- 1Institute of Physiology and Pathophysiology, University of Heidelberg, Heidelberg, Germany
- 2Interdisciplinary Center for Neurosciences (IZN), University of Heidelberg, Heidelberg, Germany
Fast neuronal network oscillations in the gamma frequency band (30–100 Hz) occur in various cortex regions, require timed synaptic excitation and inhibition with glutamate and GABA, respectively, and are associated with higher brain functions such as sensory perception, attentional selection and memory formation. However, little is known about energy and ion homeostasis during the gamma oscillation. Recent studies addressed this topic in slices of the rodent hippocampus using cholinergic and glutamatergic receptor models of gamma oscillations (GAM). Methods with high spatial and temporal resolution were applied in vitro, such as electrophysiological recordings of local field potential (LFP) and extracellular potassium concentration ([K+]o), live-cell fluorescence imaging of nicotinamide adenine dinucleotide (phosphate) and flavin adenine dinucleotide [NAD(P)H and FAD, respectively] (cellular redox state), and monitoring of the interstitial partial oxygen pressure (pO2) in depth profiles with microsensor electrodes, including mathematical modeling. The main findings are: (i) GAM are associated with high oxygen consumption rate and significant changes in the cellular redox state, indicating rapid adaptations in glycolysis and oxidative phosphorylation; (ii) GAM are accompanied by fluctuating elevations in [K+]o of less than 0.5 mmol/L from baseline, likely reflecting effective K+-uptake mechanisms of neuron and astrocyte compartments; and (iii) GAM are exquisitely sensitive to metabolic stress induced by lowering oxygen availability or by pharmacological inhibition of the mitochondrial respiratory chain. These findings reflect precise cellular adaptations to maintain adenosine-5′-triphosphate (ATP), ion and neurotransmitter homeostasis and thus neural excitability and synaptic signaling during GAM. Conversely, the exquisite sensitivity of GAM to metabolic stress might significantly contribute the exceptional vulnerability of higher brain functions in brain disease.
Gamma Oscillations and Higher Brain Functions
Neuronal information processing is primarily executed by principal cells, such as granule and pyramidal neurons that release excitatory neurotransmitter, glutamate (Bliss and Lømo, 1973; Miles and Wong, 1987; LoTurco et al., 1988; Malenka et al., 1989). It is generally thought that these projection neurons process, transfer, store and retrieve information and therefore, underlie the emergence of higher brain functions such as sensory perception, attentional selection, motor behavior, and memory formation (Buzsáki, 2006; Kullmann and Lamsa, 2007; Hájos and Paulsen, 2009; Ho et al., 2011).
Neuronal information processing, however, depends on the coordination of principal cell activity in cortical networks (Buzsáki, 2006; Traub and Whittington, 2010). Such coordination can be provided by neuronal network oscillations that show a wide spectrum of frequencies, ranging from about 0.05 Hz to 600 Hz (Buzsáki and Draguhn, 2004). Prominent examples are network oscillations in the theta (4–12 Hz), beta (13–30 Hz) and gamma (30–100 Hz) bands, which are associated with different cognitive and behavioral states (Buzsáki, 2006; Hájos and Paulsen, 2009; Uhlhaas and Singer, 2010; Watrous et al., 2015). Of course, this does not preclude the importance of slower oscillations for higher brain functions (Buzsáki, 2006; Schroeder and Lakatos, 2009).
Gamma oscillations (GAM) (30–100 Hz) have been found in many mammalian brain regions, such as visual, auditory, somatosensory and motor systems, and in the hippocampus (Kreiter and Singer, 1992; Murthy and Fetz, 1992; Franowicz and Barth, 1995; Lebedev and Nelson, 1995; Whittington et al., 1995; Gray and Viana Di Prisco, 1997). GAM are associated with rhythmic fluctuations of the membrane potential of 5–10 mV in excitatory pyramidal cells and fast-spiking inhibitory interneurons, reflecting precisely timed incidence of excitatory postsynaptic currents (EPSCs) and inhibitory postsynaptic currents (IPSCs) (Whittington et al., 1995; Penttonen et al., 1998; Fischer et al., 2002; Salkoff et al., 2015). These rhythmic fluctuations support the synchronized generation of action potentials (neuronal “spiking”) in principal cells with great precision (Buzsáki, 2006; Hájos and Paulsen, 2009; Watrous et al., 2015) and thus, permit the coordinated activation of defined sets of neurons, i.e., functional ensembles that are thought to represent the information-carrying multicellular subsets of neuronal networks (Buzsáki and Chrobak, 1995; Whittington et al., 1997; Fries et al., 2007; Traub and Whittington, 2010). GAM have a role in higher brain functions, such as voluntary movement, visual and auditory perception, attentional selection as well as memory formation (Gray et al., 1989; Pantev et al., 1991; Paulsen and Moser, 1998; Haenschel et al., 2000; Melloni et al., 2007; Montgomery and Buzsáki, 2007; Cheyne et al., 2008; Lisman and Buzsáki, 2008; van Vugt et al., 2010; Zhang et al., 2012; Popa et al., 2013). GAM in vivo occur transiently on the 100 ms time scale upon sensory input (Pantev et al., 1991; Bragin et al., 1995; Franowicz and Barth, 1995). In the human brain and dependent on the task, however, they can last for prolonged times in the range of minutes (Lehmann et al., 2001; Lutz et al., 2004). A summary of some key features of cortical GAM is given in Table 1.
Investigating Gamma Oscillations In Vitro: Experimental Models and Methods
Experimental Models in Hippocampal Slice Preparations
GAM in cortical tissue in vitro can be reliably induced by various methods, such as electrical stimulation or bath application of cholinergic or glutamatergic receptor agonists. In many studies, acute slices or organotypic slice cultures of the hippocampus have been used (Whittington et al., 1995; Fisahn et al., 1998; Hájos et al., 2009; Kann et al., 2011). An overview about the induction and features of GAM under various recording conditions, i.e., in different models, is given in Table 2. Pharmacologically induced hippocampal GAM in vitro share many features with GAM in vivo, such as intrinsic generation of GAM in the CA3 region, reversal of the phase of the local field potential (LFP) between stratum pyramidale (cell body layer of pyramidal cells) and stratum radiatum (apical dendritic compartment) of CA3, similar current source density profiles, and highest spiking probability of pyramidal cells at the negative peak of oscillation cycles (CA3, stratum pyramidale) that is followed by spiking of perisomatic inhibitory interneurons within 2 ms, consistent with monosynaptic excitation (Bragin et al., 1995; Penttonen et al., 1998; Csicsvari et al., 2003; Hájos et al., 2004; Hájos and Paulsen, 2009). However, in the majority of in vitro studies GAM are persistent for tens of minutes, show a frequency around 40 Hz, and are rarely associated with an additional, slower network rhythm (Table 2).
The synaptic mechanisms that underlie the generation of GAM have been reviewed in detail, and they largely depend on the ratio of neuronal excitation and inhibition (Bartos et al., 2007; Hájos and Paulsen, 2009). In the cortex, synaptic inhibition is mainly mediated by neurotransmitter, gamma-aminobutyric acid (GABA) that is released from the heterogeneous group of GABAergic interneurons (Mann and Paulsen, 2007; Klausberger and Somogyi, 2008; Fritschy and Panzanelli, 2014; Kaila et al., 2014). Notably, the transient activation of GABA-A receptors has a key role for the generation of GAM. This is because GAM are completely blocked by GABA-A receptor antagonist, bicuculline in various in vitro models, and studies also using transgenic mice show that synaptic excitation of fast-spiking, parvalbumin-positive interneurons is required for the generation of normal GAM in vitro and in vivo (Whittington et al., 1995; Fuchs et al., 2007; Cardin et al., 2009; Sohal et al., 2009; Gulyás et al., 2010; Korotkova et al., 2010; Oren et al., 2010). During hippocampal GAM, individual pyramidal cells generate action potentials at 1–3 Hz in vitro and in vivo, whereas fast-spiking perisomatic interneurons generate action potentials phase-coupled at almost every gamma cycle (Csicsvari et al., 2003; Hájos et al., 2004; Kann et al., 2014).
Recordings of LFP, [K+]o, pO2 and Redox State
The LFP, which is also known as micro-, depth or intracranial electroencephalogram (EEG), has been frequently used to monitor neuronal network oscillations in vitro and in vivo. LFP electrodes are small-sized, have usually a resistance of about 1–2 MOhm and are positioned in the extracellular space. The recorded extracellular potentials arise from all transmembrane ionic fluxes that underlie cellular electrical events, ranging from fast action potentials and postsynaptic potentials in neurons to slow membrane potential fluctuations in glial cells (Buzsáki et al., 2012; Einevoll et al., 2013; Hales and Pockett, 2014). Recent estimates suggest that >95% of the LFP originates in the vicinity of about 250 μm of the electrode tip (Katzner et al., 2009). Thus, the LFP recording represents a spatial average of all electrical events in a confined volume of neuronal tissue at a given point in time. Although still under debate, the prominent influence of tip geometry and impedance has not been proven yet (Nelson and Pouget, 2010).
The [K+]o can be determined with double-barreled microelectrodes in neuronal tissue. The reference barrel (LFP) is filled with 154 mM NaCl solution, and the ion-sensitive barrel with an ion-exchanger (K+ ionophore cocktail) and 100 mM KCl ([K+]o; Heinemann and Lux, 1975; Gorji and Speckmann, 2009; Papageorgiou et al., 2016). Recordings of [K+]o have been used to determine the level of neuronal activation and K+-homeostasis, including the functions of glial cells (see below). K+-sensitive microelectrodes measure the accumulation of K+ in a restricted extracellular space, irrespective of whether K+ is released from dendrites, somata or axons. The microelectrodes detect changes in [K+]o from the surrounding tissue microenvironment of less than 100 μm for most conditions of experimental K+-electrophoresis or electrical stimulation (Lux, 1974; Heinemann et al., 1986; Lux et al., 1986; Kann et al., 2003a,b).
The interstitial partial oxygen pressure (pO2) or the oxygen concentration in neuronal tissue can be determined with Clark-type oxygen microsensors, which are polarographic electrodes. Because oxygen is the final electron acceptor at the mitochondrial respiratory chain, oxygen consumption provides a valuable indirect measure of the metabolic rate in tissues (Rolfe and Brown, 1997). During the recording, oxygen diffuses from the adjacent tissue through a silicone membrane at the sensor tip and is reduced at a gold cathode within the microsensor. The resulting current is measured with a picoammeter and converted into mmHg (or mmol/L) according to calibration curves (Revsbech, 1989; Lecoq et al., 2009; Thomsen et al., 2009). Oxygen microsensors measure quite locally, i.e., with a spatial resolution of 1–2 times the outside tip diameter (8–12 μm). Oxygen consumption rates (mmol/L per min) can be calculated by recording pO2 depth profiles in slice preparations and by applying mathematical models that consider convective transport, diffusion, and activity-dependent oxygen consumption (Hall and Attwell, 2008; Huchzermeyer et al., 2013).
The cellular redox state is a useful and non-invasive tool to get insight into neuronal energy metabolism. For this purpose, live-cell fluorescence imaging of nicotinamide adenine dinucleotide (phosphate) and flavin adenine dinucleotide [NAD(P)H and FAD, respectively] have been applied. These dinucleotides serve in cellular energy transfer and have been used to get insight into activity-dependent changes in cytosolic and mitochondrial redox state, and thus adaptations in energy metabolism (Kann et al., 2003a,b; Shuttleworth et al., 2003; Kasischke et al., 2004; Brennan et al., 2006; Ivanov et al., 2011). When excited with ultraviolet light the reduced forms (NADH and NADPH) are fluorescent, while the oxidized forms are non-fluorescent. Investigators often refer to changes in NAD(P)H fluorescence because the emission spectra of NADPH and NADH overlap, and their redox states are coupled via nicotinamide nucleotide transhydrogenase (Schuchmann et al., 2001; Kann and Kovács, 2007). Cellular NAD(P)H fluorescence is primarily governed by activities of the respiratory chain (electron transport chain) and the tricarboxylic acid (TCA) cycle in mitochondria. However, relative changes in NAD(P)H fluorescence in brain slices are influenced by a variety of additional factors and need careful interpretation (Kann and Kovács, 2007; Berndt et al., 2015). Electron transport flavoproteins and α-lipoamide dehydrogenase contribute to about 75% of the flavin fluorescence in neurons (Kann and Kovács, 2007). Because both of them are also closely linked to the mitochondrial NADH pool FAD fluorescence provides insight into the mitochondrial redox state (Shuttleworth et al., 2003; Kann and Kovács, 2007). Because here the oxidized form is fluorescent, changes in FAD fluorescence are opposite to NAD(P)H fluorescence. In most studies using epifluorescence recordings (epi-illuminating light source and CCD camera), the changes in NAD(P)H fluorescence originate from neuronal and glial compartments of virtually all layers (z-axis) of the slice (Shuttleworth et al., 2003; Foster et al., 2005; Kann and Kovács, 2007; Huchzermeyer et al., 2008).
Energy Metabolism During Gamma Oscillations
Recent experimental studies have started to define the bioenergetics of cortical GAM. The available experimental evidence from many in vitro and some in vivo studies in animals and humans indicates that GAM in the hippocampus and the neocortex are associated with significant cellular adaptations to maintain energy, ion and neurotransmitter homeostasis and thus neuronal excitability and synaptic signaling. Below, we discuss the homeostatic adaptations underlying GAM, with emphasis on activity-dependent changes in pO2, cellular redox state [NAD(P)H and FAD] and [K+]o.
Recordings of LFP and pO2 with high temporal and spatial resolution revealed a positive correlation between the power of GAM and the local decrease in pO2 in acute slices and slice cultures of the hippocampus, reflecting the increase in activity-dependent oxygen consumption (Kann et al., 2011). Intriguingly, the local decrease in pO2 during GAM was significantly larger compared to repetitive electrical stimulation (10 s, 20 Hz) and close to the decrease in pO2 associated with pathological activity, i.e., seizure-like events. These findings were supported by a second study in slice cultures of the rat hippocampus demonstrating the about twofold increase in local oxygen consumption rate during GAM (about 11 mmol/L per min) compared with spontaneous asynchronous neuronal network activity (about 5 mmol/L per min). This follow-up study was based on depth profiles of local pO2 with high spatial resolution and mathematical modeling of convective transport, diffusion and activity-dependent consumption of oxygen (Huchzermeyer et al., 2013). Notably, the oxygen microsensor measured locally in stratum pyramidale, i.e., the layer where the somata of pyramidal cells are densely packed and mainly receive strong perisomatic inhibition from the complex axon arbors of GABAergic parvalbumin-positive basket cells (Sik et al., 1993; Hu et al., 2014; Kann et al., 2014). Thus, the data reflect oxygen consumption related to postsynaptic inhibition and action potential generation in pyramidal cells as well as axonal action potentials and fast rhythmic release of GABA from basket cells (Kann et al., 2014). Further experimental studies are required to determine the fractions of energy utilization in pyramidal cells (perisomatic postsynaptic potentials and action potentials at the axon initial segment) vs. GABAergic basket cells (action potentials and GABA release in the complex axon arbor).
The oxygen consumption rates during persistent GAM are higher than those obtained during spontaneous asynchronous activity or repetitive electrical stimulation in acute hippocampal and cerebellar slices reported to range from 0.7 to 1.3 mmol/L per min (Hall and Attwell, 2008; Ivanov and Zilberter, 2011; Hall et al., 2012). Several aspects need to be considered when comparing oxygen consumption rates from different experimental studies in vitro and in vivo. The high oxygen consumption rate (Huchzermeyer et al., 2013) was determined during agonist-induced persistent GAM in the pyramidal cell layer (perisomatic region) of the CA3 network in slice cultures that feature high tissue preservation and connectivity (Zhao et al., 2012; Studer et al., 2014; Schneider et al., 2015), thus reflecting a high activity state. In addition, the local CA3 network is a generator of GAM of high power within the hippocampus, and the electrophysiological and bioenergetical features of this network may not entirely apply to other neuronal networks or cortical regions (Buzsáki, 2006; Traub and Whittington, 2010; Kann et al., 2014). Another aspect is the spatial resolution. “Local pO2” in the depth profiles as determined with oxygen microsensors in slice preparations refers to a distance of about 0.2 mm (Hall and Attwell, 2008; Huchzermeyer et al., 2013). For comparison, the resolution of functional magnetic resonance imaging (fMRI) technologies in vivo is in the order of 1 mm3 and more. However, the in vitro studies suggest that GAM are associated with high energy expenditure.
These findings are in line with studies in humans using positron emission tomography with 2-deoxy-2-[18F] fluoro-D-glucose. The studies demonstrated stimulus-dependent increases in glucose metabolic rate in primary and associative visual cortices of about 40% and 60%, respectively (Phelps et al., 1981) as well as a positive correlation between spectral amplitudes of GAM and the regional glucose uptake, which was determined during seizure-free intervals in patients with non-lesional focal epilepsy (Nishida et al., 2008). Remarkably, the correlations between glucose uptake and oscillations in other human frequency bands, such as theta (4–7 Hz), alpha (8–12 Hz) and beta (16–32 Hz) were found to be either minor or absent. The tight correlation between GAM and energy expenditure is supported by studies using fMRI as a measure of neurovascular coupling. The power of GAM positively correlated with the hemodynamic fMRI response in the cat visual cortex (Niessing et al., 2005). Positive correlations between GAM and fMRI signals were also described for the human cortex during specific tasks and for nearly the entire cerebral cortex of the monkey (Lachaux et al., 2007; Schölvinck et al., 2010; Scheeringa et al., 2011). In general, there appears to be a linear relationship between neuronal activity, energy metabolism and hemodynamic responses (Sheth et al., 2004; Martin et al., 2006; Viswanathan and Freeman, 2007; Hyder et al., 2013). However, details about the relationships between GAM in local networks, energy expenditure and adaptations in blood flow need to be defined in future studies (Sumiyoshi et al., 2012).
Recently, the utilization of various energy substrates during persistent GAM was explored in rat slice cultures. It was demonstrated that GAM can be powered by various energy-rich substrates, with glucose being most effective (Galow et al., 2014). Notably, the high concentration (20 mmol/L) of either lactate or pyruvate was necessary to maintain GAM. The amplitude of the GAM, however, was significantly reduced. In another study, the addition of lactate (2 mmol/L) to lowered glucose concentration (5 mmol/L) exclusively increased the frequency by about 4 Hz, whereas the power of GAM was unchanged (Schneider et al., 2015). Therefore, lactate appears to be much less beneficial to fuel fast neuronal network oscillations compared with neuronal activity that was evoked by electrical stimulation in slice preparations (Schurr et al., 1988; Izumi et al., 1997; Schurr and Payne, 2007; Ivanov et al., 2011; Barros, 2013).
Using FAD and NAD(P)H fluorescence imaging during GAM, the concomitant changes in the mitochondrial redox state were investigated in the CA3 network of hippocampal slice cultures. GAM were associated with a shift towards reduction of the dinucleotides although the interstitial pO2 was hyperoxic (Huchzermeyer et al., 2008; Kann et al., 2011; Figure 1A). This finding might reflect an increase in the availability of energy-rich carbon molecules as a result of enhanced glycolysis in neuronal and astrocytic compartments (Kasischke et al., 2004; Brennan et al., 2006; Hertz et al., 2007; Galow et al., 2014) or an imbalance in the activities of neuronal TCA cycle and mitochondrial respiratory chain. Moreover, repetitive electrical stimulation (20 Hz, 10 s) superimposed on GAM resulted in significantly smaller shifts towards oxidation of the dinucleotides compared to controls (Kann et al., 2011; Figure 1B). This suggests that GAM are associated with near-limit utilization of mitochondrial oxidative capacity and provides further evidence for the high energy expenditure during GAM. The data might also imply that rapid and sufficient supply of oxygen and nutrients through changes in blood flow is a fundamental prerequisite for the maintenance of ion and energy homeostasis and therefore, the capability of local neuronal networks to express fast oscillations (Scheeringa et al., 2011; Sumiyoshi et al., 2012; Huchzermeyer et al., 2013; Kann et al., 2014). However, prolonged performance of neuronal mitochondria carries an inherent risk of increased generation of superoxide anion at complexes I and III of the mitochondrial respiratory chain (Morán et al., 2012; Kann, 2016). Increased superoxide anion levels can favor the accumulation of hydrogen peroxide and the generation of other reactive oxygen and nitrogen species (ROS and RNS, respectively). Some of them are reactive molecules with high biological toxicity owing to the capability to oxidize macromolecules such as lipids, DNA, and proteins (Kann and Kovács, 2007; Morán et al., 2012). In particular, fast-spiking, parvalbumin-positive basket cells might transiently generate high ROS levels because the unique electrophysiological and bioenergetical characteristics may frequently result in mismatching changes of metabolic state, Ca2+-load and pO2 in mitochondria (Kann, 2016). Such mismatches have been discussed to promote the generation of superoxide anion (Kann and Kovács, 2007; Nicholls, 2008; Adam-Vizi and Starkov, 2010). Detailed experimental studies are required to determine free radical generation and oxidative stress in excitatory and inhibitory neurons during GAM.
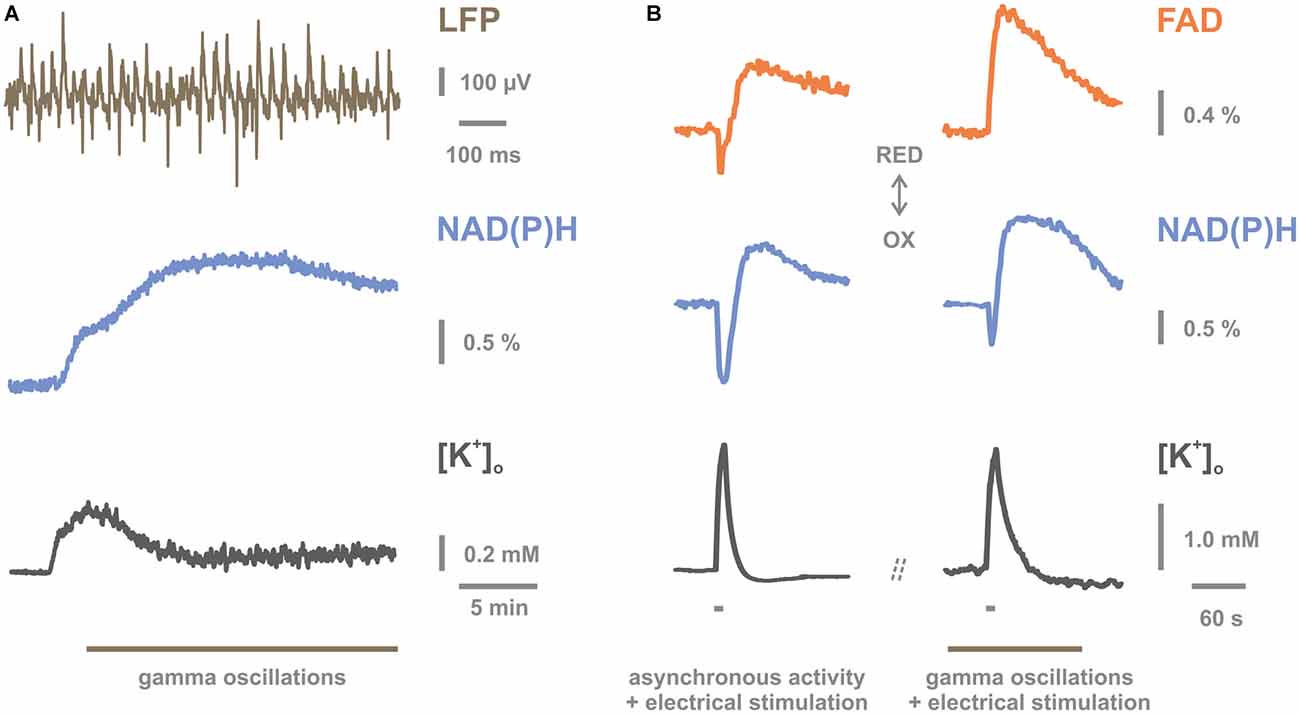
Figure 1. Mitochondrial redox state during gamma oscillations (GAM) in vitro. (A) Persistent GAM at around 40 Hz are generated in the presence of acetylcholine and physostigmine in the CA3 region of rat hippocampal slice cultures as revealed by local field potential (LFP) recordings (upper trace). Persistent GAM are associated with a long-lasting elevation in nicotinamide adenine dinucleotide (phosphate) (NAD(P)H) fluorescence (middle trace). This significant shift in the redox state towards reduction likely reflects the activation of reducing processes (tricarboxylic acid cycle and glycolysis) and/or the limitation of oxidizing processes (mitochondrial respiratory chain). Persistent GAM (symbolized by gray line) are associated with a fluctuating elevation in [K+]o of less than 0.2 mmol/L (lower trace). Note the higher temporal resolution of the upper trace illustrating GAM. (B) Simultaneous recordings of flavin adenine dinucleotide (FAD) (upper traces) and NAD(P)H (middle traces) fluorescence and [K+]o (lower traces) during spontaneous asynchronous activity (left) and persistent GAM (right). Recordings were made at identical locations during the two network activity states in a given slice culture. Superimposed repetitive electrical stimulation (10 s, 20 Hz, gray bars) resulted in FAD and NAD(P)H fluorescence transients with biphasic shapes relative to pre-stimulation baseline as well as transient changes in [K+]o. Upward deflections in fluorescence traces indicate shifts in the redox state towards reduction (gray arrow, RED), and downward deflections towards oxidation (gray arrow, OX; “REDOX” state). Biphasic transients are illustrated such that downward deflections in FAD and NAD(P)H indicate increase and decrease in emission fluorescence intensity, respectively. Note that amplitudes and kinetics of downward deflections in FAD and NAD(P)H fluorescence are clearly altered during GAM (right), likely indicating near-limit utilization of mitochondrial oxidative capacity. The K+-sensitive microelectrode was placed in stratum pyramidale, i.e., the layer where the somata of pyramidal cells are densely packed and receive strong perisomatic inhibition from the complex axon arbors of basket cells. Note that the decay time of [K+]o transients is prolonged during GAM (right). See details in the text. This figure is reproduced and modified from Kann et al. (2011) (Figures 5C, 7A,C) by permission of Oxford University Press on behalf of The Guarantors of Brain. This material is published under a Standard License and is not covered by any Creative Commons License. For commercial and non-commercial reuse, please, seek the permission ofam91cm5hbHMucGVybWlzc2lvbnNAb3VwLmNvbQ==.
Intriguingly, the electrophysiological and bioenergetic features of the hippocampal CA3 network, i.e., highest levels in gamma oscillation power, oxygen consumption, and mitochondrial performance, also correlated with the highest expression of complex I subunits (Kann et al., 2011; Wirtz and Schuelke, 2011). Complex I (NADH:ubiquinone oxidoreductase) is a member of the respiratory chain in mitochondria. It is composed of up to 46 subunits that are encoded by nuclear and mitochondrial DNA (Distelmaier et al., 2009). Complex I has been discussed to strongly control oxidative phosphorylation in mitochondria, and to be involved in the pathogenesis of various neurodegenerative diseases (Pathak and Davey, 2008; Koopman et al., 2013). The pattern of complex I gene expression in the hippocampus might reflect unique enzymatic properties of neuronal mitochondria in the CA3 network to meet the homeostatic challenges that are associated with the generation of GAM (Kann, 2012).
Conversely, a variety of studies demonstrated the fast decline of GAM in hippocampal slice preparations during metabolic stress, i.e., (i) by lowering the oxygen fraction in the ambient atmosphere to the normoxic range in the semi-interface recording condition (Huchzermeyer et al., 2008); (ii) by lowering the application rate of oxygenated recording solution in the submerged recording condition (Hájos et al., 2009); and (iii) by induction of hypoxic events (Fano et al., 2007; Pietersen et al., 2009). The fast decline of GAM was also found during pharmacological inhibition of the respiratory chain by rotenone (acting on complex I) or potassium cyanide (acting on cytochrome c oxidase in complex IV), and in the presence of protonophores that exert mitochondrial membrane uncoupling (Kann et al., 2011; Whittaker et al., 2011). Moreover, the exquisite sensitivity of GAM to mitochondrial dysfunction has been identified because other types of neuronal activity, such as electrical stimulus-evoked neuronal activation and pathological seizure-like events were more resistant to both, respiratory chain inhibition and low interstitial pO2 (Huchzermeyer et al., 2008; Kann et al., 2011). Similar observations were reported for unilateral hippocampal ischemia in vivo (Barth and Mody, 2011). These studies consistently show that hippocampal GAM are rapidly impaired during metabolic stress and are in line with data on high energy expenditure during GAM. Mechanistically, dysfunction of fast-spiking GABAergic interneurons, such as parvalbumin-positive basket cells, might be mainly responsible for this rapid impairment. This is likely because these inhibitory interneurons: (i) are crucial for the generation of GAM; and (ii) show unique bioenergetic, biophysical and electrophysiological properties (Kageyama and Wong-Riley, 1982; Gulyás et al., 2006; Hu and Jonas, 2014; Takács et al., 2015). Further details were recently summarized and discussed in reviews about the “interneuron energy hypothesis” (Kann et al., 2014; Kann, 2016).
Potassium Ion Homeostasis During Gamma Oscillations
The high energy expenditure of neurons during GAM is most likely caused by increased rates of action potentials and postsynaptic potentials. In particular, the significant and widespread increase in rates of EPSPs and IPSPs in the local network elicits strong ion fluxes across the neuronal membrane of both excitatory principal cells and inhibitory interneurons (Wong-Riley, 1989; Hájos and Paulsen, 2009; Kann et al., 2014). These ion fluxes tend to dissipate the gradients of sodium, calcium, potassium and chloride ions, ultimately utilizing potential energy. In order to keep homeostasis and neuronal excitability and thus, ensure maintenance of neuronal information processing, these ionic gradients have to be continuously restored by ion pumps, such as Na+/K+-ATPase and Ca2+-ATPase, and transporters, such as Na+/Ca2+-exchanger, Na+/H+-exchanger, and K+/Cl−-cotransporter (Somjen, 2002; Buzsáki et al., 2007; Kann et al., 2014). In addition, synthesis, release and uptake of neurotransmitters and precursors require various transport processes across neuronal and glial membranes (Bak et al., 2006; Roth and Draguhn, 2012). These transport processes are ultimately powered by cellular energy carrier, adenosine-5′-triphosphate (ATP) that is generated to a large extent by oxidative phosphorylation in neuronal mitochondria (Attwell and Laughlin, 2001; Erecińska and Silver, 2001).
However, experimental studies that explored the ion homeostasis during naturally occurring, fast neuronal network oscillations are rare. The few available studies focused on changes in [K+]o in hippocampal slice preparations (see below). [K+]o was monitored by double-barreled microelectrodes (see above). [K+]o is normally between 2.7 to 3.8 mmol/L in neuronal tissue in vivo, which is lower than in the other extracellular fluids of the body (Prince et al., 1973; Lux, 1974; Heinemann and Lux, 1977; Somjen, 2002; Gorji and Speckmann, 2009). Keeping [K+]o in this narrow range protects central neurons from undue influences on excitability and synaptic transmission because the elevation in [K+]o generally depolarizes neuronal membranes, and substantial [K+]o elevation has drastic consequences, such as the pathological occurrence of epileptic activity (Zuckermann and Glaser, 1968; Heinemann et al., 1986; Janigro et al., 1997; Somjen, 2002; Jandová et al., 2006; Steinhäuser et al., 2012). Normally, the generation of action potentials and postsynaptic potentials results in K+-release from neurons, e.g., through voltage-gated K+-channels and non-selective cation channels (Somjen, 2002; Buzsáki et al., 2007; Figure 2). At inhibitory synapses, K+-release can be evoked by pre- and postsynaptic GABA-B receptors that open K+-channels. In addition, at the postsynaptic membrane K+-release drives the extrusion of chloride through K+/Cl−-cotransporters, such as KCC2, to reverse the Cl−-influx that underlies the hyperpolarizing action of GABA-A receptors (Mann and Paulsen, 2007; Blaesse et al., 2009; Kaila et al., 2014). The activation of GABA-A receptors, however, might also cause shunting inhibition in both principal cells and interneurons (Alger and Nicoll, 1979; Andersen et al., 1980; Bartos et al., 2007; Mann and Paulsen, 2007). K+-uptake occurs mainly through Na+/K+-ATPase of neurons and astrocytes. Under certain conditions, it might be supported by astrocytic K+-transporters, K+-channels and gap-junctions that permit K+-buffering and spatial redistribution within the astrocyte syncytium (Somjen, 2002; Steinhäuser et al., 2012). There is first evidence for the role of astrocytes in GAM because functional manipulation of astrocytes markedly decreased the EEG power in the gamma frequency band in awake-behaving mice, whereas neuronal synaptic activity remained intact. The reduction in cortical GAM was accompanied by altered behavioral performance in the novel object recognition test (Lee et al., 2014). However, the details about the nature of the role of astrocytes, for example, in ion and/or neurotransmitter homeostasis, are less clear and require further experimental studies.
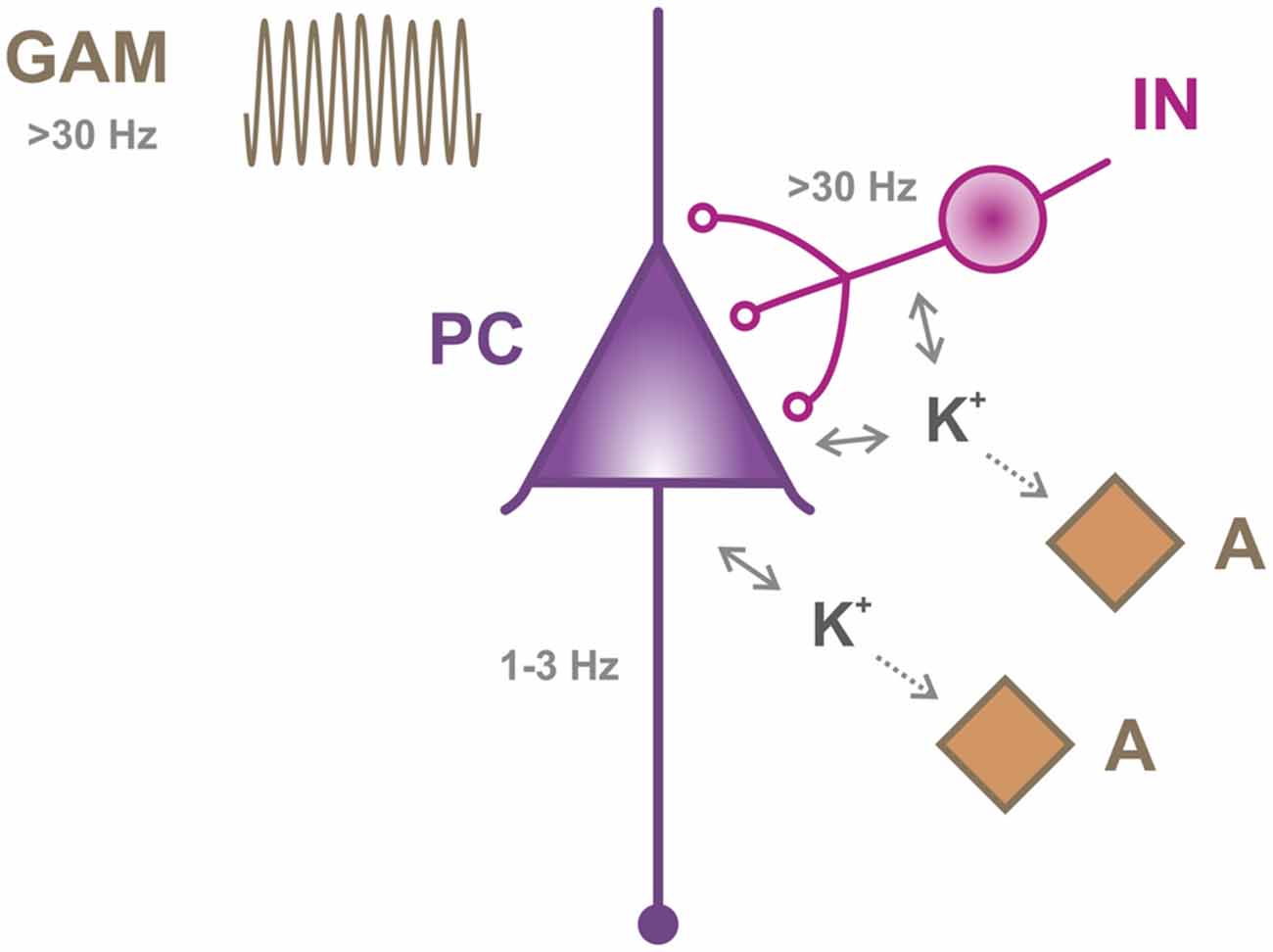
Figure 2. K+-homeostasis during gamma oscillations (GAM) in vitro. The schematic representation illustrates key features of GAM (30–100 Hz) in stratum pyramidale of hippocampal slice preparations. GAM require precise synaptic interactions of excitatory principal cells, such as pyramidal cells (PC), and fast-spiking, inhibitory interneurons (IN), such as parvalbumin-positive basket cells, which feature complex axon arbors. In the hippocampus, fast-spiking interneurons exert fast rhythmic inhibition on the perisomatic region of pyramidal cells by phasic release of GABA from presynaptic terminals (open circles). Interactions with other subtypes of interneurons and excitation of interneurons by pyramidal cells are not illustrated. During GAM, enhanced K+-release in stratum pyramidale occurs at several neuronal sites: (i) axons of interneurons and pyramidal cells that fire action potentials at different frequencies; (ii) presynaptic endings of interneurons; and (iii) perisomatic postsynaptic membranes of pyramidal cells. The K+-uptake occurs mainly in neurons and might be supported by adjacent astrocytes (A). Note that [K+]o does not exceed 3.5 mmol/L during GAM. Note that GAM are associated with a high oxygen consumption rate, indicating adaptations of adenosine-5′-triphosphate (ATP) generation in mitochondria to power ion pumps and secondary ion transport. See details in the text.
Changes in [K+]o associated with GAM were explored in the CA3 network of hippocampal slice cultures (Figure 2). The ion-sensitive microelectrodes measured [K+]o locally in stratum pyramidale, i.e., the layer where the somata of pyramidal cells are densely packed and receive strong perisomatic inhibition from the complex axon arbors of basket cells (Kann et al., 2014). It was shown that the pharmacological induction of GAM by bath application of acetylcholine at low micromolar concentrations evoked an initial transient increase in [K+]o of about 0.5 mmol/L from the baseline of 3 mmol/L. This was followed by a fluctuating elevation in [K+]o of less than 0.2 mmol/L when persistent GAM were present (Huchzermeyer et al., 2008; Kann et al., 2011; Figure 1A). In addition, repetitive electrical stimulation was superimposed on persistent GAM to get further insight into K+-homeostasis during fast neuronal network oscillations (Kann et al., 2011; Figure 1B). In these experiments, the amplitude of the electrically evoked [K+]o transient did not differ in the absence (i.e., spontaneous asynchronous activity) and presence of GAM, indicating the same level of superimposed neuronal activation. By contrast, the decay time of the evoked [K+]o transients was prolonged. This might reflect that K+-uptake mechanisms, such as Na+/K+-ATPase activity and glial K+-buffering, operate near limit during persistent GAM. Similar to GAM, sharp wave-ripples (SPW-Rs) were associated with a transient increase in [K+]o of about 0.1 mmol/L in the CA3 network of acute hippocampal slices (Behrens et al., 2007). In this model, SPW-Rs that were induced by repetitive electrical stimulation lasted for about 70 ms, with an incidence of about 8/min (Behrens et al., 2007; Hollnagel et al., 2014). The superimposed ripples had a frequency of about 190 Hz. SPW-Rs represent a different type of fast and highly synchronous neuronal network oscillations (Draguhn et al., 1998; Maier et al., 2003; Behrens et al., 2005; Schönberger et al., 2014). They occur during consummatory behaviors and non-REM sleep and are thought to represent stored information that is transferred to, for example, the neocortex during memory consolidation (Behrens et al., 2005; Hollnagel et al., 2014; Buzsáki, 2015).
These findings provide first experimental evidence that [K+]o indeed does not exceed the upper limit of about 3.5 mmol/L during fast neuronal network oscillations. This is in line with in vivo studies showing that optical stimuli transiently elevated [K+]o by about 0.5 mmol/L in the cat visual cortex (Singer and Lux, 1975; Connors et al., 1979; Somjen, 2002). Slow neuronal network oscillations, such as sleep oscillations (<1 Hz) in the cat neocortex, were associated with periodic elevations in [K+]o of about 1.8 mmol/L (Amzica and Steriade, 2000). These data contrast with in vitro and in vivo studies, in which repetitive electrical stimulation (up to 100 Hz, up to 60 s) was applied as a tool to activate neurons. In these studies much larger [K+]o transients of up to 10 mmol/L from baseline were described (Heinemann and Lux, 1975, 1977; Lothman and Somjen, 1975; Gabriel et al., 1998; Kann et al., 2003b; Huchzermeyer et al., 2008). The most likely explanation is that the artificial and robust electrical stimulation evokes action potentials at unphysiological frequencies in most of the excitatory and inhibitory neurons adjacent to the stimulation electrode (Heinemann and Lux, 1977; Janigro et al., 1997)—note that during hippocampal GAM, for example, excitatory pyramidal cells and fast-spiking interneurons generate action potentials at 1–3 Hz and >30 Hz, respectively (Kann et al., 2014). Although there is depression or attenuation of spiking according to the biophysical properties of the neuronal subtype (Wong and Prince, 1981; Madison and Nicoll, 1984; Martina and Jonas, 1997; Kann et al., 2003b; Kim et al., 2012), the resulting bulk K+-release from neurons presumably exceeds the K+-uptake mechanisms in neurons and glial cells during repetitive electrical stimulation, which is reflected by the characteristic stimulus-induced [K+]o transients. However, further experimental studies are required to determine the contribution of specific ion channels, transporters and pumps in neurons and glial cells to K+-homeostasis during different network activity states.
The maintenance of K+-homeostasis during naturally occurring fast network oscillations is likely achieved by strongly enhanced ATP generation in mitochondria—reflected by high oxygen consumption rate and near-limit utilization of oxidative capacity during GAM (see above)—to fuel ion pumps and secondary ion transport.
Conclusion
Fast neuronal network oscillations in the gamma frequency band (30–100 Hz) occur in various regions of the cortex and have been implicated in higher brain functions such as sensory perception, attentional selection and memory formation. These GAM are associated with precise cellular adaptations to maintain energy and ion homeostasis and thus neuronal excitability and synaptic signaling. Homeostasis is apparently safeguarded by strongly enhanced oxidative phosphorylation in mitochondria to generate ATP. Conversely, metabolic stress and/or ion channel dysfunction might contribute to the exceptional vulnerability of GAM and thus higher brain functions in brain disease.
Author Contributions
OK, J-OH, SE and JS wrote the manuscript. OK created the figures.
Conflict of Interest Statement
The authors declare that the research was conducted in the absence of any commercial or financial relationships that could be construed as a potential conflict of interest.
Acknowledgments
The authors thank Andrea Lewen for text editing assistance. This work was funded by the German Research Foundation (DFG) within the Collaborative Research Center (SFB) 1134 (project B02).
References
Adam-Vizi, V., and Starkov, A. A. (2010). Calcium and mitochondrial reactive oxygen species generation: how to read the facts. J. Alzheimers Dis. 20, S413–S426. doi: 10.3233/JAD-2010-100465
Alger, B. E., and Nicoll, R. A. (1979). GABA-mediated biphasic inhibitory responses in hippocampus. Nature 281, 315–317. doi: 10.1038/281315a0
Amzica, F., and Steriade, M. (2000). Neuronal and glial membrane potentials during sleep and paroxysmal oscillations in the neocortex. J. Neurosci. 20, 6648–6665.
Andersen, P., Dingledine, R., Gjerstad, L., Langmoen, I. A., and Mosfeldt Laursen, A. (1980). Two different responses of hippocampal pyramidal cells to application of gamma-amino butyric acid. J. Physiol. 305, 279–296. doi: 10.1113/jphysiol.1980.sp013363
Attwell, D., and Laughlin, S. B. (2001). An energy budget for signaling in the grey matter of the brain. J. Cereb. Blood Flow Metab. 21, 1133–1145. doi: 10.1097/00004647-200110000-00001
Bak, L. K., Schousboe, A., and Waagepetersen, H. S. (2006). The glutamate/GABA-glutamine cycle: aspects of transport, neurotransmitter homeostasis and ammonia transfer. J. Neurochem. 98, 641–653. doi: 10.1111/j.1471-4159.2006.03913.x
Barros, L. F. (2013). Metabolic signaling by lactate in the brain. Trends Neurosci. 36, 396–404. doi: 10.1016/j.tins.2013.04.002
Barth, A. M., and Mody, I. (2011). Changes in hippocampal neuronal activity during and after unilateral selective hippocampal ischemia in vivo. J. Neurosci. 31, 851–860. doi: 10.1523/JNEUROSCI.5080-10.2011
Bartos, M., Vida, I., and Jonas, P. (2007). Synaptic mechanisms of synchronized gamma oscillations in inhibitory interneuron networks. Nat. Rev. Neurosci. 8, 45–56. doi: 10.1038/nrn2044
Behrens, C. J., van den Boom, L. P., de Hoz, L., Friedman, A., and Heinemann, U. (2005). Induction of sharp wave-ripple complexes in vitro and reorganization of hippocampal networks. Nat. Neurosci. 8, 1560–1567. doi: 10.1038/nn1571
Behrens, C. J., van den Boom, L. P., and Heinemann, U. (2007). Effects of the GABAA receptor antagonists bicuculline and gabazine on stimulus-induced sharp wave-ripple complexes in adult rat hippocampus in vitro. Eur. J. Neurosci. 25, 2170–2181. doi: 10.1111/j.1460-9568.2007.05462.x
Berndt, N., Kann, O., and Holzhütter, H.-G. (2015). Physiology-based kinetic modeling of neuronal energy metabolism unravels the molecular basis of NAD(P)H fluorescence transients. J. Cereb. Blood Flow Metab. 35, 1494–1506. doi: 10.1038/jcbfm.2015.70
Blaesse, P., Airaksinen, M. S., Rivera, C., and Kaila, K. (2009). Cation-chloride cotransporters and neuronal function. Neuron 61, 820–838. doi: 10.1016/j.neuron.2009.03.003
Bliss, T. V. P., and Lømo, T. (1973). Long-lasting potentiation of synaptic transmission in the dentate area of the anaesthetized rabbit following stimulation of the perforant path. J. Physiol. 232, 331–356. doi: 10.1113/jphysiol.1973.sp010273
Bragin, A., Jandó, G., Nádasdy, Z., Hetke, J., Wise, K., and Buzsáki, G. (1995). Gamma (40–100 Hz) oscillation in the hippocampus of the behaving rat. J. Neurosci. 15, 47–60.
Brennan, A. M., Connor, J. A., and Shuttleworth, C. W. (2006). NAD(P)H fluorescence transients after synaptic activity in brain slices: predominant role of mitochondrial function. J. Cereb. Blood Flow Metab. 26, 1389–1406. doi: 10.1038/sj.jcbfm.9600292
Buzsáki, G. (2015). Hippocampal sharp wave-ripple: a cognitive biomarker for episodic memory and planning. Hippocampus 25, 1073–1088. doi: 10.1002/hipo.22488
Buzsáki, G., Anastassiou, C. A., and Koch, C. (2012). The origin of extracellular fields and currents - EEG, ECoG, LFP and spikes. Nat. Rev. Neurosci. 13, 407–420. doi: 10.1038/nrn3241
Buzsáki, G., and Chrobak, J. J. (1995). Temporal structure in spatially organized neuronal ensembles: a role for interneuronal networks. Curr. Opin. Neurobiol. 5, 504–510. doi: 10.1016/0959-4388(95)80012-3
Buzsáki, G., and Draguhn, A. (2004). Neuronal oscillations in cortical networks. Science 304, 1926–1929. doi: 10.1126/science.1099745
Buzsáki, G., Kaila, K., and Raichle, M. (2007). Inhibition and brain work. Neuron 56, 771–783. doi: 10.1016/j.neuron.2007.11.008
Cardin, J. A., Carlén, M., Meletis, K., Knoblich, U., Zhang, F., Deisseroth, K., et al. (2009). Driving fast-spiking cells induces gamma rhythm and controls sensory responses. Nature 459, 663–667. doi: 10.1038/nature08002
Cheyne, D., Bells, S., Ferrari, P., Gaetz, W., and Bostan, A. C. (2008). Self-paced movements induce high-frequency gamma oscillations in primary motor cortex. Neuroimage 42, 332–342. doi: 10.1016/j.neuroimage.2008.04.178
Connors, B., Dray, A., Fox, P., Hilmy, M., and Somjen, G. (1979). LSD’s effect on neuron populations in visual cortex gauged by transient responses of extracellular potassium evoked by optical stimuli. Neurosci. Lett. 13, 147–150. doi: 10.1016/0304-3940(79)90032-6
Csicsvari, J., Jamieson, B., Wise, K. D., and Buzsáki, G. (2003). Mechanisms of gamma oscillations in the hippocampus of the behaving rat. Neuron 37, 311–322. doi: 10.1016/s0896-6273(02)01169-8
Distelmaier, F., Koopman, W. J., van den Heuvel, L. P., Rodenburg, R. J., Mayatepek, E., Willems, P. H., et al. (2009). Mitochondrial complex I deficiency: from organelle dysfunction to clinical disease. Brain 132, 833–842. doi: 10.1093/brain/awp058
Draguhn, A., Traub, R. D., Schmitz, D., and Jefferys, J. G. R. (1998). Electrical coupling underlies high-frequency oscillations in the hippocampus in vitro. Nature 394, 189–192. doi: 10.1038/28184
Einevoll, G. T., Kayser, C., Logothetis, N. K., and Panzeri, S. (2013). Modelling and analysis of local field potentials for studying the function of cortical circuits. Nat. Rev. Neurosci. 14, 770–785. doi: 10.1038/nrn3599
Erecińska, M., and Silver, I. A. (2001). Tissue oxygen tension and brain sensitivity to hypoxia. Respir. Physiol. 128, 263–276. doi: 10.1016/s0034-5687(01)00306-1
Fano, S., Behrens, C. J., and Heinemann, U. (2007). Hypoxia suppresses kainate-induced γ-oscillations in rat hippocampal slices. Neuroreport 18, 1827–1831. doi: 10.1097/wnr.0b013e3282f13e4f
Fellous, J.-M., and Sejnowski, T. J. (2000). Cholinergic induction of oscillations in the hippocampal slice in the slow (0.5–2 Hz), theta (5–12 Hz) and gamma (35–70 Hz) bands. Hippocampus 10, 187–197. doi: 10.1002/(SICI)1098-1063(2000)10:2<187::AID-HIPO8>3.0.CO;2-M
Fisahn, A., Pike, F. G., Buhl, E. H., and Paulsen, O. (1998). Cholinergic induction of network oscillations at 40 Hz in the hippocampus in vitro. Nature 394, 186–189. doi: 10.1038/28179
Fischer, Y., Wittner, L., Freund, T. F., and Gähwiler, B. H. (2002). Simultaneous activation of gamma and theta network oscillations in rat hippocampal slice cultures. J. Physiol. 539, 857–868. doi: 10.1113/jphysiol.2001.013050
Foster, K. A., Beaver, C. J., and Turner, D. A. (2005). Interaction between tissue oxygen tension and NADH imaging during synaptic stimulation and hypoxia in rat hippocampal slices. Neuroscience 132, 645–657. doi: 10.1016/j.neuroscience.2005.01.040
Franowicz, M. N., and Barth, D. S. (1995). Comparison of evoked potentials and high-frequency (gamma-band) oscillating potentials in rat auditory cortex. J. Neurophysiol. 74, 96–112.
Fries, P., Nikolić, D., and Singer, W. (2007). The gamma cycle. Trends Neurosci. 30, 309–316. doi: 10.1016/j.tins.2007.05.005
Fritschy, J.-M., and Panzanelli, P. (2014). GABAA receptors and plasticity of inhibitory neurotransmission in the central nervous system. Eur. J. Neurosci. 39, 1845–1865. doi: 10.1111/ejn.12534
Fuchs, E. C., Zivkovic, A. R., Cunningham, M. O., Middleton, S., LeBeau, F. E. N., Bannerman, D. M., et al. (2007). Recruitment of parvalbumin-positive interneurons determines hippocampal function and associated behavior. Neuron 53, 591–604. doi: 10.1016/j.neuron.2007.01.031
Gabriel, S., Eilers, A., Kivi, A., Kovacs, R., Schulze, K., Lehmann, T.-N., et al. (1998). Effects of barium on stimulus induced changes in extracellular potassium concentration in area CA1 of hippocampal slices from normal and pilocarpine-treated epileptic rats. Neurosci. Lett. 242, 9–12. doi: 10.1016/s0304-3940(98)00012-3
Galow, L. V., Schneider, J., Lewen, A., Ta, T. T., Papageorgiou, I. E., and Kann, O. (2014). Energy substrates that fuel fast neuronal network oscillations. Front. Neurosci. 8:398. doi: 10.3389/fnins.2014.00398
Gloveli, T., Dugladze, T., Saha, S., Monyer, H., Heinemann, U., Traub, R. D., et al. (2005). Differential involvement of oriens/pyramidale interneurones in hippocampal network oscillations in vitro. J. Physiol. 562, 131–147. doi: 10.1113/jphysiol.2004.073007
Gorji, A., and Speckmann, E.-J. (2009). Epileptiform EEG spikes and their functional significance. Clin. EEG Neurosci. 40, 230–233. doi: 10.1177/155005940904000404
Gray, C. M., König, P., Engel, A. K., and Singer, W. (1989). Oscillatory responses in cat visual cortex exhibit inter-columnar synchronization which reflects global stimulus properties. Nature 338, 334–337. doi: 10.1038/338334a0
Gray, C. M., and Viana Di Prisco, G. (1997). Stimulus-dependent neuronal oscillations and local synchronization in striate cortex of the alert cat. J. Neurosci. 17, 3239–3253.
Gulyás, A. I., Buzsáki, G., Freund, T. F., and Hirase, H. (2006). Populations of hippocampal inhibitory neurons express different levels of cytochrome c. Eur. J. Neurosci. 23, 2581–2594. doi: 10.1111/j.1460-9568.2006.04814.x
Gulyás, A. I., Szabó, G. G., Ulbert, I., Holderith, N., Monyer, H., Erdélyi, F., et al. (2010). Parvalbumin-containing fast-spiking basket cells generate the field potential oscillations induced by cholinergic receptor activation in the hippocampus. J. Neurosci. 30, 15134–15145. doi: 10.1523/JNEUROSCI.4104-10.2010
Haenschel, C., Baldeweg, T., Croft, R. J., Whittington, M., and Gruzelier, J. (2000). Gamma and beta frequency oscillations in response to novel auditory stimuli: a comparison of human electroencephalogram (EEG) data with in vitro models. Proc. Natl. Acad. Sci. U S A 97, 7645–7650. doi: 10.1073/pnas.120162397
Hájos, N., Ellender, T. J., Zemankovics, R., Mann, E. O., Exley, R., Cragg, S. J., et al. (2009). Maintaining network activity in submerged hippocampal slices: importance of oxygen supply. Eur. J. Neurosci. 29, 319–327. doi: 10.1111/j.1460-9568.2008.06577.x
Hájos, N., Pálhalmi, J., Mann, E. O., Németh, B., Paulsen, O., and Freund, T. F. (2004). Spike timing of distinct types of GABAergic interneuron during hippocampal gamma oscillations in vitro. J. Neurosci. 24, 9127–9137. doi: 10.1523/JNEUROSCI.2113-04.2004
Hájos, N., and Paulsen, O. (2009). Network mechanisms of gamma oscillations in the CA3 region of the hippocampus. Neural Netw. 22, 1113–1119. doi: 10.1016/j.neunet.2009.07.024
Hales, C. G., and Pockett, S. (2014). The relationship between local field potentials (LFPs) and the electromagnetic fields that give rise to them. Front. Syst. Neurosci. 8:233. doi: 10.3389/fnsys.2014.00233
Hall, C. N., and Attwell, D. (2008). Assessing the physiological concentration and targets of nitric oxide in brain tissue. J. Physiol. 586, 3597–3615. doi: 10.1113/jphysiol.2008.154724
Hall, C. N., Klein-Flügge, M. C., Howarth, C., and Attwell, D. (2012). Oxidative phosphorylation, not glycolysis, powers presynaptic and postsynaptic mechanisms underlying brain information processing. J. Neurosci. 32, 8940–8951. doi: 10.1523/JNEUROSCI.0026-12.2012
Heinemann, U., Konnerth, A., Pumain, R., and Wadman, W. J. (1986). Extracellular calcium and potassium concentration changes in chronic epileptic brain tissue. Adv. Neurol. 44, 641–661.
Heinemann, U., and Lux, H. D. (1975). Undershoots following stimulus-induced rises of extracellular potassium concentration in cerebral cortex of cat. Brain Res. 93, 63–76. doi: 10.1016/0006-8993(75)90286-3
Heinemann, U., and Lux, H. D. (1977). Ceiling of stimulus induced rises in extracellular potassium concentration in the cerebral cortex of cat. Brain Res. 120, 231–249. doi: 10.1016/0006-8993(77)90903-9
Hertz, L., Peng, L., and Dienel, G. A. (2007). Energy metabolism in astrocytes: high rate of oxidative metabolism and spatiotemporal dependence on glycolysis/glycogenolysis. J. Cereb. Blood Flow Metab. 27, 219–249. doi: 10.1038/sj.jcbfm.9600343
Ho, V. M., Lee, J. A., and Martin, K. C. (2011). The cell biology of synaptic plasticity. Science 334, 623–638. doi: 10.1126/science.1209236
Hollnagel, J. O., Maslarova, A., ul Haq, R., and Heinemann, U. (2014). GABAB receptor dependent modulation of sharp wave-ripple complexes in the rat hippocampus in vitro. Neurosci. Lett. 574, 15–20. doi: 10.1016/j.neulet.2014.04.045
Hollnagel, J. O., ul Haq, R., Behrens, C. J., Maslarova, A., Mody, I., and Heinemann, U. (2015). No evidence for role of extracellular choline-acetyltransferase in generation of gamma oscillations in rat hippocampal slices in vitro. Neuroscience 284, 459–469. doi: 10.1016/j.neuroscience.2014.10.016
Hu, H., Gan, J., and Jonas, P. (2014). Fast-spiking, parvalbumin+ GABAergic interneurons: from cellular design to microcircuit function. Science 345:1255263. doi: 10.1126/science.1255263
Hu, H., and Jonas, P. (2014). A supercritical density of Na+ channels ensures fast signaling in GABAergic interneuron axons. Nat. Neurosci. 17, 686–693. doi: 10.1038/nn.3678
Huchzermeyer, C., Albus, K., Gabriel, H. J., Otáhal, J., Taubenberger, N., Heinemann, U., et al. (2008). Gamma oscillations and spontaneous network activity in the hippocampus are highly sensitive to decreases in pO2 and concomitant changes in mitochondrial redox state. J. Neurosci. 28, 1153–1162. doi: 10.1523/JNEUROSCI.4105-07.2008
Huchzermeyer, C., Berndt, N., Holzhütter, H.-G., and Kann, O. (2013). Oxygen consumption rates during three different neuronal activity states in the hippocampal CA3 network. J. Cereb. Blood Flow Metab. 33, 263–271. doi: 10.1038/jcbfm.2012.165
Hyder, F., Rothman, D. L., and Bennet, M. R. (2013). Cortical energy demand of signaling and nonsignaling components in brain are conserved across mammalian species and activity levels. Proc. Natl. Acad. Sci. U S A 110, 3549–3554. doi: 10.1073/pnas.1214912110
Ivanov, A., Mukhtarov, M., Bregestovski, P., and Zilberter, Y. (2011). Lactate effectively covers energy demands during neuronal network activity in neonatal hippocampal slices. Front. Neuroenergetics 3:2. doi: 10.3389/fnene.2011.00002
Ivanov, A., and Zilberter, Y. (2011). Critical state of energy metabolism in brain slices: the principal role of oxygen delivery and energy substrates in shaping neuronal activity. Front. Neuroenergetics 3:9. doi: 10.3389/fnene.2011.00009
Izumi, Y., Benz, A. M., Katsuki, H., and Zorumski, C. F. (1997). Endogenous monocarboxylates sustain hippocampal synaptic function and morphological integrity during energy deprivation. J. Neurosci. 17, 9448–9457.
Jandová, K., Päsler, D., Antonio, L. L., Raue, C., Ji, S., Njunting, M., et al. (2006). Carbamazepine-resistance in the epileptic dentate gyrus of human hippocampal slices. Brain 129, 3290–3306. doi: 10.1093/brain/awl218
Janigro, D., Gasparini, S., D’Ambrosio, R., McKhann, G. II, and DiFrancesco, D. (1997). Reduction of K+ uptake in glia prevents long-term depression maintenance and causes epileptiform activity. J. Neurosci. 17, 2813–2824.
Kageyama, G. H., and Wong-Riley, M. T. (1982). Histochemical localization of cytochrome oxidase in the hippocampus: correlation with specific neuronal types and afferent pathways. Neuroscience 7, 2337–2361. doi: 10.1016/0306-4522(82)90199-3
Kaila, K., Price, T. J., Payne, J. A., Puskarjov, M., and Voipio, J. (2014). Cation-chloride cotransporters in neuronal development, plasticity and disease. Nat. Rev. Neurosci. 15, 637–654. doi: 10.1038/nrn3819
Kann, O. (2012). The energy demand of fast neuronal network oscillations: insights from brain slice preparations. Front. Pharmacol. 2:90. doi: 10.3389/fphar.2011.00090
Kann, O. (2016). The interneuron energy hypothesis: implications for brain disease. Neurobiol. Dis. 90, 75–85. doi: 10.1016/j.nbd.2015.08.005
Kann, O., Huchzermeyer, C., Kovács, R., Wirtz, S., and Schuelke, M. (2011). Gamma oscillations in the hippocampus require high complex I gene expression and strong functional performance of mitochondria. Brain 134, 345–358. doi: 10.1093/brain/awq333
Kann, O., and Kovács, R. (2007). Mitochondria and neuronal activity. Am. J. Physiol. Cell Physiol. 292, C641–C657. doi: 10.1152/ajpcell.00222.2006
Kann, O., Kovács, R., and Heinemann, U. (2003a). Metabotropic receptor-mediated Ca2+ signaling elevates mitochondrial Ca2+ and stimulates oxidative metabolism in hippocampal slice cultures. J. Neurophysiol. 90, 613–621. doi: 10.1152/jn.00042.2003
Kann, O., Schuchmann, S., Buchheim, K., and Heinemann, U. (2003b). Coupling of neuronal activity and mitochondrial metabolism as revealed by NAD(P)H fluorescence signals in organotypic hippocampal slice cultures of the rat. Neuroscience 119, 87–100. doi: 10.1016/s0306-4522(03)00026-5
Kann, O., Papageorgiou, I. E., and Draguhn, A. (2014). Highly energized inhibitory interneurons are a central element for information processing in cortical networks. J. Cereb. Blood Flow Metab. 34, 1270–1282. doi: 10.1038/jcbfm.2014.104
Kasischke, K. A., Vishwasrao, H. D., Fisher, P. J., Zipfel, W. R., and Webb, W. W. (2004). Neural activity triggers neuronal oxidative metabolism followed by astrocytic glycolysis. Science 305, 99–103. doi: 10.1126/science.1096485
Katzner, S., Nauhaus, I., Benucci, A., Bonin, V., Ringach, D. L., and Carandini, M. (2009). Local origin of field potentials in visual cortex. Neuron 61, 35–41. doi: 10.1016/j.neuron.2008.11.016
Kim, E., Owen, B., Holmes, W. R., and Grover, L. M. (2012). Decreased afferent excitability contributes to synaptic depression during high-frequency stimulation in hippocampal area CA1. J. Neurophysiol. 108, 1965–1976. doi: 10.1152/jn.00276.2011
Klausberger, T., and Somogyi, P. (2008). Neuronal diversity and temporal dynamics: the unity of hippocampal circuit operations. Science 321, 53–57. doi: 10.1126/science.1149381
Koopman, W. J., Distelmaier, F., Smeitink, J. A., and Willems, P. H. (2013). OXPHOS mutations and neurodegeneration. EMBO J. 32, 9–29. doi: 10.1038/emboj.2012.300
Korotkova, T., Fuchs, E. C., Ponomarenko, A., von Engelhardt, J., and Monyer, H. (2010). NMDA receptor ablation on parvalbumin-positive interneurons impairs hippocampal synchrony, spatial representations and working memory. Neuron 68, 557–569. doi: 10.1016/j.neuron.2010.09.017
Kreiter, A. K., and Singer, W. (1992). Oscillatory neuronal responses in the visual cortex of the awake macaque monkey. Eur. J. Neurosci. 4, 369–375. doi: 10.1111/j.1460-9568.1992.tb00884.x
Kullmann, D. M., and Lamsa, K. P. (2007). Long-term synaptic plasticity in hippocampal interneurons. Nat. Rev. Neurosci. 8, 687–699. doi: 10.1038/nrn2207
Lachaux, J. P., Fonlupt, P., Kahane, P., Minotti, L., Hoffmann, D., Bertrand, O., et al. (2007). Relationship between task-related gamma oscillations and BOLD signal: new insights from combined fMRI and intracranial EEG. Hum. Brain Mapp. 28, 1368–1375. doi: 10.1002/hbm.20352
Lebedev, M. A., and Nelson, R. J. (1995). Rhythmically firing (20–50 Hz) neurons in monkey primary somatosensory cortex: activity patterns during initiation of vibratory-cued hand movements. J. Comput. Neurosci. 2, 313–334. doi: 10.1007/bf00961443
Lecoq, J., Tiret, P., Najac, M., Shepherd, G. M., Greer, C. A., and Charpak, S. (2009). Odor-evoked oxygen consumption by action potential and synaptic transmission in the olfactory bulb. J. Neurosci. 29, 1424–1433. doi: 10.1523/JNEUROSCI.4817-08.2009
Lee, H. S., Ghetti, A., Pinto-Duarte, A., Wang, X., Dziewczapolski, G., Galimi, F., et al. (2014). Astrocytes contribute to gamma oscillations and recognition memory. Proc. Natl. Acad. Sci. U S A 111, E3343–E3352. doi: 10.1073/pnas.1410893111
Lehmann, D., Faber, P. L., Achermann, P., Jeanmonod, D., Gianotti, L. R., and Pizzagalli, D. (2001). Brain sources of EEG gamma frequency during volitionally meditation-induced, altered states of consciousness and experience of the self. Psychiatry Res. 108, 111–121. doi: 10.1016/s0925-4927(01)00116-0
Lisman, J., and Buzsáki, G. (2008). A neural coding scheme formed by the combined function of gamma and theta oscillations. Schizophr. Bull. 34, 974–980. doi: 10.1093/schbul/sbn060
Lothman, E. W., and Somjen, G. G. (1975). Extracellular potassium activity, intracellular and extracellular potential responses in the spinal cord. J. Physiol. 252, 115–136. doi: 10.1113/jphysiol.1975.sp011137
LoTurco, J. L., Coulter, D. A., and Alkon, D. L. (1988). Enhancement of synaptic potentials in rabbit CA1 pyramidal neurons following classical conditioning. Proc. Natl. Acad. Sci. U S A 85, 1672–1676. doi: 10.1073/pnas.85.5.1672
Lutz, A., Greischar, L. L., Rawlings, N. B., Ricard, M., and Davidson, R. J. (2004). Long-term meditators self-induce high-amplitude gamma synchrony during mental practice. Proc. Natl. Acad. Sci. U S A 101, 16369–16373. doi: 10.1073/pnas.0407401101
Lux, H. D. (1974). Fast recording ion specific microelectrodes: their use in pharmacological studies in the CNS. Neuropharmacology 13, 509–517. doi: 10.1016/0028-3908(74)90140-3
Lux, H. D., Heinemann, U., and Dietzel, I. (1986). Ionic changes and alterations in the size of the extracellular space during epileptic activity. Adv. Neurol. 44, 619–639.
Madison, D. V., and Nicoll, R. A. (1984). Control of the repetitive discharge of rat CA 1 pyramidal neurones in vitro. J. Physiol. 354, 319–331. doi: 10.1113/jphysiol.1984.sp015378
Maier, N., Nimmrich, V., and Draguhn, A. (2003). Cellular and network mechanisms underlying spontaneous sharp wave-ripple complexes in mouse hippocampal slices. J. Physiol. 550, 873–887. doi: 10.1113/jphysiol.2003.044602
Malenka, R. C., Kauer, J. A., Perkel, D. J., Mauk, M. D., Kelly, P. T., Nicoll, R. A., et al. (1989). An essential role for postsynaptic calmodulin and protein kinase activity in long-term potentiation. Nature 340, 554–557. doi: 10.1038/340554a0
Mann, E. O., and Paulsen, O. (2007). Role of GABAergic inhibition in hippocampal network oscillations. Trends Neurosci. 30, 343–349. doi: 10.1016/j.tins.2007.05.003
Martin, C., Martindale, J., Berwick, J., and Mayhew, J. (2006). Investigating neural-hemodynamic coupling and the hemodynamic response function in the awake rat. Neuroimage 32, 33–48. doi: 10.1016/j.neuroimage.2006.02.021
Martina, M., and Jonas, P. (1997). Functional differences in Na+ channel gating between fast-spiking interneurones and principal neurones of rat hippocampus. J. Physiol. 505, 593–603. doi: 10.1111/j.1469-7793.1997.593ba.x
Melloni, L., Molina, C., Pena, M., Torres, D., Singer, W., and Rodriguez, E. (2007). Synchronization of neural activity across cortical areas correlates with conscious perception. J. Neurosci. 27, 2858–2865. doi: 10.1523/jneurosci.4623-06.2007
Miles, R., and Wong, R. K. S. (1987). Latent synaptic pathways revealed after tetanic stimulation in the hippocampus. Nature 329, 724–726. doi: 10.1038/329724a0
Montgomery, S. M., and Buzsáki, G. (2007). Gamma oscillations dynamically couple hippocampal CA3 and CA1 regions during memory task performance. Proc. Natl. Acad. Sci. U S A 104, 14495–14500. doi: 10.1073/pnas.0701826104
Morán, M., Moreno-Lastres, D., Marín-Buera, L., Arenas, J., Martín, M. A., and Ugalde, C. (2012). Mitochondrial respiratory chain dysfunction: implications in neurodegeneration. Free Radic. Biol. Med. 53, 595–609. doi: 10.1016/j.freeradbiomed.2012.05.009
Murthy, V. N., and Fetz, E. E. (1992). Coherent 25- to 35-Hz oscillations in the sensorimotor cortex of awake behaving monkeys. Proc. Natl. Acad. Sci. U S A 89, 5670–5674. doi: 10.1073/pnas.89.12.5670
Nelson, M. J., and Pouget, P. (2010). Do electrode properties create a problem in interpreting local field potential recordings? J. Neurophysiol. 103, 2315–2317. doi: 10.1152/jn.00157.2010
Nicholls, D. G. (2008). Oxidative stress and energy crises in neuronal dysfunction. Ann. N Y Acad. Sci. 1147, 53–60. doi: 10.1196/annals.1427.002
Niessing, J., Ebisch, B., Schmidt, K. E., Niessing, M., Singer, W., and Galuske, R. A. W. (2005). Hemodynamic signals correlate tightly with synchronized gamma oscillations. Science 309, 948–951. doi: 10.1126/science.1110948
Nishida, M., Juhász, C., Sood, S., Chugani, H. T., and Asano, E. (2008). Cortical glucose metabolism positively correlates with gamma-oscillations in nonlesional focal epilepsy. Neuroimage 42, 1275–1284. doi: 10.1016/j.neuroimage.2008.06.027
Oren, I., Hájos, N., and Paulsen, O. (2010). Identification of the current generator underlying cholinergically induced gamma frequency field potential oscillations in the hippocampal CA3 region. J. Physiol. 588, 785–797. doi: 10.1113/jphysiol.2009.180851
Oren, I., Mann, E. O., Paulsen, O., and Hájos, N. (2006). Synaptic currents in anatomically identified CA3 neurons during hippocampal gamma oscillations in vitro. J. Neurosci. 26, 9923–9934. doi: 10.1523/jneurosci.1580-06.2006
Pantev, C., Makeig, S., Hoke, M., Galambos, R., Hampson, S., and Gallen, C. (1991). Human auditory evoked gamma-band magnetic fields. Proc. Natl. Acad. Sci. U S A 88, 8996–9000. doi: 10.1073/pnas.88.20.8996
Papageorgiou, I. E., Lewen, A., Galow, L. V., Cesetti, T., Scheffel, J., Regen, T., et al. (2016). TLR4-activated microglia require IFN-γ to induce severe neuronal dysfunction and death in situ. Proc. Natl. Acad. Sci. U S A 113, 212–217. doi: 10.1073/pnas.1513853113
Pathak, R. U., and Davey, G. P. (2008). Complex I and energy thresholds in the brain. Biochim. Biophys. Acta 1777, 777–882. doi: 10.1016/j.bbabio.2008.05.443
Paulsen, O., and Moser, E. I. (1998). A model of hippocampal memory encoding and retrieval: GABAergic control of synaptic plasticity. Trends Neurosci. 21, 273–278. doi: 10.1016/s0166-2236(97)01205-8
Penttonen, M., Kamondi, A., Acsády, L., and Buzsáki, G. (1998). Gamma frequency oscillation in the hippocampus of the rat: intracellular analysis in vivo. Eur. J. Neurosci. 10, 718–728. doi: 10.1046/j.1460-9568.1998.00096.x
Phelps, M. E., Kuhl, D. E., and Mazziota, J. C. (1981). Metabolic mapping of the brain’s response to visual stimulation: studies in humans. Science 211, 1445–1448. doi: 10.1126/science.6970412
Pietersen, A. N., Lancaster, D. M., Patel, N., Hamilton, J. B., and Vreugdenhil, M. (2009). Modulation of gamma oscillations by endogenous adenosine through A1 and A2A receptors in the mouse hippocampus. Neuropharmacology 56, 481–492. doi: 10.1016/j.neuropharm.2008.10.001
Popa, D., Spolidoro, M., Proville, R. D., Guyon, N., Belliveau, L., and Léna, C. (2013). Functional role of the cerebellum in gamma-band synchronization of the sensory and motor cortices. J. Neurosci. 33, 6552–6556. doi: 10.1523/JNEUROSCI.5521-12.2013
Pöschel, B., Draguhn, A., and Heinemann, U. (2002). Glutamate-induced gamma oscillations in the dentate gyrus of rat hippocampal slices. Brain Res. 938, 22–28. doi: 10.1016/s0006-8993(02)02477-0
Prince, D. A., Lux, H. D., and Neher, E. (1973). Measurement of extracellular potassium activity in cat cortex. Brain Res. 50, 489–495. doi: 10.1016/0006-8993(73)90758-0
Revsbech, N. P. (1989). An oxygen microsensor with a guard cathode. Limnol. Oceanogr. 34, 474–478. doi: 10.4319/lo.1989.34.2.0474
Rolfe, D. F., and Brown, G. C. (1997). Cellular energy utilization and molecular origin of standard metabolic rate in mammals. Physiol. Rev. 77, 731–758.
Roth, F. C., and Draguhn, A. (2012). GABA metabolism and transport: effects on synaptic efficacy. Neural Plast. 2012:805830. doi: 10.1155/2012/805830
Salkoff, D. B., Zagha, E., Yüzgeç, Ö., and McCormick, D. A. (2015). Synaptic mechanisms of tight spike synchrony at gamma frequency in cerebral cortex. J. Neurosci. 35, 10236–10251. doi: 10.1523/JNEUROSCI.0828-15.2015
Scheeringa, R., Fries, P., Petersson, K. M., Oostenveld, R., Grothe, I., Norris, D. G., et al. (2011). Neuronal dynamics underlying high- and low-frequency EEG oscillations contribute independently to the human BOLD signal. Neuron 69, 572–583. doi: 10.1016/j.neuron.2010.11.044
Schneider, J., Lewen, A., Ta, T. T., Galow, L. V., Isola, R., Papageorgiou, I. E., et al. (2015). A reliable model for gamma oscillations in hippocampal tissue. J. Neurosci. Res. 93, 1067–1078. doi: 10.1002/jnr.23590
Schölvinck, M. L., Maier, A., Ye, F. Q., Duyn, J. H., and Leopold, D. A. (2010). Neural basis of global resting-state fMRI activity. Proc. Natl. Acad. Sci. U S A 107, 10238–10243. doi: 10.1073/pnas.0913110107
Schönberger, J., Draguhn, A., and Both, M. (2014). Lamina-specific contribution of glutamatergic and GABAergic potentials to hippocampal sharp wave-ripple complexes. Front. Neural Circuits 8:103. doi: 10.3389/fncir.2014.00103
Schroeder, C. E., and Lakatos, P. (2009). Low-frequency neuronal oscillations as instruments of sensory selection. Trends Neurosci. 32, 9–18. doi: 10.1016/j.tins.2008.09.012
Schuchmann, S., Kovacs, R., Kann, O., Heinemann, U., and Buchheim, K. (2001). Monitoring NAD(P)H autofluorescence to assess mitochondrial metabolic functions in rat hippocampal-entorhinal cortex slices. Brain Res. Brain Res. Protoc. 7, 267–276. doi: 10.1016/s1385-299x(01)00080-0
Schurr, A., and Payne, R. S. (2007). Lactate, not pyruvate, is neuronal aerobic glycolysis end product: an in vitro electrophysiological study. Neuroscience 147, 613–619. doi: 10.1016/j.neuroscience.2007.05.002
Schurr, A., West, C. A., and Rigor, B. M. (1988). Lactate-supported synaptic function in the rat hippocampal slice preparation. Science 240, 1326–1328. doi: 10.1126/science.3375817
Sheth, S. A., Nemoto, M., Guiou, M., Walker, M., Pouratian, N., and Toga, A. W. (2004). Linear and nonlinear relationships between neuronal activity, oxygen metabolism and hemodynamic responses. Neuron 42, 347–355. doi: 10.1016/s0896-6273(04)00221-1
Shuttleworth, C. W., Brennan, A. M., and Connor, J. A. (2003). NAD(P)H fluorescence imaging of postsynaptic neuronal activation in murine hippocampal slices. J. Neurosci. 23, 3196–3208.
Sik, A., Tamamaki, N., and Freund, T. F. (1993). Complete axon arborization of a single CA3 pyramidal cell in the rat hippocampus and its relationship with postsynaptic parvalbumin-containing interneurons. Eur. J. Neurosci. 5, 1719–1728. doi: 10.1111/j.1460-9568.1993.tb00239.x
Singer, W., and Lux, H. D. (1975). Extracellular potassium gradients and visual receptive fields in the cat striate cortex. Brain Res. 96, 378–383. doi: 10.1016/0006-8993(75)90751-9
Sohal, V. S., Zhang, F., Yizhar, O., and Deisseroth, K. (2009). Parvalbumin neurons and gamma rhythms enhance cortical circuit performance. Nature 459, 698–702. doi: 10.1038/nature07991
Somjen, G. G. (2002). Ion regulation in the brain: implications for pathophysiology. Neuroscientist 8, 254–267. doi: 10.1177/1073858402008003011
Steinhäuser, C., Seifert, G., and Bedner, P. (2012). Astrocyte dysfunction in temporal lobe epilepsy: K+ channels and gap junction coupling. Glia 60, 1192–1202. doi: 10.1002/glia.22313
Studer, D., Zhao, S., Chai, X., Jonas, P., Graber, W., Nestel, S., et al. (2014). Capture of activity-induced ultrastructural changes at synapses by high-pressure freezing of brain tissue. Nat. Protoc. 9, 1480–1495. doi: 10.1038/nprot.2014.099
Sumiyoshi, A., Suzuki, H., Ogawa, T., Riera, J. J., Shimokawa, H., and Kawashima, R. (2012). Coupling between gamma oscillation and fMRI signal in the rat somatosensory cortex: its dependence on systemic physiological parameters. Neuroimage 60, 738–746. doi: 10.1016/j.neuroimage.2011.12.082
Takács, V. T., Szönyi, A., Freund, T. F., Nyiri, G., and Gulyás, A. I. (2015). Quantitative ultrastructural analysis of basket and axo-axonic cell terminals in the mouse hippocampus. Brain Struct. Funct. 220, 919–940. doi: 10.1007/s00429-013-0692-6
Thomsen, K., Piilgaard, H., Gjedde, A., Bonvento, G., and Lauritzen, M. (2009). Principal cell spiking, postsynaptic excitation and oxygen consumption in the rat cerebellar cortex. J. Neurophysiol. 102, 1503–1512. doi: 10.1152/jn.00289.2009
Traub, R. D., Kopell, N., Bibbig, A., Buhl, E. H., LeBeau, F. E. N., and Whittington, M. A. (2001). Gap junctions between interneuron dendrites can enhance synchrony of gamma oscillations in distributed networks. J. Neurosci. 21, 9478–9486.
Traub, R. D., and Whittington, M. A. (2010). Cortical Oscillations in Health and Disease. New York, NY: Oxford University Press.
Tsintsadze, V., Minlebaev, M., Suchkov, D., Cunningham, M. O., and Khazipov, R. (2015). Ontogeny of kainate-induced gamma oscillations in the rat CA3 hippocampus in vitro. Front. Cell. Neurosci. 9:195. doi: 10.3389/fncel.2015.00195
Uhlhaas, P. J., and Singer, W. (2010). Abnormal neural oscillations and synchrony in schizophrenia. Nat. Rev. Neurosci. 11, 100–113. doi: 10.1038/nrn2774
van Vugt, M. K., Schulze-Bonhage, A., Litt, B., Brandt, A., and Kahana, M. J. (2010). Hippocampal gamma oscillations increase with memory load. J. Neurosci. 30, 2694–2699. doi: 10.1523/JNEUROSCI.0567-09.2010
Viswanathan, A., and Freeman, R. D. (2007). Neurometabolic coupling in cerebral cortex reflects synaptic more than spiking activity. Nat. Neurosci. 10, 1308–1312. doi: 10.1038/nn1977
Watrous, A. J., Fell, J., Ekstrom, A. D., and Axmacher, N. (2015). More than spikes: common oscillatory mechanisms for content specific neural representations during perception and memory. Curr. Opin. Neurobiol. 31, 33–39. doi: 10.1016/j.conb.2014.07.024
Whittaker, R. G., Turnbull, D. M., Whittington, M. A., and Cunningham, M. O. (2011). Impaired mitochondrial function abolishes gamma oscillations in the hippocampus through an effect on fast-spiking interneurons. Brain 134:e180. doi: 10.1093/brain/awr018
Whittington, M. A., Traub, R. D., Faulkner, H. J., Stanford, I. M., and Jefferys, J. G. R. (1997). Recurrent excitatory postsynaptic potentials induced by synchronized fast cortical oscillations. Proc. Natl. Acad. Sci. U S A 94, 12198–12203. doi: 10.1073/pnas.94.22.12198
Whittington, M. A., Traub, R. D., and Jefferys, J. G. R. (1995). Synchronized oscillations in interneuron networks driven by metabotropic glutamate receptor activation. Nature 373, 612–615. doi: 10.1038/373612a0
Wirtz, S., and Schuelke, M. (2011). Region-specific expression of mitochondrial complex I genes during murine brain development. PLoS One 6:e18897. doi: 10.1371/journal.pone.0018897
Wong, R. K. S., and Prince, D. A. (1981). Afterpotential generation in hippocampal pyramidal cells. J. Neurophysiol. 45, 86–97.
Wong-Riley, M. T. T. (1989). Cytochrome oxidase: an endogenous metabolic marker for neuronal activity. Trends Neurosci. 12, 94–101. doi: 10.1016/0166-2236(89)90165-3
Zhang, Z. G., Hu, L., Hung, Y. S., Mouraux, A., and Iannetti, G. D. (2012). Gamma-band oscillations in the primary somatosensory cortex - a direct and obligatory correlate of subjective pain intensity. J. Neurosci. 32, 7429–7438. doi: 10.1523/JNEUROSCI.5877-11.2012
Zhao, S., Studer, D., Chai, X., Graber, W., Brose, N., Nestel, S., et al. (2012). Structural plasticity of hippocampal mossy fiber synapses as revealed by high-pressure freezing. J. Comp. Neurol. 520, 2340–2351. doi: 10.1002/cne.23040
Keywords: cognition, extracellular potassium concentration, GABA-A receptor, membrane ion transport, mitochondria, Na+/K+-ATPase, neural information processing, tissue oxygen tension
Citation: Kann O, Hollnagel J-O, Elzoheiry S and Schneider J (2016) Energy and Potassium Ion Homeostasis during Gamma Oscillations. Front. Mol. Neurosci. 9:47. doi: 10.3389/fnmol.2016.00047
Received: 18 February 2016; Accepted: 30 May 2016;
Published: 16 June 2016.
Edited by:
Jochen C. Meier, Technical University Braunschweig, GermanyReviewed by:
Jakob Wolfart, University of Rostock, GermanyRüdiger Köhling, Rostock University Medical Center, Germany
Copyright © 2016 Kann, Hollnagel, Elzoheiry and Schneider. This is an open-access article distributed under the terms of the Creative Commons Attribution License (CC BY). The use, distribution and reproduction in other forums is permitted, provided the original author(s) or licensor are credited and that the original publication in this journal is cited, in accordance with accepted academic practice. No use, distribution or reproduction is permitted which does not comply with these terms.
*Correspondence: Oliver Kann, b2xpdmVyLmthbm5AcGh5c2lvbG9naWUudW5pLWhlaWRlbGJlcmcuZGU=