- 1Departamento de Neurología, Escuela de Medicina, Facultad de Medicina, Pontificia Universidad Católica de Chile, Santiago, Chile
- 2Centro de Investigación y Estudio del Consumo de Alcohol en Adolescentes, Santiago, Chile
The formation of gap junctions was initially thought to be the central role of connexins, however, recent evidence had brought to light the high relevance of unopposed hemichannels as an independent mechanism for the selective release of biomolecules during physiological and pathological conditions. In the healthy brain, the physiological opening of astrocyte hemichannels modulates basal excitatory synaptic transmission. At the other end, the release of potentially neurotoxic compounds through astroglial hemichannels and pannexons has been insinuated as one of the functional alterations that negatively affect the progression of multiple brain diseases. Recent insights in this matter have suggested encannabinoids (eCBs) as molecules that could regulate the opening of these channels during diverse conditions. In this review, we discuss and hypothesize the possible interplay between the eCB system and the hemichannel/pannexon-mediated signaling in the inflamed brain and during event of synaptic plasticity. Most findings indicate that eCBs seem to counteract the activation of major neuroinflammatory pathways that lead to glia-mediated production of TNF-α and IL-1β, both well-known triggers of astroglial hemichannel opening. In contrast to the latter, in the normal brain, eCBs apparently elicit the Ca2+-activation of astrocyte hemichannels, which could have significant consequences on eCB-dependent synaptic plasticity.
Introduction
Because neurons are the excitable cells responsible for the transmission of electrochemical impulses during major cognitive processes, they were considered for a long time the main functional units at the central nervous system (CNS; Navarrete and Araque, 2010). Nonetheless, accumulating evidence in the last two decades has showed that other cell types may also play an active and highly coordinated role in multiple brain functions (Iadecola, 2017). For example, microvascular endothelial cells are pivotal players in the neurovascular coupling, as they actively modulate both the local blood flow and transport across the blood-brain barrier (BBB), ensuring oxygen, glucose and metabolite supply on demand (Zhao et al., 2015). At the other end, along with being the major population of glial cells in the CNS, astrocytes also are the gatekeepers of the BBB and govern synaptic transmission, ionic and transmitter homeostasis, as well as antioxidant and neurovascular responses, among other functions (Allen and Eroglu, 2017). Although initially not considered an element of the chemical synapse, microglia are now recognized to have a broad spectrum of functions beyond their immune capabilities, ranging from neuronal pruning to synaptic plasticity (Li and Barres, 2017). Because they are the primary source of inflammatory mediators during infections, injuries and chronic neurodegenerative diseases, microglia finely control the inflammatory profile of astrocytes and decidedly impact the functions and fate of neurons (Kettenmann et al., 2011). Most of the abovementioned functions and features tightly depend on the complex interaction and intercellular synchronization among brain cells. In mammals, cell-to-cell communication is in part mediated by two unrelated families of plasma membrane proteins: connexins and pannexins (Wang et al., 2013a; Decrock et al., 2015).
Connexins encompass a broad protein family of 21 members in humans (20, 37 and 17 in mice, zebrafish and frog, respectively), whose major structural features include the presence of four highly conserved transmembrane domains, two extracellular loops, intracellular N- and C-termini and a cytoplasmic loop linking the second and third transmembrane segments (Esseltine and Laird, 2016; Figure 1). These proteins form two types of functional plasma membrane channels: hemichannels and gap junction channels (GJCs). Each hemichannel is the assembly of six connexins around a central aqueous pore that allow the bidirectional flux of ions and molecules between the intracellular and intracellular milieu (Sáez et al., 2010; Figure 1). Additionally, hemichannels may diffuse freely to areas of cell-to-cell contact to align and dock with compatible hemichannels from a neighboring cell to complete the formation of GJCs. The arrangement and clustering of hundreds of GJCs at membrane appositions constitute the gap junction plaque, which provide a pathway of direct cytoplasmic communication between adjacent cells (Sáez et al., 2003). These channels are the building blocks of the electrically conductive link between two neighboring neurons (electrical synapse), as well as the functional syncytium of coupled astrocytes that underlie the homeostatic regulation of ions, transmitters and metabolites at the CNS (Orellana et al., 2009; Decrock et al., 2015). For example, the essential architecture of electrically coupled retinal circuits rely on the communication through GJCs, as they are broadly found in all cell types of the retina (Bloomfield and Volgyi, 2009). While the formation of gap junctions was initially thought to be the central role of connexins, the last decade had brought to light the high relevance of unopposed hemichannels as independent mechanisms for the selective release of relevant biomolecules (e.g., ATP, glutamate, PGE2) during physiological and pathological conditions (Giaume et al., 2013; Montero and Orellana, 2015; Gajardo-Gómez et al., 2017).
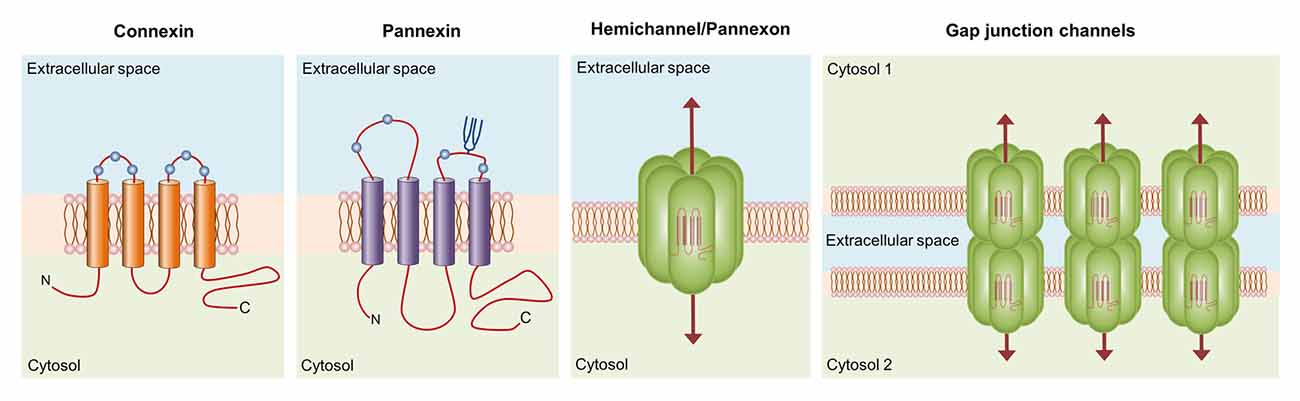
Figure 1. Basic structure of connexin and pannexin-based channels. Connexins and pannexins share a similar membrane topology with four α-helical transmembrane domains connected by two extracellular loops and one cytoplasmic loop; both the amino- and carboxy-termini are intracellular. The relative positions of the extracellular loop cysteines (green balls) and glycosylated asparagines (blue branches) are also shown. Hemichannels (also known as connexons) are formed by the oligomerization of six subunit connexins around a central pore. Pannexons are single membrane channels that are composed of six pannexin subunits. Recently, a band pattern more consistent with an octamer than a hexamer was observed in Panx2 by cross-linking studies and native gels of purified homomeric full-length and C-terminal truncation mutants (Ambrosi et al., 2010). Under resting conditions, hemichannels and pannexons remain preferentially closed, but they may be activated by diverse physiological and pathological conditions and offer a diffuse transmembrane route between the intra- and extracellular milieu. Hemichannels dock each other to form functional cell-to-cell channels termed gap junction channels (GJCs). GJCs aggregate in well-known anatomical structures called gap junctions to facilitate the intercellular cytoplasmic exchange of metabolites, second messengers and ions.
Pannexins, on the other hand, belong to a three-member family of chordate proteins homologous to the invertebrate gap junction proteins: the innexins (Bruzzone et al., 2003). Pannexins, unlike innexins and connexins, seem to have lost the capacity to directly couple contacting cells and thereby, the molecular and ionic interchange between the cytoplasmic and extracellular space is today widely recognized as their fundamental function. Although these proteins share some structural and topological features with connexins, their amino acid sequence and major post-translational modifications totally differ (Dahl and Keane, 2012). Single pannexin channels, also known as pannexons, allow the release of different paracrine molecules, including ATP, UTP, D-serine and glutamate (Bond and Naus, 2014). Pannexons formed by Pannexin 1 (Panx1), the most ubiquitous member of its family, are activated through direct protein-to-protein interactions with P2X7 receptors (P2X7R; Locovei et al., 2007), whereas the P2Y receptor (P2YR)-mediated rise in intracellular free Ca2+ concentration ([Ca2+]i) causes similar effects in these channels (Locovei et al., 2006). Consequently, the crosstalk between pannexons and purinergic receptors has been proposed to be crucial for the paracrine ATP release and amplification of cell-to-cell Ca2+ signaling (Wang et al., 2013a; Dahl, 2015). The latter is particularly true for astrocytes. They can communicate each other and with neurons by the spread of Ca2+ waves through two major interdependent mechanisms. One of them requires the physical interaction among contacting astrocytes and involves the diffusion through GJCs of cytoplasmic Ca2+-mobilizing second messengers such as IP3, cADP-ribose (cADPR) or Ca2+ itself (Scemes and Giaume, 2006; Orellana et al., 2012b). Alternatively, ATP produced by astrocytes may diffuse in a paracrine way to activate P2XRs/P2YRs, increasing the [Ca2+]i and perpetuating the propagation of Ca2+ waves as new ATP is released into the extracellular space (Guthrie et al., 1999; Anderson et al., 2004). This mechanism of ATP-induced ATP release constitutes a critical pathway through which astrocytes exert their modulatory actions over neuronal activity (Chen et al., 2013; Shen et al., 2017).
Throughout the CNS there is a complex pattern of expression for connexins and pannexins, being highly dependent on developmental stage, brain region, cell type and brain homeostatic conditions (Söhl et al., 2000; Koulakoff et al., 2012; Gaete et al., 2014; Swayne and Bennett, 2016). Up to now, most studies indicate that hemichannels and pannexons play pivotal roles at the normal CNS, including the establishment of adhesive interactions, tolerance to ischemia, fear memory consolidation, glucose and redox sensing, chemoreception, BBB permeability, spontaneous electrical activity, synaptic transmission and neuronal migration (Orellana et al., 2016). Nevertheless, during acute and chronic brain damage, these channels behave abnormally, showing an increased activity and altering their permeability properties to different crucial biomolecules. The latter phenomenon has been hypothesized as a common hallmark reflecting the homeostatic unbalance observed in diverse neuropathological conditions such as Alzheimer’s disease (AD), epilepsy, ischemia and HIV-associated neurological disorders (Domercq et al., 2010; Orellana et al., 2011a; Decrock et al., 2015; Berman et al., 2016; Robel and Sontheimer, 2016; Giaume et al., 2017). While the mechanisms linked to hemichannel/pannexon dysfunction remain still obscure, some clues coming from recent studies argue in favor of the involvement of inflammatory and redox signaling in this process (Takeuchi et al., 2006; Retamal et al., 2007; Karpuk et al., 2011; Adamson and Leitinger, 2014; Retamal, 2014; Avendaño et al., 2015). The release of potentially neurotoxic compounds through hemichannels and pannexons has been insinuated as one of the functional alterations that negatively impact the progression of multiple brain diseases, turning the study and comprehension of this field in something of high relevance (Shestopalov and Slepak, 2014; Kim et al., 2016; Giaume et al., 2017; Malik and Eugenin, 2017; Rovegno and Sáez, 2018). Accordingly, many research groups are currently focused in developing new therapeutic and pharmacological tools to tackle the exacerbated activity of hemichannels and pannexons (Moore and O’Brien, 2015; Becker et al., 2016; Willebrords et al., 2017). Recent insights in this matter have suggested cannabinoids (CBs) as molecules that could counteract the opening of hemichannels and pannexons during neuroinflammatory conditions (Froger et al., 2009; Gajardo-Gómez et al., 2017). In this review, we first describe major features of the brain endocannabinoid (eCB) system and its critical role in synaptic transmission. Later, the possible neuroprotective actions of eCBs are discussed, in particular, the inhibition of the uncontrolled opening of glial cell hemichannels. Finally, we hypothesize how astroglial hemichannels may participate in eCB-mediated synaptic transmission in the healthy brain.
The Endocannabinoid System at the CNS
Although cannabis (Cannabis sativa) has been widely used throughout history for medicinal, religious, recreational and social purposes, just in the past two decades research in the field has brought to light its multiple benefits (Corcos et al., 2005; Biswas et al., 2017; Mahvan et al., 2017; Yassin et al., 2017). In 1964, tetrahydrocannabinol (THC), the principal psychoactive constituent of cannabis, was isolated and purified, permitting the elucidation of its chemical structure (Gaoni and Mechoulam, 1964). Since then, many studies have been conducted to reveal the molecular mechanisms responsible for the psychoactive effects of cannabis-derived compounds, also known as phytocannabinoids (Zuardi, 2008). Late in the 1980s, as a result of decades of research on THC, Devane et al. (1992b) discovered the binding sites for the first CB receptor in the brain: CB1. Shortly after the cloning of the CB1 receptor (Matsuda et al., 1990), a second receptor called CB2 was isolated from human promyelocytic leukemia cells (Munro et al., 1993), whereas two eCBs agonists were identified: anandamide or N-arachidonoylethanolamine (AEA) and 2-arachidonylglycerol (2-AG; Devane et al., 1992a; Sugiura et al., 1995). Up to now, several molecules encompass the current thirteen-member list of eCBs (Pertwee, 2015), including O-arachidonoyl ethanolamine (Porter et al., 2002) and 2-arachidonyl glyceryl ether (Hanus et al., 2001); nevertheless, most studies have been focalized on AEA and 2-AG. Whether plant derived, synthetic or endogenous, CBs are lipid messengers that activate at least two Gi/o protein-coupled receptors; CB1 and CB2; and one ionotropic receptor: the transient receptor potential vanilloid 1 (TRPV1; Zygmunt et al., 1999). AEA predominantly acts as partial agonist of both CB1 and CB2 receptors and shows less relative intrinsic efficacy and affinity for CB2 than for CB1 receptors (Pertwee, 2010). On the other hand, 2-AG binds with the same affinity to both receptors and exhibits higher potency than AEA as CB1 and CB2 receptor agonist (Pertwee, 2010). AEA also binds and activates TRPV1s (Zygmunt et al., 1999), whereas 2-AG also binds GABAA receptors (Sigel et al., 2011). In concert, eCBs, their receptors and regulatory synthetic (N-acylphosphatidylethanolamine phospholipase D [NAPE-PLD] and diacyglyerol lipase α [DAGLα]) and catabolic (fatty acid amide hydrolase [FAAH], monoacylglycerol lipase [MAGL] and others) enzymes constitute the triad usually referred as the eCB system (Battista et al., 2006). This systemic pathway plays key homeostatic functions in the CNS, as well as in multiple peripheral sites including skin, cardiovascular system, gastrointestinal tract, adipose tissue and liver (Pertwee, 2012).
At the CNS, AEA originates from the metabolism of NAPE by the activity of NAPE-PLD, being this process dependent on rising [Ca2+]i upon depolarization and/or activation of ionotropic receptors (Luchicchi and Pistis, 2012). The biosynthesis of 2-AG from triacylglycerols requires the action of DAGL triggered by the activation of metabotropic receptors coupled to the PLCβ (Kreitzer and Regehr, 2002). Once postsynaptic neurons release eCBs into the synaptic cleft, they diffuse in a retrograde manner to activate presynaptic Gi/o-coupled CB1 receptors, which result in the hyperpolarization of presynaptic terminals and as a consequence decreases neurotransmitter release (Ohno-Shosaku et al., 2001). The latter occurs at least by two major mechanisms of synaptic depression. At one end, short-term stimulation of CB1 receptors may lead to presynaptic inhibition of Ca2+ influx through N- and P/Q-type voltage-gated Ca2+ channels (Kreitzer and Regehr, 2002; Nimmrich and Gross, 2012), or activation of presynaptic A-type and inward rectifier K+ channels (Kreitzer and Regehr, 2002). At the other end, long-term activation of CB1 receptors may blunt the function of adenylyl cyclase (Childers and Deadwyler, 1996), resulting in the subsequent reduction of presynaptic cAMP levels and PKA activity (Chevaleyre et al., 2007). Synaptic signaling of eCBs ceases with their re-uptake and subsequent intracellular degradation. Inactivation of AEA takes place primarily by action of FAAH (Cravatt et al., 1996), whereas 2AG is degraded by the presynaptic enzyme MAGL and α/β-Hydrolase domain-containing 6 (Dinh et al., 2002; Marrs et al., 2010). Additionally, eCBs can act as non-retrograde messengers, where 2-AG may stimulate postsynaptic CB1 or CB2 receptors, whereas AEA may open postsynaptic TRPV1s (Castillo et al., 2012).
Most of the psychoactive effects of cannabis depend on CB1 receptors and their vast expression on neurons throughout the brain, including the basal ganglia, cerebellar cortex and hippocampus (Moldrich and Wenger, 2000). Despite of being characterized in some neurons (Onaivi, 2011; Stempel et al., 2016); CB2 receptors seem mostly found in glial cells at the nervous system (see below) and immune cell in the periphery, such as macrophages, neutrophils, lymphocytes, natural killer cells and monocytes (Galiègue et al., 1995; Buckley et al., 2000; Núñez et al., 2004). What remained as an open question for a long time is whether glial cells actually express CB receptors. Although early studies described the presence of CB1 and CB2 receptors in astrocytomas and primary astroglial cultures (Bouaboula et al., 1995; Sánchez et al., 1998; Molina-Holgado F. et al., 2002; Sheng et al., 2005), other studies did not find CB receptors in astrocytes (Sagan et al., 1999; Walter and Stella, 2003; Lou et al., 2012). In microglia, first reports showed an important expression of CB2 receptors in primary cultures and cell lines from different species (Carlisle et al., 2002; Facchinetti et al., 2003; Klegeris et al., 2003; Walter et al., 2003; Ramírez et al., 2005), however, CB1 receptors were rarely detected (Waksman et al., 1999). These contradictory results may reflect differences in the approaches used, including animal strains or species, antibody specificity and efficacy and cell conditions, as well as the fact that lower expression of CB receptors could make extremely difficult their identification by western blotting or immunohistochemistry (Onaivi et al., 2012; Metna-Laurent and Marsicano, 2015). In particular, several in vitro culture conditions influence glial function and inflammatory profile, such as type of isolation, culture medium, serum supplementation, medium changes, confluence, cell age, substrates and purity (Saura, 2007; Codeluppi et al., 2011; Stansley et al., 2012; Bohlen et al., 2017). Nowadays, most in vitro and in vivo evidence indicates that both astrocytes and microglia express CB1 and CB2 receptors in rodents (Gong et al., 2006; Navarrete and Araque, 2008; Palazuelos et al., 2009; Sagredo et al., 2009; Mecha et al., 2015; Navarro et al., 2018), dogs (Fernández-Trapero et al., 2017) and humans (Benito et al., 2005, 2007), thus playing critical roles in immunomodulatory responses and synaptic plasticity (Di Marzo et al., 2015; Oliveira da Cruz et al., 2016).
Neuroprotective Actions of Cannabinoids Via the Inhibition of Hemichannels
Neuroinflammation is a pivotal determinant in the pathogenesis and progression of multiple acute and chronic neurodegenerative diseases. Microglial activation, reactive astrogliosis, production of inflammatory mediators (cytokines, chemokines, nitric oxide [NO], reactive oxygen and nitrogen species [ROS/RNS]), BBB breakdown and subsequent brain infiltration of circulating immune cells characterize this process (Becher et al., 2017). Both microglial activation and reactive astrogliosis constitute graded and multistage conserved glial reactions that counteract acute damage, restoring the homeostasis and limiting the brain parenchyma injury (Kettenmann et al., 2011; Pekny and Pekna, 2014). Nevertheless, during severe challenges and chronic brain damage, microglia and astrocytes may turn in uncontrolled source of inflammatory mediators rather than exhibiting a repair-oriented activity profile. While an efficient immune response is necessary to resolve brain threats, under the above circumstances, astrocytes and microglia may worsen disease progression by altering synaptic function, ion homeostasis, antioxidant defense and neuronal survival.
A growing body of data support the idea that eCBs are endowed with powerful immunoregulatory and anti-inflammatory properties, influencing both the CNS and peripheral tissues (Walter and Stella, 2003; Rom and Persidsky, 2013; Turcotte et al., 2015). eCBs and synthetic CB receptor agonists decrease the production of NO, ROS/RNS, free radicals and pro-inflammatory cytokines in activated glial cells, while facilitate the switching of dysfunctional microglia towards an anti-inflammatory phenotype (Waksman et al., 1999; Molina-Holgado E. et al., 2002; Molina-Holgado et al., 2003; Sheng et al., 2005; Mecha et al., 2015). Remarkably, brain levels of eCBs and glial CB receptors increase during neuroinflammation and neurodegenerative conditions, which may reflect self-neuroprotective and adaptive processes aimed at limiting the deleterious effects of inflammatory responses. In this line, CBs have been proposed as therapeutic tools to tackle several brain pathologies such as AD, multiple sclerosis (MS), Huntington’s disease (HD), traumatic brain injury (TBI), Parkinson’s disease (PD), among others (Kendall and Yudowski, 2016; Lu and Mackie, 2016). Supporting this notion, CB administration greatly mitigates the symptoms generated in animal models of MS (Lyman et al., 1989), HD (Palazuelos et al., 2009) and AD (Ramírez et al., 2005; Martín-Moreno et al., 2012), as well as a well-characterized model of chronic neuroinflammation produced by the infusion of lipopolysaccharide (LPS; Marchalant et al., 2007). Accumulating evidence suggests that neuroprotective actions of CBs depend on cellular and molecular events modulating the dysfunctional status of glial cells (Stella, 2004, 2010). At this point, one line of thought has argued that CBs may favor neuronal survival by inhibiting the uncontrolled activity of glial hemichannels and pannexons (Orellana et al., 2012c).
Inflammation has been established as a corner stone in the impaired function of hemichannels and pannexons not only in the CNS but also in peripheral organs (Kim et al., 2016; Crespo Yanguas et al., 2017). Just in the last 3 years a large list of inflammatory agents have been shown to exacerbate the opening of these channels in glial cells, such as cytokines (Abudara et al., 2015), growth factors (Garre et al., 2016), LPS (Avendaño et al., 2015), human immunodeficiency virus (Orellana et al., 2014) and ultrafine carbon black particles (Wei et al., 2014). Over the same period, similar findings have been found in multiple animal models of human disease, including amyotrophic lateral sclerosis (Almad et al., 2016), AD (Yi et al., 2016), hypercholesterolemia (Orellana et al., 2014), traumatic injury (Rovegno et al., 2015) and stress (Orellana et al., 2015). The release of cytokines from activated microglia represents a fundamental mechanism of Cx43 hemichannel activation in astrocytes in vitro (Retamal et al., 2007) and ex vivo (Abudara et al., 2015). In healthy conditions, the expression and activity of these channels is not high, but enough to ensure the release and influx of relevantly biological substances (Orellana et al., 2016). However, when astrocytes are cultured with primary microglia in presence of LPS, the opening of Cx43 hemichannels, determined from unitary current and dye uptake recordings, is prominently raised (Retamal et al., 2007). The latter response is emulated with conditioned media (CM) harvested from LPS-stimulated primary microglia, bringing up the idea that soluble factors released by microglia modulate astrocyte hemichannels (Retamal et al., 2007). ELISA, immunoneutralization and cytokine receptor blocking analysis reveal that IL-1β and TNF-α are indeed the soluble factors increasing Cx43 hemichannel opening in primary astrocytes (Retamal et al., 2007), which is consistent with previous studies showing that both cytokines reduce Cx43 expression and coupling between astrocytes (Même et al., 2006). Hence, IL-1β and TNF-α released by LPS-treated microglia regulate gap junctions and hemichannels in an opposing manner in cultured astrocytes (Retamal et al., 2007).
With this in mind, Froger et al. (2009) investigated whether endogenous (methanandamide [Meth], a non-hydrolyzable analog of AEA) and synthetic (WIN-55,212-2 [WIN] and CP 55,940 [CP]) CBs could modulate the release of IL-1β and TNF-α from LPS-treated microglia and their opposite effects on astroglial GJC and hemichannel function. They found that WIN, CP or Meth suppress the release of TNF-α and IL-1β by LPS-treated primary microglia (Froger et al., 2009), reinforcing previous reports describing that THC, as well as eCBs or synthetic CBs, blunt the expression and production of both cytokines in activated primary microglia (Puffenbarger et al., 2000; Facchinetti et al., 2003). More relevantly, these CBs prevented the astroglial uncoupling elicited by the following conditions: (i) LPS treatment in astrocyte-microglia primary mixed cultures; (ii) CM harvested from LPS-activated primary microglia; or (iii) mixture of IL-1β and TNF-α (Froger et al., 2009). Particularly, these preventive effects occurred in an additive way, indicating that WIN, CP and Meth likely act on different CB receptors. Coincident with this line of though, CB1 or CB2 receptor blockers abrogated with distinct pharmacological profiles the counteracting actions of WIN and Meth (Froger et al., 2009). The latter fit with the current notion of astrocytes expressing both CB1 and CB2 receptors (Benito et al., 2007; Navarrete and Araque, 2008; Sagredo et al., 2009; Navarro et al., 2018). In the same study, WIN and Meth were found to fully abolish the increase in Cx43 hemichannel activity in astrocytes triggered by the mixture of IL-1β and TNF-α (Froger et al., 2009). Contrary to the preventive effects observed in astroglial uncoupling, the CB-mediated inhibition of hemichannel opening was not additive and depended only on the activation of CB1 receptors (Froger et al., 2009).
As indicated above, exacerbated opening of glial hemichannels or pannexons may lead to the release of potentially neurotoxic compounds, resulting in neuronal alterations and subsequent cell death. In a follow-up study, Froger et al. (2010) tested the contribution of Cx43 hemichannel opening to NMDA-induced excitotoxicity in neuron/astrocyte co-cultures after treatment with TNF-α and IL-β. NMDA treatment significantly increased neurotoxicity in cytokine-treated co-cultures compared to the untreated ones, whereas this response did not occur in neurons co-cultured with Cx43 knock-out astrocytes or in the presence of Cx43 hemichannel blockers (Froger et al., 2010). At this point, the authors addressed the possibility that CB treatment could have a neuroprotective effect in NMDA and cytokine-induced excitotoxicity. Concordant with this notion, WIN completely prevented the increase in NMDA neurotoxicity produced by the mixture of TNF-α and IL-β (Froger et al., 2010). In the same line, recent studies by Gajardo-Gómez et al. (2017) reported that CBs ameliorate the amyloid-β peptide (Aβ)-induced neuronal death by preventing the dysfunctional opening of Cx43 hemichannels in astrocytes. Using time-lapse measurements of ethidium uptake, as well as patch clamp recordings, they showed that WIN, 2-AG and Meth, fully neutralize the Aβ-induced Cx43 hemichannel opening in cultured astrocytes and acute hippocampal slices. These responses were accompanied with a significant decline of the Aβ-induced production of IL-1β, TNF-α and NO in astrocytes (Gajardo-Gómez et al., 2017). Similar to that found by Froger et al. (2009), blockade of CB1 receptors with SR-141716A completely prevented the counteracting action of WIN, 2-AG and Meth over the Aβ-induced Cx43 hemichannel opening in cultured astrocytes. CBs also improved neuronal survival by mitigating the Aβ-induced excitotoxic release of glutamate and ATP linked to the opening of astroglial Cx43 hemichannels (Gajardo-Gómez et al., 2017). In discrepancy with primary cultures, in acute hippocampal slices the protective actions of WIN over hemichannel activity and neuronal death occurred via the activation of both CB1 and CB2 receptors, suggesting a cumulative action of CBs as they can act simultaneously in several cell types (Gajardo-Gómez et al., 2017).
How CBs impede the opening of hemichannels during pro-inflammatory conditions? A decade ago, Retamal et al. (2006) demonstrated that NO increases the opening of Cx43 hemichannels in ischemic astrocytes by inducing the S-nitrosylation of surface Cx43. Such key covalent modification also seems crucial for the cytokine-dependent opening of astroglial Cx43 hemichannels, as the inhibition of iNOS completely suppress this response in several pathological conditions (Retamal et al., 2007; Orellana et al., 2014; Avendaño et al., 2015; Gajardo-Gómez et al., 2017). In similar scenarios, blocking of p38 MAP kinase mitigates the activity of these channels, which is consistent with the fact that this pathway is a well-established cytokine target that induces iNOS expression and subsequent NO production in astrocytes (Wang et al., 2017; Figure 2). Altogether, these data denote that CB-mediated counteracting actions on hemichannel function may primarily proceeds by decreasing cytokine production and p38 MAP kinase activation, resulting in the subsequent suppression of NO production (Figure 2). In this context, the suppressive action of CBs on the NF-κβ pathway should be crucial, as it controls multiple aspects of neuroinflammation, including activation of glial cells and production of inflammatory mediators such as cytokines and NO (Shih et al., 2015; Figure 2). According with this notion, CBs blunt NF-κβ activation and reduce the production of NO and both IL-1β and TNF-α, as well as the activation of p38 MAP kinase in astrocytes (Curran et al., 2005; Sheng et al., 2009; Aguirre-Rueda et al., 2015), even in those stimulated with conditions that open hemichannels (e.g., Aβ; Aguirre-Rueda et al., 2015). Noteworthy, inhibition of NO production appears to rely on both CB1 and CB2 receptors (Sheng et al., 2005), while blockade of p38 MAP kinase activity is preferentially driven by CB2 receptors (Sheng et al., 2009). The latter may constitute a possible explication to the differential CB1/CB2 receptor pharmacology of synthetic and endogenous CBs in diverse astrocyte functions. A second but complementary mechanism of hemichannel regulation to that resulting from posttranslational modifications (e.g., S-nitrosylation) is the sorting of hemichannels to the cell surface. For instance, WIN fully abolish the Aβ-induced augment in surface levels of Cx43, signifying that modifications in surface expression of this protein may account for the preventive effects of CBs (Gajardo-Gómez et al., 2017). Future studies are required to elucidate the mechanisms associated to the CB-mediated regulation of surface Cx43.
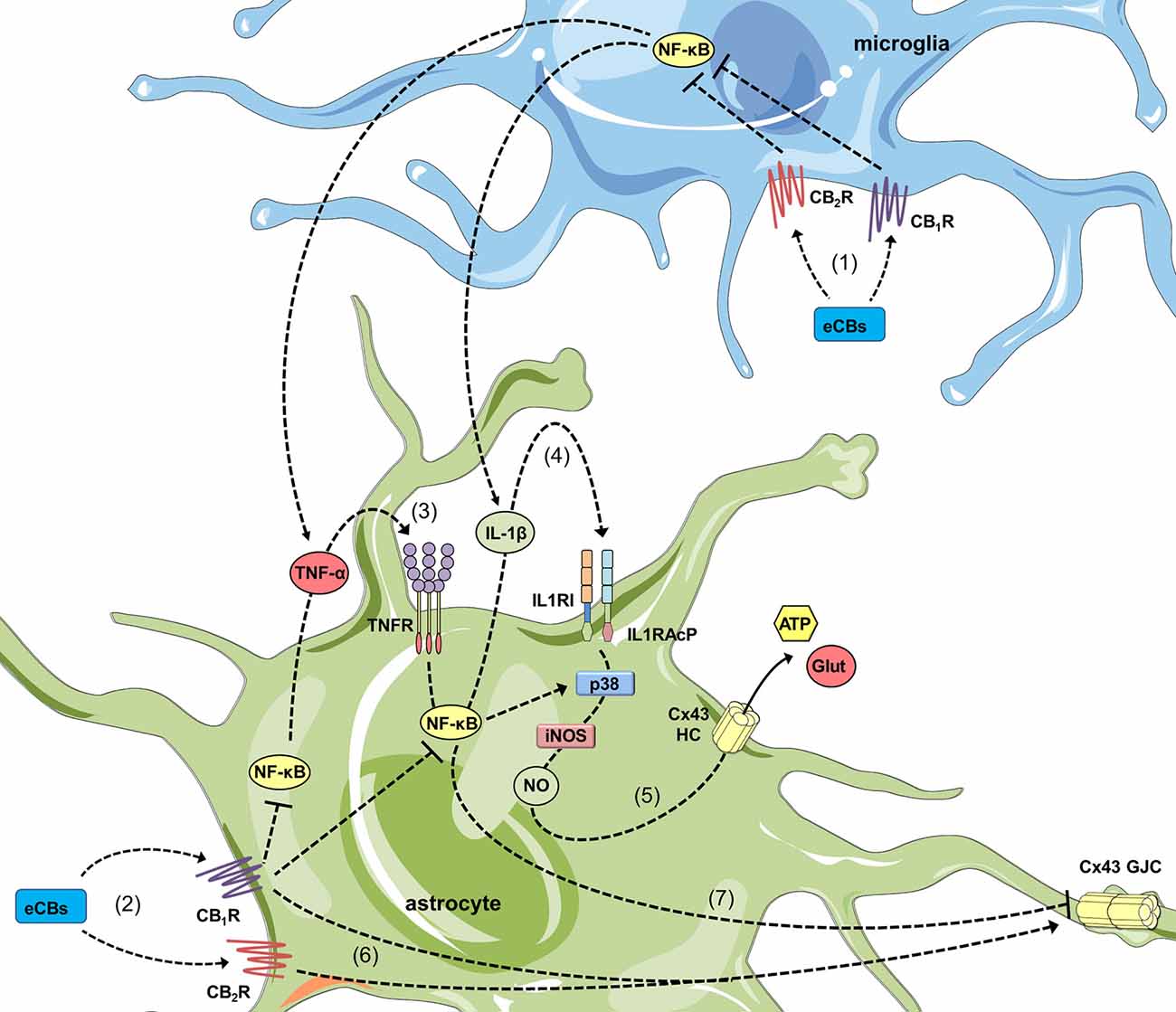
Figure 2. Cannabinoids (CBs) prevent the opposite regulation of astroglial connexin-based channels evoked by inflammatory conditions. In activated microglia, endocannabinoids (eCBs) acting on CB1Rs/CB2Rs counteract the NF-κβ-dependent release of TNF-α and IL-1β (1). In addition, in activated astrocytes, the stimulation of CB1Rs could also blunt the NF-κβ-mediated autocrine/paracrine release of TNF-α and IL-1β (2) along with the corresponding activation of TNFR1 (3) and IL1RI/IL1RAcP (4). The latter results in the inhibition of p38 MAP kinase and nitric oxide (NO) production, as well as the consequent reduction in excitotoxic release of gliotransmitters (e.g., glutamate and ATP) through astroglial Cx43 hemichannels (5). In parallel, activation of CB1Rs/CB2Rs may neutralize the reduction in gap junction communication (6) evoked by pro-inflammatory conditions (7).
The Possible Crosstalk Between Astrocyte Hemichannels and Cannabinoid System During Synaptic Transmission and Plasticity
Without a doubt, the strategic alliance between neurons and astrocytes is crucial to understand the contemporary notion of synaptic transmission and plasticity (Araque et al., 2014). Pioneering studies by Araque et al. (1998a,b) served to later coin the term “tripartite synapse”, which describes how astrocytic processes together with pre- and postsynaptic terminals constitute a functional structure that sustain the activity of neural circuits (Eroglu and Barres, 2010). In this delicate physical and functional interaction, astrocytes sense and respond to neuronal activity by releasing bioactive molecules termed “gliotransmitters” through different pathways, including vesicles, P2X7R, volume-regulated anion channels (VRACs), bestrophin-1 Ca2+-activated chloride channels, pannexons, hemichannels and transporters (Gundersen et al., 2015). In addition to embracing the synaptic cleft, astrocytes are endowed with specialized terminal processes so called “endfeet”, which ensheath capillaries, intracerebral arterioles and venules; covering almost completely the abluminal vascular surface (Simard et al., 2003). This complex crosstalk with neurons and vascular cells provides astrocytes with an incomparable architectural advantage to facilitate local and long-distance release of gliotransmitters, thereby modulating synaptic transmission and plasticity with potentially significant consequences for memory and behavior (Dallerac and Rouach, 2016).
As already mentioned, retrograde eCB signaling may mediate short-term (Kreitzer and Regehr, 2001; Wilson and Nicoll, 2001) and long-term (Gerdeman et al., 2002; Marsicano et al., 2002) mechanisms of synaptic depression at both excitatory and inhibitory synapses (Navarrete et al., 2012). Surprisingly, although CB1 receptors are usually coupled to Gi/o proteins (Piomelli, 2003), the CB1-dependent [Ca2+]i rise in astrocytes depends on the phospholipase C (PLC)-mediated IP3 production, indicating the involvement of Gq proteins rather than a “classical” inhibition of adenylate cyclase (Navarrete et al., 2012). Quite remarkably, eCB release from neurons requires astroglial Ca2+ elevations to stimulate glutamate release from astrocytes, which in turn, increases the frequency of postsynaptic NMDA receptor (NMDAR)-mediated slow inward currents (SIC) in proximal pyramidal neurons (Navarrete et al., 2012). A study from the same group described that [Ca2+]i rise elicited by astroglial CB1 receptors lead to heterosynaptic short-term facilitation of synaptic transmission, likely through glutamate released from astrocytes and subsequent activation of presynaptic metabotropic glutamate receptors type-1 (mGluR1; Navarrete and Araque, 2010). These studies reinforce the idea that while eCBs trigger transient synaptic depression at local synapses via presynaptic CB1 receptors, they also induce transient synaptic potentiation at distant synapses through activation of astrocytic CB1 receptors.
The involvement of astroglial CB1 receptors in synaptic transmission also include processes of long-term plasticity. Indeed, stimulation of astroglial CB1 receptors increases [Ca2+]i and causes glutamate release from astrocytes, which by activating presynaptic NMDARs trigger spike timing dependent long-term depression (t-LTD; Min and Nevian, 2012). Equivalent mechanisms of t-LTD have been found in the hippocampus, yet in these cases, either D-serine or glutamate seem the gliotransmitters involved in presynaptic or postsynaptic activation of NMDARs, respectively (Han et al., 2012; Andrade-Talavera et al., 2016). Noticeably, astroglial CB1 receptor-mediated t-LTD is critical for inducing the impairment of working memory in vivo, with the latter response being dependent on activation of NR2B-containing NMDARs and endocytosis of AMPARs (Han et al., 2012). Examples of astroglial participation in CB1 receptor-mediated long-term plasticity also include synaptic facilitation. Just a while ago, it was described that eCB-mediated activation of astroglial CB1 receptors elevates [Ca2+]i and induces glutamate release that acting on presynaptic mGluR1s evokes long-term potentiation (LTP) when it coincides with the postsynaptic release of NO (Gómez-Gonzalo et al., 2015). Similar events of LTP facilitation have been described in the mouse neocortex with the ATP as major gliotransmitter implicated (Rasooli-Nejad et al., 2014). These studies open up the possibility that eCBs may have opposite and complementary regulatory effects, namely, while their signaling lead to transient or LTD via presynaptic CB1 receptors in local homoneuronal synapses (Wilson and Nicoll, 2001; Chevaleyre and Castillo, 2003), they transiently (Navarrete and Araque, 2010) or persistently (Gómez-Gonzalo et al., 2015) potentiate synaptic transmission at more distant synapses through activation of astrocytes. It is unclear whether these opposite mechanisms might coexist in vivo or whether they occur only in specific physiological or pathophysiological circumstances.
While a lot has been learned about the role of astrocytes on eCB-mediated synaptic plasticity, how exactly gliotransmitters are released from astrocytes is uncertain but it could involve vesicular exocytosis in some cases. Using the light chain of tetanus toxin and Evans blue, an inhibitor of the vesicular glutamate transporter, Min and Nevian (2012) demonstrated that eCB-mediated t-LTD requires the SNARE-dependent exocytosis of astroglial glutamate. Likewise, other study showed that eCBs trigger release of ATP and D-serine from neocortical astrocytes by SNARE-complex-dependent mechanism, with ATP being crucial for LTP facilitation (Rasooli-Nejad et al., 2014). Although it is well-accepted that [Ca2+]i-dependent vesicular fusion of either large or small synaptic-like vesicles (Araque et al., 2000; Bezzi et al., 2004; Martineau et al., 2008, 2013; Kang et al., 2013) lead to glutamate and D-serine release from astrocytes, the involvement of alternative non-vesicular pathways in eCB-mediated plasticity deserve more investigation. One alternative mechanism may reside in the opening of hemichannels, either directly as a route for diffusion or indirectly by favoring Ca2+ entry that subsequently activates other [Ca2+]i-dependent gliotransmitter release pathways (Montero and Orellana, 2015). Certainly, hemichannels formed by Cx43, the predominant channels of their kind in astrocytes, have already been associated with the release of diverse gliotransmitters such as glutamate (Ye et al., 2003; Orellana et al., 2011b), ATP (Stout et al., 2002; Kang et al., 2008; Chever et al., 2014), D-serine (Meunier et al., 2017), glutathione (Rana and Dringen, 2007) and lactate (Karagiannis et al., 2016). A while ago, it was showed that lowering extracellular Ca2+ to concentrations that take place during neuronal bursting activity, causes ATP efflux via astroglial Cx43 hemichannels, which subsequently strengths inhibitory transmission by activation of neuronal P2Y1R (Torres et al., 2012). The latter study brought to light the idea that gliotransmitter release linked to the physiological opening of astroglial hemichannels may regulate synaptic transmission. This was demonstrated later on by Chever et al. (2014), who found that constitutive function of astroglial Cx43 hemichannels contributes to ATP release and tuning of basal excitatory synaptic transmission in the hippocampus.
Additionally, when studied in basal conditions, the opening of astroglial Cx43 hemichannels enhances the amplitude of slow oscillations in mitral cells of the olfactory bulb (Roux et al., 2015) and is essential for fear memory consolidation in the basolateral amygdala (BLA), a brain region crucial for anxiety and emotional memory processing (Stehberg et al., 2012). The last-mentioned study revealed that microinjection of the BLA with TAT-L2, a peptide that specifically blocks Cx43 hemichannels, does not affect short-term memory, but fully induces amnesia towards an auditory fear conditioning paradigm (Stehberg et al., 2012). TAT-L2, along with Gap19, are the most predominant pharmacological tools used to inhibit hemichannel function without affecting gap junctional communication (Iyyathurai et al., 2013). TAT-L2 is a cell-permeable mimetic peptide of the so-called L2 cytoplasmic loop region of Cx43, whereas Gap19 is a smaller nonapeptide derived from it (Iyyathurai et al., 2013). Remarkably, the TAT-L2-mediated amnesic response was prevented by co-infusing a mixture of gliotransmitters together with the peptide, including glutamate, D-serine, glycine, lactate, ATP and glutamine. Likewise, Cx43 hemichannels appear to be critical for spatial memory. Using the spontaneous alternation Y maze training, a recent study reported that blockade of astroglial Cx43 hemichannels impairs hippocampal-dependent short-term spatial memory, but not working memory (Walrave et al., 2016). Decisive evidence establishing the involvement of astroglial hemichannels in regulating synaptic transmission came from a recent study of Giaume’s group. They observed that Cx43 hemichannel inhibition and clamping [Ca2+]i in astrocytes decreases NMDA but not AMPA postsynaptic currents in the prefrontal cortex (Meunier et al., 2017). Furthermore, electrophysiological experiments of high frequency stimulation revealed D-serine release linked to astroglial Cx43 hemichannel opening is crucial for LTP of NMDA or AMPA synaptic currents (Meunier et al., 2017). This study uncover a possible mechanism to explain previous findings showing that exogenous administration of D-serine revert LTP impairment caused by inhibition of [Ca2+]i oscillations in astrocytes (Henneberger et al., 2010).
Overall, the above studies provide a solid picture about the role of astroglial hemichannels in synaptic transmission and thus their involvement on eCB-mediated neural plasticity could be critical. How hemichannels may contribute to the eCB-dependent synaptic dialog between astrocytes and neurons? Given that postsynaptic eCBs cause the [Ca2+]i-dependent release of glutamate and D-serine from astrocytes (Navarrete and Araque, 2008, 2010; Andrade-Talavera et al., 2016), one may question whether Cx43 hemichannels are involved in this process. In this line, a recent work has shown that eCBs may activate astrocytic hemichannels under basal conditions. Using two-photon in vivo microscopy, Vázquez et al. (2015) detected an increased basal activity of astroglial Cx43 hemichannels in the cortex of FAAH-null mice, which possess 15-fold augmented endogenous brain levels of AEA (Cravatt et al., 2001). Equivalent results were observed when AEA was directly applied in the mouse cortex (Vázquez et al., 2015), attributing to eCBs the ability of stimulate basal activity of astroglial hemichannels in the normal brain. It is noteworthy that CB1-dependent elevation of [Ca2+]i in astrocytes relies on PLC activation and further IP3 production (Navarrete and Araque, 2008), the latter being a well-recognized pathway involved in the activation of Cx43 hemichannels (De Bock et al., 2012; Orellana et al., 2012a; Bol et al., 2017), including in astrocytes (Alvarez et al., 2016). Indeed, the opening of Cx43 hemichannels respond to changes in cytoplasmic Ca2+ according to a bell-shaped “convex-up” pattern, with maximal activity in the 500 nM range and decreasing activities at both higher and lower [Ca2+]i (De Bock et al., 2012). Dye uptake and single-channel recordings have been used to prove this feature in astrocytes, glioma cells and other cells types (De Vuyst et al., 2009; De Bock et al., 2012; Wang et al., 2013b; Bol et al., 2017; Meunier et al., 2017). This evidence places the hemichannels as possible candidates to mediate directly or indirectly the eCB-dependent release of glutamate, D-serine or ATP from astrocytes, the latter being potentially significant for neuronal synaptic function (Figure 3).
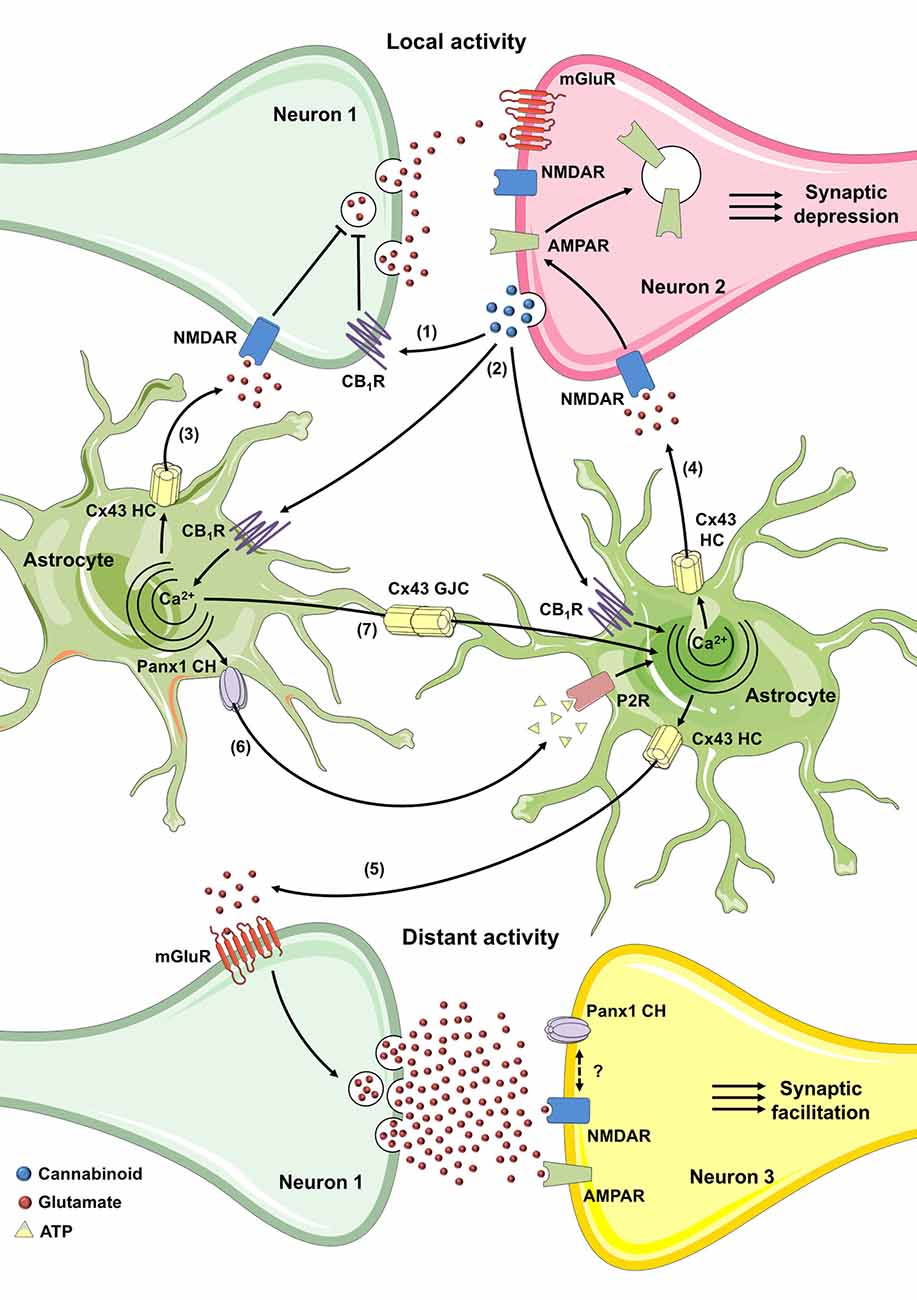
Figure 3. Possible roles of connexin- and pannexin-based channels in CB-mediated synaptic plasticity through activation of astrocytes. In the hippocampus, the activity-dependent production of eCB triggers a decrease of neurotransmitter release through the stimulation of presynaptic CB1Rs in homoneuronal synapses (1). In the cortex and hippocampus, the coincidence of postsynaptic metabotropic glutamate receptor (mGluR) activation during synaptic activity and Ca2+ influx (not depicted) caused by postsynaptic back-propagating action potentials evoke the release of eCBs (2). The latter stimulates astroglial CB1 receptors and could elicit the Ca2+-dependent release of glutamate or D-serine through Cx43 hemichannels (3), leading to the activation of presynaptic NMDA receptors (NMDARs) and subsequent induction of spike timing dependent long-term depression (t-LTD; Min and Nevian, 2012; Andrade-Talavera et al., 2016). Alternatively, in the hippocampus, astroglial CB1 receptor activation and rise of cytoplasmic Ca2+ may cause the Cx43 hemichannel-dependent release of glutamate (4) which, through the stimulation of postsynaptic NMDAR, elicits the internalization of AMPARs and further t-LTD (Han et al., 2012). At the other end, the eCB-mediated increase in cytoplasmic Ca2+ may trigger the release of glutamate through Cx43 hemichannels (5) that, acting on mGluRs induces lateral potentiation of synaptic transmission at distant synapses (Navarrete and Araque, 2010; Gómez-Gonzalo et al., 2015). The interacting coupling between NMDARs and Pannexin 1 (Panx1) channels could be a possible mechanism to potentiate the above response. Finally, purinergic signaling mediated by ATP released via Panx1 channels (6) along with gap junctional communication (7) among astrocytes could favor the widespread of eCB-mediated intracellular Ca2+ responses.
Another angle not mentioned so far is the eventual contribution of connexin/pannexin-dependent widespread of astrocyte Ca2+ responses in gliotransmission and subsequent CB-mediated potentiation of heterosynaptic plasticity at distant synapses (Navarrete and Araque, 2010; Gómez-Gonzalo et al., 2015). Although ATP seems released through different pathways (Zhang et al., 2007; Kreft et al., 2009), several studies suggests that hemichannels and pannexons are the major contributors to this phenomenon in astrocytes (Stout et al., 2002; Kang et al., 2008; Suadicani et al., 2012). Noteworthy, Panx1 channel-dependent release of ATP relies on protein-protein interactions between P2X7Rs and Panx1 (Locovei et al., 2007). In fact, Panx1 co-immunoprecipitates with P2X7Rs (Pelegrin and Surprenant, 2006; Silverman et al., 2009), and proline 451 in their C-terminal tails has been involved in this interaction (Iglesias et al., 2008; Sorge et al., 2012). P2YR stimulation also may mediate ATP efflux from astrocytes by activating the PLC/IP3/Ca2+-dependent opening of hemichannels and pannexons, as has been previously demonstrated for other cell types (Locovei et al., 2006; Orellana et al., 2012a). In addition, because hemichannels are permeable to Ca2+ (Sánchez et al., 2009; Schalper et al., 2010; Fiori et al., 2012), their dependency on [Ca2+]i may contribute to perpetuate [Ca2+]i-induced Ca2+ entry pathways associated to ATP release. Altogether, these data highlight that ATP signaling via purinergic receptors, hemichannels and pannexons may represent a plausible mechanism for underlying the CB1-dependent astroglial modulation of synaptic transmission and plasticity (Figure 3). In agreement with this notion, ATP released from eCB-stimulated astrocytes directly activates post-synaptic P2XRs, facilitating LTP due to downregulation of synaptic and extra-synaptic GABA receptors in cortical pyramidal neurons (Rasooli-Nejad et al., 2014). Desensitization of purinergic P2XRs/P2YRs and degradation of extracellular ATP by exonucleases may turn off in part ATP-dependent widespread of Ca2+ responses in astrocytes (Fields and Burnstock, 2006). Other negative feedback loops may reside in the direct counteracting action of ATP on Panx1 channels (Qiu and Dahl, 2009), as well as the inhibition of Cx43 hemichannels by [Ca2+]i over 500 nM (Meunier et al., 2017). Future studies will uncover whether opening of hemichannels and pannexons may contribute to astrocyte signaling during eCB-mediated synaptic transmission and plasticity.
In addition to contributing to astroglial-mediated release of gliotransmitters, Panx1 channels may also regulate synaptic communication given its broad expression and functionality in neurons (Thompson, 2015). Precisely, Panx1 is mainly found in the postsynaptic density of excitatory neurons (Zoidl et al., 2007) and couple NMDARs (Weilinger et al., 2012), making it well positioned to participate in eCB-mediated synaptic plasticity. Two independent groups have showed that acute hippocampal slices from adult Panx1 KO mice exhibit a significant increase in synaptic transmission, as measured in input-output curves at the hippocampal Schaffer-collateral CA1 synapse (Prochnow et al., 2012; Ardiles et al., 2014). As either adenosine application or blockade of NMDARs restored normal synaptic transmission (Prochnow et al., 2012), it is possible that loss of Panx1 may trigger extracellular adenosine depletion, thus favoring activation of postsynaptic NMDARs. Supporting this idea, stimulation of adenosine A1 receptors blunt the release of glutamate from pre-synaptic terminals (Dunwiddie and Masino, 2001) and hyperpolarizes post-synaptic neurons via ATP-sensitive K+ channels (Kawamura et al., 2010). Up to now, it is unclear whether a similar mechanism might account for transient or long-term eCB-mediated synaptic depression in the hippocampus or cortex (Han et al., 2012; Min and Nevian, 2012; Andrade-Talavera et al., 2016).
Concluding Remarks and Future Directions
As we noted before, it seems that the outcome of CBs in the functional activity of hemichannels will depend on the physiological status of the brain. Namely, under physiological conditions, eCBs may induce the controlled opening of astrocyte hemichannels and the consequent release of gliotransmitters. This may be particularly relevant for transient or long-lasting mechanisms of synaptic transmission and plasticity evoked by eCBs. At the other end, in the inflamed brain, eCBs may counteract the dysfunctional opening of hemichannels by triggering a large-scale of anti-inflammatory pathways that result in the inhibition of hemichannels and stimulation of astroglial coupling. The exact scenario and cellular cascades by which eCBs lead to this dual opposite regulation of hemichannels remain uncertain. Nevertheless, part of the explanation may rely in that eCBs may have different outcomes depending on which CB receptor they are exerting their actions. Additionally, the altered expression and production of CB receptors and eCBs during pathological conditions, respectively, may trigger different pathways that oppositely regulate the function of hemichannels. Considering the recent evidence linking the activity of GJCs, hemichannels and pannexons with major depression, addiction, autism, epilepsy and schizophrenia (Sarrouilhe et al., 2017), one may question whether the dialog between connexin/pannexin-based channels and the CB system may also impact the pathogenesis and progression of psychiatric disorders. At the other end, given the well-established role of eCB receptors in adult neurogenesis (Prenderville et al., 2015) and because connexin/pannexin function has been implicated in this process (Rozental et al., 1998; Swayne et al., 2010; Liebmann et al., 2013; Salmina et al., 2014; Swayne and Bennett, 2016), it would be interesting to unveil how the interaction between both pathways may impact neural progenitor proliferation, neuronal differentiation, maturation and survival. The mechanisms involved in all these events need to be studied further to better understand their biological implications for developing novel therapies against different brain diseases.
Author Contributions
JAO: conceived and designed the major ideas developed in the manuscript. VCL, CAS, RG-G, EFD, GIG and JAO: reviewed the literature and designed the figures; wrote and edited the manuscript. All authors read and approved the final manuscript.
Funding
This work was supported by Comisión Nacional de Investigación Científica y Tecnológica (CONICYT) and Programa de Investigación Asociativa (PIA): Grant Anillo de Ciencia y Tecnología ACT1411 (to JAO); Fondo Nacional de Desarrollo Científico y Tecnológico (FONDECYT): Grant 1160710 (to JAO).
Conflict of Interest Statement
The authors declare that the research was conducted in the absence of any commercial or financial relationships that could be construed as a potential conflict of interest.
Acknowledgments
We thank CONICYT, PIA, FONDECYT and Pontificia Universidad Católica de Chile.
References
Abudara, V., Roux, L., Dallérac, G., Matias, I., Dulong, J., Mothet, J. P., et al. (2015). Activated microglia impairs neuroglial interaction by opening Cx43 hemichannels in hippocampal astrocytes. Glia 63, 795–811. doi: 10.1002/glia.22785
Adamson, S. E., and Leitinger, N. (2014). The role of pannexin1 in the induction and resolution of inflammation. FEBS Lett. 588, 1416–1422. doi: 10.1016/j.febslet.2014.03.009
Aguirre-Rueda, D., Guerra-Ojeda, S., Aldasoro, M., Iradi, A., Obrador, E., Mauricio, M. D., et al. (2015). WIN 55,212–2, agonist of cannabinoid receptors, prevents amyloid β1–42 effects on astrocytes in primary culture. PLoS One 10:e0122843. doi: 10.1371/journal.pone.0122843
Allen, N. J., and Eroglu, C. (2017). Cell biology of astrocyte-synapse interactions. Neuron 96, 697–708. doi: 10.1016/j.neuron.2017.09.056
Almad, A. A., Doreswamy, A., Gross, S. K., Richard, J. P., Huo, Y., Haughey, N., et al. (2016). Connexin 43 in astrocytes contributes to motor neuron toxicity in amyotrophic lateral sclerosis. Glia 64, 1154–1169. doi: 10.1002/glia.22989
Alvarez, A., Lagos-Cabré, R., Kong, M., Cárdenas, A., Burgos-Bravo, F., Schneider, P., et al. (2016). Integrin-mediated transactivation of P2X7R via hemichannel-dependent ATP release stimulates astrocyte migration. Biochim. Biophys. Acta 1863, 2175–2188. doi: 10.1016/j.bbamcr.2016.05.018
Ambrosi, C., Gassmann, O., Pranskevich, J. N., Boassa, D., Smock, A., Wang, J., et al. (2010). Pannexin1 and Pannexin2 channels show quaternary similarities to connexons and different oligomerization numbers from each other. J. Biol. Chem. 285, 24420–24431. doi: 10.1074/jbc.M110.115444
Anderson, C. M., Bergher, J. P., and Swanson, R. A. (2004). ATP-induced ATP release from astrocytes. J. Neurochem. 88, 246–256. doi: 10.1111/j.1471-4159.2004.02204.x
Andrade-Talavera, Y., Duque-Feria, P., Paulsen, O., and Rodríguez-Moreno, A. (2016). Presynaptic spike timing-dependent long-term depression in the mouse hippocampus. Cereb. Cortex 26, 3637–3654. doi: 10.1093/cercor/bhw172
Araque, A., Carmignoto, G., Haydon, P. G., Oliet, S. H., Robitaille, R., and Volterra, A. (2014). Gliotransmitters travel in time and space. Neuron 81, 728–739. doi: 10.1016/j.neuron.2014.02.007
Araque, A., Li, N., Doyle, R. T., and Haydon, P. G. (2000). SNARE protein-dependent glutamate release from astrocytes. J. Neurosci. 20, 666–673.
Araque, A., Parpura, V., Sanzgiri, R. P., and Haydon, P. G. (1998a). Glutamate-dependent astrocyte modulation of synaptic transmission between cultured hippocampal neurons. Eur. J. Neurosci. 10, 2129–2142. doi: 10.1046/j.1460-9568.1998.00221.x
Araque, A., Sanzgiri, R. P., Parpura, V., and Haydon, P. G. (1998b). Calcium elevation in astrocytes causes an NMDA receptor-dependent increase in the frequency of miniature synaptic currents in cultured hippocampal neurons. J. Neurosci. 18, 6822–6829.
Ardiles, A. O., Flores-Muñoz, C., Toro-Ayala, G., Cárdenas, A. M., Palacios, A. G., Muñoz, P., et al. (2014). Pannexin 1 regulates bidirectional hippocampal synaptic plasticity in adult mice. Front. Cell. Neurosci. 8:326. doi: 10.3389/fncel.2014.00326
Avendaño, B. C., Montero, T. D., Chávez, C. E., von Bernhardi, R., and Orellana, J. A. (2015). Prenatal exposure to inflammatory conditions increases Cx43 and Panx1 unopposed channel opening and activation of astrocytes in the offspring effect on neuronal survival. Glia 63, 2058–2072. doi: 10.1002/glia.22877
Battista, N., Fezza, F., Finazzi-Agrò, A., and Maccarrone, M. (2006). The endocannabinoid system in neurodegeneration. Ital. J. Biochem. 55, 283–289.
Becher, B., Spath, S., and Goverman, J. (2017). Cytokine networks in neuroinflammation. Nat. Rev. Immunol. 17, 49–59. doi: 10.1038/nri.2016.123
Becker, D. L., Phillips, A. R., Duft, B. J., Kim, Y., and Green, C. R. (2016). Translating connexin biology into therapeutics. Semin. Cell Dev. Biol. 50, 49–58. doi: 10.1016/j.semcdb.2015.12.009
Benito, C., Kim, W. K., Chavarria, I., Hillard, C. J., Mackie, K., Tolón, R. M., et al. (2005). A glial endogenous cannabinoid system is upregulated in the brains of macaques with simian immunodeficiency virus-induced encephalitis. J. Neurosci. 25, 2530–2536. doi: 10.1523/JNEUROSCI.3923-04.2005
Benito, C., Romero, J. P., Tolón, R. M., Clemente, D., Docagne, F., Hillard, C. J., et al. (2007). Cannabinoid CB1 and CB2 receptors and fatty acid amide hydrolase are specific markers of plaque cell subtypes in human multiple sclerosis. J. Neurosci. 27, 2396–2402. doi: 10.1523/JNEUROSCI.4814-06.2007
Berman, J. W., Carvallo, L., Buckner, C. M., Luers, A., Prevedel, L., Bennett, M. V., et al. (2016). HIV-tat alters Connexin43 expression and trafficking in human astrocytes: role in NeuroAIDS. J. Neuroinflammation 13:54. doi: 10.1186/s12974-016-0510-1
Bezzi, P., Gundersen, V., Galbete, J. L., Seifert, G., Steinhäuser, C., Pilati, E., et al. (2004). Astrocytes contain a vesicular compartment that is competent for regulated exocytosis of glutamate. Nat. Neurosci. 7, 613–620. doi: 10.1038/nn1246
Biswas, P., Mishra, P., Bose, D., and Durgbanshi, A. (2017). Cannabis: a neurological remedy or a drug of abuse in india. CNS Neurol. Disord. Drug Targets 16, 576–584. doi: 10.2174/1871527316666170424115008
Bloomfield, S. A., and Volgyi, B. (2009). The diverse functional roles and regulation of neuronal gap junctions in the retina. Nat. Rev. Neurosci. 10, 495–506. doi: 10.1038/nrn2636
Bohlen, C. J., Bennett, F. C., Tucker, A. F., Collins, H. Y., Mulinyawe, S. B., and Barres, B. A. (2017). Diverse requirements for microglial survival, specification, and function revealed by defined-medium cultures. Neuron 94, 759.e8–773.e8. doi: 10.1016/j.neuron.2017.04.043
Bol, M., Wang, N., De Bock, M., Wacquier, B., Decrock, E., Gadicherla, A., et al. (2017). At the cross-point of connexins, calcium, and ATP: blocking hemichannels inhibits vasoconstriction of rat small mesenteric arteries. Cardiovasc. Res. 113, 195–206. doi: 10.1093/cvr/cvw215
Bond, S. R., and Naus, C. C. (2014). The pannexins: past and present. Front. Physiol. 5:58. doi: 10.3389/fphys.2014.00058
Bouaboula, M., Bourrié, B., Rinaldi-Carmona, M., Shire, D., Le Fur, G., and Casellas, P. (1995). Stimulation of cannabinoid receptor CB1 induces krox-24 expression in human astrocytoma cells. J. Biol. Chem. 270, 13973–13980. doi: 10.1074/jbc.270.23.13973
Bruzzone, R., Hormuzdi, S. G., Barbe, M. T., Herb, A., and Monyer, H. (2003). Pannexins, a family of gap junction proteins expressed in brain. Proc. Natl. Acad. Sci. U S A 100, 13644–13649. doi: 10.1073/pnas.2233464100
Buckley, N. E., McCoy, K. L., Mezey, E., Bonner, T., Zimmer, A., Felder, C. C., et al. (2000). Immunomodulation by cannabinoids is absent in mice deficient for the cannabinoid CB2 receptor. Eur. J. Pharmacol. 396, 141–149. doi: 10.1016/s0014-2999(00)00211-9
Carlisle, S. J., Marciano-Cabral, F., Staab, A., Ludwick, C., and Cabral, G. A. (2002). Differential expression of the CB2 cannabinoid receptor by rodent macrophages and macrophage-like cells in relation to cell activation. Int. Immunopharmacol. 2, 69–82. doi: 10.1016/s1567-5769(01)00147-3
Castillo, P. E., Younts, T. J., Chávez, A. E., and Hashimotodani, Y. (2012). Endocannabinoid signaling and synaptic function. Neuron 76, 70–81. doi: 10.1016/j.neuron.2012.09.020
Chen, J., Tan, Z., Zeng, L., Zhang, X., He, Y., Gao, W., et al. (2013). Heterosynaptic long-term depression mediated by ATP released from astrocytes. Glia 61, 178–191. doi: 10.1002/glia.22425
Chevaleyre, V., and Castillo, P. E. (2003). Heterosynaptic LTD of hippocampal GABAergic synapses: a novel role of endocannabinoids in regulating excitability. Neuron 38, 461–472. doi: 10.1016/S0896-6273(03)00235-6
Chevaleyre, V., Heifets, B. D., Kaeser, P. S., Südhof, T. C., and Castillo, P. E. (2007). Endocannabinoid-mediated long-term plasticity requires cAMP/PKA signaling and RIM1α. Neuron 54, 801–812. doi: 10.1016/j.neuron.2007.05.020
Chever, O., Lee, C. Y., and Rouach, N. (2014). Astroglial connexin43 hemichannels tune basal excitatory synaptic transmission. J. Neurosci. 34, 11228–11232. doi: 10.1523/JNEUROSCI.0015-14.2014
Childers, S. R., and Deadwyler, S. A. (1996). Role of cyclic AMP in the actions of cannabinoid receptors. Biochem. Pharmacol. 52, 819–827. doi: 10.1016/0006-2952(96)00419-4
Codeluppi, S., Gregory, E. N., Kjell, J., Wigerblad, G., Olson, L., and Svensson, C. I. (2011). Influence of rat substrain and growth conditions on the characteristics of primary cultures of adult rat spinal cord astrocytes. J. Neurosci. Methods 197, 118–127. doi: 10.1016/j.jneumeth.2011.02.011
Corcos, M., Phan, O., Nezelof, S., and Jeammet, P. (2005). [Psychopathology of the cannabis user teenager]. Rev. Prat. 55, 35–40.
Cravatt, B. F., Demarest, K., Patricelli, M. P., Bracey, M. H., Giang, D. K., Martin, B. R., et al. (2001). Supersensitivity to anandamide and enhanced endogenous cannabinoid signaling in mice lacking fatty acid amide hydrolase. Proc. Natl. Acad. Sci. U S A 98, 9371–9376. doi: 10.1073/pnas.161191698
Cravatt, B. F., Giang, D. K., Mayfield, S. P., Boger, D. L., Lerner, R. A., and Gilula, N. B. (1996). Molecular characterization of an enzyme that degrades neuromodulatory fatty-acid amides. Nature 384, 83–87. doi: 10.1038/384083a0
Crespo Yanguas, S., Willebrords, J., Johnstone, S. R., Maes, M., Decrock, E., De Bock, M., et al. (2017). Pannexin1 as mediator of inflammation and cell death. Biochim. Biophys. Acta 1864, 51–61. doi: 10.1016/j.bbamcr.2016.10.006
Curran, N. M., Griffin, B. D., O’toole, D., Brady, K. J., Fitzgerald, S. N., and Moynagh, P. N. (2005). The synthetic cannabinoid R(+)WIN 55,212–2 inhibits the interleukin-1 signaling pathway in human astrocytes in a cannabinoid receptor-independent manner. J. Biol. Chem. 280, 35797–35806. doi: 10.1074/jbc.M507959200
Dahl, G. (2015). ATP release through pannexon channels. Philos. Trans. R. Soc. Lond. B Biol. Sci. 370:20140191. doi: 10.1098/rstb.2014.0191
Dahl, G., and Keane, R. W. (2012). Pannexin: from discovery to bedside in 11±4 years? Brain Res. 1487, 150–159. doi: 10.1016/j.brainres.2012.04.058
Dallerac, G., and Rouach, N. (2016). Astrocytes as new targets to improve cognitive functions. Prog. Neurobiol. 144, 48–67. doi: 10.1016/j.pneurobio.2016.01.003
De Bock, M., Wang, N., Bol, M., Decrock, E., Ponsaerts, R., Bultynck, G., et al. (2012). Connexin 43 hemichannels contribute to cytoplasmic Ca2+ oscillations by providing a bimodal Ca2+-dependent Ca2+ entry pathway. J. Biol. Chem. 287, 12250–12266. doi: 10.1074/jbc.M111.299610
De Vuyst, E., Wang, N., Decrock, E., De Bock, M., Vinken, M., Van Moorhem, M., et al. (2009). Ca2+ regulation of connexin 43 hemichannels in C6 glioma and glial cells. Cell Calcium 46, 176–187. doi: 10.1016/j.ceca.2009.07.002
Decrock, E., De Bock, M., Wang, N., Bultynck, G., Giaume, C., Naus, C. C., et al. (2015). Connexin and pannexin signaling pathways, an architectural blueprint for CNS physiology and pathology? Cell. Mol. Life Sci. 72, 2823–2851. doi: 10.1007/s00018-015-1962-7
Devane, W. A., Breuer, A., Sheskin, T., Järbe, T. U., Eisen, M. S., and Mechoulam, R. (1992a). A novel probe for the cannabinoid receptor. J. Med. Chem. 35, 2065–2069. doi: 10.1021/jm00089a018
Devane, W. A., Hanus, L., Breuer, A., Pertwee, R. G., Stevenson, L. A., Griffin, G., et al. (1992b). Isolation and structure of a brain constituent that binds to the cannabinoid receptor. Science 258, 1946–1949. doi: 10.1126/science.1470919
Di Marzo, V., Stella, N., and Zimmer, A. (2015). Endocannabinoid signalling and the deteriorating brain. Nat. Rev. Neurosci. 16, 30–42. doi: 10.1038/nrn3876
Dinh, T. P., Freund, T. F., and Piomelli, D. (2002). A role for monoglyceride lipase in 2-arachidonoylglycerol inactivation. Chem. Phys. Lipids 121, 149–158. doi: 10.1016/s0009-3084(02)00150-0
Domercq, M., Perez-Samartin, A., Aparicio, D., Alberdi, E., Pampliega, O., and Matute, C. (2010). P2X7 receptors mediate ischemic damage to oligodendrocytes. Glia 58, 730–740. doi: 10.1002/glia.20958
Dunwiddie, T. V., and Masino, S. A. (2001). The role and regulation of adenosine in the central nervous system. Annu. Rev. Neurosci. 24, 31–55. doi: 10.1146/annurev.neuro.24.1.31
Eroglu, C., and Barres, B. A. (2010). Regulation of synaptic connectivity by glia. Nature 468, 223–231. doi: 10.1038/nature09612
Esseltine, J. L., and Laird, D. W. (2016). Next-generation connexin and pannexin cell biology. Trends Cell Biol. 26, 944–955. doi: 10.1016/j.tcb.2016.06.003
Facchinetti, F., Del Giudice, E., Furegato, S., Passarotto, M., and Leon, A. (2003). Cannabinoids ablate release of TNFα in rat microglial cells stimulated with lypopolysaccharide. Glia 41, 161–168. doi: 10.1002/glia.10177
Fernández-Trapero, M., Espejo-Porras, F., Rodríguez-Cueto, C., Coates, J. R., Pérez-Díaz, C., de Lago, E., et al. (2017). Upregulation of CB2 receptors in reactive astrocytes in canine degenerative myelopathy, a disease model of amyotrophic lateral sclerosis. Dis. Model. Mech. 10, 551–558. doi: 10.1242/dmm.028373
Fields, R. D., and Burnstock, G. (2006). Purinergic signalling in neuron-glia interactions. Nat. Rev. Neurosci. 7, 423–436. doi: 10.1038/nrn1928
Fiori, M. C., Figueroa, V., Zoghbi, M. E., Saéz, J. C., Reuss, L., and Altenberg, G. A. (2012). Permeation of calcium through purified connexin 26 hemichannels. J. Biol. Chem. 287, 40826–40834. doi: 10.1074/jbc.M112.383281
Froger, N., Orellana, J. A., Calvo, C. F., Amigou, E., Kozoriz, M. G., Naus, C. C., et al. (2010). Inhibition of cytokine-induced connexin43 hemichannel activity in astrocytes is neuroprotective. Mol. Cell. Neurosci. 45, 37–46. doi: 10.1016/j.mcn.2010.05.007
Froger, N., Orellana, J. A., Cohen-Salmon, M., Ezan, P., Amigou, E., Sáez, J. C., et al. (2009). Cannabinoids prevent the opposite regulation of astroglial connexin43 hemichannels and gap junction channels induced by pro-inflammatory treatments. J. Neurochem. 111, 1383–1397. doi: 10.1111/j.1471-4159.2009.06407.x
Gaete, P. S., Lillo, M. A., and Figueroa, X. F. (2014). Functional role of connexins and pannexins in the interaction between vascular and nervous system. J. Cell. Physiol. 229, 1336–1345. doi: 10.1002/jcp.24563
Gajardo-Gómez, R., Labra, V. C., Maturana, C. J., Shoji, K. F., Santibañez, C. A., Sáez, J. C., et al. (2017). Cannabinoids prevent the amyloid β-induced activation of astroglial hemichannels: a neuroprotective mechanism. Glia 65, 122–137. doi: 10.1002/glia.23080
Galiègue, S., Mary, S., Marchand, J., Dussossoy, D., Carrière, D., Carayon, P., et al. (1995). Expression of central and peripheral cannabinoid receptors in human immune tissues and leukocyte subpopulations. Eur. J. Biochem. 232, 54–61. doi: 10.1111/j.1432-1033.1995.tb20780.x
Gaoni, Y., and Mechoulam, R. (1964). Isolation, structure, and partial synthesis of an active constituent of hashish. J. Am. Chem. Soc. 86, 1646–1647. doi: 10.1021/ja01062a046
Garre, J. M., Yang, G., Bukauskas, F. F., and Bennett, M. V. (2016). FGF-1 triggers pannexin-1 hemichannel opening in spinal astrocytes of rodents and promotes inflammatory responses in acute spinal cord slices. J. Neurosci. 36, 4785–4801. doi: 10.1523/JNEUROSCI.4195-15.2016
Gerdeman, G. L., Ronesi, J., and Lovinger, D. M. (2002). Postsynaptic endocannabinoid release is critical to long-term depression in the striatum. Nat. Neurosci. 5, 446–451. doi: 10.1038/nn832
Giaume, C., Leybaert, L., Naus, C. C., and Sáez, J. C. (2013). Connexin and pannexin hemichannels in brain glial cells: properties, pharmacology, and roles. Front. Pharmacol. 4:88. doi: 10.3389/fphar.2013.00088
Giaume, C., Sáez, J. C., Song, W., Leybaert, L., and Naus, C. C. (2017). Connexins and pannexins in Alzheimer’s disease. Neurosci. Lett. doi: 10.1016/j.neulet.2017.09.006 [Epub ahead of print].
Gómez-Gonzalo, M., Navarrete, M., Perea, G., Covelo, A., Martín-Fernández, M., Shigemoto, R., et al. (2015). Endocannabinoids induce lateral long-term potentiation of transmitter release by stimulation of gliotransmission. Cereb. Cortex 25, 3699–3712. doi: 10.1093/cercor/bhu231
Gong, J. P., Onaivi, E. S., Ishiguro, H., Liu, Q. R., Tagliaferro, P. A., Brusco, A., et al. (2006). Cannabinoid CB2 receptors: immunohistochemical localization in rat brain. Brain Res. 1071, 10–23. doi: 10.1016/j.brainres.2005.11.035
Gundersen, V., Storm-Mathisen, J., and Bergersen, L. H. (2015). Neuroglial transmission. Physiol. Rev. 95, 695–726. doi: 10.1152/physrev.00024.2014
Guthrie, P. B., Knappenberger, J., Segal, M., Bennett, M. V., Charles, A. C., and Kater, S. B. (1999). ATP released from astrocytes mediates glial calcium waves. J. Neurosci. 19, 520–528.
Han, J., Kesner, P., Metna-Laurent, M., Duan, T., Xu, L., Georges, F., et al. (2012). Acute cannabinoids impair working memory through astroglial CB1 receptor modulation of hippocampal LTD. Cell 148, 1039–1050. doi: 10.1016/j.cell.2012.01.037
Hanus, L., Abu-Lafi, S., Fride, E., Breuer, A., Vogel, Z., Shalev, D. E., et al. (2001). 2-arachidonyl glyceryl ether, an endogenous agonist of the cannabinoid CB1 receptor. Proc. Natl. Acad. Sci. U S A 98, 3662–3665. doi: 10.1073/pnas.061029898
Henneberger, C., Papouin, T., Oliet, S. H., and Rusakov, D. A. (2010). Long-term potentiation depends on release of D-serine from astrocytes. Nature 463, 232–236. doi: 10.1038/nature08673
Iadecola, C. (2017). The neurovascular unit coming of age: a journey through neurovascular coupling in health and disease. Neuron 96, 17–42. doi: 10.1016/j.neuron.2017.07.030
Iglesias, R., Locovei, S., Roque, A., Alberto, A. P., Dahl, G., Spray, D. C., et al. (2008). P2X7 receptor-Pannexin1 complex: pharmacology and signaling. Am. J. Physiol. Cell Physiol. 295, C752–C760. doi: 10.1152/ajpcell.00228.2008
Iyyathurai, J., D’Hondt, C., Wang, N., De Bock, M., Himpens, B., Retamal, M. A., et al. (2013). Peptides and peptide-derived molecules targeting the intracellular domains of Cx43: gap junctions versus hemichannels. Neuropharmacology 75, 491–505. doi: 10.1016/j.neuropharm.2013.04.050
Kang, J., Kang, N., Lovatt, D., Torres, A., Zhao, Z., Lin, J., et al. (2008). Connexin 43 hemichannels are permeable to ATP. J. Neurosci. 28, 4702–4711. doi: 10.1523/JNEUROSCI.5048-07.2008
Kang, N., Peng, H., Yu, Y., Stanton, P. K., Guilarte, T. R., and Kang, J. (2013). Astrocytes release D-serine by a large vesicle. Neuroscience 240, 243–257. doi: 10.1016/j.neuroscience.2013.02.029
Karagiannis, A., Sylantyev, S., Hadjihambi, A., Hosford, P. S., Kasparov, S., and Gourine, A. V. (2016). Hemichannel-mediated release of lactate. J. Cereb. Blood Flow Metab. 36, 1202–1211. doi: 10.1177/0271678X15611912
Karpuk, N., Burkovetskaya, M., Fritz, T., Angle, A., and Kielian, T. (2011). Neuroinflammation leads to region-dependent alterations in astrocyte gap junction communication and hemichannel activity. J. Neurosci. 31, 414–425. doi: 10.1523/JNEUROSCI.5247-10.2011
Kawamura, M., Ruskin, D. N., and Masino, S. A. (2010). Metabolic autocrine regulation of neurons involves cooperation among pannexin hemichannels, adenosine receptors and KATP channels. J. Neurosci. 30, 3886–3895. doi: 10.1523/JNEUROSCI.0055-10.2010
Kendall, D. A., and Yudowski, G. A. (2016). Cannabinoid receptors in the central nervous system: their signaling and roles in disease. Front. Cell. Neurosci. 10:294. doi: 10.3389/fncel.2016.00294
Kettenmann, H., Hanisch, U. K., Noda, M., and Verkhratsky, A. (2011). Physiology of microglia. Physiol. Rev. 91, 461–553. doi: 10.1152/physrev.00011.2010
Kim, Y., Davidson, J. O., Gunn, K. C., Phillips, A. R., Green, C. R., and Gunn, A. J. (2016). Role of hemichannels in CNS inflammation and the inflammasome pathway. Adv. Protein Chem. Struct. Biol. 104, 1–37. doi: 10.1016/bs.apcsb.2015.12.001
Klegeris, A., Bissonnette, C. J., and McGeer, P. L. (2003). Reduction of human monocytic cell neurotoxicity and cytokine secretion by ligands of the cannabinoid-type CB2 receptor. Br. J. Pharmacol. 139, 775–786. doi: 10.1038/sj.bjp.0705304
Koulakoff, A., Mei, X., Orellana, J. A., Sáez, J. C., and Giaume, C. (2012). Glial connexin expression and function in the context of Alzheimer’s disease. Biochim. Biophys. Acta 1818, 2048–2057. doi: 10.1016/j.bbamem.2011.10.001
Kreft, M., Potokar, M., Stenovec, M., Pangrsic, T., and Zorec, R. (2009). Regulated exocytosis and vesicle trafficking in astrocytes. Ann. N Y Acad. Sci. 1152, 30–42. doi: 10.1111/j.1749-6632.2008.04005.x0
Kreitzer, A. C., and Regehr, W. G. (2001). Retrograde inhibition of presynaptic calcium influx by endogenous cannabinoids at excitatory synapses onto Purkinje cells. Neuron 29, 717–727. doi: 10.1016/s0896-6273(01)00246-x
Kreitzer, A. C., and Regehr, W. G. (2002). Retrograde signaling by endocannabinoids. Curr. Opin. Neurobiol. 12, 324–330. doi: 10.1016/s0959-4388(02)00328-8
Li, Q., and Barres, B. A. (2017). Microglia and macrophages in brain homeostasis and disease. Nat. Rev. Immunol. doi: 10.1038/nri.2017.125 [Epub ahead of print].
Liebmann, M., Stahr, A., Guenther, M., Witte, O. W., and Frahm, C. (2013). Astrocytic Cx43 and Cx30 differentially modulate adult neurogenesis in mice. Neurosci. Lett. 545, 40–45. doi: 10.1016/j.neulet.2013.04.013
Locovei, S., Scemes, E., Qiu, F., Spray, D. C., and Dahl, G. (2007). Pannexin1 is part of the pore forming unit of the P2X7 receptor death complex. FEBS Lett. 581, 483–488. doi: 10.1016/j.febslet.2006.12.056
Locovei, S., Wang, J., and Dahl, G. (2006). Activation of pannexin 1 channels by ATP through P2Y receptors and by cytoplasmic calcium. FEBS Lett. 580, 239–244. doi: 10.1016/j.febslet.2005.12.004
Lou, Z. Y., Zhao, C. B., and Xiao, B. G. (2012). Immunoregulation of experimental autoimmune encephalomyelitis by the selective CB1 receptor antagonist. J. Neurosci. Res. 90, 84–95. doi: 10.1002/jnr.22721
Lu, H. C., and Mackie, K. (2016). An introduction to the endogenous cannabinoid system. Biol. Psychiatry 79, 516–525. doi: 10.1016/j.biopsych.2015.07.028
Luchicchi, A., and Pistis, M. (2012). Anandamide and 2-arachidonoylglycerol: pharmacological properties, functional features and emerging specificities of the two major endocannabinoids. Mol. Neurobiol. 46, 374–392. doi: 10.1007/s12035-012-8299-0
Lyman, W. D., Sonett, J. R., Brosnan, C. F., Elkin, R., and Bornstein, M. B. (1989). Delta 9-tetrahydrocannabinol: a novel treatment for experimental autoimmune encephalomyelitis. J. Neuroimmunol. 23, 73–81. doi: 10.1016/0165-5728(89)90075-1
Mahvan, T. D., Hilaire, M. L., Mann, A., Brown, A., Linn, B., Gardner, T., et al. (2017). Marijuana use in the elderly: implications and considerations. Consult. Pharm. 32, 341–351. doi: 10.4140/TCP.n.2017.341
Malik, S., and Eugenin, E. A. (2017). Role of connexin and pannexin containing channels in HIV infection and NeuroAIDS. Neurosci. Lett. doi: 10.1016/j.neulet.2017.09.005 [Epub ahead of print].
Marchalant, Y., Rosi, S., and Wenk, G. L. (2007). Anti-inflammatory property of the cannabinoid agonist WIN-55212–2 in a rodent model of chronic brain inflammation. Neuroscience 144, 1516–1522. doi: 10.1016/j.neuroscience.2006.11.016
Marrs, W. R., Blankman, J. L., Horne, E. A., Thomazeau, A., Lin, Y. H., Coy, J., et al. (2010). The serine hydrolase ABHD6 controls the accumulation and efficacy of 2-AG at cannabinoid receptors. Nat. Neurosci. 13, 951–957. doi: 10.1038/nn.2601
Marsicano, G., Wotjak, C. T., Azad, S. C., Bisogno, T., Rammes, G., Cascio, M. G., et al. (2002). The endogenous cannabinoid system controls extinction of aversive memories. Nature 418, 530–534. doi: 10.1038/nature00839
Martineau, M., Galli, T., Baux, G., and Mothet, J. P. (2008). Confocal imaging and tracking of the exocytotic routes for D-serine-mediated gliotransmission. Glia 56, 1271–1284. doi: 10.1002/glia.20696
Martineau, M., Shi, T., Puyal, J., Knolhoff, A. M., Dulong, J., Gasnier, B., et al. (2013). Storage and uptake of D-serine into astrocytic synaptic-like vesicles specify gliotransmission. J. Neurosci. 33, 3413–3423. doi: 10.1523/JNEUROSCI.3497-12.2013
Martín-Moreno, A. M., Brera, B., Spuch, C., Carro, E., García-García, L., Delgado, M., et al. (2012). Prolonged oral cannabinoid administration prevents neuroinflammation, lowers β-amyloid levels and improves cognitive performance in Tg APP 2576 mice. J. Neuroinflammation 9:8. doi: 10.1186/1742-2094-9-8
Matsuda, L. A., Lolait, S. J., Brownstein, M. J., Young, A. C., and Bonner, T. I. (1990). Structure of a cannabinoid receptor and functional expression of the cloned cDNA. Nature 346, 561–564. doi: 10.1038/346561a0
Mecha, M., Feliu, A., Carrillo-Salinas, F. J., Rueda-Zubiaurre, A., Ortega-Gutiérrez, S., de Sola, R. G., et al. (2015). Endocannabinoids drive the acquisition of an alternative phenotype in microglia. Brain Behav. Immun. 49, 233–245. doi: 10.1016/j.bbi.2015.06.002
Même, W., Calvo, C. F., Froger, N., Ezan, P., Amigou, E., Koulakoff, A., et al. (2006). Proinflammatory cytokines released from microglia inhibit gap junctions in astrocytes: potentiation by β-amyloid. FASEB J. 20, 494–496. doi: 10.1096/fj.05-4297fje
Metna-Laurent, M., and Marsicano, G. (2015). Rising stars: modulation of brain functions by astroglial type-1 cannabinoid receptors. Glia 63, 353–364. doi: 10.1002/glia.22773
Meunier, C., Wang, N., Yi, C., Dallerac, G., Ezan, P., Koulakoff, A., et al. (2017). Contribution of astroglial Cx43 hemichannels to the modulation of glutamatergic currents by D-serine in the mouse prefrontal cortex. J. Neurosci. 37, 9064–9075. doi: 10.1523/JNEUROSCI.2204-16.2017
Min, R., and Nevian, T. (2012). Astrocyte signaling controls spike timing-dependent depression at neocortical synapses. Nat. Neurosci. 15, 746–753. doi: 10.1038/nn.3075
Moldrich, G., and Wenger, T. (2000). Localization of the CB1 cannabinoid receptor in the rat brain. An immunohistochemical study. Peptides 21, 1735–1742. doi: 10.1016/s0196-9781(00)00324-7
Molina-Holgado, F., Molina-Holgado, E., Guaza, C., and Rothwell, N. J. (2002). Role of CB1 and CB2 receptors in the inhibitory effects of cannabinoids on lipopolysaccharide-induced nitric oxide release in astrocyte cultures. J. Neurosci. Res. 67, 829–836. doi: 10.1002/jnr.10165
Molina-Holgado, F., Pinteaux, E., Moore, J. D., Molina-Holgado, E., Guaza, C., Gibson, R. M., et al. (2003). Endogenous interleukin-1 receptor antagonist mediates anti-inflammatory and neuroprotective actions of cannabinoids in neurons and glia. J. Neurosci. 23, 6470–6474.
Molina-Holgado, E., Vela, J. M., Arévalo-Martín, A., Almazán, G., Molina-Holgado, F., Borrell, J., et al. (2002). Cannabinoids promote oligodendrocyte progenitor survival: involvement of cannabinoid receptors and phosphatidylinositol-3 kinase/Akt signaling. J. Neurosci. 22, 9742–9753.
Montero, T. D., and Orellana, J. A. (2015). Hemichannels: new pathways for gliotransmitter release. Neuroscience 286, 45–59. doi: 10.1016/j.neuroscience.2014.11.048
Moore, K. B., and O’Brien, J. (2015). Connexins in neurons and glia: targets for intervention in disease and injury. Neural Regen. Res. 10, 1013–1017. doi: 10.4103/1673-5374.160092
Munro, S., Thomas, K. L., and Abu-Shaar, M. (1993). Molecular characterization of a peripheral receptor for cannabinoids. Nature 365, 61–65. doi: 10.1038/365061a0
Navarrete, M., and Araque, A. (2008). Endocannabinoids mediate neuron-astrocyte communication. Neuron 57, 883–893. doi: 10.1016/j.neuron.2008.01.029
Navarrete, M., and Araque, A. (2010). Endocannabinoids potentiate synaptic transmission through stimulation of astrocytes. Neuron 68, 113–126. doi: 10.1016/j.neuron.2010.08.043
Navarrete, M., Perea, G., Fernandez de Sevilla, D., Gómez-Gonzalo, M., Núñez, A., Martín, E. D., et al. (2012). Astrocytes mediate in vivo cholinergic-induced synaptic plasticity. PLoS Biol. 10:e1001259. doi: 10.1371/journal.pbio.1001259
Navarro, G., Borroto-Escuela, D., Angelats, E., Etayo, I., Reyes-Resina, I., Pulido-Salgado, M., et al. (2018). Receptor-heteromer mediated regulation of endocannabinoid signaling in activated microglia. Role of CB1 and CB2 receptors and relevance for Alzheimer’s disease and levodopa-induced dyskinesia. Brain Behav. Immun. 67, 139–151. doi: 10.1016/j.bbi.2017.08.015
Nimmrich, V., and Gross, G. (2012). P/Q-type calcium channel modulators. Br. J. Pharmacol. 167, 741–759. doi: 10.1111/j.1476-5381.2012.02069.x
Núñez, E., Benito, C., Pazos, M. R., Barbachano, A., Fajardo, O., González, S., et al. (2004). Cannabinoid CB2 receptors are expressed by perivascular microglial cells in the human brain: an immunohistochemical study. Synapse 53, 208–213. doi: 10.1002/syn.20050
Ohno-Shosaku, T., Maejima, T., and Kano, M. (2001). Endogenous cannabinoids mediate retrograde signals from depolarized postsynaptic neurons to presynaptic terminals. Neuron 29, 729–738. doi: 10.1016/s0896-6273(01)00247-1
Oliveira da Cruz, J. F., Robin, L. M., Drago, F., Marsicano, G., and Metna-Laurent, M. (2016). Astroglial type-1 cannabinoid receptor (CB1): a new player in the tripartite synapse. Neuroscience 323, 35–42. doi: 10.1016/j.neuroscience.2015.05.002
Onaivi, E. S. (2011). Commentary: functional neuronal CB2 cannabinoid receptors in the CNS. Curr. Neuropharmacol. 9, 205–208. doi: 10.2174/157015911795017416
Onaivi, E. S., Ishiguro, H., Gu, S., and Liu, Q. R. (2012). CNS effects of CB2 cannabinoid receptors: beyond neuro-immuno-cannabinoid activity. J. Psychopharmacol. 26, 92–103. doi: 10.1177/0269881111400652
Orellana, J. A., Figueroa, X. F., Sánchez, H. A., Contreras-Duarte, S., Velarde, V., and Sáez, J. C. (2011a). Hemichannels in the neurovascular unit and white matter under normal and inflamed conditions. CNS Neurol. Disord. Drug Targets 10, 404–414. doi: 10.2174/187152711794653869
Orellana, J. A., Froger, N., Ezan, P., Jiang, J. X., Bennett, M. V., Naus, C. C., et al. (2011b). ATP and glutamate released via astroglial connexin 43 hemichannels mediate neuronal death through activation of pannexin 1 hemichannels. J. Neurochem. 118, 826–840. doi: 10.1111/j.1471-4159.2011.07210.x
Orellana, J. A., Moraga-Amaro, R., Diaz-Galarce, R., Rojas, S., Maturana, C. J., Stehberg, J., et al. (2015). Restraint stress increases hemichannel activity in hippocampal glial cells and neurons. Front. Cell. Neurosci. 9:102. doi: 10.3389/fncel.2015.00102
Orellana, J. A., Retamal, M. A., Moraga-Amaro, R., and Stehberg, J. (2016). Role of astroglial hemichannels and pannexons in memory and neurodegenerative diseases. Front. Integr. Neurosci. 10:26. doi: 10.3389/fnint.2016.00026
Orellana, J. A., Sáez, J. C., Bennett, M. V., Berman, J. W., Morgello, S., and Eugenin, E. A. (2014). HIV increases the release of dickkopf-1 protein from human astrocytes by a Cx43 hemichannel-dependent mechanism. J. Neurochem. 128, 752–763. doi: 10.1111/jnc.12492
Orellana, J. A., Sáez, P. J., Cortés-Campos, C., Elizondo, R. J., Shoji, K. F., Contreras-Duarte, S., et al. (2012a). Glucose increases intracellular free Ca2+ in tanycytes via ATP released through connexin 43 hemichannels. Glia 60, 53–68. doi: 10.1002/glia.21246
Orellana, J. A., Sánchez, H. A., Schalper, K. A., Figueroa, V., and Sáez, J. C. (2012b). Regulation of intercellular calcium signaling through calcium interactions with connexin-based channels. Adv. Exp. Med. Biol. 740, 777–794. doi: 10.1007/978-94-007-2888-2_34
Orellana, J. A., von Bernhardi, R., Giaume, C., and Sáez, J. C. (2012c). Glial hemichannels and their involvement in aging and neurodegenerative diseases. Rev. Neurosci. 23, 163–177. doi: 10.1515/revneuro-2011-0065
Orellana, J. A., Sáez, P. J., Shoji, K. F., Schalper, K. A., Palacios-Prado, N., Velarde, V., et al. (2009). Modulation of brain hemichannels and gap junction channels by pro-inflammatory agents and their possible role in neurodegeneration. Antioxid. Redox Signal. 11, 369–399. doi: 10.1089/ars.2008.2130
Palazuelos, J., Aguado, T., Pazos, M. R., Julien, B., Carrasco, C., Resel, E., et al. (2009). Microglial CB2 cannabinoid receptors are neuroprotective in Huntington’s disease excitotoxicity. Brain 132, 3152–3164. doi: 10.1093/brain/awp239
Pekny, M., and Pekna, M. (2014). Astrocyte reactivity and reactive astrogliosis: costs and benefits. Physiol. Rev. 94, 1077–1098. doi: 10.1152/physrev.00041.2013
Pelegrin, P., and Surprenant, A. (2006). Pannexin-1 mediates large pore formation and interleukin-1β release by the ATP-gated P2X7 receptor. EMBO J. 25, 5071–5082. doi: 10.1038/sj.emboj.7601378
Pertwee, R. G. (2010). Receptors and channels targeted by synthetic cannabinoid receptor agonists and antagonists. Curr. Med. Chem. 17, 1360–1381. doi: 10.2174/092986710790980050
Pertwee, R. G. (2012). Targeting the endocannabinoid system with cannabinoid receptor agonists: pharmacological strategies and therapeutic possibilities. Philos. Trans. R. Soc. Lond. B Biol. Sci. 367, 3353–3363. doi: 10.1098/rstb.2011.0381
Pertwee, R. G. (2015). Endocannabinoids and their pharmacological actions. Handb. Exp. Pharmacol. 231, 1–37. doi: 10.1007/978-3-319-20825-1_1
Piomelli, D. (2003). The molecular logic of endocannabinoid signalling. Nat. Rev. Neurosci. 4, 873–884. doi: 10.1038/nrn1247
Porter, A. C., Sauer, J. M., Knierman, M. D., Becker, G. W., Berna, M. J., Bao, J., et al. (2002). Characterization of a novel endocannabinoid, virodhamine, with antagonist activity at the CB1 receptor. J. Pharmacol. Exp. Ther. 301, 1020–1024. doi: 10.1124/jpet.301.3.1020
Prenderville, J. A., Kelly, A. M., and Downer, E. J. (2015). The role of cannabinoids in adult neurogenesis. Br. J. Pharmacol. 172, 3950–3963. doi: 10.1111/bph.13186
Prochnow, N., Abdulazim, A., Kurtenbach, S., Wildförster, V., Dvoriantchikova, G., Hanske, J., et al. (2012). Pannexin1 stabilizes synaptic plasticity and is needed for learning. PLoS One 7:e51767. doi: 10.1371/journal.pone.0051767
Puffenbarger, R. A., Boothe, A. C., and Cabral, G. A. (2000). Cannabinoids inhibit LPS-inducible cytokine mRNA expression in rat microglial cells. Glia 29, 58–69. doi: 10.1002/(sici)1098-1136(20000101)29:1<58::aid-glia6>3.3.co;2-n
Qiu, F., and Dahl, G. (2009). A permeant regulating its permeation pore: inhibition of pannexin 1 channels by ATP. Am. J. Physiol. Cell Physiol. 296, C250–C255. doi: 10.1152/ajpcell.00433.2008
Ramírez, B. G., Blázquez, C., Gómez Del Pulgar, T., Guzmán, M., and de Ceballos, M. L. (2005). Prevention of Alzheimer’s disease pathology by cannabinoids: neuroprotection mediated by blockade of microglial activation. J. Neurosci. 25, 1904–1913. doi: 10.1523/JNEUROSCI.4540-04.2005
Rana, S., and Dringen, R. (2007). Gap junction hemichannel-mediated release of glutathione from cultured rat astrocytes. Neurosci. Lett. 415, 45–48. doi: 10.1016/j.neulet.2006.12.043
Rasooli-Nejad, S., Palygin, O., Lalo, U., and Pankratov, Y. (2014). Cannabinoid receptors contribute to astroglial Ca2+-signalling and control of synaptic plasticity in the neocortex. Philos. Trans. R. Soc. Lond. B Biol. Sci. 369:20140077. doi: 10.1098/rstb.2014.0077
Retamal, M. A. (2014). Connexin and Pannexin hemichannels are regulated by redox potential. Front. Physiol. 5:80. doi: 10.3389/fphys.2014.00080
Retamal, M. A., Cortés, C. J., Reuss, L., Bennett, M. V., and Sáez, J. C. (2006). S-nitrosylation and permeation through connexin 43 hemichannels in astrocytes: induction by oxidant stress and reversal by reducing agents. Proc. Natl. Acad. Sci. U S A 103, 4475–4480. doi: 10.1073/pnas.0511118103
Retamal, M. A., Froger, N., Palacios-Prado, N., Ezan, P., Sáez, P. J., Sáez, J. C., et al. (2007). Cx43 hemichannels and gap junction channels in astrocytes are regulated oppositely by proinflammatory cytokines released from activated microglia. J. Neurosci. 27, 13781–13792. doi: 10.1523/JNEUROSCI.2042-07.2007
Robel, S., and Sontheimer, H. (2016). Glia as drivers of abnormal neuronal activity. Nat. Neurosci. 19, 28–33. doi: 10.1038/nn.4184
Rom, S., and Persidsky, Y. (2013). Cannabinoid receptor 2: potential role in immunomodulation and neuroinflammation. J. Neuroimmune Pharmacol. 8, 608–620. doi: 10.1007/s11481-013-9445-9
Roux, L., Madar, A., Lacroix, M. M., Yi, C., Benchenane, K., and Giaume, C. (2015). Astroglial connexin 43 hemichannels modulate olfactory bulb slow oscillations. J. Neurosci. 35, 15339–15352. doi: 10.1523/JNEUROSCI.0861-15.2015
Rovegno, M., and Sáez, J. C. (2018). Role of astrocyte connexin hemichannels in cortical spreading depression. Biochim. Biophys. Acta 1860, 216–223. doi: 10.1016/j.bbamem.2017.08.014
Rovegno, M., Soto, P. A., Sáez, P. J., Naus, C. C., Sáez, J. C., and von Bernhardi, R. (2015). Connexin43 hemichannels mediate secondary cellular damage spread from the trauma zone to distal zones in astrocyte monolayers. Glia 63, 1185–1199. doi: 10.1002/glia.22808
Rozental, R., Morales, M., Mehler, M. F., Urban, M., Kremer, M., Dermietzel, R., et al. (1998). Changes in the properties of gap junctions during neuronal differentiation of hippocampal progenitor cells. J. Neurosci. 18, 1753–1762.
Sáez, J. C., Berthoud, V. M., Branes, M. C., Martinez, A. D., and Beyer, E. C. (2003). Plasma membrane channels formed by connexins: their regulation and functions. Physiol. Rev. 83, 1359–1400. doi: 10.1152/physrev.00007.2003
Sáez, J. C., Schalper, K. A., Retamal, M. A., Orellana, J. A., Shoji, K. F., and Bennett, M. V. (2010). Cell membrane permeabilization via connexin hemichannels in living and dying cells. Exp. Cell Res. 316, 2377–2389. doi: 10.1016/j.yexcr.2010.05.026
Sagan, S., Venance, L., Torrens, Y., Cordier, J., Glowinski, J., and Giaume, C. (1999). Anandamide and WIN 55212–2 inhibit cyclic AMP formation through G-protein-coupled receptors distinct from CB1 cannabinoid receptors in cultured astrocytes. Eur. J. Neurosci. 11, 691–699. doi: 10.1046/j.1460-9568.1999.00480.x
Sagredo, O., González, S., Aroyo, I., Pazos, M. R., Benito, C., Lastres-Becker, I., et al. (2009). Cannabinoid CB2 receptor agonists protect the striatum against malonate toxicity: relevance for Huntington’s disease. Glia 57, 1154–1167. doi: 10.1002/glia.20838
Salmina, A. B., Morgun, A. V., Kuvacheva, N. V., Lopatina, O. L., Komleva, Y. K., Malinovskaya, N. A., et al. (2014). Establishment of neurogenic microenvironment in the neurovascular unit: the connexin 43 story. Rev. Neurosci. 25, 97–111. doi: 10.1515/revneuro-2013-0044
Sánchez, C., Galve-Roperh, I., Rueda, D., and Guzmán, M. (1998). Involvement of sphingomyelin hydrolysis and the mitogen-activated protein kinase cascade in the Delta9-tetrahydrocannabinol-induced stimulation of glucose metabolism in primary astrocytes. Mol. Pharmacol. 54, 834–843. doi: 10.1124/mol.54.5.834
Sánchez, H. A., Orellana, J. A., Verselis, V. K., and Sáez, J. C. (2009). Metabolic inhibition increases activity of connexin-32 hemichannels permeable to Ca2+ in transfected HeLa cells. Am. J. Physiol. Cell Physiol. 297, C665–C678. doi: 10.1152/ajpcell.00200.2009
Sarrouilhe, D., Dejean, C., and Mesnil, M. (2017). Connexin43- and pannexin-based channels in neuroinflammation and cerebral neuropathies. Front. Mol. Neurosci. 10:320. doi: 10.3389/fnmol.2017.00320
Saura, J. (2007). Microglial cells in astroglial cultures: a cautionary note. J. Neuroinflammation 4:26. doi: 10.1186/1742-2094-4-26
Scemes, E., and Giaume, C. (2006). Astrocyte calcium waves: what they are and what they do. Glia 54, 716–725. doi: 10.1002/glia.20374
Schalper, K. A., Sánchez, H. A., Lee, S. C., Altenberg, G. A., Nathanson, M. H., and Sáez, J. C. (2010). Connexin 43 hemichannels mediate the Ca2+ influx induced by extracellular alkalinization. Am. J. Physiol. Cell Physiol. 299, C1504–C1515. doi: 10.1152/ajpcell.00015.2010
Shen, W., Nikolic, L., Meunier, C., Pfrieger, F., and Audinat, E. (2017). An autocrine purinergic signaling controls astrocyte-induced neuronal excitation. Sci. Rep. 7:11280. doi: 10.1038/s41598-017-11793-x
Sheng, W. S., Hu, S., Min, X., Cabral, G. A., Lokensgard, J. R., and Peterson, P. K. (2005). Synthetic cannabinoid WIN55,212–2 inhibits generation of inflammatory mediators by IL-1β-stimulated human astrocytes. Glia 49, 211–219. doi: 10.1002/glia.20108
Sheng, W. S., Hu, S., Ni, H. T., Rock, R. B., and Peterson, P. K. (2009). WIN55,212–2 inhibits production of CX3CL1 by human astrocytes: involvement of p38 MAP kinase. J. Neuroimmune Pharmacol. 4, 244–248. doi: 10.1007/s11481-009-9147-5
Shestopalov, V. I., and Slepak, V. Z. (2014). Molecular pathways of pannexin1-mediated neurotoxicity. Front. Physiol. 5:23. doi: 10.3389/fphys.2014.00023
Shih, R. H., Wang, C. Y., and Yang, C. M. (2015). NF-κB signaling pathways in neurological inflammation: a mini review. Front. Mol. Neurosci. 8:77. doi: 10.3389/fnmol.2015.00077
Sigel, E., Baur, R., Racz, I., Marazzi, J., Smart, T. G., Zimmer, A., et al. (2011). The major central endocannabinoid directly acts at GABAA receptors. Proc. Natl. Acad. Sci. U S A 108, 18150–18155. doi: 10.1073/pnas.1113444108
Silverman, W. R., de Rivero Vaccari, J. P., Locovei, S., Qiu, F., Carlsson, S. K., Scemes, E., et al. (2009). The pannexin 1 channel activates the inflammasome in neurons and astrocytes. J. Biol. Chem. 284, 18143–18151. doi: 10.1074/jbc.M109.004804
Simard, M., Arcuino, G., Takano, T., Liu, Q. S., and Nedergaard, M. (2003). Signaling at the gliovascular interface. J. Neurosci. 23, 9254–9262.
Söhl, G., Güldenagel, M., Beck, H., Teubner, B., Traub, O., Gutiérrez, R., et al. (2000). Expression of connexin genes in hippocampus of kainate-treated and kindled rats under conditions of experimental epilepsy. Brain Res. Mol. Brain Res. 83, 44–51. doi: 10.1016/s0169-328x(00)00195-9
Sorge, R. E., Trang, T., Dorfman, R., Smith, S. B., Beggs, S., Ritchie, J., et al. (2012). Genetically determined P2X7 receptor pore formation regulates variability in chronic pain sensitivity. Nat. Med. 18, 595–599. doi: 10.1038/nm.2710
Stansley, B., Post, J., and Hensley, K. (2012). A comparative review of cell culture systems for the study of microglial biology in Alzheimer’s disease. J. Neuroinflammation 9:115. doi: 10.1186/1742-2094-9-115
Stehberg, J., Moraga-Amaro, R., Salazar, C., Becerra, A., Echeverria, C., Orellana, J. A., et al. (2012). Release of gliotransmitters through astroglial connexin 43 hemichannels is necessary for fear memory consolidation in the basolateral amygdala. FASEB J. 26, 3649–3657. doi: 10.1096/fj.11-198416
Stella, N. (2010). Cannabinoid and cannabinoid-like receptors in microglia, astrocytes, and astrocytomas. Glia 58, 1017–1030. doi: 10.1002/glia.20983
Stempel, A. V., Stumpf, A., Zhang, H. Y., Özdoğan, T., Pannasch, U., Theis, A. K., et al. (2016). Cannabinoid type 2 receptors mediate a cell type-specific plasticity in the hippocampus. Neuron 90, 795–809. doi: 10.1016/j.neuron.2016.03.034
Stout, C. E., Costantin, J. L., Naus, C. C., and Charles, A. C. (2002). Intercellular calcium signaling in astrocytes via ATP release through connexin hemichannels. J. Biol. Chem. 277, 10482–10488. doi: 10.1074/jbc.M109902200
Suadicani, S. O., Iglesias, R., Wang, J., Dahl, G., Spray, D. C., and Scemes, E. (2012). ATP signaling is deficient in cultured Pannexin1-null mouse astrocytes. Glia 60, 1106–1116. doi: 10.1002/glia.22338
Sugiura, T., Kondo, S., Sukagawa, A., Nakane, S., Shinoda, A., Itoh, K., et al. (1995). 2-Arachidonoylglycerol: a possible endogenous cannabinoid receptor ligand in brain. Biochem. Biophys. Res. Commun. 215, 89–97. doi: 10.1002/biof.18
Swayne, L. A., and Bennett, S. A. (2016). Connexins and pannexins in neuronal development and adult neurogenesis. BMC Cell Biol. 71:S10. doi: 10.1186/s12860-016-0089-5
Swayne, L. A., Sorbara, C. D., and Bennett, S. A. (2010). Pannexin 2 is expressed by postnatal hippocampal neural progenitors and modulates neuronal commitment. J. Biol. Chem. 285, 24977–24986. doi: 10.1074/jbc.M110.130054
Takeuchi, H., Jin, S., Wang, J., Zhang, G., Kawanokuchi, J., Kuno, R., et al. (2006). Tumor necrosis factor-α induces neurotoxicity via glutamate release from hemichannels of activated microglia in an autocrine manner. J. Biol. Chem. 281, 21362–21368. doi: 10.1074/jbc.M600504200
Thompson, R. J. (2015). Pannexin channels and ischaemia. J. Physiol. 593, 3463–3470. doi: 10.1113/jphysiol.2014.282426
Torres, A., Wang, F., Xu, Q., Fujita, T., Dobrowolski, R., Willecke, K., et al. (2012). Extracellular Ca2+ acts as a mediator of communication from neurons to glia. Sci. Signal. 5:ra8. doi: 10.1126/scisignal.2002160
Turcotte, C., Chouinard, F., Lefebvre, J. S., and Flamand, N. (2015). Regulation of inflammation by cannabinoids, the endocannabinoids 2-arachidonoyl-glycerol and arachidonoyl-ethanolamide and their metabolites. J. Leukoc. Biol. 97, 1049–1070. doi: 10.1189/jlb.3RU0115-021R
Vázquez, C., Tolón, R. M., Pazos, M. R., Moreno, M., Koester, E. C., Cravatt, B. F., et al. (2015). Endocannabinoids regulate the activity of astrocytic hemichannels and the microglial response against an injury: in vivo studies. Neurobiol. Dis. 79, 41–50. doi: 10.1016/j.nbd.2015.04.005
Waksman, Y., Olson, J. M., Carlisle, S. J., and Cabral, G. A. (1999). The central cannabinoid receptor (CB1) mediates inhibition of nitric oxide production by rat microglial cells. J. Pharmacol. Exp. Ther. 288, 1357–1366.
Walrave, L., Vinken, M., Albertini, G., De Bundel, D., Leybaert, L., and Smolders, I. J. (2016). Inhibition of connexin43 hemichannels impairs spatial short-term memory without affecting spatial working memory. Front. Cell. Neurosci. 10:288. doi: 10.3389/fncel.2016.00288
Walter, L., Franklin, A., Witting, A., Wade, C., Xie, Y., Kunos, G., et al. (2003). Nonpsychotropic cannabinoid receptors regulate microglial cell migration. J. Neurosci. 23, 1398–1405.
Walter, L., and Stella, N. (2003). Endothelin-1 increases 2-arachidonoyl glycerol (2-AG) production in astrocytes. Glia 44, 85–90. doi: 10.1002/glia.10270
Wang, N., De Bock, M., Decrock, E., Bol, M., Gadicherla, A., Vinken, M., et al. (2013a). Paracrine signaling through plasma membrane hemichannels. Biochim. Biophys. Acta 1828, 35–50. doi: 10.1016/j.bbamem.2012.07.002
Wang, N., De Vuyst, E., Ponsaerts, R., Boengler, K., Palacios-Prado, N., Wauman, J., et al. (2013b). Selective inhibition of Cx43 hemichannels by Gap19 and its impact on myocardial ischemia/reperfusion injury. Basic Res. Cardiol 108:309. doi: 10.1007/s00395-012-0309-x
Wang, X., Yang, L., Yang, L., Xing, F., Yang, H., Qin, L., et al. (2017). Gypenoside IX suppresses p38 MAPK/Akt/NFκB signaling pathway activation and inflammatory responses in astrocytes stimulated by proinflammatory mediators. Inflammation 40, 2137–2150. doi: 10.1007/s10753-017-0654-x
Wei, H., Deng, F., Chen, Y., Qin, Y., Hao, Y., and Guo, X. (2014). Ultrafine carbon black induces glutamate and ATP release by activating connexin and pannexin hemichannels in cultured astrocytes. Toxicology 323, 32–41. doi: 10.1016/j.tox.2014.06.005
Weilinger, N. L., Tang, P. L., and Thompson, R. J. (2012). Anoxia-induced NMDA receptor activation opens pannexin channels via Src family kinases. J. Neurosci. 32, 12579–12588. doi: 10.1523/JNEUROSCI.1267-12.2012
Willebrords, J., Maes, M., Crespo Yanguas, S., and Vinken, M. (2017). Inhibitors of connexin and pannexin channels as potential therapeutics. Pharmacol. Ther. 180, 144–160. doi: 10.1016/j.pharmthera.2017.07.001
Wilson, R. I., and Nicoll, R. A. (2001). Endogenous cannabinoids mediate retrograde signalling at hippocampal synapses. Nature 410, 588–592. doi: 10.1038/35069076
Yassin, M., Haluza-Delay, R., Kadiri, M., Ouahrani, A. E., Mesa, J. M., and Merzouki, A. (2017). Cannabis cultivation within a religious context: a case study of Ghomara in the Rif Mountain (Northern Morocco). J. Ethn. Subst. Abuse 4, 1–22. doi: 10.1080/15332640.2017.1300972
Ye, Z. C., Wyeth, M. S., Baltan-Tekkok, S., and Ransom, B. R. (2003). Functional hemichannels in astrocytes: a novel mechanism of glutamate release. J. Neurosci. 23, 3588–3596.
Yi, C., Mei, X., Ezan, P., Mato, S., Matias, I., Giaume, C., et al. (2016). Astroglial connexin43 contributes to neuronal suffering in a mouse model of Alzheimer’s disease. Cell Death Differ. 23, 1691–1701. doi: 10.1038/cdd.2016.63
Zhang, Z., Chen, G., Zhou, W., Song, A., Xu, T., Luo, Q., et al. (2007). Regulated ATP release from astrocytes through lysosome exocytosis. Nat. Cell Biol. 9, 945–953. doi: 10.1038/ncb1620
Zhao, Z., Nelson, A. R., Betsholtz, C., and Zlokovic, B. V. (2015). Establishment and dysfunction of the blood-brain barrier. Cell 163, 1064–1078. doi: 10.1016/j.cell.2015.10.067
Zoidl, G., Petrasch-Parwez, E., Ray, A., Meier, C., Bunse, S., Habbes, H. W., et al. (2007). Localization of the pannexin1 protein at postsynaptic sites in the cerebral cortex and hippocampus. Neuroscience 146, 9–16. doi: 10.1016/j.neuroscience.2007.01.061
Zuardi, A. W. (2008). Cannabidiol: from an inactive cannabinoid to a drug with wide spectrum of action. Rev. Bras. Psiquiatr. 30, 271–280. doi: 10.1590/s1516-44462008000300015
Keywords: astrocytes, cannabis, neurons, connexins, pannexins, synaptic transmission, neuroinflammation
Citation: Labra VC, Santibáñez CA, Gajardo-Gómez R, Díaz EF, Gómez GI and Orellana JA (2018) The Neuroglial Dialog Between Cannabinoids and Hemichannels. Front. Mol. Neurosci. 11:79. doi: 10.3389/fnmol.2018.00079
Received: 14 November 2017; Accepted: 28 February 2018;
Published: 20 March 2018.
Edited by:
Ildikó Rácz, Universitätsklinikum Bonn, GermanyReviewed by:
Marie-Eve Tremblay, Laval University, CanadaBruno Pierre Guiard, Université de Toulouse, France
David Ruskin, Trinity College, United States
Copyright © 2018 Labra, Santibáñez, Gajardo-Gómez, Díaz, Gómez and Orellana. This is an open-access article distributed under the terms of the Creative Commons Attribution License (CC BY). The use, distribution or reproduction in other forums is permitted, provided the original author(s) and the copyright owner are credited and that the original publication in this journal is cited, in accordance with accepted academic practice. No use, distribution or reproduction is permitted which does not comply with these terms.
*Correspondence: Juan A. Orellana, amFvcmVsbGFAdWMuY2w=