- Department of Neuroscience, Wexner Medical Center, The Ohio State University, Columbus, OH, United States
Myelination is essential for signal processing within neural networks. Emerging data suggest that neuronal activity positively instructs myelin development and myelin adaptation during adulthood. However, the underlying mechanisms controlling activity-dependent myelination have not been fully elucidated. Myelination is a multi-step process that involves the proliferation and differentiation of oligodendrocyte precursor cells followed by the initial contact and ensheathment of axons by mature oligodendrocytes. Conventional end-point studies rarely capture the dynamic interaction between neurons and oligodendrocyte lineage cells spanning such a long temporal window. Given that such interactions and downstream signaling cascades are likely to occur within fine cellular processes of oligodendrocytes and their precursor cells, overcoming spatial resolution limitations represents another technical hurdle in the field. In this mini-review, we discuss how advanced genetic, cutting-edge imaging, and electrophysiological approaches enable us to investigate neuron-oligodendrocyte lineage cell interaction and myelination with both temporal and spatial precision.
Introduction
Myelination refers to the process of wrapping axons with compact layers of the plasma membrane, and it occurs primarily postnatally (Foran and Peterson, 1992; Baumann and Pham-Dinh, 2001; Nishiyama et al., 2009). The presence of myelin sheaths drastically increases the conduction velocity along axons. As the conduction velocity can be fine-tuned by myelin thickness and sheath length (Ford et al., 2015; Etxeberria et al., 2016), myelin is also pivotal in maintaining precise spatiotemporal activity patterns in neuronal circuits. Several recent studies particularly highlighted the importance of myelination for proper neuronal circuit activities and cognitive function in health and diseases (Pan et al., 2020; Steadman et al., 2020; Chen et al., 2021). Myelin sheaths in the central nervous system (CNS) are exclusively formed by oligodendrocytes that are generated from the differentiation of oligodendrocyte precursor cells (OPCs). Oligodendrocytes have the innate capability to wrap fibrous structures (Lee et al., 2012; Bechler et al., 2015). With the development of various pharmacological and genetic tools, it became clear that oligodendrogenesis and myelin formation are regulated by both intrinsic programs and extrinsic factors (Li and Richardson, 2016; Mayoral and Chan, 2016; Fletcher et al., 2018). Accumulating evidence has shown that social interaction (Liu et al., 2012; Makinodan et al., 2012), experience (Hughes et al., 2018), sensory inputs (Mangin et al., 2012; Hill et al., 2014), and motor learning activities (McKenzie et al., 2014; Bacmeister et al., 2020) modulate myelin adaptation and oligodendrocyte lineage cell behavior. Recent studies using state-of-the-art optogenetic (Gibson et al., 2014) and chemogenetic (Mitew et al., 2018) techniques showed that stimulating neuronal firing increased myelin thickness along the axons and oligodendrogenesis within the stimulated region. However, our understanding of the mechanisms underlying “activity-dependent myelination” is still limited.
For the past two decades, the development of various transgenic fluorescence reporter mouse lines has enabled us to directly visualize oligodendrocyte lineage cells at different stages (Mallon et al., 2002; Yuan et al., 2002; Karram et al., 2008; Zhu et al., 2008; Hughes et al., 2013). Genetic fate mapping studies using OPC specific Cre or CreER lines crossed with Cre-reporter mice have also generated vast information regarding oligodendrocyte lineage plasticity in both physiological and pathological conditions across multiple CNS regions (Lappe-Siefke et al., 2003; Rivers et al., 2008; Kang et al., 2010; Zhu et al., 2011; Tognatta et al., 2017; Huang et al., 2019). More recent studies have focused on manipulating the oligodendroglial-specific expression of key molecules in regulating oligodendroglial lineage plasticity or neuron-oligodendroglial communication. Together with the development of novel electrophysiological and optical techniques, we now have more comprehensive tools to study the underlying mechanisms for activity-dependent myelination.
Manipulating The Expression of Postsynaptic Neurotransmitter Receptors in Oligodendrocyte Lineage Cells
The discovery of neuron-OPC synaptic contacts (Bergles et al., 2000) has inspired the myelin research field for the last 2 decades. The prevalence of such synapses across different CNS regions (Lin and Bergles, 2004; Jabs et al., 2005; Lin et al., 2005; Ge et al., 2006; Káradóttir et al., 2008; Kukley et al., 2008; Mangin et al., 2008; Vélez-Fort et al., 2010), including the white matter (Kukley et al., 2007; Ziskin et al., 2007), and the fact that synaptic contacts enable neuron-OPC communication with both temporal and spatial precision position them as an ideal candidate to instruct activity-dependent myelination. Novel genetic mouse models that specifically silence or overexpress different postsynaptic neurotransmitter receptors in oligodendrocyte lineage cells can manipulate neuron-OPC synaptic transmission without interfering with neuron-neuron communication, thereby providing in-depth insights into the functional implications of these neuron-OPC synapses.
Glutamatergic postsynaptic currents in OPCs exhibit fast kinetics and are primarily mediated through AMPA receptors. In a recent study, Kougioumtzidou et al. (2017) created several murine transgenic lines to reduce or eliminate AMPA receptor-mediated postsynaptic responses by crossing Sox10-Cre line and Rosa-YFP reporter line with different combinations of Gria2flox/flox line, Gria4flox/flox line, and Gria3 null line. Triple knock-out of GluA2–4 showed almost no AMPA receptor-mediated spontaneous synaptic responses (Figure 1A) and exhibited reduced numbers of mature oligodendrocytes within the corpus callosum in mice at both P14 and P53, while the proliferation of OPCs remained unaltered (Kougioumtzidou et al., 2017). Similar results in oligodendrocyte lineage were observed with Gria2−/−Gria3 null double transgenic mice, where the AMPA receptor-mediated currents were reduced by half (Kougioumtzidou et al., 2017). However, neither single transgenic Sox10-Cre: Gria2flox/flox nor germline Gria3 null mice alone displayed any phenotype in oligodendrocyte lineage development and myelination, suggesting that there might be compensatory mechanisms between the expression of AMPA receptor subunits (Kougioumtzidou et al., 2017).
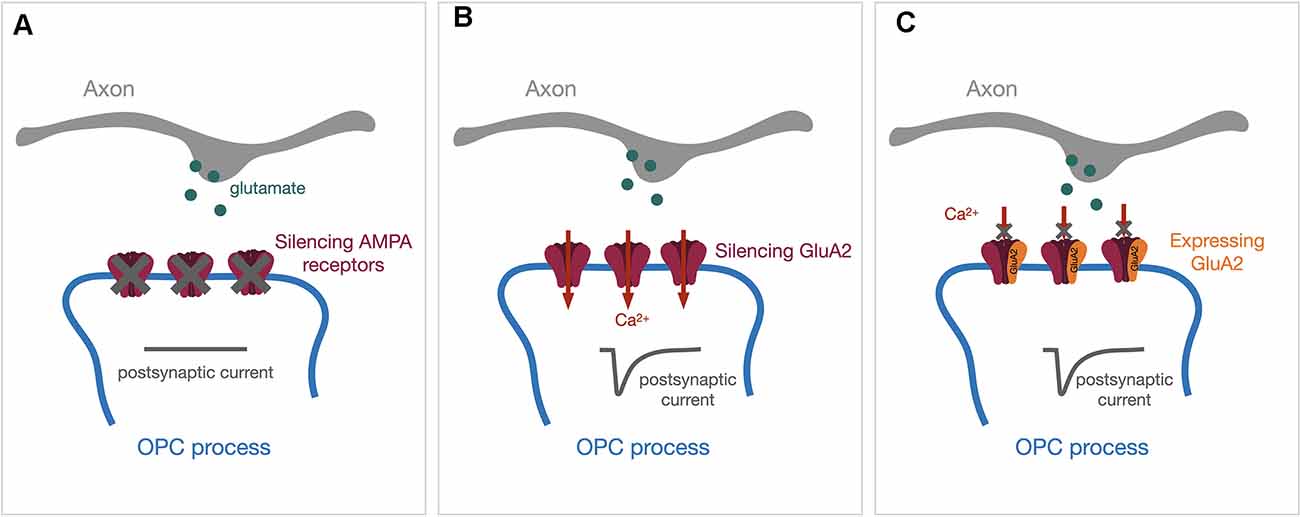
Figure 1. Genetic approaches to manipulate the expression of AMPA receptors in OPCs. Synaptic vesicles containing glutamate (green dots) will be released upon the arrival of action potentials. The release of glutamate will activate post-synaptic AMPA receptors and evoke a post-synaptic current (dark gray traces). (A) Genetically silencing all AMPA receptor subunits will diminish AMPA receptor-mediated postsynaptic currents. (B) Silencing the GluA2 subunit alone will increase Ca2+ permeability through AMPA receptors. (C) Expressing the GluA2-containing AMPA receptor will block the Ca2+ entry through AMPA receptors. OPCs, oligodendrocyte precursor cells.
Among GluA1–4 subunits, GluA2 determines the Ca2+ permeability such that only receptors lacking GluA2 are Ca2+ permeable. Previous studies demonstrated that OPCs express Ca2+ permeable AMPA receptors in the hippocampus (Bergles et al., 2000; Ge et al., 2006). In the corpus callosum, Ca2+ permeable AMPA receptors can only be detected in OPCs during adulthood (Ziskin et al., 2007), but not in the early postnatal period (Kukley et al., 2007). One emerging question is whether Ca2+ permeability through AMPA receptors regulates oligodendrocyte lineage plasticity and activity-dependent myelination. Different genetic strategies have been utilized to address this question. In the study mentioned above, Kougioumtzidou et al. (2017) did not observe any changes in oligodendrocyte lineage development and myelination from the corpus callosum at P14 using the Sox10-Cre: Gria2flox/flox line to increase Ca2+ permeability by deleting GluA2 in OPCs (Figure 1B). Conversely, Khawaja et al. (2021) recently generated an inducible GluA2 overexpression line (Sox10-CreER:Ai14:R26-Gria2) where the decreases in Ca2+ permeability through AMPA receptors (Figure 1C) resulted in an increase in OPCs only in the adult mice. Myelination in younger mice from the same line was not affected by GluA2 overexpression when 4-Hydroxytamoxifen was injected early postnatally (Khawaja et al., 2021).
Besides transgenic mouse lines, viral gene delivery approaches are routinely used for transgene expression in vivo. Although most adeno-associated viruses (AAV) serotypes exhibit dominance in neuronal tropism with constitutive promoters driving the gene expression (Burger et al., 2004; Cearley and Wolfe, 2006), several studies successfully drove oligodendrocyte-specific gene expression in vivo using AAVs with oligodendrocyte-specific promoters, such as myelin basic protein or myelin-associated glycoprotein (Chen et al., 1998; Lawlor et al., 2009; Von Jonquieres et al., 2016), or packaging AAV vectors with a novel oligotropic capsid (Powell et al., 2016; Weinberg et al., 2017). However, so far, AAV particles rarely transduce OPCs in a specific and consistent fashion in vivo. Instead, the retrovirus is a good alternative as it only transfects dividing cells (Van Praag et al., 2002), and OPCs are the major source of proliferating cells in the postnatal CNS (Dawson et al., 2003). While also aiming at manipulating AMPA receptor-dependent Ca2+ permeability, Chen et al. (2018) modified OPC-expressed AMPA receptors in vivo using retroviral vectors early postnatally. They delivered retroviral vectors expressing either full-length GluA2 with point mutations or truncated GluA2 into the corpus callosum during postnatal 2–3 weeks and reported that ~95% of transduced cells belong to oligodendrocyte lineage (Chen et al., 2018). This study showed that GluA2 point mutations increasing the AMPA receptor Ca2+ permeability enhanced OPC proliferation and suppressed their differentiation. Perturbing the trafficking of GluA2-containing AMPARs by expressing the cytoplasmic C-terminal of the GluA2 subunit decreased the OPC differentiation without affecting the proliferation during myelin development (Chen et al., 2018). While the two studies mentioned above used transgenic lines to manipulate GluA2 expression in the total OPC population, this study only targeted proliferating OPCs, which may explain the difference in their observations.
OPCs gradually lose synaptic contacts with neurons during their differentiation into oligodendrocytes, accompanied by a downregulation of ionotropic glutamate receptors (De Biase et al., 2010; Kukley et al., 2010). Nevertheless, Evonuk et al. (2020) crossed the PLP-CreER line with Gria4tm1Mony mice (Fuchs et al., 2007) to specifically knock-out GluA4 in more mature PLP+ oligodendroglial cells. They reported that deleting GluA4 ameliorated myelin degeneration and axonal damage in a mouse model of multiple sclerosis (Evonuk et al., 2020). Oligodendrocyte lineage cells also express NMDA receptors (Káradóttir et al., 2005, 2008; Salter and Fern, 2005; Micu et al., 2006). These NMDA receptors are reported to be involved in mediating myelin responses after CNS injuries (Káradóttir et al., 2005; Micu et al., 2006, 2007). It has been suggested that remyelination after white matter injury is NMDA receptor-dependent (Lundgaard et al., 2013). Genetic models that specifically delete NMDA receptor subunit NR1 in OPCs exhibited normal myelination and did not show obvious alteration in survival or proliferation of OPCs (De Biase et al., 2011; Guo et al., 2012). However, Saab et al. (2016) recently reported that oligodendroglial NMDA receptors regulate GLUT1-mediated glucose import into the myelin compartment, and the deletion of NR1 in CNP+ oligodendroglial cells led to a delay in myelin development.
GABAergic interneuron-OPC synapses primarily activate Cl−-permeable GABAA receptors in OPCs. Instead of hyperpolarizing the membrane potential, the activation of GABAA receptor depolarizes the post-synaptic membrane due to high intracellular Cl− concentration (Lin and Bergles, 2004; Passlick et al., 2013). GABAergic synaptic inputs in OPCs are therefore not electrically “inhibitory”. While direct GABAergic synaptic contacts in OPCs persist through the development to adulthood in the mouse hippocampus (Lin and Bergles, 2004), in the cortex those direct synaptic activities start decreasing after the second postnatal week and gradually switch to the volume transmission mode mediated by the extra-synaptic activation of GABAA receptors (Vélez-Fort et al., 2010). In the neocortex, OPCs are highly connected with fast-spiking parvalbumin (PV) interneurons, and γ2 subunits of GABAA receptors are exclusively expressed at post-synaptic sites of PV interneuron-OPC synapses (Orduz et al., 2015). Balia et al. (2017) bred NG2creERT2:GCamp3: γ2fl/fl mice to specifically inactivate γ2- GABAA receptor-mediated synapses in OPCs early postnatally. They reported no alteration in OPC proliferation or differentiation, but a decrease in OPC density at P30 compared to control mice, suggesting that γ2- GABAA receptors play a role in OPC self-maintenance (Balia et al., 2017). In a more recent study from the same group, Benamer et al. (2020) further characterized this transgenic line and revealed that γ2 mutant mice exhibited abnormal axonal morphology and myelin distribution in PV interneurons, along with increased nodal and internodal lengths of myelinated PV axons. Those observed myelin defects decreased the firing frequency of PV interneurons and their connectivity with excitatory neurons, leading to the impairment of sensory function in γ2 mutant mice (Benamer et al., 2020). It will be interesting for future studies to investigate the functional role of synaptic contacts between other types of interneurons and OPCs, perhaps with genetic models that specifically delete GABAA receptors in OPCs in general.
Eliciting Local Postsynaptic Responses within OPC Processes
Accumulating data have suggested that neuronal activity-induced downstream signaling often occurs locally within the thin processes of oligodendroglial cells. Axonal stimulation using electrical, optogenetic, or chemogenetic strategies has been shown to induce local myelin basic protein synthesis within OPC processes (Wake et al., 2011) and increase myelin thickness of stimulated axons (Gibson et al., 2014; Mitew et al., 2018). Confocal time-lapse imaging studies have demonstrated that cellular processes of oligodendrocyte lineage cells actively contact and form initial ensheathment with axons, but the stabilization and elongation of formed myelin sheaths are regulated by neuronal activity (Hines et al., 2015; Mensch et al., 2015; Koudelka et al., 2016). Thus far, it is not clear whether the single contacted axon’s activity is sufficient to trigger downstream signaling cascades within OPC processes locally, or whether activities from a large group of axons are required to initiate global signaling that spreads out to the whole OPC process architecture. To tease apart these two scenarios, we need to use experimental paradigms that will only activate one or just a few synapses within a spatially confined region. We previously applied local electrical stimulation by placing a mono-polar stimulating electrode approximately 5 μm away from the target OPC process segment (Figure 2A; Sun et al., 2016). When combining electrophysiology recording with 2-photon Ca2+ imaging, we observed that such a local stimulus is sufficient to trigger Ca2+ entry within the local compartment with similar kinetics as the global voltage-gated Ca2+ channel (VGCC)-mediated Ca2+ entry induced by current injection (Sun et al., 2016). The somatic depolarization upon local stimulation was well below the activation threshold for VGCCs, and we did not observe a widespread global Ca2+ signal upon local stimulation. Our results suggest that only a limited number of axons passing the target OPC process were activated upon local stimulation, and also indicate a substantial attenuation along the OPC processes, as suggested by our previous theoretical simulation (Sun and Dietrich, 2013). Our observation also suggests the expression of voltage-gated ion channels within OPC processes (Sun et al., 2016). Therefore, the local synaptic response can be amplified or dampened through the activation of ion channels within processes. Since myelin basic protein, and likely other myelin proteins could be synthesized within the OPC processes (Wake et al., 2011), future studies could utilize local electrical stimulation together with advanced in situ hybridization techniques, such as RNAscope (Wang et al., 2012), to address remaining questions like whether local synaptic inputs are sufficient to initialize the transport, local expression, and translation of myelin-specific mRNAs.
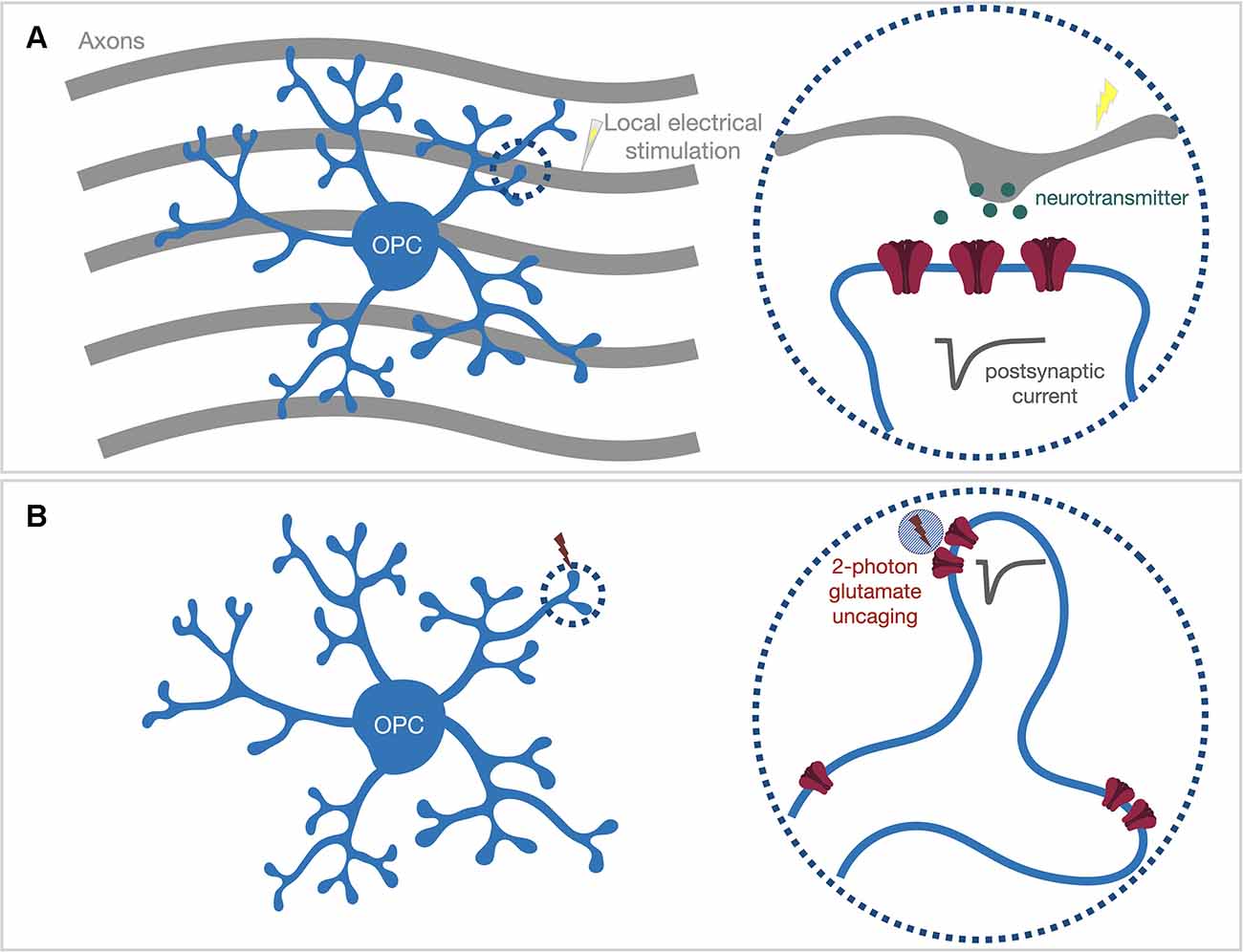
Figure 2. Locally activate postsynaptic receptors within OPC processes via either (A) local electrical stimulation or (B) 2-photon glutamate uncaging. (A) The mono-polar stimulating electrode can be placed in close proximity (~5 μm) to the target OPC process (dash circle) to only stimulate the axons passing the process segment. (B) Acute brain slices are bathed with caged-glutamate containing bath solution. The 2-photon uncaging laser (~720 nm) is pointed to the target locations by galvo scan mirrors inside the scan head. A brief uncaging laser pulse (0.6–1 ms) will uncage the caged-glutamate compound within the 2-photon excitation volume (blue shaded circle) and evoke an electrical response from post-synaptic neurotransmitter receptors (dark gray trace).
Nevertheless, the spatial resolution of local electrical stimulation is not sufficient to accurately identify which nearby synapses are activated, especially when there is no optical tool available to visualize where synapses are located along OPC processes. To circumvent this limitation, the alternative approach is to optically activate postsynaptic neurotransmitter receptors on OPC processes with high optical resolution (Figure 2B). 2-photon glutamate uncaging generates an optically controlled transient release of glutamate by brief photolysis of caged-glutamate within the diffraction-limited 2-photon excitation volume (Matsuzaki et al., 2001). A single uncaging pulse applied at the dendritic spine head of neurons can produce an AMPA receptor-mediated current response similar to a synaptic response (Matsuzaki et al., 2001). We reported that the same strategy could be used to map AMPA receptors in OPCs (Sun et al., 2016). Although OPC processes are not equipped with spine structures that clearly indicate the clustering of AMPA receptors, we demonstrated that AMPA receptors are expressed along the process (Sun et al., 2016). The fact that the same uncaging pulse (720 nm, 0.65 ms, ~35–45 mW at the surface of the specimen; Sun et al., 2016) produced variable response sizes along a process segment suggests that AMPA receptors do not distribute homogeneously, but instead, might cluster in “hot spots”. However, whether those AMPA receptor “hot spots” represent locations of neuron-OPC synapses needs further investigation.
Beyond mapping AMPA receptor distribution, 2-photon glutamate uncaging may be useful to explore the functional implication of neuron-OPC synapses. For example, it can be used to study how OPCs integrate neuronal inputs from different synaptic contact sites and initialize downstream signaling cascades. Various electrophysiological studies characterized different voltage-gated ion channel expression in OPCs (Steinhäuser et al., 1994; Kressin et al., 1995; Akopian et al., 1996; Chittajallu et al., 2004; Tong et al., 2009; De Biase et al., 2010; Kukley et al., 2010; Haberlandt et al., 2011), and many of those ion channels, e.g., calcium and potassium channels, are likely expressed on the membrane of thin processes (Sun et al., 2016). The fact that voltage signals strongly attenuate along the OPC process (Sun and Dietrich, 2013) highlights the importance of understanding how these locally expressed ion channels respond to inputs from multiple synapses and shape the post-synaptic membrane potentials within OPC process compartments. The size of integrated local post-synaptic membrane potential might determine whether downstream signaling cascades can be triggered, e.g., whether Ca2+ channels can be activated and elicit a local Ca2+ transient. While local electrical stimulation cannot accurately determine how many synapses will be activated and where those activated synapses are located, 2-photon uncaging pulses can be pointed to multiple spatially apart spots with a minimal switching time (0.6–1 ms per uncaging pulse plus 0.1 ms laser switching time; Sun et al., 2016). Therefore, when combined with Ca2+ imaging and patch-clamp recording, 2-photon uncaging is an ideal tool to investigate the voltage integration of synaptic inputs from different sites and the subsequent Ca2+ signaling within a local process compartment. Glutamate uncaging is also suited to study activity-dependent synaptic or morphological plasticity. Ge et al. (2006) reported Ca2+ permeable AMPA receptor-mediated long-term potentiation (persistent strengthening of synaptic transmission) in OPCs from the murine hippocampus. Thus far, it is unclear whether such plasticity and potentially other types of synaptic plasticity exist at an individual synapse level and whether those types of synaptic plasticity are required for local translation of myelin proteins. Additionally, it is known that OPCs are motile and have filopodia-like protrusions constantly extending and retracting (Hughes et al., 2013). Understanding whether synaptic inputs can guide those dynamic protrusion movements to form initial contact with axons will be an important direction for future investigations. Indeed, a similar approach has been used in neuronal studies to demonstrate the occurrence of long-term potentiation at individual spines (Matsuzaki et al., 2004) and repetitive uncaging pulses-induced formation of new functional spines (Kwon and Sabatini, 2011). Taken together, 2-photon glutamate uncaging combined with other techniques is a powerful tool to tackle important questions like whether local synaptic integration is functionally meaningful and whether any specific neuronal activity patterns are preferred for initiation of myelin wrapping.
Capturing The Dynamics of The Myelin Formation with Time-Lapse Imaging
Myelination is a multi-step process that involves the proliferation and differentiation of oligodendrocyte precursor cells followed by the initial contact and ensheathment of axons by mature oligodendrocytes. The whole process spans a long temporal window, and conventional end-point studies cannot capture the dynamics during the whole process. Recent studies have employed time-lapse imaging techniques based on confocal microscopy in the zebrafish model and generated crucial information about the initial myelin sheath formation along axons (Hines et al., 2015; Mensch et al., 2015; Koudelka et al., 2016). Those studies changed our previous knowledge or assumption that oligodendrocytes only select the electrically active axons to make the initial contact and subsequently start the wrapping process to form a sheath segment. Instead, oligodendrocytes constantly make contacts with axons and form initial myelin sheath segments, but at the same time, also often retract some formed sheaths. Therefore, the stabilization of those established sheaths is the key, and this step is heavily dependent on axonal vesicle release (Hines et al., 2015). Moreover, neuronal activity also influences the length of the sheaths (Hines et al., 2015) and the number of myelin sheaths each oligodendrocyte makes (Mensch et al., 2015). It is worth mentioning that to visualize oligodendrocyte lineage cells in different stages, those studies generated transgenic lines that express fluorescence protein under promoters (e.g., Sox10) that are widely expressed at almost all stages within oligodendrocyte lineage. In addition, they often chose to express membrane-anchored fluorescence protein instead of cytoplasm-expressing ones for the better visualization of myelin sheaths. Using synaptic protein-expressing transgenic zebrafish models, several studies further demonstrated the relationship between axonal vesicle exocytosis and myelin sheath formation through in vivo time-lapse imaging (Hughes and Appel, 2019; Almeida et al., 2021). To investigate how oligodendrocyte lineage cells react to axonal activity or vesicle release, recent studies also used genetically encoded Ca2+ indicators and showed that various myelin sheaths movements are accompanied by Ca2+ transients with different duration and frequencies within oligodendrocyte processes (Baraban et al., 2018; Krasnow et al., 2018; Hughes and Appel, 2020). By combining Ca2+ imaging with the single-cell RNA sequencing technique, Marisca et al. (2020) also demonstrated that subgroups of OPCs exhibited widespread heterogeneity in eliciting Ca2+ responses and initiating proliferation or differentiation upon neuronal activities.
The zebrafish larva as the animal model clearly shows the advantage of in vivo imaging due to its transparency. When looking at imaging techniques that can be applied in vivo for mouse models, the multi-photon laser scanning system surpasses the confocal system as it penetrates deeper into the tissue with less scattering and phototoxicity. However, it is worth mentioning that 2-photon microscopy generally does not reach more than 500 μm deep in the brain. Imaging deeper brain structures often involves tissue removal and the embedment of a gradient index lens (Jung et al., 2004). Future studies employing adaptive optical correction (Wang et al., 2015) or utilizing 3-photon in vivo imaging (Horton et al., 2013; Hontani et al., 2021) might partially overcome this limitation. Recent studies utilizing in vivo 2-photon imaging demonstrated how OPCs maintain hemostasis (Hughes et al., 2013), exhibit experience-dependent oligodendrogenesis and myelin adaptation (Hughes et al., 2018), and undergo adaptive remodeling during motor learning activities (Bacmeister et al., 2020). Choosing the appropriate time interval between the consecutive acquisition sessions is critical for these studies because the interval represents the temporal resolution. One must carefully set this parameter to obtain sufficient details about the target cellular event without generating a vast number of images. For example, we now know that OPCs are highly motile and can translocate to a new position within 2–3 days, even in adulthood (Hughes et al., 2013). Therefore, the interval between imaging sessions needs to be set much shorter than 2–3 days to follow the same group of cells over the whole imaging period. Longitudinal in vivo imaging studies take over days to weeks and involve re-anesthetizing the animal and re-identifying the exact imaging area between imaging sessions. Setting the imaging area with easily identified landmarks, e.g., visible blood vessels, is often helpful. Imaging processing and analysis are also challenging for in vivo time-lapse imaging studies, and it usually requires algorithms to correct moving artifacts and align all images taken at different time points. Due to technical difficulties such as motion artifacts caused by heartbeat and breathing, fewer studies employed time-lapse in vivo imaging for the spinal cord than the brain. However, the development of implantable spinal cord windows (Farrar et al., 2012; Tedeschi et al., 2016) may allow future studies to follow the dynamic communication between axons and oligodendroglial cells within the murine spinal cord. Miniaturized microscopes, also called miniscopes, can be directly head-mounted in freely moving animals and allow optical recording over an extended period (Ghosh et al., 2011). However, the conventional miniscope has lower optical resolution than the 2-photon scanning system and therefore cannot resolve finer cellular structures like dendritic spines (Silva, 2017). The ongoing development of 2-photon miniscopes (Zong et al., 2017) might overcome this problem and provide in vivo imaging in freely behaving animals with greater optical resolution. Miniscope platforms might be suited for future studies investigating longitudinal changes in oligodendroglial plasticity and myelin formation in various behavioral paradigms.
Besides imaging oligodendroglial cells and myelin sheaths with genetically labeled fluorescence proteins, a couple of label-free in vivo myelin imaging techniques have also been developed during the last decade. Coherent anti-Stokes Raman scattering (CARS) microscopy utilizes the intrinsic CH2 symmetric stretching vibration of the enriched lipid structure to image myelin sheaths (Wang et al., 2005; Fu et al., 2008). Meanwhile, spectral confocal reflectance microscopy (SCoRe; Schain et al., 2014) generates label-free myelin images by capturing the highly reflective signals from lipids using several lasers with different wavelengths. In combination with in vivo fluorescence microscopy (Schain et al., 2014), those novel label-free techniques can image dynamics between axons and myelin sheaths with great resolution and reduce the dependence on genetic models for in vivo imaging of myelin. The long-term stability and phototoxicity of these label-free imaging techniques still need to be characterized for time-lapse in vivo imaging studies spanning multiple days.
Concluding Remarks
We have now reached a widely accepted notion that neuronal activity positively regulates myelin development and adaptation during adulthood. It is clear that activity-dependent myelination is a fairly dynamic process, and future studies will require the design of specific experimental paradigms to capture the dynamics with great temporal and spatial precision. Future studies using a multidisciplinary approach addressing whether inputs from just a few synapses are sufficient to initialize the expression and translation of myelin-specific mRNAs within OPC processes and whether there is any preferred neuronal firing pattern for initiating downstream cellular events will enhance our understanding of the functional role of neuron-OPC synapses. Additionally, monitoring oligodendroglial cellular behavior and tracing key intracellular signaling molecule’s activity in vivo in various behavioral paradigms will offer further insights into the mechanisms that control activity-dependent myelination.
Author Contributions
JH and WS wrote the manuscript. WS conceived the project and supervised the overall progress. All authors contributed to the article and approved the submitted version.
Funding
This work was supported by the start-up fund from Wexner Medical Center at The Ohio State University (to WS).
Conflict of Interest
The authors declare that the research was conducted in the absence of any commercial or financial relationships that could be construed as a potential conflict of interest.
Publisher’s Note
All claims expressed in this article are solely those of the authors and do not necessarily represent those of their affiliated organizations, or those of the publisher, the editors and the reviewers. Any product that may be evaluated in this article, or claim that may be made by its manufacturer, is not guaranteed or endorsed by the publisher.
Acknowledgments
We thank Dr. Andrea Tedeschi and Dr. Maria Kukley for the helpful discussion and comments. We also thank Haven Rodocker and Alaina Scavuzzo for proofreading.
References
Akopian, G., Kressin, K., Derouiche, A., and Steinhauser, C. (1996). Identified glial cells in the early postnatal mouse hippocampus display different types of Ca2+ currents. Glia 17, 181–194. doi: 10.1002/(SICI)1098-1136(199607)17:3<181::AID-GLIA1>3.0.CO;2-4
Almeida, R. G., Williamson, J. M., Madden, M. E., Early, J. J., Voas, M. G., Talbot, W. S., et al. (2021). Myelination induces axonal hotspots of synaptic vesicle fusion that promote sheath growth. Curr. Biol. 31, 3743–3754.e5. doi: 10.1016/j.cub.2021.06.036
Bacmeister, C. M., Barr, H. J., McClain, C. R., Thornton, M. A., Nettles, D., Welle, C. G., et al. (2020). Motor learning promotes remyelination via new and surviving oligodendrocytes. Nat. Neurosci. 23, 819–831. doi: 10.1038/s41593-020-0637-3
Balia, M., Benamer, N., and Angulo, M. C. (2017). A specific GABAergic synapse onto oligodendrocyte precursors does not regulate cortical oligodendrogenesis. Glia 65, 1821–1832. doi: 10.1002/glia.23197
Baraban, M., Koudelka, S., and Lyons, D. A. (2018). Ca2+ activity signatures of myelin sheath formation and growth in vivo. Nat. Neurosci. 21, 19–23. doi: 10.1038/s41593-017-0040-x
Baumann, N., and Pham-Dinh, D. (2001). Biology of oligodendrocyte and myelin in the mammalian central nervous system. Physiol. Rev. 81, 871–927. doi: 10.1152/physrev.2001.81.2.871
Bechler, M. E., Byrne, L., and Ffrench-Constant, C. (2015). CNS myelin sheath lengths are an intrinsic property of oligodendrocytes. Curr. Biol. 25, 2411–2416. doi: 10.1016/j.cub.2015.07.056
Benamer, N., Vidal, M., Balia, M., and Angulo, M. C. (2020). Myelination of parvalbumin interneurons shapes the function of cortical sensory inhibitory circuits. Nat. Commun. 11:5151. doi: 10.1038/s41467-020-18984-7
Bergles, D. E., Roberts, J. D., Somogyi, P., and Jahr, C. E. (2000). Glutamatergic synapses on oligodendrocyte precursor cells in the hippocampus. Nature 405, 187–191. doi: 10.1038/35012083
Burger, C., Gorbatyuk, O. S., Velardo, M. J., Peden, C. S., Williams, P., Zolotukhin, S., et al. (2004). Recombinant AAV viral vectors pseudotyped with viral capsids from serotypes 1, 2, and 5 display differential efficiency and cell tropism after delivery to different regions of the central nervous system. Mol. Ther. 10, 302–317. doi: 10.1016/j.ymthe.2004.05.024
Cearley, C. N., and Wolfe, J. H. (2006). Transduction characteristics of adeno-associated virus vectors expressing cap serotypes 7, 8, 9, and Rh10 in the mouse brain. Mol. Ther. 13, 528–537. doi: 10.1016/j.ymthe.2005.11.015
Chen, T.-J., Kula, B., Nagy, B., Barzan, R., Gall, A., Ehrlich, I., et al. (2018). in vivo regulation of oligodendrocyte precursor cell proliferation and differentiation by the AMPA-receptor subunit GluA2. Cell Rep. 25, 852–861.e7. doi: 10.1016/j.celrep.2018.09.066
Chen, J.-F., Liu, K., Hu, B., Li, R.-R., Xin, W., Chen, H., et al. (2021). Enhancing myelin renewal reverses cognitive dysfunction in a murine model of Alzheimer’s disease. Neuron 109, 2292–2307.e5. doi: 10.1016/j.neuron.2021.05.012
Chen, H., Mccarty, D. M., Bruce, A. T., Suzuki, K., and Suzuki, K. (1998). Gene transfer and expression in oligodendrocytes under the control of myelin basic protein transcriptional control region mediated by adeno-associated virus. Gene Ther. 5, 50–58. doi: 10.1038/sj.gt.3300547
Chittajallu, R., Aguirre, A., and Gallo, V. (2004). NG2-positive cells in the mouse white and grey matter display distinct physiological properties. J. Physiol. 561, 109–122. doi: 10.1113/jphysiol.2004.074252
Dawson, M. R., Polito, A., Levine, J. M., and Reynolds, R. (2003). NG2-expressing glial progenitor cells: an abundant and widespread population of cycling cells in the adult rat CNS. Mol. Cell. Neurosci. 24, 476–488. doi: 10.1016/s1044-7431(03)00210-0
De Biase, L. M., Kang, S. H., Baxi, E. G., Fukaya, M., Pucak, M. L., Mishina, M., et al. (2011). NMDA receptor signaling in oligodendrocyte progenitors is not required for oligodendrogenesis and myelination. J. Neurosci. 31, 12650–12662. doi: 10.1523/JNEUROSCI.2455-11.2011
De Biase, L. M., Nishiyama, A., and Bergles, D. E. (2010). Excitability and synaptic communication within the oligodendrocyte lineage. J. Neurosci. 30, 3600–3611. doi: 10.1523/JNEUROSCI.6000-09.2010
Etxeberria, A., Hokanson, K. C., Dao, D. Q., Mayoral, S. R., Mei, F., Redmond, S. A., et al. (2016). Dynamic modulation of myelination in response to visual stimuli alters optic nerve conduction velocity. J. Neurosci. 36, 6937–6948. doi: 10.1523/JNEUROSCI.0908-16.2016
Evonuk, K. S., Doyle, R. E., Moseley, C. E., Thornell, I. M., Adler, K., Bingaman, A. M., et al. (2020). Reduction of AMPA receptor activity on mature oligodendrocytes attenuates loss of myelinated axons in autoimmune neuroinflammation. Sci. Adv. 6:eaax5936. doi: 10.1126/sciadv.aax5936
Farrar, M. J., Bernstein, I. M., Schlafer, D. H., Cleland, T. A., Fetcho, J. R., and Schaffer, C. B. (2012). Chronic in vivo imaging in the mouse spinal cord using an implanted chamber. Nat. Methods 9, 297–302. doi: 10.1038/nmeth.1856
Fletcher, J. L., Murray, S. S., and Xiao, J. (2018). Brain-derived neurotrophic factor in central nervous system myelination: a new mechanism to promote myelin plasticity and repair. Int. J. Mol. Sci. 19:4131. doi: 10.3390/ijms19124131
Foran, D. R., and Peterson, A. C. (1992). Myelin acquisition in the central nervous system of the mouse revealed by an MBP-Lac Z transgene. J. Neurosci. 12, 4890–4897. doi: 10.1523/JNEUROSCI.12-12-04890.1992
Ford, M. C., Alexandrova, O., Cossell, L., Stange-Marten, A., Sinclair, J., Kopp-Scheinpflug, C., et al. (2015). Tuning of Ranvier node and internode properties in myelinated axons to adjust action potential timing. Nat. Commun. 6:8073. doi: 10.1038/ncomms9073
Fu, Y., Huff, T. B., Wang, H.-W., Wang, H., and Cheng, J.-X. (2008). Ex vivo and in vivo imaging of myelin fibers in mouse brain by coherent anti-Stokes Raman scattering microscopy. Opt. Express 16, 19396–19409. doi: 10.1364/oe.16.019396
Fuchs, E. C., Zivkovic, A. R., Cunningham, M. O., Middleton, S., Lebeau, F. E., Bannerman, D. M., et al. (2007). Recruitment of parvalbumin-positive interneurons determines hippocampal function and associated behavior. Neuron 53, 591–604. doi: 10.1016/j.neuron.2007.01.031
Ge, W.-P., Yang, X.-J., Zhang, Z., Wang, H.-K., Shen, W., Deng, Q.-D., et al. (2006). Long-term potentiation of neuron-glia synapses mediated by Ca2+-permeable AMPA receptors. Science 312, 1533–1537. doi: 10.1126/science.1124669
Ghosh, K. K., Burns, L. D., Cocker, E. D., Nimmerjahn, A., Ziv, Y., Gamal, A. E., et al. (2011). Miniaturized integration of a fluorescence microscope. Nat. Methods 8, 871–878. doi: 10.1038/nmeth.1694
Gibson, E. M., Purger, D., Mount, C. W., Goldstein, A. K., Lin, G. L., Wood, L. S., et al. (2014). Neuronal activity promotes oligodendrogenesis and adaptive myelination in the mammalian brain. Science 344:1252304. doi: 10.1126/science.1252304
Guo, F., Maeda, Y., Ko, E. M., Delgado, M., Horiuchi, M., Soulika, A., et al. (2012). Disruption of NMDA receptors in oligodendroglial lineage cells does not alter their susceptibility to experimental autoimmune encephalomyelitis or their normal development. J. Neurosci. 32, 639–645. doi: 10.1523/JNEUROSCI.4073-11.2012
Haberlandt, C., Derouiche, A., Wyczynski, A., Haseleu, J., Pohle, J., Karram, K., et al. (2011). Gray matter NG2 cells display multiple Ca2+-signaling pathways and highly motile processes. PLoS One 6:e17575. doi: 10.1371/journal.pone.0017575
Hill, R. A., Patel, K. D., Goncalves, C. M., Grutzendler, J., and Nishiyama, A. (2014). Modulation of oligodendrocyte generation during a critical temporal window after NG2 cell division. Nat. Neurosci. 17, 1518–1527. doi: 10.1038/nn.3815
Hines, J. H., Ravanelli, A. M., Schwindt, R., Scott, E. K., and Appel, B. (2015). Neuronal activity biases axon selection for myelination in vivo. Nat. Neurosci. 18, 683–689. doi: 10.1038/nn.3992
Hontani, Y., Xia, F., and Xu, C. (2021). Multicolor three-photon fluorescence imaging with single-wavelength excitation deep in mouse brain. Sci. Adv. 7:eabf3531. doi: 10.1126/sciadv.abf3531
Horton, N. G., Wang, K., Kobat, D., Clark, C. G., Wise, F. W., Schaffer, C. B., et al. (2013). in vivo three-photon microscopy of subcortical structures within an intact mouse brain. Nat. Photonics 7, 205–209. doi: 10.1038/nphoton.2012.336
Huang, W., Guo, Q., Bai, X., Scheller, A., and Kirchhoff, F. (2019). Early embryonic NG2 glia are exclusively gliogenic and do not generate neurons in the brain. Glia 67, 1094–1103. doi: 10.1002/glia.23590
Hughes, A. N., and Appel, B. (2019). Oligodendrocytes express synaptic proteins that modulate myelin sheath formation. Nat. Commun. 10:4125. doi: 10.1038/s41467-019-12059-y
Hughes, A. N., and Appel, B. (2020). Microglia phagocytose myelin sheaths to modify developmental myelination. Nat. Neurosci. 23, 1055–1066. doi: 10.1038/s41593-020-0654-2
Hughes, E. G., Kang, S. H., Fukaya, M., and Bergles, D. E. (2013). Oligodendrocyte progenitors balance growth with self-repulsion to achieve homeostasis in the adult brain. Nat. Neurosci. 16, 668–676. doi: 10.1038/nn.3390
Hughes, E. G., Orthmann-Murphy, J. L., Langseth, A. J., and Bergles, D. E. (2018). Myelin remodeling through experience-dependent oligodendrogenesis in the adult somatosensory cortex. Nat. Neurosci. 21, 696–706. doi: 10.1038/s41593-018-0121-5
Jabs, R., Pivneva, T., Hüttmann, K., Wyczynski, A., Nolte, C., Kettenmann, H., et al. (2005). Synaptic transmission onto hippocampal glial cells with hGFAP promoter activity. J. Cell Sci. 118, 3791–3803. doi: 10.1242/jcs.02515
Jung, J. C., Mehta, A. D., Aksay, E., Stepnoski, R., and Schnitzer, M. J. (2004). in vivo mammalian brain imaging using one- and two-photon fluorescence microendoscopy. J. Neurophysiol. 92, 3121–3133. doi: 10.1152/jn.00234.2004
Kang, S. H., Fukaya, M., Yang, J. K., Rothstein, J. D., and Bergles, D. E. (2010). NG2+ CNS glial progenitors remain committed to the oligodendrocyte lineage in postnatal life and following neurodegeneration. Neuron 68, 668–681. doi: 10.1016/j.neuron.2010.09.009
Káradóttir, R., Cavelier, P., Bergersen, L. H., and Attwell, D. (2005). NMDA receptors are expressed in oligodendrocytes and activated in ischaemia. Nature 438, 1162–1166. doi: 10.1038/nature04302
Káradóttir, R., Hamilton, N. B., Bakiri, Y., and Attwell, D. (2008). Spiking and nonspiking classes of oligodendrocyte precursor glia in CNS white matter. Nat. Neurosci. 11, 450–456. doi: 10.1038/nn2060
Karram, K., Goebbels, S., Schwab, M., Jennissen, K., Seifert, G., Steinhauser, C., et al. (2008). NG2-expressing cells in the nervous system revealed by the NG2-EYFP-knockin mouse. Genesis 46, 743–757. doi: 10.1002/dvg.20440
Khawaja, R. R., Agarwal, A., Fukaya, M., Jeong, H.-K., Gross, S., Gonzalez-Fernandez, E., et al. (2021). GluA2 overexpression in oligodendrocyte progenitors promotes postinjury oligodendrocyte regeneration. Cell Rep. 35:109147. doi: 10.1016/j.celrep.2021.109147
Koudelka, S., Voas, M. G., Almeida, R. G., Baraban, M., Soetaert, J., Meyer, M. P., et al. (2016). Individual neuronal subtypes exhibit diversity in CNS myelination mediated by synaptic vesicle release. Curr. Biol. 26, 1447–1455. doi: 10.1016/j.cub.2016.03.070
Kougioumtzidou, E., Shimizu, T., Hamilton, N. B., Tohyama, K., Sprengel, R., Monyer, H., et al. (2017). Signalling through AMPA receptors on oligodendrocyte precursors promotes myelination by enhancing oligodendrocyte survival. eLife 6:e28080. doi: 10.7554/eLife.28080
Krasnow, A. M., Ford, M. C., Valdivia, L. E., Wilson, S. W., and Attwell, D. (2018). Regulation of developing myelin sheath elongation by oligodendrocyte calcium transients in vivo. Nat. Neurosci. 21, 24–28. doi: 10.1038/s41593-017-0031-y
Kressin, K., Kuprijanova, E., Jabs, R., Seifert, G., and Steinhauser, C. (1995). Developmental regulation of Na+ and K+ conductances in glial cells of mouse hippocampal brain slices. Glia 15, 173–187. doi: 10.1002/glia.440150210
Kukley, M., Capetillo-Zarate, E., and Dietrich, D. (2007). Vesicular glutamate release from axons in white matter. Nat. Neurosci. 10, 311–320. doi: 10.1038/nn1850
Kukley, M., Kiladze, M., Tognatta, R., Hans, M., Swandulla, D., Schramm, J., et al. (2008). Glial cells are born with synapses. FASEB J. 22, 2957–2969. doi: 10.1096/fj.07-090985
Kukley, M., Nishiyama, A., and Dietrich, D. (2010). The fate of synaptic input to NG2 glial cells: neurons specifically downregulate transmitter release onto differentiating oligodendroglial cells. J. Neurosci. 30, 8320–8331. doi: 10.1523/JNEUROSCI.0854-10.2010
Kwon, H.-B., and Sabatini, B. L. (2011). Glutamate induces de novo growth of functional spines in developing cortex. Nature 474, 100–104. doi: 10.1038/nature09986
Lappe-Siefke, C., Goebbels, S., Gravel, M., Nicksch, E., Lee, J., Braun, P. E., et al. (2003). Disruption of Cnp1 uncouples oligodendroglial functions in axonal support and myelination. Nat. Genet. 33, 366–374. doi: 10.1038/ng1095
Lawlor, P. A., Bland, R. J., Mouravlev, A., Young, D., and During, M. J. (2009). Efficient gene delivery and selective transduction of glial cells in the mammalian brain by AAV serotypes isolated from nonhuman primates. Mol. Ther. 17, 1692–1702. doi: 10.1038/mt.2009.170
Lee, S., Leach, M. K., Redmond, S. A., Chong, S. Y., Mellon, S. H., Tuck, S. J., et al. (2012). A culture system to study oligodendrocyte myelination processes using engineered nanofibers. Nat. Methods 9, 917–922. doi: 10.1038/nmeth.2105
Li, H., and Richardson, W. D. (2016). Evolution of the CNS myelin gene regulatory program. Brain Res. 1641, 111–121. doi: 10.1016/j.brainres.2015.10.013
Lin, S.-C., and Bergles, D. E. (2004). Synaptic signaling between GABAergic interneurons and oligodendrocyte precursor cells in the hippocampus. Nat. Neurosci. 7, 24–32. doi: 10.1038/nn1162
Lin, S.-C., Huck, J. H., Roberts, J. D., Macklin, W. B., Somogyi, P., and Bergles, D. E. (2005). Climbing fiber innervation of NG2-expressing glia in the mammalian cerebellum. Neuron 46, 773–785. doi: 10.1016/j.neuron.2005.04.025
Liu, J., Dietz, K., Deloyht, J. M., Pedre, X., Kelkar, D., Kaur, J., et al. (2012). Impaired adult myelination in the prefrontal cortex of socially isolated mice. Nat. Neurosci. 15, 1621–1623. doi: 10.1038/nn.3263
Lundgaard, I., Luzhynskaya, A., Stockley, J. H., Wang, Z., Evans, K. A., Swire, M., et al. (2013). Neuregulin and BDNF induce a switch to NMDA receptor-dependent myelination by oligodendrocytes. PLoS Biol. 11:e1001743. doi: 10.1371/journal.pbio.1001743
Makinodan, M., Rosen, K. M., Ito, S., and Corfas, G. (2012). A critical period for social experience-dependent oligodendrocyte maturation and myelination. Science 337, 1357–1360. doi: 10.1126/science.1220845
Mallon, B. S., Shick, H. E., Kidd, G. J., and Macklin, W. B. (2002). Proteolipid promoter activity distinguishes two populations of NG2-positive cells throughout neonatal cortical development. J. Neurosci. 22, 876–885. doi: 10.1523/JNEUROSCI.22-03-00876.2002
Mangin, J.-M., Kunze, A., Chittajallu, R., and Gallo, V. (2008). Satellite NG2 progenitor cells share common glutamatergic inputs with associated interneurons in the mouse dentate gyrus. J. Neurosci. 28, 7610–7623. doi: 10.1523/JNEUROSCI.1355-08.2008
Mangin, J. M., Li, P., Scafidi, J., and Gallo, V. (2012). Experience-dependent regulation of NG2 progenitors in the developing barrel cortex. Nat. Neurosci. 15, 1192–1194. doi: 10.1038/nn.3190
Marisca, R., Hoche, T., Agirre, E., Hoodless, L. J., Barkey, W., Auer, F., et al. (2020). Functionally distinct subgroups of oligodendrocyte precursor cells integrate neural activity and execute myelin formation. Nat. Neurosci. 23, 363–374. doi: 10.1038/s41593-019-0581-2
Matsuzaki, M., Ellis-Davies, G. C., Nemoto, T., Miyashita, Y., Iino, M., and Kasai, H. (2001). Dendritic spine geometry is critical for AMPA receptor expression in hippocampal CA1 pyramidal neurons. Nat. Neurosci. 4, 1086–1092. doi: 10.1038/nn736
Matsuzaki, M., Honkura, N., Ellis-Davies, G. C., and Kasai, H. (2004). Structural basis of long-term potentiation in single dendritic spines. Nature 429, 761–766. doi: 10.1038/nature02617
Mayoral, S. R., and Chan, J. R. (2016). The environment rules: spatiotemporal regulation of oligodendrocyte differentiation. Curr. Opin. Neurobiol. 39, 47–52. doi: 10.1016/j.conb.2016.04.002
McKenzie, I. A., Ohayon, D., Li, H., De Faria, J. P., Emery, B., Tohyama, K., et al. (2014). Motor skill learning requires active central myelination. Science 346, 318–322. doi: 10.1126/science.1254960
Mensch, S., Baraban, M., Almeida, R., Czopka, T., Ausborn, J., El Manira, A., et al. (2015). Synaptic vesicle release regulates myelin sheath number of individual oligodendrocytes in vivo. Nat. Neurosci. 18, 628–630. doi: 10.1038/nn.3991
Micu, I., Jiang, Q., Coderre, E., Ridsdale, A., Zhang, L., Woulfe, J., et al. (2006). NMDA receptors mediate calcium accumulation in myelin during chemical ischaemia. Nature 439, 988–992. doi: 10.1038/nature04474
Micu, I., Ridsdale, A., Zhang, L., Woulfe, J., Mcclintock, J., Brantner, C. A., et al. (2007). Real-time measurement of free Ca2+ changes in CNS myelin by two-photon microscopy. Nat. Med. 13, 874–879. doi: 10.1038/nm1568
Mitew, S., Gobius, I., Fenlon, L. R., Mcdougall, S. J., Hawkes, D., Xing, Y. L., et al. (2018). Pharmacogenetic stimulation of neuronal activity increases myelination in an axon-specific manner. Nat. Commun. 9:306. doi: 10.1038/s41467-017-02719-2
Nishiyama, A., Komitova, M., Suzuki, R., and Zhu, X. (2009). Polydendrocytes (NG2 cells): multifunctional cells with lineage plasticity. Nat. Rev. Neurosci. 10, 9–22. doi: 10.1038/nrn2495
Orduz, D., Maldonado, P. P., Balia, M., Velez-Fort, M., De Sars, V., Yanagawa, Y., et al. (2015). Interneurons and oligodendrocyte progenitors form a structured synaptic network in the developing neocortex. eLife 4:e06953. doi: 10.7554/eLife.06953
Pan, S., Mayoral, S. R., Choi, H. S., Chan, J. R., and Kheirbek, M. A. (2020). Preservation of a remote fear memory requires new myelin formation. Nat. Neurosci. 23, 487–499. doi: 10.1038/s41593-019-0582-1
Passlick, S., Grauer, M., Schafer, C., Jabs, R., Seifert, G., and Steinhauser, C. (2013). Expression of the gamma2-subunit distinguishes synaptic and extrasynaptic GABAA receptors in NG2 cells of the hippocampus. J. Neurosci. 33, 12030–12040. doi: 10.1523/JNEUROSCI.5562-12.2013
Powell, S. K., Khan, N., Parker, C. L., Samulski, R. J., Matsushima, G., Gray, S. J., et al. (2016). Characterization of a novel adeno-associated viral vector with preferential oligodendrocyte tropism. Gene Ther. 23, 807–814. doi: 10.1038/gt.2016.62
Rivers, L. E., Young, K. M., Rizzi, M., Jamen, F., Psachoulia, K., Wade, A., et al. (2008). PDGFRA/NG2 glia generate myelinating oligodendrocytes and piriform projection neurons in adult mice. Nat. Neurosci. 11, 1392–1401. doi: 10.1038/nn.2220
Saab, A. S., Tzvetavona, I. D., Trevisiol, A., Baltan, S., Dibaj, P., Kusch, K., et al. (2016). Oligodendroglial NMDA receptors regulate glucose import and axonal energy metabolism. Neuron 91, 119–132. doi: 10.1016/j.neuron.2016.05.016
Salter, M. G., and Fern, R. (2005). NMDA receptors are expressed in developing oligodendrocyte processes and mediate injury. Nature 438, 1167–1171. doi: 10.1038/nature04301
Schain, A. J., Hill, R. A., and Grutzendler, J. (2014). Label-free in vivo imaging of myelinated axons in health and disease with spectral confocal reflectance microscopy. Nat. Med. 20, 443–449. doi: 10.1038/nm.3495
Silva, A. J. (2017). Miniaturized two-photon microscope: seeing clearer and deeper into the brain. Light Sci. Appl. 6:e17104. doi: 10.1038/lsa.2017.104
Steadman, P. E., Xia, F., Ahmed, M., Mocle, A. J., Penning, A. R. A., Geraghty, A. C., et al. (2020). Disruption of oligodendrogenesis impairs memory consolidation in adult mice. Neuron 105, 150–164.e6. doi: 10.1016/j.neuron.2019.10.013
Steinhäuser, C., Kressin, K., Kuprijanova, E., Weber, M., and Seifert, G. (1994). Properties of voltage-activated Na+ and K+ currents in mouse hippocampal glial cells in situ and after acute isolation from tissue slices. Pflugers Arch. 428, 610–620. doi: 10.1007/BF00374585
Sun, W., and Dietrich, D. (2013). Synaptic integration by NG2 cells. Front. Cell. Neurosci. 7:255. doi: 10.3389/fncel.2013.00255
Sun, W., Matthews, E. A., Nicolas, V., Schoch, S., and Dietrich, D. (2016). NG2 glial cells integrate synaptic input in global and dendritic calcium signals. eLife 5:e16262. doi: 10.7554/eLife.16262
Tedeschi, A., Dupraz, S., Laskowski, C. J., Xue, J., Ulas, T., Beyer, M., et al. (2016). The calcium channel subunit alpha2delta2 suppresses axon regeneration in the adult CNS. Neuron 92, 419–434. doi: 10.1016/j.neuron.2016.09.026
Tognatta, R., Sun, W., Goebbels, S., Nave, K. A., Nishiyama, A., Schoch, S., et al. (2017). Transient Cnp expression by early progenitors causes Cre-Lox-based reporter lines to map profoundly different fates. Glia 65, 342–359. doi: 10.1002/glia.23095
Tong, X.-P., Li, X.-Y., Zhou, B., Shen, W., Zhang, Z.-J., Xu, T.-L., et al. (2009). Ca2+ signaling evoked by activation of Na+ channels and Na+/Ca2+ exchangers is required for GABA-induced NG2 cell migration. J. Cell Biol. 186, 113–128. doi: 10.1083/jcb.200811071
Van Praag, H., Schinder, A. F., Christie, B. R., Toni, N., Palmer, T. D., and Gage, F. H. (2002). Functional neurogenesis in the adult hippocampus. Nature 415, 1030–1034. doi: 10.1038/4151030a
Vélez-Fort, M., Maldonado, P. P., Butt, A. M., Audinat, E., and Angulo, M. C. (2010). Postnatal switch from synaptic to extrasynaptic transmission between interneurons and NG2 cells. J. Neurosci. 30, 6921–6929. doi: 10.1523/JNEUROSCI.0238-10.2010
Von Jonquieres, G., Fröhlich, D., Klugmann, C. B., Wen, X., Harasta, A. E., Ramkumar, R., et al. (2016). Recombinant human myelin-associated glycoprotein promoter drives selective AAV-mediated transgene expression in oligodendrocytes. Front. Mol. Neurosci. 9:13. doi: 10.3389/fnmol.2016.00013
Wake, H., Lee, P. R., and Fields, R. D. (2011). Control of local protein synthesis and initial events in myelination by action potentials. Science 333, 1647–1651. doi: 10.1126/science.1206998
Wang, F., Flanagan, J., Su, N., Wang, L.-C., Bui, S., Nielson, A., et al. (2012). RNAscope: a novel in situ RNA analysis platform for formalin-fixed, paraffin-embedded tissues. J. Mol. Diagn. 14, 22–29. doi: 10.1016/j.jmoldx.2011.08.002
Wang, H., Fu, Y., Zickmund, P., Shi, R., and Cheng, J.-X. (2005). Coherent anti-stokes Raman scattering imaging of axonal myelin in live spinal tissues. Biophys. J. 89, 581–591. doi: 10.1529/biophysj.105.061911
Wang, K., Sun, W., Richie, C. T., Harvey, B. K., Betzig, E., and Ji, N. (2015). Direct wavefront sensing for high-resolution in vivo imaging in scattering tissue. Nat. Commun. 6:7276. doi: 10.1038/ncomms8276
Weinberg, M. S., Criswell, H. E., Powell, S. K., Bhatt, A. P., and Mccown, T. J. (2017). Viral vector reprogramming of adult resident striatal oligodendrocytes into functional neurons. Mol. Ther. 25, 928–934. doi: 10.1016/j.ymthe.2017.01.016
Yuan, X., Chittajallu, R., Belachew, S., Anderson, S., Mcbain, C. J., and Gallo, V. (2002). Expression of the green fluorescent protein in the oligodendrocyte lineage: a transgenic mouse for developmental and physiological studies. J. Neurosci. Res. 70, 529–545. doi: 10.1002/jnr.10368
Zhu, X., Bergles, D. E., and Nishiyama, A. (2008). NG2 cells generate both oligodendrocytes and gray matter astrocytes. Development 135, 145–157. doi: 10.1242/dev.004895
Zhu, X., Hill, R. A., Dietrich, D., Komitova, M., Suzuki, R., and Nishiyama, A. (2011). Age-dependent fate and lineage restriction of single NG2 cells. Development 138, 745–753. doi: 10.1242/dev.047951
Ziskin, J. L., Nishiyama, A., Rubio, M., Fukaya, M., and Bergles, D. E. (2007). Vesicular release of glutamate from unmyelinated axons in white matter. Nat. Neurosci. 10, 321–330. doi: 10.1038/nn1854
Keywords: activity-dependent myelination, local stimulation, AMPA receptor, glutamate uncaging, time-lapse imaging
Citation: Heflin JK and Sun W (2021) Novel Toolboxes for the Investigation of Activity-Dependent Myelination in the Central Nervous System. Front. Cell. Neurosci. 15:769809. doi: 10.3389/fncel.2021.769809
Received: 02 September 2021; Accepted: 06 October 2021;
Published: 02 November 2021.
Edited by:
Francesca Boscia, University of Naples Federico II, ItalyReviewed by:
Maria Kukley, Achucarro Basque Center for Neuroscience, SpainRafael Góis Almeida, University of Edinburgh, United Kingdom
Ben Emery, Oregon Health and Science University, United States
Copyright © 2021 Heflin and Sun. This is an open-access article distributed under the terms of the Creative Commons Attribution License (CC BY). The use, distribution or reproduction in other forums is permitted, provided the original author(s) and the copyright owner(s) are credited and that the original publication in this journal is cited, in accordance with accepted academic practice. No use, distribution or reproduction is permitted which does not comply with these terms.
*Correspondence: Wenjing Sun, V2VuamluZy5TdW5Ab3N1bWMuZWR1