- 1Department of Life Sciences, National Chung Hsing University, Taichung, Taiwan
- 2Department of Oceanography, National Sun Yat-sen University, Kaohsiung, Taiwan
- 3Mariculture Research Center, Fisheries Research Institute, Council of Agriculture, Yulin, Taiwan
- 4Agricultural Biotechnology Center, National Chung Hsing University, Taichung, Taiwan
The fluctuation of temperature affects many physiological responses in ectothermic organisms, including feed intake, growth, reproduction, and behavior. Changes in environmental temperatures affect the acquisition of energy, whereas hepatic glycogen plays a central role in energy supply for the homeostasis of the entire body. Glycogen phosphorylase (GP), which catalyzes the rate-limiting step in glycogenolysis, is also an indicator of environmental stress. Here, we examined the effects of salinity on glycogen metabolism in milkfish livers under cold stress. A reduction of feed intake was observed in both freshwater (FW) and seawater (SW) milkfish under cold adaptation. At normal temperature (28°C), compared to the FW milkfish, the SW milkfish exhibited greater mRNA abundance of the liver isoform of GP (Ccpygl), higher GP activity, and less glycogen content in the livers. Upon hypothermal (18°C) stress, hepatic Ccpygl mRNA expression of FW milkfish surged at 3 h, declined at 6 and 12 h, increased again at 24 h, and increased significantly after 96 h. Increases in GP protein, GP activity, and the phosphorylation state and the breakdown of glycogen were also found in FW milkfish livers after 12 h of exposure at 18°C. Conversely, the Ccpygl transcript levels in SW milkfish were downregulated after 1 h of exposure at 18°C, whereas the protein abundance of GP, GP activity, and glycogen content were not significantly altered. Taken together, under 18°C cold stress, FW milkfish exhibited an acute response with the breakdown of hepatic glycogen for maintaining energy homeostasis of the entire body, whereas no change was observed in the hepatic glycogen content and GP activity of SW milkfish because of their greater tolerance to cold conditions.
Introduction
Glycogen, a polymer of glucose residues, is a crucial form of energy storage. Catabolism of stored glycogen (i.e., glycogenolysis) occurs through the action of glycogen phosphorylase (GP; EC 2.4.1.1), which releases glucose-1-phosphate from the glycogen polymer and provides glucose for energy required by the organisms. GP is a homodimeric enzyme subjected to allosteric control and exhibits transitions between “relaxed” (active) and “tense” (inhibited) conformational states (Johnson, 1992; Agius, 2015). In fish, three isoforms of GP were named according to the tissues in which they occur, including the brain (pygb), liver (pygl), and muscle (pygm). The liver and skeletal muscle are two major tissues for glycogen storage. The concentration of glycogen is higher in the liver than in the muscle (10 vs. 2% by weight) (Berg et al., 2002). In the liver, glycogen degradation and synthesis play major roles in regulating blood glucose homeostasis and supplying energy to other tissues (Vornanen and Haverinen, 2011; Polakof et al., 2012).
Elevated blood glucose levels and energy metabolism have been detected in response to stress or environmental fluctuations (Lin et al., 2011; Polakof et al., 2012; Huang et al., 2015). Water temperature is critical to the physiological responses and energy utilization strategies of teleosts. Among different species, the best physiological performance (ex. behavior, feed intake, digestion, growth, and reproduction) was usually found in the optimal ranges (Pörtner et al., 2007; Handeland et al., 2008; Pörtner, 2009; Schram et al., 2013; Payne et al., 2016). Total GP activity of the liver and heart of the crucian carp (Carassius carassius) increased during the summer (Vornanen and Haverinen, 2011). In addition, a two-stage decline in temperature is the main trigger for active foraging and synthesis of glycogen before winter dormancy in the crucian carp, whereas the hepatic glycogen store did not change when the temperature declined steadily (Varis et al., 2016). The rainbow smelt (Osmerus mordax) is a temperate species and can tolerate low temperatures from 0.4 to −1.5°C. The production of glycerol is important as an antifreeze in the plasma and other tissues, and at 0.4°C, the increase in total GP activity promoted the breakdown of glycogen allowing glycerol accumulation in the rainbow smelt (Clow et al., 2008). Conversely, at 8°C, the gilthead sea bream (Sparus aurata) typically undergoes a multi-organ dysfunction called the “winter syndrome.” Furthermore, a reduction in feed intake and accumulation of liver glycogen were detected during pre-winter periods (18°C) (Couto et al., 2008; Ibarz et al., 2010c).
The milkfish (Chanos chanos) is an economically important species in Southeast Asia and Taiwan. The average temperatures in summer and winter in Taiwan are approximately 28°C and 18°C, respectively. Cold snaps in winter, however, sometimes make the temperature lower than 15°C, leading to high mortality of this tropical euryhaline species and causing huge economic losses. Acclimation of milkfish to seawater (SW) or fresh water (FW) induces a variety of physiological responses, including changes in their tolerance to hypothermal stress. SW milkfish exhibited better hypothermal tolerance than FW milkfish (Kang et al., 2015). The FW milkfish exhibited higher gill Na+/K+-ATPase activity that might require a greater energy supply compared to that of the SW individuals (Lin et al., 2003; Kang et al., 2015). From transcriptome analyses of hypothermal milkfish (Hu et al., 2015, 2017), the fragments per kilobase of transcript per million reads mapped (FPKM) of GP was found to increase 1.28-fold in FW individuals and decrease to 0.77-fold in SW fish. Moreover, the protein abundance of lactate dehydrogenase was found to be upregulated in the liver of SW milkfish under hypothermal adaptation, indicating the requirement of a larger energy source under the stressful conditions (Chang et al., 2016b). Hence, the strategies of energy utilization in livers between FW and SW milkfish are proposed to be distinct, leading to different cold tolerance upon hypothermal stress.
Being an important factor for energy supply of fish, the feed intake was found to be lower in the gilthead sea bream under cold stress (Ibarz et al., 2007; Couto et al., 2008). The decreased energy source from feed intake led to a degradation in hepatic glycogen for maintaining blood glucose (Ibarz et al., 2010a). In this study, feed intake and hepatic glycogen content were analyzed in FW and SW milkfish under hypothermal (18°C) stress. In addition, the partial sequence of Ccpygl was identified. The Ccpygl mRNA abundance and GP activity in the livers of 18°C-exposed FW and SW milkfish were compared. Our data illustrated distinct strategies of glycogen utilization corresponding to different low temperature tolerances for FW- and SW-acclimated euryhaline milkfish.
Materials and Methods
Rearing Conditions of Milkfish
Juvenile milkfish were obtained from a local fish farm in Lukang, Taiwan. Fish were transported to the laboratory, where they were raised in four 400 L rearing tanks; two with seawater (SW 35‰) and two with FW, at 28 ± 1°C with a daily 12 h photoperiod for at least 1 month to reach a steady state (Kang et al., 2013; Chang et al., 2016c; Hu et al., 2017). The water for rearing tanks was continuously circulated through fabric-floss filters, and salinity was measured by a refractometer ATC-S (ATAGO, Tokyo, Japan). All experimental animals were fed to satiation at 15:00–16:00 everyday. The milkfish commercial diets contain 24% crude protein and 3% crude lipid (FWUSOW Industry, Taichung, Taiwan). In total, 188 milkfish were used in the present study. The protocol for the experimental fish was reviewed and approved by the Institutional Animal Care and Use Committee (IACUC) of the National Chung Hsing University (IACUC Approval No. 105-024 to THL).
Hypothermal Acclimation/Stress Experiments
For these two experiments, the temperature was maintained at 28 ± 1°C for the control group and 18 ± 0.5°C for the hypothermal group. The water for hypothermal SW and FW groups was cooled down at a constant rate (2°C h−1) with a cooling system (PF-225M, PRINCE, Tainan, Taiwan). After transfer to the 100 L experimental tanks with a hypothermal (18°C) or control temperature (28°C) from the 400 L rearing tanks, the milkfish were maintained and stabilized in the experimental tanks for at least 2 days. For the hypothermal acclimation experiments, milkfish were kept in four 100 L experimental tanks/conditions with different temperature × salinity set-ups ([1] SW/28°C, [2] FW/28°C, [3] SW/18°C, and [4] FW/18°C) for 1 week and then sampled (n = 6 for each condition). For the hypothermal stress experiments, milkfish were acclimated to 28°C followed by a temperature drop to 18°C at a rate of 2°C per hour, and subsequent sampling at 1, 3, 6, 12, 24, 48, 96, and 168 h after reaching 18°C (n = 6 for each of the salinities). During the experiments, milkfish were fed once per day. The experimental fish were euthanized the morning after the end of the experiment and anesthetized with 0.5% 2-phenoxyethanol before sampling. All efforts were made to minimize suffering and distress. The average body weight and average total length of sampled milkfish were 13.1 ± 2.3 g and 10.2 ± 1.3 cm, respectively. Milkfish livers were dissected quickly, immersed in liquid nitrogen, and stored at −80°C until the following analyses.
Feed-Intake Experiments
In addition to the hypothermal acclimation/stress experiments, 32 experimental milkfish were used in feed-intake experiments. Four experimental conditions ([1] SW/28°C, [2] FW/28°C, [3] SW/18°C, and [4] FW/18°C) of the feed-intake experiments were performed, and eight experimental animals were used in each condition. For each experimental condition, a single milkfish was reared in a 100 L experimental tank for 1 week and used for feed-intake measures only once. During the experimental period, this milkfish in the experimental tank was fed once (15:00–16:00) daily. On the 17 day of the experiment, the feed-intake of the milkfish in the experimental condition was evaluated. Several 1.5 mL-tubes of feed pellets were prepared before feeding. 0.02 g milkfish commercial feed pellets that were stable in water were first weighed and packed in each 1.5 mL tube, and the number of feed pellets in the tube was counted. After feeding, all amounts (tubes) of feed pellets and the pellet residue in the experimental tank were counted. The uptake of feed pellets of each experimental milkfish per day (g fish−1 day−1) was worked out by subtracting the amount of pellet residue in the tank from the number of feeding pellets and converting to weight (g) of feed. The average feed-intake of milkfish in each condition was derived from eight individuals.
Total RNA Extraction and Reverse Transcription
Total RNA samples were isolated using the Tripure Isolation Reagent following the manufacturer's instructions. The genomic DNA contamination in RNA samples was eliminated by using the RNAspin Mini RNA isolation kit (GE Healthcare, Piscataway, NJ, USA). RNA integrity was verified by electrophoresis in 1% agarose-gel. The purity and concentration of extracted RNA were measured with a NanoDrop 2000 (Thermo Fisher Scientific, Waltham, MA, USA). Purified RNA with an A260/A280 ratio of between 1.8 and 2.0 was used for the following experiments. First-strand cDNA was synthesized by reverse transcribing 1 μg of the total RNA and the iScript Reverse Transcription Supermix (Bio-Rad Laboratories, Hercules, CA, USA) was used according to the manufacturer's instructions. The cDNA samples were stored at −20°C before analyses.
cDNA Cloning and Sequence Analysis
The partial sequence of Ccpygl was identified (KY923199), and the primers were designed by the Primer 3 Plus based on highly conserved regions compared with other teleosts from the NCBI database (Table 1). For PCR amplification, 2 μL of cDNA from the milkfish liver was used as the template in a 50 μL PCR reaction containing 0.25 μM dNTPs, 2 U of Ex-Taq polymerase, and 0.1 μL of cloning primer. PCR products were ligated into the pGM-T vector and sequenced. The amino acid sequence of GPL was used to build a phylogenetic tree using MEGA 6, and the tree was built using the maximum likelihood method in 1,000 bootstraps.
Real-Time PCR
The mRNA expression was detected by KAPA SYBR FAST qPCR Kit Master Mix and quantified with the Mini Opticon real-time PCR system. The amplification efficiencies of the primers (Table 2) were evaluated to be 90–105%, and the r2 of the serial dilutions was evaluated to be 0.99. A single peak appeared in the melting curve analyses and the presence of a single amplification product was observed using 1.5% agarose gel. The mRNA expression of Ccgpl were normalized with the Chanos chanos 60S acidic ribosomal protein P2 (Ccrplp2) gene from the same cDNA sample. The expression levels of Ccrplp2 were not significantly different among various groups (Table 3). The PCR reactions contained 8 μL of cDNA, 2 μL of qPCR primer (2 μM), and 10 μL of SYBR Master Mix. The liver samples of the four experimental groups (FW/28°C, FW/18°C, SW/28°C, and SW/18°C) were pooled and used as the internal control (IC) among different qPCR analyses. The relative gene expression formula was 2∧–[(CtCcgpl, n – CtCcrplp2, n) – (CtCcgpl, IC – CtCcrplp2, IC)] (Livak and Schmittgen, 2001).
Immunoblotting
The immunoblotting protocol was modified from Chang et al. (2016c). The milkfish livers were suspended in SEID medium (150 mM sucrose, 10 mM EDTA, 50 mM imidazole, 0.1% sodium deoxycholate; pH 7.5) containing protease inhibitor (vol/vol: 25:1; Roche, Mannheim, Germany) and were homogenized with a Polytron PT1200E homogenizer (Lucerne, Switzerland) at maximum speed. The homogenates were then centrifuged at 10,000 × g at 4°C for 10 min. Protein concentrations of the supernatants were determined using reagents from the Protein Assay Kit (Bio-Rad), and bovine serum albumin (Sigma-Aldrich, St. Louis, MO, USA) was used as a standard. The homogenates containing 50 μg were heated at 60°C for 15 min and fractionated by electrophoresis on SDS containing 8% polyacrylamide gels. The pre-stained protein molecular weight marker (#26616, Thermo) was applied in electrophoresis. The separated proteins were transferred to 0.45 μm PVDF blotting membranes (Millipore, Bedford, MA, USA). The PVDF membranes were incubated for 1 h in PBST with 5% (wt/vol) nonfat dried milk to minimize non-specific binding. The blots were incubated with the primary antibody (GP, 1:10,000; GTX124390; Genetex, Irvine, CA, USA; GAPDH, 1:5,000; GTX100118; Genetex) overnight at 4°C, followed by incubation with the HRP-conjugated secondary antibody (goat anti-rabbit IgG, 1:10,000; GTX213110; Genetex) for 1 h at 28°C. The blots were developed with the Immobilon Western Chemiluminescent HRP substrate (Millipore). The images were photographed using the universal hood with a cooling-charge-coupled device (CCD) camera (ChemiDoc XRS+, Bio-Rad) and analyzed with ImageLab software version 3.0 (Bio-Rad) to normalize numerical values compared to the relative intensities of immunoreactive bands. The protein of GP was detected as a single immunoreactive band at 93 kDa (Figure S1).
Glycogen Phosphorylase (GP) Activity
Functional GP is capable of binding to glycogen when the enzyme is in the R (relaxed) state. The phosphorylated GP is automatically changed to the R state, whereas unphosphorylated GP in the T (tense) state is regulated by AMP of the allosteric effector and then transformed to the R state. The total GP activity consists of detected activity of both the R (determined by AMP− assay buffer) and T (determined by AMP+ assay buffer) states (Johnson, 1992; Clow et al., 2008; Vornanen and Haverinen, 2011; Bolinger and Rodnick, 2014; Agius, 2015). The GP activity was determined according to the NAPDH-linked method with modification. The direction of glycogenolysis by determination of NAPDH was enzymatically coupled to phosphoglucomutase and glucose-6-phosphate dehydrogenase. The liver from each milkfish was dissected quickly and immersed in liquid nitrogen. A 10 mg sample of liver tissue was rapidly homogenized in 1 mL buffer (100 mM imidazole, 100 mM NaF, 2 mM EDTA, 0.1 mM PMSF) using a Polytron PT1200E at the maximal speed for 5 s on ice. The homogenates were centrifuged at 5,000 × g, at 4°C for 5 min. The supernatants were used for the GP activity assay. The GP activity assay solution (50 mM potassium-phosphate buffer, 0.2 mg/mL glycogen, 0.1 mM EDTA, 15 mM MgCl2, 6.5 mM NADP+, 0.001 mg/mL glucose-1,6-bisphosphate, 10 U/mL phosphoglucomutase, 10 U/mL glucose-6-phosphate dehydrogenase) was the 5′AMP− assay buffer for determining GP activity in the R-state. Conversely, the 5′AMP+ assay buffer for determining total GP activity (R+T-state) was the GP activity assay solution plus 1.6 mM 5′AMP. The sample of 25 μL from each fish was loaded into each well, together with 175 μL of the 5′AMP− or 5′AMP+ assay buffer. Each sample was assayed in triplicate. The 96-well microplate was analyzed every 1 min for up to 5 min in the VERSAmax microplate reader at 340 nm and 28°C. Serial dilution of NADPH (50, 25, 12.5, 6.25, and 3.125 μg in 25 μL sample buffer) were prepared for the standard curve.
Glycogen Contents
For quantification of glycogen, 50 mg of milkfish livers were homogenized in ddH2O. Then the homogenates were boiled for 10 min to inactive enzymes. The boiled samples were centrifuged at 18,000 × g for 10 min to remove insoluble material. The glycogen contents were subsequently determined using the glycogen colorimetric/fluorometric assay kit (K646-100, Biovision, Milpitas, CA, USA) following the manufacturer's instructions. Then, 5 μL of the 40 × dilution of liver samples were added to each well of the 96-well microplate, and the volume was brought to 50 μL with the hydrolysis buffer incubated at 28°C for 30 min. Then, development buffer mixture was added and incubated for 30 min at 28°C. Serial dilution of glycogen (0.04, 0.08, 0.12, 0.16, and 0.2 μg in 50 μL hydrolysis buffer) was prepared for the standard curve. The absorbance was measured in the VERSAmax microplate reader at 570 nm and the glycogen standard curve was used to calculate the glycogen concentration of the samples.
Statistical Analysis
Values are expressed as the mean ± SEM (standard error of mean). The quantitative values of 1-week data from hypothermal and control groups were determined by two-way analysis of variance (ANOVA) followed by Tukey's HSD post-hoc test on R version 3.4.2 (R foundation, Vienna, Austria). Data from time-course experiments were compared using the one-way ANOVA analysis with Dunnett's pairwise method on R version 3.4.2, using the 0 h data as the control. The significance level was set at P < 0.05.
Results
Hypothermal Effects on Feed Intake between FW and SW Milkfish
The feed intake of milkfish was significantly affected by water temperature; the average feed intake per day for FW- and SW-acclimated milkfish was 0.115 ± 0.009 g and 0.115 ± 0.013 g at 28°C, whereas it was reduced to 0.025 ± 0.003 g and 0.039 ± 0.005 g, respectively, under hypothermal acclimation (Figure 1). The two-way ANOVA analyses revealed that feed intake was affected by hypothermal acclimation, whereas the synergistic interaction did not significantly affect feed intake (Table 4).
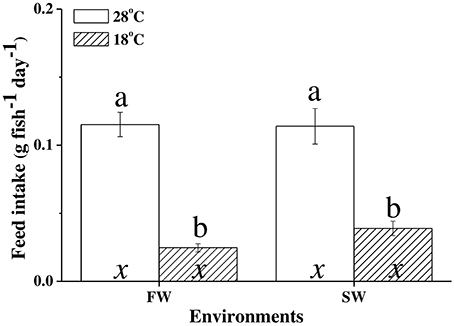
Figure 1. Feed intake (g fish−1 day−1) between hypothermal freshwater (FW) and seawater (SW) milkfish. Value are means ± SEM, n = 8. Different letters (a vs. b) indicate significant differences between the 28°C and 18°C group at the same salinity, and the letter “x” indicate no significant difference between the FW and SW group at the same temperature. The TukeyHSD pairwise comparison was used following two-way ANOVA, P < 0.05.
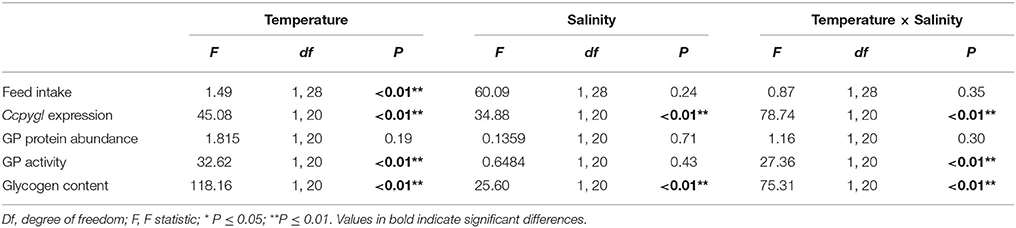
Table 4. Results of two-way ANOVA evaluating the source of temperature and salinity variations in livers of milkfish.
Phylogenetic Tree and Tissue Distribution of Ccpygl
The partial sequence of Ccpygl (1,606 bp, accession number: KY923199) was cloned from the liver of milkfish. This partial amino acid sequence was simultaneously confirmed to be the liver isoform of GP (pygl) by phylogenetic analyses in comparison with all three isoforms of GP (i.e., liver isoform, [pygl]; muscle isoform [pygm]; and brain isoform [pygb]) of other species (Figure S2). Subsequent phylogenetic analyses revealed that the amino acid sequence of Pygl of milkfish was highly similar to Pygl sequences of Mexican tetra (Astyanax mexicanus; 89%) and channel catfish (Ictalurus punctatus; 87%) and was categorized in the branch of Ostariophysi (Figure 2). Among the tissues collected from the SW milkfish, Ccpygl expression determined by qPCR was found to be primarily abundant in the liver. In addition to the liver, lower expression of Ccpygl was detected in the brain, gills, and kidney (Figure 3).
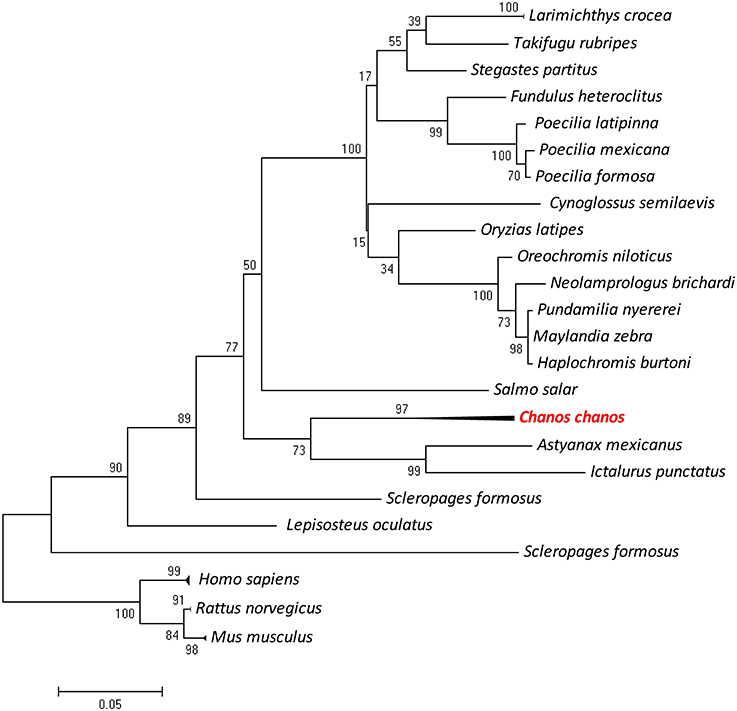
Figure 2. Phylogenetic analysis of milkfish (Chanos chanos) liver isoform of glycogen phosphorylase (Pygl) proteins based on amino acid sequences using the maximum likelihood method. The results were confirmed by 1000 bootstraps. Sequence accession numbers of different proteins are listed in Table 2.
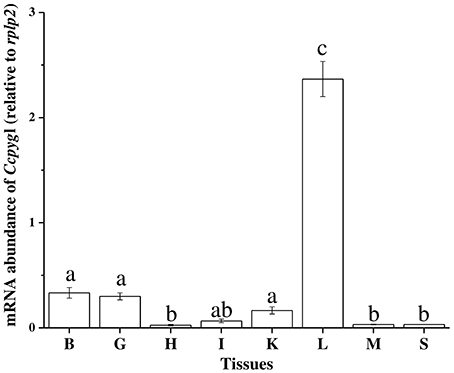
Figure 3. Tissue distribution of Ccpygl (Chanos chanos liver isoform of glycogen phosphorylase) mRNA expression of the milkfish detected by qPCR. Values are means ± SEM, n = 3. B, brain; G, gill; H, heart; I, intestine; K, kidney; L, liver; M, muscle; S, spleen.
Salinity Effects on Glycogen Catabolism in the Hypothermal Acclimation Experiments
At 28°C, the mRNA expression of Ccpygl and GP activity were significantly higher in the livers of SW milkfish than in those of FW milkfish (Figures 4A,C). The glycogen storage in the livers of SW milkfish, however, was lower than in those of the FW group (Figure 4D). Conversely, in the hypothermal acclimation experiments, the mRNA expression profiles of Ccpygl in the livers were upregulated in FW milkfish and downregulated in SW fish (Figure 4A). Hepatic GP protein (Figure 4B) and GP activity (Figure 4C) were also increased in FW milkfish under hypothermal acclimation, but not significantly different than in hypothermal SW-acclimated individuals (Figures 4B,C). Meanwhile, in the hypothermal acclimation experiments, glycogen contents were found to downregulate in the livers of FW milkfish, whereas they were slightly, but not significantly (p = 0.247), elevated in the livers of SW milkfish (Figure 4D). The two-way ANOVA analyses revealed that Ccpygl, GP activity, and glycogen content were affected by synergistic interaction between temperature and salinity (Table 4).
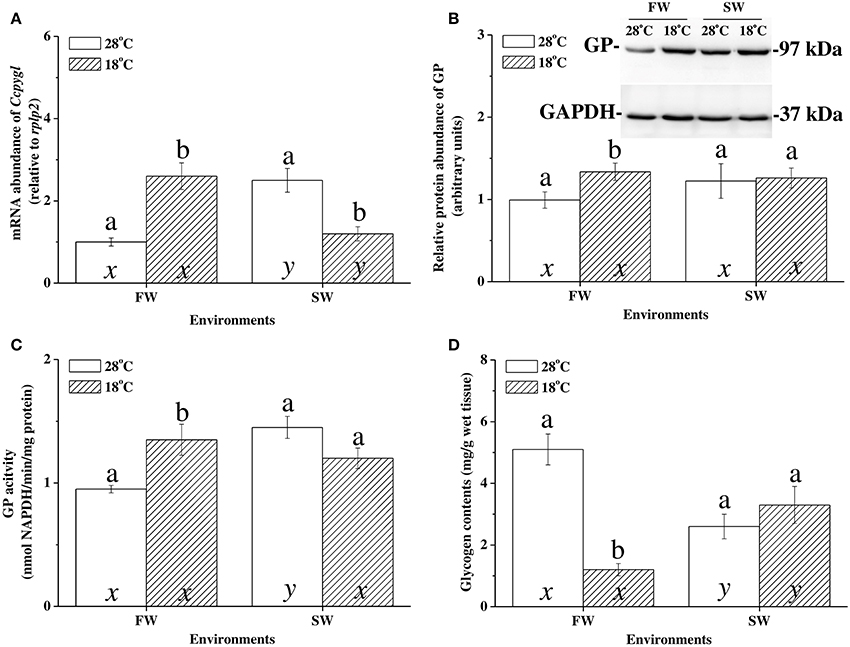
Figure 4. The hypothermal acclimation experiments revealed the glycogen catabolism pattern in livers of freshwater (FW) and seawater (SW) milkfish after 1-week hypothermal stress. (A) mRNA expression of Ccpygl, (B) abundance of GP protein, (C) GP activity, and (D) glycogen content. Values are means ± SEM, n = 6. Different letters (a vs. b and x vs. y) indicate significant differences between the 28°C and 18°C group at the same salinity, and the FW and SW group at the same temperature, respectively. The TukeyHSD pairwise comparison followed the two-way ANOVA, P < 0.05.
Acute Changes in Ccpygl mRNA Expression in the Hypothermal Stress Experiments
The acute mRNA expression of Ccpygl in milkfish livers was determined by qPCR at 0 h (control) and after hypothermal stress for 1, 3, 6, 12, 24, 48, 96, and 168 h in FW (Figure 5A) or SW (Figure 5B). In hypothermal FW, the mRNA expression of Ccpygl responded in a biphasic manner with a rapid increase peaking at 3 h and a second elevation at 96 h post transfer (Figure 5A). Conversely, in hypothermal SW, a significant downregulation of Ccpygl mRNA abundance occurred from 1 h post transfer to the end of the hypothermal stress experiment (168 h post transfer) (Figure 5B).
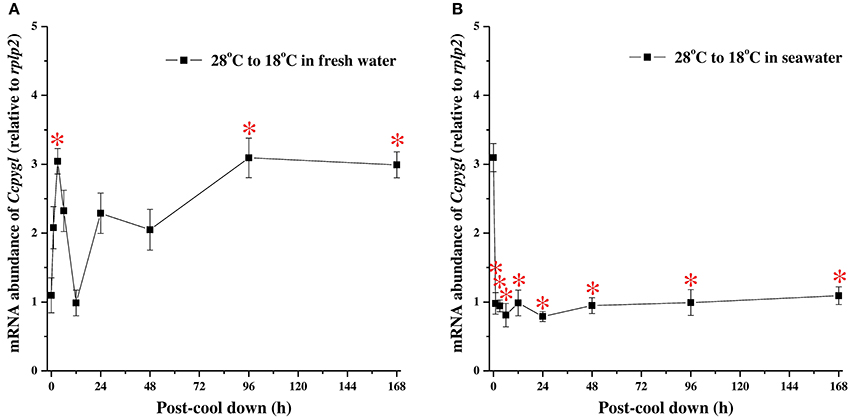
Figure 5. The hypothermal stress experiments revealed time-course mRNA expression of Ccpygl in livers of (A) fresh water (FW) and (B) seawater (SW) milkfish. Values are means ± SEM, n = 6. The asterisks indicate significant differences (P < 0.05) compared to the 0 h fish using one-way ANOVA with Dunnett's test.
Different Patterns of Hepatic Glycogen Catabolism between FW and SW Milkfish in the Hypothermal Stress Experiments
The protein abundance of GP, GP activity and glycogen contents in the livers of milkfish were analyzed at 0 (control), 12, 24, and 168 h of 18°C-exposure. The protein abundance of GP and GP activity in the livers of FW milkfish was significantly upregulated after 12, 24, and 168 h (approximately 1.5-fold) of 18°C-exposure (Figures 6A,C). In SW milkfish, however, no significant difference in protein abundance of GP and GP activity were found among different time-point groups in the hypothermal stress experiments (Figures 6B,D). Moreover, hepatic glycogen contents were significantly downregulated in FW milkfish after 12 h of hypothermal stress, and onward (Figure 6E). Conversely, in SW there was no significant change among different time-points after exposure to 18°C (Figure 6F).
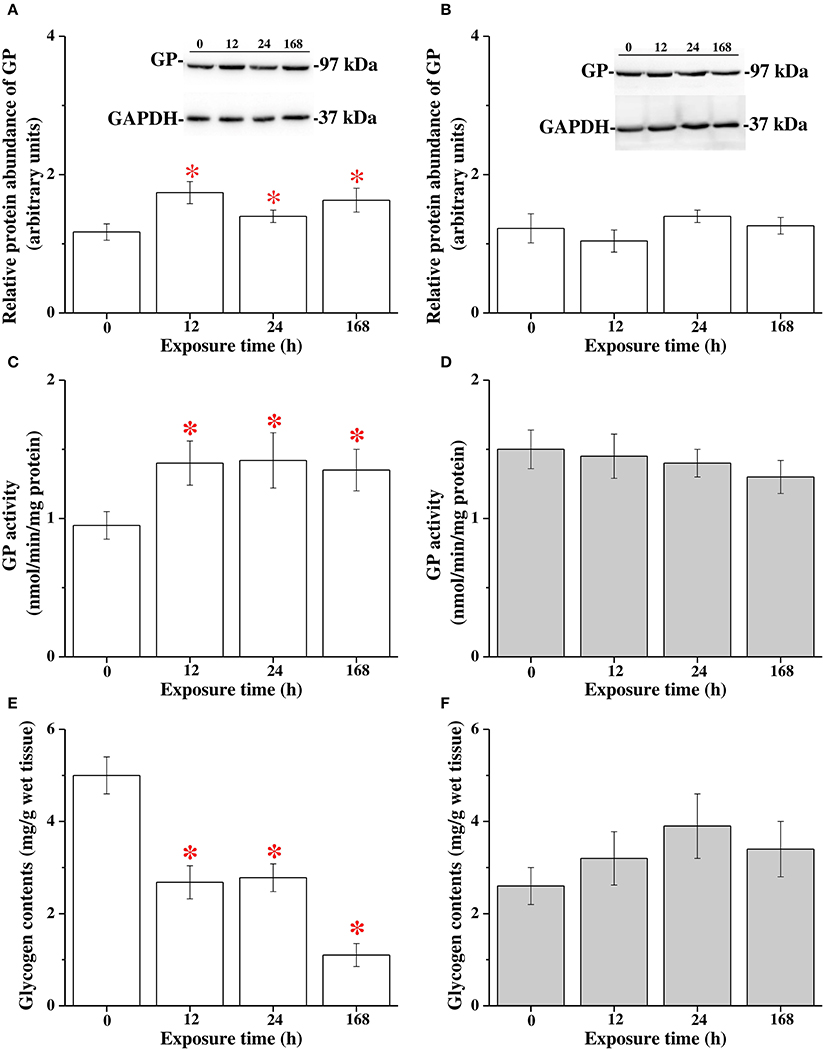
Figure 6. The hypothermal stress experiments revealed glycogen catabolism in livers of freshwater (FW) and seawater (SW) milkfish after 18°C-exposure. The protein abundance of GP in FW (A) and SW (B) groups, GP activity in FW (C) and SW (D) groups, glycogen contents in FW (E) and SW (F) groups, respectively. Values are means ± SEM, n = 6. The asterisks indicate significant differences (P < 0.05) compared to the 0 h fish using one-way ANOVA with Dunnett's test.
The Percentage of the Active form of GP in the Hypothermal Stress Experiments
The R-state is the phosphorylated state of GP with higher activity to degrade glycogen, whereas the T-state is the dephosphorylated state of GP with lower activity to breakdown glycogen. The percentage of the active form of GP activity was calculated as the results of GP (R-state; determined by AMP− assay buffer) divided by the total GP activity (R + T-state; determined by AMP+ assay buffer). Increasing percentages indicated that the levels of phosphorylation of GP in FW milkfish were significantly upregulated after 12, 24, and 168 h of exposure to 18°C (Figure 7A). However, the phosphorylation level of GP was not significantly different to that in SW milkfish in the hypothermal stress experiments (Figure 7B).
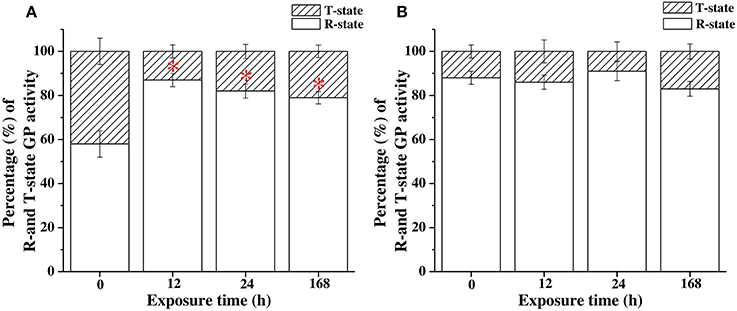
Figure 7. The percentages of active form of GP activity in livers of (A) freshwater (FW) and (B) seawater (SW) milkfish in the hypothermal stress experiments after 18°C-exposure. Values are means ± SEM, n = 6. The percentage of the activity of GP active form: GP activity (AMP−)/total GP activity (AMP+) × 100 (%). The asterisk indicates a significant difference compared to the 0 h fish by one-way ANOVA (Dunnett's comparison, P < 0.05).
Discussion
The most important finding of the present study was that under hypothermal stress, salinity affected the glycogen utilization strategy of milkfish. The mRNA expression, protein abundance, and GP activity were upregulated and correlated with glycogen catabolized (correlation coefficient: mRNA, −0.88; protein, −0.60; GP activity, −0.81) in FW-acclimated milkfish at 18°C. Moreover, mRNA expression of Ccgpl behaved in a biphasic manner, with an early and late phase, and the protein abundance of GP and phosphorylation state of GP were regulated acutely in FW-acclimated individuals under hypothermal stress. The GP activity and glycogen contents, however, were not significantly altered in SW-acclimated milkfish when exposed to 18°C. Only the mRNA expression of Ccgpl was downregulated after 1 h exposure at 18°C.
Phylogenetic Relationship of Glycogen Phosphorylase in Milkfish
Near et al. (2014) described the phylogenetic relationship of gonorynchiform fishes by using several nuclear DNA genes. The milkfish (Chanos chanos) is a primitive species originating in the Mesozoic and classified in Chanidae of Gonorynchiformes. The phylogenetic tree of ossification showed that milkfish was classified with the original ray-finned fish (Arratia and Bagarinao, 2010). The pygl gene of milkfish was classified in the Ostariophysi family. This gonorynchiform species is close to Characiformes (Astyanax mexicanus) and Siluriformes (Ictalurus punctatus) species (Arratia and Bagarinao, 2010). Three isoforms of GP were highly similar in their amino acid sequences. They were encoded by three genes, pygl, pygm, and pygb, which were mainly distributed in the liver, muscle, and brain, respectively (Tseng et al., 2007; Polakof et al., 2012). In the present study, the CcPygl sequence was confirmed according to the phylogenetic tree of these three isoforms.
Salinity Effects on Glycogen Utilization in the Livers of Milkfish
The liver is an important organ for energy metabolism, and is responsible for glycogen and glucose turnover, fatty acid synthesis, and gluconeogenesis in all teleosts (Polakof et al., 2012). Differentially expressed genes related to energy metabolism were recently reported in the livers of the gilthead sea bream (S. aurata), after hypo-osmotic challenge (Martos-Sitcha et al., 2016). The glycogen levels and GP activity were not changed under hypo-osmotic stress in the livers of the gilthead sea bream (Laiz-Carrión et al., 2005) as well as another marine species, the Senegalese sole (Solea senegalensis) (Arjona et al., 2009). Similarly, in freshwater goldfish (Carassius auratus), the levels of hepatic glycogen did not change under hyperosmotic stress (Luz et al., 2008). The hepatic glycogen contents of tilapia (Oreochromis mossambicus) and rainbow trout (Oncorhynchus mykiss) were utilized in an acute phase upon hyperosmotic challenge (Chang et al., 2007; Singer et al., 2007). Further, the hepatic glycogen may have provided carbohydrates for glycolysis to maintain branchial ionic homeostasis in tilapia, gilthead sea bream, and rainbow trout in time-course experiments (Soengas et al., 1993; Sangiao-Alvarellos et al., 2005; Chang et al., 2007). The milkfish is a marine euryhaline species with branchial Na+/K+-ATPase (NKA), the major energy-requiring pump for maintaining ionic homeostasis, more highly expressed in FW-acclimated individuals than in SW-acclimated ones. Therefore, the energy demand for ionoregulation was higher in milkfish under hypo-osmotic stress (Jana et al., 2006; Tang et al., 2010; Kang et al., 2015) reported that milkfish fry acclimated to 25‰ seawater had the lowest level of hepatic glycogen, but exhibited the best growth performance (1.2% g day−1) and muscle protein content. It is obvious that changes in environmental salinity influence strategies of energy accumulation and growth performance in milkfish (Jana et al., 2006). The present study further revealed that the energy storage or utilization strategies of milkfish obviously vary depending on the environmental salinities to which they are acclimated. On the other hand, the acute utilization strategies of hepatic glycogen in milkfish for the entire body upon hypo-osmotic challenge are still unknown. Future studies will focus on the mechanism by which hepatic glycogen in milkfish is used for maintaining ionic homeostasis upon hypo-osmotic challenge.
Temperature Effects on Glycogen Utilization in the Livers of Milkfish
Low temperature appears to be an important factor to regulate glycogen storage in the crucian carp (Carassius carassius), a temperate species. A two-step cooling down procedure (from 18°C to 7.5°C maintained for 30 days, then from 7.5 to 2°C maintained until 60 days) induced hepatic glycogen storage, whereas the direct cooling-down (to 2°C) procedure did not change the glycogen content in the livers of the crucian carp (Varis et al., 2016). In the gilthead sea bream, a subtropical species, the reduction of feed intake was significantly correlated with environmental temperatures and liver glycogen deposition was observed in the low-temperature (18°C) group rather than in the normal-temperature (25°C) group (Couto et al., 2008; Enes et al., 2008). Similarly, reduction of feed intake was also observed in the FW- and SW-acclimated milkfish under hypothermal (18°C) adaptation. When environmental temperature decreased to 8°C, however, the energy utilization strategy of sea bream changed to the production of nonpolar and polar lipids, whereas the glycogen content in the livers was not significantly changed. In environments with much lower temperatures, the production of lipids was altered to maintain membrane fluidity, and glycogen storage decreased in the liver of the sea bream (Ibarz et al., 2010a; Melis et al., 2017). Being a tropical and herbivorous species, the milkfish cannot survive in temperatures lower than 15°C and continue to use carbohydrates as their main energy resource (Chiu and Benitez, 1981; Benitez, 1983; Hu et al., 2015). On the other hand, when silver catfish (Rhamdia quelen), another tropical species, were transferred from 20°C to 15°C, their hepatic glycogen degraded (Lermen et al., 2004). In the tropical white shrimp (Litopenaeus vannamei), the catabolism of glycogen was detected under cold stress (Zhou et al., 2011). In this study, hepatic glycogen degradation was also detected in FW-acclimated milkfish, but not in SW-acclimated milkfish, under hypothermal adaptation (18°C). In aquatic organisms, hepatic glycogen storage or catabolism was affected by external temperature. When out of the optimal temperature range, reduction of feed intake and hepatic glycogen degradation were reported in aquatic organisms from tropical, subtropical, or temperate habitats. In addition, anti-oxidative responses under hypothermal stress were found in the livers of the zebrafish (Danio rerio), Nile tilapia (O. niloticus), and gilthead sea bream (Ibarz et al., 2010b; He et al., 2015; Wu et al., 2015). This antioxidant mechanism is energy consuming (Espinosa-Diez et al., 2015; Huang et al., 2015). The glycogen may be used in glycolysis to produce ATP or by the pentose phosphate pathway (Stanton, 2012). Then, the antioxidant system works depending on production of NADPH from the pentose phosphate pathway. Under hypothermal adaptation, FW milkfish encounter more oxidative stress in the liver than that by SW milkfish (Chang et al., 2016a,b, 2017). Therefore, the response of milkfish upon hypothermal and salinity stress may be correlated with the elevation of energy requirements.
Regulation of Phosphorylation State of GP between FW and SW Milkfish under Cold Stress
The activity of GP is regulated by the phosphorylation cycle, including the unphosphorylated T (tense)-state and phosphorylated R (relax)-state. The phosphorylation-state affected GP activity might be regulated by hormonal stimulation in the acute phase (Andersen and Westergaard, 2002; Milligan, 2003; Clow et al., 2008). The level of cortisol and glucagon-like peptide (GLP) in teleosts were suggested to play a role in elevating GP activity (Mommsen et al., 1999; Hallgen et al., 2003; Milligan, 2003). Barton and Peter (1982) found that the plasma cortisol levels of rainbow trout increased within 30 min and were maintained up to 4 h after rapid temperature decrease (from 10–11°C to 1°C), whereas they were recovered to the normal level after 24 h. Arjona et al. (2009) reported that plasma cortisol levels were increased 20-fold upon hypo-osmotic challenge in the Senegalese sole. In addition, 12°C exposure for 1 h induced an increase in the plasma cortisol levels in tilapia, whereas 19°C cold exposure did not cause this change (Chen et al., 2002). In addition, the GLP increased blood glucose levels via activation of gluconeogenesis in the livers of teleosts (Mojsov, 2000). The GLP levels of carp (Cyprinus carpio) blood were upregulated under acute changes in temperatures (Navarro et al., 2002). In the present study, after 12 h cold-exposure, increasing protein abundance of GP and phosphorylation levels along with an increase in GPase activity and decrease in glycogen content were found in the livers of FW milkfish, but not in SW milkfish. The total GP (R+T state) and GP (R state) allowed for identification of the elevated phosphorylation state of GP upon hypothermal stress in FW milkfish; however, no such change was noted in SW milkfish. The FW-acclimated juvenile milkfish had lower cold tolerance than the SW-acclimated fish (Kang et al., 2015). According to Milligan (2003), stress hormones might induce hepatic glycogen degradation to meet the glucose demands in FW milkfish under cold stress. Moreover, the GP activity in SW milkfish livers was not significantly different under cold stress, whereas downregulation of hepatic Ccpygl immediately after cold exposure in SW milkfish might be regulated via insulin or an insulin-like hormone (Klover and Mooney, 2004; Polakof et al., 2012).
Conclusion
The results of the molecular, biochemical, and enzymatic analyses in this study illustrated the hypothermal effects on salinity-dependent glycogen utilization strategies in juvenile milkfish. In hypothermal acclimation, the feed intake of milkfish reduced in both FW and SW milkfish. However, only the FW milkfish upregulated hepatic glycogen catabolism to supply the energy required for acclimation to hypothermal stress. The phosphorylation state, protein abundance, and transcript levels of GP in milkfish livers were further found to be adjustable in the acute phase upon hypothermal challenge, corresponding to the salinity of the acclimated environment.
Author Contributions
C-HC and T-HL conceived the study. C-HC, J-JH, and T-HL discussed and designed the experiments. C-HC, J-JH, C-YY, and L-YH carried out the animal culture. C-HC, J-JH, and C-YY performed the experiment. C-HC and T-HL contributed to writing the manuscript. Both authors read and approved the final manuscript. C-HT glycogen phosphorylase (GP) antibody and test of experimental condition of GP immunoblotting.
Funding
This study was supported by grants to T-HL from the Ministry of Science the Technology (MOST) of Taiwan (105-2313-B-005-027-MY3).
Conflict of Interest Statement
The authors declare that the research was conducted in the absence of any commercial or financial relationships that could be construed as a potential conflict of interest.
Supplementary Material
The Supplementary Material for this article can be found online at: https://www.frontiersin.org/articles/10.3389/fphys.2018.00081/full#supplementary-material
References
Agius, L. (2015). Role of glycogen phosphorylase in liver glycogen metabolism. Mol. Aspect Med. 46, 34–45. doi: 10.1016/j.mam.2015.09.002
Andersen, B., and Westergaard, N. (2002). The effect of glucose on the potency of two distinct glycogen phosphorylase inhibitors. Biochem. J. 367, 443–450. doi: 10.1042/bj20020153
Arjona, F. J., Vargas-Chacoff, L., Ruiz-Jarabo, I., Gonçalves, O., Páscoa, I., Martín del Río, M. P., et al. (2009). Tertiary stress response in Senegalese sole (Solea senegalensis Kaup, 1858) to osmotic challenge: implications for osmoregulation, energy metabolism and growth. Aquaculture 287, 419–426. doi: 10.1016/j.aquaculture.2008.10.047
Arratia, G., and Bagarinao, T. (2010). “Early ossification and development of the cranium and paired girdles of Chanos chanos (Teleostei, Gonorynchiformes),” in Gonorynchiformes and Ostariophysan Relationships: A Comprehensive Review, eds T. Grande, F. J. Poyato-Ariza, and R. Diogo (New Hampshire: Science Publishers), 73–106.
Barton, B. A., and Peter, R. E. (1982). Plasma cortisol stress response in fingerling rainbow trout, Salmo gairdneri Richardson, to various transport conditions, anesthesia, and cold shock. J. Fish Biol. 20, 39–51. doi: 10.1111/j.1095-8649.1982.tb03893.x
Benitez, L. V. (1983). “Milkfish nutrition,” in Advances in Milkfish Biology and Culture. Proceedings of the Second International Milk-Fish Aquaculture Conference, eds J. V. Juario, R. P. Ferraris, and L. V. Benitez (Iloilo City: SEAFDEC/IDRC), 133–143.
Berg, J. M., Tymoczko, J. L., and Stryer, L. (2002). “Glycogen metabolism,” in Biochemistry, 5th Edn., ed W. H. Freeman (New York, NY), 21.
Bolinger, M. T., and Rodnick, K. J. (2014). Differential effects of temperature and glucose on glycogenolytic enzymes in tissues of rainbow trout (Oncorhynchus mykiss). Comp. Biochem. Physiol. B. 171, 26–33. doi: 10.1016/j.cbpb.2014.03.003
Chang, J. C., Wu, S. M., Tseng, Y. C., Lee, Y. C., Baba, O., and Hwang, P. P. (2007). Regulation of glycogen metabolism in gills and liver of the euryhaline tilapia (Oreochromis mossambicus) during acclimation to seawater. J. Exp. Biol. 210, 3494–3504. doi: 10.1242/jeb.007146
Chang, C. H., Lo, W. Y., and Lee, T. H. (2016a). The antioxidant peroxiredoxin 6 (Prdx6) exhibits different profiles in the livers of seawater- and fresh water-acclimated milkfish, Chanos chanos, upon hypothermal challenge. Front. Physiol. 7:580. doi: 10.3389/fphys.2016.00580
Chang, C. H., Tang, C. H., Kang, C. K., Lo, W. Y., and Lee, T. H. (2016b). Comparison of integrated responses to nonlethal and lethal hypothermal stress in milkfish (Chanos chanos): a proteomics study. PLoS ONE 11:e0163538. doi: 10.1371/journal.pone.0163538
Chang, C. H., Yang, W. K., Lin, C. H., Kang, C. K., Tang, C. H., and Lee, T. H. (2016c). FXYD11 mediated modulation of Na+/K+-ATPase activity in gills of the brackish medaka (Oryzias dancena) when transferred to hypoosmotic or hyperosmotic environments. Comp. Biochem. Physiol. A 194, 19–26. doi: 10.1371/journal.pone.0055470
Chang, C. H., Lin, J. Y., Lo, W. Y., and Lee, T. H. (2017). Hypothermal stress induced differential expression profiles of the immune response gene, warm-temperature-acclimation associated 65-kDa protein (Wap65), in the liver of fresh water and seawater milkfish, Chanos chanos. Fish Shellfish Immunol. 70, 174–184. doi: 10.1016/j.fsi.2017.09.012
Chen, W. H., Sun, L. T., Tsai, C. L., Song, Y. L., and Chang, C. F. (2002). Cold-stress induced the modulation of catecholamines, cortisol, immunoglobulin M, and leukocyte phagocytosis in tilapia. Gen. Comp. Endocrinol. 126, 90–100. doi: 10.1006/gcen.2001.7772
Chiu, Y. N., and Benitez, L. V. (1981). Studies on the carbohydrates in the digestive tract of the milkfish Chanos chanos. Mar. Biol. 61, 247–254. doi: 10.1007/BF00386667
Clow, K. A., Ewart, K. V., and Driedzic, W. R. (2008). Low temperature directly activates the initial glycerol antifreeze response in isolated rainbow smelt (Osmerus mordax) liver cells. Am. J. Physiol. Regul. Integr. Comp. Physiol. 295, 961–970. doi: 10.1152/ajpregu.90372.2008
Couto, A., Enes, P., Peres, H., and Oliva-Teles, A. (2008). Effect of water temperature and dietary starch on growth and metabolic utilization of diets in gilthead sea bream (Sparus aurata) juveniles. Comp. Biochem. Physiol. A 151, 45–50. doi: 10.1016/j.cbpa.2008.05.013
Enes, P., Panserat, S., Kaushik, S., and Oliva-Teles, A. (2008). Hepatic glucokinase and glucose-6-phosphatase response to dietary glucose and starch in gilthead sea bream (Sparus aurata) juveniles reared at two temperatures. Comp. Biochem. Physiol. A 149, 80–86. doi: 10.1016/j.cbpa.2007.10.012
Espinosa-Diez, C., Miguel, V., Mennerich, D., Kietzman, T., Sánchez-Pérez, P., Cadenas, S., et al. (2015). Antioxidant responses and cellular adjustments to oxidative stress. Redox Biol. 6, 183–197. doi: 10.1016/j.redox.2015.07.008
Hallgen, N. K., Busby, E. R., and Mommsen, T. P. (2003). Cell volume affects glycogen phosphorylase activity in fish hepatocytes. J. Comp. Physiol. B 173, 591–599. doi: 10.1007/s00360-003-0369-1
Handeland, S. O., Imsland, A. K., and Stefansson, S. O. (2008). The effect of temperature and fish size on growth, feed intake, food conversion efficiency and stomach evacuation rate of Atlantic salmon post-smolts. Aquaculture 283, 36–42. doi: 10.1016/j.aquaculture.2008.06.042
He, J., Qian, J., Yang, H., Xu, P., Zhu, Z. X., and Yang, R. Q. (2015). Changes in the fatty acid composition and regulation of antioxidant enzymes and physiology of juvenile genetically improved farmed tilapia Oreochromis niloticus (L.), subjected to short-term low temperature stress. J. Therm. Biol. 53, 90–97. doi: 10.1016/j.jtherbio.2015.08.010
Hu, Y. C., Kang, C. K., Tang, C. H., and Lee, T. H. (2015). Transcriptomic analysis of metabolic pathways in milkfish that response to salinity and temperature changes. PLoS ONE 10:e0134959. doi: 10.1371/journal.pone.0134959
Hu, Y. C., Chu, K. F., Yang, W. K., and Lee, T. H. (2017). Na+,K+-ATPase β1 subunit associates with α1 subunit modulating a “higher-NKA-in-hyposmotic media response in gills of euryhaline milkfish, Chanos chanos. J. Comp. Physiol. B 187, 995–1007. doi: 10.1007/s00360-017-1066-9
Huang, C. Y., Lin, H. C., and Lin, C. H. (2015). Effects of hypoxia on ionic regulation, glycogen utilization and antioxidative ability in the gills and liver of the aquatic air-breathing fish Trichogaster microlepis. Comp. Biochem. Physiol. A 179, 25–34. doi: 10.1016/j.cbpa.2014.09.001
Ibarz, A., Blasco, J., Sala-Rabanal, M., Gallardo, A., Redondo, A., and Fernández-Borràs, J. (2007). Metabolic rate and tissue reserves in gilthead sea bream (Sparus aurata) under thermal fluctuations and fasting and their capacity for recovery. Can. J. Fish Aquat. Sci. 64, 1034–1042. doi: 10.1139/f07-079
Ibarz, A., Blasco, J., Gallardo, M. A., and Fernández-Borràs, J. (2010a). Energy reserves and metabolic status affect the acclimation of gilthead sea bream (Sparus aurata) to cold. Comp. Biochem. Physiol. A 155, 319–326. doi: 10.1016/j.cbpa.2009.11.012
Ibarz, A., Martín-Pérez, M., Blasco, J., Bellido, D., de Oliverira, E., and Fernández-Borràs, J. (2010b). Gilthead sea bream liver proteome altered at low temperatures by oxidative stress. Proteomics 10, 963–975. doi: 10.1002/pmic.200900528
Ibarz, A., Padros, F., Gallardo, M. A., Fernández-Borràs, J., Blasco, J., and Tort, L. (2010c). Low-temperature challenges to gilthead sea bream culture: review of cold-induced alterations and “Winter Synderom”. Rev. Fish. Biol. Fish. 20, 539–556. doi: 10.1007/s11160-010-9159-5
Jana, S. N., Garg, S. K., and Patra, B. C. (2006). Effect of inland water salinity on growth performance and nutritional physiology in growing milkfish, Chanos chanos (Forsskal): field and laboratory studies. J. Appl. Ichthyol. 22, 25–34. doi: 10.1111/j.1439-0426.2006.00698.x
Johnson, L. N. (1992). Glycogen phosphorylase: control by phosphorylation and allosteric effectors. FASEB J. 6, 2274–2282.
Kang, C. K., Yang, W. K., Lin, S. T., Liu, C. C., Lin, H. M., Chen, H. H., et al. (2013). The acute and regulatory phases of time-course changes in gill mitochondria-rich cells of seawater-acclimated medaka (Oryzias dancena) when exposed to hypoosmotic environments. Comp. Biochem. Physiol. A 164, 181–191. doi: 10.1016/j.cbpa.2012.08.010
Kang, C. K., Chen, Y. C., Chang, C. H., Tsai, S. C., and Lee, T. H. (2015). Seawater-acclimation abates cold effects on Na+, K+-ATPase activity in gills of the juvenile milkfish, Chanos chanos. Aquaculture 446, 67–73. doi: 10.1016/j.aquaculture.2015.04.022
Klover, P. J., and Mooney, R. A. (2004). Hepatocytes: critical for glucose homeostasis. Int. J. Biochem. Cell Biol. 36, 753–758. doi: 10.1016/j.biocel.2003.10.002
Laiz-Carrión, R., Sangiao-Alvarellos, S., Guzman, J. M., Martin del Rio, M. P., Soengas, J. L., and Mancera, J. M. (2005). Growth performance of gilthead sea bream Sparus aurata in different osmotic conditions: implication for osmoregulation and energy metabolism. Aquaculture 250, 849–861. doi: 10.1016/j.aquaculture.2005.05.021
Lermen, C. L., Lappe, R., Crestani, M., Vieira, V. P., Gioda, C. R., Schetinger, M. R., et al. (2004). Effect of different temperature regimes on metabolic and blood parameters of silver catfish Rhamdia quelen. Aquaculture 239, 497–507. doi: 10.1016/j.aquaculture.2004.06.021
Lin, Y. M., Chen, C. N., and Lee, T. H. (2003). The expression of gill Na, K-ATPase in milkfish, Chanos chanos, acclimated to seawater, brackish water and fresh water. Comp. Biochem. Physiol. A 135, 489–497. doi: 10.1016/S1095-6433(03)00136-3
Lin, Y. S., Tsai, S. C., Lin, H. C., Hsiao, C. D., and Wu, S. M. (2011). Changes of glycogen metabolism in the gills and hepatic tissue of tilapia (Oreochromis mossambicus) during short-term Cd exposure. Comp. Biochem. Physiol. C 154, 296–304. doi: 10.1016/j.cbpc.2011.06.014
Livak, K. J., and Schmittgen, T. D. (2001). Analysis of relative gene expression data using real-time quantitative PCR and the 2(-Delta Delta C(T)) method. Method 25, 402–408. doi: 10.1006/meth.2001.1262
Luz, R. K., Martinez-Alvarez, R. M., De Pedro, N., and Delgado, M. J. (2008). Growth, food intake regulation and metabolic adaptations in goldfish (Carassius auratus) exposed to different salinities. Aquaculture 276, 171–178. doi: 10.1016/j.aquaculture.2008.01.042
Martos-Sitcha, J. A., Mancera, J. M., Calduch-Giner, J. A., Yufera, M., Marinez-Rodriguez, G., and Perez-Sanchez, J. (2016). Unraveling the tissue-specific gene signatures of gilthead sea bream (Sparus aurata L.) after hyper- and hypo-osmotic challenges. PLoS ONE 11:e0148113. doi: 10.1371/journal.pone.0148113
Melis, R., Sanna, R., Braca, A., Bonaglini, E., Cappuccinelli, R., Slawski, H., et al. (2017). Molecular details on gilthead sea bream (Sparus aurata) sensitivity to low water temperature from 1H NMR metabolomics. Comp. Biochem. Physiol. A 204, 129–136. doi: 10.1016/j.cbpa.2016.11.010
Milligan, C. L. (2003). A regulatory role for cortisol in muscle glycogen metabolism in rainbow trout Oncorhynchus mykiss Walbaum. J. Exp. Biol. 206, 3167–3173. doi: 10.1242/jeb.00538
Mojsov, S. (2000). Glucagon-like peptide-1 (GLP-1) and the control of glucose metabolism in mammals and teleost fish. Am. Zool. 40, 246–258. doi: 10.1093/icb/40.2.246
Mommsen, T. P., Vijayan, M. M., and Moon, T. W. (1999). Cortisol in teleosts: dynamic mechanisms of action, and metabolic regulation. Rev. Fish Biol. Fish. 9, 211–268. doi: 10.1023/A:1008924418720
Navarro, I., Rojas, P., Capilla, E., Albalat, A., Castillo, J., Montserrat, N., et al. (2002). Insights into insulin and glucagon responses in fish. Fish Physiol. Biochem. 27, 205–216. doi: 10.1023/B:FISH.0000032726.78074.04
Near, T. J., Dornburg, A., and Friedman, M. (2014). Phylogenetic relationships and timing of diversification in gonorynchiform fishes inferred using nuclear gene DNA sequences (Teleostei: Ostariophysi). Mol. Phylogenet. Evol. 80, 297–307. doi: 10.1016/j.ympev.2014.07.013
Payne, N. L., Smith, J. A., Meulen, D. E., Taylor, M. D., Watanabe, Y. Y., Takahashi, A., et al. (2016). Temperature dependence of fish performance in the wild: links with species biogeography and physiological thermal tolerance. Funct. Ecol. 30, 903–912. doi: 10.1111/1365-2435.12618
Polakof, S., Panserat, S., Sogngas, J. L., and Moon, T. W. (2012). Glucose metabolism in fish: a review. J. Comp. Physiol. B 182, 1015–1045. doi: 10.1007/s00360-012-0658-7
Pörtner, H. O., Peck, L., and Somero, G. (2007). Thermal limits and adaptation in marine Antarctic ectotherms: an integrative view. Phil. Trans. R. Soc. B 362, 2233–2258. doi: 10.1098/rstb.2006.1947
Pörtner, H. O. (2009). Oxygen- and capacity-limitation of thermal tolerance: a matrix for integrating climate-related stressor effects in marine ecosystems. J. Exp. Biol. 213, 881–893. doi: 10.1242/jeb.037523
Sangiao-Alvarellos, S., Arjona, F. J., Martin del Rio, M. P., Miguez, J. M., Mancera, J. M., and Soengas, J. L. (2005). Time course of osmoregulatory and metabolic changes during osmotic acclimation in Sparus auratus. J. Exp. Biol. 208, 4291–4304. doi: 10.1242/jeb.01900
Schram, E., Bierman, S., Teal, L. R., Haenen, O., Vis, H., and Rijnsdorp, A. D. (2013). Thermal preference of juvenile Dover Sole (Solea solea) in relation to thermal acclimation and optimal growth temperature. PLoS ONE 8:e61357. doi: 10.1371/journal.pone.0061357
Singer, T. D., Raptis, S., Sathiyaa, R., Nichols, J. W., Playle, R. C., and Vijayan, M. M. (2007). Tissue-specific modulation of glucocorticoid receptor expression in response to salinity acclimation in rainbow trout. Comp. Biochem. Physiol. B 146, 271–278. doi: 10.1016/j.cbpb.2006.11.010
Soengas, J. L., Barciela, P., Fuentes, J., Otero, J., Andres, M. D., and Aldegunde, M. (1993). The effect of seawater transfer in liver carbohydrate metabolism of domesticated rainbow trout (Oncorhynchus mykiss). Comp. Biochem. Physiol. B 105, 337–343. doi: 10.1016/0305-0491(93)90238-Z
Stanton, R. C. (2012). Glucose-6-phosphat dehydrogenase, NAPDH, and cell survival. IUBMB Lifer. 64, 362–369. doi: 10.1002/iub.1017
Tang, C. H., Wu, W. Y., Tsai, S. C., Yoshinaga, T., and Lee, T. H. (2010). Elevated Na+/K+-ATPase responses and its potential role in triggering ion reabsorption in kidneys for homeostasis of marine euryhaline milkfish (Chanos chanos) when acclimated to hypotonic fresh water. J. Comp. Physiol. B 180, 813–824. doi: 10.1007/s00360-010-0458-x
Tseng, Y. C., Huang, C. J., Chang, C. H., Teng, W. Y., Baba, O., Fann, M. J., et al. (2007). Glycogen phosphorylase in glycogen-rich cells is involved in the energy supply for ion regulation in fish gill epithelia. Am. J. Physiol. Regul. Integr. Comp. Physiol. 293, 482–491. doi: 10.1152/ajpregu.00681.2006
Varis, J., Haverinen, J., and Vornanen, M. (2016). Lowering temperature is the trigger for glycogen build-up and winter fasting in crucian carp (Carassius carassius). Zoolog. Sci. 33, 83–91. doi: 10.2108/zs150072
Vornanen, M., and Haverinen, J. (2011). Seasonality of glycogen phosphorylase activity in crucian carp (Carassius carassius L.). J. Comp. Physiol. B 181, 917–926. doi: 10.1007/s00360-011-0580-4
Wu, S. M., Liu, J. H., Shu, L. H., and Chen, C. H. (2015). Anti-oxidative response of zebrafish (Danio rerio) gill, liver and brain tissues upon acute cold shock. Comp. Biochem. Physiol. A 187, 202–213. doi: 10.1016/j.cbpa.2015.05.016
Keywords: glycogen phosphorylase, milkfish, liver, low temperature, seawater, fresh water
Citation: Chang C-H, Huang J-J, Yeh C-Y, Tang C-H, Hwang L-Y and Lee T-H (2018) Salinity Effects on Strategies of Glycogen Utilization in Livers of Euryhaline Milkfish (Chanos chanos) under Hypothermal Stress. Front. Physiol. 9:81. doi: 10.3389/fphys.2018.00081
Received: 12 November 2017; Accepted: 23 January 2018;
Published: 12 February 2018.
Edited by:
Leonardo Julián Magnoni, Centro Interdisciplinar de Pesquisa Marine e Ambiental (CIIMAR), PortugalReviewed by:
Ivan Viegas, University of Coimbra, PortugalJuan Antonio Martos-Sitcha, Institute of Aquaculture Torre de la Sal (CSIC), Spain
Copyright © 2018 Chang, Huang, Yeh, Tang, Hwang and Lee. This is an open-access article distributed under the terms of the Creative Commons Attribution License (CC BY). The use, distribution or reproduction in other forums is permitted, provided the original author(s) and the copyright owner are credited and that the original publication in this journal is cited, in accordance with accepted academic practice. No use, distribution or reproduction is permitted which does not comply with these terms.
*Correspondence: Tsung-Han Lee, dGhsZWVAZW1haWwubmNodS5lZHUudHc=