- 1School of Physical Education, Sport and Exercise Sciences, Division of Sciences, University of Otago, Dunedin, New Zealand
- 2Department of Physiology, Division of Health Sciences, University of Otago, Dunedin, New Zealand
- 3School of Sport, Exercise and Rehabilitation Sciences, University of Birmingham, Birmingham, United Kingdom
Exercise and exogenous heat each stimulate multiple adaptations, but their roles are not well delineated, and that of the related stressor, dehydration, is largely unknown. While severe and prolonged hypohydration potentially “silences” the long-term heat acclimated phenotype, mild and transient dehydration may enhance cardiovascular and fluid-regulatory adaptations. We tested the hypothesis that exogenous heat stress and dehydration additively potentiate acute (24 h) cardiovascular and hematological outcomes following exercise. In a randomized crossover study, 10 physically-active volunteers (mean ± SD: 173 ± 11 cm; 72.1 ± 11.5 kg; 24 ± 3 year; 6 females) completed three trials of 90-min orthostatically-stressful calisthenics, in: (i) temperate conditions (22°C, 50% rh, no airflow; CON); (ii) heat (40°C, 60% rh) whilst euhydrated (HEAT), and (iii) heat with dehydration (no fluid ~16 h before and during exercise; HEAT+DEHY). Using linear mixed effects model analyses, core temperature (TCORE) rose 0.7°C more in HEAT than CON (95% CL: [0.5, 0.9]; p < 0.001), and another 0.4°C in HEAT+DEHY ([0.2, 0.5]; p < 0.001, vs. HEAT). Skin temperature also rose 1.2°C more in HEAT than CON ([0.6, 1.8]; p < 0.001), and similarly to HEAT+DEHY (p = 0.922 vs. HEAT). Peak heart rate was 40 b·min−1 higher in HEAT than in CON ([28, 51]; p < 0.001), and another 15 b·min−1 higher in HEAT+DEHY ([3, 27]; p = 0.011, vs. HEAT). Mean arterial pressure at 24-h recovery was not consistently below baseline after CON or HEAT (p ≥ 0.452), but was reduced 4 ± 1 mm Hg after HEAT+DEHY ([0, 8]; p = 0.020 vs. baseline). Plasma volume at 24 h after exercise increased in all trials; the 7% increase in HEAT was not reliably more than in CON (5%; p = 0.335), but was an additional 4% larger after HEAT+DEHY ([1, 8]; p = 0.005 vs. HEAT). Pooled-trial correlational analysis showed the rise in TCORE predicted the hypotension (r = −0.4) and plasma volume expansion (r = 0.6) at 24 h, with more hypotension reflecting more plasma expansion (r = −0.5). In conclusion, transient dehydration with heat potentiates short-term (24-h) hematological (hypervolemic) and cardiovascular (hypotensive) outcomes following calisthenics.
Introduction
Exercise is a multi-factorial, pluripotent stress that produces strain in several physiological systems. The nature of the stress dictates the profile of strain incurred, and thus the adaptive stimulus. The adaptations that occur as a result of this strain profile accrue as fitness, which improves an individual's physiological and functional capacities, and quality of life. The necessary stressors within exercise that are required to elicit a given response, or adaptation, are only partially resolved. Often the complex nature of exercise makes the delineation of these stressors difficult, and thus their individual and combined roles in adaptation also remain unclear.
Changes in blood pressure (BP) and blood volume (including plasma volume; PV) are principal components of the cardiovascular benefit of regular physical activity. However, the optimal means of facilitating these adaptations is elusive. The anti-hypertensive benefits associated with exercise (Pescatello et al., 2004, 2015) might be partly facilitated by repeated bouts of acute post-exercise hypotension (PEH; Thompson et al., 2001), and contribute to subsequent PV expansion (i.e., PVE/hypervolemia; Nagashima et al., 1999, 2000; Hayes et al., 2000). Assessing the acute cardiovascular outcomes to a conditioning stimulus, which are also important in their own right, may therefore also provide an indication of long-term cardiovascular adaptations (Liu et al., 2012). Exercise intensity moderates both PEH (Eicher et al., 2010; Halliwill et al., 2013; Graham et al., 2016) and PVE (Nadel et al., 1979; Convertino et al., 1981), such that a higher intensity elicits larger PEH and PVE, however finding an appropriate and effective exercise intensity to afford these benefits to all individuals is convoluted. Indeed, low-intensity exercise may be insufficient to confer these benefits (Wallace et al., 1999; Forjaz et al., 2004; Farinatti et al., 2011) despite its accessibility to the population. Thus, complementing low-intensity exercise with additional independent stressors may exacerbate the physiological strain, and provide an avenue for acute cardiovascular outcomes.
Heat stress and dehydration are by-products of exercise and stressors in their own right. The imposition of heat (Francesconi et al., 1983a; Ray and Gracey, 1997; Wright et al., 2010) and dehydration (Finberg et al., 1974; Nadel et al., 1980; Francesconi et al., 1983b; Brandenberger et al., 1986) increases strain in multiple systems at rest and in exercise, particularly when there is concurrent orthostatic stress (Beetham and Buskirk, 1958; Saltin and Stenberg, 1964; Ray et al., 1993; Gonzalez-Alonso et al., 1999). An increase in strain, and potential augmented adaptive stimulus, occurs despite low exercise intensity in dynamic aerobic (Finberg et al., 1974; Francesconi et al., 1983b), and isometric exercise (Ray and Gracey, 1997; Binder et al., 2012). Orthostasis and heat appear to be important stressors in stimulating PVE (Convertino et al., 1981; Nagashima et al., 1999, 2000) and potentially PEH (Halliwill et al., 2013), however the role of dehydration is equivocal and largely unexplored (Akerman et al., 2016). Considering that dehydration exacerbates the cardiovascular and hormonal (especially sympathetic and fluid regulatory) pathways associated with both PVE and PEH (Melin et al., 1997, 2001; Gonzalez-Alonso et al., 1999), it may also moderate these outcomes somewhat. Thus, identifying the separate and combined roles of these exercise- and environment-related stressors in driving or impairing adaptive processes is important for basic understanding and may aid in exercise prescription for a range of populations; especially those unable (or unwilling) to undertake moderate- and higher-intensity exercise.
The aim of the current study was therefore to determine the cumulative effects of orthostatically stressful, low-intensity exercise (dynamic body weight exercises, i.e., calisthenics), exogenous heat stress, and dehydration, on acute (24-h) cardiovascular and fluid regulatory outcomes. The separate effects of these stressors were not delineated per se, but rather their cumulative effects were examined in forming a composite stimulus (i.e., low-intensity exercise + heat stress + dehydration). This exercise type was chosen as an accessible mode and intensity for a majority of the population, whilst providing substantial cardiovascular strain, and force through all major muscle groups. We hypothesized that the addition of each stressor would provide a further stimulus for acute cardiovascular outcomes. Namely, plasma volume expansion at 24 h after exercise would be larger with the addition of each stressor, by virtue of inducing additional thermal and cardiovascular strain.
Methods
Participants
Ten habitually-active, young healthy individuals participated; six females and four males (mean ± SD, aged 24 ± 3 year, mass 72.1 ± 11.5 kg, and height 173 ± 11 cm). All were non-smokers, recreationally physically active on 3 to 5 days per week, and unacclimatised to heat. They were required to refrain from alcohol, caffeine and physical activity for 24 h before each experimental trial. All female participants had been taking the oral contraceptive pill for at least the previous 2 years, and undertook experimental trials in their self-reported early follicular phase. Written informed consent was provided by all individuals prior to participation, and all procedures were approved by the Institutional Human Ethics Committee of the University of Otago (Project No. 12/125), and conformed to the Declaration of Helsinki.
Experimental Protocol
Design
The study was a randomized, counter-balanced crossover design. Following familiarization to the exercise protocol (one occasion in CON conditions, approximately 1 week prior to first exercise condition) and all experimental procedures and instrumentations, participants reported to the laboratory for three experimental trials, or “exercise conditions.” The three exercise conditions were: calisthenics in a temperate environment [22.2 ± 0.3°C, 51.2 ± 1.5% relative humidity (RH)] in a euhydrated state (i.e., CON); calisthenics in a hot, humid environment (40.4 ± 0.4°C, 60.5 ± 1.9% RH) in a euhydrated state (HEAT); and calisthenics in a hot, humid environment in a mildly-hypohydrated state, with further dehydration during the session (HEAT+DEHY). No exogenous airflow was provided. Each condition was at the same time of day (08:00 h) and included baseline measurements, a 90-min exercise protocol, and four follow-up measurement periods over 24 h of recovery (2, 3, 5, and 24 h after exercise; Figure 1). Exercise conditions were separated by 2–7 days, with a minimum of 2 days separating a temperate to hot condition, and 4 days between hot conditions. Participants recorded physical activity, food and fluid intake, and menstrual cycle logs for the 24 h prior to, and throughout the first exercise condition, and then replicated this for the remaining two conditions.
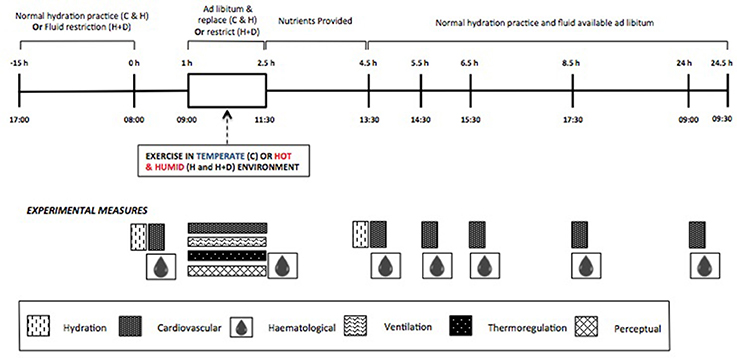
Figure 1. Schematic representation of standardizations and experimental measurements in the three exercise conditions (C, Control; H, Heat; H+D, Heat and dehydration).
The three exercise conditions were completed on days that required similar 24-h physical activity requirements and often similar duration in the same posture (i.e., walking to and from appointments, and sitting in meetings or lectures). Participants were encouraged to consume 500 mL of water with dinner the night before, and another 500 mL with breakfast on the morning of the euhydrated conditions (CON and HEAT). Further to ad libitum fluid intake (water) during the euhydrated trials, body mass (BM) was measured at 15-min intervals during exercise, and sufficient water was provided to replace any BM deficit. The dehydration protocol in HEAT+DEHY consisted of ~16-h fluid restriction (from 17:00 h the night before, to 09:00 h on day of testing; i.e., hypohydration), followed by no fluid replacement during the exercise.
Procedure
Participants arrived at the laboratory following a (self-reported) standardized fluid and meal intake. After voiding their bladder (second void of the morning) and inserting a rectal thermistor, participants were weighed semi-nude (underwear only) then rested supine before blood pressure (BP) was recorded and venous blood was drawn. Participants were then seated for 10 min to stabilize fluid compartment volumes (Ahlgrim et al., 2010) before capillary blood samples were obtained for tracking changes in plasma volume (PV). During this rest period participants were also instrumented with skin thermistors and a heart rate (HR) monitor.
Participants then entered an environmental chamber and sat for 5 min before recording of their resting HR, core (TCORE) and skin temperatures (TSKIN), and psychophysical perceptions (detailed below). This 5-min period was replicated at the end of the protocol. Participants then completed 10 min of squats at 0.2 Hz (5 min at start and end), and 70 min of calisthenics. Thus, the total “exercise protocol” was 90-min, consisting of the seated rest period, squats, and calisthenics. Respiratory gases, thermal, cardiac, and perceived strain was recorded throughout. The squat procedure was intended to provide insight into cerebrovascular autoregulation following orthostatically stressful exercise. Due to technical problems maintaining cerebrovascular measures throughout trials in the heat, these data are not of sufficient quality to present.
Participants then left the environmental chamber and sat in a temperate environment (~26°C, 27% RH) for 10 min before capillary blood samples were obtained, and lay supine for another 10 min before BP was measured and another venous blood sample was drawn (both supine). Participants voided their bladder, toweled dry and had semi-nude body mass measured. Participants were then provided with 250 mL of yogurt (37 g carbohydrate, 9 g protein, 4 g fat and 90 mg sodium) and 500 mL of fruit juice (1:1 fruit juice and water mix, 25 g carbohydrate, 1 g protein, and ~820 mg sodium) to consume as soon as possible, and return to their normal hydration level ad libitum thereafter (same juice/salt/water mix available throughout recovery). This standardized meal was chosen due to the importance of immediate protein (De Feo et al., 1992) and sodium (Luetkemeier et al., 1997) supplementation on outcome measures.
Participants returned to the laboratory 2, 3, 5, and 24 h after completing the exercise protocol for recovery measures. Participants provided a urine sample, and semi-nude body mass measurement, followed by BP measurement, venous blood (both supine after 10-min rest), and capillary blood samples after 10 min of seated rest (Figure 1).
Exercise Protocol
The exercise protocol was intended to represent a routine suitable for individuals unable to complete more traditional exercise forms. The composition and progression of exercises was designed specifically for this study, with particular emphasis on a whole-body stress and repeated changes in posture (i.e., orthostatically stressful). A detailed description of the calisthenics protocol is provided in Supplementary Data Sheet 1 (https://www.youtube.com/watch?v=FxMdPDUk8Fo) and Supplementary Figure 1. To summarize, 28 body-weight resistance movements (i.e., calisthenics), and yoga postures (herein referred to collectively as “exercises”) were completed for 70 min. The protocol consisted of ~35 min of standing exercises (7 exercises at start, 5 exercises at the end), ~15 min of supine exercises (7 exercises), ~15 min of exercises on hands and knees (6 exercises), and ~5 min of prone exercises (3 exercises).
Measurements
Semi-nude body mass was measured to a resolution of 20 g using calibrated electronic scales (Wedderburn, Dunedin, New Zealand) before and after each exercise condition, and at 15-min intervals during the two hydrated exercise conditions (i.e., CON and HEAT). Specific gravity of urine (USG) was measured using a hand-held refractometer (Atago, Tokyo, Japan) before and after exercise.
Blood pressure was measured using a stethoscope and sphygmomanometer, by the same researcher (AA) on all occasions, and reported as the mean of 3 readings over ~5 min. Heart rate was measured from the R-R interval of ventricular depolarisation, and recorded at 1-min intervals via telemetry to a wristwatch (Model RS400, Polar, Finland). Core temperature was measured using a rectal thermistor (400 series; Mallinckrodt Inc. USA) inserted 10 cm beyond the external anal sphincter. Skin temperature was measured using thermistors attached to the thigh, chest, back, and bicep using adhesive tape. Core and skin temperatures were recorded at 1-min intervals (DaqPRO 5300, Omega, USA.). Ratings of perceived exertion (RPE, ranging from 6 to 20; Borg, 1982), thermal sensation (ranging from 1 to 13), and thermal discomfort (ranging from 1 to 5; both extended from Gagge et al., 1967) were obtained using 15-, 13-, and 5-point scales respectively. Expired oxygen (O2) and carbon dioxide (CO2) concentrations and volumes were determined using open-circuit spirometry (Cosmed, CosmedSrl, Rome, Italy) to calculate oxygen consumption (O2) and partial pressure of end-tidal carbon dioxide (PETCO2). Gas analysers were calibrated before a participant's exercise session.
Arterialised capillary blood samples (75 μL) were obtained from the finger, in triplicate. Hemoglobin (Hb) concentrations were determined using reflectance photometry (OSM3; Radiometer, Copenhagen, Denmark). Hematocrit (Hct) was measured from the remaining portion (~50 μL) of each sample using a custom-made Vernier caliper reader after centrifugation (Thermo IEC, MicroCL 17, radius 8.5 cm) for 10 min at 855 g.
Venous blood samples (18 mL) were drawn without stasis from an antecubital vein. Whole-blood samples were centrifuged immediately at 4°C for 10 min, and plasma was separated and stored at −80°C for subsequent batch analysis. Plasma osmolality (POSM) was measured using a three-point calibrated (100, 290, and 1,000 mOsmol·kg−1) dew-point temperature depression method, in duplicate (triplicate if ≥2 mOsmol·kg−1 difference; Model 5,520, Vapro osmometer, Wescore, Logan, UT, USA). Plasma aldosterone (PALD) and erythropoietin (PEPO) were measured using enzyme-linked immunosorbent assay (ELISA) commercially available kits (Abnova, Taiwan; and Abcam, Australia, respectively). All samples for a given participant were analyzed within the same assay, and handled by the same researcher (AA or RK).
Plasma aldosterone concentrations were determined from calibration standards (0–1,000 pg·mL−1) and control samples, using a standard curve derived from the log transformation of the optical density of each sample. The curve was generated using commercially available software (GraphPad Prism, Version 6, GraphPad Software, CA, USA). The intra-assay coefficient of variation for PALD concentration, derived from the calibration standards, was 14.4%. Plasma erythropoietin sample concentrations were determined from calibration standards (0–100 mIU·mL−1) and control samples, using an applied four-parameter algorithm fit using a free internet-based ELISA analysis program (elisaanalysis.com). The intra-assay coefficient of variation for PEPO concentration, derived from the calibration standards, was 5.3%.
Data Analysis
Calculations and Derivations
All measurements are n = 10 unless stated otherwise. To describe the effect of the dehydration protocol (i.e., vs. normal hydration/euhydration), an unstressed baseline was estimated by averaging CON and HEAT baseline measurements, against which variables are compared over time where relevant (i.e., ΔBM, ΔBP, and ΔPV).
Area-weighted TSKIN was calculated according to International Organisation for Standardization (1992) from the following formula:
Changes in PV, associated with the exercise conditions, were calculated from changes in Hb and Hct according to the equation of Strauss et al. (1952):
where t and 0 subscripts denote measurements at time t and baseline (unstressed), respectively. Hb is in g·100 mL−1 and Hct is a fraction.
Statistics
Variables were individually analyzed longitudinally using linear mixed effect model analysis. Where appropriate, models incorporated up to seven repeated measurements (i.e., average hydrated, baseline, immediately post-exercise, 2-, 3-, 5-, and 24-h post-exercise) across three separate conditions (CON, HEAT, HEAT+DEHY). The order of condition completion was incorporated as a fixed effect, while participant was modeled as a random effect. Homogeneity of variances was assessed visually via plotting of residuals vs. model-fitted values, and formally with Levene's test, across all combinations of factors in the model. Linearity and approximate normal distribution of residuals were assessed via visual inspection of histograms and Q-Q plots of model and individual residuals, and formally with Shapiro-Wilk test. Akaike' Information Criteria (AIC) was used to determine covariance structure of model errors (following significant Levene's test), random-effect structure, and incorporation of fixed effects. In the presence of non-significant (via AIC) condition:time interaction, and thus non-inclusion in the model, “NS” is reported. Multiple comparisons were made using the least squares means contracts derived from the mixed models, and adjustments for multiple testing made with the Hochberg-Bonferroni method. In line with the study aims and hypotheses being focused on delineating the addition of discrete stressors, only comparisons between conditions of interest (i.e., HEAT vs. CON, and HEAT+DEHY vs. HEAT) were examined.
Associations between mechanistically related variables were explored using standard least squares regressions. The relations between the variables are expressed as the Pearson's correlation coefficient (denoted as r) and corresponding p-values. The magnitude of correlation effect was qualitatively assessed from corresponding according to Hopkins et al. (2009); trivial (r < 0.1), small (0.1 < r < 0.3), moderate (0.3 < r < 0.5), large/strong (0.5 < r < 0.7), and very large/strong (0.7 < r < 0.9).
The mixed-model analysis was performed using R (R Development Core Team, 2008), and correlations were performed on raw values using GraphPad Prism (Prism Version 7.00, GraphPad Software, California, USA). Descriptive statistics in text are reported as raw means ± SD, whereas comparisons of interest are reported as least-squares means with corresponding 95% confidence limits [lower limit, upper limit]. A p < 0.05 was considered statistically significant a priori. To aid in interpretation, ANOVA-derived main effects and interactions are provided, and (where appropriate) only post-hoc p-values are presented in corresponding table or text.
Results
Physical activity, diet, and menstrual cycle logs were reviewed prior to starting each of the second and third exercise conditions, and hydration compliance was checked verbally at this time. Prior to the two euhydrated conditions (CON and HEAT), if participants rated their thirst to be other than “not thirsty” (i.e., 1 on a 9-point validated Thirst Scale; Riebe et al., 1997), they ingested additional water ad libitum. All participants completed the 90-min exercise protocol.
Hydration
Body Mass Changes (Δ BM)
When compared against an average hydrated body mass (72.2 ± 11.8 kg), the overnight fluid restriction in HEAT+DEHY resulted in 1.1 ± 0.8% BM reduction (71.3 ± 11.6 kg), with a further 1.8 ± 0.8% loss by the end of exercise, leading to a net loss of 2.9 ± 1.2% (70.0 ± 11.0 kg). Net mass losses were 0.5 ± 0.6% in CON (71.8 ± 11.8 kg), and 0.1 ± 0.6% in HEAT (71.9 ± 11.3 kg; n = 9; p = 0.527 vs. CON).
Urine Specific Gravity (USG)
Baseline USG was similar between HEAT (1.007 ± 0.005) and CON (1.008 ± 0.006, p = 0.800), but was 0.019 higher in HEAT+DEHY (1.026 ± 0.005) than in HEAT (95% CL: [0.014, 0.023]; p < 0.001). Across exercise, USG was unchanged in CON (p = 0.155) and HEAT (p = 0.289), but tended to increase in HEAT+DEHY (p = 0.075). As such, post-exercise USG was similar between HEAT (1.005 ± 0.005) and CON (1.005 ± 0.004; p = 0.962), but 0.024 higher in HEAT+DEHY (1.029 ± 0.004) than in HEAT ([0.019, 0.028]; p < 0.001).
Plasma Osmolality (POSM; Figure 2)
Baseline POSM was similar between HEAT and CON (p = 0.243), and HEAT and HEAT+DEHY (p = 0.111; Figure 2). Across exercise, POSM decreased by 4 and 5 mOsm·kg−1 in CON and HEAT, respectively, but increased by a further 4 mOsm·kg−1 in HEAT+DEHY (all p ≤ 0.014). As such, post-exercise POSM was lower in HEAT than in CON (mean difference: −4 mOsm·kg−1; [−6, −1]; p = 0.002), and higher in HEAT+DEHY than in HEAT (13 mOsm·kg−1; [10, 15]; p < 0.001). Across the first 2 h of recovery, POSM remained stable in CON (p = 0.249) and HEAT (p = 0.128), but decreased by 5 mOsm·kg−1 in HEAT+DEHY (p = 0.001), remaining 4 mOsm·kg−1 higher in HEAT+DEHY than in HEAT at 2 h ([0, 9]; p = 0.046).
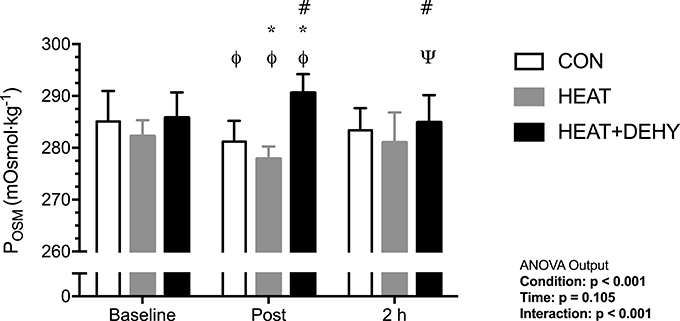
Figure 2. The additive effects of heat stress and hydration status on plasma osmolality (POSM) before, immediately after, and 2 h after 90-min exercise in CON (all n = 10), HEAT (n = 9, 9, and 8 resp.), and HEAT + DEHY (n = 9, 9, and 10 resp.). Statistical significance (ANOVA Output) is illustrated. Data presented are group mean ± SD. ΦDenotes significantly different (p < 0.05) to baseline. ΨDenotes significantly different to post-exercise measurement. *Denotes significantly different than CON. #Denotes significantly different to HEAT.
Thermal (Table 1)
Average core temperature (TCORE) was 0.4°C higher with the addition of heat stress, and an additional 0.2°C higher with dehydration (Table 1). Peak TCORE was 0.7°C higher with the addition of heat stress, and 0.3°C higher with dehydration (Table 1). Mean skin temperature (TSKIN) was 5.0°C warmer with the addition of heat stress, but not additionally higher with dehydration (Table 1). Peak TSKIN however was 5.4°C warmer with the addition of heat stress, and an additional 0.2°C warmer with dehydration (Table 1).
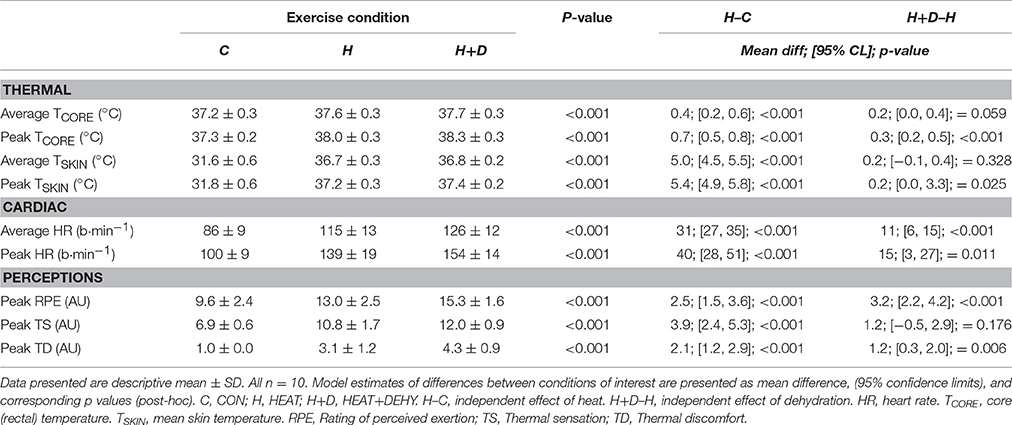
Table 1. The additive effects of heat stress and hydration status on the average and peak thermal, cardiac and perceptual strain during the 90-min exercise condition, with corresponding statistical significance (ANOVA Output) and comparisons of interest.
Heart Rate (Table 1)
Average HR was 37% higher with the addition of heat stress to exercise (i.e., CON vs. HEAT), and an additional 10% higher with dehydration (i.e., HEAT vs. HEAT+DEHY; Table 1). Similarly, peak HR was 41% higher with heat stress and 11% higher with dehydration (Table 1).
Perceptual Responses (Table 1)
Participants perceived exercise to be additively harder with the imposition of heat stress and dehydration (Table 1). They also reported higher ratings of thermal sensation with the addition of heat stress, but not additionally with dehydration (Table 1), and an additively higher thermal discomfort with heat stress and dehydration (Table 1).
Respiratory (Table 2)
Mean oxygen consumption (O2) and carbon dioxide production (CO2) were similar between conditions (Table 2), as were changes from baseline (all p ≥ 0.312; Table 2). Whereas, mean ventilation (n = 8) and its component factors (frequency and tidal volume) increased 51% more with the addition of heat stress, but not additionally with dehydration (Table 2). Mean end-tidal carbon dioxide pressure (PETCO2; Table 2) were therefore lower, by 9%, with the addition of heat stress, but not additionally with dehydration (Table 2). By end exercise, the reduction in PETCO2 was 74% larger with heat stress, but also not additionally with dehydration (Table 2).
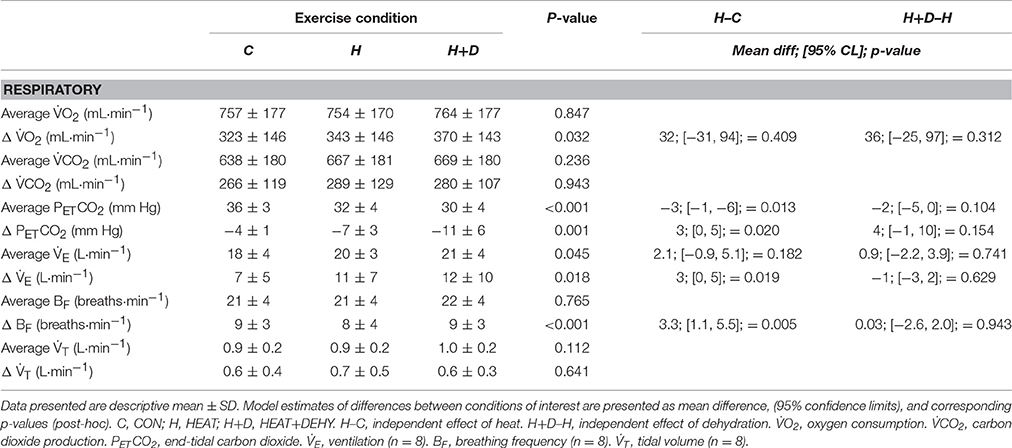
Table 2. The additive effects of heat stress and hydration status on the average and change from baseline in respiratory strain during the 90-min exercise condition, with corresponding statistical significance (ANOVA Output) and comparisons of interest.
Blood Pressure (BP; Table 3 and Figure 3)
Unstressed baseline sBP, dBP and MAP were 108 ± 6, 69 ± 7, and 82 ± 5 mm Hg, respectively. Diastolic blood pressure (dBP) did not change significantly, whereas systolic (sBP) and mean arterial pressure (MAP) showed reductions as described below (Table 3, Figure 3). Individual responses are provided in Supplementary Figure 2 for reference.
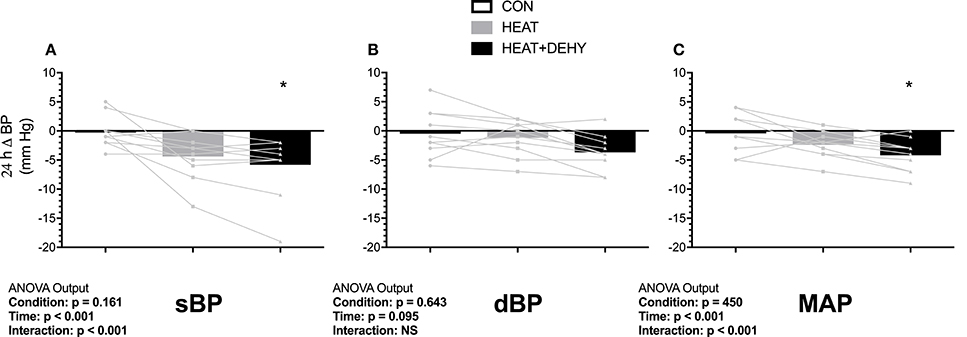
Figure 3. The additive effects of heat stress and hydration status on the 24-h change in systolic (sBP, A), diastolic (dBP, B) and mean arterial blood pressure (MAP, C) after the 90-min exercise condition. Statistical significance (ANOVA Output) is illustrated. Data presented are group mean with individual values overlaid. *Denotes significantly different (p < 0.05) than CON.

Table 3. The additive effects of heat stress and hydration status on arterial blood pressure before and after the 90-min exercise condition.
In response to exercise, sBP remained unchanged in CON (all p ≥ 0.990 vs. baseline), and in HEAT (p ≥ 0.345) except tending to be reduced at 24-h after exercise (by 5 mm Hg [0, 11]; p = 0.077). Whereas, sBP was elevated by 6 mm Hg at baseline in HEAT+DEHY ([0, 11]; p = 0.033 vs. unstressed baseline), but 7 mm Hg lower immediately post-exercise ([2, 13]; p = 0.002), then unaffected at 2–5 h (all p ≥ 0.494), and reduced again by 5 mm Hg at 24 h ([0, 11]; p = 0.049). The MAP was unchanged from baseline in CON and HEAT at all time points (p ≥ 0.452), but in HEAT+DEHY it progressed from being equivalent at baseline (p = 0.206), to a 5 mm Hg reduction at end exercise ([1, 9]; p = 0.005), equivalent from 2 to 5 h (p ≥ 0.828), and reduced again by 4 mm Hg at 24 h ([0, 8]; p = 0.020).
When comparing between stressors at a given time, the addition of heat affected neither sBP nor MAP at end exercise (p ≥ 0.753 vs. CON), whereas the addition of dehydration reduced both; sBP by 7 mm Hg ([2, 11]; p < 0.001 vs. HEAT) and MAP by 6 mm Hg ([3, 9]; p < 0.001). The BP were thereafter unaffected by the addition of either stressor in the first 5 h of recovery (all p ≥ 0.219), whereas by 24 h, sBP tended to be reduced following HEAT (by 4 mm Hg; [0, 8]; p = 0.061), comparably to HEAT+DEHY (p = 0.837 vs. HEAT; Figure 3). At 24 h, MAP was similar in HEAT and CON (p = 0.218), and in HEAT+DEHY (p = 0.398 vs. HEAT).
Association between Stress-induced Strain and Post-exercise Hypotension (PEH; Figure 4)
The association between stress-induced strain and 24-h change in MAP were explored to examine whether the addition of individual stressors (i.e., exercise, heat, and dehydration) provided a further stimulus for adaptation, by virtue of each inducing greater strain. When data from the three conditions were pooled for correlational analysis, the strain during exercise was strongly associated with the magnitude of PEH at 24 h (Figure 4).
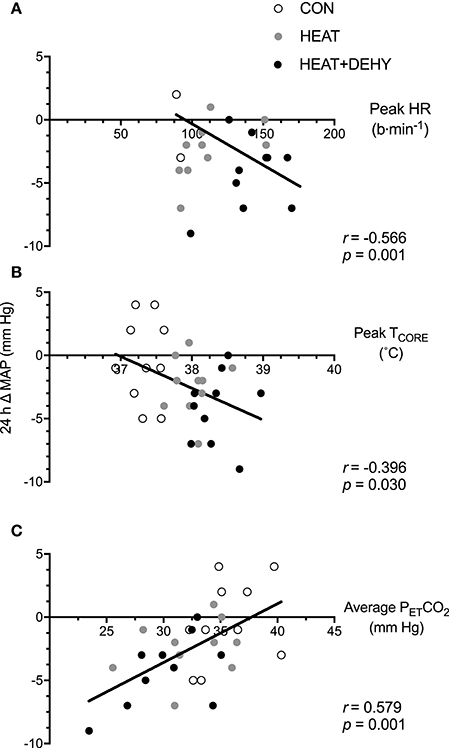
Figure 4. The relation between different forms of stress-induced strain and the post-exercise hypotensive (PEH) response at 24 h. Illustrated are the contributions of the cardiovascular strain (A; peak HR in exercise), thermoregulatory strain (B; peak TCORE in exercise), and an indicator of respiratory strain (C; average end-tidal carbon dioxide (PETCO2) in exercise) to the 24-h change in MAP.
Plasma Volume (PV; Figure 5)
As per the analysis of BP, time effects were examined against an unstressed baseline (i.e., mean of both euhydrated conditions), whereas effects of heat and dehydration at a given time were examined by comparing their respective differences from this common baseline.
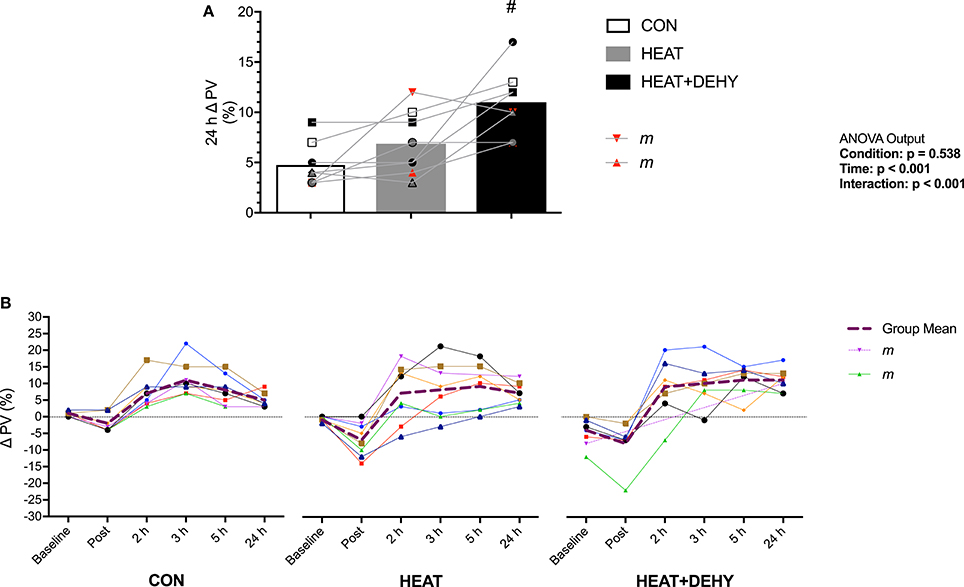
Figure 5. The additive effects of heat stress and hydration status on the change in plasma volume at 24 h after exercise (A; n = 8), and the pattern of response (B) vs. the average hydrated measure immediately after, 2, 3, 5, and 24 h after exercise in CON (all n = 8), HEAT (n = 8, 8, 8, 8, 7, and 8 resp.), and HEAT+DEHY (n = 8, 7, 7, 7, 7, and 8 resp.). Statistical significance (ANOVA Output) is illustrated. Data presented are group mean with individual values overlaid. Two male participants (“m”) are highlighted for reference. #Denotes significantly (p < 0.05) different to HEAT.
Plasma volume (PV) was reduced at baseline by 3% in HEAT+DEHY ([1, 6]; p = 0.016). Compared to baseline, PV was significantly reduced post-exercise in HEAT (p = 0.007), but not significantly in CON or DEHY (both p ≥ 0.401). This reduction was larger with heat ([0, 10]; p = 0.072), but not additionally with dehydration ([−1, 2]; p = 0.959). By 2 h after exercise PV recovered such that it was no different to baseline in CON (p = 0.114), but higher than baseline in HEAT (p = 0.049) and DEHY (p < 0.001). Plasma volume then remained elevated throughout recovery in all conditions (all p ≤ 0.017), and to a similar extent from 2 to 5 h after exercise (all p ≥ 0.420; Figure 5). However, at 24 h the PV expansion was similar between HEAT and CON ([−1, 5]; p = 0.335) but 4% larger in HEAT+DEHY ([1, 8]; p = 0.005 vs. HEAT).
Association between Stress-Induced Strain and Plasma Volume Expansion (PVE; Figure 6), and between PEH and PVE (Figure 7)
The association between indices of strain during exercise and expansion of PV by 24 h after exercise was explored to examine whether the addition of individual stressors (i.e., exercise, heat, and dehydration) provided a further stimulus for adaptation, by virtue of causing cumulative strain. Furthermore, the association between PEH and PVE (both at 24 h) was explored to examine potential mechanisms of exercise-induced hypervolemia (Figure 7). Cumulative strain was strongly associated with the magnitude of PVE at 24 h (Figure 6). Similarly, PVE at 24 h was strongly predictive of PEH at 24 h (Figure 7).
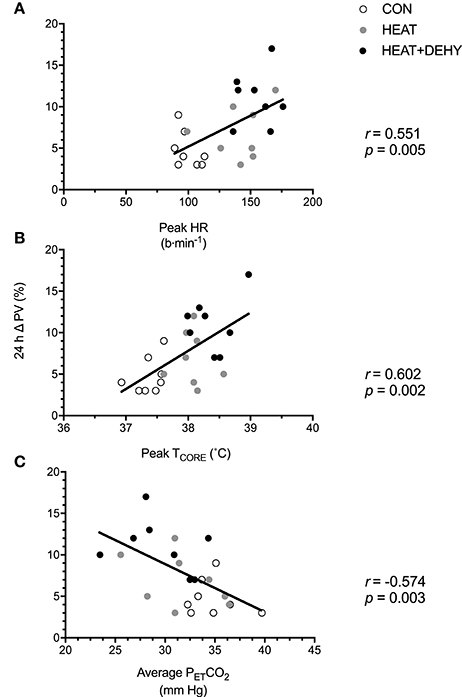
Figure 6. The relation between different forms of stress-induced strain and the post-exercise hypervolemic response (PVE; n = 8). Illustrated are the contributions of the cardiovascular strain (A; peak HR in exercise), thermoregulatory strain (B; peak TCORE in exercise), and an indicator of respiratory strain (C; average PETCO2 in exercise) to the 24-h change in PV.
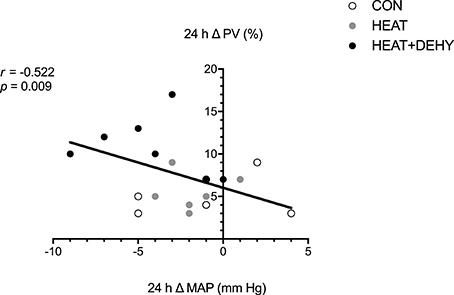
Figure 7. The relation between the post-exercise hypotensive response (24-h change in MAP), and the hypervolemic response (24-h change in PV; n = 8).
Hormones
Aldosterone (ALD; Figure 8A)
Aldosterone concentrations (n = 8) were similar between conditions at baseline (all p ≥ 0.145). Aldosterone was unchanged across exercise and at the measured (2 h) recovery point in CON (all p ≥ 0.472), whereas it increased by 41% after exercise in HEAT ([9, 73]; p = 0.010), and by 82% in HEAT+DEHY ([56, 108]; p < 0.001; Figure 8A). When comparing conditions, post-exercise ALD concentration tended to be 28% higher by virtue of heat stress ([2, 58]; p = 0.071), and 62% higher again by adding dehydration ([39, 86]; p < 0.001). These effects were no longer evident at 2 h of recovery (p ≥ 0.163 between conditions).
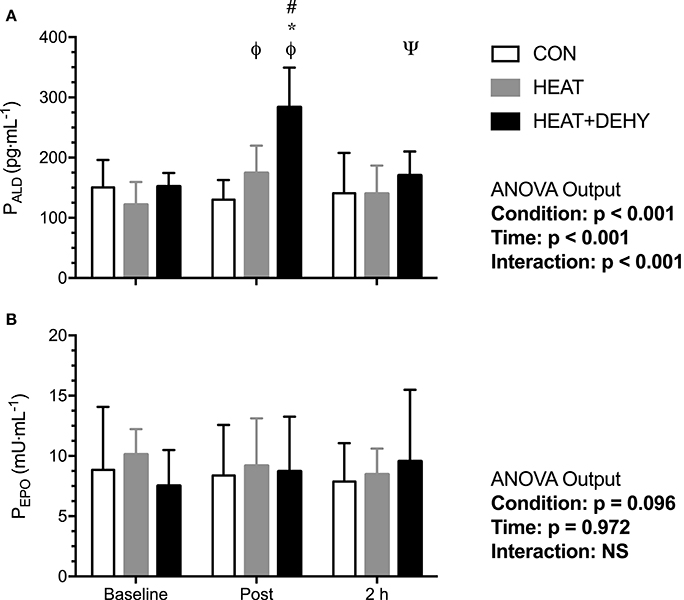
Figure 8. The effect of heat stress and hydration status on plasma aldosterone concentration (PALD, all n = 8; A) and erythropoietin concentration (PEPO; B) before, immediately after, and 2-h after the 90-min exercise in CON (all n = 8), HEAT (all n = 5), and HEAT+DEHY (all n = 6) condition. Statistical significance (ANOVA Output) is illustrated. Data presented are mean ± SD. ΦDenotes significantly different (p < 0.05) to baseline. ΨDenotes significantly different to immediately post-exercise measurement. *Denotes significantly different than CON. #Denotes significantly different to HEAT.
Erythropoietin (EPO; Figure 8B)
Erythropoietin concentrations did not change significantly with exercise in CON (n = 8), HEAT (n = 5), or DEHY (n = 6; Figure 8B).
Aldosterone-Mediated PVE (Figure 9)
The association between cardiovascular (Δ MAP; Figure 9A) and thermal strain (peak TCORE; Figure 9B) and ALD concentration were examined to identify potential thresholds and mechanisms of the ALD response. In turn, the relation between the ALD response and PVE was examined to investigate potential mechanisms for exercise-induced hypervolemia (Figure 9C). Larger reductions in MAP and larger rises in TCORE across exercise were strongly associated with post-exercise ALD concentrations, which was strongly predictive of PVE at 24 h.
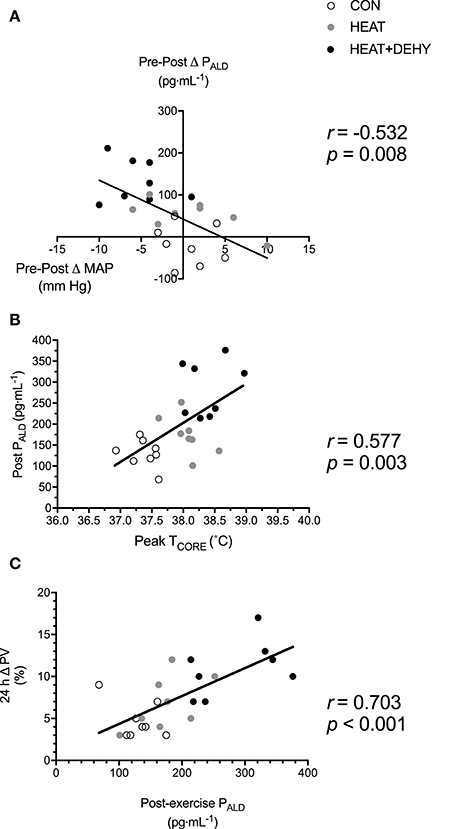
Figure 9. Potential mechanisms of increased aldosterone concentration and its role in mediating the post-exercise hypervolemic response. Illustrated is the relation between the change in MAP immediately after exercise (A), and peak TCORE in exercise (B), and PALD immediately after exercise (n = 8); and the relation between post-exercise PALD concentration and the 24-h change in PV (C).
Discussion
This is the first study, to our knowledge, to investigate the additive roles of low-intensity, orthostatically-stressful exercise, heat, and dehydration on early cardiovascular outcomes. The main novel findings were that: (i) the addition of these separate stressors combine to augment short-term (24 h) cardiovascular and hematological outcomes, particularly PEH and PVE; (ii) stress-induced strain, particularly the magnitude of TCORE elevation, closely predicts these outcomes; and (iii) within the first 24 h, PEH at least partly facilitates PVE at 24 h. Acute outcomes, such as PEH and PVE are important in their own right, but must also be considered in the context as a precursor for short- and long-term cardiovascular and hematological adaptations. Collectively, these findings indicate that manipulating the strain elicited during an exercise/conditioning bout could facilitate short-term (24 h) outcomes that may aid in exercise performance or cardiovascular health.
Post-exercise Hypotension
Low-intensity exercise provides a widely accessible means of meeting physical activity guidelines, but falls below the threshold required to meaningfully impact some important cardiovascular health parameters. In the current study, low-intensity exercise alone did not elicit a significant drop in BP at 24 h (Figure 3). This supports findings of Forjaz et al. (2004), who found no reduction in MAP 22 h after normotensive individuals cycled for 45 min at 30% O2 MAX, but significant reductions in BP after 50 and 75% 2 MAX. Similarly, Wallace et al. (1999) demonstrated no PEH 22 h after intermittent walking (50% O2 MAX) in normotensive individuals. Such data indicate that low-intensity exercise, particularly in normotensive young individuals, may be insufficient to elicit acute BP responses. Acute resistance exercise may also be insufficient in this respect (O'Connor et al., 1993), or more pronounced with large muscle mass exercise and those with hypertension (Casonatto et al., 2016). Even with resistance exercise (60% 1RM) eliciting sufficient stimulus for acute PEH, this may not eventuate over a longer period (i.e., 12 week; Moraes et al., 2012). Thus, higher intensity exercise, or a conditioning stimulus that provides a larger acute perturbation may be required to acquire the long-term (chronic) anti-hypertensive effects of exercise (Quinn, 2000; Thompson et al., 2001; Liu et al., 2012).
Adding heat stress to low-intensity exercise did not consistently affect BP. Elevated core and skin temperatures associated with exogenous heat stress (Table 1) were expected to elicit a greater degree of exercise-induced sympathetic activity (Ray and Gracey, 1997), and subsequent post-exercise residual systemic vasodilation, and thus greater baroreflex resetting and associated sympatho-inhibition (Halliwill et al., 2013). The combined stressors could conceivably also have larger transient effect on oxidative stress and the ensuing balance of oxidative stimuli and anti-oxidative capacity. We hypothesized that this would ultimately manifest in larger PEH at 24 h. Whilst systolic pressure tended to be reduced at 24 h after exercise in the heat (p = 0.077), diastolic and mean arterial pressures were not (Figure 3). Post-exercise hypotension is a complex and multifactorial response involving both peripheral and central mechanisms (Halliwill et al., 2013; Graham et al., 2016). Halliwill et al. (2013) suggest that the situational occurrence of hyperthermia may modulate the hypotensive response, however the additional thermal strain incurred in the present study did not have measurable effect. Elevated TCORE in recovery may (Kenny and Niedre, 2002) or may not (Jones et al., 2007) affect immediate PEH (≤30 min after exercise), but this is likely modulated by the preceding exercise intensity (Kenny and Niedre, 2002; Kenny et al., 2006; Jones et al., 2007). Due to the self-determined intensity of the calisthenics, participants may have attenuated the intensity of contractions in the face of a higher cardiovascular and thermal strain, and thus provided insufficient additional stimulus for PEH. While self-pacing is ecologically valid, it may be instructive to use matched paced exercise to provide a more complete mechanistic understanding.
Blood pressure was significantly reduced at 24 h only when modest (3% BM; POSM ~290 mOsmol·kg−1) dehydration was additively imposed on heat stress and low-intensity exercise (Figure 3). Furthermore, every participant demonstrated a reduction in MAP at 24 h when exposed to this combination of stressors (Figure 3), and to a meaningful extent, of 4 mm Hg (Chobanian et al., 2003). Acute dehydration augments physiological strain in multiple systems (Montain and Coyle, 1992b; Sawka et al., 1992; Gonzalez-Alonso, 1998; Gonzalez-Alonso et al., 1999), particularly via exacerbated tissue temperatures and cardiovascular strain in exercise (Montain and Coyle, 1992b; Sawka et al., 1992). This exacerbated strain facilitated PEH in the current study, presumably due at least in part to the thermal and cardiovascular strain elicited in response to this combination of stressors (Figure 4). The strain profile may therefore be as important as the work done, in determining the acute hypotensive response to exercise (Jones et al., 2007). If so, varied methods of modulating the strain induced by exercise (e.g., transient dehydration, ischemia, gravitational) may provide feasible means of eliciting (specific) short- and longer-term adaptations when not accessible via traditional and well-justified means (esp. moderate- and higher-intensity exercise).
Unfortunately, hydration status is sparsely reported in studies investigating PEH (Endo et al., 2012), likely due its complex association with muscular activity, heat production, and thermoregulatory responses. Fluid intake has been shown to abolish PEH in healthy sedentary males in a temperate environment (Endo et al., 2012), and in trained males in a hot (42°C and 20% RH) environment (Gagnon et al., 2012). Both these aforementioned studies demonstrated PEH in the 60–90 min after moderate exercise (1 h at 60% HRR, and 2 h at 120W, respectively) and modest dehydration (~1 and 2.5%, respectively). Further, the magnitude of PEH in early recovery was comparable to that of the current study (−5 mm Hg immediately after exercise; Table 3), but those studies did not report BP for the following day. Alterations in cardiac baroreflex sensitivity may partly explain the observed early-phase PEH in all three studies, as prevention of dehydration (by saline infusion or drinking) during exercise minimizes PEH and abolishes reductions to cardiac baroreflex sensitivity (Charkoudian et al., 2003). Furthermore, when coupled with higher tissue temperatures (Table 1 and Figure 4), and the subsequent cutaneous vasodilation, dehydration may facilitate larger PEH via both inhibition of central vasoconstrictor output and sustained post-exercise vasodilation of active muscle beds (i.e., via central and peripheral mechanisms; Halliwill et al., 2013).
Hypervolemia
Plasma volume was elevated by 5% at 24 h with calisthenics alone (Figure 5). This magnitude of PVE was unexpected considering its similarity to that obtained from higher-intensity exercise models (Gillen et al., 1994; Yang et al., 1998; Nagashima et al., 1999, 2000), and the fact that these participants were regularly physically active. The dynamic and orthostatically stressful nature of this exercise may therefore partly explain the observed PVE. Rapid PV reductions during exercise are attributed partly to increased capillary hydrostatic pressure and muscle tonicity (Lundvall et al., 1972), muscular activity per se (Sjogaard and Saltin, 1982), and a more upright posture (Nagashima et al., 2000). Thus, sustained isometric contractions, adjustments in posture, and dynamic movement sequences using most muscle groups in the current exercise protocol may facilitate a modest PVE despite its relatively low intensity. Plasma volume changes in the current study must also be considered in the context of potential limitations. First, the 10-min rest period prior to capillary sampling was insufficient to allow full stabilization, but was consistent for each individual between conditions, thus no systematic bias is expected. Second, we cannot discount possible confounding by incomplete washout between our conditions (Gillen et al., 1991), but any such effects would also lead to under- rather than over-estimation of hypervolemic effects of exercise or the additional stressors.
Fluid-regulatory hormonal responses to exercise are important mediators of PVE following exercise and heat exposure, and are influenced by exercise intensity and posture (Francesconi et al., 1983a; Ray et al., 1990; Gillen et al., 1991). The 5% increase in PV in CON was not accompanied by an increase in aldosterone (ALD; Figure 8A). Elevated ALD following exercise is involved in renal reabsorption of sodium and coupled reabsorption of water and subsequent PVE (Wade et al., 1985). Longer duration submaximal exercise elicits larger increases in ALD than does maximal exercise (Costill et al., 1976; Wade et al., 1987) but may have a threshold dependence (~60% O2MAX; Tanaka et al., 1986). The current study is limited in its assessment of aldosterone-mediated effects on 24-h PVE given the lack of plasma osmolality (POSM) measurements at this time point. Subsequent research should further determine the mechanisms of hypervolemia in response to different strain profiles utilizing a comprehensive assessment of body fluid regulation. Thus, the PVE in CON may therefore have been mediated by mechanisms other than those measured in the current study, including increased content of albumin due to increased lymphatic return, synthesis and decreased transcapillary escape (Gillen et al., 1991; Haskell et al., 1997; Yang et al., 1998).
Heat stress did not additively contribute to PVE (at 24 h) following low-intensity exercise (Figure 5A), despite increasing PV loss during exercise (Figure 5B). Exercise intensity (Nadel et al., 1979) and environmental temperature (Nadel et al., 1979; Maw et al., 1998) affect the PV loss during exercise, such that PV losses are larger in higher ambient temperatures regardless of hydration state (Kenefick et al., 2014). A more pronounced rise in TCORE (Table 1), and concomitant cutaneous vasodilation was expected to elevate capillary hydrostatic pressure, promoting net filtration and therefore augment the PV losses (Starling, 1896; Saltin, 1964; Senay, 1975). However, this larger loss during exercise was not compensated for by a larger rebound hypervolemia. Environmental conditions are also known to affect ALD secretion (Davies et al., 1981), with the increase in TCORE modulating this effect (Moller et al., 1989; Auernhammer and Strasburger, 1995; Wright et al., 2010). Despite a ~40% increase in ALD concentration in HEAT, this did not additively effect PVE to measurable extent; possibly due to the modest thermal strain (~38°C) coupled with the low intensity of exercise.
The combination of exercise, heat stress and dehydration resulted in the largest PVE (Figure 5). Dehydration can impair endurance exercise performance, due in part to increased tissue temperatures and cardiovascular strain (Sawka et al., 1992, 2011; Montain and Coyle, 1992a). These factors are also critically involved in acute hypervolemia (see Figure 2 in Akerman et al., 2016), however the obligatory mechanisms (if any) are largely unclear. Skin temperature was equivalently high in HEAT and HEAT+DEHY (Table 1), thereby rendering it as being unlikely in the mediation of PVE. These findings support those of Armstrong et al. (1997) and Gonzalez-Alonso et al. (1997) and indicate that hyperthermia and dehydration equally but not additively affect TSKIN in these conditions. These findings thereby also support the case for TCORE as a primary and more important modulator of PVE (Maw et al., 2000).
Fluid regulatory hormones are sensitive to changes in body water content and very likely contributed to the observed PVE in HEAT+DEHY. Hypohydration elicits larger increases in plasma renin activity (PRA) and ALD during light exercise in hot environments (Francesconi et al., 1983b; Maresh et al., 2004), whereas such exercise in a temperate environment is insufficient to stimulate these hormones (Finberg et al., 1974; Francesconi et al., 1983b). Furthermore, Francesconi et al. (1983b) also demonstrate an additive effect of heat stress on low-intensity exercise, and more-so with dehydration. The additive effect of dehydration in the current study was also a similar magnitude as Francesconi et al. (1983b), with dehydration approximately doubling the effect of heat alone on ALD concentration. Finberg et al. (1974) also found an association between PRA and cardiovascular strain in light exercise, which were attenuated when euhydration was maintained. Unlike Finberg et al. (1974), the increase in ALD in the current study was associated with the degree of thermal as well as cardiovascular strain experienced (Figure 9). It therefore appears that whilst ALD concentration, and its mediating effect on PVE (Figure 9; Nagashima et al., 1999) may be related most closely with thermal strain (i.e., TCORE), other mechanisms of PVE may also contribute.
Heat stress and physical activity act additively to vasoconstrict the splanchnic and renal beds (Rowell et al., 1968). The combination of these stressors may therefore collective facilitate the erythropoietic response to exercise (Sawka et al., 2000), but whether this occurs acutely has yet to be determined. We therefore hypothesized that the three stressors examined here would attenuate splanchnic and renal blood flow and might thus promote an erythropoietic response. However, plasma erythropoietin (EPO) was unchanged in all conditions (Figure 8B). The stimulus may therefore have been insufficient to reduce renal PO2, the primary stimulus for EPO release. Plasma volume expansion without an accompanying erythropoeitic stimulation may be beneficial in untrained people, but its functional relevance in highly endurance-trained people is less clear (Hopper et al., 1988). Whereas, PVE in conjunction with red cell volume (RCV) expansion is functionally relevant in trained individuals (Heinicke et al., 2001; Lundby et al., 2016). It is therefore valuable to know what exercise-related stimuli would expand RCV. Elevated EPO concentrations are observed in response to reductions in central venous pressure (CVP; Gunga et al., 1996a), thermal stimuli (Gunga et al., 1996b), and hypovolemia (Szygula et al., 1995), independent of hypoxia (Kirsch et al., 2005). Yet, EPO was not elevated by any of the stimuli used in this study, so their erythropoietic potential remains unclear.
PEH and PVE
The causal relation between PEH and subsequent PVE is complex and not fully understood. In the current study, the two phenomena were strongly associated, but also showed some independence, as has been observed previously (Holtzhausen and Noakes, 1995; Graham et al., 2016). For instance, PVE was consistently demonstrated in CON and HEAT despite no significant change in MAP. Whilst CVP unloading, and thus some aspect of post-exercise BP reduction must arise as a stimulus for PVE (Nagashima et al., 1999; Hayes et al., 2000), evidently PVE can also occur via other mechanisms.
Arterial pressure is generated by cardiac output and systemic vascular resistance. For PVE to occur with a simultaneous reduction in arterial BP, then presumably arterial or arteriolar resistance must decrease, even if the PVE is accommodated mostly within less resistive venous vessels. With the combination of all three stressors, a rise in cardiac output and TSKIN would facilitate higher skin blood flow and volume, and concomitant reduction in CVP. Atrial natriuretic peptide (ANP) release would be suppressed in these circumstances, thereby enhancing renin-angiotensin-aldosterone system (RAAS) activity. Considering that ALD is slow to act (Collins, 1966; Morel and Doucet, 1986; Nagashima et al., 2001), ANP suppression may also contribute to the rise in PV. Following a rise in PV, any ANP-mediated anti-diuresis may be inefficient in the face of a maximally stimulated RAAS (Altenkirch et al., 1990). However, the vasodilatory effects of ANP (Faber et al., 1988) may conceivably contribute to the occurrence of PEH, thus promoting a reduction in arterial pressure without an efficient anti-diuretic response. Histamine-mediated post-exercise vasodilation (Lockwood et al., 2005; McCord et al., 2006; Barrett-O'Keefe et al., 2013) and alterations in baroreflex control (Halliwill et al., 1996) appear to primarily facilitate PEH independent of changes in BV (Gillen et al., 1994). Post-exercise hypotension is therefore a complex phenomenon involving peripheral and central mechanisms, and its interaction with vascular volumes in still incompletely understood. Hypotheses have postulated to explain apparent links between arterial pressure, volume expansion and sympatho-vagal balance (e.g., Yun et al., 2005) but they are still tentative and warrant further examination.
Perspectives and Summary
The results of the current study indicate the importance and cumulative role of discrete stressors (low-intensity exercise, heat, dehydration) in stimulating acute cardiovascular outcomes. Of note, PEH at 24 h after exercise was affected primarily by the addition of dehydration, with the largest response (and all participants responding) when all stressors were combined, and the largest strain produced. Furthermore, PVE at 24 h after exercise was also largest when exposed to all stressors. The exacerbated strain, particularly thermal, facilitated an increase in ALD, which mediated the rise in PV and was associated with the magnitude of PEH. The current study provided associations (i.e., non-causal) between additive stressors and outcome measures. Future research should investigate dose-response relations between mediating factors of acute PEH and PVE, particularly the occurrence of incremental hyperthermia and these outcomes. The same acute outcomes may also be possible without the imposition of dehydration, but instead increasing TCORE by other means (e.g., more intense exercise or thermal stress). Whilst this is a reasonable assumption, not only does dehydration exacerbate the thermal strain of exercise in the heat (with no/low airflow at least), its independent effects may also provide a conditioning stimulus as detailed above. However, the role of dehydration in the context of acute conditioning is rarely considered. Future research should aim to identify the means by which dehydration may aid (or hinder) adaptation to exercise, rather than its well-established effects on performance acutely. In particular, the additive roles of individual stressors should be examined in a more extensive assessment; including (i) other known cardiovascular (CVP, SV, , TPR) and fluid regulatory (AVP, ANP, ALB) modulators of PEH and PVE, and (ii) the recovery kinetics of body fluid dynamics (across minutes to days), as these are seldom considered (Luttrell and Halliwill, 2015).
Acute periods of high strain, or the resultant outcomes may also be unwarranted in particular individuals. Acute PEH, whilst beneficial to most individuals, would be unwarranted in individuals with hypotension or postural orthostatic tachycardia syndrome (POTS). Similarly, dehydration is unlikely to be recommended for clinical populations, as recurrent dehydration (Clark et al., 2016), or chronic stimulation of fluid- and sodium-conserving hormones may contribute to disease progression (Thornton, 2010). However, in those undergoing conditioning periods (e.g., exercise training), the exacerbated strain may (Garrett et al., 2014) or may not (Neal et al., 2016) elicit larger changes in short-term cardiovascular and hematological adaptive processes. Equally, high levels of hypohydration may also interfere with muscle metabolic control, protein synthesis and hypothalamic adaptations to heat acclimation (Schliess et al., 2006; Schwimmer et al., 2006). Thus, whilst periods of sustained hypohydration are likely unwarranted in certain clinical populations, it is currently unclear whether transient mild dehydration (and the strain therein) plays a substantial role in the process of exercise-associated adaptation (Akerman et al., 2016).
In conclusion, acute cardiovascular and hematological outcomes following exercise therefore appear to be (at least partly) determined by the magnitude of strain induced in the conditioning stimulus. The addition of multiple independent stressors to a low-intensity exercise may exacerbate the strain, and thus the potential adaptive stimulus. This added strain might be useful when exercise performance per se is not the primary outcome; in those unable to complete traditional exercise modes (e.g., elderly or injured); are anticipating a novel acute stress (e.g., before a heat wave or surgery); or avoiding intense exercise (e.g., tapering phase before competition for athletes). Future research should investigate whether this complementary response is evident in other modes and intensities of exercise, and what facilitates such adaptive responses.
Author Contributions
AA, SL, and JC contributed to the conception and design of the study, acquisition, analysis and interpretation of the data, drafted, revised and approved the final version of the manuscript. RK contributed to analyzing the data (ELISA analysis) and reviewed and approved the final version of the manuscript.
Funding
The study received funding support from the School of Physical Education, Sport and Exercise Sciences and the Department of Physiology, University of Otago.
Conflict of Interest Statement
The authors declare that the research was conducted in the absence of any commercial or financial relationships that could be construed as a potential conflict of interest.
Acknowledgments
The authors would like to express thanks to all participants for their time and commitment to the experiment, and invaluable support from the technical team at the School of Physical Education, Sport and Exercise Sciences (Nigel, Lisa, Di). We would also like to thanks Miss Susie Ferkins for her support in completion of a pilot study and Ms Margie Lazar for her help in developing the calisthenics protocol.
Supplementary Material
The Supplementary Material for this article can be found online at: https://www.frontiersin.org/articles/10.3389/fphys.2017.00756/full#supplementary-material
Supplementary Figure 1. Example progression (from left to right, starting top left corner) of exercises during the 70-min callisthenics period of the exercise protocol.
Supplementary Figure 2. The effect of heat stress and hydration status on the systolic (sBP; A), diastolic (dBP, B) and mean arterial blood pressure (MAP, C) response to the 90-min exercise condition, and in recovery. Data presented are group mean with individual values overlaid. m denotes male participant. For statistical significance please refer to Figure 5 in the Manuscript.
References
Ahlgrim, C., Pottgiesser, T., Robinson, N., Sottas, P. E., Ruecker, G., and Schumacher, Y. O. (2010). Are 10 min of seating enough to guarantee stable haemoglobin and haematocrit readings for the athlete's biological passport? Int. J. Lab. Hematol. 32, 506–511. doi: 10.1111/j.1751-553X.2009.01213.x
Akerman, A. P., Tipton, M., Minson, C. T., and Cotter, J. D. (2016). Heat stress and dehydration in adapting for performance: good, bad, both, or neither? Temperature 3, 412–436. doi: 10.1080/23328940.2016.1216255
Altenkirch, H. U., Gerzer, R., Kirsch, K. A., Weil, J., Heyduck, B., Schultes, I., et al. (1990). Effect of prolonged physical exercise on fluid regulating hormones. Eur. J. Appl. Physiol. 61, 209–213. doi: 10.1007/BF00357601
Armstrong, L. E., Maresh, C. M., Gabaree, C. V., Hoffman, J. R., Kavouras, S. A., Kenefick, R. W., et al. (1997). Thermal and circulatory responses during exercise: effects of hypohydration, dehydration, and water intake. J. Appl. Physiol. 82, 2028–2035.
Auernhammer, C. J., and Strasburger, C. J. (1995). Effects of growth hormone and insulin-like growth factor I on the immune system. Eur. J. Endocrinol. 133, 635–645. doi: 10.1530/eje.0.1330635
Barrett-O'Keefe, Z., Kaplon, R. E., and Halliwill, J. R. (2013). Sustained post-exercise vasodilatation and histamine receptor activation following small muscle-mass exercise in humans. Exp. Physiol. 98, 268–277. doi: 10.1113/expphysiol.2012.066605
Beetham, W. P. Jr., and Buskirk, E. R. (1958). Effects of dehydration, physical conditioning and heat acclimatization on the response to passive tilting. J. Appl. Physiol. 13, 465–468.
Binder, K., Gagnon, D., Lynn, A. G., Kondo, N., and Kenny, G. P. (2012). Heat stress attenuates the increase in arterial blood pressure during isometric handgrip exercise. Eur. J. Appl. Physiol. 113, 183–190. doi: 10.1007/s00421-012-2428-3
Borg, G. A. (1982). Psychophysical bases of perceived exertion. Med. Sci. Sports Exerc. 14, 377–381. doi: 10.1249/00005768-198205000-00012
Brandenberger, G., Candas, V., Follenius, M., Libert, J. P., and Kahn, J. M. (1986). Vascular fluid shifts and endocrine responses to exercise in the heat. effect of rehydration. Eur. J. Appl. Physiol. Occup. Physiol. 55, 123–129. doi: 10.1007/BF00714993
Casonatto, J., Goessler, K. F., Cornelissen, V. A., Cardoso, J. R., and Polito, M. D. (2016). The blood pressure-lowering effect of a single bout of resistance exercise: a systematic review and meta-analysis of randomised controlled trials. Eur. J. Prev. Cardiol. 23, 1700–1714. doi: 10.1177/2047487316664147
Charkoudian, N., Halliwill, J. R., Morgan, B. J., Eisenach, J. H., and Joyner, M. J. (2003). Influences of hydration on post-exercise cardiovascular control in humans. J. Physiol. 552(Pt 2), 635–644. doi: 10.1113/jphysiol.2003.048629
Chobanian, A. V., Bakris, G. L., Black, H. R., Cushman, W. C., Green, L. A., Izzo, J. L. Jr., et al. (2003). The seventh report of the joint national committee on prevention, detection, evaluation, and treatment of high blood pressure: the JNC 7 report. JAMA 289, 2560–2572. doi: 10.1001/jama.289.19.2560
Clark, W. F., Sontrop, J. M., Huang, S. H., Moist, L., Bouby, N., and Bankir, L. (2016). Hydration and chronic kidney disease progression: a critical review of the evidence. Am. J. Nephrol. 43, 281–292. doi: 10.1159/000445959
Collins, K. J. (1966). The action of exogenous aldosterone on the secretion and composition of drug-induced sweat. Clin. Sci. 30, 207–221.
Convertino, V. A., Keil, L. C., Bernauer, E. M., and Greenleaf, J. E. (1981). Plasma volume, osmolality, vasopressin, and renin activity during graded exercise in man. J. Appl. Physiol. Respir. Environ. Exerc. Physiol. 50, 123–128.
Costill, D. L., Branam, G., Fink, W., and Nelson, R. (1976). Exercise induced sodium conservation: changes in plasma renin and aldosterone. Med. Sci. Sports 8, 209–213. doi: 10.1249/00005768-197600840-00001
Davies, J. A., Harrison, M. H., Cochrane, L. A., Edwards, R. J., and Gibson, T. M. (1981). Effect of saline loading during heat acclimatization on adrenocortical hormone levels. J. Appl. Physiol. Respir. Environ. Exerc. Physiol. 50, 605–612.
De Feo, P., Horber, F. F., and Haymond, M. W. (1992). Meal stimulation of albumin synthesis: a significant contributor to whole body protein synthesis in humans. Am. J. Physiol. 263 (4 Pt 1), E794–E799.
Eicher, J. D., Maresh, C. M., Tsongalis, G. J., Thompson, P. D., and Pescatello, L. S. (2010). The additive blood pressure lowering effects of exercise intensity on post-exercise hypotension. Am. Heart J. 160, 513–520. doi: 10.1016/j.ahj.2010.06.005
Endo, M. Y., Kajimoto, C., Yamada, M., Miura, A., Hayashi, N., Koga, S., et al. (2012). Acute effect of oral water intake during exercise on post-exercise hypotension. Eur. J. Clin. Nutr. 66, 1208–1213. doi: 10.1038/ejcn.2012.139
Faber, J. E., Gettes, D. R., and Gianturco, D. P. (1988). Microvascular effects of atrial natriuretic factor: interaction with alpha-1 and alpha-2 adrenoreceptors. Circ. Res. 63, 415–428. doi: 10.1161/01.RES.63.2.415
Farinatti, P. T., Soares, P. P., Monteiro, W. D., Duarte, A. F., and Castro, L. A. (2011). Cardiovascular responses to passive static flexibility exercises are influenced by the stretched muscle mass and the Valsalva maneuver. Clinics 66, 459–464. doi: 10.1590/S1807-59322011000300017
Finberg, J. P., Katz, M., Gazit, H., and Berlyne, G. M. (1974). Plasma renin activity after acute heat exposure in nonacclimatized and naturally acclimatized man. J. Appl. Physiol. 36, 519–523.
Forjaz, C. L., Cardoso, C. G. Jr., Rezk, C. C., Santaella, D. F., and Tinucci, T. (2004). Post-exercise hypotension and hemodynamics: the role of exercise intensity. J. Sports Med. Phys. Fitness 44, 54–62.
Francesconi, R. P., Sawka, M. N., Hubbard, R. W., and Mager, M. (1983a). Acute albumin-induced plasma volume expansion and exercise in the heat: effects on hormonal responses in men. Eur. J. Appl. Physiol. Occup. Physiol. 51, 121–128. doi: 10.1007/BF00952544
Francesconi, R. P., Sawka, M. N., and Pandolf, K. B. (1983b). Hypohydration and heat acclimation: plasma renin and aldosterone during exercise. J. Appl. Physiol. 55, 1790–1794.
Gagge, A. P., Stolwijk, J. A., and Hardy, J. D. (1967). Comfort and thermal sensations and associated physiological responses at various ambient temperatures. Environ. Res. 1, 1–20. doi: 10.1016/0013-9351(67)90002-3
Gagnon, D., Lynn, A. G., Binder, K., Boushel, R. C., and Kenny, G. P. (2012). Mean arterial pressure following prolonged exercise in the heat: influence of training status and fluid replacement. Scand. J. Med. Sci. Sports 22, e99–e107. doi: 10.1111/j.1600-0838.2012.01506.x
Garrett, A. T., Goosens, N. G., Rehrer, N. J., Patterson, M. J., Harrison, J., Sammut, I., et al. (2014). Short-term heat acclimation is effective and may be enhanced rather than impaired by dehydration. Am. J. Hum. Biol. 26, 311–320. doi: 10.1002/ajhb.22509
Gillen, C. M., Lee, R., Mack, G. W., Tomaselli, C. M., Nishiyasu, T., and Nadel, E. R. (1991). Plasma volume expansion in humans after a single intense exercise protocol. J. Appl. Physiol. 71, 1914–1920.
Gillen, C. M., Nishiyasu, T., Langhans, G., Weseman, C., Mack, G. W., and Nadel, E. R. (1994). Cardiovascular and renal function during exercise-induced blood volume expansion in men. J. Appl. Physiol. 76, 2602–2610.
Gonzalez-Alonso, J. (1998). Separate and combined influences of dehydration and hyperthermia on cardiovascular responses to exercise. Int. J. Sports Med. 19(Suppl. 2), S111–S114. doi: 10.1055/s-2007-971972
Gonzalez-Alonso, J., Mora-Rodriguez, R., Below, P. R., and Coyle, E. F. (1997). Dehydration markedly impairs cardiovascular function in hyperthermic endurance athletes during exercise. J. Appl. Physiol. 82, 1229–1236.
Gonzalez-Alonso, J., Mora-Rodriguez, R., and Coyle, E. F. (1999). Supine exercise restores arterial blood pressure and skin blood flow despite dehydration and hyperthermia. Am. J. Physiol. 277(2 Pt 2), H576–H583.
Graham, M. J., Lucas, S. J., Francois, M. E., Stavrianeas, S., Parr, E. B., Thomas, K. N., et al. (2016). Low-volume intense exercise elicits post-exercise hypotension and subsequent hypervolemia, irrespective of which limbs are exercised. Front. Physiol. 7:199. doi: 10.3389/fphys.2016.00199
Gunga, H. C., Kirsch, K., Baartz, F., Maillet, A., Gharib, C., Nalishiti, W., et al. (1996a). Erythropoietin under real and simulated microgravity conditions in humans. J. Appl. Physiol. 81, 761–773.
Gunga, H. C., Wittels, P., Gunther, T., Kanduth, B., Vormann, J., Rocker, L., et al. (1996b). Erythropoietin in 29 men during and after prolonged physical stress combined with food and fluid deprivation. Eur. J. Appl. Physiol. Occup. Physiol. 73, 11–16. doi: 10.1007/BF00262804
Halliwill, J. R., Buck, T. M., Lacewell, A. N., and Romero, S. A. (2013). Post-exercise hypotension and sustained post-exercise vasodilatation: what happens after we exercise? Exp. Physiol. 98, 7–18. doi: 10.1113/expphysiol.2011.058065
Halliwill, J. R., Taylor, J. A., and Eckberg, D. L. (1996). Impaired sympathetic vascular regulation in humans after acute dynamic exercise. J. Physiol. 495(Pt 1), 279–288. doi: 10.1113/jphysiol.1996.sp021592
Haskell, A., Nadel, E. R., Stachenfeld, N. S., Nagashima, K., and Mack, G. W. (1997). Transcapillary escape rate of albumin in humans during exercise-induced hypervolemia. J. Appl. Physiol. 83, 407–413.
Hayes, P. M., Lucas, J. C., and Shi, X. (2000). Importance of post-exercise hypotension in plasma volume restoration. Acta Physiol. Scand. 169, 115–124. doi: 10.1046/j.1365-201x.2000.00728.x
Heinicke, K., Wolfarth, B., Winchenbach, P., Biermann, B., Schmid, A., Huber, G., et al. (2001). Blood volume and hemoglobin mass in elite athletes of different disciplines. Int. J. Sports Med. 22, 504–512. doi: 10.1055/s-2001-17613
Holtzhausen, L. M., and Noakes, T. D. (1995). The prevalence and significance of post-exercise (postural) hypotension in ultramarathon runners. Med. Sci. Sports Exerc. 27, 1595–1601. doi: 10.1249/00005768-199512000-00003
Hopkins, W. G., Marshall, S. W., Batterham, A. M., and Hanin, J. (2009). Progressive statistics for studies in sports medicine and exercise science. Med. Sci. Sports Exerc. 41, 3–13. doi: 10.1249/MSS.0b013e31818cb278
Hopper, M. K., Coggan, A. R., and Coyle, E. F. (1988). Exercise stroke volume relative to plasma-volume expansion. J. Appl. Physiol. 64, 404–408.
International Organisation for Standardization (1992). Evaluation of Thermal Strain by Physiological Measurements (ISO 9886: 1992 [E]). Geneva: International Organisation for Standardization. Available online at: https://www.iso.org/standard/17767.html
Jones, H., George, K., Edwards, B., and Atkinson, G. (2007). Is the magnitude of acute post-exercise hypotension mediated by exercise intensity or total work done? Eur. J. Appl. Physiol. 102, 33–40. doi: 10.1007/s00421-007-0562-0
Kenefick, R. W., Sollanek, K. J., Charkoudian, N., and Sawka, M. N. (2014). Impact of skin temperature and hydration on plasma volume responses during exercise. J. Appl. Physiol. 117, 413–420. doi: 10.1152/japplphysiol.00415.2014
Kenny, G. P., Jay, O., Zaleski, W. M., Reardon, M. L., Sigal, R. J., Journeay, W. S., et al. (2006). Post-exercise hypotension causes a prolonged perturbation in esophageal and active muscle temperature recovery. Am. J. Physiol. Regul. Integr. Comp. Physiol. 291, R580–R588. doi: 10.1152/ajpregu.00918.2005
Kenny, G. P., and Niedre, P. C. (2002). The effect of exercise intensity on the post-exercise esophageal temperature response. Eur. J. Appl. Physiol. 86, 342–346. doi: 10.1007/s00421-001-0538-4
Kirsch, K. A., Schlemmer, M., De Santo, N. G., Cirillo, M., Perna, A., and Gunga, H. C. (2005). Erythropoietin as a volume-regulating hormone: an integrated view. Semin. Nephrol. 25, 388–391. doi: 10.1016/j.semnephrol.2005.05.007
Liu, S., Goodman, J., Nolan, R., Lacombe, S., and Thomas, S. G. (2012). Blood pressure responses to acute and chronic exercise are related in prehypertension. Med. Sci. Sports Exerc. 44, 1644–1652. doi: 10.1249/MSS.0b013e31825408fb
Lockwood, J. M., Wilkins, B. W., and Halliwill, J. R. (2005). H1 receptor-mediated vasodilatation contributes to post-exercise hypotension. J. Physiol. 563(Pt 2), 633–642. doi: 10.1113/jphysiol.2004.080325
Luetkemeier, M. J., Coles, M. G., and Askew, E. W. (1997). Dietary sodium and plasma volume levels with exercise. Sports Med. 23, 279–286. doi: 10.2165/00007256-199723050-00002
Lundby, C., Montero, D., and Joyner, M. (2016). Biology of O2max: looking under the physiology lamp. Acta Physiol. 20, 218–228. doi: 10.1111/apha.12827
Lundvall, J., Mellander, S., Westling, H., and White, T. (1972). Fluid transfer between blood and tissues during exercise. Acta Physiol. Scand. 85, 258–269. doi: 10.1111/j.1748-1716.1972.tb05259.x
Luttrell, M. J., and Halliwill, J. R. (2015). Recovery from exercise: vulnerable state, window of opportunity, or crystal ball? Front. Physiol. 6:204. doi: 10.3389/fphys.2015.00204
Maresh, C. M., Gabaree-Boulant, C. L., Armstrong, L. E., Judelson, D. A., Hoffman, J. R., Castellani, J. W., et al. (2004). Effect of hydration status on thirst, drinking, and related hormonal responses during low-intensity exercise in the heat. J. Appl. Physiol. 97, 39–44. doi: 10.1152/japplphysiol.00956.2003
Maw, G. J., Mackenzie, I. L., and Taylor, N. A. (1998). Human body-fluid distribution during exercise in hot, temperate and cool environments. Acta Physiol. Scand. 163, 297–304. doi: 10.1046/j.1365-201x.1998.00380.x
Maw, G. J., Mackenzie, I. L., and Taylor, N. A. (2000). Can skin temperature manipulation, with minimal core temperature change, influence plasma volume in resting humans? Eur. J. Appl. Physiol. 81, 159–162. doi: 10.1007/PL00013790
McCord, J. L., Beasley, J. M., and Halliwill, J. R. (2006). H2-receptor-mediated vasodilation contributes to post-exercise hypotension. J. Appl. Physiol. 100, 67–75. doi: 10.1152/japplphysiol.00959.2005
Melin, B., Jimenez, C., Savourey, G., Bittel, J., Cottet-Emard, J. M., Pequignot, J. M., et al. (1997). Effects of hydration state on hormonal and renal responses during moderate exercise in the heat. Eur. J. Appl. Physiol. Occup. Physiol. 76, 320–327. doi: 10.1007/s004210050255
Melin, B., Koulmann, N., Jimenez, C., Savourey, G., Launay, J. C., Cottet-Emard, J. M., et al. (2001). Comparison of passive heat or exercise-induced dehydration on renal water and electrolyte excretion: the hormonal involvement. Eur. J. Appl. Physiol. 85, 250–258. doi: 10.1007/s004210100448
Moller, N., Beckwith, R., Butler, P. C., Christensen, N. J., Orskov, H., and Alberti, K. G. (1989). Metabolic and hormonal responses to exogenous hyperthermia in man. Clin. Endocrinol. 30, 651–660. doi: 10.1111/j.1365-2265.1989.tb00271.x
Montain, S. J., and Coyle, E. F. (1992a). Fluid ingestion during exercise increases skin blood flow independent of increases in blood volume. J. Appl. Physiol. 73, 903–910.
Montain, S. J., and Coyle, E. F. (1992b). Influence of graded dehydration on hyperthermia and cardiovascular drift during exercise. J. Appl. Physiol. 73, 1340–1350.
Moraes, M. R., Bacurau, R. F., Simoes, H. G., Campbell, C. S., Pudo, M. A., Wasinski, F., et al. (2012). Effect of 12 weeks of resistance exercise on post-exercise hypotension in stage 1 hypertensive individuals. J. Hum. Hypertens. 26, 533–539. doi: 10.1038/jhh.2011.67
Morel, F., and Doucet, A. (1986). Hormonal control of kidney functions at the cell level. Physiol. Rev. 66, 377–468.
Nadel, E. R., Cafarelli, E., Roberts, M. F., and Wenger, C. B. (1979). Circulatory regulation during exercise in different ambient temperatures. J. Appl. Physiol. Respir. Environ. Exerc. Physiol. 46, 430–437.
Nadel, E. R., Fortney, S. M., and Wenger, C. B. (1980). Effect of hydration state of circulatory and thermal regulations. J. Appl. Physiol. 49, 715–721.
Nagashima, K., Cline, G. W., Mack, G. W., Shulman, G. I., and Nadel, E. R. (2000). Intense exercise stimulates albumin synthesis in the upright posture. J. Appl. Physiol. 88, 41–46. Available online at: http://jap.physiology.org/content/ 88/1/41
Nagashima, K., Mack, G. W., Haskell, A., Nishiyasu, T., and Nadel, E. R. (1999). Mechanism for the posture-specific plasma volume increase after a single intense exercise protocol. J. Appl. Physiol. 86, 867–873.
Nagashima, K., Wu, J., Kavouras, S. A., and Mack, G. W. (2001). Increased renal tubular sodium reabsorption during exercise-induced hypervolemia in humans. J. Appl. Physiol. 91, 1229–1236. Available online at: http://jap.physiology.org/content/91/3/1229
Neal, R. A., Massey, H. C., Tipton, M. J., Young, J. S., and Corbett, J. (2016). Effect of permissive dehydration on induction and decay of heat acclimation, and temperate exercise performance. Front. Physiol. 7:564. doi: 10.3389/fphys.2016.00564
O'Connor, P. J., Bryant, C. X., Veltri, J. P., and Gebhardt, S. M. (1993). State anxiety and ambulatory blood pressure following resistance exercise in females. Med. Sci. Sports Exerc. 25, 516–521.
Pescatello, L. S., Guidry, M. A., Blanchard, B. E., Kerr, A., Taylor, A. L., Johnson, A. N., et al. (2004). Exercise intensity alters post-exercise hypotension. J. Hypertens. 22, 1881–1888. doi: 10.1097/00004872-200410000-00009
Pescatello, L. S., MacDonald, H. V., Lamberti, L., and Johnson, B. T. (2015). Exercise for hypertension: a prescription update integrating existing recommendations with emerging research. Curr. Hypertens. Rep. 17, 87. doi: 10.1007/s11906-015-0600-y
Quinn, T. J. (2000). Twenty-four hour, ambulatory blood pressure responses following acute exercise: impact of exercise intensity. J. Hum. Hypertens. 14, 547–553. doi: 10.1038/sj.jhh.1001106
Ray, C. A., Delp, M. D., and Hartle, D. K. (1990). Interactive effect of body posture on exercise-induced atrial natriuretic peptide release. Am. J. Physiol. 258(5 Pt 1), E775–E779.
Ray, C. A., and Gracey, K. H. (1997). Augmentation of exercise-induced muscle sympathetic nerve activity during muscle heating. J. Appl. Physiol. 82, 1719–1725.
Ray, C. A., Rea, R. F., Clary, M. P., and Mark, A. L. (1993). Muscle sympathetic nerve responses to dynamic one-legged exercise: effect of body posture. Am. J. Physiol. 264(1 Pt 2), H1–H7.
Riebe, D., Maresh, C. M., Armstrong, L. E., Kenefick, R. W., Castellani, J. W., Echegaray, M. E., et al. (1997). Effects of oral and intravenous rehydration on ratings of perceived exertion and thirst. Med. Sci. Sports Exerc. 29, 117–124. doi: 10.1097/00005768-199701000-00017
Rowell, L. B., Brengelmann, G. L., Blackmon, J. R., Twiss, R. D., and Kusumi, F. (1968). Splanchnic blood flow and metabolism in heat-stressed man. J. Appl. Physiol. 24, 475–484.
Saltin, B. (1964). Circulatory response to submaximal and maximal exercise after thermal dehydration. J. Appl. Physiol. 19, 1125–1132.
Saltin, B., and Stenberg, J. (1964). Circulatory response to prolonged severe exercise. J. Appl. Physiol. 19, 833–838.
Sawka, M. N., Convertino, V. A., Eichner, E. R., Schnieder, S. M., and Young, A. J. (2000). Blood volume: importance and adaptations to exercise training, environmental stresses, and trauma/sickness. Med. Sci. Sports Exerc. 32, 332–348. doi: 10.1097/00005768-200002000-00012
Sawka, M. N., Leon, L. R., Montain, S. J., and Sonna, L. A. (2011). Integrated physiological mechanisms of exercise performance, adaptation, and maladaptation to heat stress. Compr. Physiol. 1, 1883–1928. doi: 10.1002/cphy.c100082
Sawka, M. N., Young, A. J., Latzka, W. A., Neufer, P. D., Quigley, M. D., and Pandolf, K. B. (1992). Human tolerance to heat strain during exercise: influence of hydration. J. Appl. Physiol. 73, 368–375.
Schliess, F., Richter, L., vom Dahl, S., and Haussinger, D. (2006). Cell hydration and mTOR-dependent signalling. Acta Physiol. 187, 223–229. doi: 10.1111/j.1748-1716.2006.01547.x
Schwimmer, H., Eli-Berchoer, L., and Horowitz, M. (2006). Acclimatory-phase specificity of gene expression during the course of heat acclimation and superimposed hypohydration in the rat hypothalamus. J. Appl. Physiol. 100, 1992–2003. doi: 10.1152/japplphysiol.00850.2005
Senay, L. C. Jr. (1975). Plasma volumes and constituents of heat-exposed men before and after acclimatization. J. Appl. Physiol. 38, 570–575.
Sjogaard, G., and Saltin, B. (1982). Extra- and intracellular water spaces in muscles of man at rest and with dynamic exercise. Am. J. Physiol. 243, R271–R280.
Starling, E. H. (1896). On the absorption of fluids from the connective tissue spaces. J. Physiol. 19, 312–326. doi: 10.1113/jphysiol.1896.sp000596
Strauss, M. B., Davis, R. K., Rosenbaum, J. D., and Rossmeisl, E. C. (1952). Production of increased renal sodium excretion by the hypotonic expansion of extracellular fluid volume in recumbent subjects. J. Clin. Invest. 31, 80–86. doi: 10.1172/JCI102580
Szygula, Z., Smitz, J., Roeykens, J., De Meirleir, K., and Klausen, T. (1995). An early effect of acute plasma volume expansion in humans on serum erythropoietin concentration. Eur. J. Appl. Physiol. Occup. Physiol. 72, 106–110. doi: 10.1007/BF00964123
Tanaka, H., Shindo, M., Gutkowska, J., Kinoshita, A., Urata, H., Ikeda, M., et al. (1986). Effect of acute exercise on plasma immunoreactive-atrial natriuretic factor. Life Sci. 39, 1685–1693. doi: 10.1016/0024-3205(86)90166-9
Thompson, P. D., Crouse, S. F., Goodpaster, B., Kelley, D., Moyna, N., and Pescatello, L. (2001). The acute versus the chronic response to exercise. Med. Sci. Sports Exerc. 33(6 Suppl), S438–S445; discussion S452-3. doi: 10.1097/00005768-200106001-00012
Thornton, S. N. (2010). Thirst and hydration: physiology and consequences of dysfunction. Physiol. Behav. 100, 15–21. doi: 10.1016/j.physbeh.2010.02.026
Wade, C. E., Hill, L. C., Hunt, M. M., and Dressendorfer, R. H. (1985). Plasma aldosterone and renal function in runners during a 20-day road race. Eur. J. Appl. Physiol. Occup. Physiol. 54, 456–460. doi: 10.1007/BF00422951
Wade, C. E., Ramee, S. R., Hunt, M. M., and White, C. J. (1987). Hormonal and renal responses to converting enzyme inhibition during maximal exercise. J. Appl. Physiol. 63, 1796–1800.
Wallace, J. P., Bogle, P. G., King, B. A., Krasnoff, J. B., and Jastremski, C. A. (1999). The magnitude and duration of ambulatory blood pressure reduction following acute exercise. J. Hum. Hypertens. 13, 361–366. doi: 10.1038/sj.jhh.1000797
Wright, H. E., Selkirk, G. A., and McLellan, T. M. (2010). HPA and SAS responses to increasing core temperature during uncompensable exertional heat stress in trained and untrained males. Eur. J. Appl. Physiol. 108, 987–997. doi: 10.1007/s00421-009-1294-0
Yang, R. C., Mack, G. W., Wolfe, R. R., and Nadel, E. R. (1998). Albumin synthesis after intense intermittent exercise in human subjects. J. Appl. Physiol. 84, 584–592.
Keywords: hypotension, hypervolemia, aldosterone, erythropoietin, calisthenics, adaptation, orthostasis, hypohydration
Citation: Akerman AP, Lucas SJE, Katare R and Cotter JD (2017) Heat and Dehydration Additively Enhance Cardiovascular Outcomes following Orthostatically-Stressful Calisthenics Exercise. Front. Physiol. 8:756. doi: 10.3389/fphys.2017.00756
Received: 15 May 2017; Accepted: 19 September 2017;
Published: 09 October 2017.
Edited by:
Gary W. Mack, Brigham Young University, United StatesReviewed by:
Thad E. Wilson, Osteopathic Medical School, Marian University, United StatesStephen Cheung, Brock University, Canada
Copyright © 2017 Akerman, Lucas, Katare and Cotter. This is an open-access article distributed under the terms of the Creative Commons Attribution License (CC BY). The use, distribution or reproduction in other forums is permitted, provided the original author(s) or licensor are credited and that the original publication in this journal is cited, in accordance with accepted academic practice. No use, distribution or reproduction is permitted which does not comply with these terms.
*Correspondence: James D. Cotter, amltLmNvdHRlckBvdGFnby5hYy5ueg==