- 1Spinal Cord and Brain Injury Research Group, Stark Neurosciences Research Institute, Indiana University School of Medicine, Indianapolis, IN, United States
- 2Department of Neurological Surgery, Indiana University School of Medicine, Indianapolis, IN, United States
The benefits of transplanting cultured Schwann cells (SCs) for the treatment of spinal cord injury (SCI) have been systematically investigated in experimental animals since the early 1990s. Importantly, human SC (hSC) transplantation for SCI has advanced to clinical testing and safety has been established via clinical trials conducted in the USA and abroad. However, multiple barriers must be overcome to enable accessible and effective treatments for SCI patients. This review presents available information on hSC transplantation for SCI with the intention to uncover gaps in our knowledge and discuss areas for future development. To this end, we introduce the historical progression of the work that supports existing and prospective clinical initiatives and explain the reasons for the choice of hSCs while also addressing their limitations as cell therapy products. A search of the relevant literature revealed that rat SCs have served as a preclinical model of reference since the onset of investigations, and that hSC transplants are relatively understudied, possibly due to the sophisticated resources and expertise needed for the traditional processing of hSC cultures from human nerves. In turn, we reason that additional experimentation and a reexamination of the available data are needed to understand the therapeutic value of hSC transplants taking into consideration that the manufacturing of the hSCs themselves may require further development for extended uses in basic research and clinical settings.
Introduction
Over the past few decades, much progress has been made in the understanding of the pathology and molecular mechanisms of spinal cord injuries (SCI) and the design of assorted repair strategies in experimental animals. However, developing effective treatments for humans remains a challenge. Trauma to the spinal cord often results in the formation of fluid-filled cavities and the development of astrogliotic or fibrotic scar tissue that altogether create an inhibitory environment to axonal regeneration. For this reason, the addition of cells has been chosen as the main strategy to fill the cystic cavities, reduce scarring, and ultimately support axon regrowth. Schwann cells (SCs) are one of the most studied cell types for such purposes (reviewed in Tetzlaff et al., 2011; Bunge and Wood, 2012; Deng et al., 2015a). SCs are neuroglial cells that not only drive axon regeneration and myelination in the peripheral nervous system (PNS) but also perform an analogous function when transplanted into the spinal cord. In addition, SCs can be isolated from a patient’s own nerve and expanded in vitro prior to implantation, which makes them an outstanding cell type for autotransplantation therapy in SCI (Guest et al., 2013).
SCs have been transplanted experimentally for >40 years to exploit their proregenerative and myelinating functions in traumatic and demyelinating lesions in the PNS and central nervous system (CNS) (Blakemore, 1977; Duncan et al., 1981; Kohama et al., 2001). A long-standing history of work, including clinical investigations initiated over a decade ago, has established that SC grafting for spinal cord repair is translatable to humans. To date, studies involving the delivery of hSC transplants alone (Saberi et al., 2008, 2011; Zhou et al., 2012; Anderson et al., 2017; Gant et al., 2021) and together with other cell types (Chen et al., 2014; Oraee-Yazdani et al., 2016) have indicated no undesirable effects in human participants of clinical trials. Safety measures and cell dosage in patients with subacute and chronic SCI have been addressed via USA-Food and Drug Administration (FDA)-sanctioned trials (Anderson et al., 2017; Gant et al., 2021). Nevertheless, there are basic questions about the use of hSCs that have yet to be answered, as most supportive data for a role of SCs in SCI repair have been gathered using rodent cells mainly from rats (Tetzlaff et al., 2011). While rodent SCs have been regarded as useful in basic and preclinical studies, they may be insufficient to obtain conclusive information relevant to humans, as it is becoming increasingly clear that hSCs have special characteristics and only partially mimic the rodent counterparts (reviewed in Monje, 2020).
Therefore, the goal of this review is to evaluate current knowledge of hSC transplantation for SCI in an effort to identify prospects and challenges relevant to translational medicine. Research on rodent SC transplantation has been systematically reviewed and will not be described here in detail (for reference, see Oudega and Xu, 2006; Tetzlaff et al., 2011; Bunge and Wood, 2012; Deng et al., 2015a). In the sections below, we have explained the rationale for the choice of hSCs to repair CNS injuries starting with a description of the pioneering studies that supported the cell therapy strategy. We have addressed the advantages and limitations of hSC-based therapies, analyzed the available literature to uncover gaps in our knowledge, and discussed areas for future development or exploration. The ultimate goal of this review is to highlight some of the outstanding questions that need to be resolved to maximize treatments for human SCI, considering that the therapeutic approaches will likely evolve as new information is gained from basic and clinical work.
Why Use Transplants of Cultured SCs for SCI?
Although SCs do not normally reside in the CNS, there is a strong rationale for using them in spinal cord repair because they provide a substrate for axonal growth and a means of replacing myelin (section “Therapeutic Action”). SCs are an abundant and accessible cell type amenable for culturing under Good Manufacturing Practices (GMP) and autologous introduction into the human body, thus alleviating the need for chronic immunosuppression (Bunge et al., 2017).
Therapeutically, SCs can be delivered in the following two essentially different modalities: (1) peripheral nerve grafts, i.e., undissociated cells within their connective tissue layers, and (2) purified cells in suspension obtained via in vitro culture techniques (Figure 1). Whereas uncultured tissue grafts have been chosen for nerve replacement in PNS lesions and neuroprotection in the brain (El Seblani et al., 2020), there is precedent for nerve autografting in the spinal cord of humans. Cultured SCs are preferred for the treatment of spinal cord trauma (Guest et al., 2013) and demyelinating diseases (Kohama et al., 2001) because isolated cells cause less damage during implantation and in turn, they give rise to a new and stable bridging tissue that usually adjusts well to the unique size and shape of spinal cord lesions (Guest et al., 2013).
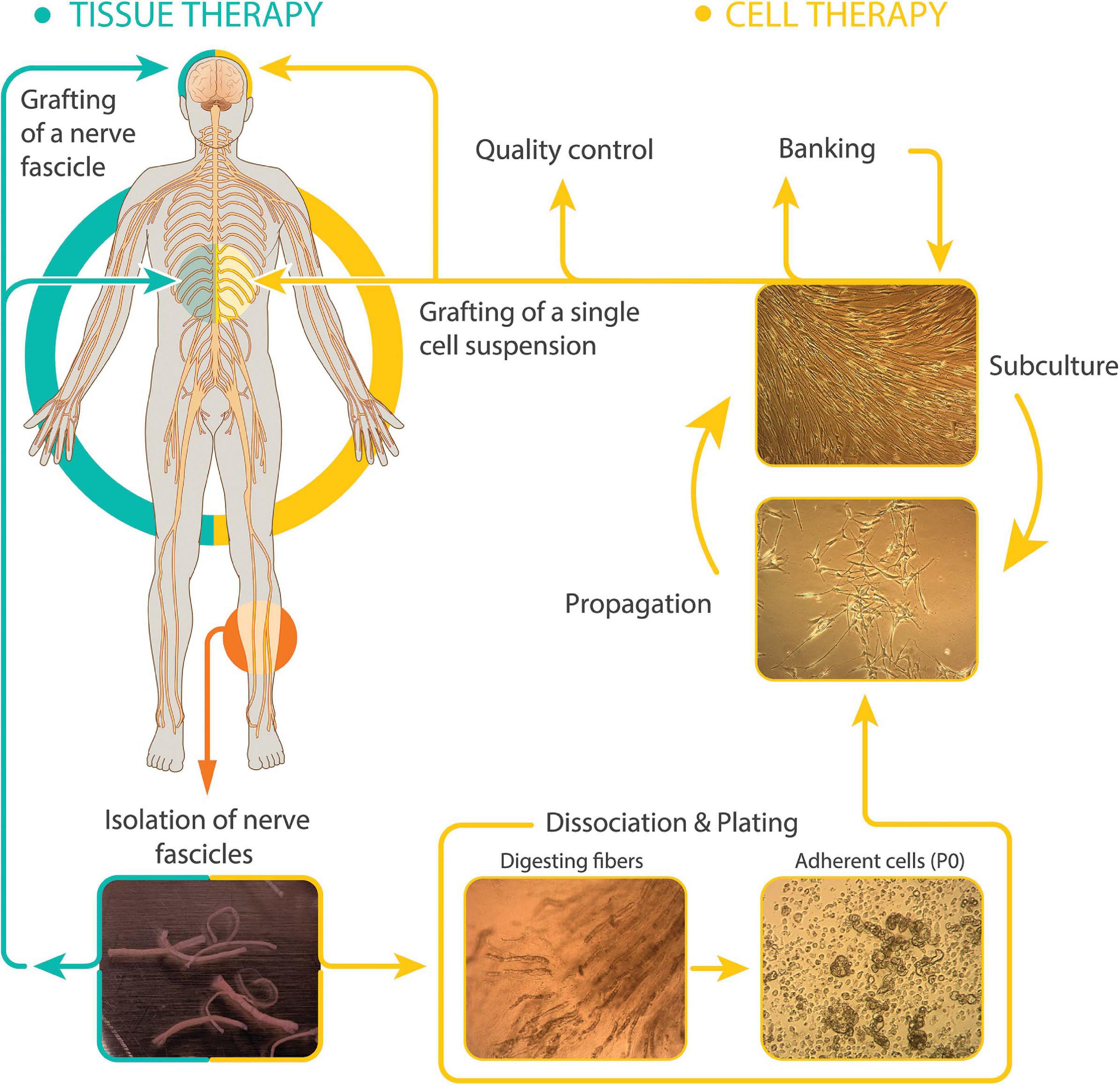
Figure 1. Modalities of hSC-based autotransplantation therapies for neuroprotection and regeneration in the CNS. Most clinical initiatives in SCI and other paradigms have used autologous cultured (right, cell therapy) or uncultured (left, tissue therapy) hSCs from sural nerve delivered within or around the injury area (diagram). The established hSCs are usually purified and expanded before collecting them as a single cell suspension for implantation. Delayed dissociation of pre-degenerated (cultured) nerve fibers induces SC activation and enhances recovery of cells at passage-zero (P0). Representative images of dissected sural nerve fascicles in culture medium (bottom left) and intermediate steps in the isolation and culturing of hSCs are shown. Culture is usually carried out up to passage-2 to generate hSCs ready to transplant and stocks for banking. Digested fibers: nerve fascicles during enzymatic dissociation. Adherent cells: primary cells right after isolation showing abundant myelin debris. Propagation (with mitogenic factors) and subculture: purified hSCs at low (lower image, subconfluent cells) and high density (upper image, confluent cells).
The feasibility of implementing a hSC-based therapy using cultured cells was evidenced when methodological advancements made it possible to isolate and propagate hSCs from the nerve fascicles of adult donors to the high numbers needed for grafting. Once established in vitro, normal hSC populations conserve essential SC characteristics (Levi et al., 1995; Rutkowski et al., 1995; Casella et al., 1996; Weiss et al., 2016) and their capacity for undesirable differentiation or aberrant (cancer) growth is extremely low (section “Safety”). hSCs can be cryopreserved without loss of viability (Kohama et al., 2001; Bastidas et al., 2017) and genetically modified ex-vivo to enhance their potency or enable visualization post-transplantation (Haastert et al., 2007). While SC cultures from multiple sources have been examined in preclinical SCI research, the ones from mature (adult) nerves are preferred (sections “Safety” and “Types of Transplantable hSCs”), despite their limitations (section “Limitations of hSC Cultures”).
Therapeutic Action
Mature SCs are endowed with remarkable plasticity. They rapidly convert into proliferative cells, often referred to as repair or activated SCs, in response to nerve (axonal) degeneration (Jessen and Mirsky, 2019). Repair SCs create an environment that is both neuroprotective and permissive to axon regeneration by virtue of the trophic factors, extracellular matrix (ECM) molecules, and adhesion molecules they express on their surface or release into the environment (Jessen and Arthur-Farraj, 2019). SCs established in cell culture retain functional capacity for nerve repair and (re)myelination. Once transplanted in the injured CNS, they can reduce the size of the lesions, attract and guide descending and ascending axons into the implant, and modestly improve locomotor function without additional interventions (reviewed in Fortun et al., 2009; Bunge and Wood, 2012; Deng et al., 2015a). Most importantly, transplanted SCs provide ensheathment and a myelin sheath to newly regenerated propriospinal axons as well as demyelinated axons resulting from secondary damage in spinal cord lesions (Deng et al., 2015b).
Safety
SC-based transplants are typically safe. To date, most preclinical investigations on rodent and hSC transplantation have used cells from adult nerves, as it is anticipated that the individuals donating the tissue, and, in turn, receiving the cultured SCs, will mostly be adults (Tetzlaff et al., 2011; Bunge et al., 2017). Mature nerves comprise highly differentiated SCs in the form of ensheathing (Remak) and/or myelinating cells. It is worth mentioning that even though mature SCs dedifferentiate substantially after injury, they remain lineage-committed (unipotent) and cannot give rise to cells of an unrelated lineage (Jessen and Mirsky, 2019). SC precursors (SCPs) and neural crest cells are not evident in adult nerve tissues based on the data from rodents (Stierli et al., 2018). Although the cellular constitution of adult human nerves has not been investigated fully, it is unlikely that multipotent cells such as SCPs or stem cells are introduced in the culture workflow, which would be undesirable for transplantation due to the risk for uncontrolled growth or differentiation of the grafted cells.
Immortalization of SCs in vitro and lack of replicative senescence has been observed in cultures of rat SCs but not of human SCs with the consideration that immortalized rat SCs are often non-cancerous and able to maintain anchorage dependent growth (Mathon et al., 2001; Funk et al., 2007). Tumor formation by transplanted rat SCs has been reported for adult skin-derived (May et al., 2018) and postnatal nerve-derived SCs subjected to excessive passaging (Langford et al., 1988). Though these findings cannot be disregarded, the risk for acquired pluri- or multi-potency, transformation or unregulated growth of SCs pre- and post-transplantation are low regardless of whether the cells are derived from developing (embryonic, neonatal) or mature tissues. First, standard culture conditions are sufficient to allow undifferentiated SCPs to spontaneously give rise to SCs on schedule, resembling their differentiation process in vivo (Mirsky and Jessen, 2018). Second, hSC cultures are highly resistant to immortalize (Emery et al., 1999) and have a limited capacity to proliferate after being isolated from the nerves (Levi et al., 1995; Monje et al., 2018). No evidence of cancer development, excessive migration or mislocalization of the hSC transplants in contusive spinal cord lesions was found in preclinical investigations of donor nerve-derived hSCs established via standard methods (Bastidas et al., 2017). In sum, the stability of nerve-derived SC cultures, and the evidence indicating their safety for implantation, has greatly facilitated the clinical development of hSC-based cell and tissue therapies.
Types of Transplantable hSCs
The sources of therapeutic hSCs can be varied because mature and developing tissues such as nerves, ganglia, or roots are suitable for the culturing of normal primary hSCs regardless of their anatomical location and donor-related characteristics (Crawford and Armati-Gulson, 1982; Rutkowski et al., 1992; Casella et al., 1996; Weiss et al., 2016). Preexistent trauma to the spinal cord does not preclude the derivation of quality-grade hSC cultures from the sural nerve, a sensory nerve most often used for autologous hSC isolation in clinical trials because of the ease of access and tolerability to the donors (Saberi et al., 2011; Aghayan et al., 2012; Anderson et al., 2017). Recent work has underscored that SCs lose their potency for nerve repair in aged animals (Painter et al., 2014). Nevertheless, empirical observations have not revealed major difficulties with the procurement and expansion of hSCs from the nerves of older adults. Optimized protocols that use mitogenic factors to achieve expansion of hSCs in vitro allow the preparation of proliferative hSC cultures from humans aged 60 years and above (discussed in Monje, 2020). Whereas donor age affects the intrinsic growth rate of hSCs in culture, age is not a restrictive factor for the preparation of healthy, expandable hSC cultures from cadaveric and organ donor tissues (Boyer et al., 1994; Bastidas et al., 2017). An important barrier to mass producing adult nerve-derived hSC cultures for transplantation is the recovery of enough numbers of viable, adherent hSCs at passage-zero (Figure 1). However, recovering expandable hSCs from pre-degenerated sural nerve fibers is not an issue of concern when autologous >10 cm long nerve explants are used together with standardized protocols for tissue dissociation, cell plating, purification, and expansion (Bunge et al., 2017). Indeed, the possibility to derive hSC cultures from the sural nerve of patients from a wide range of ages has facilitated clinical translation of hSC autotransplantation therapies in general (discussed in Guest et al., 2013; Bunge et al., 2017).
The skin is another potential donor tissue for hSC derivation. In addition to its richness in mature SCs associated with nerve terminals, the skin concomitantly possesses a reservoir of immature skin-derived precursors (SKPs) of neural crest origin capable of giving rise to SCs via directed differentiation (Biernaskie et al., 2007). Extensive preclinical data support the feasibility to obtain SC cultures from SKPs (rodents) and use them for spinal cord repair in subacute (Sparling et al., 2015; May et al., 2018) and chronic SCI models in rodents (Assinck et al., 2020). hSC cultures from skin biopsies exhibit strong neuroregenerative properties and a nearly identical transcriptional signature with respect to those derived from nerves (Stratton et al., 2017). However, the transplantation SKP-SCs can lead to uncontrolled proliferation and the formation of masses in the spinal cord based on data from adult rodent cells (May et al., 2018). SKP-hSCs have not been tested clinically, but the choice of skin rather than nerves for hSC procurement has an obvious advantage from an ethical standpoint.
Lastly, hSCs can be generated in vitro from embryonic and induced pluripotent stem cells via stepwise differentiation, or from somatic cells such as fibroblasts via transdifferentiation or direct conversion (reviewed in Huang et al., 2020). SC-like cells prepared from bone marrow- or adipose tissue-derived mesenchymal stem cells offer promise for autologous therapies to repair damage in SCI and other forms of nervous system trauma (Hopf et al., 2020). The technologies to generate SC-like cells from unconventional sources are advancing swiftly but uses in human subjects are controversial due to the instability, heterogeneity, and likely tumorigenic potential of the resulting cellular products (Lehmann and Hoke, 2016). SC-like cells of human origin may be valuable for preclinical research to gather patient-oriented data because they can be used as cellular models for genetic or pharmacological studies, toxicity screens, or other investigative approaches that can be accomplished without major ethical concerns or risks to the patients. A few noncancerous hSC lines have been created (Lehmann et al., 2012) but their use in transplantation and other functional studies is not generally recommended.
Limitations of hSC Cultures
As summarized in Table 1 and discussed in other sections, the choice of hSCs from adult nerves also encompass limitations related to the properties of the cells themselves, the biomanufacturing methods used to prepare them, and the efficacy of the transplants (section “The Knowledge Gap in our Understanding of hSCs”). Purified SCs propagated in vitro are manipulated cells irrespective of their origin and mode of preparation. Consequently, multiple steps of quality control are needed to ensure the product is suitable for human use. For this reason, the hSCs intended to be used in participants of FDA-regulated clinical trials are scrutinized extensively to confirm their identity, purity, viability, stability and sterility prior to transplantation, as well as the absence of residual components from the manufacturing process (Anderson et al., 2017). In addition, the mode of action of hSCs in nerve repair is complex, and the resulting populations often vary from donor to donor and possibly also with passaging, which makes determination of potency (biological activity) quite challenging via in vitro and in vivo assessments.
The Temporal Gap Between Basic and Clinical Science: From Rodents to Humans
The history of work on SC transplants is long-standing. By the early 1980s, it was clear that peripheral nerve tissues were supportive of long-distance axon regeneration from central neurons (Richardson et al., 1980). At that time, it was also feasible to recapitulate at least two of the constituents of the peripheral nerve that had an influence on axonal growth, i.e., the SCs and their associated ECM, in the simplified environment of a culture dish (Wood and Bunge, 1975). These early discoveries prompted an increased interest in the use of cultured SCs alone and together with ECM materials for the replacement of whole nerve grafts in animal models of trauma, neurodegeneration, and demyelination (Bunge, 1993).
The idea that cultured neuroglia—SCs in particular—could be used therapeutically to replace cells lost due to nervous system injury or disease was framed in a 1975 review article that highlighted progress on neural culture systems at that time (Bunge, 1975). This concept was supported by in vitro observations showing that SCs survived well without neurons and that their proliferation was elicited by axon membrane-bound molecules (Wood and Bunge, 1975). The discovery of defined soluble mitogenic factors for use as axon-mimetics in the ensuing years (Raff et al., 1978) confirmed that SCs obtained directly from nerve tissues remained proliferative over several passages in the absence of other cell types (Brockes et al., 1979). Since then, additional innovations allowed for the preparation of SC cultures at rising scales from mature and developing tissues as well as from different species, including humans, thereby providing researchers with an opportunity to begin exploratory research on SC grafting in the 1980s.
The goal of the earliest cultured SC transplants was to restore myelin rather than repair tissue affected by trauma in the CNS. By transferring cultured rat SCs together with their collagen substrate into demyelinating lesions in the spinal cord of immunocompromised mice (Duncan et al., 1981) and myelin-deficient rats (Duncan et al., 1988), researchers learned that SCs established in cell culture retained differentiation potential in vivo. Duncan et al. (1981) provided the first evidence on the engraftment of cultured SCs in a xenogeneic host and their association to myelin sheaths that exhibited features of peripheral myelin (Duncan et al., 1981). Regarding SCI repair, researchers used complex 3-dimensional scaffolds containing embryonic SCs and neurons from sensory ganglia (Kuhlengel et al., 1990b) prior to applying purified SCs in suspension from the nerves of adult rats (Xu et al., 1995) and humans (Guest et al., 1997b) to bridge sites of injury in the spinal cord (sections “Development and Testing of Non-human SC Grafts” and “Development and Testing of hSC Grafts in Immunodeficient Rodents”). These ground-breaking investigations sparked a wave of research in the following decade to test the efficacy of SC transplants in various animal models of SCI, understand the underlying mechanisms of repair, and find ways to improve functional outcomes via combinatorial treatments (Fortun et al., 2009; Bunge and Wood, 2012; Deng et al., 2015b). Nevertheless, it should be noted that transplantation of hSC products in SCI patients was not attempted until recently, with the first clinical study reported in year 2008 (Saberi et al., 2008) (section “hSC Transplantation in Human Subjects”).
Altogether, the work supportive of SC-based therapies has spanned nearly 5 decades and the transplantation strategy has been validated independently using SCs of various origins. Clinical trials have been initiated but this work is still in progress (Bunge et al., 2017). Key accomplishments pertaining to the preclinical development and testing of SC cultures in animal models of SCI and the onset of clinical investigations are summarized in Figure 2.
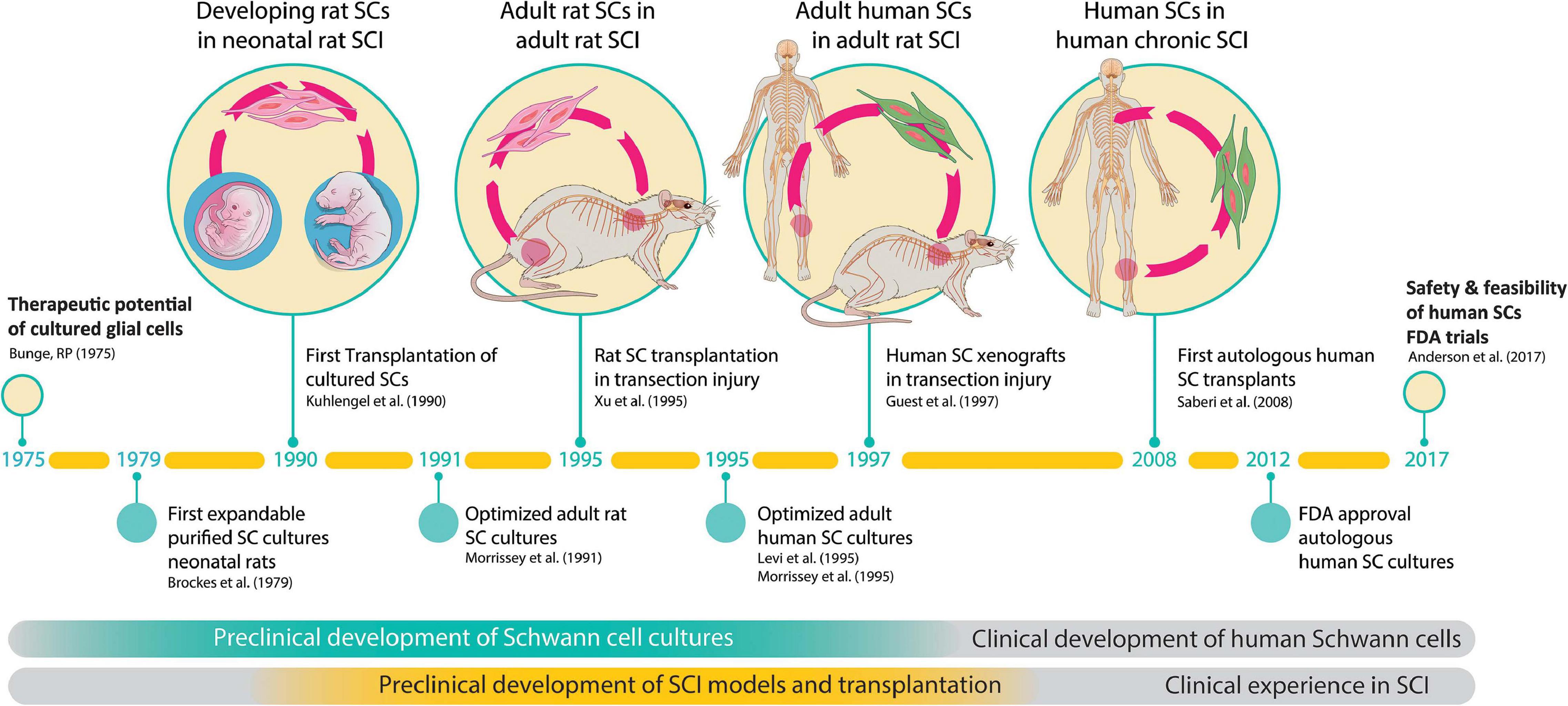
Figure 2. The path to clinical translation of SC transplantation in SCI. The timeline summarizes milestones and selected major achievements in support of the overall strategy. The history of work explaining progress made since the conception of the original idea (Bunge, 1975) to the completion of the first FDA-sanctioned trial is described in the text. Much work was done in the initial phases to (i) develop technologies for SC culturing, and (ii) obtain proof-of-concept data of SC transplants in animal models of SCI. Notice the 20-year gap between the first reported animal study (Kuhlengel et al., 1990b) and the first clinical study (Saberi et al., 2008). FDA approval for the first safety trial in the USA was gained in 2012 (Xu, 2012) and the results released in 2017 (Anderson et al., 2017).
Development and Testing of Non-human SC Grafts
Compelling data on SC transplants were presented in 2 companion publications encompassing the development of SC-containing cellularized scaffolds (Kuhlengel et al., 1990a) and their implantation into thoracolumbar lesions created in the spinal cord of newborn rats (Kuhlengel et al., 1990b). To obtain these scaffolds, cultures of dissociated embryonic dorsal root ganglion (DRG) neurons established on a collagen substrate were deprived of non-neuronal cells prior to being seeded with purified SCs from embryonic DRGs for the re-establishment of SC-neuron interactions and repopulation of the neuritic network. These co-cultures were loosely detached from their dishes and rolled up to create a scroll-like structure that was sectioned into small segments for further culture in the absence or presence of leptomeningeal cells to allow in vitro maturation of axon-associated SCs with and without vascular components. The reconstituted SC-containing scaffolds were in turn placed into cavities created by the removal of dorsal columns immediately after or at a delayed time point with respect to the injury event. The results indicated that the SC transplants filled the vacated spinal cord tissue, that both neurons and SCs survived for several months, and that the SC-grafts became well-vascularized in vivo without the contribution of leptomeningeal cells. Importantly, less differentiated grafts consisting of non-myelinating SCs without ECM components, and delayed implantation with respect to the injury event, allowed for a better fusion of the implants with the spinal cord.
Advancements in the production of large numbers of pure rat SCs from the mature sciatic nerve (Morrissey et al., 1991) allowed researchers to obtain unequivocal evidence of axon regrowth and myelination in allogenic or autologous SC-containing implants. A study by Xiao-Ming Xu et al. (1995) reporting the grafting of SC-seeded guidance channels into the transected cord of adult inbred rats was the first to show that cultured adult purified SCs suspended in a Matrigel matrix contributed to the formation of a vascularized cable tissue that supported the growth of adjacent propriospinal and sensory axons. This study further indicated that SCs alone could exert an axon growth-promoting function supportive of SC differentiation (myelination) in the spinal cord bridge without the physical and trophic support provided by transplanted sensory neurons though it was noticed that most of the SCs in the graft were associated with unmyelinated fibers (Xu et al., 1995).
Development and Testing of hSC Grafts in Immunodeficient Rodents
By the early 1990s, researchers could generate mitotically active hSC populations using pediatric and adult nerves (Rutkowski et al., 1992) as the lifespan of cultured hSCs could be extended significantly with the addition of recombinant glial growth factor (GGF or neuregulin) and cAMP-stimulating agents such as cholera toxin and forskolin (Levi et al., 1995; Rutkowski et al., 1995). Suspensions of hSCs prepared from pre-degenerated nerves and expanded in vitro with the aid of the aforementioned chemical factors were grafted into the transected sciatic nerve (Levi et al., 1994b) and the spinal cord (Guest et al., 1997b) of nude (athymic) rats, a xenograft-tolerant strain, to assess their potential for nerve repair and myelination in the PNS and CNS, respectively. Guest et al. (1997b) used semipermeable guidance channels to create solid grafts containing purified hSCs embedded in Matrigel. When these grafts were implanted across a gap in the spinal cord at the thoracic level, interdigitated interfaces resembling CNS/PNS transition zones formed between the hSC grafts and the host spinal cord. Furthermore, supportive evidence was obtained that propriospinal and DRG axons extended projections into the implants (Guest et al., 1997b), as shown for rat SCs (Xu et al., 1995).
hSC Transplantation in Human Subjects
Saberi et al. (2008, 2011) reported the first study on autologous hSCs transplantation in 33 patients with chronic SCI who had sustained thoracic and cervical injuries. These patients received several injections of a hSC suspension (sural nerve) within and on the sides of the lesion. The hSCs were prepared in the absence of mitogenic factors and animal serum, as autologous serum was used in replacement of the fetal bovine serum (Aghayan et al., 2012) that is a regular component of the hSC culture medium (Casella et al., 1996). No cases of permanent neurological worsening, increment in cavity size, abnormal tissue or tumors (as per contrast-enhanced MR imaging), or infectious complications were found in the 2 years that followed treatment. An independent study of 6 participants who received injections of autologous, activated SCs (purified, in suspension) on each side of the lesion reported no changes of concern as determined during the 5-year follow up period. Some signs of improvement in autonomic, motor, and sensory function in these patients were also recorded (Zhou et al., 2012). In this study, the hSCs were isolated from sural nerves that were ligated 1 week prior to tissue harvesting with the expectation that this procedure would increase the repair features of the isolated hSCs (activation) once established in culture.
In two additional trials, hSCs were given in a co-transplantation approach with other cell types, again confirming that the strategy is safe. In one study, patients with chronic SCI received adult hSCs (autologous, sural nerve) together with bone marrow mesenchymal stem cells through cerebrospinal fluid (Oraee-Yazdani et al., 2016). In the other study, embryonic SCs (heterologous, sciatic nerve) were transplanted together with olfactory ensheathing cells (Chen et al., 2014).
A team of scientists at University of Miami conducted two Phase I, FDA-regulated trials on hSC (sural nerve, expanded) autotransplantation for subacute (Anderson et al., 2017) and chronic SCI (Gant et al., 2021). The former was an unblinded, open-label, non-randomized, non-placebo controlled, dose escalation trial to test the safety and feasibility of obtaining and delivering autologous hSCs into the injury epicenter in 6 participants. One-year post-implantation, no serious neurological complications were associated with the transplanted cells, the spinal surgery or the cell injection system used (Anderson et al., 2017) which was designed by the same team to gently deliver cells to the spinal cord (Santamaria et al., 2018). The second trial was designed to use an individualized cavity-filling dose of hSCs in 8 patients with chronic cervical and thoracic injuries receiving rehabilitation before and after cell implantation (Gant et al., 2021). Not only did this trial confirm the safety of the approach but it also provided evidence of improvement in motor and sensory function in one individual.
The Knowledge Gap in Our Understanding of HSCs
Despite the long history of work on SC transplantation for SCI (section “The Temporal Gap Between Basic and Clinical Science: From Rodents to Humans”), studies on hSCs are surprisingly scarce. A literature search covering the past 30 years revealed a dramatic disparity in the number of indexed publications reporting research conducted using hSCs with respect to non-human SCs in SCI models of transplantation (Figure 3a). A prominent observation was the widespread use of rat SCs mostly from adult nerves in preclinical research (Figure 3c), as noted previously (Tetzlaff et al., 2011). While most hSC studies consisted of clinical investigations, fragmentary literature exists regarding hSC xenotransplants (Figure 3b). We identified only 6 studies reporting results from nerve- and stem cell-derived hSC transplantation in adult nude rats (Guest et al., 1997a, 2005; Bastidas et al., 2017), Sprague Dawley rats (Babaloo et al., 2019), and Wistar rats (Kamada et al., 2005) using dorsal hemisection (Babaloo et al., 2019), transection (Guest et al., 1997a, 2005; Yan-Wu et al., 2011) and contusion injuries (Kamada et al., 2005; Bastidas et al., 2017). There were no reports of hSC transplantation in large animal models of SCI. A handful of publications on the xenografting of hSCs (nerve-derived, primary or expanded) in peripheral nerves (Levi and Bunge, 1994; Levi et al., 1994b; Emery et al., 1999; Hood et al., 2009; Stratton et al., 2017) and demyelinating lesions in the CNS (Brierley et al., 2001; Kohama et al., 2001) are informative on basic properties of the hSCs such as survivability, tumorigenicity and scarring but the available data on particular outcome measures for SCI are rather incomplete (Figure 3d). For instance, whether hSCs proliferate and differentiate in the injured spinal cord remains relatively unexplored. Factors contributing to engraftment success and functional improvement are unknown for hSCs. What seems evident from the examination of the available data (Table 2) is that critical discrepancies exist in the expected responses of rat SCs and hSCs both in anatomical (section “Anatomical Changes”) and behavioral (section “Functional Recovery”) measures.
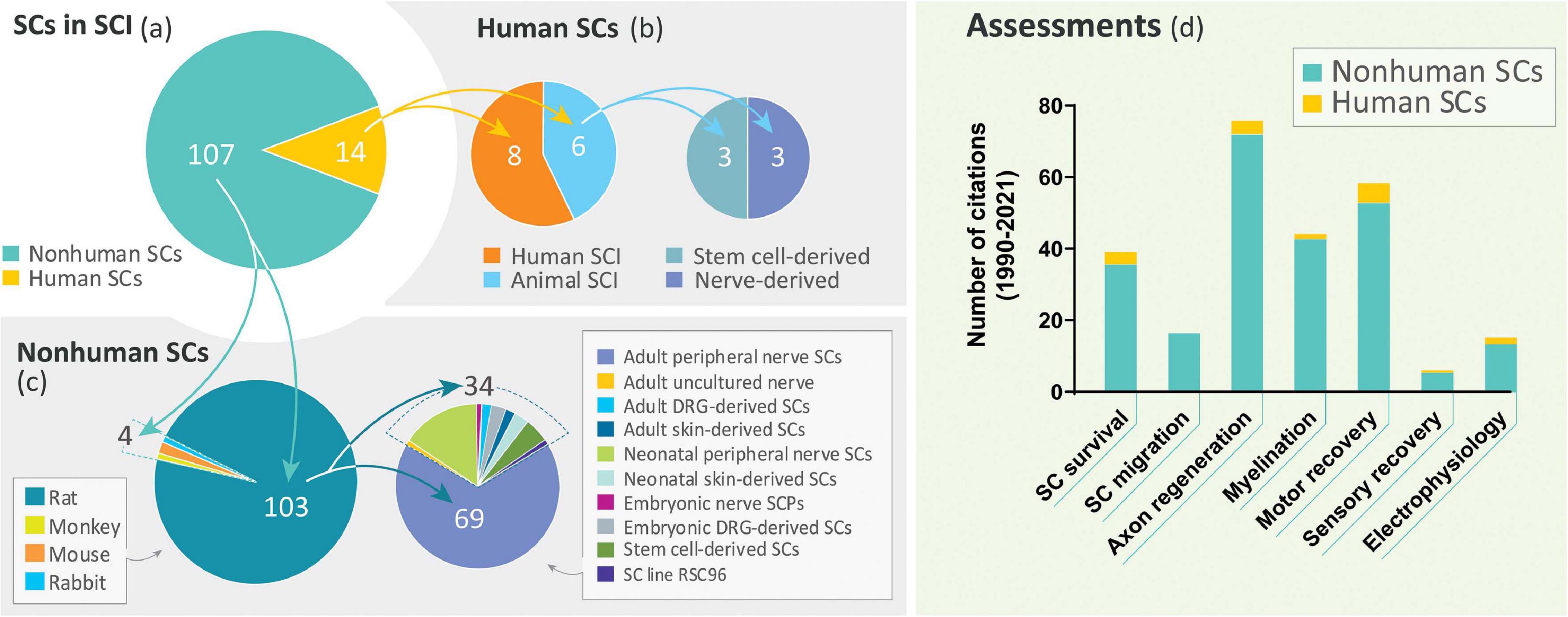
Figure 3. Literature review on SC transplantation in SCI. A PubMed search using the terms “spinal cord injury” and “Schwann cell transplantation” as entry key words retrieved only 14 publications on the transplantation of hSCs (diverse origin) in non-clinical and clinical settings during the period 1990–2021 (a,b). This time frame was selected to take into consideration that the first published study on SC transplants in SCI dates from 1990 (Kuhlengel et al., 1990b). Our search did not discriminate whether the SCs were nerve- or stem cell-derived but we excluded studies on ex-vivo transplants. Studies on non-human SCs from a variety of sources and developmental stages were extensive (88.4%) but the use of adult tissue-derived rat SCs was prevalent (c). A categorization of available studies according to the type of assessments performed in basic and clinical studies combined is shown in (d). The total number of scrutinized studies in the most relevant categories are noted in the pie charts.
Anatomical Changes
Bastidas et al. (2017) reported the first hSC transplantation study in contusive SCI (Bastidas et al., 2017), a model that mimics clinical SCI, in support of an FDA-approved clinical trial led by the same team (Anderson et al., 2017). This study encompassed a comprehensive investigation of hSC grafts in the spinal cord of nude rats on the basis of comparing independent batches of GMP-compliant, mitogen (neuregulin/forskolin)-expanded hSCs from fresh isolates and cryogenic stocks. By showing that clinical-grade hSCs (with or without cryopreservation) remained confined to the site of implantation, did not form tumors, and promoted axon ingrowth into the contusive lesion, the researchers confirmed that the cells for use in patients were bioactive and safe. However, the authors noted that hSCs proliferated slowly and the grafts contained less than 10% of the total transplanted cells. In addition, the transplanted hSCs seemed not to directly contribute myelin in the grafts and there was a high degree of lot (or donor) variability in the rate of survival and other measures of anatomical repair including CNS tissue sparing, cavitation, and axonal extension. While hSC transplants contained abundant myelinated fibers of PNS origin, the myelin sheaths were likely host-derived, as immunoreactivity for human myelin-specific antigens within the engrafted hSC populations could not be confirmed (Bastidas et al., 2017).
To conclude, the low number of studies in which hSCs have been investigated so far, and the high variability displayed by individual hSC batches, has made it difficult to ascertain the properties of hSCs in vivo, especially pertaining restorative changes in the spinal cord. Evidence for repair and (re)myelination mediated by endogenous SCs that either migrate or differentiate locally in the lesion area where hSCs are transplanted has been shown consistently (Guest et al., 1997a; Bastidas et al., 2017), aligned with reports on rat SC transplants from nerve (Hill et al., 2006) and skin (Assinck et al., 2020).
Functional Recovery
Although the data are varied and not always consistent regarding the extent of functional recovery elicited by transplanted rat SCs alone, partial recovery of motor and sensory function has been recorded when SCs are provided with other therapeutic treatments (Bunge and Wood, 2012; Deng et al., 2015a). Surprisingly, we found no published literature on the functional effect of nerve-derived hSCs (primary or expanded) in experimental animal models of SCI with the exception of one study by Guest et al. (1997b). Modestly higher scores during open field and incline plane testing in transected spinal cords were reported in animals that received bridging hSC-enriched grafts (Guest et al., 1997b).
Challenges and Open Questions
As explained above, substantial progress has been made in translating SC therapies from bench to bedside. Experimentally, SCs have been transplanted alone and together with other cell types, in suspension and as part of complex (pre-assembled) cellular systems, with or without biomaterials, and additional experimental treatments, with general positive outcomes. As discussed in section “Understanding the Therapeutic Action of hSCs,” understanding how hSCs promote repair is critical to improve current and prospective approaches to the treatment of SCI, as it is widely recognized that optimization of hSC-based therapies will require more than one bioactive component. Multiple adjunct therapies can be applied to boost the neural supportive features of non-human SCs, to increase their migration capacity and integration with endogenous spinal cord cells, and/or to change the lesion microenvironment in order to reduce the glial scar around SC transplants to make it more permissive for axon growth (reviewed in Fortun et al., 2009; Bunge and Wood, 2012; Deng et al., 2015a). However, whether adjunct therapies would be effective with hSCs cannot be inferred from the available data, as no direct comparison exists on the relative potency of hSCs with respect to those of other species.
Likewise, some limitations of the strategy must be considered. Producing clinical-grade hSCs not only requires a sophisticated infrastructure but also a great deal of time and labor to obtain a transplantable batch. Multiple steps of quality assurance are needed to ensure the product itself is safe, as chemical mitogens, animal serum, recombinant laminin, proteolytic enzymes, and other factors are introduced in the culture workflow. Perils that are communicated to patients during the informed consent process include the possibility that an ineffective isolation of the cells, or the cultures being of insufficient quality or quantity, may preclude delivery of treatment (Guest et al., 2013). These and other potential obstacles are discussed in section “Expanding Access to Transplantable hSC Products.” In this section, we reason that future refinements of hSC-based therapies should involve a reduction of the operating time, the risks of the procedure, and the costs per-batch to enable more widespread patient access to these therapeutic products.
Understanding the Therapeutic Action of hSCs
The myelin-forming capability of SCs has been essential to the formulation of a therapeutic hypothesis supportive of progression to clinical trials for SCI (Guest et al., 2013). However, functional myelination by engrafted hSCs in CNS lesions remains to be confirmed, as immunological data have shown that culture-expanded hSCs form myelin in the damaged peripheral nerve (Levi et al., 1994b) rather than in the spinal cord (Bastidas et al., 2017). Demonstrating myelination by hSCs in co-culture with DRG neurons (reconstituted systems) has proven problematic but in vitro models are insufficient to ascertain whether hSCs established in culture are inherently deficient to myelinate axons or whether the environment provided by the culture dish is insufficient to support their effective differentiation into myelinating cells (Morrissey et al., 1995; Monje et al., 2018). Multiple cell-extrinsic and cell-intrinsic factors can affect hSC (re)differentiation within CNS lesions. Competition with host SCs for access to an appropriate axonal substrate may limit the myelination efficiency of engrafted hSCs. Endogenous SCs colonize and even myelinate axons around chronic traumatic lesions in the spinal cord of humans (Guest et al., 2005) and this response may be enhanced by the transplantation of SCs, as evidenced in the rodent data (Hill et al., 2006). In addition, the isolation and expansion of hSCs may alter the (re)differentiation capability of the cells, as subculture moderately reduces myelination by transplanted hSCs in the PNS of immunodeficient rodents (Levi and Bunge, 1994; Levi et al., 1994b).
Whereas hSC grafts do not evoke undesirable effects in humans or experimental animals, it is unclear whether consistent engraftment and long-term survival of hSCs can be achieved experimentally. Therapies to prevent cell loss after transplantation may be needed. hSC survival in contusive injury (nude rats) was reported to be variable and low (Bastidas et al., 2017). This finding is aligned with reports that revealed that a majority of transplanted rat SCs die acutely post-transplantation, mainly due to necrosis and to some extent also due to apoptosis (Hill et al., 2007); however, grafts of rat SCs remain stable for >7 months and proliferation may occur as early as 1-day postgrafting (Wang and Xu, 2014). One caveat is that hSCs are antigen-presenting cells and may be sufficient to evoke an immunogenic response. hSC cultures established in vitro upregulate the expression of the major histocompatibility complex II (MHCII) (Weiss et al., 2016), which together with MHCI has been linked to the rejection of peripheral nerve allografts (Ansselin and Pollard, 1990).
Each population of transplantable hSCs is unique and a high degree of batch variability is expected, possibly due to, but not simply because of, patient-specific characteristics. Attributes that are specific to each donor are not usually evident when the cells are established in culture, but their impact may be substantial with longer survival times (Monje et al., 2018). Assorted factors can influence the bioactivity of individual cell batches, and undesirable effects on host tissues such as excessive astrogliosis or inflammation may occur. For instance, the presence of nerve-derived fibroblasts in hSC cultures has resulted in the deposition of large amounts of collagen and extensive axonal degeneration when transplanted in areas of acute demyelination in the spinal cord of adult rats (Brierley et al., 2001). Whether human fibroblasts are seemingly deleterious in traumatic SCI is unknown, but we suspect the fibroblast populations to be supportive of nerve repair because of their immaturity and their strong proregenerative transcriptional signature (Peng et al., 2020). It should be mentioned that SCs alone are usually ineffective to favorably modify the glial scar. However, evidence has pointed out that hSCs intermingle with astrocytic process from a xenogeneic host and create more permissive host-graft interfaces in the spinal cord when compared to rat SCs (Bastidas et al., 2017).
To conclude, it is expected that hSCs are beneficial irrespective of initial survival rates and donor variability but the functional consequences of hSC transplantation are ill-defined. Discriminating between true axon regeneration, i.e., axonal growth into the cavity filled with grafted hSCs, and sparing in contusive SCI can help in interpreting functional outcome. How to improve the therapeutic value of hSCs prior to and after transplantation remains an area for exploration and development.
Expanding Access to Transplantable hSC Products
For decades, basic and translational research on hSCs has been hampered by the overall lack of technologies to access and understand the cell material itself. First, the methods for processing animal nerve-derived cells are ineffective for humans, and second, the properties of cultured hSCs differ from those of other species (Monje, 2020). Preparing and maintaining hSC cultures over several weeks require sophisticated knowledge and a tailored management of culture variables to achieve sufficient cell yields at a high level of purity. Additionally, hSCs have a limited lifespan in culture and need to be prepared each time they are required unless methods are optimized to produce large enough stocks for cryogenic storage.
Even with protocols and expertise in place, one should not underestimate the impact of the high costs associated with hSC bioprocessing due to the expensive materials (including GLP-grade chemicals), dedicated personnel, and quality assurance for each individual preparation. Though autologous and freshly prepared products are the gold standard for patients, cryopreserved hSCs may be considered to increase hSC availability in preclinical research (Bastidas et al., 2017). It should be noted that hSCs can be prepared from cadaveric nerves (Boyer et al., 1994) and tissues that would otherwise be discarded during surgical procedures, such as plastic or reconstructive surgeries, amputations (Kohama et al., 2001), and aborted embryos. There is one precedent for the use of fetal (sciatic nerve) hSCs for the treatment of chronic SCI in humans (Chen et al., 2014). Using established practices, like safe cold storage of tissue biospecimens prior to cell processing (Levi et al., 1994a), can certainly aid in getting access to more varied or even non-local sources of human tissues for hSC isolation. The banking of hSCs may be considered in cases where an excess of cells is generated, thereby allowing the transfer of the cells elsewhere, or delayed use for reanalysis or redosing. Regrettably, SC-like cells from stem cells or other sources are not suitable for near-term clinical cell therapy. Important hurdles need to be overcome from the biological and regulatory standpoints for SC-like cells to be considered appropriate for human transplantation.
Limits to the expandability of hSC cultures may vary but assorted strategies can be implemented to expedite cell processing, enhance the recovery of cells, and optimize (or standardize) the quality of the preparations. With the development of pertinent innovations, such as microcarrier-based bioreactor systems, individual hSC cultures can be scaled-up significantly (Mirfeizi et al., 2017). Expedited processing of adult rat and human SC cultures can be achieved using protocols involving the immediate dissociation of nerve fibers (Andersen et al., 2016; Weiss et al., 2016). The removal of animal serum and mitogenic factors from the culture medium has already been implemented for clinical grade cultivation of hSCs (Aghayan et al., 2012). Indeed, serum-free processing (Mirfeizi et al., 2017) and other modifications of the standard protocols can ease regulatory restrictions for quality control and product release.
Alternatively, peripheral nerve tissues (uncultured) obtained as same-day products without (or with minimal) manipulation can be prepared expeditiously and used with negligible risk to patients. Autologous nerve grafts have been used to bridge a gap in the spinal cord in combination with autologous olfactory ensheathing cells and fibroblasts (Tabakow et al., 2014), and bone marrow-derived mesenchymal stem cells (Amr et al., 2014) in human subjects. Over 70 patients received implants of autologous unprocessed sural nerve fascicles in the brain as part of a combinatorial treatment for Parkinson’s disease with no reported complications (El Seblani et al., 2020). Though the implantation of unprocessed nerves is usually discouraged for SCI, no evidence indicates that it may be unsafe in humans. An advantage of autologous tissues over cultured cells is that the former products are exempt from regulatory constraints and can offer a safer and cost-effective alternative for implantation of hSCs in the CNS (El Seblani et al., 2020). Finally, whole cells may not be needed, as diverse subcellular products such as SC-derived exosomes may have sufficient therapeutic effects in promoting axon growth (Lopez-Leal et al., 2020).
Concluding Remarks
The expectations of the scientific community and the general public regarding the outcome of cell therapy trials are high in view of the lack of available options to treat human SCI and other types of CNS damage. Human studies have shown that transplanted hSCs are well tolerated in the spinal cord. Neurological improvement has been insinuated in some patients but clinical trials have not advanced to efficacy testing. As described above, investigations on hSC transplants are lagging, and crucial questions on the properties of hSCs in vivo remain unanswered. On the one hand, additional systematic experimentation and replication studies using hSCs are warranted to confirm (or disprove) the idea that rodent SC transplants are useful models for the development of hSC-based therapeutics. On the other hand, the established hSC transplantation paradigm using cultured cells obtained via traditional methods may pose a barrier to a widespread use of hSCs in basic science and in the clinic. Current technologies for hSC manufacturing restrict operations to centers equipped with considerable financial and scientific capabilities and this may affect the translation of the strategy in the longer term, especially for extending the treatment to large patient cohorts. Clinical initiatives involving cell therapies for SCI are at risk to be halted at an early stage due to the financial burden (Scott and Magnus, 2014). Simplification of the current transplantation strategy is possible because there are alternative ways to take advantage of the extraordinary regenerative capacity of peripheral nerves, in particular that of hSCs, for the development of novel biotherapeutics. Targeted innovations involving banked cells, uncultured or minimally manipulated nerve tissues or cells, and even extracellular vesicles may facilitate the development of additional products, including off-the-shelf ones, for preclinical, and perhaps clinical, applications.
To conclude, the history of work on SC transplants has highlighted the multiplicity of approaches attempted experimentally to exploit their value for SCI repair. We believe it is pertinent and timely to build upon existing knowledge, and the promising clinical results, to revitalize research on hSC therapies. Future directions toward this goal may encompass the creation of alternative hSC-based products for use alone or in support of other therapeutic approaches.
Author Contributions
PM: conceptualization, writing, figure preparation, data analysis, and funding. LD: data collection and analysis, and manuscript editing. X-MX: manuscript editing and funding. All authors contributed to the article and approved the submitted version.
Funding
This work was supported by the Craig H. Neilsen Foundation (grant 339576 to PM) and the Indiana Spinal Cord and Brain Injury Research Fund from the Indiana State Department of Health (grants 33997 and 43547 to PM and X-MX). X-MX received support from NIH R01 100531, R01 NS103481, R01 NS111776, and Merit Review Awards I01 BX002356, I01 BX003705, I01 RX002687 from the U.S. Department of Veterans Affairs. The contents of this article are the responsibility of the authors and do not necessarily represent the official views of the funding agencies.
Conflict of Interest
PM is founder of GliaBio LLC, a consulting company that focuses on glial cells.
The remaining authors declare that the research was conducted in the absence of any commercial or financial relationships that could be construed as a potential conflict of interest.
Acknowledgments
We wish to recognize Mary Bunge for her lifelong dedication to SC research and immeasurable efforts toward clinical investigations in SCI. We thank the invaluable contribution of Caitlin Hill, Martin Oudega and Tamara Weiss for many expert comments to improve the manuscript. Patti Raley and Louise Pay contributed with English editing. Christopher Brown and Valeria Nogueira assisted with illustrations.
References
Aghayan, H. R., Arjmand, B., Norouzi-Javidan, A., Saberi, H., Soleimani, M., Tavakoli, S. A., et al. (2012). Clinical grade cultivation of human Schwann cell, by the using of human autologous serum instead of fetal bovine serum and without growth factors. Cell Tissue Bank 13, 281–285. doi: 10.1007/s10561-011-9250-8
Amr, S. M., Gouda, A., Koptan, W. T., Galal, A. A., Abdel-Fattah, D. S., Rashed, L. A., et al. (2014). Bridging defects in chronic spinal cord injury using peripheral nerve grafts combined with a chitosan-laminin scaffold and enhancing regeneration through them by co-transplantation with bone-marrow-derived mesenchymal stem cells: case series of 14 patients. J. Spinal. Cord Med. 37, 54–71. doi: 10.1179/2045772312y.0000000069
Andersen, N. D., Srinivas, S., Pinero, G., and Monje, P. V. (2016). A rapid and versatile method for the isolation, purification and cryogenic storage of Schwann cells from adult rodent nerves. Sci. Rep. 6:31781.
Anderson, K. D., Guest, J. D., Dietrich, W. D., Bartlett Bunge, M., Curiel, R., Dididze, M., et al. (2017). Safety of autologous human schwann cell transplantation in subacute thoracic spinal cord injury. J,. Neurotrauma 34, 2950–2963. doi: 10.1089/neu.2016.4895
Ansselin, A. D., and Pollard, J. D. (1990). Immunopathological factors in peripheral nerve allograft rejection: quantification of lymphocyte invasion and major histocompatibility complex expression. J. Neurol. Sci. 96, 75–88. doi: 10.1016/0022-510x(90)90058-u
Assinck, P., Sparling, J. S., Dworski, S., Duncan, G. J., Wu, D. L., Liu, J., et al. (2020). Transplantation of skin precursor-derived schwann cells yields better locomotor outcomes and reduces bladder pathology in rats with chronic spinal cord injury. Stem Cell Rep. 15, 140–155. doi: 10.1016/j.stemcr.2020.05.017
Babaloo, H., Ebrahimi-Barough, S., Derakhshan, M. A., Yazdankhah, M., Lotfibakhshaiesh, N., Soleimani, M., et al. (2019). PCL/gelatin nanofibrous scaffolds with human endometrial stem cells/Schwann cells facilitate axon regeneration in spinal cord injury. J. Cell Physiol. 234, 11060–11069. doi: 10.1002/jcp.27936
Bastidas, J. G., Athauda, G., De La Cruz, W. M., Chan, R., Golshani, Y., Berrocal, M., et al. (2017). Human Schwann cells exhibit long-term cell survival, are not tumorigenic and promote repair when transplanted into the contused spinal cord. Glia 65, 1278–1301. doi: 10.1002/glia.23161
Biernaskie, J., Sparling, J. S., Liu, J., Shannon, C. P., Plemel, J. R., Xie, Y., et al. (2007). Skin-derived precursors generate myelinating Schwann cells that promote remyelination and functional recovery after contusion spinal cord injury. J. Neurosci. 27, 9545–9559. doi: 10.1523/jneurosci.1930-07.2007
Blakemore, W. F. (1977). Remyelination of CNS axons by Schwann cells transplanted from the sciatic nerve. Nature 266, 68–69. doi: 10.1038/266068a0
Boyer, P. J., Tuite, G. F., Dauser, R. C., Muraszko, K. M., Tennekoon, G. I., and Rutkowski, J. L. (1994). Sources of human Schwann cells and the influence of donor age. Exp. Neurol. 130, 53–55. doi: 10.1006/exnr.1994.1184
Brierley, C. M., Crang, A. J., Iwashita, Y., Gilson, J. M., Scolding, N. J., Compston, D. A., et al. (2001). Remyelination of demyelinated CNS axons by transplanted human schwann cells: the deleterious effect of contaminating fibroblasts. Cell Transplant. 10, 305–315. doi: 10.3727/000000001783986774
Brockes, J. P., Fields, K. L., and Raff, M. C. (1979). Studies on cultured rat Schwann cells. I. Establishment of purified populations from cultures of peripheral nerve. Brain Res. 165, 105–118. doi: 10.1016/0006-8993(79)90048-9
Bunge, M., Monje, P. V., Khan, A., and Wood, P. M. (2017). From transplanting Schwann cells in experimental rat spinal cord injury to their transplantation into human injured spinal cord in clinical trials. Prog. Brain Res. 231, 107–133. doi: 10.1016/bs.pbr.2016.12.012
Bunge, M. B., and Wood, P. M. (2012). Realizing the maximun potential of Schwann cells to promote recovery from spinal cord injury. Handbook Clin. Neurol. 109, 523–540. doi: 10.1016/b978-0-444-52137-8.00032-2
Bunge, R. P. (1975). “Changing uses of nerve tissue culture 1950-1975,” in The Nervous System. In The Basic Neurosciences, Vol. 1, ed. D. B. Tower (New York, NY: Raven Press), 31.
Bunge, R. P. (1993). Expanding roles for the Schwann cell: ensheathment, myelination, trophism and regeneration. Curr. Opin. Neurobiol. 3, 805–809. doi: 10.1016/0959-4388(93)90157-t
Casella, G. T., Bunge, R. P., and Wood, P. M. (1996). Improved method for harvesting human Schwann cells from mature peripheral nerve and expansion in vitro. Glia 17, 327–338. doi: 10.1002/(sici)1098-1136(199608)17:4<327::aid-glia7>3.0.co;2-w
Chen, L., Huang, H., Xi, H., Zhang, F., Liu, Y., Chen, D., et al. (2014). A prospective randomized double-blind clinical trial using a combination of olfactory ensheathing cells and Schwann cells for the treatment of chronic complete spinal cord injuries. Cell Transplant. 23(Suppl. 1), S35–S44.
Crawford, K., and Armati-Gulson, P. (1982). The development of human fetal dorsal root ganglia in vitro: the first 20 days. Neuropathol. Appl. Neurobiol. 8, 477–488. doi: 10.1111/j.1365-2990.1982.tb00315.x
Deng, L., Walker, C., and Xu, X.-M. (2015a). “Schwann cell-mediated axonal regeneration in the central nervous system,” in Neural Regeneration, Chap. 22, eds K.-F. So and X.-M. Xu (Oxford: Academic Press), 337–349. doi: 10.1016/b978-0-12-801732-6.00022-7
Deng, L. X., Walker, C., and Xu, X. M. (2015b). Schwann cell transplantation and descending propriospinal regeneration after spinal cord injury. Brain Res. 1619, 104–114. doi: 10.1016/j.brainres.2014.09.038
Duncan, I. D., Aguayo, A. J., Bunge, R. P., and Wood, P. M. (1981). Transplantation of rat Schwann cells grown in tissue culture into the mouse spinal cord. J. Neurol. Sci. 49, 241–252. doi: 10.1016/0022-510x(81)90082-4
Duncan, I. D., Hammang, J. P., Jackson, K. F., Wood, P. M., Bunge, R. P., and Langford, L. (1988). Transplantation of oligodendrocytes and Schwann cells into the spinal cord of the myelin-deficient rat. J. Neurocytol. 17, 351–360. doi: 10.1007/bf01187857
El Seblani, N., Welleford, A. S., Quintero, J. E., van Horne, C. G., and Gerhardt, G. A. (2020). Invited review: utilizing peripheral nerve regenerative elements to repair damage in the CNS. J. Neurosci. Methods 335:108623. doi: 10.1016/j.jneumeth.2020.108623
Emery, E., Li, X., Brunschwig, J. P., Olson, L., and Levi, A. D. (1999). Assessment of the malignant potential of mitogen stimulated human Schwann cells. J. Peripher. Nerv. Syst. 4, 107–116.
Fortun, J., Hill, C. E., and Bunge, M. B. (2009). Combinatorial strategies with Schwann cell transplantation to improve repair of the injured spinal cord. Neurosci. Lett. 456, 124–132. doi: 10.1016/j.neulet.2008.08.092
Funk, D., Fricke, C., and Schlosshauer, B. (2007). Aging Schwann cells in vitro. Eur. J. Cell Biol. 86, 207–219. doi: 10.1016/j.ejcb.2006.12.006
Gant, K., Guest, J., Palermo, A., Vedantam, A., Jimsheleishvili, G., Bunge, M. B., et al. (2021). Phase 1 safety trial of autologous human schwann cell transplantation in chronic spinal cord injury. J. Neurotrauma doi: 10.1089/neu.2020.7590
CrossRef Full Text | [Epup ahead of print]. | PubMed Abstract | Google Scholar
Guest, J., Santamaria, A. J., and Benavides, F. D. (2013). Clinical translation of autologous Schwann cell transplantation for the treatment of spinal cord injury. Curr. Opin. Organ. Transplant. 18, 682–689.
Guest, J. D., Hesse, D., Schnell, L., Schwab, M. E., Bunge, M. B., and Bunge, R. P. (1997a). Influence of IN-1 antibody and acidic FGF-fibrin glue on the response of injured corticospinal tract axons to human Schwann cell grafts. J. Neurosci. Res. 50, 888–905. doi: 10.1002/(sici)1097-4547(19971201)50:5<888::aid-jnr24>3.0.co;2-w
Guest, J. D., Hiester, E. D., and Bunge, R. P. (2005). Demyelination and Schwann cell responses adjacent to injury epicenter cavities following chronic human spinal cord injury. Exp. Neurol. 192, 384–393. doi: 10.1016/j.expneurol.2004.11.033
Guest, J. D., Rao, A., Olson, L., Bunge, M. B., and Bunge, R. P. (1997b). The ability of human Schwann cell grafts to promote regeneration in the transected nude rat spinal cord. Exp Neurol. 148, 502–522. doi: 10.1006/exnr.1997.6693
Haastert, K., Mauritz, C., Chaturvedi, S., and Grothe, C. (2007). Human and rat adult Schwann cell cultures: fast and efficient enrichment and highly effective non-viral transfection protocol. Nat. Protoc. 2, 99–104. doi: 10.1038/nprot.2006.486
Hill, C. E., Hurtado, A., Blits, B., Bahr, B. A., Wood, P. M., Bartlett Bunge, M., et al. (2007). Early necrosis and apoptosis of Schwann cells transplanted into the injured rat spinal cord. Eur. J. Neurosci. 26, 1433–1445. doi: 10.1111/j.1460-9568.2007.05771.x
Hill, C. E., Moon, L. D., Wood, P. M., and Bunge, M. B. (2006). Labeled Schwann cell transplantation: cell loss, host Schwann cell replacement, and strategies to enhance survival. Glia 53, 338–343. doi: 10.1002/glia.20287
Hood, B., Levene, H. B., and Levi, A. D. (2009). Transplantation of autologous Schwann cells for the repair of segmental peripheral nerve defects. Neurosurg. Focus. 26:E4.
Hopf, A., Schaefer, D. J., Kalbermatten, D. F., Guzman, R., and Madduri, S. (2020). Schwann cell-like cells: origin and usability for repair and regeneration of the peripheral and central nervous system. Cells 9:1990. doi: 10.3390/cells9091990
Huang, Z., Powell, R., Phillips, J. B., and Haastert-Talini, K. (2020). Perspective on schwann cells derived from induced pluripotent stem cells in peripheral nerve tissue engineering. Cells 9:2497. doi: 10.3390/cells9112497
Jessen, K. R., and Arthur-Farraj, P. (2019). Repair Schwann cell update: adaptive reprogramming, EMT, and stemness in regenerating nerves. Glia 67, 421–437. doi: 10.1002/glia.23532
Jessen, K. R., and Mirsky, R. (2019). The success and failure of the schwann cell response to nerve injury. Front. Cell Neurosci. 13:33. doi: 10.3389/fncel.2019.00033
Kamada, T., Koda, M., Dezawa, M., Yoshinaga, K., Hashimoto, M., Koshizuka, S., et al. (2005). Transplantation of bone marrow stromal cell-derived Schwann cells promotes axonal regeneration and functional recovery after complete transection of adult rat spinal cord. J. Neuropathol. Exp. Neurol. 64, 37–45. doi: 10.1093/jnen/64.1.37
Kohama, I., Lankford, K. L., Preiningerova, J., White, F. A., Vollmer, T. L., and Kocsis, J. D. (2001). Transplantation of cryopreserved adult human Schwann cells enhances axonal conduction in demyelinated spinal cord. J. Neurosci. 21, 944–950. doi: 10.1523/jneurosci.21-03-00944.2001
Kuhlengel, K. R., Bunge, M. B., and Bunge, R. P. (1990a). Implantation of cultured sensory neurons and Schwann cells into lesioned neonatal rat spinal cord. I. Methods for preparing implants from dissociated cells. J. Comp. Neurol. 293, 63–73. doi: 10.1002/cne.902930106
Kuhlengel, K. R., Bunge, M. B., Bunge, R. P., and Burton, H. (1990b). Implantation of cultured sensory neurons and Schwann cells into lesioned neonatal rat spinal cord. II. Implant characteristics and examination of corticospinal tract growth. J. Comp. Neurol. 293, 74–91. doi: 10.1002/cne.902930107
Langford, L. A., Porter, S., and Bunge, R. P. (1988). Immortalized rat Schwann cells produce tumours in vivo. J. Neurocytol. 17, 521–529. doi: 10.1007/bf01189807
Lavdas, A. A., Papastefanaki, F., Thomaidou, D., and Matsas, R. (2008). Schwann cell transplantation for CNS repair. Curr. Med. Chem. 15, 151–160. doi: 10.2174/092986708783330593
Lehmann, H. C., Chen, W., Mi, R., Wang, S., Liu, Y., Rao, M., et al. (2012). Human Schwann cells retain essential phenotype characteristics after immortalization. Stem Cells Dev. 21, 423–431. doi: 10.1089/scd.2010.0513
Lehmann, H. C., and Hoke, A. (2016). Use of engineered Schwann cells in peripheral neuropathy: Hopes and hazards. Brain Res. 1638, 97–104. doi: 10.1016/j.brainres.2015.10.040
Levi, A. D., and Bunge, R. P. (1994). Studies of myelin formation after transplantation of human Schwann cells into the severe combined immunodeficient mouse. Exp. Neurol. 130, 41–52. doi: 10.1006/exnr.1994.1183
Levi, A. D., Bunge, R. P., Lofgren, J. A., Meima, L., Hefti, F., Nikolics, K., et al. (1995). The influence of heregulins on human Schwann cell proliferation. J. Neurosci. 15, 1329–1340. doi: 10.1523/jneurosci.15-02-01329.1995
Levi, A. D., Evans, P. J., Mackinnon, S. E., and Bunge, R. P. (1994a). Cold storage of peripheral nerves: an in vitro assay of cell viability and function. Glia 10, 121–131. doi: 10.1002/glia.440100206
Levi, A. D., Guenard, V., Aebischer, P., and Bunge, R. P. (1994b). The functional characteristics of Schwann cells cultured from human peripheral nerve after transplantation into a gap within the rat sciatic nerve. J. Neurosci. 14, 1309–1319. doi: 10.1523/jneurosci.14-03-01309.1994
Lopez-Leal, R., Diaz-Viraque, F., Catalan, R. J., Saquel, C., Enright, A., Iraola, G., et al. (2020). Schwann cell reprogramming into repair cells increases miRNA-21 expression in exosomes promoting axonal growth. J. Cell Sci. 133:jcs239004.
Mathon, N. F., Malcolm, D. S., Harrisingh, M. C., Cheng, L., and Lloyd, A. C. (2001). Lack of replicative senescence in normal rodent glia. Science 291, 872–875. doi: 10.1126/science.1056782
May, Z., Kumar, R., Fuehrmann, T., Tam, R., Vulic, K., Forero, J., et al. (2018). Adult skin-derived precursor Schwann cell grafts form growths in the injured spinal cord of Fischer rats. Biomed. Mater. 13:034101. doi: 10.1088/1748-605x/aa95f8
Mirfeizi, L., Stratton, J. A., Kumar, R., Shah, P., Agabalyan, N., Stykel, M. G., et al. (2017). Serum-free bioprocessing of adult human and rodent skin-derived Schwann cells: implications for cell therapy in nervous system injury. J. Tissue Eng. Regen. Med. 11, 3385–3397. doi: 10.1002/term.2252
Mirsky, R., and Jessen, K. R. (2018). Isolation of Schwann cell precursors from rodents. Methods Mol. Biol. 1739, 3–15. doi: 10.1007/978-1-4939-7649-2_1
Monje, P. V. (2020). The properties of human Schwann cells: lessons from in vitro culture and transplantation studies. Glia 68, 797–810. doi: 10.1002/glia.23793
Monje, P. V., Sant, D., and Wang, G. (2018). Phenotypic and functional characteristics of human schwann cells as revealed by cell-based assays and RNA-SEQ. Mol. Neurobiol. 55, 6637–6660. doi: 10.1007/s12035-017-0837-3
Morrissey, T. K., Bunge, R. P., and Kleitman, N. (1995). Human Schwann cells in vitro. I. Failure to differentiate and support neuronal health under co-culture conditions that promote full function of rodent cells. J. Neurobiol. 28, 171–189. doi: 10.1002/neu.480280205
Morrissey, T. K., Kleitman, N., and Bunge, R. P. (1991). Isolation and functional characterization of Schwann cells derived from adult peripheral nerve. J. Neurosci. 11, 2433–2442. doi: 10.1523/jneurosci.11-08-02433.1991
Oraee-Yazdani, S., Hafizi, M., Atashi, A., Ashrafi, F., Seddighi, A. S., Hashemi, S. M., et al. (2016). Co-transplantation of autologous bone marrow mesenchymal stem cells and Schwann cells through cerebral spinal fluid for the treatment of patients with chronic spinal cord injury: safety and possible outcome. Spinal. Cord. 54, 102–109. doi: 10.1038/sc.2015.142
Oudega, M., and Xu, X. M. (2006). Schwann cell transplantation for repair of the adult spinal cord. J. Neurotrauma 23, 453–467. doi: 10.1089/neu.2006.23.453
Painter, M. W., Brosius Lutz, A., Cheng, Y. C., Latremoliere, A., Duong, K., Miller, C. M., et al. (2014). Diminished Schwann cell repair responses underlie age-associated impaired axonal regeneration. Neuron 83, 331–343. doi: 10.1016/j.neuron.2014.06.016
Peng, K., Sant, D., Andersen, N., Silvera, R., Camarena, V., Pinero, G., et al. (2020). Magnetic separation of peripheral nerve-resident cells underscores key molecular features of human Schwann cells and fibroblasts: an immunochemical and transcriptomics approach. Sci. Rep. 10:18433.
Raff, M. C., Abney, E., Brockes, J. P., and Hornby-Smith, A. (1978). Schwann cell growth factors. Cell 15, 813–822. doi: 10.1016/0092-8674(78)90266-0
Richardson, P. M., McGuinness, U. M., and Aguayo, A. J. (1980). Axons from CNS neurons regenerate into PNS grafts. Nature 284, 264–265. doi: 10.1038/284264a0
Rutkowski, J. L., Kirk, C. J., Lerner, M. A., and Tennekoon, G. I. (1995). Purification and expansion of human Schwann cells in vitro. Nat. Med. 1, 80–83. doi: 10.1038/nm0195-80
Rutkowski, J. L., Tennekoon, G. I., and McGillicuddy, J. E. (1992). Selective culture of mitotically active human Schwann cells from adult sural nerves. Ann. Neurol. 31, 580–586. doi: 10.1002/ana.410310603
Saberi, H., Firouzi, M., Habibi, Z., Moshayedi, P., Aghayan, H. R., Arjmand, B., et al. (2011). Safety of intramedullary Schwann cell transplantation for postrehabilitation spinal cord injuries: 2-year follow-up of 33 cases. J. Neurosurg. Spine 15, 515–525. doi: 10.3171/2011.6.spine10917
Saberi, H., Moshayedi, P., Aghayan, H. R., Arjmand, B., Hosseini, S. K., Emami-Razavi, S. H., et al. (2008). Treatment of chronic thoracic spinal cord injury patients with autologous Schwann cell transplantation: an interim report on safety considerations and possible outcomes. Neurosci. Lett. 443, 46–50. doi: 10.1016/j.neulet.2008.07.041
Santamaria, A. J., Solano, J. P., Benavides, F. D., and Guest, J. D. (2018). Intraspinal delivery of schwann cells for spinal cord injury. Methods Mol. Biol. 1739, 467–484. doi: 10.1007/978-1-4939-7649-2_31
Scott, C. T., and Magnus, D. (2014). Wrongful termination: lessons from the Geron clinical trial. Stem Cells Transl. Med. 3, 1398–1401. doi: 10.5966/sctm.2014-0147
Sparling, J. S., Bretzner, F., Biernaskie, J., Assinck, P., Jiang, Y., Arisato, H., et al. (2015). Schwann cells generated from neonatal skin-derived precursors or neonatal peripheral nerve improve functional recovery after acute transplantation into the partially injured cervical spinal cord of the rat. J. Neurosci. 35, 6714–6730. doi: 10.1523/jneurosci.1070-14.2015
Stierli, S., Napoli, I., White, I. J., Cattin, A. L., Monteza Cabrejos, A., Garcia Calavia, N., et al. (2018). The regulation of the homeostasis and regeneration of peripheral nerve is distinct from the CNS and independent of a stem cell population. Development 145:dev170316.
Stratton, J. A., Kumar, R., Sinha, S., Shah, P., Stykel, M., Shapira, Y., et al. (2017). Purification and characterization of schwann cells from adult human skin and nerve. eNeuro 4:ENEURO.0307-16.2017. doi: 10.1523/ENEURO.0307-16.2017
Tabakow, P., Raisman, G., Fortuna, W., Czyz, M., Huber, J., Li, D., et al. (2014). Functional regeneration of supraspinal connections in a patient with transected spinal cord following transplantation of bulbar olfactory ensheathing cells with peripheral nerve bridging. Cell Transplant. 23, 1631–1655. doi: 10.3727/096368914x685131
Tetzlaff, W., Okon, E. B., Karimi-Abdolrezaee, S., Hill, C. E., Sparling, J. S., Plemel, J. R., et al. (2011). A systematic review of cellular transplantation therapies for spinal cord injury. J. Neurotrauma 28, 1611–1682.
Wang, X., and Xu, X. M. (2014). Long-term survival, axonal growth-promotion, and myelination of Schwann cells grafted into contused spinal cord in adult rats. Exp. Neurol. 261, 308–319. doi: 10.1016/j.expneurol.2014.05.022
Weiss, T., Taschner-Mandl, S., Bileck, A., Slany, A., Kromp, F., Rifatbegovic, F., et al. (2016). Proteomics and transcriptomics of peripheral nerve tissue and cells unravel new aspects of the human Schwann cell repair phenotype. Glia 64, 2133–2153. doi: 10.1002/glia.23045
Wood, P. M., and Bunge, R. P. (1975). Evidence that sensory axons are mitogenic for Schwann cells. Nature 256, 662–664. doi: 10.1038/256662a0
Xu, X. M. (2012). Breaking news in spinal cord injury research: FDA approved phase I clinical trial of human, autologous schwann cell transplantation in patients with spinal cord injuries. Neural. Regen. Res. 7, 1685–1687.
Xu, X. M., Guenard, V., Kleitman, N., and Bunge, M. B. (1995). Axonal regeneration into Schwann cell-seeded guidance channels grafted into transected adult rat spinal cord. J. Comp. Neurol. 351, 145–160. doi: 10.1002/cne.903510113
Yan-Wu, G., Yi-Quan, K., Ming, L., Ying-Qian, C., Xiao-Dan, J., Shi-Zhong, Z., et al. (2011). Human umbilical cord-derived Schwann-like cell transplantation combined with neurotrophin-3 administration in dyskinesia of rats with spinal cord injury. Neurochem. Res. 36, 783–792. doi: 10.1007/s11064-011-0402-9
Keywords: cell therapy, clinical trials, regeneration, myelination, functional recovery, biotherapeutic products, Schwann cells, spinal cord injury
Citation: Monje PV, Deng L and Xu X-M (2021) Human Schwann Cell Transplantation for Spinal Cord Injury: Prospects and Challenges in Translational Medicine. Front. Cell. Neurosci. 15:690894. doi: 10.3389/fncel.2021.690894
Received: 04 April 2021; Accepted: 21 May 2021;
Published: 18 June 2021.
Edited by:
Antje Kroner, Medical College of Wisconsin, United StatesReviewed by:
Patricia Armati, The University of Sydney, AustraliaValerio Magnaghi, University of Milan, Italy
Copyright © 2021 Monje, Deng and Xu. This is an open-access article distributed under the terms of the Creative Commons Attribution License (CC BY). The use, distribution or reproduction in other forums is permitted, provided the original author(s) and the copyright owner(s) are credited and that the original publication in this journal is cited, in accordance with accepted academic practice. No use, distribution or reproduction is permitted which does not comply with these terms.
*Correspondence: Paula V. Monje, cG1vbmplQGl1LmVkdQ==; Lingxiao Deng, ZGVuZ2xAaXVwdWkuZWR1; Xiao-Ming Xu, eHUyNkBpdXB1aS5lZHU=