- 1Tissue Engineering, Regenerative Medicine and Immunoengineering Laboratory, Institute of Electronic Structure and Laser, Foundation for Research and Technology-Hellas (IESL-FORTH), Heraklion, Greece
- 2Ultrafast Laser Micro and Nano Processing Laboratory, Institute of Electronic Structure and Laser, Foundation for Research and Technology-Hellas (IESL-FORTH), Heraklion, Greece
- 3Department of Materials Science and Technology, University of Crete, Heraklion, Greece
Schwann cells (SCs), the glial cells of the peripheral nervous system (PNS), do not only form myelin sheaths thereby insulating the electrical signal propagated by the axons, but also play an essential role in the regeneration of injured axons. SCs are inextricably connected with their extracellular environment and the mechanical stimuli that are received determine their response during development, myelination and injuries. To this end, the mechanobiological response of SCs is being actively researched, as it can determine the suitability of fabricated scaffolds for tissue engineering and regenerative medicine applications. There is growing evidence that SCs are sensitive to changes in the mechanical properties of the surrounding environment (such as the type of material, its elasticity and stiffness), different topographical features provided by the environment, as well as shear stress. In this review, we explore how different mechanical stimuli affect SC behaviour and highlight the importance of exploring many different avenues when designing scaffolds for the repair of PNS injuries.
Introduction
The nervous system of vertebrates consists of the central nervous system (CNS) and the peripheral nervous system (PNS). Both are made up of neurons and glial cells, with neurons being responsible for receiving and transmitting electrical and chemical signals and glial cells providing the necessary support and protection for neurons. In the PNS, the glial cells are known as Schwann cells (SCs) and are responsible for the creation of myelin sheaths that protect and insulate the axons of peripheral neurons (Belin et al., 2017). They are also essential and indispensable for axon regeneration in the event of injury (Rosso et al., 2017) and as such, their role and function are under much investigation.
One of the main differences between the CNS and PNS is their capacity for regeneration. Injuries in the PNS are more easily repaired, as broken myelin sheaths can be phagocytosed and removed from the area of injury (Yao and Priyadarshani, 2018), while in the CNS, there are a number of limiting factors—including glial scar formation and the presence of inhibitory molecules—that make regeneration next to impossible (Huebner and Strittmatter, 2009). In the case of the PNS, when an injury is quite small, it can be repaired by suturing the two ends of the severed nerve together. Autografts have been considered the standard method of countering PNS injuries with slightly larger gap lengths, while commercially available nerve guides have been successful for nerve gaps of 20 mm or more (Bell and Haycock, 2012). However, despite the fact that there are treatments available for PNS injuries, there are still factors that limit their application, such as donor nerve shortage or immunological issues, and they have displayed moderate success (Millesi and Tsolakidis, 2005; Vindigni et al., 2009; Mahumane et al., 2018). As a result, there is a constant ongoing effort to find alternative treatment options by utilising the principles of tissue engineering.
Neural tissue engineering takes advantage of a large number of different biofabrication techniques, as well as biomaterials in order to create three-dimensional scaffolds and structures that can be used to facilitate regeneration (Boni et al., 2018; Papadimitriou et al., 2020; Doblado et al., 2021; Scaccini et al., 2021). The aim when creating scaffolds for such purposes is to mimic the physiological environment as closely as possible and provide the necessary cues to promote repair while ensuring that no localised toxicity or immune reaction is induced (Crupi et al., 2015; Doblado et al., 2021). It is well known that SCs are inextricably connected to their extracellular environment (Rosso et al., 2017). Characteristics of their physiological microenvironment include—but are not limited to—specific mechanical properties (such as elasticity and stiffness), different topographical features, as well as shear stress. All these factors are taken into account when designing scaffolds targeting PNS injuries, either individually or collectively.
Mechanobiology is related to how cells, tissues and organs sense the surrounding mechanical and physical signals and how are these signals converted into specific cellular responses such as adhesion, spreading, migration, gene expression, and cell-cell interactions in multiple cell types (Jansen et al., 2015; Belin et al., 2017). Mechanobiology relies on two main players within the cell: (i) mechanosensors, that allow cells to sense the mechanical signals provided by their environment; and; (ii) mechanotransducers, which enable cells to convert mechanical cues into biochemical signals. Various mechanosensors and mechanotransducers from both intra- and extracellular compartments have already been identified (Jansen et al., 2015). In the case of peripheral nerves and SCs, their identities are still emerging, with research focusing on how physical signals can be transmitted in SCs through the extracellular matrix (ECM), cell adhesion molecules (CAMs) and internal structures, such as the cortical cytoskeleton and the nucleus.
In this review, we explore how the different extracellular stimuli affect SC behaviour and highlight the importance of exploring many different avenues when designing scaffolds for the repair of PNS injuries.
The Effect of The Microenvironment on Schwann Cell Behaviour
The effect of mechanical properties
Schwann cells in peripheral nerves are physiologically exposed to mechanical stimuli such as shear and compressive and tensile stress, which can occur due to injuries or diseases, as well as during development and adulthood (Zhang et al., 2015; Belin et al., 2017). Peripheral nerves possess great elasticity in order to propagate action potentials throughout developmental growth (Simpson et al., 2013), mechanical compression and stretches while performing daily activities (Phillips et al., 2004). SCs are very sensitive to the surrounding stiffness and possess great plasticity. In case of injury of the peripheral nerves, myelinated SCs can dedifferentiate and guide the regeneration of peripheral axons (Jessen and Mirsky, 2016; Boerboom et al., 2017). Generally, in peripheral nerves, myelinated fibres are surrounded by 6–15 layers of connective tissue, which shield the SCs and the axons from mechanical forces originating from the external environment. It is known that the relative elasticity or stiffness of the peripheral nerves can affect the mechanical cues that the SCs are exposed to, while SC architecture and basal lamina integrity play key roles in the SC response to mechanical stress (Belin et al., 2017). In vitro studies have shown that mechanical stimulation at low levels may activate SC mitogenic pathways, independently of the regulation occurring between SCs and axons after axonal injury (Salzer and Bunge, 1980).
The mechanical properties of the ECM are defined mainly by elastin and collagen fibres that provide resilience (elasticity) and tensile strength. In peripheral nerves, there are more collagen fibres in comparison to elastin fibres (Sunderland, 1965), which contribute to the elastic properties of peripheral nerves. The main mechanotransducers that have been identified in SCs are responsible for the transmission of signals through the ECM and the SC basal side. Various studies report that SCs interact with axons on their apical side, where the biochemical signals from neurons are critical for SC migration, proliferation, survival, polarisation, differentiation, and gene expression (Monk et al., 2015; Salzer, 2015). SC function depends on the formation of adhesion complexes (through CAMs) between SCs with both axons and the ECM. All these stimuli are then transduced into biological responses, by YAP/TAZ, MRTF or LINC (Poitelon et al., 2016). In addition, forces such as mechanical compression can act on the actin cytoskeleton, leading to deformation of the nucleus and influencing chromatin organisation (Hernandez et al., 2016), while the nuclear envelope and the actin cytoskeleton work as mechanotransducers between the inner membrane of the cell and the nucleus (Plessner et al., 2015). Rosso et al. (2022) showed that the intrinsic physiological plasticity of SCs, which change their phenotype in response to physiological and patho-physiological changes in their microenvironment, in conjunction with their demonstrated mechanosensitivity, render them powerful targets for cell-based regenerative therapies.
In the field of peripheral nerve engineering, there is a wide range of biomaterials, both natural and synthetic, applied to the supporting cells involved in the repair process, such as neurons, SCs, macrophages, and blood vessels (Gregory and Phillips, 2021; Powell et al., 2021). Synthetic polymers are popular as their mechanical properties can be fine-tuned and they can be adapted to improve cell adhesion (Shahriari et al., 2017). Natural materials (typically derived from ECM components and found in hydrogel form) give the benefit of structural integrity for supporting regenerating axons, whilst maintaining the materials’ viscoelastic properties and their capability to further improve function (Bhatnagar et al., 2016). They can also be adapted to possess additional functionalities, such as controlled drug release to in situ gel formation. They can also be easily adjusted to fit defects with complicated geometries, such as that of the spinal cord. Multiple biomaterials such as chitosan (Li et al., 2014), collagen (Dalamagkas et al., 2016; Yeh et al., 2020), hyaluronic acid (Thomas et al., 2017), polycaprolactone (PCL; Chew et al., 2008; Mobasseri et al., 2014), poly (lactic acid; PLA; Miller et al., 2001; Mobasseri et al., 2014), poly (lactide-co-glycolide; PLGA; Babaliari et al., 2018a), and others (Gu et al., 2011; Bell and Haycock, 2012; Lotfi et al., 2019) have been assessed for scaffold fabrication. One of the most common materials used for hybrid polymeric conduits is gelatin/polycaprolactone (PCL), which has been shown to provide great support for in vitro neurite outgrowth and SC proliferation (Boni et al., 2018).
The surrounding ECM and microenvironment play a significant role in peripheral nerve tissue regeneration. Studies have shown that the regularity of a scaffold surface can guide migration and promote the maturation of SCs, and consequently direct the growth of dorsal root ganglion (DRG) neuritis (Ning et al., 2018; Petcu et al., 2018). In another study, Chen and co-workers evaluated the effects of cyclic tensile stimulation on the neural differentiation capabilities of human SCs. The auxetic hydrogels were found to withstand up to 20% tensile strain without tears, while only losing about 10% weight after being immersed for 14 days. The tensile forces were able to enhance cell viability and proliferation compared to static culture (Chen et al., 2020). Mechanical stimuli can also affect the remyelination of injured axons, with factors such as surface area, porosity, and surface structuring playing an important role in supporting and regulating SCs and directly influencing myelin-related gene expression (Liu et al., 2019; Park et al., 2021).
From the above, it can be seen that when it comes to assessing scaffold requirements for peripheral nerve tissue engineering, one must always take into account: (a) the static mechanical properties of the scaffolds whose aim is to imitate nerve tissue stiffness; (b) the dynamic nature of the nerve tissue; and (c) the time-varying mechanics relating to the stress gradient responses in the native tissue.
The effect of topography
The effect of topography —the surface spatial features of tissues or biomaterials—on SCs has attracted a lot of attention in the fields of neuron regeneration and regenerative medicine (Lotfi et al., 2019). Topographical cues in the micro-and nano- scale affect key SC responses such as migration, alignment, proliferation, and differentiation. The importance of these effects is highlighted by the fact that SCs provide structural support, remove debris, and direct axon regrowth in diseases associated with the PNS. Researchers attempt to mimic the natural environment of SCs to provide all the necessary cues that will enhance SC performance when developing strategies to counter neurodegenerative diseases, trauma, and disorders (Gu et al., 2011; Bell and Haycock, 2012; Lotfi et al., 2019). Fabrication of suitable scaffolds for SC seeding is gaining more popularity as a means to develop grafts that can be used as transplants for SC culture and neuron regeneration for in vivo trials (Gu et al., 2011; Deng et al., 2021).
Various topographical features have been investigated in literature (Lotfi et al., 2019). These features include but are not limited to, micro- and nano-scale surface topography and patterning (Miller et al., 2001; Schmalenberg and Uhrich, 2005; Li et al., 2014; Yiannakou et al., 2017; Angelaki et al., 2020), cell imprinted topography (Moosazadeh Moghaddam et al., 2019), scaffold geometry (grooves, filaments, wells, pillars etc.; Schmalenberg and Uhrich, 2005; Chew et al., 2008; Mitchel and Hoffman-Kim, 2011; Mobasseri et al., 2014; Tonazzini et al., 2015; Chen et al., 2019), hydrophilicity (Mobasseri et al., 2014), and roughness (Simitzi et al., 2015; Babaliari et al., 2021; Huang et al., 2021) and porosity (Li et al., 2014). Moreover, topographical characteristics are not investigated in a qualitative manner exclusively. For instance, grooves of different dimensions and spacing have been reported to have different effects on SCs (Schmalenberg and Uhrich, 2005; Li et al., 2014; Tonazzini et al., 2015). It has been shown that surface coating further mimics the ECM, which in turn boosts SC performance in axon regeneration. In 2001, Miller et al. (2001) seeded SCs and DRGs on laminin-coated poly(D, L-lactic acid) micropatterned grooves with varied groove spacing and depth and reported the effects of topography alongside the benefit of SC presence as a biological cue for neurite outgrowth and alignment. Another study reports the use of laminin-coated poly (dimethylsiloxane; PDMS) grooves for SC alignment as a means of directing neuron regeneration (Schmalenberg and Uhrich, 2005). Mobasseri et al. (2014) developed PCL/PLA films as scaffolds and reported the combined effect of different groove geometries and shapes along with scaffold wettability on SC interactions. Ahmed and Brown have also reported differential SC responses such as adhesion, alignment and migration on fibronectin fibres by comparing different combinations of substrates of fibronectin, laminin, and poly (L-lysine) and highlighting the directed orientation and enhanced speed, cell extension and cell area SCs exhibited on fibronectin fibres compared to the respective responses on non-topographical substrates (Ahmed and Brown, 1999).
Although the importance of topography cannot be understated, it is more common to investigate the topographical effects alongside other stimuli, such as electrical conductivity, shear stress, and use of growth factors, and examine their synergistic or antagonistic effects on SC behaviour. For instance, Huang et al. (2021) have developed reduced graphene oxide electrospun fibres that exhibit electrical conductivity and are suitable for SC electrical stimulation (ES). In another study, SCs combined with glial-derived neurotrophic factor (GDNF) on laminin-coated filaments exhibited significant and unidirectional axonal growth in the graft environment, reducing the inflammatory response, astrogliosis and tissue damage compared to non-coated filaments or coated filaments lacking SCs/GDNF. This demonstrated that the combination of laminin-coating and the use of SCs/GDNF was key to the success of the approach (Deng et al., 2021).
Another point of interest pertains to studies that have focused on co-culturing neurons alongside SCs since the nervous system is very complicated and multiple parameters should be taken into account for the successful application of scaffolds as in vivo transplants (Angelaki et al., 2020; Kordas et al., 2022). One such study utilised a substrate with combined morphology including both micro-cones and nano-ripples for the co-culture of SCs and neuronal cells. The authors were able to show that SCs adhesion was affected by the underlying pattern, which in turn also influenced neuronal cell behaviour (Angelaki et al., 2020).
Additionally, the focus has been directed not only on morphology, directionality and axon regeneration, but also on other key responses such as remyelination, myelin-related gene, and neurotrophic factor regulation, and examination of markers of immature or aged SCs. For example, Chew et al. (2008) used electrospun fibrous scaffolds to study SC alignment and investigate the regulation of various selected genes, myelin-specific proteins and immature SC markers. Follow-up work also highlighted the importance of topography on the spatial organisation of SCs, which in turn influenced myelination as well as neurite alignment (Siddiqui et al., 2021). Another study also used electrospun scaffolds and investigated topographical effects on myelin-related genes such as myelin-associated glycoprotein (MAG) and myelin protein zero (P0) via qRT-PCR, enabling the creation of a gene expression profile during the myelination process on scaffolds with specific alignment (Radhakrishnan et al., 2015).
It is evident that topography is one of the major factors that influence SC behaviour and plays a pivotal role in nerve regeneration applications. Topography can influence major cell responses such as adhesion, migration, alignment, and proliferation, while also affecting the regulation of multiple genes related to myelination and neurodegenerative diseases. As such, it is essential that it is taken into account and integrated into potential solutions.
The effect of shear stress
When studying cell-material interactions, more often than not conventional cell culture techniques are used, which means that the cells are cultured in flasks, Petri dishes, and other surfaces under static conditions (Coluccio et al., 2019), where they also have limited cell-cell interactions. However, within a multicellular organism, cells interact with a number of materials with different mechanical properties and topographical characteristics and are surrounded by fluid and nutrients (Zhang and Van Noort, 2011; Babaliari et al., 2018b). In order to better understand biological problems, it is important to assess cellular behaviour under conditions that reflect more closely the in vivo conditions with cell-cell, cell-matrix, and cell-soluble factor interactions (Hui and Bhatia, 2007). To achieve a more realistic environment for biological research, microfluidic devices are routinely used, as they can offer precise control over the microenvironment that influences biochemical and mechanical factors in a cell and, thus, cell functionality, such as changes in the flow-induced shear stress, the pH or O2 levels (Zhang and Van Noort, 2011).
Although shear stress—the external force acting on an object or surface parallel to the slope or plane in which it lies—is a critical component of the natural environment for the regeneration of axons (Chafik et al., 2003), the use of such systems for the study of neuronal cell behaviour is not widespread and there are very few studies available. One such study investigated the use of different substrate coatings for the use of SCs in microfluidic culturing environments, as shear stress significantly affected the effectiveness of surface coatings (Chafik et al., 2003). Gupta and co-workers studied the effect of shear stress on SC proliferation and genetic expression profiles and were able to find that under constant laminar fluid flow SCs had increased proliferation rates but displayed downregulation of MAG and myelin basic protein (MBP; Gupta and Steward, 2003; Gupta et al., 2005). Babaliari et al. (2021) have studied the combined effect of shear stress and topography on SC behaviour under dynamic culture conditions attained via continuous flow. Experiments using a precise flow-controlled microfluidic system which incorporated laser-microstructured microgrooved polyethylene terephthalate (PET) substrates revealed that, depending on the relation of the direction of the flow with respect to the topographical features (parallel or perpendicular), wall shear stress gradients act in a synergistic or antagonistic manner to topography in promoting a guided morphological cell response (Babaliari et al., 2021).
It is thus evident that flow-induced shear stress also affects neuronal behaviour. As a result, the necessity of developing in vitro biomimetic cell culture systems simulating shear stress, together with the micro/nano topography of the in vivo environment is mandatory. By utilising such systems to study the mechanobiology of peripheral glial cells, there would be a great benefit for various applications, including the creation of autologous graft substitutes for nerve tissue regeneration.
Conclusion and Future Perspectives
Over the past decades, there has been great interest in trying to understand the function of SC cells in order to fully utilise their potential in tissue engineering. As with all cells, SC function is inextricably connected to the microenvironment and it must not be ignored when attempting to understand PNS injury and disease. In this review, it has been highlighted how different aspects of the microenvironment (mechanical properties, topography and shear stress) can influence SC behaviour (Figure 1). It must be noted that all of these properties are so interconnected that integral parts of the process can be missed when they are not all taken into account. Due to the complexity of the nervous system, it is more beneficial to assess and investigate all cues provided by the natural microenvironment and apply this knowledge to the fabrication of biomimetic scaffolds. By integrating different mechanobiological aspects into scaffold design, potential synergistic, or antagonistic effects that could affect scaffold performance can be investigated. In addition, by using a multifaceted approach in scaffold design, the immunogenicity can also be modulated. By selecting materials with appropriate chemical and mechanical characteristics, as well as carefully designing the 3D architecture of the scaffolds, the immune response could be controlled and thus, lead to the reduction of adverse effects, such as scar formation (Crupi et al., 2015; Andorko and Jewell, 2017). Scaffolds that combine such features are gaining more ground as candidate conduits for in vivo PNS regeneration (Bell and Haycock, 2012; Liu et al., 2021). Hence, by choosing the appropriate materials, as well as topographical, mechanical, and chemical properties for potential scaffolds, while also utilising stimuli such as electrical stimulation and shear stress, more comprehensive solutions can be offered, which will pave the way for future state-of-the-art scaffolds to counter injuries and neurodegenerative diseases (Tupone et al., 2021).
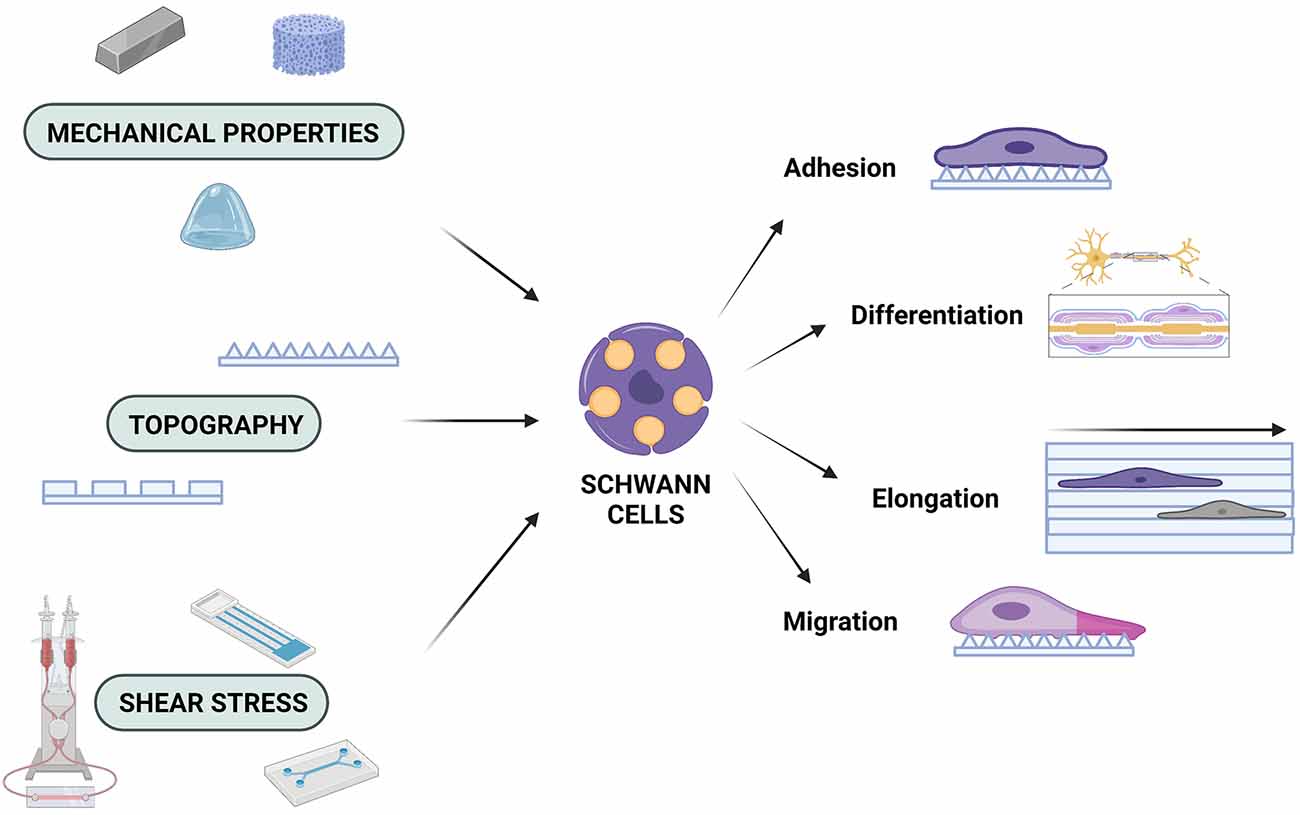
Figure 1. Mechanobiology and the Schwann cell response. SCs are affected by the mechanical properties of the engineered microenvironment, the underlying topography, and shear stress forces, which can determine their adhesion profiles, their ability to migrate and/or elongate, as well as their capacity to differentiate. Created with https://biorender.com.
Author Contributions
PM, PK, AK, and EB wrote the manuscript. PM created the figure. ES revised the manuscript. AR designed and revised the manuscript. All authors contributed to the article and approved the submitted version.
Funding
This work was funded by the projects “BioCombs4Nanofibers” (Horizon 2020 FET Open Programme—GA862016), “NeuroStimSpinal” (Horizon 2020 Research and Innovation Programme—GA829060), and by the Stavros Niarchos Foundation within the framework of the project ARCHERS (“Advancing Young Researchers’ Human Capital in Cutting Edge Technologies in the Preservation of Cultural Heritage and the Tackling of Societal Challenges”; Reference Number 2019_1193).
Conflict of Interest
The authors declare that the research was conducted in the absence of any commercial or financial relationships that could be construed as a potential conflict of interest.
Publisher’s Note
All claims expressed in this article are solely those of the authors and do not necessarily represent those of their affiliated organizations, or those of the publisher, the editors and the reviewers. Any product that may be evaluated in this article, or claim that may be made by its manufacturer, is not guaranteed or endorsed by the publisher.
References
Ahmed, Z., and Brown, R. A. (1999). Adhesion, alignment and migration of cultured Schwann cells on ultrathin fibronectin fibres. Cell Motil. Cytoskeleton 42, 331–343. doi: 10.1002/(SICI)1097-0169(1999)42:4<331::AID-CM6=3.0.CO;2-7
Andorko, J. I., and Jewell, C. M. (2017). Designing biomaterials with immunomodulatory properties for tissue engineering and regenerative medicine. Bioeng. Transl. Med. 2, 139–155. doi: 10.1002/btm2.10063
Angelaki, D., Kavatzikidou, P., Fotakis, C., Stratakis, E., and Ranella, A. (2020). Laser-induced topographies enable the spatial patterning of co-cultured peripheral nervous system cells. Mater. Sci. Eng. C Mater. Biol. Appl. 115:111144. doi: 10.1016/j.msec.2020.111144
Babaliari, E., Kavatzikidou, P., Angelaki, D., Chaniotaki, L., Manousaki, A., Siakouli-Galanopoulou, A., et al. (2018a). Engineering cell adhesion and orientation via ultrafast laser fabricated microstructured substrates. Int. J. Mol. Sci. 19:2053. doi: 10.3390/ijms19072053
Babaliari, E., Petekidis, G., and Chatzinikolaidou, M. (2018b). A precisely flow-controlled microfluidic system for enhanced pre-osteoblastic cell response for bone tissue engineering. Bioengineering (Basel) 5:66. doi: 10.3390/bioengineering5030066
Babaliari, E., Kavatzikidou, P., Mitraki, A., Papaharilaou, Y., Ranella, A., and Stratakis, E. (2021). Combined effect of shear stress and laser-patterned topography on Schwann cell outgrowth: synergistic or antagonistic? Biomater. Sci. 9, 1334–1344. doi: 10.1039/d0bm01218a
Belin, S., Zuloaga, K. L., and Poitelon, Y. (2017). Influence of mechanical stimuli on schwann cell biology. Front. Cell. Neurosci. 11:347. doi: 10.3389/fncel.2017.00347
Bell, J. H. A., and Haycock, J. W. (2012). Next generation nerve guides: materials, fabrication, growth factors and cell delivery. Tissue Eng. Part B Rev. 18, 116–128. doi: 10.1089/ten.TEB.2011.0498
Bhatnagar, D., Simon, M., and Rafailovich, M. H. (2016). “Hydrogels for Regenerative Medicine,” in Recent Advances in Biopolymers, ed F. K. Parveen (London: Intech Open), 105–123. doi: 10.5772/62044
Boerboom, A., Dion, V., Chariot, A., and Franzen, R. (2017). Molecular mechanisms involved in schwann cell plasticity. Front. Mol. Neurosci. 10:38. doi: 10.3389/fnmol.2017.00038
Boni, R., Ali, A., Shavandi, A., and Clarkson, A. N. (2018). Current and novel polymeric biomaterials for neural tissue engineering. J. Biomed. Sci. 25:90. doi: 10.1186/s12929-018-0491-8
Chafik, D., Bear, D., Bui, P., Patel, A., Jones, N. F., Kim, B. T., et al. (2003). Optimization of Schwann cell adhesion in response to shear stress in an in vitro model for peripheral nerve tissue engineering. Tissue Eng. 9, 233–241. doi: 10.1089/107632703764664701
Chen, S., Du, Z., Zou, J., Qiu, S., Rao, Z., Liu, S., et al. (2019). Promoting neurite growth and schwann cell migration by the harnessing decellularized nerve matrix onto nanofibrous guidance. ACS Appl. Mater. Interfaces 11, 17167–17176. doi: 10.1021/acsami.9b01066
Chen, Y. W., Wang, K., Ho, C. C., Kao, C. T., Ng, H. Y., and Shie, M. Y. (2020). Cyclic tensile stimulation enrichment of Schwann cell-laden auxetic hydrogel scaffolds towards peripheral nerve tissue engineering. Mater. Des. 195:108982. doi: 10.1016/j.matdes.2020.108982
Chew, S. Y., Mi, R., Hoke, A., and Leong, K. W. (2008). The effect of the alignment of electrospun fibrous scaffolds on Schwann cell maturation. Biomaterials 29, 653–661. doi: 10.1016/j.biomaterials.2007.10.025
Coluccio, M. L., Perozziello, G., Malara, N., Parrotta, E., Zhang, P., Gentile, F., et al. (2019). Microfluidic platforms for cell cultures and investigations. Microelectronic Eng. 208, 14–28. doi: 10.1016/j.mee.2019.01.004
Crupi, A., Costa, A., Tarnok, A., Melzer, S., and Teodori, L. (2015). Inflammation in tissue engineering: the Janus between engraftment and rejection. Eur. J. Immunol. 45, 3222–3236. doi: 10.1002/eji.201545818
Dalamagkas, K., Tsintou, M., and Seifalian, A. (2016). Advances in peripheral nervous system regenerative therapeutic strategies: a biomaterials approach. Mater. Sci. Eng. C Mater. Biol. Appl. 65, 425–432. doi: 10.1016/j.msec.2016.04.048
Deng, L. X., Liu, N. K., Wen, R., Yang, S. N., Wen, X., and Xu, X. M. (2021). Laminin-coated multifilament entubulation, combined with Schwann cells and glial cell line-derived neurotrophic factor, promotes unidirectional axonal regeneration in a rat model of thoracic spinal cord hemisection. Neural Regen. Res. 16, 186–191. doi: 10.4103/1673-5374.289436
Doblado, L. R., Martínez-Ramos, C., and Pradas, M. M. (2021). Biomaterials for neural tissue engineering. Front. Nanotechnol. 3:643507. doi: 10.3389/fnano.2021.643507
Gregory, H., and Phillips, J. B. (2021). Materials for peripheral nerve repair constructs: natural proteins or synthetic polymers? Neurochem. Int. 143:104953. doi: 10.1016/j.neuint.2020.104953
Gu, X., Ding, F., Yang, Y., and Liu, J. (2011). Construction of tissue engineered nerve grafts and their application in peripheral nerve regeneration. Prog. Neurobiol. 93, 204–230. doi: 10.1016/j.pneurobio.2010.11.002
Gupta, R., and Steward, O. (2003). Chronic nerve compression induces concurrent apoptosis and proliferation of Schwann cells. J. Comp. Neurol. 461, 174–186. doi: 10.1002/cne.10692
Gupta, R., Truong, L., Bear, D., Chafik, D., Modafferi, E., and Hung, C. T. (2005). Shear stress alters the expression of myelin-associated glycoprotein (MAG) and myelin basic protein (MBP) in Schwann cells. J. Orthop. Res. 23, 1232–1239. doi: 10.1016/j.orthres.2004.12.010
Hernandez, M., Patzig, J., Mayoral, S. R., Costa, K. D., Chan, J. R., and Casaccia, P. (2016). Mechanostimulation promotes nuclear and epigenetic changes in oligodendrocytes. J. Neurosci. 36, 806–813. doi: 10.1523/JNEUROSCI.2873-15.2016
Huang, Z., Sun, M., Li, Y., Guo, Z., and Li, H. (2021). Reduced graphene oxide-coated electrospun fibre: effect of orientation, coverage and electrical stimulation on Schwann cells behavior. J. Mater. Chem. B 9, 2656–2665. doi: 10.1039/d1tb00054c
Huebner, E. A., and Strittmatter, S. M. (2009). “Axon regeneration in the peripheral and central nervous systems,” in Cell Biology of the Axon. Results and Problems in Cell Differentiation, Vol. 48, ed E. Koening (Berlin, Heidelberg: Springer), 309–360.
Hui, E. E., and Bhatia, S. N. (2007). Micromechanical control of cell-cell interactions. Proc. Natl. Acad. Sci. U S A 104, 5722–5726. doi: 10.1073/pnas.0608660104
Jansen, K. A., Donato, D. M., Balcioglu, H. E., Schmidt, T., Danen, E. H. J., and Koenderink, G. H. (2015). A guide to mechanobiology: where biology and physics meet. Biochim. Biophys. Acta 1853, 3043–3052. doi: 10.1016/j.bbamcr.2015.05.007
Jessen, K. R., and Mirsky, R. (2016). The repair Schwann cell and its function in regenerating nerves. J. Physiol. 594, 3521–3531. doi: 10.1113/JP270874
Kordas, A., Manganas, P., Selimis, A., Barmparis, G. D., Farsari, M., and Ranella, A. (2022). Development of an oriented co-culture system using 3D scaffolds fabricated via non-linear lithography. Materials (Basel) 15:4349. doi: 10.3390/ma15124349
Li, G., Zhao, X., Zhao, W., Zhang, L., Wang, C., Jiang, M., et al. (2014). Porous chitosan scaffolds with surface micropatterning and inner porosity and their effects on Schwann cells. Biomaterials 35, 8503–8513. doi: 10.1016/j.biomaterials.2014.05.093
Liu, S., Niu, C., Xu, Z., Wang, Y., Liang, Y., Zhao, Y., et al. (2019). Modulation of myelin formation by combined high affinity with extracellular matrix structure of electrospun silk fibroin nanoscaffolds. J. Biomater. Sci. Polym. Ed. 30, 1068–1082. doi: 10.1080/09205063.2019.1621244
Liu, F., Xu, J., Wu, L., Zheng, T., Han, Q., Liang, Y., et al. (2021). The influence of the surface topographical cues of biomaterials on nerve cells in peripheral nerve regeneration: a review. Stem Cells Int. 2021:8124444. doi: 10.1155/2021/8124444
Lotfi, L., Khakbiz, M., Moosazadeh Moghaddam, M., and Bonakdar, S. (2019). A biomaterials approach to Schwann cell development in neural tissue engineering. J. Biomed. Mater. Res. A 107, 2425–2446. doi: 10.1002/jbm.a.36749
Mahumane, G. D., Kumar, P., du Toit, L. C., Choonara, Y. E., and Pillay, V. (2018). 3D scaffolds for brain tissue regeneration: architectural challenges. Biomater. Sci. 6, 2812–2837. doi: 10.1039/c8bm00422f
Miller, C., Jeftinija, S., and Mallapragada, S. (2001). Micropatterned Schwann cell-seeded biodegradable polymer substrates significantly enhance neurite alignment and outgrowth. Tissue Eng. 7, 705–715. doi: 10.1089/107632701753337663
Millesi, H., and Tsolakidis, S. (2005). End-to-side coaptation: an important tool in peripheral nerve surgery. Eur. Surg. 37, 228–233. doi: 10.1007/s10353-005-0171-2
Mitchel, J. A., and Hoffman-Kim, D. (2011). Cellular scale anisotropic topography guides Schwann cell motility. PLoS One 6:e24316. doi: 10.1371/journal.pone.0024316
Mobasseri, S. A., Terenghi, G., and Downes, S. (2014). Schwann cell interactions with polymer films are affected by groove geometry and film hydrophilicity. Biomed. Mater. 9:055004. doi: 10.1088/1748-6041/9/5/055004
Monk, K. R., Feltri, M. L., and Taveggia, C. (2015). New insights on Schwann cell development. Glia 63, 1376–1393. doi: 10.1002/glia.22852
Moosazadeh Moghaddam, M., Bonakdar, S., Shokrgozar, M. A., Zaminy, A., Vali, H., and Faghihi, S. (2019). Engineered substrates with imprinted cell-like topographies induce direct differentiation of adipose-derived mesenchymal stem cells into Schwann cells. Artif. Cells Nanomed. Biotechnol. 47, 1022–1035. doi: 10.1080/21691401.2019.1586718
Ning, L., Sun, H., Lelong, T., Guilloteau, R., Zhu, N., Schreyer, D. J., et al. (2018). 3D bioprinting of scaffolds with living Schwann cells for potential nerve tissue engineering applications. Biofabrication 10:035014. doi: 10.1088/1758-5090/aacd30
Papadimitriou, L., Manganas, P., Ranella, A., and Stratakis, E. (2020). Biofabrication for neural tissue engineering applications. Mater. Today Bio 6:100043. doi: 10.1016/j.mtbio.2020.100043
Park, S. E., Ahn, J., Jeong, H. E., Youn, I., Huh, D., and Chung, S. (2021). A three-dimensional in vitro model of the peripheral nervous system. NPG Asia Mater. 13:2. doi: 10.1038/s41427-020-00273-w
Petcu, E. B., Midha, R., McColl, E., Popa-Wagner, A., Chirila, T. V., and Dalton, P. D. (2018). 3D printing strategies for peripheral nerve regeneration. Biofabrication 10:032001. doi: 10.1088/1758-5090/aaaf50
Phillips, J. B., Smit, X., De Zoysa, N., Afoke, A., and Brown, R. A. (2004). Peripheral nerves in the rat exhibit localized heterogeneity of tensile properties during limb movement. J. Physiol. 557, 879–887. doi: 10.1113/jphysiol.2004.061804
Plessner, M., Melak, M., Chinchilla, P., Baarlink, C., and Grosse, R. (2015). Nuclear F-actin formation and reorganization upon cell spreading. J. Biol. Chem. 290, 11209–11216. doi: 10.1074/jbc.M114.627166
Poitelon, Y., Lopez-Anido, C., Catignas, K., Berti, C., Palmisano, M., Williamson, C., et al. (2016). YAP and TAZ control peripheral myelination and the expression of laminin receptors in Schwann cells. Nat. Neurosci. 19, 879–887. doi: 10.1038/nn.4316
Powell, R., Eleftheriadou, D., Kellaway, S., and Phillips, J. B. (2021). Natural biomaterials as instructive engineered microenvironments that direct cellular function in peripheral nerve tissue engineering. Front. Bioeng. Biotechnol. 9:674473. doi: 10.3389/fbioe.2021.674473
Radhakrishnan, J., Kuppuswamy, A. A., Sethuraman, S., and Subramanian, A. (2015). Topographic cue from electrospun scaffolds regulate myelin-related gene expressions in schwann cells. J. Biomed. Nanotechnol. 11, 512–521. doi: 10.1166/jbn.2015.1921
Rosso, G., Wehner, D., Schweitzer, C., Möllmert, S., Sock, E., Guck, J., et al. (2022). Matrix stiffness mechanosensing modulates the expression and distribution of transcription factors in Schwann cells. Bioeng. Transl. Med. 7:e10257. doi: 10.1002/btm2.10257
Rosso, G., Young, P., and Shahin, V. (2017). Implications of Schwann cells biomechanics and mechanosensitivity for peripheral nervous system physiology and pathophysiology. Front. Mol. Neurosci. 10:345. doi: 10.3389/fnmol.2017.00345
Salzer, J. L. (2015). Schwann cell myelination. Cold Spring Harb. Perspect. Biol. 7:a020529. doi: 10.1101/cshperspect.a020529
Salzer, J. L., and Bunge, R. P. (1980). Studies of Schwann cell proliferation. I. An analysis in tissue culture of proliferation during development, Wallerian degeneration and direct injury. J. Cell Biol. 84, 739–752. doi: 10.1083/jcb.84.3.739
Scaccini, L., Mezzena, R., De Masi, A., Gagliardi, M., Gambarotta, G., Cecchini, M., et al. (2021). Chitosan micro-grooved membranes with increased asymmetry for the improvement of the Schwann cell response in nerve regeneration. Int. J. Mol. Sci. 22:7901. doi: 10.3390/ijms22157901
Schmalenberg, K. E., and Uhrich, K. E. (2005). Micropatterned polymer substrates control alignment of proliferating Schwann cells to direct neuronal regeneration. Biomaterials 26, 1423–1430. doi: 10.1016/j.biomaterials.2004.04.046
Shahriari, D., Shibayama, M., Lynam, D. A., Wolf, K. J., Kubota, G., Koffler, J. Y., et al. (2017). Peripheral nerve growth within a hydrogel microchannel scaffold supported by a kink-resistant conduit. J. Biomed. Mater. Res. A 105, 3392–3399. doi: 10.1002/jbm.a.36186
Siddiqui, A. M., Brunner, R., Harris, G. M., Miller, A. L., Waletzki, B. E., Schmeichel, A. M., et al. (2021). Promoting neuronal outgrowth using ridged scaffolds coated with extracellular matrix proteins. Biomedicines 9:479. doi: 10.3390/biomedicines9050479
Simitzi, C., Efstathopoulos, P., Kourgiantaki, A., Ranella, A., Charalampopoulos, I., Fotakis, C., et al. (2015). Laser fabricated discontinuous anisotropic microconical substrates as a new model scaffold to control the directionality of neuronal network outgrowth. Biomaterials 67, 115–128. doi: 10.1016/j.biomaterials.2015.07.008
Simpson, A. H., Gillingwater, T. H., Anderson, H., Cottrell, D., Sherman, D. L., Ribchester, R. R., et al. (2013). Effect of limb lengthening on internodal length and conduction velocity of peripheral nerve. J. Neurosci. 33, 4536–4539. doi: 10.1523/JNEUROSCI.4176-12.2013
Sunderland, S. (1965). The connective tissues of peripheral nerves. Brain 88, 841–854. doi: 10.1093/brain/88.4.841
Thomas, R. C., Vu, P., Modi, S. P., Chung, P. E., Landis, R. C., Khaing, Z. Z., et al. (2017). Sacrificial crystal templated hyaluronic acid hydrogels As biomimetic 3D tissue scaffolds for nerve tissue regeneration. ACS Biomater. Sci. Eng. 3, 1451–1459. doi: 10.1021/acsbiomaterials.7b00002
Tonazzini, I., Jacchetti, E., Meucci, S., Beltram, F., and Cecchini, M. (2015). Schwann cell contact guidance versus boundary -interaction in functional wound healing along nano and microstructured membranes. Adv. Healthc. Mater. 4, 1849–1860. doi: 10.1002/adhm.201500268
Tupone, M. G., d’Angelo, M., Castelli, V., Catanesi, M., Benedetti, E., and Cimini, A. (2021). A state-of-the-art of functional scaffolds for 3D nervous tissue regeneration. Front. Bioeng. Biotechnol. 9:639765. doi: 10.3389/fbioe.2021.639765
Vindigni, V., Cortivo, R., Iacobellis, L., Abatangelo, G., and Zavan, B. (2009). Hyaluronan benzyl ester as a scaffold for tissue engineering. Int. J. Mol. Sci. 10, 2972–2985. doi: 10.3390/ijms10072972
Yao, L., and Priyadarshani, P. (2018). “Application of Schwann cells in neural tissue engineering,” in Glial Cell Engineering in Neural Regeneration, (Cham: Springer), 37–57. doi: 10.1007/978-3-030-02104-7_3
Yeh, J. Z., Wang, D. H., Cherng, J. H., Wang, Y. W., Fan, G. Y., Liou, N. H., et al. (2020). A collagen-based scaffold for promoting neural plasticity in a rat model of spinal cord injury. Polymers (Basel). 12:2245. doi: 10.3390/polym12102245
Yiannakou, C., Simitzi, C., Manousaki, A., Fotakis, C., Ranella, A., and Stratakis, E. (2017). Cell patterning via laser micro/nano structured silicon surfaces. Biofabrication 9:025024. doi: 10.1088/1758-5090/aa71c6
Zhang, C., and Van Noort, D. (2011). Cells in microfluidics. Top. Curr. Chem. 304, 295–321. doi: 10.1007/128_2011_147
Keywords: mechanobiology, Schwann cells, neural tissue engineering, mechanical properties, topography, shear stress
Citation: Manganas P, Kavatzikidou P, Kordas A, Babaliari E, Stratakis E and Ranella A (2022) The role of mechanobiology on the Schwann cells response: A tissue engineering perspective. Front. Cell. Neurosci. 16:948454. doi: 10.3389/fncel.2022.948454
Received: 19 May 2022; Accepted: 14 July 2022;
Published: 10 August 2022.
Edited by:
Ji-Fan Hu, Jilin University, ChinaReviewed by:
Gonzalo Rosso, Max Planck Institute for the Science of Light, GermanyLaura Fontenas, University of Virginia, United States
Copyright © 2022 Manganas, Kavatzikidou, Kordas, Babaliari, Stratakis and Ranella. This is an open-access article distributed under the terms of the Creative Commons Attribution License (CC BY). The use, distribution or reproduction in other forums is permitted, provided the original author(s) and the copyright owner(s) are credited and that the original publication in this journal is cited, in accordance with accepted academic practice. No use, distribution or reproduction is permitted which does not comply with these terms.
*Correspondence: Anthi Ranella, cmFudGhpQGllc2wuZm9ydGguZ3I=