- National Centre for Biological Sciences, Tata Institute of Fundamental Research, Bangalore, India
Inositol 1,4,5-trisphosphate receptors (IP3R) are Ca2+ channels on the neuronal endoplasmic reticulum (ER) membrane. They are gated by IP3, produced upon external stimulation and activation of G protein-coupled receptors on the plasma membrane (PM). IP3-mediated Ca2+ release, and the resulting depletion of the ER store, triggers entry of extracellular Ca2+ by store-operated Ca2+ entry (SOCE). Mutations in IP3R attenuate SOCE. Compromised IP3R function and SOCE during pupal development of Drosophila leads to flight deficits and mimics suppression of neuronal activity during pupal or adult development. To understand the effect of compromised IP3R function on pupal neuronal calcium signaling, we examined the effects of mutations in the IP3R gene (itpr) on Ca2+ signals in cultured neurons derived from Drosophila pupae. We observed increased spontaneous Ca2+ influx across the PM of isolated pupal neurons with mutant IP3R and also a loss of SOCE. Both spontaneous Ca2+ influx and reduced SOCE were reversed by over-expression of dOrai and dSTIM, which encode the SOCE Ca2+ channel and the ER Ca2+-sensor that regulates it, respectively. Expression of voltage-gated Ca2+ channels (cac, Ca-α1D and Ca-αT) was significantly reduced in itpr mutant neurons. However, expression of trp mRNAs and transient receptor potential (TRP) protein were increased, suggesting that TRP channels might contribute to the increased spontaneous Ca2+ influx in neurons with mutant IP3R. Thus, IP3R/SOCE modulates spontaneous Ca2+ influx and expression of PM Ca2+ channels in Drosophila pupal neurons. Spontaneous Ca2+ influx compensates for the loss of SOCE in Drosophila itpr mutant neurons.
Introduction
Inositol 1, 4, 5-trisphosphate receptors (IP3R) are Ca2+ channels on the neuronal endoplasmic reticulum (ER) membrane. IP3Rs are gated by the second messenger IP3, which is produced upon external stimulation and activation of G protein-coupled receptors (GPCRs) on the plasma membrane (PM). IP3-mediated Ca2+ release, and the resulting depletion of the ER store, triggers entry of extracellular Ca2+ by store-operated Ca2+ entry (SOCE). Drosophila mutants for the IP3R are flightless (Banerjee et al., 2004). Pan-neuronal knockdown of itpr (the gene for IP3R) and of genes encoding other calcium signaling molecules, such as GPCRs and the SOCE molecules, dSTIM and dOrai also result in flight deficits (Venkiteswaran and Hasan, 2009; Agrawal et al., 2010, 2013). These and other studies have shown that maturation of the flight circuit during pupal development requires intracellular calcium signaling through the IP3R followed by SOCE. This signaling is initiated by GPCRs and affects the transcriptional profile of developing flight circuit neurons (Agrawal et al., 2010, 2013; Pathak et al., 2015). Flight deficits in Drosophila with reduced intracellular calcium signaling during pupal stages, also correlate with reduced levels of Tyrosine Hydroxylase in dopaminergic neurons (Pathak et al., 2015), suggesting that calcium signaling through the IP3R in pupal neurons modulate neurotransmitter levels in adult Drosophila. In vertebrate neurons, neurotransmitter specification is modulated by calcium signaling through voltage-gated Ca2+ channels (VGCCs) and can be decoded by the frequency and amplitude of spontaneous Ca2+ transients (Spitzer et al., 2005; Dulcis et al., 2013); lower for excitatory neurotransmitters and higher for inhibitory neurotransmitters. Spontaneous Ca2+ signals (transients and sustained) in Drosophila pupal neurons are also mediated through VGCCs (Jiang et al., 2005; Iniguez et al., 2013). The TRPC class of PM Ca2+ channels function as polymodal cellular sensors and mediate changes in membrane voltage and intracellular calcium signals. In the developing Xenopus spinal cord TRPC channels are responsible for Ca2+ spike activity (Belgacem and Borodinsky, 2011). However a role for TRPC in generating spontaneous Ca2+ signals in Drosophila neurons is unknown.
Spontaneous Ca2+ oscillations in Drosophila occur in intact as well as in isolated brains, indicating that these signals are independent of sensory inputs (Rosay et al., 2001). In mushroom body Kenyon cells, frequency of spontaneous Ca2+ transients in isolated pupal neurons is similar to Ca2+ transients in vivo (Jiang et al., 2005). Here we have investigated the nature of spontaneous Ca2+ signals in cultured Drosophila pupal neurons where IP3/SOCE mediated intracellular Ca2+ signaling is disrupted. We show that pupal neurons from itpr mutants exhibit aberrantly high spontaneous Ca2+ influx and reduced SOCE. We propose that in itpr mutant neurons, higher spontaneous Ca2+ influx functions as a compensatory mechanism for decreased SOCE and helps maintain intracellular Ca2+ homeostasis. A possible source of the compensatory spontaneous Ca2+ influx appears to be the TRP channel.
Materials and Methods
Drosophila Strains
Single point mutants in the itpr gene were generated in an EMS (ethyl methane sulfonate) screen; detailed molecular information on these alleles has been published (Joshi et al., 2004; Srikanth et al., 2004). The UAS transgenic strains used have been published and the appropriate references are included in the results. ElavC155GAL4 (pan neuronal) was obtained from Bloomington Stock Center, Indiana University, Bloomington, IN, USA. Canton S (CS), in the background of which all mutant and transgenics were back-crossed, was used as the wild-type control. RNAi lines for itpr (1063) was obtained from National Institute of Genetics, Japan, and for dSTIM (47073), cac (104186), Ca-β (102188), Ca-α1D (51491) and Ca-α1T (48008, 31961) were obtained from Vienna Drosophila Resource Centre, Austria. Fly strains used in this study were generated by standard genetic methods using individual mutant and transgenic fly lines described above.
Primary Neuronal Culture
The protocol for pupal neuronal culture was adapted from Sicaeros and O’Dowd (2007). The brain and ventral ganglion complexes were dissected from different pupal stages of the appropriate genotypes. Dissected brain tissues were incubated for 15 min at RT with 50 U/ml papain activated by 1.32 mM cysteine in dissecting saline (5.4 mM KCl/137 mM NaCl/0.22 mM KH2PO4/0.17 mM NaH2PO4/43.8 mM sucrose/33.3 mM glucose/9.9 mM HEPES, pH 7.3 with NaOH). The brain tissue was dissociated with gentle pipetting. The lysate containing a mixture of tissue clumps and single cells was spun down, re-suspended and plated onto poly lysine-coated glass coverslip mounted to the bottom of a petri dish. Cells were resuspended and cultured in DMEM/F12-1065 (Life Technologies, Carlsbad, CA, USA), containing Glutamax-I, 2.438 sodium bicarbonate and sodium pyruvate, supplemented with 50 U/ml penicillin (Life Technologies, Carlsbad, CA, USA), 50 μg/ml streptomycin (Life Technologies, Carlsbad, CA, USA), and 10 μg/ml amphotericin B (Life Technologies, Carlsbad, CA, USA), 1 mg/ml sodium bicarbonate, 20 mM HEPES, 100 μM putrescine, 20 ng/ml progesterone, 50 μg/ml insulin, 1 μg/ml 20-hydroxyecdysone. The cells were incubated at 25°C in a humidified incubator with 5% CO2 for 14–16 h. All chemicals for cell culture were obtained from Sigma-Aldrich (St. Louis, MO, USA) unless otherwise stated.
Calcium Imaging
For measurement of spontaneous calcium influx and SOCE cells were incubated with 2.5 μM Fluo-4 AM and 0.02% Pluronic F-127 in M1 media for 30 min at room temperature in the dark. Cells were washed twice with M1 before and after dye incubation and finally covered with M1 (30 mM HEPES/150 mM NaCl/5 mM KCl/2 mM MgCl2/35 mM sucrose, pH 7.2 with NaOH) with (1 mM CaCl2) or without (2 mM EGTA) Ca2+. Data were acquired using 488 nm excitation and 520 nm emission filter sets at 15 s intervals. An Olympus IX81-ZDC2 inverted wide field microscope with epifluorescence and Z-drift compensation and a 60×/1.35 NA (oil) objective lens, was used for calcium imaging. Excitation of fluorescent Ca2+ indicator dyes was performed using specific wavelength illuminations from a halogen arc lamp with TILL Polychrome 5000 monochromator (TILL Photonics, Graefelfing, Germany) for variable bandwidth and intensity. Emitted light was detected through band pass filter sets (Chroma, Brattleboro, VT, USA). Image acquisition was performed using the Andor iXON 897E EMCCD camera and Andor iQ 2.4.2 imaging software. The time lapse acquisition mode of the software was used to follow fluorescence changes over time.
Analysis of Calcium Imaging Data
Images acquired through Andor iQ 2.4.2 on Olympus IX81-ZDC2 were analyzed with ImageJ 1.43m (NIH, Bethesda, MD, USA). Each cell was marked separately and mean fluorescence intensity was calculated in arbitrary units. Arbitrary units of fluorescence for each cell were converted to ΔF/F0 values and the highest ΔF/F0 values were tabulated; ΔF/F0 values for each assay and each genotype were plotted as a box plot or cumulative frequency distribution plot in Origin 8.0 software (Origin Lab, Northampton, MA, USA). To compare data between genotypes or assay conditions Kruskal-Wallis test for variance followed by Wilcoxon post hoc test was performed.
Quantitative PCR
Total RNA was isolated from 5 to 10 dissected CNS from suitably aged Drosophila pupae with TRIzol reagent (Invitrogen, Life Technologies, Carlsbad, CA, USA) following manufacturer’s instructions. RNA was dissolved in nuclease free water and quantified using a nanodrop machine (Thermo Scientific, Wilmington, DC, USA) and the integrity was checked on a 1.5% TAE gel. Approximately 200 ng RNA was used for cDNA preparation by Reverse Transcriptase as described in Pathak et al. (2015). Quantitative PCR (qPCR) was performed by ABI 7500 fast machine operated by ABI 7500 software using KAPA™ SYBR® FAST qPCR master mix (Kapa Biosystems, Wilmington, MA, USA) for SYBR assay I dTTP (Eurogentec, Belgium). For each genotype, three biological replicates and two technical replicates were included in the experiment. rp49 was used as internal control. A melt curve was performed after the assay to check for specificity of the reaction. The fold change of gene expression in test genotype relative to control was determined by the comparative DDCt, where DDCt = (Cttarget − Ctp49)test − (Cttarget − Ctrp49)control.
Quantitative Western Blots
Pupal CNS lysates were extracted in 50 mM Tris (pH 8), 150 mM NaCl, 1 mM EGTA and 1% (v/v) Triton X-100 with 1 mM PMSF, 0.5 μM E64 Calpain inhibitor and 100 μg/ml leupeptin. Protein extract was boiled for 5 min at 95°C in 125 mM Tris pH 6.8, 5% (v/v) Glycerol, 0.25% (w/v) SDS, 2% (v/v) β-mercaptoethanol, 10% (w/v) Bromophenol blue and resolved in 8% SDS polyacrylamide gel electrophoresis. Proteins were detected with 1:5000 anti-TRP (kind gift from Prof. Raghu Padinjat, NCBS).
Flight Test
Flies were anesthetized in ice before tethering to a tungsten wire between the head and thorax and allowed to recover for 30 min before recording flight. Flight was stimulated with a puff of air and recorded for 30 s.
Results
Pupal Neurons with Mutant IP3R Exhibit Increased Spontaneous Ca2+ Signals and Reduced Store-Operated Ca2+ Entry
To explore the role of IP3-mediated Ca2+ signaling in spontaneous Ca2+ influx and SOCE in Drosophila pupal neurons, primary neurons from the central nervous systems of 48–50 h pupae were cultured for 16–18 h and Ca2+ signals of single neurons were recorded. In presence of 2 mM extracellular CaCl2 wild type and itpr mutant neurons displayed spontaneous Ca2+ signals in culture (Figures 1A,B). The amplitude of signals was significantly higher in neurons with mutant IP3Rs (S224F/G1891S and S224F/G2117E; heteroallelic combinations) as compared with wild type (P < 0.01, Kruskal-Wallis test of variation followed by Wilcoxon post hoc test; Figures 1A,B,D, 2D). The spontaneous Ca2+ signals observed in both genotypes were sustained rather than transients. Spontaneous Ca2+ signals were not observed in minimal extracellular Ca2+ (8–11 nM) and after application of either 50 μM lanthanum chloride (Prakriya and Lewis, 2001) or 2 mM cobalt chloride (Jiang et al., 2005) in presence of 2 mM extracellular CaCl2 (Figure 1C), suggesting that the spontaneous Ca2+ signals observed were due to a Ca2+ influx across the PM and not from intracellular stores. This observation is consistent with published data reporting an absence of spontaneous Ca2+ transients in the absence of extracellular Ca2+ in cultured Drosophila pupal neurons of mushroom body Kenyon cells (Jiang et al., 2005). However, the frequency and amplitude of spontaneous Ca2+-signals observed in our study differed from what was reported in mushroom body Kenyon cells (Jiang et al., 2005), possibly due to variations in spontaneous Ca2+-signals in specific subtypes of neurons and culture conditions.
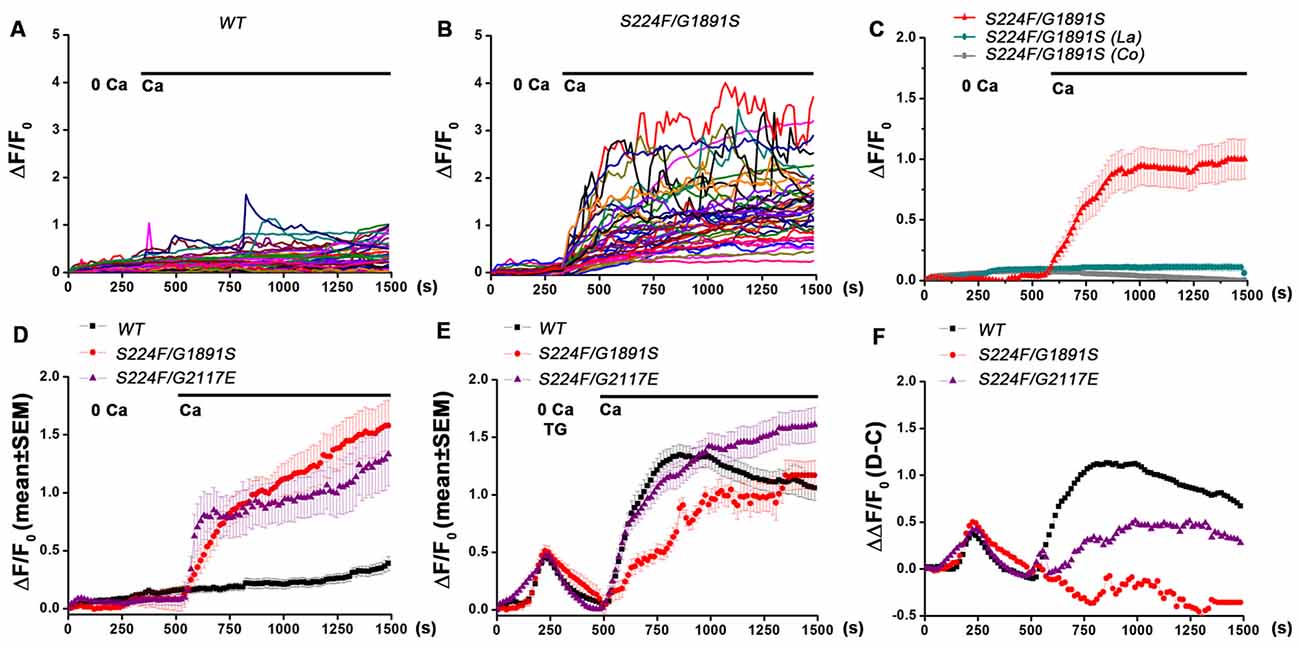
Figure 1. Drosophila pupal neurons with mutant IP3R exhibit increased spontaneous Ca2+ signals and reduced store-operated Ca2+ entry (SOCE). (A,B) Traces showing spontaneous Ca2+ signals in individual pupal neurons in absence and presence of extracellular Ca2+ in wild type and itpr mutant respectively. (C) Mean spontaneous Ca2+ signals in itpr mutant (S224F/G1891S) neurons in presence of La and Co with extracellular Ca2+. (D) Mean spontaneous Ca2+ signals in wild type and itpr mutant neurons. (E) Mean Ca2+ signals in wild type and itpr mutant neurons due to store depletion with thapsigargin followed by SOCE. (F) Ca2+ signals for SOCE after subtracting spontaneous Ca2+ influx from Ca2+ influx induced by store-Ca2+ depletion with thapsigargin (mean data obtained from several separate experiments with same time intervals for recording). N > 100 cells.
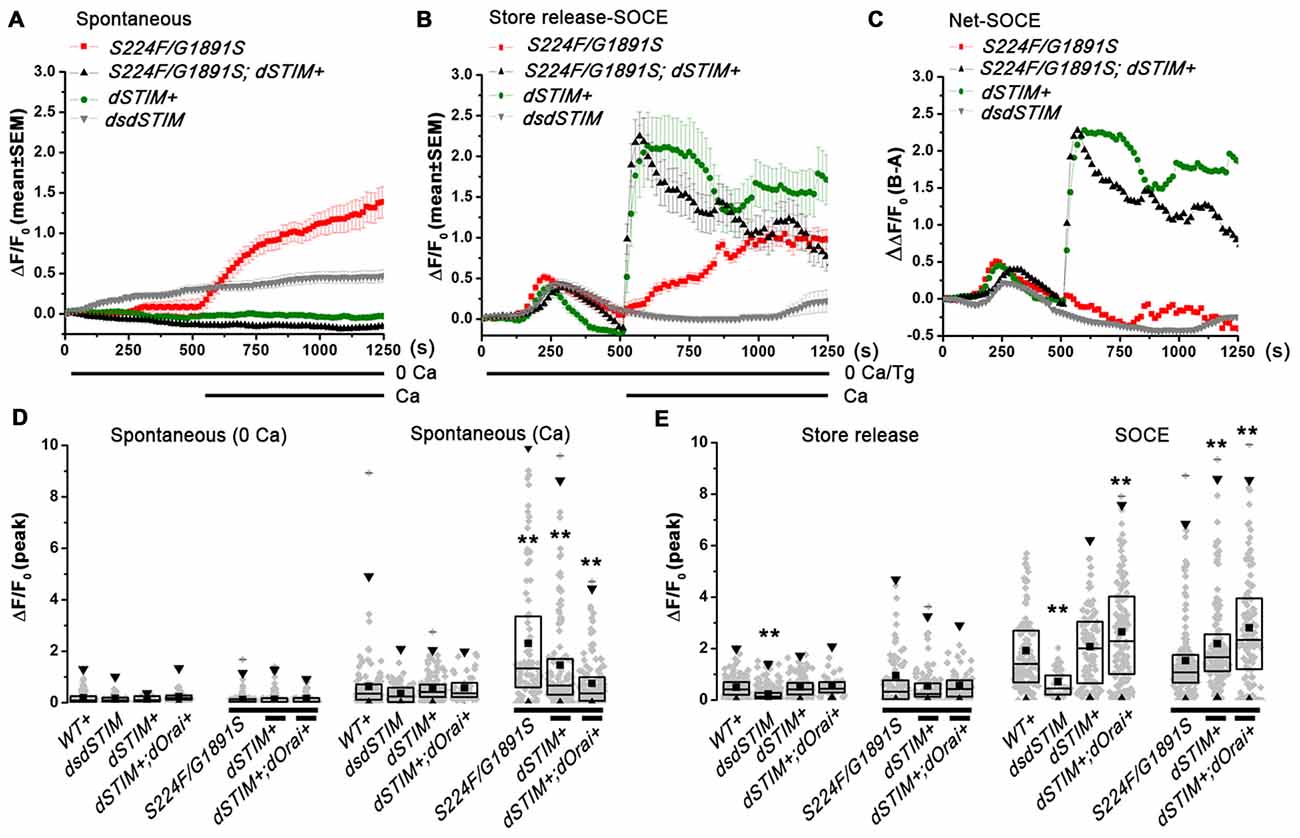
Figure 2. Aberrant spontaneous Ca2+ influx and SOCE in itpr mutant pupal neurons can be rescued by overexpression of dSTIM and dOrai. (A,B) Traces showing spontaneous Ca2+ signals and thapsigargin-Ca2+ signals respectively (mean ± SEM) in pupal neurons (N > 100). (C) Ca2+ signals for SOCE after subtracting spontaneous Ca2+ influx (A) from Ca2+ influx induced by store-Ca2+ depletion with thapsigargin (B). (D,E) Box plot representing peak ΔF/F0 with individual values for single neurons, mean, median and 25–75 quartiles for spontaneous Ca2+ influx, thapsigargin induced store depletion and SOCE, N > 100 cells, P > 0.01 compared to wild type or the mutant, Kruskal-Wallis test for variance followed by Wilcoxon post hoc test. **P < 0.01.
Drosophila larval neurons cultured from itpr mutants (S224F/G1891S; heteroallelic combination) displayed reduced SOCE after passive depletion of intracellular store-Ca2+ with 10 μM thapsigargin (Venkiteswaran and Hasan, 2009; Chakraborty and Hasan, 2012a, b; Chakraborty et al., 2016). To understand if SOCE is also compromised in pupal neurons from itpr mutants, intracellular store-Ca2+ was depleted with 10 μM thapsigargin in minimal extracellular Ca2+ (8–11 nM) followed by measurement of SOCE by replenishing 2 mM extracellular CaCl2. Because itpr mutant pupal neurons displayed higher spontaneous Ca2+ influx as compared to wild type in presence of extracellular Ca2+, SOCE was determined by subtracting spontaneous Ca2+ influx from Ca2+ influx induced by store-Ca2+ depletion with thapsigargin (Figure 1E). As in larval neurons, SOCE was significantly (P < 0.01, Kruskal-Wallis test of variation followed by Wilcoxon post hoc test) reduced in itpr mutant pupal neurons (Figure 1F).
Over-Expression of dSTIM/dOrai in itpr Mutant Neurons Restores Normal Spontaneous Ca2+ Influx and Store-Operated Ca2+ Entry
To understand if spontaneous Ca2+ influx in pupal neurons is affected by SOCE, spontaneous Ca2+ influx and SOCE was measured in pupal neurons over-expressing dSTIM and dOrai in presence or absence of mutant IP3Rs (Figure 2). Higher spontaneous Ca2+ influx was significantly reduced by over-expression of dSTIM (Figures 2A,D) and dOrai (Figure 2D) in itpr mutant neurons, suggesting that their higher spontaneous Ca2+ influx is a compensatory increase for reduced SOCE. This idea was tested further, by measuring spontaneous Ca2+ influx and SOCE in pupal neurons with knock down of dSTIM. Knock down of dSTIM (dsdSTIM) reduced SOCE in pupal neurons (Figures 2C,D); however, it did not result in significant up regulation of spontaneous Ca2+ influx (Figures 2A,D). Thus the higher spontaneous Ca2+ influx in itpr mutant neurons appears to be an adaptive effect of recurrent loss of IP3R function rather than a response to loss of SOCE. Loss of SOCE in itpr mutant larval neurons can be restored by over-expression of dSTIM and dOrai (Agrawal et al., 2010). Over-expressing dSTIM and dOrai in wild type and itpr mutant pupal neurons also increased SOCE after store-Ca2+ depletion with thapsigargin (Figures 2B,C,E). As published in larval neurons (Chakraborty et al., 2016), over-expression of dSTIM and dOrai in itpr mutant neurons did not alter store-Ca2+ depletion in pupal neurons. However, knock down of dSTIM attenuated intracellular store Ca2+ release presumably due to reduced store [Ca2+] (Figures 2B,C,E). These data suggest that loss of SOCE, in absence of a compensatory increase in spontaneous Ca2+ influx as seen in neurons with dSTIM knock-down (Figure 2A), leads to reduced Ca2+ in intracellular stores. This reiterates that increased spontaneous Ca2+ entry in itpr mutant neurons helps refill ER store in the absence of SOCE.
IP3R Function/SOCE Regulate Expression of PM Ca2+ Channels in Drosophila Pupal Neurons
To identify PM Ca2+ channels that contribute to higher spontaneous Ca2+ influx in Drosophila itpr mutant pupal neurons, we performed quantitative PCR analysis of mRNA encoding subunits of VGCCs (Ca-β, cacophony/cac, Ca-α1D and Ca-α1T), TRP channels (trp, trpl) and NCX (calx) in extracts derived from 48 h to 50 h pupal central nervous systems. The choice of candidates investigated was based on existing literature (Gu et al., 2009; Iniguez et al., 2013; Kanamori et al., 2013). The time window selected was based on previous data on the requirement of IP3R function and SOCE for the development of the Drosophila flight circuit (Banerjee et al., 2004; Richhariya et al., 2017) and on the onset of spontaneous Ca2+ transients in Drosophila pupal neurons (Jiang et al., 2005). Spontaneous Ca2+ transients in distinct subsets of Drosophila pupal neurons (in vivo and in 2 day old cultures) have been attributed to PLTX-II sensitive P/Q-type VGCC, cac (Jiang et al., 2005; Gu et al., 2009) or to T-type VGCC, Ca-αT (Iniguez et al., 2013). An increased activity of voltage-activated transient K+ current IA was observed in cac mutant neurons, associated with an increased expression of Shaker, gene for IA, and a corresponding reduction in the expression of Slowpoke, gene for [IK(Ca)] (Peng and Wu, 2007). Hence expression levels of K+ channel Slowpoke and Shaker were also analyzed as reporters for VGCC activity. Surprisingly, a significant reduction in cacophony/cac, Ca-α1D and Ca-αT mRNA levels was observed in itpr mutant neurons with a corresponding reduction in Slowpoke mRNA. Expression of Ca-β and Shaker, however, was not significantly altered (P < 0.05, Student’s t-test; Figure 3A). These data suggest that higher spontaneous Ca2+ influx in itpr mutant neurons is very likely not mediated by VGCCs. On the contrary, the normal expression of VGCCs correlates with normal IP3R function.
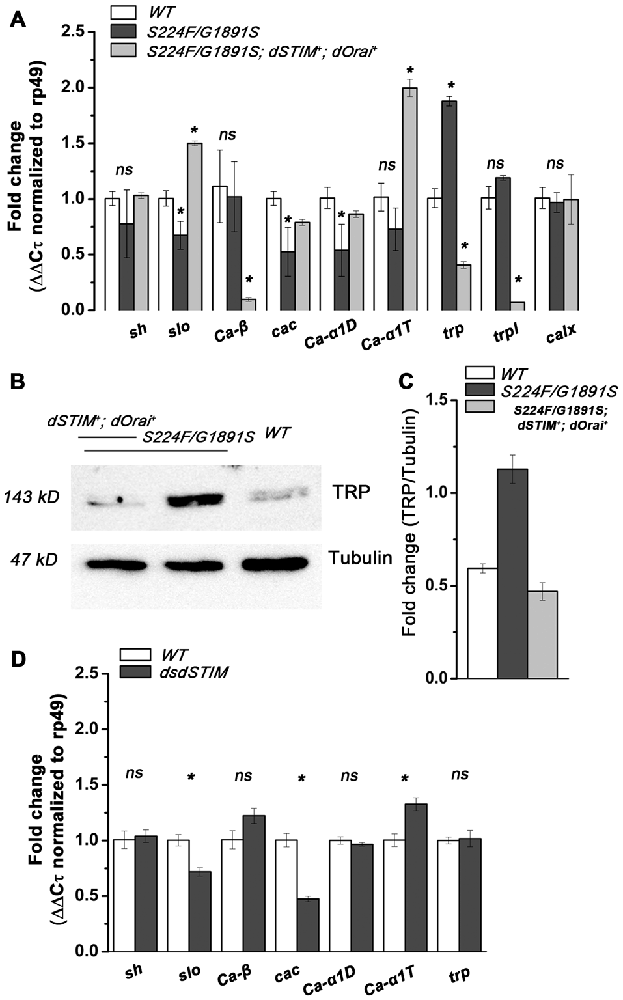
Figure 3. IP3R function/SOCE regulates expression of plasma membrane (PM) Ca2+ channels in Drosophila pupal neurons. (A) Fold change (ΔΔCτ normalized to rp49 mRNA, mean ± SEM) of mRNA levels for Slowpoke and Shaker, subunits of VGCCs (Ca-β, cacophony/cac, Ca-α1D and Ca-αT), TRP channels (trp, trpl) and NCX (calx) in extracts derived from 48–50 h pupal central nervous system in the respective genotypes, N = 3, Student’s t-test. (B) Representative western blot showing expression of TRP in the respected genotypes. (C) Fold change of TRP protein expression (mean ± SEM) in the respective genotypes, N = 3, Student’s t-test. (D) Fold change (ΔΔCτ normalized to rp49 mRNA, mean ± SEM) of mRNA levels for Slowpoke and Shaker, subunits of VGCCs (Ca-β, cacophony/cac, Ca-α1D and Ca-αT) and TRP in the respective genotypes, N = 3, Student’s t-test. *P < 0.05.
The TRPC class of PM Ca2+ channels are responsible for Ca2+ spike activity in developing Xenopus spinal cord, where Shh signaling induces synchronous Ca2+ spikes and IP3 transients at the primary cilium (Belgacem and Borodinsky, 2011). NCX (Na+/Ca2+ exchanger) expression is indirectly modulated by SOCE, through ERK1/2, in neuronal PC12 (Sirabella et al., 2012) and rat parotid acinar cells (Soltoff and Lannon, 2013). Therefore, the levels for mRNAs encoding two Drosophila TRPC proteins; TRP and TRPL (Thebault et al., 2005) and a Drosophila NCX homolog, CalX (Wu et al., 2011) were tested. A significant increase (P < 0.05, Student’s t-test) in mRNA levels of trp was observed in itpr mutant neurons, whereas expression levels of trpl and calx remained unchanged (Figure 3A). Over-expression of TRP in the pupal nervous system of itpr mutants was confirmed by measuring expression of TRP protein directly (Figures 3B,C).
Interestingly, rescue of SOCE by over-expression of dSTIM and dOrai in itpr mutant neurons reverted the change in mRNA levels of cacophony/cac, Ca-α1D, Slowpoke and trp (P < 0.05, Student’s t-test; Figure 3A). Moreover, expression of Ca-α1T and trpl (P < 0.05, Student’s t-test; Figure 3A) was also altered significantly suggesting that raising SOCE in itpr mutant neurons, by overexpression of dSTIM and dOrai, altered gene expression of VGCCs and TRPs. To understand if expression levels of cacophony/cac, Ca-α1D, Slowpoke and trp were directly sensitive to SOCE, mRNA levels were analyzed from pupal neurons with down regulation of dSTIM (Figure 3D). A significant (P < 0.05, Student’s t-test) down regulation of cac and Slowpoke was observed in dSTIM knocked down neurons whereas expression levels of Shaker, Ca-β, Ca-α1D and trp, remained unchanged. These data suggest that the expression of cac and slo are sensitive to SOCE. The reduced expression of Slowpoke possibly reports reduced activity of the P/Q-type VGCC encoded by cac, as reported earlier for cac mutant neurons (Peng and Wu, 2007). However, it is also possible that Slowpoke expression in this case is directly regulated by SOCE rather than through reduced activity of VGCCs. The absence of TRP up-regulation, as well as normal spontaneous Ca2+ influx in neurons with dSTIM knock-down supports higher expression of TRP in itpr mutant neurons as a likely cause for higher spontaneous Ca2+ influx. Over-expression of TRP thus appears to be sensitive to recurrent loss of IP3R function rather than loss of SOCE in Drosophila pupal neurons. These data support a central role for the IP3R in maintaining calcium homeostasis in Drosophila pupal neurons.
Knock-Down of VGCCs in Global or Subset of Neurons Does Not Affect Drosophila Flight
Previous results have demonstrated that knock down of either itpr or dSTIM in Drosophila neurons during pupal development leads to flight deficits in adults (Agrawal et al., 2013; Richhariya et al., 2017). Reduced expression of cac in itpr mutant neurons and in neurons with knock-down of dSTIM suggested that cac down regulation may be associated with the observed deficits in Drosophila flight. To test this hypothesis, we measured flight times in animals with knock-down of cac and other subunits of VGCCs in either all or subsets of Drosophila neurons (Figure 4A). Pan-neuronal knock-down of cac (104186) and Ca-α1D (51491) resulted in 100% lethality in late stage 3rd instar larvae and thus these animals could not be tested for flight. Pan-neuronal knock-down of Ca-β (102188) and Ca-α1T (48008, 31961) did not affect either viability or flight. We also tested flight after knock-down of these VGCC subunits in specific neuronal subsets. The RNAi lines used were based on their efficacy as described in published literature (Gu et al., 2009; Iniguez et al., 2013; Kanamori et al., 2013). Knockdowns were performed in dopaminergic, aminergic, glutamatergic, peptidergic and GABA-ergic neuronal subsets, which include neuronal classes in which IP3R and SOCE function has been previously implicated as required for adult flight (Banerjee et al., 2004; Venkiteswaran and Hasan, 2009; Agrawal and Hasan, 2015; Pathak et al., 2015). Flight times were tested up to 30 s after air-puff stimulation of tethered flight and appeared normal in all the knock down genotypes tested. Thus, the observed down regulation of VGCCs (Figures 3A,D) correlates with reduced SOCE (Figure 2E) but does not affect flight circuit function (Figure 4A). Knock-down of TRP in itpr mutant neurons and its effect on spontaneous Ca2+ influx and flight in Drosophila could not be assessed as the recombinant flies with TRP RNAi and itpr mutants didn’t survive.
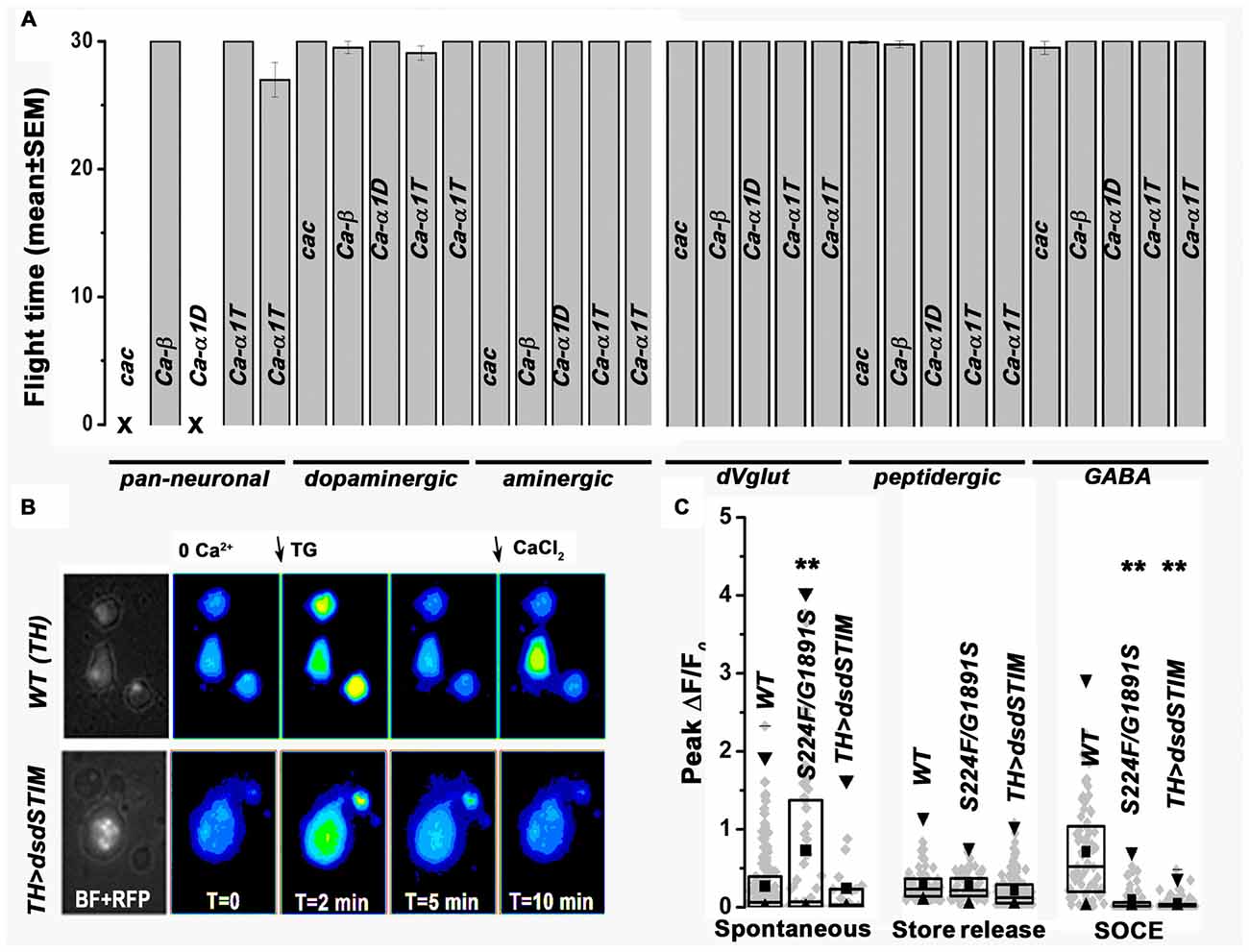
Figure 4. Neuronal knock-down of VGCCs does not affect Drosophila flight. (A) Flight time (mean ± SEM) with knockdown of VGCCs respectively in the mentioned neurons, cac (104186), Ca-β (102188), Ca-α1D (51491) and Ca-α1T (48008, 31961), N > 30 individual flies, P > 0.05, Kruskal-Wallis test for variance followed by Wilcoxon post hoc test. (B) Representative images showing store-depletion by thapsigargin and SOCE in TH positive pupal neurons in the indicated genotypes. (C) Box plot representing peak ΔF/F0 with individual values for single neurons, mean, median and 25–75 quartiles for spontaneous Ca2+ influx, thapsigargin induced store depletion and SOCE respectively, N > 50 cells, P > 0.01, Kruskal-Wallis test for variance followed by Wilcoxon post hoc test. **P < 0.01.
Spontaneous Ca2+ Influx in Pupal Dopaminergic Neurons Is Associated with Loss of Flight
Recent work investigating the role of intracellular calcium signaling in flight has shown that SOCE is required in dopaminergic neuronal subsets for transcriptional maturation of the Drosophila flight circuit during pupal development (Pathak et al., 2015). Therefore, we tested spontaneous Ca2+ influx specifically in dopaminergic neurons of itpr mutant and dSTIM knockdown pupae (Figures 4B,C). As observed in all pan-neuronal populations (Figures 1, 2), spontaneous Ca2+ influx in pupal dopaminergic neurons from a mutant IP3R (S224/G1891S) was significantly higher, whereas SOCE was lower as compared to wild-type dopaminergic neurons (Figure 4C). The IP3R is required in Drosophila neurons during pupal development for flight (Agrawal et al., 2013). Spontaneous Ca2+ signals in Drosophila neurons also originate during late stage pupal development (Jiang et al., 2005). Our data support a model where loss of flight in itpr mutant flies may be an outcome of the up regulation of spontaneous Ca2+ influx during pupal development possibly resulting in an imbalance of excitatory and inhibitory neurotransmitters in the developing flight circuit.
Discussions
Drosophila pupal neurons mutant for IP3R exhibit greater spontaneous Ca2+ influx as compared with wild type (Figures 1A,B,D) and lack SOCE even after equivalent depletion of intracellular store upon passive depletion with thapsigargin (Figures 1E,F). Our results suggest that recurrent loss of IP3R function and SOCE in Drosophila pupal neurons triggers compensatory spontaneous Ca2+ entry to maintain intracellular Ca2+ homeostasis. Restoration of SOCE by over-expression of dSTIM and dOrai restores spontaneous Ca2+ influx to wild type levels in itpr mutant neurons and also rescues flight (Figures 2A–E; Richhariya et al., 2017, respectively). However, pan-neuronal knock down of dSTIM with a GAL4 strain that expresses in post-mitotic neurons (Lin and Goodman, 1994) didn’t result in higher spontaneous Ca2+ entry (Figure 2A), indicating that loss of SOCE alone does not trigger higher spontaneous Ca2+ influx. Previous results from the lab demonstrate that over-expression of dSTIM and dOrai in Drosophila larval neurons could rescue Ca2+ release through IP3R (Agrawal et al., 2010). Thus, restoration of spontaneous Ca2+ influx in Drosophila pupal neurons could be an outcome of restored IP3R function by over-expression of dSTIM and dOrai. The cellular mechanisms underlying these observations need further elucidation. Our data suggest that loss of IP3R function and loss of SOCE, with the consequent reduction in intracellular store Ca2+ in dSTIM knocked down neurons, may influence different aspects of cell function.
We have established that expression of VGCCs and TRP are modulated by IP3R function and can be restored by raising SOCE in Drosophila pupal neurons (Figures 3A–C). Down regulation of SOCE through knock down of dSTIM altered expression of VGCCs, however did not alter expression of TRP (Figure 3D). The functional significance of reduced VGCCs expression in itpr mutant neurons (Figure 3A) is unclear because knock-down of VGCCs in Drosophila neurons (global and subsets) didn’t result in flight deficits (Figure 4A). Our results suggest that higher spontaneous Ca2+ influx in itpr mutant neurons is likely due to up regulation of PM Ca2+ channel TRP, and this may contribute to the observed flight deficits. The mechanism that triggers higher expression as well as spontaneous activation of TRP needs further exploration.
Involvement of dopaminergic neurons in Drosophila flight has been described earlier where reduced SOCE in pupal dopaminergic neurons resulted in reduced expression of Tyrosine Hydroxylase, required for synthesis of dopamine (Pathak et al., 2015). As observed in pan-neuronal population (Figures 1, 2), spontaneous Ca2+ influx in pupal dopaminergic neurons from a mutant IP3R (S224/G1891S; Figure 4C) was significantly higher, whereas SOCE was lower as compared to wild-type dopaminergic neurons (Figure 4C). Knock down of dSTIM (dsdSTIM) in dopaminergic neurons reduced SOCE (Figures 4B,C); however, it did not result in significant up regulation of spontaneous Ca2+ influx (Figure 4C). Thus, higher spontaneous Ca2+ influx in dopaminergic neurons could be one of multiple contributing factors to the flight deficits of Drosophila itpr mutants. It is known that spontaneous Ca2+ influx has a direct role in neurotransmitter specification and motor co-ordination in vertebrate neurons (Spitzer et al., 2005). Possibly, the higher spontaneous Ca2+ influx in itpr mutant neurons is an adaptive effect of dual loss of IP3R function and the accompanying loss of SOCE. Clearly, loss of SOCE alone (by knockdown of dSTIM) does not induce higher spontaneous influx. Compromised IP3R function and SOCE in Drosophila neurons leads to defects in flight motor coordination (Banerjee et al., 2004; Venkiteswaran and Hasan, 2009). However, the effect of IP3R-mediated Ca2+ release on neuronal properties during neural circuit development has not been explored. Here we show that Drosophila pupal neurons mutant for IP3R display higher spontaneous Ca2+ influx, which can be restored by over expression of dSTIM and dOrai. Direct measurement of electrophysiological properties of adult flight circuit neurons is required to understand the effect of increase in spontaneous Ca2+ influx during pupal development on these neurons. Loss of IP3R function in the vertebrate nervous system leads to defects in motor coordination and spino-cerebellar ataxia (SCA); a deletion mutation of ITPR1 in human causes SCA15/16 (van de Leemput et al., 2007; Novak et al., 2010), and the mouse knock out (KO) for Itpr1 displays ataxia (Matsumoto et al., 1996). The connection between IP3R function, spontaneous Ca2+ signals and SOCE provides a novel perspective to address fundamental questions in neurodegenerative diseases and offers new targets for subsequent development of therapeutics.
Author Contributions
SC conceived the project, designed and performed experiments, contributed reagents, analyzed data and interpreted results, prepared figures and drafted the manuscript. GH supervised SC, contributed reagents and critically evaluated the data and the manuscript.
Funding
The work was supported by core funding from National Centre for Biological Sciences (NCBS) to GH. SC was supported by senior research fellowship from Council of Scientific and Industrial Research (CSIR), India and a bridging postdoctoral fellowship from NCBS.
Conflict of Interest Statement
The authors declare that the research was conducted in the absence of any commercial or financial relationships that could be construed as a potential conflict of interest.
Acknowledgments
We thank Prof. Raghu Padinjat (NCBS) for gift of the TRP antibody, Prof. Nicholas Spitzer (University of California, San Diego, La Jolla, CA, USA) for useful discussion on the project and the Central Imaging Facility (CIFF-NCBS) for microscope use.
Abbreviations
IP3R, inositol 1,4,5-trisphosphate receptor; SOCE, store-operated calcium entry; STIM, stromal interaction molecule; TRP, transient receptor potential; VGCCs, voltage gated calcium channels.
References
Agrawal, T., and Hasan, G. (2015). Maturation of a central brain flight circuit in Drosophila requires Fz2/Ca2+ signaling. Elife 4:e07046. doi: 10.7554/elife.07046
Agrawal, T., Sadaf, S., and Hasan, G. (2013). A genetic RNAi screen for IP3/Ca2+; coupled GPCRs in Drosophila identifies the PdfR as a regulator of insect flight. PLoS Genet. 9:e1003849. doi: 10.1371/journal.pgen.1003849
Agrawal, N., Venkiteswaran, G., Sadaf, S., Padmanabhan, N., Banerjee, S., and Hasan, G. (2010). Inositol 1,4,5-trisphosphate receptor and dSTIM function in Drosophila insulin-producing neurons regulates systemic intracellular calcium homeostasis and flight. J. Neurosci. 30, 1301–1313. doi: 10.1523/JNEUROSCI.3668-09.2010
Banerjee, S., Lee, J., Venkatesh, K., Wu, C. F., and Hasan, G. (2004). Loss of flight and associated neuronal rhythmicity in inositol 1,4,5-trisphosphate receptor mutants of Drosophila. J. Neurosci. 24, 7869–7878. doi: 10.1523/JNEUROSCI.0656-04.2004
Belgacem, Y. H., and Borodinsky, L. N. (2011). Sonic hedgehog signaling is decoded by calcium spike activity in the developing spinal cord. Proc. Natl. Acad. Sci. U S A 108, 4482–4487. doi: 10.1073/pnas.1018217108
Chakraborty, S., Deb, B. K., Chorna, T., Konieczny, V., Taylor, C. W., and Hasan, G. (2016). Mutant IP3 receptors attenuate store-operated Ca2+ entry by destabilizing STIM-Orai interactions in Drosophila neurons. J. Cell Sci. 129, 3903–3910. doi: 10.1242/jcs.191585
Chakraborty, S., and Hasan, G. (2012a). Functional complementation of Drosophila itpr mutants by rat Itpr1. J. Neurogenet. 26, 328–337. doi: 10.3109/01677063.2012.697501
Chakraborty, S., and Hasan, G. (2012b). IP3R, store-operated Ca2+ entry and neuronal Ca2+ homoeostasis in Drosophila. Biochem. Soc. Trans. 40, 279–281. doi: 10.1042/BST20110618
Dulcis, D., Jamshidi, P., Leutgeb, S., and Spitzer, N. C. (2013). Neurotransmitter switching in the adult brain regulates behavior. Science 340, 449–453. doi: 10.1126/science.1234152
Gu, H., Jiang, S. A., Campusano, J. M., Iniguez, J., Su, H., Hoang, A. A., et al. (2009). Cav2-type calcium channels encoded by cac regulate AP-independent neurotransmitter release at cholinergic synapses in adult Drosophila brain. J. Neurophysiol. 101, 42–53. doi: 10.1152/jn.91103.2008
Iniguez, J., Schutte, S. S., and O’Dowd, D. K. (2013). Cav3-type α1T calcium channels mediate transient calcium currents that regulate repetitive firing in Drosophila antennal lobe PNs. J. Neurophysiol. 110, 1490–1496. doi: 10.1152/jn.00368.2013
Jiang, S. A., Campusano, J. M., Su, H., and O’Dowd, D. K. (2005). Drosophila mushroom body Kenyon cells generate spontaneous calcium transients mediated by PLTX-sensitive calcium channels. J. Neurophysiol. 94, 491–500. doi: 10.1152/jn.00096.2005
Joshi, R., Venkatesh, K., Srinivas, R., Nair, S., and Hasan, G. (2004). Genetic dissection of itpr gene function reveals a vital requirement in aminergic cells of Drosophila larvae. Genetics 166, 225–236. doi: 10.1534/genetics.166.1.225
Kanamori, T., Kanai, M. I., Dairyo, Y., Yasunaga, K., Morikawa, R. K., and Emoto, K. (2013). Compartmentalized calcium transients trigger dendrite pruning in Drosophila sensory neurons. Science 340, 1475–1478. doi: 10.1126/science.1234879
Lin, D. M., and Goodman, C. S. (1994). Ectopic and increased expression of fasciclin II alters motoneuron growth cone guidance. Neuron 13, 507–523. doi: 10.1016/0896-6273(94)90022-1
Matsumoto, M., Nakagawa, T., Inoue, T., Nagata, E., Tanaka, K., Takano, H., et al. (1996). Ataxia and epileptic seizures in mice lacking type 1 inositol 1,4,5-trisphosphate receptor. Nature 379, 168–171. doi: 10.1038/379168a0
Novak, M. J., Sweeney, M. G., Li, A., Treacy, C., Chandrashekar, H. S., Giunti, P., et al. (2010). An ITPR1 gene deletion causes spinocerebellar ataxia 15/16: a genetic, clinical and radiological description. Mov. Disord. 25, 2176–2182. doi: 10.1002/mds.23223
Pathak, T., Agrawal, T., Richhariya, S., Sadaf, S., and Hasan, G. (2015). Store-operated calcium entry through orai is required for transcriptional maturation of the flight circuit in Drosophila. J. Neurosci. 35, 13784–13799. doi: 10.1523/JNEUROSCI.1680-15.2015
Peng, I. F., and Wu, C. F. (2007). Drosophila cacophony channels: a major mediator of neuronal Ca2+ currents and a trigger for K+ channel homeostatic regulation. J. Neurosci. 27, 1072–1081. doi: 10.1523/JNEUROSCI.4746-06.2007
Prakriya, M., and Lewis, R. S. (2001). Potentiation and inhibition of Ca2+ release-activated Ca2+ channels by 2-aminoethyldiphenyl borate (2-APB) occurs independently of IP3 receptors. J. Physiol. 536, 3–19. doi: 10.1111/j.1469-7793.2001.t01-1-00003.x
Richhariya, S., Jayakumar, S., Abruzzi, K., Rosbash, M., and Hasan, G. (2017). A pupal transcriptomic screen identifies Ral as a target of store-operated calcium entry in Drosophila neurons. Sci. Rep. 7:42586. doi: 10.1038/srep42586
Rosay, P., Armstrong, J. D., Wang, Z., and Kaiser, K. (2001). Synchronized neural activity in the Drosophila memory centers and its modulation by amnesiac. Neuron 30, 759–770. doi: 10.1016/s0896-6273(01)00323-3
Sicaeros, B., and O’Dowd, D. K. (2007). Preparation of neuronal cultures from midgastrula stage Drosophila embryos. J. Vis. Exp. 5:226. doi: 10.3791/226
Sirabella, R., Secondo, A., Pannaccione, A., Molinaro, P., Formisano, L., Guida, N., et al. (2012). ERK1/2, p38, and JNK regulate the expression and the activity of the three isoforms of the Na+/Ca2+ exchanger, NCX1, NCX2, and NCX3, in neuronal PC12 cells. J. Neurochem. 122, 911–922. doi: 10.1111/j.1471-4159.2012.07838.x
Soltoff, S. P., and Lannon, W. A. (2013). Activation of ERK1/2 by store-operated calcium entry in rat parotid acinar cells. PLoS One 8:e72881. doi: 10.1371/journal.pone.0072881
Spitzer, N. C., Borodinsky, L. N., and Root, C. M. (2005). Homeostatic activity-dependent paradigm for neurotransmitter specification. Cell Calcium 37, 417–423. doi: 10.1016/j.ceca.2005.01.021
Srikanth, S., Wang, Z., Tu, H., Nair, S., Mathew, M. K., Hasan, G., et al. (2004). Functional properties of the Drosophila melanogaster inositol 1,4,5-trisphosphate receptor mutants. Biophys. J. 86, 3634–3646. doi: 10.1529/biophysj.104.040121
Thebault, S., Zholos, A., Enfissi, A., Slomianny, C., Dewailly, E., Roudbaraki, M., et al. (2005). Receptor-operated Ca2+ entry mediated by TRPC3/TRPC6 proteins in rat prostate smooth muscle (PS1) cell line. J. Cell. Physiol. 204, 320–328. doi: 10.1002/jcp.20301
van de Leemput, J., Chandran, J., Knight, M. A., Holtzclaw, L. A., Scholz, S., Cookson, M. R., et al. (2007). Deletion at ITPR1 underlies ataxia in mice and spinocerebellar ataxia 15 in humans. PLoS Genet. 3:e108. doi: 10.1371/journal.pgen.0030108
Venkiteswaran, G., and Hasan, G. (2009). Intracellular Ca2+ signaling and store-operated Ca2+ entry are required in Drosophila neurons for flight. Proc. Natl. Acad. Sci. U S A 106, 10326–10331. doi: 10.1073/pnas.0902982106
Keywords: SOCE, STIM, Orai, VGCC, Trp
Citation: Chakraborty S and Hasan G (2017) Spontaneous Ca2+ Influx in Drosophila Pupal Neurons Is Modulated by IP3-Receptor Function and Influences Maturation of the Flight Circuit. Front. Mol. Neurosci. 10:111. doi: 10.3389/fnmol.2017.00111
Received: 14 February 2017; Accepted: 04 April 2017;
Published: 20 April 2017.
Edited by:
Teresa Duda, Salus University, USAReviewed by:
Sonal Srikanth, University of California, Los Angeles, USAKrishanu Ray, Tata Institute of Fundamental Research, India
Copyright © 2017 Chakraborty and Hasan. This is an open-access article distributed under the terms of the Creative Commons Attribution License (CC BY). The use, distribution or reproduction in other forums is permitted, provided the original author(s) or licensor are credited and that the original publication in this journal is cited, in accordance with accepted academic practice. No use, distribution or reproduction is permitted which does not comply with these terms.
*Correspondence: Sumita Chakraborty, c29vbWVldGFAZ21haWwuY29t
Gaiti Hasan, Z2FpdGlAbmNicy5yZXMuaW4=
†Present address: Sumita Chakraborty, Department of Pharmacology, University of Cambridge, Cambridge, UK