- 1 Stem Cell Biology Laboratory, Institute of Molecular Biology and Genetics, Biomedical Sciences Research Center “Alexander Fleming,” Athens, Greece
- 2 Stowers Institute for Medical Research, Kansas City, MO, USA
- 3 Division of Stem Cell Biology and Developmental Genetics, Medical Research Council National Institute for Medical Research, London, UK
- 4 Institute of Oral Biology, ZZM, Faculty of Medicine, University of Zurich, Zurich, Switzerland
- 5 Department of Anatomy and Cell Biology, University of Kansas Medical Center, Kansas City, KS, USA
Embryonic cortical neural stem cells are self-renewing progenitors that can differentiate into neurons and glia. We generated neurospheres from the developing cerebral cortex using a mouse genetic model that allows for lineage selection and found that the self-renewing neural stem cells are restricted to Sox2 expressing cells. Under normal conditions, embryonic cortical neurospheres are heterogeneous with regard to Sox2 expression and contain astrocytes, neural stem cells, and neural progenitor cells sufficiently plastic to give rise to neural crest cells when transplanted into the hindbrain of E1.5 chick and E8 mouse embryos. However, when neurospheres are maintained under lineage selection, such that all cells express Sox2, neural stem cells maintain their Pax6+ cortical radial glia identity and exhibit a more restricted fate in vitro and after transplantation. These data demonstrate that Sox2 preserves the cortical identity and regulates the plasticity of self-renewing Pax6+ radial glia cells.
Introduction
Sox genes encode transcriptional regulators with HMG box DNA-binding domains, and are involved in specifying cell fates (Kamachi et al., 2000; Scaffidi and Bianchi, 2001; Wilson and Koopman, 2002; Wegner and Stolt, 2005). Sox1, Sox2, and Sox3 (SoxB1 subfamily; Wood and Episkopou, 1999; Kamachi et al., 2000) are expressed broadly within the primitive neuroepithelium during embryogenesis and typically mark uncommitted precursors within the developing (Wood and Episkopou, 1999; Avilion et al., 2003) and adult central nervous system (CNS; Ferri et al., 2004; Brazel et al., 2005).
Gain of function experiments in the chick showed that SoxB1 factors maintain the neural progenitor (NP) state and inhibits differentiation of spinal cord precursors. In contrast, suppression of SoxB1 function leads to premature cell cycle exit and initiation of neuronal differentiation (Bylund et al., 2003; Graham et al., 2003; Kan et al., 2004; Sandberg et al., 2005). In mice, SoxB1 loss of function mutations failed to reveal the role of these genes in NSCs since they result in either early lethality (Sox2) or no obvious NSC phenotypes (Sox1 and Sox3; Nishiguchi et al., 1998; Avilion et al., 2003; Malas et al., 2003; Rizzoti et al., 2004; Ekonomou et al., 2005). Recent findings suggested that Sox1 maintains cortical NP cells undifferentiated by suppressing cell cycle exit to neurogenesis (Elkouris et al., 2011). Sox2 hypomorphic mouse mutants exhibit impaired neurogenesis in the adult brain together with neurodegeneration (Ferri et al., 2004). Similarly, conditional ablation of Sox2 also caused defects in adult neurogenesis, particularly in hippocampal development and NSC maintenance which is sonic hedgehog (Shh) dependent (Favaro et al., 2009; Pevny and Nicolis, 2010). However, the precise role of Sox2 in embryonic NSCs is still elusive.
Cortical NSC can be cultured ex vivo as neurospheres which are heterogenous free-floating aggregates consisting of mixed populations of stem, progenitor, and differentiated cells. These cells eventually lose their regional identity in culture (Ellis et al., 2004; Brazel et al., 2005; Ahmed, 2009; Conti and Cattaneo, 2010), which raises important questions about the signals required for their maintenance and differentiation properties in vitro and in vivo. We used a mouse genetic model (Sox2βgeo) to investigate the molecular properties and the plasticity of a homogeneous population of cortical NSCs obtained by selection for Sox2 expression (Li et al., 1998; Zhao et al., 2004).
Materials and Methods
Mice
Sox2βgeo/+ and Sox2βgeo2/+ were maintained and genotyped as previously described (Avilion et al., 2003; Ekonomou et al., 2005). All experiments carried out on mice were approved under the UK Animal (scientific procedures) Act (Project license 80/1949; National Institute for Medical Research), the Animals Act 160/03.05.1991/revised 86/609/EEC/24.11.1986 EU directive for Animal Experimentation (Prot. No. 767/28.02.07; BSRC “Alexander Fleming”) and the IACUC animal welfare guidelines and approved protocols and licenses (Stowers Institute for Medical Research).
Neurosphere Cultures
Neurospheres were derived and maintained as previously described (Zappone et al., 2000; Elkouris et al., 2011). Cortices from Sox2βgeo/+ mice at E14.5 were dissected in ice cold DMEM–F12 medium (GIBCO-BRL) supplemented with 2 mM glutamine and antibiotics before they were triturated in DMEM–F12 medium containing 0.6% glucose, 9.6 mg/ml putrescine (SIGMA), 6.3 ng/ml progesterone (SIGMA), 5.2 ng/ml sodium selenite (SIGMA), 25 mg/ml insulin (SIGMA), 100 mg/ml transferrin (SIGMA), 20 ng/ml each of bFGF and EGF (R&D systems), 2 mM glutamine and antibiotics (NSC medium; Zappone et al., 2000). Cell suspensions were plated at clonal density (104 cells/ml) in NSC medium and primary neurospheres were grown and maintained in this medium for 3 weeks. When required, G418 was added at a final concentration of 250 mg/ml. β-galactosidase activity was assayed as described (Zappone et al., 2000; Elkouris et al., 2011). At 3 weeks, β-galactosidase+ cells were present as isolated cells (<2% of cells), in coherent patches (2–50% of cells) or dispersed throughout (>50% of cells) in 3–5, 5–7, and approximately 90% of Sox2βgeo/+ neurosphere cultures, respectively. For differentiation, single neurospheres were plated on a matrigel support and allowed to differentiate for 10 days. For the FACS analysis, Sox2βgeo/+ neurospheres were dissociated and single cells were incubated with CMFDG substrate (Molecular Probes) for 30 min on ice according to the instructions of the manufacturer, before they were subjected to cell sorting for β-galactosidase activity on a MoFlo cell sorter (Cytomation). Sox2+ (LacZ+) and Sox2− (LacZ−) cells were cultured as single cell suspension in serial dilutions and allowed to form neurospheres in the absence of G418 selection.
Immunohistochemistry
Immunohistochemistry was performed on differentiated cells fixed with 100% methanol. Whole-mount immunofluorescence was performed on live (RC2 staining) and either methanol or MEMFA (Avilion et al., 2003) fixed neurospheres. On average about 10 neurospheres were used per individual experiment with two to seven replicates. Antibody incubation was generally performed in phosphate buffered saline (PBS) solution containing 1% BSA, 0.15% glycine, and 0.1% Triton-X100 at 4°C overnight or for 1–2 h at room temperature. Images from intact neurospheres with representative staining were recorded on a Leica TCS SP confocal microscope using the TCSNT software.
Antibodies
Primary antibodies were: anti-Sox2 (rabbit purified IgG; 1:2500, R. Lovell-Badge); anti-Pax6 (mouse IgG 1:500; Chemicon); anti-nestin (mouse IgG 1:75; DSHB); anti-GFAP, Cy3-linked (mouse IgG 1:100; SIGMA); mouse RC2 (mouse IgM 1:100; DSHB); anti-βIII tubulin (TuJ1; mouse IgG 1:1000; BabCo); anti-Sox9 (rabbit purified IgG 4796; 1:1000; a gift from S. Guioli); and anti-Sox10 (mouse IgG 1:10; a gift from D. Anderson). AlexaFluor 488, 594, 555, and 647 chromophores were used with secondary antibodies (Molecular Probes). Photographs were acquired with Leica SP2 (NIMR, London) and SP5 (BFRAA, Athens) confocal microscopes using ALasAF Software (Leica).
Neurosphere Transplantations and Mouse and Chick Embryo Culture
Neurospheres were removed from culture and labeled by incubation in DiI labeling solution (0.05% w/v Dil in 0.3 M sucrose stock, diluted 1:1 in DMEM) for up to 5 min. Neurospheres were then washed multiple times in DMEM (for mouse transplants) or Ringer’s solution (for chick embryos). Depending on their size, neurospheres were either dissected into smaller pieces using glass needles for transplantation into mouse embryos or in the case of chick embryos they were transplanted as whole neurospheres at the levels of rhombomere (r) 2 and r4 in the hindbrain.
Mouse embryos were collected from timed pregnant CD1 mice at 8.5 dpc for in vitro whole embryo culture. Following dissection of conceptuses from the uterus, the parietal yolk sac was removed leaving the embryo with an intact visceral yolk sac amnion and ectoplacental cone. Post neurosphere transplantation with 0.30 μm glass needles, mouse embryos were cultured in DMEM culture medium supplemented with 50% rat serum, L-glutamine and penicillin/streptomycin (DR50) for 24 h in small glass bottles attached to a rotating drum (BTC engineering, Cambridge) at 37°C with a constant atmosphere of 5% O2, 5% CO2, 90% N2 (Sturm and Tam, 1993).
Fertilized chick eggs were incubated for approximately 36 h at 37°C in a humidified incubator to obtain embryos of the eight somite stage or earlier. Individual eggs were windowed and the embryos were visualized via injection of India Ink (1:10 dilution in Ringer’s Solution). The vitelline membrane covering the hindbrain was opened using tungsten needles after which a small slit was made in the midline of the neural tube at the desired axial level in the hindbrain. Post neurosphere transplantation, host chick embryo eggs were resealed with clear tape and returned to a 37°C incubator for either 24–48 h or up to 8–9 days.
Electroporation and in situ Hybridization
Chick embryos with eight or less somites were obtained as described above. Control plasmid pCMV–GFP was injected alone or together with pCMV–mouseSox2 or pCMV–mouseSox9 into the cranial neural tube with finely pulled injection needles. Then 0.5 mm gold electrodes (0.5 cm separation) were placed gently on the vitelline membrane on either side of the cranial neural tube and the plasmids were electroporated into the neuroepithelium using the following conditions: 5 pulses of a 25-V, 50 ms wave with a 1-s gap between pulses. After electroporation, host chick embryo eggs were resealed with clear tape and returned to a 37°C incubator for up to 24 h. Electroporated chick embryos were then processed for in situ hybridization as previously described (Wilkinson and Nieto, 1993; Wilkinson, 1995) with mouse Sox2 and chick Sox10 cRNA probes.
Results
NSC Self-Renewal Requires Sox2 Expression
To analyze the properties of Sox2βgeo/+ NSC, we cultured neuroepithelial cells from the cerebral cortex of individual E14.5 heterozygous mouse embryos for 3 weeks as primary neurospheres (Zappone et al., 2000) in two distinct populations, either without (Sox2βgeo/+) or with G418 selection (Sox2βgeo/+-selected; Figures 1A–E) without sub-cloning (Figures 1F–L ). Wild type (wt) cells were also used as controls for any in vitro effects related to Sox2 heterozygosity (Figure 1A). No significant differences were observed between wt and Sox2βgeo/+ neurospheres. Sox2βgeo/+ neurospheres had mosaic and variable patterns of lacZ activity reflecting Sox2-expressing cells, ranging from dispersed single cells (Figure 1B), to patches (Figure 1C), to a majority of Sox2+ cells (Figure 1D), a heterogeneity similar to adult NSC cultures (Brazel et al., 2005; Machon et al., 2005). In contrast, in Sox2βgeo/+-selected neurospheres, a homogeneous population of Sox2+ cells was obtained (Figure 1E, Sox2βgeo/+-selected). These neurospheres were smaller and contained 40% of the number of cells present in wt and Sox2βgeo/+ neurospheres. Since the original numbers of neurosphere-forming cells were similar for wt, Sox2βgeo/+, and Sox2βgeo/+-selected cultures (Figure 1F), the cells that failed to express Sox2 were eliminated by selection.
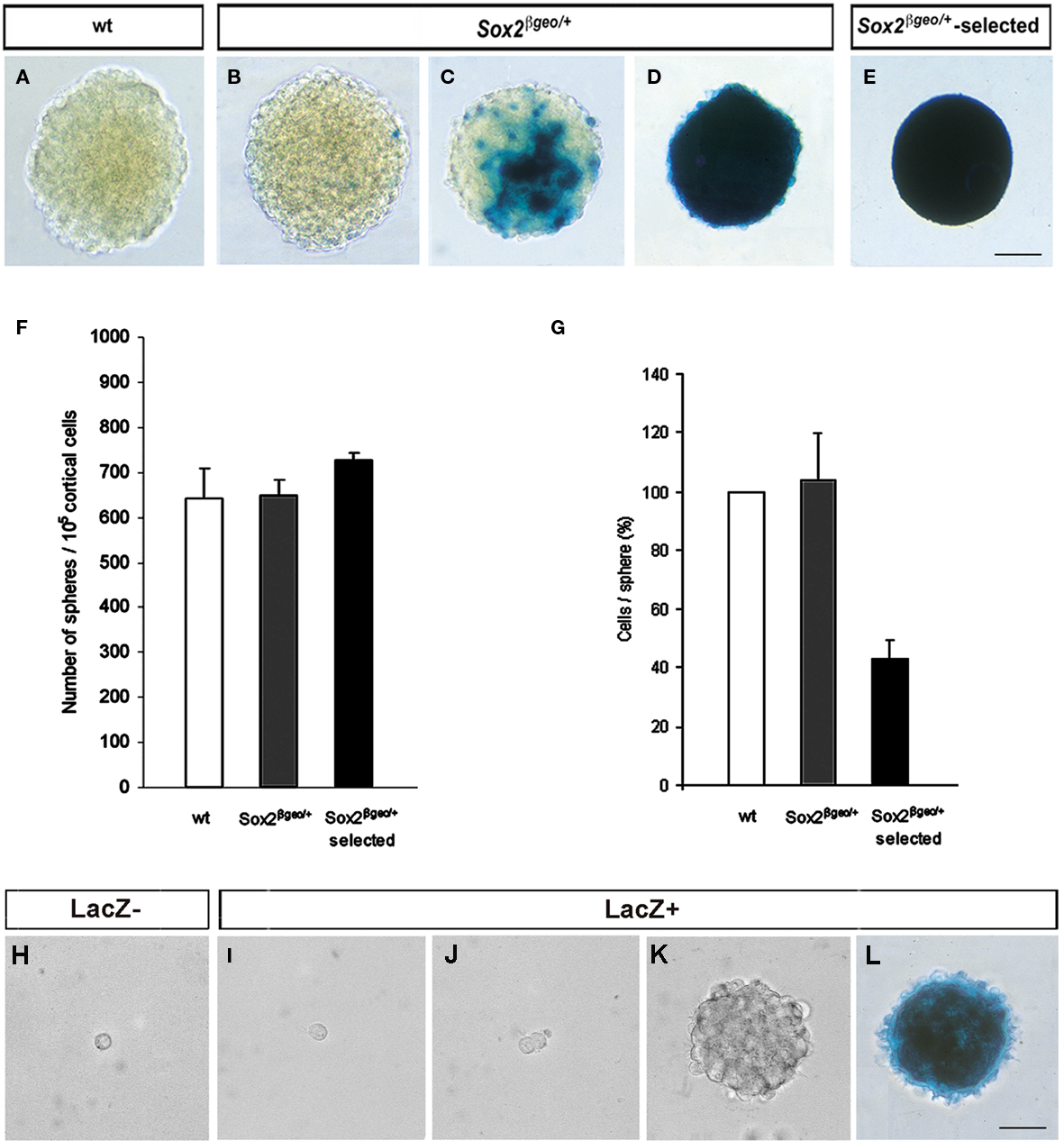
Figure 1. Cortical neurospheres contain stochastic numbers of Sox2- expressing self-renewing cells. β-galactosidase activity in wt(A), Sox2βgeo/+ (B–D), and Sox2βgeo/+-selected (E) neurospheres cultured for 3 weeks. Cortical neuroepithelial cells from E14.5 Sox2βgeo/+ embryos were grown either without [Sox2βgeo/+, (B–D)] or with G418 selection [Sox2βgeo/+-selected, (E)]. Wt littermates were used as a control [(A); scale bar = 50 μm]. Numbers of neurosphere-forming cells were similar for wt, Sox2βgeo/+, and Sox2βgeo/+-selected cells (F), but the size of Sox2βgeo/+-selected cell pool was significantly smaller (G). The data are presented as the mean ± SD (H, I). Sox2βgeo/+ neurospheres were dissociated and fractionated into Sox2βgeo/+-expressing [(I), LacZ+] and non-expressing cells [(H), LacZ−] using FACS. Each cell population was cultured at clonal density (104 cells/ml) and allowed to proliferate (J) without selective pressure. Only Sox2βgeo/+-expressing cells generated neurospheres (K) expressing variable levels of β-galactosidase activity [(L); scale bar = 50 μm].
To determine which cells were capable of self-renewal, we tested the ability of Sox2− and Sox2+ cells to give rise to secondary neurospheres (Figures 1H–L). FACS sorting for β-galactosidase expression separated the two cell populations present in dissociated unselected Sox2βgeo/+ neurospheres. When each fraction was cultured at clonal density in serial dilutions, no neurospheres were produced from the Sox2− cells, which did not survive beyond 12–24 h in culture (Figure 1H). Only Sox2+ cells divided (Figures 1I,J) and gave rise to secondary neurospheres (Figure 1K). On average, about 1% of the cells exhibited the ability to generate secondary neurospheres. As observed for the primary neurospheres (Figures 1B–D), the majority of cells within the secondary neurospheres expressed Sox2 (Figure 1L). Thus, self-renewing NSCs are restricted to Sox2-expressing cells.
NSCs Require Sox2 Expression to Maintain Their Pax6+ RG Identity in Neurosphere Cultures.
Radial glia (RG) comprise the predominant form of NP cells in the E14.5 cortex (60–70%; Gotz et al., 2002; Gotz, 2003; Malatesta et al., 2003; Gotz and Barde, 2005). These cells exhibit self-renewal properties and multipotency (Campbell and Gotz, 2002; Gotz et al., 2002; Gotz, 2003; Gotz and Barde, 2005) and express the nestin-linked epitope RC2 (Malatesta et al., 2003; Mori et al., 2005; Figure 2A). RC2+ cells co-express Sox2 in the proliferating zones of the cortex and in cell spreads (Figure 2A and inset photo). Mitogens can change the character of cells in culture and as a result neurosphere assays may not reflect the endogenous progenitor state and fate of the cortical environment in vivo ((Brazel et al., 2005; Jensen and Parmar, 2006; Conti and Cattaneo, 2010) We, therefore, examined the ability of cortical Sox2+ cells to maintain their in vivo identity in neurosphere cultures.
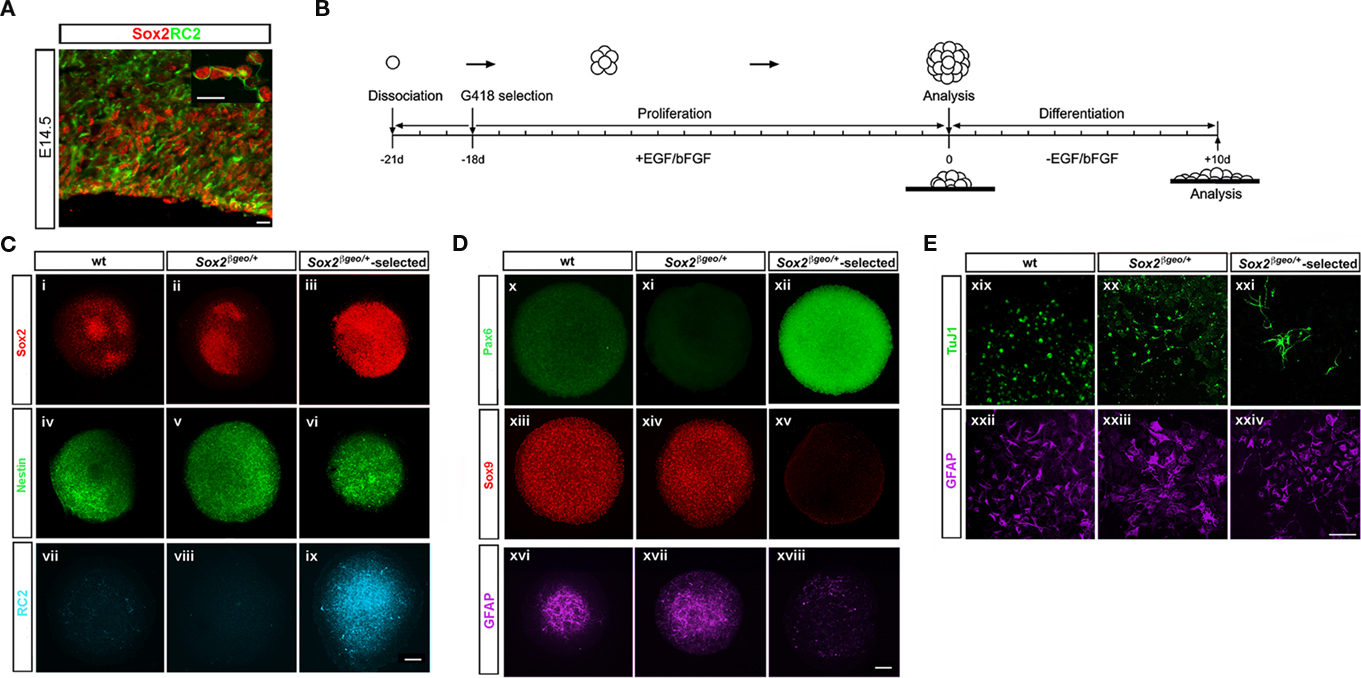
Figure 2. Sox2βgeo/+-selected NSCs possess a Pax6+ RG character. (A) Sox2 (red) marks RC2+ RG cells (green) and progenitors present in the ventricular and subventricular zone of E14.5 cortex. The inset shows co-expression of Sox2-postive/RC2+ cells in cortical cell spreads (scale bar = 25 μm). (B) Protocol for preparation and analysis of primary neurospheres. The presence of NP cells in wt, Sox2βgeo/+ neurospheres and Sox2βgeo/+-selected neurospheres was revealed by (C) Sox2 (n = 40) (i–iii), nestin (n = 40) (iv–vi), and (D) Pax6 (n = 30) (x–xii), Sox9 (n = 40) (xiii–xv) immunostaining, respectively, while the presence of glia and RG cells was manifested by GFAP (n = 50) (xvi–xviii) and RC2 (n = 40) (vii–ix) immunostaining. (E) Single wt, Sox2βgeo/+, and Sox2βgeo/+-selected neurospheres were attached to matrigel supports and allowed to differentiate. Differentiation in the periphery of each neurosphere into neurons was revealed by TuJ1 (n = 7) (xix–xxi) and to astrocytes by GFAP (n = 7) (xxii–xxiv) (scale bar = 75 μm).
Primary neurospheres from Sox2βgeo/+ embryos were cultured with or without selection (Figures 2B–E) and characterized for neural markers (Figures 2C,Di–xv). To exclude the possibility that some of the cellular phenotypes could arise from Sox2 heterozygosity, neurospheres produced from wt littermates were also analyzed. Wt and Sox2βgeo/+ neurospheres were heterogeneous with respect to Sox2+ and nestin+ cells (Figures 2Ci,ii,iv,v). In contrast, Sox2βgeo/+-selected neurospheres comprised of Sox2+ nestin+ cells (Figures 2Ciii,vi) and showed suppressed astrogenesis as evidenced by the reduction in numbers of GFAP+ cells and in levels of GFAP staining (Figure 2Dxviii) when compared to control neurosphere populations (Figures 2Dxvi,xvii). Wt and Sox2βgeo/+ neurospheres contained high numbers of Sox9+ cells (Figures 2Dxiii,xiv) and as Sox9 is implicated in the change of competence of neuroepithelial cells to generate glial lineages (Wegner and Stolt, 2005), the high number of astrocytes could be due to the presence of Sox9+ cells. Sox9+ levels were severely reduced in Sox2βgeo/+-selected neurosphere cells (Figure 2Dxv). Given the clonal origin of the neurospheres (Zappone et al., 2000), and the fact that these arise from the Sox2+ fraction (Figures 1K,L), it is most likely that the Sox9+ cells are descendents of the Sox2+ cells (Gotz and Barde, 2005). Wt and Sox2βgeo/+ neurospheres contained few RC2+ cells (Figures 2Cvii,viii). In contrast, we found that the Sox2βgeo/+-selected neurospheres were comprised of RC2+ cells (Figure 2Cix). Moreover, these RC2+ cells maintain their cortical identity by expressing high levels of Pax6 protein (Figure 3Dxii), when compared to wt and Sox2βgeo/+ neurospheres (Figures 2Dx,xi). Collectively, these data demonstrated that cortical NSCs depend on homogeneous Sox2 expression to maintain their Pax6+ RG identity ex vivo.
Radial glia cells differentiate into neurons and glial cells later in development (Gotz et al., 1998; Malatesta et al., 2000; Campbell and Gotz, 2002; Gotz et al., 2002; Gotz, 2003; Kriegstein and Gotz, 2003; Malatesta et al., 2003; Gotz and Barde, 2005). To assess the differentiation potential of Sox2βgeo/+-selected neurospheres, we allowed matrigel-attached single neurospheres to grow in the absence of selection and growth factors for 10 days (Figure 2E). GFAP+ astrocytes (Figures 2Exix-xxi) and TuJ1+ neurons (Figures 2Exxii–xxiv) were present in all neurosphere cultures. However, in wt and Sox2βgeo/+ neurospheres TuJ1+ neurons had very short axons (Figures 2Exix,xx), while in Sox2βgeo/+-selected neurospheres TuJ1+ cells had extended axons as typical cortical neurons were present. Our data implies that cells within Sox2βgeo/+ and Sox2βgeo/+-selected neurospheres have indeed distinct developmental and differentiation potential.
Sox2 Restricts the Generation of Cranial NCCs from Cortical NSCs in vivo
Embryonic stem cell-derived Pax6+ RG cells can not readily revert to a more primitive type of progenitor and as a result appear to be developmentally restricted (Gotz and Barde, 2005). To assess whether primary Sox2βgeo/+-selected Pax6+ RG cells behave in a similar manner, we performed heterotopic and heterochronic transplantations into the hindbrains of in vitro cultured mouse and in ovo cultured chick embryos (Figures 3A,B). Transplantations were performed at E8.5 (five somite stage) in mouse embryos and at E1.5 (eight somites) in chick embryos to ensure that the grafted neurospheres were incorporated into the neuroectoderm prior to the earliest waves of NCC formation and migration (Trainor, 2000, 2005; Trainor and Krumlauf, 2000; Basch et al., 2006). Wt, Sox2βgeo/+, and Sox2βgeo/+-selected neurospheres transplanted into either r2 or r4 of the hindbrain of both chick (Figures 3C–H) and mouse (Figures 3I–Q) embryos were all incorporated into the neural plate as a cohort of cells with little mixing or intermingling with their immediate neighbors. Remarkably, both wt and Sox2βgeo/+ neurospheres exhibited consistent abilities to generate migrating NCCs as evidenced by DiI lineage tracing (Figures 3C–E,I–N). Neurospheres transplanted into r2 in mouse and chick embryos generated substantial numbers of NCCs that colonized the proximo-distal extent of the first branchial arch (Figures 3D,J,M). Similarly, neurospheres transplanted into r4 in mouse and chick embryos generated substantial numbers of NCCs that colonized the proximo-distal extent of the second branchial arch (Figure 3D). The wt and Sox2βgeo/+ neurosphere cells respond appropriately to the NCC-inducing signals and migrate ventro-laterally until the first and second pharyngeal arches (Figures 3D,J,M).
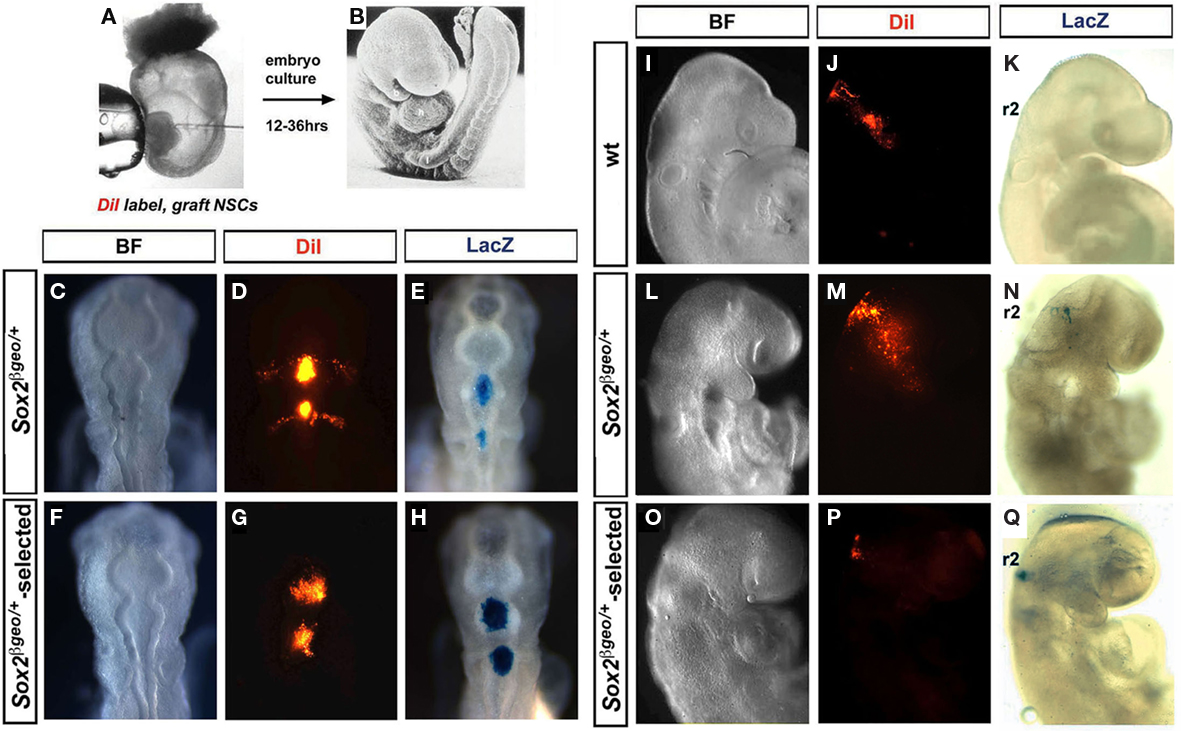
Figure 3. Sox2 restricts NSC commitment to a cranial NCC fate. Neurospheres were transplanted into the hindbrains of mouse and chick embryos (A,B) which were then cultured for up to 36 h. Transplantation of Sox2βgeo/+ (C–E) and Sox2βgeo/+-selected (F–H) neurospheres into r2 and r4 of E1.5 chick embryos. Transplantation of wt (I–K), Sox2βgeo/+ (L–N), and Sox2βgeo/+-selected (O–Q) neurospheres into r2 and r4 of E8.5 mouse embryos. Incorporation into the neural tube, the potential generation of NCCs and the identity of the transplanted cells were determined by DiI labeling (D,G,J,M,P) and β-galactosidase reporter staining (E,H,K,N,Q). Compared to wt (J–K) and Sox2βgeo/+ (C–E, L–N) neurospheres, which readily generated NCCs, Sox2βgeo/+-selected neurospheres (F–H, O–Q) exhibited a decreased ability to generate migrating NCCs.
The migration pathways adopted by DiI labeled cells derived from the transplanted neurospheres indicated that these cells might be NCCs. To rule out the possibility that the DiI+ cells were simply moving passively and being carried along by endogenous NCCs, we used Sox10, a well-known marker of migrating NCCs, to assess the NCC character of the neurosphere-derived cells in the cranial mesenchyme (Figures A1A–C in Appendix). We observed three distinct populations of cells: Sox10+ endogenous migrating neural cells (green) lateral to the neural tube and underlying the surface ectoderm, Sox10+DiI+ cells (orange) and Sox10−DiI+ (red) derived from DiI labeled neurospheres transplanted into the hindbrain (Figure A1C in Appendix). The identification of both Sox10+DiI+ (neural derivatives) and Sox10−DiI+ (mesenchymal derivatives) reflects the endogenous patterning of NCCs during normal embryonic development. Irrespective of whether neurospheres were transplanted into r2 or r4 of the hindbrains of mouse and chick embryos, we identified DiI+Tuj1+ labeled cells located proximally within the branchial arches and in close proximity to the neural tube which contributed to the formation of the trigeminal (Figures A1D–F in Appendix) and facial ganglia (Figures A1G–I in Appendix) in both species. In contrast, DiI+Tuj1− NCCs extensively populated the distal regions of the first and second branchial arches (Figures A1D–I in Appendix). This suggested that migrating cells derived from transplanted neurospheres indeed possess NCC properties. Given the appropriate embryonic niche and exposure to signals therein, E14.5 cortical wt and Sox2βgeo/+ NSCs could generate cranial NCCs and their typical neural derivatives.
However, when Sox2βgeo/+-selected neurospheres were similarly transplanted, we observed the complete absence of any NCC generation (Figures 3F–H,O–Q). This suggested that Sox2 expression inhibits the generation of NSC-derived cranial NCCs. To test this notion, we overexpressed mouse Sox2 in the neuroepithelium of E1.5 chick embryos (Figure A2 in Appendix). Whereas control GFP plasmid was continually expressed in migrating NCCs (Figures A2A,B in Appendix), neuroepithelial cells overexpressing Sox2 (Figures A2C–G in Appendix), failed to delaminate and migrate, remaining within the neural tube. NCC-specific Sox10 staining (Figures A2H,I,J in Appendix) revealed significantly fewer migrating NCCs on the Sox2 electroporated side and cranial ganglia reduced in size when compared to the control side (Figures A2I,J in Appendix). We concluded that Sox2 inhibited the generation of NCCs by restricting NSC differentiation. To test if transition from a Sox2+; Sox9− state to a Sox2−; Sox9+ state was required for NSC differentiation into NCCs, we overexpressed mouse Sox9 in E1.5 chick cranial neural tubes and observed enhanced NCC generation and consequently enlarged cranial ganglia (Figures A2K–O in Appendix). Therefore, as NSCs differentiate into NCCs, there is a clear switch in Sox expression state with Sox2 inactivated in the NCC progenitors, whereas Sox9 and then Sox10 activated in newly migrating NCCs (Melton et al., 2004).
Discussion
Cortical neurospheres are heterogeneous free-floating aggregates consisting of mixed populations of NSC, NP, and differentiated cells that eventually lose their regional identity in culture. Here, we describe, for the first time, a unique way of generating homogenous neurospheres with spatio-temporal identity that resembles the in vivo profile of proliferating cells in the embryonic cortex. Our results confirm and significantly extend previous findings by showing that Sox2 is not only important for NSC self-renewal (Zappone et al., 2000; Ferri et al., 2004; Favaro et al., 2009; Pevny and Nicolis, 2010), but also for maintaining the cortical Pax6+ identity and properties of RG cells ex vivo. Our results also show that maintenance of Sox2 expression prevents the NSC progression into committed NPs and differentiated cells. This is particularly evident in the Sox2βgeo/+-selected neurospheres, where the absence of Sox2−Sox9+ cells prevents progression to lineage-committed NPs and when these selected neurospheres are transplanted they cannot respond readily to local signals to differentiate unless they downregulate Sox2. This may reflect the normal progression of NSCs in the embryo (Pevny and Placzek, 2005) and highlights the balance required between a Sox2-mediated intrinsic program versus one dictated by the surrounding extrinsic signals considered to constitute the stem cell niche environment. The differences in properties between homogenous and heterogeneous neurospheres implies that in heterogeneous neurospheres, which consist of a mixed population of Sox2+/Sox9− NSC and Sox2−/Sox9+ NPs that are capable of generating NCCs upon back-transplantation into avian and mouse embryonic hindbrains, that it is the progenitor cells that impact significantly on the patterning of NSCs. Hence, not only is Sox2 important for the cellular memory of cortical NSCs, but also as a cell intrinsic regulator of NSC plasticity.
Cranial NCCs are a transient migratory population that exhibit a significant degree of plasticity and differentiation fates, particularly in their numerous cell and tissues contributions in the vertebrate head (Trainor and Krumlauf, 2000). NCCs are induced to form transiently at the junction between the neuroepithelium and adjacent ectoderm by the specific interplay of distinct signals from these tissues (Selleck and Bronner-Fraser, 1995) between E8.5 and E9.5 of embryonic development in mouse and between E1.5 and E2.5 in chick. These cells migrate in distinct segregated streams from the neural tube into the adjacent pharyngeal arches (Tam, 1998). Given the transient nature of NCC induction and migration from primitive neuroepithelium, which is prior to the major period or neuroepithelial maturation, it was considered highly unlikely that cranial neural crest precursor cells would persist in or could be generated from the cortex of E14.5 embryos. Our data however suggests this is indeed the case and interestingly, neural crest precursor cells have recently been shown to persist even throughout adult life in mouse whisker hair follicles (Sieber-Blum et al., 2004).
A key issue in stem cell biology is how distinct derivatives are generated from stem cell populations by a balance between cell intrinsic and cell extrinsic cues. Similar issues have been the focus of the NCC field However, the transition of a Sox2+; Sox9− neural stem cell into a Sox2−; Sox9+ differentiating cell appears to be generally conserved throughout embryonic neurogenesis. Not only is this mechanism important during cortical neurogenesis at E14.5 as we have shown, but a similar transition appears to occur during the transformation of neural stem cells into neural crest cells at E8.5 (Trainor and Krumlauf, 2001; Cheung and Briscoe, 2003). In support of this idea, it has been suggested that down-regulation of Sox2 in concert with Sox9 (Cheung and Briscoe, 2003; Cheung et al., 2005) up-regulation is required as part of a complex mechanism to generate neural crest cells from neural stem cells in avian embryos (Wakamatsu et al., 2004). Furthermore, Sox9 was shown to antagonize Sox2, and more importantly Sox9 was also demonstrated to be required for trunk NCC formation (Wakamatsu et al., 2004; Wegner and Stolt, 2005). Interestingly, this reflects the properties of Sox2βgeo/+ neurospheres and implies that neural stem cells within the cortex may retain and endogenous capacity to generate neural crest cells if provided with the appropriate environment.
The generation of NCCs from wild type and Sox2βgeo/+ neurospheres was surprising and significant since the transplanted cells were derived from the cortex of E14.5 embryos, which is nearly a week of gestation beyond the transient window of endogenous NCC formation and migration. This indicates the importance of Sox2–Sox9 signaling mechanisms in the regulation of neural stem cell maintenance and differentiation (Scott et al., 2010), but it also highlights their reiterative use throughout multiple stages of embryonic neurogenesis. Moreover, our results provocatively imply that the developmental segregation of the CNS and neural crest may be reversible even over extended periods of time. In support of this idea, migrating chick NCCs in an E2.5 embryo have been transplanted back into the ventral neural tube of younger E1.5 chick embryos. In doing so the NCCs were able to generate ventral motor neuron cell types, which are not typical NCC derivatives (Ruffins et al., 1998) lending further weight to the inherent plasticity of NCCs and the reversibility between NCCs and neuroepithelium. Lastly the differences in properties between homogenous and heterogenous neurospheres imply that in heterogeneous neurospheres, the progenitor cells impact significantly on the patterning of NSCs.
Irrespective of whether neurospheres are grown in the presence or absence of selective pressure for Sox2 expression, both populations contain Sox2+ NSCs. The failure of homogeneous Sox2+ neurospheres to generate NCCs implies that Sox2+ NSCs are difficult to reprogram when cultured in the absence of their niche provided by their associated progenitors or differentiated cells. This also implies that the Sox2+ NSC-derived Sox2− NP cells could be the ones that predominantly give rise to NCCs in response to environmental signals provided by the local environment in the graft site of the embryo. Overall, our results highlight the flexibility of NSCs and the balance required between a Sox2-mediated intrinsic program to preserve the cellular memory of cortical NSCs and another one to regulate NSC plasticity, dictated by their niche.
Conflict of Interest Statement
The authors declare that the research was conducted in the absence of any commercial or financial relationships that could be construed as a potential conflict of interest.
Acknowledgments
We thank S. Pagakis and K. Sullivan for advice on confocal microscopy; C. Atkins for FACS analysis; and S. Guioli for the Sox9 antibody. Eumorphia Remboutsika was supported by a TMR Network-funded Fellowship (EU), by the Medical Research Council (MRC; UK) and currently by the General Secretariat for Research and Technology (GSRT; ENTER-01EP67, EPAN-B26, 05NON-EU82; Greece) and the Research Promotion Foundation (Grant 7396; Cyprus). Paul A. Trainor is supported by the Stowers Institute for Medical Research, the National Institute of Dental and Craniofacial Research (Grant R01 DE 016082), and a March of Dimes Basil O’Connor scholar award (USA). Robin Lovell-Badge and Cynthia L. Andoniadou are supported by the Medical Research Council (UK; MRC file reference U117512772). Cynthia L. Andoniadou was also supported by the Beddington Fund. Maximilianos Elkouris was supported by grant 05NON-EU82 (GSRT-Greece) and an “Alexander Fleming” Fellowship (Greece). Maria Poulou was supported by PENED 03ED394 (GSRT-Greece). Angelo Iulianella was supported by a Canadian Institute of Health Research Fellowship. Thimios A. Mitsiadis was financed by funds from the University of Zurich (UZH).
References
Avilion, A. A., Nicolis, S. K., Pevny, L. H., Perez, L., Vivian, N., and Lovell-Badge, R. (2003). Multipotent cell lineages in early mouse development depend on SOX2 function. Genes Dev. 17, 126–140.
Basch, M. L., Bronner-Fraser, M., and Garcia-Castro, M. I. (2006). Specification of the neural crest occurs during gastrulation and requires Pax7. Nature 441, 218–222.
Brazel, C. Y., Limke, T. L., Osborne, J. K., Miura, T., Cai, J., Pevny, L., and Rao, M. S. (2005). Sox2 expression defines a heterogeneous population of neurosphere-forming cells in the adult murine brain. Aging Cell 4, 197–207.
Bylund, M., Andersson, E., Novitch, B. G., and Muhr, J. (2003). Vertebrate neurogenesis is counteracted by Sox1-3 activity. Nat. Neurosci. 6, 1162–1168.
Campbell, K., and Gotz, M. (2002). Radial glia: multi-purpose cells for vertebrate brain development. Trends Neurosci. 25, 235–238.
Cheung, M., and Briscoe, J. (2003). Neural crest development is regulated by the transcription factor Sox9. Development 130, 5681–5693.
Cheung, M., Chaboissier, M. C., Mynett, A., Hirst, E., Schedl, A., and Briscoe, J. (2005). The transcriptional control of trunk neural crest induction, survival, and delamination. Dev. Cell 8, 179–192.
Conti, L., and Cattaneo, E. (2010). Neural stem cell systems: physiological players or in vitro entities? Nat. Rev. Neurosci. 11, 176–187.
Ekonomou, A., Kazanis, I., Malas, S., Wood, H., Alifragis, P., Denaxa, M., Karagogeos, D., Constanti, A., Lovell-Badge, R., and Episkopou, V. (2005). Neuronal migration and ventral subtype identity in the telencephalon depend on SOX1. PLoS Biol. 3, e186. doi: 10.1371/journal.pbio.0030186
Elkouris, M., Balaskas, N., Poulou, M., Politis, P.K., Panayiotou, E., Malas, S., Thomaidou, D., and Remboutsika, E. (2011). Sox1 maintains the undifferentiated state of cortical neural progenitor cells via the suppression of Prox1-mediated cell cycle exit and neurogenesis. Stem Cells 29, 89–98.
Ellis, P., Fagan, B. M., Magness, S. T., Hutton, S., Taranova, O., Hayashi, S., Mcmahon, A., Rao, M., and Pevny, L. (2004). SOX2, a persistent marker for multipotential neural stem cells derived from embryonic stem cells, the embryo or the adult. Dev. Neurosci. 26, 148–165.
Favaro, R., Valotta, M., Ferri, A. L., Latorre, E., Mariani, J., Giachino, C., Lancini, C., Tosetti, V., Ottolenghi, S., Taylor, V., and Nicolis, S. K. (2009). Hippocampal development and neural stem cell maintenance require Sox2-dependent regulation of Shh. Nat. Neurosci. 12, 1248–1256.
Ferri, A. L., Cavallaro, M., Braida, D., Di Cristofano, A., Canta, A., Vezzani, A., Ottolenghi, S., Pandolfi, P. P., Sala, M., Debiasi, S., and Nicolis, S. K. (2004). Sox2 deficiency causes neurodegeneration and impaired neurogenesis in the adult mouse brain. Development 131, 3805–3819.
Gotz, M. (2003). Glial cells generate neurons–master control within CNS regions: developmental perspectives on neural stem cells. Neuroscientist 9, 379–397.
Gotz, M., and Barde, Y. A. (2005). Radial glial cells defined and major intermediates between embryonic stem cells and CNS neurons. Neuron 46, 369–372.
Gotz, M., Hartfuss, E., and Malatesta, P. (2002). Radial glial cells as neuronal precursors: a new perspective on the correlation of morphology and lineage restriction in the developing cerebral cortex of mice. Brain Res. Bull. 57, 777–788.
Gotz, M., Stoykova, A., and Gruss, P. (1998). Pax6 controls radial glia differentiation in the cerebral cortex. Neuron 21, 1031–1044.
Graham, V., Khudyakov, J., Ellis, P., and Pevny, L. (2003). SOX2 functions to maintain neural progenitor identity. Neuron 39, 749–765.
Jensen, J. B., and Parmar, M. (2006). Strengths and limitations of the neurosphere culture system. Mol. Neurobiol. 34, 153–161.
Kamachi, Y., Uchikawa, M., and Kondoh, H. (2000). Pairing SOX off: with partners in the regulation of embryonic development. Trends Genet. 16, 182–187.
Kan, L., Israsena, N., Zhang, Z., Hu, M., Zhao, L. R., Jalali, A., Sahni, V., and Kessler, J. A. (2004). Sox1 acts through multiple independent pathways to promote neurogenesis. Dev. Biol. 269, 580–594.
Kriegstein, A. R., and Gotz, M. (2003). Radial glia diversity: a matter of cell fate. Glia 43, 37–43.
Li, M., Pevny, L., Lovell-Badge, R., and Smith, A. (1998). Generation of purified neural precursors from embryonic stem cells by lineage selection. Curr. Biol. 8, 971–974.
Machon, O., Backman, M., Krauss, S., and Kozmik, Z. (2005). The cellular fate of cortical progenitors is not maintained in neurosphere cultures. Mol. Cell. Neurosci. 30, 388–397.
Malas, S., Postlethwaite, M., Ekonomou, A., Whalley, B., Nishiguchi, S., Wood, H., Meldrum, B., Constanti, A., and Episkopou, V. (2003). Sox1-deficient mice suffer from epilepsy associated with abnormal ventral forebrain development and olfactory cortex hyperexcitability. Neuroscience 119, 421–432.
Malatesta, P., Hack, M. A., Hartfuss, E., Kettenmann, H., Klinkert, W., Kirchhoff, F., and Gotz, M. (2003). Neuronal or glial progeny: regional differences in radial glia fate. Neuron 37, 751–764.
Malatesta, P., Hartfuss, E., and Gotz, M. (2000). Isolation of radial glial cells by fluorescent-activated cell sorting reveals a neuronal lineage. Development 127, 5253–5263.
Melton, K. R., Iulianella, A., and Trainor, P. A. (2004). Gene expression and regulation of hindbrain and spinal cord development. Front. Biosci. 9, 117–138.
Mori, T., Buffo, A., and Gotz, M. (2005). The novel roles of glial cells revisited: the contribution of radial glia and astrocytes to neurogenesis. Curr. Top. Dev. Biol. 69, 67–99.
Nishiguchi, S., Wood, H., Kondoh, H., Lovell-Badge, R., and Episkopou, V. (1998). Sox1 directly regulates the gamma-crystallin genes and is essential for lens development in mice. Genes Dev. 12, 776–781.
Pevny, L., and Placzek, M. (2005). SOX genes and neural progenitor identity. Curr. Opin. Neurobiol. 15, 7–13.
Pevny, L. H., and Nicolis, S. K. (2010). Sox2 roles in neural stem cells. Int. J. Biochem. Cell Biol. 42, 421–424.
Rizzoti, K., Brunelli, S., Carmignac, D., Thomas, P. Q., Robinson, I. C., and Lovell-Badge, R. (2004). SOX3 is required during the formation of the hypothalamo-pituitary axis. Nat. Genet. 36, 247–255.
Ruffins, S., Artinger, K. B., and Bronner-Fraser, M. (1998). Early migrating neural crest cells can form ventral neural tube derivatives when challenged by transplantation. Dev. Biol. 203, 295–304.
Sandberg, M., Kallstrom, M., and Muhr, J. (2005). Sox21 promotes the progression of vertebrate neurogenesis. Nat. Neurosci. 8, 995–1001.
Scaffidi, P., and Bianchi, M. E. (2001). Spatially precise DNA bending is an essential activity of the sox2 transcription factor. J. Biol. Chem. 276, 47296–47302.
Scott, C. E., Wynn, S. L., Sesay, A., Cruz, C., Cheung, M., Gomez Gaviro, M. V., Booth, S., Gao, B., Cheah, K. S., Lovell-Badge, R., and Briscoe, J. (2010). SOX9 induces and maintains neural stem cells. Nat. Neurosci. 13, 1181–1189.
Selleck, M. A., and Bronner-Fraser, M. (1995). Origins of the avian neural crest: the role of neural plate-epidermal interactions. Development 121, 525–538.
Sieber-Blum, M., Grim, M., Hu, Y. F., and Szeder, V. (2004). Pluripotent neural crest stem cells in the adult hair follicle. Dev. Dyn. 231, 258–269.
Sturm, K., and Tam, P. P. (1993). Isolation and culture of whole postimplantation embryos and germ layer derivatives. Meth. Enzymol. 225, 164–190.
Tam, P. P. (1998). Postimplantation mouse development: whole embryo culture and micro-manipulation. Int. J. Dev. Biol. 42, 895–902.
Trainor, P., and Krumlauf, R. (2000). Plasticity in mouse neural crest cells reveals a new patterning role for cranial mesoderm. Nat. Cell Biol. 2, 96–102.
Trainor, P. A. (2000). Craniofacial morphogenesis: a meeting in memory of Peter Thorogood. Bioessays 22, 202–204.
Trainor, P. A. (2005). Specification of neural crest cell formation and migration in mouse embryos. Semin. Cell Dev. Biol. 16, 683–693.
Trainor, P. A., and Krumlauf, R. (2001). Hox genes, neural crest cells and branchial arch patterning. Curr. Opin. Cell Biol. 13, 698–705.
Wakamatsu, Y., Endo, Y., Osumi, N., and Weston, J. A. (2004). Multiple roles of Sox2, an HMG-box transcription factor in avian neural crest development. Dev. Dyn. 229, 74–86.
Wegner, M., and Stolt, C. C. (2005). From stem cells to neurons and glia: a Soxist’s view of neural development. Trends Neurosci. 28, 583–588.
Wilkinson, D. G. (1995). RNA detection using non-radioactive in situ hybridization. Curr. Opin. Biotechnol. 6, 20–23.
Wilkinson, D. G., and Nieto, M. A. (1993). Detection of messenger RNA by in situ hybridization to tissue sections and whole mounts. Methods Enzymol. 225, 361–373.
Wilson, M., and Koopman, P. (2002). Matching SOX: partner proteins and co-factors of the SOX family of transcriptional regulators. Curr. Opin. Genet. Dev. 12, 441–446.
Wood, H. B., and Episkopou, V. (1999). Comparative expression of the mouse Sox1, Sox2 and Sox3 genes from pre-gastrulation to early somite stages. Mech. Dev. 86, 197–201.
Zappone, M. V., Galli, R., Catena, R., Meani, N., De Biasi, S., Mattei, E., Tiveron, C., Vescovi, A. L., Lovell-Badge, R., Ottolenghi, S., and Nicolis, S. K. (2000). Sox2 regulatory sequences direct expression of a (beta)-geo transgene to telencephalic neural stem cells and precursors of the mouse embryo, revealing regionalization of gene expression in CNS stem cells. Development 127, 2367–2382.
Keywords: radial glia, self-renewal, neural stem cell, stem cell niche, Sox genes, neurogenesis, gliogenesis, neural crest
Citation: Remboutsika E, Elkouris M, Iulianella A, Andoniadou CL, Poulou M, Mitsiadis TA, Trainor PA and Lovell-Badge R (2011) Flexibility of neural stem cells. Front. Physio. 2:16. doi: 10.3389/fphys.2011.00016
Received: 09 March 2011; Paper pending published: 18 March 2011;
Accepted: 28 March 2011; Published online: 11 April 2011.
Edited by:
Anna Petryk, University of Minnesota, USAReviewed by:
Daniel Graf, University of Zurich, SwitzerlandCatherine Ellen Krull, University of Michigan, USA
Copyright: © 2011 Remboutsika, Elkouris, Iulianella, Andoniadou, Poulou, Mitsiadis, Trainor and Lovell-Badge. This is an open-access article subject to a non-exclusive license between the authors and Frontiers Media SA, which permits use, distribution and reproduction in other forums, provided the original authors and source are credited and other Frontiers conditions are complied with.
*Correspondence: Eumorphia Remboutsika, Stem Cell Biology Laboratory, Biomedical Sciences Research Center Alexander Fleming, 34 Fleming Street, Vari-Attica 16672, Greece. e-mail:cmVtYm91dHNpa2FAZmxlbWluZy5ncg==