- 1Brain Science Institute, Korea Institute of Science and Technology (KIST), Seoul, South Korea
- 2Division of Bio-Medical Science & Technology, KIST School, University of Science and Technology (UST), Seoul, South Korea
- 3School of Electrical Engineering, College of Engineering, Korea University, Seoul, South Korea
- 4KHU-KIST Department of Converging Science and Technology, Kyung Hee University, Seoul, South Korea
- 5Sensor System Research Center, Korea Institute of Science and Technology (KIST), Seoul, South Korea
Globally, it is estimated there are more than 2.2 billion visually impaired people. Visual diseases such as retinitis pigmentosa, age-related macular degeneration, glaucoma, and optic neuritis can cause irreversible profound vision loss. Many groups have investigated different approaches such as microelectronic prostheses, optogenetics, stem cell therapy, and gene therapy to restore vision. However, these methods have some limitations such as invasive implantation surgery and unknown long-term risk of genetic manipulation. In addition to the safety of ultrasound as a medical imaging modality, ultrasound stimulation can be a viable non-invasive alternative approach for the sight restoration because of its ability to non-invasively control neuronal activities. Indeed, recent studies have demonstrated ultrasound stimulation can successfully modulate retinal/brain neuronal activities without causing any damage to the nerve cells. Superior penetration depth and high spatial resolution of focused ultrasound can open a new avenue in neuromodulation researches. This review summarizes the latest research results about neural responses to ultrasound stimulation. Also, this work provides an overview of technical viewpoints in the future design of a miniaturized ultrasound transducer for a non-invasive acoustic visual prosthesis for non-surgical and painless restoration of vision.
Introduction
Permanent blindness can be caused by diverse visual diseases such as retinitis pigmentosa (Yue et al., 2016), age-related macular degeneration (Jackson et al., 2002), and optic neuritis (Toosy et al., 2014). Although these diseases are incurable (Lo et al., 2020), the implantation of visual prostheses is one of the available options for vision restoration (Shepherd et al., 2013; Fernandez, 2018; Im and Kim, 2020; Kim et al., 2022). Recently, electrical stimulation is used for the activation of neurons in the retina, optic nerve, lateral geniculate nucleus, and visual cortex. For example, Argus II (Humayun et al., 2009; Farvardin et al., 2018), Alpha IMA/AMS (Zrenner et al., 2011; Stingl et al., 2015), and Orion (Beauchamp et al., 2020) have been successfully implanted onto/underneath the human retina or at the visual cortex, respectively; and they have shown clinically promising results. However, there are still several challenges to be overcome for more useful visual prostheses. Those issues include slow perception of artificial vision, limited numbers of pixels (i.e., electrodes), invasiveness of microelectrodes, and so on.
Among those issues, the invasive implantation requires considerable surgical costs and may show surgical side effects. Many efforts have recently been made to implement non-invasive visual prostheses, including the investigation of optogenetics (Barrett et al., 2014), photoswitches (Tochitsky et al., 2016), and artificial opsins (Park et al., 2018; Berry et al., 2019). In the past decade, ultrasound-based neuromodulation in the brain and peripheral nervous system has gathered huge attention due to its non-invasiveness, deep penetration power through the skull with minimal neural tissue damage (Lee et al., 2016), and sub-mm focusing capability (Kim et al., 2021). Ultrasound has long been used for both diagnostic imaging (Wells, 2006) and therapeutic treatment (Steiss and McCauley, 2004) including cancer tissue destruction (Hsiao et al., 2016), proving its safety. In recent years, ultrasound stimulation technology (UST) has been used for neuromodulation of the nerve cells of the retina (Yue et al., 2016; Jiang et al., 2019; Lo et al., 2020) and of the visual cortex (Lee et al., 2016; Lu et al., 2021). The purpose of the present study is to overview recent studies regarding UST aiming for vision restoration as well as to discuss future perspectives on the development of acoustic visual prostheses.
Working principle of ultrasound stimulation
The application of ultrasound can make the following three physical events: (1) Increase in temperature, (2) Bubble formation and cavitation, and (3) Acoustic radiation force (Yoo et al., 2022). It has been known that neural activities can be induced by the aforementioned physical changes (O’Brien, 2007; Tyler et al., 2008; Plaksin et al., 2016). First, it had been demonstrated that high-intensity (>1 W/cm2) ultrasound can evoke action potentials in peripheral neuronal cells due to the increased excitability at higher temperature (Figure 1A1; Tsui et al., 2005; Yoo et al., 2022). Because too much heat damages tissue by protein denaturation and decreases synaptic transmission (Dalecki, 2004; O’Brien, 2007), low-intensity (<500 mW/cm2) ultrasound had been tested for neuromodulation without hyperthermia effect (Dalecki, 2004; O’Brien, 2007). Interestingly, the neural activities were also modulated even with a minimal temperature rise, making the thermal mechanism less convincing.
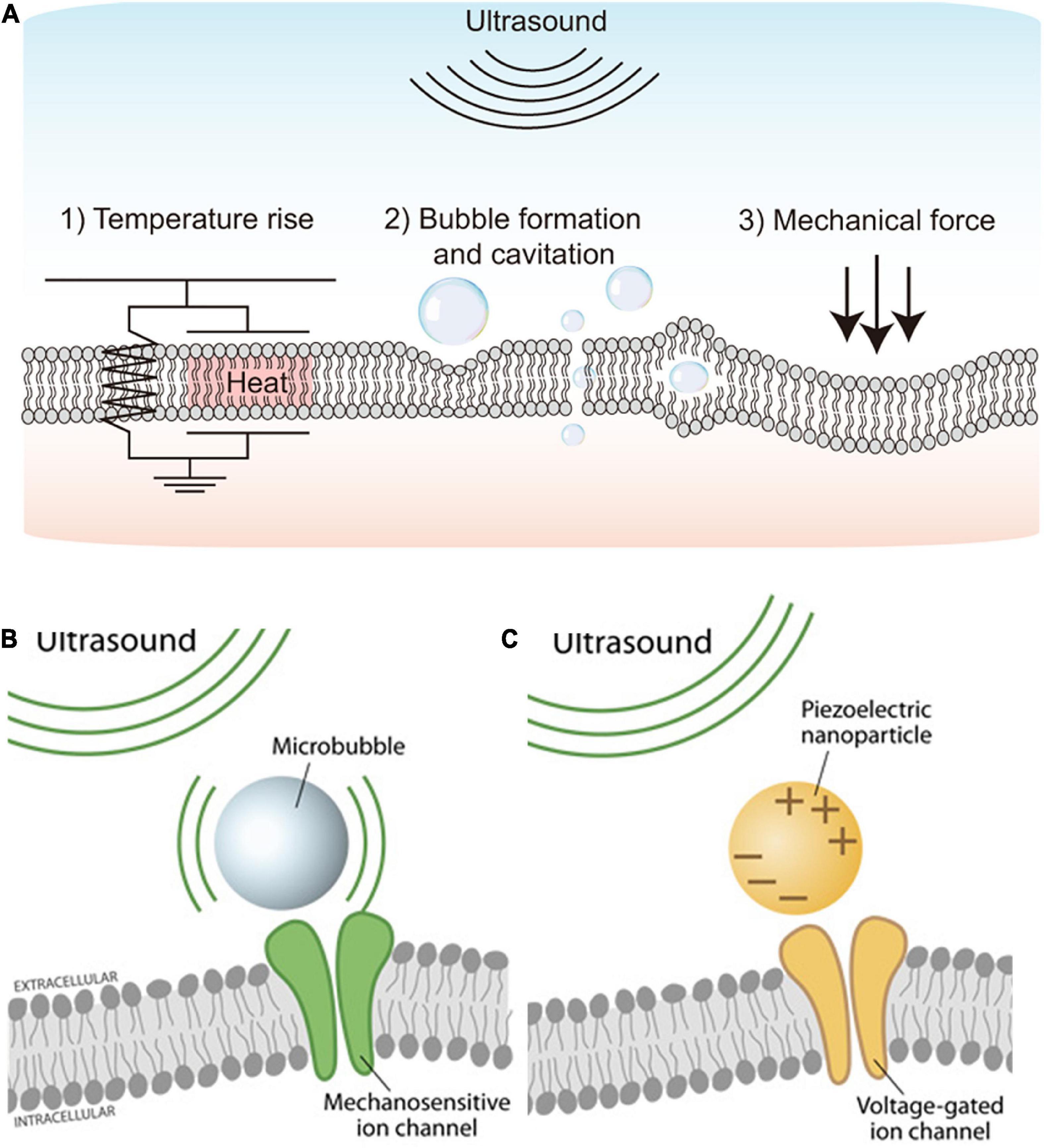
Figure 1. Schematics showing the ultrasound stimulation mechanism and ultrasound stimulation methods. (A) Physical mechanisms of ultrasound-based excitation on neurons. Biophysical effects of ultrasound such as (1) Temperature rise, (2) Bubble formation and cavitation, and (3) Mechanical force. Adapted from Yoo et al. (2022). (B) Microbubble-assisted ultrasound stimulation of mechanosensitive channels. Adapted from Rivnay et al. (2017). (C) Piezoelectric nanoparticle-assisted ultrasound stimulation of voltage-sensitive ion channel. Adapted from Rivnay et al. (2017).
Second, cavitation is a non-thermal effect caused by ultrasound (Figure 1A2), which directly modulates ion channels as well as the plasma membrane for neuromodulation (Fomenko et al., 2018). Cavitation makes gas bubbles forming, oscillating, and possibly collapsing within the tissue, resulting in stimulation of action potentials and synaptic transmission by deforming the bilayer lipid membrane (Krasovitski et al., 2011). The cavitation can happen by ultrasound waves at low frequency (1–3 MHz); however, acoustic pulses at higher frequencies (>4 MHz) are difficult to be used because oscillations in bubbles are difficult to be maintained during cavitation (Menz et al., 2019).
Lastly, acoustic radiation force is the most widely accepted potential physical mechanism of ultrasound-based neuromodulation (Figure 1A3). The mechanical force generated by steady acoustic pressure on the target neuron stretches the cell membrane and results in conformation and deformation of mechanosensitive ion channels in the cell membrane (Fomenko et al., 2018; Menz et al., 2019; Qian et al., 2022).
Mechanosensitive ion channels are transmembrane proteins that can detect and respond to mechanical stimuli; they can act as mechanosensitive nanovalve and provide a neuronal response to microbubbles generated by applied ultrasound (Figure 1B). Stretch-sensitive channels, displacement-sensitive channels, and shear stress-sensitive ion channels are commonly recognized as mechanosensitive ion channels (Morris, 1990). When the ultrasound is applied, mechanosensitive proteins undergo a conformational change that stimulates ion channels (Johns, 2002; Sukharev and Corey, 2004). Several earlier studies have focused on the significance of various mechanosensitive ion channels in various types of neurons (Menz et al., 2017; Ye et al., 2018; Wang et al., 2020; Yoo et al., 2022). Ultrasound has recently been used to trigger mechanosensitive K+, Ca2+, and Na+ channels that are found in the retina and brain (Maingret et al., 1999; Kubanek et al., 2016; Sorum et al., 2021). Those mechanosensitive proteins include MEC-4 (Kubanek et al., 2018), TRPP1/2 (Duque et al., 2022), TRPV1 (Yang et al., 2020), Piezo 1 (Qiu et al., 2019a), MscL (Ye et al., 2018), TRAAK (Sorum et al., 2021), and the K2p family (Zhao et al., 2017). Although it has not been fully understood, those mechanosensitive components are believed to play a critical role in neuromodulation using UST (Ye et al., 2018). To improve cell-type specificity, sonogenetics is also recently introduced (Ibsen et al., 2015; Qiu et al., 2019b; Yoo et al., 2022). The sonogenetics approach is a combination of ultrasound-based neuromodulation with mechanosensitive channel proteins, which can add cell type-specificity to conventional UST.
In addition to the direct activation of mechanosensitive ion channels, voltage-gated ion channels can be indirectly activated by ultrasound stimulation. For example, piezoelectric nanoparticles stimulated by external ultrasound can generate electrical charges in the target tissues, activating voltage-gated ion channels for neuromodulation (Figure 1C). A previous study demonstrated piezoelectric stimulation induces Ca2+ influx that helps in neuronal stimulation (Marino et al., 2015). This indirect electrical stimulation assisted by piezoelectric nanoparticles can serve as nano-transducers at both tissue and cell levels (Marino et al., 2017; Rivnay et al., 2017; Cafarelli et al., 2021). Earlier studies have used boron nitride nanotubes (BNNTs), barium titanate nanoparticles (BTNPs), zinc oxide (ZnO) nanowires, and polyvinylidene fluoride-trifluoroethylene (PVDF- TrFE) as piezoelectric nanoparticles (Cafarelli et al., 2021). However, in vivo proof of the feasibility and efficacy of piezoelectric nanoparticles-based ultrasound therapy for vision restoration has yet to be demonstrated. Also, more in-depth studies may be required to explore any potential toxicity and long-term biocompatibilities of piezoelectric nanoparticles (Cafarelli et al., 2021). For instance, piezoelectric nanoparticles such as lead zirconate titanate (PZT) are less biocompatible due to the lead element but further, PZT was made more biocompatible by treating its surface with titanium (Sakai et al., 2006).
Ultrasound stimulation of retina and visual cortex
Nowadays, low-intensity focused ultrasound (LIFUS) becomes widely used as a non-thermal, non-invasive approach for generating neuromodulation toward vision restoration (Baek et al., 2017; Fomenko et al., 2018; Jiang et al., 2018, 2019; Yuan et al., 2019). It has been repeatedly demonstrated that FUS can effectively stimulate the neurons in vitro (Menz et al., 2017; Cafarelli et al., 2021), ex vivo (Blackmore et al., 2019; Menz et al., 2019; Sarica et al., 2022), and in vivo (Naor et al., 2012; Lee et al., 2015). For the vision restoration purpose, an acoustic retinal prosthesis (ARP) had been proposed for the first time by demonstrating a FUS neurostimulation of the retinal cells in anesthetized wild-type rats (Naor et al., 2012). The ARP consists of an ultrasound phased array and an external camera with an image processor, connected with the cornea via an acoustic coupling component, to transmit acoustic images onto the retina. Later, a high frequency (43 MHz) ultrasound was used for stimulation of the salamander retina which generates high spatiotemporal resolution and stable visual responses (Menz et al., 2013). The acoustic pulses focused on target cells produced spiking activities like both ON and OFF types of retinal ganglion cells (RGCs) with temporal precision similar to the visual responses. Their spatial resolution was ∼100 μm but higher frequency ultrasound is expected to achieve smaller activation; however, it may damage lens tissues by generating heat. In another study, the responsiveness of RGCs in the rodent retinas to low frequency (2.25 MHz) FUS was systematically investigated (Jiang et al., 2018). Recently, RGC activities were modulated with even lower US frequency (1 MHz) at low-intensity (0.5 W/cm2) (Zhuo et al., 2022). The responses of RGCs greatly varied within each cell type as a function of different ultrasound intensities, suggesting neurophysiological properties of RGCs plays an important role in ultrasound responses. Also, the responses to ultrasound stimulation were not the same as those to light stimulation, implying some limitations for high-quality artificial vision. In the work of Jiang et al. (2018), double burst responses to ultrasound stimulation were observed; the latencies of second bursts were comparable to those of the delayed bursts that have been observed in electrical responses (Im and Fried, 2015; Im et al., 2018; Lee and Im, 2019; Kang et al., 2021). This similar temporal property suggests both ultrasound and electric stimulation may share common RGC activation mechanism(s). More recently, in vivo stimulation of blind rats’ retina using a 3.1 MHz spherically focused single-element transducer was reported for the first time (Qian et al., 2022). The ultrasound stimulation of the retina in spatial resolution of 250 μm evoked neural signals in the visual cortex (Figure 2A).
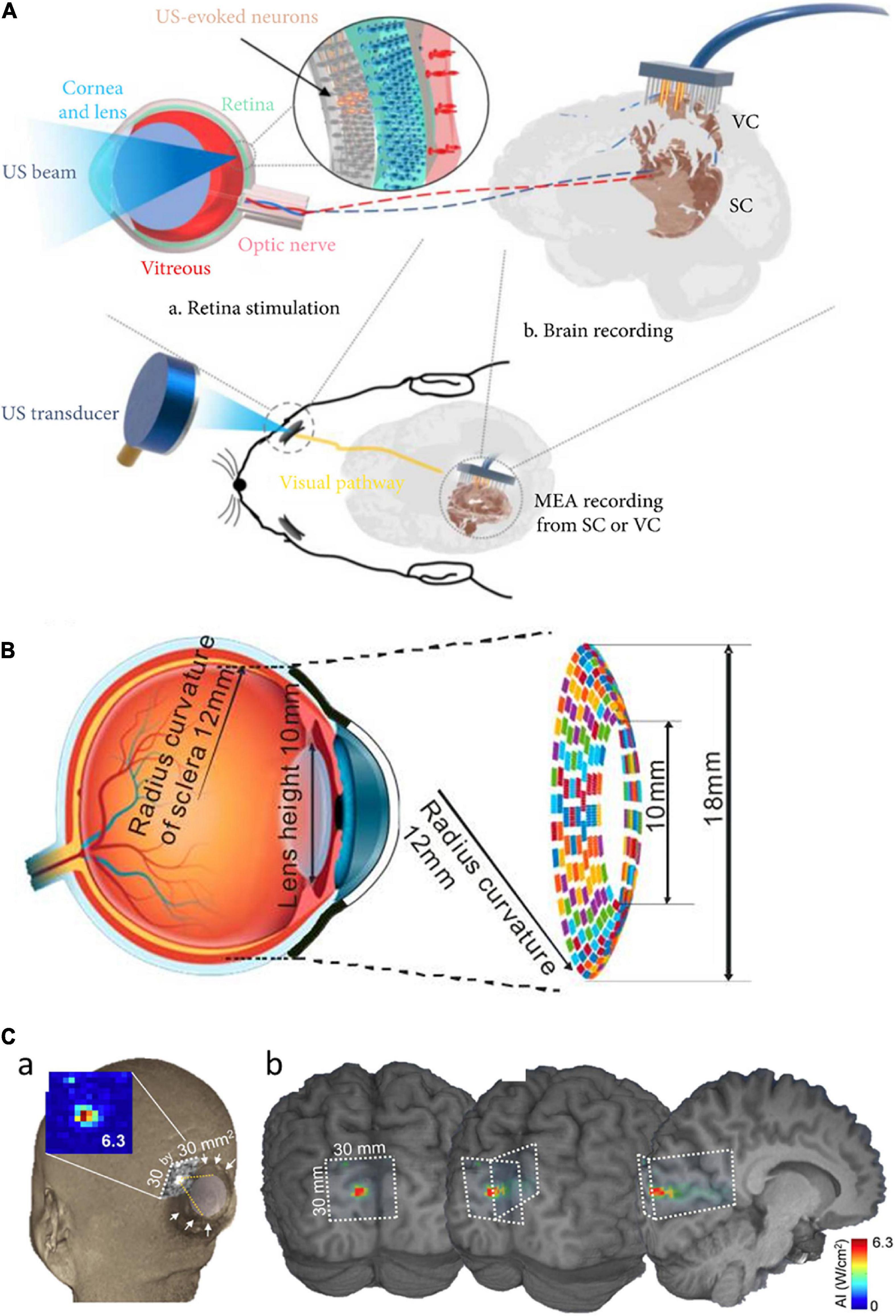
Figure 2. Focused ultrasound treatment for visual restoration. (A) Retinal neurons were excited by ultrasound waves which lead to the generation of the neural signal. These signals are transmitted to the brain via the optic nerve and the brain activity is recorded from the visual cortex or the superior colliculus. Adapted from Qian et al. (2022). (B) The circular racing array device for ultrasonic stimulation. Adapted from Yu et al. (2019). (C) In simulated acoustic intensity profiles, the acoustic focus was effectively projected to the targeted stimulatory site localized in the calcarine fissure (a), and acoustic energy was delivered to the visual cortex (b). Adapted from Lee et al. (2016).
Different areas of retinal tissue can be simultaneously stimulated using a multiple-focus ultrasound transducer array (Li et al., 2018). For easy implementation of the ARP, another study proposed a flexible wearable contact lens transducer array that covers the entire pupil, enabling multi-depth stimulation (Gao et al., 2017). The multi-focused phased array transducers can be worn like a contact lens to the external surface of the eyeball. In the simulation study, it has been estimated that acoustic stimulation at 2.5 MHz can stimulate multiple points in the retina with ∼1.3 mm lateral resolution (Gao et al., 2017). But, there was an issue limiting the application of this approach: the lens tissue in the eyeball absorbs ultrasound energy which further elevates the temperature at the treatment site that can be harmful to the eye. To solve this issue, a racing array transducer similar to a contact lens was proposed for the development of ultrasound retinal stimulation (Yu et al., 2019). The racing array transducer was composed of an array of transducer elements aligned on a concave surface and the center part of the transducer was hollow. In the racing array transducer approach (Figure 2B), the ultrasound absorption in the lens was minimized by directly applying the ultrasound to retina tissue without passing through the lens tissue, thereby avoiding retinal damage due to the potential heating. For a wide coverage of the visual field, a large flexible 2D matrix form of a capacitive micromachined ultrasonic transducer (CMUT) array can be one of the promising candidate systems (Tyler et al., 2018). The CMUT also has advantages of high temporal resolution, reduced size of the focal spot sidelobes, as well as easy monolithic integration with microelectronics.
Ultrasound stimulation technology can be applied to not only the retina but also the visual cortex (Ghezzi, 2015; Kim et al., 2015; Naor et al., 2016; Lu et al., 2021). For instance, ultrasound was successfully applied transcranially to the primary visual cortex of sheep, generating electroencephalographic potentials associated with ultrasound stimulation (Lee et al., 2016). The transcranial focused ultrasound (tFUS) approach is known to be safe and effective for transient neuromodulation (Di Biase et al., 2019). Low-intensity tFUS has elicited blood-oxygen-level-dependent (BOLD) responses in the human primary visual cortex and associated visual areas in the visual cortex, which was correlated with the perception of phosphenes (Lee et al., 2016). The on-site acoustic intensity and spatial resolution of the ultrasonic treatment were estimated using a retrospective numerical simulation of acoustic wave propagation through the skull (Figure 2C). While ultrasound has to penetrate the porous skull in the case of the cortical stimulation, the ultrasound wave for the retinal stimulation passes through a clear homogenous soft medium such as aqueous humor. This technically means that the retinal stimulation has benefits to use the non-invasiveness because the ultrasound energy is less attenuated and the pathway of the ultrasound wave can be well predictable to make higher spatiotemporal focal spots. In contrast, the stimulation of intact visual cortex may have advantages over the stimulation of degenerate retina which is known to have significant remodeling (Jones et al., 2016).
In previous studies, only normal animals and humans with no vision impairment were evaluated (Legon et al., 2018; Di Biase et al., 2019). It was quite recent that tFUS stimulation was tested to evoke neuronal activities of the visual cortices of both normal and blind rats (Lu et al., 2021). Another study compared responses to ultrasound stimulation using both Long Evans (LE) and Royal College of Surgeon (RCS) rats (Qian et al., 2022). Intriguingly, RCS (blind) rats showed much bigger response onset latencies and considerably stronger responses than those of LE (normally sighted) rats, probably due to prolonged visual deprivation.
Although tFUS has been used to modulate neuronal activity deep in the brain (Tufail et al., 2011; Legon et al., 2014, 2018; Di Biase et al., 2019; Lu et al., 2021), these approaches have some limitations such as restricted to low-frequency stimulation, low spatial resolution (>3 mm), and no cell-type selectivity. To overcome these issues, sonogenetics approach was explored (Ibsen et al., 2015). For example, a recent sonogenetics study expressed mechanosensitive ion channels (MscL) in the primary visual cortex of rodents; they achieved high spatial (∼100 μm) and temporal (<50 ms) resolution (Cadoni et al., 2021). It has been known that the activation of 100 μm area in diameter would result in the restored visual acuity of 20/400 (Palanker et al., 2020). The increased sensitivity of neuronal cells to UST was observed by heterogeneously expressed MscL, which increases Ca2+ influx (Cadoni et al., 2021). Considering these results, the sonogenetic strategy may open a new door for the application of engineered mechanosensitive channels for vision restoration in blind patients.
Issues to be addressed for the development of successful acoustic visual prostheses
Retinal UST studies (Naor et al., 2012; Qian et al., 2022) showed a spatial resolution similar to that of the Argus II (electrical stimulation system), the first and only FDA approved retinal prostheses. Despite recent promising results of the ultrasound in vision restoration studies (Menz et al., 2017; Lu et al., 2021; Rousou et al., 2021), several issues need to be addressed further for the clinical success of acoustic visual prostheses. First, the mechanism of neuromodulation of ultrasound stimulation should be more comprehensively understood. In particular, it is unclear whether the neuromodulation effect of ultrasound is universal or limited to specific cell types as well as whether the cellular compartment responding to ultrasound is existing in the degenerate retinas (Lo et al., 2020). If the underlying mechanism is revealed, the ultrasound stimulation conditions (e.g., frequency, therapy duration, duty cycle, and intensity) can be further optimized to efficiently modulate neuronal activities and/or enhance spatiotemporal resolution. For example, it has been known that the physical effect varies with changes in acoustic frequency: cavitation decreases with increasing frequency, and acoustic radiation force increases with increasing frequency (Fan et al., 2021).
Second, additional studies are essential to determine the long-term reliability and/or safety of repetitive application of ultrasonic stimulation. To date, it has been reported that repeated application of ultrasound for 36–48 h did not alter fine membrane structures (Tyler et al., 2008). However, according to the FDA safety guidelines, the acoustic intensity on the eye should be less than 50 mW/cm2, which is substantially lower than the safety threshold for other organs (720 mW/cm2) (Leary et al., 2008). Therefore, long-term mechanical damages due to continuous repetitive stimulation for dynamic artificial vision need to be additionally investigated. Also, the elevation of temperature can be unsafe for the eye. Typically, ultrasound transducers consume high power; therefore, future ultrasound-based visual prostheses must enable low-power neuromodulation not only for the light-weight batteries but also for less heat generation. Several recent attempts have provided some solutions for the power issue with the wireless energy transfer such as ultrasonic power delivery (Jiang et al., 2022).
Third, although all the parts of the LIFUS system can be mountable on the eye with wireless power transfer, further miniaturization of the whole UST system would be necessary because the size of the external stimulator is highly dependent on the stimulating power. For example, in the case of the high intensity focused ultrasound (HIFUS) system, which needs a high voltage power supply to generate high pressure from an ultrasonic transducer, additional electronics such as power amplifiers may hinder the implementation of the wearable device size. However, technological advances for increased energy efficiency in transducers, wireless power transfer, and batteries are all expected to not only reduce the size of external stimulators but also lengthen operating hours. Indeed, recent publications have demonstrated a CMUT array with monolithically integrated circuits can be chronically implantable even on a rat (Seok et al., 2021a,b). A 2D ultrasonic transducer array system would be preferred to create multi-focused patterns of activities rather than a single transducer with a raster scanning (Lo et al., 2020), which requires lots of extra bulky moving components.
Conclusion
Thanks to the non-invasiveness, UST has a potential to be a burgeoning field in neural prosthetics. Ultrasound approaches are still in their early stages, and an understanding of interaction with cells/tissues will be important for its successful advancement in the future. Both animal and human studies affirm ultrasound neuromodulation can be helpful for simple and non-invasive interference of impaired visual functions (Lee et al., 2016; Qian et al., 2022). Despite the promising feasibility results, many questions regarding the technological framework of the UST are still unanswered. Most importantly, the working principle behind the biological transduction of ultrasound is yet to be completely understood whether related to particular cell types. There are not many reports about in vivo demonstration of vision restoration. However, due to its non-invasiveness and potential for high-resolution stimulation, UST can be widely preferred. It is further expected that the use of engineered neuronal cells with mechanosensitive ion channels would provide high spatiotemporal resolution. Future research work in the abovementioned areas is needed to make the UST to be clinically applicable in blind patients.
Author contributions
JB and HR contributed to drafting the manuscript. MI conceived the study and revised the manuscript. BL and JK revised the manuscript. All authors read and approved the final manuscript.
Conflict of interest
The authors declare that the research was conducted in the absence of any commercial or financial relationships that could be construed as a potential conflict of interest.
Publisher’s note
All claims expressed in this article are solely those of the authors and do not necessarily represent those of their affiliated organizations, or those of the publisher, the editors and the reviewers. Any product that may be evaluated in this article, or claim that may be made by its manufacturer, is not guaranteed or endorsed by the publisher.
References
Baek, H., Pahk, K. J., and Kim, H. (2017). A review of low-intensity focused ultrasound for neuromodulation. Biomed. Eng. Lett. 7, 135–142. doi: 10.1007/s13534-016-0007-y
Barrett, J. M., Berlinguer-Palmini, R., and Degenaar, P. (2014). Optogenetic approaches to retinal prosthesis. Vis. Neurosci. 31, 345–354. doi: 10.1017/S0952523814000212
Beauchamp, M. S., Oswalt, D., Pouratian, N., Bosking, W. H., Correspondence, D. Y., Sun, P., et al. (2020). Dynamic stimulation of visual cortex produces form vision in sighted and blind humans. Cell 181, 774–783. doi: 10.1016/j.cell.2020.04.033
Berry, M. H., Holt, A., Salari, A., Veit, J., Visel, M., Levitz, J., et al. (2019). Restoration of high-sensitivity and adapting vision with a cone opsin. Nat. Commun. 10:1221. doi: 10.1038/s41467-019-09124-x
Blackmore, J., Shrivastava, S., Sallet, J., Butler, C. R., and Cleveland, R. O. (2019). Ultrasound neuromodulation: A review of results, mechanisms and safety. Ultrasound Med. Biol. 45, 1509–1536. doi: 10.1016/j.ultrasmedbio.2018.12.015
Cadoni, S., Demene, C., Provansal, M., Nguyen, D., Nelidova, D., Labernede, G., et al. (2021). Sonogenetic stimulation of the brain at a spatiotemporal resolution suitable for vision restoration. bioRxiv [Preprint]. doi: 10.1101/2021.11.07.467597
Cafarelli, A., Marino, A., Vannozzi, L., Puigmartí-Luis, J., Pané, S., Ciofani, G., et al. (2021). Piezoelectric nanomaterials activated by ultrasound: The pathway from discovery to future clinical adoption. ACS Nano 15, 11066–11086. doi: 10.1021/acsnano.1c03087
Dalecki, D. (2004). Mechanical bioeffects of ultrasound. Annu. Rev. Biomed. Eng. 6, 229–248. doi: 10.1146/annurev.bioeng.6.040803.140126
Di Biase, L., Falato, E., and Di Lazzaro, V. (2019). Transcranial focused ultrasound (tFUS) and transcranial unfocused ultrasound (tUS) neuromodulation: From theoretical principles to stimulation practices. Front. Neurol. 10:549. doi: 10.3389/fneur.2019.00549
Duque, M., Lee-Kubli, C. A., Tufail, Y., Magaram, U., Patel, J., Chakraborty, A., et al. (2022). Sonogenetic control of mammalian cells using exogenous transient receptor potential A1 channels. Nat. Commun. 13:600. doi: 10.1038/s41467-022-28205-y
Fan, H., Shimba, K., Ishijima, A., Sasaoka, K., Takahashi, T., Chang, C.-H., et al. (2021). Acoustic frequency-dependent physical mechanism of sub-MHz ultrasound neurostimulation. bioRxiv [Preprint]. doi: 10.1101/2021.09.11.458049
Farvardin, M., Afarid, M., Attarzadeh, A., Johari, M. K., Mehryar, M., Hossein Nowroozzadeh, M., et al. (2018). The Argus-II retinal prosthesis implantation; from the global to local successful experience. Front. Neurosci. 12:584. doi: 10.3389/fnins.2018.00584
Fernandez, E. (2018). Development of visual neuroprostheses: Trends and challenges. Bioelectron. Med. 4:12. doi: 10.1186/s42234-018-0013-8
Fomenko, A., Neudorfer, C., Dallapiazza, R. F., Kalia, S. K., and Lozano, A. M. (2018). Low-intensity ultrasound neuromodulation: An overview of mechanisms and emerging human applications. Brain Stimul. 11, 1209–1217. doi: 10.1016/j.brs.2018.08.013
Gao, M., Yu, Y., Zhao, H., Li, G., Jiang, H., Wang, C., et al. (2017). Simulation study of an ultrasound retinal prosthesis with a novel contact-lens array for noninvasive retinal stimulation. IEEE Trans. Neural Syst. Rehabil. Eng. 25, 1605–1611. doi: 10.1109/tnsre.2017.2682923
Ghezzi, D. (2015). Retinal prostheses: Progress towards the next generation implants. Front. Neurosci. 9:290. doi: 10.3389/fnins.2015.00290
Hsiao, Y. H., Kuo, S. J., Tsai, H. D., Chou, M. C., and Yeh, G. P. (2016). Clinical application of high-intensity focused ultrasound in cancer therapy. J. Cancer 7, 225–231. doi: 10.7150/jca.13906
Humayun, M. S., Dorn, J. D., Ahuja, A. K., Caspi, A., Filley, E., Dagnelie, G., et al. (2009). Preliminary 6 month results from the Argus II epiretinal prosthesis feasibility study. Annu. Int. Conf. IEEE Eng. Med. Biol. Soc. 2009, 4566–4568. doi: 10.1109/iembs.2009.5332695
Ibsen, S., Tong, A., Schutt, C., Esener, S., and Chalasani, S. H. (2015). Sonogenetics is a non-invasive approach to activating neurons in Caenorhabditis elegans. Nat. Commun. 6:8264. doi: 10.1038/ncomms9264
Im, M., and Fried, S. I. (2015). Indirect activation elicits strong correlations between light and electrical responses in ON but not OFF retinal ganglion cells. J. Physiol. 593, 3577–3596. doi: 10.1113/jp270606
Im, M., and Kim, S. W. (2020). Neurophysiological and medical considerations for better-performing microelectronic retinal prostheses. J. Neural Eng. 17:033001. doi: 10.1088/1741-2552/ab8ca9
Im, M., Werginz, P., and Fried, S. I. (2018). Electric stimulus duration alters network-mediated responses depending on retinal ganglion cell type. J. Neural Eng. 15:036010. doi: 10.1088/1741-2552/aaadc1
Jackson, G. R., Owsley, C., and Curcio, C. A. (2002). Photoreceptor degeneration and dysfunction in aging and age-related maculopathy. Ageing Res. Rev. 1, 381–396. doi: 10.1016/S1568-1637(02)00007-7
Jiang, L., Lu, G., Zeng, Y., Sun, Y., Kang, H., Burford, J., et al. (2022). Flexible ultrasound-induced retinal stimulating piezo-arrays for biomimetic visual prostheses. Nat. Commun. 13:3853. doi: 10.1038/s41467-022-31599-4
Jiang, Q., Li, G., Zhao, H., Sheng, W., Yue, L., Su, M., et al. (2018). Temporal neuromodulation of retinal ganglion cells by low-frequency focused ultrasound stimulation. IEEE Trans. Neural Syst. Rehabil. Eng. 26, 969–976. doi: 10.1109/TNSRE.2018.2821194
Jiang, X., Savchenko, O., Li, Y., Qi, S., Yang, T., Zhang, W., et al. (2019). A review of low-intensity pulsed ultrasound for therapeutic applications. IEEE Trans. Biomed. Eng. 66, 2704–2718. doi: 10.1109/TBME.2018.2889669
Johns, L. D. (2002). Nonthermal effects of therapeutic ultrasound: The frequency resonance hypothesis. J. Athl. Train. 37, 293–299.
Jones, B. W., Pfeiffer, R. L., Ferrell, W. D., Watt, C. B., Marmor, M., and Marc, R. E. (2016). Retinal remodeling in human retinitis pigmentosa. Exp. Eye Res. 150, 149–165. doi: 10.1016/j.exer.2016.03.018
Kang, J. H., Jang, Y. J., Kim, T., Lee, B. C., Lee, S. H., and Im, M. (2021). Electric stimulation elicits heterogeneous responses in on but not off retinal ganglion cells to transmit rich neural information. IEEE Trans. Neural Syst. Rehabil. Eng. 29, 300–309. doi: 10.1109/tnsre.2020.3048973
Kim, H., Park, M. Y., Lee, S. D., Lee, W., Chiu, A., and Yoo, S. S. (2015). Suppression of EEG visual-evoked potentials in rats through neuromodulatory focused ultrasound. Neuroreport 26, 211–215. doi: 10.1097/wnr.0000000000000330
Kim, S., Jo, Y., Kook, G., Pasquinelli, C., Kim, H., Kim, K., et al. (2021). Transcranial focused ultrasound stimulation with high spatial resolution. Brain Stimul. 14, 290–300. doi: 10.1016/j.brs.2021.01.002
Kim, S., Roh, H., and Im, M. (2022). Artificial visual information produced by retinal prostheses. Front. Cell. Neurosci. 16:911754. doi: 10.3389/fncel.2022.911754
Krasovitski, B., Frenkel, V., Shoham, S., and Kimmel, E. (2011). Intramembrane cavitation as a unifying mechanism for ultrasound-induced bioeffects. Proc. Natl. Acad. Sci. U.S.A. 108, 3258–3263. doi: 10.1073/PNAS.1015771108
Kubanek, J., Shi, J., Marsh, J., Chen, D., Deng, C., and Cui, J. (2016). Ultrasound modulates ion channel currents. Sci. Rep. 6:24170. doi: 10.1038/srep24170
Kubanek, J., Shukla, P., Das, A., Baccus, S. A., and Goodman, M. B. (2018). Ultrasound elicits behavioral responses through mechanical effects on neurons and ion channels in a simple nervous system. J. Neurosci. 38, 3081–3091. doi: 10.1523/jneurosci.1458-17.2018
Leary, Brendan, Vaezy, and Shahram. (2008). Marketing Clearance of Diagnostic Ultrasound Systems and Transducers Guidance for Industry and Food and Drug Administration Staff this Guidance Document Supersedes the Guidance Entitled “Information for Manufacturers Seeking Marketing Clearance of Diagnostic Ultrasound Systems and Transducers” dated Preface Public Comment. Available online at: https://www.regulations.gov (accessed on May 9, 2022).
Lee, J. I., and Im, M. (2019). Optimal electric stimulus amplitude improves the selectivity between responses of on versus off types of retinal ganglion cells. IEEE Trans. Neural Syst. Rehabil. Eng. 27, 2015–2024. doi: 10.1109/tnsre.2019.2939012
Lee, W., Kim, H., Jung, Y., Song, I. U., Chung, Y. A., and Yoo, S. S. (2015). Image-guided transcranial focused ultrasound stimulates human primary somatosensory cortex. Sci. Rep. 5:8743. doi: 10.1038/srep08743
Lee, W., Kim, H. C., Jung, Y., Chung, Y. A., Song, I. U., Lee, J. H., et al. (2016). Transcranial focused ultrasound stimulation of human primary visual cortex. Sci. Rep. 6:34020. doi: 10.1038/srep34026
Legon, W., Bansal, P., Tyshynsky, R., Ai, L., and Mueller, J. K. (2018). Transcranial focused ultrasound neuromodulation of the human primary motor cortex. Sci. Rep. 8:10007. doi: 10.1038/s41598-018-28320-1
Legon, W., Sato, T. F., Opitz, A., Mueller, J., Barbour, A., Williams, A., et al. (2014). Transcranial focused ultrasound modulates the activity of primary somatosensory cortex in humans. Nat. Neurosci. 17, 322–329. doi: 10.1038/nn.3620
Li, G., Qiu, W., Hong, J., Jiang, Q., Su, M., Mu, P., et al. (2018). Imaging-Guided Dual-Target Neuromodulation of the Mouse Brain Using Array Ultrasound. New York, N Y: IEEE. doi: 10.1109/tuffc.2018.2847252
Lo, P. A., Huang, K., Zhou, Q., Humayun, M. S., and Yue, L. (2020). Ultrasonic retinal neuromodulation and acoustic retinal prosthesis. Micromachines 11:929. doi: 10.3390/mi11100929
Lu, G., Qian, X., Castillo, J., Li, R., Jiang, L., Lu, H., et al. (2021). Transcranial focused ultrasound for noninvasive neuromodulation of the visual cortex. IEEE Trans. Ultrason. Ferroelectr. Freq. Control 68, 21–28. doi: 10.1109/tuffc.2020.3005670
Maingret, F., Fosset, M., Lesage, F., Lazdunski, M., and Honoré, E. (1999). TRAAK is a mammalian neuronal mechano-gated K+ channel. J. Biol. Chem. 274, 1381–1387. doi: 10.1074/JBC.274.3.1381
Marino, A., Arai, S., Hou, Y., Sinibaldi, E., Pellegrino, M., Chang, Y. T., et al. (2015). Piezoelectric nanoparticle-assisted wireless neuronal stimulation. ACS Nano 9, 7678–7689. doi: 10.1021/acsnano.5b03162
Marino, A., Genchi, G. G., Mattoli, V., and Ciofani, G. (2017). Piezoelectric nanotransducers: The future of neural stimulation. Nano Today 14, 9–12. doi: 10.1016/j.nantod.2016.12.005
Menz, M. D., Oralkan, Ö, Khuri-Yakub, P. T., and Baccus, S. A. (2013). Precise neural stimulation in the retina using focused ultrasound. J. Neurosci. 33, 4550–4560. doi: 10.1523/jneurosci.3521-12.2013
Menz, M. D., Ye, P., Firouzi, K., Nikoozadeh, A., Pauly, K. B., Khuri-Yakub, P., et al. (2019). Radiation force as a physical mechanism for ultrasonic neurostimulation of the ex vivo retina. J. Neurosci. 39, 6251–6264. doi: 10.1523/jneurosci.2394-18.2019
Menz, M. D., Ye, P., Firouzi, K., Pauly, K. B., Khuri-Yakub, B. T., and Baccus, S. A. (2017). Physical mechanisms of ultrasonic neurostimulation of the retina. bioRxiv [Preprint]. doi: 10.1101/231449
Morris, C. E. (1990). Mechanosensitive ion channels. J. Membr. Biol. 113, 93–107. doi: 10.1007/BF01872883
Naor, O., Hertzberg, Y., Zemel, E., Kimmel, E., and Shoham, S. (2012). Towards multifocal ultrasonic neural stimulation II: Design considerations for an acoustic retinal prosthesis. J. Neural Eng. 9:026006. doi: 10.1088/1741-2560/9/2/026006
Naor, O., Krupa, S., and Shoham, S. (2016). Ultrasonic neuromodulation. J. Neural Eng. 13:031003. doi: 10.1088/1741-2560/13/3/031003
O’Brien, W. D. (2007). Ultrasound-biophysics mechanisms. Prog. Biophys. Mol. Biol. 93, 212–255. doi: 10.1016/j.pbiomolbio.2006.07.010
Palanker, D., Le Mer, Y., Mohand-Said, S., Muqit, M., and Sahel, J. A. (2020). Photovoltaic restoration of central vision in atrophic age-related macular degeneration. Ophthalmol 127, 1097–1104. doi: 10.1016/j.ophtha.2020.02.024
Park, B., Yang, H., Ha, T. H., Park, H. S., Oh, S. J., Ryu, Y. S., et al. (2018). Artificial rod and cone photoreceptors with human-like spectral sensitivities. Adv. Mater. 30:e1706764. doi: 10.1002/adma.201706764
Plaksin, M., Kimmel, E., and Shoham, S. (2016). Cell-type-selective effects of intramembrane cavitation as a unifying theoretical framework for ultrasonic neuromodulation. eNeuro 3, 229–244. doi: 10.1523/eneuro.0136-15.2016
Qian, X., Lu, G., Thomas, B. B., Li, R., Chen, X., Shung, K. K., et al. (2022). Noninvasive ultrasound retinal stimulation for vision restoration at high spatiotemporal resolution. BME Front. 2022:9829316. doi: 10.34133/2022/9829316
Qiu, Z., Guo, J., Kala, S., Zhu, J., Xian, Q., Qiu, W., et al. (2019a). The mechanosensitive ion channel piezo1 significantly mediates in vitro ultrasonic stimulation of neurons. iScience 21, 448–457. doi: 10.1016/j.isci.2019.10.037
Qiu, Z., Kala, S., Guo, J., Xian, Q., Zhu, J., and Sun, L. (2019b). Non-invasive and selective brain stimulation by ultrasound via activation of mechanosensitive ion channels. Brain Stimul. 12:475. doi: 10.1016/j.brs.2018.12.549
Rivnay, J., Wang, H., Fenno, L., Deisseroth, K., and Malliaras, G. G. (2017). Next-generation probes, particles, and proteins for neural interfacing. Sci. Adv. 3:e1601649. doi: 10.1126/sciadv.1601649
Rousou, C., Schuurmans, C. C. L., Urtti, A., Mastrobattista, E., Storm, G., Moonen, C., et al. (2021). Ultrasound and microbubbles for the treatment of ocular diseases: From preclinical research towards clinical application. Pharmaceutics 13:1782. doi: 10.3390/pharmaceutics13111782
Sakai, T., Hoshiai, S., and Nakamachi, E. (2006). Biochemical compatibility of PZT piezoelectric ceramics covered with titanium thin film. J. Optoelectron. Adv. Mater. 8, 1435–1437.
Sarica, C., Fomenko, A., Nankoo, J. F., Darmani, G., Vetkas, A., Yamamoto, K., et al. (2022). Toward focused ultrasound neuromodulation in deep brain stimulator implanted patients: Ex-vivo thermal, kinetic and targeting feasibility assessment. Brain Stimul. 15, 376–379. doi: 10.1016/j.brs.2021.12.012
Seok, C., Yamaner, F. Y., Sahin, M., and Oralkan, Ö (2021a). A wearable ultrasonic neurostimulator - Part I: A 1D CMUT phased array system for chronic implantation in small animals. IEEE Trans. Biomed. Circuits Syst. 15, 692–704. doi: 10.1109/TBCAS.2021.3100458
Seok, C., Adelegan, O. J., Biliroglu, A. Ö, Yamaner, F. Y., and Oralkan, Ö (2021b). A wearable ultrasonic neurostimulator - Part II: A 2D CMUT phased array system with a flip-chip bonded ASIC. IEEE Trans. Biomed. Circuits Syst. 15, 705–718. doi: 10.1109/TBCAS.2021.3105064
Shepherd, R. K., Shivdasani, M. N., Nayagam, D. A. X., Williams, C. E., and Blamey, P. J. (2013). Visual prostheses for the blind. Trends Biotechnol. 31, 562–571. doi: 10.1016/j.tibtech.2013.07.001
Sorum, B., Rietmeijer, R. A., Gopakumar, K., Adesnik, H., and Brohawn, S. G. (2021). Ultrasound activates mechanosensitive TRAAK K+ channels through the lipid membrane. Proc. Natl. Acad. Sci. U.S.A. 118:e2006980118. doi: 10.1073/pnas.2006980118
Steiss, J. E., and McCauley, L. (2004). Therapeutic ultrasound. Canine Rehabil. Phys. Ther. 880, 324–336. doi: 10.1016/b978-0-7216-9555-6.50023-1
Stingl, K., Bartz-Schmidt, K. U., Besch, D., Chee, C. K., Cottriall, C. L., Gekeler, F., et al. (2015). Subretinal visual implant alpha ims–clinical trial interim report. Vision Res. 111, 149–160. doi: 10.1016/j.visres.2015.03.001
Sukharev, S., and Corey, D. P. (2004). Mechanosensitive channels: Multiplicity of families and gating paradigms. Sci. STKE 2004:re4. doi: 10.1126/stke.2192004re4
Tochitsky, I., Helft, Z., Meseguer, V., Fletcher, R. B., Vessey, K. A., Telias, M., et al. (2016). How azobenzene photoswitches restore visual responses to the blind retina. Neuron 92, 100–113. doi: 10.1016/j.neuron.2016.08.038
Toosy, A. T., Mason, D. F., and Miller, D. H. (2014). Optic neuritis. Lancet Neurol. 13, 83–99. doi: 10.1016/s1474-4422(13)70259-x
Tsui, P. H., Wang, S. H., and Huang, C. C. (2005). In vitro effects of ultrasound with different energies on the conduction properties of neural tissue. Ultrasonics 43, 560–565. doi: 10.1016/j.ultras.2004.12.003
Tufail, Y., Yoshihiro, A., Pati, S., Li, M. M., and Tyler, W. J. (2011). Ultrasonic neuromodulation by brain stimulation with transcranial ultrasound. Nat. Protoc. 6, 1453–1470. doi: 10.1038/nprot.2011.371
Tyler, W. J., Lani, S. W., and Hwang, G. M. (2018). Ultrasonic modulation of neural circuit activity. Curr. Opin. Neurobiol. 50, 222–231. doi: 10.1016/j.conb.2018.04.011
Tyler, W. J., Tufail, Y., Finsterwald, M., Tauchmann, M. L., Olson, E. J., and Majestic, C. (2008). Remote excitation of neuronal circuits using low-intensity, low-frequency ultrasound. PLoS One 3:e3511. doi: 10.1371/journal.pone.0003511
Wang, S., Meng, W., Ren, Z., Li, B., Zhu, T., Chen, H., et al. (2020). Ultrasonic neuromodulation and sonogenetics: A new era for neural modulation. Front. Physiol. 11:787. doi: 10.3389/fphys.2020.00787
Wells, P. N. T. (2006). Ultrasound imaging. Phys. Med. Biol. 51, R83–R98. doi: 10.1088/0031-9155/51/13/r06
Yang, Y., Pacia, C. P., Ye, D., Zhu, L., Baek, H., Yue, Y., et al. (2020). Sonogenetics for noninvasive and cellular-level neuromodulation in rodent brain. bioRxiv [Preprint]. doi: 10.1101/2020.01.28.919910
Ye, J., Tang, S., Meng, L., Li, X., Wen, X., Chen, S., et al. (2018). Ultrasonic control of neural activity through activation of the mechanosensitive channel MscL. Nano Lett. 18, 4148–4155. doi: 10.1021/acs.nanolett.8b00935
Yoo, S., Mittelstein, D. R., Hurt, R. C., Lacroix, J., and Shapiro, M. G. (2022). Focused ultrasound excites cortical neurons via mechanosensitive calcium accumulation and ion channel amplification. Nat. Commun. 13:493. doi: 10.1038/s41467-022-28040-1
Yu, Y., Zhang, Z., Cai, F., Su, M., Jiang, Q., Zhou, Q., et al. (2019). A novel racing array transducer for noninvasive ultrasonic retinal stimulation: A simulation study. Sensors 19:1825. doi: 10.3390/s19081825
Yuan, Y., Wang, Z., Wang, X., Yan, J., Liu, M., and Li, X. (2019). Low-intensity pulsed ultrasound stimulation induces coupling between ripple neural activity and hemodynamics in the mouse visual cortex. Cereb. Cortex 29, 3220–3223. doi: 10.1093/cercor/bhy187
Yue, L., Weiland, J. D., Roska, B., and Humayun, M. S. (2016). Retinal stimulation strategies to restore vision: Fundamentals and systems. Prog. Retin. Eye Res. 53, 21–47. doi: 10.1016/j.preteyeres.2016.05.002
Zhao, L., Feng, Y., Shi, A., Zhang, L., Guo, S., and Wan, M. (2017). Neuroprotective effect of low-intensity pulsed ultrasound against mpp+-induced neurotoxicity in pc12 cells: Involvement of k2p channels and stretch-activated ion channels. Ultrasound Med. Biol. 43, 1986–1999. doi: 10.1016/j.ultrasmedbio.2017.04.020
Zhuo, S. Y., Li, G. F., Gong, H., Qiu, W. B., Zheng, H. R., and Liang, P. J. (2022). Low-frequency, low-intensity ultrasound modulates light responsiveness of mouse retinal ganglion cells. J. Neural Eng. 19:046012. doi: 10.1088/1741-2552/ac7d75
Keywords: ultrasound stimulation, neuromodulation, artificial vision, vision restoration, visual prosthesis
Citation: Badadhe JD, Roh H, Lee BC, Kim JH and Im M (2022) Ultrasound stimulation for non-invasive visual prostheses. Front. Cell. Neurosci. 16:971148. doi: 10.3389/fncel.2022.971148
Received: 16 June 2022; Accepted: 18 July 2022;
Published: 03 August 2022.
Edited by:
Kwoon Y. Wong, University of Michigan, United StatesReviewed by:
James Weiland, University of Michigan, United StatesCheri Deng, University of Michigan, United States
Copyright © 2022 Badadhe, Roh, Lee, Kim and Im. This is an open-access article distributed under the terms of the Creative Commons Attribution License (CC BY). The use, distribution or reproduction in other forums is permitted, provided the original author(s) and the copyright owner(s) are credited and that the original publication in this journal is cited, in accordance with accepted academic practice. No use, distribution or reproduction is permitted which does not comply with these terms.
*Correspondence: Maesoon Im, bWFlc29vbi5pbUBraXN0LnJlLmty; bWFlc29vbi5pbUBnbWFpbC5jb20=