- 1Center for Neural Science, New York University, New York, NY, United States
- 2Department of Neurobiology and Behavior, School of Medicine, Stony Brook University, Stony Brook, NY, United States
Neuropeptides can exert volume modulation in neuronal networks, which account for a well-calibrated and fine-tuned regulation that depends on the sensory and behavioral contexts. For example, oxytocin (OT) and oxytocin receptor (OTR) trigger a signaling pattern encompassing intracellular cascades, synaptic plasticity, gene expression, and network regulation, that together function to increase the signal-to-noise ratio for sensory-dependent stress/threat and social responses. Activation of OTRs in emotional circuits within the limbic forebrain is necessary to acquire stress/threat responses. When emotional memories are retrieved, OTR-expressing cells act as gatekeepers of the threat response choice/discrimination. OT signaling has also been implicated in modulating social-exposure elicited responses in the neural circuits within the limbic forebrain. In this review, we describe the cellular and molecular mechanisms that underlie the neuromodulation by OT, and how OT signaling in specific neural circuits and cell populations mediate stress/threat and social behaviors. OT and downstream signaling cascades are heavily implicated in neuropsychiatric disorders characterized by emotional and social dysregulation. Thus, a mechanistic understanding of downstream cellular effects of OT in relevant cell types and neural circuits can help design effective intervention techniques for a variety of neuropsychiatric disorders.
Introduction
Well-known for its role in reproductive behaviors, the highly conserved neuropeptide oxytocin (OT) is a key modulator of neural activity in a distributed network of cells within the limbic forebrain. OT modulation of the limbic forebrain network is critical for mediating behavioral and physiological responses to internal and external stimuli – prominent among these are aversive stress/threat and appetitive social exposure. A wide range of behaviors modulated by OT include the threat and/or stress-induced defensive responses such as freezing and pro-active coping, and social exposure-elicited behaviors such as social interaction, aggression, mating, and maternal care (Carter, 1998; Ferguson et al., 2000; Mantella et al., 2003; Pedersen et al., 2006; Neumann, 2008; Bartz et al., 2011). OT modulation is important for conferring salience of emotional and social behaviors (Carter, 1998; Ferguson et al., 2000; Ciocchi et al., 2010; Bartz et al., 2011; Knobloch et al., 2012; Hasan et al., 2019). The pleiotropic effects of OT on these behaviors are thought to be driven by cascading molecular signaling in specific neural circuits across the brain (Figure 1).
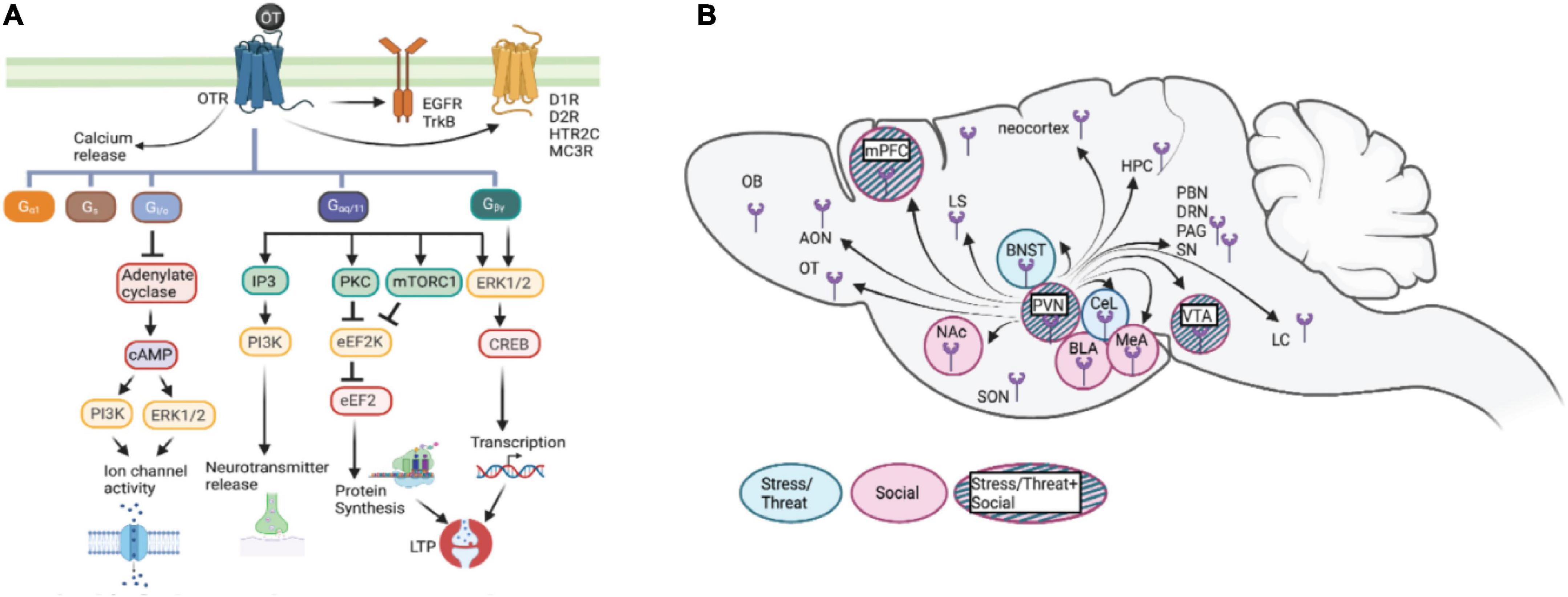
Figure 1. (A) Schematic representation of potential cascades involved in oxytocin signaling: OTR which is G-protein coupled receptor acts through various G-protein subunits, Gaq111 being the most common. This subunit either through calcium release or EGFR/TrkB transactivation brings about various downstream effects including neurotransmitter release, protein synthesis. Additionally binding of OT to its receptor also trans-activates some other receptors like dopamine receptors (01R, D2R), serotonin receptor (5HTR2c), orexin receptor (OXR) and melanocortin receptor (MC3R) forming heterodimers. Other subunits include Gpy; involved in LTP induction and protein synthesis, Gifo in regulating ion channel activity, Gs and Ga1 involved in cellular responses like proliferation and differentiation. (B) Diagram of oxytocin release in brain areas involved in stress/threat or social networks. Oxytocin stimulates the limbic forebrain through the presence of OTR and intracellular cascades, increasing the signal to noise ratio for stress/threat or social information. OT, oxytocin; OTR, oxytocin receptor; Gaq111, Gv0, Gs, Ga1; Gifo various G-protein subunits, EGFR, Epidermal growth factor; cAMP, cyclic adenosine monophosphate; IP3, Inositol 1,4,5 triphosphate; PKA, protein kinase A; pKC, Protein Kinase C; ERK, extracellular signal related kinases; eEF2, elongation factor 2; CREB, Cyclic AMP responsive element binding protein; LTP, Long term potentiation; 01R, Dopamine type1 receptor; D2R, Dopamine type 2 receptor; HTR2C, 5Hydroxytryptamine receptor 2C; MC3R, Melanocortin 3 receptor; OXR, Orexin receptor; ERK1/2, Extracellular signal-regulated kinase; mTORC1, mammalian target of rapamycin complex I; PVN, paraventricular nucleus; SON, supraoptic nucleus; mPFC, medial prefrontal cortex; OB, olfactory bulb; OT, olfactory tubercle; AON, anterior olfactory nucleus; BNST, base nucleus of the stria terminalis; CeL, centrolateral amygdala; BLA, basolateral amygdala; MeA, medial amygdala; Nae, nucleus accumbens; VTA, ventrotegmental nucleus; LS, lateral septum; HPC, hippocampus; LC, locus coeruleus; PAG, periaqueductal gray; SN, substantia nigra; PBN, parabrachial nucleus; ORN, dorsal raphe nucleus.
Oxytocin (OT) binds almost exclusively to its membrane-bound oxytocin receptor (OTR), which is a G-protein coupled receptor that can associate with one of several G proteins that mediate distinct physiological responses downstream of OT-OTR interaction in a cellular context and circuit-specific manner (Tomizawa et al., 2003; Wang and Hatton, 2007; Lin et al., 2012; Chini et al., 2017). The knowledge of various signaling pathways underlying context and experience-dependent behavioral responses induced by OT is crucial for understanding the heterogeneity of the downstream effects elicited by OT across the limbic forebrain. The critical challenge in oxytocin research is establishing causal relations between the specific behavioral effect and the underlying molecular mechanisms and circuits. The objective of this review is to provide a framework for the role that OT plays in modulating physiological responses relevant to emotional and social behaviors at different levels of the organization – from signaling inside cells to specific cell types and neural circuits, based on rodent studies. We describe here the cellular and molecular mechanisms that underlie the neuromodulation by oxytocin and how OT signaling in specific neural circuits within the limbic forebrain mediates emotional and social behaviors. In light of the array of modulatory effects that OT exerts on complex behaviors in humans and animal models, we discuss how OT signaling has been a prime focus for understanding pathophysiology and therapeutic potential in neuropsychiatric disorders.
Oxytocin signaling inside cells
In mammals, OT is synthesized in the hypothalamus, particularly in the paraventricular nucleus (PVN), the supraoptic nucleus (SON), and accessory magnocellular nuclei (AN) (Stoop, 2012). OT is released from these nuclei as a neurohormone with both paracrine and synaptic functions; therefore, oxytocin release has a dual mechanism, reaching different cortical and subcortical regions where the oxytocin receptor (OTR) is expressed (Jiménez et al., 2015; Mitre et al., 2016; Jurek and Neumann, 2018). The release of OT from PVN and SON, via axonic fibers, stimulates numerous brain regions expressing the OTR (Knobloch et al., 2012; Mitre et al., 2016) and permits the precise time-dependent, local regulation of its basal levels in addition to volume transmission (Landgraf and Neumann, 2004). Aside from axonal release, the calcium-dependent release of OT from dendrites is triggered by vesicles primed for activity-dependent release, which could lead to a functional reorganization of neuronal networks harboring the OTR, thereby affording a substrate for sustained effects (Ludwig et al., 2002).
Oxytocin binds to its cognate receptor oxytocin receptor
The various effects exerted by OT are channeled through its only receptor, the oxytocin receptor (OTR), which belongs to the G-protein coupled receptor family (Figure 1A). In rare circumstances, OT can also bind to the receptor of closely related nonapeptide vasopressin, albeit with lower affinity (Wang and Hatton, 2007). The OTR is expressed in various cell populations within a distributed network across the brain and binds OT with an affinity of about 1–10 nM (Chini et al., 2017). This cell surface receptor transmits the OT signal intracellularly, enabling it to exert its effect on various cell functions. There are several well-established pathways downstream of OT-OTR interactions, which vary at the level of brain regions, cells, and molecules involved in other outcomes of these interactions. Context-dependent signaling is central to the variability of OTR activity, hence it is important to understand how cellular context influences OT-mediated activation of various intracellular signaling cascades and the recruitment of additional signaling partners. Along the temporal scale, the physiological effects exerted by oxytocin are both immediate and/or long-lasting. The immediate effects of oxytocin can be attributed to the specific signaling cascade it activates, leading to biochemical changes. The enduring effects exerted by OT, on the other hand, are due to its relatively long half-life and the cascading intracellular messenger pathways that lead to altered gene expression (Ludwig and Leng, 2006). Additionally, OT can transactivate members of the receptor tyrosine kinase family – TrkB, which is a receptor for brain-derived neurotrophic factor (BDNF) (Mitre et al., 2022) and epidermal growth factor receptor (EGFR) (Lin et al., 2012). OT-mediated TrkB transactivation leads to clustering of the scaffold protein gephyrin and mediates inhibitory responses. Moreover, in vitro and in vivo data suggest that G protein-coupled receptors, including OTR, can form heterodimers with other multiple G protein-coupled receptors, including dopamine D1 and D2, serotonin 2C, orexin, and melanocortin 3 receptors (MCR3), providing possible mechanisms for its many physiological effects (Ringuet et al., 2021).
Oxytocin and G protein signaling
Oxytocin receptor (OTR), a G-protein coupled receptor, binds to downstream coupling partners referred to as G proteins that contain three subunits – α, β and γ (Kamato et al., 2015). While all three subunits are crucial, the specificity of the downstream signaling is generally conferred by the engaged α subunit, which can be Gαq, Gαs, Gαi, and others. OTRs have been shown to couple to Gαq and Gαi/o subtypes of G proteins, which have contrasting effects on cellular activity and cAMP abundance. An important factor in determining the G protein coupling partner of OTR is the oxytocin concentration. At a low concentration of OT (∼ 2 nM), Gαq is the partner of choice, whereas, at a high OT concentration of ∼90 nM, OTR is coupled to GαoB. The coupling partners for the intermediate concentrations of OT (11 nM – 62 nM) include Gαi3, GαoA, Gαi1, and Gαi2 (Busnelli et al., 2013).
Of physiological relevance, OTR couples predominantly to Gαq/11 subunit. Downstream of Gαq/11 coupling, OTR stimulates intracellular calcium (Ca2+) mobilization through a phospholipase C (PLC)-dependent mechanism (Park et al., 1998; Gutkowska and Jankowski, 2012). PLC stimulates hydrolysis of the phospholipid phosphatidylinositol 4,5-bisphosphate (PIP2) to diacylglycerol (DAG), which in turn activates protein kinase C (PKC) and inositol 1,4,5-trisphosphate (IP3). This cascade of molecular signaling stimulates the release of intracellular Ca2+ stores via IP3 receptors and also activates other Ca2+-activated kinases (Thibonnier et al., 1999; Viero et al., 2010). However, there is a lot of heterogeneity at the level of the physiological outcome of OT-OTR activation. For example, in neuronal cells – an increase in Ca2+ concentration leads to the formation of Ca2+-calmodulin complexes which then activate neuronal isoforms of nitric oxide (NO) synthase. NO in turn stimulates the soluble guanylate cyclase to produce cGMP. In neurosecretory cells, rising Ca2+ levels regulate their firing pattern, thereby modulating cellular excitability and leading to transmitter release (Gimpl and Fahrenholz, 2001). Further, elevated Ca2+- levels can induce alterations in gene expression, both at the levels of transcription and protein synthesis (translation). Gβ γ subunits coupled to OTR are predominantly involved in the peripheral actions of the neuropeptide (Hoare et al., 1999; Zhong et al., 2003). In neurons, however, these subunits are implicated in modulating electrical activity including dissociated hippocampal neurons (Blumenstein et al., 2004) as well as in the brainstem and spinal cord (Yevenes et al., 2003).
Oxytocin receptor (OTR) coupling to different G proteins mediates diverse effects depending on the cellular context. Whole-cell patch-clamp recordings from the supraoptic nucleus in brain slices have demonstrated that OT induces Gαq/11-mediated calcium mobilization which is followed by subsequent spike frequency reduction in response to the progressive elevation in OT concentration (Wang and Hatton, 2007). Similarly, in the midbrain, OT directly increases the firing rate of dopamine neurons in the ventral tegmental area (VTA), which is consistent with the concept that OTR is primarily coupled to Gαq/11 protein (Xiao et al., 2017). Primary cerebellar neurons display OT-mediated modulation in the levels of neurexin and neuregulin. This involves the regulation of Rho GTPases and OTR coupling with Gαq/11 (Zatkova et al., 2016). Finally, In the GN11 cell line which is derived from olfactory neurons, OTR coupling to Gαq has been shown to decrease inward rectifying potassium K + (IRK) currents, however, in contrast, the opposite effect of increasing K + involves OTR coupling to Gαi/o in a different sub-population (Gravati et al., 2010). Thus, intracellular OTR coupling to specific G proteins determines the cellular response and activity to OT-OTR binding.
Oxytocin and synapse dynamics
The effect of OT on synaptic activity and synapse formation has been well established (Bakos et al., 2018). Neurodevelopmental disorders, including autism spectrum disorders, have various synaptopathies that present with deficient oxytocin signaling (Bakos et al., 2015). The multiple properties of synapses modulated by OT include synapse formation (Blumenstein et al., 2004), synapse stabilization (Lestanova et al., 2016), synapse number (Ripamonti et al., 2017), and synaptic transmission (Jo et al., 1998). Disrupted synaptogenesis and synapse functionality can result in impaired neuronal connectivity, circuit formation, and stability (Zatkova et al., 2016). OT modulates synaptic features by acting on local synaptic sites and induces context-dependent signaling cascades. At the presynaptic membrane, activation of OTR leads to increased intracellular Ca2+ concentration, which may result in increased neurotransmitter release (Bakos et al., 2018). This increase in Ca2+ could be due to Gα q-mediated activation of the phospholipase-C leading to inositol 1,4,5-trisphosphate receptor (IP3R) induced calcium release from intracellular sources (Lambert et al., 1994) and through inhibition of the potassium Kir7.1 channels, which induces calcium entry through the voltage-dependent calcium channel (York et al., 2017).
Oxytocin modulates long-term synaptic plasticity and protein synthesis
Oxytocin exerts its effects on emotional behaviors by recruiting converging pathways in the paraventricular nucleus that promote nascent protein synthesis (Blume et al., 2008; Knobloch et al., 2012), innervating for example the central amygdala, where OTR-expressing neurons express higher synaptic strength after proactive coping threat conditioning and freezing reduction (Terburg et al., 2018). Protein synthesis is tightly regulated at the level of initiation and elongation (Shrestha and Klann, 2022). OT promotes translation elongation by activating eukaryotic elongation factor 2 (eEF2) both in a hypothalamic cell line and in vivo within the PVN (Martinetz et al., 2019). Protein synthesis is required for long-term synaptic plasticity, which is a persistent change in the synaptic strength, manifested as either long-term potentiation (LTP) or long-term depression (LTD). OT has been shown to affect both forms of synaptic plasticity depending on the brain region. In the medial amygdala (MeA), OT strongly augments LTD induction in the afferents from the accessory olfactory bulb (AOB) (Gur et al., 2014). In the rat hippocampus, OT is involved in the maintenance of LTP, which is induced upon tetanic stimulation in the CA1 region. This effect of oxytocin is exerted via two pathways – the first pathway involves the conventional Gαq/11-coupled phospholipase C pathway, whereas the second pathway involves transactivated EGFR (Wang, 2016) downstream signaling mediated by phosphatidylinositol 3 kinase (PI3K), extracellular signal-regulated kinase 1/2 (ERK1/2) and mammalian target of Rapamycin complex I (mTORC1) (Tomizawa et al., 2003; Lin et al., 2012). Importantly OTR-mediated mediated enhancement of LTP is dependent on new protein synthesis (Lin et al., 2012), indicating that OT triggers long-lasting effects on cellular physiology – which is particularly relevant for long-term social and threat-related memories.
Limbic forebrain-based oxytocin signaling network in stress/threat responses
Oxytocin-oxytocin receptor (OT-OTR) interactions mediate neuromodulatory influence on specific neural motifs within the emotional limbic forebrain for the acquisition and expression of stress/threat responses (Figure 1B). OT modulation serves the important function of fine-tuning the salience of neuronal network activity, mainly facilitating GABA-dependent increase in signal-to-noise ratio (Owen et al., 2013; Mitre et al., 2016; Oettl et al., 2016; Tirko et al., 2018), although its role in glutamatergic and astrocyte modulation has been addressed recently (Tirko et al., 2018; Wahis et al., 2021). This can be demonstrated at the level of behavior using a differential threat conditioning paradigm in which animals are exposed to two different auditory stimuli, one that is associated with the imminence of a footshock (i.e., threat) and the other that predicts safety. Pavlovian threat conditioning generates cued freezing response to threat predictive stimulus whereas instrumental signaled active avoidance results in cued avoidance responses that dominate over freezing (LeDoux and Daw, 2018; Hasan et al., 2019). OT neuromodulation of pertinent neural circuits facilitates the discrimination between stimuli that predict threat and safety, respectively. Supported by human studies, OT has been shown to facilitate adaptive response to threats and to reduce maladaptive fear, notably in social contexts (Janeček and Dabrowska, 2019; Triana-Del Río et al., 2019; van den Burg and Hegoburu, 2020), and mainly through its OT releasing long-range axons that irrigate the limbic forebrain (Sofroniew, 1983; Knobloch et al., 2012). Although acute activation of OTR in the central nervous system promotes the attenuation of paralyzing threat responses (freezing), high doses of OT administered chronically elicit anxiety-like responses (Peters et al., 2014). This dose-dependent effect may be mediated by OT binding to arginine vasopressin (AVP) receptors, which have been associated with enhanced fear (Viviani et al., 2011; Jurek and Neumann, 2018).
Oxytocin (OT) signaling is also critical for the physiological and behavioral responses to different kinds of stressors, which can be broadly categorized into physical and psychological stressors. Physical stress represents potentially life-threatening bodily harm, such as electric footshock and forced swim stressors whereas psychological stress involves anticipation of pain or threat, for instance - maternal separation, immobilization, social defeat, and predation (Sandi and Haller, 2015). Depending on the context and the stressor type, OT either impedes the already operating stress response or is released simultaneously with the onset of a stressor to moderate the outcome of the stress response (Winter et al., 2021). OT signaling in brain regions within the limbic forebrain network, including the paraventricular thalamus, amygdala, prefrontal cortex, lateral septum, and bed nucleus of stria terminalis, orchestrate the neuromodulation of stress/threat responses (Table 1).
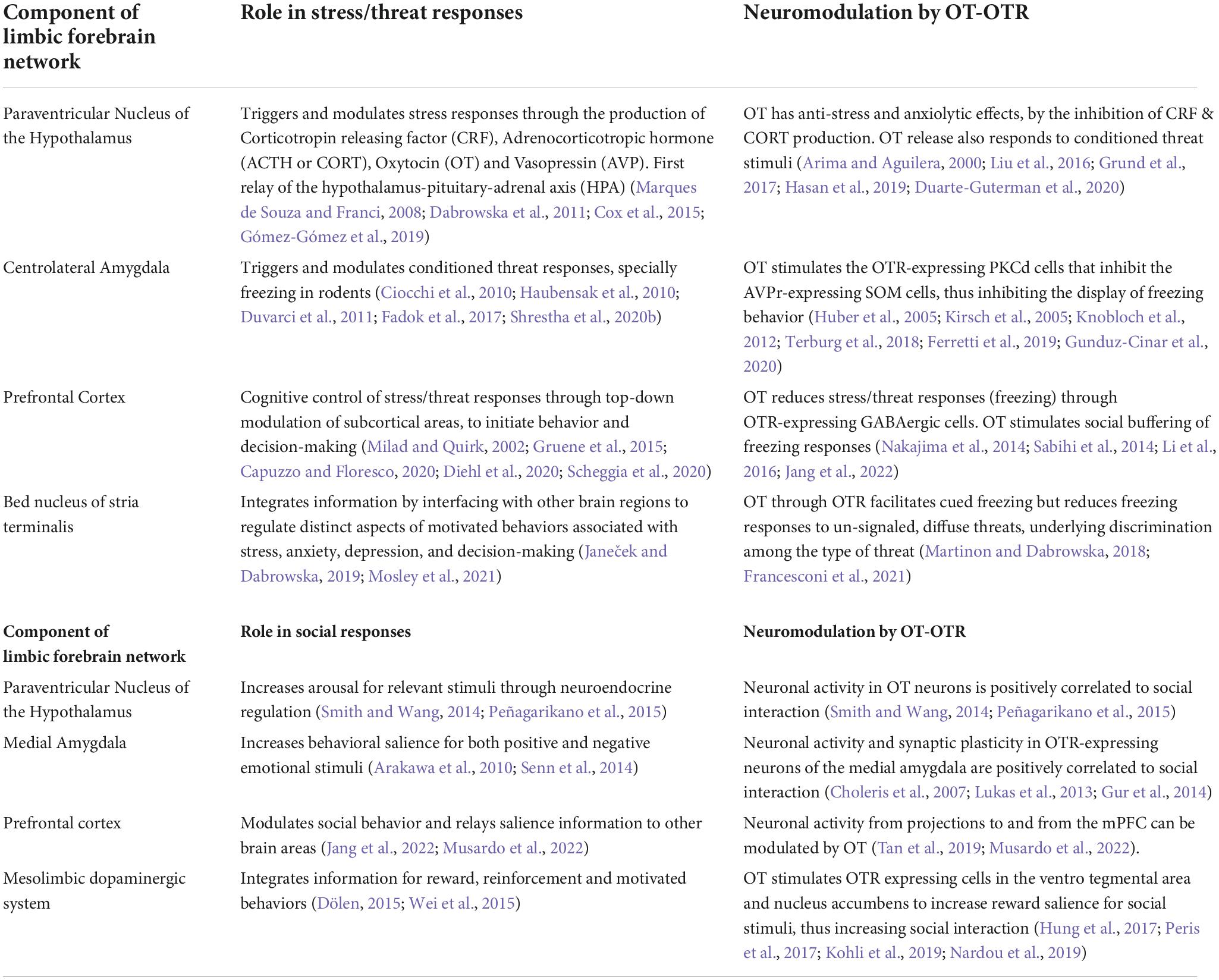
Table 1. OT-OTR signaling in limbic network modulates neuronal activity and behavioral salience in circuits for stress/threat and social responses.
Oxytocin is released by paraventricular nucleus during stress/threat responses
Oxytocin (OT) is released from the PVN during or immediately after, acute stress. Available rodent models of stress partially mimic the stress-induced pathophysiological and behavioral changes as seen in humans. In the acute model, the stressor is applied once and for a short time, while chronic stress involves repeated application of stressful stimuli over an extended period (Daviu et al., 2019). OT modulation of the stress response is particularly evident during periods of high endogenous OT levels such as the peripartum period (Slattery and Neumann, 2008). During this period, OT reduces the levels of corticotropin-releasing factor (CRF) in the PVN and the sympathetic nervous system as a physiological response to labor-induced stress. CRF release in PVN is the primary inducer of the hypothalamic-pituitary-adrenal (HPA) axis that activates generalized stress responses. For instance, in lactating women, the increase in OT during lactation appears to dampen the subsequent stress-induced secretion of corticosteroid (CORT) (Cox et al., 2015). The bidirectional inflection of OT and CRF in the PVN could underlie one of the OT-dependent neural mechanisms of stress-buffering. The cellular architecture of PVN comprises magnocellular and parvocellular neurosecretory cells, that have distinct morphology, electrophysiological properties, and pathways. While the magnocellular PVN cells innervate numerous forebrain regions and release neurohormones into the blood from the posterior pituitary, parvocellular neurosecretory cells in PVN regulate the anterior pituitary via projections to the medial eminence. Evidence for a direct interaction between the OT and CRF systems is based on the observation that parvocellular CRF neurons in the PVN co-express OT but not OTR, whereas magnocellular CRF neurons co-express CRF receptor (CRFR2), OT, and OTR, allowing these CRF neurons to respond to OT release and vice versa (Arima and Aguilera, 2000; Dabrowska et al., 2011).
Furthermore, chronic administration of OT modulates the expression of the crfr2 gene, leading to a reduction in CRFR2 membrane expression and eventually an anxiogenic phenotype (Winter et al., 2021). Additional anxiolytic mechanisms in the PVN related to OT include the stimulation of oxytocin secretion via GABA-B receptors (Marques de Souza and Franci, 2008) and activation of OT neurons via secretion of neuropeptide-S (Grund et al., 2017). Oxytocin also increases the pain threshold and stimulates various positive social interactions (Rahm et al., 2002; Uvnäs Moberg et al., 2020). During the acquisition of cued freeze suppression and/or extinction in threat conditioning paradigms, coordinated release of OT and glutamate from irrigated areas innervated by their axonal terminals to induce network plasticity in pertinent brain regions including the central amygdala, prefrontal cortex, lateral septum, and bed nucleus of the stria terminalis (BNST) (Knobloch et al., 2012; Hasan et al., 2019). Recent studies strongly suggest that the connectivity of the PVN to these areas involves experience-dependent control of spiking activity in the PVN, with hypothalamic OT neurons constituting a memory engram for the extinction of learned freezing responses (Hasan et al., 2019). Additionally, repeated exposure to OT produces long-lasting effects by affecting the activity of other transmission systems like dopamine, a mechanism that makes OT potentially clinically relevant. Interestingly, its function to modulate threat responses depends on the context and internal states. OT signals can either reduce or exacerbate such reactions, depending on the context, social environment, and hormonal status (Bosch and Neumann, 2012).
Oxytocin modulates stress/threat responses in the amygdala
The centrolateral nucleus of the amygdala (CeL) is implicated in the acquisition, storage, expression, and extinction of threat-dependent memories (Ciocchi et al., 2010; Haubensak et al., 2010; Duvarci et al., 2011; Fadok et al., 2017; Terburg et al., 2018; Winter et al., 2021) and the ensuing physiological response of retrieving those memories: freezing behavior (Ciocchi et al., 2010; Viviani et al., 2011; Whittle et al., 2021) or adaptive signaled avoidance (Fadok et al., 2017; Terburg et al., 2018; van den Burg and Hegoburu, 2020). Two prominent neuronal populations in the CeL differ in their reactivity to threat-predictive conditioned stimuli (CS): one population exhibits excitatory (CeL-On) while the other population exhibits inhibitory (CeL-Off) responses to the CS following Pavlovian threat conditioning (Ciocchi et al., 2010; Haubensak et al., 2010; Duvarci et al., 2011). Moreover, these cell types have been genetically identified and manipulated using cre- driver lines in rodents: CeL-On cells have been shown to express somatostatin (Som +) (Li et al., 2013; Penzo et al., 2015), and CeL-Off cells correspond to PKCδ + neurons, which in turn express the oxytocin receptor (Haubensak et al., 2010; Shrestha et al., 2020b). Further research has shown that, under baseline conditions, CeL-Off (PKCδ +) neurons exert a tonic inhibitory influence on cells within the centromedial nucleus (CeM), the output nucleus of the amygdala. Excitation of CeL-On (Som +) cells during CS would cause inhibition of CeL-Off neurons, resulting in disinhibition of freezing output neurons within CeM (Ciocchi et al., 2010; Haubensak et al., 2010; Fadok et al., 2017). Therefore, both CeL-On and CeL-Off neurons project to the CeM and inhibit each other (Ciocchi et al., 2010; Haubensak et al., 2010). However, an alternative view indicates a higher level of complexity for this network, in which connections between like neurons are stronger than those between different neuronal types (Hunt et al., 2017). Both CeL-Off (Som +) and CeL-On (PKCδ +) synapses are enhanced after Pavlovian threat conditioning for the expression of either freezing or active avoidance responses, respectively (Li et al., 2013; Penzo et al., 2014; Terburg et al., 2018). Therefore, these cell populations interact in a network with mutual inhibition and differentially encode memory acquisition with threat and safety cues by altering the cellular translation landscape (Shrestha et al., 2020b). In this study, blocking de novo protein synthesis in CeL-PKCδ interneurons disrupts the acquisition and consolidation of long-term inhibition of the conditioned freezing response and threat/safety discrimination (Shrestha et al., 2020b,a). Conversely, the inactivation of Som + neurons impedes the acquisition of freezing responses, while their optogenetic activation stimulates them. This also suggests that threat conditioning may disrupt competition between mutually inhibitory CeL neuron subtypes (Li et al., 2013; Penzo et al., 2014).
While OT can act on different subnuclei of the amygdala to induce freezing extinction/suppression (Gunduz-Cinar et al., 2020), the well-described mechanism of action has been done in the CeL – where PKCδ + cells are stimulated by OT and glutamate from the PVN and lateral amygdala (LA), respectively (Huber et al., 2005; Viviani et al., 2011; Terburg et al., 2018; Hasan et al., 2019). The coordinated release of OT and glutamate in CeL activates CeL-Off (PKCδ +) cells and subsequently inhibits CeL-On (Som +) cells, leading to the attenuation of conditioned freezing (Knobloch et al., 2012; Hasan et al., 2019; Wahis et al., 2021). Aside from OTR expression in PKCδ + inhibitory cells in CeL, recent reports suggest that a morphologically distinct subpopulation of astrocytes in CeL express OTR and these cells strongly mediate the anxiolytic and positive reinforcement effects of OT (Wahis et al., 2021). Consistent with this mechanism, OTR + cellular activation in CeL rescues adaptive avoidance behavior in rats deprived of inputs from the basolateral amygdala. In these animals, electrophysiological recordings showed an enhanced α-amino-3-hydroxy-5-methyl-4-isoxazolepropionic acid (AMPA)-dependent connectivity between the LA and CeL OTR + neurons but not OTR- neurons in high avoiders, i.e., animals that learned to avoid an imminent threat (Terburg et al., 2018). These findings highlight the role of OT within the centrolateral amygdala in modulating divergent defensive responses depending on the context and tone of threat/safety contingency (Ferretti et al., 2019).
Oxytocin modulates stress/threat responses in the prefrontal cortex
Neural network activity in the rodent medial prefrontal cortex (mPFC) has been associated with the acquisition of threat responses and their extinction. Along the dorsoventral axis, mPFC is divided into two subregions crucial for threat responses – the prelimbic cortex (PL) and the infralimbic cortex (IL). While PL promotes the expression of freezing, the IL is involved in its extinction (Milad and Quirk, 2002; Burgos-Robles et al., 2007; Sotres-Bayon and Quirk, 2010; Sierra-Mercado et al., 2011). Thus, these two subregions of the mPFC are differentially recruited during the perception of threat versus safety (Knapska et al., 2012; Cain, 2019). Consistent with these findings, functionally distinct neurons in the BLA project to either PL and IL regions, and mediate freezing acquisition and extinction, respectively (Senn et al., 2014; Vogel et al., 2016). mPFC networks are also responsible for the acquisition, expression, and extinction of adaptive avoidance via GABAergic modulation (Capuzzo and Floresco, 2020; Diehl et al., 2020).
Oxytocin (OT) plays an important role in modulating prefrontal GABAergic control of mPFC activity. This function of OT in mPFC circuits is crucial for extinguishing defensive threat reactions (freezing) in rodents and humans (Eckstein et al., 2015, 2019; Sabihi et al., 2017; Triana-Del Río et al., 2019). Among cortical interneurons, OTR is primarily expressed in Som + cells in the rodent mPFC (Nakajima et al., 2014; Li et al., 2016), however, OTR expression varies by sex and brain state. For instance, OTR expression increases in lactating females compared to virgins or male rodents (Mitre et al., 2016). In mPFC, OTR modulates stress responses in a sex-dependent manner, mainly through its interaction with CRF stress-dependent signaling (Li et al., 2016), and potentially with sex hormones like estradiol (Gruene et al., 2015). Also with ng this finding, in a subregion-specific manner, OT signaling reduces anxiety-like behavior through its action in PL but not in IL (Sabihi et al., 2014). Complementarily, blocking OTR in PL is anxiogenic in lactating dams but not in virgins. This mechanism appears to be GABA-dependent, as administration of OT in the PL was accompanied by increased activation of GABA neurons in the same area (Sabihi et al., 2017). This finding supports the observation that OTR is expressed primarily by PL SOM interneurons, which is also consistent with the idea that this cell population is responsible for controlling the discrimination of affective states in rodents (Scheggia et al., 2020).
Oxytocin modulates stress/threat responses in the bed nucleus of the stria terminalis
The bed nucleus of the stria terminalis (BNST) is evolutionarily and anatomically close to the CeL. Of the BNST cell types, type III consists mainly of OT-positive GABAergic neurons. In this area, OT modulates adaptive responses to threats and attenuates fearful reactions in animal models and human studies via a possible interaction with the CRF system (Janeček and Dabrowska, 2019; Mosley et al., 2021). Endogenous OT excites BNST interneurons and inhibits CeL-driven BNST output neurons by releasing GABA (Francesconi et al., 2021). The aforementioned neuromodulatory mechanism serves to enhance threat memory for a discrete cue or landmark (freezing behavior), enabling accurate and rapid adaptive responses to the threat, for example, in stress-induced social vigilance and adaptive avoidance (Martinon and Dabrowska, 2018; Steinman et al., 2019; Duque-Wilckens et al., 2020).
Limbic forebrain-based oxytocin signaling network in social behaviors
In addition to stress/threat responses, OT also plays a vital role in modulating social behaviors in rodents. Animals engage in a variety of social behaviors including social interaction, pair bonding, sexual behaviors, maternal care, and aggression (Jurek and Neumann, 2018). Among these behaviors, appetitive social behaviors toward a conspecific are determined using assays to measure social approach/avoidance, which refers to the animal’s tendency to engage in social interaction often in preference to exploring a non-social object. Motivation to engage in social approach/avoidance can be assessed as socially conditioned place preference (CPP). Social memory/recognition involves the initial sensing of a conspecific through sensory modalities followed by memory formation and recall of a previously encountered conspecific. Lastly, social threat-related responses to a conspecific involve identifying, recognizing a threat, and responding appropriately. There is compelling evidence that OT signaling plays an important role in mediating multiple aspects of social behaviors – encompassing social investigation, social motivation, social memory, and social threat.
Global manipulation of the OT system provides insight into its modulatory influence on social behaviors. Systemic administration of OT or OTR agonist (OT-A) increases time spent in social investigation and augments social preference in a social CPP task (Ramos et al., 2015; Sobota et al., 2015; Zhang et al., 2015; Kohli et al., 2019; Duarte-Guterman et al., 2020). Of note, this effect of OT is both sex-specific and age-dependent (Zhang et al., 2015; Dannenhoffer et al., 2018; Duarte-Guterman et al., 2020). Similarly, administration of an OTR antagonist decreases time spent in social investigation compared to vehicle-treated animals (Lukas et al., 2011). In a compelling study, investigators tested social discrimination in OT knockout (KO), OTR KO, and partial forebrain OTR KO mouse strains (OTR Fb KO). The transgenic subjects were all male mice of the C57BL/6J strain and tested with female Balb/c, C57BL/6J, and SW strains. Interestingly, OT KO and OTR KO mice showed impaired social memory in some strains and not others, whereas OTR Fb/Fb mice showed impaired social memory in all strains. This finding implies that OT plays a role in social recognition across different strains, who may exhibit distinct social cues and release diverse pheromones (Macbeth et al., 2009). While these studies elucidate the effects of the global OT system on social behaviors, brain region-specific manipulations of OT in the limbic forebrain have begun to reveal fascinating circuit mechanisms of this neuromodulator (Figure 1B and Table 1).
Oxytocin signaling through the paraventricular nucleus is essential for social behavior
Oxytocin (OT) signaling in the rodent hypothalamus has been strongly implicated in social behaviors. While OT is synthesized by multiple hypothalamic nuclei, the PVN OT network is particularly implicated in the social approach/avoidance and social recognition/memory. Activity in OT neurons in the PVN has been shown to increase during social interaction (Hung et al., 2017). In addition, OT mRNA abundance in PVN significantly decreased in mice that showed low levels of social interaction compared to mice that showed high levels of social interaction (Murakami et al., 2011). These findings demonstrate that OT activity in PVN is positively correlated with social behaviors. As previously discussed, chronic restraint stress leads to an increase in OT immunoreactive cells in the PVN. This effect of chronic stress is accompanied by an increase in social approach (Li et al., 2016). OT signaling within PVN is also associated with rewarding aspects of social investigation. For instance, in a social CPP test - mice that show social preference have higher OT gene expression in the PVN compared to controls (Liu et al., 2016). More direct evidence for the role of OT neurons in the PVN in social behavior comes from Resendez and colleagues (Resendez et al., 2020), who showed that chemogenetic activation of OT neurons in the mouse PVN enhances social investigation while chemogenetic inhibition of the same neurons abolishes social investigation. Two-photon calcium imaging of PVN OT neurons in behaving animals has further revealed that these neurons are activated by social stimuli and they differentially encode social and non-social stimuli (Resendez et al., 2020). Together, these findings indicate that OT signaling in the PVN affects the salience of social behaviors.
In addition, social stress increases the release of OT, following heightened PVN network activity. This leads to an increase in OT-OTR binding in networks such as the lateral septum, where OT modulates the expression and extinction of social-derived threat responses in lactating females, which appears to be augmented by sex hormones (Zoicas et al., 2014; Menon et al., 2018). Similarly, in the PVN of female rodents, increased OT release is correlated with high levels of maternal aggression (Bosch et al., 2005). Excessive or uncontrollable socially induced stress is associated with OT signaling impairments, which in turn correlate with high levels of anxiety-like behavior. Consistent with these studies, exogenous OT promotes resilience to social stress (Hale et al., 2021; Shi et al., 2021), primarily through the involvement of projections from PVN OT neurons to the prelimbic cortex (He et al., 2019).
Oxytocin signaling in the amygdala modulates social recognition/memory
Electroencephalogram (EEG) studies have shown that chronic OT administration leads to a decrease in high-frequency bands in the amygdala concurrent with increased social interaction compared to vehicle control. This indicates that OT might reduce local circuit activity within the amygdala, as effects on long-distance connectivity would have altered lower frequency bands (Sobota et al., 2015). Magnocellular OT neurons in the PVN project to the CeL and CeM subnuclei of the amygdala (Sofroniew, 1983). Besides the central amygdala, OTRs are also expressed in the BLA and medial amygdala (MeA) (Eckstein et al., 2015). To investigate the odor-induced recruitment of brain regions, Arakawa and colleagues (Arakawa et al., 2010) conducted an odor investigation test where a rat was placed in a cage with bedding that had the odor of a conspecific. Following the test, the authors carried out immunohistochemistry for cFos, a marker of cellular activity. This study showed that conspecific odor increases IEG expression in several brain regions including the olfactory bulb, MeA, BNST, and PVN. The increase in IEG expression was accompanied by an increased level of OTRs in the MeA and PVN. Further, they showed that infusion of OTR antagonist in the MeA decreases odor investigation compared to vehicle control. These findings provided compelling evidence for OT signaling within MeA in integrating conspecific odor-induced social investigation.
Moreover, OTR mRNA levels are significantly lowered in MeA of mice that showed low levels of social interaction compared to mice that had high levels of social interaction (Murakami et al., 2011). Ferguson and colleagues (Ferguson et al., 2002) examined cFos expression in OT KO and wild-type mice during a social learning task and reported similar activation in the main and accessory olfactory bulbs, the piriform cortex, and the cortical amygdala. Interestingly, wild-type mice had more activation in MeA compared to KO mice. Further, the BNST and medial preoptic area, which receive direct input from MeA, failed to show an increase in cFos expression in OT KO mice. Additional evidence for the role of MeA in social recognition comes from a study in female rats where re local infusion of antisense oligonucleotides targeting OTR resulted in impaired social memory (Choleris et al., 2007). Acute administration of OT into the MeA before memory acquisition rescued social memory (Ferguson et al., 2000), implying that local OT and OTR signaling in MeA are both necessary and sufficient for social recognition. Similar findings were reported in another study where the infusion of OTR antagonist in MeA impaired social memory in adult but not juvenile male rats (Lukas et al., 2013).
Oxytocin (OT)-induced protein synthesis is thought to be important for the consolidation of social memories. A study using social discrimination tasks found that blocking protein synthesis before memory acquisition blocked long-term social memory in rats. In vitro, exogenous administration of OT has been shown to enhance theta burst stimulation (TBS)-induced LTD in the anterior olfactory bulb (AOB)-MeA pathway. In vivo, TBS administered to the AOB before memory acquisition leads to social recognition deficits (Gur et al., 2014). Together, these findings show that MeA plays a crucial role in social memory and that OT modulates synaptic plasticity that is associated with social memory. More recent studies show that OT signaling in another amygdala subnucleus, BLA, affects social behavior. Social recognition was impaired in female mice that underwent early life stress, which coincided with an increase in dendritic complexity as well as an increase in OTR density within the BLA (Wei et al., 2015). Further, optogenetic stimulation of OTR-expressing PFC neurons that project to the BLA eliminated social recognition (Tan et al., 2019). Though these early studies are promising, more investigation into OT signaling in the BLA is needed to understand how this brain area is contributing to social approach and social memory.
Oxytocin signaling in the prefrontal cortex modulates social interaction and social threat
Oxytocin (OT) signaling in mPFC has been shown to modulate social behaviors such as social recognition and social threat. A recent study showed that a subset of glutamatergic neurons in mPFC projecting to BLA express OTRs, separately from cortical interneurons. This study demonstrated that optogenetic stimulation of the mPFC-BLA pathway impairs social recognition (Tan et al., 2019). Another study demonstrated that social isolation for over a week leads to increased subsequent social interaction, which is accompanied by increased excitatory neurotransmission from the ventral tegmental area (VTA) afferent to the mPFC. Chemogenetic inhibition of OT neurons in PVN abolishes social isolation-dependent modifications of synaptic efficacy and behavior, indicating a crucial role of OT signaling across VTA and mPFC for motivated social behavior (Musardo et al., 2022). Additionally, gene deletion of an inhibitory subunit of NMDA receptor (GluN3A KO) results in a significant reduction in OTR expression within mPFC and impaired social interaction in KO mice, which is rescued with exogenous OT administration (Lee et al., 2018).
Oxytocin (OT) signaling in mPFC also plays a key role in integrating social and threat-related information for optimal behavior outcomes. In a phenomenon known as social buffering, stress and fear responses are attenuated by acute or chronic social exposure. Jang and colleagues showed that paired rats exhibit reduced threat responses in a passive avoidance task and that OT infusion in the prelimbic cortex within mPFC of social-deprived rats results in a similar reduction in threat responses (Jang et al., 2022). Interestingly, social buffering of stress/threat response requires nascent protein synthesis in mPFC (Jang et al., 2022). Hence endogenous oxytocin seems to be fundamental for mediating the buffering effects of social interactions to diminish threat reactions like freezing (Ferrer-Pérez et al., 2020; van den Burg and Hegoburu, 2020).
Oxytocin modulation of the mesolimbic dopamine system in socio-emotional behaviors
The mesolimbic dopamine system connects the ventral tegmental area (VTA) with the ventral striatum, which includes the nucleus accumbens (NAc) and the olfactory tubercle. VTA in the telencephalon is populated by dopaminergic neurons and plays a significant role in reward, motivation, cognition, and aversion. On the other hand, the ventral striatum is populated by medium spiny neurons that express receptors for dopamine released by afferents from VTA. OTR is highly expressed in the mesolimbic dopamine circuit, including in the medial and lateral subdivisions of VTA. At a cellular level, OTRs are expressed in glutamatergic and dopaminergic neurons whose fibers project to the NAc, prefrontal cortex, and amygdala (Peris et al., 2017).
Direct activation of PVN-VTA projecting OT neurons leads to an increase in social investigation. On the other hand, inhibiting PVN-VTA OT neurons leads to abolished social preference in a social CPP test, indicating that this projection is required for social reward (Hung et al., 2017). Whole-cell recordings in the midbrain dopamine system have revealed that bath application of OT increases the firing rate of dopamine neurons in VTA (Xiao et al., 2017). Studies carried out on OT signaling in the striatum have similarly elucidated an important role of OT in mediating social behaviors. For example, Zhang and colleagues (Zhang et al., 2015) investigated the effects of OT infusion in the striatum and found that both males and females increase social interaction following the treatment. The same study also performed proteomics of striatum and found that protein levels of calcineurin and GAD67 were significantly altered upon OT administration. Specifically, calcineurin levels increased whereas GAD67 levels decreased in the striatum, indicating a possible role of OT in modulating excitatory-inhibitory balance in the striatum. Moreover, OT directly influences dopamine release in the mesolimbic circuit. Intraperitoneal injection of OT in rats leads to an increase in persistent dopamine release in the NAc (Kohli et al., 2019). Bath application of OT caused long-term depression (LTD) in medium spiny neurons in the NAc whereas application of an OTR antagonist occluded the LTD. Further investigation revealed that this synaptic plasticity was caused by a decrease in the probability of presynaptic neurotransmitter release. At the behavior level, infusion of OTR antagonist within the NAc of male mice prevented the preference for a socially conditioned context in a social CPP test (Dölen et al., 2013). The effects of OT on the mesolimbic dopamine system appear to be age-dependent. Administration of an OTR antagonist into the NAc, but not BLA, decreases time spent in the investigation of the novel conspecific, which shows that OT in the NAc is essential for motivating social behavior (Smith et al., 2017). Therefore, OT acts on the mesolimbic dopamine system to modulate reward-processing related to the social approach.
In addition to its influence on social behaviors, the mesolimbic dopamine system is recruited during contextual threat responses and is associated with neural circuit function in brain areas where OTRs are also expressed – such as the amygdala, prefrontal cortex, VTA, and striatum. There is evidence that dopaminergic neurons increase their spiking activity during the extinction of freezing responses and prevent the renewal of such responses (Bouchet et al., 2018). Dopamine also gates the associative learning of fear to switch from danger to safety conditioning (Groessl et al., 2018; Luo et al., 2018). Concerning stress-response, subchronic social isolation stress leads to OT-mediated strengthening of excitatory neurotransmission from VTA to mPFC (Musardo et al., 2022). Thus, the interactions between the mesolimbic dopamine system and OT signaling seem to be important not only for social behaviors but also for mediating stress/threat responses.
Oxytocin dysfunction and therapeutic potential in neuropsychiatric disorders
Oxytocin signaling has been implicated in a wide range of neuropsychiatric disorders, due to its crucial role in emotional and social behaviors. Among these disorders, autism spectrum disorders (ASD) are characterized by repeated or restricted interests and deficits in social interaction and social communication. Interestingly, the same brain regions that have been associated with stress/threat and social responses in rodents show abnormal activity in ASD patients (Dichter, 2012). In rodent models of ASD, OT administration has been shown to ameliorate social deficits (Peñagarikano et al., 2015; Wang et al., 2018; Hörnberg et al., 2020; Cherepanov et al., 2021). In clinical studies, intranasal OT has been used to treat ASD. So far, results have been mixed; some studies find that intranasal OT improves social deficits (Huang et al., 2021), while others report no effects on social deficits (Yamasue et al., 2020; Sikich et al., 2021). Some studies attribute the different responses to OT to differences in individual variability (Kosaka et al., 2016; Parker et al., 2017), though these results have also been challenged (Sikich et al., 2021). Further studies demonstrating the long-lasting effects of stress attenuation by OT are warranted, especially for social threats. This would provide the neurobiological basis for treatment options for social dysfunctions using exogenous OT (Neumann and Slattery, 2016).
Studies in animals strongly support the role of the OT-OTR system in the modulation of traumatic or threat memories (Triana-Del Río et al., 2019). In clinical studies, this system has been investigated in post-traumatic stress disorder (PTSD), an emotional disorder characterized by over-consolidation of traumatic/threat memories, generalization of fear, hyperactivity of the amygdala and attempts to avoid trauma-related cues. Findings from studies involving OT as a therapeutic strategy for PTSD show that an acute dose of intranasal OT increases neural responses to social reward (Nawijn et al., 2017), whereas repeated OT administration reduced symptoms of PTSD, which is correlated with decreased amygdala reactivity to fearful faces, and the attenuation of amygdala-PFC functional connectivity (Frijling, 2017). Intranasal administration of OT has been shown to trigger behavioral and neuronal responses related to threat memory processing in PTSD patients, suggesting that, similar to animal models, OT can amplify the acquisition and consolidation of threat/intrusive memories, on a comparable process of increasing the signal to noise ratio for relevant threat stimuli (Owen et al., 2013; Terburg et al., 2018). In these studies, the effects of exogenous OT are influenced by biological covariates, such as salivary cortisol, heart rate variability, sex, and PTSD polygenic risk scores (Schultebraucks et al., 2022). On the other hand, another group demonstrated that OT administration early after trauma did not attenuate PTSD symptoms in all trauma-exposed participants with acute distress; however, participants with high acute PTSD symptom severity did show beneficial effects of OT (van Zuiden et al., 2017). Following these efforts, Carmassi and colleagues (Carmassi et al., 2021) showed that baseline plasma OT levels are decreased in PTSD patients of both sexes compared to healthy controls. However, in another study, the change in peripheral OT levels did not differ by treatment condition and did not correspond to chana get in PTSD symptoms (Sippel et al., 2021).
Converging translational studies indicate that intranasal administration of OT reaches the brain to trigger physiological effects (Quintana et al., 2019, 2021; Winterton et al., 2021), correlated with a general OT-concentration increment in salivary, plasma, and cerebrospinal fluid samples (Striepens et al., 2013). However, it is still unknown if these effects reflect amplified endogenous OT release upon intranasal OT administration (as a result of positive feedback mechanisms) or the exogenously administered compound (Quintana et al., 2019, 2021). In clinical studies using OT, the statistical power is limited, thus, more systematization, larger sample sizes, and diversity in the sample populations are required, making emphasis on the exact duration of OT administration, its concentration, the dose-response effects, and the stress reactivity of the participants (Quintana et al., 2021; Winterton et al., 2021). Therefore, converging literature support the critical role of OT in social and emotional behaviors in both rodents and humans, but the therapeutic potential of OT on human neuropsychiatric disorders remains to be fully characterized.
Conclusion
While not comprehensive, we have highlighted here the studies that provide compelling evidence on how OT signaling in the limbic network modulates social and stress/threat-related behaviors. At the intersection of social behaviors and stress/threat response is social threat response when the conspecific partner is perceived as a threat. OT exerts an essential role in increasing the signal-to-noise ratio of sensory signals within a social context (Owen et al., 2013; Marlin et al., 2015; Tirko et al., 2018); this effect is potentiated when the social context becomes stressful or threatening context. OT provides resilience against social stress and socially derived threat responses. There are two prominent modulations of the OT system related to social threat, and both of them seem to relate to the increased OT release as a survival signal against the stress/threat context. First, OT is released during social buffering of acute stress responses. For example, OT signaling in the prelimbic cortex reduces freezing expression induced by acute social exposure (Jang et al., 2022), a phenomenon that has been observed in the neighboring anterior cingulate cortex (Burkett et al., 2016), and PVN (Smith and Wang, 2014), hence endogenous oxytocin seems to be fundamental for mediating the buffering effects of social interactions to diminish threat reactions like freezing (Ferrer-Pérez et al., 2020; van den Burg and Hegoburu, 2020). Secondly, social stress also increases the release of OT, as mentioned before for PVN network activity. This increases OTR binding in networks like the lateral septum, where OT modulates the expression and extinction of socially derived threat responses in lactating females, which appears to be tempered by sex hormones (Zoicas et al., 2014; Menon et al., 2018). In this brain area, OT signaling promotes rather exacerbated threat responses (freezing) and aggression responses to social threats (Guzmán et al., 2013; Meyer et al., 2020), similarly, in the PVN of rodent females, increased OT release is correlated to high levels of maternal aggression (Bosch et al., 2005). Excessive or uncontrollable socially induced stress is associated with OT signaling impairments, which are also correlated to high levels of anxiety-like behavior. This is described as the nucleus accumbens (Hou et al., 2020) or the mPFC (Shi et al., 2021). To counterbalance this effect, exogenous oxytocin promotes resilience to social stress (Hale et al., 2021; Shi et al., 2021), primarily through the involvement of projections from PVN OT neurons to PL (He et al., 2019). Therefore, coordinated regulation of neural circuits across the limbic forebrain is necessary for OT-mediated behavioral and physiological responses to social, stress, and threat-related stimuli when presented in isolation or together.
The crucial challenge in oxytocin research is determining how context-dependent intracellular signaling responses elicit a particular behavioral or physiological response. OTR-induced cellular responses and the signaling mechanisms in behaviorally relevant neural circuits may provide a better understanding of these effects. Behavioral neuroscience will significantly benefit from this knowledge. This knowledge of signaling cascades and secondary messengers will further aid in treatments using oxytocin and its analogs which will facilitate the design of better therapeutic interventions for neuropsychiatric disorders involving oxytocin.
Author contributions
RT-D, SR, JG, and PS: manuscript writing and proofreading. JL and EK: manuscript proofreading. All authors contributed to the article and approved the submitted version.
Funding
This study was supported by the Rodrigo Triana-Del Rio: Leon levy Fellowship; Joseph LeDoux:NIH-NIDA: R01 DA044445-01A1; Eric Klenn: NIH grant NS122316; and Prerana Shrestha:Sloan Fellowship #94485.
Conflict of interest
The authors declare that the research was conducted in the absence of any commercial or financial relationships that could be construed as a potential conflict of interest.
Publisher’s note
All claims expressed in this article are solely those of the authors and do not necessarily represent those of their affiliated organizations, or those of the publisher, the editors and the reviewers. Any product that may be evaluated in this article, or claim that may be made by its manufacturer, is not guaranteed or endorsed by the publisher.
References
Arakawa, H., Arakawa, K., and Deak, T. (2010). Oxytocin and vasopressin in the medial amygdala differentially modulate approach and avoidance behavior toward illness-related social odor. Neuroscience 171, 1141–1151. doi: 10.1016/j.neuroscience.2010.10.013
Arima, H., and Aguilera, G. (2000). Vasopressin and oxytocin neurons of hypothalamic supraoptic and paraventricular nuclei co-express mRNA for type-1 and type-2 corticotropin-releasing hormone receptors. J. Neuroendocrinol. 12, 833–842. doi: 10.1046/j.1365-2826.2000.00528.x
Bakos, J., Bacova, Z., Grant, S. G., Castejon, A. M., and Ostatnikova, D. (2015). Are molecules involved in neuritogenesis and axon guidance related to autism pathogenesis? Neuromol. Med. 17, 297–304.
Bakos, J., Srancikova, A., Havranek, T., and Bacova, Z. (2018). Molecular mechanisms of oxytocin signaling at the synaptic connection. Neural Plast. 2018:4864107.
Bartz, J. A., Zaki, J., Bolger, N., and Ochsner, K. N. (2011). Social effects of oxytocin in humans: Context and person matter. Trends Cogn. Sci. 15, 301–309.
Blume, A., Bosch, O. J., Miklos, S., Torner, L., Wales, L., Waldherr, M., et al. (2008). Oxytocin reduces anxiety via ERK1/2 activation: Local effect within the rat hypothalamic paraventricular nucleus. Eur. J. Neurosci. 27, 1947–1956. doi: 10.1111/j.1460-9568.2008.06184.x
Blumenstein, Y., Maximyuk, O. P., Lozovaya, N., Yatsenko, N. M., Kanevsky, N., Krishtal, O., et al. (2004). Intracellular Na+ inhibits voltage-dependent N-type Ca2+ channels by a G protein beta gamma subunit-dependent mechanism. J. Physiol. 556, 121–134. doi: 10.1113/jphysiol.2003.056168
Bosch, O. J., and Neumann, I. D. (2012). Both oxytocin and vasopressin are mediators of maternal care and aggression in rodents: From central release to sites of action. Horm. Behav. 61, 293–303. doi: 10.1016/j.yhbeh.2011.11.002
Bosch, O. J., Meddle, S. L., Beiderbeck, D. I., Douglas, A. J., and Neumann, I. D. (2005). Brain oxytocin correlates with maternal aggression: Link to anxiety. J. Neurosci. 25, 6807–6815.
Bouchet, C. A., Miner, M. A., Loetz, E. C., Rosberg, A. J., Hake, H. S., Farmer, C. E., et al. (2018). Activation of nigrostriatal dopamine neurons during fear extinction prevents the renewal of fear. Neuropsychopharmacology 43, 665–672. doi: 10.1038/npp.2017.235
Burgos-Robles, A., Vidal-Gonzalez, I., Santini, E., and Quirk, G. J. (2007). Consolidation of fear extinction requires NMDA receptor-dependent bursting in the ventromedial prefrontal cortex. Neuron 53, 871–880.
Burkett, J. P., Andari, E., Johnson, Z. V., Curry, D. C., de Waal, F. B. M., and Young, L. J. (2016). Oxytocin-dependent consolation behavior in rodents. Science 351, 375–378.
Busnelli, M., Bulgheroni, E., Manning, M., Kleinau, G., and Chini, B. (2013). Selective and potent agonists and antagonists for investigating the role of mouse oxytocin receptors. J. Pharmacol. Exp. Ther. 346, 318–327. doi: 10.1124/jpet.113.202994
Capuzzo, G., and Floresco, S. B. (2020). Prelimbic and infralimbic prefrontal regulation of active and inhibitory avoidance and reward-seeking. J. Neurosci. 40, 4773–4787. doi: 10.1523/JNEUROSCI.0414-20.2020
Carmassi, C., Marazziti, D., Mucci, F., Della Vecchia, A., Barberi, F. M., Baroni, S., et al. (2021). Decreased plasma oxytocin levels in patients with PTSD. Front. Psychol. 12:612338. doi: 10.3389/fpsyg.2021.612338
Carter, C. S. (1998). Neuroendocrine perspectives on social attachment and love. Psychoneuroendocrinology 23, 779–818. doi: 10.1016/s0306-4530(98)00055-9
Cherepanov, S. M., Gerasimenko, M., Yuhi, T., Furuhara, K., Tsuji, C., Yokoyama, S., et al. (2021). Oxytocin ameliorates impaired social behavior in a Chd8 haploinsufficiency mouse model of autism. BMC Neurosci. 22:32. doi: 10.1186/s12868-021-00631-6
Chini, B., Verhage, M., and Grinevich, V. (2017). The action radius of oxytocin release in the Mammalian CNS: From single vesicles to behavior. Trends Pharmacol. Sci. 38, 982–991. doi: 10.1016/j.tips.2017.08.005
Choleris, E., Little, S. R., Mong, J. A., Puram, S. V., Langer, R., and Pfaff, D. W. (2007). Microparticle-based delivery of oxytocin receptor antisense DNA in the medial amygdala blocks social recognition in female mice. Proc. Natl. Acad. Sci. U.S.A. 104, 4670–4675. doi: 10.1073/pnas.0700670104
Ciocchi, S., Herry, C., Grenier, F., Wolff, S. B. E., Letzkus, J. J., Vlachos, I., et al. (2010). Encoding of conditioned fear in central amygdala inhibitory circuits. Nature 468, 277–282.
Cox, E. Q., Stuebe, A., Pearson, B., Grewen, K., Rubinow, D., and Meltzer-Brody, S. (2015). Oxytocin and HPA stress axis reactivity in postpartum women. Psychoneuroendocrinology 55, 164–172.
Dabrowska, J., Hazra, R., Ahern, T. H., Guo, J.-D., McDonald, A. J., Mascagni, F., et al. (2011). Neuroanatomical evidence for reciprocal regulation of the corticotrophin-releasing factor and oxytocin systems in the hypothalamus and the bed nucleus of the stria terminalis of the rat: Implications for balancing stress and affect. Psychoneuroendocrinology 36, 1312–1326. doi: 10.1016/j.psyneuen.2011.03.003
Dannenhoffer, C. A., Kim, E. U., Saalfield, J., Werner, D. F., Varlinskaya, E. I., and Spear, L. P. (2018). Oxytocin and vasopressin modulation of social anxiety following adolescent intermittent ethanol exposure. Psychopharmacology 235, 3065–3077. doi: 10.1007/s00213-018-5003-8
Daviu, N., Bruchas, M. R., Moghaddam, B., Sandi, C., and Beyeler, A. (2019). Neurobiological links between stress and anxiety. Neurobiol. Stress 11:100191.
Dichter, G. S. (2012). Functional magnetic resonance imaging of autism spectrum disorders. Dialogues Clin. Neurosci. 14, 319–351.
Diehl, M. M., Iravedra-Garcia, J. M., Morán-Sierra, J., Rojas-Bowe, G., Gonzalez-Diaz, F. N., Valentín-Valentín, V. P., et al. (2020). Divergent projections of the prelimbic cortex bidirectionally regulate active avoidance. Elife 9:e59281. doi: 10.7554/eLife.59281
Dölen, G., Darvishzadeh, A., Huang, K. W., and Malenka, R. C. (2013). Social reward requires the coordinated activity of nucleus accumbens oxytocin and serotonin. Nature 501, 179–184. doi: 10.1038/nature12518
Duarte-Guterman, P., Lieblich, S. E., Qiu, W., Splinter, J. E. J., Go, K. A., Casanueva-Reimon, L., et al. (2020). Oxytocin has sex-specific effects on social behaviour and hypothalamic oxytocin immunoreactive cells but not hippocampal neurogenesis in adult rats. Horm. Behav. 122:104734. doi: 10.1016/j.yhbeh.2020.104734
Duque-Wilckens, N., Torres, L. Y., Yokoyama, S., Minie, V. A., Tran, A. M., Petkova, S. P., et al. (2020). Extrahypothalamic oxytocin neurons drive stress-induced social vigilance and avoidance. Proc. Natl. Acad. Sci. U.S.A. 117, 26406–26413. doi: 10.1073/pnas.2011890117
Duvarci, S., Popa, D., and Paré, D. (2011). Central amygdala activity during fear conditioning. J. Neurosci. 31, 289–294.
Eckstein, M., Almeida de Minas, A. C., Scheele, D., Kreuder, A.-K., Hurlemann, R., Grinevich, V., et al. (2019). Oxytocin for learning calm and safety. Int. J. Psychophysiol. 136, 5–14. doi: 10.1016/j.ijpsycho.2018.06.004
Eckstein, M., Becker, B., Scheele, D., Scholz, C., Preckel, K., Schlaepfer, T. E., et al. (2015). Oxytocin facilitates the extinction of conditioned fear in humans. Biol. Psychiatry 78, 194–202.
Fadok, J. P., Krabbe, S., Markovic, M., Courtin, J., Xu, C., Massi, L., et al. (2017). A competitive inhibitory circuit for selection of active and passive fear responses. Nature 542, 96–100. doi: 10.1038/nature21047
Ferguson, J. N., Young, L. J., and Insel, T. R. (2002). The neuroendocrine basis of social recognition. Front. Neuroendocrinol. 23, 200–224. doi: 10.1006/frne.2002.0229
Ferguson, J. N., Young, L. J., Hearn, E. F., Matzuk, M. M., Insel, T. R., and Winslow, J. T. (2000). Social amnesia in mice lacking the oxytocin gene. Nat. Genet. 25, 284–288.
Ferrer-Pérez, C., Reguilón, M. D., Miñarro, J., and Rodríguez-Arias, M. (2020). Endogenous oxytocin is essential for the buffering effects of pair housing against the increase in cocaine reward induced by social stress. Physiol. Behav. 221:112913. doi: 10.1016/j.physbeh.2020.112913
Ferretti, V., Maltese, F., Contarini, G., Nigro, M., Bonavia, A., Huang, H., et al. (2019). Oxytocin signaling in the central amygdala modulates emotion discrimination in mice. Curr. Biol. 29, 1938–1953.e6. doi: 10.1016/j.cub.2019.04.070
Francesconi, W., Berton, F., Olivera-Pasilio, V., and Dabrowska, J. (2021). Oxytocin excites BNST interneurons and inhibits BNST output neurons to the central amygdala. Neuropharmacology 192:108601. doi: 10.1016/j.neuropharm.2021.108601
Frijling, J. L. (2017). Preventing PTSD with oxytocin: Effects of oxytocin administration on fear neurocircuitry and PTSD symptom development in recently trauma-exposed individuals. Eur. J. Psychotraumatol. 8:1302652. doi: 10.1080/20008198.2017.1302652
Gimpl, G., and Fahrenholz, F. (2001). The oxytocin receptor system: Structure, function, and regulation. Physiol. Rev. 81, 629–683.
Gómez-Gómez, Y. M., Sánchez-Aparicio, P., Mejía-Chávez, S., García-García, F., Pascual-Mathey, L. I., Aguilera-Reyes, U., et al. (2019). c-Fos immunoreactivity in the hypothalamus and reward system of young rats after social novelty exposure. Neuroreport 30, 510–515. doi: 10.1097/WNR.0000000000001236
Gravati, M., Busnelli, M., Bulgheroni, E., Reversi, A., Spaiardi, P., Parenti, M., et al. (2010). Dual modulation of inward rectifier potassium currents in olfactory neuronal cells by promiscuous G protein coupling of the oxytocin receptor. J. Neurochem. 114, 1424–1435. doi: 10.1111/j.1471-4159.2010.06861.x
Groessl, F., Munsch, T., Meis, S., Griessner, J., Kaczanowska, J., Pliota, P., et al. (2018). Dorsal tegmental dopamine neurons gate associative learning of fear. Nat. Neurosci. 21, 952–962. doi: 10.1038/s41593-018-0174-5
Gruene, T. M., Roberts, E., Thomas, V., Ronzio, A., and Shansky, R. M. (2015). Sex-specific neuroanatomical correlates of fear expression in prefrontal-amygdala circuits. Biol. Psychiatry 78, 186–193. doi: 10.1016/j.biopsych.2014.11.014
Grund, T., Goyon, S., Li, Y., Eliava, M., Liu, H., Charlet, A., et al. (2017). Neuropeptide S activates paraventricular oxytocin neurons to induce anxiolysis. J. Neurosci. 37, 12214–12225.
Gunduz-Cinar, O., Brockway, E. T., Castillo, L. I., Pollack, G. A., Erguven, T., and Holmes, A. (2020). Selective sub-nucleus effects of intra-amygdala oxytocin on fear extinction. Behav. Brain Res. 393:112798. doi: 10.1016/j.bbr.2020.112798
Gur, R., Tendler, A., and Wagner, S. (2014). Long-term social recognition memory is mediated by oxytocin-dependent synaptic plasticity in the medial amygdala. Biol. Psychiatry 76, 377–386. doi: 10.1016/j.biopsych.2014.03.022
Gutkowska, J., and Jankowski, M. (2012). Oxytocin revisited: Its role in cardiovascular regulation. J. Neuroendocrinol. 24, 599–608.
Guzmán, Y. F., Tronson, N. C., Jovasevic, V., Sato, K., Guedea, A. L., Mizukami, H., et al. (2013). Fear-enhancing effects of septal oxytocin receptors. Nat. Neurosci. 16, 1185–1187. doi: 10.1038/nn.3465
Hale, L. H., Tickerhoof, M. C., and Smith, A. S. (2021). Chronic intranasal oxytocin reverses stress-induced social avoidance in female prairie voles. Neuropharmacology 198:108770. doi: 10.1016/j.neuropharm.2021.108770
Hasan, M. T., Althammer, F., Silva da Gouveia, M., Goyon, S., Eliava, M., Lefevre, A., et al. (2019). A fear memory engram and its plasticity in the hypothalamic oxytocin system. Neuron 103, 133–146.e8. doi: 10.1016/j.neuron.2019.04.029
Haubensak, W., Kunwar, P. S., Cai, H., Ciocchi, S., Wall, N. R., Ponnusamy, R., et al. (2010). Genetic dissection of an amygdala microcircuit that gates conditioned fear. Nature 468, 270–276. doi: 10.1038/nature09553
He, Z., Young, L., Ma, X.-M., Guo, Q., Wang, L., Yang, Y., et al. (2019). Increased anxiety and decreased sociability induced by paternal deprivation involve the PVN-PrL OTergic pathway. Elife 8:e44026. doi: 10.7554/eLife.44026
Hoare, S., Copland, J. A., Strakova, Z., Ives, K., Jeng, Y. J., Hellmich, M. R., et al. (1999). The proximal portion of the COOH terminus of the oxytocin receptor is required for coupling to g(q), but not g(i). Independent mechanisms for elevating intracellular calcium concentrations from intracellular stores. J. Biol. Chem. 274, 28682–28689.
Hörnberg, H., Pérez-Garci, E., Schreiner, D., Hatstatt-Burklé, L., Magara, F., Baudouin, S., et al. (2020). Rescue of oxytocin response and social behaviour in a mouse model of autism. Nature 584, 252–256. doi: 10.1038/s41586-020-2563-7
Hou, W., He, Z., Yang, Y., Yuan, W., Wang, L., Zhang, J., et al. (2020). The involvement of oxytocin in the effects of chronic social defeat stress on emotional behaviours in adult female mandarin voles. Eur. J. Neurosci. 52, 2853–2872.
Huang, Y., Huang, X., Ebstein, R. P., and Yu, R. (2021). Intranasal oxytocin in the treatment of autism spectrum disorders: A multilevel meta-analysis. Neurosci. Biobehav. Rev. 122, 18–27. doi: 10.1016/j.neubiorev.2020.12.028
Huber, D., Veinante, P., and Stoop, R. (2005). Vasopressin and oxytocin excite distinct neuronal populations in the central amygdala. Science 308, 245–248.
Hung, L. W., Neuner, S., Polepalli, J. S., Beier, K. T., Wright, M., Walsh, J. J., et al. (2017). Gating of social reward by oxytocin in the ventral tegmental area. Science 357, 1406–1411.
Hunt, S., Sun, Y., Kucukdereli, H., Klein, R., and Sah, P. (2017). Intrinsic circuits in the lateral central amygdala. eNeuro 4. doi: 10.1523/ENEURO.0367-16.2017
Janeček, M., and Dabrowska, J. (2019). Oxytocin facilitates adaptive fear and attenuates anxiety responses in animal models and human studies-potential interaction with the corticotropin-releasing factor (CRF) system in the bed nucleus of the stria terminalis (BNST). Cell Tissue Res. 375, 143–172. doi: 10.1007/s00441-018-2889-8
Jang, M., Jung, T., Jeong, Y., Byun, Y., and Noh, J. (2022). Oxytocin modulation in the medial prefrontal cortex of pair-exposed rats during fear conditioning. Psychoneuroendocrinology 141:105752. doi: 10.1016/j.psyneuen.2022.105752
Jiménez, A., Young, L. J., Triana-Del Río, R., LaPrairie, J. L., and González-Mariscal, G. (2015). Neuroanatomical distribution of oxytocin receptor binding in the female rabbit forebrain: Variations across the reproductive cycle. Brain Res. 1629, 329–339. doi: 10.1016/j.brainres.2015.10.043
Jo, Y. H., Stoeckel, M. E., Freund-Mercier, M. J., and Schlichter, R. (1998). Oxytocin modulates glutamatergic synaptic transmission between cultured neonatal spinal cord dorsal horn neurons. J. Neurosci. 18, 2377–2386. doi: 10.1523/JNEUROSCI.18-07-02377.1998
Jurek, B., and Neumann, I. D. (2018). The oxytocin receptor: From intracellular signaling to behavior. Physiol. Rev. 98, 1805–1908.
Kamato, D., Thach, L., Bernard, R., Chan, V., Zheng, W., Kaur, H., et al. (2015). Structure, function, pharmacology, and therapeutic potential of the G protein, Gα/q,11. Front. Cardiovasc. Med. 2:14. doi: 10.3389/fcvm.2015.00014
Kirsch, P., Esslinger, C., Chen, Q., Mier, D., Lis, S., Siddhanti, S., et al. (2005). Oxytocin modulates neural circuitry for social cognition and fear in humans. J. Neurosci. 25, 11489–11493. doi: 10.1523/JNEUROSCI.3984-05.2005
Knapska, E., Macias, M., Mikosz, M., Nowak, A., Owczarek, D., Wawrzyniak, M., et al. (2012). Functional anatomy of neural circuits regulating fear and extinction. Proc. Natl. Acad. Sci. U.S.A. 109, 17093–17098.
Knobloch, H. S., Charlet, A., Hoffmann, L. C., Eliava, M., Khrulev, S., Cetin, A. H., et al. (2012). Evoked axonal oxytocin release in the central amygdala attenuates fear response. Neuron 73, 553–566. doi: 10.1016/j.neuron.2011.11.030
Kohli, S., King, M. V., Williams, S., Edwards, A., Ballard, T. M., Steward, L. J., et al. (2019). Oxytocin attenuates phencyclidine hyperactivity and increases social interaction and nucleus accumben dopamine release in rats. Neuropsychopharmacology 44, 295–305. doi: 10.1038/s41386-018-0171-0
Kosaka, H., Okamoto, Y., Munesue, T., Yamasue, H., Inohara, K., Fujioka, T., et al. (2016). Oxytocin efficacy is modulated by dosage and oxytocin receptor genotype in young adults with high-functioning autism: A 24-week randomized clinical trial. Transl. Psychiatry 6:e872. doi: 10.1038/tp.2016.152
Lambert, R. C., Dayanithi, G., Moos, F. C., and Richard, P. (1994). A rise in the intracellular Ca2+ concentration of isolated rat supraoptic cells in response to oxytocin. J. Physiol. 478(Pt 2), 275–287.
Landgraf, R., and Neumann, I. D. (2004). Vasopressin and oxytocin release within the brain: A dynamic concept of multiple and variable modes of neuropeptide communication. Front. Neuroendocrinol. 25, 150–176. doi: 10.1016/j.yfrne.2004.05.001
LeDoux, J., and Daw, N. D. (2018). Surviving threats: Neural circuit and computational implications of a new taxonomy of defensive behaviour. Nat. Rev. Neurosci. 19, 269–282. doi: 10.1038/nrn.2018.22
Lee, J. H., Zhang, J. Y., Wei, Z. Z., and Yu, S. P. (2018). Impaired social behaviors and minimized oxytocin signaling of the adult mice deficient in the N-methyl-d-aspartate receptor GluN3A subunit. Exp. Neurol. 305, 1–12. doi: 10.1016/j.expneurol.2018.02.015
Lestanova, Z., Bacova, Z., Kiss, A., Havranek, T., Strbak, V., and Bakos, J. (2016). Oxytocin increases neurite length and expression of cytoskeletal proteins associated with neuronal growth. J. Mol. Neurosci. 59, 184–192. doi: 10.1007/s12031-015-0664-9
Li, H., Penzo, M. A., Taniguchi, H., Kopec, C. D., Huang, Z. J., and Li, B. (2013). Experience-dependent modification of a central amygdala fear circuit. Nat. Neurosci. 16, 332–339.
Li, K., Nakajima, M., Ibañez-Tallon, I., and Heintz, N. (2016). A cortical circuit for sexually dimorphic oxytocin-dependent anxiety behaviors. Cell 167, 60–72.e11. doi: 10.1016/j.cell.2016.08.067
Lin, Y.-T., Huang, C.-C., and Hsu, K.-S. (2012). Oxytocin promotes long-term potentiation by enhancing epidermal growth factor receptor-mediated local translation of protein kinase Mζ. J. Neurosci. 32, 15476–15488. doi: 10.1523/JNEUROSCI.2429-12.2012
Liu, C., Wang, J., Zhan, B., and Cheng, G. (2016). Neuronal activity and the expression of hypothalamic oxytocin and vasopressin in social versus cocaine conditioning. Behav. Brain Res. 310, 84–92. doi: 10.1016/j.bbr.2016.05.010
Ludwig, M., and Leng, G. (2006). Dendritic peptide release and peptide-dependent behaviours. Nat. Rev. Neurosci. 7, 126–136. doi: 10.1038/nrn1845
Ludwig, M., Sabatier, N., Bull, P. M., Landgraf, R., Dayanithi, G., and Leng, G. (2002). Intracellular calcium stores regulate activity-dependent neuropeptide release from dendrites. Nature 418, 85–89.
Lukas, M., Toth, I., Reber, S. O., Slattery, D. A., Veenema, A. H., and Neumann, I. D. (2011). The neuropeptide oxytocin facilitates pro-social behavior and prevents social avoidance in rats and mice. Neuropsychopharmacology 36, 2159–2168. doi: 10.1038/npp.2011.95
Lukas, M., Toth, I., Veenema, A. H., and Neumann, I. D. (2013). Oxytocin mediates rodent social memory within the lateral septum and the medial amygdala depending on the relevance of the social stimulus: Male juvenile versus female adult conspecifics. Psychoneuroendocrinology 38, 916–926. doi: 10.1016/j.psyneuen.2012.09.018
Luo, R., Uematsu, A., Weitemier, A., Aquili, L., Koivumaa, J., McHugh, T. J., et al. (2018). A dopaminergic switch for fear to safety transitions. Nat. Commun. 9:2483. doi: 10.1038/s41467-018-04784-7
Macbeth, A. H., Lee, H.-J., Edds, J., and Young, W. S. III (2009). Oxytocin and the oxytocin receptor underlie intrastrain, but not interstrain, social recognition. Genes Brain Behav. 8, 558–567. doi: 10.1111/j.1601-183X.2009.00506.x
Mantella, R. C., Vollmer, R. R., Li, X., and Amico, J. A. (2003). Female oxytocin-deficient mice display enhanced anxiety-related behavior. Endocrinology 144, 2291–2296.
Marlin, B. J., Mitre, M., D’amour, J. A., Chao, M. V., and Froemke, R. C. (2015). Oxytocin enables maternal behaviour by balancing cortical inhibition. Nature 520, 499–504.
Marques de Souza, L., and Franci, C. R. (2008). GABAergic mediation of stress-induced secretion of corticosterone and oxytocin, but not prolactin, by the hypothalamic paraventricular nucleus. Life Sci. 83, 686–692. doi: 10.1016/j.lfs.2008.09.007
Martinetz, S., Meinung, C.-P., Jurek, B., von Schack, D., van den Burg, E. H., Slattery, D. A., et al. (2019). De Novo protein synthesis mediated by the eukaryotic elongation factor 2 is required for the anxiolytic effect of oxytocin. Biol. Psychiatry 85, 802–811. doi: 10.1016/j.biopsych.2019.01.010
Martinon, D., and Dabrowska, J. (2018). Corticotropin-releasing factor receptors modulate oxytocin release in the dorsolateral bed nucleus of the stria terminalis (BNST) in male rats. Front. Neurosci. 12:183. doi: 10.3389/fnins.2018.00183
Menon, R., Grund, T., Zoicas, I., Althammer, F., Fiedler, D., Biermeier, V., et al. (2018). Oxytocin signaling in the lateral septum prevents social fear during lactation. Curr. Biol. 28, 1066–1078.e6. doi: 10.1016/j.cub.2018.02.044
Meyer, M. A. A., Anstötz, M., Ren, L. Y., Fiske, M. P., Guedea, A. L., Grayson, V. S., et al. (2020). Stress-related memories disrupt sociability and associated patterning of hippocampal activity: A role of hilar oxytocin receptor-positive interneurons. Transl. Psychiatry 10:428. doi: 10.1038/s41398-020-01091-y
Milad, M. R., and Quirk, G. J. (2002). Neurons in medial prefrontal cortex signal memory for fear extinction. Nature 420, 70–74.
Mitre, M., Marlin, B. J., Schiavo, J. K., Morina, E., Norden, S. E., Hackett, T. A., et al. (2016). A distributed network for social cognition enriched for oxytocin receptors. J. Neurosci. 36, 2517–2535. doi: 10.1523/JNEUROSCI.2409-15.2016
Mitre, M., Saadipour, K., Williams, K., Khatri, L., Froemke, R. C., and Chao, M. V. (2022). Transactivation of TrkB receptors by oxytocin and its G protein-coupled receptor. Front. Mol. Neurosci. 15:891537. doi: 10.3389/fnmol.2022.891537
Mosley, P. E., Windels, F., Morris, J., Coyne, T., Marsh, R., Giorni, A., et al. (2021). A randomised, double-blind, sham-controlled trial of deep brain stimulation of the bed nucleus of the stria terminalis for treatment-resistant obsessive-compulsive disorder. Transl. Psychiatry 11:190. doi: 10.1038/s41398-021-01307-9
Murakami, G., Hunter, R. G., Fontaine, C., Ribeiro, A., and Pfaff, D. (2011). Relationships among estrogen receptor, oxytocin and vasopressin gene expression and social interaction in male mice. Eur. J. Neurosci. 34, 469–477. doi: 10.1111/j.1460-9568.2011.07761.x
Musardo, S., Contestabile, A., Knoop, M., Baud, O., and Bellone, C. (2022). Oxytocin neurons mediate the effect of social isolation via the VTA circuits. Elife 11:e73421. doi: 10.7554/eLife.73421
Nakajima, M., Görlich, A., and Heintz, N. (2014). Oxytocin modulates female sociosexual behavior through a specific class of prefrontal cortical interneurons. Cell 159, 295–305. doi: 10.1016/j.cell.2014.09.020
Nardou, R., Lewis, E. M., Rothhaas, R., Xu, R., Yang, A., Boyden, E., et al. (2019). Oxytocin-dependent reopening of a social reward learning critical period with MDMA. Nature 569, 116–120. doi: 10.1038/s41586-019-1075-9
Nawijn, L., van Zuiden, M., Koch, S. B. J., Frijling, J. L., Veltman, D. J., and Olff, M. (2017). Intranasal oxytocin increases neural responses to social reward in post-traumatic stress disorder. Soc. Cogn. Affect. Neurosci. 12, 212–223. doi: 10.1093/scan/nsw123
Neumann, I. D. (2008). Brain oxytocin: A key regulator of emotional and social behaviours in both females and males. J. Neuroendocrinol. 20, 858–865.
Neumann, I. D., and Slattery, D. A. (2016). Oxytocin in general anxiety and social fear: A translational approach. Biol. Psychiatry 79, 213–221.
Oettl, L.-L., Ravi, N., Schneider, M., Scheller, M. F., Schneider, P., Mitre, M., et al. (2016). Oxytocin enhances social recognition by modulating cortical control of early olfactory processing. Neuron 90, 609–621. doi: 10.1016/j.neuron.2016.03.033
Owen, S. F., Tuncdemir, S. N., Bader, P. L., Tirko, N. N., Fishell, G., and Tsien, R. W. (2013). Oxytocin enhances hippocampal spike transmission by modulating fast-spiking interneurons. Nature 500, 458–462. doi: 10.1038/nature12330
Park, E. S., Won, J. H., Han, K. J., Suh, P. G., Ryu, S. H., Lee, H. S., et al. (1998). Phospholipase C-delta1 and oxytocin receptor signalling: Evidence of its role as an effector. Biochem. J. 331(Pt 1), 283–289. doi: 10.1042/bj3310283
Parker, K. J., Oztan, O., Libove, R. A., Sumiyoshi, R. D., Jackson, L. P., Karhson, D. S., et al. (2017). Intranasal oxytocin treatment for social deficits and biomarkers of response in children with autism. Proc. Natl. Acad. Sci. U.S.A. 114, 8119–8124.
Pedersen, C. A., Vadlamudi, S. V., Boccia, M. L., and Amico, J. A. (2006). Maternal behavior deficits in nulliparous oxytocin knockout mice. Genes Brain Behav. 5, 274–281. doi: 10.1111/j.1601-183X.2005.00162.x
Peñagarikano, O., Lázaro, M. T., Lu, X.-H., Gordon, A., Dong, H., Lam, H. A., et al. (2015). Exogenous and evoked oxytocin restores social behavior in the Cntnap2 mouse model of autism. Sci. Transl. Med. 7:271ra8. doi: 10.1126/scitranslmed.3010257
Penzo, M. A., Robert, V., and Li, B. (2014). Fear conditioning potentiates synaptic transmission onto long-range projection neurons in the lateral subdivision of central amygdala. J. Neurosci. 34, 2432–2437. doi: 10.1523/JNEUROSCI.4166-13.2014
Penzo, M. A., Robert, V., Tucciarone, J., De Bundel, D., Wang, M., Van Aelst, L., et al. (2015). The paraventricular thalamus controls a central amygdala fear circuit. Nature 519, 455–459.
Peris, J., MacFadyen, K., Smith, J. A., de Kloet, A. D., Wang, L., and Krause, E. G. (2017). Oxytocin receptors are expressed on dopamine and glutamate neurons in the mouse ventral tegmental area that project to nucleus accumbens and other mesolimbic targets. J. Comp. Neurol. 525, 1094–1108. doi: 10.1002/cne.24116
Peters, S., Slattery, D. A., Uschold-Schmidt, N., Reber, S. O., and Neumann, I. D. (2014). Dose-dependent effects of chronic central infusion of oxytocin on anxiety, oxytocin receptor binding and stress-related parameters in mice. Psychoneuroendocrinology 42, 225–236. doi: 10.1016/j.psyneuen.2014.01.021
Quintana, D. S., Lischke, A., Grace, S., Scheele, D., Ma, Y., and Becker, B. (2021). Advances in the field of intranasal oxytocin research: Lessons learned and future directions for clinical research. Mol. Psychiatry 26, 80–91. doi: 10.1038/s41380-020-00864-7
Quintana, D. S., Westlye, L. T., Alnæs, D., Kaufmann, T., Mahmoud, R. A., Smerud, K. T., et al. (2019). Low-dose intranasal oxytocin delivered with Breath Powered device modulates pupil diameter and amygdala activity: A randomized controlled pupillometry and fMRI study. Neuropsychopharmacology 44, 306–313. doi: 10.1038/s41386-018-0241-3
Rahm, V.-A., Hallgren, A., Högberg, H., Hurtig, I., and Odlind, V. (2002). Plasma oxytocin levels in women during labor with or without epidural analgesia: A prospective study. Acta Obstet. Gynecol. Scand. 81, 1033–1039.
Ramos, L., Hicks, C., Caminer, A., Goodwin, J., and McGregor, I. S. (2015). Oxytocin and MDMA (‘Ecstasy’) enhance social reward in rats. Psychopharmacology 232, 2631–2641.
Resendez, S. L., Namboodiri, V. M. K., Otis, J. M., Eckman, L. E. H., Rodriguez-Romaguera, J., Ung, R. L., et al. (2020). Social stimuli induce activation of oxytocin neurons within the paraventricular nucleus of the hypothalamus to promote social behavior in male mice. J. Neurosci. 40, 2282–2295. doi: 10.1523/JNEUROSCI.1515-18.2020
Ringuet, M. T., Furness, J. B., and Furness, S. G. B. (2021). G protein-coupled receptor interactions and modification of signalling involving the ghrelin receptor, GHSR1a. J. Neuroendocrinol. 9:e13077. doi: 10.1111/jne.13077
Ripamonti, S., Ambrozkiewicz, M. C., Guzzi, F., Gravati, M., Biella, G., Bormuth, I., et al. (2017). Transient oxytocin signaling primes the development and function of excitatory hippocampal neurons. Elife 6:e22466. doi: 10.7554/eLife.22466
Sabihi, S., Dong, S. M., Durosko, N. E., and Leuner, B. (2014). Oxytocin in the medial prefrontal cortex regulates maternal care, maternal aggression and anxiety during the postpartum period. Front. Behav. Neurosci. 8:258. doi: 10.3389/fnbeh.2014.00258
Sabihi, S., Dong, S. M., Maurer, S. D., Post, C., and Leuner, B. (2017). Oxytocin in the medial prefrontal cortex attenuates anxiety: Anatomical and receptor specificity and mechanism of action. Neuropharmacology 125, 1–12. doi: 10.1016/j.neuropharm.2017.06.024
Sandi, C., and Haller, J. (2015). Stress and the social brain: Behavioural effects and neurobiological mechanisms. Nat. Rev. Neurosci. 16, 290–304.
Scheggia, D., Managò, F., Maltese, F., Bruni, S., Nigro, M., Dautan, D., et al. (2020). Somatostatin interneurons in the prefrontal cortex control affective state discrimination in mice. Nat. Neurosci. 23, 47–60. doi: 10.1038/s41593-019-0551-8
Schultebraucks, K., Maslahati, T., Wingenfeld, K., Hellmann-Regen, J., Kraft, J., Kownatzki, M., et al. (2022). Intranasal oxytocin administration impacts the acquisition and consolidation of trauma-associated memories: A double-blind randomized placebo-controlled experimental study in healthy women. Neuropsychopharmacology 47, 1046–1054. doi: 10.1038/s41386-021-01247-4
Senn, V., Wolff, S. B. E., Herry, C., Grenier, F., Ehrlich, I., Gründemann, J., et al. (2014). Long-range connectivity defines behavioral specificity of amygdala neurons. Neuron 81, 428–437.
Shi, D.-D., Zhang, Y.-D., Ren, Y.-Y., Peng, S.-Y., Yuan, T.-F., and Wang, Z. (2021). Predictable maternal separation confers adult stress resilience via the medial prefrontal cortex oxytocin signaling pathway in rats. Mol. Psychiatry 26, 7296–7307. doi: 10.1038/s41380-021-01293-w
Shrestha, P., and Klann, E. (2022). Spatiotemporally resolved protein synthesis as a molecular framework for memory consolidation. Trends Neurosci. 45, 297–311. doi: 10.1016/j.tins.2022.01.004
Shrestha, P., Shan, Z., Mamcarz, M., Ruiz, K. S. A., Zerihoun, A. T., Juan, C.-Y., et al. (2020b). Amygdala inhibitory neurons as loci for translation in emotional memories. Nature 586, 407–411. doi: 10.1038/s41586-020-2793-8
Shrestha, P., Ayata, P., Herrero-Vidal, P., Longo, F., Gastone, A., LeDoux, J. E., et al. (2020a). Cell-type-specific drug-inducible protein synthesis inhibition demonstrates that memory consolidation requires rapid neuronal translation. Nat. Neurosci. 23, 281–292. doi: 10.1038/s41593-019-0568-z
Sierra-Mercado, D., Padilla-Coreano, N., and Quirk, G. J. (2011). Dissociable roles of prelimbic and infralimbic cortices, ventral hippocampus, and basolateral amygdala in the expression and extinction of conditioned fear. Neuropsychopharmacology 36, 529–538. doi: 10.1038/npp.2010.184
Sikich, L., Kolevzon, A., King, B. H., McDougle, C. J., Sanders, K. B., Kim, S.-J., et al. (2021). Intranasal oxytocin in children and adolescents with autism spectrum disorder. N. Engl. J. Med. 385, 1462–1473.
Sippel, L. M., Flanagan, J. C., Holtzheimer, P. E., Moran-Santa-Maria, M. M., Brady, K. T., and Joseph, J. E. (2021). Effects of intranasal oxytocin on threat- and reward-related functional connectivity in men and women with and without childhood abuse-related PTSD. Psychiatry Res. Neuroimaging 317:111368. doi: 10.1016/j.pscychresns.2021.111368
Slattery, D. A., and Neumann, I. D. (2008). No stress please! Mechanisms of stress hyporesponsiveness of the maternal brain. J. Physiol. 586, 377–385. doi: 10.1113/jphysiol.2007.145896
Smith, A. S., and Wang, Z. (2014). Hypothalamic oxytocin mediates social buffering of the stress response. Biol. Psychiatry 76, 281–288.
Smith, C. J. W., Mogavero, J. N., Tulimieri, M. T., and Veenema, A. H. (2017). Involvement of the oxytocin system in the nucleus accumbens in the regulation of juvenile social novelty-seeking behavior. Horm. Behav. 93, 94–98. doi: 10.1016/j.yhbeh.2017.05.005
Sobota, R., Mihara, T., Forrest, A., Featherstone, R. E., and Siegel, S. J. (2015). Oxytocin reduces amygdala activity, increases social interactions, and reduces anxiety-like behavior irrespective of NMDAR antagonism. Behav. Neurosci. 129, 389–398. doi: 10.1037/bne0000074
Sofroniew, M. V. (1983). “Morphology of vasopressin and oxytocin neurones and their central and vascular projections,” in Progress in brain research, eds B. A. Cross and G. Leng (Amsterdam: Elsevier), 101–114.
Sotres-Bayon, F., and Quirk, G. J. (2010). Prefrontal control of fear: More than just extinction. Curr. Opin. Neurobiol. 20, 231–235.
Steinman, M. Q., Duque-Wilckens, N., and Trainor, B. C. (2019). Complementary neural circuits for divergent effects of oxytocin: Social approach versus social anxiety. Biol. Psychiatry 85, 792–801. doi: 10.1016/j.biopsych.2018.10.008
Striepens, N., Kendrick, K. M., Hanking, V., Landgraf, R., Wüllner, U., Maier, W., et al. (2013). Elevated cerebrospinal fluid and blood concentrations of oxytocin following its intranasal administration in humans. Sci. Rep. 3:3440.
Tan, Y., Singhal, S. M., Harden, S. W., Cahill, K. M., Nguyen, D.-T. M., Colon-Perez, L. M., et al. (2019). Oxytocin receptors are expressed by glutamatergic prefrontal cortical neurons that selectively modulate social recognition. J. Neurosci. 39, 3249–3263. doi: 10.1523/JNEUROSCI.2944-18.2019
Terburg, D., Scheggia, D., Triana Del Rio, R., Klumpers, F., Ciobanu, A. C., Morgan, B., et al. (2018). The Basolateral amygdala is essential for rapid escape: A human and rodent study. Cell 175, 723–735.e16. doi: 10.1016/j.cell.2018.09.028
Thibonnier, M., Berti-Mattera, L. N., Dulin, N., Conarty, D. M., and Mattera, R. (1999). “Signal transduction pathways of the human V1-vascular, V2-renal, V3-pituitary vasopressin and oxytocin receptors,” in Progress in brain research, eds I. J. A. Urban, J. P. H. Burbach, and D. De Wed (Amsterdam: Elsevier), 147–161.
Tirko, N. N., Eyring, K. W., Carcea, I., Mitre, M., Chao, M. V., Froemke, R. C., et al. (2018). Oxytocin transforms firing mode of CA2 hippocampal neurons. Neuron 100, 593–608.e3. doi: 10.1016/j.neuron.2018.09.008
Tomizawa, K., Iga, N., Lu, Y.-F., Moriwaki, A., Matsushita, M., Li, S.-T., et al. (2003). Oxytocin improves long-lasting spatial memory during motherhood through MAP kinase cascade. Nat. Neurosci. 6, 384–390. doi: 10.1038/nn1023
Triana-Del Río, R., van den Burg, E., Stoop, R., and Hegoburu, C. (2019). Acute and long-lasting effects of oxytocin in cortico-limbic circuits: Consequences for fear recall and extinction. Psychopharmacology 236, 339–354. doi: 10.1007/s00213-018-5030-5
Uvnäs Moberg, K., Ekström-Bergström, A., Buckley, S., Massarotti, C., Pajalic, Z., Luegmair, K., et al. (2020). Maternal plasma levels of oxytocin during breastfeeding-A systematic review. PLoS One 15:e0235806. doi: 10.1371/journal.pone.0235806
van den Burg, E. H., and Hegoburu, C. (2020). Modulation of expression of fear by oxytocin signaling in the central amygdala: From reduction of fear to regulation of defensive behavior style. Neuropharmacology 173:108130. doi: 10.1016/j.neuropharm.2020.108130
van Zuiden, M., Frijling, J. L., Nawijn, L., Koch, S. B. J., Goslings, J. C., Luitse, J. S., et al. (2017). Intranasal oxytocin to prevent posttraumatic stress disorder symptoms: A randomized controlled trial in emergency department patients. Biol. Psychiatry 81, 1030–1040.
Viero, C., Shibuya, I., Kitamura, N., Verkhratsky, A., Fujihara, H., Katoh, A., et al. (2010). REVIEW: Oxytocin: Crossing the bridge between basic science and pharmacotherapy. CNS Neurosci. Ther. 16, e138–e156. doi: 10.1111/j.1755-5949.2010.00185.x
Viviani, D., Charlet, A., van den Burg, E., Robinet, C., Hurni, N., Abatis, M., et al. (2011). Oxytocin selectively gates fear responses through distinct outputs from the central amygdala. Science 333, 104–107. doi: 10.1126/science.1201043
Vogel, E., Krabbe, S., Gründemann, J., Wamsteeker Cusulin, J. I., and Lüthi, A. (2016). Projection-specific dynamic regulation of inhibition in amygdala micro-circuits. Neuron 91, 644–651. doi: 10.1016/j.neuron.2016.06.036
Wahis, J., Baudon, A., Althammer, F., Kerspern, D., Goyon, S., Hagiwara, D., et al. (2021). Astrocytes mediate the effect of oxytocin in the central amygdala on neuronal activity and affective states in rodents. Nat. Neurosci. 24, 529–541. doi: 10.1038/s41593-021-00800-0
Wang, Y., Zhao, S., Liu, X., Zheng, Y., Li, L., and Meng, S. (2018). Oxytocin improves animal behaviors and ameliorates oxidative stress and inflammation in autistic mice. Biomed. Pharmacother. 107, 262–269. doi: 10.1016/j.biopha.2018.07.148
Wang, Y.-F., and Hatton, G. I. (2007). Interaction of extracellular signal-regulated protein kinase 1/2 with actin cytoskeleton in supraoptic oxytocin neurons and astrocytes: Role in burst firing. J. Neurosci. 27, 13822–13834. doi: 10.1523/JNEUROSCI.4119-07.2007
Wang, Z. (2016). Transactivation of epidermal growth factor receptor by G protein-coupled receptors: Recent progress, challenges and future research. Int. J. Mol. Sci. 17:95. doi: 10.3390/ijms17010095
Wei, D., Lee, D., Cox, C. D., Karsten, C. A., Peñagarikano, O., Geschwind, D. H., et al. (2015). Endocannabinoid signaling mediates oxytocin-driven social reward. Proc. Natl. Acad. Sci. U.S.A. 112, 14084–14089. doi: 10.1073/pnas.1509795112
Whittle, N., Fadok, J., MacPherson, K. P., Nguyen, R., Botta, P., Wolff, S. B. E., et al. (2021). Central amygdala micro-circuits mediate fear extinction. Nat. Commun. 12:4156. doi: 10.1038/s41467-021-24068-x
Winter, J., Meyer, M., Berger, I., Royer, M., Bianchi, M., Kuffner, K., et al. (2021). Chronic oxytocin-driven alternative splicing of Crfr2α induces anxiety. Mol. Psychiatry doi: 10.1038/s41380-021-01141-x
Winterton, A., Westlye, L. T., Steen, N. E., Andreassen, O. A., and Quintana, D. S. (2021). Improving the precision of intranasal oxytocin research. Nat. Hum. Behav. 5, 9–18.
Xiao, L., Priest, M. F., Nasenbeny, J., Lu, T., and Kozorovitskiy, Y. (2017). Biased oxytocinergic modulation of midbrain dopamine systems. Neuron 95, 368–384.e5. doi: 10.1016/j.neuron.2017.06.003
Yamasue, H., Okada, T., Munesue, T., Kuroda, M., Fujioka, T., Uno, Y., et al. (2020). Effect of intranasal oxytocin on the core social symptoms of autism spectrum disorder: A randomized clinical trial. Mol. Psychiatry 25, 1849–1858.
Yevenes, G. E., Peoples, R. W., Tapia, J. C., Parodi, J., Soto, X., Olate, J., et al. (2003). Modulation of glycine-activated ion channel function by G-protein betagamma subunits. Nat. Neurosci. 6, 819–824. doi: 10.1038/nn1095
York, N., Halbach, P., Chiu, M. A., Bird, I. M., Pillers, D.-A. M., and Pattnaik, B. R. (2017). Oxytocin (OXT)-stimulated inhibition of Kir7.1 activity is through PIP2-dependent Ca2+ response of the oxytocin receptor in the retinal pigment epithelium in vitro. Cell. Signal. 37, 93–102. doi: 10.1016/j.cellsig.2017.06.005
Zatkova, M., Bakos, J., Hodosy, J., and Ostatnikova, D. (2016). Synapse alterations in autism: Review of animal model findings. Biomed. Pap. Med. Fac. Univ. Palacky Olomouc Czech. Repub. 160, 201–210.
Zhang, X., Li, Q., Zhang, M., Lam, S., Sham, P. C., Bu, B., et al. (2015). The effect of oxytocin on social and non-social behaviour and striatal protein expression in C57BL/6N mice. PLoS One 10:e0145638. doi: 10.1371/journal.pone.0145638
Zhong, M., Yang, M., and Sanborn, B. M. (2003). Extracellular signal-regulated kinase 1/2 activation by myometrial oxytocin receptor involves Galpha(q)Gbetagamma and epidermal growth factor receptor tyrosine kinase activation. Endocrinology 144, 2947–2956.
Keywords: oxytocin, oxytocin receptor (OTR), intracellular cascades, social behavior, emotional behavior, threat response, stress response
Citation: Triana-Del Rio R, Ranade S, Guardado J, LeDoux J, Klann E and Shrestha P (2022) The modulation of emotional and social behaviors by oxytocin signaling in limbic network. Front. Mol. Neurosci. 15:1002846. doi: 10.3389/fnmol.2022.1002846
Received: 25 July 2022; Accepted: 22 September 2022;
Published: 17 November 2022.
Edited by:
Navjot Kaur, Yale University, United StatesReviewed by:
Lei Xiao, Fudan University, ChinaIris Müller, Otto von Guericke University Magdeburg, Germany
Copyright © 2022 Triana-Del Rio, Ranade, Guardado, LeDoux, Klann and Shrestha. This is an open-access article distributed under the terms of the Creative Commons Attribution License (CC BY). The use, distribution or reproduction in other forums is permitted, provided the original author(s) and the copyright owner(s) are credited and that the original publication in this journal is cited, in accordance with accepted academic practice. No use, distribution or reproduction is permitted which does not comply with these terms.
*Correspondence: Rodrigo Triana-Del Rio, cnQ4MkBueXUuZWR1; Prerana Shrestha, cHJlcmFuYS5zaHJlc3RoYUBzdG9ueWJyb29rLmVkdQ==