- Molecular Biology Center, State Key Laboratory of Trauma, Burn, and Combined Injury, Research Institute of Surgery and Daping Hospital, Third Military Medical University, Chongqing, China
It is widely accepted that glutamate is the most important excitatory neurotransmitter in the central nervous system (CNS). However, there is also a large amount of glutamate in the blood. Generally, the concentration gradient of glutamate between intraparenchymal and blood environments is stable. However, this gradient is dramatically disrupted under a variety of pathological conditions, resulting in an amplifying cascade that causes a series of pathological reactions in the CNS and peripheral organs. This eventually seriously worsens a patient’s prognosis. These two “isolated” systems are rarely considered as a whole even though they mutually influence each other. In this review, we summarize what is currently known regarding the maintenance, imbalance and regulatory mechanisms that control the intraparenchymal-blood glutamate concentration gradient, discuss the interrelationships between these systems and further explore their significance in clinical practice.
Introduction
Glutamate is the most important excitatory neurotransmitter in the central nervous system (CNS) (Zhou and Danbolt, 2014). It is synthesized and stored in specific glutamatergic neurons until released into the synaptic cleft in response to specific stimuli. It then acts on glutamate receptors (including ionotropic and metabotropic receptors) on pre- and post-synaptic membranes and astrocytes to mediate signal transduction. It thereby plays a broad range of important roles in the brain, including roles in neuronal development (Martin and Finsterwald, 2011), learning and memory (De Leonibus et al., 2003; Naie and Manahan-Vaughan, 2004), emotion (Swanson et al., 2005; Stan et al., 2014) and neuroinflammation (Dai et al., 2010). Once in the synaptic cleft, glutamate is either re-taken up by the presynaptic membrane or promptly removed by astrocytes that are wrapped around the synapse (Rose et al., 2009). However, if excess extracellular glutamate is not cleared in a timely manner, glutamate receptors on the post-synaptic membrane will be excessively activated, resulting in excitotoxic injury, including the destruction of the Ca2+ buffer system (Tymianski and Tator, 1996), free radical-induced damage to mitochondria (Perez Velazquez et al., 1997), and the inhibition of phosphatidylcholine-specific phospholipase C (PC-PLC) (Li et al., 1998). Abnormally high levels of cytosolic Ca2+ and the massive release of inflammatory mediators in turn trigger the exocytosis-like release of glutamate from synaptic terminals, which results in the extracellular accumulation of glutamate and an amplifying cascade of excitatory toxicity that finally leads to the dysfunction and degeneration of neuronal synaptic transmission (Sattler and Tymianski, 2001). The activation of ionotropic glutamate receptors can also produce neurotoxicity when uncoupled from neuroexcitation (Shen and Slaughter, 2002). Thus, the dramatic increase in intraparenchymal glutamate finally exacerbates the brain injury, leading to a poor prognosis (Schousboe and Waagepetersen, 2005).
Under normal conditions, blood glutamate levels are maintained in a steady state, and a normal diet prevents significant fluctuations in blood glutamate levels (Zlotnik et al., 2011a). In addition to the contributions of basic metabolic reactions, such as deamination and gluconeogenesis (Brosnan, 2000), it has more recently become clear that glutamate signaling has functions in non-neuronal tissues in sites as diverse as bone (Peet et al., 1999) and the pancreas (Morley et al., 2000), skin (Frati et al., 2000; Kinkelin et al., 2000) and lungs (Dai et al., 2013) because the same vesicular release and receptor-mediated responses that have been documented at synapses in the CNS have been observed in these tissues. Moreover, researchers have also found that an excitotoxic reaction is induced by high levels of blood glutamate in these tissues that is similar to that induced in the CNS (Skerry and Genever, 2001). In addition, our previous clinical results showed that high levels of blood glutamate are closely related to the occurrence of traumatic brain injury-induced acute lung injury (TBI-ALI) (Bai et al., 2017). These data further indicate that blood glutamate plays an important role in peripheral organs.
The intraparenchymal-blood glutamate concentration gradient is maintained in a relatively stable condition under physiological conditions (Hawkins and Vina, 2016). However, in a variety of brain diseases, the glutamate levels in the blood, cerebrospinal fluid (CSF) or both can significantly increase, and the normal intraparenchymal-blood glutamate concentration gradient is thereby disrupted (see Table 1). These events have serious consequences for the brain (Stefani et al., 2017; Yang S.J. et al., 2017) and peripheral tissues (Jang et al., 2004; Wen et al., 2015) and are associated with a worse prognosis (Egerton et al., 2014). Here, we reviewed what is currently known about how the intraparenchymal-blood glutamate concentration gradient is maintained and regulated and investigated the potential clinical significance and impact of changes in this gradient on various brain insults.
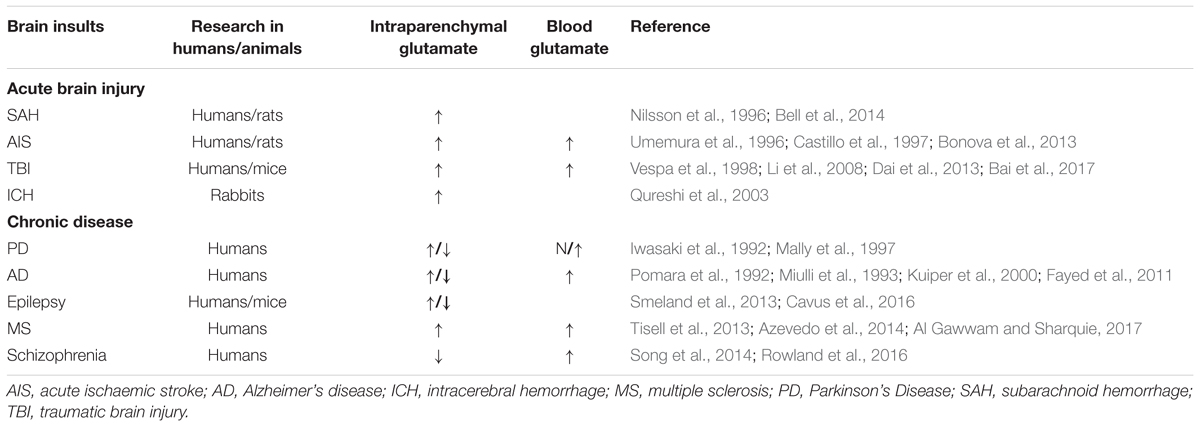
TABLE 1. Imbalanced intraparenchymal-blood glutamate concentration gradient in various brain insults.
The Formation and Maintenance of A Normal Intraparenchymal- Blood Glutamate Concentration Gradient
The glutamate concentration in the blood of healthy adults ranges from 40 to 60 μM (Bai et al., 2017). In some in vitro studies using acute brain slices, extracellular glutamate ranges from 25 to 90 nM (Cavelier and Attwell, 2005; Herman and Jahr, 2007; Le Meur et al., 2007); however, most in vivo studies using microdialysis, which is an FDA-approved method for clinical application, found much higher glutamate levels in brain, ranging from 0.2 μM to approximately 20 μM (Dash et al., 2009; De Bundel et al., 2011). Currently, researchers estimate a range from 1 to 10 μM in CSF or brain intercellular fluids (Hawkins, 2009; Li et al., 2009; Teichberg et al., 2009). Under normal conditions, the glutamate concentration is many times higher in the blood than in the CSF, and the difference between the two is nearly 50 μM, thus giving rise to the intraparenchymal-blood glutamate concentration gradient (Hawkins, 2009). The maintenance of intraparenchymal glutamate homeostasis is largely dependent on the integrity of the blood–brain barrier (BBB) limiting the influx of blood glutamate and the activity of endothelial glutamate transporters (EAATs), which constantly transport intraparenchymal glutamate into the blood (Cohen-Kashi-Malina et al., 2012).
The Integrity of the BBB Is Required for a Normal Intraparenchymal-Blood Glutamate Concentration Gradient
Glutamate is prevented from moving between the intraparenchymal and blood compartments by the BBB with intact integrity. The BBB is a physical barrier that protects the CNS from invasion by toxic substances in the blood. It has a high electrical impedance (≈2000 Ω/cm2), and restricts even the passage of ions (Crone and Olesen, 1982; Sifat et al., 2017). The BBB is composed of brain microvascular endothelial cells and junctional complexes, an endothelial basement membrane and the astrocyte end feet that surround the endothelial cells. Each layer of the BBB plays a role in restricting the flow of solutes (Abbott, 2013; Tajes et al., 2014).
Brain microvascular endothelial cells have more cytoplasmic vesicles and mitochondria than have been observed in the vessel endothelial cells of other tissues, in addition to more tight junctional complexes between cells (Oldendorf and Brown, 1975; Kniesel and Wolburg, 2000). These junctional complexes include adhesion junctions and tight junctions (TJs). The former are composed of cadherin–catenin and related proteins, while TJs mainly consist of three types of integral membrane proteins, including Claudins (Liebner et al., 2000a), Occludins (Furuse et al., 1998), and junctional adhesion molecules (JAMs) (Aurrand-Lions et al., 2001), in addition to a series of cytosolic accessory proteins, including members of the Zonula Occludens (ZO) family (Itoh et al., 1999; Wittchen et al., 1999) and cingulin (Sifat et al., 2017). These cytoplasmic proteins bind homotypically or assemble into heteropolymers, and they are responsible for the construction of the primary seal of TJs and essential for maintaining endothelial cell structure. In addition, the endothelial cell membrane is divided into the following two discrete parts by these TJs: the side facing the blood (called the luminal side) and the side facing the brain (called the abluminal side). Different populations of lipids and intrinsic proteins (e.g., glutamate transporters) reside in the luminal and abluminal spaces (Betz et al., 1980; van Meer and Simons, 1986; Tewes and Galla, 2001). The endothelial cells in the BBB are also surrounded by a continuous basement membrane that is mainly composed of collagen type IV, a variety of glycoproteins and pericytes. These proteins aggregate together to form a network that limits the flow of substances while simultaneously connecting with the surrounding tissue or extracellular matrix. They thereby play a supporting role in the BBB (Zhou et al., 2016). Embedded pericytes act alone and in association with endothelial cells or astrocytes to play key roles in maintaining the structural stability of the vessel wall (Siddharthan et al., 2007; Thanabalasundaram et al., 2010; Jo et al., 2013). Outside the basement membrane are enormous astrocyte end feet that surround approximately 85% of the surfaces of brain capillaries and play a role in regulating metabolism between brain vessels and neurons (Abbott et al., 2006). Thus, the basement membrane and astrocytic end feet are together considered the “second barrier” between the blood and brain (as shown in Figure 1).
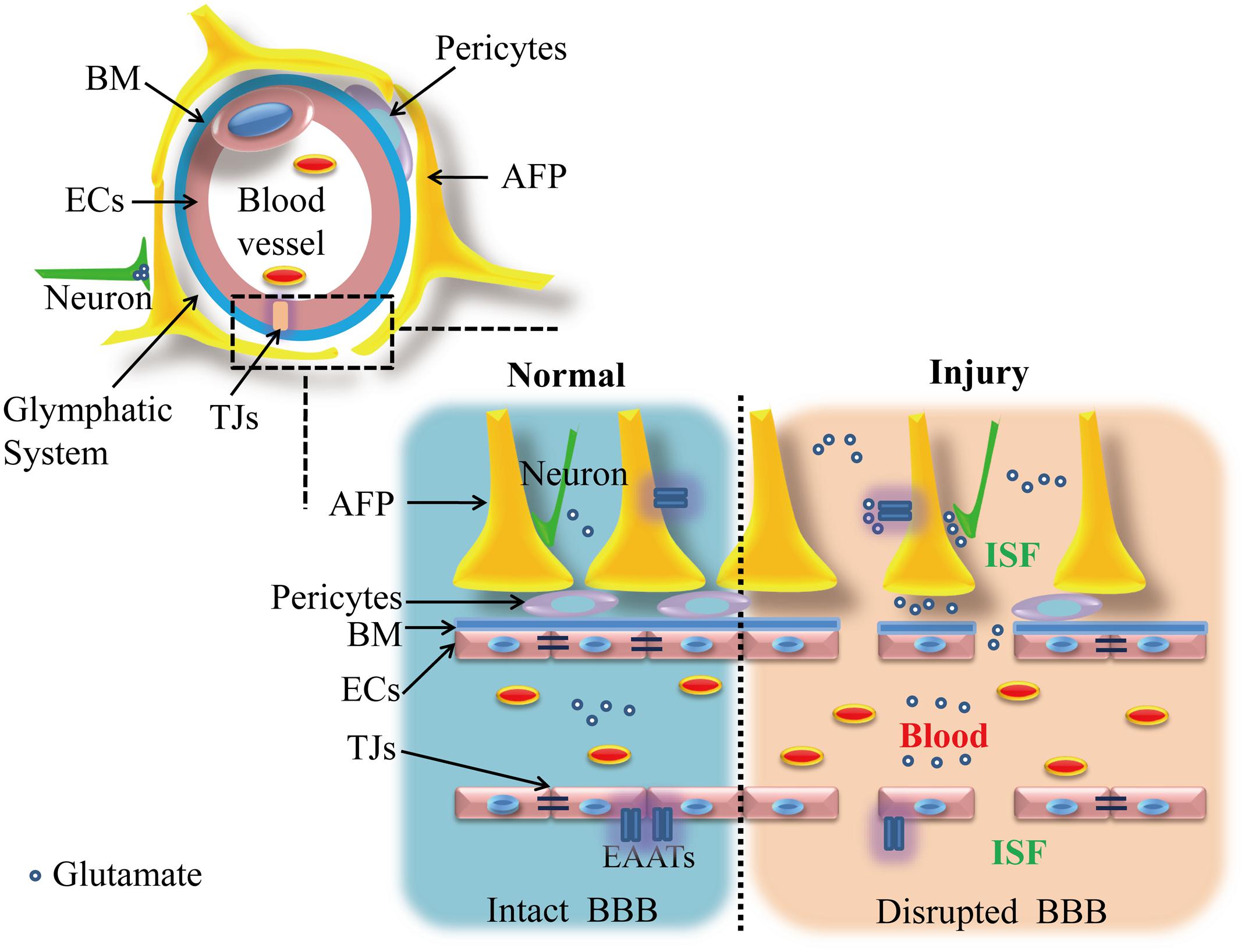
FIGURE 1. Illustration of the components of the blood–brain barrier (BBB) and distribution of glutamate under normal and injury conditions. Under normal conditions, the structure of the BBB is intact and includes a bilayer of endothelial cells (including TJs), astrocyte end feet and pericytes in combination with a basement membrane. These layers separate glutamate into two relatively isolated circumstances: brain and blood. However, after a brain injury, the BBB is disrupted, and the levels of glutamate in blood and brain both markedly increase. This figure was modified from Liu et al. (2016) with the permission of the authors. AFPs, astrocytic feet processes; BM, basement membrane; ECs, endothelial cells; EAATs, glutamate transporters; ISF, interstitial fluid; TJs, tight junctions.
Under physiological conditions, a high concentration of blood glutamate must cross at least five “films” (i.e., a bilayer of endothelial cells and astrocyte end feet in addition to the basement membrane) to enter the brain. In addition, a small amount of blood glutamate can be transported from the blood to the brain, and this process depends mainly on a Na+-independent carrier transporter (i.e., transporters, which are mainly responsible for glutamate and aspartic acid) to be transported into endothelial cells, but this occurs at a low rate, and the carrier is close to saturation (Smith, 2000; Hawkins et al., 2006a; Hawkins, 2009); additionally, a non-saturation transport that relies on the pores between endothelial cells may allow a very low rate of blood-brain flux of glutamate (Al-Sarraf and Philip, 2003). Compared to other amino acids, glutamate is transported at a relatively low rate from the blood into the brain (Benrabh and Lefauconnier, 1996; Hawkins et al., 2006b). Thus, only a very small amount of blood glutamate can normally cross the BBB into the brain (Klin et al., 2010; Cederberg et al., 2014) (see in Figure 2).
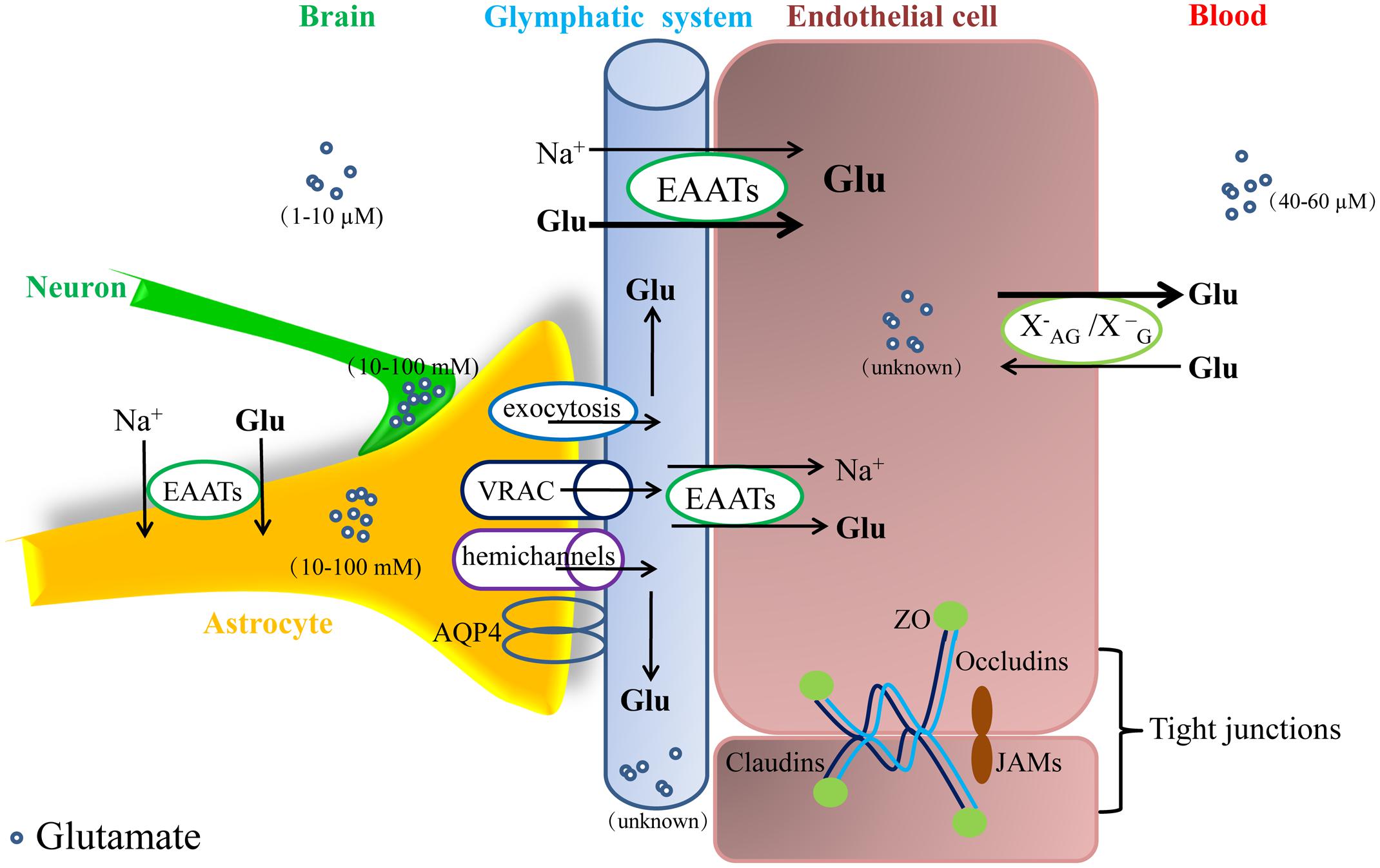
FIGURE 2. Glutamate metabolism and transport between the intraparenchymal and blood environments. The concentration of glutamate in the brain ranges from 1 to 10 μM, which is much lower than that in blood (40–60 μM) and astrocytes and neurons (10–100 mM). Under normal conditions, intraparenchymal glutamate is mainly dependent on EAATs on astrocytes and the abluminal membrane of endothelial cells for transport into cells. When the glutamate concentration in an endothelial cell exceeds the blood concentration, glutamate will be transported into the blood via facilitative transport (); however, it is difficult for blood glutamate to enter the brain via either tight junctions or carriers. EAATs, glutamate transporters.
Na+-Dependent EAATs Are the Main Force Behind the Formation of a Normal Intraparenchymal-Blood Glutamate Concentration Gradient
Despite the presence of physical barriers, however, the isolation of each compartment is not complete, and there is mutual flow between them. An active brain-to-blood efflux against the concentration gradient is thought to be the principal mechanism underlying this exchange (O’Kane et al., 1999; Hawkins et al., 2006a; Hawkins and Vina, 2016), in which the Na+-dependent EAATs (hereafter referred to as EAATs) on endothelial cells are indispensable for the maintenance of the intraparenchymal-blood glutamate concentration gradient. EAATs are a family of high-homology transmembrane proteins that are composed of 500–600 amino acids and include EAAT1/GLAST, EAAT2/GLT-1, EAAT3/EAAC1, EAAT4, and EAAT5. They also share many similarities in their molecular structures, and they possess (van den Pol et al., 1990) 8 or 10 transmembrane segments, (Zhou and Danbolt, 2014) a serine-rich motif located within the cytoplasmic or extracellular loop of the cytoplasmic or transmembrane region that contains common functional domains related to substrate-binding, (Martin and Finsterwald, 2011) a glycosylation site in the second extracellular loop of each transporter, (De Leonibus et al., 2003) the same PKA/PKC phosphorylation-regulating sites, (Naie and Manahan-Vaughan, 2004) and a large hydrophobic region near the C-terminal that is different from that of other neurotransmitter transporters. These commonalities also determine their similar regulatory mechanisms (Kanai and Hediger, 1992; Kanai et al., 1995). The expression of EAATs varies between different tissues. GLT-1 and GLAST are mainly expressed in glial cells, neurons and endothelial cells in brain, alone or in concert. EAAC1 is prevalent in the CNS (including the retina) and is mainly expressed in post-synaptic neurons (Gegelashvili and Schousboe, 1997; Fontana, 2015). EAAT4 is highly enriched in Purkinje cells of the cerebellum (Massie et al., 2001), while EAAT5 is localized to two populations of glutamatergic neurons, bipolar neurons and photoreceptors in the retina (Lee et al., 2012). Thus, EAAT1-3 are the main transporters responsible for the vast majority of intraparenchymal glutamate transport in the brain. Many studies have demonstrated the necessity of EAAT1-3 in the maintenance and regulation of glutamate homeostasis under normal and pathological conditions (see in Table 2).
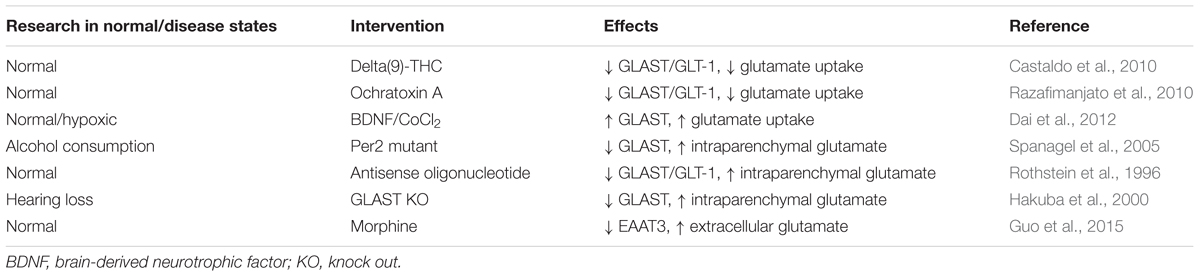
TABLE 2. Evidence for the necessity of EAAT1-3 in the maintenance and regulation of glutamate homeostasis.
Effective removal/uptake of excessive glutamate thus seems to be a crucial rescue mechanism, and failure or loss of the glutamate transport system may aggravate neurotoxic damage. In the CNS, a small proportion of the glutamate present in the synaptic cleft or intercellular fluid can undergo reuptake by the presynaptic membrane, but most of the extracellular glutamate is internalized into cells against a concentration gradient by EAATs located on glial cells or the endothelial cell membrane (Gegelashvili and Schousboe, 1998; Teichberg et al., 2009). One study has shown that when glutamate uptake is blocked, as little as 1 μM exogenous glutamate is sufficient to induce excitotoxic death in cortical neurons (Frandsen and Schousboe, 1990). Another study found that a 30-min exposure to 4 μM glutamate was sufficient to kill 50% of the neurons in astrocyte-poor cultures within 24 h, while 205 μM glutamate was required to kill the same percentage of neurons in astrocyte-rich cultures (Rosenberg et al., 1992). While the importance of the brain for blood efflux was confirmed by microinjection of radiolabelled glutamate and the kinetics of its appearance in blood (Hosoya et al., 1999; Gottlieb et al., 2003). In fact, glutamate uptake activity is so high that normal intact brain tissue is quite resistant to glutamate toxicity.
Endothelial glutamate transporters have a powerful scavenging ability that mainly depends on two processes: first and most important, EAATs are abundantly expressed on glial cells, especially astrocytes (Rothstein et al., 1994; Anderson and Swanson, 2000); additionally, astrocytes are rich in glutamine synthetase, which transforms extracellular glutamate into glutamine that can be pumped into cells to sustainably maintain a low concentration outside the cell (Danbolt, 2001). Thus, EAATs play a major role in the clearance of intraparenchymal glutamate (Zeng et al., 2010). Second, although the expression of EAATs is greatly reduced on endothelial cells (Lee et al., 2017), the brain is a highly vascularized organ [human brain contains approximately 100 million capillaries and a surface area of approximately 12 m2 (Bickel et al., 2001)]. In addition, almost every neuron in the brain has an adjacent capillary, and the average distance between a capillary and a neuron is only 8–20 μm (Schlageter et al., 1999). Therefore, EAAT-rich cerebral vessels and perivascular astrocyte end feet are particularly important for the formation and maintenance of intraparenchymal glutamate homeostasis. The driving force exerted by EAATs against the glutamate concentration gradient involves secondary active transport coupled to Na+, K+-ATPase (NKA). Intracellular Na+ is pumped out of the cell, while K+ is pumped into the cell, causing a Na+ concentration gradient from the extracellular to the intracellular compartment. EAATs cotransport one glutamate and three Na+ [or two Na+ and one H+ (Nicholls and Attwell, 1990)] into the cell. Assuming that the empty transporter is electrically neutral, the static charge of a full transport is one or two, and transport therefore generates electricity (Kanai et al., 1995).
Astrocytic and endothelial EAATs both play a crucial role in the regulation of intraparenchymal glutamate; however, endothelial EAATs play an important and unique function in the homeostasis of the intraparenchymal-blood glutamate concentration gradient since they lie directly between the brain and blood. A large number of studies have shown that endothelial EAATs are present only on the abluminal membrane (O’Kane et al., 1999; Helms et al., 2012). Under normal circumstances, the level of intraparenchymal glutamate depends on EAATs located on the endothelial abluminal membrane that transport it from the intraparenchymal space to the blood. At present, a widely accepted and confirmed view is that extracellular glutamate in the brain is continuously transported into microvascular endothelial cells, which become enriched, by abluminal EAATs against a glutamate concentration gradient. When the glutamate concentration in an endothelial cell exceeds the blood concentration, glutamate will be transported into the blood via facilitative transport (O’Kane et al., 1999; Hawkins et al., 2006a). This process is also called “concentration climbing” and is considered the most important mechanism for forming and maintaining the intraparenchymal-blood glutamate concentration gradient under physiological conditions (Helms et al., 2012). Previously, one plausible mechanism was proposed that glutamate efflux from brain extracellular fluids into the blood might involve a “glutamine-glutamate cycle” (Lee et al., 1998; O’Kane et al., 1999). In such a mechanism, the uptake of excess glutamate into astrocytes leads to its conversion into glutamine, which is released by astrocyte end feet and is subsequently pumped into endothelial cells via glutamine transporters and then converted back into glutamate. However, the results of further experiments using an isolated BBB model quickly eliminated the hypothesis that a “glutamate–glutamine” cycle makes no contribution to brain endothelial cell uptake of intercellular glutamate (Cohen-Kashi-Malina et al., 2012). In addition to this process, the glutamate in intercellular fluids can first be taken up into astrocyte end feet and then excreted via exocytosis (Wilhelm et al., 2004; Crippa et al., 2006) and volume-regulated anion channels (VRAC) (Takano et al., 2005) and hemichannels (Ye et al., 2003) before being transported into endothelial cells by glutamate transporters (as shown in Figure 2).
The Contribution of the Glymphatic System to the Formation of a Normal Intraparenchymal-Blood Glutamate Concentration Gradient
A long-held anatomical view states that the brain lacks a lymphatic system but instead uses CSF reflux. However, recent studies have suggested that in addition to endothelial cells, a novel pathway operates at the blood–brain interface, which may involve a separate paravascular highway that facilitates the rapid exchange of CSF and tissue fluids. This pathway has been referred to as the “glymphatic system” (Iliff and Nedergaard, 2013). The glymphatic system (or glymphatic clearance pathway) is a functional waste clearance pathway in the vertebrate CNS. The pathway consists of a para-arterial influx route by which CSF enters the brain parenchyma coupled to a clearance mechanism by which interstitial fluid (ISF) and extracellular solutes are removed from the interstitial compartments of the brain and spinal cord. The exchange of solutes between the CSF and the ISF is driven by arterial pulsation (Iliff et al., 2013) and regulated during sleep by the expansion and contraction of the brain extracellular space (Mendelsohn and Larrick, 2013). The clearance of soluble proteins [such as beta amyloid (Abeta), phosphorylated tau (p-tau) and Apolipoprotein E (apoE)] (Iliff et al., 2012; Achariyar et al., 2016; Sullan et al., 2017), waste products (such as lactate) (Lundgaard et al., 2017), and excess extracellular fluid (which contain small-molecule intraparenchymal glutamate and other brain injury markers) (Yang et al., 2013; Thrane et al., 2014; Plog et al., 2015) is accomplished via the convective bulk flow of the ISF and facilitated by astrocytic aquaporin 4 (AQP4) water channels (see in Figures 1, 2).
The Intraparenchymal-Blood Glutamate Concentration Gradient Is Imbalanced Under Pathological Conditions
Despite controversy, most studies have found that when various types of acute brain injury, such as subarachnoid hemorrhage (SAH), acute ischaemic stroke (AIS), intracerebral hemorrhage (ICH), or TBI occurs, glutamate levels in the brain and blood can reach extremely high levels. This elevation has also been observed in patients with chronic brain diseases, such as Parkinson’s Disease (PD), Alzheimer’s disease (AD), epilepsy, multiple sclerosis (MS) and schizophrenia (see in Table 1). Glutamate levels in the blood and CSF are significantly higher in patients with these conditions than in normal individuals, and the intraparenchymal-blood glutamate concentration gradient is also dramatically increased.
Sources of Elevated Intraparenchymal Glutamate
A tremendous amount of glutamate is stored in brain neurons and glial cells at a concentration of up to 10–100 mM (Ma et al., 2012; Mehta et al., 2013). Previous studies have shown that when a brain injury occurs, in addition to the direct destruction of neurons and glial cells, a massive amount of glutamate is released into the brain intercellular fluid by other mechanisms (Katayama et al., 1990). These include external Ca2+- or intracellular Ca2+-dependent vesicular release (Drejer et al., 1985; Katchman and Hershkowitz, 1993), release via swelling-activated anion channels (Bednar et al., 1992), an indomethacin-sensitive process in astrocytes (Parpura et al., 1994; Hassinger et al., 1995; Bezzi et al., 1998), and glutamate transporter dysfunction (Szatkowski et al., 1990; Szatkowski and Attwell, 1994). By using blockers that affect each release mechanism, researchers have demonstrated that glutamate release is largely caused by the dysfunction of glutamate transporters (Rossi et al., 2000). Conversely, this is due in part to a reduction in the expression of these transporters. For example, in patients with TBI, researchers found that reduced survival and degeneration in astrocytes resulted in a significant decrease in the expression of EAAT1/2 within 7 days after injury (van Landeghem et al., 2006; Beschorner et al., 2007), and shear or inertial force also caused changes in EAAT expression and activity-associated astrocyte deformation in TBI (Unger et al., 2012). In contrast, in such cases, the dysfunction of EAATs manifested as decreased activity and reduced transport efficiency, but reverse transport remained possible. In acute ischaemia in the hippocampus, reversed transport of neuronal EAATs resulted in a sharp increase in extracellular glutamate levels (Rossi et al., 2000). Moreover, under inflammatory conditions, which consistently accompany brain insults, the release of pro-inflammatory cytokines not only inhibited glutamate scavenging capacity (Fine et al., 1996) but also reduced EAAT2 expression in astrocytes (Rozyczka et al., 2004; Sitcheran et al., 2005).
Previously, there has been widespread controversy regarding whether the BBB is severely physically destroyed following brain injury. Although some reports have suggested that the structure of the BBB after brain injury becomes damaged and loses part of its barrier function (van Vliet et al., 2007; Weissberg et al., 2014), most studies nevertheless suggest that the direct damage (i.e., vascular rupture caused by brain contusion) is limited (Zhao et al., 2015), but that its functional components (i.e., junctional complexes between endothelial cells) might suffer more severe damage (Zhao et al., 2015; Price et al., 2016), which could last for a long time (Jiao et al., 2011). Under pathological conditions, such as shock or inflammation or the presence of a tumor, the expression of Claudin-1 and Occludin dramatically decrease in blood vessels (Liebner et al., 2000b; Papadopoulos et al., 2001). Experiments in which dye was injected into animals (Abdul-Muneer et al., 2013; Hue et al., 2014) or magnetic resonance imaging (MRI) in humans (Merali et al., 2017) confirmed the increased permeability of the BBB after a brain injury. The results of these studies suggest that increased blood glutamate can also penetrate into the brain via the functionally impaired BBB and play specific roles in the increased intraparenchymal glutamate observed after brain injury (shown in Figure 1).
The transport of intraparenchymal glutamate by the glymphatic system can also be decreased. In a variety of animal models of brain insult, including models of AD (Yang J. et al., 2017), aging (Kress et al., 2014), epilepsy (Hubbard et al., 2016), and ICH (Qiu et al., 2015), AQP4 expression is decreased and its polarity is impaired. The outflow of CSF was significantly lower in AQP4 knockout (KO) mice than in wild type mice, and the clearance rate of intercellular fluid was also greatly reduced (Plog et al., 2015). However, while the decrease in its expression had largely normalized by 7 days post-injury, AQP4 depolarization continued to be observed (Zhao et al., 2017). Moreover, a separate study also found that AQP4 is co-expressed with GLT-1 on brain perivascular astrocytes, whereas genetically knocking out AQP4 inhibited the expression of GLAST, resulting in the inhibition of intraparenchymal glutamate efflux (Li et al., 2014).
Sources of Elevated Blood Glutamate
The sources of the observed elevation in blood glutamate levels have remained unclear. Researchers have analyzed the rate of glutamate uptake in various peripheral tissues and organs after intravascular injection of [14C]-Glu and found that skeletal muscle contain the body’s largest storage pool of glutamate, accounting for approximately 59% of the total storage amount (Klin et al., 2010). In patients with acute spinal cord injury (SCI), researchers have found that an ion distribution disorder caused by the abnormal expression of NKA and its FXYD1 subunit in skeletal muscle may be the molecular basis underlying the release of glutamate from skeletal muscle after injury (Boon et al., 2012). Blood cells are another important source of blood glutamate. A comparison of patients with cerebral infarction and healthy controls has revealed that the glutamate-releasing ability of platelets is reduced in patients, suggesting that at the onset of a brain infarct, platelets are activated, which frees up a large amount of glutamate to enter the blood (Aliprandi et al., 2005; Morrell et al., 2008). Additionally, in vitro experiments have shown that the endothelial barrier function is altered by the release of soluble polymorphonuclear leukocyte (PMN)-derived glutamate during inflammatory states (Collard et al., 2002). In addition, bone might be another source of glutamate because osteoclasts also secrete L-glutamate in a Ca2+-dependent manner when stimulated with KCl or ATP (Morimoto et al., 2006).
The Regulatory Mechanisms Underlying the Imbalance in the Glutamate Concentration Gradient
The key processes goals when resolving imbalances in intraparenchymal-blood glutamate homeostasis are to reduce the elevated glutamate levels in both the blood and the brain, which includes preventing the entry of blood glutamate into the brain and enhancing the transport efficiency of glial and endothelial EAATs and the glymphatic system under pathological conditions. Thus, based on the previously discussed mechanism underlying the formation of the intraparenchymal-blood glutamate concentration gradient, we now review the regulatory mechanisms involved in modulating EAATs, TJs, the glymphatic system and glutamate itself.
Regulatory Mechanisms That Affect EAATs Expression and Function
Many factors were involved in the mRNA or protein turnover of EAATs to regulate their expression and distribution. Both glutamate and kainite dramatically increase GLAST protein expression in cultured astrocytes without significantly increasing the amount of GLAST mRNA (Gegelashvili et al., 1996). L-DOPA and ceftriaxone both increase GLT-1 expression, but they exert opposite effects on the intraparenchymal glutamate (Robelet et al., 2004; Lee S.G. et al., 2008). Studies have suggested that proteins translated from aberrant mRNAs may undergo rapid degradation and/or produce a dominant-negative effect on normal EAAT2 proteins that reduces the amount of the protein and its activity (Lin et al., 1998). Hence, while a glutamate transporter and the expression of its corresponding mRNA can differ according to the cell phenotype, cellular environment and locally active signaling pathways, the specific mechanism underlying these differences is unknown (Gegelashvili and Schousboe, 1997). Studies examining these differences at the post-translational level have primarily focused on modifications of EAATs, including their phosphorylation, glycosylation, and ubiquitination. Previous experiments have confirmed that the expression and uptake efficiency of EAATs are dependent on a PKA/PKC pathway (Casado et al., 1993; Figiel and Engele, 2000; Huang et al., 2006), and amelioration of the delayed ischaemic brain damage can be achieved by increasing both the expression and function of EAAT1 via these pathways (Yanagisawa et al., 2015; Karki et al., 2017). The effect of glycosylation on EAATs remains controversial. Observations of the N-glycosylation of GLAST demonstrated that the kinetic characteristics of GLAST are not affected (Conradt et al., 1995), while another study of EAAC1 found that glycosylation may be necessary for the activity of the transporter under hypertonic stress (Ferrer-Martinez et al., 1995). However, no clear mechanism has been identified to explain how this glycosylation is regulated. Recent evidence indicates that the turnover of EAATs in the plasma membrane is accelerated by an ubiquitin-dependent process, which is triggered by the activation of PKC (Gonzalez-Gonzalez et al., 2008; Garcia-Tardon et al., 2012). Amphetamine triggers the internalization of EAAT3 but simultaneously produces a dose-related increase in extracellular concentrations of glutamate (Del Arco et al., 1999; Underhill et al., 2014) (see also Table 3).
Because glutamate transport is associated with ion transport, the regulation of ions may significantly impact the function of transporters. In shock or TBI cases, ischaemia and hypoxia lead to a deficiency in energy synthesis, and ATP deficiency-induced mitochondrial dysfunction directly affects Na+-K+ pumps and Na+-Ca2+ and Na+-H+ exchange, resulting in a disordered charge distribution both in and outside the cell membrane. The transfer efficiency is then decreased, and in some cases, uncontrolled reverse transport occurs, resulting in the release of glutamate. Hence, these processes can eventually result in high concentrations of extracellular glutamate (Szatkowski et al., 1990; Rossi et al., 2000; Zhang et al., 2007). When the intracellular Na+ concentration increases from 15 to 30 mM, glutamate transporters began to reverse transport. However, when a Na+-H+ antiporter inhibitor was applied, it induced rapid extensive intracellular acidosis and glutamate transporter reversal but not an overload of intracellular Na+. H+ may therefore play an equally important role in regulating the direction in which EAAT is transported with Na+ (Gemba et al., 1994; Longuemare et al., 1999). Although the number of studies examining glutamate transporters has gradually increased, the precise mechanisms by which Na+, K+, and H+ lead to reverse transport remain unresolved and require further investigation (Machtens et al., 2015). In addition to these mechanisms, there may also be other routes by which extracellular glutamate concentrations can be quickly altered, including the regulation of EAAT activity, and this topic is worthy of further exploration.
Pathways Involved in the Regulation of Endothelial TJ Expression
Many signaling pathways and endo/exogenous factors have been shown to regulate the assembly of TJs (Izumi et al., 1998). Abnormalities in Ca2+ homeostasis have been implicated in the pathophysiology of brain injury (Sun et al., 2008), and one reason for this phenomenon is that Ca2+ is tightly connected to the regulation of TJs both in and outside the cell (Lacaz-Vieira and Marques, 2003). Changes in intracellular Ca2+ levels can trigger a series of PKA- or PKC-mediated molecular events that increase transendothelial resistance and promote the migration of ZO-1 from the cytoplasm to the membrane (Stevenson and Begg, 1994). Brain injury is consistently accompanied by alterations in hormones, such as insulin (Jing et al., 2017) and cortisol (Alain-Pascal et al., 2010), which also play an important role in the regulation of TJs. In both an in vitro model of BBB and in vivo research, insulin and dexamethasone were found to rapidly increase the expression of TJs and decrease permeability (Hue et al., 2015; Liu et al., 2015; Sun et al., 2015; Ito et al., 2017). Researchers observed a significant decrease in blood glutamate after injection of insulin (Zlotnik et al., 2011b); however, there was no significant correlation between blood glutamate levels and brain uptake of glutamate (Hawkins et al., 2010); in contrast, dexamethasone greatly augmented the intraparenchymal glutamate level after ischaemia (Chen et al., 1998). Blocking some chemokines/cytokines, such as IFN-γ, ameliorates both the disruption of BBB permeability and the down-regulation of TJ protein expression (Chai et al., 2014), and an increase in cortex glutamate has been observed after treatment with IFN-γ (Haroon et al., 2014). Intraparenchymal glutamate itself also induces changes in BBB permeability (Vazana et al., 2016). Additionally, the rapidly increased adenosine acting on the adenosine 2A receptor (A2AR) after a brain injury can cause cytoskeletal changes in endothelial cells while simultaneously reducing the expression of TJs, thereby increasing BBB permeability (Chen et al., 2007; Carman et al., 2011). Furthermore, the elevated intraparenchymal glutamate level is counteracted by A2AR inactivation (Li et al., 2009) (see also Table 3).
The Function of the Glymphatic System Is Closely Related to AQP4
The results of several studies have confirmed that astrocytic AQP4 plays an importantly role in clearance in the glymphatic system (Iliff et al., 2013, 2014; Murlidharan et al., 2016). As previously stated, anatomical associations have supported the notion of an interaction between endothelial cells and astrocytes (Abbott et al., 2006). Research has shown that endothelial cells promote the accumulation of AQP4 by exerting an inductive effect on extracellular matrix components such as agrin and via direct mechanical interactions with end foot processes (Camassa et al., 2015). Using an in vitro model of BBB, the results of another study confirmed that both the amount and localization of AQP4 protein in astrocytes were influenced by direct contact with endothelial cells (Haruki et al., 2013). In addition, the expression of AQP4 is influenced by other factors. Progesterone significantly reduced AQP4 expression in peri-contusion areas (Guo et al., 2006), the activation of P2X7R in astrocytes was associated with the down-regulation of AQP4 in rat brain astrocytes (Lee M. et al., 2008), and in our previous experiments, we found that AQP4 expression was significantly lower in the brain cortex in A2AR KO mice than in wild type controls following brain blast injury, suggesting that A2AR activity may affect the expression of AQP4 (Ning et al., 2013).
Pathways and Regulation of Glutamate Itself
Many enzymes or substrates are directly involved in the metabolic process of glutamate. Glutamate dehydrogenase (GDH) is important in the transdeamination of glutamate, as activation of GDH not only significantly decreases the glutamate concentration in brain (Lee et al., 2005) but also restores alpha-ketoglutarate (alpha-KG) and ATP levels after brain ischaemia (Kim et al., 2017) and increases glutamate uptake in the forebrain (Whitelaw and Robinson, 2013). Glutamine synthetase (GS) plays a key role in intraparenchymal glutamate metabolism, as after ischaemia, an increase in GS in astrocytes occurs rapidly and in parallel with proliferative changes in astrocyte organelles (Petito et al., 1992). In blood, the substrates of glutamate oxaloacetate transaminase (GOT) and glutamate pyruvate transaminase (GPT), oxaloacetate and pyruvate have also demonstrated powerful scavenging capacity (Zlotnik et al., 2012).
The Interrelationship Between Intraparenchymal and Blood Glutamate
As previously mentioned, glutamate does not exist in isolation in the brain or blood. In one study, as the glutamate concentration rose from 1 to 500 μM in the carotid artery in primary hypertension rats, the rate at which glutamate penetrated the brain increased (Al-Sarraf and Philip, 2003); additionally, systemic injection of glutamate has been reported to aggravate brain damage (Zlotnik et al., 2012). Another study showed that intravenous administration of aspartate aminotransferase (AST) (Ruban et al., 2012), pyruvate and oxaloacetate (Zlotnik et al., 2009, 2012) could significantly reduce glutamate levels in the blood in addition to accelerating the discharge of glutamate from the brain, decreasing intraparenchymal glutamate levels (Teichberg et al., 2009), significantly improving prognoses and outcomes (Campos et al., 2011), and extending the lifetimes of the mice (Zlotnik et al., 2007, 2009; Klin et al., 2010). These findings indicate that the environments in the brain and blood are mutually influenced, and blood glutamate is of great significance for the brain. However, the effect of elevated blood glutamate on the concentration of intraparenchymal glutamate and whether it is also an important source of the rapid increase in intraparenchymal glutamate remain poorly understood. Moreover, while there is no direct evidence showing that intraparenchymal glutamate levels influence blood glutamate levels, the results of our recent studies in patients with TBI indicate that the severity of brain injury is positively associated with blood glutamate levels (Bai et al., 2017).
The Significance of Potential Applications That Alter the Homeostasis of the Intraparenchymal-Blood Glutamate Concentration Gradient
In an effort to ensure that “CNS security” is made an appropriate priority in pathological cases, administering a glutamate receptor antagonist following a brain insult has, in many pre-clinical studies, indicated neuro-protective roles and improved prognoses (Furukawa et al., 2003; de Miranda et al., 2016). However, the results of clinical trials have suggested that these drugs fail to improve long-term prognoses or reduce mortality after brain injury (Davis et al., 1997; Lees, 1997; Maas et al., 2006). We hypothesized that this might be because the important role of blood glutamate (and therefore the intraparenchymal-blood glutamate concentration gradient) was ignored. Because haemofiltration (Rogachev et al., 2012) and peritoneal dialysis (Rogachev et al., 2013) have been approved to efficiently lower blood glutamate levels in patients, these measures could be used to treat acute and chronic brain disorders that are accompanied by elevated glutamate levels in both the brain and blood.
However, many unsolved issues remain. For example, is peripheral glutamate an important source of increased intraparenchymal glutamate following a brain injury? Can blood glutamate act as a diagnostic or prognostic indicator of brain injury? Only by increasing our understanding of the generation and metabolism of intraparenchymal-blood glutamate can we identify methods to regulate the glutamate concentration gradient at the source and thereby prevent the damaging effects of high levels of glutamate. Such studies would offer important and effective methods for treating the acute phase of brain injury.
Author Contributions
Both the authors, WB and Y-GZ, listed have made a substantial, direct and intellectual contribution to the work, and approved it for publication.
Funding
This work was supported by the National Natural Science Foundation of China (31171022).
Conflict of Interest Statement
The authors declare that the research was conducted in the absence of any commercial or financial relationships that could be construed as a potential conflict of interest.
Acknowledgment
The authors gratefully acknowledge Dr. Ping Li for critically reading and providing advice during the writing of this manuscript.
References
Abbott, N. J. (2013). Blood-brain barrier structure and function and the challenges for CNS drug delivery. J. Inherit. Metab. Dis. 36, 437–449. doi: 10.1007/s10545-013-9608-0
Abbott, N. J., Ronnback, L., and Hansson, E. (2006). Astrocyte-endothelial interactions at the blood-brain barrier. Nat. Rev. Neurosci. 7, 41–53. doi: 10.1038/nrn1824
Abdul-Muneer, P. M., Schuetz, H., Wang, F., Skotak, M., Jones, J., Gorantla, S., et al. (2013). Induction of oxidative and nitrosative damage leads to cerebrovascular inflammation in an animal model of mild traumatic brain injury induced by primary blast. Free Radic. Biol. Med. 60, 282–291. doi: 10.1016/j.freeradbiomed.2013.02.029
Achariyar, T. M., Li, B., Peng, W., Verghese, P. B., Shi, Y., McConnell, E., et al. (2016). Glymphatic distribution of CSF-derived apoE into brain is isoform specific and suppressed during sleep deprivation. Mol. Neurodegener. 11:74. doi: 10.1186/s13024-016-0138-8
Al Gawwam, G., and Sharquie, I. K. (2017). Serum glutamate is a predictor for the diagnosis of multiple sclerosis. Sci. World J. 2017:9320802. doi: 10.1155/2017/9320802
Alain-Pascal, B. B., Wei, H. J., Chen, X., and Zhang, J. N. (2010). Evaluation of stress hormones in traumatic brain injury patients with gastrointestinal bleeding. Chin. J. Traumatol. 13, 25–31.
Aliprandi, A., Longoni, M., Stanzani, L., Tremolizzo, L., Vaccaro, M., Begni, B., et al. (2005). Increased plasma glutamate in stroke patients might be linked to altered platelet release and uptake. J. Cereb. Blood Flow Metab. 25, 513–519. doi: 10.1038/sj.jcbfm.9600039
Al-Sarraf, H., and Philip, L. (2003). Increased brain uptake and CSF clearance of 14C-glutamate in spontaneously hypertensive rats. Brain Res. 994, 181–187. doi: 10.1016/j.brainres.2003.09.034
Anderson, C. M., and Swanson, R. A. (2000). Astrocyte glutamate transport: review of properties, regulation, and physiological functions. Glia 32, 1–14. doi: 10.1002/1098-1136(200010)32:1<1::AID-GLIA10>3.0.CO;2-W
Aurrand-Lions, M., Johnson-Leger, C., Wong, C., Du Pasquier, L., and Imhof, B. A. (2001). Heterogeneity of endothelial junctions is reflected by differential expression and specific subcellular localization of the three JAM family members. Blood 98, 3699–3707. doi: 10.1182/blood.V98.13.3699
Azevedo, C. J., Kornak, J., Chu, P., Sampat, M., Okuda, D. T., Cree, B. A., et al. (2014). In vivo evidence of glutamate toxicity in multiple sclerosis. Ann. Neurol. 76, 269–278. doi: 10.1002/ana.24202
Bai, W., Zhu, W. L., Ning, Y. L., Li, P., Zhao, Y., Yang, N., et al. (2017). Dramatic increases in blood glutamate concentrations are closely related to traumatic brain injury-induced acute lung injury. Sci. Rep. 7:5380. doi: 10.1038/s41598-017-05574-9
Bednar, M. M., Kohut, J. J., Kimelberg, H. K., Gross, J. J., and Gross, C. E. (1992). In vitro evidence supporting two mechanisms of action for the anion transport inhibitor L-644,711 in cerebral ischaemia. Neurol. Res. 14, 53–56. doi: 10.1080/01616412.1992.11740011
Bell, J. D., Thomas, T. C., Lass, E., Ai, J., Wan, H., Lifshitz, J., et al. (2014). Platelet-mediated changes to neuronal glutamate receptor expression at sites of microthrombosis following experimental subarachnoid hemorrhage. J. Neurosurg. 121, 1424–1431. doi: 10.3171/2014.3.JNS132130
Benrabh, H., and Lefauconnier, J. M. (1996). Glutamate is transported across the rat blood-brain barrier by a sodium-independent system. Neurosci. Lett. 210, 9–12. doi: 10.1016/0304-3940(96)12635-5
Beschorner, R., Dietz, K., Schauer, N., Mittelbronn, M., Schluesener, H. J., Trautmann, K., et al. (2007). Expression of EAAT1 reflects a possible neuroprotective function of reactive astrocytes and activated microglia following human traumatic brain injury. Histol. Histopathol. 22, 515–526. doi: 10.14670/HH-22.515
Betz, A. L., Firth, J. A., and Goldstein, G. W. (1980). Polarity of the blood-brain barrier: distribution of enzymes between the luminal and antiluminal membranes of brain capillary endothelial cells. Brain Res. 192, 17–28. doi: 10.1016/0006-8993(80)91004-5
Bezzi, P., Carmignoto, G., Pasti, L., Vesce, S., Rossi, D., Rizzini, B. L., et al. (1998). Prostaglandins stimulate calcium-dependent glutamate release in astrocytes. Nature 391, 281–285. doi: 10.1038/34651
Bickel, U., Yoshikawa, T., and Pardridge, W. M. (2001). Delivery of peptides and proteins through the blood-brain barrier. Adv. Drug Deliv. Rev. 46, 247–279. doi: 10.1016/S0169-409X(00)00139-3
Bonova, P., Burda, J., Danielisova, V., Nemethova, M., and Gottlieb, M. (2013). Delayed post-conditioning reduces post-ischemic glutamate level and improves protein synthesis in brain. Neurochem. Int. 62, 854–860. doi: 10.1016/j.neuint.2013.02.019
Boon, H., Kostovski, E., Pirkmajer, S., Song, M., Lubarski, I., Iversen, P. O., et al. (2012). Influence of chronic and acute spinal cord injury on skeletal muscle Na+-K+-ATPase and phospholemman expression in humans. Am. J. Physiol. Endocrinol. Metab. 302, E864–E871. doi: 10.1152/ajpendo.00625.2011
Brosnan, J. T. (2000). Glutamate, at the interface between amino acid and carbohydrate metabolism. J. Nutr. 130(4S Suppl.), 988S–990S.
Camassa, L. M., Lunde, L. K., Hoddevik, E. H., Stensland, M., Boldt, H. B., De Souza, G. A., et al. (2015). Mechanisms underlying AQP4 accumulation in astrocyte endfeet. Glia doi: 10.1002/glia.22878 [Epub ahead of print].
Campos, F., Sobrino, T., Ramos-Cabrer, P., Castellanos, M., Blanco, M., Rodriguez-Yanez, M., et al. (2011). High blood glutamate oxaloacetate transaminase levels are associated with good functional outcome in acute ischemic stroke. J. Cereb. Blood Flow Metab. 31, 1387–1393. doi: 10.1038/jcbfm.2011.4
Carman, A. J., Mills, J. H., Krenz, A., Kim, D. G., and Bynoe, M. S. (2011). Adenosine receptor signaling modulates permeability of the blood-brain barrier. J. Neurosci. 31, 13272–13280. doi: 10.1523/JNEUROSCI.3337-11.2011
Casado, M., Bendahan, A., Zafra, F., Danbolt, N. C., Aragon, C., Gimenez, C., et al. (1993). Phosphorylation and modulation of brain glutamate transporters by protein kinase C. J. Biol. Chem. 268, 27313–27317.
Castaldo, P., Magi, S., Cataldi, M., Arcangeli, S., Lariccia, V., Nasti, A. A., et al. (2010). Altered regulation of glutamate release and decreased functional activity and expression of GLT1 and GLAST glutamate transporters in the hippocampus of adolescent rats perinatally exposed to Delta(9)-THC. Pharmacol. Res. 61, 334–341. doi: 10.1016/j.phrs.2009.11.008
Castillo, J., Davalos, A., and Noya, M. (1997). Progression of ischaemic stroke and excitotoxic aminoacids. Lancet 349, 79–83. doi: 10.1016/S0140-6736(96)04453-4
Cavelier, P., and Attwell, D. (2005). Tonic release of glutamate by a DIDS-sensitive mechanism in rat hippocampal slices. J. Physiol. 564(Pt 2), 397–410. doi: 10.1113/jphysiol.2004.082131
Cavus, I., Romanyshyn, J. C., Kennard, J. T., Farooque, P., Williamson, A., Eid, T., et al. (2016). Elevated basal glutamate and unchanged glutamine and GABA in refractory epilepsy: microdialysis study of 79 patients at the yale epilepsy surgery program. Ann. Neurol. 80, 35–45. doi: 10.1002/ana.24673
Cederberg, H. H., Uhd, N. C., and Brodin, B. (2014). Glutamate efflux at the blood-brain barrier: cellular mechanisms and potential clinical relevance. Arch. Med. Res. 45, 639–645. doi: 10.1016/j.arcmed.2014.11.004
Chai, Q., He, W. Q., Zhou, M., Lu, H., and Fu, Z. F. (2014). Enhancement of blood-brain barrier permeability and reduction of tight junction protein expression are modulated by chemokines/cytokines induced by rabies virus infection. J. Virol. 88, 4698–4710. doi: 10.1128/JVI.03149-13
Chen, J., Adachi, N., Tsubota, S., Nagaro, T., and Arai, T. (1998). Dexamethasone augments ischemia-induced extracellular accumulation of glutamate in gerbil hippocampus. Eur. J. Pharmacol. 347, 67–70. doi: 10.1016/S0014-2999(98)00198-8
Chen, J. F., Sonsalla, P. K., Pedata, F., Melani, A., Domenici, M. R., Popoli, P., et al. (2007). Adenosine A2A receptors and brain injury: broad spectrum of neuroprotection, multifaceted actions and “fine tuning” modulation. Prog. Neurobiol. 83, 310–331. doi: 10.1016/j.pneurobio.2007.09.002
Cohen-Kashi-Malina, K., Cooper, I., and Teichberg, V. I. (2012). Mechanisms of glutamate efflux at the blood-brain barrier: involvement of glial cells. Cereb. J. Blood Flow Metab. 32, 177–189. doi: 10.1038/jcbfm.2011.121
Collard, C. D., Park, K. A., Montalto, M. C., Alapati, S., Buras, J. A., Stahl, G. L., et al. (2002). Neutrophil-derived glutamate regulates vascular endothelial barrier function. J. Biol. Chem. 277, 14801–14811. doi: 10.1074/jbc.M110557200
Conradt, M., Storck, T., and Stoffel, W. (1995). Localization of N-glycosylation sites and functional role of the carbohydrate units of GLAST-1, a cloned rat brain L-glutamate/L-aspartate transporter. Eur. J. Biochem. 229, 682–687. doi: 10.1111/j.1432-1033.1995.tb20514.x
Crippa, D., Schenk, U., Francolini, M., Rosa, P., Verderio, C., Zonta, M., et al. (2006). Synaptobrevin2-expressing vesicles in rat astrocytes: insights into molecular characterization, dynamics and exocytosis. J. Physiol. 570(Pt 3), 567–582. doi: 10.1113/jphysiol.2005.094052
Crone, C., and Olesen, S.P. (1982). Electrical resistance of brain microvascular endothelium. Brain Res. 241, 49–55. doi: 10.1016/0006-8993(82)91227-6
Dai, M., Xia, X. B., and Xiong, S. Q. (2012). BDNF regulates GLAST and glutamine synthetase in mouse retinal Muller cells. J. Cell Physiol. 227, 596–603. doi: 10.1002/jcp.22762
Dai, S. S., Wang, H., Yang, N., An, J. H., Li, W., Ning, Y. L., et al. (2013). Plasma glutamate-modulated interaction of A2AR and mGluR5 on BMDCs aggravates traumatic brain injury-induced acute lung injury. J. Exp. Med. 210, 839–851. doi: 10.1084/jem.20122196
Dai, S. S., Zhou, Y. G., Li, W., An, J. H., Li, P., Yang, N., et al. (2010). Local glutamate level dictates adenosine A2A receptor regulation of neuroinflammation and traumatic brain injury. J. Neurosci. 30, 5802–5810. doi: 10.1523/JNEUROSCI.0268-10.2010
Danbolt, N. C. (2001). Glutamate uptake. Prog. Neurobiol. 65, 1–105. doi: 10.1016/S0301-0082(00)00067-8
Dash, M. B., Douglas, C. L., Vyazovskiy, V. V., Cirelli, C., and Tononi, G. (2009). Long-term homeostasis of extracellular glutamate in the rat cerebral cortex across sleep and waking states. J. Neurosci. 29, 620–629. doi: 10.1523/JNEUROSCI.5486-08.2009
Davis, S. M., Albers, G. W., Diener, H. C., Lees, K. R., and Norris, J. (1997). Termination of acute stroke studies involving selfotel treatment. ASSIST steering committed. Lancet 349:32. doi: 10.1016/S0140-6736(05)62166-6
De Bundel, D., Schallier, A., Loyens, E., Fernando, R., Miyashita, H., Van Liefferinge, J., et al. (2011). Loss of system x(c)- does not induce oxidative stress but decreases extracellular glutamate in hippocampus and influences spatial working memory and limbic seizure susceptibility. J. Neurosci. 31, 5792–5803. doi: 10.1523/JNEUROSCI.5465-10.2011
De Leonibus, E., Costantini, V. J., Castellano, C., Ferretti, V., Oliverio, A., and Mele, A. (2003). Distinct roles of the different ionotropic glutamate receptors within the nucleus accumbens in passive-avoidance learning and memory in mice. Eur. J. Neurosci. 18, 2365–2373. doi: 10.1046/j.1460-9568.2003.02939.x
de Miranda, A. S., Brant, F., Vieira, L. B., Rocha, N. P., Vieira, E. L., Rezende, G. H., et al. (2016). A neuroprotective effect of the glutamate receptor antagonist MK801 on long-term cognitive and behavioral outcomes secondary to experimental cerebral malaria. Mol. Neurobiol. doi: 10.1007/s12035-016-0226-3 [Epub ahead of print].
Del Arco, A., Gonzalez-Mora, J. L., Armas, V. R., and Mora, F. (1999). Amphetamine increases the extracellular concentration of glutamate in striatum of the awake rat: involvement of high affinity transporter mechanisms. Neuropharmacology 38, 943–954. doi: 10.1016/S0028-3908(99)00043-X
Drejer, J., Benveniste, H., Diemer, N. H., and Schousboe, A. (1985). Cellular origin of ischemia-induced glutamate release from brain tissue in vivo and in vitro. J. Neurochem. 45, 145–151. doi: 10.1111/j.1471-4159.1985.tb05486.x
Egerton, A., Stone, J. M., Chaddock, C. A., Barker, G. J., Bonoldi, I., Howard, R. M., et al. (2014). Relationship between brain glutamate levels and clinical outcome in individuals at ultra high risk of psychosis. Neuropsychopharmacology 39, 2891–2899. doi: 10.1038/npp.2014.143
Fayed, N., Modrego, P. J., Rojas-Salinas, G., and Aguilar, K. (2011). Brain glutamate levels are decreased in Alzheimer’s disease: a magnetic resonance spectroscopy study. Am. J. Alzheimers Dis. Other Demen. 26, 450–456. doi: 10.1177/1533317511421780
Ferrer-Martinez, A., Felipe, A., Nicholson, B., Casado, J., Pastor-Anglada, M., and McGivan, J. (1995). Induction of the high-affinity Na(+)-dependent glutamate transport system XAG- by hypertonic stress in the renal epithelial cell line NBL-1. Biochem. J. 310(Pt 2), 689–692. doi: 10.1042/bj3100689
Figiel, M., and Engele, J. (2000). Pituitary adenylate cyclase-activating polypeptide (PACAP), a neuron-derived peptide regulating glial glutamate transport and metabolism. J. Neurosci. 20, 3596–3605.
Fine, S. M., Angel, R. A., Perry, S. W., Epstein, L. G., Rothstein, J. D., Dewhurst, S., et al. (1996). Tumor necrosis factor alpha inhibits glutamate uptake by primary human astrocytes. Implications for pathogenesis of HIV-1 dementia. J. Biol. Chem. 271, 15303–15306. doi: 10.1074/jbc.271.26.15303
Fontana, A. C. (2015). Current approaches to enhance glutamate transporter function and expression. J. Neurochem. 134, 982–1007. doi: 10.1111/jnc.13200
Frandsen, A., and Schousboe, A. (1990). Development of excitatory amino acid induced cytotoxicity in cultured neurons. Int. J. Dev. Neurosci. 8, 209–216. doi: 10.1016/0736-5748(90)90013-R
Frati, C., Marchese, C., Fisichella, G., Copani, A., Nasca, M. R., Storto, M., et al. (2000). Expression of functional mGlu5 metabotropic glutamate receptors in human melanocytes. J. Cell Physiol. 183, 364–372. doi: 10.1002/(SICI)1097-4652(200006)183:3<364::AID-JCP9>3.0.CO;2-X–401
Furukawa, T., Hoshino, S., Kobayashi, S., Asakura, T., Takahashi, M., Atsumi, T., et al. (2003). The glutamate AMPA receptor antagonist, YM872, attenuates cortical tissue loss, regional cerebral edema, and neurological motor deficits after experimental brain injury in rats. J. Neurotrauma 20, 269–278. doi: 10.1089/089771503321532851
Furuse, M., Sasaki, H., Fujimoto, K., and Tsukita, S. (1998). A single gene product, claudin-1 or -2, reconstitutes tight junction strands and recruits occludin in fibroblasts. J. Cell Biol. 143, 391–401. doi: 10.1083/jcb.143.2.391
Garcia-Tardon, N., Gonzalez-Gonzalez, I. M., Martinez-Villarreal, J., Fernandez-Sanchez, E., Gimenez, C., and Zafra, F. (2012). Protein kinase C (PKC)-promoted endocytosis of glutamate transporter GLT-1 requires ubiquitin ligase Nedd4-2-dependent ubiquitination but not phosphorylation. J. Biol. Chem. 287, 19177–19187. doi: 10.1074/jbc.M112.355909
Gegelashvili, G., Civenni, G., Racagni, G., Danbolt, N. C., Schousboe, I., and Schousboe, A. (1996). Glutamate receptor agonists up-regulate glutamate transporter GLAST in astrocytes. Neuroreport 8, 261–265. doi: 10.1097/00001756-199612200-00052
Gegelashvili, G., and Schousboe, A. (1997). High affinity glutamate transporters: regulation of expression and activity. Mol. Pharmacol. 52, 6–15.
Gegelashvili, G., and Schousboe, A. (1998). Cellular distribution and kinetic properties of high-affinity glutamate transporters. Brain Res. Bull. 45, 233–238. doi: 10.1016/S0361-9230(97)00417-6
Gemba, T., Oshima, T., and Ninomiya, M. (1994). Glutamate efflux via the reversal of the sodium-dependent glutamate transporter caused by glycolytic inhibition in rat cultured astrocytes. Neuroscience 63, 789–795. doi: 10.1016/0306-4522(94)90523-1
Gonzalez-Gonzalez, I. M., Garcia-Tardon, N., Gimenez, C., and Zafra, F. (2008). PKC-dependent endocytosis of the GLT1 glutamate transporter depends on ubiquitylation of lysines located in a C-terminal cluster. Glia 56, 963–974. doi: 10.1002/glia.20670
Gottlieb, M., Wang, Y., and Teichberg, V. I. (2003). Blood-mediated scavenging of cerebrospinal fluid glutamate. J. Neurochem. 87, 119–126. doi: 10.1046/j.1471-4159.2003.01972.x
Guo, M., Cao, D., Zhu, S., Fu, G., Wu, Q., Liang, J., et al. (2015). Chronic exposure to morphine decreases the expression of EAAT3 via opioid receptors in hippocampal neurons. Brain Res. 1628(Pt A), 40–49. doi: 10.1016/j.brainres.2015.03.037
Guo, Q., Sayeed, I., Baronne, L. M., Hoffman, S. W., Guennoun, R., and Stein, D. G. (2006). Progesterone administration modulates AQP4 expression and edema after traumatic brain injury in male rats. Exp. Neurol. 198, 469–478. doi: 10.1016/j.expneurol.2005.12.013
Hakuba, N., Koga, K., Gyo, K., Usami, S. I., and Tanaka, K. (2000). Exacerbation of noise-induced hearing loss in mice lacking the glutamate transporter GLAST. J. Neurosci. 20, 8750–8753.
Haroon, E., Woolwine, B. J., Chen, X., Pace, T. W., Parekh, S., Spivey, J. R., et al. (2014). IFN-alpha-induced cortical and subcortical glutamate changes assessed by magnetic resonance spectroscopy. Neuropsychopharmacology 39, 1777–1785. doi: 10.1038/npp.2014.25
Haruki, H., Sano, Y., Shimizu, F., Omoto, M., Tasaki, A., Oishi, M., et al. (2013). NMO sera down-regulate AQP4 in human astrocyte and induce cytotoxicity independent of complement. J. Neurol. Sci. 331, 136–144. doi: 10.1016/j.jns.2013.05.035
Hassinger, T. D., Atkinson, P. B., Strecker, G. J., Whalen, L. R., Dudek, F. E., Kossel, A. H., et al. (1995). Evidence for glutamate-mediated activation of hippocampal neurons by glial calcium waves. J. Neurobiol. 28, 159–170. doi: 10.1002/neu.480280204
Hawkins, R. A. (2009). The blood-brain barrier and glutamate. Am. J. Clin. Nutr. 90, 867S–874S. doi: 10.3945/ajcn.2009.27462BB
Hawkins, R. A., Mokashi, A., Dejoseph, M. R., Vina, J. R., and Fernstrom, J. D. (2010). Glutamate permeability at the blood-brain barrier in insulinopenic and insulin-resistant rats. Metabolism 59, 258–266. doi: 10.1016/j.metabol.2009.07.022
Hawkins, R. A., O’Kane, R. L., Simpson, I. A., and Vina, J. R. (2006a). Structure of the blood-brain barrier and its role in the transport of amino acids. J. Nutr. 136(1 Suppl.), 218S–226S.
Hawkins, R. A., Simpson, I. A., Mokashi, A., and Vina, J. R. (2006b). Pyroglutamate stimulates Na+ -dependent glutamate transport across the blood-brain barrier. FEBS Lett. 580, 4382–4386. doi: 10.1016/j.febslet.2006.06.097
Hawkins, R. A., and Vina, J. R. (2016). How glutamate is managed by the blood-brain barrier. Biology 5:37. doi: 10.3390/biology5040037
Helms, H. C., Madelung, R., Waagepetersen, H. S., Nielsen, C. U., and Brodin, B. (2012). In vitro evidence for the brain glutamate efflux hypothesis: brain endothelial cells cocultured with astrocytes display a polarized brain-to-blood transport of glutamate. Glia 60, 882–893. doi: 10.1002/glia.22321
Herman, M. A., and Jahr, C. E. (2007). Extracellular glutamate concentration in hippocampal slice. J. Neurosci. 27, 9736–9741. doi: 10.1523/JNEUROSCI.3009-07.2007
Hosoya, K., Sugawara, M., Asaba, H., and Terasaki, T. (1999). Blood-brain barrier produces significant efflux of L-aspartic acid but not D-aspartic acid: in vivo evidence using the brain efflux index method. J. Neurochem. 73, 1206–1211. doi: 10.1046/j.1471-4159.1999.0731206.x
Huang, Y., Feng, X., Sando, J. J., and Zuo, Z. (2006). Critical role of serine 465 in isoflurane-induced increase of cell-surface redistribution and activity of glutamate transporter type 3. J. Biol. Chem. 281, 38133–38138. doi: 10.1074/jbc.M603885200
Hubbard, J. A., Szu, J. I., Yonan, J. M., and Binder, D. K. (2016). Regulation of astrocyte glutamate transporter-1 (GLT1) and aquaporin-4 (AQP4) expression in a model of epilepsy. Exp. Neurol. 283(Pt A), 85–96. doi: 10.1016/j.expneurol.2016.05.003
Hue, C. D., Cao, S., Dale Bass, C. R., Meaney, D. F., and Morrison, B. I. I. I. (2014). Repeated primary blast injury causes delayed recovery, but not additive disruption, in an in vitro blood-brain barrier model. J. Neurotrauma 31, 951–960. doi: 10.1089/neu.2013.3149
Hue, C. D., Cho, F. S., Cao, S., Dale Bass, C. R., Meaney, D. F., and Morrison, B. I. I. I. (2015). Dexamethasone potentiates in vitro blood-brain barrier recovery after primary blast injury by glucocorticoid receptor-mediated upregulation of ZO-1 tight junction protein. J. Cereb. Blood Flow Metab. 35, 1191–1198. doi: 10.1038/jcbfm.2015.38
Iliff, J. J., Chen, M. J., Plog, B. A., Zeppenfeld, D. M., Soltero, M., Yang, L., et al. (2014). Impairment of glymphatic pathway function promotes tau pathology after traumatic brain injury. J. Neurosci. 34, 16180–16193. doi: 10.1523/JNEUROSCI.3020-14.2014
Iliff, J. J., and Nedergaard, M. (2013). Is there a cerebral lymphatic system? Stroke 44(6 Suppl. 1), S93–S95. doi: 10.1161/STROKEAHA.112.678698
Iliff, J. J., Wang, M., Liao, Y., Plogg, B. A., Peng, W., Gundersen, G. A., et al. (2012). A paravascular pathway facilitates CSF flow through the brain parenchyma and the clearance of interstitial solutes, including amyloid beta. Sci. Transl. Med. 4:147ra111. doi: 10.1126/scitranslmed.3003748
Iliff, J. J., Wang, M., Zeppenfeld, D. M., Venkataraman, A., Plog, B. A., Liao, Y., et al. (2013). Cerebral arterial pulsation drives paravascular CSF-interstitial fluid exchange in the murine brain. J. Neurosci. 33, 18190–18199. doi: 10.1523/JNEUROSCI.1592-13.2013
Ito, S., Yanai, M., Yamaguchi, S., Couraud, P. O., and Ohtsuki, S. (2017). Regulation of tight-junction integrity by insulin in an in vitro model of human blood-brain barrier. J. Pharm. Sci. 106, 2599–2605. doi: 10.1016/j.xphs.2017.04.036
Itoh, M., Furuse, M., Morita, K., Kubota, K., Saitou, M., and Tsukita, S. (1999). Direct binding of three tight junction-associated MAGUKs, ZO-1, ZO-2, and ZO-3, with the COOH termini of claudins. J. Cell Biol. 147, 1351–1363. doi: 10.1083/jcb.147.6.1351
Iwasaki, Y., Ikeda, K., Shiojima, T., and Kinoshita, M. (1992). Increased plasma concentrations of aspartate, glutamate and glycine in Parkinson’s disease. Neurosci. Lett. 145, 175–177. doi: 10.1016/0304-3940(92)90015-Y
Izumi, Y., Hirose, T., Tamai, Y., Hirai, S., Nagashima, Y., Fujimoto, T., et al. (1998). An atypical PKC directly associates and colocalizes at the epithelial tight junction with ASIP, a mammalian homologue of Caenorhabditis elegans polarity protein PAR-3. J. Cell Biol. 143, 95–106. doi: 10.1083/jcb.143.1.95
Jang, J. H., Kim, D. W., Sang Nam, T., Se Paik, K., and Leem, J. W. (2004). Peripheral glutamate receptors contribute to mechanical hyperalgesia in a neuropathic pain model of the rat. Neuroscience 128, 169–176. doi: 10.1016/j.neuroscience.2004.06.040
Jiao, H., Wang, Z., Liu, Y., Wang, P., and Xue, Y. (2011). Specific role of tight junction proteins claudin-5, occludin, and ZO-1 of the blood-brain barrier in a focal cerebral ischemic insult. J. Mol. Neurosci. 44, 130–139. doi: 10.1007/s12031-011-9496-4
Jing, J., Pan, Y., Zhao, X., Zheng, H., Jia, Q., Mi, D., et al. (2017). Insulin resistance and prognosis of nondiabetic patients with ischemic stroke: the ACROSS-China study (abnormal glucose regulation in patients with acute stroke across China). Stroke 48, 887–893. doi: 10.1161/STROKEAHA.116.015613
Jo, D. H., Kim, J. H., Heo, J. I., Kim, J. H., and Cho, C. H. (2013). Interaction between pericytes and endothelial cells leads to formation of tight junction in hyaloid vessels. Mol. Cells 36, 465–471. doi: 10.1007/s10059-013-0228-1
Kanai, Y., and Hediger, M. A. (1992). Primary structure and functional characterization of a high-affinity glutamate transporter. Nature 360, 467–471. doi: 10.1038/360467a0
Kanai, Y., Nussberger, S., Romero, M. F., Boron, W. F., Hebert, S. C., and Hediger, M. A. (1995). Electrogenic properties of the epithelial and neuronal high affinity glutamate transporter. J. Biol. Chem. 270, 16561–16568. doi: 10.1074/jbc.270.28.16561
Karki, P., Hong, P., Johnson, J. Jr., Pajarillo, E., Son, D. S., Aschner, M., et al. (2017). Arundic acid increases expression and function of astrocytic glutamate transporter EAAT1 Via the ERK, Akt, and NF-kappaB pathways. Mol. Neurobiol. doi: 10.1007/s12035-017-0709-x [Epub ahead of print].
Katayama, Y., Becker, D. P., Tamura, T., and Hovda, D. A. (1990). Massive increases in extracellular potassium and the indiscriminate release of glutamate following concussive brain injury. J. Neurosurg. 73, 889–900. doi: 10.3171/jns.1990.73.6.0889
Katchman, A. N., and Hershkowitz, N. (1993). Early anoxia-induced vesicular glutamate release results from mobilization of calcium from intracellular stores. J. Neurophysiol. 70, 1–7.
Kim, A. Y., Jeong, K. H., Lee, J. H., Kang, Y., Lee, S. H., and Baik, E. J. (2017). Glutamate dehydrogenase as a neuroprotective target against brain ischemia and reperfusion. Neuroscience 340, 487–500. doi: 10.1016/j.neuroscience.2016.11.007
Kinkelin, I., Brocker, E. B., Koltzenburg, M., and Carlton, S. M. (2000). Localization of ionotropic glutamate receptors in peripheral axons of human skin. Neurosci. Lett. 283, 149–152. doi: 10.1016/S0304-3940(00)00944-7
Klin, Y., Zlotnik, A., Boyko, M., Ohayon, S., Shapira, Y., and Teichberg, V. I. (2010). Distribution of radiolabeled l-glutamate and d-aspartate from blood into peripheral tissues in naive rats: significance for brain neuroprotection. Biochem. Biophys. Res. Commun. 399, 694–698. doi: 10.1016/j.bbrc.2010.07.144
Kniesel, U., and Wolburg, H. (2000). Tight junctions of the blood-brain barrier. Cell Mol. Neurobiol. 20, 57–76. doi: 10.1023/A:1006995910836
Kress, B. T., Iliff, J. J., Xia, M., Wang, M., Wei, H. S., Zeppenfeld, D., et al. (2014). Impairment of paravascular clearance pathways in the aging brain. Ann. Neurol. 76, 845–861. doi: 10.1002/ana.24271
Kuiper, M. A., Teerlink, T., Visser, J. J., Bergmans, P. L., Scheltens, P., and Wolters, E. C. (2000). L-glutamate, L-arginine and L-citrulline levels in cerebrospinal fluid of Parkinson’s disease, multiple system atrophy, and Alzheimer’s disease patients. J. Neural. Transm. 107, 183–189. doi: 10.1007/s007020050016
Lacaz-Vieira, F., and Marques, M. M. (2003). Pulses of cell Ca(2+) and the dynamics of tight junction opening and closing. J. Membr. Biol. 196, 117–127. doi: 10.1007/s00232-003-0630-2
Le Meur, K., Galante, M., Angulo, M. C., and Audinat, E. (2007). Tonic activation of NMDA receptors by ambient glutamate of non-synaptic origin in the rat hippocampus. J. Physiol. 580(Pt. 2), 373–383. doi: 10.1113/jphysiol.2006.123570
Lee, A., Anderson, A. R., Barnett, N. L., Stevens, M. G., and Pow, D. V. (2012). Alternate splicing and expression of the glutamate transporter EAAT5 in the rat retina. Gene 506, 283–288. doi: 10.1016/j.gene.2012.07.010
Lee, K. H., Huh, J. W., Choi, M. M., Yoon, S. Y., Yang, S. J., Hong, H. N., et al. (2005). Regulation of glutamate level in rat brain through activation of glutamate dehydrogenase by Corydalis ternata. Exp. Mol. Med. 37, 371–377. doi: 10.1038/emm.2005.47
Lee, M., Lee, S. J., Choi, H. J., Jung, Y. W., Frokiaer, J., Nielsen, S., et al. (2008). Regulation of AQP4 protein expression in rat brain astrocytes: role of P2X7 receptor activation. Brain Res. 1195, 1–11. doi: 10.1016/j.brainres.2007.12.023
Lee, M. L., Martinez-Lozada, Z., Krizman, E. N., and Robinson, M. B. (2017). Brain endothelial cells induce astrocytic expression of the glutamate transporter GLT-1 by a Notch-dependent mechanism. J. Neurochem. 143, 489–506. doi: 10.1111/jnc.14135
Lee, S. G., Su, Z. Z., Emdad, L., Gupta, P., Sarkar, D., Borjabad, A., et al. (2008). Mechanism of ceftriaxone induction of excitatory amino acid transporter-2 expression and glutamate uptake in primary human astrocytes. J. Biol. Chem. 283, 13116–13123. doi: 10.1074/jbc.M707697200
Lee, W. J., Hawkins, R. A., Vina, J. R., and Peterson, D. R. (1998). Glutamine transport by the blood-brain barrier: a possible mechanism for nitrogen removal. Am. J. Physiol. 274(4 Pt 1), C1101–C1107.
Lees, K. R. (1997). Cerestat and other NMDA antagonists in ischemic stroke. Neurology 49(5 Suppl. 4), S66–S69. doi: 10.1212/WNL.49.5_Suppl_4.S66
Li, W., Dai, S., An, J., Li, P., Chen, X., Xiong, R., et al. (2008). Chronic but not acute treatment with caffeine attenuates traumatic brain injury in the mouse cortical impact model. Neuroscience 151, 1198–1207. doi: 10.1016/j.neuroscience.2007.11.020
Li, W., Dai, S., An, J., Xiong, R., Li, P., Chen, X., et al. (2009). Genetic inactivation of adenosine A2A receptors attenuates acute traumatic brain injury in the mouse cortical impact model. Exp. Neurol. 215, 69–76. doi: 10.1016/j.expneurol.2008.09.012
Li, X. M., Wendu, R. L., Yao, J., Ren, Y., Zhao, Y. X., Cao, G. F., et al. (2014). Abnormal glutamate metabolism in the retina of aquaporin 4 (AQP4) knockout mice upon light damage. Neurol. Sci. 35, 847–853. doi: 10.1007/s10072-013-1610-7
Li, Y., Maher, P., and Schubert, D. (1998). Phosphatidylcholine-specific phospholipase C regulates glutamate-induced nerve cell death. Proc. Natl. Acad. Sci. U.S.A. 95, 7748–7753. doi: 10.1073/pnas.95.13.7748
Liebner, S., Fischmann, A., Rascher, G., Duffner, F., Grote, E. H., Kalbacher, H., et al. (2000a). Claudin-1 and claudin-5 expression and tight junction morphology are altered in blood vessels of human glioblastoma multiforme. Acta Neuropathol. 100, 323–331. doi: 10.1007/s004010000180
Liebner, S., Kniesel, U., Kalbacher, H., and Wolburg, H. (2000b). Correlation of tight junction morphology with the expression of tight junction proteins in blood-brain barrier endothelial cells. Eur. J. Cell Biol. 79, 707–717. doi: 10.1078/0171-9335-00101
Lin, C. L., Bristol, L. A., Jin, L., Dykes-Hoberg, M., Crawford, T., Clawson, L., et al. (1998). Aberrant RNA processing in a neurodegenerative disease: the cause for absent EAAT2, a glutamate transporter, in amyotrophic lateral sclerosis. Neuron 20, 589–602. doi: 10.1016/S0896-6273(00)80997-6
Liu, H., Zhang, J., Chen, X., Du, X. S., Zhang, J. L., Liu, G., et al. (2016). Application of iron oxide nanoparticles in glioma imaging and therapy: from bench to bedside. Nanoscale 8, 7808–7826. doi: 10.1039/c6nr00147e
Liu, Y. E., Tong, C. C., Zhang, Y. B., Jin, H. X., Gao, Y., and Hou, M. X. (2015). Effect of dexmedetomidine on rats with renal ischemia-reperfusion injury and the expression of tight junction protein in kidney. Int. J. Clin. Exp. Med. 8, 18751–18757.
Longuemare, M. C., Rose, C. R., Farrell, K., Ransom, B. R., Waxman, S. G., and Swanson, R. A. (1999). K(+)-induced reversal of astrocyte glutamate uptake is limited by compensatory changes in intracellular Na+. Neuroscience 93, 285–292. doi: 10.1016/S0306-4522(99)00152-9
Lundgaard, I., Lu, M. L., Yang, E., Peng, W., Mestre, H., Hitomi, E., et al. (2017). Glymphatic clearance controls state-dependent changes in brain lactate concentration. J. Cereb. Blood Flow Metab. 37, 2112–2124. doi: 10.1177/0271678X16661202
Ma, D., Lu, P., Yan, C., Fan, C., Yin, P., Wang, J., et al. (2012). Structure and mechanism of a glutamate-GABA antiporter. Nature 483, 632–636. doi: 10.1038/nature10917
Maas, A. I., Murray, G., Henney, H. III., Kassem, N., Legrand, V., Mangelus, M., et al. (2006). Efficacy and safety of dexanabinol in severe traumatic brain injury: results of a phase III randomised, placebo-controlled, clinical trial. Lancet Neurol. 5, 38–45. doi: 10.1016/S1474-4422(05)70253-2
Machtens, J. P., Kortzak, D., Lansche, C., Leinenweber, A., Kilian, P., Begemann, B., et al. (2015). Mechanisms of anion conduction by coupled glutamate transporters. Cell 160, 542–553. doi: 10.1016/j.cell.2014.12.035
Mally, J., Szalai, G., and Stone, T. W. (1997). Changes in the concentration of amino acids in serum and cerebrospinal fluid of patients with Parkinson’s disease. J. Neurol. Sci. 151, 159–162. doi: 10.1016/S0022-510X(97)00119-6
Martin, J. L., and Finsterwald, C. (2011). Cooperation between BDNF and glutamate in the regulation of synaptic transmission and neuronal development. Commun. Integr. Biol. 4, 14–16. doi: 10.4161/cib.4.1.13761
Massie, A., Vandesande, F., and Arckens, L. (2001). Expression of the high-affinity glutamate transporter EAAT4 in mammalian cerebral cortex. Neuroreport 12, 393–397. doi: 10.1097/00001756-200102120-00041
Mehta, A., Prabhakar, M., Kumar, P., Deshmukh, R., and Sharma, P. L. (2013). Excitotoxicity: bridge to various triggers in neurodegenerative disorders. Eur. J. Pharmacol. 698, 6–18. doi: 10.1016/j.ejphar.2012.10.032
Mendelsohn, A. R., and Larrick, J. W. (2013). Sleep facilitates clearance of metabolites from the brain: glymphatic function in aging and neurodegenerative diseases. Rejuvenation Res. 16, 518–523. doi: 10.1089/rej.2013.1530
Merali, Z., Huang, K., Mikulis, D., Silver, F., and Kassner, A. (2017). Evolution of blood-brain-barrier permeability after acute ischemic stroke. PLOS ONE 12:e0171558. doi: 10.1371/journal.pone.0171558
Miulli, D. E., Norwell, D. Y., and Schwartz, F. N. (1993). Plasma concentrations of glutamate and its metabolites in patients with Alzheimer’s disease. J. Am. Osteopath. Assoc. 93, 670–676.
Morimoto, R., Uehara, S., Yatsushiro, S., Juge, N., Hua, Z., Senoh, S., et al. (2006). Secretion of L-glutamate from osteoclasts through transcytosis. EMBO J. 25, 4175–4186. doi: 10.1038/sj.emboj.7601317
Morley, P., MacLean, S., Gendron, T. F., Small, D. L., Tremblay, R., Durkin, J. P., et al. (2000). Pharmacological and molecular characterization of glutamate receptors in the MIN6 pancreatic beta-cell line. Neurol. Res. 22, 379–385. doi: 10.1080/01616412.2000.11740687
Morrell, C. N., Sun, H., Ikeda, M., Beique, J. C., Swaim, A. M., Mason, E., et al. (2008). Glutamate mediates platelet activation through the AMPA receptor. J. Exp. Med. 205, 575–584. doi: 10.1084/jem.20071474
Murlidharan, G., Crowther, A., Reardon, R. A., Song, J., and Asokan, A. (2016). Glymphatic fluid transport controls paravascular clearance of AAV vectors from the brain. JCI Insight 1:e88034. doi: 10.1172/jci.insight.88034
Naie, K., and Manahan-Vaughan, D. (2004). Regulation by metabotropic glutamate receptor 5 of LTP in the dentate gyrus of freely moving rats: relevance for learning and memory formation. Cereb. Cortex 14, 189–198. doi: 10.1093/cercor/bhg118
Nicholls, D., and Attwell, D. (1990). The release and uptake of excitatory amino acids. Trends Pharmacol. Sci. 11, 462–468. doi: 10.1016/0165-6147(90)90129-V
Nilsson, O. G., Saveland, H., Boris-Moller, F., Brandt, L., and Wieloch, T. (1996). Increased levels of glutamate in patients with subarachnoid haemorrhage as measured by intracerebral microdialysis. Acta Neurochir. Suppl. 67, 45–47. doi: 10.1007/978-3-7091-6894-3_10
Ning, Y. L., Yang, N., Chen, X., Xiong, R. P., Zhang, X. Z., Li, P., et al. (2013). Adenosine A2A receptor deficiency alleviates blast-induced cognitive dysfunction. J. Cereb. Blood Flow Metab. 33, 1789–1798. doi: 10.1038/jcbfm.2013.127
O’Kane, R. L., Martinez-Lopez, I., DeJoseph, M. R., Vina, J. R., and Hawkins, R. A. (1999). Na(+)-dependent glutamate transporters (EAAT1, EAAT2, and EAAT3) of the blood-brain barrier. A mechanism for glutamate removal. J. Biol. Chem. 274, 31891–31895. doi: 10.1074/jbc.274.45.31891
Oldendorf, W. H., and Brown, W. J. (1975). Greater number of capillary endothelial cell mitochondria in brain than in muscle. Proc. Soc. Exp. Biol. Med. 149, 736–738. doi: 10.3181/00379727-149-38889
Papadopoulos, M. C., Saadoun, S., Woodrow, C. J., Davies, D. C., Costa-Martins, P., Moss, R. F., et al. (2001). Occludin expression in microvessels of neoplastic and non-neoplastic human brain. Neuropathol. Appl. Neurobiol. 27, 384–395. doi: 10.1046/j.0305-1846.2001.00341.x
Parpura, V., Basarsky, T. A., Liu, F., Jeftinija, K., Jeftinija, S., and Haydon, P. G. (1994). Glutamate-mediated astrocyte-neuron signalling. Nature 369, 744–747. doi: 10.1038/369744a0
Peet, N. M., Grabowski, P. S., Laketic-Ljubojevic, I., and Skerry, T. M. (1999). The glutamate receptor antagonist MK801 modulates bone resorption in vitro by a mechanism predominantly involving osteoclast differentiation. FASEB J. 13, 2179–2185.
Perez Velazquez, J. L., Frantseva, M. V., and Carlen, P. L. (1997). In vitro ischemia promotes glutamate-mediated free radical generation and intracellular calcium accumulation in hippocampal pyramidal neurons. J. Neurosci. 17, 9085–9094.
Petito, C. K., Chung, M. C., Verkhovsky, L. M., and Cooper, A. J. (1992). Brain glutamine synthetase increases following cerebral ischemia in the rat. Brain Res. 569, 275–280. doi: 10.1016/0006-8993(92)90639-Q
Plog, B. A., Dashnaw, M. L., Hitomi, E., Peng, W., Liao, Y., Lou, N., et al. (2015). Biomarkers of traumatic injury are transported from brain to blood via the glymphatic system. J. Neurosci. 35, 518–526. doi: 10.1523/JNEUROSCI.3742-14.2015
Pomara, N., Singh, R., Deptula, D., Chou, J. C., Schwartz, M. B., and LeWitt, P. A. (1992). Glutamate and other CSF amino acids in Alzheimer’s disease. Am. J. Psychiatry 149, 251–254. doi: 10.1176/ajp.149.2.251
Price, L., Wilson, C., and Grant, G. (2016). “Blood-brain barrier pathophysiology following traumatic brain injury,” in Translational Research in Traumatic Brain Injury, eds D. Laskowitz and G. Grant (Boca Raton, FL: CRC Press).
Qiu, G. P., Xu, J., Zhuo, F., Sun, S. Q., Liu, H., Yang, M., et al. (2015). Loss of AQP4 polarized localization with loss of beta-dystroglycan immunoreactivity may induce brain edema following intracerebral hemorrhage. Neurosci. Lett. 588, 42–48. doi: 10.1016/j.neulet.2014.12.053
Qureshi, A. I., Ali, Z., Suri, M. F., Shuaib, A., Baker, G., Todd, K., et al. (2003). Extracellular glutamate and other amino acids in experimental intracerebral hemorrhage: an in vivo microdialysis study. Crit. Care Med. 31, 1482–1489. doi: 10.1097/01.CCM.0000063047.63862.99
Razafimanjato, H., Garmy, N., Guo, X. J., Varini, K., Di Scala, C., Di Pasquale, E., et al. (2010). The food-associated fungal neurotoxin ochratoxin A inhibits the absorption of glutamate by astrocytes through a decrease in cell surface expression of the excitatory amino-acid transporters GLAST and GLT-1. Neurotoxicology 31, 475–484. doi: 10.1016/j.neuro.2010.06.003
Robelet, S., Melon, C., Guillet, B., Salin, P., and Kerkerian-Le Goff, L. (2004). Chronic L-DOPA treatment increases extracellular glutamate levels and GLT1 expression in the basal ganglia in a rat model of Parkinson’s disease. Eur. J. Neurosci. 20, 1255–1266. doi: 10.1111/j.1460-9568.2004.03591.x
Rogachev, B., Ohayon, S., Saad, A., Vorobiovsky, V., Gruenbaum, B. F., Leibowitz, A., et al. (2012). The effects of hemodialysis on blood glutamate levels in chronic renal failure: implementation for neuroprotection. J. Crit. Care 27, 743.e1-7. doi: 10.1016/j.jcrc.2012.07.002
Rogachev, B., Tsesis, S., Gruenbaum, B. F., Gruenbaum, S. E., Boyko, M., Klein, M., et al. (2013). The effects of peritoneal dialysis on blood glutamate levels: implementation for neuroprotection. J. Neurosurg. Anesthesiol. 25, 262–266. doi: 10.1097/ANA.0b013e318283f86a
Rose, E. M., Koo, J. C., Antflick, J. E., Ahmed, S. M., Angers, S., and Hampson, D. R. (2009). Glutamate transporter coupling to Na,K-ATPase. J. Neurosci. 29, 8143–8155. doi: 10.1523/JNEUROSCI.1081-09.2009
Rosenberg, P. A., Amin, S., and Leitner, M. (1992). Glutamate uptake disguises neurotoxic potency of glutamate agonists in cerebral cortex in dissociated cell culture. J. Neurosci. 12, 56–61.
Rossi, D. J., Oshima, T., and Attwell, D. (2000). Glutamate release in severe brain ischaemia is mainly by reversed uptake. Nature 403, 316–321. doi: 10.1038/35002090
Rothstein, J. D., Dykes-Hoberg, M., Pardo, C. A., Bristol, L. A., Jin, L., Kuncl, R. W., et al. (1996). Knockout of glutamate transporters reveals a major role for astroglial transport in excitotoxicity and clearance of glutamate. Neuron 16, 675–686. doi: 10.1016/S0896-6273(00)80086-0
Rothstein, J. D., Martin, L., Levey, A. I., Dykes-Hoberg, M., Jin, L., Wu, D., et al. (1994). Localization of neuronal and glial glutamate transporters. Neuron 13, 713–725. doi: 10.1016/0896-6273(94)90038-8
Rowland, L. M., Summerfelt, A., Wijtenburg, S. A., Du, X., Chiappelli, J. J., Krishna, N., et al. (2016). Frontal glutamate and gamma-aminobutyric acid levels and their associations with mismatch negativity and digit sequencing task performance in schizophrenia. JAMA Psychiatry 73, 166–174. doi: 10.1001/jamapsychiatry.2015.2680
Rozyczka, J., Figiel, M., and Engele, J. (2004). Endothelins negatively regulate glial glutamate transporter expression. Brain Pathol. 14, 406–414. doi: 10.1111/j.1750-3639.2004.tb00084.x
Ruban, A., Berkutzki, T., Cooper, I., Mohar, B., and Teichberg, V. I. (2012). Blood glutamate scavengers prolong the survival of rats and mice with brain-implanted gliomas. Invest. New Drugs 30, 2226–2235. doi: 10.1007/s10637-012-9794-x
Sattler, R., and Tymianski, M. (2001). Molecular mechanisms of glutamate receptor-mediated excitotoxic neuronal cell death. Mol. Neurobiol. 24, 107–129. doi: 10.1385/MN:24:1-3:107
Schlageter, K. E., Molnar, P., Lapin, G. D., and Groothuis, D. R. (1999). Microvessel organization and structure in experimental brain tumors: microvessel populations with distinctive structural and functional properties. Microvasc. Res. 58, 312–328. doi: 10.1006/mvre.1999.2188
Schousboe, A., and Waagepetersen, H. S. (2005). Role of astrocytes in glutamate homeostasis: implications for excitotoxicity. Neurotox. Res. 8, 221–225. doi: 10.1007/BF03033975
Shen, W., and Slaughter, M. M. (2002). A non-excitatory paradigm of glutamate toxicity. J. Neurophysiol. 87, 1629–1634. doi: 10.1152/jn.00532.2000
Siddharthan, V., Kim, Y. V., Liu, S., and Kim, K. S. (2007). Human astrocytes/astrocyte-conditioned medium and shear stress enhance the barrier properties of human brain microvascular endothelial cells. Brain Res. 1147, 39–50. doi: 10.1016/j.brainres.2007.02.029
Sifat, A. E., Vaidya, B., and Abbruscato, T. J. (2017). Blood-brain barrier protection as a therapeutic strategy for acute ischemic stroke. AAPS J. 19, 957–972. doi: 10.1208/s12248-017-0091-7
Sitcheran, R., Gupta, P., Fisher, P. B., and Baldwin, A. S. (2005). Positive and negative regulation of EAAT2 by NF-kappaB: a role for N-myc in TNFalpha-controlled repression. EMBO J. 24, 510–520. doi: 10.1038/sj.emboj.7600555
Skerry, T. M., and Genever, P. G. (2001). Glutamate signalling in non-neuronal tissues. Trends Pharmacol. Sci. 22, 174–181. doi: 10.1016/S0165-6147(00)01642-4
Smeland, O. B., Hadera, M. G., McDonald, T. S., Sonnewald, U., and Borges, K. (2013). Brain mitochondrial metabolic dysfunction and glutamate level reduction in the pilocarpine model of temporal lobe epilepsy in mice. J. Cereb. Blood Flow Metab. 33, 1090–1097. doi: 10.1038/jcbfm.2013.54
Smith, Q. R. (2000). Transport of glutamate and other amino acids at the blood-brain barrier. J. Nutr. 130(4S Suppl.), 1016S–1022S.
Song, J., Viggiano, A., Monda, M., and De Luca, V. (2014). Peripheral glutamate levels in schizophrenia: evidence from a meta-analysis. Neuropsychobiology 70, 133–141. doi: 10.1159/000364828
Spanagel, R., Pendyala, G., Abarca, C., Zghoul, T., Sanchis-Segura, C., Magnone, M. C., et al. (2005). The clock gene Per2 influences the glutamatergic system and modulates alcohol consumption. Nat. Med. 11, 35–42. doi: 10.1038/nm1163
Stan, A. D., Schirda, C. V., Bertocci, M. A., Bebko, G. M., Kronhaus, D. M., Aslam, H. A., et al. (2014). Glutamate and GABA contributions to medial prefrontal cortical activity to emotion: implications for mood disorders. Psychiatry Res. 223, 253–260. doi: 10.1016/j.pscychresns.2014.05.016
Stefani, M. A., Modkovski, R., Hansel, G., Zimmer, E. R., Kopczynski, A., Muller, A. P., et al. (2017). Elevated glutamate and lactate predict brain death after severe head trauma. Ann. Clin. Transl. Neurol. 4, 392–402. doi: 10.1002/acn3.416
Stevenson, B. R., and Begg, D. A. (1994). Concentration-dependent effects of cytochalasin D on tight junctions and actin filaments in MDCK epithelial cells. J. Cell Sci. 107(Pt 3), 367–375.
Sullan, M. J., Asken, B. M., Jaffee, M. S., DeKosky, S. T., and Bauer, R. M. (2017). Glymphatic system disruption as a mediator of brain trauma and chronic traumatic encephalopathy. Neurosci. Biobehav. Rev. doi: 10.1016/j.neubiorev.2017.08.016 [Epub ahead of print].
Sun, D. A., Deshpande, L. S., Sombati, S., Baranova, A., Wilson, M. S., Hamm, R. J., et al. (2008). Traumatic brain injury causes a long-lasting calcium (Ca2+)-plateau of elevated intracellular Ca levels and altered Ca2+ homeostatic mechanisms in hippocampal neurons surviving brain injury. Eur. J. Neurosci. 27, 1659–1672. doi: 10.1111/j.1460-9568.2008.06156.x
Sun, Y. N., Liu, L. B., Xue, Y. X., and Wang, P. (2015). Effects of insulin combined with idebenone on blood-brain barrier permeability in diabetic rats. J. Neurosci. Res. 93, 666–677. doi: 10.1002/jnr.23511
Swanson, C. J., Bures, M., Johnson, M. P., Linden, A. M., Monn, J. A., and Schoepp, D. D. (2005). Metabotropic glutamate receptors as novel targets for anxiety and stress disorders. Nat. Rev. Drug Discov. 4, 131–144. doi: 10.1038/nrd1630
Szatkowski, M., and Attwell, D. (1994). Triggering and execution of neuronal death in brain ischaemia: two phases of glutamate release by different mechanisms. Trends Neurosci. 17, 359–365. doi: 10.1016/0166-2236(94)90040-X
Szatkowski, M., Barbour, B., and Attwell, D. (1990). Non-vesicular release of glutamate from glial cells by reversed electrogenic glutamate uptake. Nature 348, 443–446. doi: 10.1038/348443a0
Tajes, M., Ramos-Fernandez, E., Weng-Jiang, X., Bosch-Morato, M., Guivernau, B., Eraso-Pichot, A., et al. (2014). The blood-brain barrier: structure, function and therapeutic approaches to cross it. Mol. Membr. Biol. 31, 152–167. doi: 10.3109/09687688.2014.937468
Takano, T., Kang, J., Jaiswal, J. K., Simon, S. M., Lin, J. H., Yu, Y., et al. (2005). Receptor-mediated glutamate release from volume sensitive channels in astrocytes. Proc. Natl. Acad. Sci. U.S.A. 102, 16466–16471. doi: 10.1073/pnas.0506382102
Teichberg, V. I., Cohen-Kashi-Malina, K., Cooper, I., and Zlotnik, A. (2009). Homeostasis of glutamate in brain fluids: an accelerated brain-to-blood efflux of excess glutamate is produced by blood glutamate scavenging and offers protection from neuropathologies. Neuroscience 158, 301–308. doi: 10.1016/j.neuroscience.2008.02.075
Tewes, B. J., and Galla, H. J. (2001). Lipid polarity in brain capillary endothelial cells. Endothelium 8, 207–220. doi: 10.1080/10623320109051566
Thanabalasundaram, G., Pieper, C., Lischper, M., and Galla, H. J. (2010). Regulation of the blood-brain barrier integrity by pericytes via matrix metalloproteinases mediated activation of vascular endothelial growth factor in vitro. Brain Res. 1347, 1–10. doi: 10.1016/j.brainres.2010.05.096
Thrane, A. S., Rangroo Thrane, V., and Nedergaard, M. (2014). Drowning stars: reassessing the role of astrocytes in brain edema. Trends Neurosci. 37, 620–628. doi: 10.1016/j.tins.2014.08.010
Tisell, A., Leinhard, O. D., Warntjes, J. B., Aalto, A., Smedby, O., Landtblom, A. M., et al. (2013). Increased concentrations of glutamate and glutamine in normal-appearing white matter of patients with multiple sclerosis and normal MR imaging brain scans. PLOS ONE 8:e61817. doi: 10.1371/journal.pone.0061817
Tymianski, M., and Tator, C. H. (1996). Normal and abnormal calcium homeostasis in neurons: a basis for the pathophysiology of traumatic and ischemic central nervous system injury. Neurosurgery 38, 1176–1195.
Umemura, K., Gemba, T., Mizuno, A., and Nakashima, M. (1996). Inhibitory effect of MS-153 on elevated brain glutamate level induced by rat middle cerebral artery occlusion. Stroke 27, 1624–1628. doi: 10.1161/01.STR.27.9.1624
Underhill, S. M., Wheeler, D. S., Li, M., Watts, S. D., Ingram, S. L., and Amara, S. G. (2014). Amphetamine modulates excitatory neurotransmission through endocytosis of the glutamate transporter EAAT3 in dopamine neurons. Neuron 83, 404–416. doi: 10.1016/j.neuron.2014.05.043
Unger, T., Bette, S., Zhang, J., Theis, M., and Engele, J. (2012). Connexin-deficiency affects expression levels of glial glutamate transporters within the cerebrum. Neurosci. Lett. 506, 12–16. doi: 10.1016/j.neulet.2011.10.032
van den Pol, A. N., Wuarin, J. P., and Dudek, F. E. (1990). Glutamate, the dominant excitatory transmitter in neuroendocrine regulation. Science 250, 1276–1278. doi: 10.1126/science.1978759
van Landeghem, F. K., Weiss, T., Oehmichen, M., and von Deimling, A. (2006). Decreased expression of glutamate transporters in astrocytes after human traumatic brain injury. J. Neurotrauma 23, 1518–1528. doi: 10.1089/neu.2006.23.1518
van Meer, G., and Simons, K. (1986). The function of tight junctions in maintaining differences in lipid composition between the apical and the basolateral cell surface domains of MDCK cells. EMBO J. 5, 1455–1464.
van Vliet, E. A., da Costa Araujo, S., Redeker, S., van Schaik, R., Aronica, E., and Gorter, J. A. (2007). Blood-brain barrier leakage may lead to progression of temporal lobe epilepsy. Brain 130(Pt 2), 521–534. doi: 10.1093/brain/awl318
Vazana, U., Veksler, R., Pell, G. S., Prager, O., Fassler, M., Chassidim, Y., et al. (2016). Glutamate-mediated blood-brain barrier opening: implications for neuroprotection and drug delivery. J. Neurosci. 36, 7727–7739. doi: 10.1523/JNEUROSCI.0587-16.2016
Vespa, P., Prins, M., Ronne-Engstrom, E., Caron, M., Shalmon, E., Hovda, D. A., et al. (1998). Increase in extracellular glutamate caused by reduced cerebral perfusion pressure and seizures after human traumatic brain injury: a microdialysis study. J. Neurosurg. 89, 971–982. doi: 10.3171/jns.1998.89.6.0971
Weissberg, I., Veksler, R., Kamintsky, L., Saar-Ashkenazy, R., Milikovsky, D. Z., Shelef, I., et al. (2014). Imaging blood-brain barrier dysfunction in football players. JAMA Neurol. 71, 1453–1455. doi: 10.1001/jamaneurol.2014.2682
Wen, Z. H., Chang, Y. C., and Jean, Y. H. (2015). Excitatory amino acid glutamate: role in peripheral nociceptive transduction and inflammation in experimental and clinical osteoarthritis. Osteoarthritis Cartilage 23, 2009–2016. doi: 10.1016/j.joca.2015.03.017
Whitelaw, B. S., and Robinson, M. B. (2013). Inhibitors of glutamate dehydrogenase block sodium-dependent glutamate uptake in rat brain membranes. Front. Endocrinol. 4:123. doi: 10.3389/fendo.2013.00123
Wilhelm, A., Volknandt, W., Langer, D., Nolte, C., Kettenmann, H., and Zimmermann, H. (2004). Localization of SNARE proteins and secretory organelle proteins in astrocytes in vitro and in situ. Neurosci. Res. 48, 249–257. doi: 10.1016/j.neures.2003.11.002
Wittchen, E. S., Haskins, J., and Stevenson, B. R. (1999). Protein interactions at the tight junction. Actin has multiple binding partners, and ZO-1 forms independent complexes with ZO-2 and ZO-3. J. Biol. Chem. 274, 35179–35185. doi: 10.1074/jbc.274.49.35179
Yanagisawa, M., Aida, T., Takeda, T., Namekata, K., Harada, T., Shinagawa, R., et al. (2015). Arundic acid attenuates retinal ganglion cell death by increasing glutamate/aspartate transporter expression in a model of normal tension glaucoma. Cell Death Dis. 6:e1693. doi: 10.1038/cddis.2015.45
Yang, J., Zhang, R., Shi, C., Mao, C., Yang, Z., Suo, Z., et al. (2017). AQP4 association with amyloid deposition and astrocyte pathology in the Tg-ArcSwe mouse model of Alzheimer’s disease. J. Alzheimers Dis. 57, 157–169. doi: 10.3233/JAD-160957
Yang, L., Kress, B. T., Weber, H. J., Thiyagarajan, M., Wang, B., Deane, R., et al. (2013). Evaluating glymphatic pathway function utilizing clinically relevant intrathecal infusion of CSF tracer. J. Transl. Med. 11, 107. doi: 10.1186/1479-5876-11-107
Yang, S. J., Kim, E. A., Chang, M. J., Kim, J., Na, J. M., Choi, S. Y., et al. (2017). N-adamantyl-4-methylthiazol-2-amine attenuates glutamate-induced oxidative stress and inflammation in the brain. Neurotox. Res. 32, 107–120. doi: 10.1007/s12640-017-9717-x
Ye, Z. C., Wyeth, M. S., Baltan-Tekkok, S., and Ransom, B. R. (2003). Functional hemichannels in astrocytes: a novel mechanism of glutamate release. J. Neurosci. 23, 3588–3596.
Zeng, L. H., Bero, A. W., Zhang, B., Holtzman, D. M., and Wong, M. (2010). Modulation of astrocyte glutamate transporters decreases seizures in a mouse model of tuberous sclerosis complex. Neurobiol. Dis. 37, 764–771. doi: 10.1016/j.nbd.2009.12.020
Zhang, Z., Tao, Z., Gameiro, A., Barcelona, S., Braams, S., Rauen, T., et al. (2007). Transport direction determines the kinetics of substrate transport by the glutamate transporter EAAC1. Proc. Natl. Acad. Sci. U.S.A. 104, 18025–18030. doi: 10.1073/pnas.0704570104
Zhao, Z., Nelson, A. R., Betsholtz, C., and Zlokovic, B. V. (2015). Establishment and dysfunction of the blood-brain barrier. Cell 163, 1064–1078. doi: 10.1016/j.cell.2015.10.067
Zhao, Z. A., Li, P., Ye, S. Y., Ning, Y. L., Wang, H., Peng, Y., et al. (2017). Perivascular AQP4 dysregulation in the hippocampal CA1 area after traumatic brain injury is alleviated by adenosine A2A receptor inactivation. Sci. Rep. 7:2254. doi: 10.1038/s41598-017-02505-6
Zhou, Y., and Danbolt, N. C. (2014). Glutamate as a neurotransmitter in the healthy brain. J. Neural. Transm. 121, 799–817. doi: 10.1007/s00702-014-1180-8
Zhou, Z., Pausch, F., Schlotzer-Schrehardt, U., Brachvogel, B., and Poschl, E. (2016). Induction of initial steps of angiogenic differentiation and maturation of endothelial cells by pericytes in vitro and the role of collagen IV. Histochem. Cell Biol. 145, 511–525. doi: 10.1007/s00418-015-1398-z
Zlotnik, A., Gruenbaum, B. F., Klin, Y., Gruenbaum, S. E., Ohayon, S., Sheiner, E., et al. (2011a). The effects of insulin, glucagon, glutamate, and glucose infusion on blood glutamate and plasma glucose levels in naive rats. J. Neurosurg. Anesthesiol. 23, 323–328. doi: 10.1097/ANA.0b013e3182299b15
Zlotnik, A., Gruenbaum, S. E., Artru, A. A., Rozet, I., Dubilet, M., Tkachov, S., et al. (2009). The neuroprotective effects of oxaloacetate in closed head injury in rats is mediated by its blood glutamate scavenging activity: evidence from the use of maleate. J. Neurosurg. Anesthesiol. 21, 235–241. doi: 10.1097/ANA.0b013e3181a2bf0b
Zlotnik, A., Gurevich, B., Tkachov, S., Maoz, I., Shapira, Y., and Teichberg, V. I. (2007). Brain neuroprotection by scavenging blood glutamate. Exp. Neurol. 203, 213–220. doi: 10.1016/j.expneurol.2006.08.021
Zlotnik, A., Ohayon, S., Gruenbaum, B. F., Gruenbaum, S. E., Mohar, B., Boyko, M., et al. (2011b). Determination of factors affecting glutamate concentrations in the whole blood of healthy human volunteers. J. Neurosurg. Anesthesiol. 23, 45–49. doi: 10.1097/ANA.0b013e3181f82a8f
Zlotnik, A., Sinelnikov, I., Gruenbaum, B. F., Gruenbaum, S. E., Dubilet, M., Dubilet, E., et al. (2012). Effect of glutamate and blood glutamate scavengers oxaloacetate and pyruvate on neurological outcome and pathohistology of the hippocampus after traumatic brain injury in rats. Anesthesiology 116, 73–83. doi: 10.1097/ALN.0b013e31823d7731
Keywords: glutamate, blood–brain barrier, concentration gradient, brain diseases, glutamate transporter, endothelial cell
Citation: Bai W and Zhou Y-G (2017) Homeostasis of the Intraparenchymal-Blood Glutamate Concentration Gradient: Maintenance, Imbalance, and Regulation. Front. Mol. Neurosci. 10:400. doi: 10.3389/fnmol.2017.00400
Received: 26 June 2017; Accepted: 20 November 2017;
Published: 05 December 2017.
Edited by:
Detlev Boison, Legacy Health, United StatesReviewed by:
Nikki Katherine Lytle, University of California, San Diego, United StatesChris Dulla, Tufts University School of Medicine, United States
Copyright © 2017 Bai and Zhou. This is an open-access article distributed under the terms of the Creative Commons Attribution License (CC BY). The use, distribution or reproduction in other forums is permitted, provided the original author(s) or licensor are credited and that the original publication in this journal is cited, in accordance with accepted academic practice. No use, distribution or reproduction is permitted which does not comply with these terms.
*Correspondence: Yuan-Guo Zhou, eWd6aG91QHRtbXUuZWR1LmNu;, emhvdXJpY2tAaG90bWFpbC5jb20=