- 1Department of Genetics and Molecular Neurobiology, Institute of Biology, Otto-von-Guericke University Magdeburg, Magdeburg, Germany
- 2Institute of Molecular and Clinical Immunology, Health Campus Immunology, Infectiology and Inflammation, Otto-von-Guericke University Magdeburg, Magdeburg, Germany
- 3Center for Behavioral Brain Science, Magdeburg, Germany
The serine/threonine kinase Ndr2 has been shown to control the inside-out activation of the β1subunit of integrins and the formation of neurites in both primary neurons and neurally differentiated pheochromacytoma (PC12) cells. In this study, we demonstrate that Ndr2 kinase furthermore determines the substrate specificity of neurite extension in PC12 cells via expression of α1β1 integrins. We show that stable overexpression of Ndr2 in PC12 cells increases neurite growth and extension on poly-D-lysine substrate, likely involving an increased expression of active β1 integrin in the growth tips of these cells. By contrast, the Ndr2 overexpressing cells do not show the α1β1 integrin-mediated enhancement of neurite growth on collagen IV substrate that can be seen in control cells. Moreover, they entirely fail to increase in response to activation of α1β1 integrins via a soluble KTS ligand and show a diminished accumulation of α1 integrin in neurite tips, although the expression of this subunit is induced during differentiation to comparable levels as in control cells. Finally, we demonstrate that Ndr2 overexpression similarly inhibits the α1β1 integrin-dependent dendritic growth of primary hippocampal neurons on laminin 111 substrate. By contrast, lack of Ndr2 impairs the dendritic growth regardless of the substrate. Together, these results suggest that Ndr2 regulates α1 integrin trafficking in addition to β1 integrin subunit activation and thereby controls the neurite growth on different extracellular matrix (ECM) substrates.
Introduction
Ndr2 (also termed serine/threonine kinase 38-like protein, STK38l) together with its close homolog Ndr1, as well as Lats1/2 forms the conserved family of nuclear Dbf2-related (Ndr) kinases. As targets of the Hippo signaling pathway, these kinases are involved in gene regulation and regulation of cytoskeleton dynamics during cell division and differentiation (Emoto, 2011). Earlier studies showed that the Ndr kinase homolog in yeasts, Dbf2p, is essential for the polarization of cells during mitosis (Frenz et al., 2000). Further investigations in higher organisms demonstrated a prominent expression of Ndr2 and its homologs in neuronal tissues and a role in neuronal and brain development. While the Ndr2 homolog in C. elegans, SAX-1, is required for neuronal shape and neurite initiation, Tricornered (Trc) in D. melanogaster is important for dendritic branching/tiling (Zallen et al., 2000; Emoto et al., 2006). Our own work revealed prominent expression of Ndr2 in the central nervous system and an association with actin cytoskeleton in soma, neurites and spines in isolated hippocampal neurons (Rehberg et al., 2014). We could furthermore demonstrate an enhancement of neurite outgrowth through Ndr2 overexpression, using rat pheochromocytoma (PC12) cells as a model system (Stork et al., 2004). Further research confirmed that Ndr2 is indeed critical for neuronal polarization (Yang et al., 2014) and dendritic differentiation in mammalian neurons. Accordingly, Ndr2-deficient mice and rats show arbor specific alterations and premature branching in the hippocampus and neocortex (Ultanir et al., 2012; Rehberg et al., 2014). Addressing the cellular mechanisms of these functions, we could previously show that Ndr2 controls dendritic and axonal growth in mouse hippocampal neurons by affecting the activation state and endosomal trafficking of β1 integrin subunits to the dendritic surface (Rehberg et al., 2014).
Integrins are heterodimeric membrane receptors, consisting of one alpha and one beta subunit. Eighteen α and eight β subunits form 24 different integrin dimers with specific affinities to different extracellular ligands. These distinct integrin dimers have particular roles in regulating the attachment and spreading of the cells on various extracellular matrix (ECM) components (Stukel and Willits, 2016). In addition to mediating cell adhesion, integrins transmit information from the ECM and diffusible external growth cues in order to modulate the actin cytoskeleton and intracellular signaling pathways (Schmid and Anton, 2003; Calderwood, 2004). Their availability on the cell surface is an important control mechanism of integrin activity and integrin trafficking to the membrane is tightly regulated (Caswell et al., 2009; Bridgewater et al., 2012). Moreover, depending on both intra- and extracellular factors surface integrins can assume different conformational states with low, medium and high binding affinity (Humphries et al., 2003; Shattil et al., 2010). Neurons express a variety of integrin heterodimers which allow them to detect different ECM proteins; for example α1β1 and α2β1 bind to collagens, whereas α6β1 binds to laminin (Plow et al., 2000; Hynes, 2002). However, which intracellular mechanisms control the expression and distribution of integrin subtypes during neuronal development has remained largely unresolved so far.
Based on our previous findings implicating Ndr2 in the control of β1 integrin-dependent neurite growth, we here addressed the question whether this kinase modulates neurite outgrowth in an ECM-specific manner and examined the contribution of the α1 integrin subunit to this function. To this end we first used PC12 cells, which recruit different integrin subtypes to regulate their adhesion and neurite extension in response to ECM (Tomaselli et al., 1988; Lein et al., 2000) and provide a widely used model to examine to intracellular signaling related to neurite outgrowth (Harrill and Mundy, 2011). With a previously established line of Ndr2 overexpressing PC12 cells (Stork et al., 2004), we determined the differential effect of Ndr2 kinase on neurite growth on different ECM substrates. We further investigated how enhancement of integrin adhesion by divalent cations or a synthetic α1β1-specific KTS ligand affects the Ndr2-dependent neurite extension. Moreover, we determined the effects of Ndr2 overexpression on the amount of integrin subunits in the neurite tips of differentiating PC12 cells. Finally, we confirmed the role of Ndr2 in the substrate-specific regulation of primary hippocampal neurons dendritic growth. Our data provide evidence that Ndr2 kinase regulates both α1 integrin trafficking and β1 integrin activation to control the neurite morphology of the cell on different ECM substrates in PC12 cells and primary neurons.
Materials and Methods
Mice
Primary hippocampal neurons were derived from C57BL/6JBomTac mice (Taconic) mice, bred and raised in the animal facility at the Institute of Biology, Otto-von-Guericke University Magdeburg. Animal maintenance were done according to the guidelines of State of Saxony-Anhalt, Germany and approved by the Landesverwaltungsamt Sachsen-Anhalt (permission number: 42502-2-1177 Uni MD).
Cell Culture
Rat pheochromocytoma (PC12) cells were maintained in RPMI medium supplemented with 10% horse serum, 5% fetal bovine serum and 100 U/mL Penicillin-Streptomycin (Gibco). EGFP expressing control PC12 cells (EGFP PC12) and EGFP-Ndr2 expressing PC12 cells (Ndr2 PC12) were previously established (Stork et al., 2004), stably transfected cells were maintained with 200 μg/ml G418 (Invitrogen) at 37°C under 5% CO2 incubation. For differentiation, cells were seeded (35,000 cells/cm2 for neurite growth assays and 5000 cells/cm2 for immunocytochemistry) on substrate coated glass coverslips (either with poly-d-lysine only (10 μg/cm2 in 0.15 M Borate buffer (pH 8.4), Sigma-Aldrich) or following substrates were added on poly-D-lysine (PDL) coated coverslips: fibronectin (3 μg/cm2 in water, Biochrom), laminin (5 μg/cm2 in serum-free RPMI media, BD Bioscience), gelatin (100 μg/cm2 in water, Biochrom), collagen I (10 μg/cm2 in 30% Ethanol, Sigma-Aldrich) and collagen IV (10 μg/cm2 in 30% Ethanol, Sigma-Aldrich) and cultured in low RPMI medium (RPMI with 0.2% horse serum, 1× PS and 200 μg/ml G418) supplemented with 50 ng/ml neurite growth factor (NGF, Gibco). To enhance general integrin binding, 0.3 mM MgCl2 were added to the low RPMI medium. In another set of experiments, a synthetic peptide bearing the α1β1 integrin-specific binding motif KTS (CWKTSLTSHYC, generated and purified by Pineda Antibody Service, Berlin, Germany) was applied to the cell media at a concentration of 140 μM to stimulate α1β1 integrin heterodimers specifically without altering the cell adhesion on collagen IV substrate (Moreno-Murciano et al., 2003).
Neurite Growth Assays
After 4 days of NGF treatment, cells were briefly washed with phosphate buffered saline (PBS) and fixed with 4% para-formaldehyde (PFA)/Sucrose in PBS. Images of PC12 cells were taken using an inverted Axiovert 200 M microscope (Zeiss) at 10× objective under phase-contrast filter. Cells with neurites and cells with neurites longer than 100 μm were counted separately using AxioVision 4.0 (Zeiss) and values of each trial were normalized against PDL-control conditions.
Immunocytochemistry
To stain for β1 integrin phosphorylated at threonine788/789 (β1pThr788/789), PC12 cells were stimulated with NGF as in neurite growth assays. Cells were fixed with 4% PFA/sucrose in PBS and permeabilized with 0.3% TritonX for 10 min at room temperature. Coverslips were incubated with 5% bovine serum albumin (Roth) and 5% donkey serum (Linaris) for 2 h at room temperature to block unspecific binding. Immunocytochemistry was done using a pThr788/789 β1 integrin antibody (1:200, Abcam ab5189). Cells were incubated with the primary antibody overnight at 4°C, washed with PBS and incubated with Alexa-conjugated secondary antibodies (1:1000 dilution in PBS, Invitrogen) for 1 h at room temperature. For staining of α1 integrin, PC12 cells were grown for a prolonged period of 6 days, according to Zhang et al. (1993), to allow for high expression levels. Cells were fixed, permeabilized and stained using α1 integrin primary antibody (Abcam, ab78479; diluted 1:200 in PBS). Alexa-coupled secondary antibody was applied together with rhodamine-phalloidine (1:40, R415- Molecular Probes) according to the manufacturer’s protocol to detect the actin cytoskeleton. Cells were finally mounted to slide glasses using ImmuMount (Thermo Scientific) and images were captured with Meta 510 (Zeiss) and DMI 6000 (Leica) microscopes. Images were randomized and evaluated by a researcher who was blinded with respect to the cell line. The most prominent growth tip of differentiated PC12 cells was selected and fluorescent intensities in the growth tips and cell bodies were quantified using ImageJ (NIH) software with built-in histogram and measure functions.
Western Blotting
NGF-treated PC12 cells (0 day, 3 days and 6 days NGF treatment (50 ng/ml)) were briefly washed with pre-warmed PBS and lysed in lysis buffer (1% lauryl maltoside N-dodycyl-β-D-maltoside (Merck), 1% NP-40 (Sigma Aldrich), 1 mM Na-orthovanadate, 2 mM EDTA, 50 mM Tris-HCl, 150 mM NaCl, 0.5% deoxycholic acid, 1 mM 4-(2-Aminoethyl)benzenesulfonyl fluoride hydrochloride, 1 μM Pepstatin A, 1 mM NaF and protease inhibitor cocktail (one tablet per 50 ml, Pierce)). Proteins were separated according to their size on polyacrylamide gels and transferred to PVDF membranes (Immobilon FL, Millipore). Membranes were blocked with 5% milk powder (Roth) in PBS for 2 h in room temperature and incubated with α1 integrin (1:1000 dilution in PBS+0.1% Tween; Abcam78479) and α-tubulin (1:5000 dilution in PBS+0.1% Tween; Sigma Aldrich T6199) antibodies overnight at 4°C. Membranes were briefly washed with PBS and fluorescently labeled IRDye secondary antibodies (Licor) was used with 1:15,000 dilution in PBS+0.1% Tween. After 1 h incubation at room temperature, membranes were washed three times with PBS+0.2% Tween and visualized using an Odyssey scanner (Licor).
Primary Cell Culture
Hippocampi from embryonic day 18 (E18) to E19 mice were dissected and disassociated using MACS Neural Tissue Dissociation Kit (Milteny). Disassociated cells were plated either on poly-D-lysine (10 μg/cm2, Sigma-Aldrich) or poly-D-lysine+ Laminin111 (0.45 μg/cm2, in PBS, Biolamina) coated coverslips (25,000 cells/cm2). Except for the plating media (DMEM+ 10% FBS (v/v) + L-GlutaMax (2 mM)) at day in vitro (DIV0), neurons were kept with neurobasal media containing B27 (2% v/v) and L-GlutaMax (0.5 mM) (all from Invitrogen) that had been glia conditioned for 72 h. At DIV3, neurons were transfected either with EGFP-C1 (ClonTech), EGFP-Ndr2 (Stork et al., 2004), pLL3.7_shLuc (TTG GAG AAA AGC CTT GTT T) or pLL3.7_shNdr2 (CCT CAT CTG CCA ATC CCT C; Rehberg et al., 2014) using CalPhos Mammalian Transfection kit according to the manufacturer instructions (ClonTech). All neurons were also co-transfected with pCMV-tdTomato (ClonTech) to outline the dendritic morphology. At DIV7, primary neurons were fixed with 4% paraformaldehyde and 4% sucrose in 0.1 M PBS, mounted on slides using Immuno-Mount (Thermo Scientific) and images were taken using a DMI 6000 epifluorescence microscope (Leica). Dendrites of the double-transfected neurons were selected according to their morphological features (Kaech and Banker, 2006) and were traced using QWin software (Leica). Sholl analysis of the traced dendrites was done using Fiji (ImageJ) with 10 μm radius intervals.
Statistics
Statistical analysis was performed using two-way ANOVA followed by Fisher Least Significant (LSD) test or Kruskal-Wallis test for multiple comparisons. Immunofluorescence data were log-transformed before statistical analysis. The number of cells with long neurites (>100 μm) and short neurites (<100 μm) were compared using Chi-square tests. Pairwise comparisons were done using Student’s t-test or Mann-Whitney test when appropriate and a statistical threshold for significance was set at p < 0.05. Normality of the datasets was tested using Shapiro-Wilk test.
Results
Ndr2 Is Involved in PC12 Cells Neurite Extension on Different Substrates
To investigate the role of Ndr2 kinase in neurite growth, EGFP transfected PC12 cells (EGFP PC12) and Ndr2 transfected PC12 cells (Ndr2 PC12) were cultured on different ECM substrates and treated with NGF. Both cell lines showed efficient neurite formation on PDL and collagen IV with >95% of cells forming discernable neurites under each condition. Laminin also was a favorable substrate for both lines, whereas less than 50% of control cells showed neurites on fibronectin, collagen I and gelatin. On those less efficient substrates, an increase of outgrowth in Ndr2 cells was evident, as the proportion of cells with neurites were significantly increased on fibronectin, gelatin and collagen I substrates (Figure 1A, two-way ANOVA genotype × substrate interaction: F(5,24) = 4.575, p = 0.0045). To address potential differences in neurite extension, we further analyzed cells with neurites longer than 100 μm (Figure 1B). Here we have selected PDL, Laminin and Collagen IV substrates since they resulted in neuronal differentiation in more than 90% of cells in both cell lines. Here we confirmed the previously reported increase in Ndr2 PC12 cells neurite extension of on PDL (X2(1) = 4.276, p < 0.05, Stork et al., 2004), but did not observe any change in neurite extension on laminin substrate between genotypes (X2(1) = 0.1364, p = 0.71). In contrast to PDL, on collagen IV substrate Ndr2 PC12 cells displayed significantly reduced rather than increased neurite growth compared to EGFP PC12 cells (Figures 1B,C; X2(1) = 81.45, p < 0.001). Therefore, PDL and collagen IV were chosen as substrates for further experiments to investigate how Ndr2 kinase controls substrate specific neural differentiation of PC12 cells.
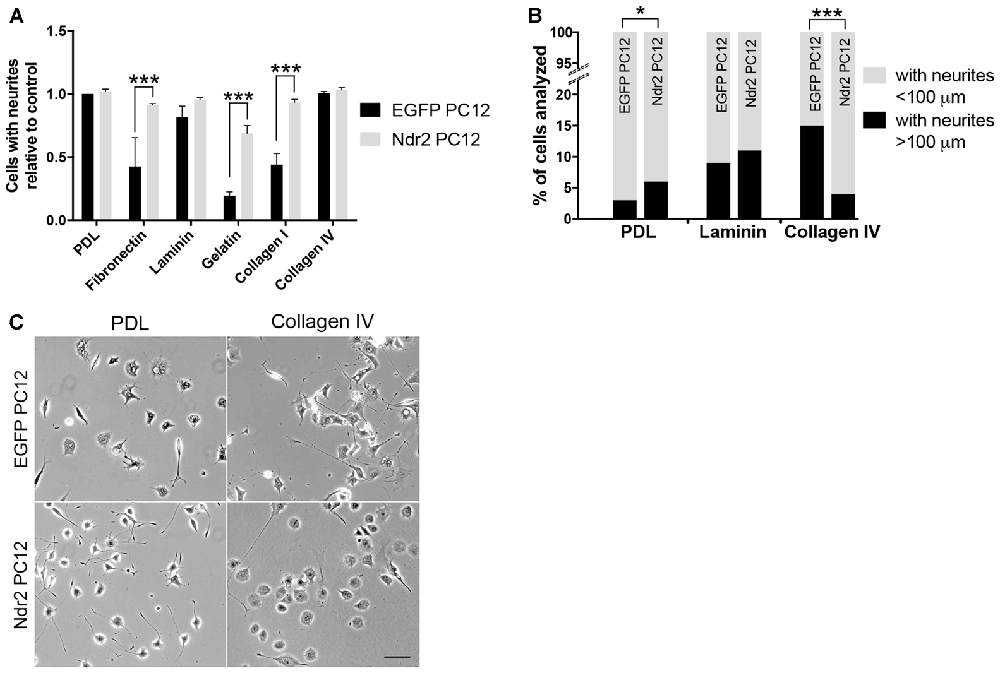
Figure 1. Substrate-specific modulation of neurite outgrowth through Nuclear Dbf2-related 2 (Ndr2). (A) The proportion of cells with neurites is increased on fibronectin, gelatin and collagen I in Ndr2 PC12 cells, while the more permissive substrates poly-D-lysine (PDL), laminin and collagen IV show generally high neurite formation in both Ndr2 rat pheochromocytoma cells (PC12) and EGFP PC12 control cells (mean + SEM, N = 3, two-way ANOVA genotype × substrate interaction: F(5,24) = 4.575, p = 0.0045). (B) In line with a generally increased neurite growth, the Ndr2 PC12 cells also show an increase in the proportion of cells bearing long neurites (>100 μm) on PDL substrate (X2(1) = 4.276, p < 0.05). However, the Ndr2 PC12 cells fail to show the enhancement of neurite extension on collagen IV substrate that is evident in EGFP PC12 controls (X2(1) = 81.45, p < 0.001). (C) Microscopic images of EGFP PC12 and Ndr2 PC12 cells on PDL and Collagen IV substrates displaying the differences in neurite numbers and morphologies. *p < 0.05; ***p < 0.001. Scale bars: 100 μm.
Ndr2 Controls the β1 Integrin Activity in Neurite Tips
Integrin receptors on the cell surface are recruited by these ECM substrates and previous studies showed that β1 integrin containing integrin heterodimers are the main receptors for their signal transmission in developing neurons (Denda and Reichardt, 2007; Lei et al., 2012). Since we have previously described the role of Ndr2 in the “inside-out” activation of β1 integrins, we next tested whether this process may be involved in the differential response of Ndr2 PC12 cells to integrin substrates. Immunohistochemical staining of β1pThr788/789, which is required for β1 integrin activation (Nilsson et al., 2006), revealed an expression of this activated β1 integrin form in both somata and neurite tips of both control cells and Ndr2 PC12 cells (Figures 2A–F). Quantification of the relative amount of β1pThr788/789 revealed no main effect of the coating substrate (two-way ANOVA, substrate effect: F(1,36) = 0.05323, p = 0.8188). However, an increase of β1pThr788/789 was observed in the neurite tips of Ndr2 PC12 cells compared to control cells (two-way ANOVA genotype effect: F(1,36)= 7.407 p < 0.01), regardless of the coating substrate (genotype × substrate interaction: F(1,36)= 0.1485, p = 0.7022; Figure 2G). Furthermore, while collagen IV substrate decreased the growth cone size of control cells, Ndr2 PC12 cells did not change their growth cone size upon collagen IV coating (genotype × substrate interaction: F(1,35) = 15.67, p = 0.0004; Figure 2H). However, β1pThr788/789 raw fluorescence results were independent of the growth cone sizes, since fluorescence intensity of β1pThr788/789 per μm2 of growth cones revealed only a genotype difference between EGFP and Ndr2 PC12 cells as in raw fluorescence results (two-way ANOVA genotype effect: F(1,36)= 4.867, p = 0.0338; data not shown).
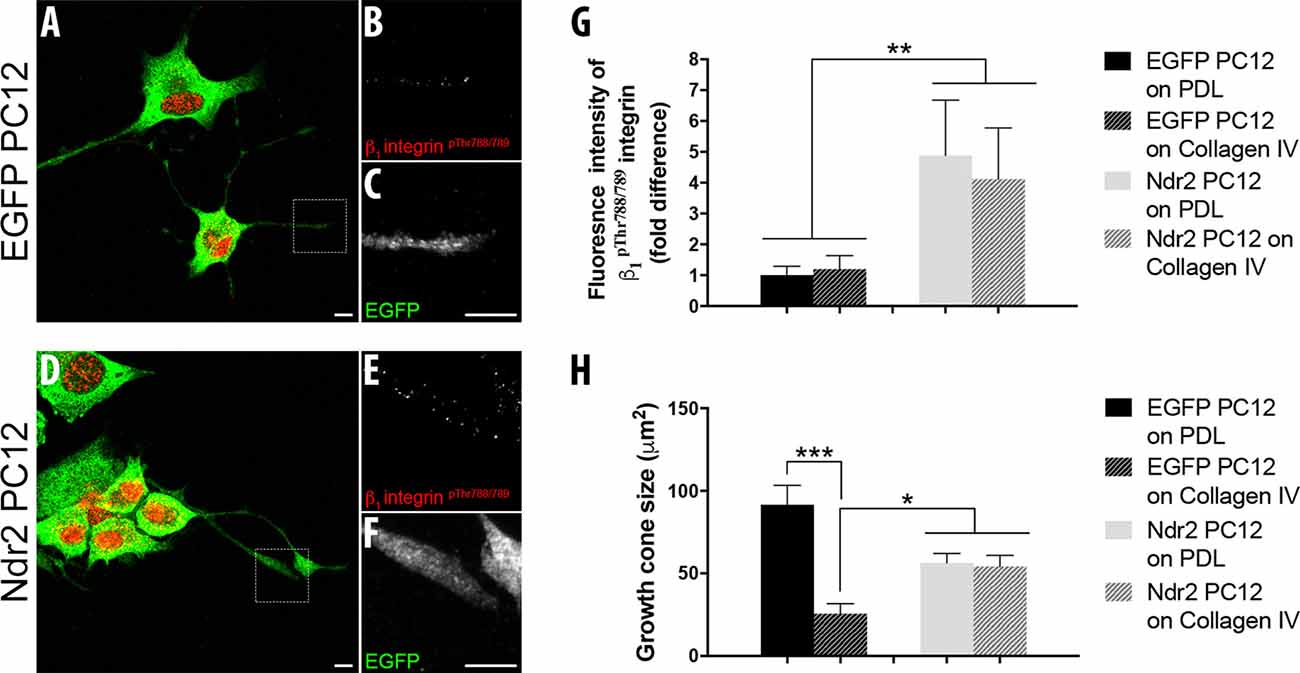
Figure 2. Ndr2 increases the expression of activated β1 integrins at neurite tips regardless of the extracellular matrix (ECM) substrate. Immunocytochemistry of differentiating EGFP PC12 cells (A–C) and Ndr2 PC12 cells (D–F). A closer look into the growth cones of Ndr2 PC12 cells reveals the co-localization of activated β1 integrin (pThr788/789) (E) and Ndr2 (F) at neurite tips. Scale bars: 10 μm. (G) Quantitative analysis of fluorescence intensity (n = 10 cells per group) reveals a significant accumulation of activated β1 integrin (pThr788/789) in neurite tips of differentiating Ndr2 PC12 cells compared to EGFP PC12 cells, both on PDL and collagen IV (two-way ANOVA genotype effect: F(1,36)= 7.407, p < 0.01). (H) Decreased growth cone size of control cells, but not of Ndr2 PC12 cells on collagen IV substrate (two-way ANOVA genotype × substrate interaction: F(1,35) = 15.67, p = 0.0004). Values are mean + SEM; *p < 0.05; **p < 0.01; ***p < 0.001.
Ndr2 Impairs α1β1 Integrin-Mediated Neurite Extension
α1β1 integrin heterodimers are the main receptors on the cell surface that respond to collagen IV substrate and α1β1 integrins show increased binding to collagen IV, rather than collagen I, during neurite extension of human neuroblastoma cells (Carmeliet et al., 1994). To identify the recognition process that underlies the growth deficiency on collagen IV, we tested the effect of integrin stimulation via Mg2+ and a KTS ligand specific to α1β1 integrins on PDL or Collagen IV substrates. Here we observed a general enhancement of outgrowth in the Ndr2 PC12 cells by Mg2+, but a complete failure to increase growth by the α1β1 integrin ligand. A significant interaction was found between Ndr2 expression and integrin stimulation (Figure 3, genotype × treatment interaction: F(6,24)= 41.05 p < 0.001).
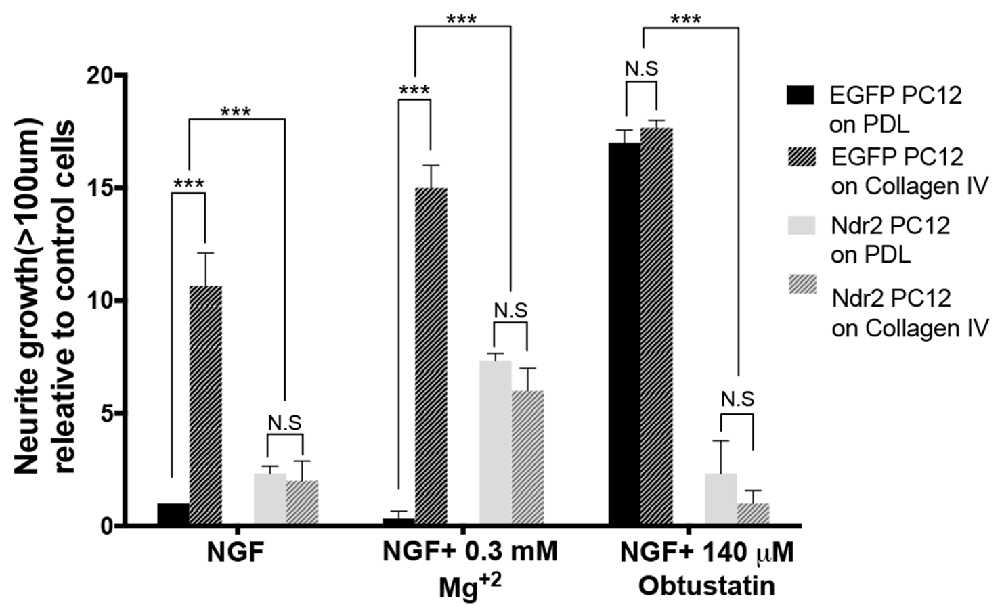
Figure 3. Lack of α1β1 integrin activation to stimulate outgrowth in Ndr2 overexpressing cells. Without additional integrin stimulation, control cells but not Ndr2 PC12 cells show an enhanced growth on collagen IV compared to PDL. Unspecific enhancement of integrin adhesion by 0.3 mM Mg2+ somewhat increases the neurite growth of Ndr2 PC12 cells on both PDL and Collagen IV, however, without reaching the high levels seen on collagen IV with control cells. The synthetic KTS ligand obtustatin induces growth in control cells on PDL to the same high level as on collagen IV. However, it does not stimulate growth of Ndr2 PC12 cells on either substrate. In summary, α1β1 stimulation by collagen IV substrate or a soluble KTS ligand stimulates neurite growth only in control cells, but not in Ndr2 PC12 (two-way ANOVA genotype × treatment interaction: F(6,24)= 41.05 p < 0.001). Values are mean + SEM; N = 3; ***p < 0.001; N.S p > 0.05.
As in the previous experiment a significant enhancement of neurite growth by collagen IV was observed in EGFP PC12 control cells, but not by Ndr2 PC12 cells upon mere NGF stimulation. Under 0.3 mM Mg2+ treatment along with NGF, EGFP PC12 cells slightly further increased growth on collagen IV, while Ndr2 cells increased growth significantly regardless of the substrate, but remained lower than control cells on collagen IV. To specifically address α1β1 integrin function we synthesized a KTS ligand peptide (CWKTSLTSHYC), derived from the larger disintegrin obtustatin (Marcinkiewicz et al., 2003; Moreno-Murciano et al., 2003) and applied it to the cell culture medium. At the concentration used in our experiments (140 μM; based on Marcinkiewicz et al., 2003), this KTS ligand did not induce any change in the flattening of the cells that would indicate a generally altered adhesion (Kruskal-Wallis test between treatments: p = 0.93), but a maximal neurite growth of PC12 control cells on both PDL and collagen IV was observed. As a side note, under 140 μM obtustatin treatment, no significant difference was observed between control cells plated on PDL and Collagen IV, suggesting that obtustatin peptide did not cause any inhibition on Collagen IV substrate. In Ndr2 PC12 cells, however, in spite of the increased expression of β1 integrin phosphorylation (Thr788/799) in the neurite tips (Figure 2G), the KTS agonist peptide like collagen IV entirely failed to induce neurite growth (Figure 3).
Ndr2 Modulates α1 Integrin Distribution During Neurite Growth
As those data indicated a specific impairment of α1β1 integrin function in Ndr2 PC12 cells, next we examined the expression and distribution of the α1 integrin subunit during differentiation (Figures 4A–F). In fact, we observed that Ndr2 PC12 cells show significantly less α1 integrin labeling at their neurite tips than EGFP PC12 cells (Figure 4G, two-tailed Student’s t-test, p < 0.001). At the same time, labeling for F-actin was not different in the neurite tips. To distinguish whether Ndr2 regulates the α1 integrin trafficking or its overall expression, we also determined the total α1 integrin expression in EGFP PC12 and Ndr2 PC12 cells (Figures 4H,I). Following either 3 days or 6 days of NGF treatment, a profound increase in α1 integrin levels was observed that was as pronounced in Ndr2 PC12 cells as in EGFP PC12 controls, similarly on PDL (two-way ANOVA, genotype effect: F(1,18)= 0.2754, p = 0.6062) and Collagen IV (two-way ANOVA, genotype effect: F(1,18)= 1.74, p = 0.2036) substrates (Figures 4J,K).
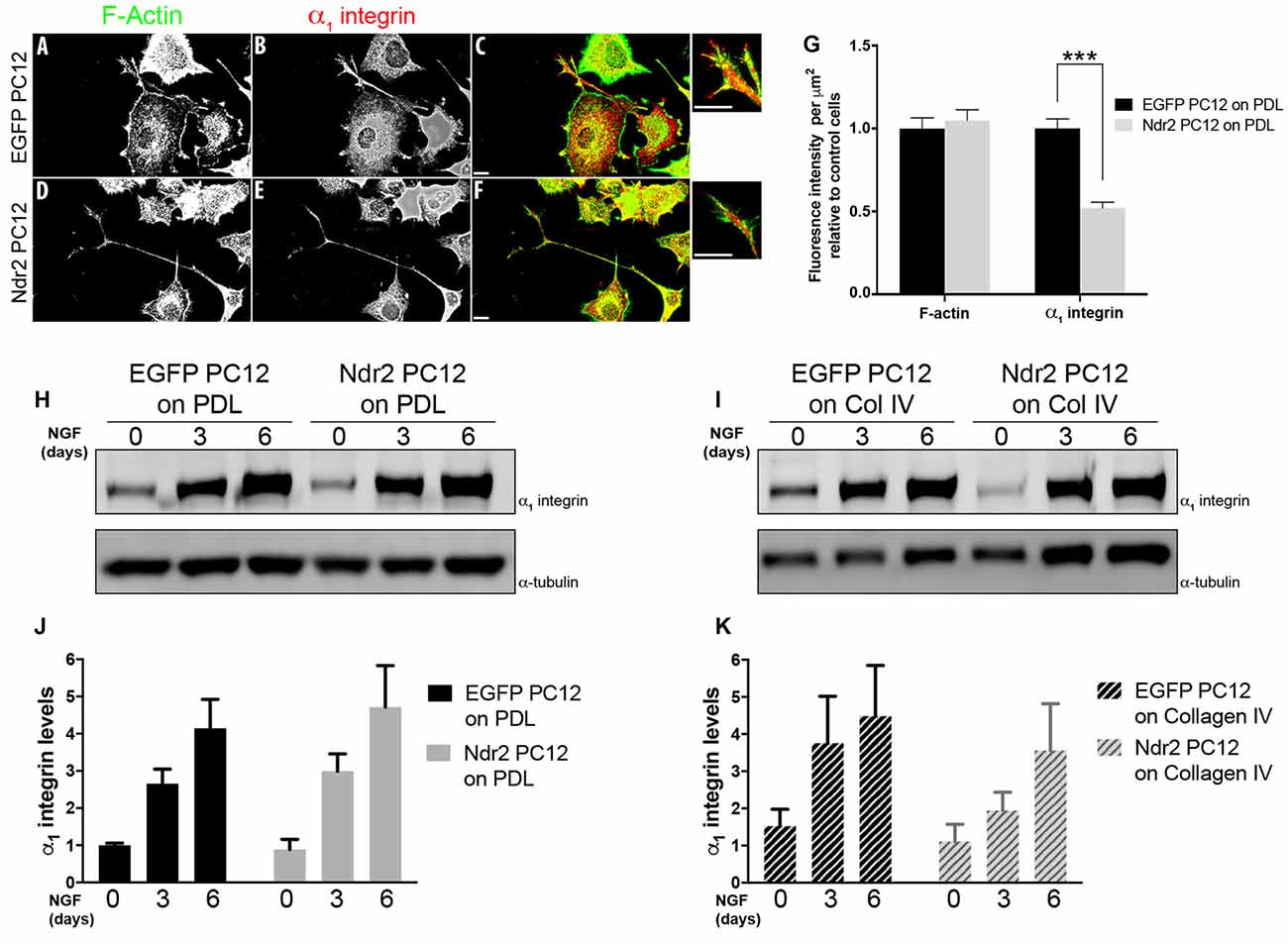
Figure 4. Reduced localization of α1 integrin to neurite tips. (A,B) Microscopic images of 6 day neurite growth factor (NGF) treated PC12 cells showing the expression and distribution F actin and of the α1 integrin receptor in control and (D,E) Ndr2 PC12 cells. (C,F) Overlay image. Scale bars: 10 μm. (G) Quantification of labeling at neurite tips (n = 46 for EGFP PC12, 49 for Ndr2 PC12 cells) reveals a significant reduction of α1 integrin levels in Ndr2 PC12 cells compared to EGFP PC12 cells (two-tailed Student’s t-test, p < 0.001), but no change in F-actin (two-tailed Student’s t-test, p = 0.595). (H,I) Immunoblotting shows the elevated expression of α1 integrin in both cell lines upon 3 days or 6 days of NGF treatment on both substrates. (J,K) Quantification of the blots shows that α1 integrin protein levels increases similarly during differentiation in EGFP PC12 and Ndr2 PC12 cells (n = 4 each; on PDL: two-way ANOVA, genotype effect: F(1,18)= 0.2754, p = 0.6062; on Collagen IV: two-way ANOVA, genotype effect: F(1,18)= 1.74, p = 0.2036). Values are mean + SEM; ***p < 0.001.
Ndr2 Controls Dendritic Branching in Primary Neurons
To investigate the role of Ndr2 in neuronal differentiation, we acutely transfected primary hippocampal neurons with Ndr2 constructs (overexpression and silencing) on different substrates. Neurons were plated on coverslips that are coated either with PDL or Laminin-111 (LN111), a previously established ECM molecule of primary neurons and a known α1β1 integrin substrate (Desban et al., 2006; Tulla et al., 2008). When control neurons (EGFP hippocampal primary culture (HPC)) were plated on LN111, their dendritic branching significantly increased compared to PDL (two-way ANOVA substrate effect: F(1,57)= 12.59, p = 0.0008; Figures 5A–C). However, Ndr2 overexpressing neurons (Ndr2 HPC) showed less dendritic branching on LN111 compared to PDL (two-way ANOVA substrate effect: F(1,57)= 15.46, p = 0.0002; Figures 5D–F), suggesting an impairment of α1 integrin dependent growth, in line with our PC12 cell results. Furthermore, analysis of the total dendritic length of these acutely-transfected neurons revealed that Ndr2 overexpression increases total dendritic length on PDL, while it reduces the total dendritic length on LN111 compared to the control neurons (one-way ANOVA: F(3,115) = 7.72, p < 0.0001; Figure 5G).
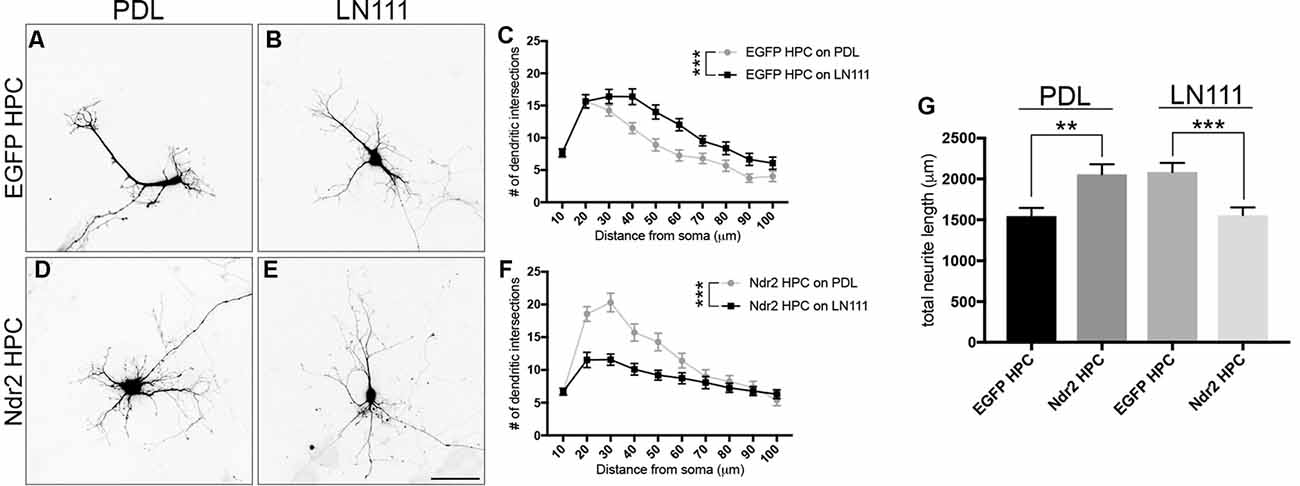
Figure 5. Overexpression of Ndr2 kinase impairs α1β1-dependent dendritic growth of hippocampal primary neurons. Primary hippocampal neurons transfected with EGFP plasmid and plated on (A) PDL and (B) Laminin-111 (LN111). (C) Sholl analysis of their dendrites reveals that LN111 substrate increases the dendrite branching significantly compared to PDL coating (two-way ANOVA between substrates: F(1,57)= 12.59, p = 0.0008, n = 30 each). (D–F) On the other hand, when Ndr2 overexpressing neurons are plated on the same substrates, Sholl analysis shows higher dendritic branching of Ndr2 hippocampal primary culture (HPC) on PDL rather than LN111 substrate (two-way ANOVA between substrates: F(1,57)= 15.46, p = 0.0002, n = 29 for PDL and 30 for LN111). (G) Total dendritic length of Ndr2 HPC is increased compared to EGFP PC12 cells on PDL and but reduced on LN111 substrate (one-way ANOVA: F(3,115) = 7.72, p < 0.0001). Values are mean + SEM; **p < 0.01; ***p < 0.001.
Finally, we tested how silencing of Ndr2 kinase in neurons affects the morphology of dendrites. shLuc transfected neurons (shLuc HPC) serving as controls increased their dendritic branching upon LN111 substrate as expected (two-way ANOVA substrate effect: F(1,54)= 12.16, p = 0.0010; Figures 6A–C). By contrast, Ndr2 knockdown neurons (shNdr2 HPC) entirely failed to increase their growth in response to LN111 substrates (two-way ANOVA substrate effect: F(1,58) = 1.262, p = 0.2660; Figures 6D–F). In fact, total dendritic lengths of shNdr2 neurons were significantly shorter than shLuc neurons on both PDL and LN111 substrates (one-way ANOVA: F(3,113)= 17.33, p < 0.0001; Figure 6G).
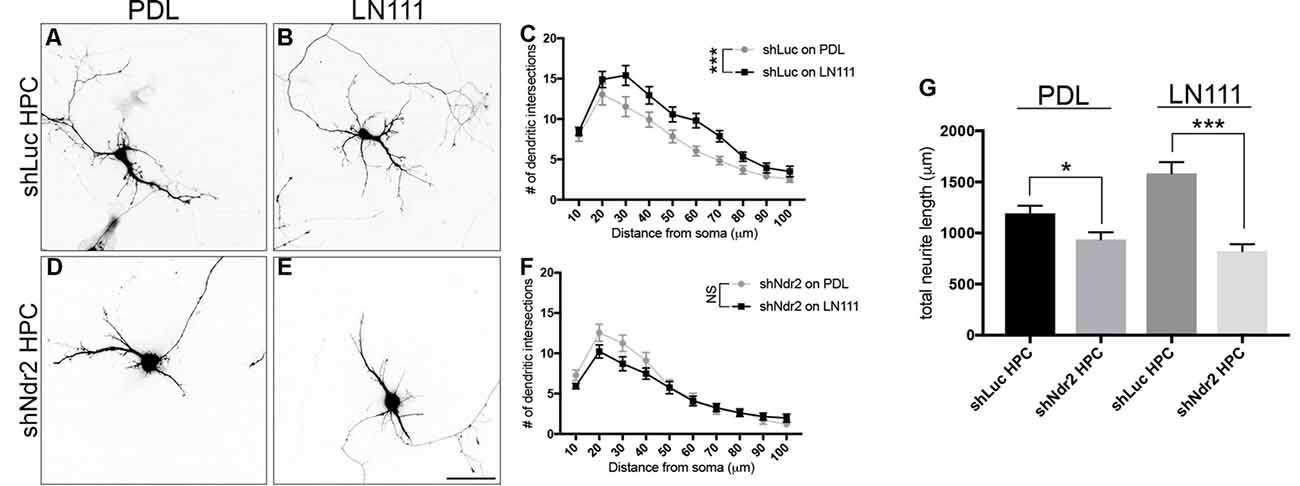
Figure 6. Ndr2 kinase is required for LN111 enhancement of dendritic growth in hippocampal neurons. Primary hippocampal neurons transfected with shLuc plasmid and plated on (A) PDL and (B) LN111. (C) Sholl analysis of their dendrites reveals that LN111 substrates increase the dendrite branching significantly compared to PDL coating (two-way ANOVA between substrates: F(1,54)= 12.16, p = 0.0010, n = 26 for PDL and 30 for LN111). (D–F) On the other hand, when Ndr2 kinase is silenced, dendritic morphology of the neurons are unchanged between substrates (two-way ANOVA between substrates: F(1,58) = 1.262, p = 0.2660, n = 30 for PDL and 31 for LN111). (G) In fact, silencing of Ndr2 kinase expression results in shorter total dendrite length compared to control neurons on both PDL and LN111 substrates (one-way ANOVA: F(3,113)= 17.33, p < 0.0001). Values are mean + SEM; *p < 0.05; ***p < 0.001.
Discussion
Neurite outgrowth is critically dependent on guidance signals such as the ECM being detected via integrins. Focal adhesion points are observed at the tip of filopodia evading the growth cone of primary neurons and on the neurite tips of differentiating PC12 cells (Eva et al., 2010). However, how cells control integrin levels and assemble specific α1β1 integrin heterodimers at these sites is still not completely understood. Our current data demonstrate that the serine/threonine kinase Ndr2 is involved in these processes in neurally differentiating PC12 cells. Ndr2 not only enhances the accumulation of β1 integrins in neurite tips of these cells but also reduces the incorporation of α1 subunits, resulting in an altered neurite outgrowth on different integrin substrates. α1 integrin subunits are only weakly expressed during the induction of neurite outgrowth, but increase at later stages during neurite extension and stabilization. Correspondingly, Ndr2 PC12 cells, although efficiently forming neurites on various substrates including collagen IV, showed a reduced collagen IV-induced growth of long neurites (>100 μm). This substrate-specific effect could also be observed in primary hippocampal neurons, where overexpression of Ndr2 prevented LN111 induced dendritic growth. Loss of Ndr2 by contrast generally inhibited dendritic growth in line with previous observations (Ultanir et al., 2012; Rehberg et al., 2014) thus also preventing the LN111 induced growth effect.
The ECM during neuron development supports the attachment of neurons and provides guidance cues for the growing neurites (Myers et al., 2011). Although it is a heterogenous mixture of different glycoproteins and proteoglycans, different ECM substrates can be classified into three major groups according to their integrin recognition sequences: laminins, fibronectins (RGD sequence containing) and collagens (GFOGER sequence containing; Barczyk et al., 2010). The basal lamina component collagen IV is furthermore characterized by its KTS sequence, which is recognized by α1β1 integrins (Kisiel et al., 2004). Laminin subtype 111 (also known as laminin-1) is another major component of basal lamina (Fiore et al., 2017) controls the migration and spreading of neural crest cells via α1β1 integrin receptors on the membrane (Desban et al., 2006). Earlier studies showed that each of the above-mentioned ligands or their combinations improve the survival/neurite growth of differentiating neurons (O’Connor et al., 2001; Fusaoka-Nishioka et al., 2011; Tonge et al., 2012).
A total of 28 different collagen subtypes have been described so far (Gordon and Hahn, 2010; Rozario and DeSimone, 2010) of which the collagen subtypes I, II, III and IV can induce neurite extension of PC12 cells in a Mg2+ dependent manner (Turner et al., 1987). Collagen I and IV were also shown to promote neuronal differentiation and neurite growth of rat cortical neurons (Ali et al., 1998; O’Connor et al., 2001). Since divalent ions prime integrin heterodimers to their activation states, the observed moderate enhancement of neurite growth in Ndr2 PC12 under the 0.3 mM Mg2+ conditions thus is in line with a mediation by integrin binding (Tiwari et al., 2011) and likely due to the increase of phosphorylated integrin (β1pThr788/789) in their neurite tips.
Thr788/789 site at the cytosolic domain of integrin β1 is required for its activation (Nilsson et al., 2006). Ndr2 has previously been shown to stimulate phosphorylation at Thr788/789 of β1 integrins via an intermediary kinase and to enhance their recycling to the cell membrane. In primary hippocampal neurons, Ndr2 thus mediates the “inside out” activation of dendritic integrins and stimulates dendritic growth and branching (Rehberg et al., 2014). Our current data further add to those findings, as we can show that silencing the Ndr2 kinase impairs the dendrite growth of hippocampal neurons regardless of the substrate (Figure 6G). Moreover, phosphorylation at the Thr788/789 activation site prevents internalized integrins from lysosomal degradation and favors recycling (Böttcher et al., 2012); accordingly, Ndr2 is associated with Rab5- and Rab11-positive early and late recycling endosomes in neurons and in PC12 cells (Rehberg et al., 2014). In the current study, we could demonstrate that this mechanism works in PC12 cells independently of the ECM substrate (PDL or collagen IV). In neuronal cells, β1subunit containing heterodimers are the major receptors for responding to those ECM substrates (Luo et al., 2007; Stukel and Willits, 2016). Previous studies also showed that outside-in stimulation of β1 integrin receptor increases the dendritic branching and its inhibition has the adverse effect on neuron morphology (Moresco et al., 2005; Marrs et al., 2006; Schlomann et al., 2009). This effect of β1 integrins on dendritic arborization is particularly evident in early development and differentiation (Warren et al., 2012).
We therefore considered that the disturbance of collagen IV- induced outgrowth in Ndr2 PC12 may have been related to an increased adhesion to the substrate and associated changes in “outside-in” signaling. We addressed this question and the specificity of the observed effects by applying a diffusible ligand mimicking collagen IV-mediated binding to integrins. While collagen IV substrate can be recognized by both α1β1and α2β1 integrins, previous studies showed that α1β1 integrin heterodimer has higher affinity and is the primary receptor that interacts with collagen IV (Kern et al., 1993; Knight et al., 2000; Becker et al., 2013). Furthermore, studies in T cells, which rely mainly on integrin receptors for adhesion, showed that α1β1 integrin expressing cells are enriched on collagen IV surfaces while α2β1expressing cells tends to localize on collagen I rich spaces (Richter et al., 2007). Strikingly, while the α1β1 integrin ligand obtustatin mimicked the collagen IV-induced neurite growth in control cells, Ndr2 overexpressing PC12 cells entirely failed to respond to the ligand.
This strongly suggests that the reduced collagen IV response of Ndr2 PC12 cells may be caused by their reduced expression of α1 integrin in neurite tips (Figures 4A–G). It has been reported that α1 integrin expression levels increase in PC12 cells upon NGF treatment, correlating with the adhesion on collagen and neurite extension levels of the cell (Zhang et al., 1993). As we detected similar induction of α1 integrin expression in Ndr2 PC12 cells and EGFP PC12 cells (Figures 4H,I), our findings indicate a disturbance of α1 integrin trafficking rather than expression regulation in Ndr2 PC12 cells.
It is plausible to assume that the Ndr2 kinase may regulate the α integrin trafficking via Rab-dependent transport mechanisms. We have previously demonstrated the association of Ndr2 with Rab5 and Rab11 positive integrin recycling vesicles (Rehberg et al., 2014) and the Rab-8 guanine exchange factor, Rabin8 has been identified as a substrate for Ndr kinases (Ultanir et al., 2012). Rabin8 stimulates endosomal transport in PC12 cells in Rab11-dependent manner and is required for neurite outgrowth in these cells (Homma and Fukuda, 2016). Previous research further demonstrated that α integrin subunits are internalized and recycled via Rab-mediated pathways and that Rab-association domains are conserved among α integrins (Pellinen et al., 2008; Caswell et al., 2009). A differential α subunit trafficking may also involve clathrin-adaptor proteins acting as intermediate regulators of vesicle trafficking (Bridgewater et al., 2012). For example, the endocytic adaptor protein Dab2 was shown to bind to and increase endocytosis of α1, α2 and α3 integrin subunits (Teckchandani et al., 2009). On the other hand α2 and α6, but not α1 integrin contain binding motifs for the adapter-protein 2 (AP-2, De Franceschi et al., 2016), which is redistributed to the membrane in PC12 cells after NGF treatment (Beattie et al., 2000). Intriguingly, Ndr2 kinase can phosphorylate and regulate the activity of AP-2 associated kinase (AAK1), which controls the AP-2 binding affinity and dendritic growth of rat hippocampal neurons (Ultanir et al., 2012).
Our data suggest, that in addition to enhancing β1 Thr788/789 phosphorylation and recovery, Ndr2 controls the selective trafficking of α1 subunit-containing integrins. While β subunits of integrins interact with intracellular proteins and are required for activation of downstream signaling cascades, α subunits critically determine the extracellular ligand specificity of the receptor (Barczyk et al., 2010; Stukel and Willits, 2016). Accordingly, β1 integrin expression is rather steady and uniform compared to α subunits which are both temporally and spatially regulated in developing cortex (Heino et al., 1989; Schmid and Anton, 2003). As integrin subunits need to form stable αβ heterodimers to exit the endoplasmic reticulum and to join the membrane trafficking/recycling pathways (Bouvard et al., 2013), α subunits appear to serve as limiting and specifying factors that determine the rate of dimerization with the β subunits as well as the integrin heterodimer sorting (Heino et al., 1989). Ndr2, by differentially regulating β1 integrin recycling and the surface localization of α1 integrin subunit localization, thus determines the substrate specificity of neurite outgrowth in PC12 cells and hippocampal neurons.
Author Contributions
KR, SK and OS designed the research. KR and YED performed the experiments. YED and OS wrote the article.
Funding
The work was supported by grants from the German Research foundation (Deutsche Forschungsgemeinschaft; GRK1167/P13 and STO488/4 to OS, CRC854/B10 to OS and SK).
Conflict of Interest Statement
The authors declare that the research was conducted in the absence of any commercial or financial relationships that could be construed as a potential conflict of interest.
Acknowledgments
We are grateful to A. Koffi von Hoff and S. Stork for expert technical assistance and D. Lang for comments on manuscript.
Abbreviations
AP-2, Adapter-protein 2; EGFP PC12, EGFP expressing control PC12 cells; Ndr2 PC12, EGFP-Ndr2 expressing PC12 cells; ECM, Extracellular matrix; HPC, Hippocampal primary culture; LN111, Laminin-111; NGF, Neurite growth factor; Ndr, Nuclear Dbf2-related; PDL, poly-D-lysine; PC12, Rat pheochromocytoma cells.
References
Ali, S. A., Pappas, I. S., and Parnavelas, J. G. (1998). Collagen type IV promotes the differentiation of neuronal progenitors and inhibits astroglial differentiation in cortical cell cultures. Dev. Brain Res. 110, 31–38. doi: 10.1016/s0165-3806(98)00091-1
Barczyk, M., Carracedo, S., and Gullberg, D. (2010). Integrins. Cell Tissue Res. 339, 269–280. doi: 10.1007/s00441-009-0834-6
Beattie, E. C., Howe, C. L., Wilde, A., Brodsky, F. M., and Mobley, W. C. (2000). NGF signals through TrkA to increase clathrin at the plasma membrane and enhance clathrin-mediated membrane trafficking. J. Neurosci. 20, 7325–7333.
Becker, H. M., Rullo, J., Chen, M., Ghazarian, M., Bak, S., Xiao, H., et al. (2013). α1β1 integrin-mediated adhesion inhibits macrophage exit from a peripheral inflammatory lesion. J. Immunol. 190, 4305–4314. doi: 10.4049/jimmunol.1202097
Böttcher, R. T., Stremmel, C., Meves, A., Meyer, H., Widmaier, M., Tseng, H., et al. (2012). Sorting nexin 17 prevents lysosomal degradation of β1 integrins by binding to the β1-integrin tail. Nat. Cell Biol. 14, 584–592. doi: 10.1038/ncb2501
Bouvard, D., Pouwels, J., De Franceschi, N., and Ivaska, J. (2013). Integrin inactivators: balancing cellular functions in vitro and in vivo. Nat. Rev. Mol. Cell Biol. 14, 430–442. doi: 10.1038/nrm3599
Bridgewater, R. E., Norman, J. C., and Caswell, P. T. (2012). Integrin trafficking at a glance. J. Cell Sci. 125, 3695–3701. doi: 10.1242/jcs.095810
Carmeliet, G., Himpens, B., and Cassiman, J. J. (1994). Selective increase in the binding of the α 1 β 1 integrin for collagen type IV during neurite outgrowth of human neuroblastoma TR 14 cells. J. Cell Sci. 107, 3379–3392.
Caswell, P. T., Vadrevu, S., and Norman, J. C. (2009). Integrins: masters and slaves of endocytic transport. Nat. Rev. Mol. Cell Biol. 10, 843–853. doi: 10.1038/nrm2799
De Franceschi, N., Arjonen, A., Elkhatib, N., Denessiouk, K., Wrobel, A. G., Wilson, T. A., et al. (2016). Selective integrin endocytosis is driven by interactions between the integrin α-chain and AP2. Nat. Struct. Mol. Biol. 23, 172–179. doi: 10.1038/nsmb.3161
Denda, S., and Reichardt, L. F. (2007). Studies on integrins in the nervous system. Meth. Enzymol. 426, 203–221. doi: 10.1016/s0076-6879(07)26010-0
Desban, N., Lissitzky, J., Rousselle, P., and Duband, J. (2006). α1β1-integrin engagement to distinct laminin-1 domains orchestrates spreading, migration and survival of neural crest cells through independent signaling pathways. J. Cell Sci. 119, 3206–3218. doi: 10.1242/jcs.03057
Emoto, K. (2011). The growing role of the Hippo—NDR kinase signalling in neuronal development and disease. J. Biochem. 150, 133–141. doi: 10.1093/jb/mvr080
Emoto, K., Parrish, J. Z., Jan, L. Y., and Jan, Y.-N. (2006). The tumour suppressor Hippo acts with the NDR kinases in dendritic tiling and maintenance. Nature 443, 210–213. doi: 10.1038/nature05090
Eva, R., Dassie, E., Caswell, P. T., Dick, G., Ffrench-Constant, C., Norman, J. C., et al. (2010). Rab11 and its effector Rab coupling protein contribute to the trafficking of β1 integrins during axon growth in adult dorsal root ganglion neurons and PC12 cells. J. Neurosci. 30, 11654–11669. doi: 10.1523/JNEUROSCI.2425-10.2010
Fiore, A. P. Z. P., Spencer, V. A., Mori, H., Carvalho, H. F., Bissell, M. J., and Bruni-Cardoso, A. (2017). Laminin-111 and the level of nuclear actin regulate epithelial quiescence via exportin-6. Cell Rep. 19, 2102–2115. doi: 10.1016/j.celrep.2017.05.050
Frenz, L. M., Lee, S. E., Fesquet, D., and Johnston, L. H. (2000). The budding yeast Dbf2 protein kinase localises to the centrosome and moves to the bud neck in late mitosis. J. Cell Sci. 113, 3399–3408.
Fusaoka-Nishioka, E., Shimono, C., Taniguchi, Y., Togawa, A., Yamada, A., Inoue, E., et al. (2011). Differential effects of laminin isoforms on axon and dendrite development in hippocampal neurons. Neurosci. Res. 71, 421–426. doi: 10.1016/j.neures.2011.08.012
Gordon, M. K., and Hahn, R. A. (2010). Collagens. Cell Tissue Res. 339, 247–257. doi: 10.1007/s00441-009-0844-4
Harrill, J. A., and Mundy, W. R. (2011). Quantitative assessment of neurite outgrowth in PC12 cells. Methods Mol. Biol. 758, 331–348. doi: 10.1007/978-1-61779-170-3_23
Heino, J., Ignotz, R. A., Hemler, M. E., Crouse, C., and Massagué, J. (1989). Regulation of cell adhesion receptors by transforming growth factor-β. Concomitant regulation of integrins that share a common β1 subunit. J. Biol. Chem. 264, 380–388.
Homma, Y., and Fukuda, M. (2016). Rabin8 regulates neurite outgrowth in both a GEF-activity-dependent and -independent manner. Mol. Biol. Cell 27, 2107–2118. doi: 10.1091/mbc.e16-02-0091
Humphries, M. J., McEwan, P. A., Barton, S. J., Buckley, P. A., Bella, J., and Mould, A. P. (2003). Integrin structure: heady advances in ligand binding, but activation still makes the knees wobble. Trends Biochem. Sci. 28, 313–320. doi: 10.1016/s0968-0004(03)00112-9
Hynes, R. O. (2002). Integrins: bidirectional, allosteric signaling machines. Cell 110, 673–687. doi: 10.1016/S0092-8674(02)00971-6
Kaech, S., and Banker, G. (2006). Culturing hippocampal neurons. Nat. Protoc. 1, 2406–2415. doi: 10.1038/nprot.2006.356
Kern, A., Eble, J., Golbik, R., and Kühn, K. (1993). Interaction of type IV collagen with the isolated integrins α1β1 and α2β1. Eur. J. Biochem. 215, 151–1519.
Kisiel, D. G., Calvete, J. J., Katzhendler, J., Fertala, A., Lazarovici, P., and Marcinkiewicz, C. (2004). Structural determinants of the selectivity of KTS-disintegrins for the α1β1 integrin. FEBS Lett. 577, 478–482. doi: 10.1016/j.febslet.2004.10.050
Knight, C. G., Morton, L. F., Peachey, A. R., Tuckwell, D. S., Farndale, R. W., Barnes, M. J., et al. (2000). The collagen-binding A-domains of integrins α1β1 and α2β1 recognize the same specific amino acid sequence, GFOGER, in native (Triple-helical) collagens. J. Biol. Chem. 275, 35–40. doi: 10.1074/jbc.275.1.35
Lein, P., Gallagher, P. J., Amodeo, J., Howie, H., and Roth, J. A. (2000). Manganese induces neurite outgrowth in PC12 cells via upregulation of α(v) integrins. Brain Res. 885, 220–230. doi: 10.1016/s0006-8993(00)02943-7
Lei, W. L., Xing, S. G., Deng, C. Y., Ju, X. C., Jiang, X. Y., and Luo, Z. G. (2012). Laminin/β1 integrin signal triggers axon formation by promoting microtubule assembly and stabilization. Cell Res. 22, 954–972. doi: 10.1038/cr.2012.40
Luo, B. H., Carman, C. V., and Springer, T. A. (2007). Structural basis of integrin regulation and signaling. Annu. Rev. Immunol. 25, 619–647. doi: 10.1146/annurev.immunol.25.022106.141618
Marcinkiewicz, C., Weinreb, P. H., Calvete, J. J., Kisiel, D. G., Mousa, S. A., Tuszynski, G. P., et al. (2003). Obtustatin: a potent selective inhibitor of α1β1 integrin in vitro and angiogenesis in vivo. Cancer Res. 63, 2020–2023.
Marrs, G. S., Honda, T., Fuller, L., Thangavel, R., Balsamo, J., Lilien, J., et al. (2006). Dendritic arbors of developing retinal ganglion cells are stabilized by β1-integrins. Mol. Cell. Neurosci. 32, 230–241. doi: 10.1016/j.mcn.2006.04.005
Moreno-Murciano, M. P., Monleón, D., Calvete, J. J., Celda, B., and Marcinkiewicz, C. (2003). Amino acid sequence and homology modeling of obtustatin, a novel non-RGD-containing short disintegrin isolated from the venom of Vipera lebetina obtusa. Protein Sci. 12, 366–371. doi: 10.1110/ps.0230203
Moresco, E. M. Y., Donaldson, S., Williamson, A., and Koleske, A. J. (2005). Integrin-mediated dendrite branch maintenance requires Abelson (Abl) family kinases. J. Neurosci. 25, 6105–6118. doi: 10.1523/JNEUROSCI.1432-05.2005
Myers, J. P., Santiago-Medina, M., and Gomez, T. M. (2011). Regulation of axonal outgrowth and pathfinding by integrin-ECM interactions. Dev. Neurobiol. 71, 901–923. doi: 10.1002/dneu.20931
Nilsson, S., Kaniowska, D., Brakebusch, C., Fässler, R., and Johansson, S. (2006). Threonine 788 in integrin subunit β1 regulates integrin activation. Exp. Cell Res. 312, 844–853. doi: 10.1016/j.yexcr.2005.12.001
O’Connor, S. M., Stenger, D. A., Shaffer, K. M., and Ma, W. (2001). Survival and neurite outgrowth of rat cortical neurons in three-dimensional agarose and collagen gel matrices. Neurosci. Lett. 304, 189–193. doi: 10.1016/s0304-3940(01)01769-4
Pellinen, T., Tuomi, S., Arjonen, A., Wolf, M., Edgren, H., Meyer, H., et al. (2008). Integrin trafficking regulated by Rab21 is necessary for cytokinesis. Dev. Cell 15, 371–385. doi: 10.1016/j.devcel.2008.08.001
Plow, E. F., Haas, T. A., Zhang, L., Loftus, J., and Smith, J. W. (2000). Ligand binding to integrins. J. Biol. Chem. 275, 21785–21788. doi: 10.1074/jbc.R000003200
Rehberg, K., Kliche, S., Madencioglu, D. A., Thiere, M., Müller, B., Meineke, B. M., et al. (2014). The serine/threonine kinase Ndr2 controls integrin trafficking and integrin-dependent neurite growth. J. Neurosci. 34, 5342–5354. doi: 10.1523/jneurosci.2728-13.2014
Richter, M., Ray, S. J., Chapman, T. J., Austin, S. J., Rebhahn, J., Mosmann, T. R., et al. (2007). Collagen distribution and expression of collagen-binding α1β1 (VLA-1) and α2β1 (VLA-2) integrins on CD4 and CD8 T cells during influenza infection. J. Immunol. 178, 4506–4516. doi: 10.4049/jimmunol.178.7.4506
Rozario, T., and DeSimone, D. W. (2010). The extracellular matrix in development and morphogenesis: a dynamic view. Dev. Biol. 341, 126–140. doi: 10.1016/j.ydbio.2009.10.026
Schlomann, U., Schwamborn, J. C., Müller, M., Fässler, R., and Püschel, A. W. (2009). The stimulation of dendrite growth by Sema3A requires integrin engagement and focal adhesion kinase. J. Cell Sci. 122, 2034–2042. doi: 10.1242/jcs.038232
Schmid, R. S., and Anton, E. S. (2003). Role of integrins in the development of the cerebral cortex. Cereb. Cortex 13, 219–224. doi: 10.1093/cercor/13.3.219
Shattil, S. J., Kim, C., and Ginsberg, M. H. (2010). The final steps of integrin activation: the end game. Nat. Rev. Mol. Cell Biol. 11, 288–300. doi: 10.1038/nrm2871
Stork, O., Zhdanov, A., Kudersky, A., Yoshikawa, T., Obata, K., and Pape, H. C. (2004). Neuronal functions of the novel serine/threonine kinase Ndr2. J. Biol. Chem. 279, 45773–45781. doi: 10.1074/jbc.m403552200
Stukel, J. M., and Willits, R. (2016). Mechanotransduction of neural cells through cell-substrate interactions. Tissue Eng. Part B. Rev. 22, 173–182. doi: 10.1089/ten.teb.2015.0380
Teckchandani, A., Toida, N., Goodchild, J., Henderson, C., Watts, J., Wollscheid, B., et al. (2009). Quantitative proteomics identifies a Dab2/integrin module regulating cell migration. J. Cell Biol. 186, 99–111. doi: 10.1083/jcb.200812160
Tiwari, S., Askari, J. A., Humphries, M. J., and Bulleid, N. J. (2011). Divalent cations regulate the folding and activation status of integrins during their intracellular trafficking. J. Cell Sci. 124, 1672–1680. doi: 10.1242/jcs.084483
Tomaselli, K. J., Damsky, C. H., and Reichardt, L. F. (1988). Purification and characterization of mammalian integrins expressed by a rat neuronal cell line (PC12): evidence that they function as α/β-heterodimeric receptors for laminin and type-IV collagen. J. Cell Biol. 107, 1241–1252. doi: 10.1083/jcb.107.3.1241
Tonge, D. A., De Burgh, H. T., Docherty, R., Humphries, M. J., Craig, S. E., and Pizzey, J. (2012). Fibronectin supports neurite outgrowth and axonal regeneration of adult brain neurons in vitro. Brain Res. 1453, 8–16. doi: 10.1016/j.brainres.2012.03.024
Tulla, M., Lahti, M., Puranen, J. S., Brandt, A. M., Käpylä, J., Domogatskaya, A., et al. (2008). Effects of conformational activation of integrin α1I and α2I domains on selective recognition of laminin and collagen subtypes. Exp. Cell Res. 314, 1734–1743. doi: 10.1016/j.yexcr.2008.01.025
Turner, D. C., Flier, L. A., and Carbonetto, S. (1987). Magnesium-dependent attachment and neurite outgrowth by PC12 cells on collagen and laminin substrata. Dev. Biol. 121, 510–525. doi: 10.1016/0012-1606(87)90187-4
Ultanir, S. K., Hertz, N. T., Li, G., Ge, W. P., Burlingame, A. L., Pleasure, S. J., et al. (2012). Chemical genetic identification of NDR1/2 kinase substrates AAK1 and Rabin8 uncovers their roles in dendrite arborization and spine development. Neuron 73, 1127–1142. doi: 10.1016/j.neuron.2012.01.019
Warren, M. S., Bradley, W. D., Gourley, S. L., Lin, Y. C., Simpson, M. A., Reichardt, L. F., et al. (2012). Integrin β1 signals through arg to regulate postnatal dendritic arborization, synapse density and behavior. J. Neurosci. 32, 2824–2834. doi: 10.1523/JNEUROSCI.3942-11.2012
Yang, R., Kong, E., Jing, J., Hergovich, A., and Püschel, A. (2014). Rassf5 and Ndr kinases regulate neuronal polarity through Par3 phosphorylation in a novel pathway. J. Cell Sci. 127, 3463–3476. doi: 10.1242/jcs.146696
Zallen, J. A., Peckol, E. L., Tobin, D. M., and Cornelia, I. B. (2000). Neuronal cell shape and neurite initiation are regulated by the Ndr kinase SAX-1, a member of the Orb6/COT-1/warts serine /threonine kinase family. Mol. Biol. Cell 11, 3177–3190. doi: 10.1091/mbc.11.9.3177
Keywords: Ndr kinase, integrin inside-out signaling, α1 integrin, neurite outgrowth, dendritic branching, pheochromocytoma, primary hippocampal neurons
Citation: Demiray YE, Rehberg K, Kliche S and Stork O (2018) Ndr2 Kinase Controls Neurite Outgrowth and Dendritic Branching Through α1 Integrin Expression. Front. Mol. Neurosci. 11:66. doi: 10.3389/fnmol.2018.00066
Received: 17 May 2017; Accepted: 16 February 2018;
Published: 06 March 2018.
Edited by:
Jean-Marc Taymans, Institut National de la Santé et de la Recherche Médicale (INSERM), FranceReviewed by:
Hansjürgen Volkmer, Universität Tübingen, GermanyChristian Gonzalez-Billault, Universidad de Chile, Chile
Copyright © 2018 Demiray, Rehberg, Kliche and Stork. This is an open-access article distributed under the terms of the Creative Commons Attribution License (CC BY). The use, distribution or reproduction in other forums is permitted, provided the original author(s) and the copyright owner are credited and that the original publication in this journal is cited, in accordance with accepted academic practice. No use, distribution or reproduction is permitted which does not comply with these terms.
*Correspondence: Oliver Stork, b2xpdmVyLnN0b3JrQG92Z3UuZGU=