- 1Li Ka Shing Faculty of Medicine, School of Biomedical Sciences, The University of Hong Kong, Hong Kong, China
- 2Department of Medicine and Therapeutics, The Chinese University of Hong Kong, Hong Kong, China
- 3Department of Medicine and Therapeutics, Institute of Digestive Disease, Li Ka Shing Institute of Health Sciences, The Chinese University of Hong Kong, Hong Kong, China
Disruptions in the orderly activation and recovery of electrical excitation traveling through the heart and the gastrointestinal (GI) tract can lead to arrhythmogenesis. For example, cardiac arrhythmias predispose to thromboembolic events resulting in cerebrovascular accidents and myocardial infarction, and to sudden cardiac death. By contrast, arrhythmias in the GI tract are usually not life-threatening and much less well characterized. However, they have been implicated in the pathogenesis of a number of GI motility disorders, including gastroparesis, dyspepsia, irritable bowel syndrome, mesenteric ischaemia, Hirschsprung disease, slow transit constipation, all of which are associated with significant morbidity. Both cardiac and gastrointestinal arrhythmias can broadly be divided into non-reentrant and reentrant activity. The aim of this paper is to compare and contrast the mechanisms underlying arrhythmogenesis in both systems to provide insight into the pathogenesis of GI motility disorders and potential molecular targets for future therapy.
Introduction
Abnormalities in the orderly activation and recovery of impulses traveling through the heart and the gastrointestinal (GI) tract can lead to arrhythmogenesis (Tse, 2015; Tse and Yeo, 2015; Tse et al., 2016k). Thus, atrial arrhythmias can cause thromboembolic events resulting in cerebrovascular accidents, whilst ventricular arrhythmias predispose to sudden cardiac death. By contrast, arrhythmias in the GI tract are usually not life-threatening and perhaps this is the reason that they are much less well characterized. However, recent studies have implicated GI arrhythmogenesis with a number of motility disorders, which are associated with significant morbidity. The aim of this article is to compare and contrast the electrophysiological mechanisms of arrhythmogenesis in both systems, drawing analogies to shed light on the GI aspects. This is followed by a discussion on the clinical relevance as exemplified by GI motility disorders and molecular targets for future therapy.
Ionic Contributions to Electrical Activity
Smooth muscle cells of the GI tract generate slow waves, whereas cardiomyocytes in the heart produce action potentials (APs); both types of electrical activity are dependent upon ionic conductances across the cell membranes. The morphology of these waveforms dependent on the cell type and location in the respective specialized conduction systems. Thus, slow waves by gastric cells are triangular with rapid depolarization and repolarization phases. Slow waves of the small and large intestinal smooth muscle cells have an initial depolarizing phase generated by the pacemaker cells, interstitial cells of Cajal of the myenteric plexus (ICC-MY) (Dickens et al., 1999), and a second phase mediated by ICC within the smooth muscle (ICC-IM) (Bauer et al., 1985; Dickens et al., 2001). Superimposed upon these slow waves are regenerative Ca2+ spikes, which only develops when the membrane potential is above a threshold; these spikes are intrinsic to the smooth muscle cells (Lee et al., 1999; Suzuki and Hirst, 1999; Lammers and Slack, 2001). Cardiac APs have a rapid upstroke, rapid repolarization and a plateau phase. The reader is directed to these articles here for a review of the ionic currents mediating GI slow waves and cardiac APs (Lammers et al., 2009; Tse et al., 2016c). Both systems show features of restitution, where the duration of electrical activity shortens in response to higher pacing rates. Thus, slow waves in the gastric antrum normally discharges at a frequency of 1–2 cycles per minute (cpm) (Bauer et al., 1985; Publicover and Sanders, 1986). Upon a higher rate of extrinsic stimulation, it can exhibit waves at 7 cpm (Sarna and Daniel, 1973). This can be explained by restitution mechanisms that result from shortening or abolishing the plateau phase (Publicover and Sanders, 1986). Similarly, cardiac restitution is responsible for normal shortening of APD observed in response to faster heart rates, and is thought to be an adaptive mechanism for preserving diastole at these rates.
Arrhythmogenic Mechanisms
Both cardiac and gastrointestinal arrhythmias can be classified into non-reentrant and reentrant mechanisms (Table 1).
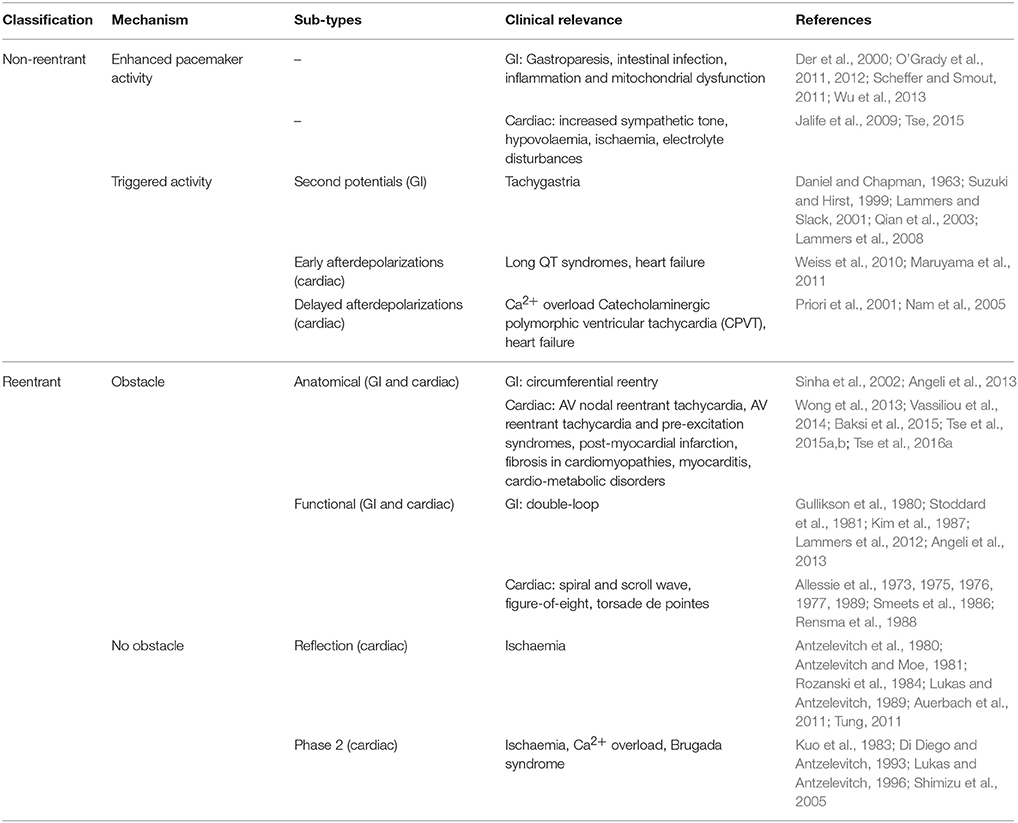
Table 1. Arrhythmogenic mechanisms in the GI and cardiovascular systems can be divided into non-reentrant and reentrant activity.
Non-Reentrant Activity
Non-reentrant activity refers to aberrant initiation due to either enhanced automaticity or triggered activity. Enhanced pacemaker activity in the heart can arise from a depolarizing shift of the maximum diastolic potential, a hyperpolarizing shift of the threshold potential or a faster rate of rise of the spontaneous depolarization (Jalife et al., 2009). In the GI tract, it has been observed in the human stomach (O'Grady et al., 2011, 2012), and the small intestine during inflammation, infection and mitochondrial disease (Der et al., 2000; Scheffer and Smout, 2011; Wu et al., 2013). By contrast, triggered activity refers to activity initiated by the preceding electrical activity (Figure 1). In the heart, it is due to early or delayed afterdepolarization phenomena (EADs and DADs, respectively), which are secondary depolarization events occurring before the subsequent AP (Cranefield, 1977; January et al., 1991), which can initiate arrhythmias (Tse, 2015). EADs are typically generated when the repolarization phase of the cardiac AP is prolonged, leading to reactivation of the L-type Ca2+ channels (ICa) (January and Riddle, 1989) or activation of the Na+-Ca2+ exchanger (INCX) secondary to spontaneous Ca2+ release from the sarcoplasmic reticulum (Szabo et al., 1994). DADs are associated with Ca2+ overload, which activates the following Ca2+-sensitive currents: the non-selective cationic current, INS, the sodium-calcium exchange current, INCX, and the calcium-activated chloride current, ICl, Ca, which together constitute the transient inward current (ITI) (Guinamard et al., 2004). These afterdepolarizations are analogous to “second potentials” that could generate the ectopic beats observed in the GI tract (Qian et al., 2003). However, the mechanism underlying their generation is different. Increased automaticity here is related to increased stretch, enhanced by acetylcholine and inhibited by adrenaline (Daniel and Chapman, 1963). Their ionic contributions are yet to be determined, but could potentially involve Ca2+ entry from the extracellular space or Ca2+ release from the endoplasmic reticulum (Suzuki and Hirst, 1999; Lammers and Slack, 2001). Premature slow waves, which presumably arise from such secondary potentials, precede and may be a prerequisite for the initiation of tachygastria (Lammers et al., 2008).
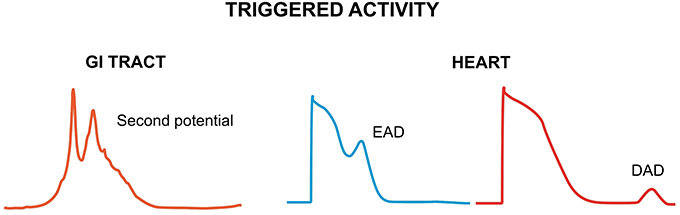
Figure 1. Triggered activity can result from second potentials in the GI tract (left) or afterdepolarizations in the heart (right). Second potentials may be due to Ca2+ entry from the extracellular space or Ca2+ release from the endoplasmic reticulum. Early afterdepolarizations (EADs) are due to reactivation of L-type Ca2+ channels or Na+-Ca2+ exchanger (NCX). Delayed afterdepolarizations (DADs) develop during Ca2+ overload, which activates Ca2+-sensitive channels: non-selective cationic channel, NCX and calcium-activated chloride channel.
Reentry
Reentry is a frequently encountered mechanism and occurs when an impulse fails to extinguish itself and re-excites a region that has recovered from refractoriness. In the heart, it can take place in the presence of an obstacle (circus-type), or in the absence of an obstacle (reflection or phase 2 reentry). Three requirements for circus-type reentry are reduced conduction velocity (CV), unidirectional conduction block and an obstacle around which the AP can circulate. This obstacle can be a permanent anatomical abnormality (anatomical reentry) (Figure 2), but can also involve a functional core of refractory tissue that arises dynamically (functional reentry) (Figure 3; Garrey, 1914).
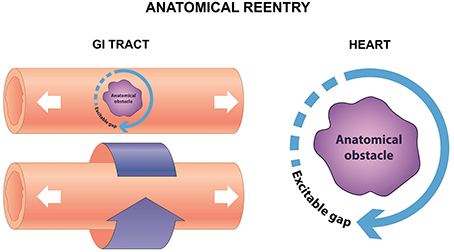
Figure 2. Anatomical reentry in the GI tract can take place in the serosal surface, or around the circumference (left). In the heart, reentry can similarly take place around an anatomical obstacle, which may be a fibrotic scar, or areas of fibrosis (right).
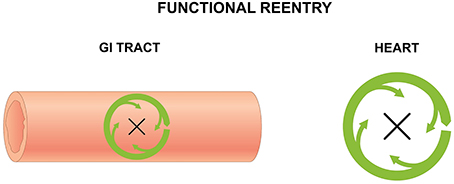
Figure 3. Functional reentry in the GI tract (left) and the heart (right) involves circular activity around a central refractory obstacle. This may arise from centripetal electrotonic forces that continuously provide subthreshold depolarization to the core, rendering it inexcitable, or from premature activation of the tissue concerned leading to absolute or relative refractoriness.
There are a number of similarities between reentry occurring in the GI tract and the heart: initiation of a premature beat precede reentry, reentry can be non-sustained or sustained. Additionally, anisotropic conduction is important in both systems in reentry (Angeli et al., 2013). However, several differences are observed (Lammers et al., 2008). Firstly, gastric tachyarrhythmia occurs at a much lower frequency of 10–15 cycles per minute, whereas ventricular tachyarrhythmia typically occurs at rates between 100 and 250 beats per minute (bpm). Secondly, unidirectional conduction block is a prerequisite of circus-type reentry (Allessie et al., 1976, 1977; Lammers et al., 1990), but this is not the case in tachygastria as shown by electrograms recorded from the canine stomach using a multi-electrode array (Lammers et al., 2008).
Anatomical Reentry
Circus-type reentry involving an anatomical obstacle was first demonstrated by the ring model using disks made from sub-umbrella tissue of a jellyfish (Mayer, 1906). Mayer made the following observations. The disks were paralyzed when they were separated from their sense organs. They do not pulsate in seawater, but did so when ring-like cuts were made from the tissue. Upon mechanical stimulation, the disks then showed “rhythmical pulsations so regular and sustained as to recall the movement of clockwork.” Later, Mines used a ring-like preparation of the tortoise heart, demonstrating that it was possible to initiate circus-type re-entry by electrical stimulation (Mines, 1913). He noted that when an excitation wave has a high CV and a long duration, the whole circuit would be excited at the same time, causing the excitation to die out. By contrast, when the wave has slow CV and a short ERP, the tissue ahead of the excitation wave would recover from refractoriness and can therefore be re-excited, resulting in circus-type re-entry. Mines predicted “a circulating excitation of this type may be responsible for some cases of paroxysmal tachycardia as observed clinically.” He was the first to formulate the three criteria for circus-type reentry mentioned above. It was later recognized that conduction of the excitation must be sufficiently slow to allow the tissue ahead in the circuit to recover from refractoriness so that it can be re-excited. It is useful to describe this excitation as a propagating wave (Weiss et al., 2005), with a wavefront that represents action potential depolarization, and a tail that represents repolarization (Weiss et al., 2000) with the assumption that APD is equal to the effective refractory period (ERP) (Tse et al., 2016i). The length of this excitation wave (λ) is given by CV × ERP (Wiener and Rosenblueth, 1946), and must be smaller than the length of the circuit in order for re-entry to be successful. Thus, reduced and increased λ is associated with greater and lesser likelihood of circus-type reentry, respectively (Smeets et al., 1986; Vaidya et al., 1999; Osadchii and Olesen, 2009; Osadchii et al., 2009, 2010; Osadchii, 2010, 2012a,b, 2014a,b, 2016; Tse et al., 2012, 2016b,c,d,e,f,g,h,j; Tse and Yan, 2016; Tse, 2016a,b,c).
Anatomical reentry is relevant in different types of tachyarrhythmias, such as AV nodal reentrant tachycardia, AV reentrant tachycardia and pre-excitation syndromes including Wolff-Parkinson-White Syndrome. It can be also the mechanism underlying atrial and ventricular tachycardia, where the AP wave circulates around a fixed fibrotic scar, such as post-myocardial infarction (Sinha et al., 2002). Moreover, micro-reentry around areas of fibrosis, which is observed in conditions such as cardiomyopathies, myocarditis and cardio-metabolic disorders of hypertension and diabetes mellitus (Wong et al., 2013; Vassiliou et al., 2014; Baksi et al., 2015; Tse et al., 2015a,b, 2016a). Anatomical reentry involving a fixed pathway has also been observed in the small intestine: re-entrant activity propagating around the circumference has been termed circumferential reentry (Angeli et al., 2013), which is analogous to the anatomical reentry (Allessie et al., 1977). In both cases, this is a fixed circuit whose length is determined by the perimeter of the anatomical obstacle, with an excitable gap between the depolarization wavefront and the repolarization tail. The revolution time is inversely proportional to the CV.
Functional Reentry
For functional reentry without an anatomical obstacle, seminal experiments in rabbit atrial preparations provided its direct evidence in support of Garrey's prediction. Allessie applied electrical stimulation at the center of the atrial preparation and found that electrical activation elicited by regular stimuli spread normally throughout the atrial tissue (Allessie et al., 1973). Contrastingly, premature stimuli elicited electrical activity that only propagated in the direction of shortened ERPs and at a reduced CV. Spatial dispersion in the refractory periods (Allessie et al., 1976) was responsible for unidirectional block of the premature AP (Allessie et al., 1975). To explain the lack of activity in this core, it was proposed that center of the circle was held above threshold by the electrotonic influences of the depolarization wavefront propagating centripetally, which rendered it inexcitable. The AP would continue to revolve around this functional core of refractory tissue. Subsequent experiments utilizing transmembrane potential recordings led to the development of the leading circle model (Allessie et al., 1977). The circuit is defined entirely by the electrophysiological properties of the tissue. The smallest circuit permitting successful re-entry, called the leading circle, is one in which the circulating wavefront can just re-excite the tissue ahead that is still in its relative refractory period. A variation of functional reentry termed spiral wave reentry was described later (Krinsky, 1966). A spiral wave is a two-dimensional wave of excitation emitted by a self-organizing source of functional reentrant activity, termed a rotor. The three-dimensional equivalent of a spiral wave is a scroll wave.
Spiral waves were described earlier in the Belousov–Zhabotinsky chemical reaction, in which cerium catalyzes the malonic acid oxidation by bromate (Belousov, 1958; Zaikin and Zhabotinsky, 1970). The ratio of cerium (IV) to cerium (III) undergoes repeated temporal oscillations, producing spiral waves with alternating colors (Müller et al., 1985; Epstein, 2006). Later, spiral waves were reproduced in theoretical models of cardiac tissue (Moe et al., 1964; Courtemanche and Winfree, 1991; Leon et al., 1994) and demonstrated in thin slices of epicardial muscle using a potentiometric dye, whose spectral properties are altered by voltage (Salzberg et al., 1973). Previous experiments have demonstrated an excitable phase singularity, although it remains non-excited and can act as a functional obstacle around which the spiral wave can travel (Ikeda et al., 1996). Spiral waves are not fixed in space but can drift (Pertsov et al., 1993). This is accompanied by a Doppler effect, in which the frequency of excitation at a given measurement site depends on its location relative to the drifting spiral wave (Davidenko et al., 1992). Therefore, the sites anterior to the wave are excited faster than those posterior to the wave. Such a mechanism may underlie torsade de pointes (Dessertenne, 1966), whereby two widely separated foci discharging at different frequencies were suggested to underlie periodic torsion of the QRS axis.
Functional reentry in the GI tract can have analogous mechanisms (Gullikson et al., 1980; Stoddard et al., 1981; Kim et al., 1987; Lammers et al., 2012; Angeli et al., 2013). In the stomach, functional reentry can take a circular route (O'Grady et al., 2011) or have a double loop morphology, consisting of two wavefronts traveling in opposite directions (Lammers et al., 2008). The latter is similar to the cardiac figure-of-eight reentry generated by two counter-rotating spiral waves separated by a small distance (El-Sherif et al., 1981). Functional reentry has also been observed in the small intestine (Lammers et al., 2012; Angeli et al., 2013), which is analogous to Allessie's leading circle model of reentrant tachycardia in the atria with the following similarities (Allessie et al., 1977). Firstly, the length of the circuit is determined by electrophysiological rather than anatomical characteristics. Secondly, the dimensions of the circuits are variable rather than fixed. Thirdly, the depolarization front and the repolarization tail are in close proximity to each other, and so there is only a partially excitable gap between the two. Fourthly, the center of the circuit contains excitable rather than inexcitable tissue, which would permit termination of the re-entrant tachycardia if an impulse shorts the circuit by crossing the circle. Finally, the time for one rotation is inversely proportional to the RP of the tissue rather than to the CV of the wave. As pointed out, these circuits can meander along the tissue and are more unstable than anatomical reentrant circuits (Lammers, 2013). This supports previous modeling studies suggesting that self-sustaining spiral waves can be generated in anisotropic smooth muscle syncytium in the intestines (Miftahof, 2005).
Autonomic Modulation
Coumel originally proposed a triads of conditions necessary for arrhythmogenesis, which are trigger, substrate and modulating factors (Coumel et al., 1967, 1978; Coumel, 1993). In both systems, arrhythmias are susceptible to autonomic modulation (Smeets et al., 1986; El-Sherif et al., 1987; Ouyang et al., 2015). In the heart, parasympathomimetic agents such as acetylcholine reduces CV, APD and ERP, thereby decreasing the excitation wavelength to promote reentry (Smeets et al., 1986; Oliveira et al., 2011). In the presence of sympathomimetic agents such as noradrenaline, the Ca2+ transient increased (Bers, 2002a,b), and forward activation of NCX, with consequent EADs and triggered activity (Patterson et al., 2006). Sympathetic activation in long QT syndromes and catecholaminergic polymorphic ventricular tachycardia can exacerbate ventricular arrhythmias (Shen and Zipes, 2014). This may be due to increased heterogeneities in repolarization and refractoriness, thereby producing a favorable substrate for reentry. Autonomic dysfunction, particularly affecting vagal nerves, is known to result in GI motility disorders in diabetes mellitus (Feldman and Schiller, 1983). Slow wave arrhythmias in the small intestine mediated by hyperglycaemia is likely the result of higher sympathetic compared to parasympathetic activity (Ouyang et al., 2015). Interestingly, diabetic rats have a higher likelihood of developing functional reentry in the small intestine compared to the control rats (Lammers et al., 2012). The underlying cause is unclear, abnormalities in the enteric nervous system or the smooth muscle itself may be affected but autonomic dysfunction can well play an important role.
Clinical Relevance
The question remains, even if arrhythmias occur in the GI tract, are they clinically significant? To answer this, the following evidence should be considered. Gastric tachy- and brady-arrhythmias have been associated with gastroparesis (Bortolotti et al., 1990), in which reduced CV of slow waves has been observed (O'Grady et al., 2012). They also appear to be predictive of dyspeptic symptoms in systemic sclerosis (McNearney et al., 2009). Gastric tachyarrhythmias can be observed following administration of opiate drugs, after anesthesia or post-operatively (Stoddard et al., 1981). Anesthetic agents can act on Ca2+ channels directly (Ahn and Karaki, 1988), thereby leading to abnormal slow wave propagation and reentrant arrhythmias. Unexplained nausea and vomiting involves recurrent arrhythmias with abnormal wave propagation and higher frequency in the distal stomach, as demonstrated by gastric serosal electrophysiological study (Abell et al., 2009). Intestinal arrhythmias occur in diabetes mellitus (Lammers et al., 2012; Ouyang et al., 2015) and mesenteric ischemia (Seidel et al., 1999; Irimia and Wikswo, 2008) and may play a role in post-operative ileus, as suggested previously (Angeli et al., 2013).
However, only limited evidence exists on the mechanisms of arrhythmias occurring in these situations, but theoretical considerations suggest reentry playing a key role. Thus, spiral waves are inducible in the myocardium or intestinal smooth muscle because of intrinsic electrical heterogeneities and anisotropic properties (Miftahof, 2005) and their formation would be made more favorable in the above pathological conditions, which increase tissue heterogeneity and anisotropy (Gizzi et al., 2010). Alternatively, inflammation could result in loss of ICC-MY activity, suggesting that pacemaker activity is impaired (Yanagida et al., 2007; Gizzi et al., 2010). A better understanding of electrophysiology is key to developing effective treatment for these motility disorders. For example, almost all anti-arrhythmic agents in the heart are modulators of ion channels, which can also be targeted in the GI tract.
Bradyarrhythmias, although not discussed in this review, are also observed in many GI pathologies. Thus, the use of opiate drugs can abolish slow waves or lead to irregular patterns of slow waves, termed amyogenesia and dysmyogenesia, respectively (Sarna and Otterson, 1990). Other causes, where loss of GI pacemaker cells is observed, include achalasia (Chen et al., 2013), gastroparesis (O'Grady et al., 2012), functional dyspepsia (Jung et al., 2012), Hirschsprung disease (Yamataka et al., 1995), and slow transit constipation (Lyford et al., 2002).
Irritable bowel syndrome, a triad of altered bowel habits, bloating and abdominal pain without an organic cause (Sinagra et al., 2016) with either a diarrhea- or constipation- predominant phenotype, is a chronic debilitating relapsing and remitting condition. Loss of ICC-MY (Eshraghian and Eshraghian, 2011), Na+ channel mutations (Saito et al., 2009) and altered microbiota profile (Tana et al., 2010; Ng et al., 2013) increasing the intestinal Cl− channel activity have been demonstrated (Chang and Talley, 2010). These changes could lead to impaired initiation of slow wave activity in the intestines. Furthermore, these abnormalities are accompanied by alterations in ICC-MY network and electrophysiological remodeling (Akbarali et al., 2010) caused by chronic inflammation (Der et al., 2000), and could conceivably serve as favorable substrates for reentrant arrhythmogenesis. Interestingly, clinical evidence does not support the notion that autonomic dysfunction plays a role in the symptoms associated with gastrointestinal motility disorders such as chronic dyspepsia or constipation (Vazeou et al., 2004).
Recently, a new syndrome characterized by Chronic Atrial and Intestinal Dysrhythmia, termed CAID syndrome, has been discovered, in which features of both sick sinus syndrome (SSS) (of alternating bradycardia-tachycardia, Chen et al., 2016b) and chronic intestinal pseudo-obstruction (CIPO) are observed (Chetaille et al., 2014). In CAID, mutation in SGOL1, a component of the cohesin complex, was the underlying cause. Both SSS and CIPO are caused by pacemaker dysfunction: SSS can be caused by loss-of-function mutations in the SCN5A gene encoding for the sodium channel, whereas CIPO is caused by loss of the ICC-MY (Feldstein et al., 2003; Struijs et al., 2008). Moreover, atrial fibrillation gut syndrome (AFGS) was used to describe reduced gastrointestinal motility, e.g., gastroparesis, following radiofrequency catheter ablation for atrial fibrillation (Lee and Lee, 2014). This may arise from vagus nerve injury from electrical injury used for ablation.
Future Treatment Options and Concluding Remarks
Improved understanding of the abnormal electrophysiology underlying GI motility disorders can lead to the development of more effective treatment options. In terms of pharmacotherapy, ion channels represent attractive targets. For example, functional constipation or constipation-predominant IBS can be managed by the chloride channel protein 2 agonist, lubiprostone (Camilleri et al., 2006; Andresen et al., 2007), whereas functional diarrhea or diarrhea -predominant IBS can be managed by its inhibitor crofelemer (Manabe et al., 2010; Yeo et al., 2013). For intervention, analogous to cardiac pacing for heart blocks, gastric electrical stimulation can be used in severe cases of gastroparesis (Abrahamsson, 2007). Similarly, colonic electrical stimulation can potentially be used for chronic functional constipation or constipation-predominant IBS (Chen et al., 2016a). Ablation has been used extensively for the management of atrial fibrillation, but it role in gastrointestinal arrhythmogenesis is unclear.
Despite the importance of GI electrophysiology, it is considerably underdeveloped compared to the cardiac electrophysiology, which is a sub-specialty of cardiology (O'Grady et al., 2014). A deeper understanding of the molecular basis and physiological mechanisms underlying GI motility disorders will enable the development of better diagnostic and therapeutic tools and the advancement of this field.
Author Contributions
GT: Design of manuscript; drafted and critically revised the manuscript for important intellectual content; preparation of figures. EL: Acquired and interpreted primary research papers; critically revised the manuscript for important intellectual content; preparation of figures. AL: Analyzed and interpreted primary research papers; critically revised the manuscript for important intellectual content. BY: Analyzed and interpreted primary research papers; critically revised the manuscript for important intellectual content. SW: Analyzed and interpreted primary research papers; critically revised the manuscript for important intellectual content.
Conflict of Interest Statement
The authors declare that the research was conducted in the absence of any commercial or financial relationships that could be construed as a potential conflict of interest.
Acknowledgments
GT was awarded a doctoral training award (DTA) from the Biotechnology and Biological Sciences Research Council (BBSRC) at the University of Cambridge. Both GT and SW thank the Croucher Foundation for supporting their clinical assistant professorships.
References
Abell, T. L., Familoni, B., Voeller, G., Werkman, R., Dean, P., Waters, B., et al. (2009). Electrophysiologic, morphologic, and serologic features of chronic unexplained nausea and vomiting: lessons learned from 121 consecutive patients. Surgery 145, 476–485. doi: 10.1016/j.surg.2008.12.006
Abrahamsson, H. (2007). Treatment options for patients with severe gastroparesis. Gut 56, 877–883. doi: 10.1136/gut.2005.078121
Ahn, H. Y., and Karaki, H. (1988). Inhibitory effects of procaine on contraction and calcium movement in vascular and intestinal smooth muscles. Br. J. Pharmacol. 94, 789–796. doi: 10.1111/j.1476-5381.1988.tb11590.x
Akbarali, H. I., Hawkins, E. G., Ross, G. R., and Kang, M. (2010). Ion channel remodeling in gastrointestinal inflammation. Neurogastroenterol. Motil. 22, 1045–1055. doi: 10.1111/j.1365-2982.2010.01560.x
Allessie, M. A., Bonke, F. I., and Schopman, F. J. (1973). Circus movement in rabbit atrial muscle as a mechanism of tachycardia. Circ. Res. 33, 54–62. doi: 10.1161/01.RES.33.1.54
Allessie, M. A., Bonke, F. I., and Schopman, F. J. (1975). The mechanism of supraventricular tachycardia induced by a single premature beat in the isolated left atrium of the rabbit. I. Circus movement as a consequence of unidirectional block of the premature impulse. Recent Adv. Stud. Cardiac Struct. Metab. 5, 303–308.
Allessie, M. A., Bonke, F. I., and Schopman, F. J. (1976). Circus movement in rabbit atrial muscle as a mechanism of tachycardia. II. The role of nonuniform recovery of excitability in the occurrence of unidirectional block, as studied with multiple microelectrodes. Circ. Res. 39, 168–177. doi: 10.1161/01.RES.39.2.168
Allessie, M. A., Bonke, F. I., and Schopman, F. J. (1977). Circus movement in rabbit atrial muscle as a mechanism of tachycardia. III. The “leading circle” concept: a new model of circus movement in cardiac tissue without the involvement of an anatomical obstacle. Circ. Res. 41, 9–18. doi: 10.1161/01.RES.41.1.9
Allessie, M. A., Schalij, M. J., Kirchhof, C. J., Boersma, L., Huybers, M., and Hollen, J. (1989). Experimental electrophysiology and arrhythmogenicity. Anisotropy and ventricular tachycardia. Eur. Heart J. 10, 2–8. doi: 10.1093/eurheartj/10.suppl_E.2
Andresen, V., Camilleri, M., Busciglio, I. A., Grudell, A., Burton, D., McKinzie, S., et al. (2007). Effect of 5 days linaclotide on transit and bowel function in females with constipation-predominant irritable bowel syndrome. Gastroenterology 133, 761–768. doi: 10.1053/j.gastro.2007.06.067
Angeli, T. R., O'Grady, G., Du, P., Paskaranandavadivel, N., Pullan, A. J., Bissett, I. P., et al. (2013). Circumferential and functional re-entry of in vivo slow-wave activity in the porcine small intestine. Neurogastroenterol. Motil. 25, e304–e314. doi: 10.1111/nmo.12085
Antzelevitch, C., Jalife, J., and Moe, G. K. (1980). Characteristics of reflection as a mechanism of reentrant arrhythmias and its relationship to parasystole. Circulation 61, 182–191. doi: 10.1161/01.CIR.61.1.182
Antzelevitch, C., and Moe, G. K. (1981). Electrotonically mediated delayed conduction and reentry in relation to “slow responses” in mammalian ventricular conducting tissue. Circ. Res. 49, 1129–1139. doi: 10.1161/01.RES.49.5.1129
Auerbach, D. S., Grzda, K. R., Furspan, P. B., Sato, P. Y., Mironov, S., and Jalife, J. (2011). Structural heterogeneity promotes triggered activity, reflection and arrhythmogenesis in cardiomyocyte monolayers. J. Physiol. 589, 2363–2381. doi: 10.1113/jphysiol.2010.200576
Baksi, A. J., Kanaganayagam, G. S., and Prasad, S. K. (2015). Arrhythmias in viral myocarditis and pericarditis. Card. Electrophysiol. Clin. 7, 269–281. doi: 10.1016/j.ccep.2015.03.009
Bauer, A. J., Reed, J. B., and Sanders, K. M. (1985). Slow wave heterogeneity within the circular muscle of the canine gastric antrum. J. Physiol. 366, 221–232. doi: 10.1113/jphysiol.1985.sp015793
Belousov, B. P. (1958). A periodically occuring reaction and its mechanism (Russian title). Sborn. Referat. Radiats. Med. 145–147.
Bers, D. M. (2002a). Calcium and cardiac rhythms: physiological and pathophysiological. Circ. Res. 90, 14–17.
Bers, D. M. (2002b). Cardiac excitation-contraction coupling. Nature 415, 198–205. doi: 10.1038/415198a
Bortolotti, M., Sarti, P., Barbara, L., and Brunelli, F. (1990). Gastric myoelectric activity in patients with chronic idiopathic gastroparesis. Neurogastroenterol. Motil. 2, 104–108. doi: 10.1111/j.1365-2982.1990.tb00015.x
Camilleri, M., Bharucha, A. E., Ueno, R., Burton, D., Thomforde, G. M., Baxter, K., et al. (2006). Effect of a selective chloride channel activator, lubiprostone, on gastrointestinal transit, gastric sensory, and motor functions in healthy volunteers. Am. J. Physiol. Gastrointest. Liver Physiol. 290, G942–G947. doi: 10.1152/ajpgi.00264.2005
Chang, J. Y., and Talley, N. J. (2010). Current and emerging therapies in irritable bowel syndrome: from pathophysiology to treatment. Trends Pharmacol. Sci. 31, 326–334. doi: 10.1016/j.tips.2010.04.008
Chen, J. H., Wang, X. Y., Liu, L. W., Yu, W., Yu, Y., Zhao, L., et al. (2013). On the origin of rhythmic contractile activity of the esophagus in early achalasia, a clinical case study. Front. Neurosci. 7:77. doi: 10.3389/fnins.2013.00077
Chen, S., Liu, L., Guo, X., Yao, S., Li, Y., Chen, S., et al. (2016a). Effects of colonic electrical stimulation using different individual parameter patterns and stimulation sites on gastrointestinal transit time, defecation, and food intake. Int. J. Colorectal Dis. 31, 429–437. doi: 10.1007/s00384-015-2457-6
Chen, Z., Sun, B., Tse, G., Jiang, J., and Xu, W. (2016b). Reversibility of both sinus node dysfunction and reduced HCN4 mRNA expression level in an atrial tachycardia pacing model of tachycardia-bradycardia syndrome in rabbit hearts. Int. J. Clin. Exp. Pathol.
Chetaille, P., Preuss, C., Burkhard, S., Côté, J.-M., Houde, C., Castilloux, J., et al. (2014). Mutations in SGOL1 cause a novel cohesinopathy affecting heart and gut rhythm. Nat. Genet. 46, 1245–1249. doi: 10.1038/ng.3113
Coumel, P. (1993). Cardiac arrhythmias and the autonomic nervous system. J. Cardiovasc. Electrophysiol. 4, 338–355. doi: 10.1111/j.1540-8167.1993.tb01235.x
Coumel, P., Cabrol, C., Fabiato, A., Gourgon, R., and Slama, R. (1967). Tachycardiamente par rythme reciproque. Arch. Mal. Coeur Vaiss. 60, 1830–1864.
Coumel, P., Fidelle, J., Lucet, V., Attuel, P., and Bouvrain, Y. (1978). Catecholamine-induced severe ventricular arrhythmias with Adams-Stokes syndrome in children: report of four cases. Br. Heart J. 40, 28–37.
Courtemanche, M., and Winfree, A. T. (1991). Re-entrant rotating waves in a Beeler-Reuter based model of two-dimensional cardiac electrical activity. Int. J. Bifurcation Chaos 1, 431–444. doi: 10.1142/S0218127491000336
Cranefield, P. F. (1977). Action potentials, afterpotentials, and arrhythmias. Circ. Res. 41, 415–423. doi: 10.1161/01.RES.41.4.415
Daniel, E. E., and Chapman, K. M. (1963). Electrical activity of the gastrointestinal tract as an indication of mechanical activity. Am. J. Dig. Dis. 8, 54–102. doi: 10.1007/BF02233560
Davidenko, J. M., Pertsov, A. V., Salomonsz, R., Baxter, W., and Jalife, J. (1992). Stationary and drifting spiral waves of excitation in isolated cardiac muscle. Nature 255, 349–351. doi: 10.1038/355349a0
Der, T., Bercik, P., Donnelly, G., Jackson, T., Berezin, I., Collins, S. M., et al. (2000). Interstitial cells of cajal and inflammation-induced motor dysfunction in the mouse small intestine. Gastroenterology 119, 1590–1599. doi: 10.1053/gast.2000.20221
Dessertenne, F. (1966). La tachycardie ventriculaire a deux foyers opposes variable. Arch. Mal. Coeur 56, 263–272.
Dickens, E. J., Edwards, F. R., and Hirst, G. D. (2001). Selective knockout of intramuscular interstitial cells reveals their role in the generation of slow waves in mouse stomach. J. Physiol. 531, 827–833. doi: 10.1111/j.1469-7793.2001.0827h.x
Dickens, E. J., Hirst, G. D., and Tomita, T. (1999). Identification of rhythmically active cells in guinea-pig stomach. J. Physiol. 514 (Pt 2), 515–531. doi: 10.1111/j.1469-7793.1999.515ae.x
Di Diego, J. M., and Antzelevitch, C. (1993). Pinacidil-induced electrical heterogeneity and extrasystolic activity in canine ventricular tissues. Does activation of ATP-regulated potassium current promote phase 2 reentry? Circulation 88, 1177–1189. doi: 10.1161/01.CIR.88.3.1177
El-Sherif, N., Gough, W. B., and Restivo, M. (1987). Reentrant ventricular arrhythmias in the late myocardial infarction period: 14. Mechanisms of resetting, entrainment, acceleration, or termination of reentrant tachycardia by programmed electrical stimulation. Pacing Clin. Electrophysiol. 10, 341–371. doi: 10.1111/j.1540-8159.1987.tb05974.x
El-Sherif, N., Smith, R. A., and Evans, K. (1981). Canine ventricular arrhythmias in the late myocardial infarction period. 8. Epicardial mapping of reentrant circuits. Circ. Res. 49, 255–265. doi: 10.1161/01.RES.49.1.255
Epstein, I. R. (2006). Predicting complex biology with simple chemistry. Proc. Natl. Acad. Sci. U.S.A. 103, 15727–15728. doi: 10.1073/pnas.0608026103
Eshraghian, A., and Eshraghian, H. (2011). Interstitial cells of Cajal: a novel hypothesis for the pathophysiology of irritable bowel syndrome. Can. J. Gastroenterol. 25, 277–279. doi: 10.1155/2011/478370
Feldman, M., and Schiller, L. R. (1983). Disorders of gastrointestinal motility associated with diabetes mellitus. Ann. Intern. Med. 98, 378–384. doi: 10.7326/0003-4819-98-3-378
Feldstein, A. E., Miller, S. M., El-Youssef, M., Rodeberg, D., Lindor, N. M., Burgart, L. J., et al. (2003). Chronic intestinal pseudoobstruction associated with altered interstitial cells of cajal networks. J. Pediatr. Gastroenterol. Nutr. 36, 492–497. doi: 10.1097/00005176-200304000-00016
Garrey, W. E. (1914). The nature of fibrillary contraction of the heart: its relation to tissue mass and form. Am. J. Physiol. 33, 397–414.
Gizzi, A., Cherubini, C., Migliori, S., Alloni, R., Portuesi, R., and Filippi, S. (2010). On the electrical intestine turbulence induced by temperature changes. Phys. Biol. 7, 16011. doi: 10.1088/1478-3975/7/1/016011
Guinamard, R., Chatelier, A., Demion, M., Potreau, D., Patri, S., Rahmati, M., et al. (2004). Functional characterization of a Ca(2+)-activated non-selective cation channel in human atrial cardiomyocytes. J. Physiol. 558, 75–83. doi: 10.1113/jphysiol.2004.063974
Gullikson, G. W., Okuda, H., Shimizu, M., and Bass, P. (1980). Electrical arrhythmias in gastric antrum of the dog. Am. J. Physiol. 239, G59–G68.
Ikeda, T., Uchida, T., Hough, D., Lee, J. J., Fishbein, M. C., Mandel, W. J., et al. (1996). Mechanism of spontaneous termination of functional reentry in isolated canine right atrium. Evidence for the presence of an excitable but nonexcited core. Circulation 94, 1962–1973. doi: 10.1161/01.CIR.94.8.1962
Irimia, A., and Wikswo, J. P. Jr. (2008). Gastrointestinal arrhythmias are associated with statistically significant fluctuations in systemic information dimension. Physiol. Meas. 29, N33–N40. doi: 10.1088/0967-3334/29/5/N01
Jalife, J., Delmar, M., Anumonwo, J., Berenfeld, O., and Kalifa, J. (eds.). (2009). “Basic mechanisms of cardiac arrhythmias,” in Basic Cardiac Electrophysiology for the Clinician, 2nd Edn (Wiley-Blackwell), 92–121.
January, C. T., Chau, V., and Makielski, J. C. (1991). Triggered activity in the heart: cellular mechanisms of early after-depolarizations. Eur. Heart J. 12, 4–9. doi: 10.1093/eurheartj/12.suppl_F.4
January, C. T., and Riddle, J. M. (1989). Early afterdepolarizations: mechanism of induction and block. A role for L-type Ca2+ current. Circ. Res. 64, 977–990. doi: 10.1161/01.RES.64.5.977
Jung, K. T., Park, H., Kim, J. H., Shin, D. J., Joung, B. Y., Lee, M. H., et al. (2012). The relationship between gastric myoelectric activity and SCN5A mutation suggesting sodium channelopathy in patients with brugada syndrome and functional dyspepsia - a pilot study. J. Neurogastroenterol. Motil. 18, 58–63. doi: 10.5056/jnm.2012.18.1.58
Kim, C. H., Zinsmeister, A. R., and Malagelada, J. R. (1987). Mechanisms of canine gastric dysrhythmia. Gastroenterology 92, 993–999.
Kuo, C. S., Munakata, K., Reddy, C. P., and Surawicz, B. (1983). Characteristics and possible mechanism of ventricular arrhythmia dependent on the dispersion of action potential durations. Circulation 67, 1356–1367. doi: 10.1161/01.CIR.67.6.1356
Lammers, W. J. (2013). Arrhythmias in the gut. Neurogastroenterol. Motil. 25, 353–357. doi: 10.1111/nmo.12116
Lammers, W. J. E. P., Ver Donck, L., Stephen, B., Smets, D., and Schuurkes, J. A. J. (2008). Focal activities and re-entrant propagations as mechanisms of gastric tachyarrhythmias. Gastroenterology 135, 1601–1611. doi: 10.1053/j.gastro.2008.07.020
Lammers, W. J., Schalij, M. J., Kirchhof, C. J., and Allessie, M. A. (1990). Quantification of spatial inhomogeneity in conduction and initiation of reentrant atrial arrhythmias. Am. J. Physiol. 259, H1254–H1263.
Lammers, W. J., and Slack, J. R. (2001). Of slow waves and spike patches. News Physiol. Sci. 16, 138–144.
Lammers, W. J., Stephen, B., and Karam, S. M. (2012). Functional reentry and circus movement arrhythmias in the small intestine of normal and diabetic rats. Am. J. Physiol. Gastrointest. Liver Physiol. 302, G684–G689. doi: 10.1152/ajpgi.00332.2011
Lammers, W. J., Ver Donck, L., Stephen, B., Smets, D., and Schuurkes, J. A. (2009). Origin and propagation of the slow wave in the canine stomach: the outlines of a gastric conduction system. Am. J. Physiol. Gastrointest. Liver Physiol. 296, G1200–G1210. doi: 10.1152/ajpgi.90581.2008
Lee, D. S., and Lee, S. J. (2014). Severe gastroparesis following radiofrequency catheter ablation for atrial fibrillation: suggestion for diagnosis, treatment, and device for gastroparesis after RFCA. Case Rep. Gastrointest. Med. 2014, 6. doi: 10.1155/2014/923637
Lee, J. C., Thuneberg, L., Berezin, I., and Huizinga, J. D. (1999). Generation of slow waves in membrane potential is an intrinsic property of interstitial cells of Cajal. Am. J. Physiol. 277, G409–G423.
Leon, L. J., Roberge, F. A., and Vinet, A. (1994). Simulation of two-dimensional anisotropic cardiac reentry: effects of the wavelength on the reentry characteristics. Ann. Biomed. Eng. 22, 592–609. doi: 10.1007/BF02368286
Lukas, A., and Antzelevitch, C. (1989). Reflected reentry, delayed conduction, and electrotonic inhibition in segmentally depressed atrial tissues. Can. J. Physiol. Pharmacol. 67, 757–764. doi: 10.1139/y89-121
Lukas, A., and Antzelevitch, C. (1996). Phase 2 reentry as a mechanism of initiation of circus movement reentry in canine epicardium exposed to simulated ischemia. Cardiovasc. Res. 32, 593–603. doi: 10.1016/0008-6363(96)00115-0
Lyford, G. L., He, C. L., Soffer, E., Hull, T. L., Strong, S. A., Senagore, A. J., et al. (2002). Pan-colonic decrease in interstitial cells of Cajal in patients with slow transit constipation. Gut 51, 496–501. doi: 10.1136/gut.51.4.496
Manabe, N., Rao, A. S., Wong, B. S., and Camilleri, M. (2010). Emerging pharmacologic therapies for irritable bowel syndrome. Curr. Gastroenterol. Rep. 12, 408–416. doi: 10.1007/s11894-010-0124-1
Maruyama, M., Lin, S. F., Xie, Y., Chua, S. K., Joung, B., Han, S., et al. (2011). Genesis of phase 3 early afterdepolarizations and triggered activity in acquired long-QT syndrome. Circ. Arrhythm. Electrophysiol. 4, 103–111. doi: 10.1161/CIRCEP.110.959064
Mayer, A. G. (1906). Rhythmical Pulsation in Scyphomedusae. Washington, DC: Carnegie Institute of Washington.
McNearney, T. A., Sallam, H. S., Hunnicutt, S. E., Doshi, D., Wollaston, D. E., Mayes, M. D., et al. (2009). Gastric slow waves, gastrointestinal symptoms and peptides in systemic sclerosis patients. Neurogastroenterol. Motil. 21, e1269–e1120. doi: 10.1111/j.1365-2982.2009.01350.x
Miftahof, R. (2005). “A novel intrinsic wave phenomenon in low excitable biological media,” in Mechanisms, Symbols, and Models Underlying Cognition: First International Work-Conference on the Interplay between Natural and Artificial Computation, IWINAC 2005, Las Palmas, Canary Islands, Spain, June 15-18, 2005, Proceedings, Part I, eds J. Mira and J. R. Álvarez (Berlin; Heidelberg: Springer Berlin Heidelberg), 38–47.
Mines, G. R. (1913). On dynamic equilibrium in the heart. J. Physiol. 46, 349–383. doi: 10.1113/jphysiol.1913.sp001596
Moe, G. K., Rheinboldt, W. C., and Abildskov, J. A. (1964). A computer model of atrial fibrillation. Am. Heart J. 67, 200–220. doi: 10.1016/0002-8703(64)90371-0
Müller, S. C., Plesser, T., and Hess, B. (1985). The structure of the core of the spiral wave in the belousov-zhabotinskii reaction. Science 230, 661–663. doi: 10.1126/science.230.4726.661
Nam, G. B., Burashnikov, A., and Antzelevitch, C. (2005). Cellular mechanisms underlying the development of catecholaminergic ventricular tachycardia. Circulation 111, 2727–2733. doi: 10.1161/CIRCULATIONAHA.104.479295
Ng, S. C., Lam, E. F., Lam, T. T., Chan, Y., Law, W., Tse, P. C., et al. (2013). Effect of probiotic bacteria on the intestinal microbiota in irritable bowel syndrome. J. Gastroenterol. Hepatol. 28, 1624–1631. doi: 10.1111/jgh.12306
O'Grady, G., Angeli, T. R., Du, P., Lahr, C., Lammers, W. J., Windsor, J. A., et al. (2012). Abnormal initiation and conduction of slow-wave activity in gastroparesis, defined by high-resolution electrical mapping. Gastroenterology 143, 589-598.e1–3. doi: 10.1053/j.gastro.2012.05.036
O'Grady, G., Egbuji, J. U., Du, P., Lammers, W. J., Cheng, L. K., Windsor, J. A., et al. (2011). High-resolution spatial analysis of slow wave initiation and conduction in porcine gastric dysrhythmia. Neurogastroenterol. Motil. 23, e345–e355. doi: 10.1111/j.1365-2982.2011.01739.x
O'Grady, G., Wang, T. H., Du, P., Angeli, T., Lammers, W. J., and Cheng, L. K. (2014). Recent progress in gastric arrhythmia: pathophysiology, clinical significance and future horizons. Clin. Exp. Pharmacol. Physiol. 41, 854–862. doi: 10.1111/1440-1681.12288
Oliveira, M., da Silva, N., Cunha, P., Ramos, R., Marques, F., Santos, S., et al. (2011). Effects of acute autonomic modulation on atrial conduction delay and local electrograms duration in paroxysmal atrial fibrillation. Int. J. Cardiol. 149, 290–295. doi: 10.1016/j.ijcard.2010.02.006
Osadchii, O. E. (2010). Mechanisms of hypokalemia-induced ventricular arrhythmogenicity. Fundam. Clin. Pharmacol. 24, 547–559. doi: 10.1111/j.1472-8206.2010.00835.x
Osadchii, O. E. (2012a). Effects of ventricular pacing protocol on electrical restitution assessments in guinea-pig heart. Exp. Physiol. 97, 807–821. doi: 10.1113/expphysiol.2012.065219
Osadchii, O. E. (2012b). Flecainide-induced proarrhythmia is attributed to abnormal changes in repolarization and refractoriness in perfused guinea-pig heart. J. Cardiovasc. Pharmacol. 60, 456–466. doi: 10.1097/FJC.0b013e31826b86cf
Osadchii, O. E. (2014a). Impact of hypokalemia on electromechanical window, excitation wavelength and repolarization gradients in guinea-pig and rabbit hearts. PLoS ONE 9:e105599. doi: 10.1371/journal.pone.0105599
Osadchii, O. E. (2014b). Impaired epicardial activation-repolarization coupling contributes to the proarrhythmic effects of hypokalaemia and dofetilide in guinea pig ventricles. Acta Physiol. 211, 48–60. doi: 10.1111/apha.12259
Osadchii, O. E. (2016). Flecainide attenuates rate adaptation of ventricular repolarization in guinea-pig heart. Scand. Cardiovasc. J. 50, 28–35. doi: 10.3109/14017431.2015.1099721
Osadchii, O. E., Bentzen, B. H., and Olesen, S. P. (2009). Chamber-specific effects of hypokalaemia on ventricular arrhythmogenicity in isolated, perfused guinea-pig heart. Exp. Physiol. 94, 434–446. doi: 10.1113/expphysiol.2008.045567
Osadchii, O. E., Larsen, A. P., and Olesen, S. P. (2010). Predictive value of electrical restitution in hypokalemia-induced ventricular arrhythmogenicity. Am. J. Physiol. Heart Circ. Physiol. 298, H210–H220. doi: 10.1152/ajpheart.00695.2009
Osadchii, O. E., and Olesen, S. P. (2009). Electrophysiological determinants of hypokalaemia-induced arrhythmogenicity in the guinea-pig heart. Acta Physiol. 197, 273–287. doi: 10.1111/j.1748-1716.2009.02028.x
Ouyang, X., Li, S., Foreman, R., Farber, J., Lin, L., Yin, J., et al. (2015). Hyperglycemia-induced small intestinal dysrhythmias attributed to sympathovagal imbalance in normal and diabetic rats. Neurogastroenterol. Motil. 27, 406–415. doi: 10.1111/nmo.12506
Patterson, E., Lazzara, R., Szabo, B., Liu, H., Tang, D., Li, Y. H., et al. (2006). Sodium-calcium exchange initiated by the Ca2+ transient: an arrhythmia trigger within pulmonary veins. J. Am. Coll. Cardiol. 47, 1196–1206. doi: 10.1016/j.jacc.2005.12.023
Pertsov, A. M., Davidenko, J. M., Salomonsz, R., Baxter, W. T., and Jalife, J. (1993). Spiral waves of excitation underlie reentrant activity in isolated cardiac muscle. Circ. Res. 72, 631–650. doi: 10.1161/01.RES.72.3.631
Priori, S. G., Napolitano, C., Tiso, N., Memmi, M., Vignati, G., Bloise, R., et al. (2001). Mutations in the cardiac ryanodine receptor gene (hRyR2) underlie catecholaminergic polymorphic ventricular tachycardia. Circulation 103, 196–200. doi: 10.1161/01.CIR.103.2.196
Publicover, N. G., and Sanders, K. M. (1986). Effects of frequency on the wave form of propagated slow waves in canine gastric antral muscle. J. Physiol. 371, 179–189. doi: 10.1113/jphysiol.1986.sp015967
Qian, L. W., Pasricha, P. J., and Chen, J. D. (2003). Origins and patterns of spontaneous and drug-induced canine gastric myoelectrical dysrhythmia. Dig. Dis. Sci. 48, 508–515. doi: 10.1023/A:1022532515172
Rensma, P. L., Allessie, M. A., Lammers, W. J., Bonke, F. I., and Schalij, M. J. (1988). Length of excitation wave and susceptibility to reentrant atrial arrhythmias in normal conscious dogs. Circ. Res. 62, 395–410. doi: 10.1161/01.RES.62.2.395
Rozanski, G. J., Jalifé, J., and Moe, G. K. (1984). Reflected reentry in nonhomogeneous ventricular muscle as a mechanism of cardiac arrhythmias. Circulation 69, 163–173. doi: 10.1161/01.CIR.69.1.163
Saito, Y. A., Strege, P. R., Tester, D. J., Locke, G. R. III Talley, N. J., Bernard, C. E., et al. (2009). Sodium channel mutation in irritable bowel syndrome: evidence for an ion channelopathy. Am. J. Physiol. Gastrointest. Liver Physiol. 296, G211–G218. doi: 10.1152/ajpgi.90571.2008
Salzberg, B. M., Davila, H. V., and Cohen, L. B. (1973). Optical recording of impulses in individual neurones of an invertebrate central nervous system. Nature 246, 508–509. doi: 10.1038/246508a0
Sarna, S. K., and Daniel, E. E. (1973). Electrical stimulation of gastric electrical control activity. Am. J. Physiol. 225, 125–131.
Sarna, S. K., and Otterson, M. F. (1990). Small intestinal amyogenesia and dysmyogenesia induced by morphine and loperamide. Am. J. Physiol. 258, G282–G289.
Scheffer, R. C., and Smout, A. J. (2011). Tachyduodenia in mitochondrial neurogastrointestinal encephalomyopathy. Neurogastroenterol. Motil. 23, 408–410. doi: 10.1111/j.1365-2982.2011.01698.x
Seidel, S. A., Hegde, S. S., Bradshaw, L. A., Ladipo, J. K., and Richards, W. O. (1999). Intestinal tachyarrhythmias during small bowel ischemia. Am. J. Physiol. 277, G993–G999.
Shen, M. J., and Zipes, D. P. (2014). Role of the autonomic nervous system in modulating cardiac arrhythmias. Circ. Res. 114, 1004–1021. doi: 10.1161/CIRCRESAHA.113.302549
Shimizu, W., Aiba, T., and Kamakura, S. (2005). Mechanisms of disease: current understanding and future challenges in Brugada syndrome. Nat. Clin. Pract. Cardiovasc. Med. 2, 408–414. doi: 10.1038/ncpcardio0268
Sinagra, E., Pompei, G., Tomasello, G., Cappello, F., Morreale, G. C., Amvrosiadis, G., et al. (2016). Inflammation in irritable bowel syndrome: myth or new treatment target? World J. Gastroenterol. 22, 2242–2255. doi: 10.3748/wjg.v22.i7.2242
Sinha, S., Stein, K. M., and Christini, D. J. (2002). Critical role of inhomogeneities in pacing termination of cardiac reentry. Chaos 12, 893–902. doi: 10.1063/1.1501176
Smeets, J. L., Allessie, M. A., Lammers, W. J., Bonke, F. I., and Hollen, J. (1986). The wavelength of the cardiac impulse and reentrant arrhythmias in isolated rabbit atrium. The role of heart rate, autonomic transmitters, temperature, and potassium. Circ. Res. 58, 96–108. doi: 10.1161/01.RES.58.1.96
Stoddard, C. J., Smallwood, R. H., and Duthie, H. L. (1981). Electrical arrhythmias in the human stomach. Gut 22, 705–712. doi: 10.1136/gut.22.9.705
Struijs, M.-C., Diamond, I. R., Pencharz, P. B., Chang, K. T. E., Viero, S., Langer, J. C., et al. (2008). Absence of the interstitial cells of Cajal in a child with chronic pseudoobstruction. J. Pediatr. Surg. 43, e25–e29. doi: 10.1016/j.jpedsurg.2008.09.017
Suzuki, H., and Hirst, G. D. S. (1999). Regenerative potentials evoked in circular smooth muscle of the antral region of guinea-pig stomach. J. Physiol. 517, 563–573. doi: 10.1111/j.1469-7793.1999.0563t.x
Szabo, B., Sweidan, R., Rajagopalan, C. V., and Lazzara, R. (1994). Role of Na+:Ca2+ exchange current in Cs(+)-induced early afterdepolarizations in Purkinje fibers. J. Cardiovasc. Electrophysiol. 5, 933–944. doi: 10.1111/j.1540-8167.1994.tb01133.x
Tana, C., Umesaki, Y., Imaoka, A., Handa, T., Kanazawa, M., and Fukudo, S. (2010). Altered profiles of intestinal microbiota and organic acids may be the origin of symptoms in irritable bowel syndrome. Neurogastroenterol. Motil. 22, e512–e115. doi: 10.1111/j.1365-2982.2009.01427.x
Tse, G. (2015). Mechanisms of cardiac arrhythmias. J. Arrhythm. 32, 75–81. doi: 10.1016/j.joa.2015.11.003
Tse, G. (2016a). Both transmural dispersion of repolarization and transmural dispersion of refractoriness are poor predictors of arrhythmogenicity: a role for the index of Cardiac Electrophysiological Balance (QT/QRS)? J. Geriatr. Cardiol.
Tse, G. (2016b). Novel conduction-repolarization indices for the stratification of arrhythmic risk. J. Geriatr. Cardiol.
Tse, G. (2016c). (Tpeak-Tend)/QRS and (Tpeak-Tend)/(QT x QRS): novel markers for predicting arrhythmic risk in Brugada syndrome. Europace.
Tse, G., Ali, A., Alpendurada, F., Prasad, S., Raphael, C. E., and Vassiliou, V. (2015a). Tuberculous constrictive pericarditis. Res Cardiovasc. Med. 4:e29614. doi: 10.5812/cardiovascmed.29614
Tse, G., Ali, A., Prasad, S. K., Vassiliou, V., and Raphael, C. E. (2015b). Atypical case of post-partum cardiomyopathy: an overlap syndrome with arrhythmogenic right ventricular cardiomyopathy? BJR Case Rep. 1:20150182. doi: 10.1259/bjrcr.20150182
Tse, G., Hothi, S. S., Grace, A. A., and Huang, C. L. (2012). Ventricular arrhythmogenesis following slowed conduction in heptanol-treated, Langendorff-perfused mouse hearts. J. Physiol. Sci. 62, 79–92. doi: 10.1007/s12576-011-0187-2
Tse, G., Lai, E. T., Tse, V., and Yeo, J. M. (2016a). Molecular and electrophysiological mechanisms underlying cardiac arrhythmogenesis in diabetes mellitus. J. Diabetes Res.
Tse, G., Lai, E. T., Yeo, J. M., and Yan, B. P. (2016b). Electrophysiological mechanisms of Bayés syndrome: insights from clinical and mouse studies. Front. Physiol. 7:188. doi: 10.3389/fphys.2016.00188
Tse, G., Lai, T. H., Yeo, J. M., Tse, V., and Wong, S. H. (2016c). Mechanisms of electrical activation and conduction in the gastrointestinal system: lessons from cardiac electrophysiology. Front. Physiol. 7:182. doi: 10.3389/fphys.2016.00182
Tse, G., Sun, B., Wong, S. T., Tse, V., and Yeo, J. M. (2016d). Ventricular anti-arrhythmic effects of hypercalcaemia treatment in hyperkalaemic, Langendorff-perfused mouse hearts. Biomed. Rep. doi: 10.3892/br.2016.577. [Epub ahead of print].
Tse, G., Tse, V., and Yeo, J. M. (2016e). Ventricular anti-arrhythmic effects of heptanol in hypokalaemic, Langendorff-perfused mouse hearts. Biomed Rep. 4, 313–324. doi: 10.3892/br.2016.577
Tse, G., Tse, V., Yeo, J. M., and Sun, B. (2016f). Atrial anti-arrhythmic effects of heptanol in Langendorff-perfused mouse hearts. PLoS ONE 11:e0148858. doi: 10.1371/journal.pone.0148858
Tse, G., Wong, S. T., Tse, V., and Yeo, J. M. (2016g). Depolarization vs. repolarization: what is the mechanism of ventricular arrhythmogenesis underlying sodium channel haploinsufficiency in mouse hearts? Acta Physiol. doi: 10.1111/apha.12694. [Epub ahead of print].
Tse, G., Wong, S. T., Tse, V., and Yeo, J. M. (2016h). Determination of action potential wavelength restitution in Scn5a+/− mouse hearts modelling human Brugada syndrome. J. Physiol.
Tse, G., Wong, S. T., Tse, V., and Yeo, J. M. (2016i). Monophasic action potential recordings: which is the recording electrode? J. Basic Clin. Physiol. Pharmacol. doi: 10.1515/jbcpp-2016-0007. [Epub ahead of print].
Tse, G., Wong, S. T., Tse, V., and Yeo, J. M. (2016j). Restitution analysis of alternans using dynamic pacing and its comparison with S1S2 restitution in heptanol-treated, hypokalaemic Langendorff-perfused mouse hearts. Biomed Rep. 4, 673–680. doi: 10.3892/br.2016.659
Tse, G., Wong, S. T., Tse, V., Lee, Y. T., Lin, H. Y., and Yeo, J. M. (2016k). Cardiac dynamics: alternans and arrhythmogenesis. J. Arrhythm. doi: 10.1016/j.joa.2016.02.009. [Epub ahead of print].
Tse, G., and Yan, B. P. (2016). Novel arrhythmic risk markers incorporating QRS dispersion: QRSd x (Tpeak-Tend)/QRS and QRSd x (Tpeak-Tend)/(QT x QRS). Ann. Noninvasive. Electrocardiol.
Tse, G., and Yeo, J. M. (2015). Conduction abnormalities and ventricular arrhythmogenesis: the roles of sodium channels and gap junctions. Int. J. Cardiol. Heart Vasc. 9, 75–82. doi: 10.1016/j.ijcha.2015.10.003
Tung, L. (2011). Expanding on forty years of reflection. J. Physiol. 589, 2107–2108. doi: 10.1113/jphysiol.2011.209239
Vaidya, D., Morley, G. E., Samie, F. H., and Jalife, J. (1999). Reentry and fibrillation in the mouse heart. A challenge to the critical mass hypothesis. Circ. Res. 85, 174–181. doi: 10.1161/01.RES.85.2.174
Vassiliou, V., Chin, C., Perperoglou, A., Tse, G., Ali, A., Raphael, C., et al. (2014). 93 Ejection fraction by cardiovascular magnetic resonance predicts adverse outcomes post aortic valve replacement. Heart 100, A53–A54. doi: 10.1136/heartjnl-2014-306118.93
Vazeou, A., Papadopoulou, A., Papadimitriou, A., Kitsou, E., Stathatos, M., and Bartsocas, C. S. (2004). Autonomic neuropathy and gastrointestinal motility disorders in children and adolescents with type 1 diabetes mellitus. J. Pediatr. Gastroenterol. Nutr. 38, 61–65. doi: 10.1097/00005176-200401000-00014
Weiss, J. N., Chen, P. S., Qu, Z., Karagueuzian, H. S., and Garfinkel, A. (2000). Ventricular fibrillation: how do we stop the waves from breaking? Circ. Res. 87, 1103–1107. doi: 10.1161/01.RES.87.12.1103
Weiss, J. N., Garfinkel, A., Karagueuzian, H. S., Chen, P. S., and Qu, Z. (2010). Early afterdepolarizations and cardiac arrhythmias. Heart Rhythm 7, 1891–1899. doi: 10.1016/j.hrthm.2010.09.017
Weiss, J. N., Qu, Z., Chen, P. S., Lin, S. F., Karagueuzian, H. S., Hayashi, H., et al. (2005). The dynamics of cardiac fibrillation. Circulation 112, 1232–1240. doi: 10.1161/CIRCULATIONAHA.104.529545
Wiener, N., and Rosenblueth, A. (1946). The mathematical formulation of the problem of conduction of impulses in a network of connected excitable elements, specifically in cardiac muscle. Arch. Inst. Cardiol. Mex. 16, 205–265.
Wong, W. T., Tian, X. Y., and Huang, Y. (2013). Endothelial dysfunction in diabetes and hypertension: cross talk in RAS, BMP4, and ROS-dependent COX-2-derived prostanoids. J. Cardiovasc. Pharmacol. 61, 204–214. doi: 10.1097/FJC.0b013e31827fe46e
Wu, R. Y., Pasyk, M., Wang, B., Forsythe, P., Bienenstock, J., Mao, Y. K., et al. (2013). Spatiotemporal maps reveal regional differences in the effects on gut motility for Lactobacillus reuteri and rhamnosus strains. Neurogastroenterol. Motil. 25, e205–e214. doi: 10.1111/nmo.12072
Yamataka, A., Kato, Y., Tibboel, D., Murata, Y., Sueyoshi, N., Fujimoto, T., et al. (1995). A lack of intestinal pacemaker (c-kit) in aganglionic bowel of patients with Hirschsprung's disease. J. Pediatr. Surg. 30, 441–444. doi: 10.1016/0022-3468(95)90051-9
Yanagida, H., Sanders, K. M., and Ward, S. M. (2007). Inactivation of inducible nitric oxide synthase protects intestinal pacemaker cells from postoperative damage. J. Physiol. 582, 755–765. doi: 10.1113/jphysiol.2006.126482
Yeo, Q. M., Crutchley, R., Cottreau, J., Tucker, A., and Garey, K. W. (2013). Crofelemer, a novel antisecretory agent approved for the treatment of HIV-associated diarrhea. Drugs Today 49, 239–252. doi: 10.1358/dot.2013.49.4.1947253
Keywords: gastrointestinal electrophysiology, cardiac electrophysiology, electrical excitation, arrhythmia, focal activity, reentry
Citation: Tse G, Lai ETH, Lee APW, Yan BP and Wong SH (2016) Electrophysiological Mechanisms of Gastrointestinal Arrhythmogenesis: Lessons from the Heart. Front. Physiol. 7:230. doi: 10.3389/fphys.2016.00230
Received: 02 May 2016; Accepted: 30 May 2016;
Published: 14 June 2016.
Edited by:
Yusuke Sata, Baker IDI Heart and Diabetes Institute, AustraliaReviewed by:
Shuji Shimizu, National Cerebral and Cardiovascular Center, JapanDaisuke Kobayashi, Baker IDI Heart and Diabetes Institute, Australia
Copyright © 2016 Tse, Lai, Lee, Yan and Wong. This is an open-access article distributed under the terms of the Creative Commons Attribution License (CC BY). The use, distribution or reproduction in other forums is permitted, provided the original author(s) or licensor are credited and that the original publication in this journal is cited, in accordance with accepted academic practice. No use, distribution or reproduction is permitted which does not comply with these terms.
*Correspondence: Gary Tse, Z2FyeS50c2VAZG9jdG9ycy5vcmcudWs=;
Sunny H. Wong, d29uZ2hlaUBjdWhrLmVkdS5oaw==