Location Matters: Navigating Regional Heterogeneity of the Neurovascular Unit
- 1Djavad Mowafaghian Centre for Brain Health, University of British Columbia, Vancouver, BC, Canada
- 2Neuro-Electronics Research Flanders, Leuven, Belgium
- 3Vlaams Instituut voor Biotechnologie, Leuven, Belgium
- 4Interuniversity Microeletronics Centre, Leuven, Belgium
- 5Department of Neurosciences, KU Leuven, Leuven, Belgium
- 6Irving Medical Center, Columbia University, New York, NY, United States
- 7Queensland Brain Institute, University of Queensland, Brisbane, QLD, Australia
The neurovascular unit (NVU) of the brain is composed of multiple cell types that act synergistically to modify blood flow to locally match the energy demand of neural activity, as well as to maintain the integrity of the blood-brain barrier (BBB). It is becoming increasingly recognized that the functional specialization, as well as the cellular composition of the NVU varies spatially. This heterogeneity is encountered as variations in vascular and perivascular cells along the arteriole-capillary-venule axis, as well as through differences in NVU composition throughout anatomical regions of the brain. Given the wide variations in metabolic demands between brain regions, especially those of gray vs. white matter, the spatial heterogeneity of the NVU is critical to brain function. Here we review recent evidence demonstrating regional specialization of the NVU between brain regions, by focusing on the heterogeneity of its individual cellular components and briefly discussing novel approaches to investigate NVU diversity.
Introduction
The varied and complex tasks performed by the brain have energy demands that far outweigh those of other organs. Accordingly, the vascular system that supplies the brain has evolved into an intricate arrangement that is unique in its cellular composition and function. The neurovascular unit (NVU) is comprised of a diverse population of cells including neurons, endothelial cells (ECs), pericytes, astrocytes, vascular smooth muscle cells (vSMCs) and others, that act in a coordinated manner to spatially and temporally match the local blood supply to the energy demand of neural activity (Iadecola, 2017). Together, NVU cells form the blood brain barrier (BBB), a regulated semipermeable border unique to the brain that shields the parenchyma from the vessel lumen (Sweeney et al., 2019).
The complexity of neurovascular communications has been recognized for a long time; however, new tools have recently allowed us to distinguish the various cell populations of the NVU in greater detail. We now know that the NVU not only harbors diverse cell populations, but that these cell populations are regionally heterogeneous. This regional heterogeneity can be broadly divided into large and local scales. On a large scale, this review will highlight recent evidence showing that NVU cells, and the communication between them, vary depending on their location within specific anatomical or functional brain region. On a more local scale, spatial NVU cellular heterogeneity is also found along the vascular tree [see reviews (Wilhelm et al., 2016; Noumbissi et al., 2018; Villabona-Rueda et al., 2019)]. Recent demonstrations of such vessel type-dependent variability include gene expression analysis of ECs that line the vessel lumen, which suggests that BBB function differs with vascular tree location (Vanlandewijck et al., 2018). Abluminal to the ECs, distinct populations of mural cells exist from arteriolar smooth muscle cells to capillary pericytes. Different phenotypes of pericytes, whether defined by morphology or molecular identity, exist along the arterio-venular tree (Hartmann et al., 2015; Vanlandewijck et al., 2018). Likewise, astrocytic gene expression and end-foot coverage is partially governed by vessel type and size (Wang M. X. et al., 2021). New studies have also uncovered previously unrecognized roles for perivascular fibroblasts and perivascular macrophages, preferentially found in the arteriolar and venular compartments (Vanlandewijck et al., 2018; Koizumi et al., 2019). It is therefore becoming increasingly recognized that spatial heterogeneity of NVU components along this arteriole-capillary-venule axis is necessary to allow local neurovascular coupling (NVC) and functional hyperemia to take place. While this vessel type-dependent heterogeneity has been reviewed recently (Wilhelm et al., 2016; Noumbissi et al., 2018; Villabona-Rueda et al., 2019), NVU function also displays heterogeneity on a wider scale, where the vascular compartment needs to match the varying demands of distinct anatomical regions of the brain. Here, we review recent evidence showing that this regional variability in neurovascular communication relies on the individual cellular components of the NVU differing between the functional and anatomical regions of the brain (Figure 1) and briefly discuss new tools available to probe this spatial heterogeneity.
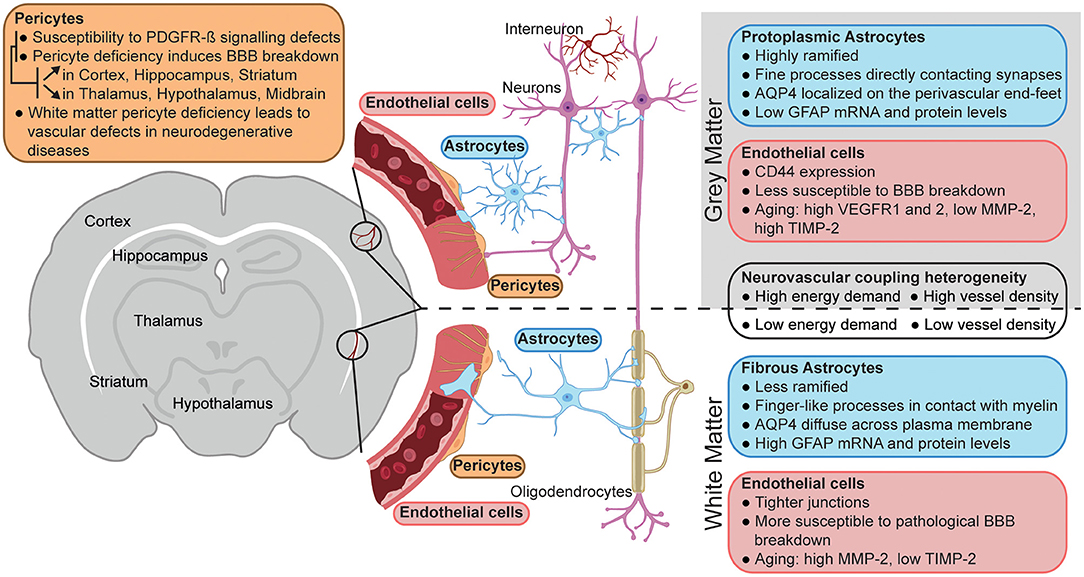
Figure 1. Neurovascular unit heterogeneity between gray and white matter. Schematic representation of the main differences between the neurovascular unit of gray (top) vs. white matter (bottom). The figure was created using BioRender.com.
Brain Region-Specific Neurovascular Coupling in Health and Disease
The brain is separated into anatomical regions that are interconnected yet functionally distinct. The relative specialization of each region allows the CNS to execute incredibly complex tasks, and their varying functions require adapted blood flow control. Furthermore, a widely different activity profile and cellular composition exists between synapse-rich gray matter areas and white matter harboring myelinated axonal fibers. More importantly for neurovascular activity, the corresponding energy demand of these diverse regions varies extensively. Glucose consumption is 2–4 times greater in gray matter (Sokoloff et al., 1977) where major energy-consuming processes like synaptic activity and action potential generation take place (Attwell and Laughlin, 2001). In white matter, resting potential maintenance and housekeeping functions in both neurons and oligodendrocytes are believed to require a comparatively lower metabolic demand (Harris and Attwell, 2012). The differential energy demands of gray matter and white matter translate into adapted microvascular structural architecture: vascular density is higher in gray matter, with arterioles and venules preferentially oriented perpendicular to cortical layers while vessels in white matter are organized parallel to axonal fibers (Cavaglia et al., 2001; Hase et al., 2019). This suggests that the coupling between local neural activity and blood flow needs to vary regionally, and recent data shows that a varying cellular composition of the NVU may underlie this adaptation.
Adding to evidence of regional heterogeneity of NVU activity under physiological conditions is the region-dependent neurovascular dysfunction observed in the pathogenesis of various brain diseases. It is now widely recognized that vascular abnormalities are key pathogenic features of cognitive decline. A group of disorders broadly termed vascular cognitive impairment and dementias (VCID) is thought to be responsible for ~20% of dementias (Iadecola, 2013; Gorelick et al., 2016; Kim et al., 2020). Cerebral small vessel disease (CSVD)—a disease of varying etiology that affects small arterioles and venules, and deep capillaries of the brain—underlies a majority of VCID cases. It results in impaired NVC, reduced blood flow, and increased BBB permeability. Neuroimaging characteristics of CSVD include subcortical infarcts and white matter hyperintensities, implying a regional component of CSVD pathogenesis (Shi and Wardlaw, 2016; Cuadrado-Godia et al., 2018). White matter abnormalities linked to cerebrovascular dysfunction are also a risk factor for Alzheimer's disease (AD) (Alber et al., 2019). The links between dementias and cerebrovascular dysfunction, specifically in white matter, have brought attention to the differential composition of the NVU between gray matter and white matter and the differential effects of NVU dysfunction on neural health. Taken together, the communication between the vascular system and neural parenchyma shows region-specific variability in health and disease, drawing interest to potential region-specific properties of the individual cell types making up the NVU.
Endothelial Cells
ECs lining CNS vessels form the first line of defense against circulating toxins and pathogens, while bearing responsibility for the entrance of indispensable nutrients and ions. This extremely selective control of permeability is achieved via three distinct features: (1) specialized tight junctions restricting paracellular flow, (2) low rates of transcytosis hampering transcellular transport, and (3) expression of membrane transporters mediating the influx of nutrients and the efflux of toxic waste (Biswas et al., 2020).
While endothelial heterogeneity along the arterio-venous tree has been extensively described (Sabbagh et al., 2018; Vanlandewijck et al., 2018), inter-regional differences in endothelial properties are subtle and mostly manifested in pathological conditions. BBB integrity is tightly linked to the expression pattern of junctional proteins in ECs and to restriction of transcytotic pathways and minor differences have been identified in the BBB signature of ECs in gray matter compared to white matter. In particular, the expression of junctional proteins occludin, claudin-5 and α-catenin is higher in white matter ECs compared to cortical ECs. Functionally, this reflects in vitro into a higher transendothelial electrical resistance of primary microvascular cells isolated from the white matter compared to those isolated from the cortex (Nyul-Toth et al., 2016). Although ECs in the white matter seemingly form a tighter paracellular barrier, white matter vessels are reported to be more susceptible than gray matter vessels to junctional fragmentation and loss of occludin and ZO-1 in pathological conditions such as HIV-associated neurocognitive deficits (Dallasta et al., 1999). Consistently with these observations, several groups have reported that white matter vasculature is more susceptible to pathological hyperpermeability. In multiple sclerosis (MS) patients, local BBB dysfunction predominantly affects white matter but not gray matter (Taheri et al., 2011). Additionally, several cognitive impairments, including VCID, AD and post-stroke dementia display marked BBB permeability specifically in the white matter, likely as a response to a marked loss of pericytes in those regions (Hase et al., 2018; Ding et al., 2020).
Regional variability of BBB-forming ECs is somewhat more prominent in the circumventricular organs (CVOs), where an active exchange between blood and the CNS is required. Compared to ECs elsewhere in the brain, ECs in CVOs have a lower expression of junctional proteins, higher rates of transcytosis and higher expression of the fenestration-forming plasmalemma vesicle-associated protein, resulting in increased permeability to tracers (Morita et al., 2016). This distinctively different signature of CVOs ECs may be due to low canonical Wnt signaling, as activation of Wnt/β-catenin pathway, by stabilizing β-catenin in ECs, induces a BBB-like phenotype (Benz et al., 2019; Wang et al., 2019). Similar to CVOs, a permeable vasculature is necessary in the choroid plexus (ChP), the site of cerebrospinal fluid (CSF) secretion. ECs in the ChP are fenestrated to allow the passage of water and small molecules. The blood-CSF barrier at the ChP is instead formed by a layer of epithelial cells surrounding choroidal vessels that regulate the permeability by expressing junctional proteins and membrane transporters (Dani et al., 2021).
Aging has very distinct and regional-specific effects on CNS vasculature. As both vascular density and branching decrease across most brain regions with a more pronounced vessel loss in white matter as a result of aging (Murugesan et al., 2012; Schager and Brown, 2020), ECs in white and gray matter activate specific pro-angiogenic pathways to maintain vessel health. In gray matter, ECs start expressing higher levels of vascular endothelial growth factor receptor-1 (VEGFR1) and VEGFR2, while Angiopoietin-1 (Ang1) is specifically upregulated in the cortex. In white matter, ECs upregulate the expression of the matrix metalloproteinase-2 (MMP-2) and downregulate its inhibitor TIMP-2; conversely, ECs in the cortex express less MMP-2 and more TIMP-2 during aging (Murugesan et al., 2012). While minimal decrease in vessel branching is observed in the hippocampus compared to cortex and white matter, it is the first brain area to show age-dependent increase in BBB permeability (Montagne et al., 2015), possibly as a response to the increase of Ang2 that destabilizes the vasculature (Murugesan et al., 2012). Studies focused specifically on hippocampal vasculature have shown a prominent switch from ligand-dependent transport to caveolar transport across the BBB in aged mice, together with a marked decrease in junctional and matrix organization (Yang et al., 2020).
Some regional heterogeneity in the expression of transporters and adhesion molecules on the EC membrane also exists. The expression of the efflux transporter P-glycoprotein (P-gp) was found to be higher in the cortex than in the cerebellum (Yasuda et al., 2015). Additionally, in epileptogenic tissue resected from the temporal lobe, P-gp expression was higher in gray matter capillaries, and its expression in both white and gray matter correlated with increased seizure recurrence (Kwan et al., 2010). Given the key role of P-gp in preventing drugs from entering the CNS parenchyma, identifying the variability in its distribution at the BBB is fundamental to address the mechanisms of pharmacoresistance (Liu et al., 2015). The expression of the adhesion molecule CD44 is restricted to ECs within gray matter (Kaaijk et al., 1997). This region-specific pattern is relevant in the context of neuroinflammation, as CD44 mediates the interaction of ECs with immune cells and its deficiency increases BBB disruption in an experimental autoimmune encephalitis (EAE) model (Flynn et al., 2013).
Aside from the features outlined in this review, the regional heterogeneity of ECs is still largely unknown. A deeper investigation in this variability is fundamental when modeling vascular pathology in vitro, since the area and method of isolation of ECs from the brain may influence their phenotype and behavior in culture.
Mural Cells
Mural cells are critical players in the control of local blood flow. They line most vessels of the brain and include vSMCs and pericytes. vSMCs are arranged in circumferential bands wrapping around arterioles where they are key mediators of vascular contractility and in a mesh-like pattern on venules. Pericytes line capillaries and post-capillary venules and are embedded within the basement membrane with a characteristic bump-on-a-log cell body. Pericytes are morphologically heterogeneous, with a cell shape highly correlated to its relative location along the arteriole-capillary-venule axis. “Ensheating pericytes” expressing contractile machinery populate 1st–4th-order branches, “thin-strand pericytes” are located along deeper capillaries and “mesh pericytes” are found on post-capillary venules. Pericyte heterogeneity is such that identification of putative subpopulations requires a combination of location, morphology, marker expression, and function. The nomenclature and the defining molecular markers attributed to each type of pericyte, and the presence of discrete subpopulations vs. a gradual continuum of phenotypes remains hotly debated (Hartmann et al., 2015; Hill et al., 2015; Attwell et al., 2016; Vanlandewijck et al., 2018). While mural cell populations along the arterial-venous tree are locally diverse, recent studies also hint at a functional diversity of mural cells, most notably pericytes, on a larger scale with brain region-dependent characteristics (Hartmann et al., 2015; Cudmore et al., 2017; Nikolakopoulou et al., 2017; Villasenor et al., 2017).
Pericytes are found lining vessels in all brain regions; however, the density of platelet-derived growth factor receptor-β (PDGFR-β)-expressing pericytes in the cortex inversely correlates with neural cell density, such that neuron-rich layers II/III of the cortex contain 40% less pericytes than layer I (Hartmann et al., 2015). Although vascular density itself may differ between cortical layers, this observation was corroborated by a study detailing a decreased density of CD13+ pericytes per capillary length in layer II/III (Cudmore et al., 2017). With pericyte presence often linked to tonic vessel constriction (Fernandez-Klett et al., 2010; Hall et al., 2014; Gonzales et al., 2020; Hartmann et al., 2021), it is suggested that a higher pericyte density may act as a buffer to blood flow pressures in upper cortical layers while lower pericyte density in cell body-rich layers may contribute to vessel relaxation necessary for NVC in areas with higher metabolic demand. A recent whole-brain analysis of pericyte density showed elevated density of PDGFRβ-expressing pericytes in deeper cortical layers (layers IV and V) relative to layer I, with pericyte density generally matching vascular density across most regions (Wu et al., 2021). Notable exceptions were thalamic areas, where a relatively higher pericyte density (relative to local vascular density) was observed.
In addition to their role in the control of vascular tone, pericytes participate in angiogenesis and vessel stabilization, as well as in the maturation and maintenance of BBB integrity. Interestingly, this key role of pericytes in BBB maintenance was shown to vary substantially among anatomical brain regions. Signaling through PDGFR-β on pericytes is key for pericyte recruitment to vessels and BBB generation and maintenance (Armulik et al., 2010; Daneman et al., 2010). Disruption of the PDGF-B/PDGFR-β signaling axis induces pericyte loss, disruption of pericyte-endothelial communication and subsequent vascular defects and BBB dysfunction (Armulik et al., 2010; Bell et al., 2010; Daneman et al., 2010; Mae et al., 2021). This property has been investigated by various groups to demonstrate region-specific contributions of pericytes to BBB function. In one model where genetic ablation of the retention motif of PDGF-B prevents pericyte recruitment (pdgf-bret/ret) (Armulik et al., 2010), the subsequent BBB leakage is elevated in the cortex, hippocampus and striatum compared to deeper areas such as the interbrain and midbrain (Villasenor et al., 2017). The regional variability in extravasation of Evans Blue and Gd-DTPA and parenchymal IgG accumulation was observed in pdgf-bret/ret mice despite a uniform reduction of CD13+ pericyte coverage throughout those same brain regions (~75% less than in controls). This suggests that the relative importance of pericytes in maintaining BBB integrity varies among brain regions, beyond their local susceptibility to PDGFR-β signaling defects. In another model of pericyte deficiency, where 7 point mutations on the pdgfrb gene (pdgfrbF7/F7) (Tallquist et al., 2003) prevents normal endothelial-pericyte signaling, regional differences in the BBB dependence on pericyte presence was confirmed. The progressive loss of CD13+ pericytes induced elevated BBB breakdown in the cortex, hippocampus and striatum as compared to thalamus (Nikolakopoulou et al., 2017). In this transgenic model, as well as in a pericyte-specific ablation model based on double expression of PDGFR-β and NG2 (Nikolakopoulou et al., 2019), BBB dysfunction directly correlated with regional pericyte loss. Taken together, the vulnerability of NVU function to pericyte loss is region-dependent, and the general resilience of thalamic areas to pericyte dysfunction may be linked to the elevated pericyte:vessel ratio these regions harbor (Wu et al., 2021).
Nevertheless, pericyte vulnerability shows a component of regional variability, which may play an important role in several pathologies. Vascular defects in white matter of the brain, and pericyte dysfunction, are believed to be partially responsible for multiple types of dementias (Sagare et al., 2013; Halliday et al., 2016; Alber et al., 2019; Nortley et al., 2019; Ding et al., 2020). In pdgfrbF7/F7 mice, pericyte loss was prominently observed in white matter tracts, such as corpus callosum, in association with BBB leakage and accumulation of blood-borne fibrinogen (Montagne et al., 2018). Such deposits of fibrinogen are thought to be toxic to oligodendrocytes, inducing demyelination and white matter damage. In multiple sclerosis (MS), the nature of the associated neurological deficits are highly dependent on the location of focal inflammatory immune cell infiltration and ensuing neural tissue lesions (Alvarez et al., 2015). When EAE, a mouse model of MS, was combined with the pdgf-bret/ret model of pericyte deficiency, the regional immune infiltration correlated with regional pericyte loss (Torok et al., 2021). The local reduction in pericyte coverage drives an increased VCAM-1 and ICAM-1 expression on ECs, thereby facilitating trafficking of pro-inflammatory CD45+ leukocytes into the brain. Since vascular defects and BBB dysfunction are hallmarks of neurodegenerative diseases, this highlights how a regional variation in pericyte susceptibility to disturbances in PDGFR-β signaling, or other yet unrecognized pathways, may in fact underlie the regional component of those diseases.
The mechanisms that dictate pericyte heterogeneity across brain regions are still poorly understood, but regionally specialized functions may drive a certain pericyte phenotype. For example, in the paraventricular nucleus, where vessel density is 3–5-fold higher than in the cortex, pericyte coverage of vessels is also significantly higher (Frahm and Tobet, 2015). In CVOs, where the BBB lacks tight junctions to allow sensing of blood-borne cues such as osmolarity, pericytes display especially high levels of NG2 and PDGFR-β (Morita et al., 2014). These levels were increased upon chronic osmotic stimulation induced via salt-loading, unlike in the cortex where they remained unchanged. Interestingly, this challenge also promoted BBB leakage in CVOs. Ontogenic variability may also play a role in the observed heterogeneity of pericytes among anatomical brain regions. Indeed, the developmental origin of brain pericytes is heterogeneous, with studies demonstrating neural crest lineage, mesenchymal lineage and hematopoietic sources (Etchevers et al., 2001; Trost et al., 2016; Yamamoto et al., 2017; Yamazaki and Mukouyama, 2018). The ontogeny of pericytes in specific brain regions remains to be elucidated but is likely to participate in the regional heterogeneity of pericyte function.
Astrocytes
Maintenance of the BBB is not a function of endothelial and mural cells alone, but a dynamic process that involves parenchymal cells including astrocytes, which express a specific molecular repertoire essential for the physiology of the vascular system.
Astrocytes are a heterogeneous subtype of glial cells that play key roles in BBB formation and upkeep, blood flow regulation and vascular contractility in response to neural activity (Abbott et al., 2010; Alvarez et al., 2013; Boulay et al., 2016). They release glia-derived neurotrophic factors and provide metabolic support to neurons (Sofroniew and Vinters, 2010; Allaman et al., 2011; Allen and Eroglu, 2017). Astrocytes display both inter- and intra-regional differences in their morphology, gene expression, and physiological role. Differences in astrocytic distribution and morphology between white and gray matter reflect the distinct functions of these CNS components and meet the specific functional requirements that each astrocytic population must fulfill. In white matter, oligodendrocytes create a myelin sheath around axonal fibers (Marques et al., 2016) that restricts direct contact of fibrous astrocytes with axonal fibers to gaps in this sheath called nodes of Ranvier (Black and Waxman, 1988; Lubetzki et al., 2020). These fibrous astrocytes are small with few but elongated, finger-like branches and organized in rows around white matter tracts (Miller, 2018; Matias et al., 2019). Conversely, gray matter—or protoplasmic—astrocytes have fine processes that envelope ~200,000 synapses in mice, and ~2 million in humans (Oberheim et al., 2006). Protoplasmic astrocytes are highly ramified cells, with greater process arborization in layers II/III than in layer IV (Lanjakornsiripan et al., 2018). Patterns of gene expression specific to gray matter astrocytes differ between cortical layers and areas (Morel et al., 2019; Batiuk et al., 2020; Bayraktar et al., 2020), suggesting a high degree of intra-regional heterogeneity in the mouse brain. Importantly, astrocytes not only contact neuronal elements, but also directly interact with the vasculature via specialized end-feet.
A critical role of astrocytes is to maintain the barrier properties of endothelial cells forming the BBB. Astrocyte cell bodies are on average located 6–10 μm from blood vessels (McCaslin et al., 2011), and their end-feet form a continuous sheath around all vessels below the pia including arterioles, capillaries, and venules (McCaslin et al., 2011). Astrocytic end-feet cover 90–99% of the brain's vasculature (Abbott et al., 2006; Mathiisen et al., 2010; Lundgaard et al., 2014). This astrocytic extension to the vessels' wall forms a subcellular domain dedicated to the gliovascular interaction with abundant aquaporin-4 (AQP4), potassium channels (KIR4.1), glucose transporter 1 (GLUT-1/SLC2A1), and connexin-43 (Abbott et al., 2006; Jessen et al., 2015), highlighting the astrocytes' role in regulating water transport as well as blood and glymphatic flow—the product of the exchange of the brain interstitial and cerebrospinal fluid mediated by AQP4 and responsible for the brain's waste clearance via the glymphatic pathway (Iliff and Simon, 2019; Rasmussen et al., 2021). Along the vascular tree, the perivascular astrocytic sheath in the somatosensory cortex is thicker in arterioles than in capillaries (Jammalamadaka et al., 2015). However, capillaries have the greatest density of astrocyte end-foot processes, and the separation between astrocytes and capillaries decreases with increasing cortical depth (McCaslin et al., 2011).
Inter-regional differences in astrocytes can be observed in their protein expression (Morel et al., 2017). A prime example of regional differences is AQP4, a channel protein selectively permeable to water that is highly expressed in astrocytic membranes at the BBB and blood-CSF barrier, indicating its role in controlling bidirectional fluid exchange (Papadopoulos and Verkman, 2013). Interestingly, in vivo AQP4 deletion did not affect BBB permeability to macromolecules, with no changes in glial fibrillary acidic protein (GFAP) expression, or microvessels ECs morphology (Saadoun et al., 2009; Haj-Yasein et al., 2011). However, the consequent uncoupling of water and potassium transport can determine susceptibility to other conditions since a decrease in AQP4 leads to an increase in propensity for seizures and cognitive decline (Yang et al., 2011) and participates in pathologies associated to brain edema (Bonomini and Rezzani, 2010; Stokum et al., 2015). While the distribution pattern of AQP4 mRNA is homogeneous across the cortex and white matter, the protein itself is expressed in the white matter at almost half of the level detected in the cortex (Nyul-Toth et al., 2016)—likely due to the lower levels of vessel density that characterize the white matter. AQP4 in protoplasmic astrocytes is preferentially localized to the perivascular end-feet, while fibrous astrocytes show a more uniform localization across the plasma membrane (Stokum et al., 2015). This difference in distribution of one of the main regulators of water homeostasis could explain why white matter is more vulnerable to swelling and edema under ischemic conditions.
Astrocytes are highly coupled via gap junctions (Cotrina and Nedergaard, 2012) comprised of connexins Cx30 and Cx43, which allow astrocytes to exchange ions and molecules below 1.5 kDa with adjacent cells by connecting their cytoplasm (Bruzzone et al., 1996). Double deletion of Cx30 and Cx43 downregulates AQP4 (Nielsen et al., 1997) and weakens the BBB, which becomes permeable to macromolecules upon an increase in vascular pressure (Ezan et al., 2012). Interestingly, the expression of these connexins is not uniform across anatomical circuits: corpus callosum astrocytes are less coupled to each other by gap junctions than their counterparts in the neocortex (Haas et al., 2006), showing that gap junctional communication between astrocytes can differ among brain regions. Whether these regional differences in connexin expression directly affect communication between astrocytes and ECs remains to be clarified.
Astrocytes express high levels of GFAP, which is also upregulated in reactive astrocytes and is often associated with the severity of CNS disorders (Escartin et al., 2019), though this correlation may be impacted by the regional differences in basal GFAP expression (Griemsmann et al., 2015; Ben Haim and Rowitch, 2017). For instance, hippocampal astrocytes display a higher level of GFAP than cortical, thalamic, or striatal astrocytes (Bushong et al., 2002; Chai et al., 2017). GFAP expression is also higher in astrocytes isolated from the corpus callosum compared to those in cortical structures (Goursaud et al., 2009). In fact, mRNA and proteins levels of GFAP are 3–7-fold higher in white matter than in gray matter (Nyul-Toth et al., 2016). Although direct investigations in the mechanistic role of GFAP in NVU function remain limited, its region-dependent expression pattern is involved in white matter vs. gray matter vascular properties since GFAP deletion increases the permeability to macromolecules of the BBB in white matter and specifically reduces vascularization in white matter compared to gray matter (Liedtke et al., 1996).
Regional identities of astrocytes are maintained over time, and evidence has shown that aged astrocytes from different brain regions present a unique molecular signature (Boisvert et al., 2018; Clarke et al., 2018). Significant differences in age-related changes in gene expression have been found between hippocampus, striatum and cortex, with an up-regulation of astrocytic reactive genes in the hippocampus and striatum—regions that show more susceptibility to oxidative stress and other environmental stressors (Saxena and Caroni, 2011)—compared to the cortex (Clarke et al., 2018). Accordingly, RNA sequencing studies on aging astrocytes from different regions showed that cortical astrocytes undergo minimal changes in gene expression (<100 differentially regulated genes) compared to cerebellar astrocytes (>500 genes). Interestingly, an age-dependent increase in GFAP expression has been shown in the hippocampus, but also in the frontal and temporal cortices (Boisvert et al., 2018). However, functional implication of GFAP upregulation during aging and CNS disorders is still controversial. A failure of astrocytes to adequately adapt to their roles in areas affected by aging may lead to a loss of neuronal populations due to insufficient vascularization, and differences in the regional expression of key proteins may be crucial to our understanding of the origin and progression of neurodegenerative diseases.
Tools to Study Regional Heterogeneity of the NVU
Investigations in the NVU diversity demonstrate region-dependent cellular variations, however direct functional investigations remain necessary to evaluate how these differences in NVU composition affect local brain functions. In this regard, the development of novel neuroimaging modalities suitable for brain-wide recordings has expanded the toolset required to probe the regional heterogeneity of the NVU that was until recently mostly addressed by single cell RNA sequencing (scRNAseq) and functional magnetic resonance imaging (fMRI). The former is a major contributor in characterizing the single cell transcriptomic profile of the cellular and vascular components of the NVU highlighting region-specific differences at the brain-wide scale (Saunders et al., 2018; Ross et al., 2020) and beyond what can be achieved with conventional imaging modalities. The latter, fMRI, is a gold standard modality suitable to capture the heterogeneity of hemodynamic responses at the whole brain scale (Devonshire et al., 2012) and between white and gray matter (Gawryluk et al., 2014). However, when considering NVU/C investigations scRNAseq lacks the functional interplay between cellular and vascular components while fMRI approach remains limited by a reduced spatiotemporal resolution when compared with other modalities (Urban et al., 2017), the need of anesthetic drugs when considering their impact on the NVC (Aksenov et al., 2015), and its lack of cellular component compensated by complementary modalities (Mishra et al., 2011; Aksenov et al., 2015).
Hereafter we focus on recent strategies allowing for cellular and/or vascular imaging under conscious conditions enabling to preserve the physiological integrity of the NVC (Masamoto and Kanno, 2012; Reimann and Niendorf, 2020). While acting at different spatiotemporal scales, multi-photon microscopy (MPM; 2- and 3-photon microscopy), miniaturized fluorescence microscopes (Miniscope), multi-fiber photometry (MFP), and functional ultrasound imaging (fUSI) modalities are complementary methods providing information on the interplay between the cellular and vascular components of the NVU regionally and at the larger, whole brain scale (Figure 2). For further comparison of neuroimaging methods described in this section, we also refer the readers to Urban et al. (2017) and Walter et al. (2020).
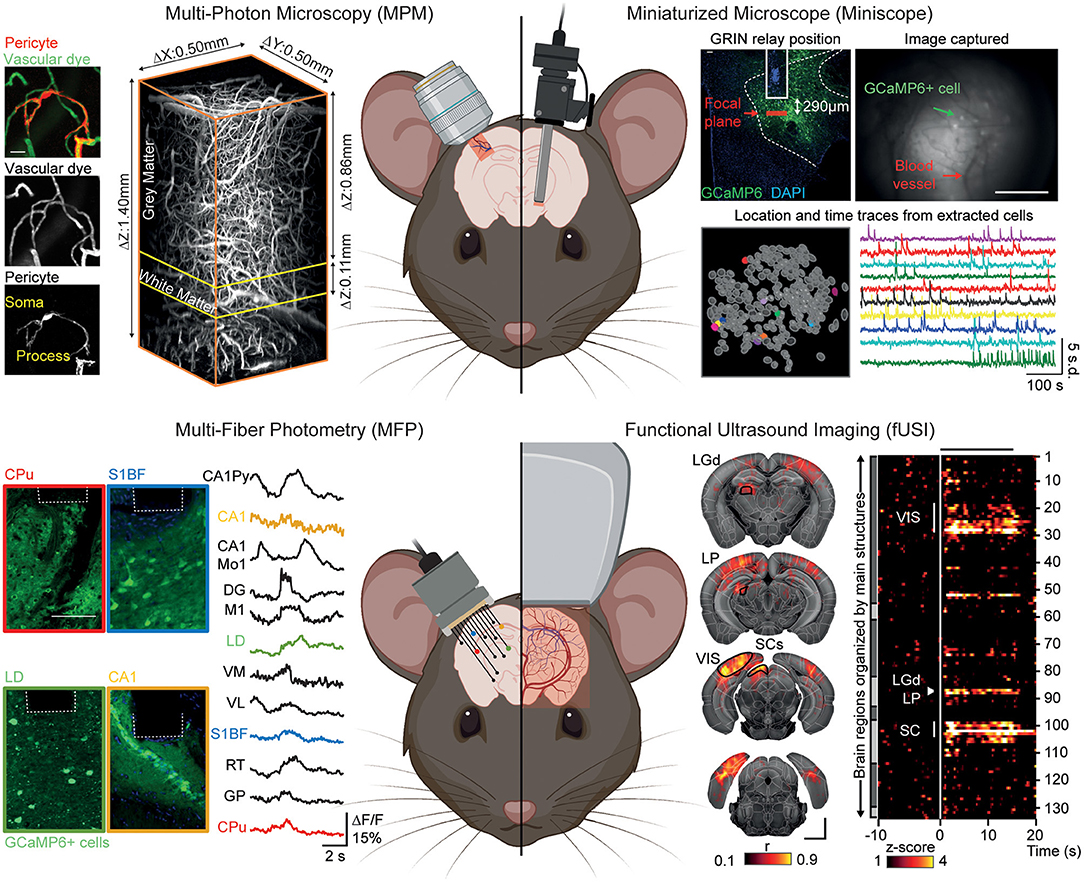
Figure 2. Neuroimaging modalities to investigate the regional heterogeneity of the neurovascular unit under awake conditions. Top left: Multi-photon microscopy (MPM) allows high-resolution imaging of cell-specific activities (here, pericytes) and vascular dynamics using fluorescent dyes in a cortical column and deeper regions. Top right: Miniaturized microscope (Miniscope) suitable for imaging of single cell activity and blood vessels from deep brain regions (here, lateral hypothalamus) by the mean of a GRIN relay. Bottom left: Multi-fiber photometry (MFP) allows for simultaneous recording of cellular changes (here, GCaMP6m-expressing cells) from across, but not limited to, 12 individual brain regions. Bottom right: Functional ultrasound imaging (fUSI) allows for high sensitivity recording of hemodynamic changes with a brain-wide coverage of more than 130 regions per hemisphere. Reprinted with permission from Li B. et al. (2020), Berthiaume et al. (2018), Resendez et al. (2016), Sych et al. (2019), and Brunner et al. (2020). The figure was created using BioRender.com.
Multi-photon microscopy (MPM) is the “gold standard” for the study of cellular dynamics, function and morphology as well as hemodynamics (Shih et al., 2012; O'Herron et al., 2016; Urban et al., 2017) in a low- to non-invasive way [i.e., cranial window (Goldey et al., 2014), thinned-skull (Drew et al., 2010), or transcranial (Wang et al., 2018)]. The capabilities of MPM (Urban et al., 2017; Li B. et al., 2020) combined with cell-specific genetically encoded activity reporters suited for probing either calcium or voltage changes (GECIs and GEVIs) (Lin and Schnitzer, 2016) has allowed the simultaneous recording of many cell populations involved in NVC [neurons (Urban et al., 2017), roles of astrocytes in the control of arteriole diameter, increase in local blood flow (Takano et al., 2006; Tran and Gordon, 2015), microglia as important regulators of blood flow during NVC (Hierro-Bujalance et al., 2018; Császár et al., 2021), pericytes control blood flow direction at capillary junctions, maintenance of capillary flow resistance and metabolic exchanges (Berthiaume et al., 2018; Gonzales et al., 2020)] and/or BBB permeability (Knowland et al., 2014). Along with cell-specific imaging, MPM can record local volumetric hemodynamic changes [blood flow and red blood cell velocity (Urban et al., 2017)] from surface pial vessels down to deep capillaries (Fan et al., 2020) with dedicated circulating fluorescent contrast agents (Miller et al., 2017). The unique combination of approaches offered by MPM makes it a tool of choice for investigating the complex interplay between cellular functions and vascular dynamics under awake conditions. Furthermore, the development of MPM allowing deeper imaging with better resolution (Miller et al., 2017; Wang et al., 2018) together with new faster and brighter GECIs (Dana et al., 2019; Inoue et al., 2019) to improve imaging capabilities in the mouse brain now allows to address the neurovascular components in the entire depth of the cortex, the white matter (Li B. et al., 2020) and down to the dorsal layers of the hippocampus (Ouzounov et al., 2017).
To better investigate the NVU of deeper brain areas, Miniscopes offer the opportunity to image up to 1,000 cells (Ziv et al., 2013) from distinct populations at single-cell resolution in freely behaving animals (Aharoni and Hoogland, 2019) using GECIs or GEVIs. Miniscope designs afford wide-field imaging of the cortical surface that can be extended to the entire cortical column by the means of microprism allowing for recording of both cell assembly and hemodynamics (Chia and Levene, 2009; Andermann et al., 2013; Low et al., 2014; Gulati et al., 2017) to various deep brain areas [e.g., hippocampus (Ziv et al., 2013), striatum (de Groot et al., 2020), hypothalamus (Resendez et al., 2016)] via gradient-index (GRIN) lens and relay. For example, using two GRIN relays Barretto et al. (2011) chronically monitored the progressive distortion of the microvasculature (i.e., architecture and velocity) due to glioma angiogenesis as well as properties of hippocampal neurons. To adapt the Miniscope design for hemodynamic measurements, Senarathna et al. (2019) added intrinsic optical signal (Grinvald et al., 1986) and laser speckle contrast (Miao et al., 2011) channels, enabling imaging of cerebral blood volume and flow over wide areas. Showing the Miniscope approach can also be used for BBB studies, Barr et al. (2020) used circulating contrast agents to image cocaine-induced BBB leakage in freely moving rats. Conceivably, Miniscopes will allow for comparative NVU imaging in deep regions of the brain, however hemodynamic recordings and BBB permeability are poorly addressed (often excluded from analysis) using these tools while so far limited to superficial layers of the cortex.
In contrast to the limited brain-wide capabilities of MPM and Miniscope, the MFP modality enables large-scale investigations through simultaneous recording of tens of cortical and subcortical regions of the mouse brain under freely-moving conditions (Pisano et al., 2019; Sych et al., 2019). As with MPM and Miniscope, MFP requires genetically encoded biosensors to collect changes in neuronal (Wang Y. et al., 2021) and astrocyte activity (Paukert et al., 2014; Schlegel et al., 2018) and supports simultaneous dual-color recordings that allows for combined recordings of different cell types (Meng et al., 2018). Despite its sensitivity and brain-wide coverage, MFP remains constrained to bulk changes and lacks single-cell resolution making investigations into specific non-neuronal cell population activity, hemodynamics [limited to O2 saturation measurement (Yu et al., 2020)] and BBB function poorly accessible so far.
From a hemodynamic point-of-view, functional ultrasound imaging (fUSI) can be considered as an alternative to fMRI by monitoring blood velocity and cerebral blood volume changes in the entire brain depth at high spatiotemporal resolution (Mace et al., 2011). Three major developments have broadened the fUSI application to neuroscience: (i) ultrasound transducer miniaturization allowed freely-behaving investigation (Urban et al., 2015), (ii) ultrasound transducer motorization achieved brain-wide coverage therefore extending the detection of activity to ~250 brain regions (Mace et al., 2018), and (iii) brain-wide volumetric imaging of behaving rodents by the mean of matrix transducer (Brunner et al., 2020). Similarly to optical modalities discussed above, several strategies have been proposed to reduce the ultrasound attenuation of the skull from transcranial (Tiran et al., 2017), thinned skull (Urban et al., 2014) to stabilized cranial window suited for awake imaging (Mace et al., 2018; Brunner et al., 2020). While fUSI enables the study of NVC regional heterogeneity (Mace et al., 2011, 2018; Sans-Dublanc et al., 2021), it has never been employed to address large scale white matter hemodynamics or BBB functions. Unlike MPM, Miniscope or MFP, fUSI cannot track cell activity via genetically-encoded indicators but can be combined with electrophysiology (Mace et al., 2018; Nunez-Elizalde et al., 2021; Sans-Dublanc et al., 2021) and cell-specific optogenetic tools (Brunner et al., 2020; Sans-Dublanc et al., 2021).
Also employed in the preclinical cerebrovascular realm, optical coherence tomography [OCT; reviewed by Yao and Wang (2014)] and optoacoustic [OA; reviewed by Ovsepian et al. (2017)] neuroimaging modalities actively support investigations on NVU/C. OCT allows for high resolution angiography of the cortical depth down to the hippocampus and including the white matter (Chong et al., 2015; Park et al., 2018). However, functional monitoring of local blood flow, velocity and O2 saturation (Gagnon et al., 2016) suitable under awake conditions (Li et al., 2020) are restricted to small cortical regions strongly limiting the investigation of the NVU and its heterogeneity in the entire brain. On the other hand, OA affords high resolution angiography of the cortical vascular tree but lacks spatial resolution and tissue penetration when probing mesoscale hemodynamics [i.e., hemoglobin oxygenation, O2 saturation (Burton et al., 2013; Tang et al., 2016)] and neural activity (Gottschalk et al., 2019). Such constraint makes OA modality limited to address the regional heterogeneity of the neurovascular unit.
Overall, imaging modalities described here range from low to high invasiveness with thinned skull or cranial window preparations (MPM and fUSI) up to insertion of device into the brain tissue (Miniscope, MFP) that may lead to structural and functional damage to the brain [i.e., tissue inflammation (Dorand et al., 2014; Bocarsly et al., 2015); brain lesions (Cole et al., 2011; Jacob et al., 2018); vessels leaking (Chia and Levene, 2009); spreading depolarization (Srienc et al., 2019)] affecting the integrity of NVU/C and inducing confounding effects in the brain cortex. These considerations further highlight the need for appropriate controls or exclusion criterion in studies using these approaches.
Beyond the established methods discussed above, the potential of combining different modalities is huge and can provide crucial information to better understand the heterogeneity and complexity driving the NVU/C at the whole brain scale. For example, scRNAseq has been performed after combined MPM and electrophysiological recordings highlighting the functional pattern and the transcriptional profile of tagged cells (Liu et al., 2020) paving the way to a better characterization of the NVU components and functions. The versatility and limited invasiveness of MFP modality allow for combination with fMRI (Schulz et al., 2012; Schlegel et al., 2018) suited for hemodynamic recordings and BBB investigations. Furthermore, the dual MFP/fUSI monitoring could provide very helpful information on the NVU heterogeneity as they generate very complementary signals (i.e., cellular and vascular) at the brain-wide scale. Similarly, Lake et al. (2020) simultaneously recorded wide-field calcium imaging with fMRI to investigate cortex-wide cell-specific activity with whole-brain hemodynamics. Moreover, Boido et al. (2019) compared the vascular and neuronal signals captured with MPM with hemodynamic changes monitored with fUSI and fMRI in the same animal.
While these studies were mostly focused on neuronal activity, the diversity of cell-specific GECI/GEVIs, optogenetic constructs and transgenic animals now available combined with the methods discussed above should support the investigation of the vascular and cellular components of the NVU at the brain-wide scale in awake rodents.
Concluding Remarks
It is now well-accepted that the NVU displays a high degree of heterogeneity, encompassing diverse cell types and varying its composition among vessel types and anatomical regions. Recent data highlighted here is likely only a hint of how the NVU exhibits specialized function to adapt to the varied environments of the brain. Intense research on the vascular landscape of areas like the choroid plexus, where a barrier more permeable than the BBB is critical to CSF production, will surely reveal new functions to the “classical” NVU cells (Liddelow, 2015; Lun et al., 2015; Kaur et al., 2016). These cells making up the NVU, once thought limited to ECs, astrocytes, pericytes and neurons, are also joined by “newcomers,” with perivascular fibroblasts, perivascular macrophages, resident microglia and others now believed to play an active role in cerebrovascular activity (Koizumi et al., 2019; Ross et al., 2020). With each of these cell types also displaying their own regional heterogeneity across the brain (Masuda et al., 2020), it is easy to imagine that even if the cerebrovascular compartment connects into one continuum, it may in fact harbor multiple somewhat distinct regions of specialized cellular composition and function. With techniques continually improving to provide better access to the cerebrovasculature, our vision of the NVU may move from a global “one NVU fits all” perspective, to a more nuanced one where the NVU is not only metabolically serving a brain region, but participating in its specialization.
Author Contributions
All authors listed have made a substantial, direct and intellectual contribution to the work, and approved it for publication.
Funding
This work was supported by Fondation Leducq (15CVD02), Canadian Institutes for Health Research (L-PB), FWO (MEDI-RESCU2-AKUL/17/049, G091719N, and 1197818N), VIB Tech-Watch (fUSI-MICE), Neuro-Electronics Research Flanders (NERF) TechDev fund (3D-fUSI project) (CB), and the Brazil Family Program for Neurology (MB).
Conflict of Interest
The authors declare that the research was conducted in the absence of any commercial or financial relationships that could be construed as a potential conflict of interest.
References
Abbott, N. J., Patabendige, A. A., Dolman, D. E., Yusof, S. R., and Begley, D. J. (2010). Structure and function of the blood-brain barrier. Neurobiol. Dis. 37, 13–25. doi: 10.1016/j.nbd.2009.07.030
Abbott, N. J., Ronnback, L., and Hansson, E. (2006). Astrocyte-endothelial interactions at the blood-brain barrier. Nat. Rev. Neurosci. 7, 41–53. doi: 10.1038/nrn1824
Aharoni, D., and Hoogland, T. M. (2019). Circuit investigations with open-source miniaturized microscopes: past, present and future. Front. Cell. Neurosci. 13:141. doi: 10.3389/fncel.2019.00141
Aksenov, D. P., Li, L., Miller, M. J., Iordanescu, G., and Wyrwicz, A. M. (2015). Effects of anesthesia on BOLD signal and neuronal activity in the somatosensory cortex. J. Cereb. Blood Flow Metab. 35, 1819–1826. doi: 10.1038/jcbfm.2015.130
Alber, J., Alladi, S., Bae, H. J., Barton, D. A., Beckett, L. A., Bell, J. M., et al. (2019). White matter hyperintensities in vascular contributions to cognitive impairment and dementia (VCID): knowledge gaps and opportunities. Alzheimers Dement. 5, 107–117. doi: 10.1016/j.trci.2019.02.001
Allaman, I., Belanger, M., and Magistretti, P. J. (2011). Astrocyte-neuron metabolic relationships: for better and for worse. Trends Neurosci. 34, 76–87. doi: 10.1016/j.tins.2010.12.001
Allen, N. J., and Eroglu, C. (2017). Cell biology of astrocyte-synapse interactions. Neuron 96, 697–708. doi: 10.1016/j.neuron.2017.09.056
Alvarez, J. I., Katayama, T., and Prat, A. (2013). Glial influence on the blood brain barrier. Glia 61, 1939–1958. doi: 10.1002/glia.22575
Alvarez, J. I., Saint-Laurent, O., Godschalk, A., Terouz, S., Briels, C., Larouche, S., et al. (2015). Focal disturbances in the blood-brain barrier are associated with formation of neuroinflammatory lesions. Neurobiol. Dis. 74, 14–24. doi: 10.1016/j.nbd.2014.09.016
Andermann, M. L., Gilfoy, N. B., Goldey, G. J., Sachdev, R. N., Wolfel, M., McCormick, D. A., et al. (2013). Chronic cellular imaging of entire cortical columns in awake mice using microprisms. Neuron 80, 900–913. doi: 10.1016/j.neuron.2013.07.052
Armulik, A., Genove, G., Mae, M., Nisancioglu, M. H., Wallgard, E., Niaudet, C., et al. (2010). Pericytes regulate the blood-brain barrier. Nature 468, 557–561. doi: 10.1038/nature09522
Attwell, D., and Laughlin, S. B. (2001). An energy budget for signaling in the grey matter of the brain. J. Cereb. Blood Flow Metab. 21, 1133–1145. doi: 10.1097/00004647-200110000-00001
Attwell, D., Mishra, A., Hall, C. N., O'Farrell, F. M., and Dalkara, T. (2016). What is a pericyte? J. Cereb. Blood Flow Metab. 36, 451–455. doi: 10.1177/0271678X15610340
Barr, J. L., Brailoiu, G. C., Abood, M. E., Rawls, S. M., Unterwald, E. M., and Brailoiu, E. (2020). Acute cocaine administration alters permeability of blood-brain barrier in freely-moving rats- Evidence using miniaturized fluorescence microscopy. Drug Alcohol Depend. 206:107637. doi: 10.1016/j.drugalcdep.2019.107637
Barretto, R. P., Ko, T. H., Jung, J. C., Wang, T. J., Capps, G., Waters, A. C., et al. (2011). Time-lapse imaging of disease progression in deep brain areas using fluorescence microendoscopy. Nat. Med. 17, 223–228. doi: 10.1038/nm.2292
Batiuk, M. Y., Martirosyan, A., Wahis, J., de Vin, F., Marneffe, C., Kusserow, C., et al. (2020). Identification of region-specific astrocyte subtypes at single cell resolution. Nat. Commun. 11:1220. doi: 10.1038/s41467-019-14198-8
Bayraktar, O. A., Bartels, T., Holmqvist, S., Kleshchevnikov, V., Martirosyan, A., Polioudakis, D., et al. (2020). Astrocyte layers in the mammalian cerebral cortex revealed by a single-cell in situ transcriptomic map. Nat. Neurosci. 23, 500–509. doi: 10.1038/s41593-020-0602-1
Bell, R. D., Winkler, E. A., Sagare, A. P., Singh, I., LaRue, B., Deane, R., et al. (2010). Pericytes control key neurovascular functions and neuronal phenotype in the adult brain and during brain aging. Neuron 68, 409–427. doi: 10.1016/j.neuron.2010.09.043
Ben Haim, L., and Rowitch, D. H. (2017). Functional diversity of astrocytes in neural circuit regulation. Nat. Rev. Neurosci. 18, 31–41. doi: 10.1038/nrn.2016.159
Benz, F., Wichitnaowarat, V., Lehmann, M., Germano, R. F., Mihova, D., Macas, J., et al. (2019). Low wnt/beta-catenin signaling determines leaky vessels in the subfornical organ and affects water homeostasis in mice. Elife 8:44. doi: 10.7554/eLife.43818.044
Berthiaume, A. A., Grant, R. I., McDowell, K. P., Underly, R. G., Hartmann, D. A., Levy, M., et al. (2018). Dynamic remodeling of pericytes in vivo maintains capillary coverage in the adult mouse brain. Cell Rep. 22, 8–16. doi: 10.1016/j.celrep.2017.12.016
Biswas, S., Cottarelli, A., and Agalliu, D. (2020). Neuronal and glial regulation of CNS angiogenesis and barriergenesis. Development 147:dev182279. doi: 10.1242/dev.182279
Black, J. A., and Waxman, S. G. (1988). The perinodal astrocyte. Glia 1, 169–183. doi: 10.1002/glia.440010302
Bocarsly, M. E., Jiang, W. C., Wang, C., Dudman, J. T., Ji, N., and Aponte, Y. (2015). Minimally invasive microendoscopy system for in vivo functional imaging of deep nuclei in the mouse brain. Biomed. Opt. Express. 6, 4546–4556. doi: 10.1364/BOE.6.004546
Boido, D., Rungta, R. L., Osmanski, B. F., Roche, M., Tsurugizawa, T., Le Bihan, D., et al. (2019). Mesoscopic and microscopic imaging of sensory responses in the same animal. Nat. Commun. 10:1110. doi: 10.1038/s41467-019-09082-4
Boisvert, M. M., Erikson, G. A., Shokhirev, M. N., and Allen, N. J. (2018). The aging astrocyte transcriptome from multiple regions of the mouse brain. Cell Rep. 22, 269–285. doi: 10.1016/j.celrep.2017.12.039
Bonomini, F., and Rezzani, R. (2010). Aquaporin and blood brain barrier. Curr. Neuropharmacol. 8, 92–96. doi: 10.2174/157015910791233132
Boulay, A. C., Cisternino, S., and Cohen-Salmon, M. (2016). Immunoregulation at the gliovascular unit in the healthy brain: a focus on connexin 43. Brain Behav. Immun. 56, 1–9. doi: 10.1016/j.bbi.2015.11.017
Brunner, C., Grillet, M., Sans-Dublanc, A., Farrow, K., Lambert, T., Mace, E., et al. (2020). A platform for brain-wide volumetric functional ultrasound imaging and analysis of circuit dynamics in awake mice. Neuron 108, 861–875.e867. doi: 10.1016/j.neuron.2020.09.020
Bruzzone, R., White, T. W., and Paul, D. L. (1996). Connections with connexins: the molecular basis of direct intercellular signaling. Eur. J. Biochem. 238, 1–27. doi: 10.1111/j.1432-1033.1996.0001q.x
Burton, N. C., Patel, M., Morscher, S., Driessen, W. H., Claussen, J., Beziere, N., et al. (2013). Multispectral opto-acoustic tomography (MSOT) of the brain and glioblastoma characterization. Neuroimage 65, 522–528. doi: 10.1016/j.neuroimage.2012.09.053
Bushong, E. A., Martone, M. E., Jones, Y. Z., and Ellisman, M. H. (2002). Protoplasmic astrocytes in CA1 stratum radiatum occupy separate anatomical domains. J. Neurosci. 22, 183–192. doi: 10.1523/JNEUROSCI.22-01-00183.2002
Cavaglia, M., Dombrowski, S. M., Drazba, J., Vasanji, A., Bokesch, P. M., and Janigro, D. (2001). Regional variation in brain capillary density and vascular response to ischemia. Brain Res. 910, 81–93. doi: 10.1016/S0006-8993(01)02637-3
Chai, H., Diaz-Castro, B., Shigetomi, E., Monte, E., Octeau, J. C., Yu, X., et al. (2017). Neural circuit-specialized astrocytes: transcriptomic, proteomic, morphological, and functional evidence. Neuron 95, 531–549.e539. doi: 10.1016/j.neuron.2017.06.029
Chia, T. H., and Levene, M. J. (2009). Microprisms for in vivo multilayer cortical imaging. J. Neurophysiol. 102, 1310–1314. doi: 10.1152/jn.91208.2008
Chong, S. P., Merkle, C. W., Leahy, C., Radhakrishnan, H., and Srinivasan, V. J. (2015). Quantitative microvascular hemoglobin mapping using visible light spectroscopic optical coherence tomography. Biomed. Opt. Express. 6, 1429–1450. doi: 10.1364/BOE.6.001429
Clarke, L. E., Liddelow, S. A., Chakraborty, C., Munch, A. E., Heiman, M., and Barres, B. A. (2018). Normal aging induces A1-like astrocyte reactivity. Proc. Natl. Acad. Sci. U.S.A. 115:E1896–E1905. doi: 10.1073/pnas.1800165115
Cole, J. T., Yarnell, A., Kean, W. S., Gold, E., Lewis, B., Ren, M., et al. (2011). Craniotomy: true sham for traumatic brain injury, or a sham of a sham? J. Neurotrauma. 28, 359–369. doi: 10.1089/neu.2010.1427
Cotrina, M. L., and Nedergaard, M. (2012). Brain connexins in demyelinating diseases: therapeutic potential of glial targets. Brain Res. 1487, 61–68. doi: 10.1016/j.brainres.2012.07.003
Császár, E., Lénárt, N., Cserép, C., Környei, Z., Fekete, R., Pósfai, B., et al. (2021). Microglia control cerebral blood flow and neurovascular coupling via P2Y12R-mediated actions. bioRxiv. doi: 10.1101/2021.02.04.429741
Cuadrado-Godia, E., Dwivedi, P., Sharma, S., Ois Santiago, A., Roquer Gonzalez, J., Balcells, M., et al. (2018). Cerebral small vessel disease: a review focusing on pathophysiology, biomarkers, and machine learning strategies. J. Stroke. 20, 302–320. doi: 10.5853/jos.2017.02922
Cudmore, R. H., Dougherty, S. E., and Linden, D. J. (2017). Cerebral vascular structure in the motor cortex of adult mice is stable and is not altered by voluntary exercise. J. Cereb. Blood Flow Metab. 37, 3725–3743. doi: 10.1177/0271678X16682508
Dallasta, L. M., Pisarov, L. A., Esplen, J. E., Werley, J. V., Moses, A. V., Nelson, J. A., et al. (1999). Blood-brain barrier tight junction disruption in human immunodeficiency virus-1 encephalitis. Am. J. Pathol. 155, 1915–1927. doi: 10.1016/S0002-9440(10)65511-3
Dana, H., Sun, Y., Mohar, B., Hulse, B. K., Kerlin, A. M., Hasseman, J. P., et al. (2019). High-performance calcium sensors for imaging activity in neuronal populations and microcompartments. Nat. Methods 16, 649–657. doi: 10.1038/s41592-019-0435-6
Daneman, R., Zhou, L., Kebede, A. A., and Barres, B. A. (2010). Pericytes are required for blood-brain barrier integrity during embryogenesis. Nature 468, 562–566. doi: 10.1038/nature09513
Dani, N., Herbst, R. H., McCabe, C., Green, G. S., Kaiser, K., Head, J. P., et al. (2021). A cellular and spatial map of the choroid plexus across brain ventricles and ages. Cell 184, 3056–3074.e21. doi: 10.1016/j.cell.2021.04.003
de Groot, A., van den Boom, B. J., van Genderen, R. M., Coppens, J., van Veldhuijzen, J., Bos, J., et al. (2020). NINscope, a versatile miniscope for multi-region circuit investigations. Elife 9:e49987. doi: 10.7554/eLife.49987
Devonshire, I. M., Papadakis, N. G., Port, M., Berwick, J., Kennerley, A. J., Mayhew, J. E., et al. (2012). Neurovascular coupling is brain region-dependent. Neuroimage 59, 1997–2006. doi: 10.1016/j.neuroimage.2011.09.050
Ding, R., Hase, Y., Ameen-Ali, K. E., Ndung'u, M., Stevenson, W., Barsby, J., et al. (2020). Loss of capillary pericytes and the blood-brain barrier in white matter in poststroke and vascular dementias and Alzheimer's disease. Brain Pathol. 30, 1087–1101. doi: 10.1111/bpa.12888
Dorand, R. D., Barkauskas, D. S., Evans, T. A., Petrosiute, A., and Huang, A. Y. (2014). Comparison of intravital thinned skull and cranial window approaches to study CNS immunobiology in the mouse cortex. Intravital 3:e29728. doi: 10.4161/intv.29728
Drew, P. J., Shih, A. Y., Driscoll, J. D., Knutsen, P. M., Blinder, P., Davalos, D., et al. (2010). Chronic optical access through a polished and reinforced thinned skull. Nat. Methods 7, 981–984. doi: 10.1038/nmeth.1530
Escartin, C., Guillemaud, O., and Carrillo-de Sauvage, M. A. (2019). Questions and (some) answers on reactive astrocytes. Glia 67, 2221–2247. doi: 10.1002/glia.23687
Etchevers, H. C., Vincent, C., Le Douarin, N. M., and Couly, G. F. (2001). The cephalic neural crest provides pericytes and smooth muscle cells to all blood vessels of the face and forebrain. Development 128, 1059–1068. doi: 10.1242/dev.128.7.1059
Ezan, P., Andre, P., Cisternino, S., Saubamea, B., Boulay, A. C., Doutremer, S., et al. (2012). Deletion of astroglial connexins weakens the blood-brain barrier. J. Cereb. Blood Flow Metab. 32, 1457–1467. doi: 10.1038/jcbfm.2012.45
Fan, J. L., Rivera, J. A., Sun, W., Peterson, J., Haeberle, H., Rubin, S., et al. (2020). High-speed volumetric two-photon fluorescence imaging of neurovascular dynamics. Nat. Commun. 11:6020. doi: 10.1038/s41467-020-19851-1
Fernandez-Klett, F., Offenhauser, N., Dirnagl, U., Priller, J., and Lindauer, U. (2010). Pericytes in capillaries are contractile in vivo, but arterioles mediate functional hyperemia in the mouse brain. Proc. Natl. Acad. Sci. U.S.A. 107, 22290–22295. doi: 10.1073/pnas.1011321108
Flynn, K. M., Michaud, M., and Madri, J. A. (2013). CD44 deficiency contributes to enhanced experimental autoimmune encephalomyelitis: a role in immune cells and vascular cells of the blood-brain barrier. Am. J. Pathol. 182, 1322–1336. doi: 10.1016/j.ajpath.2013.01.003
Frahm, K. A., and Tobet, S. A. (2015). Development of the blood-brain barrier within the paraventricular nucleus of the hypothalamus: influence of fetal glucocorticoid excess. Brain Struct. Funct. 220, 2225–2234. doi: 10.1007/s00429-014-0787-8
Gagnon, L., Smith, A. F., Boas, D. A., Devor, A., Secomb, T. W., and Sakadzic, S. (2016). Modeling of cerebral oxygen transport based on in vivo microscopic imaging of microvascular network structure, blood flow, and oxygenation. Front. Comput. Neurosci. 10:82. doi: 10.3389/fncom.2016.00082
Gawryluk, J. R., Mazerolle, E. L., and D'Arcy, R. C. (2014). Does functional MRI detect activation in white matter? A review of emerging evidence, issues, and future directions. Front. Neurosci. 8:239. doi: 10.3389/fnins.2014.00239
Goldey, G. J., Roumis, D. K., Glickfeld, L. L., Kerlin, A. M., Reid, R. C., Bonin, V., et al. (2014). Removable cranial windows for long-term imaging in awake mice. Nat. Protoc. 9, 2515–2538. doi: 10.1038/nprot.2014.165
Gonzales, A. L., Klug, N. R., Moshkforoush, A., Lee, J. C., Lee, F. K., Shui, B., et al. (2020). Contractile pericytes determine the direction of blood flow at capillary junctions. Proc. Natl. Acad. Sci. U.S.A. 117, 27022–27033. doi: 10.1073/pnas.1922755117
Gorelick, P. B., Counts, S. E., and Nyenhuis, D. (2016). Vascular cognitive impairment and dementia. Biochim. Biophys. Acta 1862, 860–868. doi: 10.1016/j.bbadis.2015.12.015
Gottschalk, S., Degtyaruk, O., Mc Larney, B., Rebling, J., Hutter, M. A., Dean-Ben, X. L., et al. (2019). Rapid volumetric optoacoustic imaging of neural dynamics across the mouse brain. Nat. Biomed. Eng. 3, 392–401. doi: 10.1038/s41551-019-0372-9
Goursaud, S., Kozlova, E. N., Maloteaux, J. M., and Hermans, E. (2009). Cultured astrocytes derived from corpus callosum or cortical grey matter show distinct glutamate handling properties. J. Neurochem. 108, 1442–1452. doi: 10.1111/j.1471-4159.2009.05889.x
Griemsmann, S., Hoft, S. P., Bedner, P., Zhang, J., von Staden, E., Beinhauer, A., et al. (2015). Characterization of panglial gap junction networks in the thalamus, neocortex, and hippocampus reveals a unique population of glial cells. Cereb. Cortex. 25, 3420–3433. doi: 10.1093/cercor/bhu157
Grinvald, A., Lieke, E., Frostig, R. D., Gilbert, C. D., and Wiesel, T. N. (1986). Functional architecture of cortex revealed by optical imaging of intrinsic signals. Nature 324, 361–364. doi: 10.1038/324361a0
Gulati, S., Cao, V. Y., and Otte, S. (2017). Multi-layer cortical Ca2+ imaging in freely moving mice with prism probes and miniaturized fluorescence microscopy. J. Vis. Exp. 124:55579. doi: 10.3791/55579
Haas, B., Schipke, C. G., Peters, O., Sohl, G., Willecke, K., and Kettenmann, H. (2006). Activity-dependent ATP-waves in the mouse neocortex are independent from astrocytic calcium waves. Cereb. Cortex. 16, 237–246. doi: 10.1093/cercor/bhi101
Haj-Yasein, N. N., Vindedal, G. F., Eilert-Olsen, M., Gundersen, G. A., Skare, O., Laake, P., et al. (2011). Glial-conditional deletion of aquaporin-4 (Aqp4) reduces blood-brain water uptake and confers barrier function on perivascular astrocyte endfeet. Proc. Natl. Acad. Sci. U.S.A. 108, 17815–17820. doi: 10.1073/pnas.1110655108
Hall, C. N., Reynell, C., Gesslein, B., Hamilton, N. B., Mishra, A., Sutherland, B. A., et al. (2014). Capillary pericytes regulate cerebral blood flow in health and disease. Nature 508, 55–60. doi: 10.1038/nature13165
Halliday, M. R., Rege, S. V., Ma, Q., Zhao, Z., Miller, C. A., Winkler, E. A., et al. (2016). Accelerated pericyte degeneration and blood-brain barrier breakdown in apolipoprotein E4 carriers with Alzheimer's disease. J. Cereb. Blood Flow Metab. 36, 216–227. doi: 10.1038/jcbfm.2015.44
Harris, J. J., and Attwell, D. (2012). The energetics of CNS white matter. J. Neurosci. 32, 356–371. doi: 10.1523/JNEUROSCI.3430-11.2012
Hartmann, D. A., Berthiaume, A. A., Grant, R. I., Harrill, S. A., Koski, T., Tieu, T., et al. (2021). Brain capillary pericytes exert a substantial but slow influence on blood flow. Nat. Neurosci. 24, 633–645. doi: 10.1038/s41593-020-00793-2
Hartmann, D. A., Underly, R. G., Grant, R. I., Watson, A. N., Lindner, V., and Shih, A. Y. (2015). Pericyte structure and distribution in the cerebral cortex revealed by high-resolution imaging of transgenic mice. Neurophotonics 2:041402. doi: 10.1117/1.NPh.2.4.041402
Hase, Y., Ding, R., Harrison, G., Hawthorne, E., King, A., Gettings, S., et al. (2019). White matter capillaries in vascular and neurodegenerative dementias. Acta Neuropathol. Commun. 7:16. doi: 10.1186/s40478-019-0666-x
Hase, Y., Horsburgh, K., Ihara, M., and Kalaria, R. N. (2018). White matter degeneration in vascular and other ageing-related dementias. J. Neurochem. 144, 617–633. doi: 10.1111/jnc.14271
Hierro-Bujalance, C., Bacskai, B. J., and Garcia-Alloza, M. (2018). In vivo imaging of microglia with multiphoton microscopy. Front. Aging Neurosci. 10:218. doi: 10.3389/fnagi.2018.00218
Hill, R. A., Tong, L., Yuan, P., Murikinati, S., Gupta, S., and Grutzendler, J. (2015). Regional blood flow in the normal and ischemic brain is controlled by arteriolar smooth muscle cell contractility and not by capillary pericytes. Neuron 87, 95–110. doi: 10.1016/j.neuron.2015.06.001
Iadecola, C. (2013). The pathobiology of vascular dementia. Neuron 80, 844–866. doi: 10.1016/j.neuron.2013.10.008
Iadecola, C. (2017). The neurovascular unit coming of age: a journey through neurovascular coupling in health and disease. Neuron 96, 17–42. doi: 10.1016/j.neuron.2017.07.030
Iliff, J., and Simon, M. (2019). CrossTalk proposal: the glymphatic system supports convective exchange of cerebrospinal fluid and brain interstitial fluid that is mediated by perivascular aquaporin-4. J. Physiol. 597, 4417–4419. doi: 10.1113/JP277635
Inoue, M., Takeuchi, A., Manita, S., Horigane, S. I., Sakamoto, M., Kawakami, R., et al. (2019). Rational engineering of XCaMPs, a multicolor GECI suite for in vivo imaging of complex brain circuit dynamics. Cell 177, 1346–1360.e1324. doi: 10.1016/j.cell.2019.04.007
Jacob, A. D., Ramsaran, A. I., Mocle, A. J., Tran, L. M., Yan, C., Frankland, P. W., et al. (2018). A compact head-mounted endoscope for in vivo calcium imaging in freely behaving mice. Curr. Protoc. Neurosci. 84:e51. doi: 10.1002/cpns.51
Jammalamadaka, A., Suwannatat, P., Fisher, S. K., Manjunath, B. S., Hollerer, T., and Luna, G. (2015). Characterizing spatial distributions of astrocytes in the mammalian retina. Bioinformatics 31, 2024–2031. doi: 10.1093/bioinformatics/btv097
Jessen, N. A., Munk, A. S., Lundgaard, I., and Nedergaard, M. (2015). The glymphatic system: a beginner's guide. Neurochem. Res. 40, 2583–2599. doi: 10.1007/s11064-015-1581-6
Kaaijk, P., Pals, S. T., Morsink, F., Bosch, D. A., and Troost, D. (1997). Differential expression of CD44 splice variants in the normal human central nervous system. J. Neuroimmunol. 73, 70–76. doi: 10.1016/S0165-5728(96)00167-1
Kaur, C., Rathnasamy, G., and Ling, E. A. (2016). The choroid plexus in healthy and diseased brain. J. Neuropathol. Exp. Neurol. 75, 198–213. doi: 10.1093/jnen/nlv030
Kim, H. W., Hong, J., and Jeon, J. C. (2020). Cerebral small vessel disease and alzheimer's disease: a review. Front. Neurol. 11:927. doi: 10.3389/fneur.2020.00927
Knowland, D., Arac, A., Sekiguchi, K. J., Hsu, M., Lutz, S. E., Perrino, J., et al. (2014). Stepwise recruitment of transcellular and paracellular pathways underlies blood-brain barrier breakdown in stroke. Neuron 82, 603–617. doi: 10.1016/j.neuron.2014.03.003
Koizumi, T., Kerkhofs, D., Mizuno, T., Steinbusch, H. W. M., and Foulquier, S. (2019). Vessel-associated immune cells in cerebrovascular diseases: from perivascular macrophages to vessel-associated microglia. Front. Neurosci. 13:1291. doi: 10.3389/fnins.2019.01291
Kwan, P., Li, H. M., Al-Jufairi, E., Abdulla, R., Gonzales, M., Kaye, A. H., et al. (2010). Association between temporal lobe P-glycoprotein expression and seizure recurrence after surgery for pharmacoresistant temporal lobe epilepsy. Neurobiol. Dis. 39, 192–197. doi: 10.1016/j.nbd.2010.04.006
Lake, E. M. R., Ge, X., Shen, X., Herman, P., Hyder, F., Cardin, J. A., et al. (2020). Simultaneous cortex-wide fluorescence Ca(2+) imaging whole-brain fMRI. Nat. Methods 17, 1262–1271. doi: 10.1038/s41592-020-00984-6
Lanjakornsiripan, D., Pior, B. J., Kawaguchi, D., Furutachi, S., Tahara, T., Katsuyama, Y., et al. (2018). Layer-specific morphological and molecular differences in neocortical astrocytes and their dependence on neuronal layers. Nat. Commun. 9:1623. doi: 10.1038/s41467-018-03940-3
Li, B., Ohtomo, R., Thunemann, M., Adams, S. R., Yang, J., Fu, B., et al. (2020). Two-photon microscopic imaging of capillary red blood cell flux in mouse brain reveals vulnerability of cerebral white matter to hypoperfusion. J. Cereb. Blood Flow Metab. 40, 501–512. doi: 10.1177/0271678X19831016
Li, Y., Rakymzhan, A., Tang, P., and Wang, R. K. (2020). Procedure and protocols for optical imaging of cerebral blood flow and hemodynamics in awake mice. Biomed. Opt. Express 11, 3288–3300. doi: 10.1364/BOE.394649
Liddelow, S. A. (2015). Development of the choroid plexus and blood-CSF barrier. Front. Neurosci. 9:32. doi: 10.3389/fnins.2015.00032
Liedtke, W., Edelmann, W., Bieri, P. L., Chiu, F. C., Cowan, N. J., Kucherlapati, R., et al. (1996). GFAP is necessary for the integrity of CNS white matter architecture and long-term maintenance of myelination. Neuron 17, 607–615. doi: 10.1016/S0896-6273(00)80194-4
Lin, M. Z., and Schnitzer, M. J. (2016). Genetically encoded indicators of neuronal activity. Nat. Neurosci. 19, 1142–1153. doi: 10.1038/nn.4359
Liu, J., Wang, M., Sun, L., Pan, N. C., Zhang, C., Zhang, J., et al. (2020). Integrative analysis of in vivo recording with single-cell RNA-seq data reveals molecular properties of light-sensitive neurons in mouse V1. Protein Cell 11, 417–432. doi: 10.1007/s13238-020-00720-y
Liu, L., Collier, A. C., Link, J. M., Domino, K. B., Mankoff, D. A., Eary, J. F., et al. (2015). Modulation of P-glycoprotein at the human blood-brain barrier by quinidine or rifampin treatment: a positron emission tomography imaging study. Drug Metab. Dispos. 43, 1795–1804. doi: 10.1124/dmd.114.058685
Low, R. J., Gu, Y., and Tank, D. W. (2014). Cellular resolution optical access to brain regions in fissures: imaging medial prefrontal cortex and grid cells in entorhinal cortex. Proc. Natl. Acad. Sci. U.S.A. 111, 18739–18744. doi: 10.1073/pnas.1421753111
Lubetzki, C., Sol-Foulon, N., and Desmazieres, A. (2020). Nodes of Ranvier during development and repair in the CNS. Nat. Rev. Neurol. 16, 426–439. doi: 10.1038/s41582-020-0375-x
Lun, M. P., Monuki, E. S., and Lehtinen, M. K. (2015). Development and functions of the choroid plexus-cerebrospinal fluid system. Nat. Rev. Neurosci. 16, 445–457. doi: 10.1038/nrn3921
Lundgaard, I., Osorio, M. J., Kress, B. T., Sanggaard, S., and Nedergaard, M. (2014). White matter astrocytes in health and disease. Neuroscience 276, 161–173. doi: 10.1016/j.neuroscience.2013.10.050
Mace, E., Montaldo, G., Cohen, I., Baulac, M., Fink, M., and Tanter, M. (2011). Functional ultrasound imaging of the brain. Nat. Methods 8, 662–664. doi: 10.1038/nmeth.1641
Mace, E., Montaldo, G., Trenholm, S., Cowan, C., Brignall, A., Urban, A., et al. (2018). Whole-brain functional ultrasound imaging reveals brain modules for visuomotor integration. Neuron 100, 1241–1251.e1247. doi: 10.1016/j.neuron.2018.11.031
Mae, M. A., He, L., Nordling, S., Vazquez-Liebanas, E., Nahar, K., Jung, B., et al. (2021). Single-cell analysis of blood-brain barrier response to pericyte loss. Circ. Res. 128:e46–e62. doi: 10.1161/CIRCRESAHA.120.317473
Marques, S., Zeisel, A., Codeluppi, S., van Bruggen, D., Mendanha Falcao, A., Xiao, L., et al. (2016). Oligodendrocyte heterogeneity in the mouse juvenile and adult central nervous system. Science 352, 1326–1329. doi: 10.1126/science.aaf6463
Masamoto, K., and Kanno, I. (2012). Anesthesia and the quantitative evaluation of neurovascular coupling. J. Cereb. Blood Flow Metab. 32, 1233–1247. doi: 10.1038/jcbfm.2012.50
Masuda, T., Sankowski, R., Staszewski, O., and Prinz, M. (2020). Microglia heterogeneity in the single-cell era. Cell Rep. 30, 1271–1281. doi: 10.1016/j.celrep.2020.01.010
Mathiisen, T. M., Lehre, K. P., Danbolt, N. C., and Ottersen, O. P. (2010). The perivascular astroglial sheath provides a complete covering of the brain microvessels: an electron microscopic 3D reconstruction. Glia 58, 1094–1103. doi: 10.1002/glia.20990
Matias, I., Morgado, J., and Gomes, F. C. A. (2019). Astrocyte heterogeneity: impact to brain aging and disease. Front. Aging Neurosci. 11:59. doi: 10.3389/fnagi.2019.00059
McCaslin, A. F., Chen, B. R., Radosevich, A. J., Cauli, B., and Hillman, E. M. (2011). In vivo 3D morphology of astrocyte-vasculature interactions in the somatosensory cortex: implications for neurovascular coupling. J. Cereb. Blood Flow Metab. 31, 795–806. doi: 10.1038/jcbfm.2010.204
Meng, C., Zhou, J., Papaneri, A., Peddada, T., Xu, K., and Cui, G. (2018). Spectrally resolved fiber photometry for multi-component analysis of brain circuits. Neuron 98, 707–717.e704. doi: 10.1016/j.neuron.2018.04.012
Miao, P., Lu, H., Liu, Q., Li, Y., and Tong, S. (2011). Laser speckle contrast imaging of cerebral blood flow in freely moving animals. J. Biomed. Opt. 16:090502. doi: 10.1117/1.3625231
Miller, D. R., Hassan, A. M., Jarrett, J. W., Medina, F. A., Perillo, E. P., Hagan, K., et al. (2017). In vivo multiphoton imaging of a diverse array of fluorophores to investigate deep neurovascular structure. Biomed. Opt. Express. 8, 3470–3481. doi: 10.1364/BOE.8.003470
Miller, S. J. (2018). Astrocyte heterogeneity in the adult central nervous system. Front. Cell. Neurosci. 12:401. doi: 10.3389/fncel.2018.00401
Mishra, A. M., Ellens, D. J., Schridde, U., Motelow, J. E., Purcaro, M. J., DeSalvo, M. N., et al. (2011). Where fMRI and electrophysiology agree to disagree: corticothalamic and striatal activity patterns in the WAG/Rij rat. J. Neurosci. 31, 15053–15064. doi: 10.1523/JNEUROSCI.0101-11.2011
Montagne, A., Barnes, S. R., Sweeney, M. D., Halliday, M. R., Sagare, A. P., Zhao, Z., et al. (2015). Blood-brain barrier breakdown in the aging human hippocampus. Neuron 85, 296–302. doi: 10.1016/j.neuron.2014.12.032
Montagne, A., Nikolakopoulou, A. M., Zhao, Z., Sagare, A. P., Si, G., Lazic, D., et al. (2018). Pericyte degeneration causes white matter dysfunction in the mouse central nervous system. Nat. Med. 24, 326–337. doi: 10.1038/nm.4482
Morel, L., Chiang, M. S. R., Higashimori, H., Shoneye, T., Iyer, L. K., Yelick, J., et al. (2017). Molecular and Functional Properties of Regional Astrocytes in the Adult Brain. J. Neurosci. 37, 8706–8717. doi: 10.1523/JNEUROSCI.3956-16.2017
Morel, L., Men, Y., Chiang, M. S. R., Tian, Y., Jin, S., Yelick, J., et al. (2019). Intracortical astrocyte subpopulations defined by astrocyte reporter Mice in the adult brain. Glia 67, 171–181. doi: 10.1002/glia.23545
Morita, S., Furube, E., Mannari, T., Okuda, H., Tatsumi, K., Wanaka, A., et al. (2016). Heterogeneous vascular permeability and alternative diffusion barrier in sensory circumventricular organs of adult mouse brain. Cell Tissue Res. 363, 497–511. doi: 10.1007/s00441-015-2207-7
Morita, S., Hourai, A., and Miyata, S. (2014). Changes in pericytic expression of NG2 and PDGFRB and vascular permeability in the sensory circumventricular organs of adult mouse by osmotic stimulation. Cell Biochem. Funct. 32, 51–61. doi: 10.1002/cbf.2971
Murugesan, N., Demarest, T. G., Madri, J. A., and Pachter, J. S. (2012). Brain regional angiogenic potential at the neurovascular unit during normal aging. Neurobiol. Aging. 33, 1004.e1001–1016. doi: 10.1016/j.neurobiolaging.2011.09.022
Nielsen, S., Nagelhus, E. A., Amiry-Moghaddam, M., Bourque, C., Agre, P., and Ottersen, O. P. (1997). Specialized membrane domains for water transport in glial cells: high-resolution immunogold cytochemistry of aquaporin-4 in rat brain. J. Neurosci. 17, 171–180. doi: 10.1523/JNEUROSCI.17-01-00171.1997
Nikolakopoulou, A. M., Montagne, A., Kisler, K., Dai, Z., Wang, Y., Huuskonen, M. T., et al. (2019). Pericyte loss leads to circulatory failure and pleiotrophin depletion causing neuron loss. Nat. Neurosci. 22, 1089–1098. doi: 10.1038/s41593-019-0434-z
Nikolakopoulou, A. M., Zhao, Z., Montagne, A., and Zlokovic, B. V. (2017). Regional early and progressive loss of brain pericytes but not vascular smooth muscle cells in adult mice with disrupted platelet-derived growth factor receptor-beta signaling. PLoS ONE 12:e0176225. doi: 10.1371/journal.pone.0176225
Nortley, R., Korte, N., Izquierdo, P., Hirunpattarasilp, C., Mishra, A., Jaunmuktane, Z., et al. (2019). Amyloid beta oligomers constrict human capillaries in Alzheimer's disease via signaling to pericytes. Science 365:eaav9518. doi: 10.1126/science.aav9518
Noumbissi, M. E., Galasso, B., and Stins, M. F. (2018). Brain vascular heterogeneity: implications for disease pathogenesis and design of in vitro blood-brain barrier models. Fluids Barriers CNS. 15:12. doi: 10.1186/s12987-018-0097-2
Nunez-Elizalde, A. O., Krumin, M., Reddy, C. B., Montaldo, G., Urban, A., Harris, K. D., et al. (2021). Neural basis of functional ultrasound signals. bioRxiv. doi: 10.1101/2021.03.31.437915
Nyul-Toth, A., Suciu, M., Molnar, J., Fazakas, C., Hasko, J., Herman, H., et al. (2016). Differences in the molecular structure of the blood-brain barrier in the cerebral cortex and white matter: an in silico, in vitro, and ex vivo study. Am. J. Physiol Heart Circ. Physiol. 310:H1702–1714. doi: 10.1152/ajpheart.00774.2015
Oberheim, N. A., Wang, X., Goldman, S., and Nedergaard, M. (2006). Astrocytic complexity distinguishes the human brain. Trends Neurosci. 29, 547–553. doi: 10.1016/j.tins.2006.08.004
O'Herron, P., Chhatbar, P. Y., Levy, M., Shen, Z., Schramm, A. E., Lu, Z., et al. (2016). Neural correlates of single-vessel haemodynamic responses in vivo. Nature 534, 378–382. doi: 10.1038/nature17965
Ouzounov, D. G., Wang, T., Wang, M., Feng, D. D., Horton, N. G., Cruz-Hernandez, J. C., et al. (2017). In vivo three-photon imaging of activity of GCaMP6-labeled neurons deep in intact mouse brain. Nat. Methods 14, 388–390. doi: 10.1038/nmeth.4183
Ovsepian, S. V., Olefir, I., Westmeyer, G., Razansky, D., and Ntziachristos, V. (2017). Pushing the boundaries of neuroimaging with optoacoustics. Neuron 96, 966–988. doi: 10.1016/j.neuron.2017.10.022
Papadopoulos, M. C., and Verkman, A. S. (2013). Aquaporin water channels in the nervous system. Nat. Rev. Neurosci. 14, 265–277. doi: 10.1038/nrn3468
Park, K. S., Shin, J. G., Qureshi, M. M., Chung, E., and Eom, T. J. (2018). Deep brain optical coherence tomography angiography in mice: in vivo, noninvasive imaging of hippocampal formation. Sci Rep. 8:11614. doi: 10.1038/s41598-018-29975-6
Paukert, M., Agarwal, A., Cha, J., Doze, V. A., Kang, J. U., and Bergles, D. E. (2014). Norepinephrine controls astroglial responsiveness to local circuit activity. Neuron 82, 1263–1270. doi: 10.1016/j.neuron.2014.04.038
Pisano, F., Pisanello, M., Lee, S. J., Lee, J., Maglie, E., Balena, A., et al. (2019). Depth-resolved fiber photometry with a single tapered optical fiber implant. Nat. Methods 16, 1185–1192. doi: 10.1038/s41592-019-0581-x
Rasmussen, M. K., Mestre, H., and Nedergaard, M. (2021). Fluid transport in the brain. Physiol. Rev. doi: 10.1152/physrev.00031.2020. [Epub ahead of print].
Reimann, H. M., and Niendorf, T. (2020). The (Un)conscious mouse as a model for human brain functions: key principles of anesthesia and their impact on translational neuroimaging. Front. Syst. Neurosci. 14:8. doi: 10.3389/fnsys.2020.00008
Resendez, S. L., Jennings, J. H., Ung, R. L., Namboodiri, V. M., Zhou, Z. C., Otis, J. M., et al. (2016). Visualization of cortical, subcortical and deep brain neural circuit dynamics during naturalistic mammalian behavior with head-mounted microscopes and chronically implanted lenses. Nat. Protoc. 11, 566–597. doi: 10.1038/nprot.2016.021
Ross, J. M., Kim, C., Allen, D., Crouch, E. E., Narsinh, K., Cooke, D. L., et al. (2020). The expanding cell diversity of the brain vasculature. Front. Physiol. 11:600767. doi: 10.3389/fphys.2020.600767
Saadoun, S., Tait, M. J., Reza, A., Davies, D. C., Bell, B. A., Verkman, A. S., et al. (2009). AQP4 gene deletion in mice does not alter blood-brain barrier integrity or brain morphology. Neuroscience 161, 764–772. doi: 10.1016/j.neuroscience.2009.03.069
Sabbagh, M. F., Heng, J. S., Luo, C., Castanon, R. G., Nery, J. R., Rattner, A., et al. (2018). Transcriptional and epigenomic landscapes of CNS and non-CNS vascular endothelial cells. Elife 7:42. doi: 10.7554/eLife.36187.042
Sagare, A. P., Bell, R. D., Zhao, Z., Ma, Q., Winkler, E. A., Ramanathan, A., et al. (2013). Pericyte loss influences Alzheimer-like neurodegeneration in mice. Nat. Commun. 4:2932. doi: 10.1038/ncomms3932
Sans-Dublanc, A., Chrzanowska, A., Reinhard, K., Lemmon, D., Nuttin, B., Lambert, T., et al. (2021). Optogenetic fUSI for brain-wide mapping of neural activity mediating collicular-dependent behaviors. Neuron. 109, 1888–1905.e10. doi: 10.1016/j.neuron.2021.04.008
Saunders, A., Macosko, E. Z., Wysoker, A., Goldman, M., Krienen, F. M., de Rivera, H., et al. (2018). Molecular diversity and specializations among the cells of the adult mouse brain. Cell 174, 1015–1030.e1016. doi: 10.1016/j.cell.2018.07.028
Saxena, S., and Caroni, P. (2011). Selective neuronal vulnerability in neurodegenerative diseases: from stressor thresholds to degeneration. Neuron 71, 35–48. doi: 10.1016/j.neuron.2011.06.031
Schager, B., and Brown, C. E. (2020). Susceptibility to capillary plugging can predict brain region specific vessel loss with aging. J. Cereb. Blood Flow Metab. 40, 2475–2490. doi: 10.1177/0271678X19895245
Schlegel, F., Sych, Y., Schroeter, A., Stobart, J., Weber, B., Helmchen, F., et al. (2018). Fiber-optic implant for simultaneous fluorescence-based calcium recordings and BOLD fMRI in mice. Nat. Protoc. 13, 840–855. doi: 10.1038/nprot.2018.003
Schulz, K., Sydekum, E., Krueppel, R., Engelbrecht, C. J., Schlegel, F., Schroter, A., et al. (2012). Simultaneous BOLD fMRI and fiber-optic calcium recording in rat neocortex. Nat. Methods 9, 597–602. doi: 10.1038/nmeth.2013
Senarathna, J., Yu, H., Deng, C., Zou, A. L., Issa, J. B., Hadjiabadi, D. H., et al. (2019). A miniature multi-contrast microscope for functional imaging in freely behaving animals. Nat. Commun. 10:99. doi: 10.1038/s41467-018-07926-z
Shi, Y., and Wardlaw, J. M. (2016). Update on cerebral small vessel disease: a dynamic whole-brain disease. Stroke Vasc. Neurol. 1, 83–92. doi: 10.1136/svn-2016-000035
Shih, A. Y., Driscoll, J. D., Drew, P. J., Nishimura, N., Schaffer, C. B., and Kleinfeld, D. (2012). Two-photon microscopy as a tool to study blood flow and neurovascular coupling in the rodent brain. J. Cereb. Blood Flow Metab. 32, 1277–1309. doi: 10.1038/jcbfm.2011.196
Sofroniew, M. V., and Vinters, H. V. (2010). Astrocytes: biology and pathology. Acta Neuropathol. 119, 7–35. doi: 10.1007/s00401-009-0619-8
Sokoloff, L., Reivich, M., Kennedy, C., Des Rosiers, M. H., Patlak, C. S., Pettigrew, K. D., et al. (1977). The [14C]deoxyglucose method for the measurement of local cerebral glucose utilization: theory, procedure, and normal values in the conscious and anesthetized albino rat. J. Neurochem. 28, 897–916. doi: 10.1111/j.1471-4159.1977.tb10649.x
Srienc, A. I., Chiang, P. P., Schmitt, A. J., and Newman, E. A. (2019). Cortical spreading depolarizations induced by surgical field blood in a mouse model of neurosurgery. J. Neurosurg. 132, 1820–1828. doi: 10.3171/2018.12.JNS181130
Stokum, J. A., Mehta, R. I., Ivanova, S., Yu, E., Gerzanich, V., and Simard, J. M. (2015). Heterogeneity of aquaporin-4 localization and expression after focal cerebral ischemia underlies differences in white versus grey matter swelling. Acta Neuropathol. Commun. 3:61. doi: 10.1186/s40478-015-0239-6
Sweeney, M. D., Zhao, Z., Montagne, A., Nelson, A. R., and Zlokovic, B. V. (2019). Blood-brain barrier: from physiology to disease and back. Physiol. Rev. 99, 21–78. doi: 10.1152/physrev.00050.2017
Sych, Y., Chernysheva, M., Sumanovski, L. T., and Helmchen, F. (2019). High-density multi-fiber photometry for studying large-scale brain circuit dynamics. Nat. Methods 16, 553–560. doi: 10.1038/s41592-019-0400-4
Taheri, S., Gasparovic, C., Shah, N. J., and Rosenberg, G. A. (2011). Quantitative measurement of blood-brain barrier permeability in human using dynamic contrast-enhanced MRI with fast T1 mapping. Magn. Reson. Med. 65, 1036–1042. doi: 10.1002/mrm.22686
Takano, T., Tian, G. F., Peng, W., Lou, N., Libionka, W., Han, X., et al. (2006). Astrocyte-mediated control of cerebral blood flow. Nat. Neurosci. 9, 260–267. doi: 10.1038/nn1623
Tallquist, M. D., French, W. J., and Soriano, P. (2003). Additive effects of PDGF receptor beta signaling pathways in vascular smooth muscle cell development. PLoS Biol. 1:e52. doi: 10.1371/journal.pbio.0000052
Tang, J., Coleman, J. E., Dai, X., and Jiang, H. (2016). Wearable 3-D photoacoustic tomography for functional brain imaging in behaving rats. Sci. Rep. 6:25470. doi: 10.1038/srep25470
Tiran, E., Ferrier, J., Deffieux, T., Gennisson, J. L., Pezet, S., Lenkei, Z., et al. (2017). Transcranial functional ultrasound imaging in freely moving awake mice and anesthetized young rats without contrast agent. Ultrasound Med. Biol. 43, 1679–1689. doi: 10.1016/j.ultrasmedbio.2017.03.011
Torok, O., Schreiner, B., Schaffenrath, J., Tsai, H. C., Maheshwari, U., Stifter, S. A., et al. (2021). Pericytes regulate vascular immune homeostasis in the CNS. Proc. Natl. Acad. Sci. U.S.A. 118:e2016587118. doi: 10.1073/pnas.2016587118
Tran, C. H., and Gordon, G. R. (2015). Astrocyte and microvascular imaging in awake animals using two-photon microscopy. Microcirculation 22, 219–227. doi: 10.1111/micc.12188
Trost, A., Lange, S., Schroedl, F., Bruckner, D., Motloch, K. A., Bogner, B., et al. (2016). Brain and retinal pericytes: origin, function and role. Front. Cell. Neurosci. 10:20. doi: 10.3389/fncel.2016.00020
Urban, A., Dussaux, C., Martel, G., Brunner, C., Mace, E., and Montaldo, G. (2015). Real-time imaging of brain activity in freely moving rats using functional ultrasound. Nat. Methods 12, 873–878. doi: 10.1038/nmeth.3482
Urban, A., Golgher, L., Brunner, C., Gdalyahu, A., Har-Gil, H., Kain, D., et al. (2017). Understanding the neurovascular unit at multiple scales: advantages and limitations of multi-photon and functional ultrasound imaging. Adv. Drug Deliv. Rev. 119, 73–100. doi: 10.1016/j.addr.2017.07.018
Urban, A., Mace, E., Brunner, C., Heidmann, M., Rossier, J., and Montaldo, G. (2014). Chronic assessment of cerebral hemodynamics during rat forepaw electrical stimulation using functional ultrasound imaging. Neuroimage 101, 138–149. doi: 10.1016/j.neuroimage.2014.06.063
Vanlandewijck, M., He, L., Mae, M. A., Andrae, J., Ando, K., Del Gaudio, F., et al. (2018). A molecular atlas of cell types and zonation in the brain vasculature. Nature 554, 475–480. doi: 10.1038/nature25739
Villabona-Rueda, A., Erice, C., Pardo, C. A., and Stins, M. F. (2019). The evolving concept of the blood brain barrier (BBB): from a single static barrier to a heterogeneous and dynamic relay center. Front. Cell. Neurosci. 13:405. doi: 10.3389/fncel.2019.00405
Villasenor, R., Kuennecke, B., Ozmen, L., Ammann, M., Kugler, C., Gruninger, F., et al. (2017). Region-specific permeability of the blood-brain barrier upon pericyte loss. J. Cereb. Blood Flow Metab. 37, 3683–3694. doi: 10.1177/0271678X17697340
Walter, A., Paul-Gilloteaux, P., Plochberger, B., Sefc, L., Verkade, P., Mannheim, J. G., et al. (2020). Correlated multimodal imaging in life sciences: expanding the biomedical horizon. Front. Phys. 8:47. doi: 10.3389/fphy.2020.00047
Wang, M. X., Ray, L., Tanaka, K. F., Iliff, J. J., and Heys, J. (2021). Varying perivascular astroglial endfoot dimensions along the vascular tree maintain perivascular-interstitial flux through the cortical mantle. Glia 69, 715–728. doi: 10.1002/glia.23923
Wang, T., Ouzounov, D. G., Wu, C., Horton, N. G., Zhang, B., Wu, C. H., et al. (2018). Three-photon imaging of mouse brain structure and function through the intact skull. Nat. Methods 15, 789–792. doi: 10.1038/s41592-018-0115-y
Wang, Y., DeMarco, E. M., Witzel, L. S., and Keighron, J. D. (2021). A selected review of recent advances in the study of neuronal circuits using fiber photometry. Pharmacol. Biochem. Behav. 201:173113. doi: 10.1016/j.pbb.2021.173113
Wang, Y., Sabbagh, M. F., Gu, X., Rattner, A., Williams, J., and Nathans, J. (2019). Beta-catenin signaling regulates barrier-specific gene expression in circumventricular organ and ocular vasculatures. Elife 8:35. doi: 10.7554/eLife.43257.035
Wilhelm, I., Nyul-Toth, A., Suciu, M., Hermenean, A., and Krizbai, I. A. (2016). Heterogeneity of the blood-brain barrier. Tissue Barriers 4:e1143544. doi: 10.1080/21688370.2016.1143544
Wu, Y. T, Bennett, H. C., Chon, U., Vanselow, D. J., Zhang, Q., Muñoz-Castañeda, R., et al. (2021). The cellular architecture of microvessels, pericytes and neuronal cell types in organizing regional brain energy homeostasis in mice. bioRxiv. doi: 10.1101/2021.05.19.444854
Yamamoto, S., Muramatsu, M., Azuma, E., Ikutani, M., Nagai, Y., Sagara, H., et al. (2017). A subset of cerebrovascular pericytes originates from mature macrophages in the very early phase of vascular development in CNS. Sci. Rep. 7:3855. doi: 10.1038/s41598-017-03994-1
Yamazaki, T., and Mukouyama, Y. S. (2018). Tissue specific origin, development, and pathological perspectives of pericytes. Front. Cardiovasc. Med. 5:78. doi: 10.3389/fcvm.2018.00078
Yang, A. C., Stevens, M. Y., Chen, M. B., Lee, D. P., Stahli, D., Gate, D., et al. (2020). Physiological blood-brain transport is impaired with age by a shift in transcytosis. Nature 583, 425–430. doi: 10.1038/s41586-020-2453-z
Yang, J., Lunde, L. K., Nuntagij, P., Oguchi, T., Camassa, L. M., Nilsson, L. N., et al. (2011). Loss of astrocyte polarization in the tg-ArcSwe mouse model of Alzheimer's disease. J. Alzheimers Dis. 27, 711–722. doi: 10.3233/JAD-2011-110725
Yao, J., and Wang, L. V. (2014). Photoacoustic brain imaging: from microscopic to macroscopic scales. Neurophotonics 1:011003. doi: 10.1117/1.NPh.1.1.011003
Yasuda, K., Cline, C., Lin, Y. S., Scheib, R., Ganguly, S., Thirumaran, R. K., et al. (2015). In vivo imaging of human MDR1 transcription in the brain and spine of MDR1-luciferase reporter mice. Drug Metab. Dispos. 43, 1646–1654. doi: 10.1124/dmd.115.065078
Yu, L., Thurston, E. M. S., Hashem, M., Dunn, J. F., Whelan, P. J., and Murari, K. (2020). Fiber photometry for monitoring cerebral oxygen saturation in freely-moving rodents. Biomed. Opt. Express. 11, 3491–3506. doi: 10.1364/BOE.393295
Keywords: neurovascular unit, regional heterogeneity, blood-brain barrier, pericytes, astrocytes, endothelial cell
Citation: Bernier LP, Brunner C, Cottarelli A and Balbi M (2021) Location Matters: Navigating Regional Heterogeneity of the Neurovascular Unit. Front. Cell. Neurosci. 15:696540. doi: 10.3389/fncel.2021.696540
Received: 16 April 2021; Accepted: 31 May 2021;
Published: 30 June 2021.
Edited by:
Fabrice Dabertrand, University of Colorado, United StatesReviewed by:
Andy Shih, Seattle Children's Research Institute, United StatesThomas Longden, University of Maryland, Baltimore, United States
Grant Robert Gordon, University of Calgary, Canada
Copyright © 2021 Bernier, Brunner, Cottarelli and Balbi. This is an open-access article distributed under the terms of the Creative Commons Attribution License (CC BY). The use, distribution or reproduction in other forums is permitted, provided the original author(s) and the copyright owner(s) are credited and that the original publication in this journal is cited, in accordance with accepted academic practice. No use, distribution or reproduction is permitted which does not comply with these terms.
*Correspondence: Matilde Balbi, m.balbi@uq.edu.au