Cortical GABAergic Interneuron/Progenitor Transplantation as a Novel Therapy for Intractable Epilepsy
- 1Translational Stem Cell Neurobiology Laboratory, Department of Cell Biology and Anatomy, New York Medical College, Valhalla, NY, United States
- 2Hall-Atwater Laboratory, Department of Biology, Program in Neuroscience and Behavior, Wesleyan University, Middletown, CT, United States
Epilepsy is a severe neurological disease affecting more than 70 million people worldwide that is characterized by unpredictable and abnormal electrical discharges resulting in recurrent seizures. Although antiepileptic drugs (AEDs) are the mainstay of epilepsy treatment for seizure control, about one third of patients with epilepsy suffer from intractable seizures that are unresponsive to AEDs. Furthermore, the patients that respond to AEDs typically experience adverse systemic side effects, underscoring the urgent need to develop new therapies that target epileptic foci rather than more systemic interventions. Neurosurgical removal of affected brain tissues or implanting neurostimulator devices are effective options only for a fraction of patients with drug-refractory seizures, so it is imperative to develop treatments that are more generally applicable and restorative in nature. Considering the abnormalities of GABAergic inhibitory interneurons in epileptic brain tissues, one strategy with considerable promise is to restore normal circuit function by transplanting GABAergic interneurons/progenitors into the seizure focus. In this review, we focus on recent studies of cortical GABAergic interneuron transplantation to treat epilepsy and discuss critical issues in moving this promising experimental therapeutic treatment into clinic.
Introduction
Epilepsy is a serious and chronic brain disorder that affects more than 70 million people worldwide (Singh and Trevick, 2016). The fundamental characteristics of epilepsy include recurrent seizures and loss of consciousness caused by unpredictable and abnormal electrical discharges (Baraban et al., 2009; Yuan et al., 2011). The etiology of epilepsy is complicated and heterogenous, including structural, genetic, infectious, metabolic, immune and/or other unknown causes (Scheffer et al., 2017). Currently, epilepsies are classified into focal epilepsies, generalized epilepsies, combined generalized and focal epilepsies, and unknown epilepsies based on the types of seizures the patients display (Fisher, 2017; Fisher et al., 2017; Scheffer et al., 2017). In various etiologies and manifestations of epilepsies, more than 30% of cases are comprised of refractory epilepsies in which medical therapies fail to manage the seizures (Engel, 2014), presenting an urgent, unmet need for new treatments. In this review, we will discuss the promise of neuronal cell-based transplantation therapies for epilepsy as a novel and restorative therapy.
Current Treatment for Epilepsy
Antiepileptic drugs (AEDs) are the mainstay of epilepsy treatments for controlling seizures (Goodwin, 2005) and they are cost-effective choices for most individuals with epilepsy. However, patients responding well to AEDs typically experience adverse systemic side effects, such as skin rashes, mental slowing, visual side effects, and behavioral disorders (Walia et al., 2006). For example, phenobarbital, which is one of the oldest AEDs, is associated with significant adverse events which may dramatically affect the quality of patients’ life (Baram and Shinnar, 2002). However, it is still widely prescribed because of its low cost and broad spectrum efficacy for multiple seizure types (Pal, 2007), By contrast, levetiracetam is one of the newer AEDs that has less severe side effects with higher tolerability, but its monthly cost is substantially higher (Hovinga, 2001; Ho et al., 2018). In addition, about one-third of patients that do not respond to any AEDs have to live with intractable seizures (Herman, 2010). Drug-resistant seizures can be potentially life-threatening and are associated with psychological impairments, excessive body injuries, and compromised quality of life (Sperling, 2004; Laxer et al., 2014). These disadvantages of AEDs underscore the need for alternate therapeutics that target epileptic foci.
Two alternatives are neurosurgical resection of the seizure focus (Kneen and Appleton, 2006) and neurostimulation (Lin and Wang, 2017). Compared to current drug therapies, patients that undergo surgical removal of seizure foci are more likely to become seizure free with better quality of life (Liu et al., 2018). However, surgical resection is a costly procedure, and can only be done successfully when the seizure focus can be clearly identified and safely removed without lasting consequences (Bertram, 2014; Jobst and Cascino, 2015). Moreover, many patients relapse after an initial seizure-free phase (de Tisi et al., 2011). The major advantages of neurostimulation over surgical resections and AEDs are that this approach does not require removal of brain tissue, and it can be applied into a specific target region to modulate affected neural circuity (Lin and Wang, 2017). Electrical neurostimulation is reported to be effective and acceptably safe (Heck et al., 2014), but it is currently useful only in a fraction of patients with drug-refractory seizures (Pais-Vieira et al., 2016). Transcranial magnetic stimulation is non-invasive, but there is no powerful evidence to support its efficacy (Lin and Wang, 2017). These limitations in the currently available treatment options for epilepsy, especially for those for refractory epilepsy, call for the development of more effective and restorative therapeutics.
Cortical Gabaergic Interneurons in Normal and Epileptic Brains
In the neocortex, excitatory pyramidal cells comprise 70–80% of neurons, while interneurons, mostly inhibitory interneurons, constitute the remaining 20–30% (Markram et al., 2004). Although inhibitory interneurons releasing Gamma-Aminobutyric Acid (GABA) are far fewer than excitatory pyramidal neurons, they serve critical roles in regulating activity within brain circuits (Buzsáki et al., 2007). During early brain development, cortical GABAergic interneurons primarily arise from Medial Ganglionic Eminence (MGE) and Caudal Ganglionic Eminence (CGE), and migrate tangentially over long distances to their final destinations in the cerebral cortex where they form local inhibitory synaptic connections with excitatory pyramidal neurons and other GABAergic interneurons (Kelsom and Lu, 2013; Tyson and Anderson, 2014; Hunt and Baraban, 2015). These interneuron progenitors from MGE and CGE generate strikingly diverse subtypes of neurons, classified by their morphology, physiology and molecular properties (Markram et al., 2004).
Interneuron abnormalities have been observed in various cases of epilepsy, especially for those that were derived from MGE. In a non-human primate model of neocortical focal epilepsy, a dramatic decrease was observed in the number of GABAergic symmetric synapses in epileptic foci (Ribak et al., 1982). In tissue from patients with temporal lobe epilepsy (TLE), hippocampal seizure foci were characterized by the loss of somatostatin (SST)+ interneurons (de Lanerolle et al., 1989). In a rodent model of TLE, key features include: altered interneuron firing, reduced numbers of inhibitory interneurons, initial loss of inhibitory synapses followed by synaptic sprouting and alterations in GABAA receptors (Sloviter, 1987; Scharfman, 1999; Kobayashi and Buckmaster, 2003; Scharfman and Myers, 2013; Chohan and Moore, 2016). With consistent observations of interneuron abnormalities in seizure foci, hypofunction/degeneration of GABAergic interneurons has been hypothesized to be key to the neural circuit dysfunction that underlies epileptogenesis and the development of recurrent spontaneous seizures (Buckmaster et al., 2002; Goldberg and Coulter, 2013; Southwell et al., 2014; Hunt and Baraban, 2015). In line with this hypothesis, it was shown that epilepsy-related loss of hilar SST+ interneurons resulted in reduced dentate gyrus inhibition (Hofmann et al., 2016). Furthermore, rapid optogenetic stimulation of hippocampal parvalbumin (PV)+ interneurons effectively suppressed spontaneous seizures (Armstrong et al., 2013; Krook-Magnuson et al., 2013), pointing to the efficacy of modulating GABAergic inhibition for therapeutic purposes.
Unlike cortical GABAergic interneurons derived from MGE, cortical GABAergic interneurons originating in the embryonic CGE, are comprised chiefly of Calretinin (CR)+ and Vasoactive Intestinal Polypeptide (VIP)+ expressing subtypes; these subtypes are not ideal for transplantation to control seizures, since they mostly target other interneurons over excitatory pyramidal neurons, and are thought to disinhibit neural circuits, which might lead to hyperexcitability (Pi et al., 2013; Hunt and Baraban, 2015; Pelkey et al., 2017).
Gabaergic Interneuron/Progenitor Transplantation to Treat Epilepsy
In the pioneering studies, transplantation of noradrenergic neurons was reported to suppress seizures in several different models of epilepsy (Barry et al., 1987; Bengzon et al., 1990; Bortolotto et al., 1990). However, considering the preponderance of interneuron pathologies in TLE, more recent studies have focused on transplanting GABAergic interneurons/progenitors into seizure foci. Preclinical studies in animal models of epilepsy have shown the efficacy of GABAergic interneuron/progenitor transplantation in ameliorating seizures (Löscher et al., 1998; Hattiangady et al., 2008; Baraban et al., 2009; Calcagnotto et al., 2010; Handreck et al., 2012; Maisano et al., 2012; Hunt et al., 2013; Cunningham et al., 2014; Hammad et al., 2014; Henderson et al., 2014; Table 1). Pioneering work by Löscher et al. (1998) revealed that transplanting rat fetal GABAergic neurons into the substania nigra (SN) led to a dramatic increase in afterdischarge thresholds and significant reductions in the severity of seizures. Although this anticonvulsant effect was not long-lasting, it did not cause adverse effects (Löscher et al., 1998). In 2012, Handreck et al. observed the anticonvulsant effects of GABA-producing cells implanted bilaterally/unilaterally into the subthalamic nucleus (STN) using a pentylenetetrazole (PTZ)-induced acute seizure model in adult rats. In addition, they observed that grafting into the STN caused more pronounced anticonvulsant effects than grafting into the SN (Handreck et al., 2012). In these two studies, GABA-producing cells were placed into sites that modulate seizures rather than the seizure focus. In future studies, it will be important to compare and determine the optimal sites for cell delivery for each type of seizure, whether it is within the seizure focus or in seizure-modulating loci.
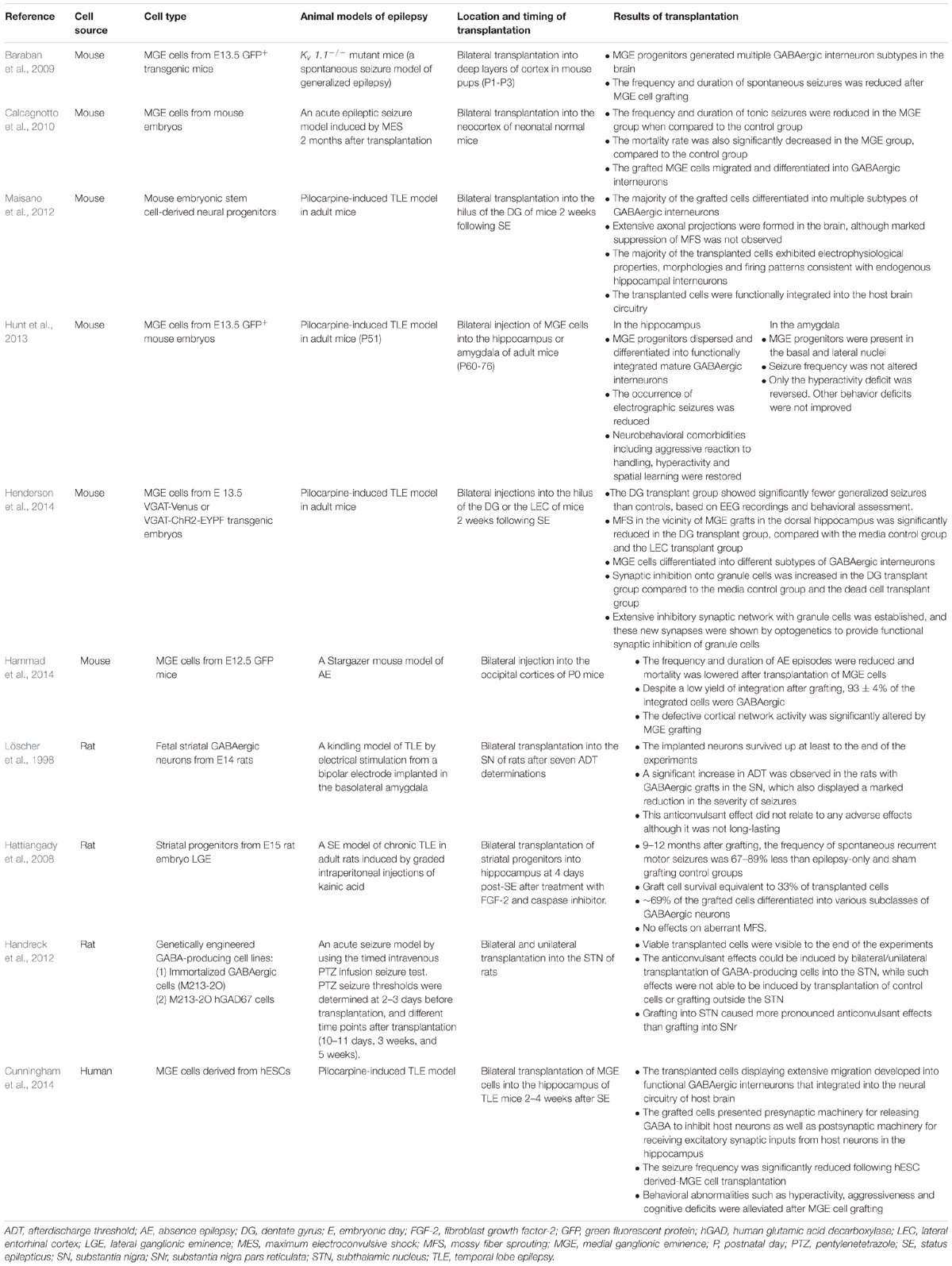
TABLE 1. A summary of GABAergic interneuron/progenitor transplantation in animal models of epilepsy.
More studies on cell therapy for epilepsy have directly targeted the seizure focus to replenish dysfunctional or damaged GABAergic interneurons, especially for TLE models in rodents. Hattiangady et al. demonstrated that bilateral grafting of interneuron progenitors into the hippocampus reduced the frequency of spontaneous seizures on a long-term basis (Hattiangady et al., 2008). In the same line, Hunt et al. (2013) and Henderson et al. (2014) reported that spontaneous seizures could be controlled by grafting mouse fetal MGE cells into the hippocampus of TLE mice, but not the amygdala nor entorhinal cortex. By optogenetic stimulation of the transplanted MGE cells, Henderson et al. (2014) further showed that the new inhibitory synaptic connections formed onto dentate granule cells were capable of inducing strong postsynaptic inhibition, suggesting that increased synaptic inhibition from the transplants onto dentate granule cells could provide a mechanism for seizure suppression in the TLE mice. Unconventionally, Chu et al. (2004) injected that human neural stem cells intravenously in adult rats with TLE, and observed that injected stem cells migrated to the damaged hippocampus, differentiated into GABAergic interneurons, and reduced seizures, warranting further exploration of this non-invasive cell delivery method to a damaged seizure focus. In addition to fetal-derived interneuron progenitors, Maisano et al. (2012) studied the effects of transplanting GABAergic interneurons derived from mouse embryonic stem cells into the dentate gyrus of mice with pilocarpine-induced TLE, and showed that these interneurons can functionally integrate the host brain circuitry and develop mature electrophysiological firing patterns characteristic of endogenous hippocampal interneurons.
In addition to more well-defined seizure foci as in TLE, MGE progenitor cells have also been transplanted to the neocortex to prevent seizures or elevate seizure thresholds. Transplantation of mouse fetal MGE cells into the neonatal mouse neocortex was effective in reducing the frequency and duration of seizures in a genetic model of epilepsy (Baraban et al., 2009). Although the transplants did not migrate throughout the entire cortical areas affected by Kv1.1 mutation, increasing inhibition in a limited area of the developing neocortex was enough to ameliorate seizures in these mice. Transplants of mouse fetal MGE cells also reduced seizure susceptibility and spread in an acute model of epilepsy induced by maximum electroconvulsive shock (Calcagnotto et al., 2010). Additionally, in stargazer mutant mice, which show spike and wave discharges resembling absence seizures (AE), transplanting fetal mouse MGE cells into the occipital neocortex reduced AE episodes and modified defective cortical network activity (Hammad et al., 2014). Taken together, these studies of GABAregic progenitor transplantation show that multiple graft locations, in both adult and developmental models of epilepsy, can suppress seizures or raise seizure thresholds. Further work is needed to define which sites provide the most effective seizure suppression for each seizure model.
Harvesting large numbers of MGE precursors from human embryos for clinical applications is impractical and raises ethical concerns, whereas differentiation of sufficient numbers of MGE progenitor cells from human pluripotent stem cells (PSCs), including human embryonic stem cells (hESCs) and human induced pluripotent stem cells (hiPSCs), is becoming more routine in many scientific laboratories (Goulburn et al., 2011; Germain et al., 2013; Liu et al., 2013a; Maroof et al., 2013; Nicholas et al., 2013; Kim et al., 2014). After transplantation into mouse brains, hPSC-derived MGE progenitors gradually mature into functional GABAergic interneurons and become synaptically integrated into host brain circuits (Nicholas et al., 2013; Kim et al., 2014). Most importantly, transplantation of human PSC-derived MGE cells into the hippocampus was efficacious in the pilocarpine model of TLE (Cunningham et al., 2014). In this study, Cunningham et al. (2014) showed that human PSC-derived MGE progenitors migrated robustly and developed into functional GABAergic interneurons that integrated into the neural circuitry of the host brain, suppressed seizure activity, and corrected behavioral abnormalities, such as hyperactivity, aggressiveness and cognitive deficits. This study provides proof-of-concept that GABAergic interneuron progenitors derived from human PSCs could provide a valuable and unlimited cell source for cell transplantation to treat intractable seizures.
There are various benefits of cell-based therapies for epilepsy, in comparison to more traditional treatment methods. Functionally distinct subtypes of GABAergic interneurons or other types of neurons could be generated and implanted into specific regions of the host brain (Bjarkam et al., 2009), thus obviating many of the adverse systemic side effects caused by AEDs. Furthermore, because developing neurons may be able to integrate into host brain circuits, they could provide synaptic inhibition on demand in an activity-dependent manner and circumvent issues associated with taking medications regularly and maintaining constant drug dosage. A self-regulating therapeutic system of grafted interneurons, activated by host excitatory neurons that releases GABA synaptically during periods of heightened hyperexcitability (Cunningham et al., 2014) would render the need to wear devices to detect and manage seizures obsolete. While resection of the seizure focus has been used as a last-resort interventional measure for refractory epilepsy, it carries the risk of surgical damage to otherwise healthy neural tissues, along with resultant aftereffects, such as morbidity and even permanent dysfunction (Jobst and Cascino, 2015). Although high-precision stereotactic stem cell transplantation would still be associated with neurosurgical risks, it has the advantage of leaving functional neural tissue in a relatively undisturbed state, and can also be truly restorative in nature.
Challenges and Opportunities
Transplantation of GABAergic interneurons/progenitors from mouse/rat embryos or mouse PSCs has been widely studied in the rodent models of epilepsy, and the initial results of human PSC-derived GABAergic progenitor transplantation are encouraging (Cunningham et al., 2014), offering hope for the realization of cell-based therapy for epilepsy (Figure 1). Nonetheless, some issues need to be addressed before transition into clinical use.
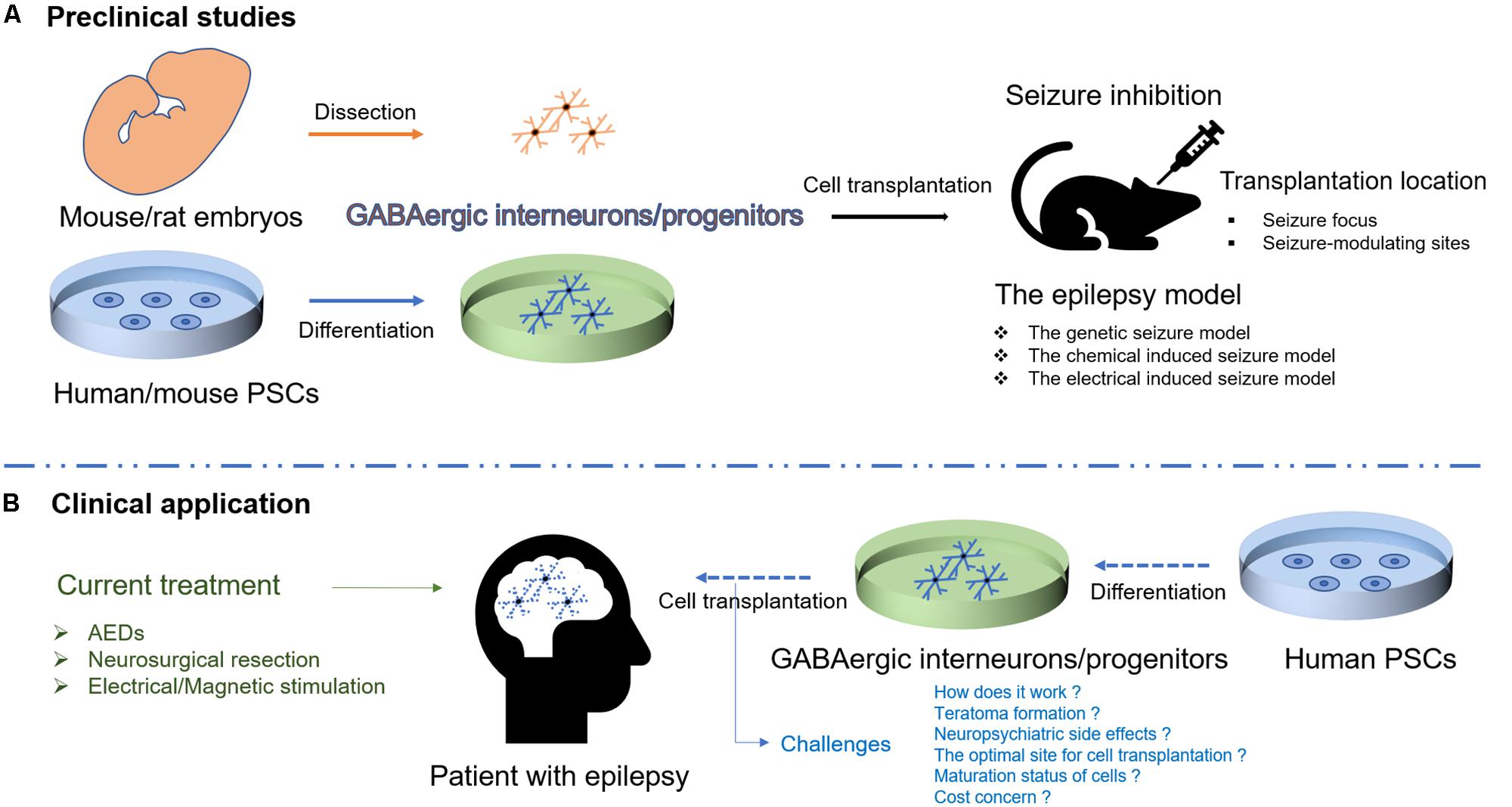
FIGURE 1. Schematic illustration of cell therapies for epilepsy. (A) Transplantation of GABAergic interneurons/progenitors from mouse/rat embryos or human/mouse PSCs has been studied in different rodent models of epilepsy. (B) Human PSC-derived GABAergic interneurons/progenitors would be a promising cell source for transplantation to target epileptic foci in humans though the challenges exist.
First, to better control seizures, basic mechanisms underlying seizure suppression have to be futher explored. Designer receptors exclusively activated by designer drugs (DREADDs) can be used to dissect the neural circuits responsible for different behaviors by activating or inhibiting specific neurons through modulating G protein-coupled receptor activity (Whissell et al., 2016). With this approach, the relationship between the activities of engrafted neurons and the seizure phenotype can be investigated in epilepsy models. Additionally, utilization of DREADDs could help neuroscientists determine whether seizure suppression is a result of the synaptic inhibition provided by the engrafted cells or other anticonvulsant mechanisms (e.g., graft release of neurotrophic factors including glial cell-line-derived neurotrophic factor) (Li et al., 2002; Simonato et al., 2006; Hattiangady and Shetty, 2011; Shetty, 2012).
Second, the safety of the cell preparations should be critically evaluated before transplantation. Teratoma formation is closely correlated to the persistence of proliferating or undifferentiated PSCs after transplantation (Blum and Benvenisty, 2008; Miura et al., 2009; Germain et al., 2012). Thus, for therapeutic purposes, isolating the optimal cell population before implantation will be critical for ensuring patient safety. Purifying the desired cell type by fluorescence activated cell sorting (FACS) is one approach that has been used successfully to reduce teratoma formation after transplantation (Chung et al., 2006). The ideal cell types should be highly migratory for optimal integration into host brain circuity after transplantation. In addition to the prevention of tumor formation, optimal cell density needs to be carefully determined to prevent potential adverse neuropsychiatric effects in vivo. None of the studies reported adverse effects of interneuron transplantation so far in the rodents. Instead, there are plethora of studies with interneuron transplantation that have shown functional benefits not only in seizure reduction but also in normalization of other behavioral abnormalities such as cognition, memory, aggressive behavior and motor symptoms in various animal models of brain disease such as epilepsy, schizophrenia and Parkinson’s (Martínez-Cerdeño et al., 2010; Liu et al., 2013b; Cunningham et al., 2014; Southwell et al., 2014; Donegan et al., 2016). Interestingly, it has been shown that the inhibition following cortical interneuron transplantation reaches a plateau with relatively low numbers of grafted cells (Southwell et al., 2014), suggesting that a larger number of cortical interneurons are not likely to cause abnormal behavior after cell transplantation. Still, the neuropsychiatric side effects need to be carefully examined in depth in the primate model that more closely matches the higher cognitive functions of the human brain. To further ensure the safety of grafts, chemogenetic modulation or suicide gene regulation may be incorporated to provide the ability to eliminate any potential adverse effects caused by the transplanted cells (Tey et al., 2007; Chen et al., 2016).
Third, the optimal site for interneuron transplantation must be determined, which is especially important when the seizure focus can not be clearly identified or there are multiple foci. As summarized above, seizure suppression has been observed following transplantation of interneurons to the seizure focus (Hunt et al., 2013; Henderson et al., 2014), but in addition, other brain regions such as SN or STN were shown to be effective sites for in modulating seizure activity in rat epilepsy models (Löscher et al., 1998; Handreck et al., 2012). Moreover, interneuron transplantation into part of epileptic brain region, providing limited innervation of the seizure focus, has also been found to reduce seizures or elevated seizure thresholds (Baraban et al., 2009; Calcagnotto et al., 2010; Hammad et al., 2014). Thus, the optimal sites of cell transplantation and distribution of the transplanted cells need to be carefully compared and determined for each seizure disorder.
Fourth, the process of maturation and differentiation in human PSC-derived MGE progenitor cells is lengthy, and appears to follow their innate in vivo time line in the human embryos (Nicholas et al., 2013). Developing interneurons integrate well into adult epileptic circuitry, receive excitatory inputs from the host brain, inhibit host neurons by releasing GABA and ameliorate seizures prior to attaining fully mature electrophysiological properties (e.g., Fast spiking) (Cunningham et al., 2014), but more efficient approaches need to be developed to speed up the maturation of transplanted cells with the purpose of accelerating the development of seizure suppression after grafting.
Finally, to overcome the requirement that recipients of grafts undergo long-term immunosuppression to prevent graft rejection, personalized stem cell treatments have been proposed. Personalized cell therapy uses the patient’s own iPSCs to generate needed neurons, however, this approach could easily cost hundreds of thousands of dollars (Lee et al., 2000). To overcome the prohibitive cost issue associated with personalized cell therapy, tissue banks could be established for human leukocyte antigen (HLA)-matching donor cells. This is an achievable goal for reducing costs and overcomes hazards associated with long-term immunosuppression and graft rejection (Taylor et al., 2012; Solomon et al., 2014; Cyranoski, 2017).
As the mechanisms for seizure control by interneuron grafts become better understood, and our ability to regulate the integration, proliferation, and function of engrafted cells becomes more refined, we envision a time in the future when patients with intractable seizure disorders may consider hPSC-based interneuron transplantation therapy as a safe and effective treatment option.
Author Contributions
QZ wrote initial drafts. SC and JRN provided expert feedback and edited the manuscript.
Funding
This study was supported by NIH Grants MH107884 (SC), NINDS grant R15NS072879-01A1 (JRN), Connecticut Regenerative Medicine Research Fund Multi-Investigator Grant No. 13-SCC-WES-01 (JRN), and a CURE Epilepsy Challenge Award (JRN), as well as Undergraduate Research Fellowship funding from Randolph and Lisa Siegel, and Wesleyan University (JRN).
Conflict of Interest Statement
The authors declare that the research was conducted in the absence of any commercial or financial relationships that could be construed as a potential conflict of interest.
References
Armstrong, C., Krook-Magnuson, E., Oijala, M., and Soltesz, I. (2013). Closed-loop optogenetic intervention in mice. Nat. Protoc. 8, 1475–1493. doi: 10.1038/nprot.2013.080
Baraban, S. C., Southwell, D. G., Estrada, R. C., Jones, D. L., Sebe, J. Y., Alfaro-Cervello, C., et al. (2009). Reduction of seizures by transplantation of cortical GABAergic interneuron precursors into Kv1.1 mutant mice. Proc. Natl. Acad. Sci. U.S.A. 106, 15472–15477. doi: 10.1073/pnas.0900141106
Barry, D. I., Kikvadze, I., Brundin, P., Bolwig, T. G., Björklund, A., and Lindvall, O. (1987). Grafted noradrenergic neurons suppress seizure development in kindling-induced epilepsy. Proc. Natl. Acad. Sci. U.S.A. 84, 8712–8715. doi: 10.1073/pnas.84.23.8712
Bengzon, J., Kokaia, M., Brundin, P., and Lindvall, O. (1990). Seizure suppression in kindling epilepsy by intrahippocampal locus coeruleus grafts: evidence for an alpha-2-adrenoreceptor mediated mechanism. Exp. Brain Res. 81, 433–437. doi: 10.1007/BF00228137
Bertram, E. H. (2014). Electrophysiology in epilepsy surgery: roles and limitations. Ann. Indian Acad. Neurol. 17, S40–S44. doi: 10.4103/0972-2327.128649
Bjarkam, C. R., Glud, A. N., Margolin, L., Reinhart, K., Franklin, R., Deding, D., et al. (2009). Safety and function of a new clinical intracerebral microinjection instrument for stem cells and therapeutics examined in the Göttingen minipig. Stereotact. Funct. Neurosurg. 88, 56–63. doi: 10.1159/000268743
Blum, B., and Benvenisty, N. (2008). The tumorigenicity of human embryonic stem cells. Adv. Cancer Res. 100, 133–158. doi: 10.1016/S0065-230X(08)00005-5
Bortolotto, Z. A., Calderazzo, L., and Cavalheiro, E. A. (1990). Some evidence that intrahippocampal grafting of noradrenergic neurons suppresses spontaneous seizures in epileptic rats. Braz. J. Med. Biol. Res. 23, 1267–1269.
Buckmaster, P. S., Yamawaki, R., and Zhang, G. F. (2002). Axon arbors and synaptic connections of a vulnerable population of interneurons in the dentate gyrus in vivo. J. Comp. Neurol. 445, 360–373. doi: 10.1002/cne.10183
Buzsáki, G., Kaila, K., and Raichle, M. (2007). Inhibition and brain work. Neuron 56, 771–783. doi: 10.1016/j.neuron.2007.11.008
Calcagnotto, M. E., Ruiz, L. P., Blanco, M. M., Santos-Junior, J. G., Valente, M. F., Patti, C., et al. (2010). Effect of neuronal precursor cells derived from medial ganglionic eminence in an acute epileptic seizure model. Epilepsia 51(Suppl. 3), 71–75. doi: 10.1111/j.1528-1167.2010.02614.x
Chen, Y., Xiong, M., Dong, Y., Haberman, A., Cao, J., Liu, H., et al. (2016). Chemical control of grafted Human PSC-Derived neurons in a mouse model of Parkinson’s Disease. Cell Stem Cell 18, 817–826. doi: 10.1016/j.stem.2016.03.014
Chohan, M. O., and Moore, H. (2016). Interneuron progenitor transplantation to Treat CNS dysfunction. Front. Neural Circuits 10:64. doi: 10.3389/fncir.2016.00064
Chu, K., Kim, M., Jung, K.-H., Jeon, D., Lee, S.-T., Kim, J., et al. (2004). Human neural stem cell transplantation reduces spontaneous recurrent seizures following pilocarpine-induced status epilepticus in adult rats. Brain Res. 1023, 213–221. doi: 10.1016/j.brainres.2004.07.045
Chung, S., Shin, B.-S., Hedlund, E., Pruszak, J., Ferree, A., Kang, U. J., et al. (2006). Genetic selection of sox1GFP-expressing neural precursors removes residual tumorigenic pluripotent stem cells and attenuates tumor formation after transplantation. J. Neurochem. 97, 1467–1480. doi: 10.1111/j.1471-4159.2006.03841.x
Cunningham, M., Cho, J.-H., Leung, A., Savvidis, G., Ahn, S., Moon, M., et al. (2014). hPSC-derived maturing GABAergic interneurons ameliorate seizures and abnormal behavior in epileptic mice. Cell Stem Cell 15, 559–573. doi: 10.1016/j.stem.2014.10.006
Cyranoski, D. (2017). Japanese man is first to receive “reprogrammed”stem cells from another person. Nature
de Lanerolle, N. C., Kim, J. H., Robbins, R. J., and Spencer, D. D. (1989). Hippocampal interneuron loss and plasticity in human temporal lobe epilepsy. Brain Res. 495, 387–395. doi: 10.1016/0006-8993(89)90234-5
de Tisi, J., Bell, G. S., Peacock, J. L., McEvoy, A. W., Harkness, W. F. J., Sander, J. W., et al. (2011). The long-term outcome of adult epilepsy surgery, patterns of seizure remission, and relapse: a cohort study. Lancet 378, 1388–1395. doi: 10.1016/S0140-6736(11)60890-8
Donegan, J. J., Tyson, J. A., Branch, S. Y., Beckstead, M. J., Anderson, S. A., and Lodge, D. J. (2016). Stem cell-derived interneuron transplants as a treatment for schizophrenia: preclinical validation in a rodent model. Mol. Psychiatry 22, 1492–1501. doi: 10.1038/mp.2016.121
Engel, J. (2014). Approaches to refractory epilepsy. Ann. Indian Acad. Neurol. 17, S12–S17. doi: 10.4103/0972-2327.128644
Fisher, R. S. (2017). The new classification of seizures by the international league against epilepsy 2017. Curr. Neurol. Neurosci. Rep. 17:48. doi: 10.1007/s11910-017-0758-6
Fisher, R. S., Cross, J. H., French, J. A., Higurashi, N., Hirsch, E., Jansen, F. E., et al. (2017). Operational classification of seizure types by the international league against epilepsy: position paper of the ILAE commission for classification and terminology. Epilepsia 58, 522–530. doi: 10.1111/epi.13670
Germain, N. D., Banda, E. C., Becker, S., Naegele, J. R., and Grabel, L. B. (2013). Derivation and isolation of NKX2.1-positive basal forebrain progenitors from human embryonic stem cells. Stem Cells Dev. 22, 1477–1489. doi: 10.1089/scd.2012.0264
Germain, N. D., Hartman, N. W., Cai, C., Becker, S., Naegele, J. R., and Grabel, L. B. (2012). Teratocarcinoma formation in embryonic stem cell-derived neural progenitor hippocampal transplants. Cell Transplant. 21, 1603–1611. doi: 10.3727/096368912X647243
Goldberg, E. M., and Coulter, D. A. (2013). Mechanisms of epileptogenesis: a convergence on neural circuit dysfunction. Nat. Rev. Neurosci. 14, 337–349. doi: 10.1038/nrn3482
Goulburn, A. L., Alden, D., Davis, R. P., Micallef, S. J., Ng, E. S., Yu, Q. C., et al. (2011). A targeted NKX2.1 human embryonic stem cell reporter line enables identification of human basal forebrain derivatives. Stem Cells 29, 462–473. doi: 10.1002/stem.587
Hammad, M., Schmidt, S. L., Zhang, X., Bray, R., Frohlich, F., and Ghashghaei, H. T. (2014). Transplantation of GABAergic interneurons into the neonatal primary visual cortex reduces absence seizures in stargazer mice. Cereb. Cortex 25, 2970–2979. doi: 10.1093/cercor/bhu094
Handreck, A., Backofen-Wehrhahn, B., Bröer, S., Löscher, W., and Gernert, M. (2012). Anticonvulsant effects by bilateral and unilateral transplantation of GABA-producing cells into the subthalamic nucleus in an acute seizure model. Cell Transplant. 23, 111–132. doi: 10.3727/096368912X658944
Hattiangady, B., Rao, M. S., and Shetty, A. K. (2008). Grafting of striatal precursor cells into hippocampus shortly after status epilepticus restrains chronic temporal lobe epilepsy. Exp. Neurol. 212, 468–481. doi: 10.1016/j.expneurol.2008.04.040
Hattiangady, B., and Shetty, A. K. (2011). Neural stem cell grafting in an animal model of chronic temporal lobe epilepsy. Curr. Protoc. Stem Cell Biol. Chapter 2:Unit2D.7. doi: 10.1002/9780470151808.sc02d07s18
Heck, C. N., King-Stephens, D., Massey, A. D., Nair, D. R., Jobst, B. C., Barkley, G. L., et al. (2014). Two-year seizure reduction in adults with medically intractable partial onset epilepsy treated with responsive neurostimulation: final results of the RNS System Pivotal trial. Epilepsia 55, 432–441. doi: 10.1111/epi.12534
Henderson, K. W., Gupta, J., Tagliatela, S., Litvina, E., Zheng, X., Van Zandt, M. A., et al. (2014). Long-term seizure suppression and optogenetic analyses of synaptic connectivity in epileptic mice with hippocampal grafts of GABAergic interneurons. J. Neurosci. 34, 13492–13504. doi: 10.1523/JNEUROSCI.0005-14.2014
Herman, S. (2010). Intractable epilepsy: relapsing, remitting, or progressive? Epilepsy Curr. 10, 146–148. doi: 10.1111/j.1535-7511.2010.01383.x
Ho, K. S., Markham, L. M., Twede, H., Lortz, A., Olson, L. M., Sheng, X., et al. (2018). A survey of antiepileptic drug responses identifies drugs with potential efficacy for seizure control in Wolf-Hirschhorn syndrome. Epilepsy Behav. 81, 55–61. doi: 10.1016/j.yebeh.2017.12.008
Hofmann, G., Balgooyen, L., Mattis, J., Deisseroth, K., and Buckmaster, P. S. (2016). Hilar somatostatin interneuron loss reduces dentate gyrus inhibition in a mouse model of temporal lobe epilepsy. Epilepsia 57, 977–983. doi: 10.1111/epi.13376
Hovinga, C. A. (2001). Levetiracetam: a novel antiepileptic drug. Pharmacotherapy 21, 1375–1388. doi: 10.1592/phco.21.17.1375.34432
Hunt, R. F., and Baraban, S. C. (2015). Interneuron transplantation as a treatment for epilepsy. Cold Spring Harb. Perspect. Med. 5:a022376. doi: 10.1101/cshperspect.a022376
Hunt, R. F., Girskis, K. M., Rubenstein, J. L., Alvarez-Buylla, A., and Baraban, S. C. (2013). GABA progenitors grafted into the adult epileptic brain control seizures and abnormal behavior. Nat. Neurosci. 16, 692–697. doi: 10.1038/nn.3392
Jobst, B. C., and Cascino, G. D. (2015). Resective epilepsy surgery for drug-resistant focal epilepsy: a review. JAMA 313, 285–293. doi: 10.1001/jama.2014.17426
Kelsom, C., and Lu, W. (2013). Development and specification of GABAergic cortical interneurons. Cell Biosci. 3:19. doi: 10.1186/2045-3701-3-19
Kim, T.-G., Yao, R., Monnell, T., Cho, J.-H., Vasudevan, A., Koh, A., et al. (2014). Efficient specification of interneurons from human pluripotent stem cells by dorsoventral and rostrocaudal modulation. Stem Cells 32, 1789–1804. doi: 10.1002/stem.1704
Kneen, R., and Appleton, R. E. (2006). Alternative approaches to conventional antiepileptic drugs in the management of paediatric epilepsy. Arch. Dis. Child. 91, 936–941. doi: 10.1136/adc.2005.080002
Kobayashi, M., and Buckmaster, P. S. (2003). Reduced inhibition of dentate granule cells in a model of temporal lobe epilepsy. J. Neurosci. 23, 2440–2452. doi: 10.1523/JNEUROSCI.23-06-02440.2003
Krook-Magnuson, E., Armstrong, C., Oijala, M., and Soltesz, I. (2013). On-demand optogenetic control of spontaneous seizures in temporal lobe epilepsy. Nat. Commun. 4:1376. doi: 10.1038/ncomms2376
Laxer, K. D., Trinka, E., Hirsch, L. J., Cendes, F., Langfitt, J., Delanty, N., et al. (2014). The consequences of refractory epilepsy and its treatment. Epilepsy Behav. 37, 59–70. doi: 10.1016/j.yebeh.2014.05.031
Lee, S. J., Klar, N., Weeks, J. C., and Antin, J. H. (2000). Predicting costs of stem-cell transplantation. J. Clin. Oncol. 18, 64–71. doi: 10.1200/JCO.2000.18.1.64
Li, S., Martin, D., Racine, R. J., and Fahnestock, M. (2002). Glial cell line-derived neurotrophic factor modulates kindling and activation-induced sprouting in hippocampus of adult rats. Exp. Neurol. 178, 49–58. doi: 10.1006/exnr.2002.8036
Lin, Y., and Wang, Y. (2017). Neurostimulation as a promising epilepsy therapy. Epilepsia Open 2, 371–387. doi: 10.1002/epi4.12070
Liu, J.-T., Liu, B., and Zhang, H. (2018). Surgical versus medical treatment of drug-resistant epilepsy: a systematic review and meta-analysis. Epilepsy Behav. 82, 179–188. doi: 10.1016/j.yebeh.2017.11.012
Liu, Y., Liu, H., Sauvey, C., Yao, L., Zarnowska, E. D., and Zhang, S.-C. (2013a). Directed differentiation of forebrain GABA interneurons from human pluripotent stem cells. Nat. Protoc. 8, 1670–1679. doi: 10.1038/nprot.2013.106
Liu, Y., Weick, J. P., Liu, H., Krencik, R., Zhang, X., and Ma, L. (2013b). Medial ganglionic eminence-like cells derived from human embryonic stem cells correct learning and memory deficits. Nature 31, 440–447. doi: 10.1038/nbt.2565
Löscher, W., Ebert, U., Lehmann, H., Rosenthal, C., and Nikkhah, G. (1998). Seizure suppression in kindling epilepsy by grafts of fetal GABAergic neurons in rat substantia nigra. J. Neurosci. Res. 51, 196–209. doi: 10.1002/(SICI)1097-4547(19980115)51:2<196::AID-JNR8>3.0.CO;2-8
Maisano, X., Litvina, E., Tagliatela, S., Aaron, G. B., Grabel, L. B., and Naegele, J. R. (2012). Differentiation and functional incorporation of embryonic stem cell-derived GABAergic interneurons in the dentate gyrus of mice with temporal lobe epilepsy. J. Neurosci. 32, 46–61. doi: 10.1523/JNEUROSCI.2683-11.2012
Markram, H., Toledo-Rodriguez, M., Wang, Y., Gupta, A., Silberberg, G., and Wu, C. (2004). Interneurons of the neocortical inhibitory system. Nat. Rev. Neurosci. 5, 793–807. doi: 10.1038/nrn1519
Maroof, A. M., Keros, S., Tyson, J. A., Ying, S.-W., Ganat, Y. M., Merkle, F. T., et al. (2013). Directed differentiation and functional maturation of cortical interneurons from human embryonic stem cells. Cell Stem Cell 12, 559–572. doi: 10.1016/j.stem.2013.04.008
Martínez-Cerdeño, V., Noctor, S. C., Espinosa, A., Ariza, J., Parker, P., Orasji, S., et al. (2010). Embryonic MGE precursor cells grafted into adult rat striatum integrate and ameliorate motor symptoms in 6-OHDA-lesioned rats. Cell Stem Cell 6, 238–250. doi: 10.1016/j.stem.2010.01.004
Miura, K., Okada, Y., Aoi, T., Okada, A., Takahashi, K., Okita, K., et al. (2009). Variation in the safety of induced pluripotent stem cell lines. Nature 27, 743–745. doi: 10.1038/nbt.1554
Nicholas, C. R., Chen, J., Tang, Y., Southwell, D. G., Chalmers, N., Vogt, D., et al. (2013). Functional maturation of hPSC-derived forebrain interneurons requires an extended timeline and mimics human neural development. Cell Stem Cell 12, 573–586. doi: 10.1016/j.stem.2013.04.005
Pais-Vieira, M., Yadav, A. P., Moreira, D., Guggenmos, D., Santos, A., Lebedev, M., et al. (2016). A Closed loop brain-machine interface for epilepsy control using dorsal column electrical stimulation. Sci. Rep. 6:32814. doi: 10.1038/srep32814
Pal, D. K. (2007). Phenobarbital for childhood epilepsy: systematic review. Paediatr. Perinat. Drug Ther. 7, 31–42. doi: 10.1185/146300905X75361
Pelkey, K. A., Chittajallu, R., Craig, M. T., Tricoire, L., Wester, J. C., and McBain, C. J. (2017). Hippocampal GABAergic Inhibitory Interneurons. Physiol. Rev. 97, 1619–1747. doi: 10.1152/physrev.00007.2017
Pi, H.-J., Hangya, B., Kvitsiani, D., Sanders, J. I., Huang, Z. J., and Kepecs, A. (2013). Cortical interneurons that specialize in disinhibitory control. Nature 503, 521–524. doi: 10.1038/nature12676
Ribak, C. E., Bradburne, R. M., and Harris, A. B. (1982). A preferential loss of GABAergic, symmetric synapses in epileptic foci: a quantitative ultrastructural analysis of monkey neocortex. J. Neurosci. 2, 1725–1735. doi: 10.1523/JNEUROSCI.02-12-01725.1982
Scharfman, H. E. (1999). The role of nonprincipal cells in dentate gyrus excitability and its relevance to animal models of epilepsy and temporal lobe epilepsy. Adv. Neurol. 79, 805–820.
Scharfman, H. E., and Myers, C. E. (2013). Hilar mossy cells of the dentate gyrus: a historical perspective. Front. Neural Circuits 6:106. doi: 10.3389/fncir.2012.00106
Scheffer, I. E., Berkovic, S., Capovilla, G., Connolly, M. B., French, J., Guilhoto, L., et al. (2017). ILAE classification of the epilepsies: position paper of the ILAE Commission for Classification and Terminology. Epilepsia 58, 512–521. doi: 10.1111/epi.13709
Shetty, A. K. (2012). Neural stem cell therapy for temporal lobe. Epilepsy 51:92. doi: 10.1093/med/9780199746545.003.0085
Simonato, M., Tongiorgi, E., and Kokaia, M. (2006). Angels and demons: neurotrophic factors and epilepsy. Trends Pharmacol. Sci. 27, 631–638. doi: 10.1016/j.tips.2006.10.002
Singh, A., and Trevick, S. (2016). The epidemiology of global epilepsy. Neurol. Clin. 34, 837–847. doi: 10.1016/j.ncl.2016.06.015
Sloviter, R. S. (1987). Decreased hippocampal inhibition and a selective loss of interneurons in experimental epilepsy. Science 235, 73–76. doi: 10.1126/science.2879352
Solomon, S., Pitossi, F., and Rao, M. S. (2014). Banking on iPSC–is it doable and is it worthwhile. Stem Cell Rev. 11, 1–10. doi: 10.1007/s12015-014-9574-4
Southwell, D. G., Nicholas, C. R., Basbaum, A. I., Stryker, M. P., Kriegstein, A. R., Rubenstein, J. L., et al. (2014). Interneurons from embryonic development to cell-based therapy. Science 344:1240622. doi: 10.1126/science.1240622
Sperling, M. R. (2004). The consequences of uncontrolled epilepsy. CNS Spectr. 9, 106–109. doi: 10.1017/S1092852900008464
Taylor, C. J., Peacock, S., Chaudhry, A. N., Bradley, J. A., and Bolton, E. M. (2012). Generating an iPSC bank for HLA-matched tissue transplantation based on known donor and recipient HLA types. Cell Stem Cell 11, 147–152. doi: 10.1016/j.stem.2012.07.014
Tey, S.-K., Dotti, G., Rooney, C. M., Heslop, H. E., and Brenner, M. K. (2007). Inducible caspase 9 suicide gene to improve the safety of allodepleted T cells after haploidentical stem cell transplantation. Biol. Blood Marrow Transplant. 13, 913–924. doi: 10.1016/j.bbmt.2007.04.005
Tyson, J. A., and Anderson, S. A. (2014). GABAergic interneuron transplants to study development and treat disease. Trends Neurosci. 37, 169–177. doi: 10.1016/j.tins.2014.01.003
Walia, K. S., Khan, E. A., Ko, D. H., Raza, S. S., and Khan, Y. N. (2006). Side effects of antiepileptics–a review. Pain Pract. 4, 194–203. doi: 10.1111/j.1533-2500.2004.04304.x
Whissell, P. D., Tohyama, S., and Martin, L. J. (2016). The use of DREADDs to deconstruct behavior. Front. Genet. 7:70. doi: 10.3389/fgene.2016.00070
Keywords: intractable epilepsy, GABAergic interneurons, cell transplantation, animal models, human pluripotent stem cells, differentiation
Citation: Zhu Q, Naegele JR and Chung S (2018) Cortical GABAergic Interneuron/Progenitor Transplantation as a Novel Therapy for Intractable Epilepsy. Front. Cell. Neurosci. 12:167. doi: 10.3389/fncel.2018.00167
Received: 31 January 2018; Accepted: 30 May 2018;
Published: 26 June 2018.
Edited by:
Jan Tønnesen, Achucarro Basque Center for Neuroscience, SpainReviewed by:
Luiz E. Mello, Federal University of São Paulo, BrazilQing Yun Wang, Beihang University, China
Copyright © 2018 Zhu, Naegele and Chung. This is an open-access article distributed under the terms of the Creative Commons Attribution License (CC BY). The use, distribution or reproduction in other forums is permitted, provided the original author(s) and the copyright owner are credited and that the original publication in this journal is cited, in accordance with accepted academic practice. No use, distribution or reproduction is permitted which does not comply with these terms.
*Correspondence: Janice R. Naegele, jnaegele@wesleyan.edu Sangmi Chung, schung8@nymc.edu; sangmichung@gmail.com