Ca2+-sensors and ROS-GC: interlocked sensory transduction elements: a review
- Research Divisions of Biochemistry and Molecular Biology, The Unit of Regulatory and Molecular Biology, Salus University, Elkins Park, PA, USA
From its initial discovery that ROS-GC membrane guanylate cyclase is a mono-modal Ca2+-transduction system linked exclusively with the photo-transduction machinery to the successive finding that it embodies a remarkable bimodal Ca2+ signaling device, its widened transduction role in the general signaling mechanisms of the sensory neuron cells was envisioned. A theoretical concept was proposed where Ca2+-modulates ROS-GC through its generated cyclic GMP via a nearby cyclic nucleotide gated channel and creates a hyper- or depolarized sate in the neuron membrane (Ca2+ Binding Proteins 1:1, 7–11, 2006). The generated electric potential then becomes a mode of transmission of the parent [Ca2+]i signal. Ca2+ and ROS-GC are interlocked messengers in multiple sensory transduction mechanisms. This comprehensive review discusses the developmental stages to the present status of this concept and demonstrates how neuronal Ca2+-sensor (NCS) proteins are the interconnected elements of this elegant ROS-GC transduction system. The focus is on the dynamism of the structural composition of this system, and how it accommodates selectivity and elasticity for the Ca2+ signals to perform multiple tasks linked with the SENSES of vision, smell, and possibly of taste and the pineal gland. An intriguing illustration is provided for the Ca2+ sensor GCAP1 which displays its remarkable ability for its flexibility in function from being a photoreceptor sensor to an odorant receptor sensor. In doing so it reverses its function from an inhibitor of ROS-GC to the stimulator of ONE-GC membrane guanylate cyclase.
Introduction
The discovery and molecular characterization of the photoreceptor ROS-GC is a land mark event in the photo-transduction field (reviewed in Pugh et al., 1997; Koch et al., 2010). It filled in the gap on the identity of the source of cyclic GMP that serves as a second messenger of the LIGHT signal; and made it possible to explain the principles of photo-transduction machinery in molecular and physiological terms.
It also impacted the core membrane guanylate cyclase field by the branching of the guanylate cyclase family into two subfamilies. The family became a transducer of both the extracellular peptide hormonal and the intracellularly genearted [Ca2+]i signals. Before, it was believed to be the sole transducer of the peptide hormone signals (reviewed in: Sharma, 2010).
Prior to its discovery in 1994, an intense search was on the identification of a guanylate cyclase unique to photo-transduction. It was known that both [Ca2+]i and cyclic GMP are the critical messengers of the photon signal in the vertebrate photoreceptors. How these signals are generated and interact with each other was not recognized, however, (early reviews: Pugh and Cobbs, 1986; Stryer, 1986). Early reports on the successful identification of photoreceptor ROS-GC contradicted each other, and created a lot of confusion. One of these reports even suggested that this Ca2+-sensitive membrane guanylate cyclase is nitric oxide sensitive, and its molecular mass is 67 kDa (Horio and Murad, 1991a,b). Another major perception was that the photo-transduction-linked membrane guanylate cyclase consists of “separate regulatory and catalytic subunits” (Stryer, 1991). In yet another report it was reported to be cloned from the human retina library (Shyjan et al., 1992). It was named retGC and via in situ hybridization was detected only in the inner segments and outer nuclear layers of the monkey's retina, the segments and the layers not linked with photo-transduction.
ROS-GC Discovery
These contradictions on the true identity of the photoreceptor ROS-GC were resolved by establishing its direct purification from the bovine outer segments (OS) (Margulis et al., 1993), the site of photo-transduction. Its protein-sequence-based molecular cloning, structure, and function demonstrated that it is not any of the earlier, including retGC, membrane guanylate cyclases (Goraczniak et al., 1994). Its theoretical molecular mass is 120, 360 Da, in general agreement with the earlier 110 kDa value reported for the biochemically purified forms of bovine and frog forms (Hayashi and Yamazaki, 1991; Koch, 1991). It was settled that unlike other family members, it is not a natriuretic peptide hormone surface receptor membrane guanylate cyclase.
The molecular identities of the photoreceptor ROS-GC from the rod outer segments (ROS) and two other members of the natriuretic peptide receptor family, ANF-RGC (Kutty et al., 1992) from the rat retina and CNP-RGC (Duda et al., 1993) from the human retina, demonstrated that the retinal neurons contain both the surface receptor and ROS-GC sub-families, but only ROS-GC is potentially linked with the photo-transduction machinery. Two important conspicuous structural differences were noted between the two sub-families. One was that ROS-GC beyond the catalytic domain contains a C-terminal extension tail of 90 amino acids, Y965-K1054; the second was that the signature ATP-regulated domain, Gly-X-X-X-Gly of the natriuretic peptide hormone receptors, CNP-RGC and ANF-RGC, is missing in ROS-GC. These differences play a key role in determining the cellular and functional specificity of the ROS-GC containing neurons.
Significantly, among all the family members, ROS-GC is the only membrane guanylate cyclase that has been cloned on the basis of its protein sequence. This approach experimentally validated the position of the N-terminus amino acid of the mature protein and demonstrated that the immature protein contains a 56 amino acid N-terminus hydrophobic signal peptide. The theoretical molecular mass of the protein with its signal peptide is 120, 361 Da and without it is 114, 360 Da.
This approach of its identification also played a key role in demonstrating that the structure of the originally cloned human retGC (Shyjan et al., 1992) was a cloning artifact. Consistent with this fact, in the January 1995 GenBank data (Accession number M92432) the structure of human retGC was revised to match it with the bovine ROS-GC. Thereby, the revised retGC became a human counter part of the bovine ROS-GC.
ROS-GC, a Two-Component Transduction System
Before the characterization of photoreceptor ROS-GC and the coined-terminology for the guanylate cyclase activating protein as GCAP, a key observation showed that a GCAP stimulates a membrane guanylate cyclase in the bovine ROS in a Ca2+-dependent fashion (Koch and Stryer, 1988). An essential feature of this GCAP was that it was a cytosolic factor. Ca2+-bound, it inhibited the membrane guanylate cyclase activity. This was the first hint that ROS-GC is a two-component [Ca2+]i transduction system and that the system had an unusual property of being inhibited by the [Ca2+]i signal. With the availability of the recombinant (r)ROS-GC, it was now possible to test and study this unusual feature of the native photoreceptor ROS-GC in its isolated form. All prior membrane guanylate cyclases were only stimulated by their peptide hormone signals.
Fortuitously, at almost the same time the cloning of ROS-GC was reported (Goraczniak et al., 1994), the cloning of two forms of GCAP, GCAP1 (Palczewski et al., 1994; Subbaraya et al., 1994; Frins et al., 1996) and GCAP2 (Dizhoor et al., 1995) was also reported. They were the Ca2+ modulators of one, or more, native ROS guanylate cyclase/s with undetermined identities. Their relationship with the cloned ROS-GC was not known.
The availabilities of the cloned forms of ROS-GC1 and GCAP1 made it possible to test if these two Ca2+ transduction elements were linked, together constituting one ROS-GC transduction system.
They indeed were (Duda et al., 1996). ROS-GC1 expressing heterologous system of COS cells responded at 10 nM [Ca2+]i to the GCAP1 stimulation in a dose dependent manner. The stimulation was incrementally inhibited by free Ca2+ with a K1/2 of 100 nM. In contrast, under identical conditions, GCAP1 had no effect on the recombinant ANF-RGC, the peptide hormone receptor. An additional important characteristic of the transduction system was that the GCAP remained bound to ROS-GC at the low and high Ca2+ levels, consistent with the physiological observations (Koutalos et al., 1995). These results demonstrated that (1) rROS-GC mimicked the native ROS-GC present in the ROS in its Ca2+-modulation, and, therefore, functionally it was identical to the native enzyme; (2) It received its Ca2+ signals through GCAP1; therefore, the GCAP was its [Ca2+]i sensor and the transmitter component; and ROS-GC was the signal receiver and the transducer component; (3) GCAP always remains bound to ROS-GC regardless of the [Ca2+]i concentration; and (4) the ROS-GC transduction system differed from the peptide hormone receptor family members in being solely the transducer of the intracellularly generated Ca2+ signals within the light-sensitive OS.
In this manner the biochemical and physiological identity of the native with its cloned form photoreceptor ROS-GC1 was established, and also its linkage with photo-transduction. It was concluded that the ROS-GC is a two-component transduction system where the Ca2+-sensor GCAP element is interlocked with the ROS-GC transducer element and the change in [Ca2+]i level defines the activity state of ROS-GC. This interlocked transduction system represented a new paradigm of the fields of membrane guanylate cyclase and the NCS proteins.
Similar conclusions based on the reconstitution studies with retGC established that GCAP2 is another NCS protein linked with photo-transduction (Dizhoor et al., 1994, 1995). In addition, the molecular identity of the second member of the ROS-GC sub-family, retGC2, from the ROS of the human and bovine retinas was established (Lowe et al., 1995; Goraczniak et al., 1997). To distinguish between the two ROS-GCs, the original ROS-GC was termed ROS-GC1 and the second as ROS-GC2.
Thus, two GCAPs and two ROS-GCs, bound to each other, reside in the OS of the rods. Because only ROS-GC1 was able to be purified directly from the ROS, it was perceived that ROS-GC1 was the dominant guanylate cyclase in ROS. This perception was validated by the direct quantitative estimation of the two isozymes in the bovine ROS. Their ratios are 96% ROS-GC1 and 4% ROS-GC2 (Helten et al., 2007). The latest estimation in the mouse shows the same pattern, yet, slightly higher concentration of ROS-GC2, 76% ROS-GC1, and 24% ROS-GC2 (Peshenko et al., 2011). However, in contrast to the bovine, the mouse study was made with the total OS instead of the isolated membrane fractions.
Because most of the studies have been conducted with ROS-GC1, the following discussion will deal with this transduction system only. It is, however, noted that GCAP2 appears to be the only natural sensor of ROS-GC2 and its target site resides between the aa736–1010 domain, quite different from the corresponding domain for the GCAP1 site in ROS-GC1 domain (Goraczniak et al., 1997). In contrast, the discussion below will indicate that both GCAP1 and GCAP2 are critical Ca2+ sensor elements of ROS-GC1.
Photo-Transduction Model
Photo-transduction is the biochemical process by which the rods and cones convert the incoming LIGHT signal into the electrical signal. Enlightened by the ROS specific features of the interlocked GCAP/ROS-GC transduction system, a model for its role in operation of the photo-transduction machinery was proposed (Pugh et al., 1997; this review covers the historical development of the membrane guanylate cyclase related photo-transduction field up to June 1997; others subsequently published are: (Pugh et al., 1999; Burns and Baylor, 2001; Koch et al., 2002, 2010; Sharma, 2002; Sharma et al., 2004; Luo et al., 2008; Stephen et al., 2008; Wensel, 2008).
This model with some advanced features is presented in Figure 1.
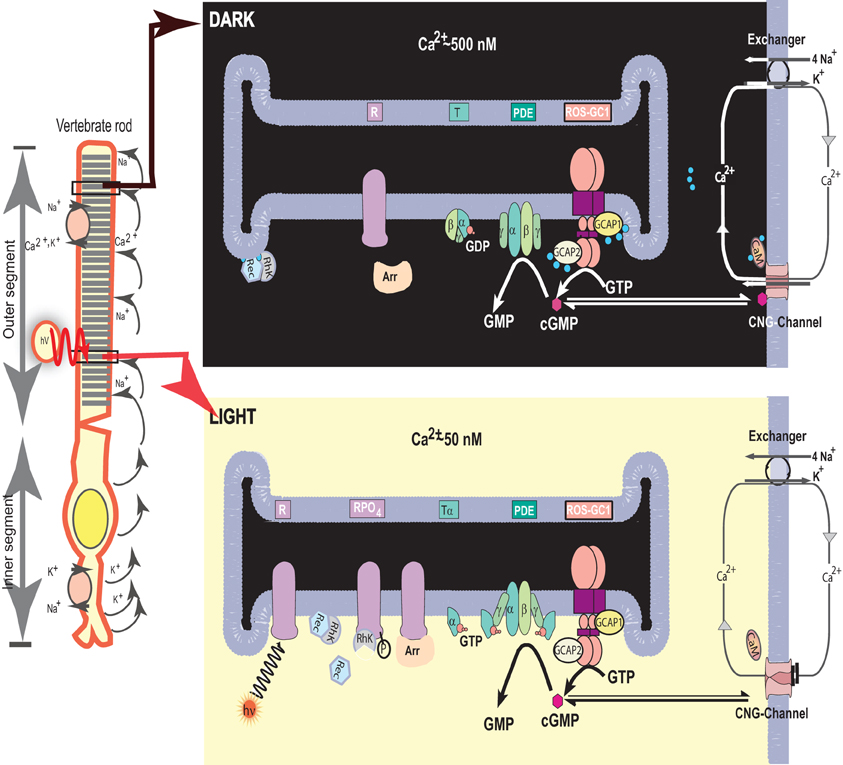
Figure 1. Photo-transduction model. Left panel. An illustration of a typical vertebrate rod. In the dark a circulating current (arrows) is present, which is outward in the inner segment and carried primarily by K+; in the outer segment the net charge flow is inward, with about 90% of the inward flow carried by Na+ and 10% by Ca2+ ions. Na+/K+ exchange pumps in the inner segment membrane and Na+/K+-Ca2+ exchangers in the outer segment membrane (see also right panels) maintain the overall ionic gradients against the dark flows. The capture of a photon (hν) by a rhodopsin molecule in one of the disc membranes of the outer segment initiates the photo-transduction cascade. Right upper panel. The components of the photo-transduction cascade are shown in the dark/resting steady-state. A high concentration of cytoplasmic cGMP keeps a fraction of CNG-channels in the plasma membrane open. Ca2+-ions enter the cell via the CNG-channel and are extruded via the Na+/K+, Ca2+-exchanger. Synthesis and hydrolysis of cGMP by ROS-GC and PDE occur at a low rate. The heterotrimeric G protein transducin (T) is in its GDP-bound state and is inactive. The Ca2+-binding proteins calmodulin (CaM) recoverin (Rec), and GCAPs bind to their target proteins, the CNG-channel, rhodopsin kinase (RhK) and ROS-GC, respectively. Right lower panel. Absorption of light by the visual pigment rhodopsin leads to the activation of the transduction cascade: the GTP-bound α-subunit of transducin activates PDE that rapidly hydrolyzes cGMP. Subsequently the CNG-channels close and the Ca2+-concentration decreases. The change in cytoplasmic [Ca2+] is sensed by Ca2+-binding proteins: CaM dissociates from the CNG-channel which leads to an increase in cGMP sensitivity of the channel, inhibition of rhodopsin kinase by recoverin is terminated and rhodopsin can be phosphorylated. GCAPs activate ROS-GC and synthesis of cGMP increases. Arrestin (Arr) binds to phosphorylated rhodopsin and interferes with the binding and further activation of transducin. Enhancement of cGMP synthesis and termination of the cascade leads to reopening of CNG-channels. [composed from Figure 1 Pugh et al. (1997) and Figure 1 Koch et al. (2002), and reprinted with the permission from those references].
In this model ROS-GC is the central component of the photo-transduction machinery. It generates cyclic GMP, the second messenger of the LIGHT signal. Its key feature is that it regulates and is regulated by the produced [Ca2+]i signal. Thereby, the accelerated production of [Ca2+]i inhibits and the decelerated production accelerates its operation. Cyclic GMP and [Ca2+]i create a feedback loop in the photo-transduction machinery. The machinery operates in the decelerated mode within 50 nM to the 250 nM [Ca2+]i range.
For almost two decades this model has served as a template in advancing the molecular, biochemical and physiological principles of the photo-transduction operation. These advancements in the model are briefly outlined below; their details are available in the cited references.
Advancements
GCAPs
As the entry of the molecular form of ROS-GC dawned on the era of decoding the molecular principles of photo-transduction machinery, so was the entrance of recoverin for the age of NCS protein field. First reported on its presence in the rods and cones of photoreceptors and its direct Ca2+-dependent regulation of guanylate cyclase, it was named recoverin referring to its function “because it promotes recovery of the dark state” (Dizhoor et al., 1991). Its linkage with true ROS-GC was concluded because it reportedly inhibited the catalytic activity of guanylate cyclase in the range of 450–40 nM of free Ca2+. However, this conclusion and its role in recovery was withdrawn (Hurley et al., 1993), yet it represents the first member of the NCS protein family. It is present in the photoreceptors; in its new role, it senses Ca2+ signals, inhibits rhodopsin kinase, and thereby, plays a role in light adaptation (Makino et al., 2004; Philippov et al., 2007).
An important contribution of recoverin's entry into the field of NCS proteins is related to the identification of its four Ca2+-specific structural motifs, these EF hands define the general Ca2+ sensor property of the NCS family. Only two are functional in recoverin, however.
The GCAPs, 1 and 2, constitute a sub-family of the NCS proteins (reviewed in Koch et al., 2010). The NCS superfamily is grouped into five subfamilies of recoverins, VILIPs, frequenins, KCHIPs, and GCAPs (reviewed in: Nef, 1996; Braunewell and Gundelfinger, 1999; McCue et al., 2010). These subfamilies perform diverse regulatory processes, which include gene expression, ion channel function, enzyme modulations like that of kinases, guanylate cyclases, and adenylate cyclases, membrane trafficking of ion channels and receptors, and control of apoptosis.
This section focuses only on the GCAPs, 1 and 2: expression, properties, and physiological functions, the reader is referred to a recent review for the details (Koch et al., 2010). However, it is noted that the number of GCAPs has expanded with their findings in several mammalian, amphibian, and teleost species. While mammals express only 2–3 GCAP isoforms, 6–8 isoforms are found in the retina of teleost fish (Rätscho et al., 2010).
Expression
GCAP1 and GCAP2 are expressed in the same concentration of 3 μM in the native bovine ROS (Hwang et al., 2003). Their quantitative, relative, and cellular concentrations in the rods and cones of any other species are not known. Immunocytochemical studies show, however, that both GCAPs are present in the outer and inner segments of rods and cones (Gorczyca et al., 1995; Frins et al., 1996). Importantly, their presence in cone synaptic pedicles can be clearly defined (Venkataraman et al., 2003).
Scattered reports based on immunohistochemical studies show varying results in the same or different species. Examples are: the expression of GCAP1 is minimal in the ROS of human, monkey, and bovine retina but is maximal in the cone OS of these species (Kachi et al., 1999); GCAP2 is expressed in both outer and inner segments of the bovine rods and cones (Dizhoor et al., 1995); monkey retinas express minimal level of GCAP2 in OS of the rods and cones and in the rod inner segments (Otto-Bruc et al., 1997). These variations might be resolved on the basis of species-specifications (Cuenca et al., 1998), yet it appears that, in general, the rods and cones of all mammals express both GCAPs.
Curiously, in some species, like human and zebrafish (but not mice), GCAP3 appears as a cone-specific isoform of GCAP (Imanishi et al., 2002). And the zebrafish isoforms GCAP4, GCAP5, and GCAP7 also exhibit cone-specific expression patterns (Imanishi et al., 2004).
Biochemical Features
Ca2+ binding
Like most other members of the NCS-protein family, GCAPs harbor four EF-hand Ca2+-binding motifs, of which three are functional (reviewed in Koch et al., 2010). These EF-hands have a nanomolar affinity for Ca2+ and they are the Ca2+-sensor elements linked with photo-transduction.
In a recent proposal for GCAP1, the transition from the DARK State to the Illuminated State of the photoreceptors occurs by the substitution of bound Ca2+ with the bound Mg2+ to ROS-GC1 (Peshenko and Dizhoor, 2006, 2007). Ca2+ associates with GCAP1 with a rate of ∼ 2 × 108 M−1 s−1 (kon) which is close to the diffusion limit (Sokal et al., 1999). The apparent dissociation constants of each EF-hand for Ca2+ are between 0.08 and 0.9 μM and between 0.1 and 1.6 μM in the absence and presence of 2 mM Mg2+, respectively (Lim et al., 2009). Thus, these nanomolar affinities result in fast dissociation rates (for example, koff = kon × KD = 2 × 108 M−1 s−1 × 0.2 × 10−6 M) = 40 s−1; 1/koff = 25 ms), consistent with the kinetics of the vertebrate photoresponse.
GCAP2 is similar, yet not identical, in its Ca2+-binding properties. The second, third, and fourth EF-hands are functional. They display an apparent KD of 300 nM (Ames et al., 1999), but no specific assignment for the affinity of each EF-hand has been made so far. It appears, however, that EF-hands two and four influence Ca2+ sensitivity of GCAP2 more than EF-hand three (Dizhoor and Hurley, 1996). Cysteine accessibility study with GCAP2 mutants shows its restricted reactivity toward Cys111 at sub-micromolar Ca2+-concentrations, indicating that within the Ca2+ concentration range where ROS-GCs are regulated, Ca2+-induces conformational changes in GCAP2 (Helten et al., 2007). Thus, these are the fluctuations in the free Ca2+ concentrations of the rods and cones that control and signal GCAPs to transmit their messages to the bound ROS-GC and be transduced in the generation of cyclic GMP, the second messenger of photo-transduction.
Myristoylation
Given the facts that GCAPs are the members of the NCS protein family and its member recoverin, also expressed in photoreceptor cells, is acylated at its N-terminus and is embodied with a striking regulatory switch termed “Ca2+-myristoyl switch” (Zozulya and Stryer, 1992), these recoverin's features were investigated for GCAPS. The findings showed that the GCAPs are, indeed, heterogeneously acylated at their N-termini; myristoyl group being of the prominent form (Palczewski et al., 1994; Olshevskaya et al., 1997). However, GCAPs do not undergo a classical calcium-myristoyl switch (Olshevskaya et al., 1997; Hwang and Koch, 2002a,b). They do not bury the myristoyl group in a hydrophobic pocket in the Ca2+-free form and expose it when in Ca2+-bound form (Zozulya and Stryer, 1992). This conclusion was validated by the crystallographic study of GCAP1 which showed that the group is always buried (Stephen et al., 2007).
Additional investigations demonstrated that the myristoyl group has a strong impact on the regulatory properties of GCAPs (Hwang and Koch, 2002a,b; Hwang et al., 2003): (1) It influences the Ca2+ sensitivity of GCAP1, but not of GCAP2, shifting its IC50 value for Ca2+ in the regulation of ROS-GC1. (2) It increases by sevenfold the affinity of GCAP1 for ROS-GC1. On the other hand, it has no effect on GCAP2. (3) It differentially regulates the catalytic efficiency, kcat/Vmax, of ROS-GC1 regulation by GCAP1 and GCAP2; the influence of the myristoyl group on catalytic efficiency is larger for GCAP1 than for GCAP2.
ROS-GC Modulation
In preceding, and then parallel, studies to those that led to the conclusion that GCAPs are differential Ca2+ sensors, a similar conclusion was arrived at. These studies involved comprehensive technology of soluble constructs of ROS-GC1, direct binding measurements by surface plasmon resonance (SPR) spectroscopy, co-immunoprecipitation and functional reconstitution utilizing progressive deletion constructs and peptide competition. The first study via the application of ROS-GC1 deletion and ANF-RGC/ROS-GC1 hybrid mutants which retained only the catalytic domain of ROS-GC1 established that the GCAP1- and GCAP2-modulated domains in ROS-GC1 are separate and that they reside on the opposite ends of the catalytic domain (Krishnan et al., 1998). The study also showed that GCAP2 signals ROS-GC1 activation under the physiological conditions of Ca2+ with a K1/2 of 10 nM, inhibits its activity with an IC50 value of 140 nM; and, importantly, it concluded that the intracellular region of ROS-GC1 “is composed of multiple modules, each designed to mediate a particular calcium-specific signaling pathway.” It will become clear from the discussion below that the architecture of the intracellular domain of ROS-GC1 is, indeed, multi-modular and each module is pre-visioned to control the intensity and shape of each type of Ca2+ signal into the given production of cyclic GMP.
The mapped region of GCAP1 constitutes two domains of ROS-GC1: transduction, M445-L456 and binding, L503-I522 (Lange et al., 1999), and the GCAP2-modulated binding and the transduction site reside in the Y965-N981 region of ROS-GC1 (Duda et al., 2005).
A comment is in order for the role of GCAP2 in Ca2+ signaling. In contrast to GCAP1, GCAP2 is the specific physiological Ca2+ sensor component of ROS-GC2 (Goraczniak et al., 1998; Haeseleer et al., 1999). Its IC50 value for Ca2+ is 150 nM; compared to GCAP1 for ROS-GC1, its EC50 value for ROS-GC2 activation is about one order of magnitude lower: compare 1 μM with 8 μM (Goraczniak et al., 1998). This feature of GCAP2 has been substantiated by the recent studies, yet they show that GCAP1 with less specificity is also the stimulant of ROS-GC2 (Helten and Koch, 2007; Peshenko et al., 2011). Importantly, the study with ROS-GC2 deletion and ANF-RGC/ROS-GC2 hybrid mutant, which retained only the catalytic domain of ROS-GC2, demonstrated that the GCAP2-modulated domain in ROS-GC2 resides at its C-terminus region of the catalytic domain. The site has not been precisely mapped, yet almost certainly it is very similar or almost identical to the corresponding ROS-GC1 site for GCAP2. Thus, it clearly indicated that the Ca2+ signal transduction mechanisms of two ROS-GCs in photo-transduction are very different.
Additional analysis indicated that GCAP1 and GCAP2 have different ranges of [Ca2+] in which they operate. Activation of ROS-GC1 by GCAP1 is half-maximal at 707 nM and that by GCAP2 at 100 nM (Hwang et al., 2003). In addition to Ca2+, Mg2+ is essential for GCAP function. It is an essential cofactor for the ROS-GC cyclization reaction. Decreasing [Mg2+] from 5 to 0.5 mM lowers, by almost one order of magnitude, the [Ca2+] IC50 values of both GCAPs (Peshenko and Dizhoor, 2004). Thus, fluctuations of [Mg2+] in a photoreceptor cell also influence the dynamic range of cyclase regulation. However, free [Mg2+] apparently does not change significantly during illumination (Chen et al., 2003).
Ca2+-Relay Model
Integration of the differential Ca2+ sensing properties of the GCAPs with the facts that their targeted sites in ROS-GC1 are also different have resulted in the current “Ca2+-relay model” of photoreceptor guanylate cyclase activation (Koch, 2006; Koch et al., 2010). The key component of this model is that there is a switch which converts GCAP1 mode to a GCAP2 mode of the ROS-GC operation during the light-induced fall in cytoplasmic [Ca2+] (Hwang et al., 2003; Koch, 2006; Burgoyne, 2007). Both GCAP1 and GCAP2 are present in almost equal concentrations and their combined concentration is equal to the concentration of a ROS-GC1 dimer (Hwang et al., 2003), “both GCAPs bind to a ROS-GC1 dimer in the dark state of the cell. In this state the ROS-GC1 activity is very low, just sufficient to maintain the cytoplasmic dark concentration of cyclic GMP by keeping a balance with the low dark-state activity of the cyclic GMP hydrolyzing enzyme, phosphodiesterase. Illumination of rod or cone cells leads to a decrease in cyclic GMP and consequently to fall in [Ca2+]i. Depending on the light conditions and on the bleaching protocol [Ca2+]i reaches an intermediate level, at which only GCAP1 becomes an activator of ROSGC1. Further decrease of [Ca2+]i (by stronger illumination for example) would also transform GCAP2 into an activator. This differential activating modus is in accordance with the observed differences in Ca2+-sensitivity and shows that ROS-GC1 is regulated by GCAP1 and GCAP2, exhibiting differences in Ca2+-sensitivity. Thus, the illumination-intensity-dependent regulation of ROS-GC1 is switched from a “GCAP1 mode” to a “GCAP2 mode” or vice versa (Hwang et al., 2003). Under constant background light the [Ca2+]i reaches a new steady state value. In this manner, these two modes operate at different light intensities depending on the free [Ca2+]i level” (Koch et al., 2010).
This model (Sharma, 2010), depicting the illumination intensity-dependent modulation of GCAPs in the Ca2+ signaling of ROS-GC1 is presented in Figure 2.
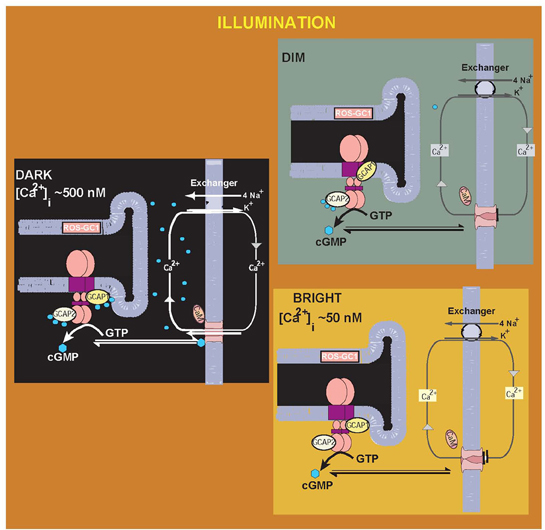
Figure 2. Illumination intensity-dependent modulation of GCAPs in the [Ca2+]i signaling of ROS-GC1: a Model. In the “DARK,” [Ca2+]i concentration is high, the Ca2+-bound sensors GCAP1 and GCAP2 are bound to ROS-GC1, ROS-GC1 activity is basal, generating ground-level cytoplasmic cyclic GMP. The cyclic GMP keeps a fraction of CNG-channels in the plasma membrane open. Ca2+ ions enter the cell via the CNG channel and are extruded via the Na+/K+, Ca2+ exchanger. The outer segments of the rods and cones are in the depolarized state. DARK to the “DIM” or intermediate LIGHT state. The initial fall of [Ca2+]i is selectively detected by GCAP1, in its Ca2+-free state GCAP1 attains the activated mode. Transition to the “Bright” LIGHT state, the CNG channels are totally closed, both GCAP1 and GCAP2 are Ca2+-free and are in the activated mode, ROS-GC1 activation is full and membranes of the rod and cone outer segments are in the hyperpolarized state. [adapted and reprinted from Figure 2: with permission from Sharma (2010)].
The “Ca2+-relay model” explains the findings on a transgenic GCAP null mice study where the role of GCAP2 could not be concluded (Howes et al., 2002). In these mice, flash responses from rods differ from the wild-type responses in two aspects. (1) The fast recovery shortly after the maximum response amplitude is missing; (2) In some cases, the flash ends in a prominent undershoot. According to the model (Hwang et al., 2003), in case 1, because GCAP1 is missing, there is no fast recovery to the single flash. In case 2, the exogenous addition of GCAP2 causes a delayed activation of ROS-GC at lower [Ca2+]i than GCAP1.
In a striking contrast to the secondary structural differences between the GCAPS modus operandi, the indication is that the Ca2+ signaling of GCAP2 causes its reversible dimerization and it is a necessary requisite to activate ROS-GCs (Olshevskaya et al., 1999). In contrast, the dimeric form of GCAP1 is inactive and does not result in activation of the ROS-GC (Hwang et al., 2004).
The knowledge gained through these studies (vide supra) has now begun to make inroads into defining the molecular events that result in migration of the Ca2+ signals to ROS-GC1 catalytic domain. Recall, the targeted domains of two GCAPs are far apart, on the opposite sides of the catalytic domain of ROS-GC1, and they perform non-overlapping tasks. The natural wisdom would be that the signaling pathways of these two GCAPs would be through distinct mechanisms.
In the first study to address this issue, the role of 657WTAPELL663 motif of ROS-GC1 in signal migration of the two GCAPs has been analyzed. The reason behind choosing this motif was that it is conserved in all members of the membrane guanylate cyclase family; and based on the ANF-RGC signaling template, it is critical for its regulatory catalytic activity (Duda et al., 2009). The question was: would this motif be also critical in ROS-GC1 signaling.
The following parameters of the motif were analyzed (Duda et al., 2011). (1) Is the motif critical for the ROS-GC1 catalytic activity? The comparative studies with a ROS-GC1 mutant in which the 657WTAPELL663 motif was deleted (Δ657WTAPELL663 mutant) demonstrated that the motif has no role on the structural integrity of the guanylate cyclase. It is, however, critical for the Ca2+ signal transduction activities of both GCAPs. Thus, it controls the total Ca2+ signaling activity of ROS-GC1. (2) Is the motif involved in the GCAPs binding to their target sites of ROS-GC1? This problem was analyzed in vivo by monitoring the interaction of GCAPs with ROS-GC1 and the deletion mutant in co-transfected cells through immnunofluorecence and in vitro by co-immunoprecipitation. The findings were that both GCAPs in their isolated forms are cytosolic proteins; yet when co-expressed with ROS-GC1 or its deletion-mutant, they are membrane bound with the respective cyclases. These results demonstrated that WTAPELL motif does not control the binding characteristics of any GCAP to its ROS-GC1 domain. This conclusion was validated by the co-immunoprecipitation experiments conducted with the COS cell membranes expressing wt-ROS-GC1 or the Δ657WTAPELL663 mutant. Both, the wt and the mutant precipitated with GCAP1 and GCAP2. (3) Which residues in the motif are critical in ROS-GC1 signaling? This question was resolved by scanning one by one the residues of the motif. W657 was found to be the most critical residue. It controlled 73% of the GCAP1 and 70% of the GCAP2 activity. The other three residues—T658, P660, and E661—individually controlled about 40% of the GCAP1 or GCAP2 signaling activity of ROS-GC1. Thus, all the four residues, marked in bold, of the 657 WTAPELL663 motif are important for the Ca2+-modulated signaling activities of the GCAPs, yet the most critical is W657 residue, accounting for about 70% of the total signaling activity.
Inroads into the Gene-Linked Retinal Diseases
For a recent comprehensive review on this topic the reader is referred to (Hunt et al., 2010; earlier findings up to the year 2002 are covered in Duda and Koch, 2002). What follows is a brief narration of the ROS-GC gene linked diseases that marked the early entry from basic to clinical medicine (reviewed in Sharma, 2010).
With the knowledge of the complete structural identity and organization of the ROS-GC1 gene (Duda et al., 1998) and of the mechanism by which its coded enzyme modulates the Ca2+ signal transduction it was now possible to investigate the human retinal diseases at the genetic levels and explain them in biochemical terms. In the first study, GCAP1 regulatory regions identified in the original study (Lange et al., 1999) gave a hint toward explaining one of the molecular causes of the rod dystrophy Leber's congenital amaurosis type 1 (LCA1), where the patients are born blind or become blind soon after birth. In these patients there is a point mutation (F514S, numbering for the bovine gene; in humans F565S) in the ROS-GC1 gene within the region L503-I522 (Perrault et al., 1996).
Biochemical studies with the heterologously expressed ROS-GC1 mutants demonstrated that this LCA mutant has almost completely (84%) lost its basal activity and sensitivity to modulation by GCAP1 (Duda et al., 1999). These studies proved therefore, that a region in proximate distance to the transmembrane region is critical for interaction and/or regulation by GCAP1 (vide supra). And this region is incapacitated in the diseased state.
A similar approach was used to investigate the second type of retinal disease that correlates with mutations of the ROS-GC1 gene, named cone-rod dystrophy type 6 (CORD 6). Patients who suffer from this disease carry one or several point mutations in the dimerization domain of ROS-GC1 (Kelsell et al., 1998). One form of the mutation is ROS-GC1-E786D,R787C,T788M. The dimer formation in this mutant is disturbed. This results in a reduced basal guanylate cyclase activity (Duda et al., 1999, 2000; Tucker et al., 1999). However, sensitivity of this mutant to GCAP1 and GCAP2 is increased. Physiological consequences of these mutations are a change in the trigger of the Ca2+-feedback and thereby causing a shift in the response-intensity curve to lower light intensities. These effects explain the photophobia often reported by CORD 6 patients and the accumulating light damage of the retina leading to loss of cone and rod vision over a time period of decades. Full accounts of the biochemical and physiological aspects of LCA1 and CORD 6 associated retinal diseases have been covered in reference (Duda and Koch, 2002).
Several point mutations in the GCAP1 gene are linked with inherited progressive cone-rod dystrophies (reviewed in: Behnen et al., 2010). These are: P50L, E89K, Y99C, D100E, N104K, I143N/T, L151F, E155G, and G159V. While the P50L mutation does not change the activating properties of GCAP1 (Newbold et al., 2001), the other mutations significantly alter the activation profile of GCAP1 (Dizhoor et al., 1998; Sokal et al., 1998; Wilkie et al., 2001; Kitiratschky et al., 2009). The Y99C mutant is constitutively active and has almost completely lost any Ca2+ sensitivity, i.e., it activates ROS-GC1 at low and higher [Ca2+] to a similar extent. P50L mutant exhibits less Ca2+-binding capacity (Newbold et al., 2001).
GCAP1-Modulated ROS-GC1 Transduction System Beyond ROS, Cone Photoreceptor Synapse
Inherited with its novel composition and its Ca2+-regulated feature, the perception in the membrane guanylate cyclase field arose that the GCAP-modulated ROS-GC transduction system is unique, linked solely to the photo-transduction machinery. If it were true, it must only reside in the OS of the rods and cones.
This question was first addressed by two groups (Liu et al., 1994; Cooper et al., 1995). Both, through immunocytochemistry, demonstrated the presence of a ROS-GC in the synaptic layers, outer plexiform layer (OPL) and inner plexiform layer (IPL) of the retina. These studies, however, made no differentiation between whether the detected cyclase was ROS-GC1 or ROS-GC2, and the presence of the GCAP component of the transduction system was not determined. Nonetheless, these studies suggested that the ROS-GC transduction system exists beyond ROS and possibly in other retinal neurons.
The presence of the GCAP1-modulated/ROS-GC1 transduction system was tested and found in the OPL through these independent extensive means: (1) functional, (2) biochemical, (3) peptide competition, (4) crosslinking, and (5) reconstitution. The tests demonstrated that the OPL contained ROS-GC1 transduction system, which in all respects was identical to the one present in native ROS (Venkataraman et al., 2003). Immunocytochemical analysis of the retina showed that ROS-GC1 and GCAP1 were co-expressed together in the cone synaptic pedicles, which reside in the presynaptic region of the bipolar neurons (Venkataraman et al., 2003). These results demonstrated that at the biochemical level the two GCAP1-modulated Ca2+ signaling ROS-GC1 transduction systems, present in ROS and OPL, are identical. This linked the GCAP1/ROS-GC1 transduction system with the pre-synaptic activity of the photoreceptor-bipolar neurons.
Its physiological relevance remains untested. It is conceivable, however, that the system is the regulator of a hypothetical CNG channel in the termini of cones. In this possibility, activation of the channel triggers release of glutamate from the termini. The GCAP1/ROS-GC1 system provides a Ca2+-dependent negative feedback control of the CNG channel. When the Ca2+ influx via voltage-dependent Ca2+ channels stops, for example, during hyperpolarization, the free Ca2+ concentration synaptic termini transiently declines. And the decline, in turn, activates the GCAP1/ROS-GC1 system. Consistent with this speculation are the findings, showing that Ca2+ levels fluctuate over a 10-fold range in photoreceptor synapses, reaching as low as 50 nM (reviewed in Krizaj and Copenhagen, 2002), the concentration at which GCAP1/ROS-GC1 transduction machinery is most accelerated, and the production of cyclic GMP is at its peak. Cyclic GMP will open the CNG channel; this would cause an influx of Ca2+, resulting in inactivation of the GCAP1/ROS-GC1 system.
The presence of the GCAP2-modulated ROS-GC Ca2+ signaling transduction system in the presynaptic region of the photoreceptor-bipolar neurons has not been yet tested. Yet GCAP2 is present in the region, it interacts with the synaptic ribbon protein, RIBEYE, and the authors speculate that it may participate in the post-NADH-modulated process (Venkatesan et al., 2010).
GCAP1-Modulated ROS-GC1 Transducton System not Unique to Sole Operations of Visual Transduction
With revelations on existence of the GCAP1-modulated Ca2+ signal transduction system within and outside of the sensory transduction neurons linked with vision, the next question was: does this transduction system exist outside the domain of vision-linked neurons?
The first studies addressing this issue were conducted in the pineolocytes. They showed that it does (Venkataraman et al., 1998, 2000; reviewed in Sharma, 2010).
Briefly, with a programmed protocol in the first task through an array of functional, biochemical, molecular, and histochemical tools the ROS-GC1 present in the bovine pinealocyte membranes was characterized. Then, it was shown that it is present with and is modulated by GCAP1 in a manner identical to the photoreceptor ROS-GC1. Importantly, the transduction system was not present in the surrounding glial cells. It was concluded that operation of the ROS-GC1 transduction machinery in the pinealocytes mimics the photo-transduction machinery.
These studies made two additional significant observations, reflecting the molecular differences between the photoreceptor and the pinealocyte photo-transduction machineries. (1) the pinealocyte's were devoid of the Ca2+-modulated GCAP2 arm of photoreceptor ROS-GC1; (2) They also did not contain the photoreceptor ROS-GC2 transduction system.
In a broadened search, the GCAP1-modulated ROS-GC1 Ca2+ signal transduction system has been characterized at the molecular, biochemical, and functional levels in the anterior region of the gustatory epithelium of the tongue, the site of gustatory transduction, linking it with the sense of taste. This finding has not been followed at the physiological level (Duda et al., 2004; reviewed in Sharma, 2010).
Being present in the sensory and sensory-linked neurons with vision and gustation transduction processes and in the pinealocytes, the subsequent question was: is the GCAP1-modulated ROS-GC1 transduction system also linked with the transduction events of the odorant?
The study focused first on the rat olfactory bulb (Duda et al., 2001). The bulb is the recipient of the odorant signal generated at the ciliated apical border. It receives the signal information in the form of action potentials. The incoming axons from the olfactory receptor cells join together and form presynaptic nets [Figure 3 in (Duda et al., 2007)]. These nets together with the dendrites of mitral and tufted cells are termed glomeruli (Shepherd and Greer, 1998). Mitral and tufted cells constitute the second-order neurons in the olfactory system (Pinching and Powell, 1971). The information received by their dendrites is processed in the soma, and transmitted, eventually, via the olfactory tract to the five specified areas of the olfactory cortex: anterior olfactory nucleus, olfactory tubercle, pyriform cortex, amygdaloidal complex, and entorhinal complex [Figure 3 in (Duda et al., 2007)]. These areas decode the sensory input and translate the original odorant signal into the perception of smell at the olfactory cortical centers.
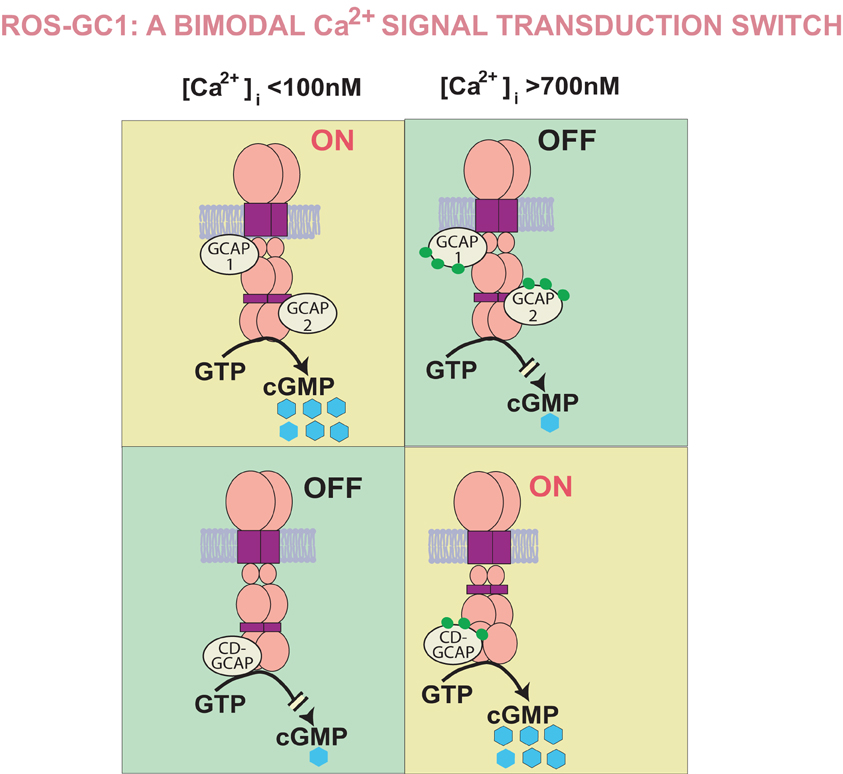
Figure 3. Bimodal Ca2+ signal transduction switch: a Model. GCAPs, 1 and 2, detect increments in free [Ca2+]i and inhibit ROS-GC1; CD-GCAPS—S100B, neurocalcin δ, hippocalcin, and frequenin—stimulate it. The range of free Ca2+ GCAPs operate in is from 100 to 500 nM and of CD-GCAPs is about 700 nM. Thus, in the GCAPs operational mode CD-GCAP switch is “OFF” and in the CD-GCAPs operational mode GCAP switch is “OFF” [reformatted and upgraded from Figure 3 with permission from Sharma et al. (2004) and Figure 4: Sharma (2010)].
Comprehensive biochemical, functional, immunohistochemical, and molecular analysis of the olfactory bulb revealed that, like the photo-transduction machinery in ROS, the bulb contains the GCAP1-modulated Ca2+ signaling ROS-GC1 transduction machinery (Duda et al., 2001). This machinery is present in the mitral cells where it is also manufactured (Duda et al., 2001). Thus, the mitral cell neurons house full genetic machinery for the production and expression of this entire transduction system in the olfactory neurons.
This study established the presence of the GCAP1/ROSGC1 signal transduction system in mitral cells of the olfactory bulb (Duda et al., 2001) and demonstrated that this transduction system is not restricted to the photic modulation; it is also present in the neurons linked with olfaction. Thus, the transduction system had the makings of being omnipresent in the neurons linked with the sensory perceptions.
GCAP1-Modulated ROS-GC1 Transducton System is also Present Outside of the Neuronal Cells
In a remarkable finding, presence of the GCAP1-modulated Ca2+ signal transduction system has been demonstrated at the molecular, protein and functional levels in the rat testes (Jankowska et al., 2007, 2010; reviewed in Jankowska and Warchol, 2010), and at the biochemical and functional levels in the human and bovine spermatozoa as well (Jankowska et al., 2010). Histochemical studies show that the transduction system is localized in a small population of spermatocytes (Jankowska et al., 2007). Physiology of the system has not been determined. Because the system is also present in the acrosomal cap, the authors have suggested that it may have a role in regulation of the acrosomal reaction (Jankowska et al., 2010). Importantly, GCAP2 was screened for its molecular presence in the rat testes but was not found, indicating absence of the GCAP2-modulated ROS-GC1 transduction in the testes.
In summary, the following conclusions are arrived at in reference to the GCAP-modulated ROS-GC transductions system:
- It is vital to the operation of the photo-transduction process.
- Only Ca2+-modulated GCAP2 and ROS-GC2 appear to be its unique photo-transduction components.
- GCAPs, 1 and 2, through diverse modes regulate ROS-GC1 activity.
- The system is present in the inner retinal neurons, yet its physiology is not determined.
- Beyond photic transmissions, its presence in the pinealocytes, olfactory bulb, and gustatory epithelium indicate that it plays a wider role in the sensory transduction processes.
- In the purest terms its presence in the spermatozoa questions the classification of GCAP1 as solely being a NCS family member.
Discovery of a CD-GCAP, S100B, Defined ROS-GC as a Calcium Bimodal Transduction Switch
The feature that the increments of [Ca2+]i progressively inhibit ROS-GC1 activity was intellectually challenging because it had never been observed before for any member of the membrane guanylate cyclase family. All members so far were stimulated by its effector ligands. Serendipitous studies (vide infra) widened its property in disclosing its remarkable nature. The ROS-GC was both an inhibitor and stimulator of [Ca2+]i signals, briefly narrated below (reviewed in: Sharma et al., 2004; Sharma and Duda, 2006; Sharma, 2010).
In a joint study, two groups reported that a retinal post-mitochondrial 100,000g supernatant fraction stimulates photoreceptor ROS-GC in a Ca2+-dependent fashion (Pozdnyakov et al., 1995). The stimulatory factor was purified as a 6–7 kDa subunit protein. It oligomerized to a functional 40 kDa and stimulated the native and the cloned form of ROSGC1 with a [Ca2+]i K1/2 of 2 μM. To distinguish it from the co-temporarily discovered GCAP that inhibited ROS-GC in a Ca2+-dependent fashion, the factor was named Ca2+-dependent guanylate cyclase activator protein (CD-GCAP) (Pozdnyakov et al., 1995). And, importantly, it was postulated that CD-GCAP may be a positive Ca2+ modulator of the yet unidentified ROS-GC type guanylate cyclase present in the synaptic layers of the bovine retina (Pozdnyakov et al., 1995), a prediction proven true by the later studies (discussed below).
The factor was cloned, sequenced both in its cloned and the native forms, and reconstituted to show that it was S100B protein (Cooper et al., 1995; Duda et al., 1996; Margulis et al., 1996; Pozdnyakov et al., 1997). And, in a remarkable discovery it was established that the ROS-GC is a Ca2+-bimodal switch. Its inhibitory mode operates through GCAP and the stimulatory through S100B. The switching modes are controlled by nanomolar to the micromolar range of [Ca2+]i.
Additional studies demonstrated that the GCAP1 and S100B-modulated domains in ROS-GC1 are far apart. They flank the catalytic domain; at its N-terminal aa503–786 segment resides the GCAP1 domain and at the C-terminal aa731–1054 segment, the CD-GCAP domain (Duda et al., 1996). The CD-GCAP and the S100B arms of the switch constitute its separate elements and they operate ROS-GC through different modes. These modes were diagrammatically depicted in a model “ROS-GC1 is a Bimodal Signal Transduction Switch” (Sharma et al., 2004). This model is reproduced in Figure 3.
There were two powerful impacts of these findings. (1) CORE, they demonstrated, for the first time, that the [Ca2+]i signals can originate downstream of the catalytic site and then be processed upstream at the catalytic site for the generation of cyclic GMP. (2) They provided a theoretical background of a general concept where via a nearby cyclic GMP-gated channel the ROS-GC transduction system could create hyper- or de-polarization in the membranes of the sensory neurons. The ensuing voltage potentials could then become the means of transmission for sensory transduction events (Sharma, 2010).
S100B is the Ca2+-Modulator of the Photoreceptor-Bipolar Synapse ROS-GC
To test the prediction that the S100B Ca2+ signaling arm of the ROS-GC1 is present in the photoreceptor-bipolar synapse region (Pozdnyakov et al., 1995), the bovine OPL was investigated (Duda et al., 2002). Serialized functional, biochemical, and immunolocalization followed by a combination of peptide competition, SPR binding, and deletion mutation studies demonstrated that upon Ca2+ binding S100B targets two sites in ROSGC1. One site comprises aa G962-N981, the other, aa I1030-Q1041. The former represents the binding site and later the transduction site. The Kd value for the binding site ranges from 198 to 395 nM.
These results confirmed that, indeed, the Ca2+ bimodal modes of the ROS-GC regulation exist in the retinal neurons and the modes define a new paradigm of Ca2+ signaling in the vertebrate neurons (Duda et al., 2002). In contrast to what happens in ROS, where increments in free Ca2+ inhibit ROS-GC1 activity, in the synapse region they stimulate ROS-GC1. The reversal in cyclase operation is caused by the substitution of GCAP in ROS with S100B in the photoreceptor-bipolar synapse. And, importantly, GCAP inhibition of ROS-GC1 occurs with Ca2+ K1/2 of about 100 nM and S100 B stimulation with a K1/2 of 850 nM. This experimentally validated the previously proposed theoretical model of S100B-modulated Ca2+ signaling of ROS-GC1 (Figure 3). It also demonstrated that S100B in the visual transduction system acts as a Ca2+ sensor protein.
Discovery of the Second CD-GCAP, Neurocalcin δ, Widened the Ca2+ Signal Transduction Modes of ROS-GC1
Besides S100 B, is there any other member of CD-GCAP linked with ROS-GC1 transduction system in the retinal neurons?
The answer is yes. First, in a heterologous system of COS cells where the sole interacting components were recombinant ROS-GC1 and recombinant bovine brain neurocalcin δ, it was demonstrated that the neurocalcin stimulated ROS-GC1 in a Ca2+-dependent manner with a K1/2 of 0.8 μM (Krishnan et al., 2004). The stimulation was specific because it did not stimulate ROS-GC2 and ANF-RGC. Also, the mechanism of stimulation was different from the prototype CD-GCAP, S100B, because the neurocalcin–modulated domain in ROS-GC1 was different from that of S100B. The common stimulatory theme of the two CD-GCAPs was explained through the comparison of their Ca2+-bound crystal structures. In 3-D terms the four helix-packing arrangements of their Ca2+-binding EF hands are very similar (Kumar et al., 1999; Sharma et al., 2004; Duda et al., 2006). Thus, spatially neurocalcin δ and S100B are structural and functional analogs.
These studies demonstrated that CD-GCAP is more than a one-member family and it is linked with the ROS-GC1 transduction system. This knowledge seeded in the evolution of a powerful theoretical concept where a single transducer ROS-GC1 component is designed to sense the multiple spatial forms of Ca2+ signals through its GCAP and CD-GCAP components and translate them into the production of a single second messenger, cyclic GMP.
Neurocalcin δ is the Ca2+-Modulator of the IPL ROS-GC1
Neurocalcin δ was purified and sequenced from the IPL of the retina (Krishnan et al., 2004). Its N-terminus is blocked and is acylated, predominantly in the myristoylated form. Accordingly, the cloned protein at its N-terminus contains a myristoylation site motif, MGXXXS, conserved in all other members of the NCS-protein family, with the only exception for the Kv-channel interacting protein subfamily; whose members, KChIP2, KChIP3, and KChIP4 are not myristoylated (Braunewell and Gundelfinger, 1999; Burgoyne and Weiss, 2001).
The IPL neurocalcin δ is a 20 kDa monomer protein. Like other Ca2+ sensor proteins (Ladant, 1995; Frins et al., 1996), it exhibits a Ca2+-dependent mobility shift. In its native form it exists as a dimer (Venkataraman et al., 2008). It has four Ca2+-binding EF-hand motifs; only three—EF2, EF3, EF4—are predicted to be functional (Okazaki et al., 1992; Terasawa et al., 1992; Vijay-Kumar and kumar, 1999).
It contains an active Ca2+ myristoyl switch (Krishnan et al., 2004) but its small fraction is always bound with the native membranes. This intrinsic binding feature has important Ca2+-dependent physiological implications. Therefore, it was studied further and validated through the analysis of the recombinant reconstituted system with the conclusions that (1) a resting cellular concentration of 100–200 nM, [Ca2+]i is able to keep neurocalcin δ membrane bound. (2) In this membrane bound state, it has a stronger affinity for ROS-GC1. (3) Once the neurocalcin δ and ROS-GC1 complex is formed, existence of this complex is independent of the Ca2+ concentration. Any one or all three of these possibilities suggested that the membrane bound neurocalcin δ can participate in the Ca2+-dependent events, occurring at millisecond time intervals, the intervals required in the visual transduction events.
In accordance with this concept, natural interaction of neurocalcin δ with ROS-GC1 was envisioned, tested and found in the IPL (Krishnan et al., 2004). Neurocalcin δ and ROS-GC1 were present together and the added presence of [Ca2+]i stimulated native ROS-GC1 activity. The results also showed that the nanomolar (resting) concentration range of Ca2+ keeps neurocalcin δ membrane bound and creates the physical and functional interaction between neurocalcin δ and ROS-GC1. Importantly, the myristoyl group of neurocalcin causes a 2-fold amplification of the saturation activity of ROS-GC1.
Kinetic parameters of the Ca2+-dependent neurocalcin δ interaction with ROS-GC1 were analyzed (Krishnan et al., 2004). Without Ca2+, neurocalcin δ had no affinity for ROS-GC1. In the presence of Ca2+, it bound ROS-GC1 with KA of 2.3 × 106 M−1 and a KD of 4.6 × 10−7 M.
These analyses demonstrated that the steps of neurocalcin δ binding to and dissociation from ROS-GC1 are Ca2+-dependent, they occur within the physiological levels of Ca2+, they are direct and of high affinity; and they occur within nano seconds, in accordance with the time span of the vision transduction steps (Krishnan et al., 2004).
That the neurocalcin δ regulation of ROS-GC1 is, indeed, unique to itself was also demonstrated with the disclosure of its target site in ROS-GC1, residing within its aa732–962 segment. It does not overlap with the GCAP1-, GCAP2-, and S100B-modulated domains of ROS-GC1 (Krishnan et al., 2004).
Neurocalcin δ Modulation of ROS-GC1 Represents a New Model of Ca2+ Signaling
Fine analysis of the aa732–962 segment pinpointed the neurocalcin δ-modulated site between aaV837-L858 of ROS-GC1. This recognition was surprising because the site resided within the core catalytic domain. This had never been observed before for any ligand of the guanylate cyclase family members.
The nature of the site was probed by direct studies with the isolated core catalytic module of ROS-GC1 (Venkataraman et al., 2008). They yielded unforeseen results, contrary to at the time held views. (1) The neurocalcin δ directly interacts with the site; (2) it does not require the adjacent N-terminally located α-helical dimerization domain structural element (aa767–811) for its interaction; (3) The core catalytic module, housing the site, is intrinsically active, i.e., it has basic guanylate cyclase activity; (4) The core module by itself is dimeric in nature, it does not require the dimerization domain structural element for being so; and (5) the core dimeric form of the catalytic module is directly regulated by the Ca2+-bound neurocalcin δ; Ca2+-unbound neurocalcin δ was ineffective.
Incorporating these features, a fold recognition based model of the core catalytic domain was built and neurocalcin δ docking simulations were carried out to define the three-dimensional features of the interacting domains of the two molecules (Venkataraman et al., 2008). This model is presented in Figure 4.
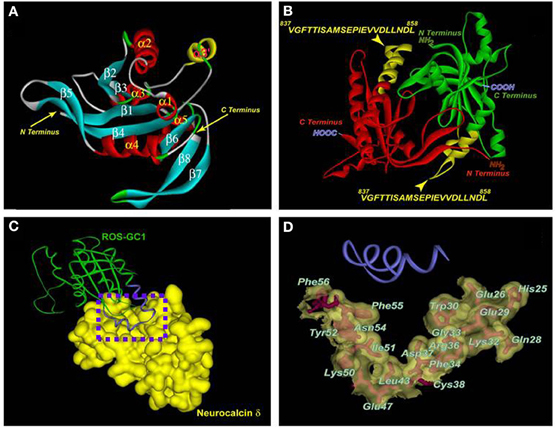
Figure 4. Three-dimensional model of the ROS-GC1 catalytic domain and molecular docking of neurocalcin δ. (A) Ribbon diagram of the ROS-GC1 catalytic domain monomer. The α helices are shown in red and the β strands in cyan. Numbering is according to the protein data base file 1AZS template. The N- and C-termini are indicated by arrows. (B) The solvent-accessible V837-L858 domain of ROS-GC1 catalytic domain. The two monomeric ROS-GC1 catalytic domains are indicated in red and green respectively. Within each monomer, the region corresponding to V837-L858 is indicated in yellow and labeled. The N- and C-termini are labeled. (C) The ROS-GC1 catalytic domain/neurocalcin δ complex. Monomers of ROS-GC1 catalytic domain and of neurocalcin δ are depicted for clarity. ROS-GC1 catalytic domain is depicted as a green ribbon and its helix-loop-helix V837-L858 region is in blue; the solvent accessible surface of neurocalcin δ is depicted in gold. A dotted box is drawn around the region of docking. (D) Residues on neurocalcin δ within 4.5 Å sphere from the interacting ROS-GC1 catalytic domain region. The docking region [dotted box in (C)] is expanded. The ROS-GC1 helix-loop-helix region (V837-L858) is depicted as a blue ribbon. The neurocalcin δ residues located within 4.5 Å sphere from the V837-L858 region are depicted as stick models in red, embedded within the solvent surface (transparent gold). The amino acid residues are labeled. Reproduced with permission from reference Venkataraman et al. (2008).
The model displayed the following features in 3-D terms.
- The two chains of the catalytic module are antiparallel. In each chain, the neurocalcin δ-binding-transduction motifs is located toward the N-terminal region of the chain. In these configurations, the two neurocalcin binding-transduction sites are distal to each other. The notable characteristics of these sites are that they are composed of helix-loop-helix structure.
- The core catalytic domain V837-L858 is the interaction site with the neurocalcin.
- The residues in and around the EF1 hand of the neurocalcin are the primary interaction sites with its target catalytic domain.
- The contact points between the catalytic domain and neurocalcin δ are designed to form a perfect fit. The neurocalcin-binding transduction motif of the catalytic domain consists of a helix-loop-helix structure. This structure is accessible to the solvent and comfortably fits in the V-shaped crevice of the neurocalcin δ. This crevice is formed by the defined EF-1 hand residues (Figure 4C). These residues form a special hydrophobic–hydrophilic patch, which may be distinctive feature of the Ca2+-dependent signaling property of neurocalcin δ.
Signal transduction model
The experimentally validated facts provided by this study and these aided by the computational modeling, allowed the proposal of a stepwise neurocalcin δ signal transduction model. Step 1, the post-phototransduction signal in the IPL neurons generates a rise of free [Ca2+]i in the lower micromolar range. Step 2, [Ca2+] binds neurocalcin δ. Step 3, neurocalcin δ undergoes Ca2+-dependent configurational change. Step 4, with an EC50 of 0.5 μM, its defined domain in EF1-hand binds its V837-L858 modulated region of the catalytic domain in ROS-GC1; catalytic domain in its native form exists as a dimer. Step 5, with kon of 7 × 104 M−1 s−1, catalytic domain is activated and generates cyclic GMP. Step 6, with koff of 4.2 × 10−2 s−1, the neurocalcin δ is dissociated and the process reverts back to the Step 1 state of the neuron. These findings defined a new transduction model for the Ca2+ signaling of ROS-GC1.
Ca2+-Modulated Odorant-Receptor ONE-GC is a Variant form of ROS-GC Transduction Sytem
The recognition that ROS-GC is a Ca2+ bimodal signal transduction switch offered the theoretical possibility of its universal linkage with the sensory transduction processes (via supra). In this pursuit, its linkage with the sense of SMELL was anticipated (reviewed in: Sharma et al., 2004; Duda et al., 2007; Sharma, 2010; Zufall and Munger, 2010). What follows is a brief recapitulation and a brief presentation of the current status of the field.
History
Ca2+-modulated ROS-GC transduction system in the olfactory transduction was first discovered in the rat olfactory bulb (vide supra) (Duda et al., 2001). After the odorant transduction step in the olfactory cilia, the bulb receives the information in the form of action potentials. The incoming axons from the olfactory receptor cells join together and form presynaptic nets. These nets are termed glomeruli (Shepherd and Greer, 1998). They are the “microprocessor units” of the olfactory bulb. Each glomerulus consists of about 20,000–25,000 axons of the olfactory receptor cells. The glomeruli synapse with the dendrites of mitral and tufted cells, constituting the second order neurons in the olfactory bulb (Pinching and Powell, 1971). The information received by the dendrites is processed in the soma, and transmitted via the olfactory tract to the five specified areas of the olfactory cortex: anterior olfactory nucleus, olfactory tubercle, pyriform cortex, amygdaloidal complex, and entorhinal complex (Figure 1 of reference Sharma, 2010). These areas decode the sensory input and translate the original odorant signal into the perception of SMELL at the olfactory cortical centers.
Programmed dissection of the bulb via the techniques of biochemistry, functional reconstitution, immunohistochemistry, and molecular constructs revealed that, like the rod and cone OS the bulb mitral cells contain and synthesize the elements of the GCAP1-modulated Ca2+-signaling ROS-GC1 transduction system (Duda et al., 2001). Importantly, the GCAP2-modulated ROS-GC signaling system is absent. Thus, these neurons have the full information both at the gene and the protein level for the production, expression, and operation of the entire transduction machinery. Intriguingly, the machinery operates with almost the same principles as established for the photo-transduction operation. These operational principles of the mitral cell have not been evaluated at the physiological level. Nonetheless, conceptually, it is possible that via a proximal hypothetical cyclic GMP-gated channel, the increments of [Ca2+]i, will decrease the production of cyclic GMP and hyperpolarize membranes of the mitral cells. This will then become the means of transmitting the original odorant signal information to the olfactory cortical centers.
Discovery of the Ca2+-Modulated ONE-GC, a Variant form of ROS-GC, in the Cilia of the Olfactory Epithelium Directed its Linkage with the Odorant Transduction
The finding of the Ca2+ signaling ROS-GC1 transduction system in the olfactory bulb provided the incentive to analyze the presence of this signaling system in the olfactory epithelium for its linkage with the process of odorant transduction. This analysis (Duda et al., 2001, 2004; reviewed in Duda et al., 2007) was aided by the prior observations with the photo-transduction machinery. Certain parallels and opposite phenotypes of the two neuronal cell systems were noted. The cilia are the counter part of the OS of rods and cones photoreceptors. OS are the sites of photo-transduction, the cilia, of the odorant transduction. Like OS, the cilia continuously shed from the olfactory receptor neurons (ORN) and undergo replacement. The OS contain the entire components of the photo-transduction system and the cilia of the odorant-transduction, a biochemical process by which an odorant signal generates electrical signal. However, it was also realized that the physiological consequences of the LIGHT signal and the ODORANT signal in their respective photo-transduction and odorant-transduction machineries are opposite; illumination results in hyperpolarization of the photoreceptor membrane, whereas odorant results in depolarization of the ORN membrane. With these considerations, intuitively, the odorant transduction-linked hypothetical ROS-GC-transduction system should be in the stimulatory mode in the semi-micromolar [Ca2+]i range in the cilia, a situation opposite to that in OS. Thus, the odorant-linked ROS-GC's sensor partner should be a CD-GCAP, instead of a GCAP in OS.
At the time this hypothesis was envisioned, the consensus was that the odorant signal transduction occurs solely through the cyclic AMP signaling pathway (reviewed in Buck, 1995; Belluscio et al., 1998; Breer, 2003; Lai et al., 2005). However, there was evidence for the presence of a membrane guanylate cyclase [GC-D] in the rat ORNs (Fülle et al., 1995). GC-D had been cloned from the total rat olfactory cDNA library. Because, no regulatory ligand for GC-D was found, it was classified as an “ORPHAN” receptor guanylate cyclase. Insitu hybridization (Fülle et al., 1995) and then immunocytochemical techniques indicated that GC-D coexisted with its cyclic GMP-PDE partner (PDE2) and, importantly, these two partners were segregated from the components of the cyclic AMP signaling pathway (Juilfs et al., 1997). Furthermore, they were present in a small subpopulation of the neuroepithelium neurons (Juilfs et al., 1997). Additional immunocytochemical studies demonstrated that this subpopulation of neurons also expresses a subunit of the cyclic GMP-selective CNG channel (Meyer et al., 2000). Thus, these neurons were not a part of the cyclic AMP signaling cascade system, instead, they were possibly wired with the cyclic GMP-selective transduction system (Juilfs et al., 1997; Meyer et al., 2000). Initial speculation was that these odorant neurons, termed necklace for their appearance, were a part of the odorant transduction system, however, this speculation was later revised to that “the function of this group of neurons may be in behavioral responses induced by hormones or pheromones, possibly related to reproduction, rather than a response to specific odorants” (Juilfs et al., 1997; Meyer et al., 2000).
To assess the possibility that a ROS-GC-like Ca2+-modulated signaling system exists in the minority subgroup of the odorant neurons and is a part of the odorant transduction system, the present authors' group in their first two studies targeted two systems: isolated rat olfactory neuroepithelium and the isolated cilia, which are the sites of odorant transduction (Duda et al., 2001, 2004). Five criteria were set forth for the ROS-GC transduction machinery to qualify as a genuine transducer of the odorant signal. (1) In its native state, it must be odorant responsive; (2) the response should be rapid; (3) it must reside in the membrane portion of the cilia, the site of odorant transduction; (4) the physiological levels of [Ca2+]i should mimic the odorant's response; and finally (5) with the defined components of the machinery, it should be possible to reconstitute the Ca2+ dependency of the machinery.
All these five criteria were met by the Ca2+-modulated myr-neurocalcin δ-ONE-GC guanylate cyclase transduction system present in the cilia (Duda et al., 2004; Sharma and Duda, 2006; Duda et al., 2007): In its native state, the machinery is odorant-responsive; its response is fast, within seconds; it resides within the membrane portion of the cilia; is modulated by the physiological concentrations of free Ca2+ with a K1/2 of 700 nM; and it can be reconstituted in a Ca2+-dependent manner by using the sole recombinant molecules of myr-neurocalcin δ and ONE-GC. These studies established the constitution and the operational principles of the odorant-linked ONE-GC transduction machinery. ONE-GC cloned from the rat olfactory neuroepithelium (Duda et al., 2001) library was found to be identical in structure to GC-D (Fülle et al., 1995). Instead of GC-D, the authors preferred the name ONE-GC because it associated with its residence in olfactory neuroepithelium (Duda et al., 2001).
Constitution and operation
The transducer component is ONE-GC and its Ca2+ sensor component is neurocalcin δ. ONE-GC is a variant form of the ROSGC subfamily because it is not inhibited by the [Ca2+]i signals; instead, it is only stimulated. ROS-GC1 and ROSGC2, the Ca2+ signal transduction components of the photo-transduction machinery, are not present in the cilia. In the resting state of the cilia, with a concentration range of 60–100 nM free Ca2+, ONE-GC transduction system is partially active and is ever ready to receive and transduce the odorant signals into the production of cyclic GMP. The neurocalcin senses Ca2+ in 300–800 nM range, undergoes conformational change, strengthens its bondage with the ONE-GC segment, a 836–1028, and turns “on” the transduction machinery. The binding of neurocalcin δ is of high affinity with a KD of 2.8 × 10−7 M, is rapid with fast association (kon of 5.7 × 103 M−1 s−1) and dissociation (koff of 1.56 × 10−3 s−1) constants. In this manner, the machinery is finely tuned to respond rapidly to the intensity-dependent fluctuations of the Ca2+ waves in the apical regions of the ciliary neurons (Duda et al., 2004).
It is noted that although neurocalcin δ contains an active Ca2+myristoyl switch, a fraction of it is always ONE-GC bound in its native state (Duda et al., 2001, 2004). It is the bound form that directly modulates the Ca2+ signals and causes activation of ONE-GC.
In this manner it was envisioned that the ONE-GC neurons via the neurocalcin-modulated Ca2+ signaling ONE-GC transduction system are linked with the odorant transduction, and one such recognized odorant was green pepper (Duda et al., 2004).
Ca2+ Sensor GCAP1 in the Phototransduction System Functions as a CD-GCAP in the Odorant ONE-GC Transduction System
A subsequent study demonstrated the identity of another Ca2+ sensor component of ONE-GC (Duda et al., 2006). It was GCAP1 but surprisingly it functioned as a CD-GCAP in the native ONE-GC neurons. There, it was bound to ONE-GC segment, aa M836-C1110 and with a Ca2+ K1/2 of 900 μM stimulated it. It was the first example and remains to be the sole example of a Ca2+ sensor that could function in an antithetical fashion, inhibiting one, ROS-GC, transduction system and stimulating the other, ONE-GC system.
ONE-GC is the Odorant Uroguanylin Receptor
An important gap related to the concept of the linkage of Ca2+-modulated ONE-GC with the odorant transduction was that (1) how it receives the odorant signal at its extracellular domain; (2) and the signal links with its Ca2+-modulated intracellular steps; and finally (3) how it is translated at its catalytic domain in the generation of cyclic GMP, the proposed second messenger of the odorant signal?
These questions began to unfold by two milestone reports (Leinders-Zufall et al., 2007; Duda and Sharma, 2008). The first, through gene-deleted mouse models, ONE-GC−/− and CNGA3−/−, and patch clamp techniques demonstrated that the wild-type mice respond to the urine odor in generating the “excitatory cyclic GMP-dependent signaling action potential firing.” The urine constituents responsible for creating these patterns of physiological behavior are uroguanylin and guanylin. It was thereby proven that the previously recognized necklace olfactory neurons were the ONE-GC neurons and they were wired with the cyclic GMP signaling pathway for the transduction of the two odorants, uroguanylin and guanylin.
This study also discounted the original proposal (Juilfs et al., 1997) and the impression it left that the ONE-GC signaling pathway is meant to signal the hypothetical pheromone activity which regulates the sexual behavior of the animals (Meyer et al., 2000), because ONE-GC gene null mice showed no abnormality in the mating and suckling behaviors (Leinders-Zufall et al., 2007).
The second report (Duda and Sharma, 2008) through in vivo cell reconstitution studies using deleted constructs of ONE-GC demonstrated that the extracellular domain of ONE-GC is the target site of the odorant uroguanylin; and the other urine odorant guanylin does not signal ONE-GC activation. Uroguanylin activates ONE-GC with an EC50 of 20 pM and saturates it at 500 pM. It was thereby established that ONE-GC is the sole uroguanylin odorant receptor.
These findings marked the solidification of the original BIMODAL concept that the Ca2+-modulated ROS-GC subfamily occurs in many forms. Through these forms, it has the potential of being a universal signal transducer of the sensory and sensory-linked neurons. ONE-GC represents a third subfamily of membrane guanylate cyclases, differing from the ROS-GC subfamily of being a direct ligand of a natriuretic peptide at its extracellular domain. Finally, contrary to the previous proposal (Fülle et al., 1995), ONE-GC is not the orphan receptor guanylate cyclase.
The other part of the report (Duda and Sharma, 2008) constituted on the mechanism by which [Ca2+]i through its sensor neurocalcin δ signals the activation of ONE-GC. Its striking feature was the disclosure of its target site, which resided directly on the ONE-GC catalytic module, aaM880-L921. There, it directly signaled its activation. The surprising aspect of this feature was that the isolated catalytic module by itself existed in the dimeric form. It did not require the co-presence of its putative dimerization domain component, revising the previous held views on the general mechanisms of membrane guanylate cyclase signaling (Garbers, 1992; Wilson and Chinkers, 1995; Ramamurthy et al., 2001).
Kinetics of the Ca2+-bound neurocalcin δ binding to the ONE-GC target site assessed by the SPR spectroscopy were: KD = 2.8 × 10−7 M; kon = 5.7 × 103 M−1s−1; koff = 1.56 × 10−3 s−1.
The study established that ONE-GC has trimodal regulation; two occur intracellularly, GCAP1- and the neurocalcin d-modulated, and one extracellularly, guanylin-modulated.
Gene Knockout Studies Demonstrated that Hippocalcin is an Additional Ca2+ Sensor Component of the Odorant Receptor ONE-GC Transduction Pathway
The hippocalcin gene deleted mouse model studies demonstrated that the hippocalcin-modulated ONE-GC transduction system also exists in the olfactory neuroepithelium and it controls ∼38% of the ONE-GC catalytic activity, ∼35% is controlled by GCAP1 and 27% by the neurocalcin (Krishnan et al., 2009). In its native state in the neuroepithelium, (1) it is bound to ONE-GC; (2) it activates ONE-GC with Ca2+K1/2 of 0.5–0.7 μM; and (3) Its EC50 value for the activation is 0.7 μM.
The Odorant, Uroguanylin, Signal Transduction Model
Given these facts that the odorant uroguanylin signals through its ONE-GC surface receptor, that the signal is amplified through its intracellular domain, that the domain is pre-bound with three of its Ca2+ sensors—the neurocalcin, GCAP1 and hippocalcin—a general odorant signal transduction model has been proposed (Figure 5). For simplicity, the model regulation is depicted for only neurocalcin, yet it is applicable to all three of its Ca2+ sensors. The specificity of each sensor resides for its target site in ONE-GC.
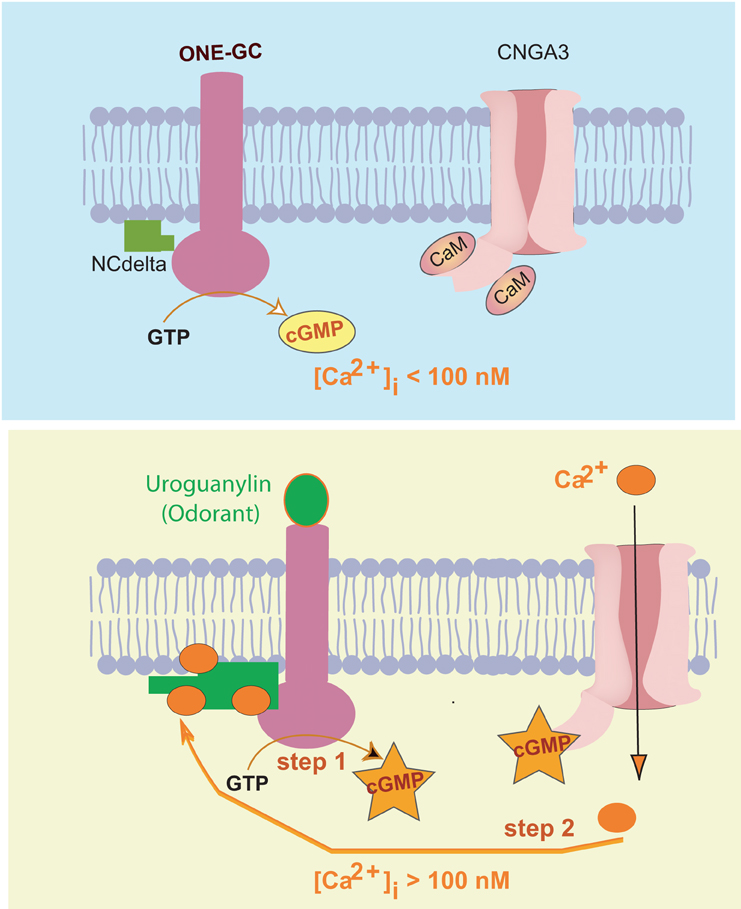
Figure 5. Two-step uroguanylin-neurocalcin δ signal transduction model. Upper panel: Resting state (free [Ca2+]i < 100 nM). ONE-GC is in its basal state, is bound to Ca2+-free neurocalcin δ (NCdelta) with low affinity, and maintains the steady-state cyclic GMP concentration. Calmodulin (CaM)-bound cyclic GMP-gated Ca2+ channel (CNGA3) is closed. Lower panel: “Step 1,” uroguanylin interacts with the receptor domain of ONE-GC causing its activation and primes ONE-GC. Cyclic GMP formed opens some of the CNGA3 channels increasing [Ca2+]i to semi-micromolar range. “Step 2”, Ca2+-bound neurocalcin δ fully interacts with uroguanylin-primed ONEGC, causing its full activation. [reproduced and reprinted with permission from: Duda and Sharma (2009)].
A small population of the ORNs contains a cyclic GMP signal transduction pathway. This pathway resides at the apical region of the cilia. Present in the region is ONE-GC membrane guanylate cyclase. Its outer domain is the uroguanylin receptor. In its inner domain, at the C-terminus, resides the catalytic domain. The M880-L921 segment of this domain is bound to Ca2+ sensor component neurocalcin δ. In resting state, the olfactory receptor neuron is in a 60–100 nM range of [Ca2+]i and ONE-GC is in its basal state.
The odorant, uroguanylin, signal starts by its interaction with the receptor domain of ONE-GC. It is processed through two sequential steps. In step one, ONE-GC is primed and activated minimally. In step 2, [Ca2+]i rises. With a K1/2 of 0.3–0.8 μM, Ca2+ binds to the neurocalcin δ, facilitating its interaction with the ONE-GC's segment M880-L921, signals full activation of ONE-GC and production of the odorant second messenger cyclic GMP.
It is envisioned that the operation of step 2 starts with the generation of a small amount of cyclic GMP in step 1. This pool of cyclic GMP opens a limited number of the cyclic GMP-gated channels causing influx of [Ca2+]i in the ORN. Ca2+ binds to the neurocalcin, which, then fully activates ONE-GC. It is noted that the ONE-GC downstream components, cyclic GMP-gated channel (CNGA3) and Ca2+ are physiologically linked.
The model is unique because it is fundamentally different from the photo-transduction and the peptide hormone receptor signal transduction models.
Ca2+-Independent, Co2 Odorant ONE-GC Signal Transduction Model
ONE-GC in addition to being a direct odorant receptor and transducer of uroguanylin possesses an additional intriguing feature. Indirectly, through carbonic anhydrase enzyme it senses atmospheric CO2 and gets accelerated in its production of cyclic GMP (Hu et al., 2007; Sun et al., 2009).
The study to decipher the biochemical and molecular differences of these two odorant signaling mechanisms demonstrated that (1) in contrast to uroguanylin, CO2 transduction mechanism is Ca2+-independent. (2) CO2 transduction site, like that of uroguanylin and neurocalcin δ, resides in the core catalytic domain, aa880–1028, of ONE-GC. (3) The site, however, does not overlap the signature neurocalcin δ domain, 908LSEPIE913. These results demonstrated an additional new transduction mechanism of the membrane guanylate cyclases, which is different from all the previously recognized modes of the membrane guanylate cyclase family.
Summation
This review has briefly chronicled the events that have resulted in the step-by-step development of the field of sensory perceptions linked with the different branches of the Ca2+-modulated ROS-GC transduction systems. Its foundation rests on the initial seminal findings made about five decades ago that cyclic GMP exists in rat urine and is synthesized through the membrane guanylate cyclase enzyme (review: Sharma, 2010). For about a decade, the field passed from the initial euphoria to a complete chaos, when, barring very few, most of the laboratories denied existence of the membrane guanylate cyclase enzyme. The field was kept alive with the efforts of those few. And was ever rekindled with the first purification of a membrane guanylate cyclase, termed ANF-RGC, which, surprisingly, was also a hormone receptor. This resulted in the belief that the membrane guanylate cyclase family is solely comprised of surface receptor guanylate cyclase. The ROS-GC discovery negated that belief and dawned a new era where its new sensory perception-linked transduction mechanisms began to be unfolded. It does so with its remarkable feature of being a two-component transduction system: Ca2+ sensor, GCAP or CD-GCAP and the transducer ROS-GC. There are numerous GCAPs and CD-GCAPs; and ROS-GCs. This architecture enables flexibility, yet specificity, for the transduction system to respond to the multitude of Ca2+ signals and generate its second messenger cyclic GMP in the sensory neurons. In this “universal Ca2+ signal transduction” concept (Figure 3) the operation of ROS-GC transduction is delicately controlled by the Ca2+ waves generated inside the neurons. It senses intensity of the waves and through its bimodal switch turns itself “on” or “off.” Through these and intermediate operations, it precisely controls the production of cyclic GMP, which, in turn, via a nearby CNG channel regulates the hyper- or depolarized state of the neuron membrane. The generated electric potential in this manner becomes a means of transmission of the sensory signals to the brain cortical centers for their perceptions.
Conflict of Interest Statement
The authors declare that the research was conducted in the absence of any commercial or financial relationships that could be construed as a potential conflict of interest.
Acknowledgments
The author (Rameshwar K. Sharma) gratefully acknowledges the continuous support for the last four decades by the numerous USPHS awards from the National Institutes of Health, the beginning awards from the National Science Foundation and the Damon Runyon Walter Winchell Cancer fund. The present research is being supported by HL 084584 and HL084584S (Teresa Duda).
References
Ames, J. B., Dizhoor, A. M., Ikura, M., Palczewski, K., and Stryer, L. (1999). Three-dimensional structure of guanylyl cyclase activating protein-2, a calcium-sensitive modulator of photoreceptor guanylyl cyclases. J. Biol. Chem. 274, 19329–19337.
Behnen, P., Dell'Orco, D., and Koch, K. W. (2010). Involvement of the calcium sensor GCAP1 in hereditary cone dystrophies. Biol. Chem. 391, 631–637.
Belluscio, L., Gold, G. H., Nemes, A., and Axel, R. (1998). Mice deficient in G(olf) are anosmic. Neuron 20, 69–81.
Braunewell, K. H., and Gundelfinger, E. D. (1999). Intracellular neuronal calcium sensor proteins: a family of EF-hand calcium-binding proteins in search of a function. Cell Tissue Res. 295, 1–12.
Breer, H. (2003). Olfactory receptors: molecular basis for recognition and discrimination of odors. Anal. Bioanal. Chem. 377, 427–433.
Burgoyne, R. D. (2007). Neuronal calcium sensor proteins: generating diversity in neuronal Ca2+ signalling. Nat. Rev. Neurosci. 8, 182–193.
Burgoyne, R. D., and Weiss, J. L. (2001). The neuronal calcium sensor family of Ca2+-binding proteins. Biochem. J. 353, 1–12. Erratum in: Biochem. J. 2001, 354, 727.
Burns, M. E., and Baylor, D. A. (2001). Activation, deactivation, and adaptation in vertebrate photoreceptor cells. Annu. Rev. Neurosci. 24, 779–805.
Chen, C., Nakatani, K., and Koutalos, Y. (2003). Free magnesium concentration in salamander photoreceptor outer segments. J. Physiol. 553, 125–135.
Cooper, N., Liu, L., Yoshida, A., Pozdnyakov, N., Margulis, A., and Sitaramayya, A. (1995). The bovine rod outer segment guanylate cyclase, ROS-GC, is present in both outer segment and synaptic layers of the retina. J. Mol. Neurosci. 6, 211–222.
Cuenca, N., Lopez, S., Howes, K., and Kolb, H. (1998). The localization of guanylyl cyclase-activating proteins in the mammalian retina. Invest. Ophthalmol. Vis. Sci. 39, 1243–1250.
Dizhoor, A. M., and Hurley, J. B. (1996). Inactivation of EF-hands makes GCAP-2 (p24) a constitutive activator of photoreceptor guanylyl cyclase by preventing a Ca2+-induced “activator-to-inhibitor” transition. J. Biol. Chem. 271, 19346–19350.
Dizhoor, A. M., Boikov, S. G., and Olshevskaya, E. V. (1998). Constitutive activation of photoreceptor guanylate cyclase by Y99C mutant of GCAP-1. Possible role in causing human autosomal dominant cone degeneration. J. Biol. Chem. 273, 17311–17314.
Dizhoor, A. M., Lowe, D. G., Olshevskaya, E. V., Laura, R. P., and Hurley, J. B. (1994). The human photoreceptor membrane guanylyl cyclase, RetGC, is present in outer segments and is regulated by calcium and a soluble activator. Neuron 12, 1345–1352.
Dizhoor, A. M., Olshevskaya, E. V., Henzel, W. J., Wong, S. C., Stults, J. T., Ankoudinova, I., and Hurley, J. B. (1995). Cloning, sequencing, and expression of a 24-kDa Ca(2+)-binding protein activating photoreceptor guanylyl cyclase. J. Biol. Chem. 270, 25200–25206.
Dizhoor, A. M., Ray, S., Kumar, S., Niemi, G., Spencer, M., Brolley, D., Walsh, K. A., Philipov, P. P., Hurley, J. B., and Stryer, L. (1991). Recoverin: a calcium sensitive activator of retinal rod guanylate cyclase. Science 251, 915–918.
Duda, T., Bharill, S., Wojtas, I., Yadav, P., Gryczynski, I., Gryczynski, Z., and Sharma, R. K. (2009). A trial natriuretic factor receptor guanylate cyclase signaling: new ATP-regulated transduction motif. Mol. Cell. Biochem. 324, 39–53.
Duda, T., Fik-Rymarkiewicz, E., Venkataraman, V., Krishnan, A., and Sharma, R. K. (2004). Calcium-modulated ciliary membrane guanylate cyclase transduction machinery: constitution and operational principles. Mol. Cell. Biochem. 267, 107–122.
Duda, T., Fik-Rymarkiewicz, E., Venkataraman, V., Krishnan, R., Koch, K. W., and Sharma, R. K. (2005). The calcium-sensor guanylate cyclase activating protein type 2 specific site in rod outer segment membrane guanylate cyclase type 1. Biochemistry 44, 7336–7345.
Duda, T., Goraczniak, R., Surgucheva, I., Rudnicka-Nawrot, M., Gorczyca, W. A., Palczewski, K., Sitaramayya, A., Baehr, W., and Sharma, R. K. (1996). Calcium modulation of bovine photoreceptor guanylate cyclase. Biochemistry 35, 8478–8482.
Duda, T., Goraczniak, R. M., and Sharma, R. K. (1996). Molecular characterization of S100A1-S100B protein in retina and its activation mechanism of bovine photoreceptor guanylate cyclase. Biochemistry 35, 6263–6266.
Duda, T., Goraczniak, R. M., Sitaramayya, A., and Sharma, R. K. (1993). Cloning and expression of an ATP-regulated human retina C-type natriuretic factor receptor guanylate cyclase. Biochemistry 32, 1391–1395.
Duda, T., Jankowska, A., Venkataraman, V., Nagele, R. G., and Sharma, R. K. (2001). A novel calcium-regulated membrane guanylate cyclase transduction system in the olfactory neuroepithelium. Biochemistry 40, 12067–12077.
Duda, T., and Koch, K. W. (2002). Retinal diseases linked with photoreceptor guanylate cyclase. Mol. Cell. Biochem. 230, 129–138.
Duda, T., Koch, K. W., Venkataraman, V., Lange, C., Beyermann, M., and Sharma, R. K. (2002). Ca(2+) sensor S100beta-modulated sites of membrane guanylate cyclase in the photoreceptor-bipolar synapse. EMBO J. 21, 2547–2556.
Duda, T., Krishnan, R., and Sharma, R. K. (2006). GCAP1: antithetical calcium sensor of ROS-GC transduction machinery. Calcium Bind. Proteins 1, 102–107.
Duda, T., Krishnan, A., Venkataraman, V., Lange, C., Koch, K. W., and Sharma, R. K. (1999). Mutations in the rod outer segment membrane guanylate cyclase in a cone-rod dystrophy cause defects in calcium signaling. Biochemistry 38, 13912–13919.
Duda, T., Pertzev, A., and Sharma, R. K. (2011). 657WTAPELL663 motif of the photoreceptor ROS-GC1: a general phototransduction switch. Biochem. Biophys. Res. Commun. 408, 236–241.
Duda, T., and Sharma, R. K. (2004). S100B-modulated Ca2+-dependent ROS-GC1 transduction machinery in the gustatory epithelium: a new mechanism in gustatory transduction. FEBS Lett. 577, 393–398.
Duda, T., and Sharma, R. K. (2008). ONE-GC membrane guanylate cyclase, a trimodal odorant signal transducer. Biochem. Biophys. Res. Commun. 367, 440–445.
Duda, T., and Sharma, R. K. (2009). Ca2+-modulated ONE-GC odorant signal transduction. FEBS Lett. 583, 1327–1330.
Duda, T., Venkataraman, V., Goraczniak, R., Lange, C., Koch, K. W., and Sharma, R. K. (1999). Functional consequences of a rod outer segment membrane guanylate cyclase (ROS-GC1) gene mutation linked with Leber's congenital amaurosis. Biochemistry 38, 509–515.
Duda, T., Venkataraman, V., Jankowska, A., Lange, C., Koch, K. W., and Sharma, R. K. (2000). Impairment of the rod outer segment membrane guanylate cyclase dimerization in a cone-rod dystrophy results in defective calcium signaling. Biochemistry 39, 12522–12533.
Duda, T., Venkataraman, V., Krishnan, A., and Sharma, R. K. (1998). Rod outer segment membrane guanylate cyclase type 1 (ROS-GC1) gene: structure, organization and regulation by phorbol ester, a protein kinase C activator. Mol. Cell. Biochem. 189, 63–70.
Duda, T., Venkataraman, V., Krishnan, A., Nagele, R. G., and Sharma, R. K. (2001). Negatively calcium-modulated membrane guanylate cyclase signaling system in the rat olfactory bulb. Biochemistry 40, 4654–4662.
Duda, T., Venkataraman, V., and Sharma, R. K. (2007). “Constitution and operational principles of the retinal and odorant-linked neurocalcin δ-dependent Ca2+ modulated ROS-GC transduction machinery,” in Neuronal Calcium Sensor Proteins. eds P. Philipov and K. W. Koch (New York, NY: Nova Science Publishers, Inc), 91–113.
Frins, S., Bönigk, W., Müller, F., Kellner, R., and Koch, K. W. (1996). Functional characterization of a guanylyl cyclase-activating protein from vertebrate rods. Cloning, heterologous expression, and localization. J. Biol. Chem. 271, 8022–8027.
Fülle, H. J., Vassar, R., Foster, D. C., Yang, R. B., Axel, R., and Garbers, D. L. (1995). A receptor guanylyl cyclase expressed specifically in olfactory sensory neurons. Proc. Natl. Acad. Sci. U.S.A. 92, 3571–3575.
Garbers, D. L. (1992). Guanylyl cyclase receptors and their endocrine, paracrine, and autocrine ligands. Cell 71, 1–4.
Goraczniak, R., Duda, T., and Sharma, R. K. (1997). Structural and functional characterization of a second subfamily member of the calcium-modulated bovine rod outer segment membrane guanylate cyclase, ROS-GC2. Biochem. Biophys. Res. Commun. 234, 666–670.
Goraczniak, R. M., Duda, T., and Sharma, R. K. (1998). Calcium modulated signaling site in type 2 rod outer segment membrane guanylate cyclase (ROS-GC2). Biochem. Biophys. Res. Commun. 245, 447–453.
Goraczniak, R. M., Duda, T., Sitaramayya, A., and Sharma, R. K. (1994). Structural and functional characterization of the rod outer segment membrane guanylate cyclase. Biochem. J. 302, 455–461.
Gorczyca, W. A., Polans, A. S., Surgucheva, I. G., Subbaraya, I., Baehr, W., and Palczewski, K. (1995). Guanylyl cyclase activating protein. A calcium-sensitive regulator of phototransduction. J. Biol. Chem. 270, 22029–22036.
Haeseleer, F., Sokal, I., Li, N., Pettenati, M., Rao, N., Bronson, D., Wechter, R., Baehr, W., and Palczewski, K. (1999). Molecular characterization of a third member of the guanylyl cyclase-activating protein subfamily. J. Biol. Chem. 274, 6526–6535.
Hayashi, F., and Yamazaki, A. (1991). Polymorphism in purified guanylate cyclase from vertebrate rod photoreceptors. Proc. Natl. Acad. Sci. U.S.A. 88, 4746–4750.
Helten, A., and Koch, K. W. (2007). Calcium-dependent conformational changes in guanylate cyclase-activating protein 2 monitored by cysteine accessibility. Biochem. Biophys. Res. Commun. 356, 687–692.
Helten, A., Säftel, W., and Koch, K. W. (2007). Expression level and activity profile of membrane bound guanylate cyclase type 2 in rod outer segments. J. Neurochem. 103, 1439–1446.
Horio, Y., and Murad, F. (1991a). Solubilization of guanylyl cyclase from bovine rod outer segments and effects of lowering Ca2+ and nitro compounds. J. Biol. Chem. 266, 3411–3415.
Horio, Y., and Murad, F. (1991b). Purification of guanylyl cyclase from rod outer segments. Biochim. Biophys. Acta 1133, 81–88.
Howes, K. A., Pennesi, M. E., Sokal, I., Church-Kopish, J., Schmidt, B., Margolis, D., Frederick, J. M., Rieke, F., Palczewski, K., Wu, S. M., Detwiler, P. B., and Baehr, W. (2002). GCAP1 rescues rod photoreceptor response in GCAP1/GCAP2 knockout mice. EMBO J. 21, 1545–1554.
Hu, J., Zhong, C., Ding, C., Chi, Q., Walz, A., Mombaerts, P., Matsunami, H., and Luo, M. (2007). Detection of near-atmospheric concentrations of CO2 by an olfactory subsystem in the mouse. Science 317, 953–957.
Hunt, D. M., Buch, P., and Michaelides, M. (2010). Guanylate cyclases and associated activator proteins in retinal disease. Mol. Cell. Biochem. 334, 157–168.
Hurley, J. B., Dizhoor, A. M., Ray, S., and Stryer, L. (1993). Recoverin's role: conclusion withdrawn. Science 260, 740.
Hwang, J. Y., and Koch, K. W. (2002a). Calcium- and myristoyl-dependent properties of guanylate cyclase-activating protein-1 and protein-2. Biochemistry 41, 13021–13028.
Hwang, J. Y., and Koch, K. W. (2002b). The myristoylation of the neuronal Ca2+-sensors guanylate cyclase-activating protein 1 and 2. Biochim. Biophys. Acta 1600, 111–117.
Hwang, J. Y., Lange, C., Helten, A., Höppner-Heitmann, D., Duda, T., Sharma, R. K., and Koch, K. W. (2003). Regulatory modes of rod outer segment membrane guanylate cyclase differ in catalytic efficiency and Ca(2+)-sensitivity. Eur. J. Biochem. 270, 3814–3821.
Hwang, J. Y., Schlesinger, R., and Koch, K. W. (2004). Irregular dimerization of guanylate cyclase-activating protein 1 mutants causes loss of target activation. Eur. J. Biochem. 271, 3785–3793.
Imanishi, Y., Li, N., Sokal, I., Sowa, M. E., Lichtarge, O., Wensel, T. G., Saperstein, D. A., Baehr, W., and Palczewski, K. (2002). Characterization of retinal guanylate cyclase-activating protein 3 (GCAP3) from zebrafish to man. Eur. J. Neurosci. 15, 63–78.
Imanishi, Y., Yang, L., Sokal, I., Filipek, S., Palczewski, K., and Baehr, W. (2004). Diversity of guanylate cyclase-activating proteins (GCAPs) in teleost fish: characterization of three novel GCAPs (GCAP4, GCAP5, GCAP7) from zebrafish (Danio rerio) and prediction of eight GCAPs (GCAP1-8) in pufferfish (Fugu rubripes). J. Mol. Evol. 59, 204–217.
Jankowska, A., Burczyńska, B., Duda, T., and Warchol, J. B. (2010). Rod outer segment membrane guanylate cyclase type 1 (ROS-GC1) calcium-modulated transduction system in the sperm. Fertil. Steril. 93, 904–912.
Jankowska, A., Burczynska, B., Duda, T., Warchol, J. B., and Sharma, R. K. (2007). Calcium-modulated rod outer segment membrane guanylate cyclase type 1 transduction machinery in the testes. J. Androl. 28, 50–58.
Jankowska, A., and Warchol, J. B. (2010). Ca(2+)-modulated membrane guanylate cyclase in the testes. Mol. Cell. Biochem. 334, 169–179.
Juilfs, D. M., Fülle, H. J., Zhao, A. Z., Houslay, M. D., Garbers, D. L., and Beavo, J. A. (1997). A subset of olfactory neurons that selectively express cGMP-stimulated phosphodiesterase (PDE2) and guanylyl cyclase-D define a unique olfactory signal transduction pathway. Proc. Natl. Acad. Sci. U.S.A. 94, 3388–3395.
Kachi, S., Nishizawa, Y., Olshevskaya, E., Yamazaki, A., Miyake, Y., Wakabayashi, T., Dizhoor, A., and Usukura, J. (1999). Detailed localization of photoreceptor guanylate cyclase activating protein-1 and -2 in mammalian retinas using light and electron microscopy. Exp. Eye Res. 68, 465–473.
Kelsell, R. E., Gregory-Evans, K., Payne, A. M., Perrault, I., Kaplan, J., Yang, R. B., Garbers, D. L., Bird, A. C., Moore, A. T., and Hunt, D. M. (1998). Mutations in the retinal guanylate cyclase (RETGC-1) gene in dominant cone-rod dystrophy. Hum. Mol. Genet. 7, 1179–1184.
Kitiratschky, V. B., Behnen, P., Kellner, U., Heckenlively, J. R., Zrenner, E., Jägle, H., Kohl, S., Wissinger, B., and Koch, K. W. (2009). Mutations in the GUCA1A gene involved in hereditary cone dystrophies impair calcium-mediated regulation of guanylate cyclase. Hum. Mutat. 30, 782–796.
Koch, K. W. (1991). Purification and identification of photoreceptor guanylate cyclase. J. Biol. Chem. 266, 8634–8637.
Koch, K. W. (2006). GCAPs, the classical neuronal calcium sensors in the retina : a Ca2+ relay model of guanylate cyclase activation. Calcium Bind. Proteins 1, 3–6.
Koch, K. W., and Stryer, L. (1988). Highly cooperative feedback control of retinal rod guanylate cyclase by calcium ions. Nature 334, 64–66.
Koch, K. W., Duda, T., and Sharma, R. K. (2002). Photoreceptor specific guanylate cyclases in vertebrate phototransduction. Mol. Cell. Biochem. 230, 97–106.
Koch, K. W., Duda, T., and Sharma, R. K. (2010). Ca(2+)-modulated vision-linked ROS-GC guanylate cyclase transduction machinery. Mol. Cell. Biochem. 334, 105–115.
Koutalos, Y., Nakatani, K., Tamura, T., and Yau, K. W. (1995). Characterization of guanylate cyclase activity in single retinal rod outer segments. J. Gen. Physiol. 106, 863–890.
Krishnan, A., Duda, T., Pertzev, A., Kobayashi, M., Takamatsu, K., and Sharma, R. K. (2009). Hippocalcin, new Ca(2+) sensor of a ROS-GC subfamily member, ONE-GC, membrane guanylate cyclase transduction system. Mol. Cell. Biochem. 325, 1–14.
Krishnan, A., Goraczniak, R. M., Duda, T., and Sharma, R. K. (1998). Third calcium-modulated rod outer segment membrane guanylate cyclase transduction mechanism. Mol. Cell. Biochem. 178, 251–259.
Krishnan, A., Venkataraman, V., Fik-Rymarkiewicz, E., Duda, T., and Sharma, R. K. (2004). Structural, biochemical, and functional characterization of the calcium sensor neurocalcin delta in the inner retinal neurons and its linkage with the rod outer segment membrane guanylate cyclase transduction system. Biochemistry 43, 2708–2723.
Krizaj, D., and Copenhagen, D. R. (2002). Calcium regulation in photoreceptors. Front. Biosci. 7, d2023–d2044.
Kumar, V. D., Vijay-Kumar, S., Krishnan, A., Duda, T., and Sharma, R. K. (1999). A second calcium regulator of rod outer segment membrane guanylate cyclase, ROS-GC1: neurocalcin. Biochemistry 38, 12614–12620.
Kutty, R. K., Fletcher, R. T., Chader, G. J., and Krishna, G. (1992). Expression of guanylate cyclase-A mRNA in the rat retina: detection using polymerase chain reaction. Biochem. Biophys. Res. Commun. 182, 851–857.
Ladant, D. (1995). Calcium and membrane binding properties of bovine neurocalcin delta expressed in Escherichia coli. J. Biol. Chem. 270, 3179–3185.
Lai, P. C., Singer, M. S., and Crasto, C. J. (2005). Structural activation pathways from dynamic olfactory receptor-odorant interactions. Chem. Senses. 30, 781–792.
Lange, C., Duda, T., Beyermann, M., Sharma, R. K., and Koch, K. W. (1999). Regions in vertebrate photoreceptor guanylyl cyclase ROS-GC1 involved in Ca(2+)-dependent regulation by guanylyl cyclase-activating protein GCAP-1. FEBS Lett. 460, 27–31.
Leinders-Zufall, T., Cockerham, R. E., Michalakis, S., Biel, M., Garbers, D. L., Reed, R. R., Zufall, F., and Munger, S. D. (2007). Contribution of the receptor guanylyl cyclase GC-D to chemosensory function in the olfactory epithelium. Proc. Natl. Acad. Sci. U.S.A. 104, 14507–14512.
Lim, S., Peshenko, I., Dizhoor, A., and Ames, J. B. (2009). Effects of Ca2+, Mg2+, and myristoylation on guanylyl cyclase activating protein 1 structure and stability. Biochemistry 48, 850–862.
Liu, X., Seno, K., Nishizawa, Y., Hayashi, F., Yamazaki, A., Matsumoto, H., Wakabayashi, T., and Usukura, J. (1994). Ultrastructural localization of retinal guanylate cyclase in human and monkey retinas. Exp. Eye Res. 59, 761–768.
Lowe, D. G., Dizhoor, A. M., Liu, K., Gu, Q., Spencer, M., Laura, R., Lu, L., and Hurley, J. B. (1995). Cloning and expression of a second photoreceptor-specific membrane retina guanylyl cyclase (RetGC), RetGC-2. Proc. Natl. Acad. Sci. U.S.A. 92, 5535–5539.
Luo, D. G., Xue, T., and Yau, K. W. (2008). How vision begins: an odyssey. Proc. Natl. Acad. Sci. U.S.A. 105, 9855–9862.
Makino, C. L., Dodd, R. L., Chen, J., Burns, M. E., Roca, A., Simon, M. I., and Baylor, D. A. (2004). Recoverin regulates light-dependent phosphodiesterase activity in retinal rods. J. Gen. Physiol. 123, 729–741. Erratum in: J. Gen. Physiol. 2005, 126, 81.
Margulis, A., Goraczniak, R. M., Duda, T., Sharma, R. K., and Sitaramayya, A. (1993). Structural and biochemical identity of retinal rod outer segment membrane guanylate cyclase. Biochem. Biophys. Res. Commun. 194, 855–861.
Margulis, A., Pozdnyakov, N., and Sitaramayya, A. (1996). Activation of bovine photoreceptor guanylate cyclase by S100 proteins. Biochem. Biophys. Res. Commun. 218, 243–247.
McCue, H. V., Haynes, L. P., and Burgoyne, R. D. (2010). The diversity of calcium sensor proteins in the regulation of neuronal function. Cold Spring Harb. Perspect. Biol. 2, a004085.
Meyer, M. R., Angele, A., Kremmer, E., Kaupp, U. B., and Muller, F. (2000). A cGMP-signaling pathway in a subset of olfactory sensory neurons. Proc. Natl. Acad. Sci. U.S.A. 97, 10595–10600.
Nef, P. (1996). “Guidebook to the calcium-binding proteins,” in Guidebook to Calcium Sensor Proteins, ed M. R. Celio (Oxford: Oxford University Press), 94.
Newbold, R. J., Deery, E. C., Walker, C. E., Wilkie, S. E., Srinivasan, N., Hunt, D. M., Bhattacharya, S. S., and Warren, M. J. (2001). The destabilization of human GCAP1 by a proline to leucine mutation might cause cone-rod dystrophy. Hum. Mol. Genet. 10, 47–54.
Okazaki, K., Watanabe, M., Ando, Y., Hagiwara, M., Terasawa, M., and Hidaka, H. (1992). Full sequence of neurocalcin, a novel calcium-binding protein abundant in central nervous system. Biochem. Biophys. Res. Commun. 185, 147–153.
Olshevskaya, E. V., Ermilov, A. N., and Dizhoor, A. M. (1999). Dimerization of guanylyl cyclase-activating protein and a mechanism of photoreceptor guanylyl cyclase activation. J. Biol. Chem. 274, 25583–25587.
Olshevskaya, E. V., Hughes, R. E., Hurley, J. B., and Dizhoor, A. M. (1997). Calcium binding, but not a calcium-myristoyl switch, controls the ability of guanylyl cyclase-activating protein GCAP-2 to regulate photoreceptor guanylyl cyclase. J. Biol. Chem. 272, 14327–14333.
Otto-Bruc, A., Fariss, R. N., Haeseleer, F., Huang, J., Buczylko, J., Surgucheva, I., Baehr, W., Milam, A. H., and Palczewski, K. (1997). Localization of guanylate cyclase-activating protein 2 in mammalian retinas. Proc. Natl. Acad. Sci. U.S.A. 94, 4727–4732.
Palczewski, K., Subbaraya, I., Gorczyca, W. A., Helekar, B. S., Ruiz, C. C., Ohguro, H., Huang, J., Zhao, X., Crabb, J. W., Johnson, R. S., and Baehr, W. (1994). Molecular cloning and characterization of retinal photoreceptor guanylyl cyclase-activating protein. Neuron 13, 395–404.
Perrault, I., Rozet, J. M., Calvas, P., Gerber, S., Camuzat, A., Dollfus, H., Châtelin, S., Souied, E., Ghazi, I., Leowski, C., Bonnemaison, M., Le Paslier, D., Frézal, J., Dufier, J. L., Pittler, S., Munnich, A., and Kaplan, J. (1996). Retinal-specific guanylate cyclase gene mutations in Leber's congenital amaurosis. Nat. Genet. 14, 461–464.
Peshenko, I. V., and Dizhoor, A. M. (2004). Guanylyl cyclase-activating proteins (GCAPs) are Ca2+/Mg2+ sensors: implications for photoreceptor guanylyl cyclase (RetGC) regulation in mammalian photoreceptors. J. Biol. Chem. 279, 16903–16906.
Peshenko, I. V., and Dizhoor, A. M. (2006). Ca2+ and Mg2+ binding properties of GCAP-1. Evidence that Mg2+-bound form is the physiological activator of photoreceptor guanylyl cyclase. J. Biol. Chem. 281, 23830–23841.
Peshenko, I. V., and Dizhoor, A. M. (2007). Activation and inhibition of photoreceptor guanylyl cyclase by guanylyl cyclase activating protein 1 (GCAP-1): the functional role of Mg2+/Ca2+ exchange in EF-hand domains. J. Biol. Chem. 282, 21645–21652.
Peshenko, I. V., Olshevskaya, E. V., Savchenko, A. B., Karan, S., Palczewski, K., Baehr, W., and Dizhoor, A. M. (2011). Enzymatic properties and regulation of the native isozymes of retinal membrane guanylyl cyclase (RetGC) from mouse photoreceptors. Biochemistry 50, 5590–5600.
Philippov, P. P., Senin, I. I., and Koch, K. W. (2007). “Recoverin: a calcium-dependent regulator of the visual transduction,” in Neuronal Calcium Sensor Proteins, eds P. Philipov and K. W. Koch (New York, NY: Nova Science Publishers, Inc), 139–151.
Pinching, A. J., and Powell, T. P. (1971). The neuropil of the periglomerular region of the olfactory bulb. J. Cell. Sci. 9, 379–409.
Pittler, S., Munnich, A., and Kaplan, J. (1996). Retinal-specific guanylate cyclase gene mutations in Leber's congenital amaurosis. Nat. Genet. 14, 461–464.
Pozdnyakov, N., Goraczniak, R., Margulis, A., Duda, T., Sharma, R. K., Yoshida, A., and Sitaramayya, A. (1997). Structural and functional characterization of retinal calcium-dependent guanylate cyclase activator protein (CD-GCAP): identity with S100beta protein. Biochemistry 36, 14159–14166.
Pozdnyakov, N., Yoshida, A., Cooper, N. G., Margulis, A., Duda, T., Sharma, R. K., and Sitaramayya, A. (1995). A novel calcium-dependent activator of retinal rod outer segment membrane guanylate cyclase. Biochemistry 34, 14279–14283.
Pugh, E. N. Jr., and Cobbs, W. H. (1986). Visual transduction in vertebrate rods and cones: a tale of two transmitters, calcium and cyclic GMP. Vision Res. 26, 1613–1643.
Pugh, E. N. Jr., Duda, T., Sitaramayya, A., and Sharma, R. K. (1997). Photoreceptor guanylate cyclases: a review. Biosci. Rep. 17, 429–473.
Pugh, E. N. Jr., Nikonov, S., and Lamb, T. D. (1999). Molecular mechanisms of vertebrate photoreceptor light adaptation. Curr. Opin. Neurobiol. 9, 410–418.
Ramamurthy, V., Tucker, C., Wilkie, S. E., Daggett, V., Hunt, D. M., and Hurley, J. B. (2001). Interactions within the coiled-coil domain of RetGC-1 guanylyl cyclase are optimized for regulation rather than for high affinity. J. Biol. Chem. 276, 26218–26229.
Rätscho, N., Scholten, A., and Koch, K. W. (2010). Diversity of sensory guanylate cyclases in teleost fishes. Mol. Cell. Biochem. 334, 207–214.
Sharma, R. K. (2002). Evolution of the membrane guanylate cyclase transduction system. Mol. Cell. Biochem. 230, 3–30.
Sharma, R. K. (2010). Membrane guanylate cyclase is a beautiful signal transduction machine: overview. Mol. Cell. Biochem. 334, 3–36.
Sharma, R. K., and Duda, T. (2006). Calcium sensor neurocalcin d-modulated ROS-GCtransduction machinery in the retinal and olfactory neurons. Calcium Bind. Proteins 1, 7–11.
Sharma, R. K., and Duda, T. (2010). ROS-GC subfamily membrane guanylate cyclase-linked transduction systems: taste, pineal gland and hippocampus. Mol. Cell. Biochem. 334, 199–206.
Sharma, R. K., Duda, T., Venkataraman, V., and Koch, K. W. (2004). Calcium modulated mammalian membrane guanylate cyclase ROS-GC transduction machinery in sensory neurons. Curr. Topics Biochem. Res. 322, 111–144.
Shepherd, G. M., and Greer, C. A. (1998). “Olfactory bulb,” in The Synaptic Organization Of The Brain, ed G. M. Shepherd (New York, NY: Oxford University Press), 159–203.
Shyjan, A. W., de Sauvage, F. J., Gillett, N. A., Goeddel, D. V., and Lowe, D. G. (1992). Molecular cloning of a retina-specific membrane guanylyl cyclase. Neuron 9, 727–737.
Sokal, I., Li, N., Surgucheva, I., Warren, M. J., Payne, A. M., Bhattacharya, S. S., Baehr, W., and Palczewski, K. (1998). GCAP1 (Y99C) mutant is constitutively active in autosomal dominant cone dystrophy. Mol. Cell. 2, 129–133.
Sokal, I., Otto-Bruc, A. E., Surgucheva, I., Verlinde, C. L., Wang, C. K., Baehr, W., and Palczewski, K. (1999). Conformational changes in guanylyl cyclase-activating protein 1 (GCAP1) and its tryptophan mutants as a function of calcium concentration. J. Biol. Chem. 274, 19829–19837.
Stephen, R., Bereta, G., Golczak, M., Palczewski, K., and Sousa, M. C. (2007). Stabilizing function for myristoyl group revealed by the crystal structure of a neuronal calcium sensor, guanylate cyclase-activating protein 1. Structure 15, 1392–1402.
Stephen, R., Filipek, S., Palczewski, K., and Sousa, M. C. (2008). Ca2+-dependent regulation of phototransduction. Photochem. Photobiol. 84, 903–910.
Subbaraya, I., Ruiz, C. C., Helekar, B. S., Zhao, X., Gorczyca, W. A., Pettenati, M. J., Rao, P. N., Palczewski, K., and Baehr, W. (1994). Molecular characterization of human and mouse photoreceptor guanylate cyclase-activating protein (GCAP) and chromosomal localization of the human gene. J. Biol. Chem. 269, 31080–31089.
Sun, L., Wang, H., Hu, J., Han, J., Matsunami, H., and Luo, M. (2009). Guanylyl cyclase-D in the olfactory CO2 neurons is activated by bicarbonate. Proc. Natl. Acad. Sci. U.S.A. 106, 2041–2046.
Terasawa, M., Nakano, A., Kobayashi, R., and Hidaka, H. (1992). Neurocalcin: a novel calcium-binding protein from bovine brain. J. Biol. Chem. 267, 19596–19599.
Tucker, C. L., Woodcock, S. C., Kelsell, R. E., Ramamurthy, V., Hunt, D. M., and Hurley, J. B. (1999). Biochemical analysis of a dimerization domain mutation in RetGC-1 associated with dominant cone-rod dystrophy. Proc. Natl. Acad. Sci. U.S.A. 96, 9039–9044.
Venkataraman, V., Duda, T., Ravichandran, S., and Sharma, R. K. (2008). Neurocalcin delta modulation of ROS-GC1, a new model of Ca(2+) signaling. Biochemistry 47, 6590–6601.
Venkataraman, V., Duda, T., and Sharma, R. K. (1998). The alpha(2D/A)-adrenergic receptor-linked membrane guanylate cyclase: a new signal transduction system in the pineal gland. FEBS Lett. 427, 69–73.
Venkataraman, V., Duda, T., Vardi, N., Koch, K. W., and Sharma, R. K. (2003). Calcium-modulated guanylate cyclase transduction machinery in the photoreceptor–bipolar synaptic region. Biochemistry 42, 5640–5648.
Venkataraman, V., Nagele, R., Duda, T., and Sharma, R. K. (2000). Rod outer segment membrane guanylate cyclase type 1-linked stimulatory and inhibitory calcium signaling systems in the pineal gland: biochemical, molecular, and immunohistochemical evidence. Biochemistry 39, 6042–6052.
Venkatesan, J. K., Natarajan, S., Schwarz, K., Mayer, S. I., Alpadi, K., Magupalli, V. G., Sung, C. H., and Schmitz, F. (2010). Nicotinamide adenine dinucleotide-dependent binding of the neuronal Ca2+ sensor protein GCAP2 to photoreceptor synaptic ribbons. J. Neurosci. 30, 6559–6576.
Vijay-Kumar, S., and Kumar, V. D. (1999). Crystal structure of recombinant bovine neurocalcin. Nat. Struct. Biol. 6, 80–88.
Wensel, T. G. (2008). Signal transducing membrane complexes of photoreceptor outer segments. Vis. Res. 48, 2052–2061.
Wilkie, S. E., Li, Y., Deery, E. C., Newbold, R. J., Garibaldi, D., Bateman, J. B., Zhang, H., Lin, W., Zack, D. J., Bhattacharya, S. S., Warren, M. J., Hunt, D. M., and Zhang, K. (2001). Identification and functional consequences of a new mutation (E155G) in the gene for GCAP1 that causes autosomal dominant cone dystrophy. Am. J. Hum. Genet. 69, 471–480.
Wilson, E. M., and Chinkers, M. (1995). Identification of sequences mediating guanylyl cyclase dimerization. Biochemistry 34, 4696–4701.
Zozulya, S., and Stryer, L. (1992). Calcium-myristoyl protein switch. Proc. Natl. Acad. Sci. U.S.A. 89, 11569–11573.
Keywords: ROS-GC guanylate cyclase, Ca2+ sensors, cyclic GMP, sensory transductions
Citation: Sharma RK and Duda T (2012) Ca2+-sensors and ROS-GC: interlocked sensory transduction elements: a review. Front. Mol. Neurosci. 5:42. doi: 10.3389/fnmol.2012.00042
Received: 08 February 2012; Accepted: 20 March 2012;
Published online: 09 April 2012.
Edited by:
Michael R. Kreutz, Leibniz-Institute for Neurobiology, GermanyReviewed by:
Karl-Wilhelm Koch, Carl von Ossietzky University Oldenburg, GermanyIvan I. Senin, A. N. Belozersky Institute Lomonosov Moscow State University, Russia
Copyright: © 2012 Sharma and Duda. This is an open-access article distributed under the terms of the Creative Commons Attribution Non Commercial License, which permits non-commercial use, distribution, and reproduction in other forums, provided the original authors and source are credited.
*Correspondence: Distinguished Professor Rameshwar K. Sharma, Research Divisions of Biochemistry and Molecular Biology, The Unit of Regulatory and Molecular Biology, Salus University, 8360 Old York Road, Elkins Park, PA 19027, USA. e-mail: rsharma@salus.edu