- 1Laboratory of Neuroimmunology, Department of Physiology and Pharmacology, Sapienza University of Rome, Rome, Italy
- 2IRCCS Neuromed, Pozzilli, Italy
Astrocytes are highly plastic cells whose activity is essential to maintain the cerebral homeostasis, regulating synaptogenesis and synaptic transmission, vascular and metabolic functions, ions, neuro- and gliotransmitters concentrations. In pathological conditions, astrocytes may undergo transient or long-lasting molecular and functional changes that contribute to disease resolution or exacerbation. In recent years, many studies demonstrated that non-neoplastic astrocytes are key cells of the tumor microenvironment that contribute to the pathogenesis of glioblastoma, the most common primary malignant brain tumor and of secondary metastatic brain tumors. This Mini Review covers the recent development of research on non-neoplastic astrocytes as tumor-modulators. Their double-edged capability to promote cancer progression or to represent potential tools to counteract brain tumors will be discussed.
1 Introduction
Although neurons are the excitable and firing cells of the brain driving the nervous system signaling, glial cells in the brain parenchyma are key players for the correct functioning and the homeostasis of the central nervous system. Among glial cells, “star-like” astrocytes are the cells whose relative number, size, number of ramified processes and volume increased with phylogeny and brain complexity (Nedergaard et al., 2003). Depending on the different regions, astrocytes represent 20−40% of all brain cells (Herculano-Houzel, 2014) and show different morphology, ranging from protoplasmic to spherical shape (Emsley and Macklis, 2006; Oberheim et al., 2006). In the brain, each astrocyte occupies a specific territory, with less of 5% of overlap with neighboring astrocytes (Ogata and Kosaka, 2002). Within their specific competence territory, astrocytes contact blood vessels and up to hundred-thousands of different synapses (Halassa et al., 2007); moreover, due to the presence of connexin gap junctions between different astrocytes, these cells are organized in networks (D’Ambrosio et al., 1998; Giaume et al., 2010) that appear to be organized in functional domain (Giaume et al., 2010). Generally believed to mainly have a supportive function (Kettenmann and Ransom, 2005), astrocytic cells play many active roles. During development, astrocytes play a role in guiding the migration of neuronal axons and neuroblast (Powell and Geller, 1999), and the formation of developing synapses (Ullian et al., 2001; Christopherson et al., 2005); moreover, they can drive microglial synapse engulfment, or actively engulf synapses and sculpt neuronal circuits (Chung et al., 2013; Vainchtein et al., 2018). With their terminal processes (end-feet), astrocytes contribute to the formation and maintenance of brain-blood integrity (Abbott, 2002); thank to the presence of several plasma membrane transporters, during neuronal activity they can buffer extracellular K+ concentration and water content (Simard and Nedergaard, 2004), regulate the extracellular pH and remove excessive glutamate from the synapses (Rose et al., 2018). Astrocytes sense neuronal activity via metabotropic neurotransmitter receptors, and are able to provide energy substrate to neurons through the so call “astrocyte-neuron lactate shuttle” (Magistretti and Pellerin, 1999); in addition, astrocytic networks can support the high energy demand of neuronal activity, also at site distant from blood vessels (Rouach et al., 2008), thus ensuring glia-neurons metabolic coupling necessary for memory formation (Suzuki et al., 2011; Gao et al., 2016). Also, astroglial endfeets that enwrap blood vessels are characterized by high levels of connexins expression (Rouach et al., 2008) and Ca2+ signaling within astrocytes can trigger the release of vasoactive molecules that modulate local or regional cerebral blood flow (Koehler et al., 2009; Institoris et al., 2022). Being part of the “tripartite” synapse (Araque et al., 1999), astrocytes respond to neurotransmitter release by presynaptic terminals with an increase in intracellular Ca2+, and consequent release of “gliotransmitters” that can act regulating synaptic plasticity at local synapse (Fellin et al., 2006; Di Castro et al., 2011). Moreover, intracellular calcium increase can be spread to other connected astrocytes (Bazargani and Attwell, 2016; Goenaga et al., 2023) resulting in neurotransmitter release and modulation of synapses at the level of network activity (Fellin, 2009; Miguel-Quesada et al., 2023).
In non-physiological conditions, such as CNS (central nervous system) injuries, disease or brain tumor, astrocytes lose their “quiescent” state, become “reactive” and undergo changes in molecular expression, progressive cellular hypertrophy and in some cases also proliferation and scar formation (Lukaszevicz et al., 2002; Sofroniew, 2009; Faideau et al., 2010; Acevedo-Arozena et al., 2011; Cuevas-Diaz Duran et al., 2019; Makarava et al., 2019). These changes are regulated in a context-specific manner, and lead to altered astrocytic activities, either loss or gain of functions, that can be either detrimental or beneficial to the brain (Sofroniew, 2005).
2 CNS primary tumors originating from astrocytes
CNS primary tumors are the most frequent in children between 0 and 14 years of age and are the eighth most frequent in adults (van den Bent et al., 2023). These tumors are extremely heterogeneous, depending not only on the tissue of origin but also on the genetic and/or molecular modifications that characterize them and on the ethnicity of the affected population; all these aspects define the average outcome of patients (Louis et al., 2021). A first distinction is between malignant and non-malignant tumors; the first ones are able to invade the surrounding tissue and have a terrible outcome; the others are classified based on histological and molecular characteristics. Among the malignant CNS tumors, gliomas are the most common, and glioblastoma (GBM) is the most aggressive and frequent primary malignant CNS tumor, with the prognosis of an overall survival of 7−17 months after surgical removal (Molinaro et al., 2020). Among the non-malignant tumors, the most common is meningioma (Louis et al., 2016).
Despite malignant brain tumors can originate from neuronal stem cells or oligodendrocyte precursor cells, astrocytes represent the cellular origin at least for a defined number of cases (Zong et al., 2015). In fact, a specific mouse model carrying co-deletion of the tumor suppressor Tp53, Pten and Rb1 genes was created using the site-specific recombinase technology (Cre-Lox/GFAP) in adult mice (Chow et al., 2011). This model shows deletion of the three tumor suppressor genes widespread in mature astrocytes but also in a subpopulation of GFAP-expressing neuronal stem cells (NSCs) in the brain proliferative niches (subventricular zone and subgranular layer). Most tumors grew in these niches but more than 20% of tumors appeared in the non-proliferative areas (specifically in cortex, brainstem, cerebellum, and spinal cord). These data demonstrate that mature astrocytes are a cell type from which malignant CNS tumors arise even if the majority originates from stem cells.
Astrocytes can originate malignant CNS tumors not only by alteration of their proliferation and/or cell survival process, but also in case of alterations in the differentiation state maintenance. Indeed, it has been shown that astrocytes dedifferentiated into NSCs - after stimulation with TNFα - become susceptible to the process of cancerization by irradiation; in contrast mature astrocytes do not undergo transformation upon the same oncogenic stress (Dufour et al., 2009).
3 Metastatic brain tumors
Metastatic brain tumors are secondary tumors that develop from cells of a primary systemic tumor that invade the brain and are often the main cause of mortality (Achrol et al., 2019). The most common primary tumors that develop brain metastasis are the lung (40−50%), breast (15−20%), skin (5−10%), and gastrointestinal (4−6%) tumors, and most patients develop more than one brain lesion. Similar to glioma, standard of care to treat brain metastasis is the surgical removal, followed by radiation- and chemo- therapies; nevertheless, these treatments have a reduced efficacy, with most patients developing local recurrence in less than one year (Brastianos et al., 2013). Brain metastasis formation requires several steps such as detachment from the primary tumor, invasion of surrounding tissue, intravasation in blood vessel, dissemination and arrest in brain capillary, extravasation through non-fenestrated capillaries, colonization of surrounding tissue and local proliferation and neo-angiogenesis (Svokos et al., 2014). The interaction between circulating tumor cells and blood–brain barrier (BBB) components is mainly mediated by cytokines and chemokines (Seike et al., 2011); tumor cell proliferation and angiogenesis in the brain depend on the release of local growth factors (Hoshide and Jandial, 2017).
4 Brain tumor/non-neoplastic astrocyte crosstalk
It is well established that the crosstalk between brain tumor cells and the surrounding microenvironment is determinant for tumor progression. In particular 50% of glioma tumor mass is made by the infiltration of brain-resident microglia, and peripheral macrophages (Hambardzumyan et al., 2016) that actively contribute to tumor proliferation and invasion, but also to the formation of an immune suppressive environment (Catalano et al., 2020). Indeed, other infiltrating immune cells are present, these are primarily T lymphocytes, and also rare NK, dendritic and B cells (Gieryng et al., 2017). In particular the infiltrating T cells display an exhausted phenotype and undergo programmed cell death (Woroniecka et al., 2018), resulting in the inability to contrast the tumor.
In this context, astrocytes in the proximity of the tumor became reactive (Nagashima et al., 2002; O’Brien et al., 2013) as a consequence of their ability to sense the tumor and to engage a crosstalk with the tumor microenvironment (TME), becoming part of it. Reactive astrocytes appear to play an important role in supporting glioma growth since, as it has been recently found, genetic ablation of tumor-associated astrocytes in glioma GL261 bearing mice, not only stalls GBM progression but drives the tumors into regression and prolongs animal survival (Perelroizen et al., 2022). The ability of astrocytes to modulate tumor growth seems to depend on the phenotype of glioma cells. In fact, if the glioma is minimally invasive (i.e., U87MG glioma cell line), astrocytes are able to totally block its migration and probably to stimulate a robust immune response. If the glioma is highly invasive (i.e., LN229 glioma cell line), astrocytes increase the migratory capacity of tumor cells by inducing over-expression of genes related to migratory signaling pathways, such as STAT3 (signal transducer and activator of transcription) and HGF/MET (hepatocyte growth factor/mesenchymal-epithelial transition factor) (Cui et al., 2023).
Also in the case of brain metastasis derived from a primary systemic tumor, the interaction between metastatic cells and the surrounding brain TME plays a fundamental role in the establishment of brain metastasis (Gwak, 2023). Among cells of the TME, reactive astrocytes are the most active host cell population, that immediately localizes to individual invading tumor cells and continuously associates with growing metastatic lesions (Lorger and Felding-Habermann, 2010). Moreover, in a model of spontaneous brain metastasis, in immunocompetent mice, Schwartz et al. (2016) demonstrated that astrocytes acquire a proinflammatory phenotype in the brain metastatic niche before the formation of macrometastasis.
The crosstalk between astrocytes and tumoral cells within the brain is mediated by the release of soluble factors, the release of extracellular vesicles (EVs) and through the direct contact between cells, due to gap junctions or to tunneling nanotubes (Figure 1).
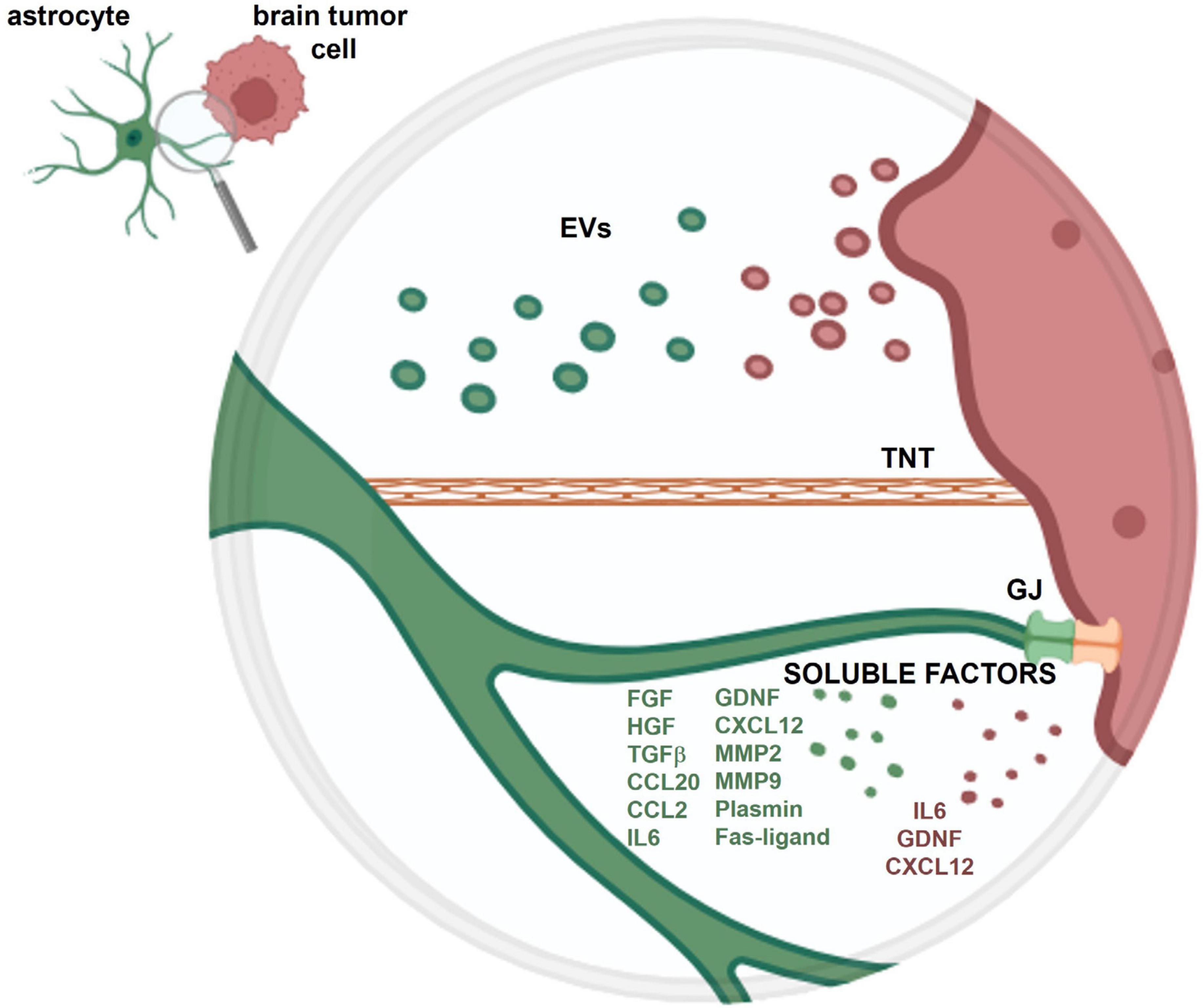
Figure 1. Schematic representation of the crosstalk between an astrocyte and a brain tumor cell. The crosstalk is mediated by soluble factors and extracellular vesicles (EVs) released from both cell types and through the direct contact between cells, via gap junctions (GJ) and tunneling nanotubes (TNT). More detailed information on the role of these different types of crosstalk is provided in the present review.
4.1 Crosstalk via secreted molecules
The secretome of astrocytes depends on the surrounding milieu. For this reason, the mechanisms underlying the interaction between astrocytes and glioma cells are not linear. In fact, the release of many molecules by astrocytes is affected by the ongoing TME and by factors released by tumor cells, and largely contributes to the growth and invasion of the glioma and metastatic brain tumors.
Astrocytes stimulated with the ligand of the receptor activator of nuclear factor kappa-B (RANKL), that is overexpressed by invasive glioma cell lines (Jin et al., 2011), release factors that induce glioma cells invasion such as fibroblast growth factor (FGF), hepatocyte growth factor (HGF), and transforming growth factor β (TGF-β) (Kim et al., 2014). Under hypoxic conditions (that resemble the hypoxic microenvironment in which astrocytes and glioblastoma coexist), astrocytes increase the release of the chemokine CCL20. Astrocytic CCL20 promotes glioma cell invasion through the increase in tumor cell expression of the hypoxia-inducible factor HIF-1α (Jin et al., 2018). Similarly, the chemokine CCL2, that in the brain is mainly produced by astrocytes, turned out to be a key element for metastatic brain tumor cell migration both in vitro and in vivo (Hajal et al., 2021). Cytokine IL6 represents another example of the reciprocal activation between glioma and astrocytes; it is released by the tumor and acts in a paracrine way increasing its own secretion by astrocytes (Liu et al., 2010, Chen W. et al., 2016). Increased release of IL6 promotes migration and invasion of glioma cells through the activation of the transcription factor STAT3 (Chen et al., 2020). This reciprocal activation has not been highlighted for GDNF (glial-derived neurotrophic factor) that does not exert paracrine effect to induce astrogliosis (Ku et al., 2013), but when released by astrocytes enhances the growth and invasion of the tumor (Shabtay-Orbach et al., 2015), and when released by the tumor acts autocrinally to strengthen glioma growth (Lu et al., 2010).
In the central nervous system astrocytes are among the major producers of CXCL12 (Trettel et al., 2020) and express its specific receptor CXCR4. CXCL12 with an autocrine effect activates CXCR4 promoting astrocyte proliferation through the ERK1-2/PI-3K (extracellular signal-regulated kinase 1-2/phosphatidylinositol 3-kinase) signaling pathway (Bajetto et al., 1999; Barbero et al., 2002). Glioma cells also release CXCL12 and overexpress its receptor CXCR4 (Salmaggi et al., 2005; Bian et al., 2007). Stimulation of this axis activates not only ERKs but also AKTs proliferative pathways promoting tumor growth and invasion (Barbero et al., 2003; Rubin et al., 2003). CXCL12 is also able to attract CXCR4 + myeloid derived suppressor cells to create an immunosuppressive microenvironment that favors the growth of glioma (Alghamri et al., 2022), as reported for other tumors (Obermajer et al., 2011; Benedicto et al., 2018). CXCL12 has higher affinity for the receptor CXCR7 (Esencay et al., 2013) whose high expression correlates with poor glioblastoma patient survival (Deng et al., 2017) and similarly to CXCR4 activation, triggers glioma proliferation and invasion (Liu et al., 2015). Astrocytes express CXCR7 (Odemis et al., 2010), whose activation reinforces the CXCR4-mediated proliferative signaling. The expression of CXCR7, and not of CXCR4, on astrocytes is modulated by microenvironmental conditions, such as hypoxic conditions induced by tumoral cells. This distinction highlights the complex role of the trio CXCL12/CXCR4/CXCR7 in the bidirectional interaction between astrocytes and glioma cells. Of note, there is another aspect that generates greater complexity in this interaction. Astrocytes under specific stimuli are able to produce the matrix metalloproteinases MMP2 and MMP9 (Ogier et al., 2006), both overexpressed in GBM patients and correlating with patient poor prognosis. These enzymes also mediate the proteolytic processing of CXCL12 into the specific CXCL12(5-67) peptide, a neurotoxic protein that binds CXCR3, whose expression in GBM patients also correlates with a patient’s poor prognosis. Even if the direct effect of the chemokine peptide CXCL12(5-67) on glioma cells has not yet been evaluated, CXCR3 activation increases glioma cell invasion whilst CXCR3 downregulation inhibits glioma stem cells viability (Pu et al., 2015; Boyé et al., 2017).
Astrocytes are among the brain parenchymal cells that first make contact with extravasated metastatic cells. Reactive astrocytes close to extravasating metastatic tumor cells in the brain, also overexpress and release MMP9 favoring the development of brain metastasis (Lorger and Felding-Habermann, 2010). Using different types of breast cancer or lung cancer cell lines, to induce brain metastatization in mice, it has been shown that, by sensing brain infiltrating cancer cells, astrocytes became reactive and attempt to defend against metastatic invasion by releasing both Plasmin (PA) and Fas-ligand, that induce cancer cell death. Moreover plasmin induces the destroying of L1CAM (L1 cell adhesion molecule) expressed by cancer cells, preventing their ability to coopt brain capillaries. (Valiente et al., 2014). However, some metastatic cells can express high levels of antiPA-serpin (that prevents PA formation) preventing cell death and fostering vascular cooption (Valiente et al., 2014).
In addition, it has been shown that proinflammatory astrocytes are instigated to overcome brain tissue damage due to the entrance of metastatic cells into the brain. Later on, reactive astrocytes are hijacked by brain-metastasizing tumor cells in order to express SerpinE1 and SerpinA3N genes, that support metastasis growth (Schwartz et al., 2016).
4.2 Crosstalk via extracellular vesicles
In addition to the secretome, that acts close to the cell of origin, EVs that can act also far from the donor cell. These particles are made up of a phospholipid bilayer that contains protein, lipid and genetic materials which is completely transferred to the recipient cell. Tumors and among them gliomas, release huge amounts of EVs as a tumorigenic mechanism, being their content able to activate transforming signaling pathways in target cells; for example, tumor-derived EVs induce transformed features to normal adjacent cells (i.e., fibroblasts, stromal and epithelial cells) such as anchorage-independent growth and enhanced or aberrant growth capability (Webber et al., 2010; Antonyak et al., 2011; Paggetti et al., 2015). Glioma-derived EVs, as described in general for cancer-derived vesicles, could also be shared between tumoral cells. Tumor-derived EVs transfer drug-resistance molecules from drug-resistant cells to drug-sensitive ones as observed in breast cancer (Lv et al., 2014).
In addition, they activate macrophages, B and NK cells, induce maturation of dendritic cells and promote generation of myeloid-derived suppressor cells (Liu et al., 2006; Valenti et al., 2006; Yu et al., 2007; Clayton et al., 2008). All these effects belong to the pleiotropic mechanism aimed by tumor EVs to promote an immune-suppressive microenvironment supporting the cancer development. Glioma-derived EVs target different immune cells supporting their defective response, one of the major hallmarks of tumor occurrence. They transfer onco-miRs as for example miR155, miR214, miR21 (Zonari et al., 2013; Feng and Tsao, 2016; Abels et al., 2019; Yang et al., 2019; Xu et al., 2021; Orso et al., 2023) or tumorigenic transcription factors (such as Stat3) into tissue-resident microglia, infiltrating myeloid-derived macrophages (Gabrusiewicz et al., 2018; Johnson et al., 2018; Xu et al., 2021) and tumor-infiltrating regulatory T cells (Li et al., 2017).
With respect to astrocytes, GBM EVs stimulate astrocyte release of a huge amount of growth factors, cytokines and chemokines (few examples are TNFα, CCL20, IL10 and CCL2, see above) (Oushy et al., 2018), that mediate autocrine effect promoting astrocytes migration and paracrine effects inducing tumor cells migration and invasion (Kucharzewska et al., 2013; Mu et al., 2013; Taheri et al., 2018). Tumor EVs induce an upregulation of genes important for extracellular matrix remodeling (i.e., MMP2 and MMP9). GBM EVs also show transforming capability toward astrocytes, perhaps by transferring oncogenes how demonstrated for the oncogenic form of the epidermal growth factor receptor (EGF), called EGFRvIII, horizontally transferred among glioma cells to induce the activation of EGFRvIII-dependent oncogenes (Al-Nedawi et al., 2008).
The effect of EVs released by astrocytes in most cases enhances brain tumor development. In fact, it has been demonstrated that astrocytes derived EVs transfer miRs that inhibit the important tumor suppressor PTEN in metastatic tumor cells (Zhang et al., 2015). Notably, PTEN loss is responsible for the increased release of CCL2 (Hajal et al., 2021) that autocrinally reinforces the migration of tumor cells. Astrocytes derived EVs also contain factors such as fibroblast growth factor-2 and vascular endothelial growth factor (Proia et al., 2008) that could be shared with glioma cells in which exert a proliferative action (Haley and Kim, 2014; Bian et al., 2000; Jimenez-Pascual et al., 2020).
4.3 Crosstalk via gap junctions
Astrocytes are highly interconnected through gap junctions that allow for fast ions and metabolites exchange. Gap junctions are made up of two hemichannels, each expressed on a different cell. Each hemichannel, called connexin, consists of six protein subunits (Scott et al., 2012). Connexin 43 (Cx43) represents the most abundant subunit on astrocytes (Rash et al., 2001, Xing et al., 2019). Even if Cx43 is overexpressed in the tumoral core, contributing to the increase of GBM-GBM cell communication, it is also over expressed in a subset of reactive astrocytes close to tumor cells. Selective deletion of Cx43 in reactive astrocytes attenuates glioma invasion in vivo (Sin et al., 2016). In line with this finding, it has been found that glioma-astrocyte gap junctions enable the transfer from GBM cells to astrocytes of many miRs (such as miR19) that downregulates the expression of cadherins, integrins, focal adhesion kinases, and other adhesion molecules. This process favors a reduced adhesion of astrocytes to the basement membrane, thereby opening a gateway that favors tumor cell invasion (McCutcheon and Spray, 2022). In addition, Cx43 mediates the transfer of cGAMP from brain metastatic cancer cells to astrocytes inducing the release of factors (such as TNFα) that activate the NF-kB pathways on cancer cells (Chen Q. et al., 2016), thus promoting metastasis progression (Wang et al., 2017). Cx43 gap junctions between metastatic brain cancer cells and astrocytes are favored by the over expression of the brain specific cell adhesion molecule protocadherin 7 (PCDH7) in metastatic cells (Chen Q. et al., 2016).
The expression of genes related to drug resistance, anti-apoptosis and survival in glioma cells also depends on genetic material that is transferred from astrocytes to glioma cells through gap junctions (Lin et al., 2016). Among these genetic materials are also microRNAs such as miR5096 that can activate pro-invasive pathways in cancer cells (Hong et al., 2015), or miR152-3p that can reduce cell migration and invasion of glioma cells (Fukuda et al., 2021).
The tight junctions between astrocytes and vessel smooth muscle cells are unsettled by tumor cells that creep between the two healthy cell types (Watkins et al., 2014). In this manner, tumor cells take control of vessel tone by modulating K+ efflux, and thus dilate or constrict arterioles by the same mechanism used by astrocytes (Zonta et al., 2003).
4.4 Crosstalk via tunneling nanotubes
Another direct contact between astrocytes and glioma cells is represented by tunneling nanotubes (TNTs), thin and long protuberances (up to 550 μm) of the cell cytoplasm. TNTs allow the transfer of ions, molecules, and organelles from the donor to the target cell (Davis and Sowinski, 2008). Astrocyte-glioma nanotubes initiating from astrocytes are able to reduce the proliferation of tumor cells (Zhang and Zhang, 2015). Tumoral TNTs, called tumor microtubes, are structurally different showing less F-actin content, and being long-live and thicker than non-tumoral TNTs. They contribute to tumor growth, by distributing potentially toxic material for tumor cells, such as calcium (Li et al., 2020), to neighboring cells keeping its intracellular levels within non-lethal limits (Osswald et al., 2015). Besides TNTs can translocate larger structures such as cellular organelles that can change the functionality of recipient cells. For example, tumor microtubes transfer mitochondria from glioma to healthy astrocytes. These mitochondria transform the metabolism of recipient cells (i.e., non-neoplastic astrocytes) into a tumor-like metabolism, especially with regard to the utilization of glutamine as major energy source instead of glucose and lipids used by healthy astrocytes (Valdebenito et al., 2021); thus, non-neoplastic astrocytes become resistant to the hypoxic environment induced by the fast proliferation of tumor cells (Beppu et al., 2002). The in vivo discovery of a microtubes-mediated functional coupling between GBM cells and astrocytes is recent (Venkataramani et al., 2022) and highlights a potentially relevant aspect for diagnostic and therapeutic purposes.
5 Non-neoplastic astrocytes counteract GBM
Although non-neoplastic astrocytes engage direct and indirect dialogue with glioma cells contributing to tumor progression, recent data suggest that these cells can also represent key elements to contrast tumor progression (Fletcher-Sananikone et al., 2021; Serpe et al., 2022).
One strategy to counteract glioma might be represented by EVs released by non-neoplastic astrocytes. Recently (Serpe et al., 2022) we have found that EVs derived from glioma-stimulated astrocytes increase glioma proliferation and in vivo tumor volume. In contrast, EVs derived from normal astrocytes are able, in vitro, to reduce glioma cell proliferation, migration and invasion capability. Moreover in vivo, administration of these EVs reduces glioma tumor volume, proliferation rate, and in addition, impairs cell volume regulation. We found that among molecules transported by these EVs there is miR124. Such molecules are able to reduce the expression in glioma cells of LRCC8C protein, a subunit of the volume regulated anion channels (VRACs) that play a role in the modification of cell volume, necessary for cell migration and proliferation. Glioma cells kill surrounding neurons by releasing glutamate in order to create the necessary space to grow (Ye and Sontheimer, 1999). VRACs are also permeable to excitatory amino acids including glutamate (Feustel et al., 2004); thus, we speculate that the reduction of VRAC expression might contribute to reduction in glioma release of glutamate.
Another strategy could be the use of non-neoplastic astrocytes as therapeutic targets. After surgical resection of glioma, the standard of care is patients’ treatment with up to 60 Gy of fractionated ionized irradiation (IR) with concurrent adjuvant chemotherapy, such as Temozolomide (Stupp et al., 2005). However, despite the positive response following IR therapy, later on the recurrence of a more invasive and resistant glioma lead to a fatal prognosis (Osuka and Van Meir, 2017, Scoccianti et al., 2021). Recently, it has been demonstrated in vivo, using syngeneic GL261 mouse model of glioma, and in vitro, using coculture of normal astrocytes and GL261 glioma cells, that upon irradiation normal astrocytes became senescent and release factors, including HGF. HGF then activates the Met receptor on glioma, promoting tumor invasiveness. In vivo, blocking Met activation by pharmacological approach results in attenuation of tumor growth and increased mice survival. Further, the elimination of senescent astrocytes using a senolytic drug results in delayed tumor growth in pre-irradiated brains (Fletcher-Sananikone et al., 2021).
6 Conclusion
In this Mini Review different strategies of the crosstalk between surrounding non-neoplastic astrocytes and brain primary or metastatic tumor cells have been reported. Astrocytes react to brain tumor cells engaging in undirect and direct dialogues mainly to support the tumor growth. Astrocytic release of soluble factors fosters migration, invasion, and growth of primary brain tumor cells. In brain metastasis these factors, released by astrocytes close to the extravasated cancer cells, support the brain entering.
Extracellular vesicles released by primary tumor cells promote astrocytic polarization toward an immunosuppressive phenotype and astrocytic release of soluble factors that contribute to the tumor progression. EVs released by astrocytes transfer miRs and growth factors into metastatic cancer cells that support metastatization into the brain.
Direct exchange of molecules occurs through gap junctions or tunneling nanotubes between astrocytes and brain tumor cells also support their crosstalk.
However, recent data suggest that EVs from non-neoplastic astrocytes or selective elimination of non-neoplastic astrocytes may be used to counteract brain tumors. Considering these findings, it is possible to speculate that in the future, administration of EVs obtained from non-neoplastic astrocytes (for example derived from patient differentiated IPSCs), and/or targeting non-neoplastic astrocytes by using senolytic therapy, could represent an alternative or coadiuvant therapeutic approach to limit brain tumor progression and to contrast glioma recurrence.
Author contributions
MC: Conceptualization, Data curation, Supervision, Writing – original draft, Writing – review & editing, Funding acquisition. CL: Funding acquisition, Supervision, Writing – review & editing. FT: Conceptualization, Data curation, Supervision, Writing – original draft, Writing – review & editing.
Funding
The author(s) declare financial support was received for the research, authorship, and/or publication of this article. This research was funded by the Sapienza University of Rome, grant RM12117A80E90BF3 to MC.; Italian Ministry of University, grant PRIN2020Z73J5A to CL.; AIRC Foundation for Cancer Research in Italy, grant IG-23010 to CL.; grant PNC SALUTE – D3 4 Health - Digital Driven Diagnostics, prognostics and therapeutics for sustainable Health care - PNC0001, Spoke 3 Linea tematica 2, CUP B53C22006120001 to CL.
Conflict of interest
The authors declare that the research was conducted in the absence of any commercial or financial relationships that could be construed as a potential conflict of interest.
The author(s) declared that they were an editorial board member of Frontiers, at the time of submission. This had no impact on the peer review process and the final decision.
Publisher’s note
All claims expressed in this article are solely those of the authors and do not necessarily represent those of their affiliated organizations, or those of the publisher, the editors and the reviewers. Any product that may be evaluated in this article, or claim that may be made by its manufacturer, is not guaranteed or endorsed by the publisher.
References
Abbott, N. J. (2002). Astrocyte-endothelial interactions and blood-brain barrier permeability. J. Anat. 200, 629–638. doi: 10.1046/j.1469-7580.2002.00064.x
Abels, E. R., Maas, S. L. N., Nieland, L., Wei, Z., Cheah, P. S., Tai, E., et al. (2019). Glioblastoma-associated microglia reprogramming is mediated by functional transfer of extracellular miR-21. Cell Rep. 28, 3105–3119. doi: 10.1016/j.celrep.2019.08.036
Acevedo-Arozena, A., Kalmar, B., Essa, S., Ricketts, T., Joyce, P., Kent, R., et al. (2011). A comprehensive assessment of the SOD1G93A low-copy transgenic mouse, which models human amyotrophic lateral sclerosis. Dis. Model Mech. 4, 686–700. doi: 10.1242/dmm.007237
Achrol, A. S., Rennert, R. C., Anders, C., Soffietti, R., Ahluwalia, M. S., Nayak, L., et al. (2019). Brain metastases. Nat. Rev. Dis. Primers 5:5. doi: 10.1038/s41572-018-0055-y
Alghamri, M. S., Banerjee, K., Mujeeb, A. A., Mauser, A., Taher, A., Thalla, R., et al. (2022). Systemic delivery of an adjuvant CXCR4-CXCL12 signaling inhibitor encapsulated in synthetic protein nanoparticles for glioma immunotherapy. ACS Nano. 16, 8729–8750. doi: 10.1021/acsnano.1c07492
Al-Nedawi, K., Meehan, B., Micallef, J., Lhotak, V., May, L., Guha, A., et al. (2008). Intercellular transfer of the oncogenic receptor EGFRvIII by microvesicles derived from tumour cells. Nat. Cell Biol. 10, 619–624. doi: 10.1038/ncb1725
Antonyak, M. A., Li, B., Boroughs, L. K., Johnson, J. L., Druso, J. E., and Bryant, K. L. (2011). Cancer cell-derived microvesicles induce transformation by transferring tissue transglutaminase and fibronectin to recipient cells. Proc. Natl. Acad. Sci. U. S. A. 108, 4852–4857. doi: 10.1073/pnas.1017667108
Araque, A., Parpura, V., Sanzgiri, R. P., and Haydon, P. G. (1999). Tripartite synapses: Glia, the unacknowledged partner. Trends Neurosci. 22, 208–215. doi: 10.1016/S0166-2236(98)01349-6
Bajetto, A., Bonavia, R., Barbero, S., Piccioli, P., Costa, A., Florio, T., et al. (1999). Glial and neuronal cells express functional chemokine receptor CXCR4 and its natural ligand stromal cell-derived factor 1. J. Neurochem. 73, 2348–2357. doi: 10.1046/j.1471-4159.1999.0732348.x
Barbero, S., Bajetto, A., Bonavia, R., Porcile, C., Piccioli, P., and Pirani, P. (2002). Expression of the chemokine receptor CXCR4 and its ligand stromal cell-derived factor 1 in human brain tumors and their involvement in glial proliferation in vitro. Ann. N. Y. Acad Sci. 973, 60–69. doi: 10.1111/j.1749-6632.2002.tb04607.x
Barbero, S., Bonavia, R., Bajetto, A., Porcile, C., Pirani, P., Ravetti, J. L., et al. (2003). Stromal cell-derived factor 1alpha stimulates human glioblastoma cell growth through the activation of both extracellular signal-regulated kinases 1/2 and Akt. Cancer Res. 63, 1969–1974.
Bazargani, N., and Attwell, D. (2016). Astrocyte calcium signaling: the third wave. Nat. Neurosci. 19, 182–189. doi: 10.1038/nn.4201
Benedicto, A., Romayor, I., and Arteta, B. (2018). CXCR4 receptor blockage reduces the contribution of tumor and stromal cells to the metastatic growth in the liver. Oncol. Rep. 39, 2022–2030. doi: 10.3892/or.2018.6254
Beppu, T., Kamada, K., Yoshida, Y., Arai, H., Ogasawara, K., and Ogawa, A. (2002). Change of oxygen pressure in glioblastoma tissue under various conditions. J. Neurooncol. 58, 47–52. doi: 10.1023/a:1015832726054
Bian, X. W., Du, L. L., Shi, J. Q., Cheng, Y. S., and Liu, F. X. (2000). Correlation of bFGF, FGFR-1 and VEGF expression with vascularity and malignancy of human astrocytomas. Anal. Quant. Cytol. Histol. 22, 267–274.
Bian, X. W., Yang, S. X., Chen, J. H., Ping, Y. F., Zhou, X. D., Wang, Q. L., et al. (2007). Preferential expression of chemokine receptor CXCR4 by highly malignant human gliomas and its association with poor patient survival. Neurosurgery 61, 570–579. doi: 10.1227/01.NEU.0000290905.53685.A2
Boyé, K., Pujol, N., Alves, I., Chen, Y. P., Daubon, T., Lee, Y. Z., et al. (2017). The role of CXCR3/LRP1 cross-talk in the invasion of primary brain tumors. Nat. Commun. 8:1571. doi: 10.1038/s41467-017-01686-y
Brastianos, P. K., Curry, W. T., and Oh, K. S. (2013). Clinical discussion and review of the management of brain metastases. J. Natl. Compr. Canc. Netw. 11, 1153–1164. doi: 10.6004/jnccn.2013.0133
Catalano, M., D’Alessandro, G., Trettel, F., and Limatola, C. (2020). Role of infiltrating microglia/macrophages in Glioma. Adv. Exp. Med. Biol. 1202, 281–298. doi: 10.1007/978-3-030-30651-9_14
Chen, Q., Boire, A., Jin, X., Valiente, M., Er, E. E., Lopez-Soto, A., et al. (2016). Carcinoma-astrocyte gap junctions promote brain metastasis by cGAMP transfer. Nature 533, 493–498. doi: 10.1038/nature18268
Chen, W., Xia, T., Wang, D., Huang, B., Zhao, P., Wang, J., et al. (2016). Human astrocytes secrete IL-6 to promote glioma migration and invasion through upregulation of cytomembrane MMP14. Oncotarget 7, 62425–62438.
Chen, Z., Yuan, S. J., Li, K., Zhang, Q., Li, T. F., An, H. C., et al. (2020). Doxorubicin-polyglycerol-nanodiamond conjugates disrupt STAT3/IL-6-mediated reciprocal activation loop between glioblastoma cells and astrocytes. J. Control Rel. 320, 469–483. doi: 10.1016/j.jconrel.2020.01.044
Chow, L. M., Endersby, R., Zhu, X., Rankin, S., Qu, C., Zhang, J., et al. (2011). Cooperativity within and among Pten, p53, and Rb pathways induces high-grade astrocytoma in adult brain. Cancer Cell. 19, 305–316. doi: 10.1016/j.ccr.2011.01.039
Christopherson, K., Ullian, E., Stokes, C., Mullowney, C., Hell, J. W., Agah, A., et al. (2005). Thrombospondins are astrocyte-secreted proteins that promote CNS synaptogenesis. Cell 120, 421–433. doi: 10.1016/j.cell.2004.12.020
Chung, W.-S. S., Clarke, L. E., Wang, G. X., Stafford, B. K., Sher, A., Chakraborty, C., et al. (2013). Astrocytes mediate synapse elimination through MEGF10 and MERTK pathways. Nature 504, 394–400. doi: 10.1038/nature12776
Clayton, A., Mitchell, J. P., Court, J., Linnane, S., Mason, M. D., and Tabi, Z. (2008). Human tumor-derived exosomes down-modulate NKG2D expression. J. Immunol. 180, 7249–7258. doi: 10.4049/jimmunol.180.11.7249
Cuevas-Diaz Duran, R., Wang, C. Y., Zheng, H., Deneen, B., and Wu, J. Q. (2019). Brain region-specific gene signatures revealed by distinct astrocyte subpopulations unveil links to glioma and neurodegenerative diseases. eNeuro 6, ENEURO.288–ENEURO.218. doi: 10.1523/ENEURO.0288-18.2019
Cui, Y., Lee, P., Reardon, J. J., Wang, A., Lynch, S., Otero, J. J., et al. (2023). Evaluating glioblastoma tumour sphere growth and migration in interaction with astrocytes using 3D collagen-hyaluronic acid hydrogels. J. Mater. Chem. B. 11, 5442–5459. doi: 10.1039/d3tb00066d
D’Ambrosio, R., Wenzel, J., Schwartzkroin, P. A., and McKhann, G. M. (1998). Functional specialization and topographic segregation of hippocampal astrocytes. J. Neurosci. 18, 4425–4438. doi: 10.1523/JNEUROSCI.18-12-04425.1998
Davis, D. M., and Sowinski, S. (2008). Membrane nanotubes: dynamic long-distance connections between animal cells. Nat. Rev. Mol. Cell Biol. 9, 431–436. doi: 10.1038/nrm2399
Deng, L., Zheng, W., Dong, X., Liu, J., Zhu, C., Lu, D., et al. (2017). Chemokine receptor CXCR7 is an independent prognostic biomarker in glioblastoma. Cancer Biomark. 20, 1–6. doi: 10.3233/CBM-151430
Di Castro, M. A., Chuquet, J., Liaudet, N., Bhaukaurally, K., Santello, M., Bouvier, D., et al. (2011). Local Ca2+ detection and modulation of synaptic release by astrocytes. Nat. Neurosci. 14, 1276–1284. doi: 10.1038/nn.2929
Dufour, C., Cadusseau, J., Varlet, P., Surena, A. L., de Faria, G. P., Dias-Morais, A., et al. (2009). Astrocytes reverted to a neural progenitor-like state with transforming growth factor alpha are sensitized to cancerous transformation. Stem Cells 27, 2373–2382. doi: 10.1002/stem.155
Emsley, J. G., and Macklis, J. D. (2006). Astroglial heterogeneity closely reflects the neuronal-defined anatomy of the adult murine CNS. Neuron Glia Biol. 2, 175–186. doi: 10.1017/S1740925X06000202
Esencay, M., Sarfraz, Y., and Zagzag, D. (2013). CXCR7 is induced by hypoxia and mediates glioma cell migration towards SDF-1α. BMC Cancer 13:347. doi: 10.1186/1471-2407-13-347
Faideau, M., Kim, J., Cormier, K., Gilmore, R., Welch, M., Auregan, G., et al. (2010). In vivo expression of polyglutamine-expanded huntingtin by mouse striatal astrocytes impairs glutamate transport: a correlation with Huntington’s disease subjects. Hum. Mol. Genet. 19, 3053–3067. doi: 10.1093/hmg/ddq212
Fellin, T. (2009). Communication between neurons and astrocytes: relevance to the modulation of synaptic and network activity. J. Neurochem. 108, 533–544. doi: 10.1111/j.1471-4159.2008.05830.x
Fellin, T., Sul, J. Y., D’Ascenzo, M., Takano, H., Pascual, O., and Haydon, P. G. (2006). Bidirectional astrocyte-neuron communication: the many roles of glutamate and ATP. Novartis Found Symp. 217, 275–281. doi: 10.1002/9780470032244.ch16
Feng, Y. H., and Tsao, C. J. (2016). Emerging role of microRNA-21 in cancer. Biomed. Rep. 5, 395–402. doi: 10.3892/br.2016.747
Feustel, P. J., Jin, Y., and Kimelberg, H. K. (2004). Volume-regulated anion channels are the predominant contributors to release of excitatory amino acids in the ischemic cortical penumbra. Stroke 35, 1164–1168. doi: 10.1161/01.STR.0000124127.57946.a1
Fletcher-Sananikone, E., Kanji, S., Tomimatsu, N., Di Cristofaro, L. F. M., Kollipara, R. K., Saha, D., et al. (2021). Elimination of radiation-induced senescence in the brain tumor microenvironment attenuates glioblastoma recurrence. Cancer Res. 81, 5935–5947. doi: 10.1158/0008-5472.CAN-21-0752
Fukuda, S., Akiyama, M., Niki, Y., Kawatsura, R., Harada, H., and Nakahama, K. I. (2021). Inhibitory effects of miRNAs in astrocytes on C6 glioma progression via connexin 43. Mol. Cell Biochem. 476, 2623–2632. doi: 10.1007/s11010-021-04118-0
Gabrusiewicz, K., Li, X., Wei, J., Hashimoto, Y., Marisetty, A. L., Ott, M., et al. (2018). Glioblastoma stem cell-derived exosomes induce M2 macrophages and PD-L1 expression on human monocytes. Oncoimmunology 7, e1412909. doi: 10.1080/2162402X.2017.1412909
Gao, V., Suzuki, A., Magistretti, P. J., Lengacher, S., Pollonini, G., Steinman, M. Q., et al. (2016). Astrocytic β2-adrenergic receptors mediate hippocampal long-term memory consolidation. Proc. Natl. Acad. Sci. U. S. A. 113, 8526–8531. doi: 10.1073/pnas.1605063113
Giaume, C., Koulakoff, A., Roux, L., Holcman, D., and Rouach, N. (2010). Astroglial networks: a step further in neuroglial and gliovascular interactions. Nat. Rev. Neurosci. 11, 87–99. doi: 10.1038/nrn2757
Gieryng, A., Pszczolkowska, D., Walentynowicz, K. A., Rajan, W. D., and Kaminska, B. (2017). Immune microenvironment of gliomas. Lab. Invest. 97, 498–518. doi: 10.1038/labinvest.2017.19
Goenaga, J., Araque, A., Kofuji, P., and Herrera Moro, and Chao, D. (2023). Calcium signaling in astrocytes and gliotransmitter release. Front. Synaptic Neurosci. 15:1138577. doi: 10.3389/fnsyn.2023.1138577
Gwak, H. S. (2023). Molecular biology of brain metastases. Brain Tumor Res. Treat. 11, 8–15. doi: 10.14791/btrt.2022.0045
Hajal, C., Shin, Y., Li, L., Serrano, J. C., Jacks, T., and Kamm, R. D. (2021). The CCL2-CCR2 astrocyte-cancer cell axis in tumor extravasation at the brain. Sci. Adv. 7, eabg8139. doi: 10.1126/sciadv.abg8139
Halassa, M. M., Fellin, T., Takano, H., Dong, J. H., and Haydon, P. G. (2007). Synaptic islands defined by the territory of a single astrocyte. J. Neurosci. 27, 6473–6477. doi: 10.1523/JNEUROSCI.1419-07.2007
Haley, E. M., and Kim, Y. (2014). The role of basic fibroblast growth factor in glioblastoma multiforme and glioblastoma stem cells and in their in vitro culture. Cancer Lett. 346, 1–5. doi: 10.1016/j.canlet.2013.12.003
Hambardzumyan, D., Gutmann, D. H., and Kettenmann, H. (2016). The role of microglia and macrophages in glioma maintenance and progression. Nat. Neurosci. 19, 20–27. doi: 10.1038/nn.4185
Herculano-Houzel, S. (2014). The glia/neuron ratio: how it varies uniformly across brain structures and species and what that means for brain physiology and evolution. Glia 62, 1377–1391. doi: 10.1002/glia.22683
Hong, X., Sin, W. C., Harris, A. L., and Naus, C. C. (2015). Gap junctions modulate glioma invasion by direct transfer of microRNA. Oncotarget 6, 15566–15577. doi: 10.18632/oncotarget.3904
Hoshide, R., and Jandial, R. (2017). The role of the neural niche in brain metastasis. Clin. Exp. Meta. 34, 369–376. doi: 10.1007/s10585-017-9857-7
Institoris, A., Vandal, M., Peringod, G., Catalano, C., Tran, C. H., Yu, X., et al. (2022). Astrocytes amplify neurovascular coupling to sustained activation of neocortex in awake mice. Nat. Commun. 13:7872. doi: 10.1038/s41467-022-35383-2
Jimenez-Pascual, A., Mitchell, K., Siebzehnrubl, F. A., and Lathia, J. D. (2020). FGF2: a novel druggable target for glioblastoma? Expert. Opin. Ther. Targets 24, 311–318. doi: 10.1080/14728222.2020.1736558
Jin, P., Shin, S., Chun, Y., Shin, H., Shin, Y. J., Lee, Y., et al. (2018). Astrocyte-derived CCL20 reinforces HIF-1-mediated hypoxic responses in glioblastoma by stimulating the CCR6-NF-κB signaling pathway. Oncogene 37, 3070–3087. doi: 10.1038/s41388-018-0182-7
Jin, X., Jeon, H. Y., Joo, K. M., Kim, J. K., Jin, J., Kim, S. H., et al. (2011). Frizzled 4 regulates stemness and invasiveness of migrating glioma cells established by serial intracranial transplantation. Cancer Res. 71, 3066–3075. doi: 10.1158/0008-5472.CAN-10-1495
Johnson, D. E., O’Keefe, R. A., and Grandis, J. R. (2018). Targeting the IL-6/JAK/STAT3 signalling axis in cancer. Nat. Rev. Clin. Oncol. 15, 234–248.
Kettenmann, H., and Ransom, B. R. (2005). Neuroglia, 2nd Edn. New York, NY: Oxford University Press.
Kim, J. K., Jin, X., Sohn, Y. W., Jin, X., Jeon, H. Y., Kim, E. J., et al. (2014). Tumoral RANKL activates astrocytes that promote glioma cell invasion through cytokine signaling. Cancer Lett. 28 353, 194–200. doi: 10.1016/j.canlet.2014.07.034
Koehler, R. C., Roman, R. J., and Harder, D. R. (2009). Astrocytes and the regulation of cerebral blood flow. Trends Neurosci. 32, 160–169. doi: 10.1016/j.tins.2008.11.005
Ku, M. C., Wolf, S. A., Respondek, D., Matyash, V., Pohlmann, A., Waiczies, S., et al. (2013). GDNF mediates glioblastoma-induced microglia attraction but not astrogliosis. Acta Neuropathol. 125, 609–620. doi: 10.1007/s00401-013-1079-8
Kucharzewska, P., Christianson, H. C., Welch, J. E., Svensson, K. J., Fredlund, E., Ringnér, M., et al. (2013). Exosomes reflect the hypoxic status of glioma cells and mediate hypoxia-dependent activation of vascular cells during tumor development. Proc. Natl. Acad. Sci. U. S. A. 110, 7312–7317. doi: 10.1073/pnas.1220998110
Li, P., Feng, J., Liu, Y., Liu, Q., Fan, L., Liu, Q., et al. (2017). Novel therapy for glioblastoma multiforme by restoring LRRC4 in tumor cells: lrrc4 inhibits tumor-infitrating regulatory t cells by cytokine and programmed cell death 1-containing exosomes. Front. Immunol. 8:1748. doi: 10.3389/fimmu.2017.01748
Li, X., Spelat, R., Bartolini, A., Cesselli, D., Ius, T., Skrap, M., et al. (2020). Mechanisms of malignancy in glioblastoma cells are linked to mitochondrial Ca2+ uniporter upregulation and higher intracellular Ca2+ levels. J. Cell Sci. 133, jcs237503. doi: 10.1242/jcs.237503
Lin, Q., Liu, Z., Ling, F., and Xu, G. (2016). Astrocytes protect glioma cells from chemotherapy and upregulate survival genes via gap junctional communication. Mol. Med. Rep. 13, 1329–1335. doi: 10.3892/mmr.2015.4680
Liu, C., Yu, S., Zinn, K., Wang, J., Zhang, L., Jia, Y., et al. (2006). Murine mammary carcinoma exosomes promote tumor growth by suppression of NK cell function. J. Immunol. 176, 1375–1385. doi: 10.4049/jimmunol.176.3.1375
Liu, Q., Li, G., Li, R., Shen, J., He, Q., Deng, L., et al. (2010). IL-6 promotion of glioblastoma cell invasion and angiogenesis in U251 and T98G cell lines. J. Neuro-oncol. 100, 165–176. doi: 10.1007/s11060-010-0158-0
Liu, Y., Carson-Walter, E., and Walter, K. A. (2015). Targeting chemokine receptor CXCR7 inhibits glioma cell proliferation and mobility. Anticancer Res. 35, 53–64.
Lorger, M., and Felding-Habermann, B. (2010). Capturing changes in the brain microenvironment during initial steps of breast cancer brain metastasis. Am. J. Pathol. 176, 2958–2971. doi: 10.2353/ajpath.2010.090838
Louis, D. N., Perry, A., Reifenberger, G., von Deimling, A., Figarella-Branger, D., Cavenee, W. K., et al. (2016). The 2016 World Health Organization classification of tumors of the central nervous system: A summary. Acta Neuropathol. 131, 803–820. doi: 10.1007/s00401-016-1545-1
Louis, D. N., Perry, A., Wesseling, P., Brat, D. J., Cree, I. A., Figarella-Branger, D., et al. (2021). The 2021 WHO classification of tumors of the central nervous system: a summary. Neuro Oncol. 23, 1231–1251. doi: 10.1093/neuonc/noab106
Lu, D. Y., Leung, Y. M., Cheung, C. W., Chen, Y. R., and Wong, K. L. (2010). Glial cell line-derived neurotrophic factor induces cell migration and matrix metalloproteinase-13 expression in glioma cells. Biochem. Pharmacol. 80, 1201–1209. doi: 10.1016/j.bcp.2010.06.046
Lukaszevicz, A. C., Sampaïo, N., Guégan, C., Benchoua, A., Couriaud, C., Chevalier, E., et al. (2002). High sensitivity of protoplasmic cortical astroglia to focal ischemia. J. Cereb. Blood Flow Metab. 22, 289–298. doi: 10.1097/00004647-200203000-00006
Lv, M. M., Zhu, X. Y., Chen, W. X., Zhong, S. L., Hu, Q., Ma, T. F., et al. (2014). Exosomes mediate drug resistance transfer in MCF-7 breast cancer cells and a probable mechanism is delivery of P-glycoprotein. Tumour Biol. 35, 10773–10779. doi: 10.1007/s13277-014-2377-z
Magistretti, P. J., and Pellerin, L. (1999). Cellular mechanisms of brain energy metabolism and their relevance to functional brain imaging. Philos. Trans. R. Soc. Lond. B Biol. Sci. 354, 1155–1163.
Makarava, N., Chang, J. C., Kushwaha, R., and Baskakov, I. V. (2019). Region-specific response of astrocytes to prion infection. Front. Neurosci. 13:1048. doi: 10.3389/fnins.2019.01048
McCutcheon, S., and Spray, D. C. (2022). Glioblastoma-astrocyte connexin 43 gap junctions promote tumor invasion. Mol. Cancer Res. 20, 319–331. doi: 10.1158/1541-7786.MCR-21-0199
Miguel-Quesada, C., Zaforas, M., Herrera-Pérez, S., Lines, J., Fernández-López, E., Alonso-Calviño, E., et al. (2023). Astrocytes adjust the dynamic range of cortical network activity to control modality-specific sensory information processing. Cell Rep. 42:112950. doi: 10.1016/j.celrep.2023.112950
Molinaro, A. M., Hervey-Jumper, S., Morshed, R. A., Young, J., Han, S. J., Chunduru, P., et al. (2020). Association of Maximal Extent of Resection of Contrast-Enhanced and Non–Contrast-Enhanced Tumor With Survival Within Molecular Subgroups of Patients With Newly Diagnosed Glioblastoma. JAMA Oncol. 6, 495–503. doi: 10.1001/jamaoncol.2019.6143
Mu, W., Rana, S., and Zöller, M. (2013). Host matrix modulation by tumor exosomes promotes motility and invasiveness. Neoplasia 15, 875–887. doi: 10.1593/neo.13786
Nagashima, G., Suzuki, R., Asai, J., and Fujimoto, T. (2002). Immunohistochemical analysis of reactive astrocytes around glioblastoma: an immunohistochemical study of postmortem glioblastoma cases. Clin. Neurol. Neurosurg. 104, 125–131. doi: 10.1016/s0303-8467(01)00197-4
Nedergaard, M., Ransom, B., and Goldman, S. A. (2003). New roles for astrocytes: redefining the functional architecture of the brain. Trends Neurosci. 26, 523–530. doi: 10.1016/j.tins.2003.08.008
Oberheim, N. A., Wang, X., Goldman, S., and Nedergaard, M. (2006). Astrocytic complexity distinguishes the human brain. Trends Neurosci. 29, 547–553. doi: 10.1016/j.tins.2006.08.004
Obermajer, N., Muthuswamy, R., Odunsi, K., Edwards, R. P., and Kalinski, P. (2011). PGE(2)-induced CXCL12 production and CXCR4 expression controls the accumulation of human MDSCs in ovarian cancer environment. Cancer Res. 71, 7463–7470. doi: 10.1158/0008-5472.CAN-11-2449
O’Brien, E. R., Howarth, C., and Sibson, N. R. (2013). The role of astrocytes in CNS tumors: pre-clinical models and novel imaging approaches. Front. Cell Neurosci. 16:40. doi: 10.3389/fncel.2013.00040
Odemis, V., Boosmann, K., Heinen, A., Küry, P., and Engele, J. (2010). CXCR7 is an active component of SDF-1 signalling in astrocytes and Schwann cells. J. Cell Sci. 123, 1081–1088. doi: 10.1242/jcs.062810
Ogata, K., and Kosaka, T. (2002). Structural and quantitative analysis of astrocytes in the mouse hippocampus. Neuroscience 113, 221–233. doi: 10.1016/s0306-4522(02)00041-6
Ogier, C., Bernard, A., Chollet, A. M., Le Diguardher, T., Hanessian, S., Charton, G., et al. (2006). Matrix metalloproteinase-2 (MMP-2) regulates astrocyte motility in connection with the actin cytoskeleton and integrins. Glia 54, 272–284. doi: 10.1002/glia.20349
Orso, F., Virga, F., Dettori, D., Dalmasso, A., Paradzik, M., Savino, A., et al. (2023). Stroma-derived miR-214 coordinates tumor dissemination. J. Exp. Clin. Cancer Res. 42:20. doi: 10.1186/s13046-022-02553-5
Osswald, M., Jung, E., Sahm, F., Solecki, G., Venkataramani, V., Blaes, J., et al. (2015). Brain tumour cells interconnect to a functional and resistant network. Nature 528, 93–98. doi: 10.1038/nature16071
Osuka, S., and Van Meir, E. G. (2017). Overcoming therapeutic resistance in glioblastoma: the way forward. J. Clin. Invest. 127, 415–426. doi: 10.1172/JCI89587
Oushy, S., Hellwinkel, J. E., Wang, M., Nguyen, G. J., Gunaydin, D., Harland, T. A., et al. (2018). Glioblastoma multiforme-derived extracellular vesicles drive normal astrocytes towards a tumour-enhancing phenotype. Philos. Trans. R. Soc. Lond. B Biol. Sci. 373:20160477. doi: 10.1098/rstb.2016.0477
Paggetti, J., Haderk, F., Seiffert, M., Janji, B., Distler, U., Ammerlaan, W., et al. (2015). Exosomes released by chronic lymphocytic leukemia cells induce the transition of stromal cells into cancer-associated fibroblasts. Blood 126, 1106–1117. doi: 10.1182/blood-2014-12-618025
Perelroizen, R., Philosof, B., Budick-Harmelin, N., Chernobylsky, T., Ron, A., Katzir, R., et al. (2022). Astrocyte immunometabolic regulation of the tumour microenvironment drives glioblastoma pathogenicity. Brain 145, 3288–3307. doi: 10.1093/brain/awac222
Powell, E. M., and Geller, H. (1999). Dissection of astrocyte-mediatedcues in neuronal guidance and process extension. Glia 26, 73–83.
Proia, P., Schiera, G., Mineo, M., Ingrassia, A. M., Santoro, G., Savettieri, G., et al. (2008). Astrocytes shed extracellular vesicles that contain fibroblast growth factor-2 and vascular endothelial growth factor. Int. J. Mol. Med. 21, 63–67.
Pu, Y., Li, S., Zhang, C., Bao, Z., Yang, Z., and Sun, L. (2015). High expression of CXCR3 is an independent prognostic factor in glioblastoma patients that promotes an invasive phenotype. J. Neurooncol. 122, 43–51. doi: 10.1007/s11060-014-1692-y
Rash, J. E., Yasumura, T., Dudek, F. E., and Nagy, J. I. (2001). Cell-specific expression of connexins and evidence of restricted gap junctional coupling between glial cells and between neurons. J. Neurosci. 21, 1983–2000. doi: 10.1523/JNEUROSCI.21-06-01983.2001
Rose, C. R., Felix, L., Zeug, A., Dietrich, D., Reiner, A., and Henneberger, C. (2018). Astroglial glutamate signaling and uptake in the hippocampus. Front. Mol. Neurosci. 10:451. doi: 10.3389/fnmol.2017.00451
Rouach, N., Koulakoff, A., Abudara, V., Willecke, K., and Giaume, C. (2008). Astroglial metabolic networks sustain hippocampal synaptic transmission. Science 322, 1551–1555. doi: 10.1126/science.1164022
Rubin, J. B., Kung, A. L., Klein, R. S., Chan, J. A., Sun, Y., Schmidt, K., et al. (2003). A small-molecule antagonist of CXCR4 inhibits intracranial growth of primary brain tumors. Proc. Natl. Acad. Sci. U. S. A. 100, 13513–13518. doi: 10.1073/pnas.2235846100
Salmaggi, A., Gelati, M., Pollo, B., Marras, C., Silvani, A., Balestrini, M. R., et al. (2005). CXCL12 expression is predictive of a shorter time to tumor progression in low-grade glioma: a single-institution study in 50 patients. J. Neurooncol. 74, 287–293. doi: 10.1007/s11060-004-7327-y
Schwartz, H., Blacher, E., Amer, M., Livneh, N., Abramovitz, L., Klein, A., et al. (2016). Incipient Melanoma Brain Metastases Instigate Astrogliosis and Neuroinflammation. Cancer Res. 76, 4359–4371. doi: 10.1158/0008-5472.CAN-16-0485
Scoccianti, S., Perna, M., Olmetto, E., Delli Paoli, C., Terziani, F., Ciccone, L. P., et al. (2021). Local treatment for relapsing glioblastoma: A decision-making tree for choosing between reirradiation and second surgery. Crit. Rev. Oncol. Hematol. 157:103184. doi: 10.1016/j.critrevonc.2020.103184
Scott, C. A., Tattersall, D., O’Toole, E. A., and Kelsell, D. P. (2012). Connexins in epidermal homeostasis and skin disease. Biochim. Biophys. Acta 1818, 1952–1961. doi: 10.1016/j.bbamem.2011.09.004
Seike, T., Fujita, K., Yamakawa, Y., Kido, M. A., Takiguchi, S., Teramoto, N., et al. (2011). Interaction between lung cancer cells and astrocytes via specific inflammatory cytokines in the microenvironment of brain metastasis. Clin. Exp. Metastasis. 28, 13–25. doi: 10.1007/s10585-010-9354-8
Serpe, C., Michelucci, A., Monaco, L., Rinaldi, A., De Luca, M., Familiari, P., et al. (2022). Astrocytes-derived small extracellular vesicles hinder glioma growth. Biomedicines 10:2952. doi: 10.3390/biomedicines10112952
Shabtay-Orbach, A., Amit, M., Binenbaum, Y., Na’ara, S., and Gil, Z. (2015). Paracrine regulation of glioma cells invasion by astrocytes is mediated by glial-derived neurotrophic factor. Int. J. Cancer. 137, 1012–1020. doi: 10.1002/ijc.29380
Simard, M., and Nedergaard, M. (2004). The neurobiology of glia in the context of water and ion homeostasis. Neuroscience 129, 877–896. doi: 10.1016/j.neuroscience.2004.09.053
Sin, W. C., Aftab, Q., Bechberger, J. F., Leung, J. H., Chen, H., and Naus, C. C. (2016). Astrocytes promote glioma invasion via the gap junction protein connexin43. Oncogene 35, 1504–1516. doi: 10.1038/onc.2015.210
Sofroniew, M. V. (2005). Reactive astrocytes in neural repair and protection. Neuroscientist 5:226. doi: 10.1177/1073858405278321
Sofroniew, M. V. (2009). Molecular dissection of reactive astrogliosis and glial scar formation. Trends Neurosci. 32, 638–647. doi: 10.1016/j.tins.2009.08.002
Stupp, R., Mason, W. P., van den Bent, M. J., Weller, M., Fisher, B., Taphoorn, M. J., et al. (2005). Radiotherapy plus concomitant and adjuvant temozolomide for glioblastoma. N. Engl. J. Med. 352, 987–996. doi: 10.1056/NEJMoa043330
Suzuki, A., Stern, S. A., Bozdagi, O., Huntley, G. W., Walker, R. H., Magistretti, P. J., et al. (2011). Astrocyte-neuron lactate transport is required for long-term memory formation. Cell 144, 810–823. doi: 10.1016/j.cell.2011.02.018
Svokos, K. A., Salhia, B., and Toms, S. A. (2014). Molecular biology of brain metastasis. Int. J. Mol. Sci. 15, 9519–9530. doi: 10.3390/ijms15069519
Taheri, B., Soleimani, M., Aval, S. F., Memari, F., and Zarghami, N. (2018). C6 glioma-derived microvesicles stimulate the proliferative and metastatic gene expression of normal astrocytes. Neurosci. Lett. 685, 173–178. doi: 10.1016/j.neulet.2018.08.034
Trettel, F., Di Castro, M. A., and Limatola, C. (2020). Chemokines: Key molecules that orchestrate communication among neurons, microglia and astrocytes to preserve brain function. Neuroscience 439, 230–240. doi: 10.1016/j.neuroscience.2019.07.035
Ullian, E. K., Sapperstein, S. K., Christopherson, K. S., and Barres, B. A. (2001). Control of synapse number by glia. Science 291, 657–661. doi: 10.1126/science.291.5504.657
Vainchtein, I. D., Chin, G., Cho, F. S., Kelley, K. W., Miller, J. G., Chien, E. C., et al. (2018). Astrocyte-derived interleukin-33 promotes microglial synapse engulfment and neural circuit development. Science 359, 1269–1273. doi: 10.1126/science.aal3589
Valdebenito, S., Malik, S., Luu, R., Loudig, O., Mitchell, M., Okafo, G., et al. (2021). Tunneling nanotubes, TNT, communicate glioblastoma with surrounding non-tumor astrocytes to adapt them to hypoxic and metabolic tumor conditions. Sci. Rep. 11:14556. doi: 10.1038/s41598-021-93775-8
Valenti, R., Huber, V., Filipazzi, P., Pilla, L., Sovena, G., Villa, A., et al. (2006). Human tumor-released microvesicles promote the differentiation of myeloid cells with transforming growth factor-beta-mediated suppressive activity on T lymphocytes. Cancer Res. 66, 9290–9298. doi: 10.1158/0008-5472.CAN-06-1819
Valiente, M., Obenauf, A. C., Jin, X., Chen, Q., Zhang, X. H., Lee, D. J., et al. (2014). Serpins promote cancer cell survival and vascular co-option in brain metastasis. Cell 156, 1002–1016. doi: 10.1016/j.cell.2014.01.040
van den Bent, M. J., Geurts, M., French, P. J., Smits, M., Capper, D., Bromberg, J. E. C., et al. (2023). Primary brain tumours in adults. Lancet 402, 1564–1579. doi: 10.1016/S0140-6736(23)01054-1
Venkataramani, V., Yang, Y., Schubert, M. C., Reyhan, E., Tetzlaff, S. K., Wißmann, N., et al. (2022). Glioblastoma hijacks neuronal mechanisms for brain invasion. Cell 185, 2899–2917.e1. doi: 10.1016/j.cell.2022.06.054
Wang, W., Mani, A. M., and Wu, Z. H. (2017). DNA damage-induced nuclear factor-kappa B activation and its roles in cancer progression. J. Cancer Metastasis Treat. 3, 45–59. doi: 10.20517/2394-4722.2017.03
Watkins, S., Robel, S., Kimbrough, I. F., Robert, S. M., Ellis-Davies, G., and Sontheimer, H. (2014). Disruption of astrocyte-vascular coupling and the blood-brain barrier by invading glioma cells. Nat. Commun. 5:4196. doi: 10.1038/ncomms5196
Webber, J., Steadman, R., Mason, M. D., Tabi, Z., and Clayton, A. (2010). Cancer exosomes trigger fibroblast to myofibroblast differentiation. Cancer Res. 70, 9621–9630. doi: 10.1158/0008-5472.CAN-10-1722
Woroniecka, K., Chongsathidkiet, P., Rhodin, K., Kemeny, H., Dechant, C., Farber, S. H., et al. (2018). T-Cell Exhaustion Signatures Vary with Tumor Type and Are Severe in Glioblastoma. Clin. Cancer Res. 24, 4175–4186. doi: 10.1158/1078-0432.CCR-17-1846
Xing, L., Yang, T., Cui, S., and Chen, G. (2019). Connexin Hemichannels in Astrocytes: Role in CNS Disorders. Front. Mol. Neurosci. 12:23. doi: 10.3389/fnmol.2019.00023
Xu, J., Zhang, J., Zhang, Z., Gao, Z., Qi, Y., Qiu, W., et al. (2021). Hypoxic glioma-derived exosomes promote M2-like macrophage polarization by enhancing autophagy induction. Cell Death Dis. 12:373. doi: 10.1038/s41419-021-03664-1
Yang, J. K., Liu, H. J., Wang, Y., Li, C., Yang, J. P., and Yang, L. (2019). Exosomal miR-214-5p released from glioblastoma cells modulates inflammatory response of microglia after lipopolysaccharide stimulation through targeting CXCR5. CNS Neurol. Disord. Drug Targets 18, 78–87. doi: 10.2174/1871527317666181105112009
Ye, Z. C., and Sontheimer, H. (1999). Glioma cells release excitotoxic concentrations of glutamate. Cancer Res. 59, 4383–4391.
Yu, S., Liu, C., Su, K., Wang, J., Liu, Y., Zhang, L., et al. (2007). Tumor exosomes inhibit differentiation of bone marrow dendritic cells. J. Immunol. 178, 6867–6875. doi: 10.4049/jimmunol.178.11.6867
Zhang, L., Zhang, S., Yao, J., Lowery, F. J., Zhang, Q., Huang, W. C., et al. (2015). Microenvironment-induced PTEN loss by exosomal microRNA primes brain metastasis outgrowth. Nature 527, 100–104. doi: 10.1038/nature15376
Zhang, L., and Zhang, Y. (2015). Tunneling nanotubes between rat primary astrocytes and C6 glioma cells alter proliferation potential of glioma cells. Neurosci. Bull. 31, 371–378. doi: 10.1007/s12264-014-1522-4
Zonari, E., Pucci, F., Saini, M., Mazzieri, R., Politi, L. S., Gentner, B., et al. (2013). A role for miR-155 in enabling tumor-infiltrating innate immune cells to mount effective antitumor responses in mice. Blood 122, 243–252. doi: 10.1182/blood-2012-08-449306
Zong, H., Parada, L. F., and Baker, S. J. (2015). Cell of origin for malignant gliomas and its implication in therapeutic development. Cold Spring Harb. Perspect Biol. 7, a020610. doi: 10.1101/cshperspect.a020610
Keywords: non-neoplastic astrocytes, primary brain tumors, metastatic brain tumors, glioma, tumor microenvironment
Citation: Catalano M, Limatola C and Trettel F (2024) Non-neoplastic astrocytes: key players for brain tumor progression. Front. Cell. Neurosci. 17:1352130. doi: 10.3389/fncel.2023.1352130
Received: 07 December 2023; Accepted: 26 December 2023;
Published: 16 January 2024.
Edited by:
Miren Revuelta, University of the Basque Country, SpainReviewed by:
Shweta Pradip Jadhav, Consultant, Carlsbad, CA, United StatesCopyright © 2024 Catalano, Limatola and Trettel. This is an open-access article distributed under the terms of the Creative Commons Attribution License (CC BY). The use, distribution or reproduction in other forums is permitted, provided the original author(s) and the copyright owner(s) are credited and that the original publication in this journal is cited, in accordance with accepted academic practice. No use, distribution or reproduction is permitted which does not comply with these terms.
*Correspondence: Flavia Trettel, ZmxhdmlhLnRyZXR0ZWxAdW5pcm9tYTEuaXQ=