- 1Department of Neuromuscular Diseases, UCL Queen Square Institute of Neurology, University College London, London, United Kingdom
- 2UK Dementia Research Institute, University College London, London, United Kingdom
- 3Discoveries Centre for Regenerative and Precision Medicine, University College London Campus, London, United Kingdom
Dominant, missense mutations in the widely and constitutively expressed GARS1 gene cause peripheral neuropathy that usually begins in adolescence and principally impacts the upper limbs. Caused by a toxic gain-of-function in the encoded glycyl-tRNA synthetase (GlyRS) enzyme, the neuropathology appears to be independent of the canonical role of GlyRS in aminoacylation. Patients display progressive, life-long weakness and wasting of muscles in hands followed by feet, with frequently associated deficits in sensation. When dysfunction is observed in motor and sensory nerves, there is a diagnosis of Charcot-Marie-Tooth disease type 2D (CMT2D), or distal hereditary motor neuropathy type V if the symptoms are purely motor. The cause of this varied sensory involvement remains unresolved, as are the pathomechanisms underlying the selective neurodegeneration characteristic of the disease. We have previously identified in CMT2D mice that neuropathy-causing Gars mutations perturb sensory neuron fate and permit mutant GlyRS to aberrantly interact with neurotrophin receptors (Trks). Here, we extend this work by interrogating further the anatomy and function of the CMT2D sensory nervous system in mutant Gars mice, obtaining several key results: (1) sensory pathology is restricted to neurons innervating the hindlimbs; (2) perturbation of sensory development is not common to all mouse models of neuromuscular disease; (3) in vitro axonal transport of signaling endosomes is not impaired in afferent neurons of all CMT2D mouse models; and (4) Gars expression is selectively elevated in a subset of sensory neurons and linked to sensory developmental defects. These findings highlight the importance of comparative neurological assessment in mouse models of disease and shed light on key proposed neuropathogenic mechanisms in GARS1-linked neuropathy.
Introduction
Characterized by distal dysfunction of motor and sensory nerves, Charcot-Marie-Tooth disease (CMT) is a hereditary peripheral neuropathy that usually presents in adolescence and affects 1 in 2,500–5,000 people, which makes it the most common inherited neuromuscular condition (Pipis et al., 2019). Classically, the disease can be categorized as CMT1, typified by demyelination and thus reduced nerve conduction velocity, CMT2 in which there is axon loss but no diminished nerve conduction velocity, and intermediate CMT that shares features of both CMT1 and CMT2 (Reilly et al., 2011). Consistent with length-dependency, patients display slowly progressive, bilateral muscle weakness and sensory deficits predominantly in the extremities, typically starting in the feet. CMT is on a phenotypic spectrum with distal hereditary motor neuropathy (dHMN) and hereditary sensory/autonomic neuropathy (HSN/HSAN), which have mainly motor and sensory/autonomic involvement, respectively, and can be caused by mutations in the same gene (Pisciotta and Shy, 2018).
To date, mutations in more than 100 different genetic loci have been linked to CMT (Rossor et al., 2013; Pipis et al., 2019). Many causative CMT1 genes are selectively expressed by myelinating Schwann cells or have myelin-specific functions, providing mechanistic justification for pathology. However, CMT2-associated genes are involved in a variety of processes critical to general cell viability (e.g., mitochondrial dynamics, endolysosomal sorting, ubiquitination, heat shock response), and the pathomechanisms underlying neuronal selectivity remain relatively obscure. Fitting with this, the widely and constitutively active aminoacyl-tRNA synthetase (ARS) enzymes, which covalently bind specific amino acids to their partner tRNAs for protein translation (Ibba and Soll, 2000), represent the largest protein family implicated in CMT etiology. To date, dominant mutations in six ARS-encoding genes (GARS1, YARS1, AARS1, HARS1, WARS1, and MARS1) have been linked to CMT with varying degrees of evidence for pathogenicity (Wei et al., 2019).
Encoding glycyl-tRNA synthetase (GlyRS), which charges glycine, GARS1 is the first and best-studied ARS gene linked to CMT (designated CMT type 2D, CMT2D, OMIM: 601472; Antonellis et al., 2003). Uncharacteristically and contravening length-dependency, CMT2D patients frequently display upper limb predominance with weakness beginning in dorsal interosseus muscles of the hand and progressing to involve lower limbs in about only half of patients (Antonellis et al., 2018; Sivakumar et al., 2005). Genetic studies across yeast, Drosophila melanogaster and mouse models for CMT2D indicate that, although neuropathy-causing mutations can abolish canonical GlyRS function and loss-of-function pathogenesis hypotheses prevail (Meyer-Schuman and Antonellis, 2017), the disease is most likely caused by a toxic gain-of-function (Boczonadi et al., 2018; Wei et al., 2019). Commensurate with mutant protein toxicity, wild-type GARS1 overexpression in CMT2D mice has no discernible rescue effect on neuromuscular pathologies, while the increased dosage of disease-causing Gars alleles causes more severe neuropathy (Motley et al., 2011). Moreover, all assessed GlyRS mutants possess a similar conformational opening that excavates neomorphic surfaces usually buried within the structure of the wild-type enzyme (He et al., 2011, 2015). Given that GlyRS is secreted from several different cell types (Park et al., 2012, 2018; Grice et al., 2015; He et al., 2015), these uncovered protein regions can mediate aberrant deleterious interactions both inside and outside the cell (He et al., 2015; Sleigh et al., 2017a; Mo et al., 2018), likely accounting for non-cell-autonomous aspects of pathology (Grice et al., 2015, 2018). While some of these mis-interactions are with neuronally-enriched proteins, the pathomechanisms underlying neuronal selectivity in CMT2D remain unresolved. Nevertheless, recent studies indicate that impairments in the processes of axonal transport (Benoy et al., 2018; Mo et al., 2018) and protein translation (Niehues et al., 2015) may be playing a causative role.
Several different mouse models are available for CMT2D (Seburn et al., 2006; Achilli et al., 2009; Morelli et al., 2019), which have mutations in endogenous mouse Gars, causing phenotypes akin to human neuropathy. These mice display loss of lower motor neuron connectivity and disturbed neurotransmission at the neuromuscular junction (NMJ), causing muscle weakness and motor function deficits (Sleigh et al., 2014a; Spaulding et al., 2016). Furthermore, there appears to be a pre-natal perturbation of sensory neuron fate in dorsal root ganglia (DRG), such that CMT2D mice have more nociceptive (noxious stimulus-sensing) neurons and fewer mechanosensitive (touch-sensing) and proprioceptive (body position-sensing) neurons (Sleigh et al., 2017a). Perhaps causing this and providing a rationale for neuronal selectivity, mutant GlyRS mis-interacts with the extracellular region of tropomyosin receptor kinase (Trk) receptors. These largely neuron-specific transmembrane proteins mediate the development and survival of sensory neurons by binding with differential affinity to neurotrophins secreted from distal target cells/tissues (e.g., Schwann cells and muscles; Huang and Reichardt, 2003). Activated neurotrophin-Trk receptor complexes are internalized in the periphery, sorted into signaling endosomes, and then retrogradely transported along microtubules to neuronal somas, where they elicit transcriptional events fundamental to nerve survival (Villarroel-Campos et al., 2018).
The earliest manifestation of CMT2D in many individuals is transient cramping and pain in the hands upon cold exposure (Antonellis et al., 2018). In addition to muscle weakness, this is followed by compromised reflexes and loss of sensation to vibration, touch, temperature, and pin-prick (Sivakumar et al., 2005). Some of these symptoms are reflected in the phenotypes observed in Gars-neuropathy mice, highlighting their potential for studying sensory pathomechanisms (Sleigh et al., 2017a).
However, the motor symptoms of CMT2D patients are the focus of the clinical investigation, given their relative severity. Moreover, GARS1 neuropathy patients can show little to no sensory involvement and are thus diagnosed with dHMN type V (OMIM 600794; Antonellis et al., 2003). The pathological impact of mutant GlyRS on the sensory nervous system is therefore relatively under-studied and requires further attention if we are to elucidate the cause of its varied involvement in GARS1-linked neuropathy. Here, we have thus extended our sensory analyses in CMT2D mice to better understand the importance of anatomical location to pathology and to assess the relevance of some proposed disease mechanisms in afferent nerves.
Materials and Methods
Animals
All experiments were carried out following the guidelines of the UCL Queen Square Institute of Neurology Genetic Manipulation and Ethics Committees and following the European Community Council Directive of 24 November 1986 (86/609/EEC). GarsC201R/+ (RRID:MGI 3849420) and SOD1G93A (RRID:IMSR_JAX 002726) mouse handling and experiments were carried out under license from the UK Home Office following the Animals (Scientific Procedures) Act 1986 and were approved by the UCL Queen Square Institute of Neurology Ethical Review Committee. GarsNmf249/+ (RRID:MGI 5308205) tissue was provided by Drs. Emily Spaulding and Robert Burgess (The Jackson Laboratory, Bar Harbor, ME, USA), as previously described (Sleigh et al., 2017a). GarsC201R/+ and GarsNmf249/+ mice were maintained as heterozygote breeding pairs on a C57BL/6J background and genotyped as previously described (Seburn et al., 2006; Achilli et al., 2009). Both males and females were used in the analyses of mutant Gars alleles, as no clear sex-specific differences have yet been observed or reported. Genotyped using standard procedures (Gurney et al., 1994), transgenic male mice heterozygous for the mutant human SOD1 gene (G93A) on a mixed C57BL/6-SJL background [B6SJLTg (SOD1*G93A)1Gur/J] and wild-type male littermate controls were used for the SOD1G93A experiments. GarsC201R/+ mice sacrificed for 1-month and 3-month timepoints were 29–37 and 89–97 days old, respectively. The GarsNmf249/+ mice used at 1 month were P31–32, while SOD1G93A mice were P30–31 and P100–101.
Tissue Dissection
DRG were extracted from either non-perfused or saline-perfused mice as previously described (Sleigh et al., 2016, 2020b). The most caudal pair of floating ribs and the large size of lumbar level 4 (L4) DRG and associated axon bundles were used as markers to consistently and accurately define the spinal level. The forepaws of embryonic day 13.5 (E13.5) embryos were removed between the wrist and elbow joints, as outlined elsewhere (Wickramasinghe et al., 2008).
Tissue Immunofluorescence
Dissected DRG and E13.5 forepaws were processed for immunofluorescence and analyzed as previously described in detail (Sleigh et al., 2017a). The following antibodies were used: rabbit anti-GlyRS (1/200, Abcam, ab42905, RRID:AB_732519), rabbit anti-LysRS (1/200, Abcam, ab129080, RRID:AB_11155689), mouse anti-neurofilament (1/50, 2H3, developed and deposited by Jessell, T.M./Dodd, J., Developmental Studies Hybridoma Bank, supernatant), mouse anti-NF200 (1/500, Sigma, N0142, RRID:AB_477257) and rabbit anti-peripherin (1/500, Merck Millipore, AB1530, RRID:AB_90725). The analyses of L1-L5 and C4-C8 wild-type DRG were performed at different times, as were the assessments of lumbar DRG dissected from the different genetic strains.
Western Blotting of DRG Lysates
Probing and analysis of DRG lysate western blots were performed as previously described (Sleigh et al., 2017a), using the following antibodies: mouse anti-Gapdh (1/3,000, Merck Millipore, MAB374, RRID:AB_2107445), anti-GlyRS (1/2,000), anti-LysRS (1/500), anti-NF200 (1/1,000), anti-peripherin (1/1,000), rabbit anti-TrkB (1/1,000, BD Biosciences, 610101, RRID:AB_397507) and rabbit anti-TyrRS (1/500, Abcam, ab150429, RRID:AB_2744675). Ten microgram of DRG lysate was loaded per lane.
Culturing Primary DRG Neurons
Twenty to twenty-four lumbar to thoracic DRG (Figure 1B) were dissociated and cultured on 35 mm glass-bottom dishes (MatTek, P35G-1.5-14-C) in the presence of freshly added 20 ng/ml mouse glial cell line-derived neurotrophic factor (GDNF, PeproTech, 450-44) as detailed elsewhere (Sleigh et al., 2017a). To reduce variability, a wild-type and GarsC201R/+ littermate of the same sex were dissected and cultured in parallel for each experimental replicate.
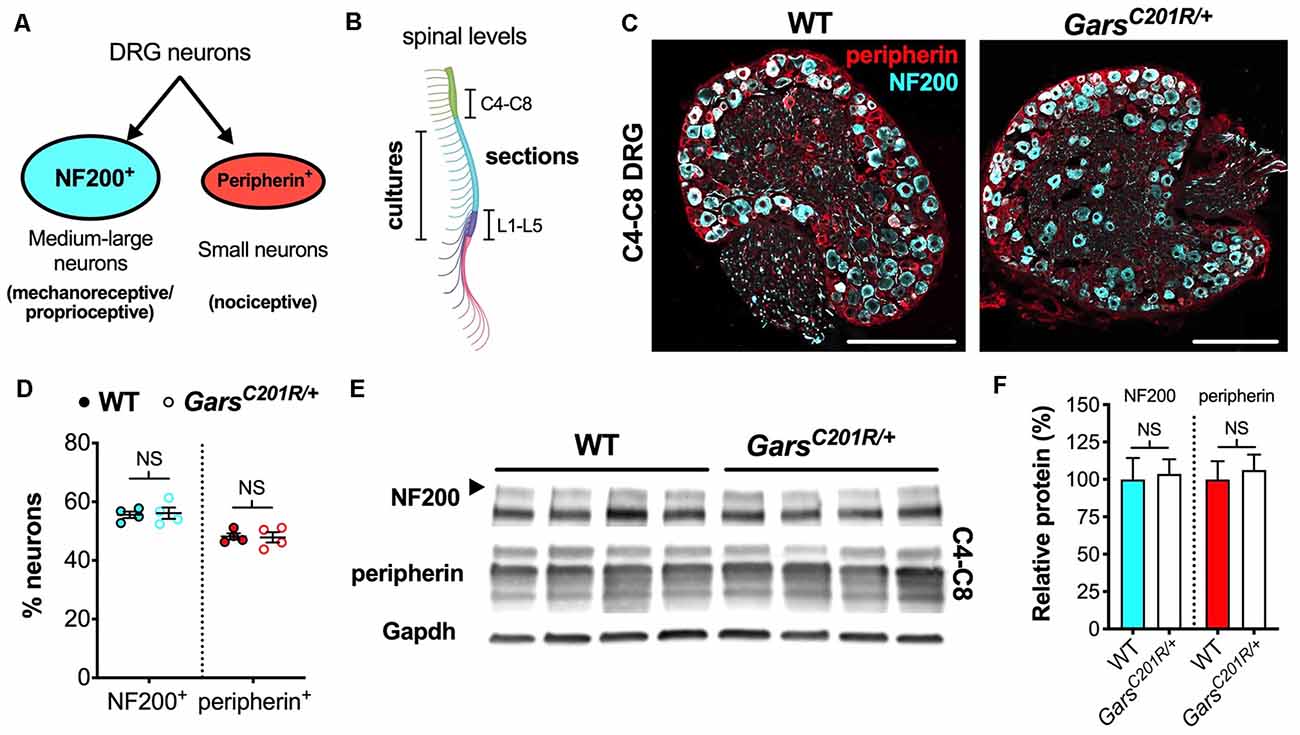
Figure 1. Sensory neuron development is not impaired in GarsC201R/+ dorsal root ganglion (DRG) at cervical spinal levels. (A) Neurons found in sensory ganglia can be classified into NF200+ cells, which are mainly medium-large in size and function as either mechanoreceptors or proprioceptors, and peripherin+ cells that are generally small and nociceptive. (B) DRG used in this study were taken from cervical spinal level 4 (C4) to C8 and lumbar level 1 (L1) to L5 for immunofluorescence analysis and from thoracic to lumbar levels for primary cultures. The schematic was created with BioRender (https://biorender.com). (C) Representative immunofluorescence images of 1-month-old wild-type and GarsC201R/+ cervical DRG sections stained for NF200 (cyan) and peripherin (red). Scale bars = 200 μm. (D) There was no difference in the proportions of NF200+ (P = 0.812, unpaired t-test) or peripherin+ (P = 0.885, unpaired t-test) neurons between genotypes in C4-C8 ganglia, n = 4. (E) Representative western blot of C4-C8 DRG lysates from 1-month-old wild-type and GarsC201R/+ mice probed for NF200, peripherin, and the loading control Gapdh. (F) Consistent with the immunofluorescence analysis (D), there was no difference between genotypes in levels of NF200 (P = 0.835, unpaired t-test) or peripherin (P = 0.702, unpaired t-test) protein, n = 5. NS, not significant; WT, wild-type. See also Supplementary Figures S1, S2.
In vitro Signaling Endosome Transport Assay
The atoxic binding fragment of tetanus neurotoxin (HCT) was bacterially expressed and labeled with the AlexaFluor647 antibody labeling kit (Life Technologies, A-20186) as previously outlined (Gibbs et al., 2016). Twenty-four hours post-plating of dissociated DRG neurons, HCT-647 was added to the neuronal media at a final concentration of approximately 1.5 μg/ml (30 nM), before gentle mixing by rotation and returning to 37°C for 25 min. HCT-containing medium was then aspirated, the cells were washed with 2 ml pre-warmed medium, before being slowly flooded with 2 ml standard medium containing all supplements. Within 10–90 min of the media change, endosome transport was imaged on an inverted LSM780 laser scanning microscope (Zeiss) inside an environmental chamber pre-warmed and set throughout the experiment to 37°C. An area containing a single neuronal process retrogradely transporting fluorescent endosomes was imaged using a 63× Plan-Apochromat oil immersion objective (Zeiss). Images were taken at 100× digital zoom (1,024 × 1,024, 1% laser power) every 2.4 s on average. Before selecting a neuronal process for analysis, it was first traced back to the cell body to confirm the directionality of transport and imaged for area measurement (see below). Cultures from wild-type and GarsC201R/+ mice were imaged in the same session and, to avoid introducing time-dependent biases, their order was alternated across replicates. Two males and two females of each genotype were analyzed at each timepoint.
Endosome Transport Analysis
Individual endosomes were manually tracked using Tracker (Kinetic Imaging Limited) as described previously (Sleigh et al., 2020a). Briefly, endosomes were included in the analysis if they could be observed for five consecutive frames and did not pause for >10 consecutive frames. Endosomes moving solely in the anterograde direction were infrequent and not included in the analysis. Individual frame-to-frame step speeds are included in the frequency histogram (an average of 2, 370 ± 135 frame-to-frame speeds per animal), meaning that an endosome tracked across 21 consecutive frames will generate 20 frame-to-frame speeds in this graph. To determine the endosome speed per animal, individual endosome speeds were calculated, and then the mean of these determined (an average of 95.1 ± 3.5 endosomes per animal). All speed analyses include frames and time during which endosomes may have been paused, i.e., the speed across the entire tracked run length is reported and not the speed solely when motile. An endosome was considered to have paused if it remained in the same position for two or more consecutive frames. The “% time paused” is a calculation of the length of time all tracked endosomes remained stationary, while the “% pausing endosomes” details the proportion of endosomes that displayed at least one pause while being tracked. An average of 26.2 endosomes was tracked per neuron, and at least three individual neurons were assessed per animal replicate.
Image Analysis
Cell body areas of neurons analyzed in endosome transport assays were measured using the freehand tool on ImageJ1 to draw around the circumference of the somas. The diameters of neuronal processes imaged for transport were measured in ImageJ using the straight-line tool. The average of five measurements across the width of the process was calculated. To determine in which cells GlyRS levels were highest in GarsNmf249/+ lumbar DRG sections, all cells with increased GlyRS expression were first identified by eye in the single fluorescence channel. Cells positive for NF200 were then independently designated in the second channel. The percentage of GlyRS-elevated cells also positive for N200 was then calculated. Similarly, the percentage of NF200+ cells without an increase in GlyRS was also determined. All sections used for GlyRS analysis were stained and imaged in parallel with the same confocal settings to permit side-by-side comparison.
Statistical Analysis
Data were assumed to be normally distributed unless evidence to the contrary could be provided by the D’Agostino and Pearson omnibus normality test. GraphPad Prism 8 (version 8.4.0, La Jolla, CA, USA) was used for all statistical analyses. Means ± standard error of the mean are plotted, as well as individual data points in all graphs except for those depicting western blot densitometry. Unpaired t-tests and two-way ANOVAs were used throughout the study. Rather than ANOVAs, unpaired t-tests were used to analyze the percentages of NF200+ and peripherin+ neurons separately, because the two markers are not independently expressed. Similarly, western blot densitometry was also analyzed using unpaired t-tests; since expression was calculated relative to wild-type levels for each individual protein, the expression of proteins in wild-type animals are not statistically comparable.
Results
Altered Sensory Development Occurs Specifically in Lumbar Segments of CMT2D Mice
In previous work, we showed that sensory neuron fate is altered during development in the mild GarsC201R/+ and more severe GarsNmf249/+ mouse models for CMT2D, the extent of which correlated with overall model severity (Sleigh et al., 2017a). In that study, by co-staining DRG for NF200, a marker of medium-large area mechanosensitive/proprioceptive neurons, and peripherin, which identifies small area nociceptive neurons (Figure 1A), we determined that mutant Gars DRG had fewer touch- and body position-sensing (NF200+) neurons and a concomitant increase in noxious stimulus-sensing (peripherin+) neurons. We reported that this phenotype was present at birth and did not change up to 3 months of age, suggesting it is developmental in origin and non-progressive. Ganglia assessed in these original experiments were isolated from lumbar level 1 (L1) to L5, which contains neurons that innervate the lower leg (Mohan et al., 2014); however, CMT2D patients frequently display upper limb predominance (Antonellis et al., 2018).
To determine whether the phenotype is also observed in forelimb-innervating ganglia (Tosolini et al., 2013), we isolated and immunohistochemically analyzed cervical level 4 (C4) to C8 DRG from 1-month-old wild-type and GarsC201R/+ mice (Figure 1B). Co-labelling DRG for NF200 and peripherin (Figure 1C) and calculating the percentages of neurons expressing each marker, we saw no difference between genotypes (Figure 1D). Corroborating this, western blotting of cervical DRG lysates showed no difference in NF200 or peripherin protein levels (Figures 1E,F). Together, these data indicate that there is no impairment in sensory neuron identity in the C4-C8 ganglia of GarsC201R/+ mice.
To confirm and extend the lumbar phenotype, we assessed levels of the protein TrkB, which binds brain-derived neurotrophic factor (BDNF) and neurotrophin-4 (NT-4) to ensure the survival of a mechanosensitive sub-population of NF200+ neurons (Montaño et al., 2010). We found that lumbar ganglia of GarsC201R/+ mice have less total TrkB, consistent with there being fewer NF200+ neurons in the mutant DRG, whereas C4-C8 ganglia showed no difference (Supplementary Figure S1).
We then statistically compared the proportions of wild-type cervical DRG neurons with previously published data from 1-month-old wild-type L1-L5 DRG (NF200+ 40.7 ± 1.9%; peripherin+ 61.5 ± 2.1%; Sleigh et al., 2017a). We found that the ratio of subtypes is more even in cervical ganglia, which possess significantly more NF200+ and significantly fewer peripherin+ neurons than lumbar DRG (Supplementary Figure S2A).
In the past, we also identified a sensory neurodevelopmental phenotype in embryonic GarsC201R/+ hindlimbs (Sleigh et al., 2017a). Dissecting hind paws from embryonic day 13.5 (E13.5) mice and staining neurons for neurofilament (2H3), we observed impaired arborization of nociceptive neurons found in the developing dorsal floor plate. To evaluate whether this phenotype is also seen in forelimbs, we analyzed forepaws from E13.5 embryos (Figure 2A). Similar to the hind paws, there was no difference in sensory nerve growth between genotypes, assessed by measuring the distance from nerve growth cone to digit tip (Figure 2B). However, CMT2D forepaws did not display the nociceptive nerve branching defect present in lower limbs (Figure 2C). Therefore, similar to the DRG, developing sensory neurons originating at cervical spinal levels do not show the impairments found in lumbar afferent nerves.
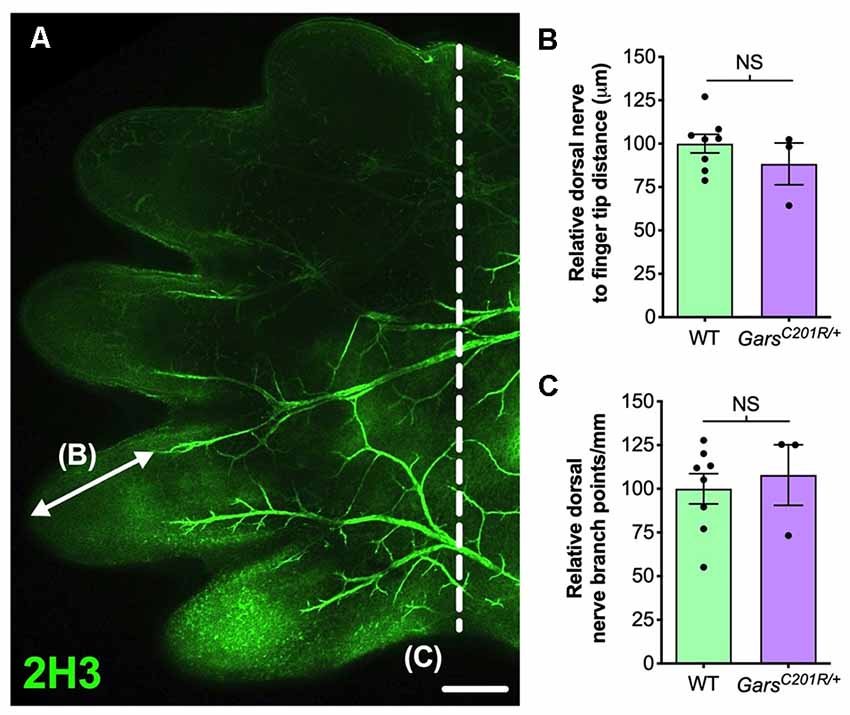
Figure 2. Sensory neurodevelopment appears normal in the forelimb of GarsC201R/+ embryos. (A) A representative single confocal plane, tile scan image of the dorsal aspect of an E13.5 wild-type forepaw stained for neurofilament (2H3, green). The arrow depicts distance from a major nerve branch ending to the tip of a finger, which was measured for B. The nerves to the left of the dashed line were used for branch analysis in (C). Scale bar = 250 μm. (B,C) There was no difference between wild-type and GarsC201R/+ mice in sensory nerve extension into forepaw extremities (B, P = 0.328, unpaired t-test), nor in the amount of branching (C, P = 0.662, unpaired t-test), n = 3–8. NS, not significant; WT, wild-type.
Sensory Populations Are Unaltered in a Mouse Model of ALS
We believe that the small, yet physiologically relevant, distortion of sensory populations in lumbar ganglia of CMT2D mice may be associated with aberrant mutant GlyRS-Trk receptor binding during development. To see whether it extends to other mouse models of neuromuscular disease, we analyzed L1-L5 DRG from SOD1G93A mice, an established model of SOD1-associated amyotrophic lateral sclerosis (ALS), which displays a plethora of defects and dysfunctional pathways in peripheral, albeit mainly motor, nerves (Kim et al., 2015; Nardo et al., 2016). Lumbar DRG were dissected and immunohistochemically processed from SOD1G93A and littermate control males at P30–31 (Figure 3A) and P100–101 (Figure 3C), representing pre-symptomatic and late disease stages, respectively. No distinctions in sensory populations were observed at either age (Figures 3B,D), suggesting that the sensory subtype switch is not observed in all mouse models of neuromuscular diseases.
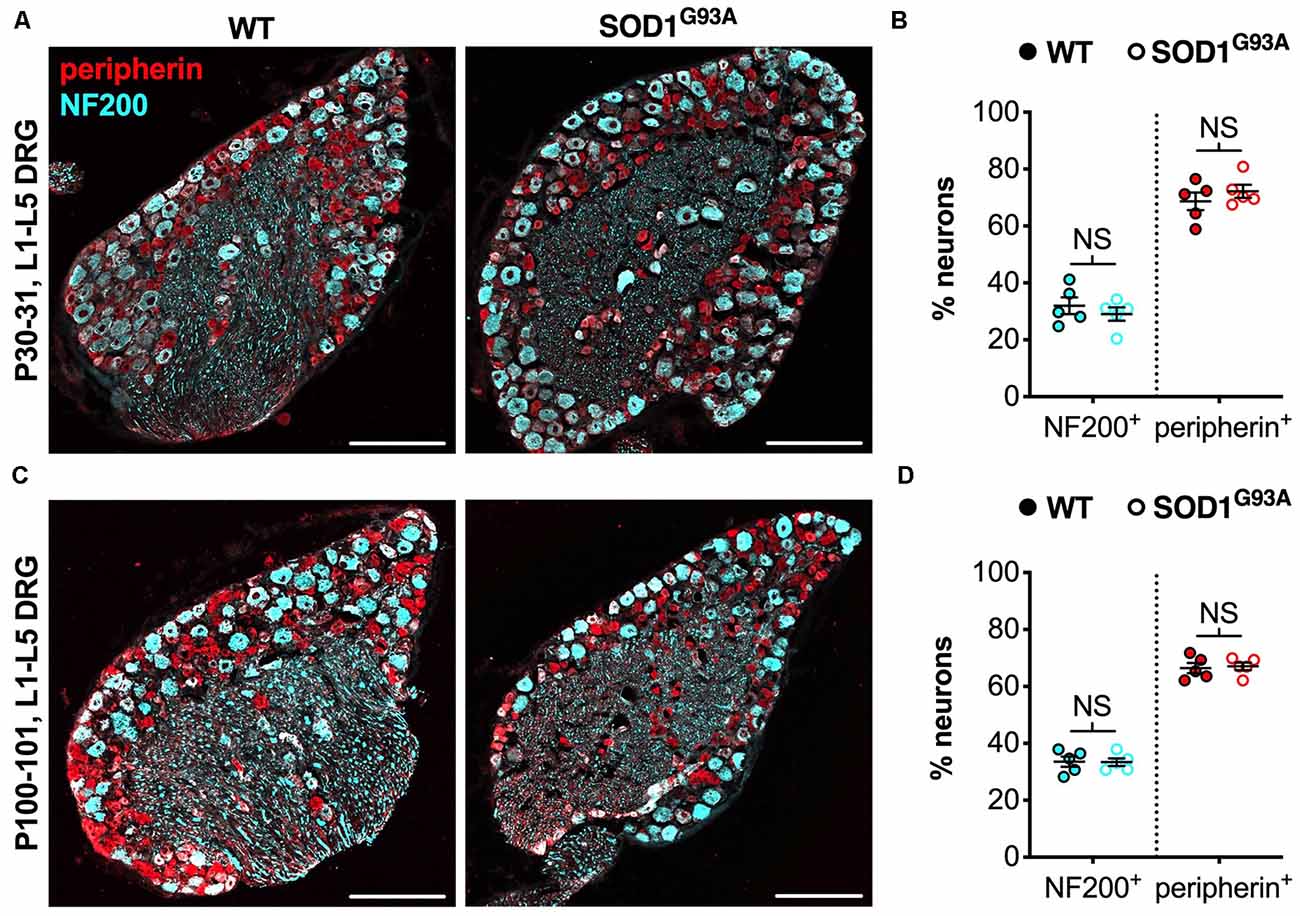
Figure 3. The CMT2D lumbar DRG sensory subtype switch is not observed in SOD1G93A mice. (A,C) Representative immunofluorescence images of L1-L5 DRG sections from P30–31 (A) and P100–101 (C) wild-type and SOD1G93A mice stained for NF200 (cyan) and peripherin (red). Scale bars = 200 μm. (B,D) There was no difference in the percentage of NF200+ and peripherin+ neurons between wild-type and SOD1G93A mice at P30–31 (NF200 P = 0.460, peripherin P = 0.391; unpaired t-tests) nor P100–101 (NF200 P = 0.938, peripherin P = 0.782; unpaired t-tests), n = 5. NS, not significant; WT, wild-type. See also Supplementary Figure S2.
SOD1G93A and GarsC201R/+ mice are maintained on different genetic backgrounds, and it appeared as though there may be a small difference in neuron populations between wild-types of the two strains. We, therefore, compared neuron proportions at 1 and 3 months in lumbar DRG from wild-type mice on a mixed C57BL/6-SJL background (SOD1G93A control) vs. a pure C57BL/6J background (GarsC201R/+ control). We observed a small, but significant difference between strains in the percentage of NF200+, but not peripherin+, neurons at 1 month, but not 3 months (Supplementary Figures S2B,C).
Long-Range Signaling Endosome Transport Is Unaffected in CMT2D Sensory Neurons
Axonal transport is reliant upon motor proteins traversing microtubule networks to deliver diverse cargoes from one end of an axon to the other (Guedes-Dias and Holzbaur, 2019). Anterograde transport from the cell body to the axonal terminal is key for delivering organelles, proteins, and RNAs towards peripheral synapses. Connecting the axon tip to the cell body, retrograde transport is needed for long-range delivery of autophagosomes and survival-promoting neurotrophic factors. Pre-symptomatic disturbances in axonal trafficking are thought to underlie, or at least contribute to, several neurological diseases (Sleigh et al., 2019). Indeed, primary DRG neurons cultured from 12 day old GarsNmf249/+ mice display reduced retrograde transport speeds of nerve growth factor (NGF)-loaded endosomes (Mo et al., 2018), while reduced mitochondrial motility was also identified in sensory processes of 12-month-old GarsC201R/+ mice (Benoy et al., 2018). Disruption of two different cargoes suggests a broad transport impairment (e.g., due to microtubule dysfunction); however, GarsC201R/+ neurons were cultured from late symptomatic mice, thus the defective trafficking observed in this model may simply be a secondary consequence of neuropathology.
To analyze GarsC201R/+ transport in early symptomatic sensory neurons, we cultured primary thoracic and lumbar DRG neurons from 1 and 3-month-old mice and assessed retrograde signaling endosome trafficking. These spinal levels were combined to obtain sufficient cell numbers for the assay (Figure 1B), and the time points were chosen to allow comparison with several other phenotypes assessed previously in this model (Sleigh et al., 2014b, 2017a,b). Cultures were incubated with fluorescently labeled atoxic binding fragment of tetanus neurotoxin (HCT-647), which is taken up by neurons and loaded into signaling endosomes containing Trk receptors and p75 neurotrophin receptor (p75NTR), when applied to media (Deinhardt et al., 2006, 2007). Time-lapse confocal microscopy was performed to enable tracking of individual endosomes (Figure 4A). Sensory neurons cultured from DRG do not always display a visible axon initial segment and may bear several morphologically indistinguishable axon-like extensions/processes (Nascimento et al., 2018), thus the trafficking analyzed may not always be “axonal” transport per se.
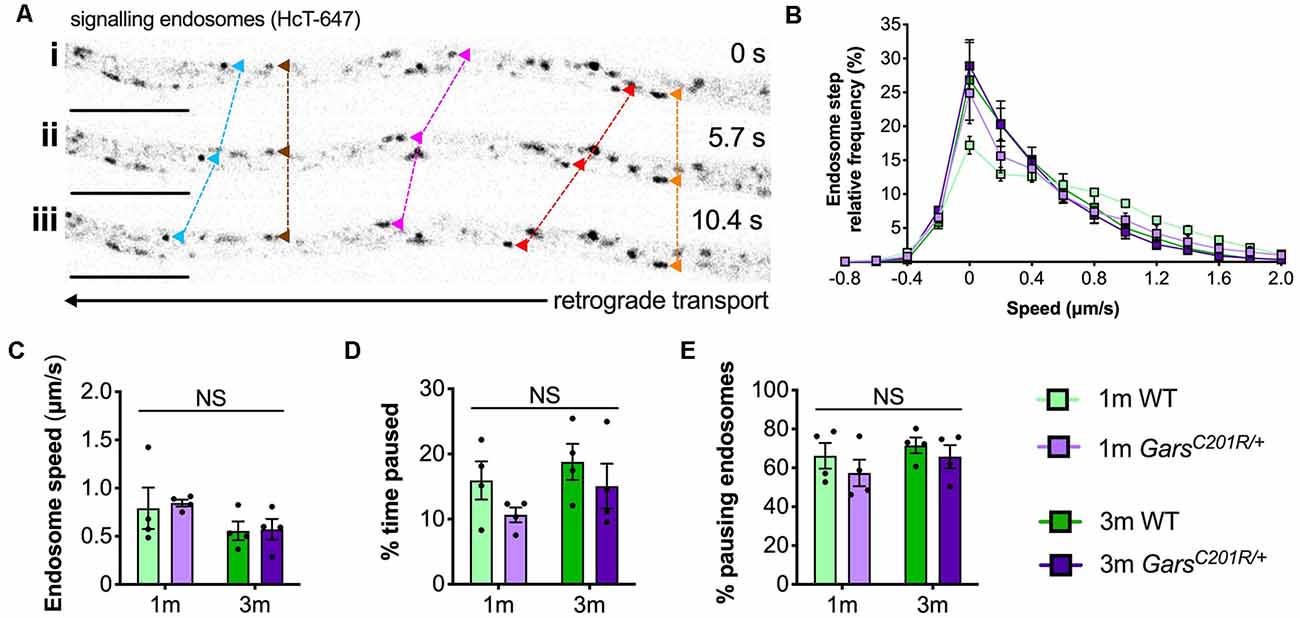
Figure 4. Axonal transport of signaling endosomes is unaffected in large area GarsC201R/+ sensory neurons. (A) Individual signaling endosomes loaded with fluorescently labeled HCT (HCT-647, grayscale inverted) were tracked and analyzed from primary sensory neurons. Image series (i-iii) depicts retrograde (right to left) trafficking of distinct endosomes (arrowheads linked by dashed lines across time series). The imaged neuron was cultured from a P97 wild-type female. Scale bars = 10 μm. (B) Frame-to-frame speed distribution curves indicate that there was no clear distinction in endosome kinetics between genotypes or timepoints. (C–E) This was confirmed by analyzing average endosome speeds (C, genotype P = 0.792, age P = 0.076, interaction P = 0.884; two-way ANOVA), the percentage of time endosomes were paused for (D, genotype P = 0.123, age P = 0.206, interaction P = 0.779; two-way ANOVA), and the percentage of pausing endosomes (E, genotype P = 0.245, age P = 0.273, interaction P = 0.805; two-way ANOVA), n = 4. 1 m, 1 month; 3 m, 3 months; NS, not significant; WT, wild-type. See also Supplementary Figures S3, S4.
Contrary to the previous studies, overlapping histograms of endosome frame-to-frame speeds suggest that there is little difference in endosome dynamics between genotypes and timepoints (Figure 4B). This was confirmed by analyzing average endosome speeds (Figure 4C), the percentage of time that endosomes were paused (Figure 4B), and the percentage of pausing endosomes (Figure 4E) per animal. There was also no difference in transport parameters between wild-type and GarsC201R/+ when axons were used as the experimental unit (Supplementary Figure S3); however, irrespective of the genotype, older cultures did display a general slowing of endosome speeds linked to increased pausing when compared in this manner.
Transport was assessed in larger area neurons only, because there is a less frequent overlap of moving endosomes in wider processes, likely due to lower microtubule density (Ochs et al., 1978), permitting greater tracking accuracy. Although not confirmed immunohistochemically, analyzed cells were therefore very likely to be medium-large NF200+ sensory neurons (i.e., mechanosensitive or proprioceptive). Nonetheless, given the distortion in sensory subtypes in CMT2D lumbar DRG (Sleigh et al., 2017a) it is possible that different neuron populations were analysed between genotypes. Thus, we measured cell body areas and process diameters from the neurons in which endosome transport was assessed (Supplementary Figure S4). There were no differences in these morphological properties, indicating that similar neurons were analyzed across genotypes and timepoints.
Elevated GlyRS Levels in Select Neurons Are Linked to the Sensory Subtype Switch
GlyRS protein levels have previously been reported to be elevated in both GarsC201R/+ and GarsNmf249/+ brains (Achilli et al., 2009; Stum et al., 2011), perhaps as a compensatory response to impaired protein function. To determine whether GlyRS levels are also altered in sensory neurons, we extracted and performed western blotting on C4-C8 and L1-L5 DRG from 1-month-old GarsC201R/+ mice (Figure 5A). There was no difference in GlyRS levels in cervical ganglia; however, GlyRS was upregulated more than 2-fold in L1-L5 DRG (Figure 5B). This was corroborated by GlyRS immunofluorescence analysis in C4-C8 (Figure 5C) and L1-L5 (Figure 5D) ganglia. We also assessed L1-L5 DRG of 1-month-old GarsNmf249/+ mice and saw the same pattern of enhanced GlyRS fluorescence in a subset of DRG neurons (Figure 5E), thus indicating that the increase of mutant GlyRS levels in L1-L5 DRG is an early event in CMT2D pathogenesis.
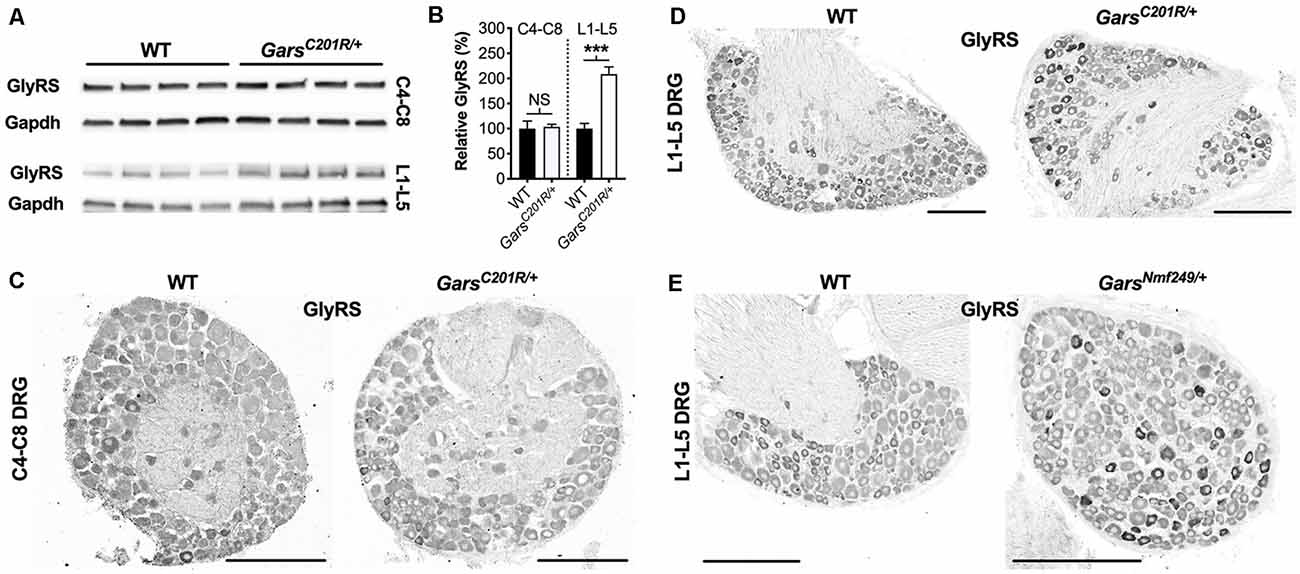
Figure 5. GlyRS protein levels are elevated in lumbar, but not cervical, DRG of CMT2D mice. (A) Representative western blot of C4-C8 (top) and L1-L5 (bottom) DRG lysates from 1-month-old wild-type and GarsC201R/+ mice probed for GlyRS and the loading control Gapdh. (B) There was no difference between genotypes in GlyRS levels in cervical ganglia (P = 0.825; unpaired t-test; n = 5); however, GlyRS was elevated in mutant L1-L5 DRG (***P < 0.001, NS not significant; unpaired t-test; n = 4). (C,D) This was confirmed by immunofluorescence analysis of GlyRS in the cervical (C) and lumbar (D) DRG sections from 1-month-old wild-type and GarsC201R/+ mice, n = 4. GlyRS levels appear higher in many individual neurons with larger cell bodies in the mutant lumbar DRG (top right image). (E) These findings were replicated when comparing sections of L1-L5 ganglia from 1-month-old wild-type and GarsNmf249/+ mice, n = 3. Scale bars = 200 μm. WT, wild-type.
Upon closer inspection, GlyRS immunofluorescence is marginally higher in some of the smaller area neurons of wild-type lumbar DRG; however, in both Gars mutants, the upregulation appears to be in larger neurons. To better characterize this, we co-stained GarsNmf249/+ lumbar DRG for GlyRS and NF200 (Figure 6A). We found that ≈87% of neurons with increased GlyRS levels were also NF200+ (Figure 6B), which is a particularly high proportion considering that these mutant ganglia consist of only ≈22% NF200+ neurons (Sleigh et al., 2017a). This suggests that there is a preferential increase of GlyRS in mechanosensitive and proprioceptive neurons. We then quantified the percentage of NF200+ neurons that showed elevated GlyRS and found that ≈32% showed the phenotype (Figure 6B), indicating that GlyRS is differentially upregulated even within this neuronal population, perhaps in a subgroup with a particular function.
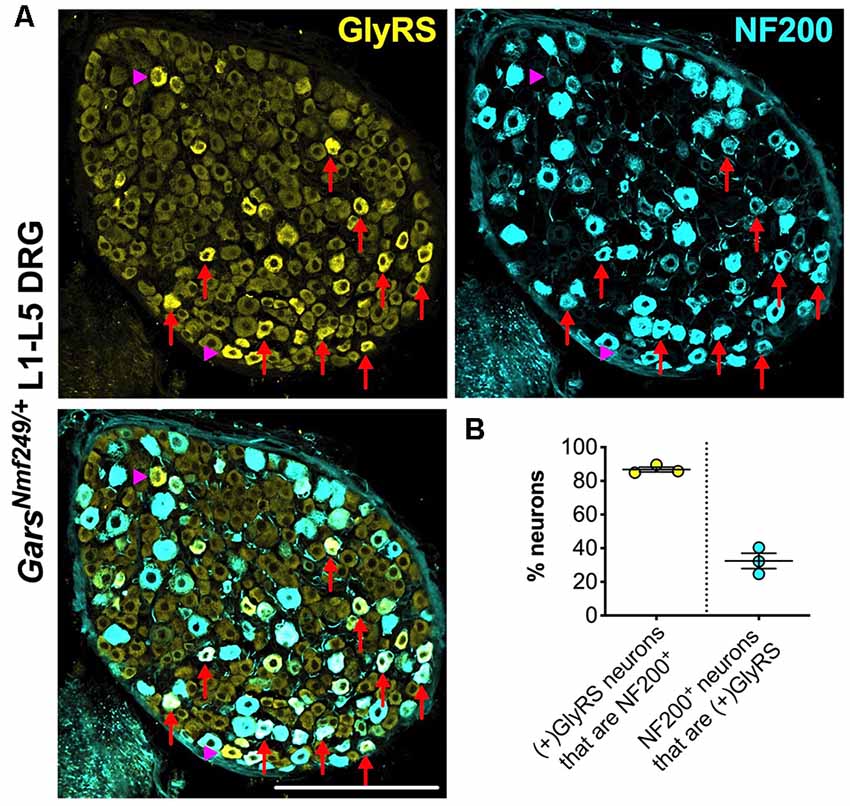
Figure 6. GlyRS is preferentially increased in some, but not all, NF200+ sensory neurons of GarsNm249/+ mice. (A) Representative immunofluorescence image of an L1-L5 DRG section from a 1-month-old GarsNm249/+ mouse stained for GlyRS (yellow) and NF200 (cyan). Red arrows and magenta arrowheads highlight N200+ and NF200− neurons, respectively, in which GlyRS was increased. Scale bar = 200 μm. (B) The majority of neurons in which GlyRS was upregulated, designated “(+)GlyRS,” express NF200; however, not all NF200+ cells have increased GlyRS levels, n = 3.
A global increase in ARS proteins would perhaps suggest dysfunction in a cellular process linked to aminoacylation, for instance, protein translation, which is impaired a gain-of-function manner in CMT2D fly models (Niehues et al., 2015). We, therefore, assessed whether upregulation is GlyRS-specific by analyzing lumbar DRG levels of Kars-encoded lysyl-tRNA synthetase (LysRS) and Yars-encoded tyrosyl-tRNA synthetase (TyrRS; Figure 7A). LysRS was chosen because, with GlyRS, it is the only other dual-localized synthetase functioning in both cytoplasm and mitochondria, while TyrRS was chosen because there is strong evidence that mutations in its encoding gene, YARS1, cause CMT (Wei et al., 2019). GarsC201R/+ L1-L5 DRG shows no change in LysRS levels, but a small yet significant rise in TyrRS (Figure 7B). We confirmed the lack of LysRS upregulation by staining lumbar ganglia from GarsNmf249/+ mice (Figure 7C). TyrRS immunohistochemistry was attempted, but the resulting staining pattern was not consistent with a cytoplasmic tRNA synthetase, suggestive of non-specific staining.
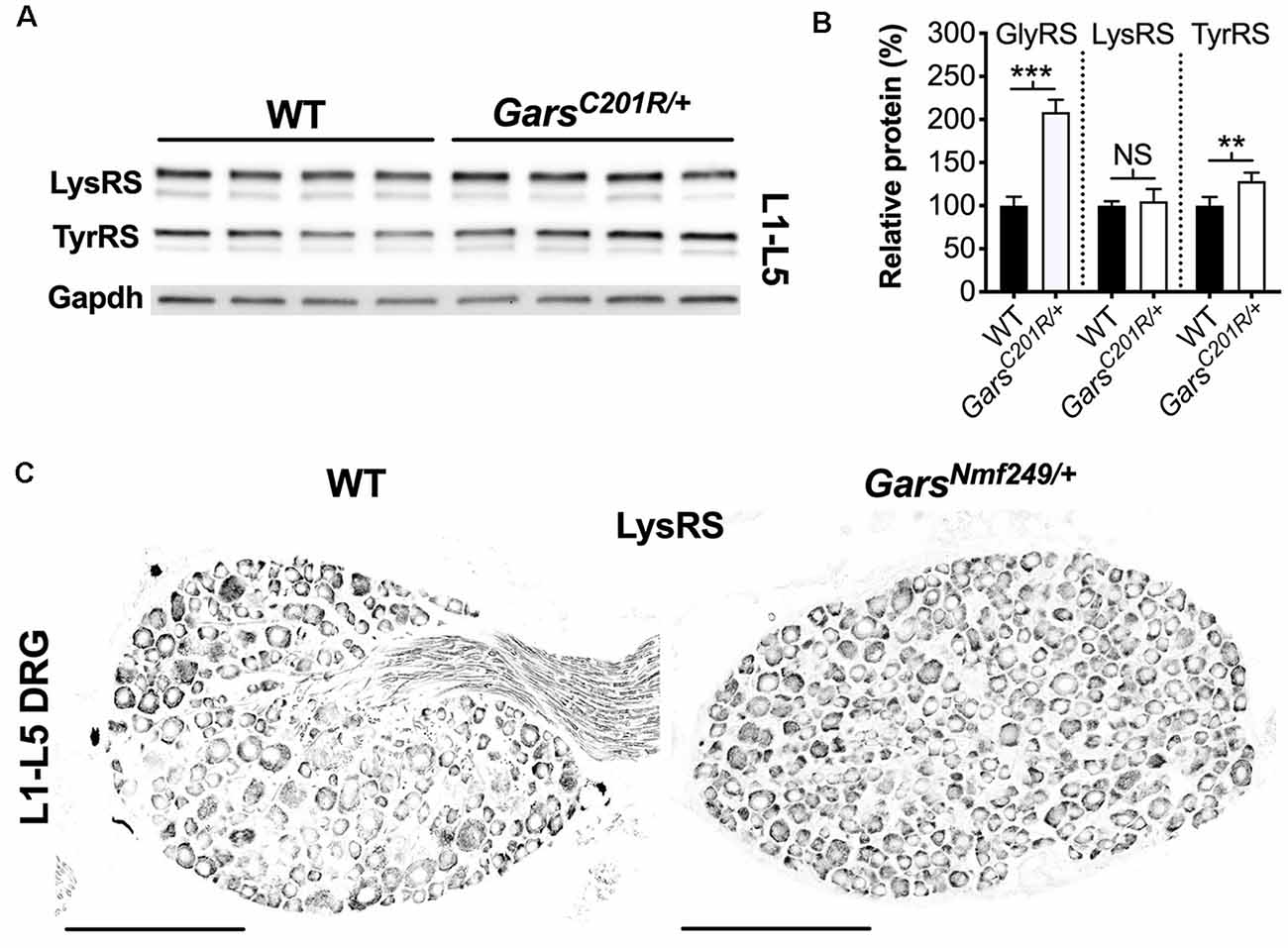
Figure 7. Not all aminoacyl-tRNA synthetase (ARS) proteins are upregulated in lumbar DRG of CMT2D mice. (A) Representative western blot of L1-L5 DRG lysates from 1-month-old wild-type and GarsC201R/+ mice probed for aminoacyl-tRNA synthetase enzymes LysRS and TyrRS, and the loading control Gapdh. (B) Densitometry analysis indicates that LysRS levels did not differ between genotypes. There was a small, but significant, increase in TyrRS levels in GarsC201R/+ lumbar DRG, but the change was much less than that observed for GlyRS (densitometry analysis from Figure 5 included for comparison). ***P < 0.001, **P < 0.01, NS, not significant; unpaired t-test, n = 4. (C) Representative immunofluorescence analysis of LysRS in L1-L5 ganglia sections from 1-month-old wild-type and GarsNmf249/+ mice. Unlike GlyRS (Figure 6), but consistent with the GarsC201R/+ LysRS western blot (A,B), individual neurons did not display an increase in LysRS levels, n = 3. N.b., the positive LysRS signal observed in axons (wild-type image) was also seen in the secondary only control and was therefore likely to be non-specific. Scale bars = 200 μm. WT, wild-type.
Together, these data indicate that there is a selective increase in Gars expression that occurs predominantly in NF200+ cells and only at spinal levels displaying a developmental perturbation of sensory neuron fate.
Discussion
Sensory Phenotypes Are Restricted to the Lower Limbs of CMT2D Mice
CMT2D mice display developmental phenotypes in sensory neurons innervating the hind paws (Sleigh et al., 2017a). To extend these analyses and determine whether the upper limb predominance of patients is replicated, we assessed afferent nerves from cervical spinal levels in GarsC201R/+ mice. The lumbar phenotypes of subtype switching and impaired axon branching were not present in sensory neurons targeting forepaws (Figures 1, 2, and Supplementary Figure S1), suggesting that anatomical location has a considerable bearing on neurodevelopmental pathology. Given that these phenotypes are developmental and do not progress in severity from P1 to 3 months, it is unlikely that they occur in cervical DRG later in the disease.
Although mutations in GARS1 frequently cause hands to be affected before and more severely than feet, there are examples where the opposite occurs (Sivakumar et al., 2005; Forrester et al., 2020); albeit it is unclear as to whether this also applies to sensory symptoms. Similarly, the GarsC201R mutation could therefore simply preferentially impact lower limbs. However, restriction of weakness to CMT2D patient feet is rare. So, what could be driving the differential pathology between lumbar and cervical ganglia in mice? It may be due to a distinction in the proportions of sensory subtypes. In wild-type mice, NF200 is expressed by ≈41% of neurons in L1-L5 DRG (Sleigh et al., 2017a), whereas ≈56% are N200+ in cervical ganglia (Supplementary Figure S2A). If mutant GlyRS aberrantly interacts with Trk receptors pre-natally, thus impacting sensory development and skewing the proportions of functional subclasses (Sleigh et al., 2017a), then GlyRS is likely to have less impact on ganglia that have a more equal balance between NF200+ and peripherin+ cells, as is observed in C4-C8 DRG. Alternatively, spinal level distinctions may be caused by differences in the amount or kinetics of GlyRS secretion or Trk expression. DRG at different spinal levels develop asynchronously (Lawson and Biscoe, 1979) and possess divergent transcription factor profiles (Lai et al., 2016), which may also contribute to the lower limb predominance observed in CMT2D mice. Indeed, the transcription factors neurogenin 1 and 2, which drive two distinct waves of neurogenesis required for segregation of major classes of Trk-expressing sensory neurons, are differentially required by cervical and more caudal sensory ganglia (Ma et al., 1999).
Irrespective of the cause, experiments presented here highlight the importance of comparative anatomy in mouse models of neuromuscular disorders to enhance understanding of pathomechanisms. It remains to be seen whether such variations are also observed in the motor nervous system of CMT2D mice, although differential susceptibility of muscles to NMJ denervation has been previously reported (Seburn et al., 2006; Sleigh et al., 2014b, 2020; Spaulding et al., 2016).
Developmental Perturbation of Sensory Fate Is Not a Common Phenotype
A difference in sensory neuron populations has also been reported in lumbar DRG of mice modeling spinal muscular atrophy (SMA; Shorrock et al., 2018). We, therefore, aimed to determine whether this phenotype is a general feature of mouse models of neuromuscular disease, as this would cast doubt on the aberrant binding of mutant GlyRS to Trk receptors as being the cause of impaired sensory development in mutant Gars mice. SOD1G93A mice modeling SOD1-linked ALS show a variety of defects in sensory neurons (Sassone et al., 2016; Vaughan et al., 2018; Seki et al., 2019); however, they do not display a subtype switch in lumbar DRG (Figure 3). Moreover, we recently found that mice modeling a developmental form of SMA caused by loss-of-function mutations in BICD2 also do not show this phenotype (Rossor et al., 2020). Together, these data suggest that perturbed sensory development is not observed in all mouse models of neurodegeneration.
We did, however, see a small difference in lumbar DRG between wild-type littermates of SOD1G93A and GarsC201R/+ mice (Supplementary Figures S2B,C), which are maintained on different genetic backgrounds, suggesting that genetic background may subtly influence sensory neuron populations, likely contributing to previously reported disparities in sensation between strains (Crawley et al., 1997). This result is not overly surprising considering that mouse genetic background can influence even gross anatomical features such as the number of spinal levels (Rigaud et al., 2008).
Long-Range Transport Is Not Universally Impaired in CMT2D Sensory Neurons
Deficits in axonal transport contribute to many different genetic neuropathies (Prior et al., 2017; Beijer et al., 2019), and its early involvement in disease may be a common driver in peripheral nerve selectivity typical of many forms of CMT2. Indeed, disruption of long-range trafficking has been identified in sensory neurons cultured from CMT2D DRG (Benoy et al., 2018; Mo et al., 2018). Contrastingly, we found no difference in retrograde transport of signaling endosomes between wild-type and GarsC201R/+ at 1 and 3 months of age (Figure 4 and Supplementary Figure S3). Nonetheless, if thoracic DRG show limited to no pathology, by combining thoracic DRG with L1-L5 ganglia we may have masked a lumbar-specific DRG transport phenotype. Additionally, the medium and associated supplements in which DRG neurons were cultured vary across studies. Neuronal activity can impact the rate and quantity of axonal transport (Sajic et al., 2013; Wang et al., 2016), while proteins such as neurotrophic factors, which are present in primary neuron media, can affect neuronal activity (Dombert et al., 2017). Though unlikely, it is possible therefore that the medium in which our neurons were grown may have selectively enhanced GarsC201R/+ transport masking a trafficking deficiency.
Mo et al. (2018) identified a slow-down in NGF-containing endosomes tracked in sensory neurons cultured from 12-day old GarsNmf249/+ mice. Firstly, if transport disruption correlates with the overall disease burden, then the more severe mutant allele is more likely to display a defect than the milder GarsC201R/+ mutant. Equally as important, the assayed neurons in the two studies were perhaps different. NGF binds to TrkA, which is expressed by nociceptors (Supplementary Figure S1A), thus transport was probably assessed in noxious stimulus-sensing peripherin+ neurons from GarsNmf249/+ mice. The atoxic binding fragment of tetanus neurotoxin that we used to assess transport is taken up into multiple populations of signaling endosomes (Villarroel-Campos et al., 2018); however, we focused our analyses on large area neurons with wide processes (Supplementary Figure S4), likely to be either TrkB+ mechanoreceptors or TrkC+ proprioceptors.
Benoy et al. (2018) identified that in vitro sensory neurons cultured from 12-month-old GarsC201R/+ mice had an almost complete impairment in mitochondrial motility. The disparity with this study could simply reflect the analyzed cargo, i.e., mitochondrial, but not endosomal, transport is altered in this model. Alternatively, the difference may be due to the age at which the neurons were tested (1 and 3 months vs. 12 months). Supporting this idea, we have previously shown that GarsC201R/+ sensory neurons cultured from 1-month-old animals show normal neurite/process outgrowth (Sleigh et al., 2017a); however, this was defective in 12-month-old cells (Benoy et al., 2018). Decreased neuronal health may, therefore, be contributing to reduced mitochondrial motility, as may the process of aging. Indeed, we have previously reported that the dynamics of signaling endosome transport in vivo remain unaltered in aged wild-type mice (Sleigh and Schiavo, 2016; Sleigh et al., 2020a), whereas mitochondrial transport is known to be altered in old animals (Mattedi and Vagnoni, 2019).
Our long-range retrograde transport data indicate that cargo trafficking is not globally disrupted in all CMT2D sensory neurons during early disease stages. To unravel the significance of axonal transport impairments to CMT2D etiology, it will be important to assess the trafficking of a variety of different cargoes both in sensory and motor neurons of mutant Gars models. Given the complexity of the in vivo environment, the kinetics of axonal transport is not always replicated in vitro (Sleigh et al., 2017c), hence the analysis of mutant Gars mouse transport should also be extended to peripheral nerves in vivo (Gibbs et al., 2016, 2018).
GlyRS Elevation Is Not a Simple Compensatory Mechanism
Neuropathy-causing GARS1 mutations differentially impact the enzymatic activity, with some fully ablating it, whilst others having little effect (Oprescu et al., 2017). The charging function of GlyRSC201R and GlyRSP278KY in GarsC201R/+ and GarsNmf249/+ mice, respectively, were originally reported as unaffected (Seburn et al., 2006; Achilli et al., 2009; Stum et al., 2011). However, a re-evaluation under Michaelis-Menten kinetic conditions suggests that GlyRSP278KY has severely decreased kinetics and cannot support yeast viability, commensurate with a loss-of-function (Morelli et al., 2019). Moreover, GlyRSC201R aminoacylation was analyzed indirectly in brain lysates that had a 3.8-fold increase in GlyRS (Achilli et al., 2009), which could mask a charging deficiency. Accordingly, brains from severe homozygous GarsC201R/C201R mice showed a 60% decrease in aminoacylation despite an 8.2-fold increase in GlyRS. Further supporting a GlyRSC201R loss-of-function, wild-type GARS1 overexpression in sub-viable homozygous GarsC201R/C201R animals can restore post-natal viability (Motley et al., 2011). Similar to GarsC201R/+ brains, GlyRS levels were reported to be higher in GarsNmf249/+ cerebellum, although this was not quantified (Stum et al., 2011). It is, therefore, possible that GlyRS levels are elevated in CMT2D tissues as a compensatory response to diminished aminoacylation.
To test this hypothesis in sensory tissue, we analyzed the GlyRS protein in CMT2D DRG (Figure 5). Coinciding with the perturbation of sensory neuron fate, we observed enhanced GlyRS levels in lumbar, but not cervical, ganglia of mutant Gars mice. Furthermore, the increase was not observed in all lumbar sensory neurons, but preferentially in a portion of NF200+ neurons (Figure 6). This argues against GlyRS upregulation being a compensatory response to impaired charging, an alteration in a non-canonical function (e.g. Johanson et al., 2003; Park et al., 2012; Mo et al., 2016), or that mutant GlyRS protein stability is altered, because if any of those scenarios were true, then GlyRS increase would also likely occur in cervical DRG and across all sensory neurons equally. That is unless there is a greater requirement for glycine charging in cell bodies of larger sensory neurons with the longest axons (i.e., those innervating lower, but not upper, limbs). Contradictory to this idea, GlyRS levels were enhanced in only about a third of NF200+ neurons in mutant lumbar DRG, suggesting that a particular subset may be selectively impacted by the disease.
To further tease apart the basis for increased Gars expression, we assessed levels of additional ARS proteins. We found that LysRS remained unchanged, but that there was a small increase in TyrRS in CMT2D DRG (Figure 7). This indicates that there is no global increase in tRNA synthetase in response to GlyRSC201R expression. We, therefore, observe a GlyRS-specific upregulation, preferentially occurring in a subdivision of NF200+ neurons and only in ganglia that display neuropathology. Why might this be the case? Neuropathy-associated GARS1 mutations have been shown to impair GlyRS localization in neuron-like cell lines (Antonellis et al., 2006; Nangle et al., 2007), which could cause build-up in the soma, although, once again, if this were the cause of increased GlyRS levels then it would probably not be so selectively upregulated. The GlyRS elevation is only present in DRG that display a developmental perturbation in sensory neuron fate, suggesting that the two phenotypes may be linked. Perhaps the NF200+ neurons resident in lumbar ganglia are under stresses not experienced by neighboring subtypes. The integrated stress response (ISR), which is linked to amino acid deprivation (Pakos-Zebrucka et al., 2016), maybe especially activated in these cells. Consistent with impaired protein translation reported in CMT2D fly models (Niehues et al., 2015), the ISR causes a global downregulation of cap-dependent translation of mRNAs, except for a select few that possess upstream open reading frames (uORFs) in their 5′-UTRs, which under non-stressed conditions usually restrict translation initiation of the main downstream ORF (Barbosa et al., 2013). Although not classically thought of as an ISR-associated gene, human and mouse GARS1 express two mRNA isoforms, one of which possesses an uORF that may, under conditions of stress, play a role in the observed GlyRS increase (Alexandrova et al., 2015). However, some KARS1 variants also possess an uORF (AceView, NCBI) and LysRS levels remained unchanged. Alternatively, the increase may be an active, compensatory response by a subset of NF200+ cells to combat degeneration. Indeed, the NF200+ class of neurons includes vibration-sensing mechanoreceptors, which are most impacted in CMT2D patients (Sivakumar et al., 2005).
Conclusion
Sensory dysfunction of GARS1-neuropathy patients and mouse models of CMT2D is chronically understudied. This is unsurprising given the relative severity of motor symptoms; however, by studying pathology in both types of peripheral nerve and performing comparative anatomical studies on mouse motor and sensory nervous systems, we are much more likely to determine key pathomechanisms causing the selective pathology characteristic of CMT. Here, we have made four key discoveries: (1) sensory pathology is not equal across all CMT2D ganglia, thus anatomical location dictates disease involvement; (2) perturbed sensory neuron fate is not a general feature of different neuromuscular disease models, supporting its specificity to GARS1 neuropathy; (3) signaling endosome trafficking in a sub-population of GarsC201R/+ sensory neurons remains unaffected, indicating that a widespread CMT2D defect in axonal transport is unlikely; and (4) Gars expression is selectively enhanced in NF200+ lumbar DRG neurons and is thus linked to the subtype switch, perhaps in response to active degeneration.
Data Availability Statement
The raw data supporting the conclusions of this article will be made available by the authors, without undue reservation.
Ethics Statement
The animal study was reviewed and approved by the UCL Queen Square Institute of Neurology Genetic Manipulation and Ethics Committees and performed in accordance with the European Community Council Directive of 24 November 1986 (86/609/EEC). GarsC201R/+ (RRID:MGI 3849420) and SOD1G93A (RRID:IMSR_JAX 002726) mouse handling and experiments were carried out under license from the UK Home Office in accordance with the Animals (Scientific Procedures) Act 1986 and were approved by the UCL Queen Square Institute of Neurology Ethical Review Committee.
Author Contributions
JS conceived the experiments, analyzed the data and wrote the manuscript. JS, AM, TA, and YZ performed the research. GS provided expertise and discussion. All authors contributed to the article and approved the submitted version.
Funding
This work was supported by the Medical Research Council Career Development Award (MR/S006990/1; JS), the Wellcome Trust Sir Henry Wellcome Postdoctoral Fellowship (103191/Z/13/Z; JS), the Wellcome Trust Senior Investigator Award (107116/Z/15/Z; GS), the European Union’s Horizon 2020 Research and Innovation Programme under grant agreement 739572 (GS), and the UK Dementia Research Institute Foundation award (UKDRI-1005; GS).
Conflict of Interest
The authors declare that the research was conducted in the absence of any commercial or financial relationships that could be construed as a potential conflict of interest.
Acknowledgments
We would like to thank Drs. Emily Spaulding and Robert Burgess (The Jackson Laboratory, Bar Harbor, ME, USA) for providing GarsNmf249/+ tissue and commenting on the manuscript, James Dick (University College London) for genotyping the P100-101 SODG93A and wild-type mice, and Drs. Alexander M. Rossor and David Villarroel-Campos (University College London) for critical comments on the manuscript.
Footnotes
Supplementary Material
The Supplementary Material for this article can be found online at: https://www.frontiersin.org/articles/10.3389/fncel.2020.00232/full#supplementary-material.
References
Achilli, F., Bros-Facer, V., Williams, H. P., Banks, G. T., AlQatari, M., Chia, R., et al (2009). An ENU-induced mutation in mouse glycyl-tRNA synthetase (GARS) causes peripheral sensory and motor phenotypes creating a model of charcot-marie-tooth type 2d peripheral neuropathy. Dis. Model. Mech. 2, 359–373. doi: 10.1242/dmm.002527
Alexandrova, J., Paulus, C., Rudinger-Thirion, J., Jossinet, F., and Frugier, M. (2015). Elaborate uORF/IRES features control expression and localization of human glycyl-tRNA synthetase. RNA Biol. 12, 1301–1313. doi: 10.1080/15476286.2015.1086866
Antonellis, A., Ellsworth, R. E., Sambuughin, N., Puls, I., Abel, A., Lee-Lin, S.-Q., et al (2003). Glycyl tRNA synthetase mutations in charcot-marie-tooth disease type 2d and distal spinal muscular atrophy type V. Am. J. Hum. Genet. 72, 1293–1299. doi: 10.1086/375039
Antonellis, A., Goldfarb, L. G., and Sivakumar, K. (2018). “GARS1-associated axonal neuropathy,” in GeneReviews®, eds. M. P., Adam, H. H., Ardinger, R. A., Pagon, S. E., Wallace, L. J. H., Bean, H. C., Mefford et al. (Seattle, WA: University of Washington), 1993–2020.
Antonellis, A., Lee-Lin, S.-Q., Wasterlain, A., Leo, P., Quezado, M., Goldfarb, L. G., et al (2006). Functional analyses of glycyl-tRNA synthetase mutations suggest a key role for tRNA-charging enzymes in peripheral axons. J. Neurosci. 26, 10397–10406. doi: 10.1523/jneurosci.1671-06.2006
Barbosa, C., Peixeiro, I., and Romão, L. (2013). Gene expression regulation by upstream open reading frames and human disease. PLoS Genet. 9:e1003529. doi: 10.1371/journal.pgen.1003529
Beijer, D., Sisto, A., Van Lent, J., Baets, J., and Timmerman, V. (2019). Defects in axonal transport in inherited neuropathies. J. Neuromuscul. Dis. 6, 401–419. doi: 10.3233/jnd-190427
Benoy, V., Van Helleputte, L., Prior, R., d’Ydewalle, C., Haeck, W., Geens, N., et al (2018). HDAC6 is a therapeutic target in mutant GARS-induced charcot-marie-tooth disease. Brain 141, 673–687. doi: 10.1093/brain/awx375
Boczonadi, V., Jennings, M. J., and Horvath, R. (2018). The role of tRNA synthetases in neurological and neuromuscular disorders. FEBS Lett. 592, 703–717. doi: 10.1002/1873-3468.12962
Crawley, J. N., Belknap, J. K., Collins, A., Crabbe, J. C., Frankel, W., Henderson, N., et al (1997). Behavioral phenotypes of inbred mouse strains: implications and recommendations for molecular studies. Psychopharmacology 132, 107–124. doi: 10.1007/s002130050327
Deinhardt, K., Reversi, A., Berninghausen, O., Hopkins, C. R., and Schiavo, G. (2007). Neurotrophins redirect p75NTR from a clathrin-independent to a clathrin-dependent endocytic pathway coupled to axonal transport. Traffic 8, 1736–1749. doi: 10.1111/j.1600-0854.2007.00645.x
Deinhardt, K., Salinas, S., Verastegui, C., Watson, R., Worth, D., Hanrahan, S., et al (2006). Rab5 and Rab7 control endocytic sorting along the axonal retrograde transport pathway. Neuron 52, 293–305. doi: 10.3410/f.1052837.504763
Dombert, B., Balk, S., Lüningschrör, P., Moradi, M., Sivadasan, R., Saal-Bauernschubert, L., et al (2017). BDNF/trkB induction of calcium transients through Cav2.2 calcium channels in motoneurons corresponds to F-actin assembly and growth cone formation on β2-chain laminin (221). Front. Mol. Neurosci. 10:346. doi: 10.3389/fnmol.2017.00346
Forrester, N., Rattihalli, R., Horvath, R., Maggi, L., Manzur, A., Fuller, G., et al (2020). Clinical and genetic features in a series of eight unrelated patients with neuropathy due to glycyl-tRNA synthetase (GARS) variants. J. Neuromuscul. Dis. 7, 137–143. doi: 10.3233/jnd-200472
Gibbs, K. L., Kalmar, B., Rhymes, E. R., Fellows, A. D., Ahmed, M., Whiting, P., et al (2018). Inhibiting p38 MAPK alpha rescues axonal retrograde transport defects in a mouse model of ALS. Cell Death Dis. 9:596. doi: 10.1038/s41419-018-0624-8
Gibbs, K. L., Kalmar, B., Sleigh, J. N., Greensmith, L., and Schiavo, G. (2016). In vivo imaging of axonal transport in murine motor and sensory neurons. J. Neurosci. Methods 257, 26–33. doi: 10.1016/j.jneumeth.2015.09.018
Grice, S. J., Sleigh, J. N., and Cader, M. Z. (2018). Plexin-semaphorin signaling modifies neuromuscular defects in a Drosophila model of peripheral neuropathy. Front. Mol. Neurosci. 11:55. doi: 10.3389/fnmol.2018.00055
Grice, S. J., Sleigh, J. N., Motley, W. W., Liu, J.-L., Burgess, R. W., Talbot, K., et al (2015). Dominant, toxic gain-of-function mutations in gars lead to non-cell autonomous neuropathology. Hum. Mol. Genet. 24, 4397–4406. doi: 10.1093/hmg/ddv176
Guedes-Dias, P., and Holzbaur, E. L. F. (2019). Axonal transport: driving synaptic function. Science 366:eaaw9997. doi: 10.1126/science.aaw9997
Gurney, M. E., Pu, H., Chiu, A. Y., Dal Canto, M. C., Polchow, C. Y., Alexander, D. D., et al (1994). Motor neuron degeneration in mice that express a human cu, zn superoxide dismutase mutation. Science 264, 1772–1775. doi: 10.1126/science.7985031
He, W., Bai, G., Zhou, H., Wei, N., White, N. M., Lauer, J., et al (2015). CMT2D neuropathy is linked to the neomorphic binding activity of glycyl-tRNA synthetase. Nature 526, 710–714. doi: 10.1038/nature15510
He, W., Zhang, H.-M., Chong, Y. E., Guo, M., Marshall, A. G., and Yang, X.-L. (2011). Dispersed disease-causing neomorphic mutations on a single protein promote the same localized conformational opening. Proc. Natl. Acad. Sci. U S A 108, 12307–12312. doi: 10.1073/pnas.1104293108
Huang, E. J., and Reichardt, L. F. (2003). Trk receptors: roles in neuronal signal transduction. Annu. Rev. Biochem. 72, 609–642. doi: 10.1146/annurev.biochem.72.121801.161629
Ibba, M., and Soll, D. (2000). Aminoacyl-tRNA synthesis. Annu. Rev. Biochem. 69, 617–650. doi: 10.1146/annurev.biochem.69.1.617
Johanson, K., Hoang, T., Sheth, M., and Hyman, L. E. (2003). GRS1, a yeast tRNA synthetase with a role in mRNA 3’ end formation. J. Biol. Chem. 278, 35923–35930. doi: 10.1074/jbc.m304978200
Kim, R. B., Irvin, C. W., Tilva, K. R., and Mitchell, C. S. (2015). State of the field: An informatics-based systematic review of the SOD1–G93 a amyotrophic lateral sclerosis transgenic mouse model. Amyotroph. Lateral Scler. Frontotemporal Degener. 17, 1–14. doi: 10.3109/21678421.2015.1047455
Lai, H. C., Seal, R. P., and Johnson, J. E. (2016). Making sense out of spinal cord somatosensory development. Development 143, 3434–3448. doi: 10.1242/dev.139592
Lawson, S. N., and Biscoe, T. J. (1979). Development of mouse dorsal root ganglia: an autoradiographic and quantitative study. J. Neurocytol. 8, 265–274. doi: 10.1007/bf01236122
Ma, Q., Fode, C., Guillemot, F., and Anderson, D. J. (1999). Neurogenin1 and neurogenin2 control two distinct waves of neurogenesis in developing dorsal root ganglia. Genes Dev. 13, 1717–1728. doi: 10.1101/gad.13.13.1717
Mattedi, F., and Vagnoni, A. (2019). Temporal control of axonal transport: the extreme case of organismal ageing. Front. Cell. Neurosci. 13:393. doi: 10.3389/fncel.2019.00393
Meyer-Schuman, R., and Antonellis, A. (2017). Emerging mechanisms of aminoacyl-tRNA synthetase mutations in recessive and dominant human disease. Hum. Mol. Genet. 26, R114–R127. doi: 10.1093/hmg/ddx231
Mo, Z., Zhang, Q., Liu, Z., Lauer, J., Shi, Y., Sun, L., et al (2016). Neddylation requires glycyl-tRNA synthetase to protect activated E2. Nat. Struct. Mol. Biol. 23, 730–737. doi: 10.1038/nsmb.3250
Mo, Z., Zhao, X., Liu, H., Hu, Q., Chen, X.-Q., Pham, J., et al (2018). Aberrant GlyRS-HDAC6 interaction linked to axonal transport deficits in charcot-marie-tooth neuropathy. Nat. Commun. 9:1007. doi: 10.1038/s41467-018-03461-z
Mohan, R., Tosolini, A. P., and Morris, R. (2014). Targeting the motor end plates in the mouse hindlimb gives access to a greater number of spinal cord motor neurons: an approach to maximize retrograde transport. Neuroscience 274, 318–330. doi: 10.1016/j.neuroscience.2014.05.045
Montaño, J. A., Pérez-Piñera, P., García-Suárez, O., Cobo, J., and Vega, J. A. (2010). Development and neuronal dependence of cutaneous sensory nerve formations: lessons from neurotrophins. Microsc. Res. Tech. 73, 513–529. doi: 10.1002/jemt.20790
Morelli, K. H., Griffin, L. B., Pyne, N. K., Wallace, L. M., Fowler, A. M., Oprescu, S. N., et al (2019). Allele-specific RNA interference prevents neuropathy in charcot-marie-tooth disease type 2d mouse models. J. Clin. Invest. 129, 5568–5583. doi: 10.1172/JCI130600
Motley, W. W., Seburn, K. L., Nawaz, M. H., Miers, K. E., Cheng, J., Antonellis, A., et al (2011). Charcot-marie-tooth-linked mutant GARS is toxic to peripheral neurons independent of wild-type GARS levels. PLoS Genet. 7:e1002399. doi: 10.1371/journal.pgen.1002399
Nangle, L. A., Zhang, W., Xie, W., Yang, X.-L., and Schimmel, P. (2007). Charcot-marie-tooth disease-associated mutant tRNA synthetases linked to altered dimer interface and neurite distribution defect. Proc. Natl. Acad. Sci. U S A 104, 11239–11244. doi: 10.1073/pnas.0705055104
Nardo, G., Trolese, M. C., Tortarolo, M., Vallarola, A., Freschi, M., Pasetto, L., et al (2016). New insights on the mechanisms of disease course variability in ALS from mutant SOD1 mouse models. Brain Pathol. 26, 237–247. doi: 10.1111/bpa.12351
Nascimento, A. I., Mar, F. M., and Sousa, M. M. (2018). The intriguing nature of dorsal root ganglion neurons: linking structure with polarity and function. Prog. Neurobiol. 168, 86–103. doi: 10.1016/j.pneurobio.2018.05.002
Niehues, S., Bussmann, J., Steffes, G., Erdmann, I., Köhrer, C., Sun, L., et al (2015). Impaired protein translation in Drosophila models for charcot-marie-tooth neuropathy caused by mutant tRNA synthetases. Nat. Commun. 6:7520. doi: 10.1038/ncomms8520
Ochs, S., Erdman, J., Jersild, R. A., and McAdoo, V. (1978). Routing of transported materials in the dorsal root and nerve fiber branches of the dorsal root ganglion. J. Neurobiol. 9, 465–481. doi: 10.1002/neu.480090606
Oprescu, S. N., Griffin, L. B., Beg, A. A., and Antonellis, A. (2017). Predicting the pathogenicity of aminoacyl-tRNA synthetase mutations. Methods 113, 139–151. doi: 10.1016/j.ymeth.2016.11.013
Pakos-Zebrucka, K., Koryga, I., Mnich, K., Ljujic, M., Samali, A., and Gorman, A. M. (2016). The integrated stress response. EMBO Rep. 17, 1374–1395. doi: 10.15252/embr.201642195
Park, M. C., Kang, T., Jin, D., Han, J. M., Kim, S. B., Park, Y. J., et al (2012). Secreted human glycyl-tRNA synthetase implicated in defense against ERK-activated tumorigenesis. Proc. Natl. Acad. Sci. U S A 109, E640–E647. doi: 10.1073/pnas.1200194109
Park, S.-R., Kim, H.-J., Yang, S.-R., Park, C. H., Lee, H.-Y., and Hong, I.-S. (2018). A novel endogenous damage signal, glycyl tRNA synthetase, activates multiple beneficial functions of mesenchymal stem cells. Cell Death Differ. 25, 2023–2036. doi: 10.1038/s41418-018-0099-2
Pipis, M., Rossor, A. M., Laura, M., and Reilly, M. M. (2019). Next-generation sequencing in charcot-marie-tooth disease: opportunities and challenges. Nat. Rev. Neurol. 15, 644–656. doi: 10.1038/s41582-019-0254-5
Pisciotta, C., and Shy, M. E. (2018). Neuropathy. Handb. Clin. Neurol. 148, 653–665. doi: 10.1016/B978-0-444-64076-5.00042-9
Prior, R., Van Helleputte, L., Benoy, V., and Van Den Bosch, L. (2017). Defective axonal transport: a common pathological mechanism in inherited and acquired peripheral neuropathies. Neurobiol. Dis. 105, 300–320. doi: 10.1016/j.nbd.2017.02.009
Reilly, M. M., Murphy, S. M., and Laurá, M. (2011). Charcot-marie-tooth disease. J. Peripher. Nerv. Syst. 16, 1–14. doi: 10.1111/j.1529-8027.2011.00324.x
Rigaud, M., Gemes, G., Barabas, M.-E., Chernoff, D. I., Abram, S. E., Stucky, C. L., et al (2008). Species and strain differences in rodent sciatic nerve anatomy: implications for studies of neuropathic pain. Pain 136, 188–201. doi: 10.1016/j.pain.2008.01.016
Rossor, A. M., Polke, J. M., Houlden, H., and Reilly, M. M. (2013). Clinical implications of genetic advances in charcot-marie-tooth disease. Nat. Rev. Neurol. 9, 562–571. doi: 10.1038/nrneurol.2013.179
Rossor, A. M., Sleigh, J. N., Groves, M., Muntoni, F., Reilly, M. M., Hoogenraad, C. C., et al (2020). Loss of BICD2 in muscle drives motor neuron loss in a developmental form of spinal muscular atrophy. Acta Neuropathol. Commun. 8:34. doi: 10.1186/s40478-020-00909-6
Sajic, M., Mastrolia, V., Lee, C. Y., Trigo, D., Sadeghian, M., Mosley, A. J., et al (2013). Impulse conduction increases mitochondrial transport in adult mammalian peripheral nerves in vivo. PLoS Biol. 11:e1001754. doi: 10.1371/journal.pbio.1001754
Sassone, J., Taiana, M., Lombardi, R., Porretta-Serapiglia, C., Freschi, M., Bonanno, S., et al (2016). ALS mouse model SOD1G93A displays early pathology of sensory small fibers associated to accumulation of a neurotoxic splice variant of peripherin. Hum. Mol. Genet. 25, 1588–1599. doi: 10.1093/hmg/ddw035
Seburn, K. L., Nangle, L. A., Cox, G. A., Schimmel, P., and Burgess, R. W. (2006). An active dominant mutation of glycyl-tRNA synthetase causes neuropathy in a charcot-marie-tooth 2d mouse model. Neuron 51, 715–726. doi: 10.3410/f.1047563.497530
Seki, S., Yamamoto, T., Quinn, K., Spigelman, I., Pantazis, A., Olcese, R., et al (2019). Circuit-specific early impairment of proprioceptive sensory neurons in the SOD1G93A mouse model for ALS. J. Neurosci. 39, 8798–8815. doi: 10.1523/jneurosci.1214-19.2019
Shorrock, H. K., van der Hoorn, D., Boyd, P. J., Llavero Hurtado, M., Lamont, D. J., Wirth, B., et al (2018). UBA1/GARS-dependent pathways drive sensory-motor connectivity defects in spinal muscular atrophy. Brain 141, 2878–2894. doi: 10.1093/brain/awy237
Sivakumar, K., Kyriakides, T., Puls, I., Nicholson, G. A., Funalot, B., Antonellis, A., et al (2005). Phenotypic spectrum of disorders associated with glycyl-tRNA synthetase mutations. Brain 128, 2304–2314. doi: 10.1093/brain/awh590
Sleigh, J. N., and Schiavo, G. (2016). Older but not slower: aging does not alter axonal transport dynamics of signalling endosomes in vivo. Matters 201605000018. doi: 10.19185/matters.201605000018
Sleigh, J. N., Burgess, R. W., Gillingwater, T. H., and Cader, M. Z. (2014a). Morphological analysis of neuromuscular junction development and degeneration in rodent lumbrical muscles. J. Neurosci. Methods 227, 159–165. doi: 10.1016/j.jneumeth.2014.02.005
Sleigh, J. N., Dawes, J. M., West, S. J., Wei, N., Spaulding, E. L., Gómez-Martín, A., et al (2017a). Trk receptor signaling and sensory neuron fate are perturbed in human neuropathy caused by Gars mutations. Proc. Natl. Acad. Sci. USA 114, E3324–E3333. doi: 10.1073/pnas.1614557114
Sleigh, J. N., Gómez-Martín, A., Wei, N., Bai, G., Yang, X.-L., and Schiavo, G. (2017b). Neuropilin 1 sequestration by neuropathogenic mutant glycyl-tRNA synthetase is permissive to vascular homeostasis. Sci. Rep. 7:9216. doi: 10.1038/s41598-017-10005-w
Sleigh, J. N., Grice, S. J., Burgess, R. W., Talbot, K., and Cader, M. Z. (2014b). Neuromuscular junction maturation defects precede impaired lower motor neuron connectivity in charcot-marie-tooth type 2d mice. Hum. Mol. Genet. 23, 2639–2650. doi: 10.1093/hmg/ddt659
Sleigh, J. N., Rossor, A. M., Fellows, A. D., Tosolini, A. P., and Schiavo, G. (2019). Axonal transport and neurological disease. Nat. Rev. Neurol. 15, 691–703. doi: 10.1038/s41582-019-0257-2
Sleigh, J. N., Mech, A. M., and Schiavo, G. (2020). Developmental demands contribute to early neuromuscular degeneration in CMT2D mice. Cell Death Dis. 11:564. doi: 10.1038/s41419-020-02798-y
Sleigh, J. N., Tosolini, A. P., Gordon, D., Devoy, A., Fratta, P., Fisher, E. M. C., et al (2020a). Mice carrying ALS mutant TDP-43, but not mutant FUS, display in vivo defects in axonal transport of signaling endosomes. Cell Rep. 30, 3655–3662.e2. doi: 10.1016/j.celrep.2020.02.078
Sleigh, J. N., Vagnoni, A., Twelvetrees, A. E., and Schiavo, G. (2017c). Methodological advances in imaging intravital axonal transport. F1000Res. 6:200. doi: 10.12688/f1000research.10433.1
Sleigh, J. N., Weir, G. A., and Schiavo, G. (2016). A simple, step-by-step dissection protocol for the rapid isolation of mouse dorsal root ganglia. BMC Res. Notes 9:82. doi: 10.1186/s13104-016-1915-8
Sleigh, J. N., West, S. J., and Schiavo, G. (2020b). A video protocol for rapid dissection of mouse dorsal root ganglia from defined spinal levels. BMC Res. Notes 13:302. doi: 10.21203/rs.3.rs-25927/v1
Spaulding, E. L., Sleigh, J. N., Morelli, K. H., Pinter, M. J., Burgess, R. W., and Seburn, K. L. (2016). Synaptic deficits at neuromuscular junctions in two mouse models of charcot-marie-tooth type 2d. J. Neurosci. 36, 3254–3267. doi: 10.1523/jneurosci.1762-15.2016
Stum, M., McLaughlin, H. M., Kleinbrink, E. L., Miers, K. E., Ackerman, S. L., Seburn, K. L., et al (2011). An assessment of mechanisms underlying peripheral axonal degeneration caused by aminoacyl-tRNA synthetase mutations. Mol. Cell. Neurosci. 46, 432–443. doi: 10.1016/j.mcn.2010.11.006
Tosolini, A. P., Mohan, R., and Morris, R. (2013). Targeting the full length of the motor end plate regions in the mouse forelimb increases the uptake of fluoro-gold into corresponding spinal cord motor neurons. Front. Neurol. 4:58. doi: 10.3389/fneur.2013.00058
Vaughan, S. K., Sutherland, N. M., Zhang, S., Hatzipetros, T., Vieira, F., and Valdez, G. (2018). The ALS-inducing factors, TDP43A315T and SOD1G93A, directly affect and sensitize sensory neurons to stress. Sci. Rep. 8:16582. doi: 10.1111/ejn.14682/v3/review1
Villarroel-Campos, D., Schiavo, G., and Lazo, O. M. (2018). The many disguises of the signalling endosome. FEBS Lett. 592, 3615–3632. doi: 10.1002/1873-3468.13235
Wang, T., Martin, S., Nguyen, T. H., Harper, C. B., Gormal, R. S., Martínez-Mármol, R., et al (2016). Flux of signalling endosomes undergoing axonal retrograde transport is encoded by presynaptic activity and TrkB. Nat. Commun. 7:12976. doi: 10.1038/ncomms12976
Wei, N., Zhang, Q., and Yang, X.-L. (2019). Neurodegenerative charcot-marie-tooth disease as a case study to decipher novel functions of aminoacyl-tRNA synthetases. J. Biol. Chem. 294, 5321–5339. doi: 10.1074/jbc.rev118.002955
Keywords: aminoacyl-tRNA synthetase (ARS), amyotrophic lateral sclerosis (ALS), axonal transport, Charcot-Marie-Tooth disease (CMT), glycyl-tRNA synthetase (GlyRS), neurotrophin receptors (Trks), sensory neuron, signaling endosome
Citation: Sleigh JN, Mech AM, Aktar T, Zhang Y and Schiavo G (2020) Altered Sensory Neuron Development in CMT2D Mice Is Site-Specific and Linked to Increased GlyRS Levels. Front. Cell. Neurosci. 14:232. doi: 10.3389/fncel.2020.00232
Received: 27 April 2020; Accepted: 01 July 2020;
Published: 11 August 2020.
Edited by:
David J. Adams, University of Wollongong, AustraliaReviewed by:
Sinead Madeleine Murphy, Tallaght University Hospital, IrelandAlbena Jordanova, University of Antwerp, Belgium
Copyright © 2020 Sleigh, Mech, Aktar, Zhang and Schiavo. This is an open-access article distributed under the terms of the Creative Commons Attribution License (CC BY). The use, distribution or reproduction in other forums is permitted, provided the original author(s) and the copyright owner(s) are credited and that the original publication in this journal is cited, in accordance with accepted academic practice. No use, distribution or reproduction is permitted which does not comply with these terms.
*Correspondence: James N. Sleigh, ai5zbGVpZ2hAdWNsLmFjLnVr
†ORCID: James N. Sleigh orcid.org/0000-0002-3782-9045
Yuxin Zhang orcid.org/0000-0003-0243-2520
Giampietro Schiavo orcid.org/0000-0002-4319-8745