- 1Department of Biochemistry and Physiology, Faculty of Pharmacy and Food Science, University of Barcelona, Barcelona, Spain
- 2Centro de Investigación Biomédica en Red Enfermedades Neurodegenerativas (CiberNed), National Institute of Health Carlos III, Madrid, Spain
- 3Department of Biochemistry and Molecular Biomedicine, Universitat de Barcelona, Barcelona, Spain
- 4Basic Sciences Department, Faculty of Medicine and Health Sciences, Universitat Internacional de Catalunya, Sant Cugat del Vallès, Spain
- 5University of Bordeaux, INSERM, Neurocentre Magendie, Bordeaux, France
- 6School of Chemistry, University of Barcelona, Barcelona, Spain
- 7Institut de Neurociències, Universitat de Barcelona (UBNeuro), Barcelona, Spain
There is evidence of ghrelinergic-cannabinoidergic interactions in the central nervous system (CNS) that may impact on the plasticity of reward circuits. The aim of this article was to look for molecular and/or functional interactions between cannabinoid CB1 and ghrelin GHS-R1a receptors. In a heterologous system and using the bioluminescence resonance energy transfer technique we show that human versions of cannabinoid CB1 and ghrelin GHS-R1a receptors may form macromolecular complexes. Such receptor heteromers have particular properties in terms of CB1/Gi-mediated signaling and in terms of GHS-R1a-Gq-mediated signaling. On the one hand, just co-expression of CB1R and GHS-R1a led to impairment of cannabinoid signaling. On the other hand, cannabinoids led to an increase in ghrelin-derived calcium mobilization that was stronger at low concentrations of the CB1 receptor agonist, arachidonyl-2’-chloroethylamide (ACEA). The expression of CB1-GHS-R1a receptor complexes in striatal neurons was confirmed by in situ proximity ligation imaging assays. Upregulation of CB1-GHS-R1a- receptor complexes was found in striatal neurons from siblings of pregnant female mice on a high-fat diet. Surprisingly, the expression was upregulated after treatment of neurons with ghrelin (200 nM) or with ACEA (100 nM). These results help to better understand the complexities underlying the functional interactions of neuromodulators in the reward areas of the brain.
Introduction
Cell surface cannabinoid receptors were identified as targets of natural compounds present in Cannabis sativa, specially of Δ9-tetrahydrocannabinol (Δ9-THC; (6aR, 10aR)-6,6,9-trimethyl-3-pentyl-6a,7,8,10a-tetrahydro-6H-benzo[c]chromen-1-ol; CAS registry number: #1972-08-3). So far, two cannabinoid receptors have been cloned and pharmacologically characterized, the CB1 and the CB2 receptors. They belong to class A rhodopsin-like G-protein coupled receptors (GPCRs) and both have Gi as the canonical heterotrimeric G protein to which they couple (Alexander et al., 2021). Subsequent to the discovery of cannabinoid receptors, the main compounds that act as endogenous agonists were identified, 2-arachidonoylglycerol (2-AG) and anandamide (N-arachidonoylethanolamine). Further components of the endocannabinoid system are the enzymes that synthesize and degrade 2-AG and anandamide (Lu and Mackie, 2016). Cannabis smoking leads to psychotropic events that are due to Δ9-THC acting on the CB1 receptor (CB1R), which is reportedly the most abundant GPCR in the central nervous system, being expressed in almost any region of the brain and both in neurons and glia (Elphick and Egertová, 2001; Mackie, 2005). In addition, it is well established that cannabis use has orexigenic properties (Pagotto et al., 2006).
Ghrelin has been considered as the “hunger hormone” (Funahashi et al., 2003; Abizaid and Horvath, 2008; Schellekens et al., 2010; Cassidy and Tong, 2017). Ghrelin, a 28-amino acid peptide produced by specialized cells of the gastrointestinal tract, activates central mechanisms that control food intake (Funahashi et al., 2003; Abizaid and Horvath, 2008; Schellekens et al., 2010; Cassidy and Tong, 2017). However, in mammals, there are overlapping mechanisms, both central and peripheral, that control food intake. Ghrelin acts via a specific receptor, GHS-R1a, that belongs to the family of GPCRs, couples to Gq heterotrimeric G protein and is expressed in a variety of cells and tissues (Pradhan et al., 2013; Alexander et al., 2019). In previous studies, we have reported physiologically relevant interactions in which the GHS-R1a receptor is involved. A functional unit composed of GHS-R1a and the dopamine D1 receptor mediates, at least in part, the hunger-suppressing actions of cocaine; in a macromolecular complex that also includes the non-GPCR sigma-1 receptor, a dual coupling is possible; that is, the coupling to two G proteins makes it possible for ghrelin to act through cAMP rather than through Ca2+ and dopamine to signal via increases in cytoplasmic Ca2+ rather than through cAMP (Casanovas et al., 2021). The ghrelin receptor is also able to interact with the CB2 cannabinoid receptor in both heterologous cells and in cells of the central nervous system. Cannabinoids acting on the CB2 receptor do not alter the cytosolic Ca2+ increases triggered by ghrelin. However, ghrelin receptor activation led to a blockade of CB2 receptor-mediated Gi-dependent signaling (Lillo et al., 2021). The aim of this article was to investigate the potential molecular and/or functional interactions between CB1 and GHS-R1a receptors. As the risk of obesity is higher in the progeny of obese parents, the interaction between these two receptors was also studied in neurons isolated from fetuses of mothers on a high-fat diet (Abu-Rmeileh et al., 2008).
Materials and Methods
Reagents
ACEA, ghrelin (human), rimonabant hydrochloride, and YIL 781 hydrochloride were purchased from Tocris Bioscience (Bristol, United Kingdom). Concentrated (10 mM) stock solutions prepared in DMSO, milli-Q® H2O (Merck/Millipore, Darmstadt, Germany), or ethanol were stored at −20°C. In each experimental session, aliquots of concentrated solutions of compounds were thawed and conveniently diluted in the appropriate experimental solution.
Diet-Induced Obesity Model
C57BL/6J female mice were used for the experiments. All animals were subjected to a 12 h/12 h light/ dark cycle in a temperature- and humidity-controlled room and were allowed free access to water and standard laboratory chow. C57BL/6J mice were randomly assigned to a high-fat diet (HFD; 60% kcal from fat; catalog no. D12492, Research Diets, New Brunswick, NJ, USA) or standard diet (STD; 10% kcal from fat; catalog no. D12450B, Research Diets) for 60 days. Primary striatal neurons were obtained from fetuses of mothers on STD or HFD diets. Pregnant animals were killed by cervical dislocation during the light phase. All animal procedures were performed in agreement with European guidelines (2010/63/EU) and approved by the University of Barcelona Ethical Committee, which reports to the regional Government (Protocol #9659; Generalitat de Catalunya, May 24, 2019).
Cell Culture and Transient Transfection
Human embryonic kidney HEK-293T (lot 612968) cells were acquired from the American Type Culture Collection (ATCC). They were amplified and frozen in liquid nitrogen in several aliquots. Cells from each aliquot were used until passage 19. HEK-293T cells were grown in Dulbecco’s modified Eagle’s medium (DMEM; Gibco, Paisley, Scotland, United Kingdom) supplemented with 2 mM L-glutamine, 100 U/ml penicillin/streptomycin, MEM Non-Essential Amino Acid Solution (1/100), and 5% (v/v) heat-inactivated Fetal Bovine Serum (FBS; all supplements were from Invitrogen, Paisley, Scotland, United Kingdom). Cells were maintained in a humid atmosphere of 5% CO2 at 37°C.
Cells were transiently transfected with the corresponding cDNAs using the PEI (PolyEthylenImine, Sigma-Aldrich, St. Louis, MO, USA) method as previously described (Carriba et al., 2008; Hradsky et al., 2011; Navarro et al., 2012). Four hours after transfection, growth medium was replaced by a complete medium. Experiments were carried out 48 h later.
To prepare primary striatal neurons, brains from fetuses of pregnant mice were removed (gestational age: 17 days). Neurons were isolated as described in Hradsky et al. (2013) and plated at a confluence of 40,000 cells/0.32 cm2. Briefly, the samples were dissected and, after careful removal of the meninges, digested for 20 min at 37°C with 0.25% trypsin. Trypsinization was stopped by adding an equal volume of culture medium (Dulbecco’s modified Eagle medium-F-12 nutrient mixture, Invitrogen). Cells were brought to a single cell suspension by repeated pipetting followed by passage through a 100 μm-pore mesh. Pelleted (7 min, 200× g) cells were resuspended in supplemented DMEM and seeded at a density of 3.5 × 105 cells/ml. The next day, the medium was replaced by neurobasal medium supplemented with 2 mM L-glutamine, 100 U/ml penicillin/streptomycin, and 2% (v/v) B27 medium (Gibco). Neuronal cultures were used for assays after 15 days of culture. Using NeuN as a marker, the percentage of neurons in the cultures was >90%.
Expression Vectors
The human cDNAs for the CB1R, GHS-R1a, and D1R cloned in pcDNA3.1 were amplified without their stop codons using sense and antisense primers. The primers harbored either unique BamHI and KpnI sites for CB1R and HindIII and BamHI sites for GHS-R1a and D1R. The fragments were subcloned to be in frame with an enhanced yellow fluorescent protein (pEYFP-N1; Clontech, Heidelberg, Germany) and the Renilla luciferase protein (Rluc; pRluc-N1; PerkinElmer, Wellesley, MA) on the C-terminal end of the receptor to produce CB1R-YFP, D1R-Rluc, and GHS-R1a-Rluc.
Immunofluorescence
HEK-293T cells transfected with cDNAs for CB1R-YFP and GHS-R1a-Rluc were fixed in 4% paraformaldehyde for 15 min and then washed twice with PBS containing 20 mM glycine before permeabilization with the same buffer containing 0.2% Triton X-100 (5 min incubation). The samples were treated for 1 h with blocking solution (PBS containing 1% bovine serum albumin) and labeled with a mouse anti-Rluc (1/100; MAB4400, Millipore, Burlington, MA, USA) as primary antibody and subsequently treated with Cy3-conjugated anti-mouse IgG (1/200; 715-166-150; Jackson ImmunoResearch) as the secondary antibody (1 h each). The samples were washed several times and mounted with 30% Mowiol (Calbiochem, San Diego, USA). Nuclei were stained with Hoechst (1/100). Samples were observed under a Zeiss 880 confocal microscope (Carl Zeiss, Oberkochen, Germany).
Bioluminescence Resonance Energy Transfer (BRET) Assay
HEK-293T cells growing in 6-well plates were transiently cotransfected with a constant amount of cDNA encoding for GHS-R1a fused to Renilla luciferase (GHS-R1a-Rluc) and with increasing amounts of cDNA corresponding to CB1 receptor fused to the yellow fluorescent protein (CB1R-YFP). For negative control, cells were cotransfected with a constant amount of cDNA encoding for D1R-Rluc and with increasing amounts of cDNA for CB1R-YFP. Forty-eight hours post-transfection cells were washed twice in quick succession with HBSS (137 mM NaCl; 5 mM KCl; 0.34 mM Na2HPO4; 0.44 mM KH2PO4; 1.26 mM CaCl2; 0.4 mM MgSO4; 0.5 mM MgCl2; and 10 mM HEPES, pH 7.4) supplemented with 0.1% glucose (w/v), detached by gently pipetting and resuspended in the same buffer. To have an estimation of the number of cells per plate, protein concentration was determined using a Bradford assay kit (Bio-Rad, Munich, Germany) with bovine serum albumin dilutions for standardization. To quantify YFP-fluorescence expression, cells were distributed (20 μg protein) in 96-well microplates (black plates with a transparent bottom; Porvair, Leatherhead, UK). Fluorescence was read using a Mithras LB 940 (Berthold, Bad Wildbad, Germany) equipped with a high-energy xenon flash lamp, using a 10-nm bandwidth excitation and emission filters at 485 and 530 nm, respectively. YFP-fluorescence expression was determined as the fluorescence of the sample minus the fluorescence of cells expressing only protein-Rluc. For the BRET measurements, the equivalent of 20 μg of cell suspension was distributed in 96-well microplates (white plates; Porvair), and 5 μM coelenterazine H was added (PJK GMBH, Kleinblittersdorf, Germany). Then, 1 min after coelenterazine H addition, the readings were collected using a Mithras LB 940 (Berthold, Bad Wildbad, Germany), which allowed the integration of the signals detected in the short-wavelength filter at 485 nm (440–500 nm) and the long-wavelength filter at 530 nm (510–590 nm). To quantify receptor-Rluc expression, luminescence readings were collected 10 min after 5 μM coelenterazine H addition. The net BRET is defined as [(long-wavelength emission)/(short-wavelength emission)]-Cf where Cf corresponds to [(long-wavelength emission)/(short-wavelength emission)] for the Rluc construct expressed alone in the same experiment. The BRET curves were fitted assuming a single phase by a non-linear regression equation using the GraphPad Prism software (San Diego, CA, USA). BRET values are given as milli-BRET units (mBU: 1000 × net BRET).
cAMP Determination
HEK-293T cells transfected with the cDNAs for CB1R (1 μg) and/or GHS-R1a (1.5 μg) and neuronal primary cultures were plated in 6-well plates. Two hours before initiating the experiment, the cell-culture medium was replaced by the non-supplemented DMEM medium. Then, cells were detached, resuspended in the non-supplemented DMEM medium containing 50 μM zardaverine, and plated in 384-well microplates (2,500 cells/well). Cells were pretreated (15 min) with the corresponding antagonists (1 μM rimonabant for CB1R and 1 μM YIL 781 for GHS-R1a) or vehicle and stimulated with agonists (1 nM, 10 nM, 100 nM, and 1 μM ACEA for CB1R or 200 nM ghrelin for GHS-R1a; 15 min) before the addition of 0.5 μM FK or vehicle. Finally, the reaction was stopped by the addition of the Eu-cAMP tracer and the ULight-conjugated anti-cAMP monoclonal antibody prepared in the “cAMP detection buffer” (PerkinElmer). All steps were performed at 25°. Homogeneous time-resolved fluorescence energy transfer (HTRF) measures were performed after 60 min incubation at RT using the Lance Ultra cAMP kit (PerkinElmer, Waltham, MA, USA). Fluorescence at 665 nm was analyzed on a PHERAstar Flagship microplate reader equipped with an HTRF optical module (BMG Lab Technologies, Offenburg, Germany).
MAPK Phosphorylation Assays
To determine extracellular signal-regulated kinase 1/2 (ERK1/2) phosphorylation, HEK-293T transfected cells and primary striatal neurons were plated (50,000 cells/well) in transparent Deltalab 96-well plates and kept in the incubator for 15 days. Two hours before the experiment, the medium was replaced by non-supplemented DMEM medium. Next, the cells were pre-treated at RT for 10 min with antagonists (1 μM rimonabant for CB1R and YIL 781 for GHS-R1a) or vehicle and stimulated for an additional 7 min with selective agonists (1 nM, 10 nM, 100 nM, 1 μM ACEA for CB1R and 200 nM ghrelin for GHS-R1a). Then, cells were washed twice with cold PBS before the addition of 30 μl/well “Ultra lysis buffer” -PerkinElmer- (15 min treatment). Afterward, 10 μl of each supernatant was placed in white ProxiPlate 384-well plates and ERK1/2 phosphorylation was determined using an AlphaScreen®SureFire® kit (PerkinElmer), following the instructions of the supplier, and using an EnSpire® Multimode Plate Reader (PerkinElmer, Waltham, MA, USA). The reference value (100%) was the value achieved in the absence of any treatment (basal). Agonist effects were given in percentage with respect to the basal value.
Real-Time Determination of Calcium Ion Cytoplasmic Level Variation
HEK-293T cells were transfected with the cDNAs for CB1R (1 μg) and/or GHS-R1a (1.5 μg) in the presence of 1 μg cDNA for the calmodulin-based calcium GCaMP6 sensor (Chen et al., 2013) using the PEI method. 48 h after transfection, cells were detached using Mg+2-free Locke’s buffer (pH 7.4; 154 mM NaCl, 5.6 mM KCl, 3.6 mM NaHCO3, 2.3 mM CaCl2, 5.6 mM glucose and 5 mM HEPES) supplemented with 10 μM glycine. 1,500 cells per well were plated in 96-well black, clear-bottom, microtiter plates. Then, cells were incubated for 10 min with the CB1R and GHS-R1a antagonists (1 μM rimonabant or 1 μM YIL 781), and subsequently stimulated with selective agonists (1 nM, 10 nM, 100 nM, 1 μM ACEA, or 200 nM ghrelin). Upon excitation at 488 nm, real-time 515 nm fluorescence emission due to calcium-ion complexed GCaMP6 was recorded on the EnSpire® Multimode Plate Reader (every 5 s, 100 flashes per well).
Proximity Ligation Assays (PLAs)
Physical interaction between CB1R and GHS-R1a was detected using the Duolink in situ PLA detection Kit (OLink; Bioscience, Uppsala, Sweden) following the instructions of the supplier. Primary neurons were grown on glass coverslips, fixed in 4% paraformaldehyde for 15 min, washed with PBS containing 20 mM glycine to quench the aldehyde groups, and permeabilized with the same buffer containing 0.05% Triton X-100 (20 min). Then, samples were successively washed with PBS. After 1 h incubation at 37°C with the blocking solution in a pre-heated humidity chamber, primary neurons were incubated overnight in the antibody diluent medium with a mixture of equal amounts of mouse anti-CB1R (1/100; sc-293419, Santa Cruz Technologies, Dallas, TX, USA) and rabbit anti-GHS-R1a (1/100; ab95250, Abcam, Cambridge, United Kingdom) to detect CB1R-GHS-R1a complexes. Neurons were processed using the PLA probes detecting primary antibodies (Duolink II PLA probe plus and Duolink II PLA probe minus) diluted in the antibody diluent solution (1:5). Ligation and amplification were done as indicated by the supplier. Samples were mounted using the mounting medium with Hoechst (1/100; Sigma-Aldrich) to stain nuclei. Samples were observed in a Zeiss 880 confocal microscope (Carl Zeiss, Oberkochen, Germany) equipped with an apochromatic 63× oil immersion objective (N.A. 1.4) and a 405 nm and a 561 nm laser lines. For each field of view, a stack of two channels (one per staining) and four Z stacks with a step size of 1 μm were acquired. The number of neurons containing one or more red spots vs. total cells (blue nucleus) was determined, and the unpaired t-test was used to compare the values (red dots/cell) obtained.
Results
The CB1R May Interact With the GHS-R1a
Cannabis sativa L consumption has an orexigenic effect via a mechanism in which a hormone of the endocrine system, ghrelin, participates. To identify whether or not there are functional interactions between the cannabinoidergic and the orexinergic systems, we first tested a potential interaction between the CB1R and the functional form of the ghrelin receptor, GHS-R1a. Immunocytochemical assays in HEK-293T cells transfected with the cDNA of the GHS-R1a fused to Renilla luciferase (Rluc) and/or the cDNA for the CB1R fused to the Yellow Fluorescent Protein (YFP) led to detect the receptors at the plasma membrane level with a marked colocalization when coexpressed (Figure 1A).
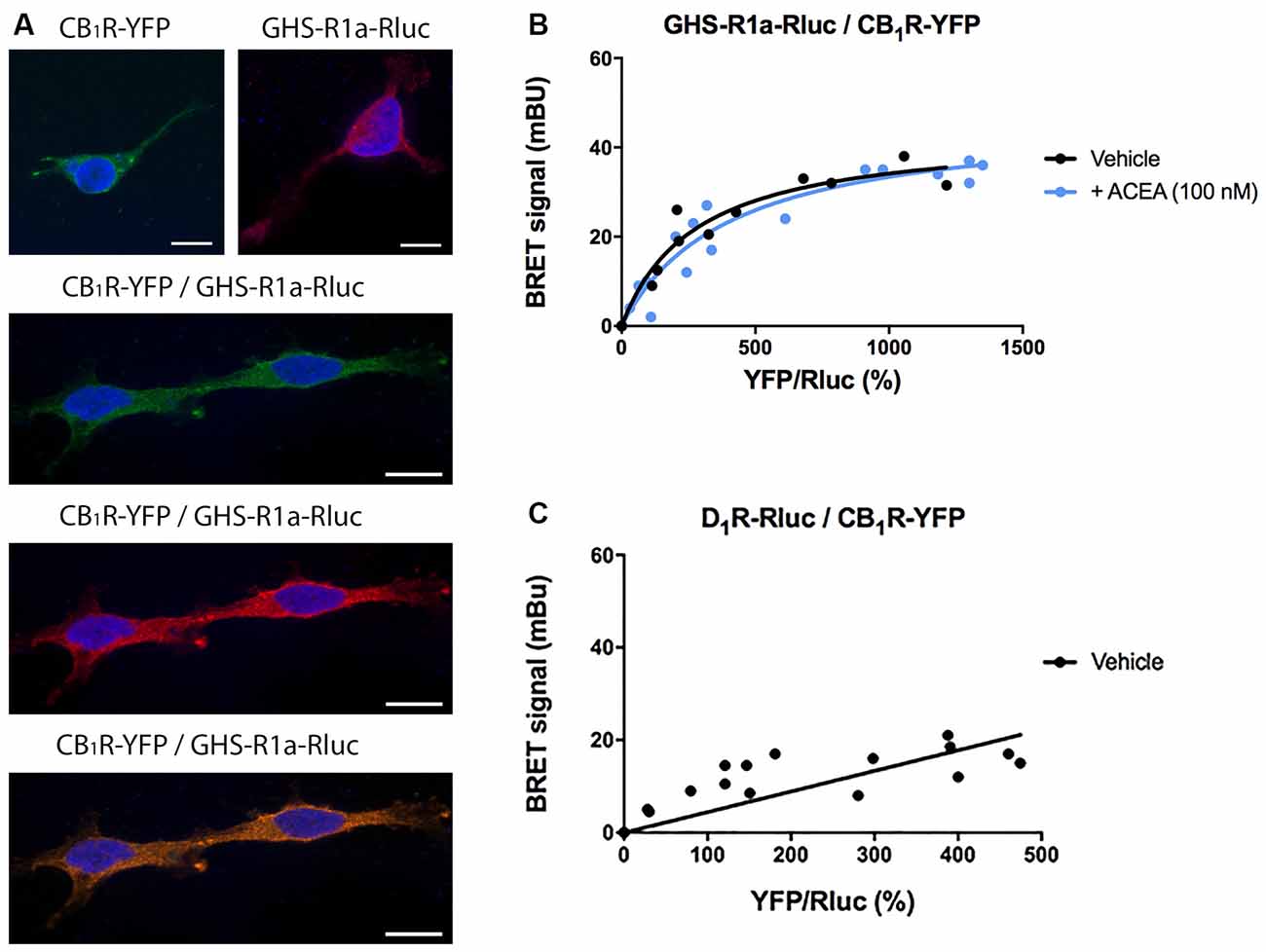
Figure 1. Molecular interaction between GHS-R1a and CB1 receptors expressed in HEK-293T cells. (A) Confocal microscopy images of HEK-293T cells transfected with cDNAs for GHS-R1a-Rluc (1.5 μg) and/or for CB1R-YFP (1 μg). GHS-R1a-Rluc (red) was identified by immunocytochemistry using an anti-Rluc antibody (Merck-Millipore, 1/100). The CB1R-YFP (green) was identified by the fluorescence due to YFP. Colocalization is shown in yellow. Cell nuclei were stained with Hoechst (blue). Scale bar: 10 μm. (B,C) BRET saturation experiments were performed using HEK-293T cells co-transfected with a constant amount of GHS-R1a-Rluc cDNA (1.5 μg) and increasing amounts of CB1R-YFP cDNA (0–2 μg) and treated with ACEA (100 nM) or vehicle. As a negative control, HEK-293T cells were transfected with a constant amount of D1R-Rluc cDNA (1.5 μg) and increasing amounts of CB1R-YFP cDNA (0–2 μg). BRET data are expressed as the mean ± SEM of eight independent experiments performed in duplicates. mBU: milliBRET units.
As colocalization may be found for proteins that are close (approximately 200 nm apart) but may not be directly interacting, a Bioluminescence Resonance Energy Transfer (BRET) assay was performed in HEK-293T cells cotransfected with a constant amount of the cDNA for GHS-R1a-Rluc and increasing amounts of cDNA for CB1R-YFP. A saturation curve (BRETmax = 44 ± 4 mBU, BRET50 = 280 ± 70) was obtained, demonstrating a direct interaction between the two receptors in this heterologous expression system (Figure 1B). When the same experiment was performed in cells pretreated for 30 min with the selective CB1R agonist, arachidonyl-2’-chloroethylamide (ACEA, 100 nM), no significant differences were observed (BRETmax = 47 ± 4 mBU, BRET50 = 400 ± 10). This result indicates that CB1R-GHS-R1a interaction is not affected by the activation of the CB1R. As a negative control, HEK-293T cells were transfected with a constant amount of dopamine D1 receptor-Rluc cDNA and increasing amounts of CB1R-YFP cDNA; the nonspecific linear signal indicates a lack of interaction between these two proteins (Figure 1C).
CB1R-Mediated Signaling Is Blocked in the CB1-GHS-R1a Receptor Heteromer (CB1R-GHS-R1aHet)
After identifying a direct interaction between CB1R and GHS-R1a, the functional consequences of the interaction were investigated. Signaling assays were performed considering that the CB1R couples to Gi and that, although the canonical protein that couples to GHS-R1a receptor is Gq, the ghrelin receptor may also couple to Gi. The activation of any of the receptors in the presence of forskolin (FK), which activates adenylate cyclase, led to a decrease in cytosolic cAMP levels in HEK-293T cells expressing CB1R or GHS-R1a. In cells expressing the CB1R, the selective agonist, arachidonyl-2’-chloroethylamide (ACEA), led to a dose-response decrease in (FK)-stimulated cAMP levels that were not affected by pretreatment with ghrelin. Moreover, ghrelin treatment induced no effect over cannabinoid CB1R, demonstrating ligand selectivity. The effect of ACEA was specific as it was blocked by a selective CB1R antagonist, rimonabant (Figure 2A). In cells expressing the GHS-R1a, 200 nM ghrelin induced a significant (circa 30%) decrease of FK-induced cAMP level that was not affected by ACEA but that was completely counteracted by YIL 781, a selective GHS-R1a receptor antagonist (Figure 2B). It was confirmed that ACEA did not induce any effect over the GHS-R1a. When the Gq coupling was assayed using the GCaMP6 sensor of cytoplasmic Ca2+, no signal was obtained in cells expressing the CB1R (Figure 2C), while in cells expressing the GHS-R1a receptor ghrelin lead to a transient peak of cytosolic [Ca2+] that was not modified by preincubation with ACEA but that was prevented upon preincubation with the ghrelin receptor antagonist (Figure 2D).
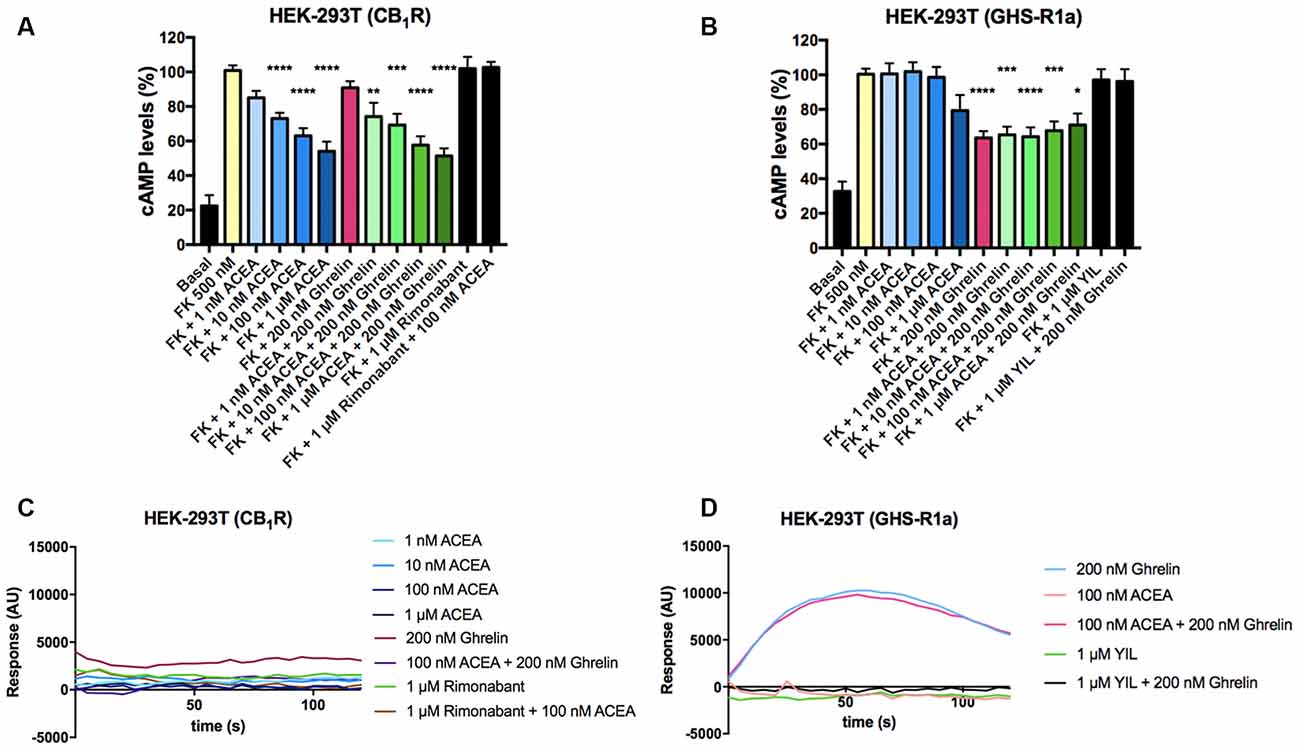
Figure 2. Functional characterization of GHS-R1a and CB1 receptors expressed in HEK-293T cells. (A,B) HEK-293T cells transfected with cDNA encoding for either CB1R (1 μg; A) or GHS-R1a (1.5 μg; B) were pre-treated with selective antagonists, 1 μM rimonabant -CB1R- or 1 μM YIL 781 -GHS-R1a-, and subsequently treated with the selective agonists, ACEA (1 nM, 10 nM, 100 nM, 1 μM) -CB1R- or ghrelin (200 nM) -GHS-R1a-. cAMP levels after 0.5 μM forskolin (FK) stimulation were detected by the Lance Ultra cAMP kit. Results are expressed in % respect to levels obtained upon FK stimulation (100%). The values are the mean ± SEM of 10 independent experiments performed in triplicates. One-way ANOVA followed by Bonferroni’s multiple comparison post-hoc test were used for statistical analysis. *p < 0.05, **p < 0.01, ***p < 0.001, ****p < 0.0001 vs. FK treatment. (C,D) HEK-293T cells expressing an engineered calcium sensor, 6GCaMP, and CB1R (C) or GHS-R1a (D) were pre-treated with selective antagonists for 10 min followed by agonist stimulation. Real-time traces of cytoplasmic Ca2+ levels detected by EnSpire® Multimode Plate Reader (PerkinElmer, Waltham, MA, USA) over time are shown (six independent experiments).
In HEK-293T cells expressing CB1 and GHS-R1a receptors, the ghrelin-induced decrease of FK-stimulated cAMP levels was significant although lower than that in cells only expressing the ghrelin receptor (37% vs. 15%; Figure 3A). Interestingly, the presence of the ghrelin receptor uncoupled CB1R activation from Gi protein, at least at a functional level. In fact, ACEA even at the highest concentration (1 μM) was not able to significantly reduce FK-induced cAMP levels. Functionality was, however, found when cells were treated with ghrelin and there was no additive effect at concentration of 10 nM ACEA or higher; the lower concentration of ACEA (1 nM) showed a trend to increase the ghrelin-induced effect (Figure 3A). Results related to Gq coupling were noteworthy as ACEA, which did not lead to calcium ion mobilization by itself, significantly increased the effect of ghrelin. Remarkably, the potentiation was much stronger at low doses of ACEA (1 nM) than at higher concentrations (Figure 3B). In particular, the 40 s post-activation increase provided by the presence of 1 nM, 10 nM or 100 nM ACEA over the signal provided by 200 nM ghrelin, was (in percentage), 140 ± 30, 65 ± 30, and 71 ± 15, respectively. Finally, as GHS-R1a and CB1 receptor agonists lead to activation of the mitogen-activated protein kinase (MAPK) pathway (Mousseaux et al., 2006; Daigle et al., 2008; Navarro et al., 2018b), we tested the properties of the heteromer in the link to the MAPK signaling pathway. Again, the effect of the cannabinoid receptor agonist was suppressed when the two receptors were co-expressed, while the effect of ghrelin was not modified by low doses of ACEA but was diminished when higher doses were used (Figure 3C). In a control assay, it was confirmed that ACEA does increase ERK1/2 phosphorylation, as previously reported (Navarro et al., 2018a, b). These data demonstrate that cannabinoids may regulate GHS-R1a function depending on the concentration and that GHS-R1a expression suppresses cannabinoid receptor-mediated events supposedly by the establishment of heteromeric complexes.
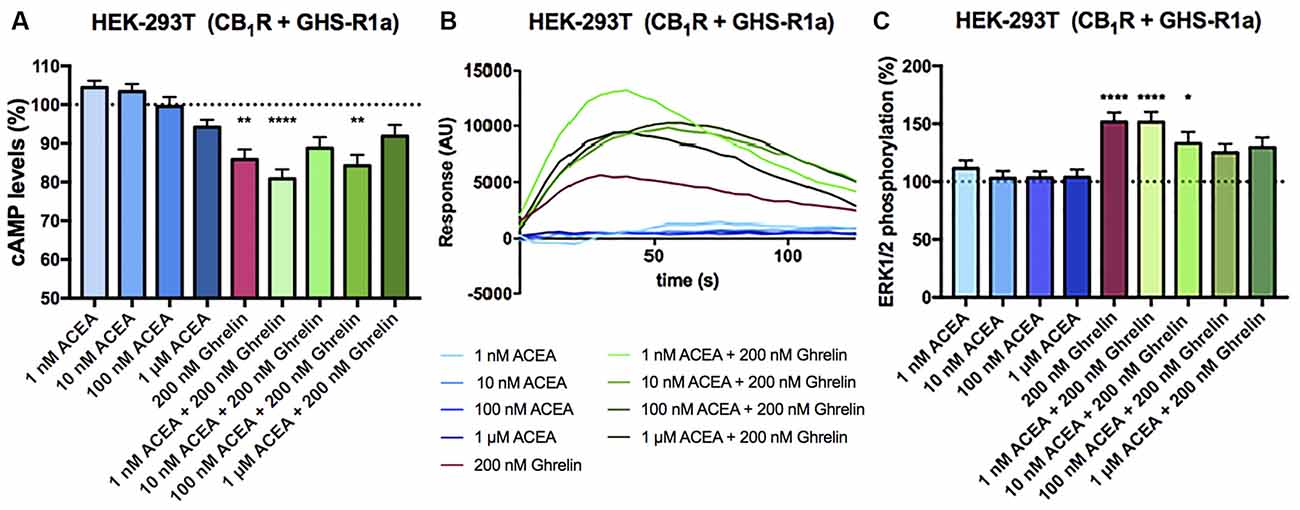
Figure 3. Functional characterization of the CB1-GHS-R1aHet expressed in HEK-293T cells. (A–C) HEK-293T cells were transfected with cDNAs encoding for GHS-R1a (1.5 μg) and for CB1R (1 μg; A,C) or cDNAs encoding for GHS-R1a (1.5 μg), CB1R (1 μg) and the 6GCaMP calcium sensor (1 μg; B) and stimulated with selective agonists, 200 nM ghrelin -for GHS-R1a- and 1 nM, 10 nM, 100 nM, and 1 μM ACEA -for CB1R-, individually or in combination. cAMP levels were analyzed by the Lance Ultra cAMP kit and results are expressed in % respect to levels obtained upon 0.5 μM FK stimulation (A; 100%, dotted line). Representative traces of intracellular Ca2+ responses over time are shown (eight independent experiments; B). ERK 1/2 phosphorylation was determined by an AlphaScreen®SureFire® kit (PerkinElmer) using an EnSpire® Multimode Plate Reader (PerkinElmer, Waltham, MA, USA; C); (basal is represented as 100%, dotted line). In cAMP accumulation and MAPK pathway signaling-related assays, the values are the mean ± SEM of eight independent experiments performed in triplicates. One-way ANOVA followed by Bonferroni’s multiple comparison post-hoc test were used for statistical analysis. *p < 0.05, **p < 0.01, ****p < 0.0001; vs. FK treatment in cAMP or vs. basal in ERK1/2 phosphorylation assays.
Cross-Talk Characterization
In the 90s different laboratories proved interactions between GPCRs to form heteromeric complexes. These complexes can be detected by energy transfer techniques in heterologous expression systems or by proximity ligation assays (PLA) in natural sources, either primary cultures or tissue sections. An often-found property of a heteromer formed by two GPCRs is that the antagonist of one of the receptors not only blocks the signaling originated at the receptor but also the signaling originated at the partner receptor. Such cross-antagonism, which is due to conformational changes transmitted from one receptor to the other, may serve as a print to detect the heteromer. Another possibility is that coactivation leads to a smaller effect than that obtained upon activating only one receptor of the complex; this phenomenon is known as negative crosstalk. Finally, in some cases, the antagonist of one receptor may restore the signaling via the partner receptor in the heteromer.
To study the effect of antagonists, HEK-293T cells expressing CB1 and GHS-R1a receptors were pretreated with the selective antagonists before agonist stimulation. As observed in Figure 4A, the CB1R antagonist, rimonabant, did not block Gi-mediated ghrelin-induced effect, whereas the GHS-R1a antagonist, YIL 781, which completely blocked ghrelin-induced decrease of FK-induced cAMP levels, did not restore the CB1R-Gi coupling. Accordingly, there was no cross-antagonism in GHS-R1a/Gi-mediated signaling when the CB1 receptor was blocked by a selective antagonist. Similar were results in Figure 4B, i.e., there was no cross-antagonism in GHS-R1a receptor/Gq-mediated signaling when the CB1R receptor was blocked by a selective antagonist. In summary, neither cross-antagonism nor restoration of CB1R-Gi coupling was observed when addressing direct Gi- or direct Gq-induced outputs using selective antagonists.
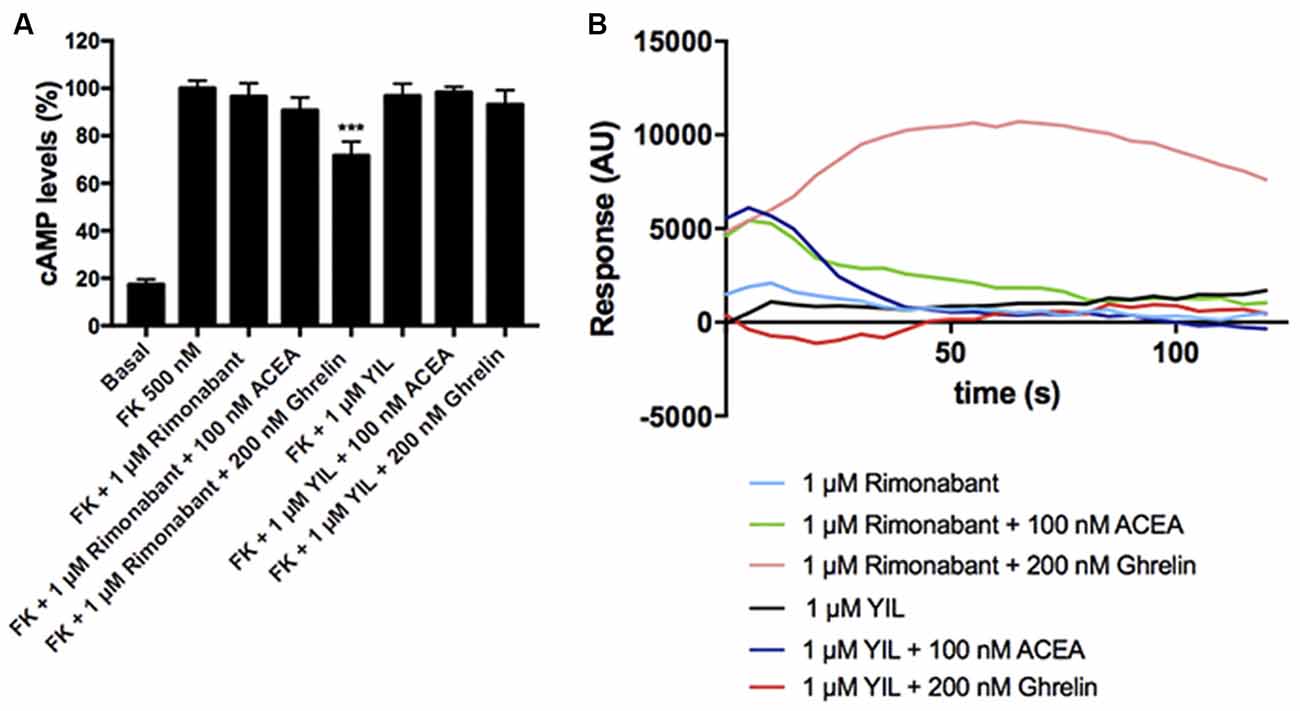
Figure 4. Effect of antagonists in the functionality of the CB1-GHS-R1aHet. HEK-293T cells were transfected with cDNAs encoding for GHS-R1a (1.5 μg) and for CB1R (1 μg; A) or with cDNAs encoding for GHS-R1a (1.5 μg), CB1R (1 μg), and the 6GCaMP calcium sensor (1 μg; B). Cells were pre-treated with antagonists, 1 μM YIL 781 -for GHS-R1a- or 1 μM rimonabant -for CB1R-, and subsequently stimulated with selective agonists, 200 nM ghrelin -for GHS-R1a- or 100 nM ACEA -for CB1R-. cAMP levels were analyzed by the Lance Ultra cAMP kit and results were expressed in % respect to levels obtained upon 0.5 μM FK stimulation (A; 100%). Representative real- time traces of cytoplasmic Ca2+ responses are shown (eight independent experiments; B). In cAMP accumulation assays, the values are the mean ± SEM of eight independent experiments performed in triplicates. One-way ANOVA followed by Bonferroni’s multiple comparison post-hoc test were used for statistical analysis. ***p < 0.001; vs. FK treatment.
Effect of Ghrelin and/or ACEA in Primary Striatal Neurons
After demonstrating the functionality of CB1R-GHS-R1aHet in transfected HEK-293T cells, we moved to a more physiologic environment to look for the expression of the receptor complex. We investigated the expression and function of CB1R-GHS-R1aHet in primary striatal neurons. First, we analyzed the effect of ACEA and ghrelin on FK-induced cAMP. As observed in Figure 5, ACEA was able to induce a dose-dependent effect. Ghrelin also decreased the FK-induced cAMP levels. Coactivation with ghrelin and 1 nM ACEA resulted in an additive effect, but not at higher ACEA concentrations. Moreover, the effect of ACEA was not affected by the antagonists of the GHS-R1a receptor and, reciprocally, the effect of ghrelin was not counteracted by the antagonists of the CB1R (Figure 5B). On the other hand, when analyzing ERK1/2 phosphorylation in striatal primary neurons, both ACEA and ghrelin led to a significant increase in phosphorylation levels. In addition, no cross-antagonism was detected in primary neurons pretreated with rimonabant followed by ghrelin stimulation (Figures 5C,D). These results suggest that the proportion of CB1R-GHS-R1aHets in primary striatal cultures is relatively low and that CB1 and GHS-R1a receptors may be forming complexes with other GPCRs (see “Discussion” section).
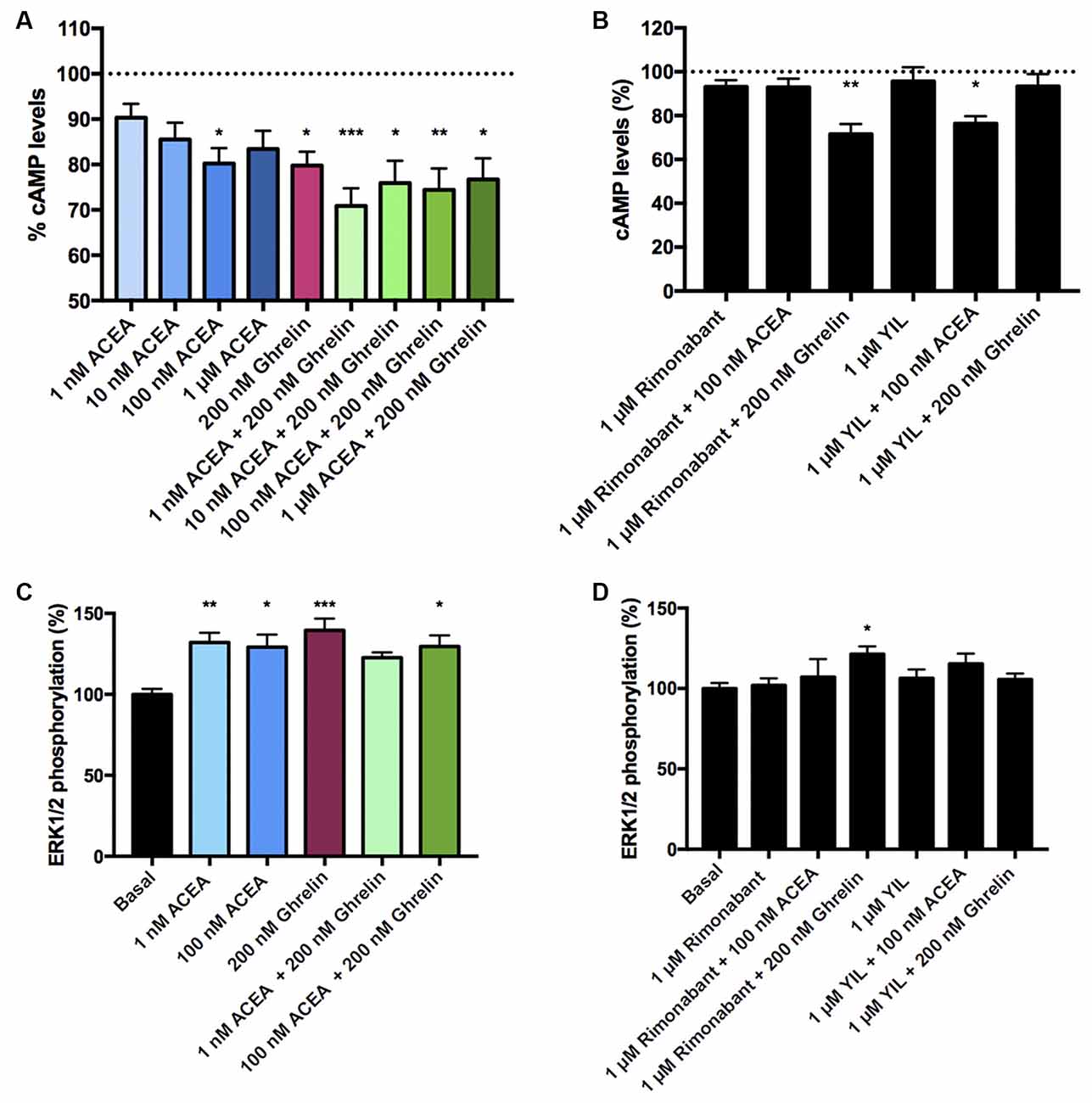
Figure 5. CB1R-GHS-R1aHet signaling in primary striatal neurons from C57BL/6J mice. Primary striatal neurons obtained from C57BL/6J brain fetuses were pre-treated with selective antagonists, 1 μM YIL 781 -for GHS-R1a- or 1 μM rimonabant -for CB1R- or vehicle and subsequently stimulated with selective agonists, 200 nM ghrelin -for GHS-R1a- or 1 nM, 10 nM, 100 nM, and 1 μM ACEA -for CB1R-, individually or in combination. cAMP levels (A,B) were collected by the Lance Ultra cAMP kit and results are expressed in % respect to levels obtained upon 0.5 μM FK stimulation (100%, dotted line). ERK1/2 phosphorylation (C,D) was analyzed using an AlphaScreen®SureFire® kit (PerkinElmer) and results are expressed in % respect to basal levels. Values are the mean ± SEM of six independent experiments performed in triplicates. One-way ANOVA followed by Bonferroni’s multiple comparison post-hoc tests were used for statistical analysis. *p < 0.05, **p < 0.01, ***p < 0.001; vs. FK treatment (A,B) or vs. basal (C,D).
The CB1R-GHS-R1aHet Is Overexpressed in Striatal Neurons Isolated From the Brain of the Progeny of Mothers Under a High-Fat Diet
One of the aims of this study was to correlate the expression of CB1R-GHS-R1a receptor complexes in a situation of unbalanced energy homeostasis. For this purpose, we used primary striatal neurons isolated from fetuses of mothers fed a standard (STD) or high fat (HFD) diet (see “Materials and Methods” section). The expression of CB1R-GHS-R1aHets was assessed by in situ proximity ligation assay (PLA). An important increase in the expression of CB1-GHS-R1a complexes was observed in striatal neurons from fetuses of HFD mothers (18 red dots/cell vs. 3 red dots/cell in neurons from fetuses of STD mothers; Figures 6A,B). Moreover, when primary neurons from fetuses of HFD mothers were treated with 1 nM ACEA, a significant decrease in complex expression (12 red dots/cell) was observed; however, a higher concentration of the compound, 100 nM, led to a marked increase in the number of complexes (36 red dots/cell). Remarkably, treatment with 200 nM ghrelin produced a robust increase in CB1R-GHS-R1a complex expression (>40 red dots/cell; Figure 6C). In summary, the expression of the CB1R-GHS-R1aHet was higher in the progeny of HFD mothers and may be regulated by ghrelin and, differentially, by cannabinoid concentration.
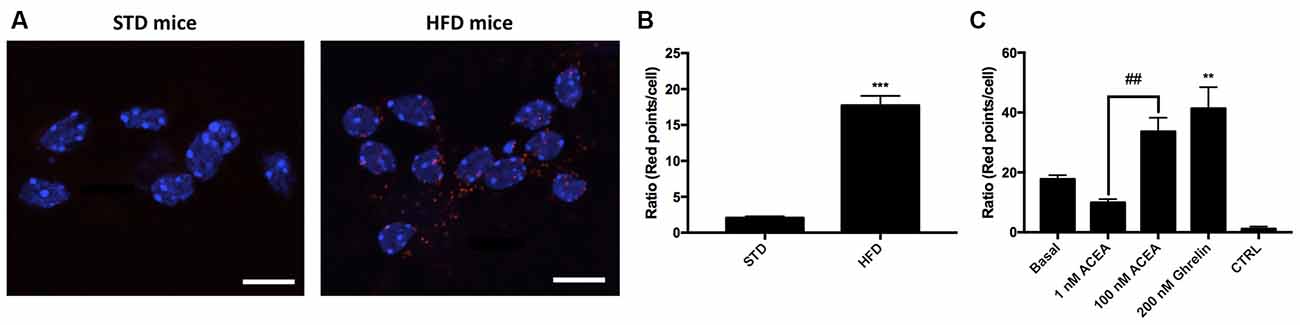
Figure 6. Expression and function of CB1-GHS-R1aHets in primary striatal neurons isolated from fetuses of pregnant C57BL/6J female mice subjected to a standard (STD) or high-fat (HFD) diet. (A) CB1-GHS-R1aHets were detected by the in situ proximity ligation assay (PLA) in primary striatal neurons isolated from the striatum of fetuses from STD or HFD pregnant mothers. Experiments were performed in samples from six animals. (B,C) The number of red dots/cell was quantified using Andy’s algorithm Fiji’s plug-in; nuclei were stained using Hoechst (blue). In (B) the number of dots/cell in HFD samples are compared with that in STD samples. In (C) neurons obtained from fetuses from HFD mothers were stimulated with 200 nM ghrelin -for GHS-R1a- or 1 nM and 100 nM ACEA -for CB1R- and analyzed by PLA (C). The negative control was obtained omitting one of the primary antibodies. Scale bar: 10 μm. One-way ANOVA followed by Bonferroni’s multiple comparison post-hoc test was used for statistical analysis on comparing to basal or to STD: ***p < 0.001, vs. STD (B) **p < 0.01, vs. basal (C). Bonferroni’s multiple comparison test showed significance in comparing results from ACEA treatments: ##p < 0.01 (C).
Discussion
Cannabis has been known and used for years by various civilizations and is used even today. Cannabis use is perceived in two almost opposite ways. One is related to the psychotropic properties of one of its components, (6aR, 10aR)-6,6,9-trimethyl-3-pentyl-6a,7,8,10a-tetrahydro-6H-benzo[c]chromen-1-ol, commonly known as THC (or Δ9THC; CAS registry number #1972-08-3). It is under question whether the exposure to THC is addictive although it is known that the compound affects homeostatic synaptic plasticity. The good side is the possibility that compounds in Cannabis sativa L may be useful to combat a variety of diseases. For instance, dronabinol (Marinol®), with an identical chemical structure to THC but of synthetic origin, has been already approved for human use. Also approved are a preparation having an equal amount of THC and cannabidiol (Sativex®) and a preparation of pure cannabidiol in vegetable oil (Epidiolex®). The main therapeutic indications of these drugs are anti-emetic and spasticity management. Interestingly, a CB1R antagonist, rimonabant, was approved to combat obesity. The discovery that blockade of the CB1R could lead to a drop in body weight by reducing food consumption correlated with the well-known orexigenic properties of Cannabis consumption. Unfortunately, the compound, which is brain permeable, was withdrawn due to serious adverse events related to alterations in central functions. Although it was hypothesized that CB1R antagonists unable to cross the blood-brain barrier could overcome the side effects, there is evidence that the anti-orexigenic actions of CB1R antagonists are due to, at least in part, the blockade of receptors in the central nervous system where the CB1 is the most abundant GPCR (Carai et al., 2006; Christensen et al., 2007; Sam et al., 2011; Tudge et al., 2015). In this scenario, we aimed at defining possible interactions between cannabinoid receptors and the receptor for the so-called “hunger” hormone, ghrelin. In a recent article, we have shown the interaction of the cannabinoid CB2 receptor (CB2R) with the GHS-R1a receptor in a heterologous expression system and in physiological cell models (Lillo et al., 2021). Therefore, the first aim of the present study was to address the possible interaction between the ghrelin receptor and the CB1R and to characterize the functional consequences of such interaction. Both, the previous and the present studies demonstrate that the GHS-R1a receptor may interact with either CB1 or CB2 receptors and that the resulting heteromers may occur in physiological environments.
At the functional level, allosteric interactions within CB1R-GHS-R1aHets and of CB2R-GHS-R1aHets lead to the blockade of cannabinoid receptor/Gi-mediated signaling. The blockade occurs just by simple co-expression, i.e., it does not require the activation of the GHS-R1a receptor. Taking these results together, cells expressing cannabinoid and ghrelin receptors heteromers on the cell surface would not be responsive to cannabinoids, or even to endocannabinoids, unless there is a pool of cell surface CB1Rs that are not interacting with the ghrelin receptor.
Several phytocannabinoids are able to cross the blood-brain barrier (Sagredo et al., 2007; Lafuente et al., 2011; Espejo-Porras et al., 2013; García et al., 2016; Libro et al., 2016; Palomo-Garo et al., 2016; Zeissler et al., 2016; Valdeolivas et al., 2017; Haider et al., 2018; Franco et al., 2020) and reach the brain reward circuits in which ghrelin acts. Ghrelin receptors in reward circuits mediate the control of food intake (Guan et al., 1997; Funahashi et al., 2003; Geelissen et al., 2003; Argente-Arizón et al., 2015; Cassidy and Tong, 2017). Our data indicate a blockade of Gi/CB1R coupling but a potentiation induced by cannabinoids of the GHS-R1a/Gq-mediated events, namely a potentiation in calcium-mediated signaling. In our opinion, the higher potentiation at low doses of the CB1R agonist (1 nM) is physiologically relevant. It is tempting to speculate that the atypical effect depending on the dose, i.e., higher potentiation at lower doses underlies previous results in humans and mice showing that low doses of THC are associated with hyperphagia, whereas high doses suppress it (Simon and Cota, 2017). As previously highlighted, endocannabinoid tone may be important in controlling the inputs received by the reward circuits and that impact on food intake, especially as it relates to the hedonic part of eating (Coccurello and Maccarrone, 2018). Whereas cannabinoids acting on the CB1 receptor affected calcium mobilization mediated by GHS-R1a-Gq coupling, this is not the case for the CB2R-GHS-R1aHet (Lillo et al., 2021). As further discussed below, caution must be taken when trying to make general conclusions as the allosteric-cross interactions will occur in neurons expressing CB1R and GHS-R1a receptors and also CB1R-GHS-R1aHets; i.e., not all neurons express the two receptors, and a given neuron may express the CB1R-GHS-R1aHet plus other CB1R-containing heteromers (see, in http://www.gpcr-hetnet.com/, GPCRs that interact with the CB1R; accessed on October 22, 2021).
As the risk to be obese is higher in families with a history of overweighed individuals, we reasoned that the expression of the heteromer could be altered in the offspring of high-fat-diet mouse mothers as they have more risk to be obese. Compared with samples from fetuses of mothers subjected to STD, there was a marked increase of CB1R-GHS-R1aHets expression in striatal neurons from siblings of pregnant female mice under a high-fat diet. Such an increase in heteromer expression might be implicated in the obesity predisposition of the progeny of obese parents (Abu-Rmeileh et al., 2008). Upregulation of the CB1R-GHS-R1aHet in siblings of mothers fed with HFD suggests that already at birth, these mice have a compromised CB1R function. In addition, we observed that the number of heteromers markedly increased by activation of either the CB1 with 100 nM ACEA or the GHS-R1a receptors with ghrelin. The upregulation induced by ACEA treatment in neurons did not occur in transfected HEK-293T cells, where ACEA pretreatment does not alter the BRET saturation curve. Also noteworthy is the fact that treatments are short, i.e., changes in expression are not due to regulation of gene expression but to conformational rearrangements within the receptor-receptor and receptor-G protein interactions. Another piece of information is that, in our hands, the CB1R/Gi-mediated effect of 100 nM ACEA observed in striatal primary neurons was completely blocked in transfected HEK-293T cells expressing the CB1R-GHS-R1aHet. This result indicates that not all striatal neurons express the heteromer and/or that the CB1R in a given neuron may be interacting with receptors other than the GHS-R1aHet, i.e., with receptors that are not allosterically blocking CB1R-mediated signaling. In the so called “hedonic eating” by Coccurello and Maccarrone (2018), dopamine plays a key role in the reward circuits. Accordingly, dopamine receptors may be considered in the overall picture. In this sense, the CB1R may interact with dopamine receptors; it has been reported that the cannabinoid receptor may, at least, interact with the dopamine D2 receptor (Jarrahian et al., 2004; Navarro et al., 2008; Marcellino et al., 2008; Przybyla and Watts, 2010; Khan and Lee, 2014; Köfalvi et al., 2020), reviewed in García et al. (2016). Cannabinoid CB1/dopamine D2 receptor-receptor interaction is bidirectional and may result in functional antagonism, i.e., a CB1R agonist blocking the D2R-mediated modulation of locomotor activity (Marcellino et al., 2008) or in a shift from Gi to Gs coupling and signaling (Bagher et al., 2017).
In conclusion, the benefits of cannabinoids acting on populations of striatal neurons expressing CB1R-GHS-R1aHet would be lost by the blockade exerted by the ghrelin receptor, thus prevailing the effect of such cannabinoids on other systems such as the dopaminergic. On the other hand, the CB1R-GHSR1aHet coactivated by ghrelin and cannabinoids provides a more robust calcium response. Such bursts in the concentration of cytosolic calcium must be relevant since calcium regulates almost any event of neuronal physiology. Our results suggest the potential for GHS-R1a receptor antagonists, which could offer a double benefit: (i) reduce food intake and (ii) revert the detrimental effects of HFD on the functionality of the CB1R in striatal neurons.
It is quite likely that the CB1R-GHSR1aHet does occur in given subpopulations of neurons. Therefore, the next stage would be an accurate description, at the anatomical and cellular levels, of the regions and specific neurons where the two receptors are co-expressed.
Data Availability Statement
The raw data supporting the conclusions of this article will be made available by the authors, without undue reservation.
Ethics Statement
The animal study was reviewed and approved by University of Barcelona Ethical Committee, which reports to the regional Government (Protocol #9659; Generalitat de Catalunya, May 24, 2019).
Author Contributions
GN and RF: conceptualization, supervision, and writing—original draft. RF: data curation. AL, JL, and GN: formal analysis. AL, JL, IR, FD, CM, and GN: investigation, writing—review and editing. AL, JL, and IR: methodology. AL: project administration. CM, GN, and RF: resources. GN: validation. All authors have read and agreed to the published version of the manuscript. All authors contributed to the article and approved the submitted version.
Funding
This work was partially supported by the AARFD-17-503612 grant the US Alzheimer’s Association, and by grants SAF2017-84117-R and PID2020-113430RB-I00 funded by Spanish MCIN/AEI/10.13039/501100011033 and, as appropriate, by “ERDF A way of making Europe”, by the “European Union” or by the “European Union Next Generation EU/PRTR”. The research group of the University of Barcelona is considered of excellence (grup consolidat #2017 SGR 1497) by the Regional Catalonian Government, which does not provide any specific funding for reagents or for payment of services or Open Access fees.
Conflict of Interest
The authors declare that the research was conducted in the absence of any commercial or financial relationships that could be construed as a potential conflict of interest.
Publisher’s Note
All claims expressed in this article are solely those of the authors and do not necessarily represent those of their affiliated organizations, or those of the publisher, the editors and the reviewers. Any product that may be evaluated in this article, or claim that may be made by its manufacturer, is not guaranteed or endorsed by the publisher.
Acknowledgments
In memoriam, María Teresa Miras-Portugal a great scientist in the Purinergic field and a beloved friend of RF.
References
Abizaid, A., and Horvath, T. L. (2008). Brain circuits regulating energy homeostasis. Regul. Pept. 149, 3–10. doi: 10.1016/j.regpep.2007.10.006
Abu-Rmeileh, N. M. E., Hart, C. L., McConnachie, A., Upton, M. N., Lean, M. E. J., and Watt, G. C. M. (2008). Contribution of midparental BMI and other determinants of obesity in adult offspring. Obesity 16, 1388–1393. doi: 10.1038/oby.2008.211
Alexander, S. P., Christopoulos, A., Davenport, A. P., Kelly, E., Mathie, A., Peters, J. A., et al. (2019). The concise guide to pharmacology 2019/20: G protein-coupled receptors. Br. J. Pharmacol. 176, S21–S141. doi: 10.1111/bph.14748
Alexander, S. P., Christopoulos, A., Davenport, A. P., Kelly, E., Mathie, A., Peters, J. A., et al. (2021). The concise guide to pharmacology 2021/22: G protein-coupled receptors. Br. J. Pharmacol. 178, S27–S156. doi: 10.1111/bph.15538
Argente-Arizón, P., Freire-Regatillo, A., Argente, J., and Chowen, J. A. (2015). Role of non-neuronal cells in body weight and appetite control. Front. Endocrinol. 6:42. doi: 10.3389/fendo.2015.00042
Bagher, A. M., Laprairie, R. B., Toguri, J. T., Kelly, M. E. M., and Denovan-Wright, E. M. (2017). Bidirectional allosteric interactions between cannabinoid receptor 1 (CB1) and dopamine receptor 2 long (D2L) heterotetramers. Eur. J. Pharmacol. 813, 66–83. doi: 10.1016/j.ejphar.2017.07.034
Carai, M. A. M., Colombo, G., Maccioni, P., and Gessa, G. L. (2006). Efficacy of rimonabant and other cannabinoid CB1 receptor antagonists in reducing food intake and body weight: preclinical and clinical data. CNS Drug Rev. 12, 91–99. doi: 10.1111/j.1527-3458.2006.00091.x
Carriba, P., Navarro, G., Ciruela, F., Ferré, S., Casadó, V., Agnati, L., et al. (2008). Detection of heteromerization of more than two proteins by sequential BRET-FRET. Nat. Methods 5, 727–733. doi: 10.1038/nmeth.1229
Casanovas, M., Jiménez-Rosés, M., Cordomí, A., Lillo, A., Vega-Quiroga, I., Izquierdo, J., et al. (2021). Discovery of a macromolecular complex mediating the hunger suppressive actions of cocaine: structural and functional properties. Addict. Biol. 26:e13017. doi: 10.1111/adb.13017
Cassidy, R. M., and Tong, Q. (2017). Hunger and satiety gauge reward sensitivity. Front. Endocrinol. 8:104. doi: 10.3389/fendo.2017.00104
Chen, T.-W., Wardill, T. J., Sun, Y., Pulver, S. R., Renninger, S. L., Baohan, A., et al. (2013). Ultrasensitive fluorescent proteins for imaging neuronal activity. Nature 499, 295–300. doi: 10.1038/nature12354
Christensen, R., Kristensen, P. K., Bartels, E. M., Bliddal, H., and Astrup, A. (2007). Efficacy and safety of the weight-loss drug rimonabant: a meta-analysis of randomised trials. Lancet 370, 1706–1713. doi: 10.1016/S0140-6736(07)61721-8
Coccurello, R., and Maccarrone, M. (2018). Hedonic eating and the “delicious circle”: from lipid-derived mediators to brain dopamine and back. Front. Neurosci. 12:271. doi: 10.3389/fnins.2018.00271
Daigle, T. L., Kearn, C. S., and Mackie, K. (2008). Rapid CB1 cannabinoid receptor desensitization defines the time course of ERK1/2 MAP kinase signaling. Neuropharmacology 54, 36–44. doi: 10.1016/j.neuropharm.2007.06.005
Elphick, M. R., and Egertová, M. (2001). The neurobiology and evolution of cannabinoid signalling. Philos. Trans. R. Soc. B Biol. Sci. 356, 381–408. doi: 10.1098/rstb.2000.0787
Espejo-Porras, F., Fernández-Ruiz, J., Pertwee, R. G., Mechoulam, R., and García, C. (2013). Motor effects of the non-psychotropic phytocannabinoid cannabidiol that are mediated by 5-HT1Areceptors. Neuropharmacology 75, 155–163. doi: 10.1016/j.neuropharm.2013.07.024
Franco, R., Rivas-Santisteban, R., Reyes-Resina, I., Casanovas, M., Pérez-Olives, C., Ferreiro-Vera, C., et al. (2020). Pharmacological potential of varinic-, minor-, and acidic phytocannabinoids. Pharmacol. Res. 158:104801. doi: 10.1016/j.phrs.2020.104801
Funahashi, H., Takenoya, F., Guan, J.-L., Kageyama, H., Yada, T., and Shioda, S. (2003). Hypothalamic neuronal networks and feeding-related peptides involved in the regulation of feeding. Anat. Sci. Int. 78, 123–138. doi: 10.1046/j.0022-7722.2003.00055.x
García, C., Palomo-Garo, C., Gómez-Gálvez, Y., and Fernández-Ruiz, J. (2016). Cannabinoid-dopamine interactions in the physiology and physiopathology of the basal ganglia. Br. J. Pharmacol. 173, 2069–2079. doi: 10.1111/bph.13215
Geelissen, S. M. E., Beck, I. M. E., Darras, V. M., Kühn, E. R., Van der Geyten, S., Kuhn, E. R., et al. (2003). Distribution and regulation of chicken growth hormone secretagogue receptor isoforms. Gen. Comp. Endocrinol. 134, 167–174. doi: 10.1016/s0016-6480(03)00250-8
Guan, X., Yu, H., Palyha, O., McKee, K., Feighner, S., Sirinathsinghji, D., et al. (1997). Distribution of mRNA encoding the growth hormone secretagogue receptor in brain and peripheral tissues. Mol. Brain Res. 48, 23–29. doi: 10.1016/s0169-328x(97)00071-5
Haider, A., Spinelli, F., Herde, A. M., Mu, B., Keller, C., Margelisch, M., et al. (2018). Evaluation of 4-oxo-quinoline-based CB2 PET radioligands in R6/2 chorea huntington mouse model and human ALS spinal cord tissue. Eur. J. Med. Chem. 145, 746–759. doi: 10.1016/j.ejmech.2017.12.097
Hradsky, J., Mikhaylova, M., Karpova, A., Kreutz, M. R., and Zuschratter, W. (2013). Super-resolution microscopy of the neuronal calcium-binding proteins Calneuron-1 and Caldendrin. Methods Mol. Biol. 963, 147–169. doi: 10.1007/978-1-62703-230-8_10
Hradsky, J., Raghuram, V., Reddy, P. P., Navarro, G., Hupe, M., Casado, V., et al. (2011). Post-translational membrane insertion of tail-anchored transmembrane EF-hand Ca2+sensor calneurons requires the TRC40/Asna1 protein chaperone. J. Biol. Chem. 286, 36762–36776. doi: 10.1074/jbc.M111.280339
Jarrahian, A., Watts, V. J., and Barker, E. L. (2004). D2 dopamine receptors modulate Gα-subunit coupling of the CB1 cannabinoid receptor. J. Pharmacol. Exp. Ther. 308, 880–886. doi: 10.1124/jpet.103.057620
Khan, S. S., and Lee, F. J. S. (2014). Delineation of domains within the cannabinoid CB1 and dopamine D2 receptors that mediate the formation of the heterodimer complex. J. Mol. Neurosci. 53, 10–21. doi: 10.1007/s12031-013-0181-7
Köfalvi, A., Moreno, E., Cordomí, A., Cai, N. S., Fernández-Dueñas, V., Ferreira, S. G., et al. (2020). Control of glutamate release by complexes of adenosine and cannabinoid receptors. BMC Biol. 18:9. doi: 10.1186/s12915-020-0739-0
Lafuente, H., Alvarez, F. J., Pazos, M. R., Alvarez, A., Rey-Santano, M. C., Mielgo, V., et al. (2011). Cannabidiol reduces brain damage and improves functional recovery after acute hypoxia-ischemia in newborn pigs. Pediatr. Res. 70, 272–277. doi: 10.1203/PDR.0b013e3182276b11
Libro, R., Diomede, F., Scionti, D., Piattelli, A., Grassi, G., Pollastro, F., et al. (2016). Cannabidiol modulates the expression of Alzheimer’s disease-related genes in mesenchymal stem cells. Int. J. Mol. Sci. 18:26. doi: 10.3390/ijms18010026
Lillo, J., Lillo, A., Zafra, D. A., Miralpeix, C., Rivas-Santisteban, R., Casals, N., et al. (2021). Identification of the ghrelin and cannabinoid CB2 receptor heteromer functionality and marked upregulation in striatal neurons from offspring of mice under a high-fat diet. Int. J. Mol. Sci. 22:8928. doi: 10.3390/ijms22168928
Lu, H.-C. C., and Mackie, K. (2016). An introduction to the endogenous cannabinoid system. Biol. Psychiatry 79, 516–525. doi: 10.1016/j.biopsych.2015.07.028
Mackie, K. (2005). Distribution of cannabinoid receptors in the central and peripheral nervous system. Handb. Exp. Pharmacol. 168, 299–325. doi: 10.1007/3-540-26573-2_10
Marcellino, D., Carriba, P., Filip, M., Borgkvist, A., Frankowska, M., Bellido, I., et al. (2008). Antagonistic cannabinoid CB1/dopamine D2 receptor interactions in striatal CB1/D2 heteromers. A combined neurochemical and behavioral analysis. Neuropharmacology 54, 815–823. doi: 10.1016/j.neuropharm.2007.12.011
Mousseaux, D., Gallic, L. L., Ryan, J., Oiry, C., Gagne, D., Fehrentz, J.-A., et al. (2006). Regulation of ERK1/2 activity by ghrelin-activated growth hormone secretagogue receptor 1A involves a PLC/PKCε pathway. Br. J. Pharmacol. 148, 350–365. doi: 10.1038/sj.bjp.0706727
Navarro, G., Borroto-Escuela, D., Angelats, E., Etayo, I., Reyes-Resina, I., Pulido-Salgado, M., et al. (2018a). Receptor-heteromer mediated regulation of endocannabinoid signaling in activated microglia. Relevance for Alzheimer’s disease and levo-dopa-induced dyskinesia. Brain Behav. Immun. 67, 139–151. doi: 10.1016/j.bbi.2017.08.015
Navarro, G., Reyes-Resina, I., Rivas-Santisteban, R., Sánchez de Medina, V., Morales, P., Casano, S., et al. (2018b). Cannabidiol skews biased agonism at cannabinoid CB1 and CB2 receptors with smaller effect in CB1-CB2 heteroreceptor complexes. Biochem. Pharmacol. 157, 148–158. doi: 10.1016/j.bcp.2018.08.046
Navarro, G., Carriba, P., Gandía, J., Ciruela, F., Casadó, V., Cortés, A., et al. (2008). Detection of heteromers formed by cannabinoid CB1, dopamine D2, and adenosine A2A G-protein-coupled receptors by combining bimolecular fluorescence complementation and bioluminescence energy transfer. ScientificWorldJournal 8, 1088–1097. doi: 10.1100/tsw.2008.136
Navarro, G., Hradsky, J., Lluís, C., Casadó, V., McCormick, P. J., Kreutz, M. R., et al. (2012). NCS-1 associates with adenosine A2A receptors and modulates receptor function. Front. Mol. Neurosci. 5:53. doi: 10.3389/fnmol.2012.00053
Pagotto, U., Cervino, C., Vicennati, V., Marsicano, G., Lutz, B., and Pasquali, R. (2006). How many sites of action for endocannabinoids to control energy metabolism? Int. J. Obes. 30, S39–S43. doi: 10.1038/sj.ijo.0803277
Palomo-Garo, C., Gómez-Gálvez, Y., García, C., and Fernández-Ruiz, J. (2016). Targeting the cannabinoid CB 2 receptor to attenuate the progression of motor deficits in LRRK2-transgenic mice. Pharmacol. Res. 110, 181–192. doi: 10.1016/j.phrs.2016.04.004
Pradhan, G., Samson, S. L., and Sun, Y. (2013). Ghrelin: much more than a hunger hormone. Curr. Opin. Clin. Nutr. Metab. Care 16, 619–624. doi: 10.1097/MCO.0b013e328365b9be
Przybyla, J. A., and Watts, V. J. (2010). Ligand-induced regulation and localization of cannabinoid CB1 and dopamine D2L receptor heterodimers. J. Pharmacol. Exp. Ther. 332, 710–719. doi: 10.1124/jpet.109.162701
Sagredo, O., García-Arencibia, M., de Lago, E., Finetti, S., Decio, A., and Fernández-Ruiz, J. (2007). Cannabinoids and neuroprotection in basal ganglia disorders. Mol. Neurobiol. 36, 82–91. doi: 10.1007/s12035-007-0004-3
Sam, A. H., Salem, V., and Ghatei, M. A. (2011). Rimonabant: from RIO to ban. J. Obes. 2011:432607. doi: 10.1155/2011/432607
Schellekens, H., Dinan, T. G., and Cryan, J. F. (2010). Lean mean fat reducing “ghrelin” machine: hypothalamic ghrelin and ghrelin receptors as therapeutic targets in obesity. Neuropharmacology 58, 2–16. doi: 10.1016/j.neuropharm.2009.06.024
Simon, V., and Cota, D. (2017). Mechanisms in endocrinology: endocannabinoids and metabolism: past, present, and future. Eur. J. Endocrinol. 176, R309–R324. doi: 10.1530/EJE-16-1044
Tudge, L., Williams, C., Cowen, P. J., and McCabe, C. (2015). Neural effects of cannabinoid CB1 neutral antagonist tetrahydrocannabivarin on food reward and aversion in healthy volunteers. Int. J. Neuropsychopharmacol. 18:pyu094. doi: 10.1093/ijnp/pyu094
Valdeolivas, S., Sagredo, O., Delgado, M., Pozo, M., and Fernández-Ruiz, J. (2017). Effects of a sativex-like combination of phytocannabinoids on disease progression in R6/2 mice, an experimental model of Huntington’s disease. Int. J. Mol. Sci. 18:684. doi: 10.3390/ijms18040684
Keywords: CB1 cannabinoid receptor, hunger hormone, marihuana consumption, orexigenic, obesity, addiction, ghrelin receptor (GHS-R1a), cannabinoids
Citation: Lillo A, Lillo J, Raïch I, Miralpeix C, Dosrius F, Franco R and Navarro G (2021) Ghrelin and Cannabinoid Functional Interactions Mediated by Ghrelin/CB1 Receptor Heteromers That Are Upregulated in the Striatum From Offspring of Mice Under a High-Fat Diet. Front. Cell. Neurosci. 15:786597. doi: 10.3389/fncel.2021.786597
Received: 30 September 2021; Accepted: 08 November 2021;
Published: 09 December 2021.
Edited by:
Yamina Berchiche, Dr. GPCR, United StatesReviewed by:
Serge N. Schiffmann, Université libre de Bruxelles, BelgiumRoberto Coccurello, National Research Council (CNR), Italy
Copyright © 2021 Lillo, Lillo, Raïch, Miralpeix, Dosrius, Franco and Navarro. This is an open-access article distributed under the terms of the Creative Commons Attribution License (CC BY). The use, distribution or reproduction in other forums is permitted, provided the original author(s) and the copyright owner(s) are credited and that the original publication in this journal is cited, in accordance with accepted academic practice. No use, distribution or reproduction is permitted which does not comply with these terms.
*Correspondence: Rafael Franco, cmZyYW5jbzEyM0BnbWFpbC5jb20=; Gemma Navarro, ZGltYXJ0dHNAaG90bWFpbC5jb20=
† These authors have contributed equally to this work