- 1Laboratory of Neural Differentiation, Institute of Neuroscience, Université catholique de Louvain, Brussels, Belgium
- 2Department of Biology, University of Victoria, Victoria, BC, Canada
- 3The Cyprus Institute of Neurology and Genetics, Nicosia, Cyprus
- 4Center for Advanced Biotechnology and Medicine and Department of Pediatrics, Rutgers University - Robert Wood Johnson Medical School, Piscataway, NJ, USA
- 5Inserm U 1127, CNRS UMR 7225, Sorbonne Universités, UPMC University Paris 06 UMR S 1127, Institut du Cerveau et de la Moelle épinière (ICM), Paris, France
- 6Laboratory of Cell Physiology, Institute of Neuroscience, Université catholique de Louvain, Brussels, Belgium
- 7State Key Laboratory of Ophthalmology, Zhongshan Ophthalmic Center, Sun Yat-sen University, Guangzhou, China
Spinal ventral interneurons regulate the activity of motor neurons, thereby controlling motor activities. Interneurons arise during embryonic development from distinct progenitor domains distributed orderly along the dorso-ventral axis of the neural tube. A single ventral progenitor population named p2 generates at least five V2 interneuron subsets. Whether the diversification of V2 precursors into multiple subsets occurs within the p2 progenitor domain or involves a later compartment of early-born V2 interneurons remains unsolved. Here, we provide evidence that the p2 domain produces an intermediate V2 precursor compartment characterized by the transient expression of the transcriptional repressor Vsx1. These cells display an original repertoire of cellular markers distinct from that of any V2 interneuron population. They have exited the cell cycle but have not initiated neuronal differentiation. They coexpress Vsx1 and Foxn4, suggesting that they can generate the known V2 interneuron populations as well as possible additional V2 subsets. Unlike V2 interneurons, the generation of Vsx1-positive precursors does not depend on the Notch signaling pathway but expression of Vsx1 in these cells requires Pax6. Hence, the p2 progenitor domain generates an intermediate V2 precursor compartment, characterized by the presence of the transcriptional repressor Vsx1, that contributes to V2 interneuron development.
Introduction
During CNS development, the generation of numerous neuronal populations distinct in their functional properties, location and connectivity is a prerequisite for building circuitries that regulate complex behaviors like locomotion. In vertebrates, locomotor activity is controlled by multiple regions of the CNS but is eventually triggered by local neuronal circuits that are established in the ventral spinal cord. These circuits are composed of motor neurons and of several types of interneurons that regulate motor neuron activity (Goulding, 2009; Grillner and Jessell, 2009; Garcia-Campmany et al., 2010; Grossmann et al., 2010; Lu et al., 2015). Although cardinal populations of ventral interneurons have been thoroughly described, the molecular mechanisms that regulate the generation and the diversification of these cells remain only partly elucidated.
Upon activation of unique combinations of transcription factors in response to morphogen gradients, distinct ventral interneuron progenitor domains called p0 to p3 are defined in the developing spinal cord. These progenitor domains generate four cardinal classes of ventral interneurons termed V0 to V3 (Grillner and Jessell, 2009; Garcia-Campmany et al., 2010; Lu et al., 2015). Interestingly, these cardinal populations further diversify into numerous subsets characterized by distinct combinations of molecular markers, use of different neurotransmitters, specific projection patterns and differential contributions to the locomotor circuits (Moran-Rivard et al., 2001; Pierani et al., 2001; Karunaratne et al., 2002; Sapir et al., 2004; Al-Mosawie et al., 2007; Crone et al., 2008; Zhang et al., 2008; Zagoraiou et al., 2009; Panayi et al., 2010; Dougherty et al., 2013; Francius et al., 2013; Panayiotou et al., 2013; Azim et al., 2014; Zhang et al., 2014; Britz et al., 2015). For example, V2 interneurons eventually subdivide into at least five different subtypes including V2a, V2b, V2c, V2d and Pax6+V2 interneurons (Zhou et al., 2000; Karunaratne et al., 2002; Al-Mosawie et al., 2007; Panayi et al., 2010; Dougherty et al., 2013; Panayiotou et al., 2013). This diversification process enables ventral interneuron subpopulations to ensure different aspects of the stereotypic pattern of locomotor activity, including left-right alternation (V0 + V2a), flexor-extensor alternation (V1 + V2b), speed (V1) and robustness and rhythmicity (V2d + V3; Lanuza et al., 2004; Gosgnach et al., 2006; Crone et al., 2008; Zhang et al., 2008, 2014; Dougherty et al., 2013; Talpalar et al., 2013; Azim et al., 2014; Britz et al., 2015).
Within the p2 domain, segregation of the V2a vs. V2b lineages depends on differential activation of the Dll4/Notch pathway (Del Barrio et al., 2007; Peng et al., 2007; Joshi et al., 2009; Skaggs et al., 2011), which relies on the mosaic expression of several transcriptional regulators including Foxn4 and Ascl1 (Li et al., 2005; Del Barrio et al., 2007; Misra et al., 2014). However, it remains unclear whether activation of the Notch pathway, V2a/V2b segregation and generation of the other V2 interneuron subsets occur within the p2 progenitor domain or in a later cell compartment of early-born V2 interneurons. In the present study, we identify an early intermediate V2 precursor compartment characterized by the transient expression of the transcription factor Vsx1. Vsx1 is a transcriptional repressor of the Paired-like CVC (Prd-L:CVC) homeobox gene family (Chow et al., 2001; Ohtoshi et al., 2001). In the mouse, it is expressed in gastrula stage embryos (Ohtoshi et al., 2001) and in several bipolar cone interneurons of the retina where it regulates different aspects of their terminal differentiation (Chow et al., 2001, 2004; Ohtoshi et al., 2001, 2004; Kerschensteiner et al., 2008; Shi et al., 2011, 2012). Here we show that, in the mouse developing spinal cord, Vsx1-positive cells are transiently detected from e9.5 onward, are molecularly distinct from any V2 interneuron populations and generate all the V2 populations and possibly additional V2 subsets that remain to be characterized. Loss-of-function analyses indicate that Pax6 is required for the expression of Vsx1 in this cell compartment.
Materials and Methods
Mouse Strains
All experiments were performed in accordance with the European Community Council directive of 24 November 1986 (86-609/ECC) and the decree of 20 October 1987 (87-848/EEC). Mice were treated according to the principles of laboratory animal care, and experiments and mouse housing were approved by the Animal Welfare Committee of the Université catholique de Louvain (Permit Number: 2013/UCL/MD/11). The day of vaginal plug was considered as embryonic day (e)0.5. Minimum numbers of three embryos of the same genotype were analyzed in each experiment. The Ascl1+/−, Hnf6+/−; onecut 2(Oc2)+/−, Presenilin-1 (PS1)+/−, Pax6+/Sey and Vsx1+/τLacZ mutant mice were previously described (Hill et al., 1992; Guillemot et al., 1993; Wong et al., 1997; Jacquemin et al., 2000; Gong et al., 2003; Chow et al., 2004; Li et al., 2004; Clotman et al., 2005). Although β-galactosidase production was evident in a ventral population in Vsx1τLacZ/τLacZ spinal cords, it was barely detectable in Vsx1+/τLacZ heterozygous embryos, probably due to the negative auto-regulatory loop reported to control Vsx1 expression levels in the retina (Chow et al., 2004). Furthermore, β-galactosidase distribution was diffuse and punctuated, hindering the identification of the cells wherein it was present (data not shown). Therefore, a novel Vsx1+/nlsLacZ line was generated using the PG00233_Z_5_A10 allele developed by the Knock-Out Mouse Project (KOMP). Vsx1 inactivation was confirmed by genotyping PCR and by complete loss of the Vsx1 protein. However, β-galactosidase was never detected in this line (data not shown). Nevertheless, Vsx1nlsLacZ/nlsLacZ embryos were analyzed for the development of V2 interneuron populations.
Immunofluorescence Labelings
Mouse embryos were fixed in PBS/4% PFA at 4°C for 15–30 min according to the developmental stage. Fixed mouse embryos were washed in PBS before incubation in PBS/30% sucrose overnight at 4°C. They were embedded in PBS/7.5% gelatin/15% sucrose and frozen at −55°C. Embryos were cut at 14 μm in a Leica CM3050 cryostat.
Cryosections were saturated with PBS/0.1% Triton/10% horse serum for 30 min and incubated with the primary antibodies diluted in the same solution at 4°C overnight. For Vsx1 labeling, cryosections were permeabilized with PBS/1% Triton for 30 min at room temperature and saturated for 30 min with PBS/0.1% Triton/1% horse serum. Anti-Vsx1 antibody diluted in the same solution was incubated for 2 h at room temperature. After three washes in PBS/0.1% Triton, the secondary antibodies, diluted in PBS/0.1% Triton/10% horse serum, were added for 30 min at room temperature. Slides were washed three times in PBS/0.1% Triton before a final wash in PBS/DAPI, and were mounted with Fluorescent mounting medium (DAKO).
The following primary antibodies and dilution were used: mouse anti-Ascl1 at 1:200 (BD #556604), guinea-pig anti-Ascl1 at 1:10,000 (Kim et al., 2008), mouse anti-beta III tubulin at 1:5000 (Chemicon #MAB1637), goat anti-BhlhB5 at 1:1000 (Santa Cruz #sc-6045), rabbit or rat anti-BhlhB5 at 1:2000 (Ross et al., 2012), sheep anti-Chx10 (Vsx2) at 1:500 (Exalpha Biologicals #X1179P), goat anti Dll4 at 1:200 (R&D System #AF1389), rabbit or guinea pig anti-Foxd3 at 1:5000 (Müller et al., 2005), rat anti-Gata3 at 1: 20 (Panayi et al., 2010), guinea-pig anti-Insm1 at 1:10,000 (Welcker et al., 2013), mouse anti-Islet 1/2 at 1:6000 (DSHB #39.4D5), mouse anti-Lhx3 at 1:1000 (DSHB #67.4E12), mouse anti-Lhx1/5 at 1:2000 (DSHB # 4F2), rat anti-Nkx6.1 at 1:2 (Ono et al., 2007), guinea pig anti-OC-1 at 1:6000 (Espana and Clotman, 2012), sheep anti-OC-1 at 1:250 (R&D System #AF6277), rat anti-OC-2 at 1:400 (Clotman et al., 2005), mouse anti-p27kip1 at 1:2000 (BD), mouse anti-Pax6 at 1:1000 (DSHB #PAX6), mouse anti-phospho-Histone H3 at 1:1000 (Abcam ab14955), rabbit or guinea pig anti-Prdm8 at 1:1000 (Ross et al., 2012), goat anti-Prox1 at 1:100 (R&D Systems # AF2727), mouse anti-Shox2 at 1:500 (Abcam #ab55740), goat anti-Sox 1 at 1:500 (Santa Cruz #sc-17318), rabbit anti-Vsx1 at 1:500 (Clark et al., 2008).
Secondary antibodies from Life Science were used at 1:2000 and were donkey anti-mouse/AlexaFluor 594 or 488 or 647, anti-rabbit/AlexaFluor 647, anti-rat/AlexaFluor 594, anti-goat/AlexaFluor 488, anti-sheep/AlexaFluor 594, anti-mouse IgG1/AlexaFluor 594 or anti-mouse IgG2a/AlexaFluor 488. Secondary antibodies from Jackson ImmunoResearch were used at 1:1000 and were donkey-anti chicken/Dylight 488 or 594, anti-mouse/AlexaFluor 647, anti-rat/AlexaFluor 647, anti-sheep/AlexaFluor 594 or 647, anti-rabbit/AlexaFluor 488 or 594, anti-guinea pig/AlexaFluor 488 or 647. All secondary antibodies gave signals in the spinal cord only in the presence of corresponding primary antibodies.
Double in situ Hybridization (ISH)
Mouse embryos were fixed overnight in PBS/4% PFA at 4°C and processed as for immunolabeling experiments. Fourteen micrometer cryostat sections were cut and double in situ hybridization (ISH) protocol was performed essentially as previously described with slight modifications (Beguin et al., 2013; Pelosi et al., 2014). Sections were simultaneously hybridized overnight at 65°C with a DIG-conjugated Foxn4 (NM_148935.2, nucleotides 78–1643 (Francius et al., 2015)) and a fluorescein-labeled Vsx1 (NM_054068.2, nucleotides 121–2728, provided by C. Cepko) riboprobe. After hybridization, sections were washed four times in 50% Formamide, 1× SSC, 0.1% Tween-20 for 1 h at 65°C, twice in MABT buffer (100 mM maleic acid, 150 mM NaCl, 0.1% Tween20, pH 7.5) for 30 min before blocking in blocking buffer (MABT, 2% blocking reagent from Roche, 20% inactivated horse serum) for 2 h at room temperature. Sections were then incubated overnight with anti-DIG-alkaline phosphatase (AP)-conjugate antibody (Roche) at 4°C. After washing for 30 min in MABT, Foxn4 probe was visualized by AP-catalyzed chromogenic reaction using NBT-BCIP substrates (Roche) according to manufacturer’s instructions. The color reaction was stopped in 1× PBS and AP was inactivated by incubating the slides for 30 min with 0.1 M glycine/HCl pH 2.2. The sections were then washed twice in MABT buffer for 30 min, blocked again in blocking buffer for 2 h, and incubated to a 1:2000 dilution of anti-Fluo-AP-conjugate antibody (Roche) in blocking buffer overnight at 4°C. Slides were washed and incubated with HNPP/Fast Red kit (Roche) according to the manufacturer’s instructions to visualize Vsx1 expression. The reaction was stopped by washing in 1× PBS. Sections were then counterstained with DAPI and slides were mounted with Fluorescent mounting medium (DAKO).
Imaging and Quantitative Analyses
Immunofluorescence and double ISH images of cryosections at thoracic level of the developing spinal cord were acquired on a Zeiss Axio Cell Observer Z1 confocal microscope with the Zeiss AxioVision Rel. 4.8 software and processed with Adobe Photoshop CS5 software. Double ISH images were also acquired using an EVOS® FL Auto Imaging System (ThermoFisher Scientific) and related software. Quantifications were performed on red or green or blue layer of acquired confocal images and double or triple labeling cells were processed by subtractive method (Francius and Clotman, 2010). For each embryo (n ≥ 3), both sides of three sections at thoracic level were quantified using the count analysis tool of Adobe Photoshop CS5 software.
Raw data were exported from Adobe Photoshop CS5 software to SigmaPlot v12.3 software and processed in order to generate histogram figures. All data were analyzed and histograms were made with SigmaPlot software. Adequate statistical tests were applied based on the number of comparisons and on the variance in each group. For analysis of cell quantification based on comparison of two groups (control or mutant), standard Student’s t-tests were performed. Quantitative analyses were considered significant at p < 0.05. Three asterisks (***) indicate p < 0.001.
Results
The p2 Progenitor Domain Generates an Early V2 Population
In mouse embryonic spinal cord, neurogenesis starts around e9.5. The earliest differentiating neurons are motor neurons, which originate from the pMN progenitor domain. We recently described that the production of V1 interneurons is also initiated at e9.5 (Stam et al., 2012). At this early stage, the presence of the OC transcription factors, namely OC-1 (or HNF-6), OC-2 and OC-3, was restricted to differentiating neurons and was observed in motor neurons and V1 interneurons (Francius and Clotman, 2010; Roy et al., 2012; Stam et al., 2012; Francius et al., 2013). Surprisingly, OC-1 was additionally detected in cells located between motor neurons and prospective Renshaw cells (Figures 1A–A″′; Stam et al., 2012). As the p2 progenitors are located between the pMN and the p1 domains, we postulated that these unidentified cells were most likely early-born V2 cells containing OC factors. To test this hypothesis we assessed the distribution of Ascl1, which is specifically detected in the p2 domain and early V2 interneurons (Gong et al., 2003; Wildner et al., 2006; Parras et al., 2007). Co-detection of OC-1 and Ascl1 confirmed that early-born OC-1+ cells derived from the p2 domain (Figures 1B–B″′). Given that OC factors are detected only in post-mitotic neurons (Francius and Clotman, 2010), we concluded that these cells were early V2 interneurons. However, V2 interneurons are reported to be generated from e10.5 onward (Zhou et al., 2000; Li et al., 2005; Peng et al., 2007). Accordingly, the number of V2a or V2b identified by the presence of Chx10 or Gata3, respectively, was restricted at e9.5 (1–5 cells per hemisection; Figures 1C–C″,F–F″, 2C) but significantly increased from e10.5 on (Figures 2A,C; Nardelli et al., 1999; Zhou et al., 2000; Smith et al., 2002). At e9.5, OC-1 was present in V2a and V2b interneurons, as well as in other cells located lateral to the p2 progenitor domain (Figures 1C–C″). Taken together, these observations suggested either that OC-1 was detected in early V2a/V2b interneurons before the onset of Chx10/Gata3 expression or that OC-1 was present in an additional unknown V2 subset. The latter hypothesis was consistent with results from a genetic lineage-tracing of Foxn4+ progenitors, which indicated that the p2 domain produces additional unidentified V2 interneurons (Li et al., 2010).
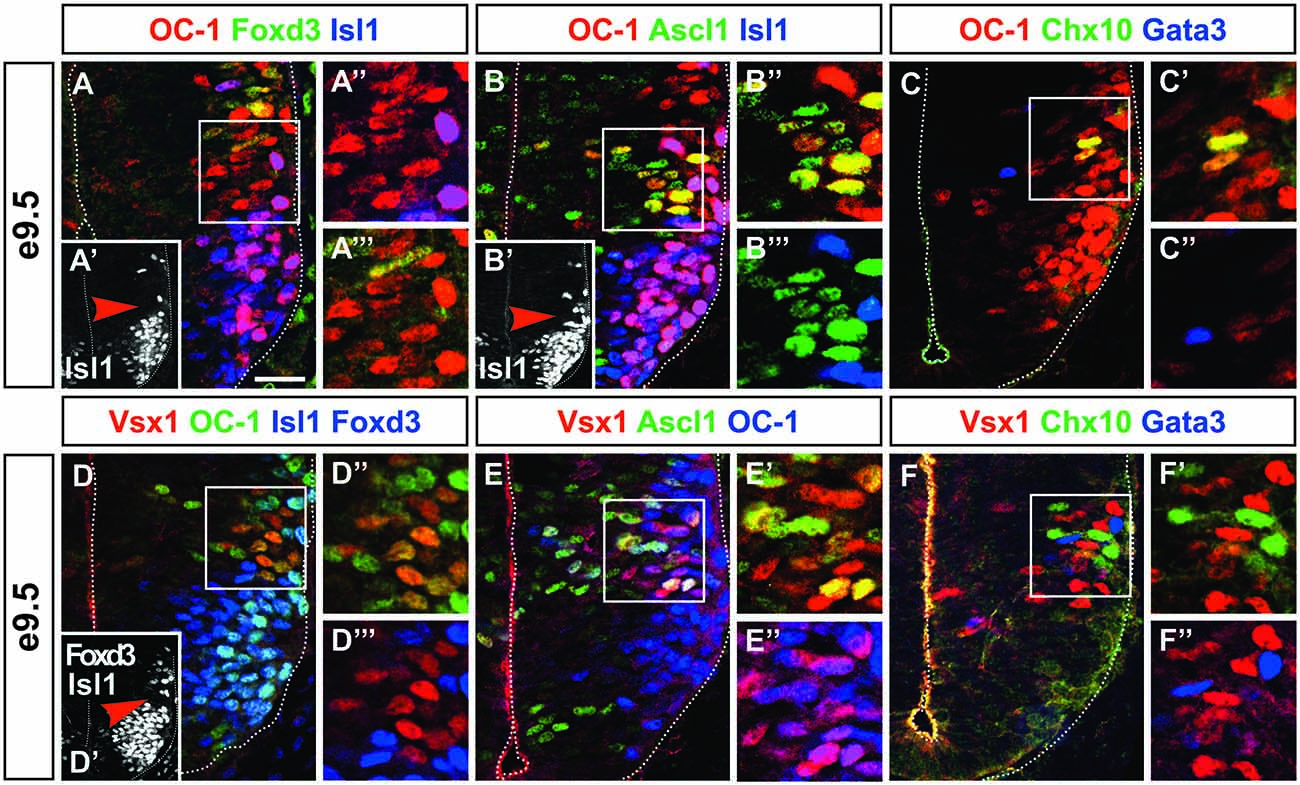
Figure 1. Onecut (OC)-1 and Vsx1 are present in an early V2 interneuron population. (A–A″′) At e9.5, OC-1 is detected in cells located between motor neurons (Isl1+, inset A′; arrowhead indicates the location of A″ and A″′ insets) and V1 interneurons (Foxd3+). (B–B″′) These cells derive from the p2 progenitor domain (Ascl1+) and are not motor neurons (Isl1+, inset B′; arrowhead indicates the location of B″ and B″′ insets). (C–C″) OC-1 is detected in the V2 domain in cells that are neither V2a (Chx10+) nor V2b (Gata3+) interneurons. (D–D″′) Vsx1 is detected in cells containing OC-1 located between motor neurons (Isl1+, inset D′; arrowhead indicates the location of D″ and D″′ insets) and V1 interneurons (Foxd3+). (E–E″) Vsx1 is present in cells that derive from the p2 domain (Ascl1+) and contain OC-1. (F–F″) Vsx1+ cells are distinct from early V2a (Chx10+) and V2b (Gata3+) interneurons. Scale bar = 50 μm.
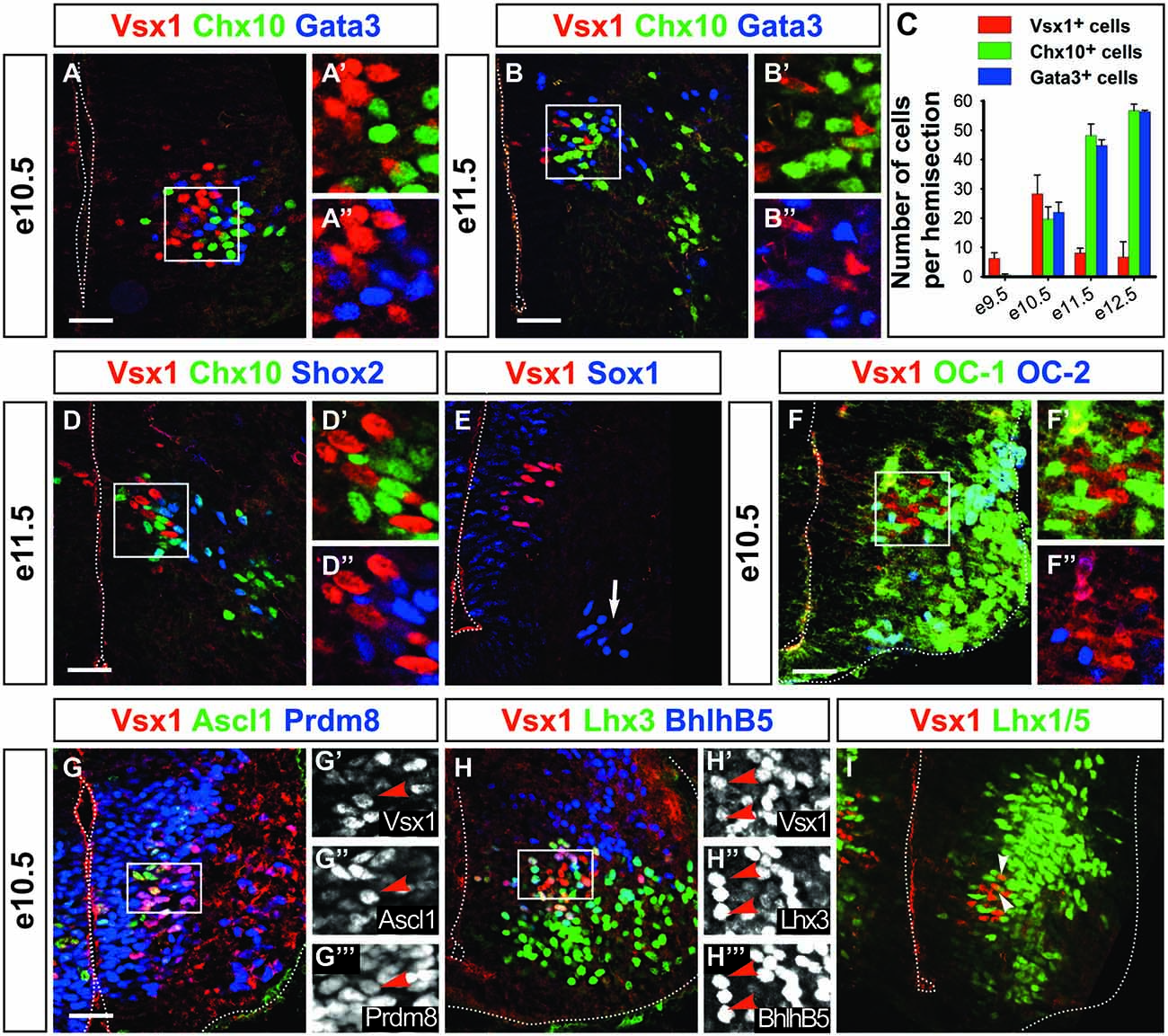
Figure 2. Vsx1 identifies a population distinct from V2 interneurons. At e10.5 (A–A″) and e11.5 (B–B″), Vsx1 is present in cells that intermingle with, but are distinct from V2a (Chx10+) and V2b (Gata3+) interneurons. (C) Quantification of V2a, V2b and Vsx1+ cells at different stages (mean values ± SEM, n = 3). (D–D″) Vsx1 is not detected in V2d interneurons (Shox2 + Chx10−). (E) Vsx1+ cells are also distinct from V2c cells (Sox1+, arrow). (F–F″) At e10.5, OC-1 is present at low levels in Vsx1+ cells, while OC-2 is undetectable. (G–G″′) Ascl1 is present in Vsx1+ cells, confirming their p2 origin. Proteins present in V2a, including Prdm8 (G–G″′), Lhx3 and Bhlhb5 (H–H″′), are also detected. (I) In contrast, Lhx1/5, which is found in V2b, is barely detectable (arrowheads). Scale bars = 50 μm.
To distinguish between these possibilities, we searched for specific markers of these cells. Several transcription factors present in V2 interneurons are also detected in differentiating neurons of the retina (Glaser et al., 1992; Liu et al., 1994; Tomita et al., 1996; Gouge et al., 2001). Thus, specific markers of this V2 subset may be found among retinal proteins that are not yet associated with a specific spinal population. Vsx1 is the single paralog of the V2a marker Chx10 in the mouse genome and is expressed as Chx10 in the developing retina (Chow et al., 2001; Ohtoshi et al., 2001). Interestingly, Vsx1 expression in retinal cells is inhibited by Chx10 (Clark et al., 2008) and Chx10 expression is repressed by Vsx1 in Type 7 cone bipolar cells (Shi et al., 2011). In the zebrafish embryonic spinal cord, the Vsx1 ortholog is detected in common V2a/V2b progenitors and is transiently maintained in the V2a lineage (Batista et al., 2008; Kimura et al., 2008). In Xenopus and chick, Vsx1 expression was attributed to V2a interneurons based on its distribution similar to Chx10 (Chen and Cepko, 2000; D’Autilia et al., 2006). However, co-labeling of Vsx1 and other V2 markers in the mouse embryonic spinal cord was not reported yet.
Therefore, we characterized the distribution of Vsx1 in the mouse developing spinal cord. First, we assessed whether Vsx1 is present in the early OC-1+ V2 cells identified at e9.5. At this stage, Vsx1 was detected in cells containing OC-1 that were neither motor neurons nor early V1 interneurons (Figures 1D–D″′). As observed for OC-1 (Figures 1B–B″′), these cells contained Ascl1, confirming that they derived from the p2 domain (Figures 1E–E″). To determine whether these may correspond to V2a or V2b interneurons, the distribution of Vsx1 was compared to that of Chx10 and Gata3. However, Vsx1 was detected in cells lacking Chx10 or Gata3 (Figures 1F–F″), leaving open the question whether they corresponded to early V2 precursors or to a distinct population of V2 interneurons.
Vsx1 Defines a Specific V2 Subset
To further investigate this question, we compared the distribution of Vsx1 and V2 markers at later developmental stages (Figures 2A–E). We first noticed that the number of Vsx1+ cells increased from e9.5 to e10.5, then decreased to e12.5 and became undetectable at later stages (Figures 2A–C and data not shown), indicating a transient expression of Vsx1 in the mouse spinal cord as observed in zebrafish embryos (Passini et al., 1998). At all developmental stages, Vsx1 was found in cells located in close vicinity to V2a and V2b interneurons. However, co-detection of Vsx1 with Chx10 or Gata3 was never observed (Figures 2A–B″). Vsx1 was also present at e11.5 in cells located nearby V2d interneurons, characterized by the presence of Shox2 in the absence of Chx10 (Dougherty et al., 2013), but Shox2 was not detected in Vsx1+ cells (Figures 2D–D″). Finally, Vsx1 distribution was compared to that of Sox1, which labels V2c interneurons in addition to spinal progenitors (Panayi et al., 2010). However, V2c are located more ventrally and were distinct from cells containing Vsx1 (Figure 2E, arrow). Hence, Vsx1 is a specific, albeit transient, marker of a novel cell subset distinct from described V2 populations.
Spinal neurons that share lineage relationship or developmental origin often exhibit common markers. To assess whether Vsx1+ cells might relate to any V2 population, we established a repertoire of markers detected in these cells. As OC-1 was detected in Vsx1+ cells at early developmental stages (Figures 1D–D″), we assessed whether OC factors were co-detected with Vsx1 in more differentiated cells. At e10.5, OC-1 levels decreased sharply but the protein was still detected in 66% of the Vsx1+ cells (Figures 2F–F″), while OC-2 (Figures 2F–F″) and OC-3 (data not shown) were absent. As observed at e9.5, Ascl1 was present at e10.5 in the Vsx1+ cells, confirming their p2 origin (Figures 2G–G″′). Lhx3 is present in pMN and in p2 progenitors and is maintained in the medial motor column and in V2a interneurons but not in other V2 subsets. Lhx3 was detected in 95% of the Vsx1+ cells (Figures 2H–H″′), suggesting that they might relate to V2a interneurons. Accordingly, Prdm8 and Bhlhb5, which are present in V2a interneurons (Skaggs et al., 2011; Francius et al., 2013), were detected in 97% and 87% of the Vsx1+ cells, respectively (Figures 2G–H″′). In contrast Lhx1/5, which is present in V2b but is excluded from V2a interneurons, was barely detectable in 7% of the Vsx1+ complement (Figure 2I, arrowheads). Thus, as previously reported in other species (Chen and Cepko, 2000; D’Autilia et al., 2006; Batista et al., 2008; Kimura et al., 2008), Vsx1+ cells share some characteristics with V2a interneurons but Vsx1 is not detected in known V2 populations.
Vsx1 Is Restricted to an Early V2 Compartment Prior to Neuronal Differentiation
The lack of differentiated V2 interneuron markers in Vsx1+ cells and their medial location in the intermediate zone (Figures 1, 2) prompted us to assess whether they were differentiating neurons. Co-labeling experiments from e9.5 to e11.5 showed that only a minority of the Vsx1+ cells, decreasing from 14% to 0%, respectively, contained the neuronal differentiation marker β-III-tubulin (Figures 3A–D). This suggested that Vsx1 was present before the onset of neuronal differentiation in p2-derived cells. Accordingly, Sox1, which labels the spinal progenitors in the ventricular zone, was detected in medial Vsx1+ cells located within the subventricular zone (Figures 2E, 3C–C″). These observations supported the hypothesis that Vsx1 is expressed in early V2 precursors before the onset of expression of their population-specific differentiation markers. Consistent with this idea, β-III-tubulin was detected in all the V2a and V2b cells at e10.5 (Figures 3E–E″) and the cyclin-dependent kinase inhibitor protein p27Kip1 was present in V2a and V2b interneurons but not in Vsx1+ cells (Figures 3F–F″, Chx10 and Gata3 both in blue). This suggested that Vsx1 expression occurs earlier than that of Chx10 and Gata3 in V2 interneurons. Hence, Vsx1 may be restricted to an early V2 compartment prior to V2 interneuron diversification.
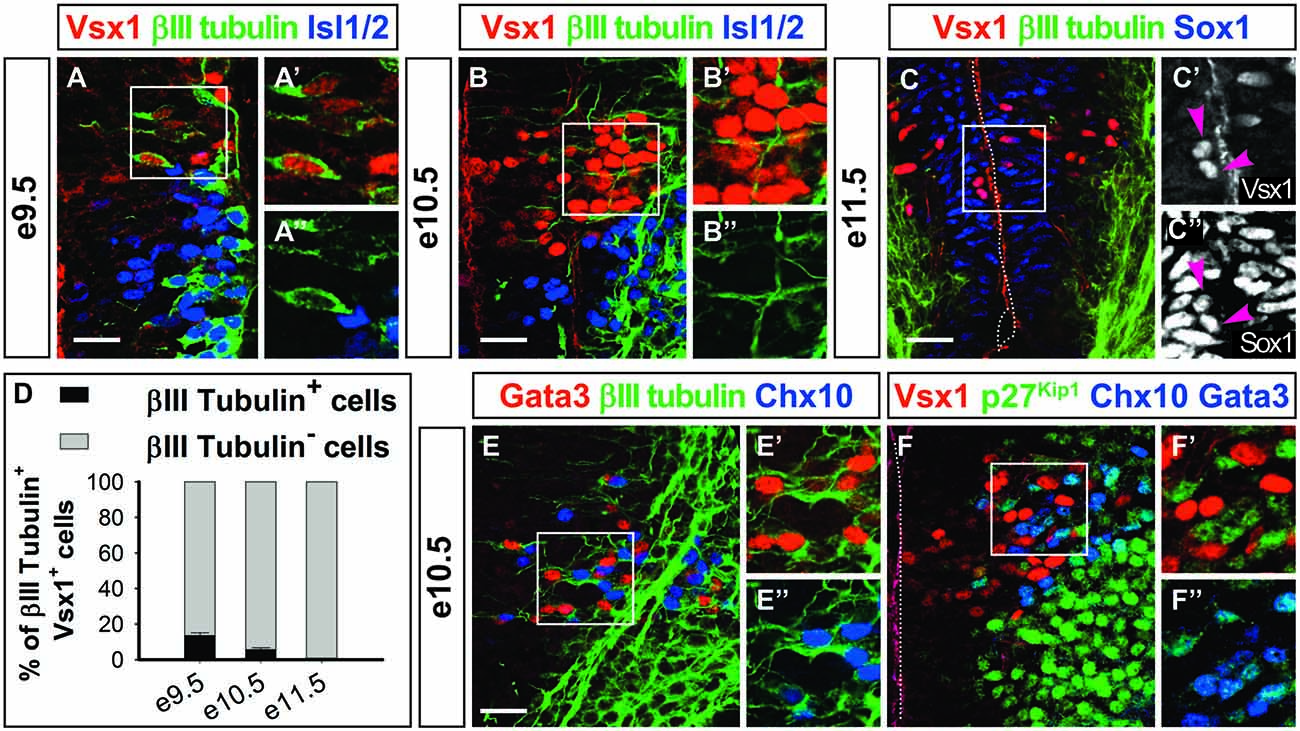
Figure 3. Vsx1 is present before the onset of neuronal differentiation. (A–B″) At e9.5 (A–A″) and e10.5 (B–B″), the majority of the Vsx1+ cells are devoid of β-III-tubulin. In contrast, most of the motor neurons (Isl1/2+) contain β-III-tubulin at this stage. (C–C″) Co-labeling of β-III-tubulin and Vsx1 is never observed at e11.5, and the Vsx1+ cells are located between the neural progenitors (Sox1+) and the differentiating neurons (β-III-tubulin+). Vsx1 is detected in some Sox1+ progenitors (arrowheads). (D) Quantifications (mean percentage ± SD, n = 3) shows that only a minority of Vsx1+ cells contain β-III-tubulin at e9.5 and e10.5 and none at e11.5. (E–E″) In contrast, at e10.5, β-III-tubulin is readily detected in differentiating V2a (Chx10+) or V2b (Gata3+) interneurons. (F–F″) Accordingly, p27Kip is present in V2a and V2b interneurons (Chx10+ and Gata3+, both in blue) but not in Vsx1+ cells. Scale bars = 50 μm.
To test this idea, we compared the distribution of Vsx1 with that of Prox1 and Insm1. These two factors define an early intermediate compartment containing late progenitors (i.e., progenitors in their last division) and newborn neurons (Duggan et al., 2008; Misra et al., 2008). Indeed, we found that Vsx1 was present in cells containing both Prox1 and Insm1 (Figures 4A–A″), demonstrating that Vsx1 is restricted to an early intermediate V2 subset. The presence of Ascl1 in this subset but not in differentiating V2a or V2b interneurons (Figures 1B–B″, 2G–G″, 4B–B″, Chx10 and Gata3 both in blue) suggested that the Vsx1+ cells may correspond to precursors of the described V2 populations. We thus investigated whether Vsx1+ cells contained other determinants of V2 development. The segregation of V2a and V2b interneurons depends on a regulatory network involving Ascl1 and Foxn4 that eventually controls production of the Notch ligand Dll4 and thereby differentially modulates activation of Notch signaling in V2 precursors (Li et al., 2005; Del Barrio et al., 2007; Peng et al., 2007; Misra et al., 2014; Zou et al., 2015). Dll4 was present in Vsx1+ cells at the time of V2a/V2b production (Figures 4C–C″, arrowheads), although it was not exclusive to the Vsx1+ population. Similarly, Foxn4 expression was detected by double ISH in the cells expressing Vsx1 (Figures 4D–F′). As V2 precursors expressing Foxn4 have been shown to generate all the described V2 interneuron populations as well as additional V2 subsets (Li et al., 2010), our data demonstrate that Vsx1+ cells are precursors of V2a/V2b interneurons and of other V2 subsets that also derive from Foxn4- and Dll4-containing progenitors (Panayi et al., 2010; Panayiotou et al., 2013; Zou et al., 2015).
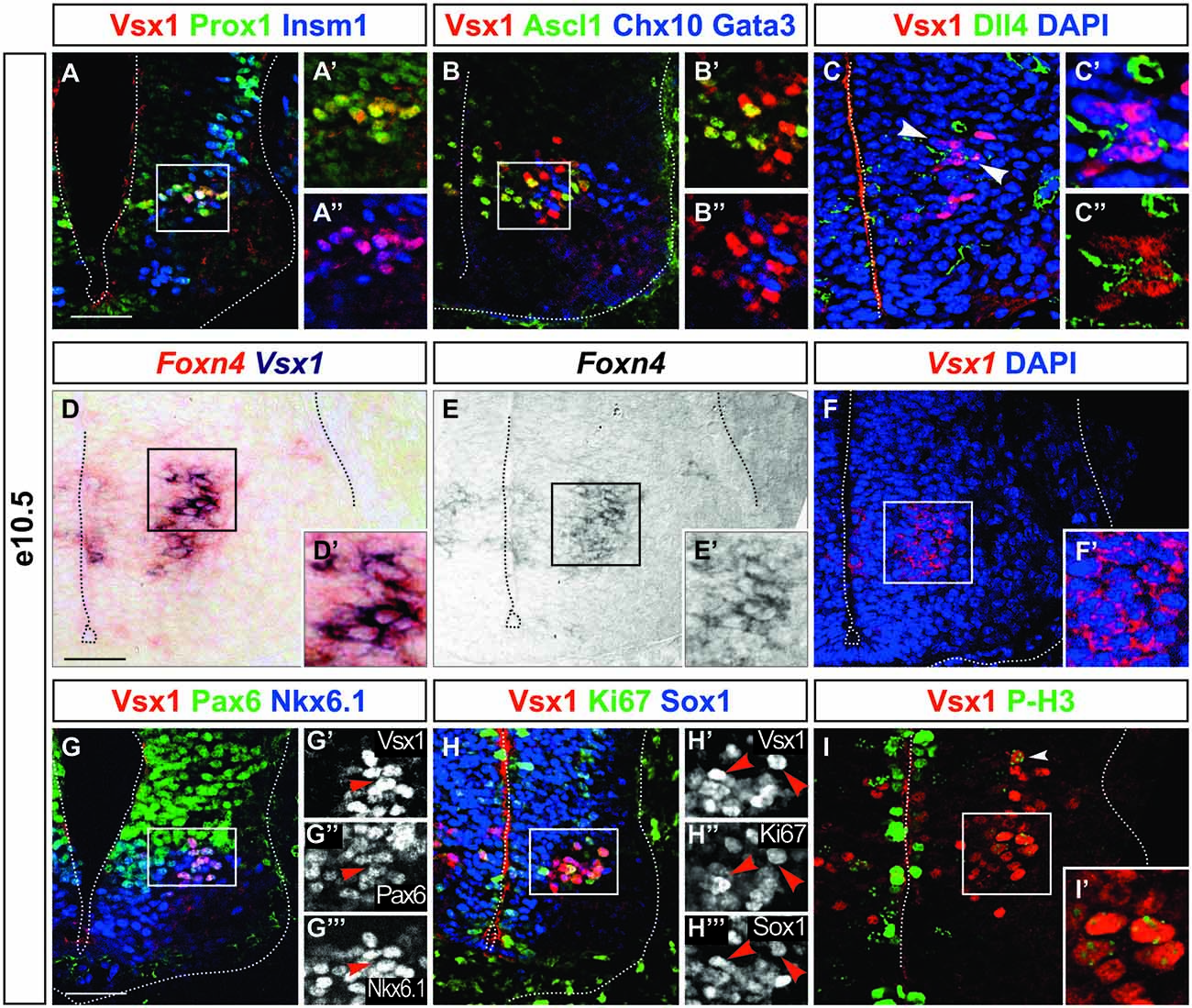
Figure 4. Vsx1 is present in an early intermediate compartment during V2 interneuron differentiation. (A–A″) Vsx1 is detected in the intermediate compartment characterized by the presence of Prox1 and Insm1. (B–B″) Ascl1 is co-detected with Vsx1, which is not the case for Chx10 and Gata3 (both in blue). (C–C″) Dll4 is detected in Vsx1+ cells (arrowheads). (D–F′) Vsx1 mRNA is detected by double in situ hybridization (ISH) in cells expressing Foxn4. (G–G″′) Pax6 and Nkx6.1 are maintained in cells containing Vsx1. (H–H″′) Although Sox1 is present at low levels in some Vsx1+ cells, Ki-67 is barely detectable. (I,I′) Only remaining traces (arrowhead) of phospho-HistoneH3 is detected in cells containing Vsx1, as compared to the strong labeling of the dividing progenitors in the ventricular zone. Scale bars = 50 μm.
We then assessed whether Vsx1 is detected in dividing cells of the ventricular zone or in intermediate precursor cells. Pax6 and Nkx6.1, which are present in the p2 progenitors, were detected in the Vsx1+ cells. However, these cells were clustered nearby the lateral border of the ventricular zone rather than the lumen (Figures 4G–G″′). Consistently, although Sox1 was present at low levels (Figures 2E, 4H–H″′), the proliferation marker Ki-67 was barely detectable in Vsx1+ cells (Figures 4H–H″′) and only remaining traces of phosphorylated-Histone H3, specific to the metaphase of mitotic division, were found (Figures 4I,I′, arrowhead). Taken together, these observations suggest that Vsx1 is present in cells that just completed their last mitotic division but did not yet initiate neuronal differentiation, defining an early intermediate V2 interneuron compartment. As observed respectively in motor neurons and in V1 interneurons, Nkx6.1 and Pax6 are maintained in these cells even though they are no longer dividing.
The Developmental Determinants of V2 Interneurons Are not Required for the Production of Vsx1+ Cells
Given the possibility that Vsx1+ cells may constitute precursors of V2 populations, we assessed whether their production depends on factors necessary for proper V2 interneuron development. The segregation of the V2a and V2b lineages relies on the asymmetrical activation of the Notch pathway by Dll4, which stimulates Notch signaling in surrounding cells and thereby promotes V2b differentiation, restricting V2a differentiation to cells with lower Notch activity (Li et al., 2005; Del Barrio et al., 2007; Peng et al., 2007; Misra et al., 2014; Zou et al., 2015). Proper activation of this pathway requires mosaic expression of several transcriptional regulators including Foxn4 and Ascl1 in the p2 domain (Li et al., 2005; Del Barrio et al., 2007; Xiang and Li, 2013; Misra et al., 2014). To assess whether the production of the Vsx1+ cells also depends on this network, we evaluated the phenotype of these populations in embryos mutant for Foxn4, Ascl1 or PS1. Lack of Foxn4 resulted as expected in a strong imbalance in the generation of V2 interneuron subsets, with V2a being produced in excess at the expense of V2b cells (Figures 5A–B″). However, the generation of Vsx1+ cells was not affected (Figures 5A–C). Similarly, inactivation of Ascl1 resulted in excessive production of V2a interneurons at the expense of V2b, but the number of Vsx1+ cells was not altered (Figures 5D–F). Consistently, loss of PS1, which results in severe diminution of Notch activation (Struhl and Greenwald, 2001), did not alter the specification of Vsx1+ cells (Figures 5G–I). These observations indicate that the generation of Vsx1+ cells does not depend on Notch signaling, but remain consistent with the hypothesis that these cells may constitute the precursor population wherein differential activation of the Notch pathway determines further V2 interneuron diversification.
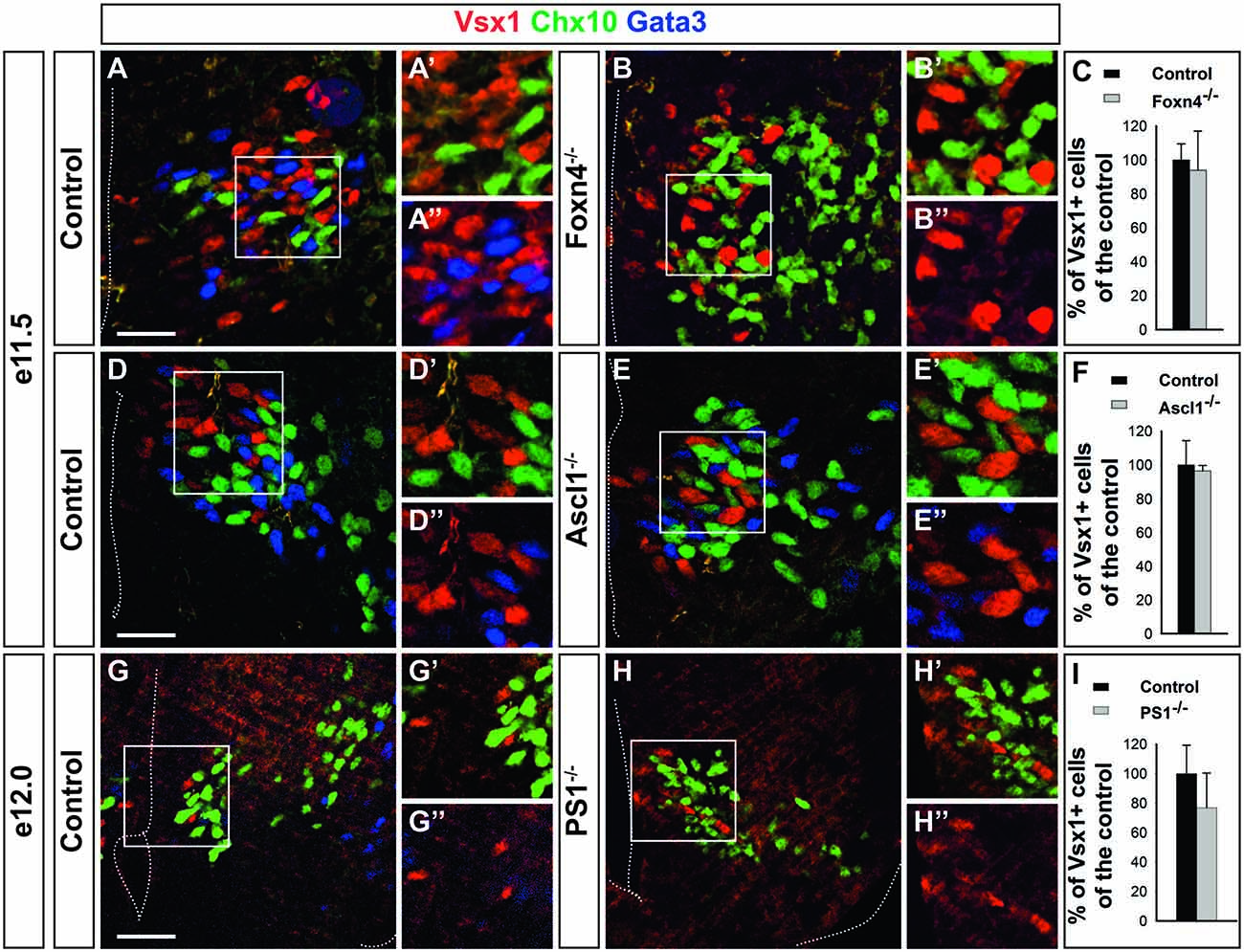
Figure 5. The generation of Vsx1+ cells does not require Notch signaling. (A–C) The generation of Vsx1+ cells is not altered in Foxn4 mutant embryos, although production of V2b interneurons (Gata3+) is abolished in favor of V2a interneurons (Chx10+). (D–F) Vsx1+ cell production is not altered in Ascl1 mutant embryos whereas supernumerary V2a interneurons are generated at the expense of V2b cells. (G–I) In Presenilin-1 (PS1) mutant embryos, the number of Vsx1+ cells was similar to that of control embryos whereas V2b interneurons were replaced by V2a interneurons. Scale bars = 50 μm. Quantifications are expressed as mean percentage ± SD, n = 4.
Pax6 Is Required for Proper Expression of Vsx1 in V2 Precursors
We then turned our analyses to the other transcription factors expressed during Vsx1+ cell development. OC factors are present in the early Vsx1+ cells (Figures 1, 2). The role of OC factors has been evaluated in Oc1/Oc2−/− double-mutant embryos, which also lack Oc3 in the CNS (Roy et al., 2012). However, the number of Vsx1+ cells was not changed in the spinal cord of these mutants, suggesting that OC proteins are not necessary for their production (Figures 6A–C).
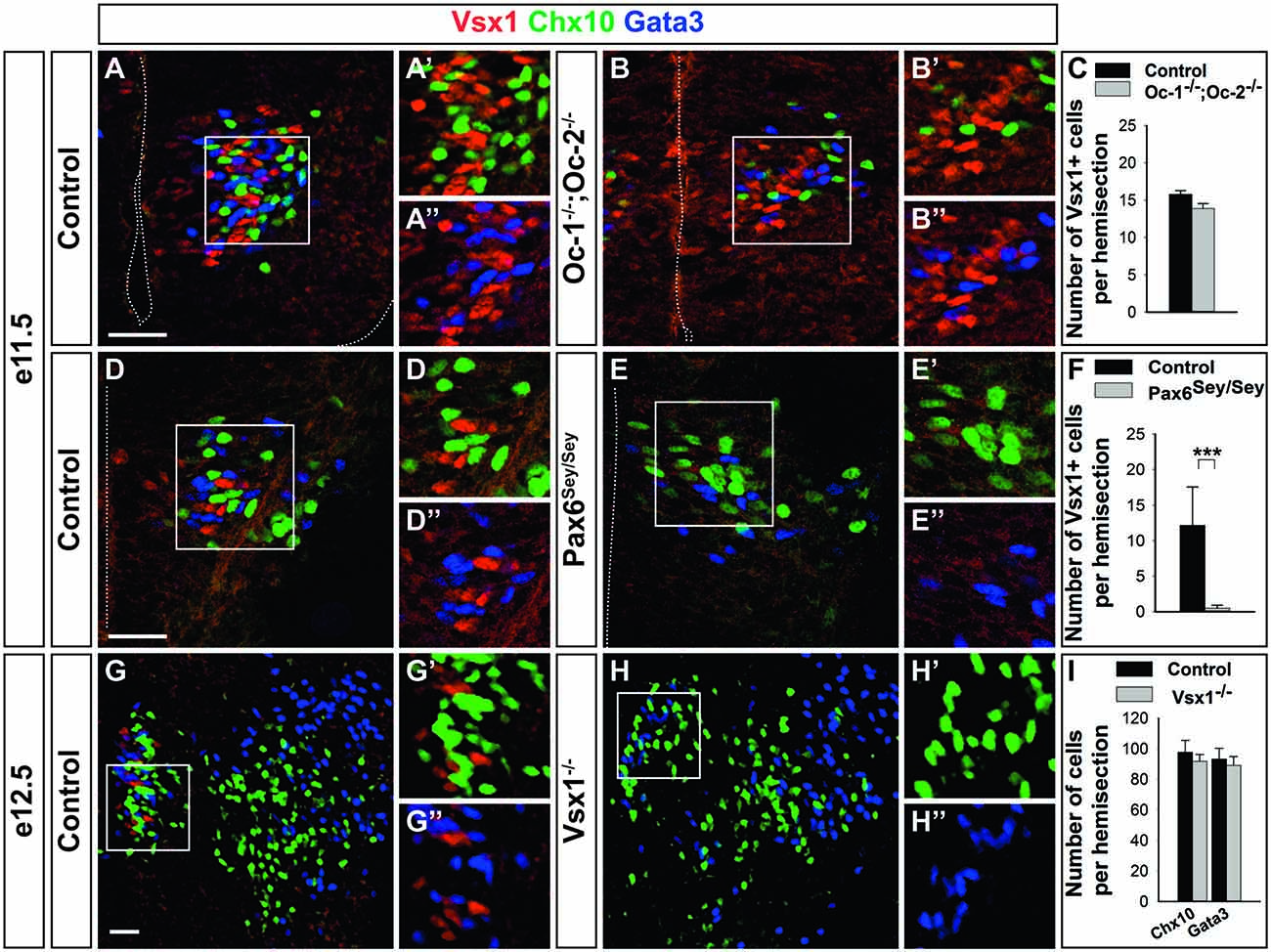
Figure 6. Pax6 is necessary for Vsx1 expression. (A–C) The generation of Vsx1+, Chx10 + and Gata3 + cells, is not affected in Oc1−/−Oc2−/− embryos. (D–F) In Pax6Sey/Sey embryos, the number of Vsx1+ cells is dramatically reduced (***p < 0.001) without similar alteration of V2a or V2b production. (G–I) In embryos lacking Vsx1, the production of V2a and of V2b interneurons is unaffected. Scale bars = 50 μm. Quantifications are expressed as mean values ± SEM, n = 3.
Next, we investigated the role of Pax6 in Vsx1+ cell generation. Pax6 is required for patterning the ventral spinal cord during neurogenesis (Ericson et al., 1997) and for proper generation of V1 interneurons (Burrill et al., 1997; Sapir et al., 2004; Gosgnach et al., 2006). In Small eye (Sey/Sey) mutant embryos, which lack Pax6 (Glaser et al., 1992), the production of V0 and V2b populations is not affected (Burrill et al., 1997; Sapir et al., 2004; Gosgnach et al., 2006; Zhang et al., 2014) while the number of V2a interneurons is only slightly decreased (Ericson et al., 1997; Gosgnach et al., 2006). The persistence of Pax6 in the early Vsx1+ cells suggested that this factor may contribute to their development. Accordingly, Vsx1 was barely detectable in Sey/Sey embryos at e11.5 (Figures 6D–F) and e12.5 (data not shown), although the production of V2a and V2b interneurons was conserved. The presence of V2a and V2b interneurons indicated that the p2 progenitor domain was not re-specified and that the V2a/V2b precursors were preserved. In contrast, the loss of Vsx1 unveiled a selective requirement for Pax6 in the expression of Vsx1 in this early intermediate V2 compartment. Furthermore, these observations suggest that Vsx1 may be dispensable for proper generation of V2 interneuron subsets.
To test this hypothesis, we assessed the role of Vsx1 in V2 interneuron development. In the absence of any other specific marker of the Vsx1+ cells, this compartment could not be analyzed in the Vsx1 mutant embryos. In contrast, as Vsx1-containing precursors generate all the V2 subsets, we addressed a possible role for Vsx1 in V2 interneuron development. An inverse relationship between the levels of Chx10 and Vsx1 was previously reported in bipolar cells of the retina (Clark et al., 2008). To investigate whether a similar relationship also exists in spinal cord, the distribution of Chx10 was first assessed in Vsx1 mutant embryos. However, the number of Chx10-positive cells was similar at e12.5 in control and in mutant embryos, indicating that the absence of Vsx1 does neither impact on V2a interneuron production nor on Chx10 expression. Accordingly, the number of V2b interneurons was not affected and the V2a/V2b ratio was preserved (Figures 6G–I). Taken together, these observations confirm that Vsx1 is not required for the production of described V2 interneuron populations, suggesting that the integrity of the early V2 precursor compartment is also preserved in Vsx1 mutant embryos. Consistently, Vsx1 mutant mice did not display any alteration in their motor behavior (data not shown).
Discussion
During spinal cord development, ventral progenitor domains produce multiple neuronal populations that variously contribute to the activity of motor circuits. Here, we provide evidence that the p2 progenitor domain generates an early intermediate compartment characterized by the presence of Vsx1 prior to neuronal differentiation. This early compartment very likely produces the collection of known V2 interneuron populations, and may also generate additional V2 subsets that remain to be identified (Figure 7).
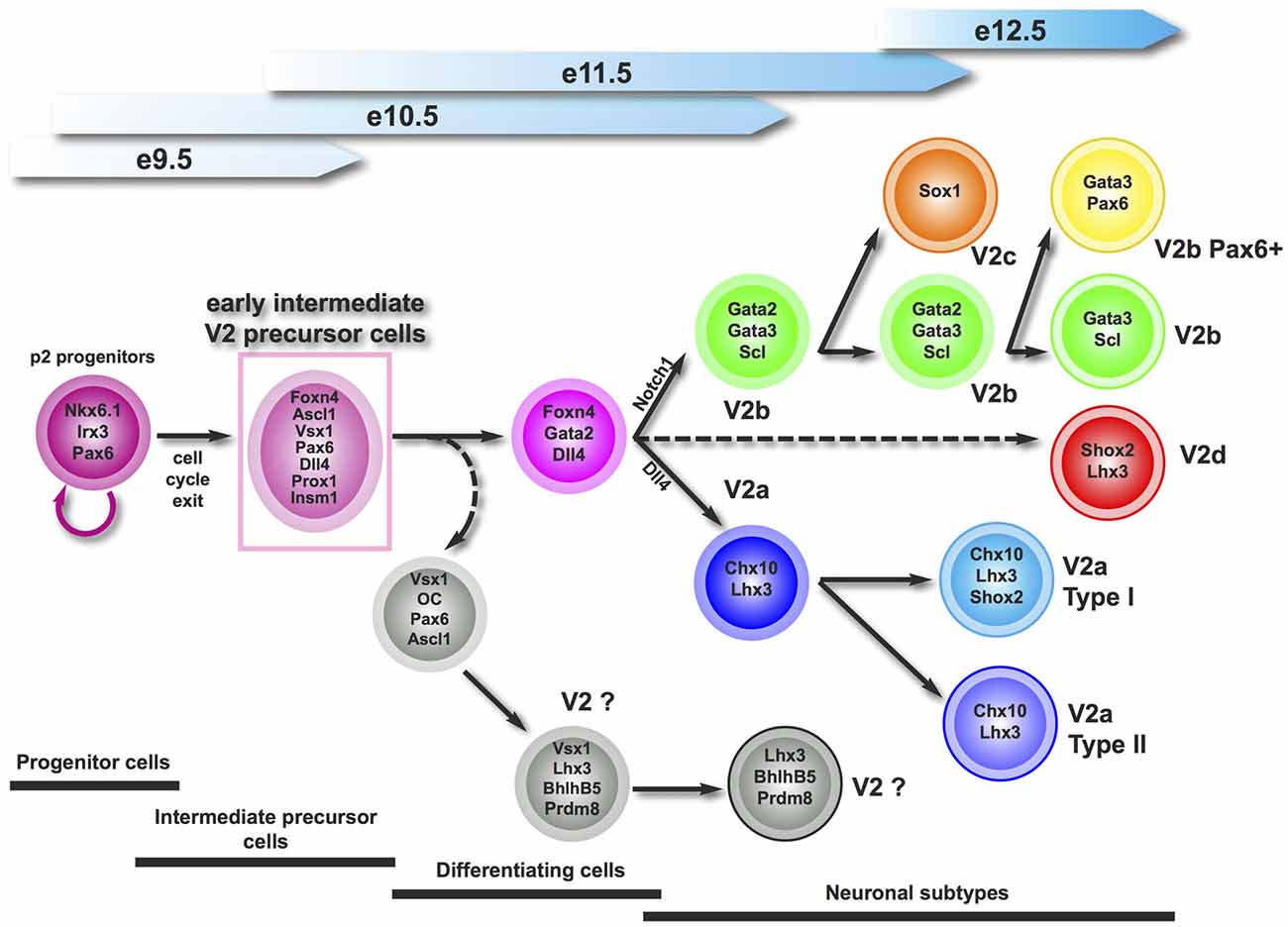
Figure 7. Proposed working model for the generation of V2 interneuron subtypes. Vsx1 defines an early intermediate precursor compartment that generates all the described V2 interneuron subsets, and may produce additional V2 subpopulation(s) that remain(s) to be characterized.
In the zebrafish embryonic spinal cord, Vsx1 is detected in common V2a/V2b progenitors and its expression is transiently maintained in the V2a lineage (Batista et al., 2008; Kimura et al., 2008). In Xenopus and chick, the expression of Vsx1 was attributed to V2a interneurons based on its distribution similar to that of Chx10 (Chen and Cepko, 2000; D’Autilia et al., 2006). Here, we demonstrate that, in contrast to other species, murine Vsx1 is restricted to an early intermediate compartment that likely comprises precursors of the known V2 subsets. Although they contain factors known as progenitor markers, including Sox1, Nkx6.1 and Pax6, Vsx1+ cells are not proliferating as evidenced by the absence of proliferation or mitosis markers. Conversely, Vsx1+ cells are not differentiating neurons, as they neither display cyclin-dependant kinase inhibitor protein of V2 interneurons (Gui et al., 2007) and very few of them produce β-III-tubulin. Therefore, they constitute an early intermediate compartment of V2 postmitotic precursors (Figure 7).
Consistent with this idea, Vsx1+ cells contain Prox1 and Insm1, which specifically label compartments comprising late progenitors and newborn neurons and are transiently expressed before the onset of neuronal differentiation (Duggan et al., 2008; Misra et al., 2008). Prox1 acts downstream of the pro-neural proteins Ascl1 and Neurog-2 to implement neurogenesis in the spinal interneurons and may antagonize the anti-neurogenic activity of Sox proteins (Misra et al., 2008) including Sox1, which is present in spinal progenitors. Insm1 is thought to play a role in the termination of progenitor proliferation throughout the nervous system (Duggan et al., 2008). Prox1 and Insm1 may affect the onset and extent of terminal and penultimate neurogenic divisions, and thus the total number of neurons produced, in any area and stage of neurogenesis. In the spinal intermediate V2 compartment defined by the presence of Vsx1, one may additionally propose that Prox1 and Insm1 could delay the onset of neuronal differentiation and thereby open a time-window necessary for the activation of signaling pathways, including Notch, that will determine the diversification of V2 precursors in multiple V2 populations.
Our data suggest that Vsx1+ cells are the precursors of most of the V2 interneurons (Figure 7) including V2a and V2b subsets (Li et al., 2005; Del Barrio et al., 2007; Peng et al., 2007; Misra et al., 2014; Zou et al., 2015). Vsx1 is never co-detected with the V2a or V2b specific markers Chx10 or Gata3 although some Vsx1+ cells very likely differentiate into V2a interneurons as they contain Dll4 (Zou et al., 2015). This indicates a sharp downregulation of Vsx1 expression at the onset of V2 diversification. Interestingly, Chx10 expression is repressed by Vsx1 in Type 7 cone bipolar cells (Shi et al., 2011) and Vsx1 expression in retinal cells is inhibited by Chx10 (Clark et al., 2008). Such a cross-repression loop may also exist between these two Prd-L:CVC factors during V2a differentiation and may contribute to rapidly inhibiting Vsx1 expression in differentiating V2a cells. However, Chx10 distribution was not expanded in the absence of Vsx1, indicating that the latter is not required to restrict Chx10 expression to the differentiating V2a interneurons. In contrast, lack of co-detection of Vsx1 and Gata3 suggests either that other mechanisms downregulate Vsx1 expression at the onset of V2b differentiation or that V2b interneurons do not derive from Vsx1+ cells. The latter possibility is unlikely since we detected Vsx1 in the cells expressing Foxn4 and V2b interneurons derive from Foxn4-positive precursors (Del Barrio et al., 2007; Li et al., 2010). Hence, the mechanisms that sharply downregulate Vsx1 expression at the onset of V2 diversification and the necessity of this tight regulation require further investigations.
Our observations strongly suggest that Vsx1 defines an early intermediate V2 compartment that generates multiple V2 subsets. Whether Vsx1+ cells constitute only precursors of the known V2 populations or additionally produce other V2 subsets (Figure 7) remains an open question. The existence of an additional V2 population that derives from Foxn4-expressing cells has been demonstrated (Li et al., 2010), and Vsx1 and Foxn4 are extensively coexpressed in the early V2 precursors. Therefore, spinal Vsx1+ cells very likely produce yet unknown V2 subsets.
Our study of the developmental determinants of the Vsx1+ cells identified a novel role for Pax6 in the developing spinal cord. Pax6 is initially present in all progenitor domains of the neural tube and, following neural patterning, is excluded from the p3 domain (Ericson et al., 1997). It is also transiently maintained in V1 (Stam et al., 2012) and in Vsx1+ cells (this study). It is necessary for the production of V1 interneurons and slightly impacts on the generation of V2a cells but not of the adjacent V0 or V2b populations (Burrill et al., 1997; Ericson et al., 1997; Sapir et al., 2004; Gosgnach et al., 2006; Zhang et al., 2014). Here we provide evidence that Pax6 is additionally necessary for the expression of Vsx1 in an early intermediate V2 compartment. The loss of Vsx1 expression in the spinal cord of Sey mutants is unlikely to result from defective dorsoventral patterning or altered neurogenesis in the p2 domain since V2b cells are generated properly and V2a interneurons are only slightly decreased (Burrill et al., 1997; Ericson et al., 1997; Sapir et al., 2004; Gosgnach et al., 2006; Zhang et al., 2014). It more likely manifests a requirement of Pax6 for the expression of Vsx1 in this early V2 compartment. Identification of other specific markers for these cells will be required to test this hypothesis. In the retina, Pax6 and Vsx1 are present in different cellular compartments (de Melo et al., 2003), suggesting that Vsx1 in the spinal cord may have been recruited into genetic networks different from those that operate in the eye. Consistently, Vsx1 expression is reactivated after spinal cord injury in the adult zebrafish and a large majority of the Vsx1+ cells observed after lesion also contains Pax6 (Kuscha et al., 2012).
In the zebrafish embryonic spinal cord, Vsx1 is detected in common V2a/V2b progenitors and is transiently maintained in part of the V2a lineage. The onset of Vsx1 expression seems to occur in committed intermediate progenitors, i.e., cells that will undergo a last division to produce pairs of neurons between which Notch signaling will be activated to segregate V2a and V2b interneurons. However, these studies did not exclude the existence of an additional V2 population wherein Vsx1 would be individually present (Batista et al., 2008; Kimura et al., 2008). Compared to our data, these observations suggest that Vsx1 is initially expressed in a pattern reminiscent of its paralog Chx10, although with an earlier onset, and has been recruited during vertebrate evolution to earlier multipotent neuronal precursors able to generate a large array of V2 subsets. Increased neuronal production and diversification in this early intermediate precursor compartment may have provided motor circuits with specific properties necessary for terrestrial tetrapod locomotion. The detection of murine Vsx1 in this early compartment and the presence in these cells of several markers also found in V2a interneurons are consistent with this late evolution. Functional studies will enable to determine the roles of their derivative populations in the spinal motor circuits and to assess whether they contribute to the execution of original motor capacities present in higher vertebrates.
Funding
This work was supported by grants from the “Fonds spéciaux de recherche” (FSR) of the Université catholique de Louvain including a FSR-COFUND (Marie Curie) to MH-F, by a “Projet de recherche (PDR)” #T.0117.13 and an “Equipement (EQP)” funding #U.N027.14 of the Fonds de la Recherche Scientifique (F.R.S.-FNRS), by the “Actions de Recherche Concertées (ARC)” #10/15-026 of the “Direction générale de l’Enseignement non obligatoire et de la Recherche scientifique—Direction de la Recherche scientifique—Communauté française de Belgique” and granted by the “Académie universitaire “Louvain””, and by the “Association Belge contre les Maladies neuro-Musculaires asbl” (ABMM) to FC; by the National Institutes of Health (EY020849) to MX; by the Canadian Institutes for Health Research to RLC; and by the Institut National de la Santé et de la Recherche Médicale Grant R05245DS to CP. KM was supported by a grant from the New Jersey Commission on Spinal Cord Research, and HH was funded by the Neuropôle de Recherche Francilien/Région de l’Ile-de-France and by the Fondation pour l’Aide à la Recherche sur la Sclerose En Plaque. MH-F was a Postdoctoral Researcher and FC is a Research Associate of the F.R.S.-FNRS, respectively. SD and AH hold a specialization grant from the F.R.I.A. (Belgium).
Author Contributions
CF, MH-F, SD, BP and FC designed the experiments. All authors participated in performing the experiments and discussed the data. CF, MH-F, BP, SD and FC contributed to drafting the manuscript.
Conflict of Interest Statement
The authors declare that the research was conducted in the absence of any commercial or financial relationships that could be construed as a potential conflict of interest.
Acknowledgments
We thank members of the NEDI lab for material, technical support and discussions. We are grateful to Drs. A. Pierani and M. Barber for mouse embryos, and to Drs. D. Kurek, F. Grosveld, S.E. Ross, J. Welcker, Y. Ono, J. Johnson, K. Sharma and C. Cepko for reagents. The antibodies anti Isl1/2 (39.4D5), anti Lhx1/5 (4F2) and anti Lhx3 (67.4E12) developed by Thomas M. Jessell and Susan Brenner-Morton and anti Pax6 developed by Atsushi Kawakami were obtained from the Developmental Studies Hybridoma Bank, created by the NICHD of the NIH and maintained at The University of Iowa, Department of Biology, Iowa City, IA 52242.
References
Al-Mosawie, A., Wilson, J. M., and Brownstone, R. M. (2007). Heterogeneity of V2-derived interneurons in the adult mouse spinal cord. Eur. J. Neurosci. 26, 3003–3015. doi: 10.1111/j.1460-9568.2007.05907.x
Azim, E., Jiang, J., Alstermark, B., and Jessell, T. M. (2014). Skilled reaching relies on a V2a propriospinal internal copy circuit. Nature 508, 357–363. doi: 10.1038/nature13021
Batista, M. F., Jacobstein, J., and Lewis, K. E. (2008). Zebrafish V2 cells develop into excitatory CiD and Notch signalling dependent inhibitory VeLD interneurons. Dev. Biol. 322, 263–275. doi: 10.1016/j.ydbio.2008.07.015
Beguin, S., Crépel, V., Aniksztejn, L., Becq, H., Pelosi, B., Pallesi-Pocachard, E., et al. (2013). An epilepsy-related ARX polyalanine expansion modifies glutamatergic neurons excitability and morphology without affecting GABAergic neurons development. Cereb. Cortex 23, 1484–1494. doi: 10.1093/cercor/bhs138
Britz, O., Zhang, J., Grossmann, K. S., Dyck, J., Kim, J. C., Dymecki, S., et al. (2015). Correction: a genetically defined asymmetry underlies the inhibitory control of flexor-extensor locomotor movements. Elife 4:e13038. doi: 10.7554/eLife.13038
Burrill, J. D., Moran, L., Goulding, M. D., and Saueressig, H. (1997). PAX2 is expressed in multiple spinal cord interneurons, including a population of EN1+ interneurons that require PAX6 for their development. Development 124, 4493–4503.
Chen, C.-M., and Cepko, C. L. (2000). Expression of Chx10 and Chx10–1 in the developing chicken retina. Mech. Dev. 90, 293–297. doi: 10.1016/s0925-4773(99)00251-8
Chow, R. L., Snow, B., Novak, J., Looser, J., Freund, C., Vidgen, D., et al. (2001). Vsx1, a rapidly evolving paired-like homeobox gene expressed in cone bipolar cells. Mech. Dev. 109, 315–322. doi: 10.1016/s0925-4773(01)00585-8
Chow, R. L., Volgyi, B., Szilard, R. K., Ng, D., McKerlie, C., Bloomfield, S. A., et al. (2004). Control of late off-center cone bipolar cell differentiation and visual signaling by the homeobox gene Vsx1. Proc. Natl. Acad. Sci. U S A 101, 1754–1759. doi: 10.1073/pnas.0306520101
Clark, A. M., Yun, S., Veien, E. S., Wu, Y. Y., Chow, R. L., Dorsky, R. I., et al. (2008). Negative regulation of Vsx1 by its paralog Chx10/Vsx2 is conserved in the vertebrate retina. Brain Res. 1192, 99–113. doi: 10.1016/j.brainres.2007.06.007
Clotman, F., Jacquemin, P., Plumb-Rudewiez, N., Pierreux, C. E., Van der Smissen, P., Dietz, H. C., et al. (2005). Control of liver cell fate decision by a gradient of TGF β signaling modulated by Onecut transcription factors. Genes Dev. 19, 1849–1854. doi: 10.1101/gad.340305
Crone, S. A., Quinlan, K. A., Zagoraiou, L., Droho, S., Restrepo, C. E., Lundfald, L., et al. (2008). Genetic ablation of V2a ipsilateral interneurons disrupts left-right locomotor coordination in mammalian spinal cord. Neuron 60, 70–83. doi: 10.1016/j.neuron.2008.08.009
D’Autilia, S., Decembrini, S., Casarosa, S., He, R.-Q., Barsacchi, G., Cremisi, F., et al. (2006). Cloning and developmental expression of the Xenopus homeobox gene Xvsx1. Dev. Genes Evol. 216, 829–834. doi: 10.1007/s00427-006-0109-0
Del Barrio, M. G., Taveira-Marques, R., Muroyama, Y., Yuk, D. I., Li, S., Wines-Samuelson, M., et al. (2007). A regulatory network involving Foxn4, Mash1 and delta-like 4/Notch1 generates V2a and V2b spinal interneurons from a common progenitor pool. Development 134, 3427–3436. doi: 10.1242/dev.005868
de Melo, J., Qiu, X., Du, G., Cristante, L., and Eisenstat, D. D. (2003). Dlx1, Dlx2, Pax6, Brn3b and Chx10 homeobox gene expression defines the retinal ganglion and inner nuclear layers of the developing and adult mouse retina. J. Comp. Neurol. 461, 187–204. doi: 10.1002/cne.10674
Dougherty, K. J., Zagoraiou, L., Satoh, D., Rozani, I., Doobar, S., Arber, S., et al. (2013). Locomotor rhythm generation linked to the output of spinal shox2 excitatory interneurons. Neuron 80, 920–933. doi: 10.1016/j.neuron.2013.08.015
Duggan, A., Madathany, T., de Castro, S. C., Gerrelli, D., Guddati, K., and García-Añoveros, J. (2008). Transient expression of the conserved zinc finger gene INSM1 in progenitors and nascent neurons throughout embryonic and adult neurogenesis. J. Comp. Neurol. 507, 1497–1520. doi: 10.1002/cne.21629
Ericson, J., Rashbass, P., Schedl, A., Brenner-Morton, S., Kawakami, A., van Heyningen, V., et al. (1997). Pax6 controls progenitor cell identity and neuronal fate in response to graded Shh signaling. Cell 90, 169–180. doi: 10.1016/s0092-8674(00)80323-2
Espana, A., and Clotman, F. (2012). Onecut transcription factors are required for the second phase of development of the A13 dopaminergic nucleus in the mouse. J. Comp. Neurol. 520, 1424–1441. doi: 10.1002/cne.22803
Francius, C., and Clotman, F. (2010). Dynamic expression of the Onecut transcription factors HNF-6, OC-2 and OC-3 during spinal motor neuron development. Neuroscience 165, 116–129. doi: 10.1016/j.neuroscience.2009.09.076
Francius, C., Harris, A., Rucchin, V., Hendricks, T. J., Stam, F. J., Barber, M., et al. (2013). Identification of multiple subsets of ventral interneurons and differential distribution along the rostrocaudal axis of the developing spinal cord. PLoS One 8:e70325. doi: 10.1371/journal.pone.0070325
Francius, C., Ravassard, P., Hidalgo-Figueroa, M., Mallet, J., Clotman, F., and Nardelli, J. (2015). Genetic dissection of Gata2 selective functions during specification of V2 interneurons in the developing spinal cord. Dev. Neurobiol. 75, 721–737. doi: 10.1002/dneu.22244
Garcia-Campmany, L., Stam, F. J., and Goulding, M. (2010). From circuits to behaviour: motor networks in vertebrates. Curr. Opin. Neurobiol. 20, 116–125. doi: 10.1016/j.conb.2010.01.002
Glaser, T., Walton, D. S., and Maas, R. L. (1992). Genomic structure, evolutionary conservation and aniridia mutations in the human PAX6 gene. Nat. Genet. 2, 232–239. doi: 10.1038/ng1192-232
Gong, S., Zheng, C., Doughty, M. L., Losos, K., Didkovsky, N., Schambra, U. B., et al. (2003). A gene expression atlas of the central nervous system based on bacterial artificial chromosomes. Nature 425, 917–925. doi: 10.1038/nature02033
Gosgnach, S., Lanuza, G. M., Butt, S. J., Saueressig, H., Zhang, Y., Velasquez, T., et al. (2006). V1 spinal neurons regulate the speed of vertebrate locomotor outputs. Nature 440, 215–219. doi: 10.1038/nature04545
Gouge, A., Holt, J., Hardy, A. P., Sowden, J. C., and Smith, H. K. (2001). Foxn4—a new member of the forkhead gene family is expressed in the retina. Mech. Dev. 107, 203–206. doi: 10.1016/s0925-4773(01)00465-8
Goulding, M. (2009). Circuits controlling vertebrate locomotion: moving in a new direction. Nat. Rev. Neurosci. 10, 507–518. doi: 10.1038/nrn2608
Grillner, S., and Jessell, T. M. (2009). Measured motion: searching for simplicity in spinal locomotor networks. Curr. Opin. Neurobiol. 19, 572–586. doi: 10.1016/j.conb.2009.10.011
Grossmann, K. S., Giraudin, A., Britz, O., Zhang, J., and Goulding, M. (2010). Genetic dissection of rhythmic motor networks in mice. Prog. Brain Res. 187, 19–37. doi: 10.1016/b978-0-444-53613-6.00002-2
Gui, H., Li, S., and Matise, M. P. (2007). A cell-autonomous requirement for Cip/Kip cyclin-kinase inhibitors in regulating neuronal cell cycle exit but not differentiation in the developing spinal cord. Dev. Biol. 301, 14–26. doi: 10.1016/j.ydbio.2006.10.035
Guillemot, F., Lo, L.-C., Johnson, J. E., Auerbach, A., Anderson, D. J., and Joyner, A. L. (1993). Mammalian achaete-scute homolog 1 is required for the early development of olfactory and autonomic neurons. Cell 75, 463–476. doi: 10.1016/0092-8674(93)90381-y
Hill, R. E., Favor, J., Hogan, B. L., Ton, C. C., Saunders, G. F., Hanson, I. M., et al. (1992). Mouse Small eye results from mutations in a paired-like homeobox-containing gene. Nature 355:750. doi: 10.1038/355750a0
Jacquemin, P., Durviaux, S. M., Jensen, J., Godfraind, C., Gradwohl, G., Guillemot, F., et al. (2000). Transcription factor hepatocyte nuclear factor 6 regulates pancreatic endocrine cell differentiation and controls expression of the proendocrine gene ngn3. Mol. Cell. Biol. 20, 4445–4454. doi: 10.1128/mcb.20.12.4445-4454.2000
Joshi, K., Lee, S., Lee, B., Lee, J. W., and Lee, S. K. (2009). LMO4 controls the balance between excitatory and inhibitory spinal V2 interneurons. Neuron 61, 839–851. doi: 10.1016/j.neuron.2009.02.011
Karunaratne, A., Hargrave, M., Poh, A., and Yamada, T. (2002). GATA proteins identify a novel ventral interneuron subclass in the developing chick spinal cord. Dev. Biol. 249, 30–43. doi: 10.1006/dbio.2002.0754
Kerschensteiner, D., Liu, H., Cheng, C. W., Demas, J., Cheng, S. H., Hui, C. C., et al. (2008). Genetic control of circuit function: Vsx1 and Irx5 transcription factors regulate contrast adaptation in the mouse retina. J. Neurosci. 28, 2342–2352. doi: 10.1523/JNEUROSCI.4784-07.2008
Kim, E. J., Battiste, J., Nakagawa, Y., and Johnson, J. E. (2008). Ascl1 (Mash1) lineage cells contribute to discrete cell populations in CNS architecture. Mol. Cell. Neurosci. 38, 595–606. doi: 10.1016/j.mcn.2008.05.008
Kimura, Y., Satou, C., and Higashijima, S. (2008). V2a and V2b neurons are generated by the final divisions of pair-producing progenitors in the zebrafish spinal cord. Development 135, 3001–3005. doi: 10.1242/dev.024802
Kuscha, V., Frazer, S. L., Dias, T. B., Hibi, M., Becker, T., and Becker, C. G. (2012). Lesion-induced generation of interneuron cell types in specific dorsoventral domains in the spinal cord of adult zebrafish. J. Comp. Neurol. 520, 3604–3616. doi: 10.1002/cne.23115
Lanuza, G. M., Gosgnach, S., Pierani, A., Jessell, T. M., and Goulding, M. (2004). Genetic identification of spinal interneurons that coordinate left-right locomotor activity necessary for walking movements. Neuron 42, 375–386. doi: 10.1016/s0896-6273(04)00249-1
Li, S., Misra, K., Matise, M. P., and Xiang, M. (2005). Foxn4 acts synergistically with Mash1 to specify subtype identity of V2 interneurons in the spinal cord. Proc. Natl. Acad. Sci. U S A 102, 10688–10693. doi: 10.1073/pnas.0504799102
Li, S., Misra, K., and Xiang, M. (2010). A Cre transgenic line for studying V2 neuronal lineages and functions in the spinal cord. Genesis 48, 667–672. doi: 10.1002/dvg.20669
Li, S., Mo, Z., Yang, X., Price, S. M., Shen, M. M., and Xiang, M. (2004). Foxn4 controls the genesis of amacrine and horizontal cells by retinal progenitors. Neuron 43, 795–807. doi: 10.1016/j.neuron.2004.08.041
Liu, I. S., Chen, J. D., Ploder, L., Vidgen, D., van der Kooy, D., Kalnins, V. I., et al. (1994). Developmental expression of a novel murine homeobox gene (Chx10): evidence for roles in determination of the neuroretina and inner nuclear layer. Neuron 13, 377–393. doi: 10.1016/0896-6273(94)90354-9
Lu, D. C., Niu, T., and Alaynick, W. A. (2015). Molecular and cellular development of spinal cord locomotor circuitry. Front. Mol. Neurosci. 8:25. doi: 10.3389/fnmol.2015.00025
Misra, K., Gui, H., and Matise, M. P. (2008). Prox1 regulates a transitory state for interneuron neurogenesis in the spinal cord. Dev. Dyn. 237, 393–402. doi: 10.1002/dvdy.21422
Misra, K., Luo, H., Li, S., Matise, M., and Xiang, M. (2014). Asymmetric activation of Dll4-Notch signaling by Foxn4 and proneural factors activates BMP/TGFbeta signaling to specify V2b interneurons in the spinal cord. Development 141, 187–198. doi: 10.1242/dev.092536
Moran-Rivard, L., Kagawa, T., Saueressig, H., Gross, M. K., Burrill, J., and Goulding, M. (2001). Evx1 is a postmitotic determinant of v0 interneuron identity in the spinal cord. Neuron 29, 385–399. doi: 10.1016/s0896-6273(01)00213-6
Müller, T., Anlag, K., Wildner, H., Britsch, S., Treier, M., and Birchmeier, C. (2005). The bHLH factor Olig3 coordinates the specification of dorsal neurons in the spinal cord. Genes Dev. 19, 733–743. doi: 10.1101/gad.326105
Nardelli, J., Thiesson, D., Fujiwara, Y., Tsai, F. Y., and Orkin, S. H. (1999). Expression and genetic interaction of transcription factors GATA-2 and GATA-3 during development of the mouse central nervous system. Dev. Biol. 210, 305–321. doi: 10.1006/dbio.1999.9278
Ohtoshi, A., Justice, M. J., and Behringer, R. R. (2001). Isolation and characterization of Vsx1, a novel mouse CVC paired-like homeobox gene expressed during embryogenesis and in the retina. Biochem. Biophys. Res. Commun. 286, 133–140. doi: 10.1006/bbrc.2001.5372
Ohtoshi, A., Wang, S. W., Maeda, H., Saszik, S. M., Frishman, L. J., Klein, W. H., et al. (2004). Regulation of retinal cone bipolar cell differentiation and photopic vision by the CVC homeobox gene Vsx1. Curr. Biol. 14, 530–536. doi: 10.1016/j.cub.2004.02.027
Ono, Y., Nakatani, T., Sakamoto, Y., Mizuhara, E., Minaki, Y., Kumai, M., et al. (2007). Differences in neurogenic potential in floor plate cells along an anteroposterior location: midbrain dopaminergic neurons originate from mesencephalic floor plate cells. Development 134, 3213–3225. doi: 10.1242/dev.02879
Panayi, H., Panayiotou, E., Orford, M., Genethliou, N., Mean, R., Lapathitis, G., et al. (2010). Sox1 is required for the specification of a novel p2-derived interneuron subtype in the mouse ventral spinal cord. J. Neurosci. 30, 12274–12280. doi: 10.1523/JNEUROSCI.2402-10.2010
Panayiotou, E., Panayi, E., Lapathitis, G., Francius, C., Clotman, F., Kessaris, N., et al. (2013). Pax6 is expressed in subsets of V0 and V2 interneurons in the ventral spinal cord in mice. Gene Expr. Patterns 13, 328–334. doi: 10.1016/j.gep.2013.06.004
Parras, C. M., Hunt, C., Sugimori, M., Nakafuku, M., Rowitch, D., and Guillemot, F. (2007). The proneural gene Mash1 specifies an early population of telencephalic oligodendrocytes. J. Neurosci. 27, 4233–4242. doi: 10.1523/JNEUROSCI.0126-07.2007
Passini, M. A., Kurtzman, A. L., Canger, A. K., Asch, W. S., Wray, G. A., Raymond, P. A., et al. (1998). Cloning of zebrafish vsx1: expression of a paired-like homeobox gene during CNS development. Dev. Genet. 23, 128–141. doi: 10.1002/(SICI)1520-6408(1998)23:2<128::AID-DVG5>3.3.CO;2-Q
Pelosi, B., Migliarini, S., Pacini, G., Pratelli, M., and Pasqualetti, M. (2014). Generation of Pet1210-Cre transgenic mouse line reveals non-serotonergic expression domains of Pet1 both in CNS and periphery. PLoS One 9:e104318. doi: 10.1371/journal.pone.0104318
Peng, C. Y., Yajima, H., Burns, C. E., Zon, L. I., Sisodia, S. S., Pfaff, S. L., et al. (2007). Notch and MAML signaling drives Scl-dependent interneuron diversity in the spinal cord. Neuron 53, 813–827. doi: 10.1016/j.neuron.2007.02.019
Pierani, A., Moran-Rivard, L., Sunshine, M. J., Littman, D. R., Goulding, M., and Jessell, T. M. (2001). Control of interneuron fate in the developing spinal cord by the progenitor homeodomain protein Dbx1. Neuron 29, 367–384. doi: 10.1016/s0896-6273(01)00212-4
Ross, S. E., McCord, A. E., Jung, C., Atan, D., Mok, S. I., Hemberg, M., et al. (2012). Bhlhb5 and Prdm8 form a repressor complex involved in neuronal circuit assembly. Neuron 73, 292–303. doi: 10.1016/j.neuron.2011.09.035
Roy, A., Francius, C., Rousso, D. L., Seuntjens, E., Debruyn, J., Luxenhofer, G., et al. (2012). Onecut transcription factors act upstream of Isl1 to regulate spinal motoneuron diversification. Development 139, 3109–3119. doi: 10.1242/dev.078501
Sapir, T., Geiman, E. J., Wang, Z., Velasquez, T., Mitsui, S., Yoshihara, Y., et al. (2004). Pax6 and engrailed 1 regulate two distinct aspects of renshaw cell development. J. Neurosci. 24, 1255–1264. doi: 10.1523/JNEUROSCI.3187-03.2004
Shi, Z., Jervis, D., Nickerson, P. E., and Chow, R. L. (2012). Requirement for the paired-like homeodomain transcription factor VSX1 in type 3a mouse retinal bipolar cell terminal differentiation. J. Comp. Neurol. 520, 117–129. doi: 10.1002/cne.22697
Shi, Z., Trenholm, S., Zhu, M., Buddingh, S., Star, E. N., Awatramani, G. B., et al. (2011). Vsx1 regulates terminal differentiation of type 7 ON bipolar cells. J. Neurosci. 31, 13118–13127. doi: 10.1523/JNEUROSCI.2331-11.2011
Skaggs, K., Martin, D. M., and Novitch, B. G. (2011). Regulation of spinal interneuron development by the Olig-related protein Bhlhb5 and Notch signaling. Development 138, 3199–3211. doi: 10.1242/dev.057281
Smith, E., Hargrave, M., Yamada, T., Begley, C. G., and Little, M. H. (2002). Coexpression of SCL and GATA3 in the V2 interneurons of the developing mouse spinal cord. Dev. Dyn. 224, 231–237. doi: 10.1002/dvdy.10093
Stam, F. J., Hendricks, T. J., Zhang, J., Geiman, E. J., Francius, C., Labosky, P. A., et al. (2012). Renshaw cell interneuron specialization is controlled by a temporally restricted transcription factor program. Development 139, 179–190. doi: 10.1242/dev.071134
Struhl, G., and Greenwald, I. (2001). Presenilin-mediated transmembrane cleavage is required for Notch signal transduction in Drosophila. Proc. Natl. Acad. Sci. U S A 98, 229–234. doi: 10.1073/pnas.011530298
Talpalar, A. E., Bouvier, J., Borgius, L., Fortin, G., Pierani, A., and Kiehn, O. (2013). Dual-mode operation of neuronal networks involved in left-right alternation. Nature 500, 85–88. doi: 10.1038/nature12286
Tomita, K., Nakanishi, S., Guillemot, F., and Kageyama, R. (1996). Mash1 promotes neuronal differentiation in the retina. Genes Cells 1, 765–774. doi: 10.1111/j.1365-2443.1996.tb00016.x
Welcker, J. E., Hernandez-Miranda, L. R., Paul, F. E., Jia, S., Ivanov, A., Selbach, M., et al. (2013). Insm1 controls development of pituitary endocrine cells and requires a SNAG domain for function and for recruitment of histone-modifying factors. Development 140, 4947–4958. doi: 10.1242/dev.097642
Wildner, H., Muller, T., Cho, S. H., Bröhl, D., Cepko, C. L., Guillemot, F., et al. (2006). dILA neurons in the dorsal spinal cord are the product of terminal and non-terminal asymmetric progenitor cell divisions, and require Mash1 for their development. Development 133, 2105–2113. doi: 10.1242/dev.02345
Wong, P. C., Zheng, H., Chen, H., Becher, M. W., Sirinathsinghji, D. J., Trumbauer, M. E., et al. (1997). Presenilin 1 is required for Notch1 and DII1 expression in the paraxial mesoderm. Nature 387, 288–292. doi: 10.1038/387288a0
Xiang, M., and Li, S. (2013). Foxn4: a multi-faceted transcriptional regulator of cell fates in vertebrate development. Sci. China Life Sci. 56, 985–993. doi: 10.1007/s11427-013-4543-8
Zagoraiou, L., Akay, T., Martin, J. F., Brownstone, R. M., Jessell, T. M., and Miles, G. B. (2009). A cluster of cholinergic premotor interneurons modulates mouse locomotor activity. Neuron 64, 645–662. doi: 10.1016/j.neuron.2009.10.017
Zhang, J., Lanuza, G. M., Britz, O., Wang, Z., Siembab, V. C., Zhang, Y., et al. (2014). V1 and v2b interneurons secure the alternating flexor-extensor motor activity mice require for limbed locomotion. Neuron 82, 138–150. doi: 10.1016/j.neuron.2014.02.013
Zhang, Y., Narayan, S., Geiman, E., Lanuza, G. M., Velasquez, T., Shanks, B., et al. (2008). V3 spinal neurons establish a robust and balanced locomotor rhythm during walking. Neuron 60, 84–96. doi: 10.1016/j.neuron.2008.09.027
Zhou, Y., Yamamoto, M., and Engel, J. D. (2000). GATA2 is required for the generation of V2 interneurons. Development 127, 3829–3838.
Keywords: Vsx1, V2 interneurons, spinal cord, embryonic development, neural differentiation, spinal locomotor circuits
Citation: Francius C, Hidalgo-Figueroa M, Debrulle S, Pelosi B, Rucchin V, Ronellenfitch K, Panayiotou E, Makrides N, Misra K, Harris A, Hassani H, Schakman O, Parras C, Xiang M, Malas S, Chow RL and Clotman F (2016) Vsx1 Transiently Defines an Early Intermediate V2 Interneuron Precursor Compartment in the Mouse Developing Spinal Cord. Front. Mol. Neurosci. 9:145. doi: 10.3389/fnmol.2016.00145
Received: 30 July 2016; Accepted: 30 November 2016;
Published: 26 December 2016.
Edited by:
Jean-Marc Taymans, French Institute of Health and Medical Research (INSERM), FranceReviewed by:
Alice Davy, Paul Sabatier University, FrancePhilip Forsyth Copenhaver, Oregon Health & Science University, USA
Copyright © 2016 Francius, Hidalgo-Figueroa, Debrulle, Pelosi, Rucchin, Ronellenfitch, Panayiotou, Makrides, Misra, Harris, Hassani, Schakman, Parras, Xiang, Malas, Chow and Clotman. This is an open-access article distributed under the terms of the Creative Commons Attribution License (CC BY). The use, distribution and reproduction in other forums is permitted, provided the original author(s) or licensor are credited and that the original publication in this journal is cited, in accordance with accepted academic practice. No use, distribution or reproduction is permitted which does not comply with these terms.
*Correspondence: Frédéric Clotman, ZnJlZGVyaWMuY2xvdG1hbkB1Y2xvdXZhaW4uYmU=
†Present address: Cédric Francius, Équipe Développement des Circuits Neuronaux, Institut du Fer á Moulin (IFM), Inserm UMRS-839, Université Pierre and Marie Curie, Paris, France
María Hidalgo-Figueroa, Neuropsychopharmacology Research Group, University of Cadiz, Cadiz, Spain
‡ These authors have contributed equally to this work.