- 1Biomedical Neuroscience Institute (BNI), Faculty of Medicine, Universidad de Chile, Santiago, Chile
- 2Physiology and Biophysics Program, Institute of Biomedical Sciences, Faculty of Medicine, Universidad de Chile, Santiago, Chile
- 3Pathophysiology Program, Institute of Biomedical Sciences, Faculty of Medicine, Universidad de Chile, Santiago, Chile
- 4Systemic and Cellular Neurophysiology, Physiology Institute I, Medical Faculty, University of Freiburg, Freiburg Germany
- 5Pathology and Physiology Department, Medical School, Faculty of Medicine, Universidad de Valparaíso, Valparaíso, Chile
- 6Department of Neuroscience and Center of Molecular Studies of the Cell, Faculty of Medicine, Universidad de Chile, Santiago, Chile
The induction of both long-term potentiation (LTP) and long-term depression (LTD) of synaptic transmission entails pre- and postsynaptic Ca2+ signals, which represent transient increments in cytoplasmic free Ca2+ concentration. In diverse synapse types, Ca2+ release from intracellular stores contributes to amplify the Ca2+ signals initially generated by activation of neuronal Ca2+ entry pathways. Here, we used hippocampal slices from young male rats to evaluate whether pharmacological activation or inhibition of Ca2+ release from the endoplasmic reticulum (ER) mediated by ryanodine receptor (RyR) channels modifies LTD induction at Schaffer collateral-CA1 synapses. Pre-incubation of slices with ryanodine (1 μM, 1 h) or caffeine (1 mM, 30 min) to promote RyR-mediated Ca2+ release facilitated LTD induction by low frequency stimulation (LFS), but did not affect the amplitude of synaptic transmission, the profiles of field excitatory postsynaptic potentials (fEPSP) or the paired-pulse (PP) responses. Conversely, treatment with inhibitory ryanodine (20 μM, 1 h) to suppress RyR-mediated Ca2+ release prevented LTD induction, but did not affect baseline synaptic transmission or PP responses. Previous literature reports indicate that LTD induction requires presynaptic CaMKII activity. We found that 1 h after applying the LTD induction protocol, slices displayed a significant increase in CaMKII phosphorylation relative to the levels exhibited by un-stimulated (naïve) slices. In addition, LTD induction (1 h) enhanced the phosphorylation of the presynaptic protein Synapsin I at a CaMKII-dependent phosphorylation site, indicating that LTD induction stimulates presynaptic CaMKII activity. Pre-incubation of slices with 20 μM ryanodine abolished the increased CaMKII and Synapsin I phosphorylation induced by LTD, whereas naïve slices pre-incubated with inhibitory ryanodine displayed similar CaMKII and Synapsin I phosphorylation levels as naïve control slices. We posit that inhibitory ryanodine suppressed LTD-induced presynaptic CaMKII activity, as evidenced by the suppression of Synapsin I phosphorylation induced by LTD. Accordingly, we propose that presynaptic RyR-mediated Ca2+ signals contribute to LTD induction at Schaffer collateral-CA1 synapses.
Introduction
Evidence gathered in the last three decades supports long-term potentiation (LTP) and long-term depression (LTD) as likely cellular correlates of associative learning and long-term memory (Dudek and Bear, 1992; Bliss and Collingridge, 1993; Bear and Abraham, 1996; Malenka and Bear, 2004; Whitlock et al., 2006; Raymond, 2007; Citri and Malenka, 2008; Lynch et al., 2014; Nabavi et al., 2014). Both LTP and LTD require increments in postsynaptic intracellular Ca2+ concentration (Mulkey and Malenka, 1992), which occur initially by means of Ca2+ influx through N-methyl-D-aspartate (NMDA) receptors or voltage-gated Ca2+ channels (Dudek and Bear, 1992; Mulkey and Malenka, 1992; Citri and Malenka, 2008). In addition, LTD induction entails presynaptic CaMKII activation, whereas postsynaptic CaMKII stimulation is required to evoke LTP (Stanton and Gage, 1996; Margrie et al., 1998).
Substantial evidence implicates ensuing amplification of Ca2+ entry signals by means of Ca2+ release from intracellular stores mediated by the two types of Ca2+ release channels present in the endoplasmic reticulum (ER), the inositol 1,4,5-trisphosphate (IP3) receptor (IP3R) and the ryanodine receptor (RyR) channels (Berridge, 1998, 2002). The IP3R channels, which play an important role in neuronal function, reside predominantly in the cerebellum (Hisatsune and Mikoshiba, 2017) and the hippocampus (Sharp et al., 1993; Hertle and Yeckel, 2007). The RyR channels, which are widely expressed in the brain (Furuichi et al., 1994; Zhao et al., 2000), play central roles in synaptic plasticity (Reyes and Stanton, 1996; Lu and Hawkins, 2002; Raymond and Redman, 2002; Mellentin et al., 2007; Grigoryan et al., 2012). In addition, RyR channel activation facilitates learning and memory formation whereas inhibition of RyR activity or expression impairs these processes (Zhao et al., 2000; Edwards and Rickard, 2006; Galeotti et al., 2008; Adasme et al., 2011; Hidalgo and Arias-Cavieres, 2016; More et al., 2018).
As implied by its name, RyR channels bind selectively and with high affinity the plant alkaloid ryanodine (Jenden and Fairhurst, 1969). Ryanodine at low concentrations (≤1 μM) activates RyR channels, while higher ryanodine concentrations (≥10 μM) inhibit RyR single channel activity (Meissner, 1986; Bull et al., 1989; Mackrill, 2010). We have reported previously that inhibitory ryanodine concentrations (≥10 μM) suppress RyR-mediated Ca2+ release induced by high-frequency stimulation of primary hippocampal neurons (Riquelme et al., 2011) but do not affect ER calcium content (Adasme et al., 2015). Considering that ryanodine binds predominantly to open RyR channels, full inhibition of RyR-mediated Ca2+ release requires prolonged incubation (≥1 h) with inhibitory ryanodine. In addition, caffeine at mM concentration also promotes full RyR channel activation (McPherson et al., 1991); however, whereas ryanodine only targets RyR channels, caffeine also inhibits cyclic nucleotide phosphodiesterases (Sutherland and Rall, 1958) and IP3R channel activity (Bezprozvanny et al., 1994), and antagonizes adenosine receptors (Rall, 1982).
Previous reports have indicated that RyR channels play important roles in the LTP and LTD responses recorded in different hippocampal regions (Baker et al., 2013; Paula-Lima et al., 2014). In Schaffer collateral-CA1 synapses, RyR channel inhibition prevents LTP induction or maintenance in CA3–CA1 synapses, depending on the type of stimulation protocol used, theta-burst stimulation (TBS) or high-frequency stimulation (Lu and Hawkins, 2002; Raymond and Redman, 2002; Mellentin et al., 2007; Grigoryan et al., 2012). Inhibition of RyR channels prevents LTD induction in dentate gyrus (DG) synapses (Wang et al., 1997), while in CA3–CA3 synapses, presynaptic ryanodine-sensitive Ca2+ stores are required for NMDA receptor (NMDAR)-dependent LTD induction (Unni et al., 2004). Depletion of intracellular Ca2+ stores does not affect baseline synaptic transmission in CA3–CA1 synapses but blocks LTD induction in young rats (Reyes and Stanton, 1996). Moreover, based on the findings that bath application of inhibitory ryanodine (10 μM) blocks LTD induction whereas filling CA1 pyramidal neurons with ryanodine (2 μM to 5 mM) does not, the authors proposed that LTD induction requires Ca2+ release from presynaptic ryanodine-sensitive Ca2+ stores and from postsynaptic (presumably IP3-gated) stores (Reyes and Stanton, 1996). In contrast, a subsequent report proposed that postsynaptic calcium stores are critical for LTD induction (Nakano et al., 2004). In CA3–CA1 synapses, LTD induction at homo- and heterosynaptic sites requires functional RyR and IP3R channels, respectively (Nishiyama et al., 2000). Furthermore, knockout mice for the type-3 RyR (RyR3) isoform do not display LTD in CA3–CA1 synapses (Futatsugi et al., 1999), albeit the synaptic location of RyR3 channels was not established. In addition, RyR channels contribute to both the defective synaptic plasticity (Kumar and Foster, 2005) and the neuronal Ca2+ dysregulation displayed by aged animals (Gant et al., 2006, 2015; Bodhinathan et al., 2010). In particular, we recently reported that aged rats display increased RyR2 and RyR3 protein levels and significantly enhanced LTD in CA3–CA1 synapses (Arias-Cavieres et al., 2017). These combined results indicate that RyR channels play key roles in LTD induction in Schaffer collateral-CA1 synapses.
To examine if modifying RyR channel activity affects circuit excitability in hippocampal slices from young male rats (28–35 days old), we studied in Schaffer collateral-CA1 synapses the effects of low (1 μM) or high (20 μM) concentrations of ryanodine or of 1 mM caffeine on input/output (I/O) responses. We evaluated as well the effects of ryanodine and caffeine on fast presynaptic transmitter release dynamics by studying CA1 paired-pulse (PP) responses after stimulation of Schaffer collateral fibers, and assessed the effects of both drugs on LTD induction by low frequency stimulation (LFS) of hippocampal slices. We also examined in control slices and in slices treated with 20 μM ryanodine if LTD induction modified the phosphorylation levels of CaMKII, a protein expressed in pre- and postsynaptic terminals that has a key role in LTD induction (Stanton and Gage, 1996; Margrie et al., 1998; Coultrap and Bayer, 2012). To evaluate presynaptic CaMKII activity, we measured the phosphorylation of the presynaptic protein Synapsin I at a site that undergoes specific Ca2+-dependent phosphorylation by CaMKII (Cesca et al., 2010).
Materials and Methods
Materials
All reagents used were of analytical grade. Stock solutions of ryanodine (Tocris, Bristol, UK) and caffeine (Sigma, St. Louis, MO, USA) were dissolved in water and stored in aliquots at −20°C before thawing and dilution to their final concentrations in artificial cerebrospinal fluid (ACSF) solution (in mM: 124 NaCl, 5 KCl, 1 MgCl2, 2 CaCl2, 1.25 NaH2PO4, 26 NaHCO3, pH 7.4, 10 glucose). The ACSF solution was oxygenated with a mixture of 95% O2/5% CO2. Antibodies: anti-CamKII (pan) (D11A10) rabbit monoclonal antibody (#4436, 1:3,000) was from Cell Signaling Technology (Danvers, MA, USA). Phospho CaMKII (phospho T286) rabbit polyclonal antibody (ab32678, 1:5,000), anti-synapsin I rabbit polyclonal antibody (ab64581, 1:2,500) and anti-synapsin I (phospho S603) rabbit polyclonal antibody (ab13879, 1:1,500) were from Abcam (Cambridge, MA, USA).
Animals
Young (4–5 weeks) male Sprague Dawley rats were obtained from the Universidad de Chile animal facility. Food and water were provided ad libitum. Lights were maintained on a 12–12 light/dark cycle. All experiments were carried out following the guidelines provided by National Institute of Health (USA) and the regulations for the Care and Use of Animals for Scientific Purposes; the Bioethics Committee, F. Medicine, Universidad de Chile, approved all protocols used in this work.
Hippocampal Slice Preparation
Animals were sacrificed by decapitation under halothane anesthesia and their brains were quickly removed. The hippocampal tissue was removed, dissected and immersed in cold dissection buffer (in mM: 212.7 sucrose, 10 glucose, 5 KCl, 1.25 NaH2PO4, 2 MgCl2, 1 CaCl2, 26 NaHCO3, pH 7.4) and cut into 400 μm transversal slices with a VT 1000 S vibratome (Leica, Wetzlar, Germany). Hippocampal slices were transferred to an immersion storage chamber and were kept at room temperature for 1 h in ACSF solution, bubbled with 95% O2/5% CO2.
Electrophysiology
For field recordings slices were perfused with ACSF bubbled with 95% O2/5% CO2 (30 ± 2°C) at a rate of 2 ml/min. Synaptic transmission at the Schaffer collaterals-CA1 synapse was evoked by square current pulses (0.2 ms) delivered with a concentric bipolar stimulating electrode (FHC Inc., Bowdoinham, ME, USA) placed at the Schaeffer collateral–commissural fibers (Arias-Cavieres et al., 2017); field excitatory postsynaptic potentials (fEPSP) were recorded with ACSF-filled glass microelectrodes (2–3 MΩ) placed into the CA1 stratum radiatum region. Pulses of 50, 100, 150, 200 or 250 microamperes were applied to generate I/O response curves using a constant current stimulator (AM system, Washington, DC, USA). Signals were amplified and filtered at 10 kHz using a differential amplifier (AM system, Washington, DC, USA); signals were digitized using a national instruments board (NI PCI6221, Austin, TX, USA) and recorded using custom-made routines written in Igor Pro (WaveMetrics Inc., Lake Oswego, OR, USA). The experiment was discarded if a population spike was detected (Andersen et al., 1980).
PP experiments were performed to evaluate synaptic release dynamics. PPs were evoked every 15 s with time delays ranging from 20 ms to 640 ms. Plasticity experiments were performed after synaptic transmission and PP determinations. Pulses were delivered every 15 s, using stimulation intensities that evoked half-maximal fEPSP amplitudes. After collecting baseline responses for 15 min, an LFS protocol (1 Hz/900 pulses) was applied and fEPSPs were registered for 60 min to test for LTD induction.
Data Analysis of Electrophysiological Records
Data analysis was done using custom-made software written in Igor Pro (WaveMetrics Inc., Lake Oswego, OR, USA). Fiber volley (FV; Andersen et al., 1980) and fEPSP amplitudes were measured as the peak negative response from baseline. Rise times were measured as the time elapsed between reaching 20–80 percent of the peak fEPSP amplitude, and half-width time as the width of fEPSP traces at half amplitude. Decay constant (tau) vales were determined by fitting a single exponential function to the after-peak fEPSP waveform. Results from PP experiments are presented as fEPSP slope ratios. Plasticity experiments are presented as percent change of the initial fEPSP slopes.
Western Blot Analysis
Two-three hippocampal slices (400 μm each) from each rat were pooled and extracts were prepared as described (Arias-Cavieres et al., 2017). Proteins were resolved by SDS-PAGE using 3.5%–8% Tris-acetate gels (RyR2) and 10% gels (Synapsin I and CaMKII). Proteins were transferred to polyvinylidenedifluoride (PVDF) membranes, and probed with CaMKII, phospho-CaMKII, Synapsin I and phospho-Synapsin I antibodies. Image acquisition was performed by means of the Chemidoc™ MP System (Bio-Rad laboratories, Hercules, CA, USA); the ImageJ Lab software was used for band density analysis.
Immunohistochemistry
Free-floating sections for immunofluorescence were prepared as previously described (Muñoz et al., 2016). Naïve slices or slices exposed to LTD induction protocols (plus or minus 20 μM ryanodine in both cases), were fixed for 30 min in 4% paraformaldehyde/4% sucrose and then placed in 30% sucrose (w/v). The fixed slices were washed three times with phosphate-based saline buffer, embedded in medium for frozen tissue specimens and sectioned at −20°C with a cryostat to generate sections of 25 μm width. Free-floating sections were incubated overnight at 4°C with permeabilization/blocking buffer (0.7% Triton X-100, 0.1% sodium borohydride, 10% goat serum), and were subsequently incubated for 12 h at 4°C with phospho-CaMKII rabbit polyclonal antibody (phospho T286, ab32678, 1:500), or with phospho-Synapsin I rabbit polyclonal antibody (phospho S603, ab13879, 1:500). Both antibodies were from Abcam (Cambridge, MA, USA). After this incubation period, washed sections were incubated for 2 h with donkey-anti-rabbit Alexa Fluor 546 antibody (1:500, Fisher Scientific, Waltham, MA, USA). Nuclei were visualized with Hoechst 33342 (1:1,000, Fisher Scientific, Waltham, MA, USA). Images were acquired in a confocal microscope (Nikon Eclipse C180i, Melville, NY, USA).
Statistics
Statistical analysis was performed using the GraphPad Software (San Diego, CA, USA), as detailed in figure legends. All values represent Mean ± SE. For independent data sets, statistical differences between two mean values were assessed by two-tailed unpaired Student’s t-test provided values presented normal distribution, determined by the Shapiro-Wilk test. The nonparametric Mann-Whitney U test was used if values did not present normal distribution or the sample number was ≤ 5. Differences were considered statistically significant at p < 0.05.
Results
Effects of Ryanodine and Caffeine on Input-Output Responses
First, we explored if activating (1 μM) or inhibitory (20 μM) concentrations of ryanodine, as well as 1 mM caffeine, influenced signaling at the Schaffer collateral-CA1 synapses. To this aim, I/O curves were generated from recordings collected after 15 min of perfusion with control ACSF or with ACSF containing 1 μM ryanodine. At all tested stimulus intensities, 1 μM ryanodine had no effect on FV amplitudes (Figure 1A), fEPSP amplitudes (Figure 1B) or fEPSP slopes (Figure 1C). We evaluated next these three parameters in slices perfused for 60 min with 20 μM ryanodine. Treatment with inhibitory ryanodine did not affect FV amplitudes (Figure 1D), fEPSP amplitudes (Figure 1E) and fEPSP slopes (Figure 1F). Consistent with the lack of effect of stimulatory ryanodine, RyR activation by application of 1 mM caffeine did not affect FV amplitudes (Figure 1G), fEPSP amplitudes (Figure 1H) and fEPSP slopes (Figure 1I).
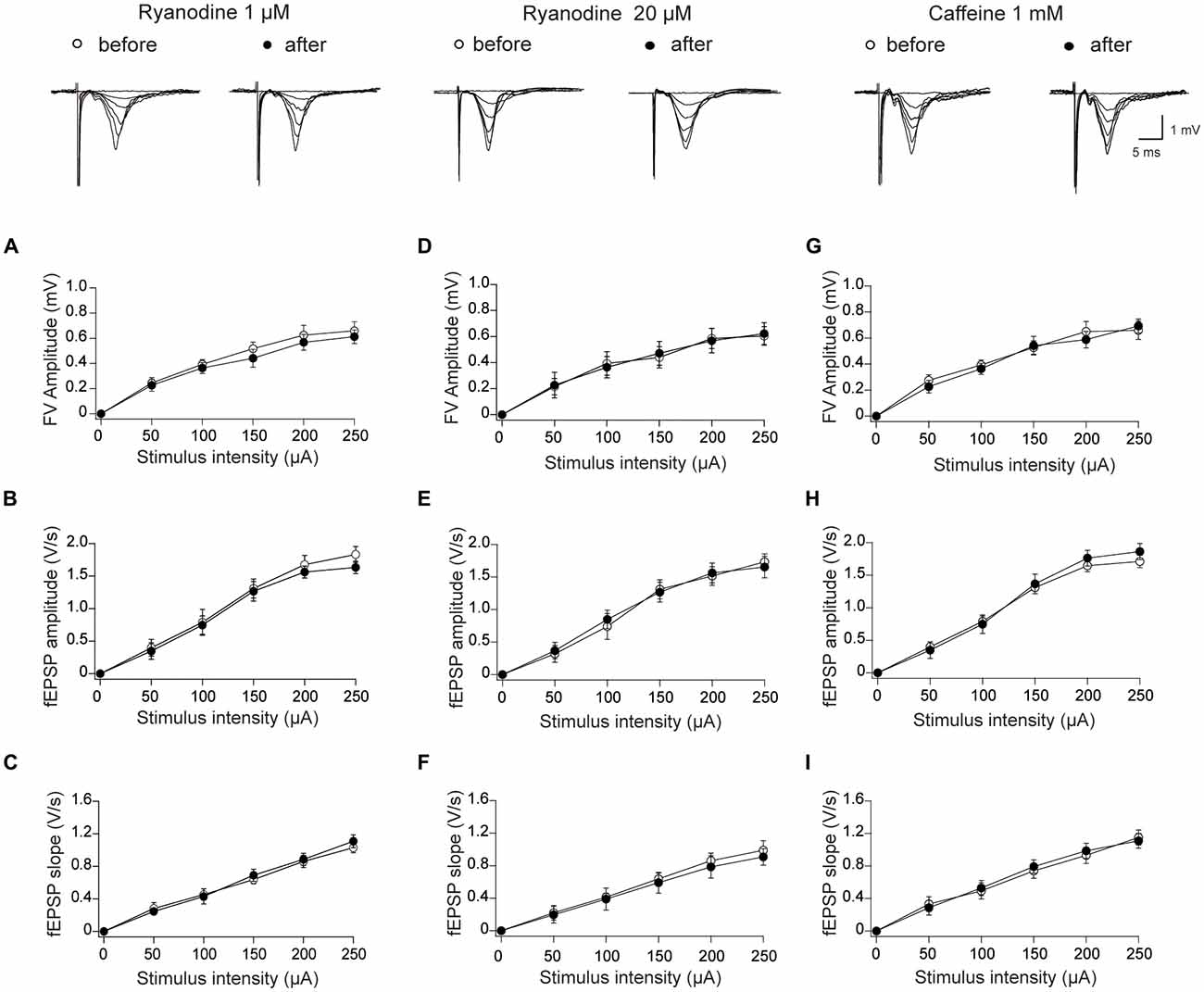
Figure 1. Effects of stimulatory and inhibitory ryanodine concentrations and of caffeine on basal synaptic transmission in the CA3–CA1 hippocampal synapse. Left panels: slices were treated with 1 μM ryanodine; representative field excitatory postsynaptic potential (fEPSP) traces registered before and 15 min after ryanodine addition are illustrated on top of the panels. (A) Fiber volley (FV) amplitude vs. stimulus intensity; (B) amplitude vs. stimulus intensity; (C) fEPSP slopes vs. stimulus intensity. Central panels: slices were treated with 20 μM ryanodine; representative fEPSP traces registered before and 60 min after ryanodine addition are illustrated on top. (D) FV amplitude vs. stimulus intensity; (E) fEPSP amplitude vs. stimulus intensity; (F) fEPSP slopes vs. stimulus intensity. Right Panels: slices were treated with 1 mM caffeine; representative fEPSP traces registered before and 15 min after caffeine addition are illustrated on top. (G) FV amplitude vs. stimulus intensity; (H) fEPSP amplitude vs. stimulus intensity; (I) fEPSP slopes vs. stimulus intensity. Values represent Mean ± SEM; (12, 3). The first number in parentheses indicates the number of hippocampal slices and the second the number of animals used. Statistical significance of values was assessed by Mann-Whitney test (p > 0.05 in all cases).
We also tested the effects of ryanodine and caffeine on additional fEPSP parameters, and found that 1 μM stimulatory ryanodine did not affect fEPSP rise times (Figure 2A), half-widths (Figure 2B) or decay rates (Figure 2C). Treatment with inhibitory ryanodine (20 μM) did not affect fEPSP rise times (Figure 2D) but caused a modest increase in half-widths (Figure 2E) and decay constant tau values (Figure 2F) displayed by fEPSPs evoked by strong stimulation (200 and 250 μA). As observed following treatment with stimulatory ryanodine, RyR activation by application of 1 mM caffeine had no effect on fEPSP rise times (Figure 2G), half-widths (Figure 2H) or decay rates (Figure 2I).
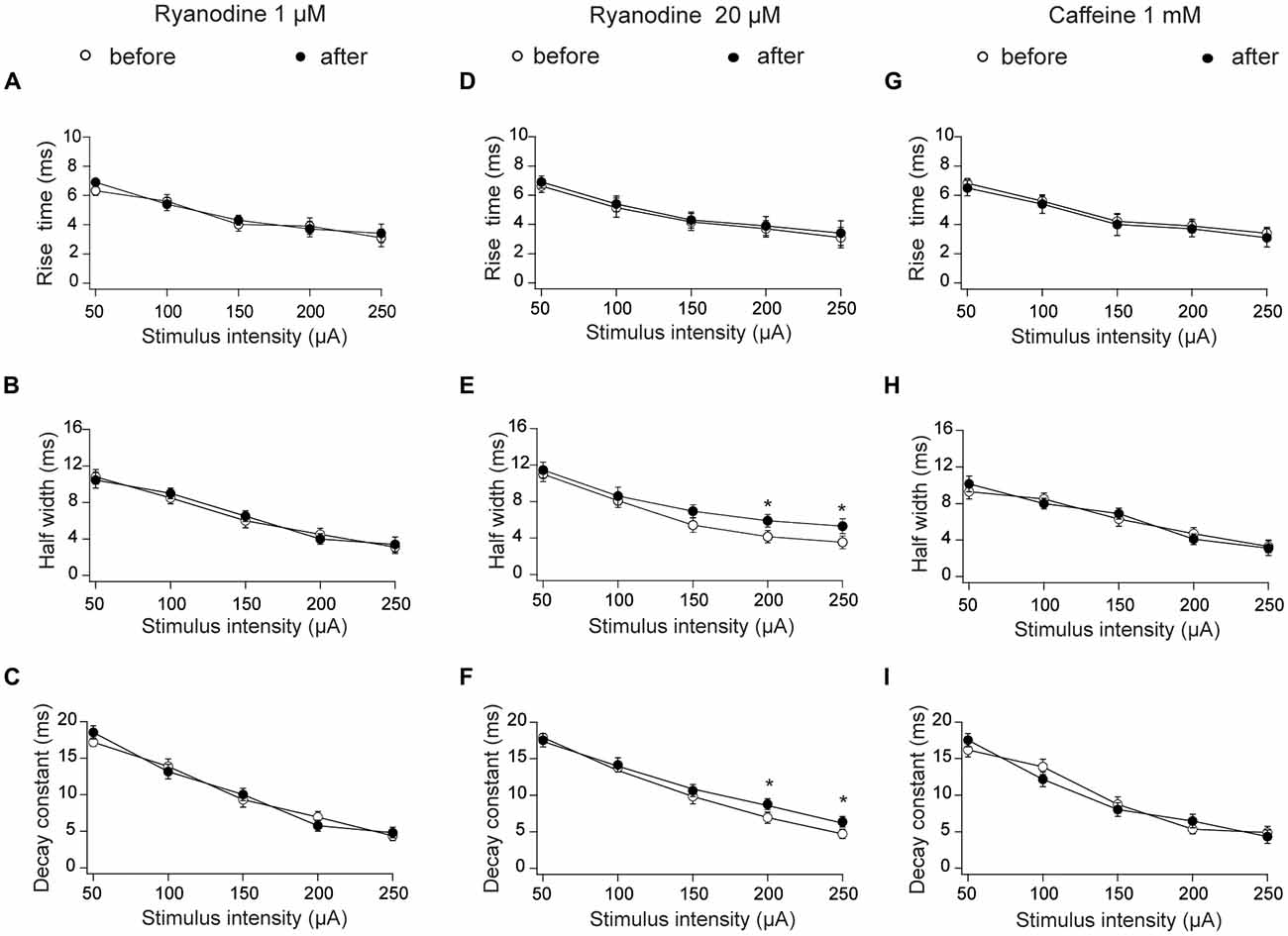
Figure 2. Effects of stimulatory and inhibitory ryanodine concentrations and of caffeine on fEPSP rise times, half-widths and decay constants tau measured in CA3–CA1 hippocampal synapses. Left panels: slices were treated with 1 μM ryanodine. (A) fEPSP rise times vs. stimulus intensity. (B) fEPSP half-widths vs. stimulus intensity; (C) fEPSP decay constants (tau) vs. stimulus intensity. Central panels: slices were treated with 20 μM ryanodine for 60 min. (D) fEPSP rise times vs. stimulus intensity; (E) fEPSP half-widths vs. stimulus intensity; (F) fEPSP decay constants (tau) vs. stimulus intensity. Right panels: slices were treated for 15 min with 1 mM caffeine. (G) fEPSP rise times vs. stimulus intensity; (H) fEPSP half-widths vs. stimulus intensity; (I) fEPSP decay constants (tau) vs. stimulus intensity. Values represent Mean ± SE; (12, 3). The first number in parentheses indicates the number of hippocampal slices and the second the number of animals used. Statistical significance of values was assessed Mann-Whitney test (*p < 0.05).
Based on these combined results, we conclude that RyR activation with ryanodine or caffeine does not modify the electrical signals recorded during basal synaptic transmission, and that RyR inhibition does not affect fEPSP properties in the stimulation range < 200 μA, a range that was used in all subsequent experiments.
Activation or Inhibition of RyR Channels Does Not Affect Paired-Pulse Responses
To analyze further if RyR-mediated Ca2+ release modifies the PP response, we evaluated this response in control conditions and after treatment of slices with stimulatory or inhibitory ryanodine concentrations, or with caffeine (Figure 3A). Treatment with 1 μM ryanodine (Figure 3B) or 1 mM caffeine (Figure 3D) to promote RyR channel activation did not affect fEPSP slope ratios, independent of the delay between pulses. Likewise, perfusion with 20 μM ryanodine did not affect the PP responses (Figure 3C). Hence, we suggest that RyR inhibition does not affect the generation of presynaptic local Ca2+ signals involved in this fast response.
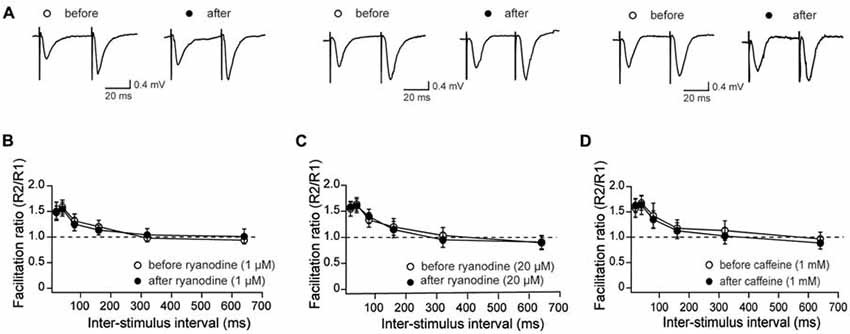
Figure 3. Effects of stimulatory and inhibitory ryanodine concentrations and of caffeine on paired-pulse (PP) responses measured in CA3–CA1 hippocampal synapses. (A) Representative fEPSP traces showing PP responses before and after addition of 1 μM ryanodine (left panels), 20 μM ryanodine (center panels) and 1 mM caffeine (right panels). (B) Effects of 1 μM ryanodine applied for 15 min on PP facilitation. The graph illustrates the facilitation ratio vs. inter-stimulus intervals. (D) Effects of 1 mM caffeine applied for 15 min on PP facilitation. The graph illustrates the facilitation ratio vs. inter-stimulus intervals. (C) Effects of 20 μM ryanodine applied for 60 min on PP facilitation. The graph illustrates the facilitation ratio vs. inter-stimulus intervals. Values represent Mean ± SE; (13, 4) for ryanodine-treated slices; (12, 3) for caffeine-treated slices and (16, 4) for the control. The first number in parentheses indicates the number of hippocampal slices and the second the number of animals used.
Modulation of LTD Induction by Ryanodine and Caffeine
We evaluated next whether activation or inhibition of RyR channels affects LTD induction by LFS at 1 Hz. To this aim, we tested first the effects of incubating hippocampal slices with stimulatory (1 μM) ryanodine for 15 min before application of the LFS protocol, and found that this treatment significantly enhanced LTD induction (Figures 4A,B). Relative to the initial slope values, defined as 100%, 60 min after application of the LFS protocol control slices displayed fEPSP slopes (% values) of 81.4 ± 4.8, whereas slices treated with 1 μM ryanodine exhibited fEPSP slopes (% values) of 59.7 ± 7.2. Consistently, pre-incubation with 1 mM caffeine to activate RyR channels also enhanced LTD induction to the same extent as stimulatory ryanodine concentrations (Figures 4C,D). Caffeine-treated slices displayed fEPSP slope values (in %), measured 60 min after LFS, of 61.8 ± 6, which were significantly lower than the % values of 83.8 ± 5.4 displayed by control slices.
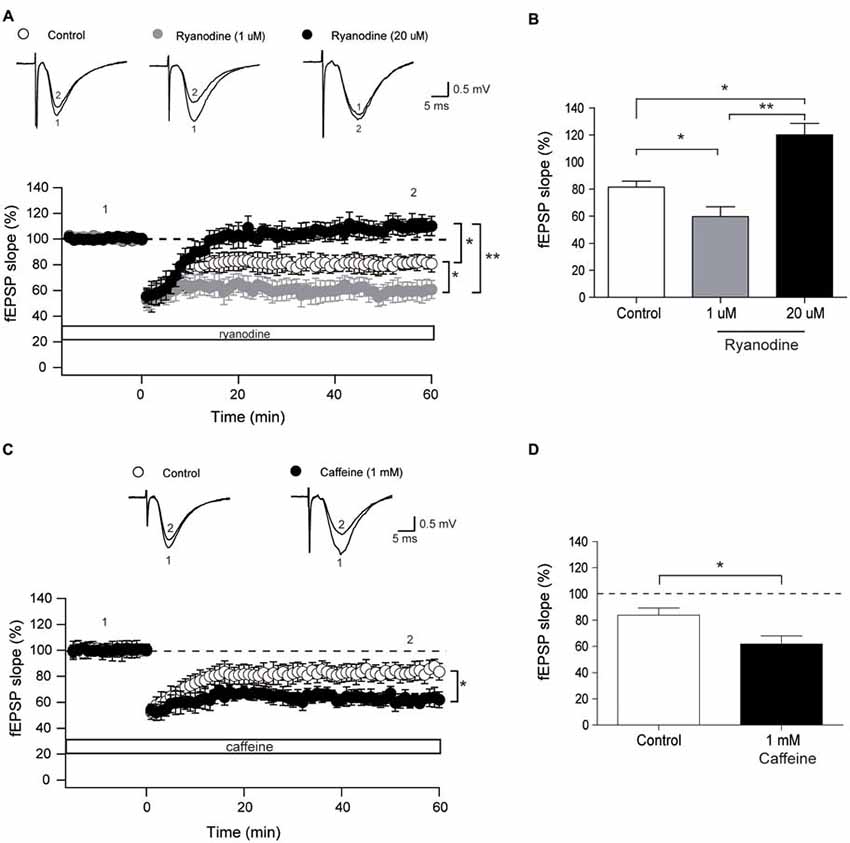
Figure 4. Stimulatory and inhibitory ryanodine concentrations and caffeine modify the long-term depression (LTD) response. (A) Time course of fEPSP slopes recorded (CA3–CA1) before and after application of the low frequency stimulation (LFS) protocol to control hippocampal slices (14, 4) or to slices treated with 1 μM ryanodine (14, 3) or 20 μM ryanodine (13, 4). Representative fEPSP traces recorded 1–5 min before (trace 1) and 60 min after applying the LFS protocol (trace 2) to control slices, or recorded in slices treated with 1 μM or 20 μM ryanodine are shown on top of the graph. Open symbols: control slices; gray symbols: slices treated with 1 μM ryanodine; black symbols: slices treated with 20 μM ryanodine. (B) Average magnitudes of fEPSP slopes recorded during the last 10 min after stimulation. (C) Time course of fEPSP slopes recorded (CA3–CA1) before and after application of the LFS protocol to control hippocampal slices (15, 3) or to slices treated with 1 mM caffeine (12, 4). The first number in parentheses indicates the number of hippocampal slices and the second the number of animals used. Representative fEPSP traces recorded 1–5 min before (trace 1) and 60 min (trace 2) after applying the LFS protocol to control slices and to slices treated with 1 mM caffeine are shown on top of the graph. Open symbols: control slices; black symbols: slices treated with 1 mM caffeine. (D) Average magnitudes of fEPSP slopes recorded during the last 10 min after stimulation of control or caffeine-treated slices. Values represent Mean ± SE. Statistical significance of values was assessed by Mann-Whitney test (*p < 0.05; **p < 0.01).
Incubation of hippocampal slices for 60 min with 20 μM ryanodine to abolish RyR channel activity did not affect basal transmission but completely prevented LTD induction (Figures 4A,B) with % fEPSP values of 120.1 ± 7.4, measured 60 min after the application of the LFS protocol.
LTD Increases the Phosphorylation Levels of CaMKII and Synapsin I
We evaluated next in slices if LTD-inducing protocols modified the Ca2+-dependent phosphorylation of CaMKII and of the presynaptic protein Synapsin I. As illustrated in Figure 5, LTD induction for 60 min caused a significant increase in the phosphorylation levels of CaMKII (α and β) and of Synapsin I relative to the levels displayed by unstimulated slices (naïve). Slices pre-incubated for 1 h with 20 μM ryanodine before applying the LTD induction protocol displayed phosphorylation levels of CaMKII-α, CaMKII-β and Synapsin I that were not significantly different from the levels displayed by unstimulated slices (Figure 5). In addition, naïve slices pre-incubated for 1 h with 20 μM ryanodine displayed comparable CaMKII and Synapsin I phosphorylation levels as naïve control slices (Figure 5). The protein contents of CaMKII-α, CaMKII-β and Synapsin I were not affected by inhibitory ryanodine or exposure to the LTD induction protocol (Supplementary Figure S1).
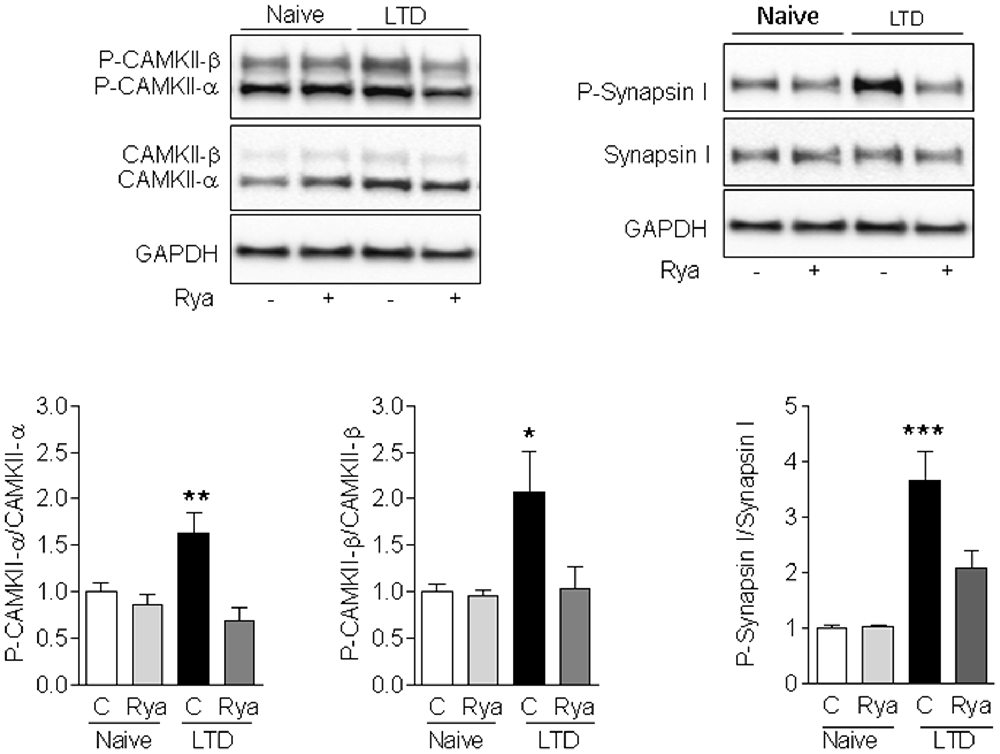
Figure 5. LTD-induced phosphorylation of CaMKII and Synapsin I. Induction of LTD for 60 min in control (C) slices caused a significant increase in the phosphorylation levels of Synapsin I (left panels), CaMKII-α (center panels) and CaMKII-β (right panels) relative to the levels displayed by unstimulated slices (naïve). Slices pre-incubated for 1 h with 20 μM ryanodine (Rya) before applying the LTD induction protocol displayed significantly lower increments in the phosphorylation levels of Synapsin I and CaMKII-α, whereas the phosphorylation levels of CaMKII-β were not significantly different from the levels displayed by unstimulated slices. Values represent Mean ± SE (n = 3). Statistical analysis was performed with one-way ANOVA, followed by Tukey’s post hoc test. *p < 0.05 vs. naïve; **p < 0.01 vs. naïve; ***p < 0.001 vs. naïve.
In agreement with these findings, immunohistochemistry analysis of hippocampal sections containing the CA1 region showed that LTD induction increased CaMKII phosphorylation relative to naïve slices in the soma of CA1 neurons and in neurite projections (Figure 6A). LTD induction also increased CaMKII-dependent Synapsin I phosphorylation in neurite projections; inhibitory ryanodine markedly reduced both increments (Figure 6B).
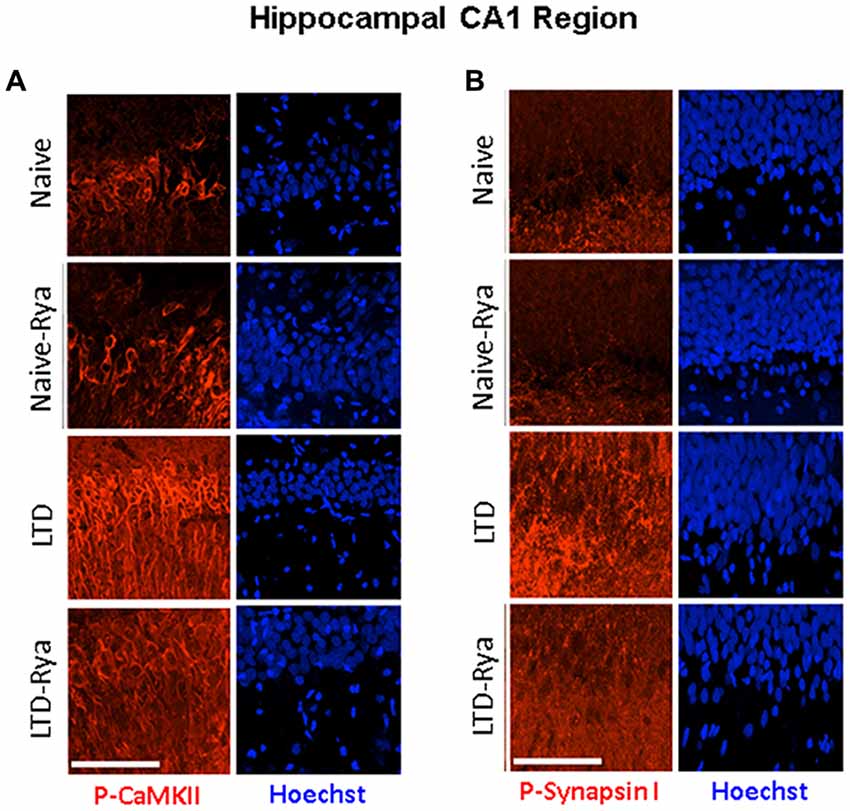
Figure 6. The LTD induction protocol increased (A) CaMKII and (B) Synapsin I phosphorylation levels in hippocampal sections. The figure illustrates representative immunohistochemistry images. (A) CA1-containing hippocampal section stained with an antibody against phospho-CaMKII or (B) with an antibody against phospho-Synapsin-I, which detects CaMKII-dependent phosphorylation of phospho-Ser-603. Three independent experiments yielded similar results. For experimental details, see text. The calibration bar represents 50 μm.
Discussion
Summary of Results
The results presented in this work show that treatment of hippocampal slices with concentrations of ryanodine (1 μM) or caffeine (1 mM) that are known to stimulate RyR channel activity did not change the parameters of the fEPSP waveforms in the synaptic response. Inhibitory ryanodine (20 μM), however, produced a modest increase in the half-width and the tau decay values of fEPSPs, but this response was observed only when applying strong stimulation. In addition, low or high concentrations of ryanodine, as well as 1 mM caffeine, did not modify basal synaptic transmission or PP responses. Treatment with 1 μM ryanodine or 1 mM caffeine enhanced LTD induction, while inhibitory ryanodine prevented the induction of LTD and decreased CaMKII and Synapsin I phosphorylation relative to the levels exhibited by control slices 1 h after LTD induction.
Ryanodine and Caffeine Do Not Affect Fiber Volley Amplitude and Basal fEPSP Properties
The negative deflection of FV records evoked by extracellular stimulation represents the extracellular counterpart of action potentials in the presynaptic fibers (Henze et al., 2000). In our recordings, we found that 1 μM or 20 μM ryanodine, or 1 mM caffeine did not modify FV amplitude, suggesting that these drugs do not affect presynaptic components involved in basal synaptic transmission. These results add novel findings to the characterization of this presynaptic response.
We observed that both ryanodine and caffeine did not affect basal fEPSP amplitudes and slopes, in agreement with the previously reported lack of effect of 0.2 μM ryanodine on these fEPSP properties (Grigoryan et al., 2012). Based on these combined results, we suggest that RyR-mediated Ca2+ signals do not participate in modulating basal α-amino-3-hydroxy-5-methyl-4-isoxazolepropionic acid (AMPA) or NMDAR kinetics. A previous report, however, showed that more prolonged incubation (30 min) with a higher caffeine concentration (5 mM) modifies fEPSP slopes recorded in the ventral hippocampus but does not alter fEPSP slopes recorded in the dorsal hippocampus (Grigoryan et al., 2012), suggesting different responses to RyR activation by caffeine in these two regions. In our experiments, we did not differentiate between ventral and dorsal hippocampus and only tested the effects of incubation for 15 min with 1 mM caffeine.
In addition, albeit 1 μM ryanodine or caffeine did not modify the half-width times or the exponential decay constant tau, we found that inhibitory ryanodine modified these two parameters to a small extent but only under strong stimulation conditions. Possibly, strong stimulation enhances the emergence of RyR-mediated Ca2+ signals that promote repolarization by recruiting postsynaptic Ca2+-gated K+ channels. The hippocampus expresses both large-conductance (BK) and low conductance (SK) Ca2+-activated K+ channels (Chen et al., 2014). The BK channels are expressed in presynaptic terminals of Schaffer collateral fibers of stratum radiatum but do not contribute significantly to transmitter release under basal experimental conditions (Hu et al., 2001). In contrast, SK channels are expressed in hippocampal CA1 pyramidal cells (Stocker and Pedarzani, 2000; Bond et al., 2004; Chen et al., 2014). Moreover, SK2 is the most abundant isoform expressed in dendritic spines of CA1 pyramidal cells (Chen et al., 2014); this isoform is activated by Ca2+ entry through NMDARs and voltage-gated Ca2+ channels (Chen et al., 2014), and possibly by Ca2+ generated by RyR-mediated CICR from the ER in response to strong stimulation. We suggest, accordingly, that RyR channel inhibition decreases the activity of postsynaptic SK channels, causing the small increases of tau values and half-width times produced in response to strong stimulation.
Ryanodine or Caffeine Do Not Affect Paired-Pulse Responses
We show in the present work that low or high concentrations of ryanodine or 1 mM caffeine did not modify the paired-pulse facilitation response, in agreement with a previous report showing that incubation for 30 min with 1 mM caffeine does not modify this response (Grigoryan et al., 2012). We add to these findings the lack of effect of low or high concentrations of ryanodine. We propose that RyR-mediated Ca2+ release does not participate in the fast-presynaptic neurotransmitter release that underlies pair pulse facilitation, which occurs in the tens of ms time range, but is required to stimulate slower Ca2+-dependent pathways underlying LTD induction, as discussed below.
Ryanodine and Caffeine Modify LTD Induction
Previous reports have implicated Ca2+ release from the ER in the induction of LTD in young (Mulkey and Malenka, 1992; Reyes and Stanton, 1996; Nakano et al., 2004) and aged rodents (Kumar and Foster, 2005). In particular, thapsigargin and cyclopiazonic acid, two sarco/ER Ca2+-ATPase inhibitors that deplete intracellular Ca2+ stores (Seidler et al., 1989; Thastrup et al., 1990), inhibit LTD induction by 1 Hz stimulation in the hippocampal CA3–CA1 synapse in young rats (Reyes and Stanton, 1996). A later report, however, described that LTD induction by 1 Hz stimulation was not affected by thapsigargin and cyclopiazonic acid; yet, 0.5 or 2 Hz stimulation, which induced much smaller LTD, required Ca2+ stores (Nakano et al., 2004).
A few studies have implicated IP3R channels in LTD induction in CA1 neurons of guinea pig hippocampal slices (Taufiq et al., 2005), in postsynaptic cerebellar Purkinje neurons (Finch and Augustine, 1998) and in Schaffer collateral-CA1 synapses (Jo et al., 2010). Alternatively, other reports have implicated RyR channels in LTD induction in young (Reyes and Stanton, 1996; Nakano et al., 2004) and aged rodents (Kumar and Foster, 2005). Moreover, the RyR3 channel isoform was implicated in LTD induction, based on the finding that one train of LFS does not induce LTD in RyR3-deficient mice (Futatsugi et al., 1999). Here, we present additional results that confirm the role of RyR channels in LTD induction by 1 Hz, by showing that stimulatory ryanodine and caffeine enhanced LTD induction while inhibitory ryanodine abolished this response.
In addition to RyR channels, several neuronal sources contribute to elicit the postsynaptic Ca2+ signals required for LTD induction in response to LFS, including NMDARs (Dudek and Bear, 1992; Bear and Abraham, 1996; Li et al., 2007), AMPA receptors (Sanderson et al., 2016) and metabotropic receptors (Taufiq et al., 2005; Citri and Malenka, 2008; Hisatsune and Mikoshiba, 2017). As mentioned above, a role for IP3R channels in LTD induction in different synapse types has been reported (Finch and Augustine, 1998; Taufiq et al., 2005; Jo et al., 2010). Yet, Ca2+ release mediated by IP3R1 channels, which undergo activation via G-protein-coupled metabotropic glutamate receptors (Hisatsune and Mikoshiba, 2017), is unlikely to participate in LTD induction. Mice lacking the IP3R1 type-1 (IP3R1) isoform, the more abundant IP3R isoform expressed in the hippocampus (Furuichi et al., 1994; Hertle and Yeckel, 2007; Hisatsune and Mikoshiba, 2017), display robust LTD induction in response to LFS protocols (Fujii et al., 2000; Nagase et al., 2003).
Inhibitory Ryanodine Reduces the Enhanced Phosphorylation of CaMKII and Synapsin I Caused by LTD Induction
We found that 1 h after LTD induction slices exhibited a significant increase in CaMKII (α and β) and Synapsin I phosphorylation; these increments did not occur in slices treated with inhibitory ryanodine prior to exposure to the LTD induction protocol. In addition, LTD induction enhanced RyR-mediated Synapsin I phosphorylation by CaMKII, which we interpret as evidence of presynaptic CaMKII activation following LTD induction. The elimination by inhibitory ryanodine of the enhanced CaMKII-dependent Synapsin I phosphorylation indicates RyR-mediated Ca2+ signals mediate the LTD-induced stimulation of presynaptic CaMKII activity. Based on these novel results, we suggest that LTD induction requires presynaptic functional RyR channels to generate Ca2+ signals that stimulate CaMKII activity in presynaptic terminals.
In addition, we found that inhibitory ryanodine partially prevented the enhanced CaMKII phosphorylation displayed by the soma of CA1 neurons 1 h after LTD induction. Whether this postsynaptic increase is relevant for LTD induction remains the subject of future studies, albeit an earlier report showed that LTD induction requires presynaptic but not postsynaptic CaMKII activation (Stanton and Gage, 1996).
Several studies indicate that Synapsin I phosphorylation, which displayed a significant increase following LTD induction (Figures 5, 6), leads to an increase in the readily releasable vesicle pool and enhances vesicle availability for exocytosis (Chi et al., 2003). Yet, studies in Synapsin knockout mice suggest that CaMKII-mediated Synapsin I phosphorylation has a limited role in controlling neurotransmitter release (Gitler et al., 2008; Wang, 2008; Song and Augustine, 2015). To our knowledge, there is no information in the literature implicating Synapsin I phosphorylation as part of the cellular mechanisms underlying LTD induction, which for NMDA-dependent LTD at CA3–CA1 synapses entail long-term reduction of release from the rapidly recycling presynaptic vesicle pool (Zhang et al., 2006).
Conclusion
Based on the present findings, we propose that the Ca2+ signals generated by activation of presynaptic RyR channels enhance LTD induction while their inhibition precludes this response. Accordingly, we suggest that RyR-mediated Ca2+ release from presynaptic intracellular stores contributes to the activation of downstream Ca2+-dependent pathways and signaling molecules, including CaMKII, which are required for LTD induction.
Author Contributions
AA-C designed and performed all experiments, analyzed results, wrote the first draft of the manuscript and revised all subsequent version of the manuscript. GB analyzed results, and revised all versions of the manuscript. GS performed immunoblots, analyzed the ensuing results and contributed to manuscript writing. CE provided the software for analysis of results. PM performed electrophysiological and immunohistochemistry experiments, analyzed the results generated by them and contributed to manuscript writing. CH supervised and financed the work, analyzed results and wrote the final version of the manuscript.
Funding
This research was supported by FONDECYT (1140545; 1170053) and BNI (P-09-015F).
Conflict of Interest Statement
The authors declare that the research was conducted in the absence of any commercial or financial relationships that could be construed as a potential conflict of interest.
Acknowledgments
We thank the excellent technical help provided by Nicole Henriquez, Luis Montecinos and Carolina Estay.
Abbreviations
ACSF, artificial cerebrospinal fluid; AMPA, α-amino-3-hydroxy-5-methyl-4-isoxazolepropionic acid; BK, large-conductance Ca2+-activated K+ channels; CICR, Ca2+-induced Ca2+ release; ER, endoplasmic reticulum; fEPSP, field excitatory postsynaptic potential; FV, fiber volley; I/O, input/output; IP3, inositol 1,4,5-trisphosphate; IP3R, IP3 receptor; LFS, low frequency stimulation; LTD, long-term depression; LTP, long-term potentiation; NMDA, N-methyl-D-aspartate; PP, Paired Pulse; RyR, ryanodine receptor; SK, low-conductance Ca2+-activated K+ channels; TBS, theta-burst stimulation.
Supplementary Material
The Supplementary Material for this article can be found online at: https://www.frontiersin.org/articles/10.3389/fncel.2018.00403/full#supplementary-material
FIGURE S1 | The LTD induction protocol does not modify hippocampal CaMKII and Synapsin I protein contents. The protein contents of CaMKII-alpha (left panel), CaMKII-beta (center panel) and Synapsin I (right panel), measured 1 h after applying the LTD induction protocol to control (C) or to slices treated with inhibitory ryanodine (Rya), were not significantly different from the respective protein contents of naïve slices. Values represent Mean ± SE (n = 3). Statistical analysis was performed with one-way ANOVA, followed by Tukey’s post hoc test.
References
Adasme, T., Haeger, P., Paula-Lima, A. C., Espinoza, I., Casas-Alarcon, M. M., Carrasco, M. A., et al. (2011). Involvement of ryanodine receptors in neurotrophin-induced hippocampal synaptic plasticity and spatial memory formation. Proc. Natl. Acad. Sci. U S A 108, 3029–3034. doi: 10.1073/pnas.1013580108
Adasme, T., Paula-Lima, A., and Hidalgo, C. (2015). Inhibitory ryanodine prevents ryanodine receptor-mediated Ca2+ release without affecting endoplasmic reticulum Ca2+ content in primary hippocampal neurons. Biochem. Biophys. Res. Commun. 458, 57–62. doi: 10.1016/j.bbrc.2015.01.065
Andersen, P., Sundberg, S. H., Sveen, O., Swann, J. W., and Wigstrom, H. (1980). Possible mechanisms for long-lasting potentiation of synaptic transmission in hippocampal slices from guinea-pigs. J. Physiol. 302, 463–482. doi: 10.1113/jphysiol.1980.sp013256
Arias-Cavieres, A., Adasme, T., Sanchez, G., Munoz, P., and Hidalgo, C. (2017). Aging impairs hippocampal-dependent recognition memory and LTP and prevents the associated RyR up-regulation. Front. Aging Neurosci. 9:111. doi: 10.3389/fnagi.2017.00111
Baker, K. D., Edwards, T. M., and Rickard, N. S. (2013). The role of intracellular calcium stores in synaptic plasticity and memory consolidation. Neurosci. Biobehav. Rev. 37, 1211–1239. doi: 10.1016/j.neubiorev.2013.04.011
Bear, M. F., and Abraham, W. C. (1996). Long-term depression in hippocampus. Annu. Rev. Neurosci. 19, 437–462. doi: 10.1146/annurev.neuro.19.1.437
Berridge, M. J. (1998). Neuronal calcium signaling. Neuron 21, 13–26. doi: 10.1016/s0896-6273(00)80510-3
Berridge, M. J. (2002). The endoplasmic reticulum: a multifunctional signaling organelle. Cell Calcium 32, 235–249. doi: 10.1016/s0143416002001823
Bezprozvanny, I., Bezprozvannaya, S., and Ehrlich, B. E. (1994). Caffeine-induced inhibition of inositol(1,4,5)-trisphosphate-gated calcium channels from cerebellum. Mol. Biol. Cell 5, 97–103. doi: 10.1091/mbc.5.1.97
Bliss, T. V., and Collingridge, G. L. (1993). A synaptic model of memory: long-term potentiation in the hippocampus. Nature 361, 31–39. doi: 10.1038/361031a0
Bodhinathan, K., Kumar, A., and Foster, T. C. (2010). Redox sensitive calcium stores underlie enhanced after hyperpolarization of aged neurons: role for ryanodine receptor mediated calcium signaling. J. Neurophysiol. 104, 2586–2593. doi: 10.1152/jn.00577.2010
Bond, C. T., Herson, P. S., Strassmaier, T., Hammond, R., Stackman, R., Maylie, J., et al. (2004). Small conductance Ca2+-activated K+ channel knock-out mice reveal the identity of calcium-dependent afterhyperpolarization currents. J. Neurosci. 24, 5301–5306. doi: 10.1523/jneurosci.0182-04.2004
Bull, R., Marengo, J. J., Suarez-Isla, B. A., Donoso, P., Sutko, J. L., and Hidalgo, C. (1989). Activation of calcium channels in sarcoplasmic reticulum from frog muscle by nanomolar concentrations of ryanodine. Biophys. J. 56, 749–756. doi: 10.1016/s0006-3495(89)82722-5
Cesca, F., Baldelli, P., Valtorta, F., and Benfenati, F. (2010). The synapsins: key actors of synapse function and plasticity. Prog. Neurobiol. 91, 313–348. doi: 10.1016/j.pneurobio.2010.04.006
Chen, S., Benninger, F., and Yaari, Y. (2014). Role of small conductance Ca2+-activated K+ channels in controlling CA1 pyramidal cell excitability. J. Neurosci. 34, 8219–8230. doi: 10.1523/jneurosci.0936-14.2014
Chi, P., Greengard, P., and Ryan, T. A. (2003). Synaptic vesicle mobilization is regulated by distinct synapsin I phosphorylation pathways at different frequencies. Neuron 38, 69–78. doi: 10.1016/s0896-6273(03)00151-x
Citri, A., and Malenka, R. C. (2008). Synaptic plasticity: multiple forms, functions, and mechanisms. Neuropsychopharmacology 33, 18–41. doi: 10.1038/sj.npp.1301559
Coultrap, S. J., and Bayer, K. U. (2012). CaMKII regulation in information processing and storage. Trends Neurosci. 35, 607–618. doi: 10.1016/j.tins.2012.05.003
Dudek, S. M., and Bear, M. F. (1992). Homosynaptic long-term depression in area CA1 of hippocampus and effects of N-methyl-D-aspartate receptor blockade. Proc. Natl. Acad. Sci. U S A 89, 4363–4367. doi: 10.1073/pnas.89.10.4363
Edwards, T. M., and Rickard, N. S. (2006). Pharmaco-behavioural evidence indicating a complex role for ryanodine receptor calcium release channels in memory processing for a passive avoidance task. Neurobiol. Learn. Mem. 86, 1–8. doi: 10.1016/j.nlm.2005.12.012
Finch, E. A., and Augustine, G. J. (1998). Local calcium signalling by inositol-1,4,5-trisphosphate in purkinje cell dendrites. Nature 369, 753–756. doi: 10.1038/25541
Fujii, S., Matsumoto, M., Igarashi, K., Kato, H., and Mikoshiba, K. (2000). Synaptic plasticity in hippocampal CA1 neurons of mice lacking type 1 inositol-1,4,5-trisphosphate receptors. Learn. Mem. 7, 312–320. doi: 10.1101/lm.34100
Furuichi, T., Furutama, D., Hakamata, Y., Nakai, J., Takeshima, H., and Mikoshiba, K. (1994). Multiple types of ryanodine receptor/Ca2+ release channels are differentially expressed in rabbit brain. J. Neurosci. 14, 4794–4805. doi: 10.1523/jneurosci.14-08-04794.1994
Futatsugi, A., Kato, K., Ogura, H., Li, S. T., Nagata, E., Kuwajima, G., et al. (1999). Facilitation of NMDAR-independent LTP and spatial learning in mutant mice lacking ryanodine receptor type 3. Neuron 24, 701–713. doi: 10.1016/s0896-6273(00)81123-x
Galeotti, N., Quattrone, A., Vivoli, E., Norcini, M., Bartolini, A., and Ghelardini, C. (2008). Different involvement of type 1, 2 and 3 ryanodine receptors in memory processes. Learn. Mem. 15, 315–323. doi: 10.1101/lm.929008
Gant, J. C., Chen, K. C., Kadish, I., Blalock, E. M., Thibault, O., Porter, N. M., et al. (2015). Reversal of aging-related neuronal Ca2+ dysregulation and cognitive impairment by delivery of a transgene encoding FK506-binding protein 12.6/1b to the hippocampus. J. Neurosci. 35, 10878–10887. doi: 10.1523/jneurosci.1248-15.2015
Gant, J. C., Sama, M. M., Landfield, P. W., and Thibault, O. (2006). Early and simultaneous emergence of multiple hippocampal biomarkers of aging is mediated by Ca2+-induced Ca2+ release. J. Neurosci. 26, 3482–3490. doi: 10.1523/jneurosci.4171-05.2006
Gitler, D., Cheng, Q., Greegard, P., and Augustine, D. J. (2008). Synapsin IIa controls the reserve pool of glutamatergic synaptic vesicles. J. Neurosci. 28, 10835–10843. doi: 10.1523/jneurosci.0924-08.2008
Grigoryan, G., Korkotian, E., and Segal, M. (2012). Selective facilitation of LTP in the ventral hippocampus by calcium stores. Hippocampus 22, 1635–1644. doi: 10.1002/hipo.22000
Henze, D. A., Borhegyi, Z., Csicsvari, J., Mamiya, A., Harris, K. D., and Buzsaki, G. (2000). Intracellular features predicted by extracellular recordings in the hippocampus in vivo. J. Neurophysiol. 84, 390–400. doi: 10.1152/jn.2000.84.1.390
Hertle, D. N., and Yeckel, M. F. (2007). Distribution of inositol-1,4,5-trisphosphate receptor isotypes and ryanodine receptor isotypes during maturation of the rat hippocampus. Neuroscience 150, 625–638. doi: 10.1016/j.neuroscience.2007.09.058
Hidalgo, C., and Arias-Cavieres, A. (2016). Calcium, reactive oxygen species and synaptic plasticity. Physiology 31, 201–215. doi: 10.1152/physiol.00038.2015
Hisatsune, C., and Mikoshiba, K. (2017). IP3 receptor mutations and brain diseases in human and rodents. J. Neurochem. 141, 790–807. doi: 10.1111/jnc.13991
Hu, H., Shao, L. R., Chavoshy, S., Gu, N., Trieb, M., Behrens, R., et al. (2001). Presynaptic Ca2+-activated K+ channels in glutamatergic hippocampal terminals and their role in spike repolarization and regulation of transmitter release. J. Neurosci. 21, 9585–9597. doi: 10.1523/jneurosci.21-24-09585.2001
Jenden, D. J., and Fairhurst, A. S. (1969). The pharmacology of ryanodine. Pharmacol. Rev. 21, 1–25.
Jo, J., Son, G., Winters, B., Kim, M., Whitcomb, D., Dickinson, B., et al. (2010). Muscarinic receptors induce LTD of NMDAR EPSCs via a mechanism involving hippocalcin, AP2 and PSD-95. Nat. Neurosci. 13, 1216–1226. doi: 10.1038/nn.2636
Kumar, A., and Foster, T. C. (2005). Intracellular calcium stores contribute to increased susceptibility to LTD induction during aging. Brain Res. 1031, 125–128. doi: 10.1016/j.brainres.2004.10.023
Li, R., Huang, F. S., Abbas, A. K., and Wigstrom, H. (2007). Role of NMDA receptor subtypes in different forms of NMDA-dependent synaptic plasticity. BMC Neurosci. 8:55. doi: 10.1186/1471-2202-8-55
Lu, Y. F., and Hawkins, R. D. (2002). Ryanodine receptors contribute to cGMP-induced late-phase LTP and CREB phosphorylation in the hippocampus. J. Neurophysiol. 88, 1270–1278. doi: 10.1152/jn.2002.88.3.1270
Lynch, G., Cox, C. D., and Gall, C. M. (2014). Pharmacological enhancement of memory or cognition in normal subjects. Front. Syst. Neurosci. 8:90. doi: 10.3389/fnsys.2014.00090
Mackrill, J. J. (2010). Ryanodine receptor calcium channels and their partners as drug targets. Biochem. Pharmacol. 79, 1535–1543. doi: 10.1016/j.bcp.2010.01.014
Malenka, R. C., and Bear, M. F. (2004). LTP and LTD: an embarrassment of riches. Neuron 44, 5–21. doi: 10.1016/j.neuron.2004.09.012
Margrie, T. W., Rostas, J. A., and Sah, P. (1998). Presynaptic long-term depression at a central glutamatergic synapse: a role for CaMKII. Nat. Neurosci. 1, 378–383. doi: 10.1038/1589
McPherson, P. S., Kim, Y. K., Valdivia, H., Knudson, C. M., Takekura, H., Franzini-Armstrong, C., et al. (1991). The brain ryanodine receptor: a caffeine-sensitive calcium release channel. Neuron 7, 17–25. doi: 10.1016/0896-6273(91)90070-g
Meissner, G. (1986). Ryanodine activation and inhibition of the Ca2+ release channel of sarcoplasmic reticulum. J. Biol. Chem. 261, 6300–6306.
Mellentin, C., Jahnsen, H., and Abraham, W. C. (2007). Priming of long-term potentiation mediated by ryanodine receptor activation in rat hippocampal slices. Neuropharmacology 52, 118–125. doi: 10.1016/j.neuropharm.2006.07.009
More, J. Y., Bruna, B. A., Lobos, P. E., Galaz, J. L., Figueroa, P. L., Namias, S., et al. (2018). Calcium release mediated by redox-sensitive RyR2 channels has a central role in hippocampal structural plasticity and spatial memory. Antioxid. Redox Signal. 29, 1125–1146. doi: 10.1089/ars.2017.7277
Mulkey, R. M., and Malenka, R. C. (1992). Mechanisms underlying induction of homosynaptic long-term depression in area CA1 of the hippocampus. Neuron 9, 967–975. doi: 10.1016/0896-6273(92)90248-c
Muñoz, P., Estay, C., Díaz, P., Elgueta, C., Ardiles, Á. O., and Lizana, P. A. (2016). Inhibition of DNA methylation impairs synaptic plasticity during an early time window in rats. Neural Plast. 2016:4783836. doi: 10.1155/2016/4783836
Nabavi, S., Fox, R., Proulx, C. D., Lin, J. Y., Tsien, R. Y., and Malinow, R. (2014). Engineering a memory with LTD and LTP. Nature 511, 348–352. doi: 10.1038/nature13294
Nagase, T., Ito, K. I., Kato, K., Kaneko, K., Kohda, K., Matsumoto, M., et al. (2003). Long-term potentiation and long-term depression in hippocampal CA1 neurons of mice lacking the IP(3) type 1 receptor. Neuroscience 117, 821–830. doi: 10.1016/s0306-4522(02)00803-5
Nakano, M., Yamada, S., Udagawa, R., and Kato, N. (2004). Frequency-dependent requirement for calcium store-operated mechanisms in induction of homosynaptic long-term depression at hippocampus CA1 synapses. Eur. J. Neurosci. 19, 2881–2887. doi: 10.1111/j.0953-816x.2004.03390.x
Nishiyama, M., Hong, K., Mikoshiba, K., Poo, M., and Kato, K. (2000). Calcium stores regulate the polarity and input specificity of synaptic modification. Nature 408, 584–588. doi: 10.1038/35046067
Paula-Lima, A. C., Adasme, T., and Hidalgo, C. (2014). Contribution of Ca2+ release channels to hippocampal synaptic plasticity and spatial memory: potential redox modulation. Antioxid. Redox Signal. 21, 892–914. doi: 10.1089/ars.2013.5796
Rall, T. W. (1982). Evolution of the mechanisms of action of methylxanthines: from calcium mobilizers to antagonists of adenosine receptors. Pharmacologist 24, 277–287.
Raymond, C. R. (2007). LTP forms 1, 2 and 3: different mechanisms for the "long" in long-term potentiation. Trends Neurosci. 30, 167–175. doi: 10.1016/j.tins.2007.01.007
Raymond, C. R., and Redman, S. J. (2002). Different calcium sources are narrowly tuned to the induction of different forms of LTP. J. Neurophysiol. 88, 249–255. doi: 10.1152/jn.2002.88.1.249
Reyes, M., and Stanton, P. K. (1996). Induction of hippocampal long-term depression requires release of Ca2+ from separate presynaptic and postsynaptic intracellular stores. J. Neurosci. 16, 5951–5960. doi: 10.1523/jneurosci.16-19-05951.1996
Riquelme, D., Alvarez, A., Leal, N., Adasme, T., Espinoza, I., Valdés, J. A., et al. (2011). High-frequency field stimulation of primary neurons enhances ryanodine receptor-mediated Ca2+ release and generates hydrogen peroxide, which jointly stimulate NF-κB activity. Antioxid. Redox Signal. 14, 1245–1259. doi: 10.1089/ars.2010.3238
Sanderson, J. L., Gorski, J. A., and Dell’Acqua, M. L. (2016). NMDA receptor-dependent ltd requires transient synaptic incorporation of Ca2+-permeable AMPARs mediated by AKAP150-anchored PKA and calcineurin. Neuron 89, 1000–1015. doi: 10.1016/j.neuron.2016.01.043
Seidler, N. W., Jona, I., Vegh, M., and Martonosi, A. (1989). Cyclopiazonic acid is a specific inhibitor of the Ca2+-ATPase of sarcoplasmic reticulum. J. Biol. Chem. 264, 17816–17823.
Sharp, A. H., McPherson, P. S., Dawson, T. M., Aoki, C., Campbell, K. P., and Snyder, S. H. (1993). Differential immunohistochemical localization of inositol 1,4,5-trisphosphate- and ryanodine-sensitive Ca2+ release channels in rat brain. J. Neurosci. 13, 3051–3063. doi: 10.1523/jneurosci.13-07-03051.1993
Song, S.-H., and Augustine, G. J. (2015). Synapsin isoforms and synaptic vesicle trafficking. Mol. Cells 38, 936–940. doi: 10.14348/molcells.2015.0233
Stanton, P. K., and Gage, A. T. (1996). Distinct synaptic loci of Ca2+/calmodulin-dependent protein kinase II necessary for long-term potentiation and depression. J. Neurophysiol. 76, 2097–2101. doi: 10.1152/jn.1996.76.3.2097
Stocker, M., and Pedarzani, P. (2000). Differential distribution of three Ca2+-activated K+ channel subunits, SK1, SK2 and SK3, in the adult rat central nervous system. Mol. Cell. Neurosci. 15, 476–493. doi: 10.1006/mcne.2000.0842
Sutherland, E. W., and Rall, T. W. (1958). Fractionation and characterization of a cyclic adenine ribonucleotide formed by tissue particles. J. Biol. Chem. 232, 1077–1091.
Taufiq, A. M., Fujii, S., Yamazaki, Y., Sasaki, H., Kaneko, K., Li, J., et al. (2005). Involvement of IP3 receptors in LTP and LTD induction in guinea pig hippocampal CA1 neurons. Learn. Mem. 12, 594–600. doi: 10.1101/lm.17405
Thastrup, O., Cullen, P. J., Drøbak, B. K., Hanley, M. R., and Dawson, A. P. (1990). Thapsigargin, a tumor promoter, discharges intracellular Ca2+ stores by specific inhibition of the endoplasmic reticulum Ca2+-ATPase. Proc. Natl. Acad. Sci. U S A 87, 2466–2470. doi: 10.1073/pnas.87.7.2466
Unni, V. K., Zakharenko, S. S., Zablow, L., DeCostanzo, A. J., and Siegelbaum, S. A. (2004). Calcium release from presynaptic ryanodine-sensitive stores is required for long-term depression at hippocampal CA3-CA3 pyramidal neuron synapses. J. Neurosci. 24, 9612–9622. doi: 10.1523/jneurosci.5583-03.2004
Wang, Z.-W. (2008). Regulation of synaptic transmission by presynaptic CaMKII and BK channels. Mol. Neurobiol. 38, 153–166. doi: 10.1007/s12035-008-8039-7
Wang, Y., Rowan, M. J., and Anwyl, R. (1997). Induction of LTD in the dentate gyrus in vitro is NMDA receptor independent, but dependent on Ca2+ influx via low-voltage-activated Ca2+ channels and release of Ca2+ from intracellular stores. J. Neurophysiol. 77, 812–825. doi: 10.1152/jn.1997.77.2.812
Whitlock, J. R., Heynen, A. J., Shuler, M. G., and Bear, M. F. (2006). Learning induces long-term potentiation in the hippocampus. Science 313, 1093–1097. doi: 10.1126/science.1128134
Zhang, X. L., Zhou, Z. Y., Winterer, J., Múller, W., and Stanton, P. K. (2006). NMDA-dependent, but not group I metabotropic glutamate receptor-dependent, long-term depression at schaffer collateral-CA1 synapses is associated with long-term reduction of release from the rapidly recycling presynaptic vesicle pool. J. Neurosci. 26, 10270–10280. doi: 10.1523/jneurosci.3091-06.2006
Keywords: calcium signals, ryanodine, caffeine, basal synaptic transmission, low-frequency stimulation
Citation: Arias-Cavieres A, Barrientos GC, Sánchez G, Elgueta C, Muñoz P and Hidalgo C (2018) Ryanodine Receptor-Mediated Calcium Release Has a Key Role in Hippocampal LTD Induction. Front. Cell. Neurosci. 12:403. doi: 10.3389/fncel.2018.00403
Received: 20 November 2017; Accepted: 18 October 2018;
Published: 06 November 2018.
Edited by:
Alessandro Tozzi, University of Perugia, ItalyReviewed by:
Patric Kevin Stanton, New York Medical College, United StatesAshok Kumar, University of Florida, United States
Copyright © 2018 Arias-Cavieres, Barrientos, Sánchez, Elgueta, Muñoz and Hidalgo. This is an open-access article distributed under the terms of the Creative Commons Attribution License (CC BY). The use, distribution or reproduction in other forums is permitted, provided the original author(s) and the copyright owner(s) are credited and that the original publication in this journal is cited, in accordance with accepted academic practice. No use, distribution or reproduction is permitted which does not comply with these terms.
*Correspondence: Cecilia Hidalgo, Y2hpZGFsZ29AbWVkLnVjaGlsZS5jbA==
† These authors share the first authorship