-rich repeat kinase 2 (LRRK2) also regulates stability of microtubules by modulating tubulin acetylation (Gandhi et al., 2008; Law et al., 2014). LRRK2 is known to participate in several signaling cascades that modulate neurite growth, vesicle trafficking, endocytosis, and autophagy, although it is unclear whether all these functions require the microtubule-bound form of LRRK2 (Berwick and Harvey, 2011). Thus, signaling and scaffolding molecules can control microtubule dynamics; and microtubules themselves could function as signaling hubs by sequestering kinases such as DLK and LRRK2.
Microtubule Assembly, Stability, and Dynamics
Despite the importance of microtubules, their assembly within neurons is poorly understood. Two models have been proposed for the origin of axonal microtubules: (i) microtubules are clipped off from the centrosome by the severing enzyme, Katanin, before entering the axon (Baas et al., 2005) and (ii) multiple nucleation centers are created along the neuronal process from the severing of existing axonal microtubules (Roll-Mecak and Vale, 2006). Tubulin oligomers transported along the axon via slow transport (Terada et al., 2000; Wang and Brown, 2002) are eventually likely to be incorporated into microtubules. Once assembled, microtubules are dynamic, with cyclic phases of both growth and shrinkage. This behavior of microtubules has been termed “dynamic instability” (Box 1). The plus ends of microtubules are much more dynamic than their minus ends (Walker et al., 1988).
Box 1. Experimental methods to monitor dynamic microtubules
A key insight into microtubule polymerization came in the 1980s when coexistence of growing as well as shrinking populations of microtubules were observed in vitro by combining biochemical methods, immunofluorescence, and electron microscopy. This process was termed “dynamic instability” and was further confirmed in cells using similar methods (Cassimeris et al., 1988; Mitchison and Kirschner, 1988; Okabe and Hirokawa, 1988; Sammak and Borisy, 1988).
After these early studies, imaging techniques carried out at the time scales of 2 s or faster have been critical in observing microtubule dynamics in vivo (Shelden and Wadsworth, 1993). A major advance in the field was the development of fluorescent speckle microscopy (FSM). Here low concentrations of fluorescently labeled tubulin subunits were incorporated into a microtubule (Waterman-Storer and Salmon, 1998). The speckles provided markers along microtubules whose positions did not change unless they grew or shrunk. This technique unambiguously permitted tracking assembly and disassembly of microtubules.
The identification of microtubule end binding EB proteins now conveniently allows investigators to track the growing ends of microtubules using EB-GFP fusions (Stepanova et al., 2003). However, this method only assesses growth of +ends and does not provide the detailed information obtained using FSM and hence gives an incomplete understanding of microtubule dynamics.
A recent advance combined an electrically tunable lens with fluorescent microscopy thus providing rapid focusing (Nakai et al., 2015) which allows temporal resolution within 10 ms and a spatial resolution of 40 nm making it an attractive tool to assess microtubule dynamics in vivo. Such newer technologies may allow us to effectively monitor microtubule dynamics in healthy and diseased neurons.
Neurons possess more stable microtubules compared to other cell types (Okabe and Hirokawa, 1988; Seitz-Tutter et al., 1988; Stepanova et al., 2003). These stable microtubules have half-lives of several hours and co-exist with dynamic microtubules with half-lives of several minutes (Li and Black, 1996; Conde and Cáceres, 2009). A cap of non-hydrolyzed GTP Tubulin subunits at the growing end of a microtubule (Figures 1B,C) is thought to protect against depolymerization and stabilize the plus ends of microtubules (Carlier and Pantaloni, 1981; Mitchison and Kirschner, 1988). Another group of proteins that interacts with the fast growing ends of microtubules are the “plus end-tracking proteins” or +TIPs which include the cytoplasmic linker proteins (CLIPs), the CLIP-associated proteins (CLASPs), Adenomatous polyposis coli protein (APC), the Microtubule-actin crosslinking factor (MACF) and the end-binding (EB) proteins (Akhmanova and Steinmetz, 2010) (Figure 1C). The EB proteins are now widely used to track growing ends of microtubules (Stepanova et al., 2003; Akhmanova and Steinmetz, 2010) (Box 1). Further, several +TIP proteins regulate different aspects of microtubule dynamics (Table 1), including promoting interaction of microtubule ends with actin and other cellular structures (Akhmanova and Steinmetz, 2010).
Lengths of neuronal microtubules are also known to vary and are controlled by the severing enzymes Spastin and Katanin, that can cut microtubules throughout their length (McNally and Vale, 1993; Karabay et al., 2004; Yu et al., 2005, 2008). Katanin levels are higher throughout the axon during neuronal development but the enzyme is mostly restricted to the cell body once axons reach their targets (Karabay et al., 2004; Yu et al., 2005). Katanin is therefore likely to sever microtubules, generating nucleating centers or tubulin subunits required for the process of axon extension during neuronal development. On the other hand, Spastin is concentrated at sites where axons branch (Yu et al., 2008), consistent with the presence of shorter microtubules at such locations (Yu et al., 1994). Further, certain MAPs may regulate the severing properties of enzymes, for example microtubule-bound Tau renders the microtubules more resistant to severing by Katanin but not Spastin (Qiang et al., 2006; Yu et al., 2008). Together these processes lead to axonal microtubules of various lengths. In several organisms these lengths vary from one micrometer to over hundred micrometers long, averaging around 100 μm in vertebrate neurons (Bray and Bunge, 1981; Chalfie and Thomson, 1982; Letourneau, 1982; Yu et al., 2007). An increased proportion of unstable and short microtubules might lead to varied neuronal defects such as impaired axonal transport and increased branching (Figure 2B) (Dent et al., 2003; Yu et al., 2008).
Role of Tubulin Subunits in Microtubule Dynamics
Mutations in α- and β-Tubulin subunits can themselves influence the stability and dynamics of microtubules. Both α and β-Tubulins have an N-terminal domain, an intermediate domain and a C-terminal domain. Residues of the N-terminal domain are important for protein folding and conformation and contain the guanine nucleotide binding region (Downing and Nogales, 1998; Löwe et al., 2001). The residues in the C-terminal domain bind both MAPs and motor proteins (Littauer et al., 1986; Löwe et al., 2001). In C. elegans, approximately fifty mutations have been mapped to the mec-7 β-tubulin gene and several to the mec-12 α-tubulin gene (Savage et al., 1989, 1994; Fukushige et al., 1999; Baran et al., 2010; Bounoutas et al., 2011; Kirszenblat et al., 2013; Hsu et al., 2014). A missense mutation in the intermediate domain of MEC-7 induces ectopic neurite outgrowth, causes mis-localization of synaptic vesicles and reduces regeneration after injury (Kirszenblat et al., 2013). A recent study in C. elegans has also shown that a missense mutation in the C-terminal of MEC-12 α-tubulin leads to unbundling of microtubules and an increased affinity to Dynein that likely leads to the observed cargo mis-trafficking defects (Hsu et al., 2014). In both the above studies, lesions in distinct tubulin domains share similar cellular phenotypes. Several mutations in tubulin genes of C. elegans and other model systems map to many different locations along the proteins and lead to a multiplicity of neuronal phenotypes (Table 2). As yet it is unclear whether mutations in specific tubulin domains are associated with a single or specific constellation of phenotypes. Nonetheless, since mutations in all three domains of the tubulins have been associated with neuronal defects (Table 2), each protein domains appears to be critical for microtubule function. The aspect of microtubule structure or function altered in these cases is not uniformly well documented.
Specific mutations in a human β-tubulin, TUBB3, have been shown in vitro and in cultured cells to impair α/β heterodimer formation leading to lower stability of microtubules (Poirier et al., 2010). These and other β-tubulin mutations have been associated with malformations in cortical development (MCD) in human patients and cortical neuron migration and axonal guidance defects in mouse models (Poirier et al., 2007, 2010; Jaglin et al., 2009). Such studies show that mutations in α- and β-tubulin that alter microtubule dynamics can lead to neurodevelopmental defects. Mutations in tubulin genes have also been documented in patients with many types of neurodegenerative diseases, although their relationship to microtubule dynamics or stability is yet unclear (Table 3).
Post-translation Modifications as Markers of Microtubule Stability
The α and β-Tubulin subunits themselves are modified by tyrosination, acetylation, and polyamination (Hammond et al., 2008; Fukushima et al., 2009; Janke and Chloë Bulinski, 2011). Some of these post-translational modifications mark stable microtubules (Westermann and Weber, 2003; Peris et al., 2006, 2009; Ikegami and Setou, 2010). For instance, stable microtubules are usually detyrosinated and acetylated (Fukushima et al., 2009). Detyrosination reduces microtubule depolymerisation (Peris et al., 2009) and detyrosinated microtubules are enriched in axons suggesting that axons contain more stable microtubules compared to dendrites (Witte et al., 2008; Hammond et al., 2010). Studies performed in cultured rat sympathetic neurons show that tyrosinated microtubules are enriched in the most proximal and distal regions of growing axons (Baas and Black, 1990; Brown et al., 1992). Further, a single microtubule can contain both stable and labile domains differing in their tyrosinated and acetylated Tubulin content (Baas and Black, 1990; Brown et al., 1992, 1993). Recently, polyamination of α- and β-Tubulins by a transglutaminase was also shown to promote axonal microtubule stabilization (Song et al., 2013). Such studies suggest that Tubulin modifications can affect both local stability within a microtubule and stability of microtubules along the axon. These islands of modifications within a microtubule may act as local disassembly brakes when microtubules undergo depolymerization. Additionally, the stable and dynamic regions of a microtubule could act as binding hotspots for specific microtubule-associated proteins such as LRRK2 to less stable microtubules (Law et al., 2014) and the motor Kinesin-1 to stable microtubules (Reed et al., 2006).
Effect of MAPs on Microtubule Dynamics
Much of the increased stability of microtubules in neurons is due to the presence of a large number of MAPs that shift the dynamics toward assembly and promote stability (Figure 1B). These include classical MAPs such as Tau (MAPT), MAP1a, MAP1b, and MAP2 as well as STOP (Stable Tubule Only Protein), Doublecortin, and the microtubule crosslinking proteins Plakins/Plectins (Chapin and Bulinski, 1992; Matus, 1994; Bosc et al., 1996; Horesh et al., 1999; Leung et al., 2002).
MAP2 and Tau are present exclusively on stable microtubules, with the former being found in dendrites and the latter largely in axons (Bernhardt and Matus, 1984; Dehmelt and Halpain, 2005). The well-studied AD-associated Tau protein in healthy neurons binds to and promotes microtubule polymerization and stability (Weingarten et al., 1975; Cleveland et al., 1977; Panda et al., 2003). A recent study reported a novel function of Tau as a recruiter of EB proteins to microtubule plus-ends, further suggesting, that Tau might contribute to microtubule stability at the +end via EB proteins (Sayas et al., 2015). Mutational studies have shown that MAPs are important for maintaining neuronal morphology. For example, loss of MAP1A shows decreased density of microtubules, abnormal focal swellings in dendrites and degeneration of Purkinje neurons in mice (Liu et al., 2015). MAP1B deficient mice neurons show that this protein sequesters microtubule end-binding proteins EB1 or EB3. This sequestration prevents hyper-stabilization of microtubules and halts axon growth (Tortosa et al., 2013). Thus, interaction of MAPs with microtubules modulates its dynamics and thereby, neuronal function.
Unstable Microtubules in Neurodegenerative Diseases
Cytoskeletal dysfunctions have been proposed as an underlying mechanism in many neurodegenerative diseases. AD, a widely studied neurodegenerative disease, has been associated with altered microtubule dynamics (Matsuyama and Jarvik, 1989). AD and other tauopathies are characterized by the presence of neurofibrillary tangles (NFTs), composed mainly of hyper-phosphorylated or modified Tau protein (Avila et al., 2004; Iqbal et al., 2010; Serrano-Pozo et al., 2011). Tau also contributes to neuronal morphology and axon outgrowth (Weingarten et al., 1975; Cleveland et al., 1977; Harada et al., 1994; Dawson et al., 2001; Panda et al., 2003; Yu et al., 2008; Sayas et al., 2015). Tau-mediated neurodegeneration could occur via defective axonal transport, faulty signaling or altered gene regulation and may not occur exclusively through microtubule effects (Trinczek et al., 1999; Shulman and Feany, 2003; Blard et al., 2007; Guthrie et al., 2009; Ambegaokar and Jackson, 2011; Morris et al., 2011; McCormick et al., 2013). Nonetheless, some pathological features in Tau-associated diseases could be a consequence of decreased microtubule stability.
A characteristic feature associated with AD is the accumulation of aluminum (Matsuyama and Jarvik, 1989; Walton, 2013) which, when incorporated in microtubules, make them more sensitive to depolymerization (Walton, 2006, 2009). The late onset of AD symptoms can also be attributed to the gradual accumulation of aluminum to levels toxic enough to tip the balance toward microtubule depolymerization and thus possibly neuron degeneration. Similarly, Parkin, an E3 ubiquitin ligase linked to PD, strongly binds to α/β Tubulin heterodimers and stabilizes microtubules (Ren et al., 2003; Yang et al., 2005). Reduction in neurite length, number of neurite branches and synaptic terminals, seen in PD patients, has been attributed to increased microtubule depolymerization in the absence of functional Parkin (Ren et al., 2015). Early loss of neurites, with delayed damage to the soma or “dying back axonopathy,” is a common feature of several neurodegenerative diseases (Stokin et al., 2005; Goto et al., 2009; Dadon-Nachum et al., 2010). Studies in vertebrates and invertebrates show that disruption of pre-synaptic microtubules precedes synapse retraction and degeneration (Zhai et al., 2003; Luo and O'Leary, 2005; Pielage et al., 2005; Stephan et al., 2015). Neurotoxins that lead to Parkinsonism, such as MPP+ (Cappelletti et al., 2005) and 6-hydroxydopamine (Patel and Chu, 2014), also alter microtubule dynamics, causing a decrease in length and number of microtubules and shortening of neurite. Reduction in microtubule stability also underlies the behavioral and axonal transport defects seen in PD-associated mutations in Leucine Rich Repeat Kinase 2 (LRRK2) (Godena et al., 2014). These examples suggest, that decreased stability of microtubules is a common feature in many neurodegenerative diseases and could initiate “dying-back” of the axon (Figure 2B).
Hyper-stabilized Microtubules in Neurodegeneration
Although a major fraction of microtubules in differentiated neurons is stable, pools of dynamic microtubules undergoing polymerization and depolymerization are also present (Hu et al., 2008). Stable microtubule pools may be important for maintaining neuronal morphology and synaptic integrity (Lewcock et al., 2007; Jaworski et al., 2009); however, dynamic pools within a neuron are important for turnover of microtubules as well as regeneration of neurons after injury (Stone et al., 2010; Chen and Chisholm, 2011; Ghosh-Roy et al., 2012). Microtubules stabilized using taxol, in healthy neurons, are known to increase the axonal diameter, enlarge growth cones and reduce neurite extension (Letourneau et al., 1986; Chuckowree and Vickers, 2003; Ferrari-Toninelli et al., 2008) (Figure 2C). Taxol stabilizes microtubules and can arrest mitosis and induce apoptosis (Jordan and Wilson, 1998). Therefore, it has been used as an effective treatment against cancer cells (Klauber et al., 1997; Jordan and Wilson, 1998; Dumontet and Sikic, 1999). However, taxol and other microtubule-stabilizing agents used as anti-cancer drugs show adverse effects on the peripheral nervous system in clinical studies. Such drugs have been shown to induce degeneration and fragmentation of sensory axons, reduce axonal length and also reduce axonal transport in animal models (Cavaletti et al., 1995; Lee and Swain, 2006; Scripture et al., 2006; Scuteri et al., 2006; Gornstein and Schwarz, 2014).
Genetic mutations in the microtubule-severing enzyme Spastin, is most commonly associated with hereditary spastic paraplegia (HSP) (Hazan et al., 1999). Spastin loss of function results in local accumulation of detyrosinated microtubules (Tarrade et al., 2006) and reduced number of dynamic plus-ends marked by EB3 along the axon shaft (Fassier et al., 2013). These mutations also results in axonal swellings which can be rescued by treatment with microtubule-destabilizing drugs such as Nocodazole (Fassier et al., 2013). This hyper-stability of microtubules likely triggers the progressive degeneration of corticospinal tracts in the Spastin-dependent HSP cases (Hazan et al., 1999; Evans et al., 2005). Collectively these studies demonstrate that reduced dynamics of microtubules is also detrimental to neuronal health.
Microtubule Dynamics is Necessary for Neuronal Function
Many neurodegenerative diseases are also associated with memory loss (Sagar et al., 1988; Levy et al., 2002; Walsh and Selkoe, 2004; Yamasaki et al., 2007; Shankar et al., 2008). Behavioral paradigms that lead to memory formation also show increase in dynamic microtubules that allows for the necessary synaptic plasticity (Gu et al., 2008; Jaworski et al., 2009; Fanara et al., 2010). Likewise, stabilizing microtubules using taxol prevents memory formation in mice (Atarod et al., 2015). One early symptom in AD is impairment in memory formation while loss of long-term memory appears in more advanced stages of the disease (Selkoe, 2002; Celone et al., 2006). These studies suggest that disruption of microtubule dynamics could be an important parameter, leading to an inability to form new memories as seen in the early stages of some neurodegenerative diseases.
Perspective: Microtubule Dynamics and Cellular Lingchi?
Given the critical role of microtubules in a variety of cellular processes in the neuron, several of which have been described above, it is not very surprising that this polymer shows changes in multiple neurodegenerative diseases such as AD, PD, Charcot Marie Tooth (CMT) etc. (Evans et al., 2005; Bunker et al., 2006; Gillardon, 2009; Tanabe and Takei, 2009; Cartelli et al., 2010; Tischfield et al., 2010). Amongst the many changes in microtubules that can occur, we think that change in dynamics is a significant “intermediate” phenotype that should be investigated. Microtubule dynamics, and conversely their stability, probably result from the sum of multiple microtubule binding proteins and modifications of α and β-Tubulins. The number of stable and dynamic microtubules has the potential to change a variety of steps (hence Lingchi) including the number of signaling hubs, morphology of the neuron as well as transport rates (Figure 2). Such changes could cumulatively lead to irreversible effects over the time scales of years, often the typical progression time scales in several neurodegenerative diseases.
Recognizing the importance of microtubule dynamics as an intermediate step in disease progression, some studies have focused on increasing stability of microtubules as a therapeutic target in treating neurodegenerative diseases. Axonal transport has been improved by stabilizing or destabilizing microtubules in some models of neurodegenerative disease (Fanara et al., 2007; Cartelli et al., 2010; Zhang et al., 2012; Fassier et al., 2013) and has been suggested as a therapeutic intervention (Ballatore et al., 2012; Brunden et al., 2014). Additionally, targeting Notch signaling as a microtubule stabilizer has also been proposed as therapeutic treatment for neurodegenerative conditions (Bonini et al., 2013). These studies suggest that stabilizing microtubules will improve disrupted axonal transport that could be critical in neurodegenerative disease progression.
However, what appears essential for neuronal function, is not merely stable microtubules but the right balance between stable and dynamic microtubules. This likely impacts numerous cellular processes, not restricted merely to axonal transport (Figure 2). For example, during neuronal polarization, a single neurite, which would be the future axonal process, shows increased microtubule stability (Witte et al., 2008). Moreover, shifting the dynamics toward greater stability by application of taxol results in multiple axons (Witte et al., 2008). The ratio of stable to dynamic microtubules also appears to be critical in allowing neurons to maintain their synaptic plasticity and form new memories (Jaworski et al., 2009; Fanara et al., 2010). In mouse models of neurodegenerative diseases like CMT, it was shown that the disease pathology has two distinct temporal phases, with an early pre-symptomatic phase showing hyper-stable microtubules followed by a later symptomatic phase, where microtubules are unstable (Almeida-Souza et al., 2011a,b; d'Ydewalle et al., 2011). This suggests that one way to slow down disease progression could be to provide temporally defined, correct dosage of agents that control the balance of stable and dynamic microtubules in a disease-specific manner. Additionally, systematic temporal studies examining microtubule dynamics in both cell and animal models, combined with behavioral assays in animal models, may allow investigators to gain a deeper understanding of how the complex cellular roles of microtubules contribute to neurodegenerative disease progression.
Conflict of Interest Statement
The authors declare that the research was conducted in the absence of any commercial or financial relationships that could be construed as a potential conflict of interest.
Acknowledgments
We thank Amruta Vasudevan and Madhushree Kamak for comments on the manuscript. Research in the SPK lab is supported by HHMI-IECS to SPK and TIFR. NR is supported by HHMI-IECS to SPK. JD is supported by CSIR-UGC.
References
Akhmanova, A., and Hoogenraad, C. C. (2005). Microtubule plus-end-tracking proteins: mechanisms and functions. Curr. Opin. Cell Biol. 17, 47–54. doi: 10.1016/j.ceb.2004.11.001
Akhmanova, A., and Hoogenraad, C. C. (2015). Microtubule minus-end-targeting proteins. Curr. Biol. 25, R162–R171. doi: 10.1016/j.cub.2014.12.027
Akhmanova, A., and Steinmetz, M. O. (2008). Tracking the ends: a dynamic protein network controls the fate of microtubule tips. Nat. Rev. Mol. Cell Biol. 9, 309–322. doi: 10.1038/nrm2369
Akhmanova, A., and Steinmetz, M. O. (2010). Microtubule +TIPs at a glance. J. Cell Sci. 123, 3415–3419. doi: 10.1242/jcs.062414
Allen, C., and Borisy, G. G. (1974). Structural polarity and directional growth of microtubules of Chlamydomonas flagella. J. Mol. Biol. 90, 381–402. doi: 10.1016/0022-2836(74)90381-7
Almeida-Souza, L., Asselbergh, B., d'Ydewalle, C., Moonens, K., Goethals, S., de Winter, V., et al. (2011b). Small heat-shock protein HSPB1 mutants stabilize microtubules in charcot-marie-tooth neuropathy. J. Neurosci. 31, 15320–15328. doi: 10.1523/JNEUROSCI.3266-11.2011
Almeida-Souza, L., Timmerman, V., and Janssens, S. (2011a). Microtubule dynamics in the peripheral nervous system. Bioarchitecture 1, 267–270. doi: 10.4161/bioa.1.6.19198
Ambegaokar, S. S., and Jackson, G. R. (2011). Functional genomic screen and network analysis reveal novel modifiers of tauopathy dissociated from tau phosphorylation. Hum. Mol. Genet. 20, 4947–4977. doi: 10.1093/hmg/ddr432
Arnold, S. E., Toledo, J. B., Appleby, D. H., Xie, S. X., Wang, L.-S., Baek, Y., et al. (2013). A comparative survey of the topographical distribution of signature molecular lesions in major neurodegenerative diseases. J. Comp. Neurol. 521, 4339–4355. doi: 10.1002/cne.23430
Atarod, D., Eskandari-Sedighi, G., Pazhoohi, F., Karimian, S. M., Khajeloo, M., and Riazi, G. H. (2015). Microtubule dynamicity is more important than stability in memory formation: an in vivo study - Springer. J. Mol. Neurosci. 56, 313–319. doi: 10.1007/s12031-015-0535-4
Avila, J., Lucas, J. J., Perez, M., and Hernández, F. (2004). Role of Tau protein in both physiological and pathological conditions. Physiol. Rev. 84, 361–384. doi: 10.1152/physrev.00024.2003
Baas, P. W., and Black, M. M. (1990). Individual microtubules in the axon consist of domains that differ in both composition and stability. J. Cell Biol. 111, 495–509. doi: 10.1083/jcb.111.2.495
Baas, P. W., Black, M. M., and Banker, G. A. (1989). Changes in microtubule polarity orientation during the development of hippocampal neurons in culture. J. Cell Biol. 109, 3085–3094. doi: 10.1083/jcb.109.6.3085
Baas, P. W., Deitch, J. S., Black, M. M., and Banker, G. A. (1988). Polarity orientation of microtubules in hippocampal neurons: uniformity in the axon and nonuniformity in the dendrite. Proc. Natl. Acad. Sci. U.S.A. 85, 8335–8339. doi: 10.1073/pnas.85.21.8335
Baas, P. W., Karabay, A., and Qiang, L. (2005). Microtubules cut and run. Trends Cell Biol. 15, 518–524. doi: 10.1016/j.tcb.2005.08.004
Baird, F. J., and Bennett, C. L. (2013). Microtubule defects & Neurodegeneration. J. Genet. Syndr. Gene Ther. 4:203. doi: 10.4172/2157-7412.1000203
Ballatore, C., Brunden, K. R., Huryn, D. M., Trojanowski, J. Q., Lee, V. M.-Y., and Smith, A. B. (2012). Microtubule stabilizing agents as potential treatment for Alzheimer's disease and related neurodegenerative tauopathies. J. Med. Chem. 55, 8979–8996. doi: 10.1021/jm301079z
Baran, R., Castelblanco, L., Tang, G., Shapiro, I., Goncharov, A., and Jin, Y. (2010). Motor neuron synapse and axon defects in a C. elegans alpha-tubulin mutant. PLoS ONE 5:e9655. doi: 10.1371/journal.pone.0009655
Beharry, C., Cohen, L. S., Di, J., Ibrahim, K., Briffa-Mirabella, S., and Alonso Adel, C. (2014). Tau-induced neurodegeneration: mechanisms and targets. Neurosci. Bull. 30, 346–358. doi: 10.1007/s12264-013-1414-z
Bernhardt, R., and Matus, A. (1984). Light and electron microscopic studies of the distribution of microtubule-associated protein 2 in rat brain: a difference between dendritic and axonal cytoskeletons. J. Comp. Neurol. 226, 203–221. doi: 10.1002/cne.902260205
Berwick, D. C., and Harvey, K. (2011). LRRK2 signaling pathways: the key to unlocking neurodegeneration? Trends Cell Biol. 21, 257–265. doi: 10.1016/j.tcb.2011.01.001
Blard, O., Feuillette, S., Bou, J., Chaumette, B., Frébourg, T., Campion, D., et al. (2007). Cytoskeleton proteins are modulators of mutant tau-induced neurodegeneration in Drosophila. Hum. Mol. Genet. 16, 555–566. doi: 10.1093/hmg/ddm011
Bonini, S. A., Ferrari-Toninelli, G., Montinaro, M., and Memo, M. (2013). Notch signalling in adult neurons: a potential target for microtubule stabilization. Ther. Adv. Neurol. Disord. 6, 375–385. doi: 10.1177/1756285613490051
Bosc, C., Cronk, J. D., Pirollet, F., Watterson, D. M., Haiech, J., Job, D., et al. (1996). Cloning, expression, and properties of the microtubule-stabilizing protein STOP. Proc. Natl. Acad. Sci. U.S.A. 93, 2125–2130. doi: 10.1073/pnas.93.5.2125
Bounoutas, A., Kratz, J., Emtage, L., Ma, C., Nguyen, K. C., and Chalfie, M. (2011). Microtubule depolymerization in Caenorhabditis elegans touch receptor neurons reduces gene expression through a p38 MAPK pathway. Proc. Natl. Acad. Sci. U.S.A. 108, 3982–3987. doi: 10.1073/pnas.1101360108
Bray, D., and Bunge, M. B. (1981). Serial analysis of microtubules in cultured rat sensory axons. J. Neurocytol. 10, 589–605. doi: 10.1007/BF01262592
Brown, A., Li, Y., Slaughter, T., and Black, M. M. (1993). Composite microtubules of the axon: quantitative analysis of tyrosinated and acetylated tubulin along individual axonal microtubules. J. Cell Sci. 104, 339–352.
Brown, A., Slaughter, T., and Black, M. M. (1992). Newly assembled microtubules are concentrated in the proximal and distal regions of growing axons. J. Cell Biol. 119, 867–882. doi: 10.1083/jcb.119.4.867
Brunden, K. R., Trojanowski, J. Q., Smith, A. B. III Lee, V. M.-Y., and Ballatore, C. (2014). Microtubule-stabilizing agents as potential therapeutics for neurodegenerative disease. Bioorg. Med. Chem. 22, 5040–5049. doi: 10.1016/j.bmc.2013.12.046
Bunker, J. M., Kamath, K., Wilson, L., Jordan, M. A., and Feinstein, S. C. (2006). FTDP-17 mutations compromise the ability of Tau to regulate microtubule dynamics in cells. J. Biol. Chem. 281, 11856–11863. doi: 10.1074/jbc.M509420200
Burton, P. R., and Paige, J. L. (1981). Polarity of axoplasmic microtubules in the olfactory nerve of the frog. Proc. Natl. Acad. Sci. U.S.A. 78, 3269–3273. doi: 10.1073/pnas.78.5.3269
Cappelletti, G., Surrey, T., and Maci, R. (2005). The parkinsonism producing neurotoxin MPP+ affects microtubule dynamics by acting as a destabilising factor. FEBS Lett. 579, 4781–4786. doi: 10.1016/j.febslet.2005.07.058
Carlier, M. F., and Pantaloni, D. (1981). Kinetic analysis of guanosine 5′-triphosphate hydrolysis associated with tubulin polymerization. Biochemistry 20, 1918–1924. doi: 10.1021/bi00510a030
Cartelli, D., Ronchi, C., Maggioni, M. G., Rodighiero, S., Giavini, E., and Cappelletti, G. (2010). Microtubule dysfunction precedes transport impairment and mitochondria damage in MPP+-induced neurodegeneration. J. Neurochem. 115, 247–258. doi: 10.1111/j.1471-4159.2010.06924.x
Cassimeris, L., Pryer, N. K., and Salmon, E. D. (1988). Real-time observations of microtubule dynamic instability in living cells. J. Cell Biol. 107, 2223–2231. doi: 10.1083/jcb.107.6.2223
Cavaletti, G., Tredici, G., Braga, M., and Tazzari, S. (1995). Experimental peripheral neuropathy induced in adult rats by repeated intraperitoneal administration of taxol. Exp. Neurol. 133, 64–72. doi: 10.1006/exnr.1995.1008
Cederquist, G. Y., Luchniak, A., Tischfield, M. A., Peeva, M., Song, Y., Menezes, M. P., et al. (2012). An inherited TUBB2B mutation alters a kinesin-binding site and causes polymicrogyria, CFEOM and axon dysinnervation. Hum. Mol. Genet. 21, 5484–5499. doi: 10.1093/hmg/dds393
Celone, K. A., Calhoun, V. D., Dickerson, B. C., Atri, A., Chua, E. F., Miller, S. L., et al. (2006). Alterations in memory networks in mild cognitive impairment and Alzheimer's disease: an independent component analysis. J. Neurosci. 26, 10222–10231. doi: 10.1523/JNEUROSCI.2250-06.2006
Chalfie, M., and Thomson, J. N. (1982). Structural and functional diversity in the neuronal microtubules of Caenorhabditis elegans. J. Cell Biol. 93, 15–23. doi: 10.1083/jcb.93.1.15
Chapin, S. J., and Bulinski, J. C. (1992). Microtubule stabilization by assembly-promoting microtubule-associated proteins: a repeat performance. Cell Motil. Cytoskeleton 23, 236–243. doi: 10.1002/cm.970230403
Chazaud, C., and Rossant, J. (2006). Disruption of early proximodistal patterning and AVE formation in Apc mutants. Development 133, 3379–3387. doi: 10.1242/dev.02523
Chen, B. L., Hall, D. H., and Chklovskii, D. B. (2006). Wiring optimization can relate neuronal structure and function. Proc. Natl. Acad. Sci. U. S. A. 103, 4723–4728. doi: 10.1073/pnas.0506806103
Chen, C.-H., Lee, A., Liao, C.-P., Liu, Y.-W., and Pan, C.-L. (2014). RHGF-1/PDZ-RhoGEF and retrograde DLK-1 signaling drive neuronal remodeling on microtubule disassembly. Proc. Natl. Acad. Sci. U.S.A. 111, 16568–16573. doi: 10.1073/pnas.1410263111
Chen, L., and Chisholm, A. D. (2011). Axon regeneration mechanisms: insights from C. elegans. Trends Cell Biol. 21, 577–584. doi: 10.1016/j.tcb.2011.08.003
Chuckowree, J. A., and Vickers, J. C. (2003). Cytoskeletal and morphological alterations underlying axonal sprouting after localized transection of cortical neuron axons in vitro. J. Neurosci. 23, 3715–3725.
Cleveland, D. W., Hwo, S.-Y., and Kirschner, M. W. (1977). Purification of tau, a microtubule-associated protein that induces assembly of microtubules from purified tubulin. J. Mol. Biol. 116, 207–225. doi: 10.1016/0022-2836(77)90213-3
Conde, C., and Cáceres, A. (2009). Microtubule assembly, organization and dynamics in axons and dendrites. Nat. Rev. Neurosci. 10, 319–332. doi: 10.1038/nrn2631
Craig, A. M., and Banker, G. (1994). Neuronal Polarity. Annu. Rev. Neurosci. 17, 267–310. doi: 10.1146/annurev.ne.17.030194.001411
Cueva, J. G., Hsin, J., Huang, K. C., and Goodman, M. B. (2012). Posttranslational acetylation of α-tubulin constrains protofilament number in native microtubules. Curr. Biol. 22, 1066–1074. doi: 10.1016/j.cub.2012.05.012
Cushion, T. D., Paciorkowski, A. R., Pilz, D. T., Mullins, J. G. L., Seltzer, L. E., Marion, R. W., et al. (2014). De novo mutations in the beta-tubulin gene TUBB2A cause simplified gyral patterning and infantile-onset epilepsy. Am. J. Hum. Genet. 94, 634–641. doi: 10.1016/j.ajhg.2014.03.009
d'Ydewalle, C., Krishnan, J., Chiheb, D. M., Van Damme, P., Irobi, J., Kozikowski, A. P., et al. (2011). HDAC6 inhibitors reverse axonal loss in a mouse model of mutant HSPB1-induced Charcot-Marie-Tooth disease. Nat. Med. 17, 968–974. doi: 10.1038/nm.2396
Dadon-Nachum, M., Melamed, E., and Offen, D. (2010). The “Dying-Back” phenomenon of motor neurons in ALS. J. Mol. Neurosci. 43, 470–477. doi: 10.1007/s12031-010-9467-1
Dawson, H. N., Ferreira, A., Eyster, M. V., Ghoshal, N., Binder, L. I., and Vitek, M. P. (2001). Inhibition of neuronal maturation in primary hippocampal neurons from τ deficient mice. J. Cell Sci. 114, 1179–1187.
Dehmelt, L., and Halpain, S. (2005). The MAP2/Tau family of microtubule-associated proteins. Genome Biol. 6:204. doi: 10.1186/gb-2004-6-1-204
Dent, E. W., and Baas, P. W. (2014). Microtubules in neurons as information carriers. J. Neurochem. 129, 235–239. doi: 10.1111/jnc.12621
Dent, E. W., and Kalil, K. (2001). Axon branching requires interactions between dynamic microtubules and actin filaments. J. Neurosci. 21, 9757–9769.
Dent, E. W., Tang, F., and Kalil, K. (2003). Axon guidance by growth cones and branches: common cytoskeletal and signaling mechanisms. Neuroscientist 9, 343–353. doi: 10.1177/1073858403252683
De Vos, K. J., Grierson, A. J., Ackerley, S., and Miller, C. C. J. (2008). Role of axonal transport in neurodegenerative diseases*. Annu. Rev. Neurosci. 31, 151–173. doi: 10.1146/annurev.neuro.31.061307.090711
Diamantopoulos, G. S., Perez, F., Goodson, H. V., Batelier, G., Melki, R., Kreis, T. E., et al. (1999). Dynamic localization of CLIP-170 to microtubule plus ends is coupled to microtubule assembly. J. Cell Biol. 144, 99–112. doi: 10.1083/jcb.144.1.99
Dombeck, D. A., Kasischke, K. A., Vishwasrao, H. D., Ingelsson, M., Hyman, B. T., and Webb, W. W. (2003). Uniform polarity microtubule assemblies imaged in native brain tissue by second-harmonic generation microscopy. Proc. Natl. Acad. Sci. U.S.A. 100, 7081–7086. doi: 10.1073/pnas.0731953100
Downing, K. H., and Nogales, E. (1998). Tubulin structure: insights into microtubule properties and functions. Curr. Opin. Struct. Biol. 8, 785–791. doi: 10.1016/S0959-440X(98)80099-7
Dumontet, C., and Sikic, B. I. (1999). Mechanisms of action of and resistance to antitubulin agents: microtubule dynamics, drug transport, and cell death. J. Clin. Oncol. 17, 1061–1061.
Encalada, S. E., and Goldstein, L. S. B. (2014). Biophysical challenges to axonal transport: motor-cargo deficiencies and neurodegeneration. Annu. Rev. Biophys. 43, 141–169. doi: 10.1146/annurev-biophys-051013-022746
Evans, K. J., Gomes, E. R., Reisenweber, S. M., Gundersen, G. G., and Lauring, B. P. (2005). Linking axonal degeneration to microtubule remodeling by Spastin-mediated microtubule severing. J. Cell Biol. 168, 599–606. doi: 10.1083/jcb.200409058
Fanara, P., Banerjee, J., Hueck, R. V., Harper, M. R., Awada, M., Turner, H., et al. (2007). Stabilization of hyperdynamic microtubules is neuroprotective in amyotrophic lateral sclerosis. J. Biol. Chem. 282, 23465–23472. doi: 10.1074/jbc.M703434200
Fanara, P., Husted, K. H., Selle, K., Wong, P.-Y. A., Banerjee, J., Brandt, R., et al. (2010). Changes in microtubule turnover accompany synaptic plasticity and memory formation in response to contextual fear conditioning in mice. Neuroscience 168, 167–178. doi: 10.1016/j.neuroscience.2010.03.031
Fassier, C., Tarrade, A., Peris, L., Courageot, S., Mailly, P., Dalard, C., et al. (2013). Microtubule-targeting drugs rescue axonal swellings in cortical neurons from spastin knockout mice. Dis. Model. Mech. 6, 72–83. doi: 10.1242/dmm.008946
Ferrari-Toninelli, G., Bonini, S. A., Bettinsoli, P., Uberti, D., and Memo, M. (2008). Microtubule stabilizing effect of notch activation in primary cortical neurons. Neuroscience 154, 946–952. doi: 10.1016/j.neuroscience.2008.04.025
Franker, M. A. M., and Hoogenraad, C. C. (2013). Microtubule-based transport – basic mechanisms, traffic rules and role in neurological pathogenesis. J. Cell Sci. 126, 2319–2329. doi: 10.1242/jcs.115030
Fukushige, T., Siddiqui, Z. K., Chou, M., Culotti, J. G., Gogonea, C. B., Siddiqui, S. S., et al. (1999). MEC-12, an alpha-tubulin required for touch sensitivity in C. elegans. J. Cell Sci. 112, 395–403.
Fukushima, N., Furuta, D., Hidaka, Y., Moriyama, R., and Tsujiuchi, T. (2009). Post-translational modifications of tubulin in the nervous system. J. Neurochem. 109, 683–693. doi: 10.1111/j.1471-4159.2009.06013.x
Gandhi, P. N., Wang, X., Zhu, X., Chen, S. G., and Wilson-Delfosse, A. L. (2008). The Roc domain of leucine-rich repeat kinase 2 is sufficient for interaction with microtubules. J. Neurosci. Res. 86, 1711–1720. doi: 10.1002/jnr.21622
Garcia, M. L., and Cleveland, D. W. (2001). Going new places using an old MAP: tau, microtubules and human neurodegenerative disease. Curr. Opin. Cell Biol. 13, 41–48. doi: 10.1016/S0955-0674(00)00172-1
Ghosh-Roy, A., Goncharov, A., Jin, Y., and Chisholm, A. D. (2012). Kinesin-13 and tubulin posttranslational modifications regulate microtubule growth in axon regeneration. Dev. Cell 23, 716–728. doi: 10.1016/j.devcel.2012.08.010
Ghosh-Roy, A., Wu, Z., Goncharov, A., Jin, Y., and Chisholm, A. D. (2010). Calcium and cyclic AMP promote axonal regeneration in Caenorhabditis elegans and require DLK-1 kinase. J. Neurosci. 30, 3175–3183. doi: 10.1523/JNEUROSCI.5464-09.2010
Gillardon, F. (2009). Leucine-rich repeat kinase 2 phosphorylates brain tubulin-beta isoforms and modulates microtubule stability – a point of convergence in Parkinsonian neurodegeneration? J. Neurochem. 110, 1514–1522. doi: 10.1111/j.1471-4159.2009.06235.x
Giniger, E. (1998). A role for Abl in notch signaling. Neuron 20, 667–681. doi: 10.1016/S0896-6273(00)81007-7
Glass, C. K., Saijo, K., Winner, B., Marchetto, M. C., and Gage, F. H. (2010). Mechanisms underlying inflammation in neurodegeneration. Cell 140, 918–934. doi: 10.1016/j.cell.2010.02.016
Godena, V. K., Brookes-Hocking, N., Moller, A., Shaw, G., Oswald, M., Sancho, R. M., et al. (2014). Increasing microtubule acetylation rescues axonal transport and locomotor deficits caused by LRRK2 Roc-COR domain mutations. Nat. Commun. 5:5245. doi: 10.1038/ncomms6245
Goedert, M., and Jakes, R. (2005). Mutations causing neurodegenerative tauopathies. Biochim. Biophys. Acta 1739, 240–250. doi: 10.1016/j.bbadis.2004.08.007
Gomis-Rüth, S., Wierenga, C. J., and Bradke, F. (2008). Plasticity of polarization: changing dendrites into axons in neurons integrated in neuronal circuits. Curr. Biol. 18, 992–1000. doi: 10.1016/j.cub.2008.06.026
Goodwin, P. R., Sasaki, J. M., and Juo, P. (2012). Cyclin-dependent kinase 5 regulates the polarized trafficking of neuropeptide-containing dense-core vesicles in Caenorhabditis elegans motor neurons. J. Neurosci. 32, 8158–8172. doi: 10.1523/JNEUROSCI.0251-12.2012
Gornstein, E., and Schwarz, T. L. (2014). The paradox of paclitaxel neurotoxicity: mechanisms and unanswered questions. Neuropharmacology 76, 175–183. doi: 10.1016/j.neuropharm.2013.08.016
Goto, A., Wang, Y.-L., Kabuta, T., Setsuie, R., Osaka, H., Sawa, A., et al. (2009). Proteomic and histochemical analysis of proteins involved in the dying-back-type of axonal degeneration in the gracile axonal dystrophy (gad) mouse. Neurochem. Int. 54, 330–338. doi: 10.1016/j.neuint.2008.12.012
Grigoriev, I., Gouveia, S. M., van der Vaart, B., Demmers, J., Smyth, J. T., Honnappa, S., et al. (2008). STIM1 is a MT-plus-end-tracking protein involved in remodeling of the ER. Curr. Biol. 18, 177–182. doi: 10.1016/j.cub.2007.12.050
Groden, J., Thliveris, A., Samowitz, W., Carlson, M., Gelbert, L., Albertsen, H., et al. (1991). Identification and characterization of the familial adenomatous polyposis coli gene. Cell 66, 589–600. doi: 10.1016/0092-8674(81)90021-0
Gu, J., Firestein, B. L., and Zheng, J. Q. (2008). Microtubules in dendritic spine development. J. Neurosci. 28, 12120–12124. doi: 10.1523/JNEUROSCI.2509-08.2008
Gunawardena, S., and Goldstein, L. S. B. (2004). Cargo-carrying motor vehicles on the neuronal highway: transport pathways and neurodegenerative disease. J. Neurobiol. 58, 258–271. doi: 10.1002/neu.10319
Guthrie, C. R., Schellenberg, G. D., and Kraemer, B. C. (2009). SUT-2 potentiates tau-induced neurotoxicity in Caenorhabditis elegans. Hum. Mol. Genet. 18, 1825–1838. doi: 10.1093/hmg/ddp099
Hall, D. H., and Hedgecock, E. M. (1991). Kinesin-related gene unc-104 is required for axonal transport of synaptic vesicles in C. elegans. Cell 65, 837–847. doi: 10.1016/0092-8674(91)90391-B
Hammond, J. W., Cai, D., and Verhey, K. J. (2008). Tubulin modifications and their cellular functions. Curr. Opin. Cell Biol. 20, 71–76. doi: 10.1016/j.ceb.2007.11.010
Hammond, J. W., Huang, C.-F., Kaech, S., Jacobson, C., Banker, G., and Verhey, K. J. (2010). Posttranslational modifications of tubulin and the polarized transport of Kinesin-1 in neurons. Mol. Biol. Cell 21, 572–583. doi: 10.1091/mbc.E09-01-0044
Harada, A., Oguchi, K., Okabe, S., Kuno, J., Terada, S., Ohshima, T., et al. (1994). Altered microtubule organization in small-calibre axons of mice lacking tau protein. Nature 369, 488–491. doi: 10.1038/369488a0
Hazan, J., Fonknechten, N., Mavel, D., Paternotte, C., Samson, D., Artiguenave, F., et al. (1999). Spastin, a new AAA protein, is altered in the most frequent form of autosomal dominant spastic paraplegia. Nat. Genet. 23, 296–303. doi: 10.1038/15472
Heidemann, S. R., Landers, J. M., and Hamborg, M. A. (1981). Polarity orientation of axonal microtubules. J. Cell Biol. 91, 661–665. doi: 10.1083/jcb.91.3.661
Hendricks, M., and Jesuthasan, S. (2009). PHR regulates growth cone pausing at intermediate targets through microtubule disassembly. J. Neurosci. 29, 6593–6598. doi: 10.1523/JNEUROSCI.1115-09.2009
Hinckelmann, M.-V., Zala, D., and Saudou, F. (2013). Releasing the brake: restoring fast axonal transport in neurodegenerative disorders. Trends Cell Biol. 23, 634–643. doi: 10.1016/j.tcb.2013.08.007
Hirai, S., Kawaguchi, A., Hirasawa, R., Baba, M., Ohnishi, T., and Ohno, S. (2002). MAPK-upstream protein kinase (MUK) regulates the radial migration of immature neurons in telencephalon of mouse embryo. Development 129, 4483–4495.
Hirokawa, N., and Noda, Y. (2008). Intracellular transport and kinesin superfamily proteins, KIFs: structure, function, and dynamics. Physiol. Rev. 88, 1089–1118. doi: 10.1152/physrev.00023.2007
Honnappa, S., Okhrimenko, O., Jaussi, R., Jawhari, H., Jelesarov, I., Winkler, F. K., et al. (2006). Key interaction modes of Dynamic +TIP networks. Mol. Cell 23, 663–671. doi: 10.1016/j.molcel.2006.07.013
Hoogenraad, C. C., and Bradke, F. (2009). Control of neuronal polarity and plasticity – a renaissance for microtubules? Trends Cell Biol. 19, 669–676. doi: 10.1016/j.tcb.2009.08.006
Horesh, D., Sapir, T., Francis, F., Wolf, S. G., Caspi, M., Elbaum, M., et al. (1999). Doublecortin, a stabilizer of microtubules. Hum. Mol. Genet. 8, 1599–1610. doi: 10.1093/hmg/8.9.1599
Horton, A. C., and Ehlers, M. D. (2003). Neuronal polarity and trafficking. Neuron 40, 277–295. doi: 10.1016/S0896-6273(03)00629-9
Hsu, J.-M., Chen, C.-H., Chen, Y.-C., McDonald, K. L., Gurling, M., Lee, A., et al. (2014). Genetic analysis of a novel tubulin mutation that redirects synaptic vesicle targeting and causes neurite degeneration in C. elegans. PLoS Genet. 10:e1004715. doi: 10.1371/journal.pgen.1004715
Hu, X., Viesselmann, C., Nam, S., Merriam, E., and Dent, E. W. (2008). Activity-dependent dynamic microtubule invasion of dendritic spines. J. Neurosci. 28, 13094–13105. doi: 10.1523/JNEUROSCI.3074-08.2008
Hurd, D. D., and Saxton, W. M. (1996). Kinesin mutations cause motor neuron disease phenotypes by disrupting fast axonal transport in drosophila. Genetics 144, 1075–1085.
Ikegami, K., and Setou, M. (2010). Unique post-translational modifications in specialized microtubule architecture. Cell Struct. Funct. 35, 15–22. doi: 10.1247/csf.09027
Iqbal, K., Liu, F., Gong, C.-X., and Grundke-Iqbal, I. (2010). Tau in Alzheimer disease and related tauopathies. Curr. Alzheimer Res. 7, 656–664. doi: 10.2174/156720510793611592
Ischiropoulos, H., and Beckman, J. S. (2003). Oxidative stress and nitration in neurodegeneration: cause, effect, or association? J. Clin. Invest. 111, 163–169. doi: 10.1172/JCI200317638
Jacobs, J. R., and Stevens, J. K. (1986). Changes in the organization of the neuritic cytoskeleton during nerve growth factor-activated differentiation of PC12 cells: a serial electron microscopic study of the development and control of neurite shape. J. Cell Biol. 103, 895–906. doi: 10.1083/jcb.103.3.895
Jaglin, X. H., Poirier, K., Saillour, Y., Buhler, E., Tian, G., Bahi-Buisson, N., et al. (2009). Mutations in the β-tubulin gene TUBB2B result in asymmetrical polymicrogyria. Nat. Genet. 41, 746–752. doi: 10.1038/ng.380
Janke, C., and Chloë Bulinski, J. C. (2011). Post-translational regulation of the microtubule cytoskeleton: mechanisms and functions. Nat. Rev. Mol. Cell Biol. 12, 773–786. doi: 10.1038/nrm3227
Janmey, P. A. (1998). The cytoskeleton and cell signaling: component localization and mechanical coupling. Physiol. Rev. 78, 763–781.
Jaworski, J., Kapitein, L. C., Gouveia, S. M., Dortland, B. R., Wulf, P. S., Grigoriev, I., et al. (2009). Dynamic microtubules regulate dendritic spine morphology and synaptic plasticity. Neuron 61, 85–100. doi: 10.1016/j.neuron.2008.11.013
Jordan, M. A., and Wilson, L. (1998). Microtubules and actin filaments: dynamic targets for cancer chemotherapy. Curr. Opin. Cell Biol. 10, 123–130. doi: 10.1016/S0955-0674(98)80095-1
Karabay, A., Yu, W., Solowska, J. M., Baird, D. H., and Baas, P. W. (2004). Axonal growth is sensitive to the levels of Katanin, a protein that severs microtubules. J. Neurosci. 24, 5778–5788. doi: 10.1523/JNEUROSCI.1382-04.2004
Kirszenblat, L., Neumann, B., Coakley, S., and Hilliard, M. A. (2013). A dominant mutation in mec-7/β-tubulin affects axon development and regeneration in Caenorhabditis elegans neurons. Mol. Biol. Cell 24, 285–296. doi: 10.1091/mbc.E12-06-0441
Klauber, N., Parangi, S., Flynn, E., Hamel, E., and D'Amato, R. J. (1997). Inhibition of angiogenesis and breast cancer in mice by the microtubule inhibitors 2-methoxyestradiol and taxol. Cancer Res. 57, 81–86.
Kline-Smith, S. L., and Walczak, C. E. (2002). The Microtubule-destabilizing Kinesin XKCM1 regulates microtubule dynamic instability in cells. Mol. Biol. Cell 13, 2718–2731. doi: 10.1091/mbc.E01-12-0143
Kodama, A., Karakesisoglou, I., Wong, E., Vaezi, A., and Fuchs, E. (2003). ACF7: an essential integrator of microtubule dynamics. Cell 115, 343–354. doi: 10.1016/S0092-8674(03)00813-4
Kornack, D. R., and Giger, R. J. (2005). Probing microtubule +TIPs: regulation of axon branching. Curr. Opin. Neurobiol. 15, 58–66. doi: 10.1016/j.conb.2005.01.009
Lansbergen, G., Grigoriev, I., Mimori-Kiyosue, Y., Ohtsuka, T., Higa, S., Kitajima, I., et al. (2006). CLASPs attach microtubule plus ends to the cell cortex through a complex with LL5β. Dev. Cell 11, 21–32. doi: 10.1016/j.devcel.2006.05.012
Law, B. M. H., Spain, V. A., Leinster, V. H. L., Chia, R., Beilina, A., Cho, H. J., et al. (2014). A direct interaction between leucine-rich repeat kinase 2 and specific β-tubulin isoforms regulates tubulin acetylation. J. Biol. Chem. 289, 895–908. doi: 10.1074/jbc.M113.507913
Layden, B. T., Saengsawang, W., Donati, R. J., Yang, S., Mulhearn, D. C., Johnson, M. E., et al. (2008). Structural model of a complex between the heterotrimeric G protein, Gsα, and tubulin. Biochim. Biophys. Acta 1783, 964–973. doi: 10.1016/j.bbamcr.2008.02.017
Lee, H., Engel, U., Rusch, J., Scherrer, S., Sheard, K., and Van Vactor, D. (2004). The microtubule plus end tracking protein orbit/MAST/CLASP acts downstream of the tyrosine kinase Abl in mediating axon guidance. Neuron 42, 913–926. doi: 10.1016/j.neuron.2004.05.020
Lee, J. J., and Swain, S. M. (2006). Peripheral neuropathy induced by microtubule-stabilizing agents. J. Clin. Oncol. 24, 1633–1642. doi: 10.1200/JCO.2005.04.0543
Letourneau, P. C. (1982). Analysis of microtubule number and length in cytoskeletons of cultured chick sensory neurons. J. Neurosci. 2, 806–814.
Letourneau, P. C., Shattuck, T. A., and Ressler, A. H. (1986). Branching of sensory and sympathetic neurites in vitro is inhibited by treatment with taxol. J. Neurosci. 6, 1912–1917.
Leung, C. L., Green, K. J., and Liem, R. K. H. (2002). Plakins: a family of versatile cytolinker proteins. Trends Cell Biol. 12, 37–45. doi: 10.1016/S0962-8924(01)02180-8
Leung, C. L., Sun, D., Zheng, M., Knowles, D. R., and Liem, R. K. H. (1999). Microtubule Actin Cross-Linking Factor (Macf). J. Cell Biol. 147, 1275–1286. doi: 10.1083/jcb.147.6.1275
Levy, G., Jacobs, D. M., Tang, M.-X., Côté, L. J., Louis, E. D., Alfaro, B., et al. (2002). Memory and executive function impairment predict dementia in Parkinson's disease. Mov. Disord. 17, 1221–1226. doi: 10.1002/mds.10280
Lewcock, J. W., Genoud, N., Lettieri, K., and Pfaff, S. L. (2007). The ubiquitin ligase Phr1 regulates axon outgrowth through modulation of microtubule dynamics. Neuron 56, 604–620. doi: 10.1016/j.neuron.2007.09.009
Li, Y., and Black, M. M. (1996). Microtubule assembly and turnover in growing axons. J. Neurosci. 16, 531–544.
Littauer, U. Z., Giveon, D., Thierauf, M., Ginzburg, I., and Ponstingl, H. (1986). Common and distinct tubulin binding sites for microtubule-associated proteins. Proc. Natl. Acad. Sci. U.S.A. 83, 7162–7166. doi: 10.1073/pnas.83.19.7162
Liu, G., and Dwyer, T. (2014). Microtubule dynamics in axon guidance. Neurosci. Bull. 30, 569–583. doi: 10.1007/s12264-014-1444-6
Liu, Y., Lee, J. W., and Ackerman, S. L. (2015). Mutations in the microtubule-associated protein 1A (Map1a) gene cause Purkinje cell degeneration. J. Neurosci. 35, 4587–4598. doi: 10.1523/JNEUROSCI.2757-14.2015
Liu, Z., Steward, R., and Luo, L. (2000). Drosophila Lis1 is required for neuroblast proliferation, dendritic elaboration and axonal transport. Nat. Cell Biol. 2, 776–783. doi: 10.1038/35041011
Lohmann, K., Wilcox, R. A., Winkler, S., Ramirez, A., Rakovic, A., Park, J.-S., et al. (2013). Whispering dysphonia (DYT4 dystonia) is caused by a mutation in the TUBB4 gene. Ann. Neurol. 73, 537–545. doi: 10.1002/ana.23829
Löwe, J., Li, H., Downing, K. H., and Nogales, E. (2001). Refined structure of αβ-tubulin at 3.5 Å resolution1. J. Mol. Biol. 313, 1045–1057. doi: 10.1006/jmbi.2001.5077
Luo, L., and O'Leary, D. D. M. (2005). Axon retraction and degeneration in development and disease. Annu. Rev. Neurosci. 28, 127–156. doi: 10.1146/annurev.neuro.28.061604.135632
Lyons, D. A., Naylor, S. G., Mercurio, S., Dominguez, C., and Talbot, W. S. (2008). KBP is essential for axonal structure, outgrowth and maintenance in zebrafish, providing insight into the cellular basis of Goldberg-Shprintzen syndrome. Development 135, 599–608. doi: 10.1242/dev.012377
Maday, S., Twelvetrees, A. E., Moughamian, A. J., and Holzbaur, E. L. F. (2014). Axonal transport: cargo-specific mechanisms of motility and regulation. Neuron 84, 292–309. doi: 10.1016/j.neuron.2014.10.019
Maniar, T. A., Kaplan, M., Wang, G. J., Shen, K., Wei, L., Shaw, J. E., et al. (2012). UNC-33 (CRMP) and ankyrin organize microtubules and localize kinesin to polarize axon-dendrite sorting. Nat. Neurosci. 15, 48–56. doi: 10.1038/nn.2970
Matsuyama, S. S., and Jarvik, L. F. (1989). Hypothesis: microtubules, a key to Alzheimer disease. Proc. Natl. Acad. Sci. U.S.A. 86, 8152–8156. doi: 10.1073/pnas.86.20.8152
Matus, A. (1994). Stiff microtubules and neuronal morphology. Trends Neurosci. 17, 19–22. doi: 10.1016/0166-2236(94)90030-2
McCormick, A. V., Wheeler, J. M., Guthrie, C. R., Liachko, N. F., and Kraemer, B. C. (2013). Dopamine D2 Receptor antagonism suppresses tau aggregation and neurotoxicity. Biol. Psychiatry 73, 464–471. doi: 10.1016/j.biopsych.2012.08.027
McNally, F. J., and Vale, R. D. (1993). Identification of katanin, an ATPase that severs and disassembles stable microtubules. Cell 75, 419–429. doi: 10.1016/0092-8674(93)90377-3
Millecamps, S., and Julien, J.-P. (2013). Axonal transport deficits and neurodegenerative diseases. Nat. Rev. Neurosci. 14, 161–176. doi: 10.1038/nrn3380
Miller, A. L., Wang, Y., Mooseker, M. S., and Koleske, A. J. (2004). The Abl-related gene (Arg) requires its F-actin–microtubule cross-linking activity to regulate lamellipodial dynamics during fibroblast adhesion. J. Cell Biol. 165, 407–420. doi: 10.1083/jcb.200308055
Mimori-Kiyosue, Y., Grigoriev, I., Lansbergen, G., Sasaki, H., Matsui, C., Severin, F., et al. (2005). CLASP1 and CLASP2 bind to EB1 and regulate microtubule plus-end dynamics at the cell cortex. J. Cell Biol. 168, 141–153. doi: 10.1083/jcb.200405094
Mitchison, T., and Kirschner, M. (1988). Cytoskeletal dynamics and nerve growth. Neuron 1, 761–772. doi: 10.1016/0896-6273(88)90124-9
Miyatake, S., Osaka, H., Shiina, M., Sasaki, M., Takanashi, J., Haginoya, K., et al. (2014). Expanding the phenotypic spectrum of TUBB4A-associated hypomyelinating leukoencephalopathies. Neurology 82, 2230–2237. doi: 10.1212/WNL.0000000000000535
Morales, J., Hiesinger, P. R., Schroeder, A. J., Kume, K., Verstreken, P., Jackson, F. R., et al. (2002). Drosophila fragile X protein, DFXR, regulates neuronal morphology and function in the brain. Neuron 34, 961–972. doi: 10.1016/S0896-6273(02)00731-6
Morris, M., Maeda, S., Vossel, K., and Mucke, L. (2011). The many faces of Tau. Neuron 70, 410–426. doi: 10.1016/j.neuron.2011.04.009
Nakai, Y., Ozeki, M., Hiraiwa, T., Tanimoto, R., Funahashi, A., Hiroi, N., et al. (2015). High-speed microscopy with an electrically tunable lens to image the dynamics of in vivo molecular complexes. Rev. Sci. Instrum. 86:013707. doi: 10.1063/1.4905330
Okabe, S., and Hirokawa, N. (1988). Microtubule dynamics in nerve cells: analysis using microinjection of biotinylated tubulin into PC12 cells. J. Cell Biol. 107, 651–664. doi: 10.1083/jcb.107.2.651
Panda, D., Samuel, J. C., Massie, M., Feinstein, S. C., and Wilson, L. (2003). Differential regulation of microtubule dynamics by three- and four-repeat tau: implications for the onset of neurodegenerative disease. Proc. Natl. Acad. Sci. U.S.A. 100, 9548–9553. doi: 10.1073/pnas.1633508100
Paschal, B. M., and Vallee, R. B. (1987). Retrograde transport by the microtubule-associated protein MAP 1C. Nature 330, 181–183. doi: 10.1038/330181a0
Paschen, W., and Mengesdorf, T. (2005). Endoplasmic reticulum stress response and neurodegeneration. Cell Calcium 38, 409–415. doi: 10.1016/j.ceca.2005.06.019
Patel, V. P., and Chu, C. T. (2014). Decreased SIRT2 activity leads to altered microtubule dynamics in oxidatively-stressed neuronal cells: implications for Parkinson's disease. Exp. Neurol. 257, 170–181. doi: 10.1016/j.expneurol.2014.04.024
Peris, L., Thery, M., Fauré, J., Saoudi, Y., Lafanechère, L., Chilton, J. K., et al. (2006). Tubulin tyrosination is a major factor affecting the recruitment of CAP-Gly proteins at microtubule plus ends. J. Cell Biol. 174, 839–849. doi: 10.1083/jcb.200512058
Peris, L., Wagenbach, M., Lafanechère, L., Brocard, J., Moore, A. T., Kozielski, F., et al. (2009). Motor-dependent microtubule disassembly driven by tubulin tyrosination. J. Cell Biol. 185, 1159–1166. doi: 10.1083/jcb.200902142
Pielage, J., Fetter, R. D., and Davis, G. W. (2005). Presynaptic spectrin is essential for synapse stabilization. Curr. Biol. 15, 918–928. doi: 10.1016/j.cub.2005.04.030
Poirier, K., Keays, D. A., Francis, F., Saillour, Y., Bahi, N., Manouvrier, S., et al. (2007). Large spectrum of lissencephaly and pachygyria phenotypes resulting from de novo missense mutations in tubulin alpha 1A (TUBA1A). Hum. Mutat. 28, 1055–1064. doi: 10.1002/humu.20572
Poirier, K., Saillour, Y., Bahi-Buisson, N., Jaglin, X. H., Fallet-Bianco, C., Nabbout, R., et al. (2010). Mutations in the neuronal beta tubulin subunit TUBB3 result in malformation of cortical development and neuronal migration defects. Hum. Mol. Genet. 128, 45–57. doi: 10.1093/hmg/ddq377
Qiang, L., Yu, W., Andreadis, A., Luo, M., and Baas, P. W. (2006). Tau protects microtubules in the axon from severing by Katanin. J. Neurosci. 26, 3120–3129. doi: 10.1523/JNEUROSCI.5392-05.2006
Reed, N. A., Cai, D., Blasius, T. L., Jih, G. T., Meyhofer, E., Gaertig, J., et al. (2006). Microtubule acetylation promotes kinesin-1 binding and transport. Curr. Biol. 16, 2166–2172. doi: 10.1016/j.cub.2006.09.014
Reiner, O. (2013). LIS1 and DCX: implications for brain development and human disease in relation to microtubules. Scientifica 2013:e393975. doi: 10.1155/2013/393975
Ren, Y., Jiang, H., Hu, Z., Fan, K., Wang, J., Janoschka, S., et al. (2015). Parkin mutations reduce the complexity of neuronal processes in iPSC-derived human neurons. Stem Cells 33, 68–78. doi: 10.1002/stem.1854
Ren, Y., Zhao, J., and Feng, J. (2003). Parkin binds to alpha/beta tubulin and increases their ubiquitination and degradation. J. Neurosci. 23, 3316–3324.
Rogers, S. L., Wiedemann, U., Häcker, U., Turck, C., and Vale, R. D. (2004). Drosophila RhoGEF2 associates with microtubule plus ends in an EB1-dependent manner. Curr. Biol. 14, 1827–1833. doi: 10.1016/j.cub.2004.09.078
Roll-Mecak, A., and Vale, R. D. (2006). Making more microtubules by severing: a common theme of noncentrosomal microtubule arrays? J. Cell Biol. 175, 849–851. doi: 10.1083/jcb.200611149
Roy, S. (2014). Seeing the unseen – the hidden world of slow axonal transport. Neuroscientist 20, 71–81. doi: 10.1177/1073858413498306
Roychowdhury, S., Panda, D., Wilson, L., and Rasenick, M. M. (1999). G protein α subunits activate tubulin GTPase and modulate microtubule polymerization dynamics. J. Biol. Chem. 274, 13485–13490. doi: 10.1074/jbc.274.19.13485
Sagar, H. J., Cohen, N. J., Sullivan, E. V., Corkin, S., and Growdon, J. H. (1988). Remote memory function in Alzheimer's disease and Parkinson's disease. Brain 111, 185–206. doi: 10.1093/brain/111.1.185
Sammak, P. J., and Borisy, G. G. (1988). Direct observation of microtubule dynamics in living cells. Nature 332, 724–726. doi: 10.1038/332724a0
Savage, C., Hamelin, M., Culotti, J. G., Coulson, A., Albertson, D. G., and Chalfie, M. (1989). mec-7 is a beta-tubulin gene required for the production of 15-protofilament microtubules in Caenorhabditis elegans. Genes Dev. 3, 870–881. doi: 10.1101/gad.3.6.870
Savage, C., Xue, Y., Mitani, S., Hall, D., Zakhary, R., and Chalfie, M. (1994). Mutations in the Caenorhabditis elegans beta-tubulin gene mec-7: effects on microtubule assembly and stability and on tubulin autoregulation. J. Cell Sci. 107, 2165–2175.
Sayas, C. L., Tortosa, E., Bollati, F., Ramírez-Ríos, S., Arnal, I., and Avila, J. (2015). Tau regulates the localization and function of End-binding proteins 1 and 3 in developing neuronal cells. J. Neurochem. 133, 653–667. doi: 10.1111/jnc.13091
Schappi, J. M., Krbanjevic, A., and Rasenick, M. M. (2014). Tubulin, actin and heterotrimeric G proteins: coordination of signaling and structure. Biochim. Biophys. Acta 1838, 674–681. doi: 10.1016/j.bbamem.2013.08.026
Scripture, C. D., Figg, W. D., and Sparreboom, A. (2006). Peripheral neuropathy induced by paclitaxel: recent insights and future perspectives. Curr. Neuropharmacol. 4, 165–172. doi: 10.2174/157015906776359568
Scuteri, A., Nicolini, G., Miloso, M., Bossi, M., Cavaletti, G., Windebank, A. J., et al. (2006). Paclitaxel Toxicity in Post-mitotic Dorsal Root Ganglion (DRG) Cells. Anticancer Res. 26, 1065–1070.
Seitz-Tutter, D., Langford, G. M., and Weiss, D. G. (1988). Dynamic instability of native microtubules from squid axons is rare and independent of gliding and vesicle transport. Exp. Cell Res. 178, 504–512. doi: 10.1016/0014-4827(88)90418-1
Selkoe, D. J. (2002). Alzheimer's disease is a synaptic failure. Science 298, 789–791. doi: 10.1126/science.1074069
Serrano-Pozo, A., Frosch, M. P., Masliah, E., and Hyman, B. T. (2011). Neuropathological alterations in Alzheimer disease. Cold Spring Harb. Perspect. Med. 1:a006189. doi: 10.1101/cshperspect.a006189
Shankar, G. M., Li, S., Mehta, T. H., Garcia-Munoz, A., Shepardson, N. E., Smith, I., et al. (2008). Amyloid-β protein dimers isolated directly from Alzheimer's brains impair synaptic plasticity and memory. Nat. Med. 14, 837–842. doi: 10.1038/nm1782
Shelden, E., and Wadsworth, P. (1993). Observation and quantification of individual microtubule behavior in vivo: microtubule dynamics are cell-type specific. J. Cell Biol. 120, 935–945. doi: 10.1083/jcb.120.4.935
Shi, S.-H., Cheng, T., Jan, L. Y., and Jan, Y.-N. (2004). APC and GSK-3β are involved in mPar3 targeting to the nascent axon and establishment of neuronal polarity. Curr. Biol. 14, 2025–2032. doi: 10.1016/j.cub.2004.11.009
Shulman, J. M., and Feany, M. B. (2003). Genetic modifiers of tauopathy in Drosophila. Genetics 165, 1233–1242.
Simons, C., Wolf, N. I., McNeil, N., Caldovic, L., Devaney, J. M., Takanohashi, A., et al. (2013). A de novo mutation in the β-tubulin gene TUBB4A results in the leukoencephalopathy hypomyelination with atrophy of the basal ganglia and cerebellum. Am. J. Hum. Genet. 92, 767–773. doi: 10.1016/j.ajhg.2013.03.018
Skovronsky, D. M., Lee, V. M.-Y., and Trojanowski, J. Q. (2006). Neurodegenerative diseases: new concepts of pathogenesis and their therapeutic implications. Annu. Rev. Pathol. Mech. Dis. 1, 151–170. doi: 10.1146/annurev.pathol.1.110304.100113
Smith, B. N., Ticozzi, N., Fallini, C., Gkazi, A. S., Topp, S., Kenna, K. P., et al. (2014). Exome-wide rare variant analysis identifies TUBA4A mutations associated with familial ALS. Neuron 84, 324–331. doi: 10.1016/j.neuron.2014.09.027
Song, Y., Kirkpatrick, L. L., Schilling, A. B., Helseth, D. L., Chabot, N., Keillor, J. W., et al. (2013). Transglutaminase and polyamination of tubulin: posttranslational modification for stabilizing axonal microtubules. Neuron 78, 109–123. doi: 10.1016/j.neuron.2013.01.036
Stepanova, T., Slemmer, J., Hoogenraad, C. C., Lansbergen, G., Dortland, B., Zeeuw, C. I. D., et al. (2003). Visualization of microtubule growth in cultured neurons via the use of EB3-GFP (end-binding protein 3-green fluorescent protein). J. Neurosci. 23, 2655–2664.
Stepanova, T., Smal, I., van Haren, J., Akinci, U., Liu, Z., Miedema, M., et al. (2010). History-dependent catastrophes regulate axonal microtubule behavior. Curr. Biol. 20, 1023–1028. doi: 10.1016/j.cub.2010.04.024
Stephan, R., Goellner, B., Moreno, E., Frank, C. A., Hugenschmidt, T., Genoud, C., et al. (2015). Hierarchical microtubule organization controls axon caliber and transport and determines synaptic structure and stability. Dev. Cell 33, 5–21. doi: 10.1016/j.devcel.2015.02.003
Stokin, G. B., Lillo, C., Falzone, T. L., Brusch, R. G., Rockenstein, E., Mount, S. L., et al. (2005). Axonopathy and transport deficits early in the pathogenesis of Alzheimer's disease. Science 307, 1282–1288. doi: 10.1126/science.1105681
Stone, M. C., Nguyen, M. M., Tao, J., Allender, D. L., and Rolls, M. M. (2010). Global up-regulation of microtubule dynamics and polarity reversal during regeneration of an axon from a dendrite. Mol. Biol. Cell 21, 767–777. doi: 10.1091/mbc.E09-11-0967
Sun, D., Leung, C. L., and Liem, R. K. (2001). Characterization of the microtubule binding domain of microtubule actin crosslinking factor (MACF): identification of a novel group of microtubule associated proteins. J. Cell Sci. 114, 161–172.
Takalo, M., Salminen, A., Soininen, H., Hiltunen, M., and Haapasalo, A. (2013). Protein aggregation and degradation mechanisms in neurodegenerative diseases. Am. J. Neurodegener. Dis. 2, 1–14.
Tanabe, K., and Takei, K. (2009). Dynamic instability of microtubules requires dynamin 2 and is impaired in a Charcot-Marie-Tooth mutant. J. Cell Biol. 185, 939–948. doi: 10.1083/jcb.200803153
Tanaka, T., Serneo, F. F., Higgins, C., Gambello, M. J., Wynshaw-Boris, A., and Gleeson, J. G. (2004). Lis1 and doublecortin function with dynein to mediate coupling of the nucleus to the centrosome in neuronal migration. J. Cell Biol. 165, 709–721. doi: 10.1083/jcb.200309025
Tarrade, A., Fassier, C., Courageot, S., Charvin, D., Vitte, J., Peris, L., et al. (2006). A mutation of spastin is responsible for swellings and impairment of transport in a region of axon characterized by changes in microtubule composition. Hum. Mol. Genet. 15, 3544–3558. doi: 10.1093/hmg/ddl431
Terada, S., Kinjo, M., and Hirokawa, N. (2000). Oligomeric tubulin in large transporting complex is transported via kinesin in squid giant axons. Cell 103, 141–155. doi: 10.1016/S0092-8674(00)00094-5
Tischfield, M. A., Baris, H. N., Wu, C., Rudolph, G., Van Maldergem, L., He, W., et al. (2010). Human TUBB3 mutations perturb microtubule dynamics, kinesin interactions, and axon guidance. Cell 140, 74–87. doi: 10.1016/j.cell.2009.12.011
Tortosa, E., Galjart, N., Avila, J., and Sayas, C. L. (2013). MAP1B regulates microtubule dynamics by sequestering EB1/3 in the cytosol of developing neuronal cells. EMBO J. 32, 1293–1306. doi: 10.1038/emboj.2013.76
Trinczek, B., Ebneth, A., Mandelkow, E. M., and Mandelkow, E. (1999). Tau regulates the attachment/detachment but not the speed of motors in microtubule-dependent transport of single vesicles and organelles. J. Cell Sci. 112, 2355–2367.
Valakh, V., Frey, E., Babetto, E., Walker, L. J., and DiAntonio, A. (2015). Cytoskeletal disruption activates the DLK/JNK pathway, which promotes axonal regeneration and mimics a preconditioning injury. Neurobiol. Dis. 77, 13–25. doi: 10.1016/j.nbd.2015.02.014
Valakh, V., Walker, L. J., Skeath, J. B., and DiAntonio, A. (2013). Loss of the spectraplakin short stop activates the DLK injury response pathway in drosophila. J. Neurosci. 33, 17863–17873. doi: 10.1523/JNEUROSCI.2196-13.2013
Vale, R. D., Reese, T. S., and Sheetz, M. P. (1985b). Identification of a novel force-generating protein, kinesin, involved in microtubule-based motility. Cell 42, 39–50. doi: 10.1016/S0092-8674(85)80099-4
Vale, R. D., Schnapp, B. J., Mitchison, T., Steuer, E., Reese, T. S., and Sheetz, M. P. (1985a). Different axoplasmic proteins generate movement in opposite directions along microtubules in vitro. Cell 43, 623–632. doi: 10.1016/0092-8674(85)90234-X
Valetti, C., Wetzel, D. M., Schrader, M., Hasbani, M. J., Gill, S. R., Kreis, T. E., et al. (1999). Role of dynactin in endocytic traffic: effects of dynamitin overexpression and colocalization with CLIP-170. Mol. Biol. Cell 10, 4107–4120. doi: 10.1091/mbc.10.12.4107
Vallee, R. B., and Tsai, J.-W. (2006). The cellular roles of the lissencephaly gene LIS1, and what they tell us aboutbrain development. Genes Dev. 20, 1384–1393. doi: 10.1101/gad.1417206
Wade, R. H. (2009). On and around microtubules: an overview. Mol. Biotechnol. 43, 177–191. doi: 10.1007/s12033-009-9193-5
Walker, R. A., O'Brien, E. T., Pryer, N. K., Soboeiro, M. F., Voter, W. A., Erickson, H. P., et al. (1988). Dynamic instability of individual microtubules analyzed by video light microscopy: rate constants and transition frequencies. J. Cell Biol. 107, 1437–1448.
Walsh, D. M., and Selkoe, D. J. (2004). Deciphering the molecular basis of memory failure in Alzheimer's disease. Neuron 44, 181–193. doi: 10.1016/j.neuron.2004.09.010
Walton, J. R. (2006). Aluminum in hippocampal neurons from humans with Alzheimer's disease. Neurotoxicology 27, 385–394. doi: 10.1016/j.neuro.2005.11.007
Walton, J. R. (2009). Brain lesions comprised of aluminum-rich cells that lack microtubules may be associated with the cognitive deficit of Alzheimer's disease. Neurotoxicology 30, 1059–1069. doi: 10.1016/j.neuro.2009.06.010
Walton, J. R. (2013). Aluminum involvement in the progression of Alzheimer's disease. J. Alzheimers Dis. 35, 7–43. doi: 10.3233/JAD-121909
Wang, L., and Brown, A. (2002). Rapid movement of microtubules in axons. Curr. Biol. 12, 1496–1501. doi: 10.1016/S0960-9822(02)01078-3
Warita, H., Itoyama, Y., and Abe, K. (1999). Selective impairment of fast anterograde axonal transport in the peripheral nerves of asymptomatic transgenic mice with a G93A mutant SOD1 gene. Brain Res. 819, 120–131. doi: 10.1016/S0006-8993(98)01351-1
Watanabe, T., Noritake, J., Kakeno, M., Matsui, T., Harada, T., Wang, S., et al. (2009). Phosphorylation of CLASP2 by GSK-3β regulates its interaction with IQGAP1, EB1 and microtubules. J. Cell Sci. 122, 2969–2979. doi: 10.1242/jcs.046649
Waterman-Storer, C. M., and Salmon, E. D. (1998). How microtubules get fluorescent speckles. Biophys. J. 75, 2059–2069. doi: 10.1016/S0006-3495(98)77648-9
Weingarten, M. D., Lockwood, A. H., Hwo, S. Y., and Kirschner, M. W. (1975). A protein factor essential for microtubule assembly. Proc. Natl. Acad. Sci. U.S.A. 72, 1858–1862. doi: 10.1073/pnas.72.5.1858
Westermann, S., and Weber, K. (2003). Post-translational modifications regulate microtubule function. Nat. Rev. Mol. Cell Biol. 4, 938–948. doi: 10.1038/nrm1260
Witte, H., Neukirchen, D., and Bradke, F. (2008). Microtubule stabilization specifies initial neuronal polarization. J. Cell Biol. 180, 619–632. doi: 10.1083/jcb.200707042
Wittmann, T., and Waterman-Storer, C. M. (2001). Cell motility: can Rho GTPases and microtubules point the way? J. Cell Sci. 114, 3795–3803.
Xiong, X., Wang, X., Ewanek, R., Bhat, P., Diantonio, A., and Collins, C. A. (2010). Protein turnover of the Wallenda/DLK kinase regulates a retrograde response to axonal injury. J. Cell Biol. 191, 211–223. doi: 10.1083/jcb.201006039
Yamasaki, T. R., Blurton-Jones, M., Morrissette, D. A., Kitazawa, M., Oddo, S., and LaFerla, F. M. (2007). Neural stem cells improve memory in an inducible mouse model of neuronal loss. J. Neurosci. 27, 11925–11933. doi: 10.1523/JNEUROSCI.1627-07.2007
Yan, D., Wu, Z., Chisholm, A. D., and Jin, Y. (2009). The DLK-1 kinase promotes mRNA stability and local translation in C. elegans synapses and axon regeneration. Cell 138, 1005–1018. doi: 10.1016/j.cell.2009.06.023
Yang, F., Jiang, Q., Zhao, J., Ren, Y., Sutton, M. D., and Feng, J. (2005). Parkin stabilizes microtubules through strong binding mediated by three independent domains. J. Biol. Chem. 280, 17154–17162. doi: 10.1074/jbc.M500843200
Yu, W., Ahmad, F. J., and Baas, P. W. (1994). Microtubule fragmentation and partitioning in the axon during collateral branch formation. J. Neurosci. 14, 5872–5884.
Yu, W., Qiang, L., and Baas, P. W. (2007). Microtubule-severing in the axon: implications for development, disease, and regeneration after injury. J Environ. Biomed 1, 1–7.
Yu, W., Qiang, L., Solowska, J. M., Karabay, A., Korulu, S., and Baas, P. W. (2008). The microtubule-severing proteins spastin and Katanin participate differently in the formation of axonal branches. Mol. Biol. Cell 19, 1485–1498. doi: 10.1091/mbc.E07-09-0878
Yu, W., Solowska, J. M., Qiang, L., Karabay, A., Baird, D., and Baas, P. W. (2005). Regulation of microtubule severing by Katanin subunits during neuronal development. J. Neurosci. 25, 5573–5583. doi: 10.1523/JNEUROSCI.0834-05.2005
Zhai, Q., Wang, J., Kim, A., Liu, Q., Watts, R., Hoopfer, E., et al. (2003). Involvement of the ubiquitin-proteasome system in the early stages of Wallerian degeneration. Neuron 39, 217–225. doi: 10.1016/S0896-6273(03)00429-X
Zhang, B., Carroll, J., Trojanowski, J. Q., Yao, Y., Iba, M., Potuzak, J. S., et al. (2012). The microtubule-stabilizing agent, Epothilone D, reduces axonal dysfunction, neurotoxicity, cognitive deficits, and Alzheimer-like pathology in an interventional study with aged Tau transgenic mice. J. Neurosci. 32, 3601–3611. doi: 10.1523/JNEUROSCI.4922-11.2012
Zheng, Y., Wildonger, J., Ye, B., Zhang, Y., Kita, A., Younger, S. H., et al. (2008). Dynein is required for polarized dendritic transport and uniform microtubule orientation in axons. Nat. Cell Biol. 10, 1172–1180. doi: 10.1038/ncb1777
Keywords: microtuble stability, Alzheimer's disease, Parkinson disease, dying back, hyperstable microtubules, microtubule signaling hubs
Citation: Dubey J, Ratnakaran N and Koushika SP (2015) Neurodegeneration and microtubule dynamics: death by a thousand cuts. Front. Cell. Neurosci. 9:343. doi: 10.3389/fncel.2015.00343
Received: 26 April 2015; Accepted: 18 August 2015;
Published: 09 September 2015.
Edited by:
Yogesh P. Wairkar, University of Texas Medical Branch, USAReviewed by:
Christopher D. Link, University of Colorado, USAChris Li, City College of the City University of New York, USA
Copyright © 2015 Dubey, Ratnakaran and Koushika. This is an open-access article distributed under the terms of the Creative Commons Attribution License (CC BY). The use, distribution or reproduction in other forums is permitted, provided the original author(s) or licensor are credited and that the original publication in this journal is cited, in accordance with accepted academic practice. No use, distribution or reproduction is permitted which does not comply with these terms.
*Correspondence: Sandhya P. Koushika, Department of Biological Sciences, Tata Institute of Fundamental Research, Dr. Homi Bhabha Road, Colaba, Mumbai-400005, India,c3Brb3VzaGlrYUB0aWZyLnJlcy5pbg==
†These authors have contributed equally to this work.