- 1Exercise Biology Group, Faculty of Sport and Health Sciences, Technical University of Munich, Munich, Germany
- 2German Mouse Clinic, Institute of Experimental Genetics, Helmholtz Zentrum München, Neuherberg, Germany
- 3Chair of Experimental Genetics, School of Life Science Weihenstephan, Technische Universität München, Freising, Germany
- 4German Center for Diabetes Research (DZD), Neuherberg, Germany
Skeletal muscle mass differs greatly in mice and humans and this is partially inherited. To identify muscle hypertrophy candidate genes we conducted a systematic review to identify genes whose experimental loss or gain-of-function results in significant skeletal muscle hypertrophy in mice. We found 47 genes that meet our search criteria and cause muscle hypertrophy after gene manipulation. They are from high to small effect size: Ski, Fst, Acvr2b, Akt1, Mstn, Klf10, Rheb, Igf1, Pappa, Ppard, Ikbkb, Fstl3, Atgr1a, Ucn3, Mcu, Junb, Ncor1, Gprasp1, Grb10, Mmp9, Dgkz, Ppargc1a (specifically the Ppargc1a4 isoform), Smad4, Ltbp4, Bmpr1a, Crtc2, Xiap, Dgat1, Thra, Adrb2, Asb15, Cast, Eif2b5, Bdkrb2, Tpt1, Nr3c1, Nr4a1, Gnas, Pld1, Crym, Camkk1, Yap1, Inhba, Tp53inp2, Inhbb, Nol3, Esr1. Knock out, knock down, overexpression or a higher activity of these genes causes overall muscle hypertrophy as measured by an increased muscle weight or cross sectional area. The mean effect sizes range from 5 to 345% depending on the manipulated gene as well as the muscle size variable and muscle investigated. Bioinformatical analyses reveal that Asb15, Klf10, Tpt1 are most highly expressed hypertrophy genes in human skeletal muscle when compared to other tissues. Many of the muscle hypertrophy-regulating genes are involved in transcription and ubiquitination. Especially genes belonging to three signaling pathways are able to induce hypertrophy: (a) Igf1-Akt-mTOR pathway, (b) myostatin-Smad signaling, and (c) the angiotensin-bradykinin signaling pathway. The expression of several muscle hypertrophy-inducing genes and the phosphorylation of their protein products changes after human resistance and high intensity exercise, in maximally stimulated mouse muscle or in overloaded mouse plantaris.
Introduction
In humans, skeletal muscle mass, fiber numbers, fiber size, and strength vary greatly. In 18–29 year old women and men muscle mass is 34 ± 6% and 42 ± 4% of the whole body mass, respectively (Janssen et al., 2000). Females on average have a lower muscle mass than males (Janssen et al., 2000) which can partially be explained by low levels of the male sex hormone testosterone, which promotes muscle hypertrophy (Sinha-Hikim et al., 2002). Humans have over 600 muscles and within muscles the number of fibers and their cross sectional area differs greatly. For example, in the vastus lateralis Lexell et al. counted between 393,000 and 903,000 muscle fibers in nine males aged 15–22 years. The average area of type 1 and type 2 fibers per individual ranged from 2,146 to 6,279 μm2 and 2,142 to 5,535 μm2, respectively (Lexell et al., 1988). Similarly, in 1,121,088 males aged 16–25 years the mean elbow flexion strength was 387 ± 84 N, hand grip strength 616 ± 98 N and knee extension strength 569 ± 118 N, respectively. This means that ≈5% of individuals could extend their leg either with a maximal force of either <333 N or more than 805 N (Silventoinen et al., 2008), highlighting the large variation of strength. Muscle mass and function are additionally lost during normal aging (Mitchell et al., 2012) which has been termed sarcopenia (Rosenberg, 1997). In summary, muscle mass and function variables vary greatly in human populations and decline with normal aging.
What are the consequences of this large variation in muscle mass and function? Skeletal muscle is the largest organ in terms of percent of body mass. Muscle takes up circulating glucose, releases amino acids into the circulation during fasting, and low muscle strength is associated with an increased risk of falls (Wolfe, 2006). In a prospective study, researchers additionally found that strength was associated with a significantly higher all-cause and cancer mortality in both individuals below 60 years of age and above 60 years (Ruiz et al., 2008). Thus, muscle mass and function not only matter for athletic performance but also influence our health, how well we age and how long we live.
What factors influence muscle mass and strength and how much is this influenced by variations in the DNA sequence (i.e., genetics)? As almost all traits, muscle mass and function depend on both nature (i.e., genetics or DNA sequence variation) and nurture, which is environmental factors such as resistance training (American College of Sports Medicine, 2009) and nutrition. In twin and family/sib-pair studies researchers estimated the heritability of strength. The results varied greatly from 0.14 to 0.97 (Peeters et al., 2009) perhaps showing the limitations of studies aimed at estimating heritability. In the largest twin study, elbow, hand grip, and knee extension strength were estimated to be 56, 66, and 61% inherited, respectively (Silventoinen et al., 2008). In the extreme, patients with monogenetic muscle diseases can have very poor muscle function (Kaplan, 2011) whereas some elite athletes in strength and power sports have extreme muscle mass and function.
Transgenic mouse models have helped us to identify genes where variations in the DNA sequence cause disease or influence traits including muscle mass and function. Genetic modifications involve naturally occurring mutations or genetically engineered ones. In 2007 Mario R. Capecchi, Martin J. Evans and Oliver Smithies won the Nobel Prize in Physiology or Medicine “for their discoveries of principles of introducing specific gene modifications in mice by the use of embryonic stem cells” or short transgenic mice technology. In contrast to chemically induced random point mutation generation e.g., by ethylnitrosourea (ENU; Russell et al., 1979), transgenic methods are designed to make genes non-functional (i.e., knock out/down or loss-of-function) or to increase the function or expression of a gene (i.e., knock in or gain-of-function). The added DNA might be inserted randomly or to targeted sequences. The transgene construct could contain at minimum a defined promotor (widespread or skeletal muscle/tissue-specific), start and stop codons as well as ribosomal recognition sites, and typically also includes selection markers such as antibiotics resistance or reporter genes like β-galactosidase (lacZ). Gene targeting in mice makes use of homologous recombination which exchanges endogenous DNA with a modified DNA sequence. To further specify gene inactivation at a given time point or in defined tissues conditional mutagenesis is performed e.g., using the Cre/loxP recombination system (Nagy, 2000). Cre is a recombinase from the bacteriophage P1 that mediates excision of gene sequences between loxP sites introduced to the gene of interest by homologous recombination (Turan and Bode, 2011). There is a variety of tissue-specific and drug-inducible Cre lines available allowing temporal and spatial gene function analysis. The next generation of transgenesis methods makes use of engineered nucleases targeting directly the gene of interest thus reducing time and cost for mouse breeding. These include Zinc-finger nucleases (ZFNs; Maeder et al., 2008), Transcription Activator-Like Effector Nucleases (TALENs; Engler et al., 2008) and the CRISPR-Cas system (Cong et al., 2013; Mali et al., 2013). Especially CRISPR-Cas allows researchers to modify several genes which mimics multigenic human diseases and phenotypes. In addition, adeno-associated virus (AAV) vectors are used for in vivo gene transfer and are already applied for human gene therapy also in muscle (for review see Boisgerault and Mingozzi, 2015).
Transgenic methods in mice have led to the discovery of genes whose gain or loss-of-function results in muscle hypertrophy in mice. The most prominent example for this is the myostatin knock out mouse (gene symbol Mstn or Gdf8). A loss of Mstn in mice or humans both roughly doubles muscle mass (McPherron et al., 1997; Schuelke et al., 2004). Thus, genes whose experimentally induced mutation or change of expression/activity affects muscle mass or function in mice are “candidate genes” for muscle mass and function in humans.
To date, there is no systematic compilation of genes whose mutation causes muscle hypertrophy in mice. The aim of this systematic review was therefore to systematically search the literature for published studies where a gain or loss-of-function mutation of a gene induces skeletal muscle hypertrophy. Through this analysis we identify 47 genes whose mutation induces muscle hypertrophy in mice. Additionally, we performed bioinformatical analyses for these 47 genes to determine their expression pattern in human tissues and different muscle fibers as well as to find out whether these genes change their expression or become phosphorylated in response to high intensity and resistance (strength) exercise in human skeletal muscle, maximal mouse muscle contraction and synergist ablation-overloaded mouse plantaris muscle.
Methods
Systematic Literature Search
To identify publications that identify genes whose transgensis results in muscle hypertrophy, we carried out a systematic review according to the PRISMA guidelines (Moher et al., 2009) and searched the literature according to the PICO framework (Schardt et al., 2007). First, we searched PubMed (RRID:SCR_004846) using the following search terms: “mouse AND transgenesis AND (muscle mass OR muscle weight).” This search was repeated in PubReminer (http://hgserver2.amc.nl/cgi-bin/miner/miner2.cgi) to identify more relevant MeSH terms and keywords. From the PubReminer results we selected the search terms shown in Table S1. To narrow the number of studies, we added the search term “AND skeletal muscle” to exclude studies that reported hypertrophy of other tissues. Finally, we searched as follows: “((((((((mice) OR mouse) OR “mouse model”) OR mice transgenic)) AND ((((((gene transfer techniques) OR “overexpression”) OR “knockout”) OR mutagenesis) OR retroviridae) OR gene deletion)) AND (((((((((“muscle mass”) OR hypertrophy) OR “muscle weight”) OR “hypermuscular”) OR “muscle growth”) OR “muscle fiber size”) OR “cross sectional area”) OR hyperplasia) OR phenotype))) AND skeletal muscle.”
We included articles from peer-reviewed journals, written in English, which studied muscle size in gene-manipulated mouse models in vivo. Studies were eligible when no pathologies were reported for the duration of the study as we aimed to identify genes that can potentially be targeted for hypertrophy without causing disease. Also, to exclude confounding factors on muscle growth from, for example, regeneration or muscle loading, gene manipulation had to be the only intervention used in the study. Furthermore, a measure of muscle mass had to be reported (e.g., muscle weight, cross sectional area, muscle diameter, or fiber number). In the case a muscle mass-influencing gene was reported more than once in the literature, only the first mention was included.
We excluded studies where one or more of the following applied:
1) rat or in vitro study,
2) no transgenesis or double mutation or miRNA manipulation,
3) mice showed disease or pathologies or were older than 12 months,
4) no effect, no outcome measures, or muscle atrophy,
5) no use of a wildtype or other control group,
6) not first mention of effect of gene on muscle mass.
From every relevant study we extracted the following information: author, gene name, protein name, method of gene manipulation, output measure, muscle(s) studied, muscle size values for transgenic and control mice, difference between transgenic and control mice in percentage, age of mice, mouse strain, additional measurements, and remarks, and compiled this information in Supplementary Table S2. Often the output measure values did not appear in the text, but in a bar graph. In that case, we manually estimated the relative difference between transgenic and control mouse from the bar graph (indicated in Table 1 with “*”). Also, we adopted the official gene name from Uniprot (RRID:SCR_002380) or Entrez Gene (RRID:SCR_002473). Therefore, it is possible that the mentioned gene name varies from an alias used in the original publication. Finally, note that we write the gene name in lowercase when it is unclear if the source of the overexpressed gene was human or other.
Bioinformatical Analyses
To determine whether the muscle hypertrophy-inducing genes are expressed specifically in skeletal muscle or elsewhere, we retrieved expression figures from the Genotype-Tissue Expression (GTEx; RRID:SCR_001618; GTEx Consortium, 2015) database and pasted the figures into worksheet 1 in Supplementary Table S3.
To compare the expression of muscle hypertrophy-inducing genes in mouse type 1 and type 2b fibers, we downloaded the microarray dataset GSE23244 (Chemello et al., 2011) from Gene Omnibus (RRID:SCR_007303) and retrieved the data with GEO2R. We then copied for all 47 muscle hypertrophy-inducing genes the adjusted p-values (adj.P.Val) and the log fold changes (logFC) into worksheet 2 in Supplementary Table S3. Positive logFC values indicate that a gene is more expressed in type 1 fibers. Negative logFC values indicate that the gene is more expressed in type 2b fibers.
To identify secreted hypertrophy-inducing genes we retrieved a list of genes/proteins that are predicted to be secreted from the Human Protein Atlas (https://www.proteinatlas.org/; Uhlén et al., 2015) and performed an overlap analysis using a web-based tool to identify genes in two lists (http://jura.wi.mit.edu/bioc/tools/compare.php).
To find out whether the 47 muscle hypertrophy-inducing genes interact through direct interaction of the proteins they encode or through functional interaction, we performed a STRING database analysis (Szklarczyk et al., 2015; https://string-db.org/; RRID:SCR_005223) which illustrates such interactions. The data are presented as an interaction figure and as a table in worksheet 3 in Supplementary Table S3.
To identify common functions and other links for the 47 muscle hypertrophy-inducing genes we performed bioinformatical enrichment analyses using DAVID (Huang da et al., 2009; https://david.ncifcrf.gov/summary.jsp; RRID:SCR_001881) and pasted the data into worksheets 4 and 5 in Supplementary Table S3. Subsequently we ordered the raw data so that functional categories and gene ontology, that were significantly enriched, are at the top of the list. For the functional enrichment analysis we used a background list of proteins that are expressed in skeletal muscle (Deshmukh et al., 2015; worksheet 6 in Supplementary Table S3).
To see if the identified genes are linked to human phenotypes we used the GWAS catalog (MacArthur et al., 2017; https://www.ebi.ac.uk/gwas/; RRID:SCR_012745), a website that allows to query Genome-wide association studies.
To study whether hypertrophy-inducing genes change their expression in skeletal muscle after resistance (strength) or endurance exercise, we downloaded the transcriptome microarray dataset GSE59088 (Vissing and Schjerling, 2014) from Gene Omnibus (https://www.ncbi.nlm.nih.gov/geo/; RRID:SCR_007303) and plotted the gene expression data 2.5 h and 5 h after human resistance exercise in relation to pre exercise.
To see how the expression of muscle hypertrophy-inducing genes changes during overload-induced hypertrophy in synergist-ablated mouse plantaris muscle, we retrieved the microarray dataset GSE47098 data from Chaillou et al. (2013). We copied and pasted for all 47 hypertrophy-inducing genes the data into worksheet 7 of Supplementary Table S3 and calculate their expression relative to the unstimulated plantaris (day 0).
We also investigated whether hypertrophy-inducing genes change their phosphorylation after exercise. For this, we downloaded supplementary data from two phosphoproteome studies. The first investigated protein phosphorylation changes after a single bout of high intensity training in human muscle (Hoffman et al., 2015, Supplementary Table S1 of that paper). The second studied protein phosphorylation in mouse skeletal muscle after electrically evoked maximal-intensity contractions (Potts et al., 2017; supporting information file tjp12447-sup-0001-Table S1.xlsx). We downloaded the supplementary files mentioned and copied and pasted the relevant data into worksheet 8 of Supplementary Table S3.
Results
We searched PubMed using our systematic search strategy and identified 1,982 papers with publication dates until June 2017. Based on the title or abstract we excluded 1,861 studies. After this 132 articles remained that were assessed full-text for eligibility. Twenty-seven more articles were identified by reviewing the reference lists of the full-text articles or other sources. Finally, we read 159 full-text and analyzed 45 articles quantitatively. The PRISMA flowchart describing our search and selection is in the Supplementary Material (Figure S1).
Gene Manipulations That Result in Skeletal Muscle Hypertrophy
The 45 analyzed articles report 47 genes whose gain or loss-of-function through transgenesis increased skeletal muscle mass significantly between 5 and 345% (Table 1). To illustrate the muscle hypertrophy-inducing effect of transgenesis in different muscles, we plotted increases in muscle weight per muscle (Figures 1A, 2A), as well as increases in muscle or myofiber cross sectional area (Figures 1B, 2B). Figure 1 shows genes whose gain-of-function increases muscle mass and Figure 2 reports genes whose loss-of-function increases muscle mass. Overexpression of Fst and Ski most increased muscle weight (Figure 1A) and fiber cross sectional area (CSA; Figure 1B), respectively. In contrast, among the knock out genes the loss-of function of Acvr2b and Mstn increased muscle mass most (Figure 2A). The extent of muscle hypertrophy can vary greatly after transgenesis of the same gene. For example, Ucn3 overexpression increases the muscle weight of the soleus by 85% but in the tibialis anterior (TA) only by 20%. Intriguingly, knock out of Esr1 or Nol3 increased muscle mass of the soleus but caused atrophy of the TA or plantaris, respectively (Figures 2A,B).
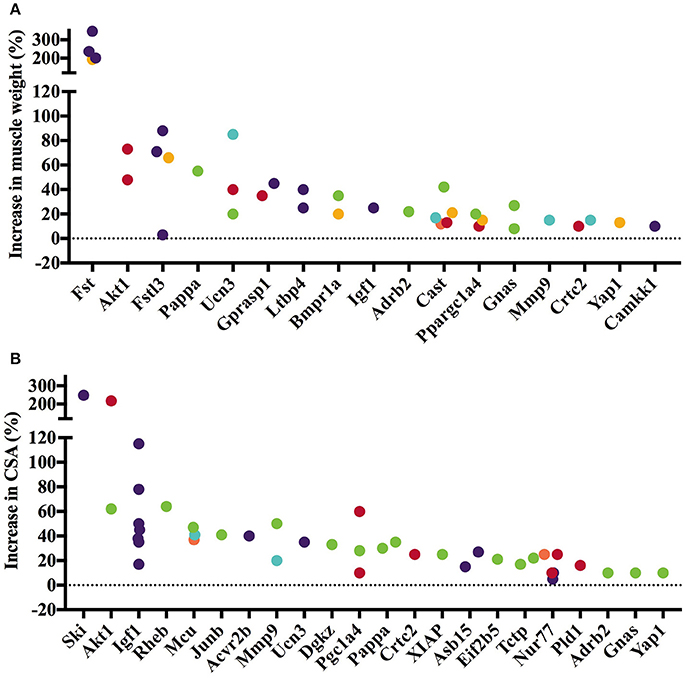
Figure 1. Gene knock-in or overexpression increases muscle weight and cross-sectional area (CSA). Increase in muscle weight (A) and fiber or muscle CSA (B) differs across genes and between muscles. Individual muscles are color-coded as follows: Gastrocnemius, Soleus, Tibialis anterior, Quadriceps, Extensor digitorum longus, Other. Data is based on values collected in Supplementary Table S2.
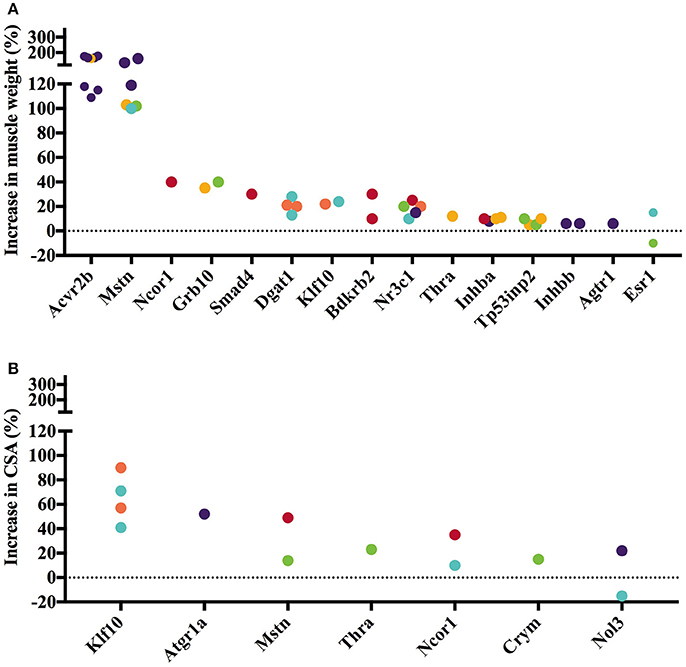
Figure 2. Gene knock out or loss-of-function increases muscle weight and cross-sectional area (CSA). Increase in muscle weight (A) and fiber or muscle CSA (B) after gene knock out differs across genes and between muscles. Individual muscles are color-coded as follows: Gastrocnemius, Soleus, Tibialis anterior, Quadriceps, Extensor digitorum longus, Other. Data is based on values collected in Supplementary Table S2.
In What Tissues and in What Muscle Fibers Are Muscle Hypertrophy-Associated Genes Expressed?
To find out whether the muscle hypertrophy-associated genes are mainly expressed selectively in skeletal muscle, we retrieved tissue-specific gene expression data from the GTEx Portal database (GTEx Consortium, 2015; worksheet 1 in Supplementary Table S3). This analysis revealed that Asb15, Klf10, and Tpt1 were the only genes that were most expressed in skeletal muscle when compared to other human tissues. The genes Cast, Mcu, Mstn, Nol3, and Ppargc1a were highly expressed in skeletal muscle (top 10 of all tissues) whereas the remainder of the genes were generally more expressed in tissues other than skeletal muscle. Together this suggest that only a minority of muscle hypertrophy genes are genes that are highly or specifically expressed in skeletal muscle.
Skeletal muscle is a heterogeneous tissue comprising of slow type 1, intermediate type 2a, and fast 2x and 2b (only in rodents) muscle fibers. To test for muscle fiber-specific gene expression, we retrieved the transcriptome microarray dataset GSE23244 from Gene Omnibus that reported gene expression levels for mouse type 1 and type 2b muscle fibers (Chemello et al., 2011). We could not find expression data for 13 genes. Of the remaining genes, Mstn, Gnas and Nol3 were differently expressed in-between type 1 and type 2b fibers with Mstn being 20-fold more expressed in type 2b fibers whereas Gnas and Nol3 are significantly but moderately more expressed in type 1 fibers.
How Many Hypertrophy Genes Are Predicted to be Secreted?
To identify genes that encode proteins that are predicted to be secreted we downloaded a list of secreted proteins from the Human Protein Atlas (Uhlén et al., 2015) and overlapped them with the list of hypertrophy-inducing genes. This revealed that 11 out of the 47 genes are predicted to be secreted (Fst, Fstl3, Gnas, Igf1, Inhba, Inhbb, Ltbp4, Mmp9, Mstn, Pappa, Ucn3).
Do Muscle Hypertrophy Genes Interact?
We performed a STRING analysis to detect functional association between the 47 hypertrophy-associated genes. This is defined as “a specific and productive functional relationship between two proteins, likely contributing to a common biological purpose” (Szklarczyk et al., 2015). This analysis revealed multiple functional associations that are illustrated in worksheet 3 of Supplementary Table 3. One important functional association cluster is linked to myostatin-Smad signaling and includes the genes Mstn, Fst, Fstl3, Inhba, Inhbb, Acvr2b, Bmpr1a, Smad4, and Ski. Another cluster comprises genes linked to the Igf1-Akt-mTOR signaling network and includes Igf1, Akt1, and Rheb as central members of this network plus other genes that encode proteins that functionally associate. Finally, Agtr1a, Bdkrb2, Adrb2, and Gnas are linked to angiogensin-bradykinin and G-protein coupled receptor signaling. Together this suggests that hypertrophy-regulating genes belong to several signaling systems that are capable of inducing skeletal muscle hypertrophy.
Do the Muscle Hypertrophy Genes Have Similar Functions?
To learn more about the biological functions of the 47 muscle mass-increasing genes in mice, we performed functional enrichment analyses using DAVID (Huang da et al., 2009; RRID:SCR_001881). This analysis revealed that 28% of the muscle hypertrophy-inducing genes are associated with ubiquitination and 21% regulates transcription (worksheet 4 and 5 in Supplementary Table S3).
Are Muscle Hypertrophy Genes Linked to Human Phenotypes in GWAS Studies?
To see if the identified genes are linked to human phenotypes we used the Genome Wide Association Studies (GWAS) catalog (MacArthur et al., 2017; RRID:SCR_012745). No muscle phenotypes in humans are linked with the genes we found. Still, some muscle and growth related associations are found. PPARD, PAPPA, and ESR1 associate with height (Allen et al., 2010; Wood et al., 2014) and PLD1 is linked to the body mass index (BMI Ng et al., 2012). Also, Tpt1, which is highly expressed in skeletal muscle, is associated with type 2 diabetes (Anderson et al., 2015).
Do Muscle Hypertrophy Genes Change Their Expression in Response to Strength (Resistance) Exercise?
To find out whether the muscle hypertrophy-causing genes are induced or repressed by resistance exercise in humans, we accessed transcriptome data from Vissing and Schjerling (2014). This revealed that the expression of some of these genes (IGF1, PPARGC1A, BMPR1A, ASB15, CAST, KLF10, and AGTR1) changes significantly by more than 10% after resistance exercise in human muscle (Figure 3).
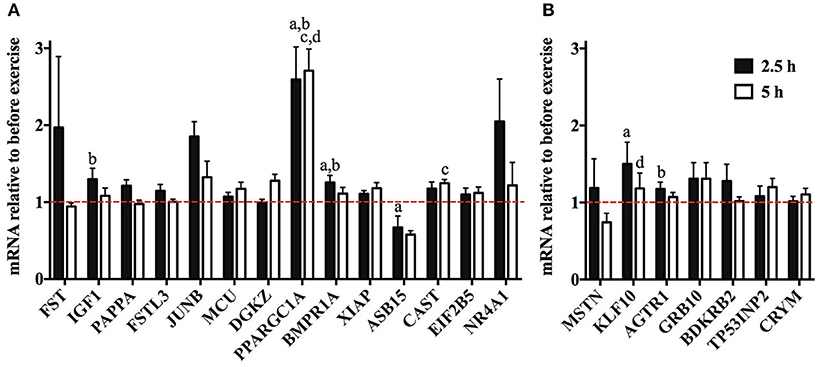
Figure 3. Expression of muscle mass regulating genes after resistance exercise. Transcriptome data GSE23244 from Vissing and Schjerling (2014) was accessed to discover which muscle mass regulating genes are induced or repressed 2.5 h or 5 h relative to resting value after a bout of resistance exercise. (A) Expression of genes whose overexpression or activation increases muscle mass in mice. (B) Expression of genes whose knock out increases muscle mass. Genes whose mean expression increased or decreased by more than 10% are shown in this figure. aDifferent at 2.5 h compared to pre-training, bDifferent compared to control group at 2.5 h, cDifferent at 5 h compared to pre-training, dDifferent compared to control group at 5 h (n = 6). Note that the data for pan PPARGC1A are shown. However, muscle hypertrophy is only stimulated by the PGC-1α4 protein isoform (Ruas et al., 2012).
Does the Expression of Hypertrophy Genes Change During Overload-Induced Hypertrophy in a Way That Is Consistent With Their Function?
To find out how the expression of muscle hypertrophy-increasing genes changes in a mouse plantaris that is hypertrophying because of synergist ablation, we re-analyzed transcriptome data from Chaillou et al. (2013). We expected increased expression of genes whose gain-of-function causes muscle hypertrophy and decreased expression of genes whose loss-of-function causes hypertrophy. Generally, genes whose change-of-function has a large effect on muscle size changed their expression as expected (e.g., Acvr2b, Akt1, Fst, Fstl3, Igf1, and Mstn; Figure 4; Supplementary Table S3, worksheet 7). Of these genes, Mstn expression was one of the genes with the largest drop of expression in the hypertrophying plantaris muscle genome wide. Overall, only roughly half of the hypertrophy-inducing genes changed their expression as expected whereas the other half did change their expression in an unexpected way or their expression remained stable.
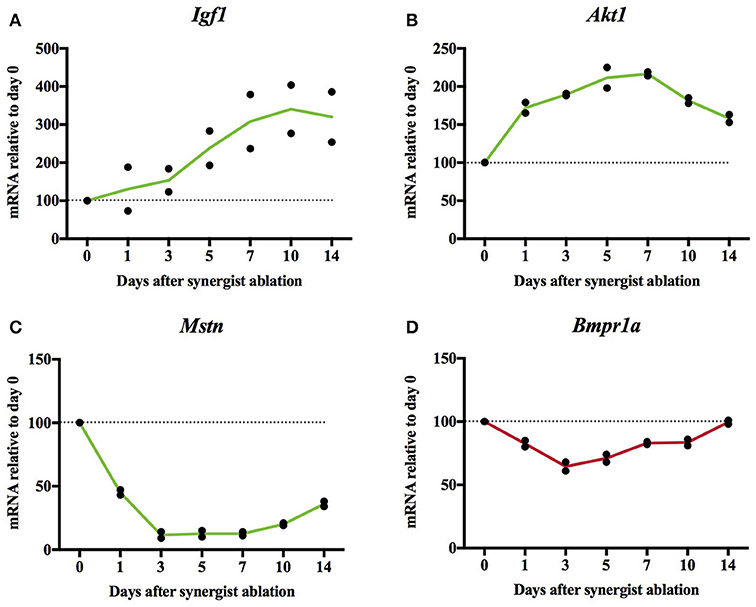
Figure 4. Relative mRNA expression of Igf1 (A), Akt1 (B), Mstn (C), and Bmpr1a (D) in synergist ablation-overloaded mouse plantaris muscle. Igf1, Akt1, Mstn change their expression which is consistent with the hypertrophy of the plantaris. In contrast, Bmpr1a did not change its expression as expected as the overexpression of Bmpr1a causes hypertrophy (Sartori et al., 2013) whilst its expression decreased in the hypertrophying plantaris (see Supplementary Table S3, worksheet 7 for further figures). Original data derived from microarray dataset GSE47098 data from Chaillou et al. (2013).
Do Hypertrophy-Inducing Proteins Change Their Phosphorylation After Human High-Intensity Exercise and Maximal Mouse Muscle Contraction?
Protein phosphorylation is a common mechanism of signal transduction that is essential for many skeletal muscle adaptations to exercise. To test whether hypertrophy-inducing proteins are phosphorylated in muscle and whether their phosphorylation changes in response to human high intensity exercise or maximal mouse muscle contraction, we re-analyzed the phosphoproteomic datasets of Potts et al. (2017) and Hoffman et al. (2015) (Supplementary Table S3, worksheet 8). In their non-biased analyses the authors detected phosphoproteins for the hypertrophy-inducing genes ADRB2, AKT1, CAMKK1, Cast/CAST, CRTC2, Dgkz/DGKZ, Eif2b5, GNAS, GRB10, Ltbp4, Nr3c1, Pld1, and Yap1/YAP1 (lower cases denote the mouse gene symbols from (Potts et al., 2017) and the upper case gene symbols the human gene symbols from Hoffman et al. (2015). However, only the proteins encoded by Cast/CAST, CAMKK1, CAST, and DGKZ significantly changed their phosphorylation in response to mouse muscle stimulation or human high intensity exercise.
Discussion
Through a systematic literature search we identified 47 genes whose genetic manipulation results in significant skeletal muscle hypertrophy in mice when compared to wildtype controls confirming that muscle mass and muscle hypertrophy is a polygenic trait. Of these 47 genes, 18 had to be knocked out (loss-of-function) and 29 were knocked in or overexpressed (gain-of-function) to induce muscle hypertrophy. This shows that muscle mass is regulated by both muscle growth factors and muscle mass inhibitors, which have been termed “chalones” (Lee, 2004). The 47 hypertrophy-inducing genes are candidate genes that encode proteins that are potentially involved in developmental growth, adult muscle mass, hypertrophy in response to resistance (strength) training and feeding, and their dysregulation might contribute to the loss of muscle mass and function during normal aging, termed sarcopenia (Rosenberg, 1997) as well as several other forms of atrophy.
The first example where one of these genes has served as a candidate gene for a human genetic discovery is the Mstn (McPherron et al., 1997) which served as a candidate gene for discoverig a homozygous, intronic MSTN mutation in a hypermuscular child (Schuelke et al., 2004). However, Mstn mutants are also a good example to highlight that increased muscle size does not always translate into proportionally improved muscle function. For example, Mstn loss-of-function mice have more muscle mass but are not stronger than wildtype mice. The consequence is a reduced force-to-muscle mass ratio or muscle quality (Amthor et al., 2007). However, the relation between muscle mass and function appears to be species dependent as Mstn variants in whippet dogs are associated with increased running performance when compared to wildtype dogs (Mosher et al., 2007). Also the anecdotal evidence from the human MSTN knockout suggests that MSTN loss-of-function carriers had not only increased muscle mass but also more strength (Schuelke et al., 2004).
To further study the 47 muscle hypertrophy-inducing genes, we performed several bioinformatical analyses to learn more about the tissue-specific expression, functional association, and phenotype association as well as their response to resistance (strength) exercise, and synergist ablation-induced hypertrophy. Together this dataset gives an update on the genetics of skeletal muscle hypertrophy that adds to narrative reviews on the regulation of skeletal muscle mass (Schiaffino et al., 2013; Egerman and Glass, 2014; Marcotte et al., 2015). Whilst this is a systematic review, it is not unbiased as the authors of the original papers subjectively chose genes for mouse transgenesis. Here, the International Mouse Phenotyping Consortium (IMPC) might help to extend the list of hypertrophy-inducing genes in a less biased fashion. The aim of the IMPC is to generate knock out mice for 20.000 known or predicted mouse genes (http://www.mousephenotype.org/). These knock out mice are subjected to an extensive pipeline of phenotyping tests in order to discover the biological functions of each gene (Brown and Moore, 2012; Dickinson et al., 2016). In the IMPC phenotype pipeline skeletal muscle hypertrophy, muscle mass and fiber size are not directly measured but investigations of embryos, body weight, body composition as well as grip strength are phenotyping measures that can suggest skeletal muscle hypertrophy which can then be followed up with a specific skeletal muscle analysis.
One key question is whether the muscle hypertrophy associated genes belong to one major muscle hypertrophy pathway, to several, or whether most genes are functionally unrelated. To answer this question, we bioinformatically assessed human tissue-specific (GTEx Portal) and mouse muscle fiber-specific gene expression (Chemello et al., 2011), functional associations (STRING analysis) as well as a functional enrichment analysis using DAVID to identify commonalities among hypertrophy-inducing genes (Huang da et al., 2009). Only three genes (Asb15, Klf10, Tpt1) are most expressed in skeletal muscle and of all 47 genes only Asb15 seems to be selectively expressed in skeletal and cardiac muscle. Mstn is generally expressed at low levels and selectively in fast type 2 muscle fibers (Chemello et al., 2011). Most other genes are most expressed in tissues other than muscle. Collectively this suggests that muscle hypertrophy-inducing genes are rarely specifically expressed in skeletal muscle but are more often expressed in multiple tissues or mainly in other tissues. This is important information for identifying muscle atrophy drug targets as the targeting of skeletal muscle-specific genes and proteins makes side effects less likely. Therapeutically relevant is also the finding that 11 out of the 47 genes are predicted to be secreted (Fst, Fstl3, Gnas, Igf1, Inhba, Inhbb, ltbp4, Mmp9, Mstn, Papa, Ucn3) as secreted proteins can be targeted better than intracellular proteins.
Analyzing functional associations with the functional association search programme STRING points to three pathways whose genes can induce muscle hypertrophy. They are the (a) Igf1-Akt-mTOR (Marcotte et al., 2015), (b) myostatin-Smad (Sartori and Sandri, 2015), and (c) angiotensin-bradykinin signaling pathways. Whilst it is extensively shown that Igf1-Akt-mTOR and myostatin-Smad signaling regulate muscle mass, there is less information on a link between angiotensin-bradykinin signaling and muscle mass. In humans, the angiotensin-converting enzyme ACE D deletion allele was associated with greater strength gain after resistance training but the study only involved 33 subjects (Folland et al., 2000). Also, an ACE inhibitor attenuated load-induced muscle hypertrophy in rats (Gordon et al., 2001) suggesting that the angiotensin-bradykinin system is involved in regulating muscle size. Still, Igf1-Akt-mTOR, myostatin-Smad and angiotension-bradykinin signaling are not the only pathways contributing to muscle growth. A growth stimulus from resistance, or high intensity exercise, increases transcription of many genes that do not have a direct link with these pathways (Vissing and Schjerling, 2014; Hoffman et al., 2015).
In addition to the 47 genes we identified, IGSF1 might also be a candidate gene for rare and common DNA variants influencing muscle mass. Recently, a gene wide association study has linked DNA variation of the IGSF1 gene to body size in dog breeds (Plassais et al., 2017). This is of interest because a single nucleotide variation in the IGSF9B locus was associated with human hand grip strength (Willems et al., 2017).
Resistance (strength) exercise is a key intervention to promote skeletal muscle hypertrophy (Schoenfeld, 2010). To find out whether the 47 muscle hypertrophy-inducing genes are potential regulators of the muscle hypertrophy response to resistance exercise, we re-analyzed a dataset of gene expression data obtained pre, 2.5 and 5 h after a bout of resistance exercise (Vissing and Schjerling, 2014). This revealed that IGF1, PPARGC1A, BMPR1A, ASB15, CAST, KLF10, and AGTR1 are significantly altered by resistance exercise (Figure 3; note that muscle hypertrophy is only stimulated by the PGC-1α4 isoform Ruas et al., 2012). Interestingly, while KLF10 and AGTR1 expression increases after a single bout of resistance exercise, we identified that knock out of these genes in mice increases muscle mass (Kammoun et al., 2016; Zempo et al., 2016). Similarly, ASB15 is repressed by resistance exercise and in the synergist ablation-overloaded plantaris whilst its overexpression results in muscle hypertrophy in mice (McDaneld et al., 2006). Thus, the direction of the change of the expression of hypertrophy-inducing genes at 2.5 and 5 h after resistance exercise is not always consistent with their function.
A chronic overload-induced muscle hypertrophy model is synergist ablation. Here, we re-analyzed the data of the synergist ablation-overloaded plantaris from Chaillou et al. (2013) which measured gene expression at days 1, 3, 5, 7, 10, and 14 which gives a detailed time course of gene expression during a hypertrophy stimulus. We found that genes such as Akt1, Igf1, and Mstn whose gain-or-loss of function has a large effect on muscle size changed their expression as predicted. This suggests that many hypertrophy-inducing genes contribute to synergist ablation-induced hypertrophy through a regulation of their gene expression. However, other genes such as Bmpr1a (Figure 4), change their expression not as expected (e.g., increases of the expression of genes whose loss-of-function causes muscle hypertrophy) or remain relatively stable. Some hypertrophy-inducing proteins are also phosphorylated in skeletal muscle and the proteins encoded by Cast/CAST, CAMKK1, CAST, and DGKZ significantly change their phosphorylation in response to high intensity exercise or maximal muscle contraction.
Thalacker-Mercer et al. (2013) compared gene expression with the response to resistance exercise and found that the hypertrophy-inducing genes DGKZ, MSTN, IGF1, ESR1, ACVR2Bb, SKI, and AKT1 are differentially expressed in extreme responders vs. non-responders. Genetic variability between individuals likely determines the muscle adaptation to exercise. For instance, it has been shown that gains in lean mass, muscle fiber/muscle hypertrophy, and power in humans are associated with DNA sequence variants of the ACE gene (Montgomery et al., 1999; Valdivieso et al., 2017).
Limitations of this systematic review are the strict inclusion and exclusion criteria determined before collecting the relevant literature. As a result of our search strategy we might have missed relevant studies. To identify relevant papers for our review we initially only screened abstracts and titles. Studies that observed a muscle phenotype, but failed to report this in the title or abstract are possibly overlooked. We decided using only PubMed to identify papers for our review, however, additional databases could have given extra results. We excluded double knock out studies which sometimes can provide important insight. For example, the combined knock out of Mstn and the expression of a Fst transgene roughly quadrupled muscle weight suggesting that the hypertrophy-inducing genes can have additive effects (Lee, 2007). Also we did not include chemically induced or naturally occurring mutants nor did we include studies where muscle hypertrophy was accompanied by a pathological phenotype. Note, that although we excluded genes whose manipulation was confounded by a pathology, we acknowledge that in this case the manipulated gene can still play a role in muscle hypertrophy. A follow-up literature evaluation for the identified hypertrophy genes was not performed. We only assessed the original papers that report an effect on muscle mass after gene gain or loss-of-function. For example, we did not assess whether studies other than the original one, report pathologies such as increased fibrosis or decreased life span. Most muscle phenotypes are determined at 2–3 months of age (Supplementary Table S2) and decreased life span for example would not become apparent. Also, we do not know whether the increases in muscle mass reported in the studies are maintained through life.
Summary and Conclusion
In summary, we found 47 genes whose transgenesis in mice results in muscle hypertrophy. These genes are candidate genes for rare and common DNA variants influencing human muscle mass. They also encode proteins that are potential targets for muscle addressing drug discovery, especially when secreted or expressed in a muscle-specific fashion. Most hypertrophy-inducing genes do not appear to change their expression or phosphorylation in a hypertrophy-promoting direction in the minutes and hours after human resistance and high intensity exercise. This is different, however, during day 1–14 of plantaris overload through synergist ablation. Here, especially hypertrophy-inducing genes with a large effect size such as Akt1, Igf1, and Mstn change their expression in a direction that is consistent with their involvement in hypertrophy regulation (Chaillou et al., 2013).
Author Contributions
HW and LB conceptualized review. SV and FN conducted systematic literature search. SV performed literature analysis. MS screened bioinformatic databases. SV and HW drafted manuscript. SV, HW, LB, and MHdA edited manuscript.
Conflict of Interest Statement
The authors declare that the research was conducted in the absence of any commercial or financial relationships that could be construed as a potential conflict of interest.
Acknowledgments
This work was supported by the German Research Foundation (DFG) and the Technical University of Munich within the Open Access Publishing Funding Programme and the German Federal Ministry of Education and Research (Infrafrontier grant 01KX1012).
Supplementary Material
The Supplementary Material for this article can be found online at: https://www.frontiersin.org/articles/10.3389/fphys.2018.00553/full#supplementary-material
References
American College of Sports Medicine (2009). Progression models in resistance training for healthy adults. Med. Sci. Sports Exerc. 41, 687–708. doi: 10.1249/MSS.0b013e3181915670
Amthor, H., Macharia, R., Navarrete, R., Schuelke, M., Brown, S. C., Otto, A., et al. (2007). Lack of myostatin results in excessive muscle growth but impaired force generation. Proc. Natl. Acad. Sci. U.S.A. 104, 1835–1840. doi: 10.1073/pnas.0604893104
Anderson, D., Cordell, H. J., Fakiola, M., Francis, R. W., Syn, G., Scaman, E. S., et al. (2015). First genome-wide association study in an Australian aboriginal population provides insights into genetic risk factors for body mass index and type 2 diabetes. PLoS ONE 10:e0119333. doi: 10.1371/journal.pone.0119333
Bakkar, N., Wang, J., Ladner, K. J., Wang, H., Dahlman, J. M., Carathers, M., et al. (2008). IKK/NF-kappaB regulates skeletal myogenesis via a signaling switch to inhibit differentiation and promote mitochondrial biogenesis. J. Cell Biol. 180, 787–802. doi: 10.1083/jcb.200707179
Bodine, S. C., Stitt, T. N., Gonzalez, M., Kline, W. O., Stover, G. L., Bauerlein, R., et al. (2001). Akt/mTOR pathway is a crucial regulator of skeletal muscle hypertrophy and can prevent muscle atrophy in vivo. Nat. Cell Biol. 3, 1014–1019. doi: 10.1038/ncb1101-1014
Boisgerault, F., and Mingozzi, F. (2015). The skeletal muscle environment and its role in immunity and tolerance to AAV vector-mediated gene transfer. Curr. Gene Ther. 15, 381–394. doi: 10.2174/1566523215666150630121750
Brown, M., Ning, J., Ferreira, J. A., Bogener, J. L., and Lubahn, D. B. (2009). Estrogen receptor-α and -β and aromatase knockout effects on lower limb muscle mass and contractile function in female mice. Am. J. Physiol. Endocrinol. Metab. 296, E854–E861. doi: 10.1152/ajpendo.90696.2008
Brown, S. D., and Moore, M. W. (2012). The international mouse phenotyping consortium: past and future perspectives on mouse phenotyping. Mamm. Genome 23, 632–640. doi: 10.1007/s00335-012-9427-x
Bruno, N. E., Kelly, K. A., Hawkins, R., Bramah-Lawani, M., Amelio, A. L., Nwachukwu, J. C., et al. (2014). Creb coactivators direct anabolic responses and enhance performance of skeletal muscle. EMBO J. 33, 1027–1043. doi: 10.1002/embj.201386145
Chaillou, T., Lee, J. D., England, J. H., Esser, K. A., and McCarthy, J. J. (2013). Time course of gene expression during mouse skeletal muscle hypertrophy. J. Appl. Physiol. 115, 1065–1074. doi: 10.1152/japplphysiol.00611.2013
Chemello, F., Bean, C., Cancellara, P., Laveder, P., Reggiani, C., and Lanfranchi, G. (2011). Microgenomic analysis in skeletal muscle: expression signatures of individual fast and slow myofibers. PLoS ONE 6:e16807. doi: 10.1371/journal.pone.0016807
Coleman, M. E., DeMayo, F., Yin, K. C., Lee, H. M., Geske, R., Montgomery, C., et al. (1995). Myogenic vector expression of insulin-like growth factor I stimulates muscle cell differentiation and myofiber hypertrophy in transgenic mice. J. Biol. Chem. 270, 12109–12116. doi: 10.1074/jbc.270.20.12109
Cong, L., Ran, F. A., Cox, D., Lin, S., Barretto, R., Habib, N., et al. (2013). Multiplex genome engineering using CRISPR/Cas systems. Science 339, 819–823. doi: 10.1126/science.1231143
GTEx Consortium (2015). Human genomics. The Genotype-Tissue Expression (GTEx) pilot analysis: multitissue gene regulation in humans. Science 348, 648–660. doi: 10.1126/science.1262110
Dahiya, S., Bhatnagar, S., Hindi, S. M., Jiang, C., Paul, P. K., Kuang, S., et al. (2011). Elevated levels of active matrix metalloproteinase-9 cause hypertrophy in skeletal muscle of normal and dystrophin-deficient mdx mice. Hum. Mol. Genet. 20, 4345–4359. doi: 10.1093/hmg/ddr362
de Picoli Souza, K., Batista, E. C., Silva, E. D., Reis, F. C., Silva, S. M., Araujo, R. C., et al. (2010). Effect of kinin B2 receptor ablation on skeletal muscle development and myostatin gene expression. Neuropeptides 44, 209–214. doi: 10.1016/j.npep.2009.12.001
Deshmukh, A. S., Murgia, M., Nagaraj, N., Treebak, J. T., Cox, J., and Mann, M. (2015). Deep proteomics of mouse skeletal muscle enables quantitation of protein isoforms, metabolic pathways, and transcription factors. Mol. Cell. Proteomics 14, 841–853. doi: 10.1074/mcp.M114.044222
Dickinson, M. E., Flenniken, A. M., Ji, X., Teboul, L., Wong, M. D., White, J. K., et al. (2016). High-throughput discovery of novel developmental phenotypes. Nature 537, 508–514. doi: 10.1038/nature19356
Egerman, M. A., and Glass, D. J. (2014). Signaling pathways controlling skeletal muscle mass. Crit. Rev. Biochem. Mol. Biol. 49, 59–68. doi: 10.3109/10409238.2013.857291
Engler, C., Kandzia, R., and Marillonnet, S. (2008). A one pot, one step, precision cloning method with high throughput capability. PLoS ONE 3:e3647. doi: 10.1371/journal.pone.0003647
Ferey, J. L. A., Brault, J. J., Smith, C. A. S., and Witczak, C. A. (2014). Constitutive activation of CaMKKα signaling is sufficient but not necessary for mTORC1 activation and growth in mouse skeletal muscle. Am. J. Physiol. Endocrinol. Metab. 307, E686–E694. doi: 10.1152/ajpendo.00322.2014
Folland, J., Leach, B., Little, T., Hawker, K., Myerson, S., Montgomery, H., et al. (2000). Angiotensin-converting enzyme genotype affects the response of human skeletal muscle to functional overload. Exp. Physiol. 85, 575–579. doi: 10.1111/j.1469-445X.2000.02057.x
Goodman, C. A., Coenen, A. M., Frey, J. W., You, J.-S., Barker, R. G., Frankish, B. P., et al. (2017). Insights into the role and regulation of TCTP in skeletal muscle. Oncotarget 8, 18754–18772. doi: 10.18632/oncotarget.13009
Goodman, C. A., Miu, M. H., Frey, J. W., Mabrey, D. M., Lincoln, H. C., Ge, Y., et al. (2010). A phosphatidylinositol 3-kinase/protein kinase B-independent activation of mammalian target of rapamycin signaling is sufficient to induce skeletal muscle hypertrophy. Mol. Biol. Cell 21, 3258–3268. doi: 10.1091/mbc.E10-05-0454
Gordon, S. E., Davis, B. S., Carlson, C. J., and Booth, F. W. (2001). ANG II is required for optimal overload-induced skeletal muscle hypertrophy. Am. J. Physiol. Endocrinol. Metab. 280, 150–159. doi: 10.1152/ajpendo.2001.280.1.E150
Hagg, A., Colgan, T. D., Thomson, R. E., Qian, H., Lynch, G. S., and Gregorevic, P. (2016). Using AAV vectors expressing the β2-adrenoceptor or associated Gα proteins to modulate skeletal muscle mass and muscle fibre size. Sci. Rep. 6:23042. doi: 10.1038/srep23042
Hoffman, N. J., Parker, B. L., Chaudhuri, R., Fisher-Wellman, K. H., Kleinert, M., Humphrey, S. J., et al. (2015). Global phosphoproteomic analysis of human skeletal muscle reveals a network of exercise-regulated kinases and AMPK substrates. Cell Metab. 22, 922–935. doi: 10.1016/j.cmet.2015.09.001
Hu, J., Du, J., Zhang, L., Price, S. R., Klein, J. D., and Wang, X. H. (2010). XIAP reduces muscle proteolysis induced by CKD. J. Am. Soc. Nephrol. 21, 1174–1183. doi: 10.1681/ASN.2009101011
Huang, da W, Sherman, B. T., and Lempicki, R. A. (2009). Systematic and integrative analysis of large gene lists using DAVID bioinformatics resources. Nat. Protoc. 4, 44–57. doi: 10.1038/nprot.2008.211
Jaafar, R., De Larichaudy, J., Chanon, S., Euthine, V., Durand, C., Naro, F., et al. (2013). Phospholipase D regulates the size of skeletal muscle cells through the activation of mTOR signaling. Cell Commun. Signal. 11:55. doi: 10.1186/1478-811X-11-55
Jamieson, P. M., Cleasby, M. E., Kuperman, Y., Morton, N. M., Kelly, P. A., Brownstein, D. G., et al. (2011). Urocortin 3 transgenic mice exhibit a metabolically favourable phenotype resisting obesity and hyperglycaemia on a high-fat diet. Diabetologia 54, 2392–2403. doi: 10.1007/s00125-011-2205-6
Janssen, I., Heymsfield, S. B., Wang, Z. M., and Ross, R. (2000). Skeletal muscle mass and distribution in 468 men and women aged 18–88 yr. J. Appl. Physiol. 89, 81–88. doi: 10.1152/jappl.2000.89.1.81
Kammoun, M., Pouletaut, P., Canon, F., Subramaniam, M., Hawse, J. R., Vayssade, M., et al. (2016). Impact of TIEG1 deletion on the passive mechanical properties of fast and slow twitch skeletal muscles in female mice. PLoS ONE 11:e0164566. doi: 10.1371/journal.pone.0164566
Kaplan, J.-C. (2011). The 2012 version of the gene table of monogenic neuromuscular disorders. Neuromuscul. Disord. 21, 833–861. doi: 10.1016/j.nmd.2011.10.008
Lai, K. M., Gonzalez, M., Poueymirou, W. T., Kline, W. O., Na, E., Zlotchenko, E., et al. (2004). Conditional activation of akt in adult skeletal muscle induces rapid hypertrophy. Mol. Cell. Biol. 24, 9295–9304. doi: 10.1128/MCB.24.21.9295-9304.2004
Lango Allen, H., Estrada, K., Lettre, G., Berndt, S. I., Weedon, M. N., Rivadeneira, F., et al. (2010). Hundreds of variants clustered in genomic loci and biological pathways affect human height. Nature 467, 832–838. doi: 10.1038/nature09410
Lamar, K.-M., Bogdanovich, S., Gardner, B. B., Gao, Q. Q., Miller, T., Earley, J. U., et al. (2016). Overexpression of latent TGFβ binding protein 4 in muscle ameliorates muscular dystrophy through myostatin and TGFβ. PLoS Genet. 12:e1006019. doi: 10.1371/journal.pgen.1006019
Lee, S. J. (2007). Quadrupling muscle mass in mice by targeting TGF-beta signaling pathways. PLoS ONE 2:e789. doi: 10.1371/journal.pone.0000789
Lee, S.-J. (2004). Regulation of muscle mass by myostatin. Annu. Rev. Cell Dev. Biol. 20, 61–86. doi: 10.1146/annurev.cellbio.20.012103.135836
Lee, S.-J., Lee, Y.-S., Zimmers, T. A., Soleimani, A., Matzuk, M. M., Tsuchida, K., et al. (2010). Regulation of muscle mass by follistatin and activins. Mol. Endocrinol. 24, 1998–2008. doi: 10.1210/me.2010-0127
Lee, S. J., and McPherron, A. C. (2001). Regulation of myostatin activity and muscle growth. Proc. Natl. Acad. Sci. U.S.A. 98, 9306–9311. doi: 10.1073/pnas.151270098
Lexell, J., Taylor, C. C., and Sjöström, M. (1988). What is the cause of the ageing atrophy? J. Neurol. Sci. 84, 275–294. doi: 10.1016/0022-510X(88)90132-3
Liu, L., Yu, S., Khan, R. S., Ables, G. P., Bharadwaj, K. G., Hu, Y., et al. (2011). DGAT1 deficiency decreases PPAR expression and does not lead to lipotoxicity in cardiac and skeletal muscle. J. Lipid Res. 52, 732–744. doi: 10.1194/jlr.M011395
Luquet, S., Lopez-Soriano, J., Holst, D., Fredenrich, A., Melki, J., Rassoulzadegan, M., et al. (2003). Peroxisome proliferator-activated receptor delta controls muscle development and oxidative capability. FASEB J. 17, 2299–2301. doi: 10.1096/fj.03-0269fje
MacArthur, J., Bowler, E., Cerezo, M., Gil, L., Hall, P., Hastings, E., et al. (2017). The new NHGRI-EBI catalog of published genome-wide association studies (GWAS Catalog). Nucleic Acids Res. 45, D896–D901. doi: 10.1093/nar/gkw1133
Maeder, M. L., Thibodeau-Beganny, S., Osiak, A., Wright, D. A., Anthony, R. M., Eichtinger, M., et al. (2008). Rapid “open-source” engineering of customized zinc-finger nucleases for highly efficient gene modification. Mol. Cell 31, 294–301. doi: 10.1016/j.molcel.2008.06.016
Mali, P., Yang, L., Esvelt, K. M., Aach, J., Guell, M., DiCarlo, J. E., et al. (2013). RNA-guided human genome engineering via Cas9. Science 339, 823–826. doi: 10.1126/science.1232033
Mammucari, C., Gherardi, G., Zamparo, I., Raffaello, A., Boncompagni, S., Chemello, F., et al. (2015). The mitochondrial calcium uniporter controls skeletal muscle trophism in vivo. Cell Rep. 10, 1269–1279. doi: 10.1016/j.celrep.2015.01.056
Marcotte, G. R., West, D. W., and Baar, K. (2015). The molecular basis for load-induced skeletal muscle hypertrophy. Calcif. Tissue Int. 96, 196–210. doi: 10.1007/s00223-014-9925-9
Mayhew, D. L., Hornberger, T. A., Lincoln, H. C., and Bamman, M. M. (2011). Eukaryotic initiation factor 2B epsilon induces cap-dependent translation and skeletal muscle hypertrophy. J. Physiol. 589(Pt 12), 3023–3037. doi: 10.1113/jphysiol.2010.202432
McDaneld, T. G., Hannon, K., and Moody, D. E. (2006). Ankyrin repeat and SOCS box protein 15 regulates protein synthesis in skeletal muscle. Am. J. Physiol. Regul. Integr. Comp. Physiol. 290, R1672–R1682. doi: 10.1152/ajpregu.00239.2005
McPherron, A. C., Lawler, A. M., and Lee, S. J. (1997). Regulation of skeletal muscle mass in mice by a new TGF-β superfamily member. Nature 387, 83–90. doi: 10.1038/387083a0
Mitchell, A. S., Smith, I. C., Gamu, D., Donath, S., Tupling, A. R., and Quadrilatero, J. (2015). Functional, morphological, and apoptotic alterations in skeletal muscle of ARC deficient mice. Apoptosis 20, 310–326. doi: 10.1007/s10495-014-1078-9
Mitchell, W. K., Williams, J., Atherton, P., Larvin, M., Lund, J., and Narici, M. (2012). Sarcopenia, dynapenia, and the impact of advancing age on human skeletal muscle size and strength; a quantitative review. Front. Physiol. 3:260. doi: 10.3389/fphys.2012.00260
Moher, D., Liberati, A., Tetzlaff, J., and Altman, D., and The PRISMA Group. (2009). Preferred reporting items for systematic reviews and meta-analysis: the PRISMA statement. PLoSMed. 151, 264–269. doi: 10.1371/journal.pmed.1000097
Monestier, O., Brun, C., Heu, K., Passet, B., Malhouroux, M., Magnol, L., et al. (2012). Ubiquitous Gasp1 overexpression in mice leads mainly to a hypermuscular phenotype. BMC Genomics 13:541. doi: 10.1186/1471-2164-13-541
Montgomery, H., Clarkson, P., Barnard, M., Bell, J., Brynes, A., Dollery, C., et al. (1999). Angiotensin-converting-enzyme gene insertion/deletion polymorphism and response to physical training. Lancet 353, 541–545. doi: 10.1016/S0140-6736(98)07131-1
Mosher, D. S., Quignon, P., Bustamante, C. D., Sutter, N. B., Mellersh, C. S., Parker, H. G., et al. (2007). A mutation in the myostatin gene increases muscle mass and enhances racing performance in heterozygote dogs. PLoS Genet. 3:e79. doi: 10.1371/journal.pgen.0030079
Musarò, A., McCullagh, K., Paul, A., Houghton, L., Dobrowolny, G., Molinaro, M., et al. (2001). Localized Igf-1 transgene expression sustains hypertrophy and regeneration in senescent skeletal muscle. Nat. Genet. 27, 195–200. doi: 10.1038/84839
Nagy, A. (2000). Cre recombinase: the universal reagent for genome tailoring. Genesis 26, 99–109. doi: 10.1002/(SICI)1526-968X(200002)26:2<99::AID-GENE1>3.0.CO;2-B
Ng, M. C., Hester, J. M., Wing, M. R., Li, J., Xu, J., Hicks, P. J., et al. (2012). Genome-wide association of BMI in African Americans. Obesity 20, 622–627. doi: 10.1038/oby.2011.154
Otani, K., Han, D.-H., Ford, E. L., Garcia-Roves, P. M., Ye, H., Horikawa, Y., et al. (2004). Calpain system regulates muscle mass and glucose transporter GLUT4 turnover. J. Biol. Chem. 279, 20915–20920. doi: 10.1074/jbc.M400213200
Peeters, M. W., Thomis, M. A., Beunen, G. P., and Malina, R. M. (2009). Genetics and sports: an overview of the pre-molecular biology Era. Med. Sport Sci. 54, 28–42. doi: 10.1159/000235695
Pessemesse, L., Schlernitzauer, A., Sar, C., Levin, J., Grandemange, S., Seyer, P., et al. (2012). Depletion of the p43 mitochondrial T3 receptor in mice affects skeletal muscle development and activity. FASEB J. 26, 748–756. doi: 10.1096/fj.11-195933
Plassais, J., Rimbault, M., Williams, F. J., Davis, B. W., Schoenebeck, J. J., and Ostrander, E. A. (2017). Analysis of large versus small dogs reveals three genes on the canine X chromosome associated with body weight, muscling and back fat thickness. PLoS Genet. 13:e1006661. doi: 10.1371/journal.pgen.1006661
Potts, G. K., McNally, R. M., Blanco, R., You, J.-S., Hebert, A. S., Westphall, M. S., et al. (2017). A map of the phosphoproteomic alterations that occur after a bout of maximal-intensity contractions. J. Physiol. 595, 5209–5226. doi: 10.1113/JP273904
Raffaello, A., Milan, G., Masiero, E., Carnio, S., Lee, D., Lanfranchi, G., et al. (2010). JunB transcription factor maintains skeletal muscle mass and promotes hypertrophy. J. Cell Biol. 191, 101–113. doi: 10.1083/jcb.201001136
Rehage, M., Mohan, S., Wergedal, J. E., Bonafede, B., Tran, K., Hou, D., et al. (2007). Transgenic overexpression of pregnancy-associated plasma protein-A increases the somatic growth and skeletal muscle mass in mice. Endocrinology 148, 6176–6185. doi: 10.1210/en.2007-0274
Rosenberg, I. H. (1997). Sarcopenia: origins and clinical relevance. J. Nutr. 127(5 Suppl.), 990S−991S. doi: 10.1093/jn/127.5.990S
Ruas, J. L., White, J. P., Rao, R. R., Kleiner, S., Brannan, K. T., Harrison, B. C., et al. (2012). A PGC-1α isoform induced by resistance training regulates skeletal muscle hypertrophy. Cell 151, 1319–1331. doi: 10.1016/j.cell.2012.10.050
Ruiz, J. R., Sui, X., Lobelo, F., Morrow, J. R., Jackson, A. W., Sjöström, M., et al. (2008). Association between muscular strength and mortality in men: prospective cohort study. BMJ 337:a439. doi: 10.1136/bmj.a439
Russell, W. L., Kelly, E. M., Hunsicker, P. R., Bangham, J. W., Maddux, S. C., and Phipps, E. L. (1979). Specific-locus test shows ethylnitrosourea to be the most potent mutagen in the mouse. Proc. Natl. Acad. Sci. U.S.A. 76, 5818–5819. doi: 10.1073/pnas.76.11.5818
Sala, D., Ivanova, S., Plana, N., Ribas, V., Duran, J., Bach, D., et al. (2014). Autophagy-regulating TP53INP2 mediates muscle wasting and is repressed in diabetes. J. Clin. Invest. 124, 1914–1927. doi: 10.1172/JCI72327
Sartori, R., and Sandri, M. (2015). Bone and morphogenetic protein signalling and muscle mass. Curr. Opin. Clin. Nutr. Metab. Care 18, 215–220. doi: 10.1097/MCO.0000000000000172
Sartori, R., Schirwis, E., Blaauw, B., Bortolanza, S., Zhao, J., Enzo, E., et al. (2013). BMP signaling controls muscle mass. Nat. Genet. 45, 1309–1318. doi: 10.1038/ng.2772
Schardt, C., Adams, M. B., Owens, T., Keitz, S., and Fontelo, P. (2007). Utilization of the PICO framework to improve searching PubMed for clinical questions. BMC Med. Inform. Decis. Mak. 7:16. doi: 10.1186/1472-6947-7-16
Schiaffino, S., Dyar, K. A., Ciciliot, S., Blaauw, B., and Sandri, M. (2013). Mechanisms regulating skeletal muscle growth and atrophy. FEBS J. 280, 4294–4314 doi: 10.1111/febs.12253
Schoenfeld, B. J. (2010). The mechanisms of muscle hypertrophy and their application to resistance training. J. Strength Cond. Res. 24, 2857–2872. doi: 10.1519/JSC.0b013e3181e840f3
Schuelke, M., Wagner, K. R., Stolz, L. E., Hübner, C., Riebel, T., Kömen, W., et al. (2004). Myostatin mutation associated with gross muscle hypertrophy in a child. N. Engl. J. Med. 351, 2682–2688. doi: 10.1056/NEJMoa040933
Seko, D., Ogawa, S., Li, T.-S., Taimura, A., and Ono, Y. (2016). μ-Crystallin controls muscle function through thyroid hormone action. FASEB J. 30, 1733–1740. doi: 10.1096/fj.15-280933
Shimizu, N., Maruyama, T., Yoshikawa, N., Matsumiya, R., Ma, Y., Ito, N., et al. (2015). A muscle-liver-fat signalling axis is essential for central control of adaptive adipose remodelling. Nat. Commun. 6:6693. doi: 10.1038/ncomms7693
Silventoinen, K., Magnusson, P. K., Tynelius, P., Kaprio, J., and Rasmussen, F. (2008). Heritability of body size and muscle strength in young adulthood: a study of one million Swedish men. Genet. Epidemiol. 32, 341–349. doi: 10.1002/gepi.20308
Sinha-Hikim, I., Artaza, J., Woodhouse, L., Gonzalez-Cadavid, N., Singh, A. B., Lee, M. I., et al. (2002). Testosterone-induced increase in muscle size in healthy young men is associated with muscle fiber hypertrophy. Am. J. Physiol. Endocrinol. Metab. 283, E154–E164. doi: 10.1152/ajpendo.00502.2001
Smith, F. M., Holt, L. J., Garfield, A. S., Charalambous, M., Koumanov, F., Perry, M., et al. (2007). Mice with a disruption of the imprinted Grb10 gene exhibit altered body composition, glucose homeostasis, and insulin signaling during postnatal life. Mol. Cell. Biol. 27, 5871–5886. doi: 10.1128/MCB.02087-06
Sutrave, P., Kelly, A. M., and Hughes, S. H. (1990). ski can cause selective growth of skeletal muscle in transgenic mice. Genes Dev. 4, 1462–1472. doi: 10.1101/gad.4.9.1462
Szklarczyk, D., Franceschini, A., Wyder, S., Forslund, K., Heller, D., Huerta-Cepas, J., et al. (2015). STRING v10: protein-protein interaction networks, integrated over the tree of life. Nucleic Acids Res. 43, D447–D452. doi: 10.1093/nar/gku1003
Thalacker-Mercer, A., Stec, M., Cui, X., Cross, J., Windham, S., and Bamman, M. (2013). Cluster analysis reveals differential transcript profiles associated with resistance training-induced human skeletal muscle hypertrophy. Physiol. Genomics 45, 499–507. doi: 10.1152/physiolgenomics.00167.2012
Tontonoz, P., Cortez-Toledo, O., Wroblewski, K., Hong, C., Lim, L., Carranza, R., et al. (2015). The orphan nuclear receptor Nur77 is a determinant of myofiber size and muscle mass in mice. Mol. Cell. Biol. 35, 1125–1138. doi: 10.1128/MCB.00715-14
Turan, S., and Bode, J. (2011). Site-specific recombinases: from tag-and-target- to tag-and-exchange-based genomic modifications. FASEB J. 25, 4088–4107. doi: 10.1096/fj.11-186940
Uhlén, M., Fagerberg, L., Hallstrom, B. M., Lindskog, C., Oksvold, P., Mardinoglu, A., et al. (2015). Tissue-based map of the human proteome. Science 347, 1260419–1260419. doi: 10.1126/science.1260419
Valdivieso, P., Vaughan, D., Laczko, E., Brogioli, M., Waldron, S., Rittweger, J., et al. (2017). The metabolic response of skeletal muscle to endurance exercise is modified by the ACE-I/D gene polymorphism and training state. Front. Physiol. 8:993. doi: 10.3389/fphys.2017.00993
Vissing, K., and Schjerling, P. (2014). Simplified data access on human skeletal muscle transcriptome responses to differentiated exercise. Sci. Data 1:140041. doi: 10.1038/sdata.2014.41
Watt, K. I., Turner, B. J., Hagg, A., Zhang, X., Davey, J. R., Qian, H., et al. (2015). The Hippo pathway effector YAP is a critical regulator of skeletal muscle fibre size. Nat. Commun. 6:6048. doi: 10.1038/ncomms7048
Willems, S. M., Wright, D. J., Day, F. R., Trajanoska, K., Joshi, P. K., Morris, J. A., Ohlsson, C., et al. (2017). Large-scale GWAS identifies multiple loci for hand grip strength providing biological insights into muscular fitness. Nature Commun 8:16015. doi: 10.1038/ncomms16015
Wolfe, R. R. (2006). The underappreciated role of muscle in health and disease. Am. J. Clin. Nutr. 84, 475–482. doi: 10.1093/ajcn/84.3.475
Wood, A. R., Esko, T., Yang, J., Vedantam, S., Pers, T. H., Gustafsson, S., et al. (2014). Defining the role of common variation in the genomic and biological architecture of adult human height. Nat. Genet. 46, 1173–1186. doi: 10.1038/ng.3097
Yamamoto, H., Williams, E. G., Mouchiroud, L., Cantó, C., Fan, W., Downes, M., et al. (2011). NCoR1 is a conserved physiological modulator of muscle mass and oxidative function. Cell 147, 827–839. doi: 10.1016/j.cell.2011.10.017
You, J.-S., Lincoln, H. C., Kim, C.-R., Frey, J. W., Goodman, C. A., Zhong, X.-P., et al. (2014). The role of diacylglycerol kinase ζ and phosphatidic acid in the mechanical activation of mammalian target of rapamycin (mTOR) signaling and skeletal muscle hypertrophy. J. Biol. Chem. 289, 1551–1563. doi: 10.1074/jbc.M113.531392
Keywords: skeletal muscle, hypertrophy, gene manipulation, mutation, GWAS, sarcopenia, myostatin, resistance exercise
Citation: Verbrugge SAJ, Schönfelder M, Becker L, Yaghoob Nezhad F, Hrabě de Angelis M and Wackerhage H (2018) Genes Whose Gain or Loss-Of-Function Increases Skeletal Muscle Mass in Mice: A Systematic Literature Review. Front. Physiol. 9:553. doi: 10.3389/fphys.2018.00553
Received: 20 December 2017; Accepted: 30 April 2018;
Published: 22 May 2018.
Edited by:
Bert Blaauw, Università degli Studi di Padova, ItalyReviewed by:
Troy A. Hornberger, University of Wisconsin-Madison, United StatesMartin Flück, Universität Zürich, Switzerland
Copyright © 2018 Verbrugge, Schönfelder, Becker, Yaghoob Nezhad, Hrabě de Angelis and Wackerhage. This is an open-access article distributed under the terms of the Creative Commons Attribution License (CC BY). The use, distribution or reproduction in other forums is permitted, provided the original author(s) and the copyright owner are credited and that the original publication in this journal is cited, in accordance with accepted academic practice. No use, distribution or reproduction is permitted which does not comply with these terms.
*Correspondence: Henning Wackerhage, aGVubmluZy53YWNrZXJoYWdlQHR1bS5kZQ==