- 1Department of Neurology, The First Hospital of Jilin University, Changchun, China
- 2Department of Life Sciences, National Natural Science Foundation of China, Beijing, China
Mounting evidence suggests that neuroinflammation is not just a consequence but a vital contributor to the development and progression of Parkinson’s disease (PD). Microglia in particular, may contribute to the induction and modulation of inflammation in PD. Upon stimulation, microglia convert into activated phenotypes, which exist along a dynamic continuum and bear different immune properties depending on the disease stage and severity. Activated microglia release various factors involved in neuroinflammation, such as cytokines, chemokines, growth factors, reactive oxygen species (ROS), reactive nitrogen species (RNS), and prostaglandins (PGs). Further, activated microglia interact with other cell types (e.g., neurons, astrocytes and mast cells) and are closely associated with α-synuclein (α-syn) pathophysiology and iron homeostasis disturbance. Taken together, microglial activation and microglia-mediated inflammatory responses play essential roles in the pathogenesis of PD and elucidation of the complexity and imbalance of microglial activation may shed light on novel therapeutic approaches for PD.
Generic View of PD Pathogenesis
Parkinson’s disease, a prevalent movement disorder which affects almost 2% of the aged population worldwide, is the second most common neurodegenerative disease (Hirsch et al., 2016). Pathologically, PD is characterized by the selective and progressive degeneration of dopaminergic neurons in the SNpc and dopaminergic terminals in the striatum. The exact molecular mechanisms of neuronal loss are not fully understood, but several pathways and mechanisms are known to be implicated in PD pathophysiology, including neuroinflammation, impairment of autophagy, oxidative stress, severe endoplasmic reticulum stress, mitochondrial dysfunction, dysfunction of axonal transport, motor circuit pathophysiology, α-syn aggregation, and prion-like cell-to-cell transmission of α-syn (Charvin et al., 2018). Although it may not be the initial trigger of PD, neuroinflammation is considered to be a vital promoter to the development of PD, in which microglia perform significant roles (Hirsch and Hunot, 2009; Wang et al., 2015c; Skaper et al., 2018).
McGeer et al. (1988) first proposed that innate immunity was involved in PD in 1988 when a substantial number of HLA-DR-positive reactive microglia were detected in the SN of brains from post-mortem PD patients using immunohistochemical staining. Another neuropathologic study showed that the number of reactive microglia expressing MHC class II molecules not only in the SN and putamen but also in the hippocampus, cingulate cortex, temporal cortex and transentorhinal cortex was increased as the neuronal degeneration proceeded (Imamura et al., 2003). Similar up-regulated expression of MHC, as well as T-cell infiltration, has been reported in the SN and striatum of MPTP mouse model of PD (Kurkowska-Jastrzebska et al., 1999). Moreover, GWAS further indicated that common genetic variation in the HLA region was related to sporadic PD (Hamza et al., 2010; Hill-Burns et al., 2011; Nalls et al., 2011). In vivo studies using PET confirmed that microglia were widespread activated at the early stage of PD and levels remained relatively stable, possibly driving the disease process via inflammatory reaction (Ouchi et al., 2005; Gerhard et al., 2006; Edison et al., 2013; Iannaccone et al., 2013; Femminella et al., 2016; Ghadery et al., 2017).
The brain of PD patients and animal models induced by MPTP or 6-OHDA showed several signs of increased inflammatory reaction and programmed cell death (or apoptosis) of neurons and/or neuroglia (Nagatsu et al., 2000; Hunot and Hirsch, 2003): (a) microgliosis which was observerd in various toxin-based models, such as MPTP, 6-OHDA, and rotenone (Marinova-Mutafchieva et al., 2009; Fricke et al., 2016; Ojha et al., 2016; Manocha et al., 2017), as well as in mutant α-syn transgenic models of PD (Lee et al., 2002; Su et al., 2009); (b) elevated levels of inflammatory cytokines (Mogi et al., 1994a, b, 1996), such as IL-1β, IL-2, IL-4, IL-6, IFN-γ and TNF-α, TGF-α, TGF-β1, TGF-β2, EGF, bFGF; (c) increased levels of cytokine receptors, such as TNF-α receptor R1 (p55); (d) increased levels of caspase activities, such as caspase-1 and caspase-3; (e) enzymes related to inflammation (Knott et al., 2000), such as COX-1, COX-2, and iNOS (Knott et al., 2000; Iravani et al., 2002; Ruano et al., 2006); (f) reduced levels of neurotrophins, such as NGF and BDNF; (g) elevated levels of bcl-2 and soluble Fas, which could protect and promote apoptosis, respectively.
A remarkable percentage of monocytic precursors were found in the peripheral blood of patients with PD (Funk et al., 2013). Moreover, increased effector/memory T cells (Tem), defined as CD45RO+ and FAS+ CD4+ T cells, and decreased CD31+ and α4β7+ CD4+ T cells were associated with progressive Unified PD Rating Scale III (UPDRS-III) scores (Saunders et al., 2012). Collectively, the immune responses, notably the CD4+-cell sub-set imbalance and Tem activation, may mirror the pathobiology of PD in the CNS.
Several meta-analyses revealed the association between the use of NSAIDs and the risk of PD (Samii et al., 2009; Gagne and Power, 2010; Gao et al., 2011; Rees et al., 2011; Poly et al., 2019), whereas the relationship was inconclusive. NSAIDs as a whole seemed not to be related to the risk of PD (Samii et al., 2009; Poly et al., 2019). Interestingly, subgroup analysis indicated that use of nonaspirin NSAIDs may have a protective effect, though not shared by aspirin (Gagne and Power, 2010; Rees et al., 2011) or acetaminophen (Gagne and Power, 2010; Gao et al., 2011). One prospective study suggested that the use of nonaspirin NSAIDs may reduce PD risk in males but not in females (Hernan et al., 2006). One population-based study reported that the effect of aspirin also differed by gender, namely a protective role only in females, especially for longer duration of use (≥24 months) or at higher doses (≥14 pills/week) (Wahner et al., 2007). This gender-specific association between NSAIDs and lower PD risk warrants further investigation. As regards ibuprofen, whether there is a potential therapeutic effect or not is still controversial (Chen et al., 2005; Samii et al., 2009; Gao et al., 2011). Further, in MPTP-induced mouse models, indomethacin exerted anti-inflammatory effects and promoted survival of new neurons in the hippocampus without reversing dopaminergic neuronal loss (Hain et al., 2018). Rofecoxib and ibuprofen were also shown to directly protect MPTP-induced damage (Gupta et al., 2009; Swiatkiewicz et al., 2013). In an in vitro study, 6-OHDA-induced PC12 cells pretreated with celecoxib, indomethacin and ibuprofen for 24 h showed significantly increased cell viability, GSH content, and cytoplasmic level of NF-κB, as well as decreased levels of ROS and several apoptosis biomarkers, including cleaved caspase-3, Bax, P-SAPK/JNK and cleaved PARP (Ramazani et al., 2019). Further studies are still needed to elucidate the underlying inflammation process in PD and possible protective effects of NSAIDs.
Taken together, neuroinflammation appears to play central roles in the development of PD. Glial cells, particularly microglia, are involved in this scenario.
Generic View of Microglial Functions
Microglia are resident macrophage-like immune cells in the CNS (Lawson et al., 1990). “Resting” microglia exhibit a ramified morphology with elongated motile cytoplasmic protrusions and processes; they constantly survey the CNS microenvironment and dynamically interact with other neighboring elements, such as neuronal cell bodies, astrocytes, and blood vessels (Davalos et al., 2005; Nimmerjahn et al., 2005; Szalay et al., 2016; Catalin et al., 2017).
Microglia actively contribute to brain development, injury repair, as well as homeostasis maintenance and surveillance (Michell-Robinson et al., 2015; Colonna and Butovsky, 2017). In the developing CNS, microglia control its patterning by regulating apoptosis of neuronal subpopulations (Wakselman et al., 2008) as well as neuron survival and proliferation (Ueno et al., 2013), and control the wiring of neurons by modulating synapse function and maturation, activity-dependent synaptic pruning, as well as synapse number (Wake et al., 2009; Paolicelli et al., 2011; Schafer et al., 2012; Kierdorf and Prinz, 2017). Acting similarly to peripheral macrophages, microglia in the mature CNS are able to phagocytize excess synapses, dead cells, protein aggregates, pathogens, and other particulate and dissolvable antigens that may endanger the CNS (Neumann et al., 2009; Sierra et al., 2010; Kettenmann et al., 2011; Colonna and Butovsky, 2017). Moreover, microglia secrete cytokines, chemokines, growth factors, ROS and RNS, and PGs (Gonzalez et al., 2014). These functions of microglia can be regulated by interactions with the BBB and other cellular elements, including astrocytes, neurons, infiltrating T cells and mast cells (Gonzalez et al., 2014; Figure 1). Microglia show brain region-dependent diversity and selective regional sensitivities to aging, which could underlie region-specific sensitivities to microglial dysregulation and involvement in age-related neurodegeneration (Grabert et al., 2016).
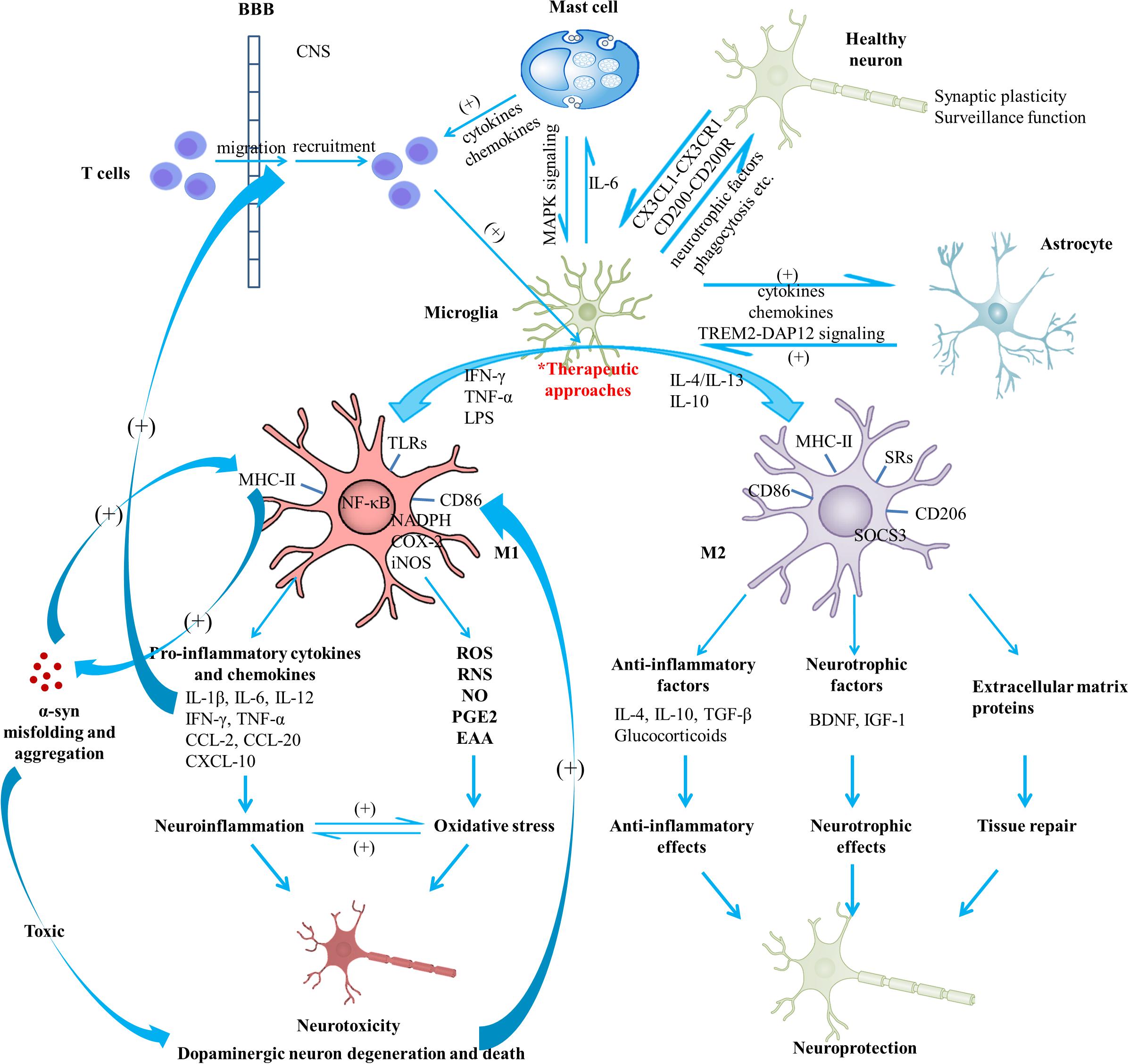
Figure 1. Schematic view of microglial activation and cross-talks between microglia and other immune factors in the pathogenesis of Parkinson’s disease. With regard to PD pathogenesis, microglial activation and microglia-mediated inflammatory responses play essential roles. The M1 polarized state can be induced by TNF-α, IFN-γ, and LPS; present phenotype markers such as MHC-II and CD86; are associated with the production of pro-inflammatory cytokines (IL-1β, IL-6, IL-12, IFN-γ, and TNF-α), chemokines (CCL-2, CCL-20) and CXCL-10; are capable of releasing cytotoxic substances (ROS, RNS, NO, EAA) and PGE2 due to the activation of NADPH oxidase, iNOS, as well as expression of COX-2, contributing to the enhanced oxidative stress. Neuroinflammation and oxidative stress interact with each other to engender a vicious cycle, exerting toxic effects on dopaminergic neurons and leading to the exacerbation of the neurodegenerative process. On the other hand, M2 can be induced by IL-4, IL-10, and IL-13; generally present intracellular components (e.g., SOCS3), cell surface markers (SRs, CD206, CD86, and MHC-II); are capable of producing mediators such as anti-inflammatory cytokines (e.g., IL-4, IL-10, and TGF-β), glucocorticoids, neurotrophic factors (e.g., BDNF and IGF-1), and extracellular matrix proteins; exert anti-inflammatory and neurotrophic effects and play vital roles in tissue repair. Notably, activated microglia may exist along a dynamic continuum rather than be simply polarized into two categories, which is regulated by interactions with other cellular elements, including astrocytes, neurons, infiltrating T cells and mast cells. TREM2-DAP12 signaling complex, the CD200-CD200R and the CX3CL1-CX3CR1 axes are considered to be involved in the astrocyte-microglia and neuron-glia cross-talks. Activated mast cells could induce microglial activation via the MAPK signaling pathway. In turn, microglia-derived IL-6 could induce surface TLR2 and TLR4 expression and consequent cytokine release of mast cells, which contribute to the recruitment of immune cells to the injured areas. In addition, microglial activation has been indicated to promote the prion-like behavior of α-syn misfolding and aggregation. In turn, misfolded α-syn act as chemoattractants to direct microglial migration toward damaged neurons, promote the pro-inflammatory microglia, and exert toxic effects on neurons. Dopaminergic neurons in a death process can trigger microglial activation and inflammatory factor production which can promote recruitment of peripheral leucocytes (mainly T cells). In this way, a complex inflammatory network forms and aggravates degeneration of dopaminergic neurons. A variety of agents or approaches might exert neuroprotective effects due to their regulatory roles on microglial activation. BBB, blood-brain barrier; BDNF, brain-derived neurotrophic factor; COX, cyclooxygenase; CXCL, chemokine (C-X-C motif) ligand; DAP12, DNAX activation protein 12; EAA, excitatory amino acids; IFN, interferon; IGF-1, insulin-like growth factor-1; IL, interleukin; iNOS, inducible nitric oxide synthase; LPS, lipopolysaccharide; MAPK, mitogen-activated protein kinase; NADPH, nicotinamide adenine dinucleotide phosphate; NO, nitric oxide; PD, Parkinson’s disease; PGE2, prostaglandin E2; RNS, reactive nitrogen species; ROS, reactive oxygen species; SOCS3, suppressor of cytokine signaling 3; SR, scavenger receptor; TGF, transforming growth factor; TLR, toll-like receptor; TNF, tumor necrosis factor; TREM2, triggering receptor expressed on myeloid cells 2; α-syn, α-synuclein.
Polarization of Microglia and Its Roles in Neuroinflammation
In various neurodegenerative diseases or in response to any insult, microglia become activated, including morphological changes, alterations of gene expression and surface markers, and proliferation (Wolf et al., 2017). Activated microglia may present epithelioid, rod, amoeboid, multinucleated or “dystrophic” morphological appearance (Boche et al., 2013). Growing evidence shows that microglial activation in the CNS is heterogeneous, similarly to peripheral macrophages, which is often simplified into two polarization states: the M1 phenotype (known as “classical activation”) and the M2 phenotype (known as “alternative activation”) (Orihuela et al., 2016). Activation of microglia can be induced by various factors through numerous pattern recognition receptors like TLRs, SRs and cytokine receptors and chemokine receptors. Microglial cells may present distinctive phenotypes and exhibit specific effects depending on the nature, intensity and duration of the stimulus (Ransohoff and Perry, 2009; Gosselin et al., 2017; Joers et al., 2017; Figure 1).
The M1 polarized microglia with an IL-12high, IL-23high, IL-10low phenotype, present markers such as MHC-II, COX-2, iNOS, and CD86 (Martinez et al., 2006; Chhor et al., 2013), which are associated with the production of pro-inflammatory cytokines (IL-1β, IL-6, IL-12, IFN-γ, and TNF-α), chemokines (CCL-2, CCL-20), CXCL-10, cytotoxic substances (ROS, RNS, NO, EAA), and prostaglandin E2 (PGE2) (Le et al., 2001; Mantovani et al., 2005; Pais et al., 2008). M1 microglia are able to promote neurogenesis and astrocytogenesis from NSPCs (Butovsky et al., 2006; Vay et al., 2018), participate as inducer and effector cells in polarized Th1 responses, and mediate resistance against tumor cells and intracellular parasites (Mantovani et al., 2005). This polarized phenotype can be induced in experimental animals by using IFN-γ or LPS. IFN-γ mediates M1 polarization of microglia mainly through the JAK/STAT intracellular signal transduction pathway; also by “priming”, enhancing TLR-activated signal transduction and then reinforcing microglial responsiveness to inflammatory stimuli (e.g., TLR ligands and TNF-α) (Hu and Ivashkiv, 2009). Studies in vivo and in vitro on macrophage showed that TRPC1-mediated Ca2+ influx is crucial for IFN-γ-induced polarization to the M1 phenotype (Chauhan et al., 2018). LPS, an endotoxin in the outer membrane of Gram-negative bacteria, induces M1 polarization via binding to TLR4, which is coupled to myeloid differentiation protein 2, with participation of LPS-binding protein and co-receptors CD14 (Chow et al., 1999; Takeda and Akira, 2004). The TLR4-mediated signaling typically involves an MyD88-dependent pathway, in which activation of transcription factor NF-κB is followed by production of several inflammatory cytokines (e.g., IL-1β, IL-6, TNF-α), and an MyD88-independent pathway, in which activation of transcription factors NF-κB and IRF-3 is followed by the production of IFN-β mediating IFN-β-induced STAT1-dependent gene expression (Chow et al., 1999; Kawai et al., 2001; Toshchakov et al., 2002; Takeda and Akira, 2004). In this way, expressions of numerous M1-associated cytokines, chemokines and other inflammatory factors are increased. These cytokines activate iNOS and NADPH oxidase, leading to the production of NO, ROS, and RNS (Bove and Perier, 2012; Zeng et al., 2012).
The mechanism of M2 polarization in microglia, as compared to macrophages, is much less clear. The characteristics of M2 microglia may parallel those of macrophages to some extent (Chhor et al., 2013; Miron et al., 2013). The M2 state in macrophages can be divided into three sub-classes named M2a, M2b, and M2c, respectively (Martinez and Gordon, 2014), which might be extrapolated to microglia. The M2 polarized microglia with an IL-12low, IL-23low, IL-10high phenotype, generally present high levels of scavenger, mannose-type and galactose-type receptors (Mantovani et al., 2005), which are associated with the production of anti-inflammatory cytokines (e.g., IL-4, IL-10, and TGF-β), glucocorticoids, neurotrophic factors (e.g., BDNF and IGF-1), and extracellular matrix proteins (e.g., fibronectin) (Subramaniam and Federoff, 2017). M2 microglia are able to promote oligodendrogenesis and neurogenesis (especially oligodendrogenesis) from NSPCs via the PPAR-γ signaling pathway (Butovsky et al., 2006; Vay et al., 2018) and perform vital roles in various situations, such as immune regulation, inflammation inhibition and tissue repair (Michell-Robinson et al., 2015). The activation mechanisms of these three sub-classes as well as their functions are different. The M2a activation state, which performs phagocytosis, functions to suppress inflammation, and contributes to tissue repair, can be induced by IL-4 or IL-13 (Stein et al., 1992; Joers et al., 2017; Subramaniam and Federoff, 2017). IL-4 per se mediates M2a polarization through binding to various receptor pairs, then stimulating JAK1 or JAK3, activating STAT6 and resulting in expression of M2a-associated genes, such as intracellular components (e.g., SOCS3), cell surface markers (SRs and CD206), Ym1 (chitinase-like protein), Fizz1 (found in inflammatory zone) and IL-10 (Stein et al., 1992; Joers et al., 2017; Subramaniam and Federoff, 2017). The M2b polarized state, which is involved in inflammation regulation and selective phagocytosis, can be induced by agonists of TLRs and IL-1 receptors. TLRs become activated by fusing Fc gamma receptors, which bind to immunoglobulin G complexes, resulting in macrophage/microglia polarization to an M2b phenotype and then leading to the secretion of IL-10 and the production of cell surface markers (MHC-II, CD86) (Franco and Fernandez-Suarez, 2015). The M2c polarized state, which plays vital roles in immune regulation, matrix deposition and tissue remodeling, can be induced by IL-10 and glucocorticoid hormones (Subramaniam and Federoff, 2017). IL-10 can activate JAK1 through binding to IL-10 receptor 1 and 2 subunits, then resulting in the translocation of STAT3 to the nucleus, which consequently inhibits the production of M1-associated pro-inflammatory cytokines (Subramaniam and Federoff, 2017).
In general, the M1 phenotype is considered to be the first line of defense with pro-inflammatory roles, while the M2 phenotype to suppress inflammation and to promote tissue repair. Of note is that activated microglia may exist along a dynamic continuum and get different profiles depending on their surrounding microenvironment, rather than be simply polarized into either category (Figure 1).
As mentioned above, the over-simplified “polarization” designation of activated microglia is still controversial. Additional microglia phenotypes which do not align with the traditional binary classification have been discovered (Holtman et al., 2015; Szulzewsky et al., 2015; Morganti et al., 2016; Cho et al., 2019). Therefore, polarization of microglia or the continuum from M1 to M2 state has not been fully supported by experimental data; more importantly, the microglial polarization paradigm may impede rather than facilitate research progress as this schema tends to simplify data interpretation (Ransohoff, 2016). Current research is focused on the characterization of activated microglia for discovering new drug target.
Activation of Microglia and Pathogenesis of PD, What Have We Learned?
The complexity and imbalance of microglial activation has become an area of intense focus, aiming to elucidate a potential mechanism of PD pathogenesis and to identify potential targets for anti-inflammatory treatment (Du et al., 2017).
Microglial Activation and Associated Neuroinflammation and Oxidative Stress
Studies in PD patients, alongside with mounting data in experimental models of this disease, clearly indicate that microglial activation and microglia-mediated inflammation exert dual (beneficial/harmful) effects in PD pathophysiology, as discussed above. A variant in the TREM2 gene of microglia, p.R47H, is a risk factor for PD (Rayaprolu et al., 2013). In the MPTP-induced mouse models of progressive PD, the degeneration of dopaminergic neurons was associated with gradual polarization of microglia to an M1 (pro-inflammatory) phenotype (Pisanu et al., 2014), which could become even more obvious with age (Yao and Zhao, 2018). Furthermore, microglia seemed to present dynamically changing phenotypes depending on disease-stage, accounting for the dynamic changes of pro-inflammatory and anti-inflammatory cytokines (Pisanu et al., 2014; Joers et al., 2017).
Activated microglia proliferate and exhibit a stage- and region-specific secretion of cytokines and mediators (neurotoxic molecules including NO, ROS, RNS and pro-inflammatory cytokines), leading to progressive degeneration of dopaminergic neurons, while microglia in the SNpc are more abundant (approximately 4.5 times) than in other brain regions (Grabert et al., 2016; Lopez Gonzalez et al., 2016). In addition, dopaminergic neurons in the SNpc have decreased anti-oxidant potentials, while the redox reaction of DA could be enhanced by the intensified generation of ROS, resulting in the production of toxic DA metabolites (Whitton, 2007; Tansey and Goldberg, 2010; L’Episcopo et al., 2018). All these features render midbrain dopaminergic neurons more susceptible to oxidative/inflammatory attacks (Whitton, 2007; Tansey and Goldberg, 2010; L’Episcopo et al., 2018). Hence, neuroinflammatory mechanisms could contribute to neuronal degeneration via cascade events.
With regard to the mechanism(s) of dopaminergic neuron degeneration and death, non-cell-autonomous mechanisms might play vital roles as well (Hirsch and Hunot, 2009). Activation of glial cells (especially microglia), triggered by dopaminergic neurons in a death process, could induce inflammatory responses as well as oxidative stress and cytokine-receptor-mediated apoptosis, which in turn promote recruitment of peripheral leucocytes (mainly T cells) and lead to an inflammatory network around neuronal injury (Hirsch and Hunot, 2009). The non-cell-autonomous mechanism is considered to drive dopaminergic cell death and hence neurodegeneration in PD (Hirsch and Hunot, 2009). During microglial activation, activation of NADPH oxidase, iNOS, as well as expression of COX-2 results in the synthesis of high levels of ROS, RNS, NO, and PGE2, contributing to the enhanced oxidative stress, which not only exerts toxic effects on dopaminergic neurons but also further amplifies the pro-inflammatory microenvironment (Taylor et al., 2013). Peroxynitrite, a potent toxin produced when NADPH oxidase and iNOS are present together, promotes the nitration of proteins (e.g., tyrosine) and further production of hydroxyl radicals, which can damage lipids, proteins, DNA and RNA, resulting in dysfunction and eventual death of neurons (Gao and Hong, 2008; Taylor et al., 2013; L’Episcopo et al., 2018). In this way, neuroinflammation and oxidative stress interact to engender a vicious cycle, leading to the exacerbation of neurodegeneration (Figure 1). However, microgliosis and increase of pro-inflammatory molecules could be detected at the early stage and well before dopaminergic neuron death in the α-syn transgenic models (Su et al., 2009), implying that cell death is not necessarily a prerequisite for the activation of microglia and mutated α-syn could also initiate the vicious cycle.
The interaction between α-syn and microglia is considered to be vital in the pathogenesis of PD (Figure 1). α-syn, a presynaptic protein secreted by neurons in small amounts which prevalently exists as monomers in physiology, is over-expressed and aggregates into oligomers or protofibrils in PD (Theillet et al., 2016). Oligomers/protofibrils could propagate between cells as free floating proteins or via extracellular vesicles (Li et al., 2008; Ingelsson, 2016), exert toxic effects on the synapses, resulting in disruption of electrophysiological properties (Calo et al., 2016; Ingelsson, 2016; Bridi and Hirth, 2018; La Vitola et al., 2018; Prots et al., 2018; Longhena et al., 2019), and act as chemoattractants to direct microglial migration toward damaged neurons (Wang et al., 2015a). In addition, α-syn over-expression promotes the polarization of microglia to the pro-inflammatory phenotype with elevated production of cytokines such as IL-1β, IL-6, and TNF-α as well as COX-2, iNOS and free radicals (Zhang et al., 2005, 2017; Austin et al., 2006; Su et al., 2008, 2009; Theodore et al., 2008; Sacino et al., 2014; Ferreira and Romero-Ramos, 2018). Oligomeric α-syn induces the M1 phenotype by directly engaging the TLR1/2 heterodimer, resulting in the nuclear translocation of NF-κB and the elevated production of these cytokines by an MyD88-dependent pathway (Kim et al., 2013; Daniele et al., 2015; Ho, 2019; Kouli et al., 2019): After α-syn activates TLR1/2 heterodimer, the C-terminal intracellular TIR domains of TLR interact with MyD88, activating the IRAK complex (Lin et al., 2010), which in return activates the TRAF6 via its K63-linked auto-ubiquitination. Then, the subsequent activation of the TAK1 complex and its downstream effector IKK complex lead to the degradation of IκBα and the production of pro-inflammatory cytokines via the activation of MAPK and translocation of NF-κB, p38 and JNK (Symons et al., 2006; Kawai and Akira, 2007, 2010). TLR4 could also mediate α-syn-dependent activation of microglia, including the production of ROS and pro-inflammatory cytokines as well as the phagocytic activity of microglia (Fellner et al., 2013; Janda et al., 2018; Hughes et al., 2019). Scavenger receptor CD36 and P2X7 receptor are also involved in α-syn-mediated microglial activation (Su et al., 2008; Jiang et al., 2015). Moreover, α-syn-stimulated microglia secrete MMPs, activating PAR-1 and amplifying microglial inflammatory signals (Lee et al., 2010). In this way, α-syn is a vital regulator of microglial activation and phagocytosis. Activated microglia which exert enhanced phagocytosis of α-syn in turn, could on one hand directly play a beneficial role in controlling α-syn spread (Stefanova et al., 2011), and on the other hand, notably, promote the prion-like behavior of α-syn misfolding, aggregation and spread (Hansen et al., 2011; George et al., 2019; Olanow et al., 2019). This may be associated with the increased accumulation of intracellular α-syn in microglia and accelerated secretion of α-syn into extracellular space via exosomal pathways (Porro et al., 2019; Xia et al., 2019), as well as the toxic effects of cytokines generated from activated microglia on the vulnerable neuronal cells (Olanow et al., 2019). Taken together, microglia-derived oxidative stress is considered to bridge α-syn pathogenic alteration and neuroinflammation, contributing to chronic progression of neurodegeneration in PD (Lawand et al., 2015). Those mentioned receptors on microglia could be therapeutic targets for microglial activation and α-syn pathogenesis.
Cross-Talks Between Microglia and Other Cell Types
The microglial polarization status and associated neuroinflammation are tightly connected with astrocyte-microglia and neuron-glia interactions via ligand-receptor pairings (Figure 1). TREM2, exclusively expressed by microglia, could enhance the phagocytosis function of microglia (Takahashi et al., 2005, 2007; Kawabori et al., 2015), and attenuate inflammatory responses by negatively regulating the TLR4-mediated activation of NF-κB signaling and shifting M1 polarization to the M2 phenotype (Jiang et al., 2016; Ren et al., 2018; Zhang et al., 2018). In addition, TREM2-DAP12 signaling complex exerts vital roles in promoting phagocytosis, microglial activation, as well as microglial survival (Belloli et al., 2017; Konishi and Kiyama, 2018; Mecca et al., 2018). The fractalkine receptor CX3CR1, another receptor specifically expressed in microglia, plays a fundamental role in the communication between microglia and neurons by binding to neuronal fractalkine, namely CX3CL1 (Harrison et al., 1998). Accumulating evidence suggests that the CX3CL1-CX3CR1 axis is involved in homeostatic suppression of microglial activation and regulation of chemoattraction and synaptic plasticity, inhibiting microglia-mediated inflammatory responses and neurotoxicity (Cardona et al., 2006; Pabon et al., 2011; Sheridan and Murphy, 2013; Mecca et al., 2018). CD200, a transmembrane glycoprotein present on neurons, can regulate microglial activation status by binding to microglial CD200R. Deficits in the CD200-CD200R axis could exacerbate activation of microglia and dopaminergic neurodegeneration (Wang et al., 2011; Zhang et al., 2011).
Notably, the mast cell-glia cross-talk is also involved in regulation of neuroinflammation (Skaper et al., 2017, 2018; Figure 1). In inflammatory tissues, the expression of ligand-receptor pairings is increased, enhancing chemotactic actions of glial cells and mast cells and facilitating intercellular communication. Activated mast cells could induce microglial activation and subsequent production of TNF-α and IL-6 via the MAPK signaling pathway (Zhang et al., 2016). In turn, microglia-derived IL-6 could induce expression of surface TLR2 and TLR4 and consequent release of cytokines by mast cells, which recruit immune cells (including microglia) to the injured areas (Pietrzak et al., 2011; Aguirre et al., 2013). Conceivably, these two cell types form a feedback loop in concert to promote neuroinflammation.
Activation of Microglia and Imbalance of Iron Metabolism
Importantly, disturbance of iron homeostasis is a cardinal feature and contributes to the pathogenesis of multiple neurodegenerative diseases including PD, and microglia are capable of modulating the neuronal iron metabolism (Song et al., 2018). Iron is essential for oxygen transport and the related metabolic activities, especially for brain, the highest oxygen-consuming organ. In addition, iron is necessary for various activities exclusively in the brain, such as the maintenance of myelin, the synthesis and metabolism of neurotransmitters including serotonin, norepinephrine and DA (Ward et al., 2014; Crielaard et al., 2017). Iron accumulation is a physiological feature of aging brain, the degree of which seems to be even greater in PD (Ward et al., 2014). The first observation of abnormal deposits of iron in post-mortem brain especially in the basal ganglia of one PD patient was reported by Lhermitte et al. (1924) in 1924, and was later verified by pathological studies (Sofic et al., 1988; Riederer et al., 1989), magnetic resonance imaging studies (Zhang J. et al., 2010; Wang C. et al., 2013; Lehericy et al., 2014) and transcranial ultrasound (Becker and Berg, 2001; Zecca et al., 2005). However, there has long been a debate as to whether iron is a cause or a consequence of this disorder. Abnormal iron accumulation can induce oxidative stress via ROS, especially hydroxyl radical production, leading to the oxidation and modification of proteins, carbohydrates, lipids, and DNA (Ward et al., 2014). Loss of transferrin receptor 1 (involved in iron uptake) rather than ferroportin could cause age-progressive degeneration of dopaminergic neurons and motor deficits in mice (Matak et al., 2016). Iron might modulate the expression of α-syn and facilitate its aggregation and toxicity via oxidative stress-induced nitration and phosphorylation, contributing to degeneration of dopaminergic neurons (Song et al., 2018). Furthermore, neurodegeneration in the injured regions could be exacerbated by the strong redox coupling of iron and DA (Hare and Double, 2016). Regarding microglial contributions to the disturbance of iron metabolism in PD, the expressed transporters/molecules and the released factors such as pro-inflammatory factors, neurotropic factors and lactoferrin, exert fundamental roles (Song et al., 2018). Microglia are more efficient to accumulate and safely store abundant iron than astrocytes and neurons (Bishop et al., 2011), as evidenced by the finding that ferritin was abundant in microglia while rarely detected in astrocytes and neurons (Healy et al., 2016). Even in the case of acute oxidative stress-induced ferritin reduction, the increased iron storage capacity of microglia might be achieved by elevated iron saturation of the existing ferritin molecules (Mehlhase et al., 2005), coupled with the aggregation of activated microglia around degenerative neurons. Further, pro-inflammatory factors released by activated microglia (e.g., IL-1β, IL-6, and TNF-α) contribute to the iron deposition by up-regulating the expression of IRP1 and iron transporters (e.g., DMT1) through production of ROS and RNS (Urrutia et al., 2013; Wang J. et al., 2013). These findings provide compelling evidence that microglia could aggravate iron-mediated neurodegeneration in PD. Understanding iron metabolism and roles of microglia in iron dyshomeostasis might provide new therapeutic strategies for PD via restoring brain iron homeostasis.
Microbiome-Gut-Brain Axis
In the last decade, the microbiome-gut-brain axis in the pathogenesis of neurodegenerative disorders has attracted increasingly more attention. The gut microbiota could affect BBB permeability (Braniste et al., 2014), and microbiome-derived SCFAs were involved in regulation of microglia homeostasis (Erny et al., 2015). Further, a multi-faceted TLR signaling network is essential for the immune-mediated processes both in the gut and in the CNS, exerting inflammatory or regulatory effects in the pathogenesis of PD (Houser and Tansey, 2017; Caputi and Giron, 2018). Dietary tryptophan metabolites produced by symbiotic microbiota could influence microglial activities, TGF-α production, and neuroinflammatory pathology in EAE, a mouse model of MS (Rothhammer et al., 2018). Future investigations should be focused on whether probiotics or antibiotics or dietary protocols can be utilized to modulate microglial function and to alleviate progress of neurodegenerative diseases including PD.
In brief, as far as PD pathogenesis is concerned, microglial activation and microglia-mediated inflammatory responses play essential roles. Further, α-syn-microglia, neuron-glia, astrocyte-microglia, and mast cell-glia cross-talks (Figure 1), as well as the microbiome-gut-brain axis, are considered to be involved in the regulation of these processes.
Potentials of Microglia as a Therapeutic Target for PD
Drugs are currently available to relieve PD symptoms by limiting the extent of inflammation (Glass et al., 2010), and thus to enhance the life quality of patients. Nevertheless, these drugs are incapable of repairing or regenerating the damaged neurons (Song and Suk, 2017). Given that the balance of M1/M2 polarization states of microglia has been concerned in connection with the pathogenesis of neurodegenerative disorders, including PD, some small molecular compounds have been studied to determine their potentials to modulate the balance and to elucidate the underlying mechanisms.
Novel Agents or Approaches Targeting the M1 Polarization State
Drugs targeting pro-inflammatory and pro-killing M1 polarization state could potentially help reduce neuronal damage caused by inflammation. These drugs listed in Table 1 can be divided into several categories:
Firstly, regulation or modification of receptors on microglia, such as TLRs and CB2 receptors, provides a novel pharmacological target for neurodegenerative disorders including PD (Maresz et al., 2005; Cassano et al., 2017; Fiebich et al., 2018; Navarro et al., 2018). In patients with PD, stage and region-dependent TLR2 expression is consistent with region-specific polarization states of microglia, indicating that TLR2 plays a critical role in the microglia-associated responses in this disorder (Doorn et al., 2014). Candesartan cilexetil, an FDA-approved drug for treating cerebrovascular diseases, hypertension, and chronic heart failure, also able to inhibit the expression of TLR2 and TLR4 (Dasu et al., 2009), might reverse the pro-inflammatory phenotype of microglia activated by oligomeric α-syn (Daniele et al., 2015). Moreover, one recent study showed that the antibiotic rifampin and its autoxidation product rifampicin quinone prevented α-syn-induced TLR2- and P2X7-dependent microglial inflammatory responses in vitro (Acuna et al., 2019), which needs further investigation to confirm their potentials as anti-parkinsionian drugs. Interestingly, endurance exercise could exert neuroprotective effects in MPTP-induced PD mice by regulating the expression of TLR2 and its downstream signaling such as MyD88, TRAF6, and TAK1 (Jang et al., 2017; Koo et al., 2017). As previously discussed, TLR4, another receptor involved in microglial activation, is a potential therapeutic target. Hughes et al. found that pretreatment with TAK-242 or RSLA, small molecule antagonists of TLR4, significantly attenuated α-syn-mediated oxidative stress, TNF-α production by microglia and neuronal death in vitro (Hughes et al., 2019), but has not been tested in vivo. The endocannabinoid system is considered to be closely associated with the neuropathological processes of PD (Navarrete et al., 2018). A naturally occurring agonist of CB2 receptors, β-caryophyllene, could negatively regulate microglial activation and suppress expressions of pro-inflammatory cytokines, whereby eliciting significant neuroprotective effects on nigral dopaminergic neurons (Javed et al., 2016; Ojha et al., 2016). JWH133, another selective CB2 receptor agonist, could protect the MPTP induced PD mice by alleviating BBB disruption and by suppressing the peripheral immune cell infiltration as well as by reducing the M1 microglia-production of iNOS and pro-inflammatory cytokines (Chung et al., 2016).
Secondly, the JAK/STAT signaling pathway and the NF-κB signaling pathway are vital for the polarization of microglia to the M1 state. Targeting these signaling pathways may serve as a potential to regulate M1 activation. For example, α-asarone, a chemical component found in various plants (e.g., Annonaceae and Araceae species), was shown to significantly suppress M1 polarization and to attenuate the production of pro-inflammatory cytokines by inhibiting the NF-κB signaling, and to eventually mitigate PD-like behavioral anomalies in the MPTP-induced mouse model (Kim et al., 2015). Tanshinone I, a bioactive flavonoid, could selectively inhibit the activation of NF-κB signaling and the production of M1-pro-inflammatory molecules including IL-1β, IL-6, TNF-α and NO, partially reserve the expression of M2-anti-inflammatory markers such as IL-10, and prevent nigrostriatal dopaminergic neurodegeneration (Wang et al., 2015b).
Thirdly, some compounds targeting NADPH oxidase, such as apocynin, resveratrol and diphenyleneiodonium, could inhibit microglial activation, suppress the generation of various toxic factors (e.g., ROS, RNS, and pro-inflammatory cytokines), and promote microglial phenotype toward anti-inflammatory state, thereby offering promising candidates for clinical trials in patients with neurodegenerative diseases including PD and AD (Zhang F. et al., 2010; Choi et al., 2012; Wang et al., 2014, 2015d; Sharma and Nehru, 2016).
Finally, targeting pro-inflammatory cytokines (e.g., IL-1β and TNF-α) may provide new possibilities to inhibit M1 polarization. In mouse models induced by MPTP or 6-OHDA, selective suppression of solTNF via intranigral lentiviral delivery of DN-TNF (McCoy et al., 2008; Harms et al., 2011) or nigral infusion of DN-TNF compound XENP345 (McCoy et al., 2006) or peripheral application of DN-TNF inhibitor XPro1595 (Barnum et al., 2014) could alleviate glial activation, dopaminergic neuron loss and final behavioral deficits, suggesting that blocking the solTNF signaling by DN-TNF gene transfer or novel agents is therapeutically feasible for PD.
Interestingly, ghrelin, an endogenous ligand for growth hormone secretagogue receptor 1a, was demonstrated to prevent the activation of microglia, the production of pro-inflammatory factors (e.g., TNF-α and IL-1β) and the activation of iNOS, and to attenuate dopaminergic neuron loss in the MPTP-induced mouse models of PD (Jiang et al., 2008; Moon et al., 2009). The inhibitory effect of ghrelin seems to be exerted by suppressing the expression of MMP-3 in stressed dopaminergic neurons (Moon et al., 2009).
In addition, several drugs approved for other distinct diseases [e.g., lenalidomide for multiple myeloma or myelodysplastic syndrome (Valera et al., 2015), zonisamide for epilepsy (Hossain et al., 2018), minocycline for infectious diseases (Wu et al., 2002; Kobayashi et al., 2013), dimethyl fumaratefor MS (Campolo et al., 2017)] and some bioactive compounds [e.g., ginsenoside Rg1 (Zhou et al., 2015; Heng et al., 2016), piperine (Yang et al., 2015), curcumin (Ghasemi et al., 2019), rosmarinic acid (Lv et al., 2019), astilbin (Zhu et al., 2019)] are capable of inhibiting activation of microglia and attenuating production of pro-inflammatory cytokines in mouse models of PD. These drugs might offer novel alternative therapeutic approaches for PD. However, the underlying mechanisms or involved signaling pathways are not fully understood and require further investigations.
Several promising targets have been proposed to modulate microglial activation. For example, microglia-mediated immune responses could be enhanced by CD73-derived adenosine A2A signaling. CD73 inactivation substantially limited adenosine production, inhibited A2A receptor (A2AR)-mediated pro-inflammatory state of microglia, enhanced the activity of dopaminergic neurons, and mitigated the motor symptoms in animal models of PD (Meng et al., 2019). Histamine-4 receptor is another regulator of microglial activation. Lateral ventricle infusion of H4R antagonist JNJ7777120 could suppress the pro-inflammatory activation of microglia, prevent the degeneration of dopaminergic neurons, reduce the Lewy body-like neuropathology, and improve parkinsonian behaviors (Zhou et al., 2019). Another potential target for PD, NLRP3 inflammasome in microglia can be activated by fibrillar α-syn (Gordon et al., 2018). Oral administration of a small-molecule inhibitor of NLRP3 inflammasome, MCC950, effectively prevented dopaminergic degeneration and α-syn pathology, and alleviated motor behaviors in PD models (Gordon et al., 2018).
Novel Agents or Approaches Capable of Enhancing the M2 Phenotype or Induce the Phenotypic Switch From M1 to M2
Some molecules (e.g., IL-10, cyclic AMP, and vitamin D), which have the capability of enhancing the anti-inflammatory phenotype or of inducing the phenotypic switch from pro-inflammatory to anti-inflammatory, may have therapeutic feasibility for PD (Table 2).
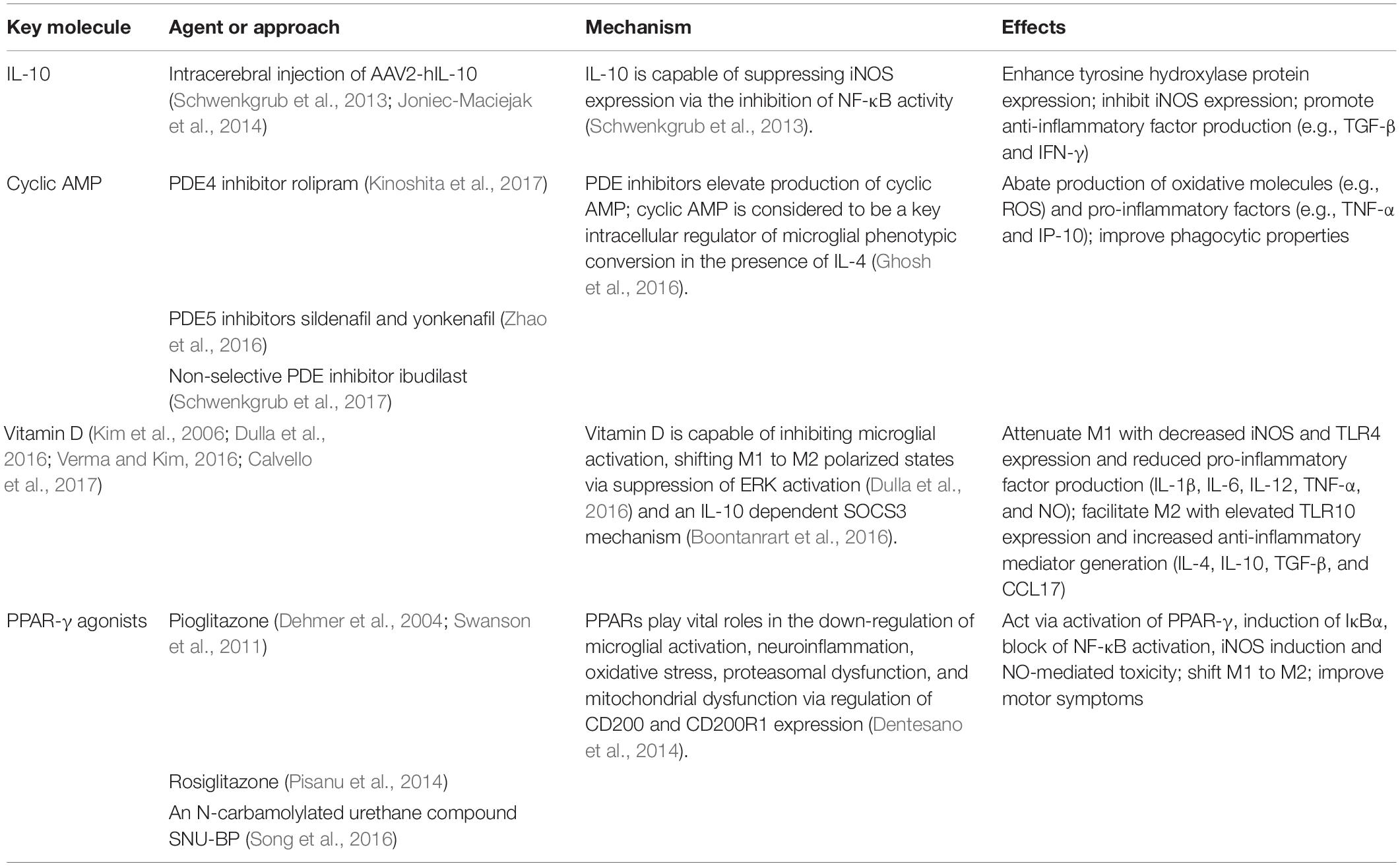
Table 2. Novel agents or approaches capable of enhancing M2 phenotype or induce the phenotypic switch from M1 to M2.
IL-10 is a potent anti-inflammatory cytokine capable of suppressing iNOS expression via the inhibition of the NF-κB activity (Schwenkgrub et al., 2013). Several studies have demonstrated that intracerebral injection of AAV2-hIL-10 in MPTP-induced mouse models of PD could enhance the expression of the striatal tyrosine hydroxylase protein associated with down-regulated expressions of pro-inflammatory iNOS, and promote the production of anti-inflammatory factors like TGF-β, suggesting the neuroprotective properties of AAV2-hIL-10 (Schwenkgrub et al., 2013; Joniec-Maciejak et al., 2014).
Cyclic AMP is considered to be a key intracellular regulator of microglial phenotypic conversion (Ghosh et al., 2016). The M1 to M2a conversion could be promoted by cyclic AMP combined with IL-4, but neither alone, in in vitro microglia cell culture. The M2a-converted microglia showed abated production of oxidative molecules (e.g., ROS) and pro-inflammatory factors (e.g., TNF-α and IP-10), as well as improved phagocytic properties. The cyclic AMP-protein kinase A signaling pathway was involved in the conversion process (Ghosh et al., 2016). Based on this finding, application of synthetic analogs of cyclic AMP, activators of adenylyl cyclase, or inhibitors of PDE might suppress the pro-inflammatory phenotype of microglial cells via elevation of cyclic AMP. Notably, PDE inhibitors, such as rolipram for PDE4, sildenafil and yonkenafil for PDE5 (Zhao et al., 2016), and non-selective PDE inhibitors (e.g., ibudilast), have been proposed as potential therapeutic drugs for neurodegenerative disorders, including MS (Pifarre et al., 2011), AD (Myeku et al., 2016; Kumar and Singh, 2017; Rabal et al., 2018; Xu et al., 2018), and PD (Kinoshita et al., 2017; Schwenkgrub et al., 2017).
Vitamin D is capable of protecting dopaminergic neurons against neuroinflammation and oxidative stress via inhibition of microglial activation, shifting M1 to M2 polarized states, which has been demonstrated in mouse models of PD induced by 6-OHDA/MPTP (Kim et al., 2006; Calvello et al., 2017; Lima et al., 2018) and in microglial HMO6/BV-2 cells (Boontanrart et al., 2016; Dulla et al., 2016; Verma and Kim, 2016). Vitamin D treatment could attenuate M1 polarization with decreased expression of iNOS and TLR4 and reduced production of pro-inflammatory factors (IL-1β, IL-6, IL-12, TNF-α, and NO), and facilitate M2 polarization with elevated expression of TLR10 and increased generation of anti-inflammatory mediators (IL-4, IL-10, TGF-β, and chemokine CCL17) and typical hallmarks of M2 microglia (CD163, CD204, and CD206) (Kim et al., 2006; Dulla et al., 2016; Verma and Kim, 2016; Calvello et al., 2017). Regulatory effects of vitamin D on microglial activation might be associated with suppression of ERK activation (Dulla et al., 2016) and an IL-10 dependent SOCS3 mechanism (Boontanrart et al., 2016).
Peroxisome proliferator-activated receptors, nuclear receptors involved in the pathogenesis of neurodegenerative disorders, have garnered much attention as a promising therapeutic target. PPARs down-regulate microglial activation, neuroinflammation and oxidative stress (Agarwal et al., 2017). PPAR-γ agonists, such as pioglitazone and rosiglitazone, could modulate microglial polarization, thus playing neuroprotective roles on dopaminergic neurons. Oral administration of pioglitazone improved motor symptoms of parkinsonian rhesus monkeys induced by MPTP via anti-inflammatory and neuroprotective effects (Swanson et al., 2011). Pioglitazone might sequentially act via activation of PPAR-γ, induction of IκBα, blockade of NF-κB activation, iNOS induction and NO-mediated toxicity in an MPTP-induced mouse model of PD (Dehmer et al., 2004). In addition, an in vitro study indicated that the anti-inflammatory and neuroprotective effects of PPAR-γ agonists might be closely associated with the interaction between CD200 and CD200R1 (Dentesano et al., 2014). Similarly, rosiglitazone could boost the M2 (anti-inflammatory) phenotype, and regulate cytokine production, whereby alleviating the degeneration of dopaminergic neurons in both SNpc and striatum (Pisanu et al., 2014). However, clinical studies as to whether glitazone protected against PD yielded controversial results (Brauer et al., 2015; Connolly et al., 2015; Ninds Exploratory Trials in Parkinson Disease (Net-Pd) Fs-Zone Investigators., 2015; Brakedal et al., 2017). Notably, a phase 2, multi-center, double-blind, placebo-controlled, randomized clinical trial reported that pioglitazone seemed incapable of modifying progression in early PD (Ninds Exploratory Trials in Parkinson Disease (Net-Pd) Fs-Zone Investigators., 2015). Some novel PPAR-γ agonists, such as an N-carbamylated urethane compound SNU-BP (Song et al., 2016), may inhibit M1-pro-inflammatory cytokine and NO production, and enhance M2 marker expression. Further studies are still needed to confirm the therapeutic potentials of PPAR agonists in neurodegeneration and to explore the underlying mechanisms.
In summary, a variety of drugs may play neuroprotective roles in PD by regulating microglial activation and microglia-induced inflammation; nevertheless, the therapeutic mechanisms are not fully understood (Tables 1, 2). In view of roles of iron metabolism in PD pathogenesis, the protective effects of iron chelation has been explored in the MPTP mouse model by both genetic (transgenic expression of the iron binding protein ferritin) and pharmacological (oral administration of the bioavailable metal chelator clioquinol) means (Kaur et al., 2003). Deferiprone, another oral iron chelator used to treat systemic iron overload diseases, was demonstrated to increase hippocampal BDNF levels, rescue memory deficits and ameliorate iron-induced harmful effects (Alcalde et al., 2018). Further investigations are warranted to explore the underlying mechanisms of beneficial effects of these drugs and to discover other novel potentially therapeutic approaches. It is noteworthy, however, that the pharmacological modulation of microglia could also have adverse effects as microglia have such physiological functions mentioned aboved. One recent study found that blockade of CD22, a canonical B-cell receptor which is up-regulated on aged microglia, reprogramed microglia toward a homeostatic transcriptional state and restored homeostatic microglial phagocytosis of α-syn fibrils (Pluvinage et al., 2019).
Apart from these pharmacological treatments, rTMS have been reported to have modulating effect (promoting/suppressing) on microglial activation, which may depend on the intensity and frequency of rTMS (Cullen and Young, 2016; Sasso et al., 2016; Cacace et al., 2017). Further research are needed to clarify the specific effect of rTMS on microglia as well as on astrocytes and neurons. In addition, accumulating evidence indicates that physical exercise could inhibit microglial activation and regulate neuroinflammation as well as improve the synaptic plasticity, whereby enhancing brain function and attenuating neurodegeneration (Mee-Inta et al., 2019).
Future Perspectives
Accumulating studies on PD pathogenesis have focused on activation of microglia and cross-talks between microglia and other immune factors. Activated microglia bear pro-inflammatory or anti-inflammatory properties depending on the nature, intensity and duration of the stimulus and associated with disease stage and severity. Importantly, activated microglia may exist along a dynamic continuum rather than be simply polarized into two categories. Activation of microglia triggered by damaged dopaminergic neurons could cause neuroinflammation, oxidative stress and cytokine-receptor-mediated apoptosis, which in turn enhance peripheral leucocyte recruitment. In this way, a vicious cycle comes into being, resulting in the exacerbation of the neurodegenerative process. In addition, cross-talks of α-syn-microglia, neuron-glia, astrocyte-microglia, and mast cell-glia as well as the microbiome-gut-brain axis are thought to be involved in the regulation of microglial activation and neuroinflammation. The existing available drugs of PD are able to mitigate symptoms by limiting the extent of inflammation (Glass et al., 2010). Remarkably, the strategy of the biological therapy should be to restore the immune balance, rather than simply inhibit the immune responses. A variety of agents or approaches, including candesartan cilexetil, rifampicin/rifampicin quinone, TAK-242, RSLA, β-caryophyllene, JWH133, α-asarone, tanshinone I, apocynin, resveratrol, diphenyleneiodonium, DN-TNF, lenalidomide, zonisamide, minocycline, dimethyl fumarate, some bioactive compounds extracted from various plants, JNJ7777120, MCC950, AAV2-hIL-10, Vitamin D, PDE inhibitors (rolipram, ibudilast), and PPAR-γ agonists (pioglitazone, rosiglitazone), might exert neuroprotective effects due to their regulatory effects on microglial activation. In addition, intervention of iron metabolism balance or microbiome-gut-brain axis as well as rTMS and physical exercise may offer emerging therapeutic strategies. In conclusion, microglial activation is a key point to understand the pathogenesis of PD and a potential therapeutic target for PD. Further investigations should focus on the dual role of reactive microglia to elucidate PD pathogenesis and detect emerging agents/approaches for PD.
Author Contributions
C-YL, XW, and CL searched the literature and drafted the manuscript. C-YL and H-LZ critically revised the manuscript. All authors listed have made a substantial, direct, and intellectual contribution to the work, and approved it for publication.
Conflict of Interest
The authors declare that the research was conducted in the absence of any commercial or financial relationships that could be construed as a potential conflict of interest.
Abbreviations
α-syn, α-synuclein; 6-OHDA, 6-hydroxydopamine; AAV2-hIL-10, adeno-associated viral vector containing the cDNA for human interleukin-10; AD, Alzheimer’s disease; BBB, blood-brain barrier; BDNF, brain-derived neurotrophic factor; bFGF, basic fibroblast growth factor; CB2, cannabinoid type 2; CNS, central nervous system; COX, cyclooxygenase; CX3CR1, CX3C chemokine receptor 1; CXCL, chemokine (C-X-C motif) ligand; cyclic AMP, cyclic adenosine monophosphate; DA, dopamine; DAP12, DNAX activation protein 12; DMT1, divalent metal transporter 1; DN-TNF, dominant-negative tumor necrosis factor; EAA, excitatory amino acids; EAE, experimental autoimmune encephalomyelitis; EGF, epidermal growth factor; ERK, extracellular signal-regulated kinase; GSH, glutathione; GWAS, genome-wide association studies; H4R, histamine-4 receptor; HLA, human leucocyte antigen; I κ B α, inhibitory protein- κ-Bα; IFN, interferon; IGF-1, insulin-like growth factor-1; IKK, I κ B kinase; IL, interleukin; iNOS, inducible nitric oxide synthase; IP-10, interferon-gamma-induced protein-10; IRAK, interleukin-1 receptor-associated kinase; IRF, interferon regulatory factor; IRP1, iron regulatory protein 1; JAK, Janus kinase; LPS, lipopolysaccharide; MAPK, mitogen-activated protein kinase; MHC, major histocompatibility complex; MMP, matrix metalloproteinase; MPTP, 1-methyl-4-phenyl-1,2,3,6-tetrahydropyridine; MS, multiple sclerosis; NADPH, nicotinamide adenine dinucleotide phosphate; NF- κ B, nuclear factor kappa B; NGF, nerve growth factor; NLRP3, NLR family pyrin domain containing 3; NO, nitric oxide; NSAID, nonsteroidal anti-inflammatory drug; NSPC, neural stem/progenitor cell; PAR-1, protease-activated receptor-1; PARP, poly ADP ribose polymerase; PD, Parkinson’s disease; PDE, phosphodiesterase; PET, positron emission tomography; PG, prostaglandins; PPAR, peroxisome proliferator-activated receptor; P-SAPK/JNK, phospho-stress-activated protein kinases/c-Jun N-terminal kinases; RNS, reactive nitrogen species; ROS, reactive oxygen species; rTMS, repetitive transcranial magnetic stimulation; SCFAs, short-chain fatty acids; SN, substantia nigra; SNpc, substantia nigra (SN) pars compacta; SOCS3, suppressor of cytokine signaling 3; solTNF, soluble tumor necrosis factor; SR, scavenger receptor; STAT, signal transducer and activator of transcription; TAK1, TGF- β-activated protein kinase 1; Tem, effector/memory T cells; TGF, transforming growth factor; TIR, toll-interleukin 1 receptor; TLR, toll-like receptor; TNF, tumor necrosis factor; TRAF6, TNF receptor-associated factor 6; TREM2, triggering receptor expressed on myeloid cells 2; TRPC1, transient receptor potential canonical 1; UPDRS-III, Unified Parkinson’s Disease Rating Scale III
References
Acuna, L., Hamadat, S., Corbalan, N. S., Gonzalez-Lizarraga, F., Dos-Santos-Pereira, M., Rocca, J., et al. (2019). Rifampicin and its derivative rifampicin quinone reduce microglial inflammatory responses and neurodegeneration induced in vitro by alpha-synuclein fibrillary aggregates. Cells 8:E776. doi: 10.3390/cells8080776
Agarwal, S., Yadav, A., and Chaturvedi, R. K. (2017). Peroxisome proliferator-activated receptors (PPARs) as therapeutic target in neurodegenerative disorders. Biochem. Biophys. Res. Commun. 483, 1166–1177. doi: 10.1016/j.bbrc.2016.08.043
Aguirre, A., Maturana, C. J., Harcha, P. A., and Saez, J. C. (2013). Possible involvement of TLRs and hemichannels in stress-induced CNS dysfunction via mastocytes, and glia activation. Mediators Inflamm. 2013:893521. doi: 10.1155/2013/893521
Alcalde, L. A., de Freitas, B. S., Machado, G. D. B., de Freitas Crivelaro, P. C., Dornelles, V. C., Gus, H., et al. (2018). Iron chelator deferiprone rescues memory deficits, hippocampal BDNF levels and antioxidant defenses in an experimental model of memory impairment. Biometals 31, 927–940. doi: 10.1007/s10534-018-0135-1
Austin, S. A., Floden, A. M., Murphy, E. J., and Combs, C. K. (2006). Alpha-synuclein expression modulates microglial activation phenotype. J. Neurosci. 26, 10558–10563. doi: 10.1523/jneurosci.1799-06.2006
Barnum, C. J., Chen, X., Chung, J., Chang, J., Williams, M., Grigoryan, N., et al. (2014). Peripheral administration of the selective inhibitor of soluble tumor necrosis factor (TNF) XPro(R)1595 attenuates nigral cell loss and glial activation in 6-OHDA hemiparkinsonian rats. J. Parkinsons Dis. 4, 349–360. doi: 10.3233/jpd-140410
Becker, G., and Berg, D. (2001). Neuroimaging in basal ganglia disorders: perspectives for transcranial ultrasound. Mov. Disord. 16, 23–32. doi: 10.1002/1531-8257(200101)16:1<23::aid-mds1003>3.0.co;2-2
Belloli, S., Pannese, M., Buonsanti, C., Maiorino, C., Di Grigoli, G., Carpinelli, A., et al. (2017). Early upregulation of 18-kDa translocator protein in response to acute neurodegenerative damage in TREM2-deficient mice. Neurobiol. Aging 53, 159–168. doi: 10.1016/j.neurobiolaging.2017.01.010
Bishop, G. M., Dang, T. N., Dringen, R., and Robinson, S. R. (2011). Accumulation of non-transferrin-bound iron by neurons, astrocytes, and microglia. Neurotox. Res. 19, 443–451. doi: 10.1007/s12640-010-9195-x
Boche, D., Perry, V. H., and Nicoll, J. A. (2013). Review: activation patterns of microglia and their identification in the human brain. Neuropathol. Appl. Neurobiol. 39, 3–18. doi: 10.1111/nan.12011
Boontanrart, M., Hall, S. D., Spanier, J. A., Hayes, C. E., and Olson, J. K. (2016). Vitamin D3 alters microglia immune activation by an IL-10 dependent SOCS3 mechanism. J. Neuroimmunol. 292, 126–136. doi: 10.1016/j.jneuroim.2016.01.015
Bove, J., and Perier, C. (2012). Neurotoxin-based models of Parkinson’s disease. Neuroscience 211, 51–76. doi: 10.1016/j.neuroscience.2011.10.057
Brakedal, B., Flones, I., Reiter, S. F., Torkildsen, O., Dolle, C., Assmus, J., et al. (2017). Glitazone use associated with reduced risk of Parkinson’s disease. Mov. Disord. 32, 1594–1599. doi: 10.1002/mds.27128
Braniste, V., Al-Asmakh, M., Kowal, C., Anuar, F., Abbaspour, A., Toth, M., et al. (2014). The gut microbiota influences blood-brain barrier permeability in mice. Sci. Transl. Med. 6:263ra158. doi: 10.1126/scitranslmed.3009759
Brauer, R., Bhaskaran, K., Chaturvedi, N., Dexter, D. T., Smeeth, L., and Douglas, I. (2015). Glitazone Treatment and incidence of Parkinson’s Disease among people with diabetes: a retrospective cohort study. PLoS Med. 12:e1001854. doi: 10.1371/journal.pmed.1001854
Bridi, J. C., and Hirth, F. (2018). Mechanisms of alpha-synuclein induced synaptopathy in Parkinson’s Disease. Front. Neurosci. 12:80. doi: 10.3389/fnins.2018.00080
Butovsky, O., Ziv, Y., Schwartz, A., Landa, G., Talpalar, A. E., Pluchino, S., et al. (2006). Microglia activated by IL-4 or IFN-gamma differentially induce neurogenesis and oligodendrogenesis from adult stem/progenitor cells. Mol. Cell. Neurosci. 31, 149–160. doi: 10.1016/j.mcn.2005.10.006
Cacace, F., Mineo, D., Viscomi, M. T., Latagliata, E. C., Mancini, M., Sasso, V., et al. (2017). Intermittent theta-burst stimulation rescues dopamine-dependent corticostriatal synaptic plasticity and motor behavior in experimental parkinsonism: possible role of glial activity. Mov. Disord. 32, 1035–1046. doi: 10.1002/mds.26982
Calo, L., Wegrzynowicz, M., Santivanez-Perez, J., and Grazia Spillantini, M. (2016). Synaptic failure and alpha-synuclein. Mov. Disord. 31, 169–177. doi: 10.1002/mds.26479
Calvello, R., Cianciulli, A., Nicolardi, G., De Nuccio, F., Giannotti, L., Salvatore, R., et al. (2017). Vitamin D treatment attenuates neuroinflammation and dopaminergic neurodegeneration in an animal model of Parkinson’s Disease. Shifting M1 to M2 microglia responses. J. Neuroimmune Pharmacol. 12, 327–339. doi: 10.1007/s11481-016-9720-7
Campolo, M., Casili, G., Biundo, F., Crupi, R., Cordaro, M., Cuzzocrea, S., et al. (2017). The Neuroprotective Effect of Dimethyl Fumarate in an MPTP-Mouse Model of Parkinson’s Disease: involvement of Reactive Oxygen Species/Nuclear Factor-kappaB/Nuclear Transcription Factor Related to NF-E2. Antioxid. Redox Signal. 27, 453–471. doi: 10.1089/ars.2016.6800
Caputi, V., and Giron, M. C. (2018). Microbiome-gut-brain axis and toll-like receptors in Parkinson’s Disease. Int. J. Mol. Sci. 19:E1689. doi: 10.3390/ijms19061689
Cardona, A. E., Pioro, E. P., Sasse, M. E., Kostenko, V., Cardona, S. M., Dijkstra, I. M., et al. (2006). Control of microglial neurotoxicity by the fractalkine receptor. Nat. Neurosci. 9, 917–924. doi: 10.1038/nn1715
Cassano, T., Calcagnini, S., Pace, L., De Marco, F., Romano, A., and Gaetani, S. (2017). Cannabinoid receptor 2 signaling in neurodegenerative disorders: from pathogenesis to a promising therapeutic target. Front. Neurosci. 11:30. doi: 10.3389/fnins.2017.00030
Catalin, B., Stopper, L., Balseanu, T. A., and Scheller, A. (2017). The in situ morphology of microglia is highly sensitive to the mode of tissue fixation. J. Chem. Neuroanat. 86, 59–66. doi: 10.1016/j.jchemneu.2017.08.007
Charvin, D., Medori, R., Hauser, R. A., and Rascol, O. (2018). Therapeutic strategies for Parkinson disease: beyond dopaminergic drugs. Nat. Rev. Drug Discov. 17, 804–822. doi: 10.1038/nrd.2018.136
Chauhan, A., Sun, Y., Sukumaran, P., Quenum Zangbede, F. O., Jondle, C. N., Sharma, A., et al. (2018). M1 macrophage polarization is dependent on TRPC1-mediated calcium entry. Science 8, 85–102. doi: 10.1016/j.isci.2018.09.014
Chen, H., Jacobs, E., Schwarzschild, M. A., McCullough, M. L., Calle, E. E., Thun, M. J., et al. (2005). Nonsteroidal antiinflammatory drug use and the risk for Parkinson’s disease. Ann. Neurol. 58, 963–967. doi: 10.1002/ana.20682
Chhor, V., Le Charpentier, T., Lebon, S., Ore, M. V., Celador, I. L., Josserand, J., et al. (2013). Characterization of phenotype markers and neuronotoxic potential of polarised primary microglia in vitro. Brain Behav. Immun. 32, 70–85. doi: 10.1016/j.bbi.2013.02.005
Cho, C. E., Damle, S. S., Wancewicz, E. V., Mukhopadhyay, S., Hart, C. E., Mazur, C., et al. (2019). A modular analysis of microglia gene expression, insights into the aged phenotype. BMC Genomics 20:164. doi: 10.1186/s12864-019-5549-9
Choi, S. H., Aid, S., Kim, H. W., Jackson, S. H., and Bosetti, F. (2012). Inhibition of NADPH oxidase promotes alternative and anti-inflammatory microglial activation during neuroinflammation. J. Neurochem. 120, 292–301. doi: 10.1111/j.1471-4159.2011.07572.x
Chow, J. C., Young, D. W., Golenbock, D. T., Christ, W. J., and Gusovsky, F. (1999). Toll-like receptor-4 mediates lipopolysaccharide-induced signal transduction. J. Biol. Chem. 274, 10689–10692. doi: 10.1074/jbc.274.16.10689
Chung, Y. C., Shin, W. H., Baek, J. Y., Cho, E. J., Baik, H. H., Kim, S. R., et al. (2016). CB2 receptor activation prevents glial-derived neurotoxic mediator production, BBB leakage and peripheral immune cell infiltration and rescues dopamine neurons in the MPTP model of Parkinson’s disease. Exp. Mol. Med. 48:e205. doi: 10.1038/emm.2015.100
Colonna, M., and Butovsky, O. (2017). Microglia function in the central nervous system during health and neurodegeneration. Annu. Rev. Immunol. 35, 441–468. doi: 10.1146/annurev-immunol-051116-052358
Connolly, J. G., Bykov, K., and Gagne, J. J. (2015). Thiazolidinediones and parkinson disease: a cohort study. Am. J. Epidemiol. 182, 936–944. doi: 10.1093/aje/kwv109
Crielaard, B. J., Lammers, T., and Rivella, S. (2017). Targeting iron metabolism in drug discovery and delivery. Nat. Rev. Drug Discov. 16, 400–423. doi: 10.1038/nrd.2016.248
Cullen, C. L., and Young, K. M. (2016). How Does Transcranial Magnetic Stimulation Influence Glial Cells in the Central Nervous System? Front. Neural Circuits 10:26. doi: 10.3389/fncir.2016.00026
Daniele, S. G., Beraud, D., Davenport, C., Cheng, K., Yin, H., and Maguire-Zeiss, K. A. (2015). Activation of MyD88-dependent TLR1/2 signaling by misfolded alpha-synuclein, a protein linked to neurodegenerative disorders. Sci. Signal. 8:ra45. doi: 10.1126/scisignal.2005965
Dasu, M. R., Riosvelasco, A. C., and Jialal, I. (2009). Candesartan inhibits Toll-like receptor expression and activity both in vitro and in vivo. Atherosclerosis 202, 76–83. doi: 10.1016/j.atherosclerosis.2008.04.010
Davalos, D., Grutzendler, J., Yang, G., Kim, J. V., Zuo, Y., Jung, S., et al. (2005). ATP mediates rapid microglial response to local brain injury in vivo. Nat. Neurosci. 8, 752–758. doi: 10.1038/nn1472
Dehmer, T., Heneka, M. T., Sastre, M., Dichgans, J., and Schulz, J. B. (2004). Protection by pioglitazone in the MPTP model of Parkinson’s disease correlates with I kappa B alpha induction and block of NF kappa B and iNOS activation. J. Neurochem. 88, 494–501. doi: 10.1046/j.1471-4159.2003.02210.x
Dentesano, G., Serratosa, J., Tusell, J. M., Ramon, P., Valente, T., Saura, J., et al. (2014). CD200R1 and CD200 expression are regulated by PPAR-gamma in activated glial cells. Glia 62, 982–998. doi: 10.1002/glia.22656
Doorn, K. J., Moors, T., Drukarch, B., van de Berg, W., Lucassen, P. J., and van Dam, A. M. (2014). Microglial phenotypes and toll-like receptor 2 in the substantia nigra and hippocampus of incidental Lewy body disease cases and Parkinson’s disease patients. Acta Neuropathol. Commun. 2:90. doi: 10.1186/s40478-014-0090-1
Du, L., Zhang, Y., Chen, Y., Zhu, J., Yang, Y., and Zhang, H. L. (2017). Role of microglia in neurological disorders and their potentials as a therapeutic target. Mol. Neurobiol. 54, 7567–7584. doi: 10.1007/s12035-016-0245-0
Dulla, Y. A., Kurauchi, Y., Hisatsune, A., Seki, T., Shudo, K., and Katsuki, H. (2016). Regulatory mechanisms of vitamin D3 on production of nitric oxide and pro-inflammatory cytokines in microglial BV-2 cells. Neurochem. Res. 41, 2848–2858. doi: 10.1007/s11064-016-2000-3
Edison, P., Ahmed, I., Fan, Z., Hinz, R., Gelosa, G., Ray Chaudhuri, K., et al. (2013). Microglia, amyloid, and glucose metabolism in Parkinson’s disease with and without dementia. Neuropsychopharmacology 38, 938–949. doi: 10.1038/npp.2012.255
Erny, D., Hrabe, de Angelis, A. L., Jaitin, D., Wieghofer, P., Staszewski, O., et al. (2015). Host microbiota constantly control maturation and function of microglia in the CNS. Nat. Neurosci. 18, 965–977. doi: 10.1038/nn.4030
Fellner, L., Irschick, R., Schanda, K., Reindl, M., Klimaschewski, L., Poewe, W., et al. (2013). Toll-like receptor 4 is required for alpha-synuclein dependent activation of microglia and astroglia. Glia 61, 349–360. doi: 10.1002/glia.22437
Femminella, G. D., Ninan, S., Atkinson, R., Fan, Z., Brooks, D. J., and Edison, P. (2016). Does microglial activation influence hippocampal volume and neuronal function in alzheimer’s disease and parkinson’s disease dementia? J. Alzheimers Dis. 51, 1275–1289. doi: 10.3233/jad-150827
Ferreira, S. A., and Romero-Ramos, M. (2018). Microglia response during parkinson’s disease: alpha-synuclein intervention. Front. Cell. Neurosci. 12:247. doi: 10.3389/fncel.2018.00247
Fiebich, B. L., Batista, C. R. A., Saliba, S. W., Yousif, N. M., and de Oliveira, A. C. P. (2018). Role of Microglia TLRs in neurodegeneration. Front. Cell. Neurosci. 12:329. doi: 10.3389/fncel.2018.00329
Franco, R., and Fernandez-Suarez, D. (2015). Alternatively activated microglia and macrophages in the central nervous system. Prog. Neurobiol. 131, 65–86. doi: 10.1016/j.pneurobio.2015.05.003
Fricke, I. B., Viel, T., Worlitzer, M. M., Collmann, F. M., Vrachimis, A., Faust, A., et al. (2016). 6-hydroxydopamine-induced Parkinson’s disease-like degeneration generates acute microgliosis and astrogliosis in the nigrostriatal system but no bioluminescence imaging-detectable alteration in adult neurogenesis. Eur. J. Neurosci. 43, 1352–1365. doi: 10.1111/ejn.13232
Funk, N., Wieghofer, P., Grimm, S., Schaefer, R., Buhring, H. J., Gasser, T., et al. (2013). Characterization of peripheral hematopoietic stem cells and monocytes in Parkinson’s disease. Mov. Disord. 28, 392–395. doi: 10.1002/mds.25300
Gagne, J. J., and Power, M. C. (2010). Anti-inflammatory drugs and risk of Parkinson disease: a meta-analysis. Neurology 74, 995–1002. doi: 10.1212/WNL.0b013e3181d5a4a3
Gao, H. M., and Hong, J. S. (2008). Why neurodegenerative diseases are progressive: uncontrolled inflammation drives disease progression. Trends Immunol. 29, 357–365. doi: 10.1016/j.it.2008.05.002
Gao, X., Chen, H., Schwarzschild, M. A., and Ascherio, A. (2011). Use of ibuprofen and risk of Parkinson disease. Neurology 76, 863–869. doi: 10.1212/WNL.0b013e31820f2d79
George, S., Rey, N. L., Tyson, T., Esquibel, C., Meyerdirk, L., Schulz, E., et al. (2019). Microglia affect alpha-synuclein cell-to-cell transfer in a mouse model of Parkinson’s disease. Mol. Neurodegener. 14:34. doi: 10.1186/s13024-019-0335-3
Gerhard, A., Pavese, N., Hotton, G., Turkheimer, F., Es, M., Hammers, A., et al. (2006). In vivo imaging of microglial activation with [11C](R)-PK11195 PET in idiopathic Parkinson’s disease. Neurobiol. Dis. 21, 404–412. doi: 10.1016/j.nbd.2005.08.002
Ghadery, C., Koshimori, Y., Coakeley, S., Harris, M., Rusjan, P., Kim, J., et al. (2017). Microglial activation in Parkinson’s disease using [(18)F]-FEPPA. J. Neuroinflamm. 14:8. doi: 10.1186/s12974-016-0778-1
Ghasemi, F., Bagheri, H., Barreto, G. E., Read, M. I., and Sahebkar, A. (2019). Effects of curcumin on microglial cells. Neurotox. Res. 36, 12–26. doi: 10.1007/s12640-019-00030-0
Ghosh, M., Xu, Y., and Pearse, D. D. (2016). Cyclic AMP is a key regulator of M1 to M2a phenotypic conversion of microglia in the presence of Th2 cytokines. J. Neuroinflamm. 13:9. doi: 10.1186/s12974-015-0463-9
Glass, C. K., Saijo, K., Winner, B., Marchetto, M. C., and Gage, F. H. (2010). Mechanisms underlying inflammation in neurodegeneration. Cell 140, 918–934. doi: 10.1016/j.cell.2010.02.016
Gonzalez, H., Elgueta, D., Montoya, A., and Pacheco, R. (2014). Neuroimmune regulation of microglial activity involved in neuroinflammation and neurodegenerative diseases. J. Neuroimmunol. 274, 1–13. doi: 10.1016/j.jneuroim.2014.07.012
Gordon, R., Albornoz, E. A., Christie, D. C., Langley, M. R., Kumar, V., Mantovani, S., et al. (2018). Inflammasome inhibition prevents alpha-synuclein pathology and dopaminergic neurodegeneration in mice. Sci. Transl. Med. 10:eaah4066. doi: 10.1126/scitranslmed.aah4066
Gosselin, D., Skola, D., Coufal, N. G., Holtman, I. R., Schlachetzki, J. C. M., Sajti, E., et al. (2017). An environment-dependent transcriptional network specifies human microglia identity. Science 356:eaal3222. doi: 10.1126/science.aal3222
Grabert, K., Michoel, T., Karavolos, M. H., Clohisey, S., Baillie, J. K., Stevens, M. P., et al. (2016). Microglial brain region-dependent diversity and selective regional sensitivities to aging. Nat. Neurosci. 19, 504–516. doi: 10.1038/nn.4222
Gupta, A., Dhir, A., Kumar, A., and Kulkarni, S. K. (2009). Protective effect of cyclooxygenase (COX)-inhibitors against drug-induced catatonia and MPTP-induced striatal lesions in rats. Pharmacol. Biochem. Behav. 94, 219–226. doi: 10.1016/j.pbb.2009.07.018
Hain, E. G., Sparenberg, M., Rasinska, J., Klein, C., Akyuz, L., and Steiner, B. (2018). Indomethacin promotes survival of new neurons in the adult murine hippocampus accompanied by anti-inflammatory effects following MPTP-induced dopamine depletion. J. Neuroinflamm. 15:162. doi: 10.1186/s12974-018-1179-4
Hamza, T. H., Zabetian, C. P., Tenesa, A., Laederach, A., Montimurro, J., Yearout, D., et al. (2010). Common genetic variation in the HLA region is associated with late-onset sporadic Parkinson’s disease. Nat. Genet. 42, 781–785. doi: 10.1038/ng.642
Hansen, C., Angot, E., Bergstrom, A. L., Steiner, J. A., Pieri, L., Paul, G., et al. (2011). alpha-Synuclein propagates from mouse brain to grafted dopaminergic neurons and seeds aggregation in cultured human cells. J. Clin. Invest. 121, 715–725. doi: 10.1172/jci43366
Hare, D. J., and Double, K. L. (2016). Iron and dopamine: a toxic couple. Brain 139, 1026–1035. doi: 10.1093/brain/aww022
Harms, A. S., Barnum, C. J., Ruhn, K. A., Varghese, S., Trevino, I., Blesch, A., et al. (2011). Delayed dominant-negative TNF gene therapy halts progressive loss of nigral dopaminergic neurons in a rat model of Parkinson’s disease. Mol. Ther. 19, 46–52. doi: 10.1038/mt.2010.217
Harrison, J. K., Jiang, Y., Chen, S., Xia, Y., Maciejewski, D., McNamara, R. K., et al. (1998). Role for neuronally derived fractalkine in mediating interactions between neurons and CX3CR1-expressing microglia. Proc. Natl. Acad. Sci. U.S.A. 95, 10896–10901. doi: 10.1073/pnas.95.18.10896
Healy, S., McMahon, J., Owens, P., and FitzGerald, U. (2016). Significant glial alterations in response to iron loading in a novel organotypic hippocampal slice culture model. Sci. Rep. 6:36410. doi: 10.1038/srep36410
Heng, Y., Zhang, Q. S., Mu, Z., Hu, J. F., Yuan, Y. H., and Chen, N. H. (2016). Ginsenoside Rg1 attenuates motor impairment and neuroinflammation in the MPTP-probenecid-induced parkinsonism mouse model by targeting alpha-synuclein abnormalities in the substantia nigra. Toxicol. Lett. 243, 7–21. doi: 10.1016/j.toxlet.2015.12.005
Hernan, M. A., Logroscino, G., and Garcia Rodriguez, L. A. (2006). Nonsteroidal anti-inflammatory drugs and the incidence of Parkinson disease. Neurology 66, 1097–1099. doi: 10.1212/01.wnl.0000204446.82823.28
Hill-Burns, E. M., Factor, S. A., Zabetian, C. P., Thomson, G., and Payami, H. (2011). Evidence for more than one Parkinson’s disease-associated variant within the HLA region. PLoS One 6:e27109. doi: 10.1371/journal.pone.0027109
Hirsch, E. C., and Hunot, S. (2009). Neuroinflammation in Parkinson’s disease: a target for neuroprotection? Lancet Neurol. 8, 382–397. doi: 10.1016/s1474-4422(09)70062-6
Hirsch, L., Jette, N., Frolkis, A., Steeves, T., and Pringsheim, T. (2016). The incidence of parkinson’s disease: a systematic review and meta-analysis. Neuroepidemiology 46, 292–300. doi: 10.1159/000445751
Ho, M. S. (2019). Microglia in parkinson’s disease. Adv. Exp. Med. Biol. 1175, 335–353. doi: 10.1007/978-981-13-9913-8_13
Holtman, I. R., Raj, D. D., Miller, J. A., Schaafsma, W., Yin, Z., Brouwer, N., et al. (2015). Induction of a common microglia gene expression signature by aging and neurodegenerative conditions: a co-expression meta-analysis. Acta Neuropathol. Commun. 3:31. doi: 10.1186/s40478-015-0203-5
Hossain, M. M., Weig, B., Reuhl, K., Gearing, M., Wu, L. J., and Richardson, J. R. (2018). The anti-parkinsonian drug zonisamide reduces neuroinflammation: role of microglial Nav 1.6. Exp. Neurol. 308, 111–119. doi: 10.1016/j.expneurol.2018.07.005
Houser, M. C., and Tansey, M. G. (2017). The gut-brain axis: is intestinal inflammation a silent driver of Parkinson’s disease pathogenesis? NPJ Parkinsons Dis. 3:3. doi: 10.1038/s41531-016-0002-0
Hu, X., and Ivashkiv, L. B. (2009). Cross-regulation of signaling pathways by interferon-gamma: implications for immune responses and autoimmune diseases. Immunity 31, 539–550. doi: 10.1016/j.immuni.2009.09.002
Hughes, C. D., Choi, M. L., Ryten, M., Hopkins, L., Drews, A., Botia, J. A., et al. (2019). Picomolar concentrations of oligomeric alpha-synuclein sensitizes TLR4 to play an initiating role in Parkinson’s disease pathogenesis. Acta Neuropathol. 137, 103–120. doi: 10.1007/s00401-018-1907-y
Hunot, S., and Hirsch, E. C. (2003). Neuroinflammatory processes in Parkinson’s disease. Ann. Neurol. 53, S49–S58. doi: 10.1002/ana.10481
Iannaccone, S., Cerami, C., Alessio, M., Garibotto, V., Panzacchi, A., Olivieri, S., et al. (2013). In vivo microglia activation in very early dementia with Lewy bodies, comparison with Parkinson’s disease. Parkinsonism Relat. Disord. 19, 47–52. doi: 10.1016/j.parkreldis.2012.07.002
Imamura, K., Hishikawa, N., Sawada, M., Nagatsu, T., Yoshida, M., and Hashizume, Y. (2003). Distribution of major histocompatibility complex class II-positive microglia and cytokine profile of Parkinson’s disease brains. Acta Neuropathol. 106, 518–526. doi: 10.1007/s00401-003-0766-2
Ingelsson, M. (2016). Alpha-synuclein oligomers-neurotoxic molecules in Parkinson’s Disease and other lewy body disorders. Front. Neurosci. 10:408. doi: 10.3389/fnins.2016.00408
Iravani, M. M., Kashefi, K., Mander, P., Rose, S., and Jenner, P. (2002). Involvement of inducible nitric oxide synthase in inflammation-induced dopaminergic neurodegeneration. Neuroscience 110, 49–58. doi: 10.1016/s0306-4522(01)00562-0
Janda, E., Boi, L., and Carta, A. R. (2018). Microglial phagocytosis and its regulation: a therapeutic target in Parkinson’s Disease? Front. Mol. Neurosci. 11:144. doi: 10.3389/fnmol.2018.00144
Jang, Y., Koo, J. H., Kwon, I., Kang, E. B., Um, H. S., Soya, H., et al. (2017). Neuroprotective effects of endurance exercise against neuroinflammation in MPTP-induced Parkinson’s disease mice. Brain Res. 1655, 186–193. doi: 10.1016/j.brainres.2016.10.029
Javed, H., Azimullah, S., Haque, M. E., and Ojha, S. K. (2016). Cannabinoid type 2 (CB2) receptors activation protects against oxidative stress and neuroinflammation associated dopaminergic neurodegeneration in rotenone model of parkinson’s disease. Front. Neurosci. 10:321. doi: 10.3389/fnins.2016.00321
Jiang, H., Li, L. J., Wang, J., and Xie, J. X. (2008). Ghrelin antagonizes MPTP-induced neurotoxicity to the dopaminergic neurons in mouse substantia nigra. Exp. Neurol. 212, 532–537. doi: 10.1016/j.expneurol.2008.05.006
Jiang, T., Hoekstra, J., Heng, X., Kang, W., Ding, J., Liu, J., et al. (2015). P2X7 receptor is critical in alpha-synuclein–mediated microglial NADPH oxidase activation. Neurobiol. Aging 36, 2304–2318. doi: 10.1016/j.neurobiolaging.2015.03.015
Jiang, T., Zhang, Y. D., Chen, Q., Gao, Q., Zhu, X. C., Zhou, J. S., et al. (2016). TREM2 modifies microglial phenotype and provides neuroprotection in P301S tau transgenic mice. Neuropharmacology 105, 196–206. doi: 10.1016/j.neuropharm.2016.01.028
Joers, V., Tansey, M. G., Mulas, G., and Carta, A. R. (2017). Microglial phenotypes in Parkinson’s disease and animal models of the disease. Prog. Neurobiol. 155, 57–75. doi: 10.1016/j.pneurobio.2016.04.006
Joniec-Maciejak, I., Ciesielska, A., Wawer, A., Sznejder-Pacholek, A., Schwenkgrub, J., Cudna, A., et al. (2014). The influence of AAV2-mediated gene transfer of human IL-10 on neurodegeneration and immune response in a murine model of Parkinson’s disease. Pharmacol. Rep. 66, 660–669. doi: 10.1016/j.pharep.2014.03.008
Kaur, D., Yantiri, F., Rajagopalan, S., Kumar, J., Mo, J. Q., Boonplueang, R., et al. (2003). Genetic or pharmacological iron chelation prevents MPTP-induced neurotoxicity in vivo: a novel therapy for Parkinson’s disease. Neuron 37, 899–909. doi: 10.1016/s0896-6273(03)00126-0
Kawabori, M., Kacimi, R., Kauppinen, T., Calosing, C., Kim, J. Y., Hsieh, C. L., et al. (2015). Triggering receptor expressed on myeloid cells 2 (TREM2) deficiency attenuates phagocytic activities of microglia and exacerbates ischemic damage in experimental stroke. J. Neurosci. 35, 3384–3396. doi: 10.1523/jneurosci.2620-14.2015
Kawai, T., and Akira, S. (2007). Signaling to NF-kappaB by Toll-like receptors. Trends Mol. Med. 13, 460–469. doi: 10.1016/j.molmed.2007.09.002
Kawai, T., and Akira, S. (2010). The role of pattern-recognition receptors in innate immunity: update on Toll-like receptors. Nat. Immunol. 11, 373–384. doi: 10.1038/ni.1863
Kawai, T., Takeuchi, O., Fujita, T., Inoue, J., Muhlradt, P. F., Sato, S., et al. (2001). Lipopolysaccharide stimulates the MyD88-independent pathway and results in activation of IFN-regulatory factor 3 and the expression of a subset of lipopolysaccharide-inducible genes. J. Immunol. 167, 5887–5894. doi: 10.4049/jimmunol.167.10.5887
Kettenmann, H., Hanisch, U. K., Noda, M., and Verkhratsky, A. (2011). Physiology of microglia. Physiol. Rev. 91, 461–553. doi: 10.1152/physrev.00011.2010
Kierdorf, K., and Prinz, M. (2017). Microglia in steady state. J. Clin. Invest. 127, 3201–3209. doi: 10.1172/JCI90602
Kim, B. W., Koppula, S., Kumar, H., Park, J. Y., Kim, I. W., More, S. V., et al. (2015). alpha-Asarone attenuates microglia-mediated neuroinflammation by inhibiting NF kappa B activation and mitigates MPTP-induced behavioral deficits in a mouse model of Parkinson’s disease. Neuropharmacology 97, 46–57. doi: 10.1016/j.neuropharm.2015.04.037
Kim, C., Ho, D. H., Suk, J. E., You, S., Michael, S., Kang, J., et al. (2013). Neuron-released oligomeric alpha-synuclein is an endogenous agonist of TLR2 for paracrine activation of microglia. Nat. Commun. 4:1562. doi: 10.1038/ncomms2534
Kim, J. S., Ryu, S. Y., Yun, I., Kim, W. J., Lee, K. S., Park, J. W., et al. (2006). 1alpha,25-dihydroxyvitamin D(3) protects dopaminergic neurons in rodent models of Parkinson’s disease through inhibition of microglial activation. J. Clin. Neurol. 2, 252–257. doi: 10.3988/jcn.2006.2.4.252
Kinoshita, K. I., Muroi, Y., Unno, T., and Ishii, T. (2017). Rolipram improves facilitation of contextual fear extinction in the 1-methyl-4-phenyl-1,2,3,6-tetrahydropyridine-induced mouse model of Parkinson’s disease. J. Pharmacol. Sci. 134, 55–58. doi: 10.1016/j.jphs.2017.04.002
Knott, C., Stern, G., and Wilkin, G. P. (2000). Inflammatory regulators in Parkinson’s disease: iNOS, lipocortin-1, and cyclooxygenases-1 and -2. Mol. Cell. Neurosci. 16, 724–739. doi: 10.1006/mcne.2000.0914
Kobayashi, K., Imagama, S., Ohgomori, T., Hirano, K., Uchimura, K., Sakamoto, K., et al. (2013). Minocycline selectively inhibits M1 polarization of microglia. Cell Death Dis. 4:e525. doi: 10.1038/cddis.2013.54
Konishi, H., and Kiyama, H. (2018). Microglial TREM2/DAP12 signaling: a double-edged sword in neural diseases. Front. Cell. Neurosci. 12:206. doi: 10.3389/fncel.2018.00206
Koo, J. H., Jang, Y. C., Hwang, D. J., Um, H. S., Lee, N. H., Jung, J. H., et al. (2017). Treadmill exercise produces neuroprotective effects in a murine model of Parkinson’s disease by regulating the TLR2/MyD88/NF-kappaB signaling pathway. Neuroscience 356, 102–113. doi: 10.1016/j.neuroscience.2017.05.016
Kouli, A., Horne, C. B., and Williams-Gray, C. H. (2019). Toll-like receptors and their therapeutic potential in Parkinson’s disease and alpha-synucleinopathies. Brain Behav. Immun. 81, 41–51. doi: 10.1016/j.bbi.2019.06.042
Kumar, A., and Singh, N. (2017). Inhibitor of Phosphodiestearse-4 improves memory deficits, oxidative stress, neuroinflammation and neuropathological alterations in mouse models of dementia of Alzheimer’s Type. Biomed. Pharmacother. 88, 698–707. doi: 10.1016/j.biopha.2017.01.059
Kurkowska-Jastrzebska, I., Wronska, A., Kohutnicka, M., Czlonkowski, A., and Czlonkowska, A. (1999). The inflammatory reaction following 1-methyl-4-phenyl-1,2,3, 6-tetrahydropyridine intoxication in mouse. Exp. Neurol. 156, 50–61. doi: 10.1006/exnr.1998.6993
La Vitola, P., Balducci, C., Cerovic, M., Santamaria, G., Brandi, E., Grandi, F., et al. (2018). Alpha-synuclein oligomers impair memory through glial cell activation and via Toll-like receptor 2. Brain Behav. Immun. 69, 591–602. doi: 10.1016/j.bbi.2018.02.012
Lawand, N. B., Saade, N. E., El-Agnaf, O. M., and Safieh-Garabedian, B. (2015). Targeting alpha-synuclein as a therapeutic strategy for Parkinson’s disease. Expert Opin. Ther. Targets 19, 1351–1360. doi: 10.1517/14728222.2015.1062877
Lawson, L. J., Perry, V. H., Dri, P., and Gordon, S. (1990). Heterogeneity in the distribution and morphology of microglia in the normal adult mouse brain. Neuroscience 39, 151–170. doi: 10.1016/0306-4522(90)90229-w
Le, W., Rowe, D., Xie, W., Ortiz, I., He, Y., and Appel, S. H. (2001). Microglial activation and dopaminergic cell injury: an in vitro model relevant to Parkinson’s disease. J. Neurosci. 21, 8447–8455. doi: 10.1523/jneurosci.21-21-08447.2001
Lee, E. J., Woo, M. S., Moon, P. G., Baek, M. C., Choi, I. Y., Kim, W. K., et al. (2010). Alpha-synuclein activates microglia by inducing the expressions of matrix metalloproteinases and the subsequent activation of protease-activated receptor-1. J. Immunol. 185, 615–623. doi: 10.4049/jimmunol.0903480
Lee, M. K., Stirling, W., Xu, Y., Xu, X., Qui, D., Mandir, A. S., et al. (2002). Human alpha-synuclein-harboring familial Parkinson’s disease-linked Ala-53 –> Thr mutation causes neurodegenerative disease with alpha-synuclein aggregation in transgenic mice. Proc. Natl. Acad. Sci. U.S.A. 99, 8968–8973. doi: 10.1073/pnas.132197599
Lehericy, S., Bardinet, E., Poupon, C., Vidailhet, M., and Francois, C. (2014). 7 Tesla magnetic resonance imaging: a closer look at substantia nigra anatomy in Parkinson’s disease. Mov. Disord. 29, 1574–1581. doi: 10.1002/mds.26043
L’Episcopo, F., Tirolo, C., Serapide, M. F., Caniglia, S., Testa, N., Leggio, L., et al. (2018). Microglia polarization, gene-environment interactions and wnt/beta-catenin signaling: emerging roles of glia-neuron and glia-stem/neuroprogenitor crosstalk for dopaminergic neurorestoration in aged parkinsonian brain. Front. Aging Neurosci. 10:12. doi: 10.3389/fnagi.2018.00012
Lhermitte, J., Kraus, W. M., and McAlpine, D. (1924). Original Papers: on the occurrence of abnormal deposits of iron in the brain in parkinsonism with special reference to its localisation. J. Neurol. Psychopathol. 5, 195–208. doi: 10.1136/jnnp.s1-5.19.195
Li, J. Y., Englund, E., Holton, J. L., Soulet, D., Hagell, P., Lees, A. J., et al. (2008). Lewy bodies in grafted neurons in subjects with Parkinson’s disease suggest host-to-graft disease propagation. Nat. Med. 14, 501–503. doi: 10.1038/nm1746
Lima, L. A. R., Lopes, M. J. P., Costa, R. O., Lima, F. A. V., Neves, K. R. T., Calou, I. B. F., et al. (2018). Vitamin D protects dopaminergic neurons against neuroinflammation and oxidative stress in hemiparkinsonian rats. J. Neuroinflamm. 15:249. doi: 10.1186/s12974-018-1266-6
Lin, S. C., Lo, Y. C., and Wu, H. (2010). Helical assembly in the MyD88-IRAK4-IRAK2 complex in TLR/IL-1R signalling. Nature 465, 885–890. doi: 10.1038/nature09121
Longhena, F., Faustini, G., Spillantini, M. G., and Bellucci, A. (2019). Living in promiscuity: the multiple partners of alpha-synuclein at the synapse in physiology and pathology. Int. J. Mol. Sci. 20:E141. doi: 10.3390/ijms20010141
Lopez Gonzalez, I., Garcia-Esparcia, P., Llorens, F., and Ferrer, I. (2016). Genetic and transcriptomic profiles of inflammation in neurodegenerative diseases: alzheimer, parkinson, creutzfeldt-jakob and tauopathies. Int. J. Mol. Sci. 17:206. doi: 10.3390/ijms17020206
Lv, R., Du, L., Liu, X., Zhou, F., Zhang, Z., and Zhang, L. (2019). Rosmarinic acid attenuates inflammatory responses through inhibiting HMGB1/TLR4/NF-kappaB signaling pathway in a mouse model of Parkinson’s disease. Life Sci. 223, 158–165. doi: 10.1016/j.lfs.2019.03.030
Manocha, G. D., Floden, A. M., Puig, K. L., Nagamoto-Combs, K., Scherzer, C. R., and Combs, C. K. (2017). Defining the contribution of neuroinflammation to Parkinson’s disease in humanized immune system mice. Mol. Neurodegener. 12:17. doi: 10.1186/s13024-017-0158-z
Mantovani, A., Sica, A., and Locati, M. (2005). Macrophage polarization comes of age. Immunity 23, 344–346. doi: 10.1016/j.immuni.2005.10.001
Maresz, K., Carrier, E. J., Ponomarev, E. D., Hillard, C. J., and Dittel, B. N. (2005). Modulation of the cannabinoid CB2 receptor in microglial cells in response to inflammatory stimuli. J. Neurochem. 95, 437–445. doi: 10.1111/j.1471-4159.2005.03380.x
Marinova-Mutafchieva, L., Sadeghian, M., Broom, L., Davis, J. B., Medhurst, A. D., and Dexter, D. T. (2009). Relationship between microglial activation and dopaminergic neuronal loss in the substantia nigra: a time course study in a 6-hydroxydopamine model of Parkinson’s disease. J. Neurochem. 110, 966–975. doi: 10.1111/j.1471-4159.2009.06189.x
Martinez, F. O., and Gordon, S. (2014). The M1 and M2 paradigm of macrophage activation: time for reassessment. F1000Prime Rep. 6:13. doi: 10.12703/p6-13
Martinez, F. O., Gordon, S., Locati, M., and Mantovani, A. (2006). Transcriptional profiling of the human monocyte-to-macrophage differentiation and polarization: new molecules and patterns of gene expression. J. Immunol. 177, 7303–7311. doi: 10.4049/jimmunol.177.10.7303
Matak, P., Matak, A., Moustafa, S., Aryal, D. K., Benner, E. J., Wetsel, W., et al. (2016). Disrupted iron homeostasis causes dopaminergic neurodegeneration in mice. Proc. Natl. Acad. Sci. U.S.A. 113, 3428–3435. doi: 10.1073/pnas.1519473113
McCoy, M. K., Martinez, T. N., Ruhn, K. A., Szymkowski, D. E., Smith, C. G., Botterman, B. R., et al. (2006). Blocking soluble tumor necrosis factor signaling with dominant-negative tumor necrosis factor inhibitor attenuates loss of dopaminergic neurons in models of Parkinson’s disease. J. Neurosci. 26, 9365–9375. doi: 10.1523/jneurosci.1504-06.2006
McCoy, M. K., Ruhn, K. A., Martinez, T. N., McAlpine, F. E., Blesch, A., and Tansey, M. G. (2008). Intranigral lentiviral delivery of dominant-negative TNF attenuates neurodegeneration and behavioral deficits in hemiparkinsonian rats. Mol. Ther. 16, 1572–1579. doi: 10.1038/mt.2008.146
McGeer, P. L., Itagaki, S., Boyes, B. E., and McGeer, E. G. (1988). Reactive microglia are positive for HLA-DR in the substantia nigra of Parkinson’s and Alzheimer’s disease brains. Neurology 38, 1285–1291.
Mecca, C., Giambanco, I., Donato, R., and Arcuri, C. (2018). Microglia and aging: the role of the TREM2-DAP12 and CX3CL1-CX3CR1 axes. Int. J. Mol. Sci. 19:E318. doi: 10.3390/ijms19010318
Mee-Inta, O., Zhao, Z. W., and Kuo, Y. M. (2019). Physical exercise inhibits inflammation and microglial activation. Cells 8:E691. doi: 10.3390/cells8070691
Mehlhase, J., Sandig, G., Pantopoulos, K., and Grune, T. (2005). Oxidation-induced ferritin turnover in microglial cells: role of proteasome. Free Radic. Biol. Med. 38, 276–285. doi: 10.1016/j.freeradbiomed.2004.10.025
Meng, F., Guo, Z., Hu, Y., Mai, W., Zhang, Z., Zhang, B., et al. (2019). CD73-derived adenosine controls inflammation and neurodegeneration by modulating dopamine signalling. Brain 142, 700–718. doi: 10.1093/brain/awy351
Michell-Robinson, M. A., Touil, H., Healy, L. M., Owen, D. R., Durafourt, B. A., Bar-Or, A., et al. (2015). Roles of microglia in brain development, tissue maintenance and repair. Brain 138, 1138–1159. doi: 10.1093/brain/awv066
Miron, V. E., Boyd, A., Zhao, J. W., Yuen, T. J., Ruckh, J. M., Shadrach, J. L., et al. (2013). M2 microglia and macrophages drive oligodendrocyte differentiation during CNS remyelination. Nat. Neurosci. 16, 1211–1218. doi: 10.1038/nn.3469
Mogi, M., Harada, M., Kondo, T., Riederer, P., Inagaki, H., Minami, M., et al. (1994a). Interleukin-1 beta, interleukin-6, epidermal growth factor and transforming growth factor-alpha are elevated in the brain from parkinsonian patients. Neurosci. Lett. 180, 147–150. doi: 10.1016/0304-3940(94)90508-8
Mogi, M., Harada, M., Riederer, P., Narabayashi, H., Fujita, K., and Nagatsu, T. (1994b). Tumor necrosis factor-alpha (TNF-alpha) increases both in the brain and in the cerebrospinal fluid from parkinsonian patients. Neurosci. Lett. 165, 208–210. doi: 10.1016/0304-3940(94)90746-3
Mogi, M., Harada, M., Narabayashi, H., Inagaki, H., Minami, M., and Nagatsu, T. (1996). Interleukin (IL)-1 beta, IL-2, IL-4, IL-6 and transforming growth factor-alpha levels are elevated in ventricular cerebrospinal fluid in juvenile parkinsonism and Parkinson’s disease. Neurosci. Lett. 211, 13–16. doi: 10.1016/0304-3940(96)12706-3
Moon, M., Kim, H. G., Hwang, L., Seo, J. H., Kim, S., Hwang, S., et al. (2009). Neuroprotective effect of ghrelin in the 1-methyl-4-phenyl-1,2,3,6-tetrahydropyridine mouse model of Parkinson’s disease by blocking microglial activation. Neurotox. Res. 15, 332–347. doi: 10.1007/s12640-009-9037-x
Morganti, J. M., Riparip, L. K., and Rosi, S. (2016). Call off the Dog(ma): M1/M2 polarization is concurrent following traumatic brain injury. PLoS One 11:e0148001. doi: 10.1371/journal.pone.0148001
Myeku, N., Clelland, C. L., Emrani, S., Kukushkin, N. V., Yu, W. H., Goldberg, A. L., et al. (2016). Tau-driven 26S proteasome impairment and cognitive dysfunction can be prevented early in disease by activating cAMP-PKA signaling. Nat. Med. 22, 46–53. doi: 10.1038/nm.4011
Nagatsu, T., Mogi, M., Ichinose, H., and Togari, A. (2000). Changes in cytokines and neurotrophins in Parkinson’s disease. J. Neural Transm. Suppl. 2000, 277–290. doi: 10.1007/978-3-7091-6301-6_19
Nalls, M. A., Plagnol, V., Hernandez, D. G., Sharma, M., Sheerin, U. M., Saad, M., et al. (2011). Imputation of sequence variants for identification of genetic risks for Parkinson’s disease: a meta-analysis of genome-wide association studies. Lancet 377, 641–649. doi: 10.1016/s0140-6736(10)62345-8
Navarrete, F., Garcia-Gutierrez, M. S., Aracil-Fernandez, A., Lanciego, J. L., and Manzanares, J. (2018). Cannabinoid CB1 and CB2 receptors, and monoacylglycerol lipase gene expression alterations in the basal ganglia of patients with parkinson’s disease. Neurotherapeutics 15, 459–469. doi: 10.1007/s13311-018-0603-x
Navarro, G., Borroto-Escuela, D., Angelats, E., Etayo, I., Reyes-Resina, I., Pulido-Salgado, M., et al. (2018). Receptor-heteromer mediated regulation of endocannabinoid signaling in activated microglia. Role of CB1 and CB2 receptors and relevance for Alzheimer’s disease and levodopa-induced dyskinesia. Brain Behav. Immun. 67, 139–151. doi: 10.1016/j.bbi.2017.08.015
Neumann, H., Kotter, M. R., and Franklin, R. J. (2009). Debris clearance by microglia: an essential link between degeneration and regeneration. Brain 132, 288–295. doi: 10.1093/brain/awn109
Nimmerjahn, A., Kirchhoff, F., and Helmchen, F. (2005). Resting microglial cells are highly dynamic surveillants of brain parenchyma in vivo. Science 308, 1314–1318. doi: 10.1126/science.1110647
Ninds Exploratory Trials in Parkinson Disease (Net-Pd) Fs-Zone Investigators. (2015). Pioglitazone in early Parkinson’s disease: a phase 2, multicentre, double-blind, randomised trial. Lancet Neurol. 14, 795–803. doi: 10.1016/s1474-4422(15)00144-1
Ojha, S., Javed, H., Azimullah, S., and Haque, M. E. (2016). beta-Caryophyllene, a phytocannabinoid attenuates oxidative stress, neuroinflammation, glial activation, and salvages dopaminergic neurons in a rat model of Parkinson disease. Mol. Cell. Biochem. 418, 59–70. doi: 10.1007/s11010-016-2733-y
Olanow, C. W., Savolainen, M., Chu, Y., Halliday, G. M., and Kordower, J. H. (2019). Temporal evolution of microglia and alpha-synuclein accumulation following foetal grafting in Parkinson’s disease. Brain 142, 1690–1700. doi: 10.1093/brain/awz104
Orihuela, R., McPherson, C. A., and Harry, G. J. (2016). Microglial M1/M2 polarization and metabolic states. Br. J. Pharmacol. 173, 649–665. doi: 10.1111/bph.13139
Ouchi, Y., Yoshikawa, E., Sekine, Y., Futatsubashi, M., Kanno, T., Ogusu, T., et al. (2005). Microglial activation and dopamine terminal loss in early Parkinson’s disease. Ann. Neurol. 57, 168–175. doi: 10.1002/ana.20338
Pabon, M. M., Bachstetter, A. D., Hudson, C. E., Gemma, C., and Bickford, P. C. (2011). CX3CL1 reduces neurotoxicity and microglial activation in a rat model of Parkinson’s disease. J. Neuroinflamm. 8:9. doi: 10.1186/1742-2094-8-9
Pais, T. F., Figueiredo, C., Peixoto, R., Braz, M. H., and Chatterjee, S. (2008). Necrotic neurons enhance microglial neurotoxicity through induction of glutaminase by a MyD88-dependent pathway. J. Neuroinflamm. 5:43. doi: 10.1186/1742-2094-5-43
Paolicelli, R. C., Bolasco, G., Pagani, F., Maggi, L., Scianni, M., Panzanelli, P., et al. (2011). Synaptic pruning by microglia is necessary for normal brain development. Science 333, 1456–1458. doi: 10.1126/science.1202529
Pietrzak, A., Wierzbicki, M., Wiktorska, M., and Brzezinska-Blaszczyk, E. (2011). Surface TLR2 and TLR4 expression on mature rat mast cells can be affected by some bacterial components and proinflammatory cytokines. Mediators Inflamm. 2011:427473. doi: 10.1155/2011/427473
Pifarre, P., Prado, J., Baltrons, M. A., Giralt, M., Gabarro, P., Feinstein, D. L., et al. (2011). Sildenafil (Viagra) ameliorates clinical symptoms and neuropathology in a mouse model of multiple sclerosis. Acta Neuropathol. 121, 499–508. doi: 10.1007/s00401-010-0795-6
Pisanu, A., Lecca, D., Mulas, G., Wardas, J., Simbula, G., Spiga, S., et al. (2014). Dynamic changes in pro- and anti-inflammatory cytokines in microglia after PPAR-γ agonist neuroprotective treatment in the MPTPp mouse model of progressive Parkinson’s disease. Neurobiol. Dis. 71, 280–291. doi: 10.1016/j.nbd.2014.08.011
Pluvinage, J. V., Haney, M. S., Smith, B. A. H., Sun, J., Iram, T., Bonanno, L., et al. (2019). CD22 blockade restores homeostatic microglial phagocytosis in ageing brains. Nature 568, 187–192. doi: 10.1038/s41586-019-1088-4
Poly, T. N., Islam, M. M. R., Yang, H. C., and Li, Y. J. (2019). Non-steroidal anti-inflammatory drugs and risk of Parkinson’s disease in the elderly population: a meta-analysis. Eur. J. Clin. Pharmacol. 75, 99–108. doi: 10.1007/s00228-018-2561-y
Porro, C., Panaro, M. A., Lofrumento, D. D., Hasalla, E., and Trotta, T. (2019). The multiple roles of exosomes in Parkinson’s disease: an overview. Immunopharmacol. Immunotoxicol. 41, 469–476. doi: 10.1080/08923973.2019.1650371
Prots, I., Grosch, J., Brazdis, R. M., Simmnacher, K., Veber, V., Havlicek, S., et al. (2018). alpha-Synuclein oligomers induce early axonal dysfunction in human iPSC-based models of synucleinopathies. Proc. Natl. Acad. Sci. U.S.A. 115, 7813–7818. doi: 10.1073/pnas.1713129115
Rabal, O., Sanchez-Arias, J. A., Cuadrado-Tejedor, M., de Miguel, I., Perez-Gonzalez, M., Garcia-Barroso, C., et al. (2018). Design, synthesis, biological evaluation and in vivo testing of dual phosphodiesterase 5 (PDE5) and histone deacetylase 6 (HDAC6)-selective inhibitors for the treatment of Alzheimer’s disease. Eur. J. Med. Chem. 150, 506–524. doi: 10.1016/j.ejmech.2018.03.005
Ramazani, E., Tayarani-Najaran, Z., and Fereidoni, M. (2019). Celecoxib, indomethacin, and ibuprofen prevent 6-hydroxydopamine-induced PC12 cell death through the inhibition of NFkappaB and SAPK/JNK pathways. Iran. J. Basic Med. Sci. 22, 477–484. doi: 10.22038/ijbms.2019.34011.8091
Ransohoff, R. M. (2016). A polarizing question: do M1 and M2 microglia exist? Nat. Neurosci. 19, 987–991. doi: 10.1038/nn.4338
Ransohoff, R. M., and Perry, V. H. (2009). Microglial physiology: unique stimuli, specialized responses. Annu. Rev. Immunol. 27, 119–145. doi: 10.1146/annurev.immunol.021908.132528
Rayaprolu, S., Mullen, B., Baker, M., Lynch, T., Finger, E., Seeley, W. W., et al. (2013). TREM2 in neurodegeneration: evidence for association of the p.R47H variant with frontotemporal dementia and Parkinson’s disease. Mol. Neurodegener. 8:19. doi: 10.1186/1750-1326-8-19
Rees, K., Stowe, R., Patel, S., Ives, N., Breen, K., Clarke, C. E., et al. (2011). Non-steroidal anti-inflammatory drugs as disease-modifying agents for Parkinson’s disease: evidence from observational studies. Cochrane Database Syst. Rev. 87:CD008454. doi: 10.1002/14651858.CD008454.pub2
Ren, M., Guo, Y., Wei, X., Yan, S., Qin, Y., Zhang, X., et al. (2018). TREM2 overexpression attenuates neuroinflammation and protects dopaminergic neurons in experimental models of Parkinson’s disease. Exp. Neurol. 302, 205–213. doi: 10.1016/j.expneurol.2018.01.016
Riederer, P., Sofic, E., Rausch, W. D., Schmidt, B., Reynolds, G. P., Jellinger, K., et al. (1989). Transition metals, ferritin, glutathione, and ascorbic acid in parkinsonian brains. J. Neurochem. 52, 515–520. doi: 10.1111/j.1471-4159.1989.tb09150.x
Rothhammer, V., Borucki, D. M., Tjon, E. C., Takenaka, M. C., Chao, C. C., Ardura-Fabregat, A., et al. (2018). Microglial control of astrocytes in response to microbial metabolites. Nature 557, 724–728. doi: 10.1038/s41586-018-0119-x
Ruano, D., Revilla, E., Gavilan, M. P., Vizuete, M. L., Pintado, C., Vitorica, J., et al. (2006). Role of p38 and inducible nitric oxide synthase in the in vivo dopaminergic cells’ degeneration induced by inflammatory processes after lipopolysaccharide injection. Neuroscience 140, 1157–1168. doi: 10.1016/j.neuroscience.2006.02.073
Sacino, A. N., Brooks, M., McKinney, A. B., Thomas, M. A., Shaw, G., Golde, T. E., et al. (2014). Brain injection of alpha-synuclein induces multiple proteinopathies, gliosis, and a neuronal injury marker. J. Neurosci. 34, 12368–12378. doi: 10.1523/jneurosci.2102-14.2014
Samii, A., Etminan, M., Wiens, M. O., and Jafari, S. (2009). NSAID use and the risk of Parkinson’s disease: systematic review and meta-analysis of observational studies. Drugs Aging 26, 769–779. doi: 10.2165/11316780-000000000-00000
Sasso, V., Bisicchia, E., Latini, L., Ghiglieri, V., Cacace, F., Carola, V., et al. (2016). Repetitive transcranial magnetic stimulation reduces remote apoptotic cell death and inflammation after focal brain injury. J. Neuroinflamm. 13:150. doi: 10.1186/s12974-016-0616-5
Saunders, J. A., Estes, K. A., Kosloski, L. M., Allen, H. E., Dempsey, K. M., Torres-Russotto, D. R., et al. (2012). CD4+ regulatory and effector/memory T cell subsets profile motor dysfunction in Parkinson’s disease. J. Neuroimmune Pharmacol. 7, 927–938. doi: 10.1007/s11481-012-9402-z
Schafer, D. P., Lehrman, E. K., Kautzman, A. G., Koyama, R., Mardinly, A. R., Yamasaki, R., et al. (2012). Microglia sculpt postnatal neural circuits in an activity and complement-dependent manner. Neuron 74, 691–705. doi: 10.1016/j.neuron.2012.03.026
Schwenkgrub, J., Joniec-Maciejak, I., Sznejder-Pacholek, A., Wawer, A., Ciesielska, A., Bankiewicz, K., et al. (2013). Effect of human interleukin-10 on the expression of nitric oxide synthases in the MPTP-based model of Parkinson’s disease. Pharmacol. Rep. 65, 44–49. doi: 10.1016/s1734-1140(13)70962-9
Schwenkgrub, J., Zaremba, M., Joniec-Maciejak, I., Cudna, A., Mirowska-Guzel, D., and Kurkowska-Jastrzebska, I. (2017). The phosphodiesterase inhibitor, ibudilast, attenuates neuroinflammation in the MPTP model of Parkinson’s disease. PLoS One 12:e0182019. doi: 10.1371/journal.pone.0182019
Sharma, N., and Nehru, B. (2016). Apocyanin, a microglial NADPH oxidase inhibitor prevents dopaminergic neuronal degeneration in lipopolysaccharide-induced parkinson’s disease model. Mol. Neurobiol. 53, 3326–3337. doi: 10.1007/s12035-015-9267-2
Sheridan, G. K., and Murphy, K. J. (2013). Neuron-glia crosstalk in health and disease: fractalkine and CX3CR1 take centre stage. Open Biol. 3, 130181. doi: 10.1098/rsob.130181
Sierra, A., Encinas, J. M., Deudero, J. J., Chancey, J. H., Enikolopov, G., Overstreet-Wadiche, L. S., et al. (2010). Microglia shape adult hippocampal neurogenesis through apoptosis-coupled phagocytosis. Cell Stem Cell. 7, 483–495. doi: 10.1016/j.stem.2010.08.014
Skaper, S. D., Facci, L., Zusso, M., and Giusti, P. (2017). Neuroinflammation. Mast cells, and glia: dangerous liaisons. Neuroscientist 23, 478–498. doi: 10.1177/1073858416687249
Skaper, S. D., Facci, L., Zusso, M., and Giusti, P. (2018). An inflammation-centric view of neurological disease: beyond the neuron. Front. Cell. Neurosci. 12:72. doi: 10.3389/fncel.2018.00072
Sofic, E., Riederer, P., Heinsen, H., Beckmann, H., Reynolds, G. P., Hebenstreit, G., et al. (1988). Increased iron (III) and total iron content in post mortem substantia nigra of parkinsonian brain. J. Neural Transm. 74, 199–205. doi: 10.1007/bf01244786
Song, G. J., Nam, Y., Jo, M., Jung, M., Koo, J. Y., Cho, W., et al. (2016). A novel small-molecule agonist of PPAR-gamma potentiates an anti-inflammatory M2 glial phenotype. Neuropharmacology 109, 159–169. doi: 10.1016/j.neuropharm.2016.06.009
Song, G. J., and Suk, K. (2017). Pharmacological modulation of functional phenotypes of microglia in neurodegenerative diseases. Front. Aging Neurosci. 9:139. doi: 10.3389/fnagi.2017.00139
Song, N., Wang, J., Jiang, H., and Xie, J. (2018). Astroglial and microglial contributions to iron metabolism disturbance in Parkinson’s disease. Biochim. Biophys. Acta Mol. Basis Dis. 1864, 967–973. doi: 10.1016/j.bbadis.2018.01.008
Stefanova, N., Fellner, L., Reindl, M., Masliah, E., Poewe, W., and Wenning, G. K. (2011). Toll-like receptor 4 promotes alpha-synuclein clearance and survival of nigral dopaminergic neurons. Am. J. Pathol. 179, 954–963. doi: 10.1016/j.ajpath.2011.04.013
Stein, M., Keshav, S., Harris, N., and Gordon, S. (1992). Interleukin 4 potently enhances murine macrophage mannose receptor activity: a marker of alternative immunologic macrophage activation. J. Exp. Med. 176, 287–292. doi: 10.1084/jem.176.1.287
Su, X., Federoff, H. J., and Maguire-Zeiss, K. A. (2009). Mutant alpha-synuclein overexpression mediates early proinflammatory activity. Neurotox. Res. 16, 238–254. doi: 10.1007/s12640-009-9053-x
Su, X., Maguire-Zeiss, K. A., Giuliano, R., Prifti, L., Venkatesh, K., and Federoff, H. J. (2008). Synuclein activates microglia in a model of Parkinson’s disease. Neurobiol. Aging 29, 1690–1701. doi: 10.1016/j.neurobiolaging.2007.04.006
Subramaniam, S. R., and Federoff, H. J. (2017). Targeting microglial activation states as a therapeutic avenue in Parkinson’s Disease. Front. Aging Neurosci. 9:176. doi: 10.3389/fnagi.2017.00176
Swanson, C. R., Joers, V., Bondarenko, V., Brunner, K., Simmons, H. A., Ziegler, T. E., et al. (2011). The PPAR-gamma agonist pioglitazone modulates inflammation and induces neuroprotection in parkinsonian monkeys. J. Neuroinflamm. 8:91. doi: 10.1186/1742-2094-8-91
Swiatkiewicz, M., Zaremba, M., Joniec, I., Czlonkowski, A., and Kurkowska-Jastrzebska, I. (2013). Potential neuroprotective effect of ibuprofen, insights from the mice model of Parkinson’s disease. Pharmacol. Rep. 65, 1227–1236. doi: 10.1016/s1734-1140(13)71480-4
Symons, A., Beinke, S., and Ley, S. C. (2006). MAP kinase kinase kinases and innate immunity. Trends Immunol. 27, 40–48. doi: 10.1016/j.it.2005.11.007
Szalay, G., Martinecz, B., Lenart, N., Kornyei, Z., Orsolits, B., Judak, L., et al. (2016). Microglia protect against brain injury and their selective elimination dysregulates neuronal network activity after stroke. Nat. Commun. 7:11499. doi: 10.1038/ncomms11499
Szulzewsky, F., Pelz, A., Feng, X., Synowitz, M., Markovic, D., Langmann, T., et al. (2015). Glioma-associated microglia/macrophages display an expression profile different from M1 and M2 polarization and highly express Gpnmb and Spp1. PLoS One 10:e0116644. doi: 10.1371/journal.pone.0116644
Takahashi, K., Prinz, M., Stagi, M., Chechneva, O., and Neumann, H. (2007). TREM2-transduced myeloid precursors mediate nervous tissue debris clearance and facilitate recovery in an animal model of multiple sclerosis. PLoS Med. 4:e124. doi: 10.1371/journal.pmed.0040124
Takahashi, K., Rochford, C. D., and Neumann, H. (2005). Clearance of apoptotic neurons without inflammation by microglial triggering receptor expressed on myeloid cells-2. J. Exp. Med. 201, 647–657. doi: 10.1084/jem.20041611
Takeda, K., and Akira, S. (2004). TLR signaling pathways. Semin. Immunol. 16, 3–9. doi: 10.1016/j.smim.2003.10.003
Tansey, M. G., and Goldberg, M. S. (2010). Neuroinflammation in Parkinson’s disease: its role in neuronal death and implications for therapeutic intervention. Neurobiol. Dis. 37, 510–518. doi: 10.1016/j.nbd.2009.11.004
Taylor, J. M., Main, B. S., and Crack, P. J. (2013). Neuroinflammation and oxidative stress: co-conspirators in the pathology of Parkinson’s disease. Neurochem. Int. 62, 803–819. doi: 10.1016/j.neuint.2012.12.016
Theillet, F. X., Binolfi, A., Bekei, B., Martorana, A., Rose, H. M., Stuiver, M., et al. (2016). Structural disorder of monomeric alpha-synuclein persists in mammalian cells. Nature 530, 45–50. doi: 10.1038/nature16531
Theodore, S., Cao, S., McLean, P. J., and Standaert, D. G. (2008). Targeted overexpression of human alpha-synuclein triggers microglial activation and an adaptive immune response in a mouse model of Parkinson disease. J. Neuropathol. Exp. Neurol. 67, 1149–1158. doi: 10.1097/NEN.0b013e31818e5e99
Toshchakov, V., Jones, B. W., Perera, P. Y., Thomas, K., Cody, M. J., Zhang, S., et al. (2002). TLR4, but not TLR2, mediates IFN-beta-induced STAT1alpha/beta-dependent gene expression in macrophages. Nat. Immunol. 3, 392–398. doi: 10.1038/ni774
Ueno, M., Fujita, Y., Tanaka, T., Nakamura, Y., Kikuta, J., Ishii, M., et al. (2013). Layer V cortical neurons require microglial support for survival during postnatal development. Nat. Neurosci. 16, 543–551. doi: 10.1038/nn.3358
Urrutia, P., Aguirre, P., Esparza, A., Tapia, V., Mena, N. P., Arredondo, M., et al. (2013). Inflammation alters the expression of DMT1, FPN1 and hepcidin, and it causes iron accumulation in central nervous system cells. J. Neurochem. 126, 541–549. doi: 10.1111/jnc.12244
Valera, E., Mante, M., Anderson, S., Rockenstein, E., and Masliah, E. (2015). Lenalidomide reduces microglial activation and behavioral deficits in a transgenic model of Parkinson’s disease. J. Neuroinflamm. 12:93. doi: 10.1186/s12974-015-0320-x
Vay, S. U., Flitsch, L. J., Rabenstein, M., Rogall, R., Blaschke, S., Kleinhaus, J., et al. (2018). The plasticity of primary microglia and their multifaceted effects on endogenous neural stem cells in vitro and in vivo. J. Neuroinflamm. 15:226. doi: 10.1186/s12974-018-1261-y
Verma, R., and Kim, J. Y. (2016). 1,25-Dihydroxyvitamin D3 Facilitates M2 Polarization and Upregulates TLR10 Expression on Human Microglial Cells. Neuroimmunomodulation 23, 75–80. doi: 10.1159/000444300
Wahner, A. D., Bronstein, J. M., Bordelon, Y. M., and Ritz, B. (2007). Nonsteroidal anti-inflammatory drugs may protect against Parkinson disease. Neurology 69, 1836–1842. doi: 10.1212/01.wnl.0000279519.99344.ad
Wake, H., Moorhouse, A. J., Jinno, S., Kohsaka, S., and Nabekura, J. (2009). Resting microglia directly monitor the functional state of synapses in vivo and determine the fate of ischemic terminals. J. Neurosci. 29, 3974–3980. doi: 10.1523/jneurosci.4363-08.2009
Wakselman, S., Bechade, C., Roumier, A., Bernard, D., Triller, A., and Bessis, A. (2008). Developmental neuronal death in hippocampus requires the microglial CD11b integrin and DAP12 immunoreceptor. J. Neurosci. 28, 8138–8143. doi: 10.1523/jneurosci.1006-08.2008
Wang, C., Fan, G., Xu, K., and Wang, S. (2013). Quantitative assessment of iron deposition in the midbrain using 3D-enhanced T2 star weighted angiography (ESWAN): a preliminary cross-sectional study of 20 Parkinson’s disease patients. Magn. Reson. Imaging 31, 1068–1073. doi: 10.1016/j.mri.2013.04.015
Wang, J., Song, N., Jiang, H., Wang, J., and Xie, J. (2013). Pro-inflammatory cytokines modulate iron regulatory protein 1 expression and iron transportation through reactive oxygen/nitrogen species production in ventral mesencephalic neurons. Biochim. Biophys. Acta 1832, 618–625. doi: 10.1016/j.bbadis.2013.01.021
Wang, Q., Chu, C. H., Oyarzabal, E., Jiang, L., Chen, S. H., Wilson, B., et al. (2014). Subpicomolar diphenyleneiodonium inhibits microglial NADPH oxidase with high specificity and shows great potential as a therapeutic agent for neurodegenerative diseases. Glia 62, 2034–2043. doi: 10.1002/glia.22724
Wang, S., Chu, C. H., Stewart, T., Ginghina, C., Wang, Y., Nie, H., et al. (2015a). alpha-Synuclein, a chemoattractant, directs microglial migration via H2O2-dependent Lyn phosphorylation. Proc. Natl. Acad. Sci. U.S.A. 112, E1926–E1935. doi: 10.1073/pnas.1417883112
Wang, S., Jing, H., Yang, H., Liu, Z., Guo, H., Chai, L., et al. (2015b). Tanshinone I selectively suppresses pro-inflammatory genes expression in activated microglia and prevents nigrostriatal dopaminergic neurodegeneration in a mouse model of Parkinson’s disease. J. Ethnopharmacol. 164, 247–255. doi: 10.1016/j.jep.2015.01.042
Wang, Q., Liu, Y., and Zhou, J. (2015c). Neuroinflammation in Parkinson’s disease and its potential as therapeutic target. Transl. Neurodegener. 4:19. doi: 10.1186/s40035-015-0042-0
Wang, Q., Qian, L., Chen, S. H., Chu, C. H., Wilson, B., Oyarzabal, E., et al. (2015d). Post-treatment with an ultra-low dose of NADPH oxidase inhibitor diphenyleneiodonium attenuates disease progression in multiple Parkinson’s disease models. Brain 138, 1247–1262. doi: 10.1093/brain/awv034
Wang, X. J., Zhang, S., Yan, Z. Q., Zhao, Y. X., Zhou, H. Y., Wang, Y., et al. (2011). Impaired CD200-CD200R-mediated microglia silencing enhances midbrain dopaminergic neurodegeneration: roles of aging, superoxide, NADPH oxidase, and p38 MAPK. Free Radic. Biol. Med. 50, 1094–1106. doi: 10.1016/j.freeradbiomed.2011.01.032
Ward, R. J., Zucca, F. A., Duyn, J. H., Crichton, R. R., and Zecca, L. (2014). The role of iron in brain ageing and neurodegenerative disorders. Lancet Neurol. 13, 1045–1060. doi: 10.1016/s1474-4422(14)70117-6
Whitton, P. S. (2007). Inflammation as a causative factor in the aetiology of Parkinson’s disease. Br. J. Pharmacol. 150, 963–976. doi: 10.1038/sj.bjp.0707167
Wolf, S. A., Boddeke, H. W., and Kettenmann, H. (2017). Microglia in physiology and disease. Annu. Rev. Physiol. 79, 619–643. doi: 10.1146/annurev-physiol-022516-034406
Wu, D. C., Jackson-Lewis, V., Vila, M., Tieu, K., Teismann, P., Vadseth, C., et al. (2002). Blockade of microglial activation is neuroprotective in the 1-methyl-4-phenyl-1,2,3,6-tetrahydropyridine mouse model of Parkinson disease. J. Neurosci. 22, 1763–1771. doi: 10.1523/jneurosci.22-05-01763.2002
Xia, Y., Zhang, G., Han, C., Ma, K., Guo, X., Wan, F., et al. (2019). Microglia as modulators of exosomal alpha-synuclein transmission. Cell Death Dis. 10:174. doi: 10.1038/s41419-019-1404-9
Xu, Y., Zhu, N., Xu, W., Ye, H., Liu, K., Wu, F., et al. (2018). Inhibition of phosphodiesterase-4 reverses abeta-induced memory impairment by regulation of HPA axis related cAMP signaling. Front. Aging Neurosci. 10:204. doi: 10.3389/fnagi.2018.00204
Yang, W., Chen, Y. H., Liu, H., and Qu, H. D. (2015). Neuroprotective effects of piperine on the 1-methyl-4-phenyl-1,2,3,6-tetrahydropyridine-induced Parkinson’s disease mouse model. Int. J. Mol. Med. 36, 1369–1376. doi: 10.3892/ijmm.2015.2356
Yao, K., and Zhao, Y. F. (2018). Aging modulates microglia phenotypes in neuroinflammation of MPTP-PD mice. Exp. Gerontol. 111, 86–93. doi: 10.1016/j.exger.2018.07.010
Zecca, L., Berg, D., Arzberger, T., Ruprecht, P., Rausch, W. D., Musicco, M., et al. (2005). In vivo detection of iron and neuromelanin by transcranial sonography: a new approach for early detection of substantia nigra damage. Mov. Disord. 20, 1278–1285. doi: 10.1002/mds.20550
Zeng, K. W., Zhao, M. B., Ma, Z. Z., Jiang, Y., and Tu, P. F. (2012). Protosappanin A inhibits oxidative and nitrative stress via interfering the interaction of transmembrane protein CD14 with Toll-like receptor-4 in lipopolysaccharide-induced BV-2 microglia. Int. Immunopharmacol. 14, 558–569. doi: 10.1016/j.intimp.2012.09.004
Zhang, F., Shi, J. S., Zhou, H., Wilson, B., Hong, J. S., and Gao, H. M. (2010). Resveratrol protects dopamine neurons against lipopolysaccharide-induced neurotoxicity through its anti-inflammatory actions. Mol. Pharmacol. 78, 466–477. doi: 10.1124/mol.110.064535
Zhang, J., Zhang, Y., Wang, J., Cai, P., Luo, C., Qian, Z., et al. (2010). Characterizing iron deposition in Parkinson’s disease using susceptibility-weighted imaging: an in vivo MR study. Brain Res. 1330, 124–130. doi: 10.1016/j.brainres.2010.03.036
Zhang, Q. S., Heng, Y., Yuan, Y. H., and Chen, N. H. (2017). Pathological alpha-synuclein exacerbates the progression of Parkinson’s disease through microglial activation. Toxicol. Lett. 265, 30–37. doi: 10.1016/j.toxlet.2016.11.002
Zhang, S., Wang, X. J., Tian, L. P., Pan, J., Lu, G. Q., Zhang, Y. J., et al. (2011). CD200-CD200R dysfunction exacerbates microglial activation and dopaminergic neurodegeneration in a rat model of Parkinson’s disease. J. Neuroinflamm. 8:154. doi: 10.1186/1742-2094-8-154
Zhang, W., Wang, T., Pei, Z., Miller, D. S., Wu, X., Block, M. L., et al. (2005). Aggregated alpha-synuclein activates microglia: a process leading to disease progression in Parkinson’s disease. FASEB J. 19, 533–542. doi: 10.1096/fj.04-2751com
Zhang, X., Dong, H., Li, N., Zhang, S., Sun, J., Zhang, S., et al. (2016). Activated brain mast cells contribute to postoperative cognitive dysfunction by evoking microglia activation and neuronal apoptosis. J. Neuroinflamm. 13:127. doi: 10.1186/s12974-016-0592-9
Zhang, Y., Feng, S., Nie, K., Li, Y., Gao, Y., Gan, R., et al. (2018). TREM2 modulates microglia phenotypes in the neuroinflammation of Parkinson’s disease. Biochem. Biophys. Res. Commun. 499, 797–802. doi: 10.1016/j.bbrc.2018.03.226
Zhao, S., Yang, J., Wang, L., Peng, S., Yin, J., Jia, L., et al. (2016). NF-kappab upregulates type 5 phosphodiesterase in N9 microglial cells: inhibition by sildenafil and yonkenafil. Mol. Neurobiol. 53, 2647–2658. doi: 10.1007/s12035-015-9293-0
Zhou, P., Homberg, J. R., Fang, Q., Wang, J., Li, W., Meng, X., et al. (2019). Histamine-4 receptor antagonist JNJ7777120 inhibits pro-inflammatory microglia and prevents the progression of Parkinson-like pathology and behaviour in a rat model. Brain Behav. Immun. 76, 61–73. doi: 10.1016/j.bbi.2018.11.006
Zhou, T. T., Zu, G., Wang, X., Zhang, X. G., Li, S., Liang, Z. H., et al. (2015). Immunomodulatory and neuroprotective effects of ginsenoside Rg1 in the MPTP(1-methyl-4-phenyl-1,2,3,6-tetrahydropyridine) -induced mouse model of Parkinson’s disease. Int. Immunopharmacol. 29, 334–343. doi: 10.1016/j.intimp.2015.10.032
Keywords: Parkinson’s disease, microglia, neuroinflammation, microglial activation, polarization
Citation: Liu C-Y, Wang X, Liu C and Zhang H-L (2019) Pharmacological Targeting of Microglial Activation: New Therapeutic Approach. Front. Cell. Neurosci. 13:514. doi: 10.3389/fncel.2019.00514
Received: 21 August 2019; Accepted: 31 October 2019;
Published: 19 November 2019.
Edited by:
Victoria Campos-Peña, National Institute of Neurology and Neurosurgery (INNN), MexicoReviewed by:
Lorenzo Di Cesare Mannelli, University of Florence, ItalyVeronica Ghiglieri, University of Perugia, Italy
Copyright © 2019 Liu, Wang, Liu and Zhang. This is an open-access article distributed under the terms of the Creative Commons Attribution License (CC BY). The use, distribution or reproduction in other forums is permitted, provided the original author(s) and the copyright owner(s) are credited and that the original publication in this journal is cited, in accordance with accepted academic practice. No use, distribution or reproduction is permitted which does not comply with these terms.
*Correspondence: Cai-Yun Liu, Y2FpeXVuXzA3NzBAMTYzLmNvbQ==; Hong-Liang Zhang, ZHJ6aGxAaG90bWFpbC5jb20=