- 1Department of Gerontology and Geriatrics, Shengjing Hospital of China Medical University, Shenyang, China
- 2Department of Otolaryngology Head and Neck Surgery, Shengjing Hospital of China Medical University, Shenyang, China
Neurodegenerative diseases (NDDs), including Alzheimer’s disease (AD), Parkinson’s disease (PD), Huntington’s disease (HD), and amyotrophic lateral sclerosis (ALS), are progressive and ultimately fatal. NDD onset is influenced by several factors including heredity and environmental cues. Long noncoding RNAs (lncRNAs) are a class of noncoding RNA molecules with: (i) lengths greater than 200 nucleotides, (ii) diverse biological functions, and (iii) highly conserved structures. They directly interact with molecules such as proteins and microRNAs and subsequently regulate the expression of their targets at the genetic, transcriptional, and post-transcriptional levels. Emerging studies indicate the important roles of lncRNAs in the progression of neurological diseases including NDDs. Additionally, improvements in detection technologies have enabled quantitative lncRNA detection and application to circulating fluids in clinical settings. Here, we review current research on lncRNAs in animal models and patients with NDDs. We also discuss the potential applicability of circulating lncRNAs as biomarkers in NDD diagnostics and prognostics. In the future, a better understanding of the roles of lncRNAs in NDDs will be essential to exploit these new therapeutic targets and improve noninvasive diagnostic methods for diseases.
Introduction
Neurodegenerative diseases (NDDs) are characterized by the loss of neuronal cells in the nervous system (Rittiner et al., 2020). Common NDDs include Alzheimer’s disease (AD), Parkinson’s disease (PD), Huntington’s disease (HD), and amyotrophic lateral sclerosis (ALS; Ang et al., 2010). Currently, no effective treatments are available for NDDs, thus posing a heavy economic burden on society, families, and individuals, and severely affecting the lifestyle and health of patients and caregivers (Liao et al., 2019). Studies have identified a variety of pathophysiological mechanisms involved in NDD pathogenesis, including oxidative stress, mitochondrial dysfunction (Lin and Beal, 2006), excitotoxic amino acids (Garcez et al., 2019), and inflammatory reactions (Chen et al., 2016). Despite decades of research, the causes of NDDs remain unknown. In recent years, noncoding RNA (ncRNA) has gained importance as a major research direction in the study of NDD pathogenesis (Riva et al., 2016). In patients with AD compared with healthy controls, the antisense transcription of BACE1 is up-regulated, thus leading to increased BACE1 protein expression. Similarly, overexpressed BACE1 increases levels of Aβ peptide, the main component of amyloid plaques (Koelsch, 2017). In other research, RNA sequencing from white blood cells has shown lower expression of 13 lncRNAs in patients with PD than in healthy controls, thereby providing an experimental basis for further lncRNA research on PD pathogenesis (Soreq et al., 2014). Although the roles of ncRNAs in NDDs are difficult to fully elucidate, studies are increasingly showing that ncRNAs play important roles in both normal and pathological neurobiology (Maoz et al., 2017; Wu and Kuo, 2020).
Currently, commercially viable biomarkers for wide use in the early diagnosis of NDDs in clinical practice are lacking. In addition, although many peripheral blood biomarkers for predicting disease prognosis, treatment, and outcome have been evaluated at different disease stages, they have not yet entered clinical trials for evaluation. In recent years, circulating Aβ42 and Aβ40, α-synuclein (α-syn), transactive response DNA-binding protein of 43 (TDP-43), and other body fluid biomarkers from the blood or urine have emerged (Atik et al., 2016; Feneberg et al., 2018; Palmqvist et al., 2019); however, they remain far from clinical application.
lncRNAs generally refer to ncRNA transcripts >200 nucleotides (nt) in length. Researchers identified these noncoding sequences as early as the 1970 s. According to the prevailing understanding of evolutionary biology at the time, lncRNAs were predicted to have no biological function and were therefore deemed “junk DNA.” However, as research advanced, studies increasingly suggested that this “junk DNA” has important biological functions (Chen Y. et al., 2019). In fact, lncRNAs exert their biological functions by acting as signaling molecules, protein complex scaffolds, and/or molecular decoys to enhance gene transcription (Yang et al., 2019). Similarly, lncRNAs play important roles in the development and differentiation of the central nervous system (CNS). Deep sequencing data from tissues and cells have indicated that lncRNAs have stronger cell and tissue specificity than coding RNAs (Kozomara and Griffiths-Jones, 2014). A recent brain development study using RNA-seq, chromatin immunoprecipitation-sequencing, and lncRNA and mRNA cluster analysis, has observed that lncRNA is more tissue-specific than mRNA (Ramos et al., 2013). In addition, the number of lncRNAs far exceeds that of coding genes, thus potentially enabling them to serve as disease diagnostic and prognostic biomarkers.
Studies investigating the involvement of ncRNAs in NDD pathogenesis have primarily focused on microRNAs (miRNAs), whereas the exact lncRNA mechanisms involved in NDD pathogenesis remain unclear (Riva et al., 2016; Haque et al., 2020). In addition, many studies have focused on circulating miRNAs as NDD biomarkers (Roser et al., 2018), but lncRNAs have not been fully evaluated. Compared with lncRNAs, miRNAs have been more widely studied as diagnostic biomarkers for NDDs (Kou et al., 2020; Zhao Y. et al., 2020), and several meta-analyses have suggested that miRNAs have clinical practical value as biomarkers for the diagnosis of neoplastic diseases (Zhou et al., 2018; Jayaraj et al., 2019). The stable presence of circulating miRNA in the serum, particularly after the elucidation of exosomes, has enriched the understanding and detection of circulating miRNA (Cha et al., 2019; Chen J. J. et al., 2019). Many databases for miRNA research are available, and studies on the mechanisms of miRNA involvement in NDDs are updated in a timely manner. In contrast, few clinical studies, particularly well-designed controlled clinical studies, have been conducted on lncRNAs; therefore, such research remains in the preliminary stages. Additionally, the slow updating of public databases that can be used to retrieve lncRNAs has restricted research progress on lncRNAs in NDDs (Maracaja-Coutinho et al., 2019). However, lncRNA research has rapidly emerged and provided growing evidence of lncRNAs as potential circulating diagnostic biomarkers in recent years. Herein, we review the involvement of lncRNAs in NDD pathogenesis (Figure 1), analyze their applications as biomarkers in clinical practice and discuss the inclusion of lncRNAs as novel therapeutic strategies for various NDDs.
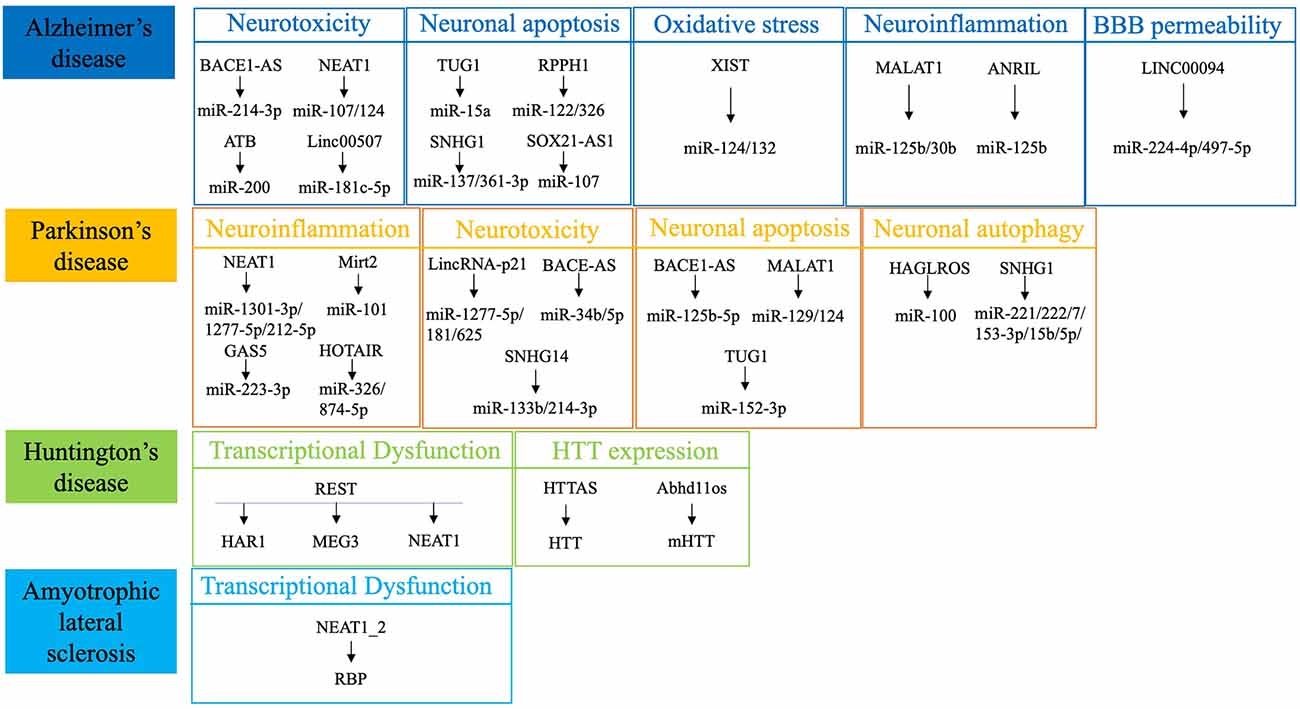
Figure 1. Summary of the most dysregulated lncRNAs in neurodegenerative diseases. REST, RE1-silencing transcription factor; HTT, Huntingtin; RBP, RNA-binding protein.
Overview and Classification of lncRNAs
lncRNAs are widely distributed in the cytoplasm and nucleus and are mainly derived from protein coding gene interruption, chromatin recombination, noncoding gene replication reverse transposition products, adjacent fragment duplication within noncoding RNA, and insertion of transposable factors into genes (Yang et al., 2017). Most lncRNAs are transcribed by RNA polymerase II and processed by splicing, 5′ capping, and 3′ polyadenylation (Xing and Chen, 2018). Studies have shown that lncRNA expression exhibited tissue, temporal and spatial specificity; i.e., lncRNAs were differentially expressed among tissues, and their expression levels varied among developmental stages in the same tissue or organ (Liu et al., 2018). With the increase in interest in lncRNA research, several functions have been attributed to these molecules, including roles in gene transcription, splicing, protein translation, protein localization, stem cell pluripotency, and cell structural integrity (Engreitz et al., 2016). Depending on the relationship between lncRNAs and protein coding genes, these lncRNAs can be divided into intergenic, antisense, intronic, sense, and bidirectional categories. Similarly, depending on their implicated roles in molecular mechanisms, these lncRNAs can be divided into signal, decoy, guide, and scaffold molecules (Lee, 2012).
How lncRNAs are produced is unclear, but they have been suggested to have arisen through the following mechanisms: (i) in early evolutionary processes, the open reading frame of a protein-coding gene becomes mutated and gene structure is destroyed; (ii) after chromatin rearrangement, two un-transcribed and distant sequences merge and produce a multi-exon lncRNA; (iii) noncoding genes are produced by reverse translocation; (iv) two consecutive repetitive events generate lncRNAs with repetitive sequences inside ncRNAs; and (v) transposable components insert into genes and produce lncRNAs. Most lncRNAs exhibit specific tissue and cell expression during tissue differentiation and development and similarly have characteristic expression patterns in CNS diseases (Ransohoff et al., 2018; Sarropoulos et al., 2019).
lncRNA Regulatory Mechanisms
lncRNAs are typically involved in cell function regulation as secondary or tertiary structures in functions such as DNA replication, RNA transcription, protein translation, cell proliferation, and differentiation (Robinson et al., 2020). The structural characteristics of lncRNAs facilitate their complex and precise regulatory roles in gene expression, with functions such as epigenetic phenomena including gene silencing, DNA methylation, histone modification, dormant transposon activation, RNA editing, and gene methylation (Johnsson et al., 2014). As new epigenetic regulatory molecules, lncRNAs have been implicated in epigenetic regulation (Heo et al., 2013). Gene transcription is a highly complex biological process, and lncRNAs appear to regulate gene transcription by mimicking DNA elements, competitively binding transcription factors, or conducting variable splicing (Wang et al., 2008). During gene expression, post-transcriptional gene regulation requires a series of post-transcriptional processes including gene processing, modification, and regulation, through incorporating RNA splicing, processing, splicing, maturation, metabolism, and stability regulation (Filipowicz et al., 2005). In an important step in mRNA processing and metabolism, the shearing of mRNA precursors is often regulated by lncRNAs.
Several studies have proposed that lncRNAs have pathophysiological roles in regulating miRNA expression (Huang, 2018). Because of the unique length characteristics of lncRNA molecules, miRNA recognition action sites have been identified along lncRNAs. These sites specifically bind miRNAs, thereby affecting in vivo lncRNA stability, mediating lncRNA degradation, and regulating cell biological functions (Huang, 2018). In addition, beyond acting as protein bait molecules that regulate target gene expression, lncRNAs also act as bait molecules for miRNAs: they adsorb miRNAs via target mimicry and inhibit further effects on target molecules. Moreover, lncRNAs may be used as molecular bait to indirectly regulate target gene expression by suppressing miRNA effects on their targets. lncRNAs also competitively bind mRNA to miRNA, thus directly regulating target gene expression (Wang et al., 2020a).
NDD-Related lncRNAs
AD-Related lncRNAs
AD is an NDD characterized by progressive cognitive decline and memory loss. Characteristic pathological manifestations include senile plaques, intracellular neurofibrillary tangles, and extracellular Aβ deposition. The disease etiology and pathogenesis have yet to be elucidated (Fernandez et al., 2019). In recent years, several genomic studies have shown that lncRNA expression was closely associated with AD pathogenesis (Idda et al., 2018).
Aβ is a polypeptide containing 39–43 amino acids, which is produced by proteolysis of the amyloid precursor protein (APP) via β- and γ-secretases (Zhou R. et al., 2020). Aβ deposition not only is associated with neuronal degeneration but also activates a series of pathological events. The polypeptide is the main cause of neuronal degeneration and cell death in AD brains (Sevigny et al., 2016). Faghihi et al. have shown that the antisense transcript of β-secretase (BACE1), i.e., lncRNA BACE1-AS, increased Aβ1–42 expression in patients with AD through a feed-forward regulatory mechanism (Table 1). BACE1-AS levels are elevated in patients with AD and in APP transgenic mice, thus suggesting that BACE1-AS levels may serve as a potential diagnostic biomarker and therapeutic target for AD (Faghihi et al., 2008). Liu et al. have constructed an AD senile plaque model by examining neuroblastoma cells (SH-SY5Y) with synthesized Aβ1–42, and down-regulating lncRNA BACE1-AS through siRNA silencing. The ability of BACE1 to shear APP and produce Aβ1–42 decreased, thus suggesting that lncRNA BACE1-AS is an important factor in Aβ1–42 formation (Liu et al., 2014). In addition, in vivo BACE1-AS knockdown mediated by lentiviral expression has been found to improve the memory and learning ability of SAMP8 mice, inhibit BACE1 and APP production, and prevent phosphorylation of the tau protein in mouse hippocampi (Zhang W. et al., 2018). He et al. have shown that BACE1-AS was up-regulated and miR-214-3p was down-regulated in the plasma from patients with AD as well as in SK-N-SH cells treated with Aβ. Additionally, BACE1-AS acts as a sponge for miR-214-3p, and miR-214-3p silencing reverses the effects of BACE1-AS knockdown on isoflurane-mediated apoptosis in Aβ-induced SK-N-SH cells. These data suggest that BACE1-AS aggravates isoflurane-induced neurotoxicity in AD via miR-214-3p sponging (He et al., 2020).
Nuclear paraspeckle assembly transcript 1 (NEAT1) is a widely expressed lncRNA in a variety of mammalian cell types (Wang et al., 2020b). Ke et al. have shown that NEAT1 expression was elevated in Aβ-induced SH-SY5Y neuroblastoma cells, whereas NEAT1 knockdown attenuated the Aβ-induced inhibition of cell viability, apoptosis promotion, and p-Tau levels. MiR-107 abundance is diminished in Aβ-treated SH-SY5Y neuroblastoma cells, and NEAT1 overexpression reverses Aβ-induced injury. Furthermore, NEAT1 acts as a decoy for miR-107, and NEAT1 aggravates Aβ-induced neuronal injury by sponging miR-107, thus suggesting a novel strategy for AD treatment (Ke et al., 2019). Spreafico et al. have observed that NEAT1 expression levels were elevated in the temporal cortex and hippocampus in patients with AD, and have further observed a strong positive correlation between NEAT1 expression and cyclin-dependent kinase 5 regulatory subunit 1 (CDK5R1), which was closely associated with AD onset and progression. Moreover, NEAT1 has a neuroprotective role in AD by compensating for increased CDK5R1 levels by sponging the miR-15/107 family (Spreafico et al., 2018). Zhao et al. (2019) have confirmed that NEAT1 was considerably up-regulated in an AD mouse model and identified a reversible regulatory relationship with miR-124. NEAT1 knockdown, or miR-124 over-expression has been found to elicit protective effects in a cellular AD model induced by Aβ. Moreover, NEAT1 has been suggested to function as a regulatory factor promoting AD development by modulating the miR-124/BACE1 axis; this pathway may be a potential novel therapeutic target for AD treatment (Zhao et al., 2019). In addition, NEAT1 interacts with NEDD4L and consequently promotes PTEN-induced putative kinase 1 (PINK1) ubiquitination and degradation, thus resulting in PINK1-dependent autophagy (Huang et al., 2020).
Expression of the lncRNA MEG3 has been found to be down-regulated in an Aβ25–35 induced AD rat model by Yi et al., who have suggested that MEG3 up-regulation improved memory and spatial learning abilities, decreased Aβ deposition in the hippocampus, and mitigated oxidative stress and inflammatory injury by inactivating the phosphatidylinositol 3-kinase and protein kinase B (PI3K/AKT) pathway in this model (Yi et al., 2019).
The lncRNA BC1 has been shown to be overexpressed in the brains of AD model mice. Exogenous BC1 expression in excitatory pyramidal neurons induces Aβ peptide accumulation, spatial learning, and memory impairments in these mice. Moreover, BC1 induces APP mRNA translation via its association with the Fragile X syndrome protein (FMRP). Thus, inhibiting the association of BC1 or BC1-FMRP in an AD mouse model decreases Aβ accumulation in the brain, and prevents spatial learning and memory loss (Zhang T. et al., 2018).
Massone et al. (2011) have described the novel lncRNA 17A, which was stably expressed in SH-SY5Y neuroblastoma cells, and was also expressed in the human brain and up-regulated in cerebral tissue from patients with AD. Moreover, 17A expression in neuroblastoma cells enhances Aβ secretion and Aβ x-42/Aβ x-40 peptide ratios by inducing responses to inflammatory stimuli.
Neuroinflammation plays a major role in AD (Calsolaro and Edison, 2016). MALAT1 expression has been found to be elevated in a PC12 cell line AD model. MALAT1 overexpression inhibits neuronal apoptosis, decreases IL-6 and TNF-α levels, promotes neurite outgrowth, and increases IL-10 level by sponging miR-125b; therefore, MALAT1 may have a neuroprotective role against AD (Ma et al., 2019). Li L. et al. (2020) have demonstrated that MALAT1 served as a sponge for mR-30b, thereby resulting in up-regulation of cannabinoid receptor 1 (CNR1) expression. Moreover, phosphorylation of PI3K and AKT was stimulated when MALAT1 or CNR1 is over-expressed. In addition, MALAT1 has been found to promote neuronal recovery after AD onset in a rat model, via the miR-30b/CNR1 network and PI3K/AKT signaling activation.
The lncRNA at the INK4 locus (lnc-ANRIL) has been identified in several neurological diseases with pathologies associated with inflammation and neural dysfunction (Zhou B. et al., 2020). In Aβ1–42 assaulted nerve growth factor (NGF)-stimulated PC12 cells, lnc-ANRIL is up-regulated, whereas lnc-ANRIL knockdown inhibits inflammatory cytokine expression and increases neurite outgrowth by regulating miR-125a expression (Zhou B. et al., 2020).
Oxidative stress has also been implicated in AD pathogenesis and progression (Tobore, 2019). Yue et al. have demonstrated that the expression of the lncRNA XIST was significantly up-regulated in in vivo and in vitro AD models. The silencing of lncRNA XIST negatively regulates miR-124 and positively regulates BACE1 expression in N2a cells, thus suggesting that lncRNA XIST may be a new potential target for AD treatment (Yue et al., 2020). Additionally, Wang X. et al. (2018) have shown that lncRNA XIST knockdown alleviated Aβ25–35-induced oxidative stress, toxicity, and apoptosis in primary cultured rat hippocampal neurons via miR-132 up-regulation. These observations underpin the potential of manipulating lncRNA XIST in AD treatment.
Cai et al. (2017) have revealed that the lncRNA RPPH1 was up-regulated in an AD mouse model, thereby up-regulating CDC42 expression and promoting hippocampal neuron dendritic spine formation. Gu et al. (2020b) have demonstrated that both lncRNA RPPH1 and miR-122 were up-regulated in an AD mouse model, and that lncRNA RPPH1 activated Wnt/β-catenin signaling, thus ameliorating Aβ induced neuronal apoptosis in SK-N-SH cells via direct targeting of miR-122. In addition, the overexpression of lncRNA RPPH1 down-regulates the endoplasmic reticulum stress (ERS) related proteins CHOP and GRP78, as well as cleaved caspase 12. Moreover, lncRNA RPPH1 directly targets miR-326 and counteracts its inhibitory effects on PKM2 expression, thereby attenuating the ERS status and apoptosis induced by Aβ25–35 (Gu et al., 2020a).
Other lncRNAs have been implicated in AD pathogenesis via several molecular pathways. The lncRNA-ATB was the first lncRNA found to be activated by transforming growth factor-β (TGF- β) (Saito et al., 2015). lncRNA-ATB promotes epithelial-mesenchymal transformation in gastric cancer by regulating TGF-β and the miR-200 family (Saito et al., 2015). In Aβ25–35-induced PC12 cells, and the cerebrospinal fluid (CSF) and serum from patients with AD, lncRNA-ATB expression levels are significantly elevated (Wang J. et al., 2018). Furthermore, Wang J. et al. (2018) have indicated that the suppression of lncRNA-ATB protected PC12 cells against Aβ25–35-induced neurotoxicity via regulation of the miR-200/ZNF217 axis, thereby providing new insights for AD prevention.
The lncRNA EBF3-AS is abundantly expressed in the brain and has been reported to be dysregulated in an AD mouse model (Gu et al., 2018). Gu et al. have shown that lncRNA EBF3-AS expression was up-regulated in the hippocampus in APP/PS1 mice. Knockdown of lncRNA EBF3-AS by siRNA inhibits Aβ25–35 induced apoptosis, thus suggesting that lncRNA EBF3-AS promotes AD neuronal apoptosis and indicating its potential as a new therapeutic target for AD treatment (Gu et al., 2018).
Inhibition of BC200, a highly expressed lncRNA in AD, may be an effective method for AD therapy (Li et al., 2018). Li et al. (2018) have established an AD cell model with overexpression of Aβ1–42 to investigate the effects of BC200 on cell viability and apoptosis. They have observed that BC200 knockdown suppressed BACE1 expression, thus suggesting that this may be a putative target for AD therapeutics.
The lncRNA SNHG1 has been reported to show increased expression in SH-SY5Y and human primary neuronal (HPN) cells, and lncRNA SNHG1 knockdown partially reverses the effects of Aβ25–35 treatment on apoptosis, cell viability, and caspase-3 activity (Wang et al., 2019). In addition, SNHG1 appears to function as a competing endogenous RNA (ceRNA) for miR-137, which negatively regulates kringle containing transmembrane protein 1 (KREMEN1) expression by targeting its 3′ untranslated region. Wang et al. (2019) have indicated that SNHG1 knockdown exerted neuronal protective effects by repressing KRENEN1 and by acting as a ceRNA for miR-137, in an in vitro AD cell model. Gao et al. (2020) have also suggested that SNHG1 expression was up-regulated in an in vitro AD cell model. Thus, SNHG1 may promote cell injury by regulating the miR-361-3p/ZNF217 axis; this knowledge may provide a theoretical basis for AD molecular therapy.
The lncRNA taurine up-regulated gene 1 (TUG1) was first recognized in 2005 as an important element in retinal development in rodents. This lncRNA has also been shown to participate in oncogenic processes by sponging miRNAs (Li et al., 2016). Li X. et al. (2020) have observed that TUG1 expression was up-regulated in an AD mouse model, whereas TUG1 knockdown improved memory and spatial learning ability, decreased pathological neuronal injury, and inhibited neuronal apoptosis by elevating miR-15a levels and suppressing Rho-associated kinase 1 (ROCK1) expression in an AD mouse model.
Linc00507 is significantly elevated in the brain in APP/PS transgenic mice and in SH-SY5Y cells (Yan et al., 2020). It directly binds miR-181c-5p and regulates the expression of microtubule-associated protein Tau (MAPT) and tau-tubulin kinase-1 (TTBK1) as a ceRNA. In addition, linc00507 mediates tau protein hyperphosphorylation by activating the P25/P35/GSK3β signaling pathway sponging miR-181c-5p (Yan et al., 2020).
IAβ1–42-treated SH-SY5Y cells show increased expression of the lncRNA SOX21 antisense RNA1 (SOX21-AS1) and decreased expression of miR-107 (Xu et al., 2020a). SOX21-AS1 acts as a sponge for miR-107, whereas silenced SOX21-AS1 attenuates Aβ1–42, mediated p-Tau levels, and SH-SY5Y cell viability by sponging miR-107, thus suggesting a possible role in AD therapeutics (Xu et al., 2020a).
Expression of the lncRNA brain-derived neurotrophic factor (BDNF)-AS is increased by Aβ25–35-induced neurotoxicity in PC12 cells, thus inhibiting BDNF expression and inducing apoptosis in PC12 cells (Guo et al., 2018). Furthermore, silenced lncRNA BDNF-AS up-regulates BDNF expression and cell viability, inhibits apoptosis, and exerts protective functions in Aβ25–35-induced neurotoxicity in PC12 cells (Guo et al., 2018).
Zhu et al. (2019) have shown that linc00094 increased in Aβ1–42 co-incubated with microvascular endothelial cells in an in vitro blood-brain barrier model. Silencing of linc00094 inhibits endophilin-1 expression by up-regulating miR-224-4p/miR-497-5p, increasing ZO-1, occludin, and claudin-5 expression, and ultimately alleviating blood-brain barrier permeability in an AD microenvironment. These data suggest that the linc00094/miR-224–5p (miR-497-5p)/endophilin-1 axis may have a crucial role in regulating blood-brain barrier permeability in the AD microenvironment (Zhu et al., 2019).
PD-Associated lncRNAs
PD, a common NDD caused by the gradual loss of dopaminergic neurons in the substantia nigra striatum of the midbrain, is characterized by static tremors, dyskinesia, and an abnormal postural gait. In severe cases, dementia appears, but the exact cause remains unknown.
Autophagy has been shown to be associated with the central mechanisms underlying PD. In a recent study, lncRNA SNHG1 has been reported to be significantly elevated in PD (Qian et al., 2019). The authors have demonstrated that lncRNA SNHG1 knockdown promoted autophagy and prevents 1-methyl-4-phenylpyridinium (MPP+)-induced cell death, whereas SNHG1 down-regulation further attenuated MPP+-induced decreases in LC3-II levels and cytotoxicity via the miR-221/222/p27/mTOR pathway. Over-expression of the lncRNA SNHG1 also aggravates MPP+-induced cytotoxicity in SH-SY5Y cells by regulating PTEN/AKT/mTOR signaling via miR-153–3p sponging. These data suggest that SNHG1 may serve as a therapeutic target for PD neuroprotection and treatment (Qian et al., 2019; Zhao et al., 2020a).
NEAT1 is closely associated with pathological changes in the CNS. Yan et al. have observed that NEAT1 expression was elevated in a PD mouse model (Yan et al., 2018). Moreover, NEAT1 positively regulates PINK1 protein levels by inhibiting degradation of the PINK1 protein, whereas NEAT1 knockdown effectively suppresses in vivo 1-methyl-4-phenyl-1,2,3, 6-tetrahydropyridine (MPTP)-induced autophagy, thus alleviating dopaminergic neuronal injury. These data indicate that NEAT1 promotes MPTP-induced autophagy in PD via PINK1 stabilization (Yan et al., 2018). NEAT1 down-regulation also decreases α-syn-induced activation of NLRP3 inflammation by inhibiting GJB1 expression via miR-1301-3p sponging (Sun et al., 2020). Additionally, up-regulated NEAT1 appears to contribute to MPP+-induced neuronal injury via the NEAT1-miR-1277-5p-ARHGAP26 ceRNA pathway (Zhou et al., 2020a). NEAT1 knockdown also suppresses MPP+-induced inflammation, apoptosis, and cytotoxicity in SK-N-SH cells by regulating both miR-212-5p and RAB3IP expression. These data suggest a possible therapeutic strategy based on NEAT1 in patients with PD (Liu et al., 2020).
HAGLROS expression has been found to be elevated in an MPTP-induced PD mouse model and in MPP+-induced SH-SY5Y cells (Peng et al., 2019). HAGLROS regulates autophagy and apoptosis in MPP+ induced SH-SY5Y cells by sponging miR-100, whereas up-regulated HAGLROS appears to contribute to PD development by inhibiting apoptosis and autophagy, effects that might be achieved through regulation of the miR100/ATG10 axis and PI3K/AKT/mTOR pathway (Peng et al., 2019).
Fan et al. (2020) have shown that the lncRNA BDNF-AS was also dysregulated in PD. lncRNA BDNF-AS was up-regulated in both in vivo and vitro PD models. In addition, lncRNA BDNF-AS knockdown has been found to elevate SH-SY5Y cell viability, and inhibit autophagy and apoptosis in an MPTP-induced PD model by regulating miR-125b-5p, thus suggesting that lncRNA BDNF-AS may serve as a potential therapeutic target for PD.
Pathological changes in PD are mediated by the formation of Lewy bodies (LB). Because α-syn is the main component of LB, α-syn alterations may affect PD pathogenesis. SNHG14 expression is elevated in the brain tissue in PD mice (Zhang et al., 2019). Moreover, miR-133b down-regulates α-syn expression by targeting its mRNA 3′ untranslated region, whereas SNHG14 has a reversible regulatory relationship with miR-133b. The authors have also demonstrated that SNHG14 silencing mitigated dopaminergic neuron injury by down-regulating α-syn through targeting miR-133b, thus potentially ameliorating PD symptoms (Zhang et al., 2019). Additionally, SNHG14 knockdown appeared to protect SK-N-SH cells against MPP+-induced cytotoxicity through up-regulation of miR-214-3p. KLF4 is a direct target of miR-214-3p, and SNHG14 appears to regulate KLF4 expression by acting as a miR-214-3p sponge and therefore may be a promising target for PD intervention (Zhou et al., 2020b).
The lincRNA-p21 has been reported to be elevated in PD. Up-regulated lincRNA-p21 sponges miR-1277-5p and indirectly increases α-syn expression, thus suppressing SH-SY5Y viability and activating apoptosis in these cells. These data suggest that lincRNA-p21 may serve as a novel target for PD (Xu et al., 2018). In addition, lincRNA-p21 competitively binds the miR-181 family and induces microglial activation via the miR-181/PKC-δ pathway (Ye et al., 2018). Moreover, lincRNA-p21 inhibits MPP+-induced neuronal injury by sponging miR-625 and up-regulating TRPM2 in SH-SY5Y cells. These data have increased the understanding of PD pathogenesis (Ding et al., 2019).
UCA1 may also regulate PD progression by modulating α-syn expression (Lu et al., 2018). UCA1 and α-syn are highly expressed in brain tissue in a PD mouse model and in MPP+-induced SH-SY5Y cells. UCA1 overexpression considerably up-regulates the mRNA and protein expression of α-syn, whereas UCA1 knockdown decreases caspase-3 activity and MPP+-induced neuronal apoptosis in SH-SY5Y cells (Lu et al., 2018). Similarly, Cai et al. have shown that UCA1 down-regulation increased dopamine levels, inhibited apoptosis and oxidative stress in substantia nigra neurons, and decreased neuroinflammatory responses in a rat model of PDs. Furthermore, UCA1 down-regulation inhibits the activation of the PI3K/AKT signaling pathway in the substantia nigra in a rat model of PD, thus suggesting a protective mechanism toward dopaminergic neurons in a rat PD model (Cai et al., 2019).
MALAT1 has also been implicated in PD pathogenesis. The expression of MALAT1 has been found to be diminished in a PD mouse model, whereas its overexpression up-regulates α-syn levels by inhibiting miR-129 expression (Xia et al., 2019). MALAT1 also promotes apoptosis by sponging miR-124 in PD mouse models, thus providing a potential therapeutic foundation for the clinical use of MALAT1 against PD (Liu et al., 2017).
Neuroinflammation plays an important role in PD pathogenesis. SNHG1 has been found to be elevated in brain specimens from patients with PD and in MPP+-induced SH-SY5Y cells. Cao et al. have observed that SNHG1 functioned as a ceRNA for miR-7, thus regulating nod-like receptor protein 3 (NLRP3) expression and activating the NLRP3 inflammasome pathway. These findings suggest that SNHG1 promotes neuroinflammation in PD pathogenesis by modulating the miR-7/NLRP3 pathway (Cao et al., 2018).
The lncRNA Mirt2 has also been shown to decrease inflammatory reactions in numerous cell types (Han et al., 2019). Mirt2 has been suggested to elevate SH-SY5Y cell resistance to inflammation, whereas Mirt2 overexpression generates anti-inflammatory properties by suppressing miR-101 expression and inhibiting the TNF-α-triggered NF-κB/p38MAPK pathway (Han et al., 2019).
The lncRNA GAS5 has also been shown to be associated with inflammatory responses. GAS5 has been found to be up-regulated in a PD mouse model and to positively regulate NLRP3 expression by competitively sponging miR-223-3p. These findings suggest that GAS5 accelerates PD progression by targeting the miR-223-3p/NLRP3 axis (Xu et al., 2020b).
Oxidative stress injury to dopaminergic neurons is another important pathological mechanism in PD pathogenesis and progression. The lncRNA BACE1-AS has been implicated in PD pathogenesis through regulating dopaminergic neuron oxidative stress injury. Li Y. et al. (2020) have observed that BACE1-AS levels are elevated in the substantia nigra of a rat model of PD, whereas down-regulated BACE1-AS decreases levels of α-syn, inducible nitric oxide synthase, and glutamine levels. In addition, down-regulated BACE1-AS has been found to repress apoptosis and oxidative stress injury in the substantia nigra neurons in a rat model of PDs by up-regulating miR-34b-5p.
In MPP+-induced PD-like cytotoxicity, NORAD expression levels are down-regulated in SH-SY5Y cells. In contrast, up-regulated NORAD protects against MPP+-induced cytotoxicity in these cells by rescuing MPP+-induced cellular destruction and apoptosis, as well as decreasing caspases 3/7, reactive oxygen species, and lactate dehydrogenase levels (Song et al., 2019).
Hox transcript antisense intergenic RNA (HOTAIR) is an approximately 2.2 kb lncRNA transcribed from the HOXC locus (Wang et al., 2017). The transcript is up-regulated in a PD mouse model and in SH-SY5Y cells pretreated with MPP+ and is believed to be involved in PD pathogenesis through up-regulating LRRK2 expression (Wang et al., 2017). HOTAIR is highly expressed in a variety of tumors, in which it may promote malignant biological behavior, but its role in PD pathogenesis remains unclear. Zhang Q. et al. (2020) have demonstrated that HOTAIR was up-regulated in PD model mice and MPP+ induced SH-SY5Y cells. HOTAIR knockdown notably ameliorates PD symptoms in vivo. In addition, HOTAIR silencing significantly inhibits neuronal damage by repressing NLRP3 mediated pyroptosis activation via regulation of the miR-326/ELAVL1 axis in PD mice (Zhang Q. et al., 2020). Moreover, HOTAIR appears to aggregate MPP+-triggered neuronal injury by sponging miR-874-5p; therefore, modulating HOTAIR expression may provide a therapeutic mechanism for PD treatment (Zhao et al., 2020b).
DAPK1, first identified during γ-interferon-induced programmed cell death, is a key factor in the CNS, including PD etiology (Lu et al., 2020). DAPK1 is up-regulated and negatively correlated with miR-124-3p levels in SH-SY5Y cells treated with MPP+. MALAT1 regulates miR-124-3p, whereas MALAT1 knockdown ameliorates animal behavioral changes and decreases apoptosis by up-regulating miR-124-3p and down-regulating DAPK1 in an MPTP induced PD mouse model. These data implicate the MALAT1/miR-124-3p/DAPK1 signaling cascade in PD pathogenesis (Lu et al., 2020). Additionally, MALAT1 is highly expressed in the brains of MPTP-induced PD model mice (Cai et al., 2020). Knockdown of MALAT1 inhibits elevated NRF2 expression, thereby inhibiting inflammasome activation and the production of reactive oxygen species in PD mice (Cai et al., 2020).
The lncRNA H19 is associated with PD progression (Zhang Y. et al., 2020); lncRNA H19 inhibits neuronal apoptosis in MPTP-induced PD mice and MPP+ treated neuroblastoma cells by regulating the miR-585-3p/PIK3R3 pathway (Zhang Y. et al., 2020). In addition, H19 over-expression protects against dopaminergic neuronal loss in this PD pathway by impairing the miR-301b-3p-targeted inhibition of HPRT1 expression. These data suggest a potential theoretical strategy for PD treatment by targeting lncRNAs (Jiang et al., 2020).
TUG1 expression is up-regulated and miR-152-3p is down-regulated in a PD mouse model (Zhai et al., 2020). TUG1 sponges and regulates miR-152-3p expression, whereas miR-152-3p negatively regulates PTEN expression. Therefore, TUG1 knockdown exerts a protective effect against PD via the miR-152-3p/PTEN pathway (Zhai et al., 2020).
HD-Related lncRNAs
HD is a rare autosomal dominant neurodegenerative disorder characterized by the loss of neurons in the striatum and cerebral cortex. The Huntingtin (HTT) protein has a CAG repeat sequence in the first exon, which encodes polyglutamine. Excessive amplification of the CAG repeat in gene coding regions causes polyglutamine chain amplification in the protein and results in disease. Wild-type Huntingtin regulates the nuclear translocation of RE1-silencing transcription factor (REST), whereas mutated Huntingtin causes abnormal REST nuclear translocation, thereby leading to abnormal expression of REST downstream target genes, including protein-coding genes and ncRNAs (Shimojo, 2008). After investigating the brain expression profiles in patients with HD, Johnson et al. (2010) have observed that expression of the lncRNA Human Accelerated Region 1 (HAR1) significantly decreased in the striatum, because REST localized to HAR1 loci via specific DNA regulatory elements, thereby inhibiting HAR1 transcription. Smeenk et al. (2011) have found that the lncRNA NEAT1 was up-regulated in patients with HD, and plays a different role with REST in the pathogenesis of HD. MEG3 has also been found to contain binding sites for REST within 10 kb of its transcription start site, thus suggesting that MEG3 may also be a REST target. Therefore, the substantial down-regulation of MEG3 in the brain tissues of patients with HD may be partly due to REST suppression (Johnson, 2012).
LINC0341 and RPS20P22 are both up-regulated in patients with HD, whereas LINC00342 is down-regulated, thereby revealing changes in neurological specific lncRNA expression patterns in patients with HD (Johnson, 2012). In the HD cell model, HTTAS overexpression has been found to decrease HTT transcription levels; however, siRNA knockdown of HTTAS v1 increases HTT transcription levels. These data provide strong evidence of the existence of an HTT antisense strand (Chung et al., 2011).
Studies by Francelle et al. (2015) have shown that Abhd11os expression in an HD mouse model was significantly diminished. In contrast, overexpression of Abhd11os elicited neuroprotective effects against the mutant HTT N-terminal fragment; however, Abhd11os knockout also elicited cytotoxic effects. In addition, the loss of lncRNA Abhd11os promotes vulnerability in the HD striatum (Francelle et al., 2015).
Sunwoo et al. (2017) have analyzed lncRNA expression patterns in HD via gene chips and found that lncRNA NEAT1 was highly expressed in the brains of patients with HD and in R6/2 mice. Furthermore, NEAT1 plays a neuroprotective role in HD; however, the specific mechanism remains unclarified (Sunwoo et al., 2017).
ALS-Related lncRNAs
ALS is a fatal NDD and is the most common form of motor neuron disease. Although the pathogenesis of ALS is not fully elucidated, its characteristic pathological manifestations are progressive exacerbation of limb weakness, muscle atrophy, and pyramidal tract signs, accompanied by bulbar palsy, dysphagia, and respiratory muscle involvement (Al-Chalabi and Hardiman, 2013; Bonafede and Mariotti, 2017). Approximately 5%–10% of patients with ALS have a family history of the disease, but the genes associated with ALS remain to be fully characterized (Sunwoo et al., 2017).
Expression of the lncRNA NEAT1 is significantly up-regulated in early ALS motor neurons. NEAT1/paraspeckle-related defects are closely associated with NDD occurrence. Previous studies have identified FUS as a disease-related ALS gene; FUS directly binds NEAT1 and localizes to nuclear paraspeckles. In in vivo studies on ALS, FUS has been shown to have undergone mutation, thus resulting in its relocalization from the nucleus to the cytoplasm and in nuclear paraspeckle functional defects. Moreover, in vitro experiments have shown that siRNA-mediated FUS interference hinders nuclear paraspeckle formation. Data from Nishimoto et al. have shown that NEAT1_2 was minimally expressed in the motor neurons of healthy people but highly expressed in the motor neurons of people with early-stage ALS, thus suggesting its utility as a biomarker for early ALS diagnostics. In addition, NEAT1_2 may serve as the backbone of RNA and RNA-binding protein (RBP) in the nuclei in ALS motor neurons, thereby regulating the expression of ALS-related RBP and exemplifying the roles of lncRNAs in ALS pathological processes (Nishimoto et al., 2013).
Circulating lncRNAs
lncRNAs participate in multiple biological processes and are associated with the occurrence and progression of several NDDs. In recent years, the detection of circulating lncRNAs in body fluids from patients with NDD has been demonstrated to be an effective diagnostic method (Table 2). Abnormal lncRNA expression is found not only in tissues and cells but also in various body fluids including the blood, urine, saliva, and CSF. Ren et al. have found that after repeated freezing and thawing, even in the presence of ribonucleases, lncRNAs from circulating plasma remained stable (Ren et al., 2013).
Gene chips and next-generation sequencing technologies have established an extensive archive of transcripts from the human genome. On the basis of these technologies, research on lncRNAs has rapidly developed. Several circulating lncRNAs have been shown to be effective in clinical diagnostic and prognostic applications, and have served as robust biomarkers for NDDs. Single or combined circulating lncRNAs have also demonstrated diagnostic performance comparable or superior to that of conventional NDD markers. In the next section, we further explore the potential of lncRNAs as diagnostic biomarkers for NDD.
Potential of lncRNAs as Diagnostic Ndd Biomarkers
BACE1-AS is up-regulated in the AD brain and potentially the bloodstream (Fotuhi et al., 2019). Fotuhi et al. have evaluated the AD diagnostic value of BACE1-AS in 45 patients with AD and 36 healthy controls. BACE1-AS levels were lower in patients with pre-AD, but higher in patients with full-AD, than in healthy controls. Receiver operating characteristic curve analysis has shown that BACE1-AS levels can be used to discriminate among pre-AD, full-AD, and healthy control samples with high sensitivity and specificity, thereby highlighting its potential utility as a biomarker for AD progression and diagnosis (Fotuhi et al., 2019).
Wang D. et al. (2020) have assessed whether plasma exosomal lncRNA levels in combination with image data from the entorhinal cortex and hippocampus could be used as a combinatorial AD biomarker, in a study on 72 patients with AD and 62 healthy controls. The plasma exosomal BACE1AS levels were significantly higher in the patients with AD than the healthy controls. Receiver operating characteristic curve analysis indicated an area under the curve of 0.761 for BACE1AS, with 87.5% sensitivity and 61.3% specificity. In addition, series parallel testing integrating BACE1AS levels with right entorhinal cortex volume and thickness have shown significantly increased specificity and sensitivity, at 96.15% and 90.91%, respectively.
In a study involving 88 patients with AD, Feng et al. have reported similar data: the plasma BACE1 levels were significantly elevated in patients with AD and distinguished patients with AD from healthy controls (88% specificity for AD). These observations suggest that BACE1 may be a potential candidate biomarker for predicting AD (Feng et al., 2018).
Zhuang et al. (2020) have detected and explored the clinical value of MALAT1 levels in CSF and plasma samples from 120 patients with AD. The MALAT1 levels were down-regulated in both the CSF and plasma, a finding associated with lower disease severity. The CSF MALAT1 levels also predicted MMSE score declines at years 1, 2, and 3 in patients with AD (Zhuang et al., 2020).
Similar studies have been conducted in patients with PD. Wang Q. et al. (2020) have performed next-generation sequencing of exosomal lncRNAs in peripheral blood samples from 32 patients with PD. Levels of lnc-MKRN2-42:1 were significantly lower in patients with PD. In addition, lnc-MKRN2-42:1 was positively associated with MDS-UPDRS III scores in patients with PD, thus suggesting that this lncRNA may be associated with PD occurrence and development. Lnc-MKRN2-42:1 has therefore been proposed as a biomarker for PD diagnosis and disease monitoring.
In a cohort of 72 patients with PD and 22 healthy controls, Fan et al. (2019) have identified 122 differentially expressed lncRNAs in the circulating leukocytes of patients with PD. Four lncRNAs (lnc-MOK-6:1, HOTAIRM1, RF01976.1-201, and AC131056.3-001) were further confirmed to be up-regulated in these patients. Moreover, the dysregulated lncRNAs AC131056.3-001 and HOTAIRM1 may contribute to PD pathogenesis by enhancing apoptosis in dopaminergic neurons.
Cheng et al. (2020) have evaluated the diagnostic value of serum TUG1 levels for PD in a case-control study including 97 patients with PD and 84 healthy controls. The TUG1 levels were significantly higher in patients with PD, thus suggesting that TUG1 could adequately distinguish patients with PD from healthy controls, with an area under the curve of 0.902. In addition, serum TUG1 was positively correlated with UPDRS levels, thus suggesting that TUG1 may be a possible target for PD early diagnosis and therapeutic intervention. Moreover, NEAT1 levels have been found to be up-regulated in PD blood samples, thus suggesting its potential as a PD biomarker (Boros et al., 2020).
The lncRNAs entering the circulatory system are stable in the blood or other body fluids (urine, saliva); thus, lncRNAs could be easily detected in these samples. However, the current detection of circulating lncRNAs still has many deficiencies and cannot be directly used in the early diagnosis of various NDDs in clinical practice. First, a consensus is lacking regarding the reference genes for circulating lncRNAs, thus preventing the determination of which genes are stable and are suitable reference genes. Further research remains to be performed on how to use appropriate reference genes to calculate the expression of circulating lncRNA and how to improve the accuracy of detection. Second, many circulating lncRNAs are difficult to detect in the circulatory system despite their lower expression than that of other circulating nucleic acids after pre-amplification. Therefore, the detection of circulating lncRNA must be improved in the selection and establishment of methods. Third, circulating lncRNAs with differential expression have been found to lack specificity for individual NDDs. For example, NEAT1 shows differential expression in AD, PD, and ALS. Therefore, combined detection based on multiple lncRNAs and combined diagnostic application with traditional serum biomarkers should greatly improve the diagnostic value and will be an important direction for development in the future. Current research on lncRNAs is primarily at the expression level. Similarly, clinical sample numbers are inadequate, and an incomplete understanding of the regulatory mechanisms precludes direct application in clinical practice. The lncRNA detection technologies and methods, including sample preparation, extraction methods, and internal reference selection, must be expanded and improved to meet these requirements.
Clinical Applications and Limitations of lncRNAs
Unlike mRNA molecules, lncRNAs are functional molecules. Their highly specific expression suggests that lncRNAs may serve as good biomarkers. In addition, lncRNA use in diagnostics and research on the therapeutic targeting of lncRNAs has received substantial attention. For pathogenic lncRNAs, the following blocking/silencing methodologies are primarily used. (1) Silencing lncRNA: (i) RNA interference to inhibit lncRNA expression; (ii) antisense oligonucleotides to block lncRNA activity; and (iii) RNaseH to induce lncRNA degradation. (2) Small molecule inhibitors that mask lncRNA binding sites and interacting proteins to block functions. (3) Small molecule inhibitors that bind lncRNAs, thus affecting folding and conformation.
For lncRNAs with neuroprotective or therapeutic effects, modern biological techniques can facilitate their increased expression at specific locations in the CNS for therapeutic purposes. For example, Espinoza et al. (2020) have used an adenovirus 9-mediated delivery system to increase SINEUP expression in the striatum in wild-type mice, thus indicating endogenous glial cell line-derived neurotrophic factor (GDNF) protein expression and potentiation of the DA system’s functions. In addition, SINEUP-GDNF has been found to ameliorate motor deficits and neurodegeneration of DA neurons in a PD neurochemical mouse model.
However, the current understanding of lncRNAs limits their clinical application. In addition, although lncRNAs are considered tissue and disease-specific, this specificity is not strong. For example, TUG1 is dysregulated in not only NDDs but also cancer and other diseases. Moreover, the viral system used to deliver lncRNA may have low efficiency in targeting NDDs, thus limiting the possibilities for using lncRNA as therapeutic strategy in the treatment of NDDs. Further research is needed to pursue these directions in the future.
Conclusions
The recent discovery of lncRNA has revolutionized the understanding of this anthropogenic group. lncRNA research remains in its infancy, and nomenclature schemes, structures, properties, and functional aspects remain to be standardized. In recent years, researchers have observed that lncRNAs played important roles in CNS development and NDD via epigenetic, translational, and posttranslational modifications. With the continued in-depth study of lncRNAs, specific lncRNAs are expected not only to be helpful in elucidating disease pathophysiological processes but also to become novel biomarkers and drug targets. Although very little is known about lncRNA function, and much bench research awaits, continued lncRNA exploration will doubtlessly provide new directions for NDD treatment and clinical drug development.
Author Contributions
MZ and PH drafted and revised the manuscript. ZB drafted and modified the figures and tables. All authors approved the final version of the manuscript and have agreed to be accountable for all aspects of the work, to ensure that questions associated with the accuracy or integrity of any part of the work are appropriately investigated and resolved. All authors contributed to the article and approved the submitted version.
Conflict of Interest
The authors declare that the research was conducted in the absence of any commercial or financial relationships that could be construed as a potential conflict of interest.
Publisher’s Note
All claims expressed in this article are solely those of the authors and do not necessarily represent those of their affiliated organizations, or those of the publisher, the editors and the reviewers. Any product that may be evaluated in this article, or claim that may be made by its manufacturer, is not guaranteed or endorsed by the publisher.
References
Al-Chalabi, A., and Hardiman, O. (2013). The epidemiology of ALS: a conspiracy of genes, environment and time. Nat. Rev. Neurol. 9, 617–628. doi: 10.1038/nrneurol.2013.203
Ang, E.T., Tai, Y.K., Lo, S.Q., Seet, R., and Soong, T.W. (2010). Neurodegenerative diseases: exercising toward neurogenesis and neuroregeneration. Front. Aging Neurosci. 2:25. doi: 10.3389/fnagi.2010.00025
Atik, A., Stewart, T., and Zhang, J. (2016). Alpha-synuclein as a biomarker for Parkinson’s disease. Brain Pathol. 26, 410–418. doi: 10.1111/bpa.12370
Bonafede, R., and Mariotti, R. (2017). ALS pathogenesis and therapeutic approaches: The role of mesenchymal stem cells and extracellular vesicles. Front. Cell. Neurosci. 11:80. doi: 10.3389/fncel.2017.00080
Boros, F. A., Maszlag-Török, R., Vécsei, L., and Klivényi, P. (2020). Increased level of NEAT1 long non-coding RNA is detectable in peripheral blood cells of patients with Parkinson’s disease. Brain Res. 1730:146672. doi: 10.1016/j.brainres.2020.146672
Cai, Y., Sun, Z., Jia, H., Luo, H., Ye, X., Wu, Q., et al. (2017). Rpph1 upregulates CDC42 expression and promotes hippocampal neuron dendritic spine formation by competing with miR-330-5p. Front. Mol. Neurosci. 10:27. doi: 10.3389/fnmol.2017.00027
Cai, L. J., Tu, L., Huang, X. M., Huang, J., Qiu, N., Xie, G. H., et al. (2020). LncRNA MALAT1 facilitates inflammasome activation via epigenetic suppression of Nrf2 in Parkinson’s disease. Mol. Brain 13:130. doi: 10.1186/s13041-020-00656-8
Cai, L., Tu, L., Li, T., Yang, X., Ren, Y., Gu, R., et al. (2019). Downregulation of lncRNA UCA1 ameliorates the damage of dopaminergic neurons, reduces oxidative stress and inflammation in Parkinson’s disease through the inhibition of the PI3K/Akt signaling pathway. Int. Immunopharmacol. 75:105734. doi: 10.1016/j.intimp.2019.105734
Calsolaro, V., and Edison, P. (2016). Neuroinflammation in Alzheimer’s disease: current evidence and future directions. Alzheimers Dement. 12, 719–732. doi: 10.1016/j.jalz.2016.02.010
Cao, B., Wang, T., Qu, Q., Kang, T., and Yang, Q. (2018). Long noncoding RNA SNHG1 promotes neuroinflammation in Parkinson’s disease via regulating miR-7/NLRP3 pathway. Neuroscience 388, 118–127. doi: 10.1016/j.neuroscience.2018.07.019
Cha, D. J., Mengel, D., Mustapic, M., Liu, W., Selkoe, D. J., Kapogiannis, D., et al. (2019). miR-212 and miR-132 are downregulated in neurally derived plasma exosomes of Alzheimer’s patients. Front. Neurosci. 13:1208. doi: 10.3389/fnins.2019.01208
Chen, Y., Li, H. Y., Zeng, F., Chen, L., Zhou, F. Y., Peng, Z. Y., et al. (2019). LincRNA plays a role in the effect of CYP46A1 polymorphism in Alzheimer’s disease-related pathology. Front. Aging Neurosci. 11:381. doi: 10.3389/fnagi.2019.00381
Chen, J. J., Yang, G., Yan, Q. Q., Zhao, J., and Li, S. (2019). Exosome-encapsulated microRNAs as promising biomarkers for Alzheimer’s disease. Rev. Neurosci. 31, 77–87. doi: 10.1515/revneuro-2019-0001
Chen, W. W., Zhang, X., and Huang, W. J. (2016). Role of neuroinflammation in neurodegenerative diseases (Review). Mol. Med. Rep. 13, 3391–3396. doi: 10.1515/revneuro-2019-0001
Cheng, J., Duan, Y., Zhang, F., Shi, J., Li, H., Wang, F., et al. (2020). The role of lncRNA TUG1 in the Parkinson disease and its effect on microglial inflammatory response. Neuromolecular Med. 23, 327–334. doi: 10.1007/s12017-020-08626-y
Chung, D. W., Rudnicki, D. D., Yu, L., and Margolis, R. L. (2011). A natural antisense transcript at the Huntington’s disease repeat locus regulates HTT expression. Hum. Mol. Genet. 20, 3467–3477. doi: 10.1093/hmg/ddr263
Ding, X. M., Zhao, L. J., Qiao, H. Y., Wu, S. L., and Wang, X. H. (2019). Long non-coding RNA-p21 regulates MPP(+)-induced neuronal injury by targeting miR-625 and derepressing TRPM2 in SH-SY5Y cells. Chem. Biol. Interact. 307, 73–81. doi: 10.1016/j.cbi.2019.04.017
Engreitz, J. M., Haines, J. E., Perez, E. M., Munson, G., Chen, J., Kane, M., et al. (2016). Local regulation of gene expression by lncRNA promoters, transcription and splicing. Nature 539, 452–455. doi: 10.1038/nature20149
Espinoza, S., Scarpato, M., Damiani, D., Managò, F., Mereu, M., Contestabile, A., et al. (2020). SINEUP non-coding RNA targeting GDNF rescues motor deficits and neurodegeneration in a mouse model of Parkinson’s disease. Mol. Ther. 28, 642–652. doi: 10.1016/j.ymthe.2019.08.005
Faghihi, M. A., Modarresi, F., Khalil, A. M., Wood, D. E., Sahagan, B. G., Morgan, T. E., et al. (2008). Expression of a noncoding RNA is elevated in Alzheimer’s disease and drives rapid feed-forward regulation of beta-secretase. Nat. Med. 14, 723–730. doi: 10.1038/nm1784
Fan, Y., Li, J., Yang, Q., Gong, C., Gao, H., Mao, Z., et al. (2019). Dysregulated long non-coding RNAs in Parkinson’s disease contribute to the apoptosis of human neuroblastoma cells. Front. Neurosci. 13:1320. doi: 10.3389/fnins.2019.01320
Fan, Y., Zhao, X., Lu, K., and Cheng, G. (2020). LncRNA BDNF-AS promotes autophagy and apoptosis in MPTP-induced Parkinson’s disease via ablating microRNA-125b-5p. Brain Res. Bull. 157, 119–127. doi: 10.1016/j.brainresbull.2020.02.003
Feneberg, E., Gray, E., Ansorge, O., Talbot, K., and Turner, M. R. (2018). Towards a TDP-43-based biomarker for ALS and FTLD. Mol. Neurobiol. 55, 7789–7801. doi: 10.1007/s12035-018-0947-6
Feng, L., Liao, Y. T., He, J. C., Xie, C. L., Chen, S. Y., Fan, H. H., et al. (2018). Plasma long non-coding RNA BACE1 as a novel biomarker for diagnosis of Alzheimer disease. BMC Neurol. 18:4. doi: 10.1186/s12883-017-1008-x
Fernandez, C. G., Hamby, M. E., Mcreynolds, M. L., and Ray, W. J. (2019). The role of APOE4 in disrupting the homeostatic functions of astrocytes and microglia in aging and Alzheimer’s disease. Front. Aging Neurosci. 11:14. doi: 10.3389/fnagi.2019.00014
Filipowicz, W., Jaskiewicz, L., Kolb, F. A., and Pillai, R. S. (2005). Post-transcriptional gene silencing by siRNAs and miRNAs. Curr. Opin. Struct. Biol. 15, 331–341. doi: 10.1016/j.sbi.2005.05.006
Fotuhi, S. N., Khalaj-Kondori, M., Hoseinpour Feizi, M. A., and Talebi, M. (2019). Long non-coding RNA BACE1-AS may serve as an Alzheimer’s disease blood-based biomarker. J. Mol. Neurosci. 69, 351–359. doi: 10.1007/s12031-019-01364-2
Francelle, L., Galvan, L., Gaillard, M. C., Petit, F., Bernay, B., Guillermier, M., et al. (2015). Striatal long noncoding RNA Abhd11os is neuroprotective against an N-terminal fragment of mutant huntingtin in vivo. Neurobiol. Aging 36, e1607–1616. doi: 10.1016/j.neurobiolaging.2014.11.014
Gao, Y., Zhang, N., Lv, C., Li, N., Li, X., Li, W., et al. (2020). IncRNA SNHG1 knockdown alleviates amyloid-β-induced neuronal injury by regulating ZNF217 via sponging miR-361–3p in Alzheimer’s disease. J. Alzheimers Dis. 77, 85–98. doi: 10.3233/JAD-191303
Garcez, M. L., Jacobs, K. R., and Guillemin, G. J. (2019). Microbiota alterations in Alzheimer’s disease: involvement of the kynurenine pathway and inflammation. Neurotox. Res. 36, 424–436. doi: 10.1007/s12640-019-00057-3
Gu, C., Chen, C., Wu, R., Dong, T., Hu, X., Yao, Y., et al. (2018). Long noncoding RNA EBF3-AS promotes neuron apoptosis in Alzheimer’s disease. DNA Cell Biol. 37, 220–226. doi: 10.1089/dna.2017.4012
Gu, R., Liu, R., Wang, L., Tang, M., Li, S. R., Hu, X., et al. (2020a). LncRNA RPPH1 attenuates Aβ(25–35)-induced endoplasmic reticulum stress and apoptosis in SH-SY5Y cells via miR-326/PKM2. Int. J. Neurosci. 1:8. doi: 10.1080/00207454.2020.1746307
Gu, R., Wang, L., Tang, M., Li, S. R., Liu, R., Hu, X., et al. (2020b). LncRNA Rpph1 protects amyloid-β induced neuronal injury in SK-N-SH cells via miR-122/Wnt1 axis. Int. J. Neurosci. 130, 443–453. doi: 10.1080/00207454.2019.1692834
Guo, C. C., Jiao, C. H., and Gao, Z. M. (2018). Silencing of LncRNA BDNF-AS attenuates Aβ(25–35)-induced neurotoxicity in PC12 cells by suppressing cell apoptosis and oxidative stress. Neurol. Res. 40, 795–804. doi: 10.1080/01616412.2018.1480921
Han, Y., Kang, C., Kang, M., Quan, W., Gao, H., Zhong, Z., et al. (2019). Long non-coding RNA Mirt2 prevents TNF-α-triggered inflammation via the repression of microRNA-101. Int. Immunopharmacol. 76:105878. doi: 10.1016/j.intimp.2019.105878
Haque, M. E., Akther, M., Jakaria, M., Kim, I. S., Azam, S., Choi, D. K., et al. (2020). Targeting the microglial NLRP3 inflammasome and its role in Parkinson’s disease. Mov. Disord. 35, 20–33. doi: 10.1002/mds.27874
He, W., Chi, S., Jin, X., Lu, J., Zheng, W., Yan, J., et al. (2020). Long Non-Coding RNA BACE1-AS modulates isoflurane-induced neurotoxicity to Alzheimer’s disease through sponging miR-214–3p. Neurochem. Res. 45, 2324–2335. doi: 10.1007/s11064-020-03091-2
Heo, J. B., Lee, Y. S., and Sung, S. (2013). Epigenetic regulation by long noncoding RNAs in plants. Chromosome Res. 21, 685–693. doi: 10.1007/s10577-013-9392-6
Huang, Y. (2018). The novel regulatory role of lncRNA-miRNA-mRNA axis in cardiovascular diseases. J. Cell. Mol. Med. 22, 5768–5775. doi: 10.1111/jcmm.13866
Huang, Z., Zhao, J., Wang, W., Zhou, J., and Zhang, J. (2020). Depletion of LncRNA NEAT1 rescues mitochondrial dysfunction through NEDD4L-dependent PINK1 degradation in animal models of Alzheimer’s disease. Front. Cell. Neurosci. 14:28. doi: 10.3389/fncel.2020.00028
Idda, M. L., Munk, R., Abdelmohsen, K., and Gorospe, M. (2018). Noncoding RNAs in Alzheimer’s disease. Wiley. Interdiscip. Rev. RNA 9:e1463. doi: 10.1002/wrna.1463
Jayaraj, R., Nayagam, S. G., Kar, A., Sathyakumar, S., Mohammed, H., Smiti, M., et al. (2019). Clinical theragnostic relationship between drug-resistance specific miRNA expressions, chemotherapeutic resistance and sensitivity in breast cancer: a systematic review and meta-znalysis. Cells 8:1250. doi: 10.3390/cells8101250
Jiang, J., Piao, X., Hu, S., Gao, J., and Bao, M. (2020). LncRNA H19 diminishes dopaminergic neuron loss by mediating microRNA-301b-3p in Parkinson’s disease via the HPRT1-mediated Wnt/β-catenin signaling pathway. Aging (Albany NY) 12, 8820–8836. doi: 10.18632/aging.102877
Johnson, R. (2012). Long non-coding RNAs in Huntington’s disease neurodegeneration. Neurobiol. Dis. 46, 245–254. doi: 10.1016/j.nbd.2011.12.006
Johnson, R., Richter, N., Jauch, R., Gaughwin, P. M., Zuccato, C., Cattaneo, E., et al. (2010). Human accelerated region 1 noncoding RNA is repressed by REST in Huntington’s disease. Physiol. Genomics 41, 269–274. doi: 10.1152/physiolgenomics.00019.2010
Johnsson, P., Lipovich, L., Grandér, D., and Morris, K. V. (2014). Evolutionary conservation of long non-coding RNAs; sequence, structure, function. Biochim. Biophys. Acta 1840, 1063–1071. doi: 10.1016/j.bbagen.2013.10.035
Ke, S., Yang, Z., Yang, F., Wang, X., Tan, J., Liao, B., et al. (2019). Long noncoding RNA NEAT1 aggravates Aβ-induced neuronal damage by targeting miR-107 in Alzheimer’s disease. Yonsei Med. J. 60, 640–650. doi: 10.3349/ymj.2019.60.7.640
Koelsch, G. (2017). BACE1 Function and inhibition: implications of intervention in the Amyloid pathway of Alzheimer’s disease pathology. Molecules 22:1723. doi: 10.3390/molecules22101723
Kou, X., Chen, D., and Chen, N. (2020). The regulation of microRNAs in Alzheimer’s disease. Front. Neurol. 11:288. doi: 10.3389/fneur.2020.00288
Kozomara, A., and Griffiths-Jones, S. (2014). miRBase: annotating high confidence microRNAs using deep sequencing data. Nucleic Acids Res. 42, D68–D73. doi: 10.1093/nar/gkt1181
Lee, J. T. (2012). Epigenetic regulation by long noncoding RNAs. Science 338, 1435–1439. doi: 10.1126/science.1231776
Li, J., An, G., Zhang, M., and Ma, Q. (2016). Long non-coding RNA TUG1 acts as a miR-26a sponge in human glioma cells. Biochem. Biophys Res. Commun. 477, 743–748. doi: 10.1016/j.bbrc.2016.06.129
Li, Y., Fang, J., Zhou, Z., Zhou, Q., Sun, S., Jin, Z., et al. (2020). Downregulation of lncRNA BACE1-AS improves dopamine-dependent oxidative stress in rats with Parkinson’s disease by upregulating microRNA-34b-5p and downregulating BACE1. Cell Cycle 19, 1158–1171. doi: 10.1080/15384101.2020.1749447
Li, X., Wang, S. W., Li, X. L., Yu, F. Y., and Cong, H. M. (2020). Knockdown of long non-coding RNA TUG1 depresses apoptosis of hippocampal neurons in Alzheimer’s disease by elevating microRNA-15a and repressing ROCK1 expression. Inflamm. Res. 69, 897–910. doi: 10.1007/s00011-020-01364-8
Li, L., Xu, Y., Zhao, M., and Gao, Z. (2020). Neuro-protective roles of long non-coding RNA MALAT1 in Alzheimer’s disease with the involvement of the microRNA-30b/CNR1 network and the following PI3K/AKT activation. Exp. Mol. Pathol. 117:104545. doi: 10.1016/j.yexmp.2020.104545
Li, H., Zheng, L., Jiang, A., Mo, Y., and Gong, Q. (2018). Identification of the biological affection of long noncoding RNA BC200 in Alzheimer’s disease. Neuroreport 29, 1061–1067. doi: 10.1097/WNR.0000000000001057
Liao, Q., Li, Z., Zeng, H., Feng, X., Huang, W., Fu, C., et al. (2019). Is the evidence strong enough for acupuncture ameliorates clinical symptoms in patients with amyotrophic lateral sclerosis: a protocol for a systematic review and meta-analysis. Medicine (Baltimore) 98:e15218. doi: 10.1097/MD.0000000000015218
Lin, M. T., and Beal, M. F. (2006). Mitochondrial dysfunction and oxidative stress in neurodegenerative diseases. Nature 443, 787–795. doi: 10.1038/nature05292
Liu, T., Huang, Y., Chen, J., Chi, H., Yu, Z., Wang, J., et al. (2014). Attenuated ability of BACE1 to cleave the amyloid precursor protein via silencing long noncoding RNA BACE1-AS expression. Mol. Med. Rep. 10, 1275–1281. doi: 10.3892/mmr.2014.2351
Liu, J., Li, Y., Tong, J., Gao, J., Guo, Q., Zhang, L., et al. (2018). Long non-coding RNA-dependent mechanism to regulate heme biosynthesis and erythrocyte development. Nat. Commun. 9:4386. doi: 10.1038/s41467-018-06883-x
Liu, R., Li, F., and Zhao, W. (2020). Long noncoding RNA NEAT1 knockdown inhibits MPP(+)-induced apoptosis, in-ammation and cytotoxicity in SK-N-SH cells by regulating miR-212–5p/RAB3IP axis. Neurosci. Lett. 731:135060. doi: 10.1016/j.neulet.2020.135060
Liu, W., Zhang, Q., Zhang, J., Pan, W., Zhao, J., Xu, Y., et al. (2017). Long non-coding RNA MALAT1 contributes to cell apoptosis by sponging miR-124 in Parkinson disease. Cell Biosci. 7:19. doi: 10.1186/s13578-017-0147-5
Lu, Y., Gong, Z., Jin, X., Zhao, P., Zhang, Y., Wang, Z., et al. (2020). LncRNA MALAT1 targeting miR-124–3p regulates DAPK1 expression contributes to cell apoptosis in Parkinson’s Disease. J. Cell Biochem. [Online ahead of print]. doi: 10.1002/jcb.29711
Lu, M., Sun, W. L., Shen, J., Wei, M., Chen, B., Qi, Y. J., et al. (2018). LncRNA-UCA1 promotes PD development by upregulating SNCA. Eur. Rev. Med. Pharmacol. Sci. 22, 7908–7915. doi: 10.26355/eurrev_201811_16417
Ma, P., Li, Y., Zhang, W., Fang, F., Sun, J., Liu, M., et al. (2019). Long non-coding RNA MALAT1 inhibits neuron apoptosis and neuroinflammation while stimulates neurite outgrowth and its correlation with MiR-125b mediates PTGS2, CDK5 and FOXQ1 in Alzheimer’s disease. Curr. Alzheimer Res. 16, 596–612. doi: 10.2174/1567205016666190725130134
Maoz, R., Garfinkel, B. P., and Soreq, H. (2017). Alzheimer’s disease and ncRNAs. Adv. Exp. Med. Biol. 978, 337–361. doi: 10.1007/978-3-319-53889-1_18
Maracaja-Coutinho, V., Paschoal, A. R., Caris-Maldonado, J. C., Borges, P. V., Ferreira, A. J., Durham, A. M., et al. (2019). Noncoding RNAs databases: current status and trends. Methods Mol. Biol. 1912, 251–285. doi: 10.1007/978-1-4939-8982-9_10
Massone, S., Vassallo, I., Fiorino, G., Castelnuovo, M., Barbieri, F., Borghi, R., et al. (2011). 17A, a novel non-coding RNA, regulates GABA B alternative splicing and signaling in response to inflammatory stimuli and in Alzheimer disease. Neurobiol. Dis. 41, 308–317. doi: 10.1016/j.nbd.2010.09.019
Nishimoto, Y., Nakagawa, S., Hirose, T., Okano, H. J., Takao, M., Shibata, S., et al. (2013). The long non-coding RNA nuclear-enriched abundant transcript 1_2 induces paraspeckle formation in the motor neuron during the early phase of amyotrophic lateral sclerosis. Mol. Brain 6:31. doi: 10.1186/1756-6606-6-31
Palmqvist, S., Janelidze, S., Stomrud, E., Zetterberg, H., Karl, J., Zink, K., et al. (2019). Performance of fully automated plasma assays as screening tests for Alzheimer disease-related β-amyloid status. JAMA Neurol. 76, 1060–1069. doi: 10.1001/jamaneurol.2019.1632
Peng, T., Liu, X., Wang, J., Liu, Y., Fu, Z., Ma, X., et al. (2019). Long noncoding RNA HAGLROS regulates apoptosis and autophagy in Parkinson’s disease via regulating miR-100/ATG10 axis and PI3K/Akt/mTOR pathway activation. Artif. Cells Nanomed Biotechnol. 47, 2764–2774. doi: 10.1080/21691401.2019.1636805
Qian, C., Ye, Y., Mao, H., Yao, L., Sun, X., Wang, B., et al. (2019). Downregulated lncRNA-SNHG1 enhances autophagy and prevents cell death through the miR-221/222 /p27/mTOR pathway in Parkinson’s disease. Exp. Cell. Res. 384:111614. doi: 10.1016/j.yexcr.2019.111614
Ramos, A. D., Diaz, A., Nellore, A., Delgado, R. N., Park, K. Y., Gonzales-Roybal, G., et al. (2013). Integration of genome-wide approaches identifies lncRNAs of adult neural stem cells and their progeny in vivo. Cell Stem Cell 12, 616–628. doi: 10.1016/j.stem.2013.03.003
Ransohoff, J. D., Wei, Y., and Khavari, P. A. (2018). The functions and unique features of long intergenic non-coding RNA. Nat. Rev. Mol. Cell Biol. 19, 143–157. doi: 10.1038/nrm.2017.104
Ren, S., Wang, F., Shen, J., Sun, Y., Xu, W., Lu, J., et al. (2013). Long non-coding RNA metastasis associated in lung adenocarcinoma transcript 1 derived miniRNA as a novel plasma-based biomarker for diagnosing prostate cancer. Eur. J. Cancer 49, 2949–2959. doi: 10.1016/j.ejca.2013.04.026
Rittiner, J. E., Moncalvo, M., Chiba-Falek, O., and Kantor, B. (2020). Gene-editing technologies paired with viral vectors for translational research into neurodegenerative diseases. Front. Mol. Neurosci. 13:148. doi: 10.3389/fnmol.2020.00148
Riva, P., Ratti, A., and Venturin, M. (2016). The long non-coding RNAs in neurodegenerative diseases: novel mechanisms of pathogenesis. Curr. Alzheimer Res. 13, 1219–1231. doi: 10.2174/1567205013666160622112234
Robinson, E. K., Covarrubias, S., and Carpenter, S. (2020). The how and why of lncRNA function: an innate immune perspective. Biochim. Biophys. Acta Gene Regul. Mech. 1863:194419. doi: 10.1016/j.bbagrm.2019.194419
Roser, A. E., Caldi Gomes, L., Schünemann, J., Maass, F., and Lingor, P. (2018). Circulating miRNAs as diagnostic biomarkers for Parkinson’s disease. Front. Neurosci. 12:625. doi: 10.3389/fnins.2018.00625
Saito, T., Kurashige, J., Nambara, S., Komatsu, H., Hirata, H., Ueda, M., et al. (2015). A long non-coding RNA activated by transforming growth factor-β is an independent prognostic marker of gastric cancer. Ann. Surg. Oncol. 22, S915–922. doi: 10.1245/s10434-015-4554-8
Sarropoulos, I., Marin, R., Cardoso-Moreira, M., and Kaessmann, H. (2019). Developmental dynamics of lncRNAs across mammalian organs and species. Nature 571, 510–514. doi: 10.1038/s41586-019-1341-x
Sevigny, J., Chiao, P., Bussière, T., Weinreb, P. H., Williams, L., Maier, M., et al. (2016). The antibody aducanumab reduces Aβ plaques in Alzheimer’s disease. Nature 537, 50–56. doi: 10.1038/nature19323
Shimojo, M. (2008). Huntingtin regulates RE1-silencing transcription factor/neuron-restrictive silencer factor (REST/NRSF) nuclear trafficking indirectly through a complex with REST/NRSF-interacting LIM domain protein (RILP) and dynactin p150 Glued. J. Biol. Chem. 283, 34880–34886. doi: 10.1074/jbc.M804183200
Smeenk, L., Van Heeringen, S. J., Koeppel, M., Gilbert, B., Janssen-Megens, E., Stunnenberg, H. G., et al. (2011). Role of p53 serine 46 in p53 target gene regulation. PLoS One 6:e17574. doi: 10.1371/journal.pone.0017574
Song, Q., Geng, Y., Li, Y., Wang, L., and Qin, J. (2019). Long noncoding RNA NORAD regulates MPP+-induced Parkinson’s disease model cells. J. Chem. Neuroanat. 101:101668. doi: 10.1016/j.jchemneu.2019.101668
Soreq, L., Guffanti, A., Salomonis, N., Simchovitz, A., Israel, Z., Bergman, H., et al. (2014). Long non-coding RNA and alternative splicing modulations in Parkinson’s leukocytes identified by RNA sequencing. PLoS Comput. Biol. 10:e1003517. doi: 10.1371/journal.pcbi.1003517
Spreafico, M., Grillo, B., Rusconi, F., Battaglioli, E., and Venturin, M. (2018). Multiple layers of CDK5R1 regulation in Alzheimer’s disease implicate long non-coding RNAs. Int. J. Mol. Sci. 19:2022. doi: 10.3390/ijms19072022
Sun, Q., Zhang, Y., Wang, S., Yang, F., Cai, H., Xing, Y., et al. (2020). NEAT1 decreasing suppresses Parkinson’s disease progression via acting as miR-1301–3p sponge. J. Mol. Neurosci. 71, 369–378. doi: 10.1007/s12031-020-01660-2
Sunwoo, J. S., Lee, S. T., Im, W., Lee, M., Byun, J. I., Jung, K. H., et al. (2017). Altered expression of the long noncoding RNA NEAT1 in Huntington’s disease. Mol. Neurobiol. 54, 1577–1586. doi: 10.1007/s12035-016-9928-9
Tobore, T. O. (2019). On the central role of mitochondria dysfunction and oxidative stress in Alzheimer’s disease. Neurol. Sci. 40, 1527–1540. doi: 10.1007/s10072-019-03863-x
Wang, X., Arai, S., Song, X., Reichart, D., Du, K., Pascual, G., et al. (2008). Induced ncRNAs allosterically modify RNA-binding proteins in cis to inhibit transcription. Nature 454, 126–130. doi: 10.1038/nature06992
Wang, Z., Cheng, H., Qi, L., and Sui, D. (2020a). Comprehensive analysis of long non-coding RNA using an associated competitive endogenous RNA network in Wilms tumor. Mol. Med. Rep. 22, 105–116. doi: 10.3892/mmr.2020.11124
Wang, Z., Li, K., and Huang, W. (2020b). Long non-coding RNA NEAT1-centric gene regulation. Cell. Mol. Life Sci. 77, 3769–3779. doi: 10.1007/s00018-020-03503-0
Wang, Q., Han, C.-L., Wang, K.-L., Sui, Y.-P., Li, Z.-B., Chen, N., et al. (2020). Integrated analysis of exosomal lncRNA and mRNA expression profiles reveals the involvement of lnc-MKRN2–42:1 in the pathogenesis of Parkinson’s disease. CNS Neurosci. Ther. 26, 527–537. doi: 10.1111/cns.13277
Wang, H., Lu, B., and Chen, J. (2019). Knockdown of lncRNA SNHG1 attenuated Aβ(25–35)-inudced neuronal injury via regulating KREMEN1 by acting as a ceRNA of miR-137 in neuronal cells. Biochem. Biophys. Res. Commun. 518, 438–444. doi: 10.1016/j.bbrc.2019.08.033
Wang, D., Wang, P., Bian, X., Xu, S., Zhou, Q., Zhang, Y., et al. (2020). Elevated plasma levels of exosomal BACE1-AS combined with the volume and thickness of the right entorhinal cortex may serve as a biomarker for the detection of Alzheimer’s disease. Mol. Med. Rep. 22, 227–238. doi: 10.3892/mmr.2020.11118
Wang, X., Wang, C., Geng, C., and Zhao, K. (2018). LncRNA XIST knockdown attenuates Aβ(25–35)-induced toxicity, oxidative stress and apoptosis in primary cultured rat hippocampal neurons by targeting miR-132. Int. J. Clin. Exp. Pathol. 11, 3915–3924.
Wang, S., Zhang, X., Guo, Y., Rong, H., and Liu, T. (2017). The long noncoding RNA HOTAIR promotes Parkinson’s disease by upregulating LRRK2 expression. Oncotarget 8, 24449–24456. doi: 10.18632/oncotarget.15511
Wang, J., Zhou, T., Wang, T., and Wang, B. (2018). Suppression of lncRNA-ATB prevents amyloid-β-induced neurotoxicity in PC12 cells via regulating miR-200/ZNF217 axis. Biomed. Pharmacother. 108, 707–715. doi: 10.1016/j.biopha.2018.08.155
Wu, Y. Y., and Kuo, H. C. (2020). Functional roles and networks of non-coding RNAs in the pathogenesis of neurodegenerative diseases. J. Biomed. Sci. 27:49. doi: 10.1186/s12929-020-00636-z
Xia, D., Sui, R., and Zhang, Z. (2019). Administration of resveratrol improved Parkinson’s disease-like phenotype by suppressing apoptosis of neurons via modulating the MALAT1/miR-129/SNCA signaling pathway. J. Cell Biochem. 120, 4942–4951. doi: 10.1002/jcb.27769
Xing, Y. H., and Chen, L. L. (2018). Processing and roles of snoRNA-ended long noncoding RNAs. Crit. Rev. Biochem. Mol. Biol. 53, 596–606. doi: 10.1080/10409238.2018.1508411
Xu, W., Li, K., Fan, Q., Zong, B., and Han, L. (2020a). Knockdown of long non-coding RNA SOX21-AS1 attenuates amyloid-β-induced neuronal damage by sponging miR-107. Biosci. Rep. 40:BSR20194295. doi: 10.1042/BSR20194295
Xu, W., Zhang, L., Geng, Y., Liu, Y., and Zhang, N. (2020b). Long noncoding RNA GAS5 promotes microglial inflammatory response in Parkinson’s disease by regulating NLRP3 pathway through sponging miR-223–3p. Int. Immunopharmacol. 85:106614. doi: 10.1016/j.intimp.2020.106614
Xu, X., Zhuang, C., Wu, Z., Qiu, H., Feng, H., Wu, J., et al. (2018). LincRNA-p21 inhibits cell viability and promotes cell apoptosis in Parkinson’s disease through activating α-synuclein expression. Biomed. Res. Int. 2018:8181374. doi: 10.1155/2018/8181374
Yan, W., Chen, Z. Y., Chen, J. Q., and Chen, H. M. (2018). LncRNA NEAT1 promotes autophagy in MPTP-induced Parkinson’s disease through stabilizing PINK1 protein. Biochem. Biophys. Res. Commun. 496, 1019–1024. doi: 10.1016/j.bbrc.2017.12.149
Yan, Y., Yan, H., Teng, Y., Wang, Q., Yang, P., Zhang, L., et al. (2020). Long non-coding RNA 00507/miRNA-181c-5p/TTBK1/MAPT axis regulates tau hyperphosphorylation in Alzheimer’s disease. J. Gene Med. 22:e3268. doi: 10.1002/jgm.3268
Yang, Z., Jiang, S., Shang, J., Jiang, Y., Dai, Y., Xu, B., et al. (2019). LncRNA: Shedding light on mechanisms and opportunities in fibrosis and aging. Ageing Res. Rev. 52, 17–31. doi: 10.1016/j.arr.2019.04.001
Yang, H., Shang, D., Xu, Y., Zhang, C., Feng, L., Sun, Z., et al. (2017). The LncRNA connectivity map: Using LncRNA signatures to connect small molecules, lncRNAs and diseases. Sci. Rep. 7:6655. doi: 10.1038/s41598-017-06897-3
Ye, Y., He, X., Lu, F., Mao, H., Zhu, Z., Yao, L., et al. (2018). A lincRNA-p21/miR-181 family feedback loop regulates microglial activation during systemic LPS- and MPTP- induced neuroinflammation. Cell Death Dis. 9:803. doi: 10.1038/s41419-018-0821-5
Yi, J., Chen, B., Yao, X., Lei, Y., Ou, F., Huang, F., et al. (2019). Upregulation of the lncRNA MEG3 improves cognitive impairment, alleviates neuronal damage and inhibits activation of astrocytes in hippocampus tissues in Alzheimer’s disease through inactivating the PI3K/Akt signaling pathway. J. Cell Biochem. 120, 18053–18065. doi: 10.1002/jcb.29108
Yue, D., Guanqun, G., Jingxin, L., Sen, S., Shuang, L., Yan, S., et al. (2020). Silencing of long noncoding RNA XIST attenuated Alzheimer’s disease-related BACE1 alteration through miR-124. Cell Biol. Int. 44, 630–636. doi: 10.1002/cbin.11263
Zhai, K., Liu, B., and Gao, L. (2020). Long-noncoding RNA TUG1 promotes Parkinson’s disease via modulating MiR-152–3p/PTEN pathway. Hum. Gene Ther. 31, 1274–1287. doi: 10.1089/hum.2020.106
Zhang, Q., Huang, X. M., Liao, J. X., Dong, Y. K., Zhu, J. L., He, C. C., et al. (2020). LncRNA HOTAIR promotes neuronal damage through facilitating NLRP3 mediated-pyroptosis activation in Parkinson’s disease via regulation of miR-326/ELAVL1 axis. Cell Mol. Neurobiol. [Online ahead of print]. doi: 10.1007/s10571-020-00946-8
Zhang, T., Pang, P., Fang, Z., Guo, Y., Li, H., Li, X., et al. (2018). Expression of BC1 impairs spatial learning and memory in Alzheimer’s disease via APP translation. Mol. Neurobiol. 55, 6007–6020. doi: 10.1007/s12035-017-0820-z
Zhang, L. M., Wang, M. H., Yang, H. C., Tian, T., Sun, G. F., Ji, Y. F., et al. (2019). Dopaminergic neuron injury in Parkinson’s disease is mitigated by interfering lncRNA SNHG14 expression to regulate the miR-133b/ α-synuclein pathway. Aging (Albany NY) 11, 9264–9279. doi: 10.18632/aging.102330
Zhang, Y., Xia, Q., and Lin, J. (2020). LncRNA H19 Attenuates apoptosis in MPTP-induced Parkinson’s disease through regulating miR-585–3p/PIK3R3. Neurochem. Res. 45, 1700–1710. doi: 10.1007/s11064-020-03035-w
Zhang, W., Zhao, H., Wu, Q., Xu, W., and Xia, M. (2018). Knockdown of BACE1-AS by siRNA improves memory and learning behaviors in Alzheimer’s disease animal model. Exp. Ther. Med. 16, 2080–2086. doi: 10.3892/etm.2018.6359
Zhao, J., Geng, L., Chen, Y., and Wu, C. (2020a). SNHG1 promotes MPP(+)-induced cytotoxicity by regulating PTEN/AKT/mTOR signaling pathway in SH-SY5Y cells via sponging miR-153–3p. Biol. Res. 53:1. doi: 10.1186/s40659-019-0267-y
Zhao, J., Li, H., and Chang, N. (2020b). LncRNA HOTAIR promotes MPP+-induced neuronal injury in Parkinson’s disease by regulating the miR-874–5p/ATG10 axis. EXCLI J. 19, 1141–1153. doi: 10.17179/excli2020-2286
Zhao, Y., Jaber, V., Alexandrov, P. N., Vergallo, A., Lista, S., Hampel, H., et al. (2020). microRNA-based biomarkers in Alzheimer’s disease (AD). Front. Neurosci. 14:585432. doi: 10.3389/fnins.2020.585432
Zhao, M. Y., Wang, G. Q., Wang, N. N., Yu, Q. Y., Liu, R. L., Shi, W. Q., et al. (2019). The long-non-coding RNA NEAT1 is a novel target for Alzheimer’s disease progression via miR-124/BACE1 axis. Neurol. Res. 41, 489–497. doi: 10.1080/01616412.2018.1548747
Zhou, B., Li, L., Qiu, X., Wu, J., Xu, L., Shao, W., et al. (2020). Long non-coding RNA ANRIL knockdown suppresses apoptosis and pro-inflammatory cytokines while enhancing neurite outgrowth via binding microRNA-125a in a cellular model of Alzheimer’s disease. Mol. Med. Rep. 22, 1489–1497. doi: 10.3892/mmr.2020.11203
Zhou, Q., Liu, J., Quan, J., Liu, W., Tan, H., Li, W., et al. (2018). MicroRNAs as potential biomarkers for the diagnosis of glioma: a systematic review and meta-analysis. Cancer Sci. 109, 2651–2659. doi: 10.1111/cas.13714
Zhou, R., Yang, G., and Shi, Y. (2020). Macromolecular complex in recognition and proteolysis of amyloid precursor protein in Alzheimer’s disease. Curr. Opin. Struct. Biol. 61, 1–8. doi: 10.1016/j.sbi.2019.09.004
Zhou S., Zhang, D., Guo, J., Chen, Z., Chen, Y., Zhang, J., et al. (2020a). Deficiency of NEAT1 prevented MPP(+)-induced inflammatory response, oxidative stress and apoptosis in dopaminergic SK-N-SH neuroblastoma cells via miR-1277–5p/ARHGAP26 axis. Brain Res. 1750:147156. doi: 10.1016/j.brainres.2020.147156
Zhou S., Zhang, D., Guo, J., Zhang, J., and Chen, Y. (2020b). Knockdown of SNHG14 alleviates MPP(+)-induced injury in the cell model of Parkinson’s disease by targeting the miR-214–3p/KLF4 axis. Front. Neurosci. 14:930. doi: 10.3389/fnins.2020.00930
Zhu, L., Lin, M., Ma, J., Liu, W., Gao, L., Wei, S., et al. (2019). The role of LINC00094/miR-224–5p (miR-497-5p)/endophilin-1 axis in Memantine mediated protective effects on blood-brain barrier in AD microenvironment. J. Cell. Mol. Med. 23, 3280–3292. doi: 10.1111/jcmm.14214
Keywords: biomarkers, circulating, lncRNA, miRNA, neurodegenerative disease
Citation: Zhang M, He P and Bian Z (2021) Long Noncoding RNAs in Neurodegenerative Diseases: Pathogenesis and Potential Implications as Clinical Biomarkers. Front. Mol. Neurosci. 14:685143. doi: 10.3389/fnmol.2021.685143
Received: 24 March 2021; Accepted: 19 July 2021;
Published: 04 August 2021.
Edited by:
Fabrizio Michetti, Catholic University of the Sacred Heart, ItalyReviewed by:
Gema Navarro, Independent Researcher, Madrid, SpainGiau Van Vo, Gachon University, South Korea
Copyright © 2021 Zhang, He and Bian. This is an open-access article distributed under the terms of the Creative Commons Attribution License (CC BY). The use, distribution or reproduction in other forums is permitted, provided the original author(s) and the copyright owner(s) are credited and that the original publication in this journal is cited, in accordance with accepted academic practice. No use, distribution or reproduction is permitted which does not comply with these terms.
*Correspondence: Zhigang Bian, YmlhbnpnMDgxMEAxNjMuY29t