- 1iBET - Instituto de Biologia Experimental e Tecnológica, Oeiras, Portugal
- 2CEDOC - Chronic Diseases Research Center, Faculdade de Ciências Médicas, Universidade Nova de Lisboa, Lisbon, Portugal
- 3ITQB-NOVA, Instituto de Tecnologia Química e Biológica António Xavier, Universidade Nova de Lisboa, Oeiras, Portugal
- 4Instituto de Medicina Molecular, Faculdade de Medicina, Universidade de Lisboa, Lisbon, Portugal
Diabetes affects hundreds of millions of patients worldwide. Despite the advances in understanding the disease and therapeutic options, it remains a leading cause of death and of comorbidities globally. Islet amyloid polypeptide (IAPP), or amylin, is a hormone produced by pancreatic β-cells. It contributes to the maintenance of glucose physiological levels namely by inhibiting insulin and glucagon secretion as well as controlling adiposity and satiation. IAPP is a highly amyloidogenic polypeptide forming intracellular aggregates and amyloid structures that are associated with β-cell death. Data also suggest the relevance of unprocessed IAPP forms as seeding for amyloid buildup. Besides the known consequences of hyperamylinemia in the pancreas, evidence has also pointed out that IAPP has a pathological role in cognitive function. More specifically, IAPP was shown to impair the blood–brain barrier; it was also seen to interact and co-deposit with amyloid beta peptide (Aß), and possibly with Tau, within the brain of Alzheimer's disease (AD) patients, thereby contributing to diabetes-associated dementia. In fact, it has been suggested that AD results from a metabolic dysfunction in the brain, leading to its proposed designation as type 3 diabetes. Here, we have first provided a brief perspective on the IAPP amyloidogenic process and its role in diabetes and AD. We have then discussed the potential interventions for modulating IAPP proteotoxicity that can be explored for therapeutics. Finally, we have proposed the concept of a “diabetes brain phenotype” hypothesis in AD, which may help design future IAPP-centered drug developmentstrategies against AD.
Introduction
Amyloidogenesis is a process by which peptides spontaneously self-assemble into higher order structures, namely oligomers, protofibrils, and mature amyloid fibrils (Martins et al., 2008; Maurer-Stroh et al., 2010; Hauser et al., 2014). These mature amyloid fibrils are highly ordered structures with fibrillar aggregates derived from different amyloidogenic amino acid sequences that share common features (Maurer-Stroh et al., 2010). The current consensus is that the amyloid fibrils are not the main cause of toxicity (Martins et al., 2008; Kuperstein et al., 2010; Hauser et al., 2014). This seems to be mostly down to precursor oligomers and protofibrils, which are associated with a number of the so-called amyloid diseases, including type 2 diabetes mellitus (T2DM), Alzheimer's disease (AD), Parkinson's disease, and cataracts (Hauser et al., 2014; Cremades and Dobson, 2018).
T2DM, the most prevalent type of diabetes, is an islet amyloid polypeptide (IAPP)-associated pathology (Cukierman et al., 2005; Westermark et al., 2011; Yang and Song, 2013). Dementia also represents a major public concern, affecting 50 million people worldwide. AD, the most common form of dementia in North America (Alzheimer's Association, 2016; Bondi et al., 2017; Lane et al., 2018), is associated with amyloid beta peptide 42 (Aß-42) (Martins et al., 2008; Kuperstein et al., 2010). The amyloid hypothesis on AD pathology is, however, called into question by the undeniable role of Tau aggregation and other important players, as has been reviewed (Makin, 2018).
There is much evidence to support the close association between T2DM and AD. IAPP (also known as amylin) and Aß-42 were proven to co-deposit, contributing to AD onset and progression (Jackson et al., 2013; Wijesekara et al., 2017). In addition, it the molecular interaction between Tau and IAPP was recently proved (Arya et al., 2019). At last, AD is associated with insulin resistance and an imbalance of glucose levels in the brain (Cukierman et al., 2005; Yang and Song, 2013), earning the designation of type 3 diabetes (T3DM) (de la Monte, 2014; Kandimalla et al., 2017; Leszek et al., 2017). Given these links, we have reviewed the mechanisms of IAPP dysfunction in diabetes and dementia, particularly in AD, thus adding to the recent view of multi-factorial contributions to both diseases. Furthermore, we have also discussed the potential interventions for modulating IAPP proteotoxicity that can be explored for therapeutics, encouraging new venues for treatment.
IAPP and Diabetes
Diabetes mellitus (DM) is one of the major causes of premature illness and mortality worldwide (Federation, 2009). High blood glucose levels and glucose intolerance, as a consequence of a defective insulin production/secretion by pancreatic β cells (β-cells) or insulin sensitivity (Stumvoll et al., 2005; Tan et al., 2019), are the typical clinical features of the disease. In T2DM, impairment and loss of β-cell mass has been associated with diverse pathological phenomena, including glucolipotoxicity, islet cholesterol accumulation, and islet inflammation (Poitout and Robertson, 2002; Ishikawa et al., 2008; Brunham et al., 2010; Donath and Shoelson, 2011). Equally important are the current views that regard IAPP dyshomeostasis, intracellular accumulation of IAPP oligomers, and IAPP amyloid deposition in the islets of Langerhans as detrimental events in β-cell dysfunction and disease (Kanatsuka et al., 2018).
IAPP is a 37-amino acid neuroendocrine hormone that plays an important role in regulating metabolism and glucose homeostasis (Figure 1A). In circulation, IAPP and insulin act as synergistic partners: they stimulate the uptake of blood glucose into muscle and fat tissues and inhibit the endogenous glucose output from the liver, thus stabilizing the blood sugar levels in post-meal conditions (Zhang et al., 2016). Physiologically, IAPP also reduces the secretion of nutrient-stimulated glucagon, regulates gastric emptying and satiation (Lutz, 2010; Akter et al., 2016), and regulates blood pressure while having an effect on the renin-angiotensin system (Wookey et al., 1998).
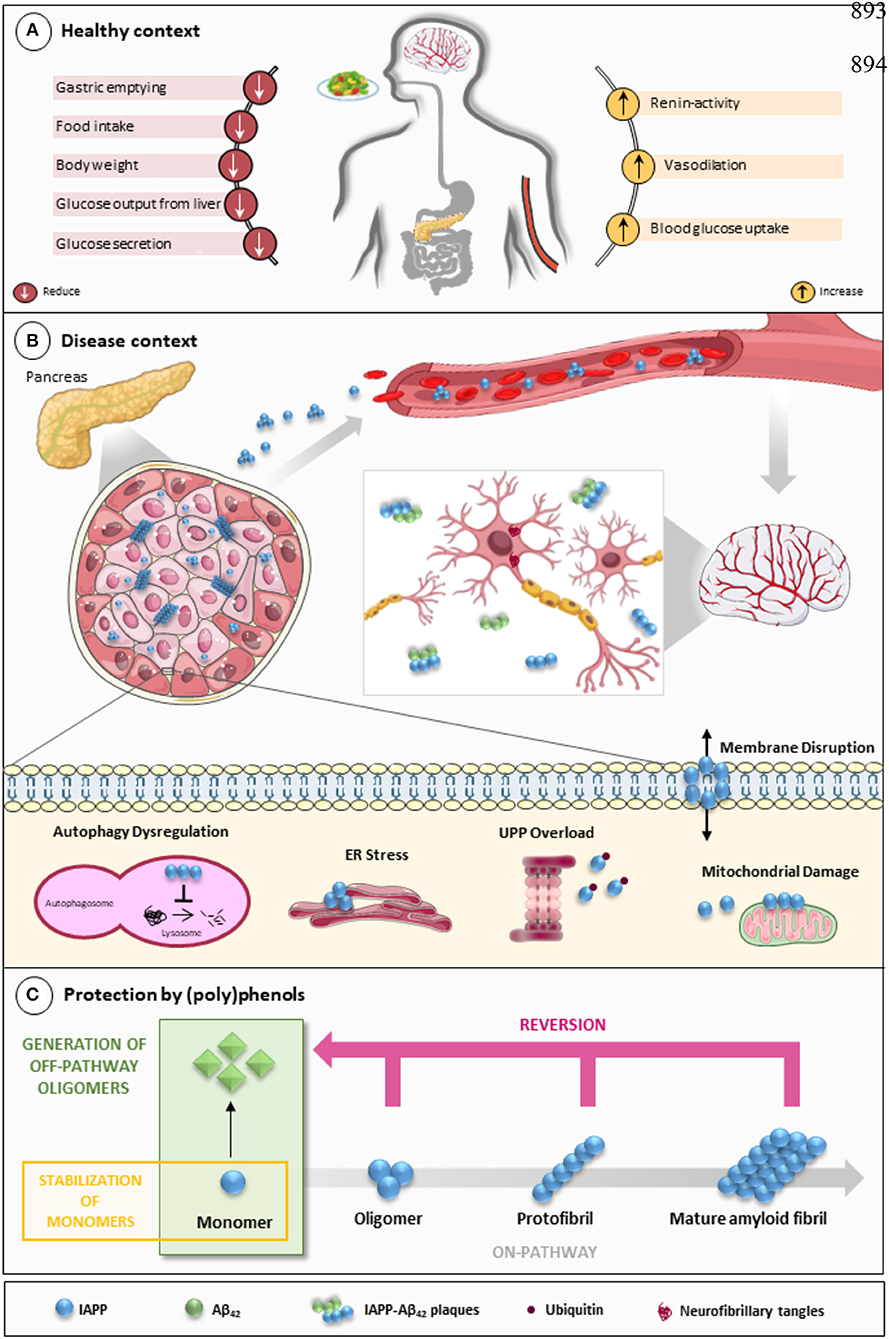
Figure 1. IAPP on physiological and pathological contexts and (poly)phenols-mediated protection. (A) In healthy conditions, IAPP is co-secreted with insulin to regulate glucose metabolism and homeostasis in a post-meal condition. Several functions are attributed to IAPP: slowing down gastric emptying, thereby reducing food intake and body weight; reducing glucose output from liver and glucagon secretion; and stimulating the renin-angiotensin system, vasodilation, and blood glucose uptake. (B) In disease conditions, IAPP pathological species deposit in the pancreas and in brain microvasculature where they induce the injury of small vessels and reach the brain parenchyma. In the brain environment, IAPP forms heterogeneous deposits with Aβ molecules increasing neurotoxicity. Proteostasis imbalance caused by Aβ/IAPP and tau may promote a set of molecular changes that culminate in glucose homeostasis dysregulation, cell death, and neurodegeneration. The molecular pathways of β-cell dysfunction are depicted: autophagy dysregulation; ER stress; UPP overload; membrane instability; and mitochondrial damage. (C) Protection mediated by (poly)phenols is associated with the stabilization of IAPP monomers, the remodeling of amyloids, protofibrils, and toxic oligomers to non-fibrillogenic “off-pathway” oligomers and monomers. Aβ, Amyloid beta; ER, Endoplasmic Reticulum; IAPP, Islet Amyloid Polypeptide; Ub, Ubiquitin; UPP, Ubiquitin Proteasome Pathway.
IAPP and insulin are co-secreted and processed by proprotein convertase (PC) 1/3, PC 2, and carboxypeptidase E (Yonemoto et al., 2008). During its biogenesis, IAPP is synthesized as an 89-residue preprohormone (Sanke et al., 1988). Its signal peptide is cleaved throughout the transport into the endoplasmic reticulum (ER) to form proIAPP (Akter et al., 2016), which is then processed in the late Golgi complex. To yield the mature active form of the hormone, IAPP suffers amidation of the C-terminal end, and a disulphide bond is formed between cysteines at positions two and seven (Westermark et al., 2011; Akter et al., 2016; Bower and Hay, 2016). Once produced, mature IAPP is co-packaged with insulin in secretory granules of β-cells to then be co-released in response to glucose (Kahn et al., 1993; Gedulin et al., 1997; Zhang et al., 2016). In a pre-diabetes/diabetes phenotypes, the increased production of insulin is accompanied by augmented IAPP levels (Kahn et al., 1991; Mulder et al., 1996). The overload and impairment of β-cell processing machinery leads to the accumulation of unprocessed IAPP forms (Westermark et al., 2000; Paulsson et al., 2006). These events, together with the overwhelming of the ER, generate a feed-forward cycle that promotes IAPP oligomerization, fibril formation, and β-cell injury. Elevated proIAPP levels and amyloid deposition in β-cells lacking PC1/3 and PC2 (Marzban et al., 2006), as well as the presence of proIAPP in intracellular fibrils (Paulsson et al., 2006), corroborate this idea. Despite this, the role of unprocessed IAPP forms in the disease is not fully understood.
Under pathological conditions, increased IAPP expression and the generation of aberrant IAPP intermediates favor misfolding, which leads to the formation of toxic aggregates through a seeding-nucleation model, similar to prion replication (Mukherjee et al., 2017). As misfolded molecules accumulate, they build up into intracellular oligomers and larger amyloid fibrils, which deposit in surrounding tissues, thus disrupting the normal islet architecture and functioning (Zhang et al., 2016). Deposits of aggregated IAPP are present in the pancreas of about 90% of T2DM patients, thus representing a histopathological hallmark of the disease (Westermark and Grimelius, 1973; Mukherjee et al., 2017). Corroborating the toxicity of these aggregates in diabetes, the IAPP allele S20G, which raises IAPP aggregation propensity (Sakagashira et al., 2000), has been associated with premature onset diabetes and has accelerated the decline of endogenous insulin secretion when compared to non-S20G T2DM individuals (Morita et al., 2011). Moreover, a transgenic mice model expressing human IAPP (hIAPP) spontaneously developed amyloidosis, showing impaired insulin production, β-cell loss, and fasting hyperglycemia (Janson et al., 1996).
Although the link between IAPP aggregation and β-cell loss seems to be convincing, there are some questions that remain poorly understood, including (a) the initiation site and triggers of amyloid formation, (b) the mechanisms of IAPP-mediated toxicity in β-cell death, and (c) the nature of toxic IAPP species (Kanatsuka et al., 2018). Initially, mature amyloid fibrils were presumed to be the pathological structures (Lorenzo and Yankner, 1996), however, the current consensus is that toxicity is mostly associated with soluble oligomers and protofibrils, which may act as the trigger agents for β-cell depletion and diabetes onset (Haataja et al., 2008; Zhao et al., 2009; Zhang et al., 2016).
Oligomeric IAPP species form ion-leaking pores in the cell membranes (Gurlo et al., 2010; Li et al., 2016b), leading to enhanced membrane fluidity, calcium dysregulation, and decreased cell viability (Huang et al., 2010). IAPP oligomers have also been found within disturbed mitochondrial membranes in transgenic hIAPP mice and T2DM patients (Gurlo et al., 2010). Unstable mitochondrial membrane potential induced by toxic oligomers is thought to be involved in the overproduction of reactive oxygen species (ROS), which are currently considered to be potential initiators of IAPP toxicity (Konarkowska et al., 2005). ER stress and impairment of proteasome function have also been associated with hIAPP-induced toxicity (Casas et al., 2007; Gurlo et al., 2010), however, in studies with cultured islets producing IAPP at more physiological levels, ER stress was not detected (Hull et al., 2009).
In heterozygous hIAPP+ mice with β cell–specific Atg7 deficiency (hIAPP+Atg7Δβcell mice), the accumulation of toxic oligomers, the loss of β-cells, and diabetes development is linked to autophagy disruption, and this is suggestive of a role for autophagy in IAPP toxicity (Kim et al., 2014). Moreover, inhibition of lysosomal degradation in HIP (hIAPP transgenic) rats increases hIAPP-mediated toxicity, whereas autophagy stimulation protects β-cells against hIAPP-induced apoptosis (Rivera et al., 2011). Chronic inflammation is also observed in local and systemic amyloidosis due to the activation of the NLRP3 inflammasome by hIAPP aggregates (Masters et al., 2010). A general view of IAPP pathological mechanisms is given in Figure 1B.
IAPP Pathology in the Brain
AD was considered for a long period to be caused by Aβ amyloidogenesis and/or Tau aggregation (Makin, 2018). Indeed, the presence of extracellular Aβ-42 amyloid plaques and intracellular aggregates of hyperphosphorylated Tau are the classical diagnostic markers of the disease (Glenner et al., 1984; Gotz, 2001; Gong et al., 2003). Aβ exists mainly in two forms, Aβ-40 and Aβ-42, composed of 40 and 42 amino acids, respectively, and the increase of the Aβ-42/Aβ-40 ratio is strongly correlated with AD severity (Kuperstein et al., 2010). Given the importance of these players in disease pathophysiology, AD research has been so focused on them that other possible agents have been somewhat overlooked.
More recently, IAPP has emerged as a novel player in AD pathology (de la Monte and Wands, 2008; Wijesekara et al., 2017; Norwitz et al., 2019; Qiu et al., 2019). Notwithstanding, the mechanisms by which IAPP contributes to AD pathology are still unclear and deserve further enquiry. It is known that IAPP and Aβ interact with each other and that IAPP promotes Aβ aggregation in a seeding-like manner, leading to the formation of cross-seeded oligomers (Andreetto et al., 2010; Rezaei-Ghaleh et al., 2011; Yan et al., 2014; Hu et al., 2015; Bakou et al., 2017; Moreno-Gonzalez et al., 2017; Ge et al., 2018; Armiento et al., 2019). Interestingly, an aggregation blocker mimicking IAPP has been proven to work against Aβ (Yan et al., 2007).
Hyperamylinemia has been pointed out as a possible trigger for IAPP misfolding and aggregation, which may cause damage in the brain (Jackson et al., 2013) and other organs by various mechanisms that include the toxic gain-of-function of IAPP aggregates and the loss of IAPP physiological functions (Westermark et al., 2011; Despa et al., 2012, 2014). In addition, IAPP dyshomeostais may affect other organs, particularly the brain, in Aβ-42-dependent and -independent manners. This is illustrated by studies showing that IAPP deposition impairs brain function regardless of Aβ-42 pathology (Srodulski et al., 2014) and that the brain of AD patients can also have IAPP deposits, alone or in the presence of Aβ-42 (Fawver et al., 2014), even if clinical signs of diabetes are absent (Jackson et al., 2013; Oskarsson et al., 2015). A remarkable aspect is the fact that the IAPP analog pramlintide is able to have a neuroprotective effect, both in AD pathogenesis as well as on cognition in general (Adler et al., 2014). This is in line with observations that the key regions involved in Aβ-42-IAPP interaction—the interface amino acid residues—are at the same time high-affinity binding sites in both the cross- and self-aggregation of these molecules (Andreetto et al., 2010). Pramlintide possibly modulates these interactions by preventing them or promoting the formation of biologically inactive fibrils. However, the in silico cross seeding of Aβ-42 and IAPP fibril-like oligomers still needs to be complemented with further experimental evidence to support this hypothesis (Berhanu et al., 2013). In addition to Aβ-42, it was also reported that the major component of cerebrovascular plaques in the AD brain, the Aβ-40, can cross-seed IAPP fibrillization, suggesting that these two peptides might populate states that cross-interact (O'Nuallain et al., 2004). Other mechanisms by which IAPP dyshomeostasis exacerbates Aβ-42 toxicity in the brain may include ROS generation (Jhamandas and MacTavish, 2004; Lim et al., 2010) and the breakdown of insulin degrading enzyme activity, which is responsible for insulin, IAPP, and Aβ degradation (Kurochkin and Goto, 1994; McDermott and Gibson, 1997).
As IAPP produced in the pancreas was shown to cross the blood–brain barrier (Banks et al., 1995; Banks and Kastin, 1998) and to act on brain receptors, another important aspect of IAPP pathophysiology in the brain is its role in neuronal network function. Therefore, the effects of IAPP on neuronal and glial cells have been investigated (Chaitanya et al., 2011; Xi et al., 2019). As the primary site of IAPP action, the area postrema (AP) is the brain structure best characterized in terms of IAPP effects. While IAPP was shown to promote the formation of AP neuronal projections in neonatal rodents, in adult Wistar rats, IAPP injections were reported (1) to affect genes controlling neurogenesis, particularly NeuroD1, (2) to increase the number of newly proliferated AP-cells, and (3) to promote differentiation of these cells into neurons (Liberini et al., 2016). A study to investigate the mechanism by which IAPP modulates neuronal excitability in AP neurons in rat brainstem slices revealed that IAPP induced changes in excitatory responses of neurons not displaying the hyperpolarization-activated cation current. Furthermore, this study revealed that IAPP receptors were mainly located on presynaptic glutamatergic terminals connecting these neurons and that IAPP can increase glutamate release enough to cause cell firing (Fukuda et al., 2013). Likewise, hIAPP was shown to cause a dose-dependent membrane depolarization and an increase in firing frequency in neurons of the diagonal band of Broca, a cholinergic basal forebrain nucleus, in rats (Li and and Li, 2012). Hence, IAPP dysregulation may have important implications in neuronal function. IAPP receptors were also proven to be mediators of the deleterious actions of Aβ-42 in human neurons (Jhamandas et al., 2011). In this sense, amylin receptors are seen as potential targets for AD therapies (Fu et al., 2017).
AD is also considered a metabolic disease to a large extent. It is clear that the brain loses its capacity to deal with glucose and to respond to insulin and insulin-like growth factor (IGF) (Rivera et al., 2005; Liu et al., 2011; Talbot et al., 2012). The inability to respond to insulin and IGF leads to brain “starvation” and neuronal loss (de la Monte et al., 2009; de la Monte, 2012). Moreover, reducing the activity of the insulin/IGF signaling cascade seems to protect from AD-like neurodegeneration in nematodes, possibly by promoting more densely packed (and less toxic) amyloid fibrils (Cohen and Goedert, 2004; El-Ami et al., 2014). Thus, the link between AD and insulin/IGF exists, but it is not easy to decipher. However, some of the mechanisms involved are becoming clear. For example, the kinases that promote Tau phosphorylation, causing cell death, become increasingly activated due to insulin resistance (Schubert et al., 2003, 2004). Then, Aβ-42 and its precursor protein levels also increase in the brain as a result of insulin resistance (Messier and Teutenberg, 2005). One can state that, what could be called the “brain diabetes phenotype,” i.e., increased resistance to insulin and to IGF, can result in the appearance of classical AD molecular biomarkers. Besides these clear links between diabetes and AD-related peptides and proteins, the physiological functioning of insulin and IGF promotes neuronal growth, differentiation, and the formation of synapses, the lack of which is associated with dementia (Takeda et al., 2010; Westwood et al., 2014). Overall, insulin and IGF are required for synaptic plasticity and are necessary for the cognitive function, the mechanisms of which are only partially explained (Qiu et al., 1998; Wickelgren, 1998; Zhao and Alkon, 2001). Oxidative stress is also associated with AD and diabetes as well as advanced glycation end products (Ramasamy et al., 2011; Silveira et al., 2019).
Although studies focusing on IAPP, insulin, and IGF are stimulating and may lead to exciting developments, one must be careful to draw definitive conclusions regarding multi-factorial diseases such as AD, even if it has been analyzed through the prism of the glucose metabolism. The road to a treatment for AD is full of failed starts and drug-development pipeline failures even if one (partially) understands the mechanism involved (Berhanu et al., 2013). The fact that aging implies reductions in insulin and IAPP release (Dechenes et al., 1998) provides important clues that, in retrospect, should not have been overlooked for so long (Despa and Decarli, 2013). The most powerful process may be related to IGF-I, which has been shown to protect and rescue hippocampal neurons from Aβ-42 neurotoxicity and IAPP-induced toxicity, as a two-in-one solution. This was already reported over 20 years ago (Doré et al., 1997), but, inexplicably, it was somewhat ignored. This is no longer the case: the role of IAPP in AD is not overlooked, as IAPP is even seen as the second amyloid of AD pathology, a promising approach to understand IAPP in relation to AD (Fawver et al., 2014). A curious finding is that Aβ-42 directly activates the amylin-3 receptor subtype, which may have major implications in AD pathology (Fu et al., 2012) as well as in the “brain diabetes phenotype” that we have proposed here. Moreover, it may also explain why pramlintide, which acts on rat and human amylin receptors (Gingell et al., 2014), can be protective in AD. Interestingly, Aβ-42 expressed on human neurons can bind to amylin receptors (Jhamandas et al., 2011), thereby triggering activation of apoptotic genes, as IAPP does (Jhamandas and Mactavish, 2012). The activity of these molecules on the brain may lead to neuronal death, particularly in AD patients, thus explaining their phenotypic profiles (Kawarabayashi et al., 2001; Dubois et al., 2016; Li and Huang, 2016; Li et al., 2016a).
Strategies for Reducing IAPP Proteotoxicity Using Natural Compounds
The links between IAPP and AD have not gone unnoticed, with some authors presenting relevant reviews on the topic and hinting at possible therapeutic strategies (Despa and Decarli, 2013; Jackson et al., 2013; Bharadwaj et al., 2017; Mietlicki-Baase, 2018). The role of IAPP is undeniably relevant in both diabetes and AD. Therefore, attempting to modulate the oligomerization process or block its cytotoxicity is an appealing venue for therapeutic strategies. Different approaches have been attempted to block protein aggregation (Figure 1C). Efforts have been made to interfere with the oligomerization process itself by (i) stabilizing the monomer, (ii) remodeling small oligomers from a fibrillogenic to non-fibrillogenic form, thereby creating “off-pathway” oligomers, and (iii) reverting fibrils to monomers or other intermediate species (Pithadia et al., 2016; Table 1). Another strategy is to revert the pathological effects of oligomers in cellular homeostasis, such as ER stress, mitochondrial damage, cell membrane permeabilization, autophagy impairment, inflammation, and β-cell death (Kiriyama and Nochi, 2018).
The pleiotropic action of (poly)phenols toward chronic diseases, particularly diabetes, is well-documented (Bahadoran et al., 2013; Panickar, 2013; Jasmin and Jaitak, 2019; Silveira et al., 2019). Most importantly, (poly)phenols have been linked to the inhibition of aggregation of proteins such as IAPP and Aβ-42 (Pithadia et al., 2016; Sequeira and Poppitt, 2017; Dhouafli et al., 2018). It has been shown that different classes of (poly)phenols may interfere with different steps of the oligomerization process (Ladiwala et al., 2011). The lower toxicity of these compounds compared to synthetic molecules gives them an advantage as future therapeutics. However, there is an urgent need for the validation of their therapeutic potential in pre-clinical studies, as most of the evidences derives from cell-free and in vitro assays (Table 1).
Epigallocatechin gallate (EGCG) and resveratrol are the most-studied compounds. EGCG has been proved to remodel IAPP oligomers, create “off-pathway” intermediates, and prevent monomers from shifting into β-sheet structures, a critical step in early-stage aggregation processes (Bieschke et al., 2010; Young et al., 2014a; Nedumpully-Govindan et al., 2016). Resveratrol has also been suggested as an inhibitor of both IAPP and Aβ-42 pathological effects. It was reported to lower intracellular and secreted levels of Aβ-42 and also to stimulate intracellular degradation (Marambaud et al., 2005). However, resveratrol seems to be less effective than EGCG and inefficient in preventing amyloid formation (Tu et al., 2015). In addition, (poly)phenols have an important role in reducing oligomer-induced cytotoxicity by modulating oxidative stress (Chakrabarti et al., 2013), inflammation (Apetz et al., 2014), and autophagy (Rigacci et al., 2015). A compilation of (poly)phenols as bioactive components modulating IAPP toxicity is given in Table 1.
Concluding Remarks
This study shows how an “old story” can originate ground-breaking knowledge and create new venues for a therapeutic approach. The first high-impact paper describing IAPP as a relevant factor for T2DM was published in 1994 (Lorenzo et al., 1994). Since then, even though it took a long time for this field to be pursued, knowledge has come a long way. It is now clear that direct brain microvascular injury, leading to white matter disease, is unequivocally originated by elevated IAPP levels in diabetes (Ly et al., 2017), further supporting the “diabetes brain phenotype” hypothesis that we have proposed here.
This change of approach is as cutting-edge as the finding that amyloid fibrils precursors, but not the amyloid fibrils themselves, are the cause of toxicity (Martins et al., 2008). We believe that this study, and others that reflect on the role of IAPP in AD in an unbiased manner (Mietlicki-Baase, 2018) complemented by further experiments, will certainly pave the road to future IAPP-centered drug development strategies against AD, as we considering it as the result of a “diabetes brain phenotype.” Such a view will certainly yield major therapeutic advances.
Author Contributions
AR and SF wrote the manuscript. IM wrote and revised the manuscript. RM designed the layout and wrote and revised the manuscript.
Funding
We acknowledge iNOVA4Health—UID/Multi/04462/2019, a program financially supported by Fundação para a Ciência e Tecnologia/Ministério da Educação e Ciência, through national funds and co-funded by FEDER under the PT2020 Partnership Agreement. Funding from the INTERFACE Programme, through the Innovation, Technology and Circular Economy Fund (FITEC) is gratefully acknowledged. This study was also supported by FCT via PTDC/BIA-MOL31104/2017 and UID/Multi/04462/2019-SubProj iNOVA4Health 44 to RM and PD/BD/135504/2018 to AR. Sociedade Portuguesa de Diabetologia for the Nuno Castelo-Branco Prize−2016, attributed to RM, was also acknowledged. IM acknowledges FCT-MCTES Program Concurso de Estímulo ao Emprego Científico (CEECIND/01670/2017).
Conflict of Interest
The authors declare that the research was conducted in the absence of any commercial or financial relationships that could be construed as a potential conflict of interest.
References
Aarabi, M. H., and Mirhashemi, S. M. (2017). To estimate effective antiamyloidogenic property of melatonin and fisetin and their actions to destabilize amyloid fibrils. Pak. J. Pharm. Sci. 30, 1589–1593.
Adler, B. L., Yarchoan, M., Hwang, H. M., Louneva, N., Blair, J. A., Palm, R., et al. (2014). Neuroprotective effects of the amylin analogue pramlintide on Alzheimer's disease pathogenesis and cognition. Neurobiol. Aging 35, 793–801. doi: 10.1016/j.neurobiolaging.2013.10.076
Aitken, J. F., Loomes, K. M., Riba-Garcia, I., Unwin, R. D., Prijic, G., Phillips, A. S., et al. (2017). Rutin suppresses human-amylin/hIAPP misfolding and oligomer formation in-vitro, and ameliorates diabetes and its impacts in human-amylin/hIAPP transgenic mice. Biochem. Biophys. Res. Commun. 482, 625–631. doi: 10.1016/j.bbrc.2016.11.083
Akter, R., Cao, P., Noor, H., Ridgway, Z., Tu, L.-H., Wang, H., et al. (2016). Islet amyloid polypeptide: structure, function, and pathophysiology. J. Diabetes Res. 2016:2798269. doi: 10.1155/2016/2798269
Alzheimer's Association (2016). 2016 Alzheimer's disease facts and figures. Alzheimers Dement. 12, 459–509. doi: 10.1016/j.jalz.2016.03.001
Andreetto, E., Yan, L. M., Tatarek-Nossol, M., Velkova, A., Frank, R., and Kapurniotu, A. (2010). Identification of hot regions of the Abeta-IAPP interaction interface as high-affinity binding sites in both cross- and self-association. Angew. Chem. Int. Ed. Engl. 49, 3081–3085. doi: 10.1002/anie.200904902
Apetz, N., Munch, G., Govindaraghavan, S., and Gyengesi, E. (2014). Natural compounds and plant extracts as therapeutics against chronic inflammation in Alzheimer's disease–a translational perspective. CNS Neurol. Disord. Drug Targets 13, 1175–1191. doi: 10.2174/1871527313666140917110635
Armiento, V., Spanopoulou, A., and Kapurniotu, A. (2019). Peptide-based molecular strategies to interfere with protein misfolding, aggregation, and cell degeneration. Angew. Chem. Int. Ed. 59, 3372–84. doi: 10.1002/anie.201906908
Arya, S., Claud, S. L., Cantrell, K. L., and Bowers, M. T. (2019). Catalytic prion-like cross-talk between a key alzheimer's disease tau-fragment r3 and the type 2 diabetes peptide IAPP. ACS Chem. Neurosci. 10, 4757–4765. doi: 10.1021/acschemneuro.9b00516
Bahadoran, Z., Mirmiran, P., and Azizi, F. (2013). Dietary polyphenols as potential nutraceuticals in management of diabetes: a review. J. Diabetes. Metab. Disord. 12:43. doi: 10.1186/2251-6581-12-43
Bakou, M., Hille, K., Kracklauer, M., Spanopoulou, A., Frost, C. V., Malideli, E., et al. (2017). Key aromatic/hydrophobic amino acids controlling a cross-amyloid peptide interaction versus amyloid self-assembly. J. Biol. Chem. 292, 14587–14602. doi: 10.1074/jbc.M117.774893
Banks, W. A., and Kastin, A. J. (1998). Differential permeability of the blood-brain barrier to two pancreatic peptides: insulin and amylin. Peptides 19, 883–889. doi: 10.1016/S0196-9781(98)00018-7
Banks, W. A., Kastin, A. J., Maness, L. M., Huang, W., and Jaspan, J. B. (1995). Permeability of the blood-brain barrier to amylin. Life Sci. 57, 1993–2001. doi: 10.1016/0024-3205(95)02197-Q
Berhanu, W. M., Yaşar, F., and Hansmann, U. H. E. (2013). In silico cross seeding of Aβ and amylin fibril-like oligomers. ACS Chem. Neurosci. 4, 1488–1500. doi: 10.1021/cn400141x
Bharadwaj, P., Wijesekara, N., Liyanapathirana, M., Newsholme, P., Ittner, L., Fraser, P., et al. (2017). The link between type 2 diabetes and neurodegeneration: roles for amyloid-beta, amylin, and tau proteins. J. Alzheimers Dis. 59, 421–432. doi: 10.3233/JAD-161192
Bieschke, J., Russ, J., Friedrich, R. P., Ehrnhoefer, D. E., Wobst, H., Neugebauer, K., et al. (2010). EGCG remodels mature alpha-synuclein and amyloid-beta fibrils and reduces cellular toxicity. Proc. Natl. Acad. Sci. U.S.A. 107, 7710–7715. doi: 10.1073/pnas.0910723107
Bondi, M. W., Edmonds, E. C., and Salmon, D. P. (2017). Alzheimer's disease: past, present, and future. J. Int. Neuropsychol. Soc. 23, 818–831. doi: 10.1017/S135561771700100X
Bower, R. L., and Hay, D. L. (2016). Amylin structure-function relationships and receptor pharmacology: implications for amylin mimetic drug development. Br. J. Pharmacol. 173, 1883–1898. doi: 10.1111/bph.13496
Brunham, L. R., Kruit, J. K., Hayden, M. R., and Verchere, C. B. (2010). Cholesterol in beta-cell dysfunction: the emerging connection between HDL cholesterol and type 2 diabetes. Curr. Diab. Rep. 10, 55–60. doi: 10.1007/s11892-009-0090-x
Bruno, E., Pereira, C., Roman, K. P., Takiguchi, M., Kao, P. Y., Nogaj, L. A., et al. (2013). IAPP aggregation and cellular toxicity are inhibited by 1,2,3,4,6-penta-O-galloyl-beta-D-glucose. Amyloid 20, 34–38. doi: 10.3109/13506129.2012.762761
Cao, P., and Raleigh, D. P. (2012). Analysis of the inhibition and remodeling of islet amyloid polypeptide amyloid fibers by flavanols. Biochemistry 51, 2670–2683. doi: 10.1021/bi2015162
Casas, S., Gomis, R., Gribble, F. M., Altirriba, J., Knuutila, S., and Novials, A. (2007). Impairment of the ubiquitin-proteasome pathway is a downstream endoplasmic reticulum stress response induced by extracellular human islet amyloid polypeptide and contributes to pancreatic beta-cell apoptosis. Diabetes 56, 2284–2294. doi: 10.2337/db07-0178
Chaitanya, G. V., Cromer, W. E., Wells, S. R., Jennings, M. H., Couraud, P. O., Romero, I. A., et al. (2011). Gliovascular and cytokine interactions modulate brain endothelial barrier in vitro. J Neuroinflammation 8:162. doi: 10.1186/1742-2094-8-162
Chakrabarti, S., Sinha, M., Thakurta, I. G., Banerjee, P., and Chattopadhyay, M. (2013). Oxidative stress and amyloid beta toxicity in Alzheimer's disease: intervention in a complex relationship by antioxidants. Curr. Med. Chem. 20, 4648–4664. doi: 10.2174/09298673113209990152
Cheng, B., Gong, H., Li, X., Sun, Y., Chen, H., Zhang, X., et al. (2013). Salvianolic acid B inhibits the amyloid formation of human islet amyloid polypeptide and protects pancreatic beta-cells against cytotoxicity. Proteins 81, 613–621. doi: 10.1002/prot.24216
Cheng, B., Gong, H., Li, X., Sun, Y., Zhang, X., Chen, H., et al. (2012). Silibinin inhibits the toxic aggregation of human islet amyloid polypeptide. Biochem. Biophys. Res. Commun. 419, 495–499. doi: 10.1016/j.bbrc.2012.02.042
Cohen, P., and Goedert, M. (2004). GSK3 inhibitors: development and therapeutic potential. Nat. Rev. Drug Discov. 3, 479–487. doi: 10.1038/nrd1415
Cremades, N., and Dobson, C. M. (2018). The contribution of biophysical and structural studies of protein self-assembly to the design of therapeutic strategies for amyloid diseases. Neurobiol. Dis. 109(Pt B), 178–190. doi: 10.1016/j.nbd.2017.07.009
Cukierman, T., Gerstein, H. C., and Williamson, J. D. (2005). Cognitive decline and dementia in diabetes–systematic overview of prospective observational studies. Diabetologia 48, 2460–2469. doi: 10.1007/s00125-005-0023-4
Daval, M., Bedrood, S., Gurlo, T., Huang, C. J., Costes, S., Butler, P. C., et al. (2010). The effect of curcumin on human islet amyloid polypeptide misfolding and toxicity. Amyloid 17, 118–128. doi: 10.3109/13506129.2010.530008
de la Monte, S. M. (2012). Contributions of brain insulin resistance and deficiency in amyloid-related neurodegeneration in Alzheimer's disease. Drugs 72, 49–66. doi: 10.2165/11597760-000000000-00000
de la Monte, S. M. (2014). Type 3 diabetes is sporadic Alzheimers disease: mini-review. Eur. Neuropsychopharmacol. 24, 1954–1960. doi: 10.1016/j.euroneuro.2014.06.008
de la Monte, S. M., Longato, L., Tong, M., and Wands, J. R. (2009). Insulin resistance and neurodegeneration: roles of obesity, type 2 diabetes mellitus and non-alcoholic steatohepatitis. Curr. Opin. Investig. Drugs 10, 1049–1060.
de la Monte, S. M., and Wands, J. R. (2008). Alzheimer's disease is type 3 diabetes-evidence reviewed. J. Diabetes Sci. Technol. 2, 1101–1113. doi: 10.1177/193229680800200619
Dechenes, C. J., Verchere, C. B., Andrikopoulos, S., and Kahn, S. E. (1998). Human aging is associated with parallel reductions in insulin and amylin release. Am. J. Physiol. 275, E785–91. doi: 10.1152/ajpendo.1998.275.5.E785
Despa, F., and Decarli, C. (2013). Amylin: what might be its role in Alzheimer's disease and how could this affect therapy? Expert Rev. Proteomics 10, 403–405. doi: 10.1586/14789450.2013.841549
Despa, S., Margulies, K. B., Chen, L., Knowlton, A. A., Havel, P. J., Taegtmeyer, H., et al. (2012). Hyperamylinemia contributes to cardiac dysfunction in obesity and diabetes: a study in humans and rats. Circ. Res. 110, 598–608. doi: 10.1161/CIRCRESAHA.111.258285
Despa, S., Sharma, S., Harris, T. R., Dong, H., Li, N., Chiamvimonvat, N., et al. (2014). Cardioprotection by controlling hyperamylinemia in a “humanized” diabetic rat model. J. Am. Heart Assoc. 3:e001015. doi: 10.1161/JAHA.114.001015
Dhouafli, Z., Cuanalo-Contreras, K., Hayouni, E. A., Mays, C. E., Soto, C., and Moreno-Gonzalez, I. (2018). Inhibition of protein misfolding and aggregation by natural phenolic compounds. Cell Mol. Life Sci. 75, 3521–3538. doi: 10.1007/s00018-018-2872-2
Donath, M. Y., and Shoelson, S. E. (2011). Type 2 diabetes as an inflammatory disease. Nat. Rev. Immunol. 11, 98–107. doi: 10.1038/nri2925
Doré, S., Kar, S., and Quirion, R. (1997). Insulin-like growth factor I protects and rescues hippocampal neurons against β-amyloid- and human amylin-induced toxicity. Proc. Natl. Acad. Sci. U.S.A. 94, 4772–4777. doi: 10.1073/pnas.94.9.4772
Dubois, B., Padovani, A., Scheltens, P., Rossi, A., and Dell'Agnello, G. (2016). Timely diagnosis for Alzheimer's disease: a literature review on benefits and challenges. J. Alzheimers Dis. 49, 617–631. doi: 10.3233/JAD-150692
El-Ami, T., Moll, L., Carvalhal Marques, F., Volovik, Y., Reuveni, H., and Cohen, E. (2014). A novel inhibitor of the insulin/IGF signaling pathway protects from age-onset, neurodegeneration-linked proteotoxicity. Aging Cell 13, 165–174. doi: 10.1111/acel.12171
Engel, M. F., vandenAkker, C. C., Schleeger, M., Velikov, K. P., Koenderink, G. H., and Bonn, M. (2012). The polyphenol EGCG inhibits amyloid formation less efficiently at phospholipid interfaces than in bulk solution. J. Am. Chem. Soc. 134, 14781–14788. doi: 10.1021/ja3031664
Evers, F., Jeworrek, C., Tiemeyer, S., Weise, K., Sellin, D., Paulus, M., et al. (2009). Elucidating the mechanism of lipid membrane-induced IAPP fibrillogenesis and its inhibition by the red wine compound resveratrol: a synchrotron X-ray reflectivity study. J. Am. Chem. Soc. 131, 9516–9521. doi: 10.1021/ja8097417
Fawver, J. N., Ghiwot, Y., Koola, C., Carrera, W., Rodriguez-Rivera, J., Hernandez, C., et al. (2014). Islet amyloid polypeptide (IAPP): a second amyloid in Alzheimer's disease. Curr. Alzheimer Res. 11, 928–940. doi: 10.2174/1567205011666141107124538
Federation ID. (2009). IDF Diabetes Atlas, 4th Edn. Montreal, CA: International Diabetes Federation.
Franko, A., Rodriguez Camargo, D. C., Böddrich, A., Garg, D., Rodriguez Camargo, A., Rathkolb, B., et al. (2018). Epigallocatechin gallate (EGCG) reduces the intensity of pancreatic amyloid fibrils in human islet amyloid polypeptide (hIAPP) transgenic mice. Sci. Rep. 8:1116. doi: 10.1038/s41598-017-18807-8
Fu, W., Patel, A., Kimura, R., Soudy, R., and Jhamandas, J. H. (2017). Amylin receptor: a potential therapeutic target for Alzheimer's disease. Trends Mol. Med. 23, 709–720. doi: 10.1016/j.molmed.2017.06.003
Fu, W., Ruangkittisakul, A., MacTavish, D., Shi, J. Y., Ballanyi, K., and Jhamandas, J. H. (2012). Amyloid beta (Abeta) peptide directly activates amylin-3 receptor subtype by triggering multiple intracellular signaling pathways. J. Biol. Chem. 287, 18820–18830. doi: 10.1074/jbc.M111.331181
Fukuda, T., Hirai, Y., Maezawa, H., Kitagawa, Y., and Funahashi, M. (2013). Electrophysiologically identified presynaptic mechanisms underlying amylinergic modulation of area postrema neuronal excitability in rat brain slices. Brain Res. 1494, 9–16. doi: 10.1016/j.brainres.2012.11.051
Gao, M., Estel, K., Seeliger, J., Friedrich, R. P., Dogan, S., Wanker, E. E., et al. (2015). Modulation of human IAPP fibrillation: cosolutes, crowders and chaperones. Phys. Chem. Chem. Phys. 17, 8338–8348. doi: 10.1039/C4CP04682J
Ge, X., Yang, Y., Sun, Y., Cao, W., and Ding, F. (2018). Islet amyloid polypeptide promotes amyloid-beta aggregation by binding-induced helix-unfolding of the amyloidogenic core. ACS Chem. Neurosci. 9, 967–975. doi: 10.1021/acschemneuro.7b00396
Gedulin, B. R., Rink, T. J., and Young, A. A. (1997). Dose-response for glucagonostatic effect of amylin in rats. Metabolism 46, 67–70. doi: 10.1016/S0026-0495(97)90170-0
Gingell, J. J., Burns, E. R., and Hay, D. L. (2014). Activity of pramlintide, rat and human amylin but not Abeta1-42 at human amylin receptors. Endocrinology 155, 21–26. doi: 10.1210/en.2013-1658
Glenner, G. G., Wong, C. W., Quaranta, V., and Eanes, E. D. (1984). The amyloid deposits in Alzheimer's disease: their nature and pathogenesis. Appl. Pathol. 2, 357–369.
Gong, Y., Chang, L., Viola, K. L., Lacor, P. N., Lambert, M. P., Finch, C. E., et al. (2003). Alzheimer's disease-affected brain: presence of oligomeric A beta ligands (ADDLs) suggests a molecular basis for reversible memory loss. Proc. Natl. Acad. Sci. U.S.A. 100, 10417–10422. doi: 10.1073/pnas.1834302100
Gotz, J. (2001). Tau and transgenic animal models. Brain Res. Brain Res. Rev. 35, 266–286. doi: 10.1016/S0165-0173(01)00055-8
Gurlo, T., Ryazantsev, S., Huang, C.-,j., Yeh, M. W., Reber, H. A., Hines, O. J., et al. (2010). Evidence for proteotoxicity in beta cells in type 2 diabetes: toxic islet amyloid polypeptide oligomers form intracellularly in the secretory pathway. Am. J. Pathol. 176, 861–869. doi: 10.2353/ajpath.2010.090532
Haataja, L., Gurlo, T., Huang, C. J., and Butler, P. C. (2008). Islet amyloid in type 2 diabetes, and the toxic oligomer hypothesis. Endocr. Rev. 29, 303–316. doi: 10.1210/er.2007-0037
Hauser, C. A., Maurer-Stroh, S., and Martins, I. C. (2014). Amyloid-based nanosensors and nanodevices. Chem. Soc. Rev. 43, 5326–5345. doi: 10.1039/C4CS00082J
Hu, R., Zhang, M., Chen, H., Jiang, B., and Zheng, J. (2015). Cross-seeding interaction between beta-amyloid and human islet amyloid polypeptide. ACS Chem. Neurosci. 6, 1759–1768. doi: 10.1021/acschemneuro.5b00192
Huang, C.-J., Gurlo, T., Haataja, L., Costes, S., Daval, M., Ryazantsev, S., et al. (2010). Calcium-activated calpain-2 is a mediator of beta cell dysfunction and apoptosis in type 2 diabetes. J.Biol. Chem. 285, 339–348. doi: 10.1074/jbc.M109.024190
Hull, R. L., Zraika, S., Udayasankar, J., Aston-Mourney, K., Subramanian, S. L., and Kahn, S. E. (2009). Amyloid formation in human IAPP transgenic mouse islets and pancreas, and human pancreas, is not associated with endoplasmic reticulum stress. Diabetologia 52, 1102–1111. doi: 10.1007/s00125-009-1329-4
Ishikawa, M., Iwasaki, Y., Yatoh, S., Kato, T., Kumadaki, S., Inoue, N., et al. (2008). Cholesterol accumulation and diabetes in pancreatic beta-cell-specific SREBP-2 transgenic mice: a new model for lipotoxicity. J. Lipid. Res. 49, 2524–2534. doi: 10.1194/jlr.M800238-JLR200
Jackson, K., Barisone, G. A., Diaz, E., Jin, L. W., DeCarli, C., and Despa, F. (2013). Amylin deposition in the brain: a second amyloid in Alzheimer disease? Ann. Neurol. 74, 517–526. doi: 10.1002/ana.23956
Janson, J., Soeller, W. C., Roche, P. C., Nelson, R. T., Torchia, A. J., Kreutter, D. K., et al. (1996). Spontaneous diabetes mellitus in transgenic mice expressing human islet amyloid polypeptide. Proc. Natl. Acad. Sci. U.S.A. 93, 7283–7288. doi: 10.1073/pnas.93.14.7283
Jasmin, and Jaitak, V. (2019). A review on molecular mechanism of flavonoids as antidiabetic agents. Mini. Rev. Med. Chem. 19, 762–786. doi: 10.2174/1389557519666181227153428
Jesus, A. R., Dias, C., Matos, A. M., de Almeida, R. F. M., Viana, A. S., Marcelo, F., et al. (2014). Exploiting the therapeutic potential of 8-β-d-glucopyranosylgenistein: synthesis, antidiabetic activity, and molecular interaction with islet amyloid polypeptide and amyloid β-peptide (1–42). J. Med. Chem. 57, 9463–9472. doi: 10.1021/jm501069h
Jhamandas, J. H., Li, Z., Westaway, D., Yang, J., Jassar, S., and MacTavish, D. (2011). Actions of β-amyloid protein on human neurons are expressed through the amylin receptor. Am. J. Pathol. 178, 140–149. doi: 10.1016/j.ajpath.2010.11.022
Jhamandas, J. H., and MacTavish, D. (2004). Antagonist of the amylin receptor blocks beta-amyloid toxicity in rat cholinergic basal forebrain neurons. J. Neurosci. 24, 5579–5584. doi: 10.1523/JNEUROSCI.1051-04.2004
Jhamandas, J. H., and Mactavish, D. (2012). β-Amyloid protein (Aβ) and human amylin regulation of apoptotic genes occurs through the amylin receptor. Apoptosis 17, 37–47. doi: 10.1007/s10495-011-0656-3
Jiang, P., Li, W., Shea, J. E., and Mu, Y. (2011). Resveratrol inhibits the formation of multiple-layered β-sheet oligomers of the human islet amyloid polypeptide segment 22-27. Biophys. J. 100, 1550–1558. doi: 10.1016/j.bpj.2011.02.010
Kahn, S. E., Fujimoto, W. Y., D'Alessio, D. A., Ensinck, J. W., and Porte, D. Jr. (1991). Glucose stimulates and potentiates islet amyloid polypeptide secretion by the B-cell. Horm. Metab. Res. 23, 577–580. doi: 10.1055/s-2007-1003759
Kahn, S. E., Verchere, C. B., D'Alessio, D. A., Cook, D. L., and Fujimoto, W. Y. (1993). Evidence for selective release of rodent islet amyloid polypeptide through the constitutive secretory pathway. Diabetologia 36, 570–573. doi: 10.1007/BF02743276
Kamihira-Ishijima, M., Nakazawa, H., Kira, A., Naito, A., and Nakayama, T. (2012). Inhibitory mechanism of pancreatic amyloid fibril formation: formation of the complex between tea catechins and the fragment of residues 22-27. Biochemistry 51, 10167–10174. doi: 10.1021/bi3012274
Kanatsuka, A., Kou, S., and Makino, H. (2018). Correction to: IAPP/amylin and betacell failure: implication of the risk factors of type 2 diabetes. Diabetol. Int. 9, 143–157. doi: 10.1007/s13340-018-0363-1
Kandimalla, R., Thirumala, V., and Reddy, P. H. (2017). Is Alzheimer's disease a Type 3 Diabetes? A critical appraisal. Biochim. Biophys. Acta Mol. Basis. Dis. 1863, 1078–1089. doi: 10.1016/j.bbadis.2016.08.018
Kawarabayashi, T., Younkin, L. H., Saido, T. C., Shoji, M., Ashe, K. H., and Younkin, S. G. (2001). Age-dependent changes in brain, CSF, and plasma amyloid (beta) protein in the Tg2576 transgenic mouse model of Alzheimer's disease. J. Neurosci. 21, 372–381. doi: 10.1523/JNEUROSCI.21-02-00372.2001
Kim, J., Cheon, H., Taek Jeong, Y., Quan, W., Kim, K., Min Cho, J., et al. (2014). Amyloidogenic peptide oligomer accumulation in autophagy-deficient β cells induces diabetes. J. Clin. Invest. 124, 3311–3324. doi: 10.1172/JCI69625
Kiriyama, Y., and Nochi, H. (2018). Role and cytotoxicity of amylin and protection of pancreatic islet beta-cells from amylin cytotoxicity. Cell 7:95. doi: 10.3390/cells7080095
Konarkowska, B., Aitken, J. F., Kistler, J., Zhang, S., and Cooper, G. J. (2005). Thiol reducing compounds prevent human amylin-evoked cytotoxicity. FEBS J. 272, 4949–4959. doi: 10.1111/j.1742-4658.2005.04903.x
Kuperstein, I., Broersen, K., Benilova, I., Rozenski, J., Jonckheere, W., Debulpaep, M., et al. (2010). Neurotoxicity of Alzheimer's disease Abeta peptides is induced by small changes in the Aβ42 to Aβ40 ratio. EMBO J. 29, 3408–3420. doi: 10.1038/emboj.2010.211
Kurochkin, I. V., and Goto, S. (1994). Alzheimer's beta-amyloid peptide specifically interacts with and is degraded by insulin degrading enzyme. FEBS Lett. 345, 33–37. doi: 10.1016/0014-5793(94)00387-4
Ladiwala, A. R., Dordick, J. S., and Tessier, P. M. (2011). Aromatic small molecules remodel toxic soluble oligomers of amyloid beta through three independent pathways. J. Biol. Chem. 286, 3209–3218. doi: 10.1074/jbc.M110.173856
Lane, C. A., Hardy, J., and Schott, J. M. (2018). Alzheimer's disease. Eur. J. Neurol. 25, 59–70. doi: 10.1111/ene.13439
Lee, Y. H., Lin, Y., Cox, S. J., Kinoshita, M., Sahoo, B. R., Ivanova, M., et al. (2019). Zinc boosts EGCG's hIAPP amyloid Inhibition both in solution and membrane. Biochim. Biophys. Acta Proteins Proteom. 1867, 529–536. doi: 10.1016/j.bbapap.2018.11.006
Leszek, J., Trypka, E., Tarasov, V. V., Ashraf, G. M., and Aliev, G. (2017). Type 3 diabetes mellitus: a novel implication of Alzheimers disease. Curr. Top. Med. Chem. 17, 1331–1335. doi: 10.2174/1568026617666170103163403
Li, W., and Huang, E. (2016). An update on type 2 diabetes mellitus as a risk factor for dementia. J. Alzheimers Dis. 53, 393–402. doi: 10.3233/JAD-160114
Li, W., Wang, T., and Xiao, S. (2016a). Type 2 diabetes mellitus might be a risk factor for mild cognitive impairment progressing to Alzheimer's disease. Neuropsychiatr. Dis. Treat. 12, 2489–2495. doi: 10.2147/NDT.S111298
Li, X., Wan, M., Gao, L., and Fang, W. (2016b). Mechanism of inhibition of human islet amyloid polypeptide-induced membrane damage by a small organic fluorogen. Sci. Rep. 6:21614. doi: 10.1038/srep21614
Li, Z. M., and Li, X. F. (2012). Functional coupling reactions of human amylin receptor and nicotinic acetylcholine receptors in rat brain neurons. Acta Psychol. Sin. 64, 69–74.
Liberini, C. G., Borner, T., Boyle, C. N., and Lutz, T. A. (2016). The satiating hormone amylin enhances neurogenesis in the area postrema of adult rats. Mol. Metab. 5, 834–843. doi: 10.1016/j.molmet.2016.06.015
Lim, Y. A., Rhein, V., Baysang, G., Meier, F., Poljak, A., Raftery, M. J., et al. (2010). Abeta and human amylin share a common toxicity pathway via mitochondrial dysfunction. Proteomics 10, 1621–1633. doi: 10.1002/pmic.200900651
Liu, Y., Liu, F., Grundke-Iqbal, I., Iqbal, K., and Gong, C. X. (2011). Deficient brain insulin signalling pathway in Alzheimer's disease and diabetes. J. Pathol. 225, 54–62. doi: 10.1002/path.2912
Lolicato, F., Raudino, A., Milardi, D., and La Rosa, C. (2015). Resveratrol interferes with the aggregation of membrane-bound human-IAPP: a molecular dynamics study. Eur. J. Med. Chem. 92, 876–881. doi: 10.1016/j.ejmech.2015.01.047
López, L. C., Varea, O., Navarro, S., Carrodeguas, J. A., Sanchez de Groot, N., Ventura, S., et al. (2016). Benzbromarone, quercetin, and folic acid inhibit amylin aggregation. Int. J. Mol. Sci. 17, 964. doi: 10.3390/ijms17060964
Lorenzo, A., Razzaboni, B., Weir, G. C., and Yankner, B. A. (1994). Pancreatic islet cell toxicity of amylin associated with type-2 diabetes mellitus. Nature 368, 756–760. doi: 10.1038/368756a0
Lorenzo, A., and Yankner, B. A. (1996). Amyloid fibril toxicity in Alzheimer's disease and diabetes. Ann. N. Y. Acad. Sci. 777, 89–95. doi: 10.1111/j.1749-6632.1996.tb34406.x
Lutz, T. A. (2010). The role of amylin in the control of energy homeostasis. Am. J. Physiol. Regul. Integr. Comp. Physiol. 298, R1475–1484. doi: 10.1152/ajpregu.00703.2009
Lv, W., Zhang, J., Jiao, A., Wang, B., Chen, B., and Lin, J. (2019). Resveratrol attenuates hIAPP amyloid formation and restores the insulin secretion ability in hIAPP-INS1 cell line via enhancing autophagy. Can. J. Physiol. Pharmacol. 97, 82–89. doi: 10.1139/cjpp-2016-0686
Ly, H., Verma, N., Wu, F., Liu, M., Saatman, K. E., Nelson, P. T., et al. (2017). Brain microvascular injury and white matter disease provoked by diabetes-associated hyperamylinemia. Ann. Neurol. 82, 208–222. doi: 10.1002/ana.24992
Makin, S. (2018). The amyloid hypothesis on trial. Nature 559, S4–S7. doi: 10.1038/d41586-018-05719-4
Marambaud, P., Zhao, H., and Davies, P. (2005). Resveratrol promotes clearance of Alzheimer's disease amyloid-beta peptides. J. Biol. Chem. 280, 37377–37382. doi: 10.1074/jbc.M508246200
Martins, I. C., Kuperstein, I., Wilkinson, H., Maes, E., Vanbrabant, M., Jonckheere, W., et al. (2008). Lipids revert inert Abeta amyloid fibrils to neurotoxic protofibrils that affect learning in mice. EMBO J. 27, 224–233. doi: 10.1038/sj.emboj.7601953
Marzban, L., Rhodes, C. J., Steiner, D. F., Haataja, L., Halban, P. A., and Verchere, C. B. (2006). Impaired NH2-terminal processing of human proislet amyloid polypeptide by the prohormone convertase PC2 leads to amyloid formation and cell death. Diabetes 55, 2192–2201. doi: 10.2337/db05-1566
Masters, S. L., Dunne, A., Subramanian, S. L., Hull, R. L., Tannahill, G. M., Sharp, F. A., et al. (2010). Activation of the NLRP3 inflammasome by islet amyloid polypeptide provides a mechanism for enhanced IL-1β in type 2 diabetes. Nat. Immunol. 11, 897–904. doi: 10.1038/ni.1935
Maurer-Stroh, S., Debulpaep, M., Kuemmerer, N., Lopez de la Paz, M., Martins, I. C., Reumers, J., et al. (2010). Exploring the sequence determinants of amyloid structure using position-specific scoring matrices. Nat. Methods 7, 237–242. doi: 10.1038/nmeth.1432
McDermott, J. R., and Gibson, A. M. (1997). Degradation of Alzheimer's beta-amyloid protein by human and rat brain peptidases: involvement of insulin-degrading enzyme. Neurochem. Res. 22, 49–56. doi: 10.1023/A:1027325304203
Meng, F., Abedini, A., Plesner, A., Verchere, C. B., and Raleigh, D. P. (2010). The flavanol (-)-epigallocatechin 3-gallate inhibits amyloid formation by islet amyloid polypeptide, disaggregates amyloid fibrils, and protects cultured cells against IAPP-induced toxicity. Biochemistry 49, 8127–8133. doi: 10.1021/bi100939a
Messier, C., and Teutenberg, K. (2005). The role of insulin, insulin growth factor, and insulin-degrading enzyme in brain aging and Alzheimer's disease. Neural. Plast. 12, 311–328. doi: 10.1155/NP.2005.311
Mietlicki-Baase, E. G. (2018). Amylin in Alzheimer's disease: pathological peptide or potential treatment? Neuropharmacology 136(Pt B), 287–297. doi: 10.1016/j.neuropharm.2017.12.016
Mirhashemi, S. M. (2012). To evaluate likely antiamyloidogenic property of ferulic acid and baicalein against human islet amyloid polypeptide aggregation, in vitro study. Afr. J. Pharm. Pharmaco. 6, 671–676. doi: 10.5897/AJPP12.033
Mishra, R., Sellin, D., Radovan, D., Gohlke, A., and Winter, R. (2009). Inhibiting islet amyloid polypeptide fibril formation by the red wine compound resveratrol. Chembiochem 10, 445–449. doi: 10.1002/cbic.200800762
Moreno-Gonzalez, I., Edwards Iii, G., Salvadores, N., Shahnawaz, M., Diaz-Espinoza, R., and Soto, C. (2017). Molecular interaction between type 2 diabetes and Alzheimer's disease through cross-seeding of protein misfolding. Mol. Psychiatry 22, 1327–1334. doi: 10.1038/mp.2016.230
Morita, S., Sakagashira, S., Ueyama, M., Shimajiri, Y., Furuta, M., and Sanke, T. (2011). Progressive deterioration of insulin secretion in Japanese type2 diabetic patients in comparison with those who carry the S20G mutation of the islet amyloid polypeptide gene: a long-term follow-up study. J. Diabetes Investig. 2, 287–292. doi: 10.1111/j.2040-1124.2011.00102.x
Mukherjee, A., Morales-Scheihing, D., Salvadores, N., Moreno-Gonzalez, I., Gonzalez, C., Taylor-Presse, K., et al. (2017). Induction of IAPP amyloid deposition and associated diabetic abnormalities by a prion-like mechanism. J. Exp. Med. 214, 2591–2610. doi: 10.1084/jem.20161134
Mulder, H., Ahren, B., and Sundler, F. (1996). Islet amyloid polypeptide and insulin gene expression are regulated in parallel by glucose in vivo in rats. Am. J. Physiol. 271(6 Pt 1), E1008–E1014. doi: 10.1152/ajpendo.1996.271.6.E1008
Nedumpully-Govindan, P., Kakinen, A., Pilkington, E. H., Davis, T. P., Chun Ke, P., and Ding, F. (2016). Stabilizing Off-pathway oligomers by polyphenol nanoassemblies for iapp aggregation inhibition. Sci. Rep. 6:19463. doi: 10.1038/srep19463
Noor, H., Cao, P., and Raleigh, D. P. (2012). Morin hydrate inhibits amyloid formation by islet amyloid polypeptide and disaggregates amyloid fibers. Protein Sci. 21, 373–382. doi: 10.1002/pro.2023
Norwitz, N. G., Mota, A. S., Norwitz, S. G., and Clarke, K. (2019). Multi-Loop model of alzheimer disease: an integrated perspective on the Wnt/GSK3beta, alpha-synuclein, and type 3 diabetes hypotheses. Front. Aging Neurosci. 11:184. doi: 10.3389/fnagi.2019.00184
O'Nuallain, B., Williams, A. D., Westermark, P., and Wetzel, R. (2004). Seeding specificity in amyloid growth induced by heterologous fibrils. J. Biol. Chem. 279, 17490–17499. doi: 10.1074/jbc.M311300200
Oskarsson, M. E., Paulsson, J. F., Schultz, S. W., Ingelsson, M., Westermark, P., and Westermark, G. T. (2015). In vivo seeding and cross-seeding of localized amyloidosis: a molecular link between type 2 diabetes and Alzheimer disease. Am. J. Pathol. 185, 834–846. doi: 10.1016/j.ajpath.2014.11.016
Palhano, F. L., Lee, J., Grimster, N. P., and Kelly, J. W. (2013). Toward the molecular mechanism(s) by which EGCG treatment remodels mature amyloid fibrils. J. Am. Chem. Soc. 135, 7503–7510. doi: 10.1021/ja3115696
Panickar, K. S. (2013). Effects of dietary polyphenols on neuroregulatory factors and pathways that mediate food intake and energy regulation in obesity. Mol. Nutr. Food Res. 57, 34–47. doi: 10.1002/mnfr.201200431
Paulsson, J. F., Andersson, A., Westermark, P., and Westermark, G. T. (2006). Intracellular amyloid-like deposits contain unprocessed pro-islet amyloid polypeptide (proIAPP) in beta cells of transgenic mice overexpressing the gene for human IAPP and transplanted human islets. Diabetologia 49, 1237–1246. doi: 10.1007/s00125-006-0206-7
Pithadia, A., Brender, J. R., Fierke, C. A., and Ramamoorthy, A. (2016). Inhibition of IAPP aggregation and toxicity by natural products and derivatives. J. Diabetes Res. 2016:2046327. doi: 10.1155/2016/2046327
Poitout, V., and Robertson, R. P. (2002). Minireview: Secondary beta-cell failure in type 2 diabetes–a convergence of glucotoxicity and lipotoxicity. Endocrinology 143, 339–342. doi: 10.1210/endo.143.2.8623
Qiu, Q., Lin, X., Sun, L., Zhu, M. J., Wang, T., Wang, J. H., et al. (2019). Cognitive decline is related to high blood glucose levels in older Chinese adults with the ApoE ε3/ε3 genotype. Transl. Neurodegener. 8:12. doi: 10.1186/s40035-019-0151-2
Qiu, W. Q., Walsh, D. M., Ye, Z., Vekrellis, K., Zhang, J., Podlisny, M. B., et al. (1998). Insulin-degrading enzyme regulates extracellular levels of amyloid beta-protein by degradation. J. Biol. Chem. 273, 32730–32738. doi: 10.1074/jbc.273.49.32730
Radovan, D., Opitz, N., and Winter, R. (2009). Fluorescence microscopy studies on islet amyloid polypeptide fibrillation at heterogeneous and cellular membrane interfaces and its inhibition by resveratrol. FEBS Lett. 583, 1439–1445. doi: 10.1016/j.febslet.2009.03.059
Ramasamy, R., Yan, S. F., and Schmidt, A. M. (2011). Receptor for AGE (RAGE): signaling mechanisms in the pathogenesis of diabetes and its complications. Ann. N. Y. Acad. Sci. 1243, 88–102. doi: 10.1111/j.1749-6632.2011.06320.x
Ren, B., Liu, Y., Zhang, Y., Cai, Y., and Gong, X. (2018). Genistein: a dual inhibitor of both amyloid beta and human islet amylin peptides. ACS Chem. Neurosci. 9, 1215–1224. doi: 10.1021/acschemneuro.8b00039
Rezaei-Ghaleh, N., Andreetto, E., Yan, L. M., Kapurniotu, A., and Zweckstetter, M. (2011). Interaction between amyloid beta peptide and an aggregation blocker peptide mimicking islet amyloid polypeptide. PLoS ONE 6:e20289. doi: 10.1371/journal.pone.0020289
Rigacci, S., Guidotti, V., Bucciantini, M., Parri, M., Nediani, C., Cerbai, E., et al. (2010). Oleuropein aglycon prevents cytotoxic amyloid aggregation of human amylin. J. Nutr. Biochem. 21, 726–735. doi: 10.1016/j.jnutbio.2009.04.010
Rigacci, S., Miceli, C., Nediani, C., Berti, A., Cascella, R., Pantano, D., et al. (2015). Oleuropein aglycone induces autophagy via the AMPK/mTOR signalling pathway: a mechanistic insight. Oncotarget 6, 35344–35357. doi: 10.18632/oncotarget.6119
Rivera, E. J., Goldin, A., Fulmer, N., Tavares, R., Wands, J. R., and de la Monte, S. M. (2005). Insulin and insulin-like growth factor expression and function deteriorate with progression of Alzheimer's disease: link to brain reductions in acetylcholine. J. Alzheimers Dis. 8, 247–268. doi: 10.3233/JAD-2005-8304
Rivera, J. F., Gurlo, T., Daval, M., Huang, C. J., Matveyenko, A. V., Butler, P. C., et al. (2011). Human-IAPP disrupts the autophagy/lysosomal pathway in pancreatic β-cells: protective role of p62-positive cytoplasmic inclusions. Cell Death Differ. 18, 415–426. doi: 10.1038/cdd.2010.111
Sakagashira, S., Hiddinga, H. J., Tateishi, K., Sanke, T., Hanabusa, T., Nanjo, K., et al. (2000). S20G mutant amylin exhibits increased in vitro amyloidogenicity and increased intracellular cytotoxicity compared to wild-type amylin. Am. J. Pathol. 157, 2101–2109. doi: 10.1016/S0002-9440(10)64848-1
Sanke, T., Bell, G. I., Sample, C., Rubenstein, A. H., and Steiner, D. F. (1988). An islet amyloid peptide is derived from an 89-amino acid precursor by proteolytic processing. J. Biol. Chem. 263, 17243–17246.
Schubert, M., Brazil, D. P., Burks, D. J., Kushner, J. A., Ye, J., Flint, C. L., et al. (2003). Insulin receptor substrate-2 deficiency impairs brain growth and promotes tau phosphorylation. J. Neurosci. 23, 7084–7092. doi: 10.1523/JNEUROSCI.23-18-07084.2003
Schubert, M., Gautam, D., Surjo, D., Ueki, K., Baudler, S., Schubert, D., et al. (2004). Role for neuronal insulin resistance in neurodegenerative diseases. Proc. Natl. Acad. Sci. U.S.A 101, 3100–3105. doi: 10.1073/pnas.0308724101
Sciacca, M. F. M., Chillemi, R., Sciuto, S., Greco, V., Messineo, C., Kotler, S. A., et al. (2018). A blend of two resveratrol derivatives abolishes hIAPP amyloid growth and membrane damage. Biochim. Biophys. Acta Biomembr. 1860, 1793–1802. doi: 10.1016/j.bbamem.2018.03.012
Sequeira, I. R., and Poppitt, S. D. (2017). Unfolding novel mechanisms of polyphenol flavonoids for better glycaemic control: targeting pancreatic Islet Amyloid Polypeptide (IAPP). Nutrients 9:788. doi: 10.3390/nu9070788
Shoval, H., Weiner, L., Gazit, E., Levy, M., Pinchuk, I., and Lichtenberg, D. (2008). Polyphenol-induced dissociation of various amyloid fibrils results in a methionine-independent formation of ROS. Biochim. Biophys. Acta 1784, 1570–1577. doi: 10.1016/j.bbapap.2008.08.007
Silveira, A. C., Dias, J. P., Santos, V. M., Oliveira, P. F., Alves, M. G., Rato, L., et al. (2019). The action of polyphenols in diabetes mellitus and alzheimer's disease: a common agent for overlapping pathologies. Curr. Neuropharmacol. 17, 590–613. doi: 10.2174/1570159X16666180803162059
Sparks, S., Liu, G., Robbins, K. J., and Lazo, N. D. (2012). Curcumin modulates the self-assembly of the islet amyloid polypeptide by disassembling alpha-helix. Biochem. Biophys. Res. Commun. 422, 551–555. doi: 10.1016/j.bbrc.2012.05.013
Srodulski, S., Sharma, S., Bachstetter, A. B., Brelsfoard, J. M., Pascual, C., Xie, X. S., et al. (2014). Neuroinflammation and neurologic deficits in diabetes linked to brain accumulation of amylin. Mol. Neurodegener. 9:30. doi: 10.1186/1750-1326-9-30
Stumvoll, M., Goldstein, B. J., and van Haeften, T. W. (2005). Type 2 diabetes: principles of pathogenesis and therapy. Lancet 365, 1333–1346. doi: 10.1016/S0140-6736(05)61032-X
Suzuki, Y., Brender, J. R., Hartman, K., Ramamoorthy, A., and Marsh, E. N. G. (2012). Alternative pathways of human islet amyloid polypeptide aggregation distinguished by (19)f nuclear magnetic resonance-detected kinetics of monomer consumption. Biochemistry 51, 8154–8162. doi: 10.1021/bi3012548
Takeda, S., Sato, N., Uchio-Yamada, K., Sawada, K., Kunieda, T., Takeuchi, D., et al. (2010). Diabetes-accelerated memory dysfunction via cerebrovascular inflammation and Aβ deposition in an Alzheimer mouse model with diabetes. Proc. Natl. Acad. Sci. U.S.A. 107, 7036–7041. doi: 10.1073/pnas.1000645107
Talbot, K., Wang, H. Y., Kazi, H., Han, L. Y., Bakshi, K. P., Stucky, A., et al. (2012). Demonstrated brain insulin resistance in Alzheimer's disease patients is associated with IGF-1 resistance, IRS-1 dysregulation, and cognitive decline. J. Clin. Invest. 122, 1316–1338. doi: 10.1172/JCI59903
Tan, S. Y., Mei Wong, J. L., Sim, Y. J., Wong, S. S., Mohamed Elhassan, S. A., Tan, S. H., et al. (2019). Type 1 and 2 diabetes mellitus: a review on current treatment approach and gene therapy as potential intervention. Diabetes Metab. Syndr. 13, 364–372. doi: 10.1016/j.dsx.2018.10.008
Tu, L. H., Young, L. M., Wong, A. G., Ashcroft, A. E., Radford, S. E., and Raleigh, D. P. (2015). Mutational analysis of the ability of resveratrol to inhibit amyloid formation by islet amyloid polypeptide: critical evaluation of the importance of aromatic-inhibitor and histidine-inhibitor interactions. Biochemistry 54, 666–676. doi: 10.1021/bi501016r
Velander, P., Wu, L., Ray, W. K., Helm, R. F., and Xu, B. (2016). Amylin amyloid inhibition by flavonoid baicalein: key roles of its vicinal dihydroxyl groups of the catechol moiety. Biochemistry 55, 4255–4258. doi: 10.1021/acs.biochem.6b00578
Wang, Q., Guo, J., Jiao, P., Liu, H., and Yao, X. (2014). Exploring the influence of EGCG on the beta-sheet-rich oligomers of human islet amyloid polypeptide (hIAPP1-37) and identifying its possible binding sites from molecular dynamics simulation. PLoS ONE 9:e94796. doi: 10.1371/journal.pone.0094796
Wang, Q., Ning, L., Niu, Y., Liu, H., and Yao, X. (2015a). Molecular mechanism of the inhibition and remodeling of human islet amyloid polypeptide (hIAPP(1-37)) oligomer by resveratrol from molecular dynamics simulation. J. Phys. Chem. B 119, 15–24. doi: 10.1021/jp507529f
Wang, Q., Zhou, S., Wei, W., Yao, X., Liu, H., and Hu, Z. (2015b). Computational insights into the inhibition and destabilization of morin on the oligomer of full-length human islet amyloid polypeptide. Phys. Chem. Chem. Phys. 17, 29103–29112. doi: 10.1039/C5CP03991F
Westermark, G. T., Steiner, D. F., Gebre-Medhin, S., Engstrom, U., and Westermark, P. (2000). Pro islet amyloid polypeptide (ProIAPP) immunoreactivity in the islets of Langerhans. Ups J. Med. Sci. 105, 97–106. doi: 10.1517/03009734000000057
Westermark, P., Andersson, A., and Westermark, G. T. (2011). Islet amyloid polypeptide, islet amyloid, and diabetes mellitus. Physiol. Rev. 91, 795–826. doi: 10.1152/physrev.00042.2009
Westermark, P., and Grimelius, L. (1973). The pancreatic islet cells in insular amyloidosis in human diabetic and non-diabetic adults. Acta Pathol. Microbiol. Scand. A 81, 291–300. doi: 10.1111/j.1699-0463.1973.tb03538.x
Westwood, A. J., Beiser, A., Decarli, C., Harris, T. B., Chen, T. C., He, X.-M., et al. (2014). Insulin-like growth factor-1 and risk of Alzheimer dementia and brain atrophy. Neurology 82, 1613–1619. doi: 10.1212/WNL.0000000000000382
Wickelgren, I. (1998). Tracking insulin to the mind. Science 280, 517–519. doi: 10.1126/science.280.5363.517
Wijesekara, N., Ahrens, R., Sabale, M., Wu, L., Ha, K., Verdile, G., et al. (2017). Amyloid-beta and islet amyloid pathologies link Alzheimer's disease and type 2 diabetes in a transgenic model. FASEB J. 31, 5409–5418. doi: 10.1096/fj.201700431R
Wookey, P. J., Cao, Z., and Cooper, M. E. (1998). Interaction of the renal amylin and renin-angiotensin systems in animal models of diabetes and hypertension. Miner. Electrolyte Metab. 24, 389–399. doi: 10.1159/000057400
Wu, L., Velander, P., Liu, D., and Xu, B. (2017). Olive component oleuropein promotes beta-cell insulin secretion and protects beta-cells from amylin amyloid-induced cytotoxicity. Biochemistry 56, 5035–5039. doi: 10.1021/acs.biochem.7b00199
Xi, X. X., Sun, J., Chen, H. C., Chen, A. D., Gao, L. P., Yin, J., et al. (2019). High-fat diet increases amylin accumulation in the hippocampus and accelerates brain aging in hIAPP transgenic mice. Front. Aging Neurosci. 11:225. doi: 10.3389/fnagi.2019.00225
Xu, Z. X., Ma, G. L., Zhang, Q., Chen, C. H., He, Y. M., Xu, L. H., et al. (2017). Inhibitory mechanism of epigallocatechin gallate on fibrillation and aggregation of amidated human islet amyloid polypeptide. Chemphyschem 18, 1611–1619. doi: 10.1002/cphc.201700057
Xu, Z. X., Zhang, Q., Ma, G. L., Chen, C. H., He, Y. M., Xu, L. H., et al. (2016). Influence of aluminium and egcg on fibrillation and aggregation of human islet amyloid polypeptide. J. Diabetes Res. 2016:1867059. doi: 10.1155/2016/1867059
Yan, L. M., Velkova, A., and Kapurniotu, A. (2014). Molecular characterization of the hetero-assembly of β-amyloid peptide with islet amyloid polypeptide. Curr. Pharm. Des. 20, 1182–1191. doi: 10.2174/13816128113199990064
Yan, L. M., Velkova, A., Tatarek-Nossol, M., Andreetto, E., and Kapurniotu, A. (2007). IAPP mimic blocks Abeta cytotoxic self-assembly: cross-suppression of amyloid toxicity of Abeta and IAPP suggests a molecular link between Alzheimer's disease and type II diabetes. Angew. Chem. Int. Ed. Engl. 46, 1246–1252. doi: 10.1002/anie.200604056
Yang, J., Sun, Y., Xu, F., Liu, W., Hayashi, T., Hattori, S., et al. (2019a). Silibinin protects rat pancreatic β-cell through up-regulation of estrogen receptors' signaling against amylin- or Aβ1−42 -induced reactive oxygen species/reactive nitrogen species generation. Phytother. Res. 33, 998–1009. doi: 10.1002/ptr.6293
Yang, J., Sun, Y., Xu, F., Liu, W., Mai, Y., Hayashi, T., et al. (2019b). Silibinin ameliorates amylin-induced pancreatic beta-cell apoptosis partly via upregulation of GLP-1R/PKA pathway. Mol. Cell Biochem. 452, 83–94. doi: 10.1007/s11010-018-3414-9
Yang, Y., and Song, W. (2013). Molecular links between Alzheimer's disease and diabetes mellitus. Neuroscience 250, 140–150. doi: 10.1016/j.neuroscience.2013.07.009
Yonemoto, I. T., Kroon, G. J. A., Dyson, H. J., Balch, W. E., and Kelly, J. W. (2008). Amylin proprotein processing generates progressively more amyloidogenic peptides that initially sample the helical state. Biochemistry 47, 9900–9910. doi: 10.1021/bi800828u
Young, L. M., Cao, P., Raleigh, D. P., Ashcroft, A. E., and Radford, S. E. (2014a). Ion mobility spectrometry-mass spectrometry defines the oligomeric intermediates in amylin amyloid formation and the mode of action of inhibitors. J. Am. Chem. Soc. 136, 660–670. doi: 10.1021/ja406831n
Young, L. M., Saunders, J. C., Mahood, R. A., Revill, C. H., Foster, R. J., Tu, L.-H., et al. (2014b). Screening and classifying small-molecule inhibitors of amyloid formation using ion mobility spectrometry–mass spectrometry. Nat. Chem. 7, 73–81. doi: 10.1038/nchem.2129
Yu, X. L., Li, Y. N., Zhang, H., Su, Y. J., Zhou, W. W., Zhang, Z. P., et al. (2015). Rutin inhibits amylin-induced neurocytotoxicity and oxidative stress. Food Funct. 6, 3296–3306. doi: 10.1039/C5FO00500K
Zelus, C., Fox, A., Calciano, A., Faridian, B. S., Nogaj, L. A., and Moffet, D. A. (2012). Myricetin inhibits Islet Amyloid Polypeptide (IAPP) Aggregation and rescues living mammalian cells from IAPP toxicity. Open Biochem. J. 6, 66–70. doi: 10.2174/1874091X01206010066
Zhang, X.-X., Pan, Y.-H., Huang, Y.-M., and Zhao, H.-L. (2016). Neuroendocrine hormone amylin in diabetes. World J. Diabetes 7, 189–197. doi: 10.4239/wjd.v7.i9.189
Zhao, H. L., Sui, Y., Guan, J., He, L., Gu, X. M., Wong, H. K., et al. (2009). Amyloid oligomers in diabetic and nondiabetic human pancreas. Transl. Res. 153, 24–32. doi: 10.1016/j.trsl.2008.10.009
Zhao, W. Q., and Alkon, D. L. (2001). Role of insulin and insulin receptor in learning and memory. Mol. Cell Endocrinol. 177, 125–134. doi: 10.1016/S0303-7207(01)00455-5
Keywords: Aß-42, Alzheimer's disease, amylin, diabetes, IAPP, protein aggregation
Citation: Raimundo AF, Ferreira S, Martins IC and Menezes R (2020) Islet Amyloid Polypeptide: A Partner in Crime With Aβ in the Pathology of Alzheimer's Disease. Front. Mol. Neurosci. 13:35. doi: 10.3389/fnmol.2020.00035
Received: 21 November 2019; Accepted: 20 February 2020;
Published: 20 March 2020.
Edited by:
Maria Rosário Almeida, University of Porto, PortugalReviewed by:
Fernando Peña-Ortega, National Autonomous University of Mexico, MexicoCong Liu, University of Chinese Academy of Sciences, China
Copyright © 2020 Raimundo, Ferreira, Martins and Menezes. This is an open-access article distributed under the terms of the Creative Commons Attribution License (CC BY). The use, distribution or reproduction in other forums is permitted, provided the original author(s) and the copyright owner(s) are credited and that the original publication in this journal is cited, in accordance with accepted academic practice. No use, distribution or reproduction is permitted which does not comply with these terms.
*Correspondence: Ivo C. Martins, aXZvbWFydGluc0BtZWRpY2luYS51bGlzYm9hLnB0; Regina Menezes, cm1lbmV6ZXNAaWJldC5wdA==; cmVnaW5hLm1lbmV6ZXNAbm1zLnVubC5wdA==
†These authors have contributed equally to this work