- 1Laboratório de Bioquímica e Biologia Molecular de Vetores, Instituto de Química, Universidade Federal do Rio de Janeiro, Rio de Janeiro, Brazil
- 2Instituto Nacional de Ciência e Tecnologia em Entomologia Molecular-CNPq, Rio de Janeiro, Brazil
- 3Department of Molecular and Cellular Biology, University of California, Davis, Davis, CA, United States
Olfactory proteins mediate a wide range of essential behaviors for insect survival. Odorant binding proteins (OBPs) are small soluble olfactory proteins involved in the transport of odor molecules (=odorants) through the sensillum lymph to odorant receptors, which are housed on the dendritic membrane of olfactory sensory neurons also known as olfactory receptor neurons. Thus, a better understanding of the role(s) of OBPs from Rhodnius prolixus, one of the main vectors of Chagas disease, may ultimately lead to new strategies for vector management. Here we aimed at functionally characterize OBPs from R. prolixus. Genes of interest were selected using conventional bioinformatics approaches and subsequent quantification by qPCR. We screened and estimated expression in different tissues of 17 OBPs from R. prolixus adults. These analyses showed that 11 OBPs were expressed in all tissues, whereas six OBP genes were specific to antennae. Two OBP genes, RproOBP6 and RproOBP13, were expressed in both male and female antennae thus suggesting that they might be involved in the recognition of semiochemicals mediating behaviors common to both sexes, such host finding (for a blood meal). Transcripts for RproOBP17 and RproOBP21 were enriched in female antennae and possibly involved in the detection of oviposition attractants or other semiochemicals mediating female-specific behaviors. By contrast, RproOBP26 and RproOBP27 might be involved in the reception of sex pheromones given that their transcripts were highly expressed in male antennae. To test this hypothesis, we silenced RproOBP27 using RNAi and examined the sexual behavior of the phenotype. Indeed, adult males treated with dsOBP27 spent significantly less time close to females as compared to controls. Additionally, docking analysis suggested that RproOBP27 binds to putative sex pheromones. We therefore concluded that RproOBP27 might be a pheromone-binding protein.
Introduction
Chemical signals are essential to promote specific behaviors in different species (Gaillard et al., 2004). Insects, in particular, depend on the correct identification of volatile compounds (semiochemicals) for survival and reproduction (Cruz-Lopez et al., 2001; Syed and Leal, 2009; Pitts et al., 2014). Rhodnius prolixus is one of the main vectors of the protozoan Trypanosoma cruzi, the etiological agent of Chagas disease. According to estimates based on 2010 WHO data, 5,742,167 people in 21 Latin American countries are infected (WHO, 2015). New cases due to vector transmission were estimated to 29,925/year (WHO, 2015). Several proteins participate in insect chemosensation, including odorant binding proteins (OBPs), which transports odor molecules through the sensillum lymph to odorant receptors (ORs) (Fan et al., 2011; Leal, 2012; Brito et al., 2016; Pelosi et al., 2018); ORs located in the membrane of olfactory sensorial neurons (Benton, 2006), which recognize volatile odorant molecules (de Bruyne and Baker, 2008); and ionotropic receptors (IRs), which detect diverse chemical ligands from the environment (Benton et al., 2009). OBPs represent the first contact between semiochemicals from the environment and the olfactory sensory system since they are responsible for transporting hydrophobic ligands to their specific ORs (Wojtasek and Leal, 1999; Fan et al., 2011). OBPs are small soluble proteins secreted by accessory cells into the antenna sensillar lymph surrounding the olfactory sensory neurons (Brito et al., 2016; Pelosi et al., 2018). Initially, OBPs were identified and characterized at molecular level in Drosophila melanogaster (Brito et al., 2016). After that, other studies reported that OBPs were identified in different insect species, including the disease vectors Anopheles gambiae (Vogt, 2002; Mastrobuoni et al., 2013), Aedes aegypti (Zhou et al., 2008), Culex quinquefasciatus (Pelletier and Leal, 2009), and Glossina morsitans morsitans (Liu et al., 2010). In hemipterans, the first characterized OBP was Lygus antennal protein (LAP) from the phytophagous insect Lygus lineolaris (Dickens et al., 1998). LAP expression was shown to be adult-specific, initiating development in antennae during the transitional period that precedes adult molt (Vogt et al., 1999). Subsequently, it was reported that in the alfalfa plant bug Adelphocoris lineolatus, some OBP genes exhibited high differential expression in male and female antennae (Gu et al., 2011a). More recently, the genome of the hematophagous hemipteran R. prolixus was released and has been predicted to encode 27 putative OBP genes (Mesquita et al., 2015). However, only 17 OBPs were actually identified in the antenna proteome (Oliveira et al., 2017), suggesting that these proteins could be associated with odor detection. Hemipteran insects have many intricate behaviors such as male aggregation (Vitta et al., 2009; Pontes and Lorenzo, 2012), oviposition aggregation (Rolandi and Schilman, 2017), food ingestion (Diaz-Albiter et al., 2016; Franco et al., 2016), and avoidance behavior (Zermoglio et al., 2015). Despite the importance of R. prolixus as a vector of Chagas disease, the role(s) of OBPs in odor recognition has not yet been investigated, even though there is strong evidence that these insects use chemical signals to mediate sexual communication. It is already known that males can be oriented toward air currents carrying volatiles produced by female metasternal glands (MGs) (Pontes et al., 2008, 2014). Recently, several studies have used the RNA interference (RNAi) technique to identify OBP functions in insects (Biessmann et al., 2010; Pelletier et al., 2010; Zhang et al., 2016). Moreover, it might be possible to link behavior to OBP(s) by gene silencing (He et al., 2011; Swarup et al., 2011; Deng et al., 2013; Li et al., 2016; Shorter et al., 2016; Lin et al., 2017). In fact, RNAi based studies have shown that OBPs are involved in the detection of oviposition attractants (Biessmann et al., 2010; Pelletier et al., 2010), plant volatiles (He et al., 2011; Li et al., 2016; Zhang et al., 2016), host molecules (Deng et al., 2013), in the survival of insects (He et al., 2011; Lin et al., 2017), and regulates mating behavior (Shorter et al., 2016). Therefore, the purpose of this study was to investigate the role of the 17 OBPs previously identified in antenna proteome (Oliveira et al., 2017), in R. prolixus chemical communication. Results revealed that 11 OBPs were expressed in all tissues, whereas six OBPs were shown to be antennae-specific. RproOBP6 and RproOBP13 were expressed in both male and female antennae. RproOBP17 and RproOBP21 were enriched in female antennae. In contrast, RproOBP26 and RproOBP27 were significantly expressed in male antennae, which suggests these proteins could play a role in male specific behaviors. Interestingly, RproOBP26 was also reported overexpressed in the insect gut (Ribeiro et al., 2014), suggesting that RproOBP26 might be involve in multiple roles. The potential role of RproOBP27 in the detection of odorants was further investigated by RNAi because this protein is male antennae-specific and thus a putative pheromone-binding protein. Additionally, docking analysis suggested that RproOBP27 favorably binds the most abundant chemicals (putative sex pheromones) identified in female MGs (Pontes et al., 2008), which indicates this OBP could be involved in the detection of female-derived semiochemicals. In a behavioral assay, males injected with dsOBP27 spent significantly less time close to females when compared to controls, strongly suggesting RproOBP27 plays a role in the reception of female-derived semiochemicals.
Materials and Methods
Insect Rearing
Rhodnius prolixus were taken from a colony at Insect Biochemistry Laboratory/Federal University of Rio de Janeiro/Brazil. Insects were maintained at 28°C and 80–90% relative humidity under a photoperiod of 12 h of light/12 h dark. Insects used in this work were unmated males fed on rabbit blood at 3-week intervals. Male R. prolixus injected with dsRNA were kept on cages maintained under the same conditions. In dsRNA experiments, unfed male nymphs (5th instar, N5) were injected with 1 μg of dsRNA (dsOBP27 or dsβ-gal) diluted in 1 μL of RNase-free water into the metathoracic cavity using a 10 μL Hamilton syringe. Nymphs were fed on rabbit blood 7 days after dsRNA treatment.
Ethics Statement
All animal care and experimental protocols were conducted following the guidelines of the institutional care and use committee (Committee for Evaluation of Animal Use for Research from Federal University of Rio de Janeiro), which are based on the National Institute of Health Guide and Use of Laboratory Animals (ISBNo-309-05377-3). The protocols were approved by the Committee for Evaluation of Animal Use for Research (CAUAP) from the Federal University of Rio de Janeiro, under register number CEAU-UFRJ#1200.001568/2013-87, 155/13. Technicians dedicated to the animal facility at Federal University of Rio de Janeiro carried out all aspects related to rabbit husbandry under strict guidelines to ensure careful and consistent handling of the animals.
Tissue Isolation, RNA Extraction, and cDNA Synthesis
Antennae, proboscis, legs, and heads (without antennae and proboscis) from 30 blood-fed male and 30 blood-fed female were dissected using forceps. Tissues were transferred to polypropylene tube separately, frozen in liquid nitrogen and triturated with plastic pestle. Total RNA was extracted from different tissues using TRIzol (Invitrogen, Carlsbad, CA, United States) according to the manufacturer’s instructions. RNA concentrations were determined at 260 nm on a UV-1800 spectrophotometer (Shimadzu, Inc., Kyoto, Japan). RNA integrity was evaluated in 1% agarose gel. RNAs were treated with RNase-free DNAse I (Fermentas International, Inc., Burlington, ON, Canada), 1 μg of RNA was used for cDNA synthesis with High-Capacity cDNA Reverse Transcription kit and random primers (Applied Biosystems, Foster City, CA, United States).
Spatial Transcript Quantification
Gene sequences of 17 RproOBPs were downloaded from R. prolixus genome database1 for primer design using OligoPerfectTM Designer – Thermo Fisher Scientific tool. All primer sequences are listed in Supplementary Table S1. PCR studies were performed using GoTaq® Green Master Mix kit (Promega, Madison, WI, United States). R. prolixus’ ribosomal gene 18S (RproR18S) was used as the reference gene (Majerowicz et al., 2011). PCRs were performed on Veriti® Thermal Cycler-96 well thermocycler (Applied Biosystems, Foster City, CA, United States), consisting of 35 cycles for RproOBPs and 25 cycles for RproR18S under the following conditions, 94°C for 3 min, followed by denaturation steps at 94°C for 30 s, annealing temperature was set according to each primer pair (Supplementary Table S1) for 30 s and the extension step at 72°C for 1 min and 30 s, finally followed by 72°C for 10 min. cDNA from antennae, proboscis, legs, and heads (without antennae and proboscis) obtained from adults were used as templates for PCR. PCR products were analyzed on a 1% agarose gel stained with GelRedTM (Biotium, Hayward, CA, United States) in TAE buffer pH 8 (40 mM Tris-acetate, 1 mM EDTA). Gels were digitalized on DNR MiniBIS Pro Bio-Imaging Systems (BioAmerica Inc., Miami, FL, United States). qPCRs were performed on a StepOneTM Real-Time PCR System (Applied Biosystems) thermocycler using Power SYBR® Green PCR Master Kit (Applied Biosystems). cDNA from adult antennae, proboscis, legs and heads (without antennae and proboscis) were used as templates for qPCRs. Oligonucleotide concentrations consisted of 400 nM for RproR18S and 600 nM for RproOBPs. Reactions were carried out in three biological replicates and three technical replicates for each sample, in a 48-well optical plate with the following initial cycle, 50°C for 2 min; 95°C for 10 min; followed by denaturation steps at 94°C for 15 s then 60°C for 15 s and extension at 72°C for 1 min for 40 cycles; dissociation curves were obtained under standard conditions of the instrument. RproR18S gene was used as reference gene for the normalization of Ct (threshold cycle) values. The relative gene expression of the RproOBPs was determined by 2-ΔΔCt method (Livak and Schmittgen, 2001). Data were presented as mean ± standard error of three independent experiments in biological triplicates.
dsRNA Synthesis and Gene Silencing Assays
Fragments of PCR product encoding RproOBP27, size 146 bp, were amplified by PCR using cDNA from blood-fed male adults antennae produced as described above. The following conditions were used for amplification: one cycle for 3 min at 94°C, following by 35 cycles of 30 s at 94°C for denaturation, 30 s at 59°C for annealing and the extension step at 72°C for 1 min and 30 s, followed by 72°C for 10 min. The primers used for amplification of templates for dsRNA synthesis are listed at Supplementary Table S1. These primers contained a T7 polymerase binding sequence required for dsRNA synthesis. These products were used as the template for the transcription reactions using the enzyme T7 RNA polymerase with MEGAscriptRNAi kit (Ambion, Austin, TX, United States), according to manufacturer’s protocol. The β-galactosidase protein (β-gal) gene from Culex quinquefasciatus (Xu et al., 2014) cloned into pGEM-T (Promega) was amplified by PCR using T7 minimal promoter primers under the following conditions: one cycle for 3 min at 94°C, following by 35 cycles of 30 s at 94°C for denaturation, 30 s at 56°C for annealing and the extension step at 72°C for 1 min and 30 s, followed by 72°C for 10 min. The PCR product generated was used as the template for β-gal dsRNA synthesis used as a control in the silencing assay. Following in vitro synthesis, all dsRNAs were purified using phenol-chloroform (1:1), quantified using a spectrophotometer at 260 nm and analyzed by 1% agarose gel electrophoresis stained with GelRedTM. RNAi experiments were performed as described by Franco et al., 2016. Briefly, 1 μL of dsRNA (1 μg/μL RNase-free water) was injected into the metathoracic cavity of starved N5 males (N = 20 for each dsRNA treatment), using a 10 μL Hamilton syringe, after 7 days insects were blood fed and monitored during 21 days until ecdysis. The resulting dsRNA-treated adults were fed on rabbit blood. In bioassays, insects from the different groups were tested individually.
Starved N5 males treated with dsRNA as described above (N = 20 for dsOBP27 and N = 20 for dsβ-gal) were kept under controlled temperature and humidity conditions. Mortality was monitored from the 3rd to the 20th day after dsRNA injection. The number of survival N5 in this period was registered. The effect of dsRNA injection on blood feeding was performed as described by Franco et al., 2016. The dsOBP27- and dsβ-gal-treated N5s were weighed 2 h before and 2 h after feeding. The ingested mass (mg) was calculated by the weight difference after and before feeding.
Female Recognition Bioassay
The ability of adult males treated with dsRNA to recognize females was accessed using a bioassay adapted from Zermoglio et al. (2015) (Supplementary Figure S5). Adult males injected with dsRNA in the N5 stage were used in the bioassay. dsRNA-treated N5 males were blood-fed (N = 20 dsOBP27; N = 20 dsβ-gal) 7 days after injection. Males were then blood-fed 7 days after molt. Bioassays were conducted 1 week after blood meals. A polystyrene tube (falcon tube) with approximately 10 cm long and 2 cm in diameter was used (Supplementary Figure S5). This tube was divided into three zones: female zone (FZ), intermediate zone (IZ), and male release zone (MZ). A gate divides the MZ from IZ. A protective mesh was used to separate MZ and IZ from FZ. An adult female was placed in front of the protection mesh attached by a tape on the tube. Then a male was placed in the MZ and the gate was opened after 5 min of acclimation. The time spent by males to move across the tube toward the female was measured using a digital chronometer and estimated in a maximum period of 300 s. When the insect reached the FZ, the chronometer was reset and started again to record the interval of time that male stayed near the female. The bioassay was repeated 3 times for each insect in each group (dsOBP27 and dsβ-gal).
Docking Studies
Since 3D structures have not yet been characterized for Rhodnius OBPs, the primary sequence of mature RproOBP27 was used to construct a 3D model for in silico docking studies. Three-dimensional modeling was developed using the online protein threading program PHYRE2 (Kelley et al., 2015). Stereochemical quality and accuracy of the predicted model were evaluated using the software PROCHECK (Laskowski et al., 1996) and Verify3D (Eisenberg et al., 1997). The most abundant compounds identified as volatiles emitted by MGs of females and reported as being able to modulate male orientation (Pontes et al., 2008, 2014) were selected for docking studies: 2-methyl-3-buten-2-ol, (2S)-pentanol, (3E)-2-methyl-3-penten-2-ol, and (2R/2S)-4-methyl-3-penten-2-ol. We used thermodynamic principle that ligands tightly bind the active site of a protein when the free binding energy of the process is low (Du et al., 2016). Therefore, such parameter was used to estimate binding affinities of the MGs ligands to RproOBP27. Three-dimensional structures of compounds were obtained from PubChem2 (Kim et al., 2016). Molecular docking with RproOBP27 and each of the selected ligands was carried out 100 times using Docking Server (Bikadi and Hazai, 2009) and the free binding-energy scoring function was considered to estimate binding affinity.
Statistical Analysis
Statistical analysis of qPCRs and bioassays were performed using t-test followed by the Mann–Whitney test (GraphPad PRISM 6.00 software, San Diego, CA, United States). qPCRs analyses were done by using three biological and three technique replicates for each gene. Bioassays were carried out independently in three technique replicates. Bars represent the standard error of three replicate, asterisks indicate statistically significant differences (P < 0.05).
Results
Spatial Expression of OBPs
Previous results showed that 17 OBPs were expressed in the adult antennae (Oliveira et al., 2017), which suggests that at least 17 genes predicted as OBPs in the genome actually encodes antennal functional proteins. In order to investigate which of those 17 OBP genes were antennae-specific, different tissues of adult insects [antennae, proboscis, legs, and heads (without antennae and proboscis)] were screened by PCR. Spatial expression showed that 11 OBPs (RproOBP1, RproOBP7, RproOBP11, RproOBP12, RproOBP14, RproOBP18, RproOBP20, RproOBP22, RproOBP23, RproOBP24, and RproOBP29) were expressed in multiple tissues (Figure 1; all original gels appear in Supplementary Figures), which strongly suggests that proteins produced by these genes are not specifically related to odorant transport. In contrast, four OBPs (RproOBP6, RproOBP13, RproOBP17, and RproOBP21) were detected specifically in adult antennae, although minor bands for RproOBP13 and RproOBP21 were detected in other tissues (Figure 2). Two OBPs were highly expressed in the male antennae, RproOBP26 and RproOBP27 (Figure 2), with minor RproOBP27 bands being observed in male proboscis and male and female legs (Figure 2; see also the original gels in the Supplementary Figures S1–S4). To further investigate these qualitative profiles, OBPs that were found to be enriched in the antenna were quantified by qPCR. Proboscis, legs, and heads (without antenna and proboscis) were also analyzed by qPCR. Considering that we did not identify any transcripts in proboscis, heads, and legs, the above described bands in these tissues (detected by conventional PCR) were probably not specific bands for the tested genes (Figure 2). Quantitative results confirmed that RproOBP6 and RproOBP13 were expressed exclusively in male and female adult antennae and did not exhibit transcripts in other tissues (Figures 3A,B). In addition, RproOBP17 and RproOBP21 were enriched in female antennae (P < 0.05) (Figures 3C,D). On the other hand, RproOBP26 and RproOBP27 were shown to have high and specific expression in male antenna (P < 0.05) (Figures 3E,F).
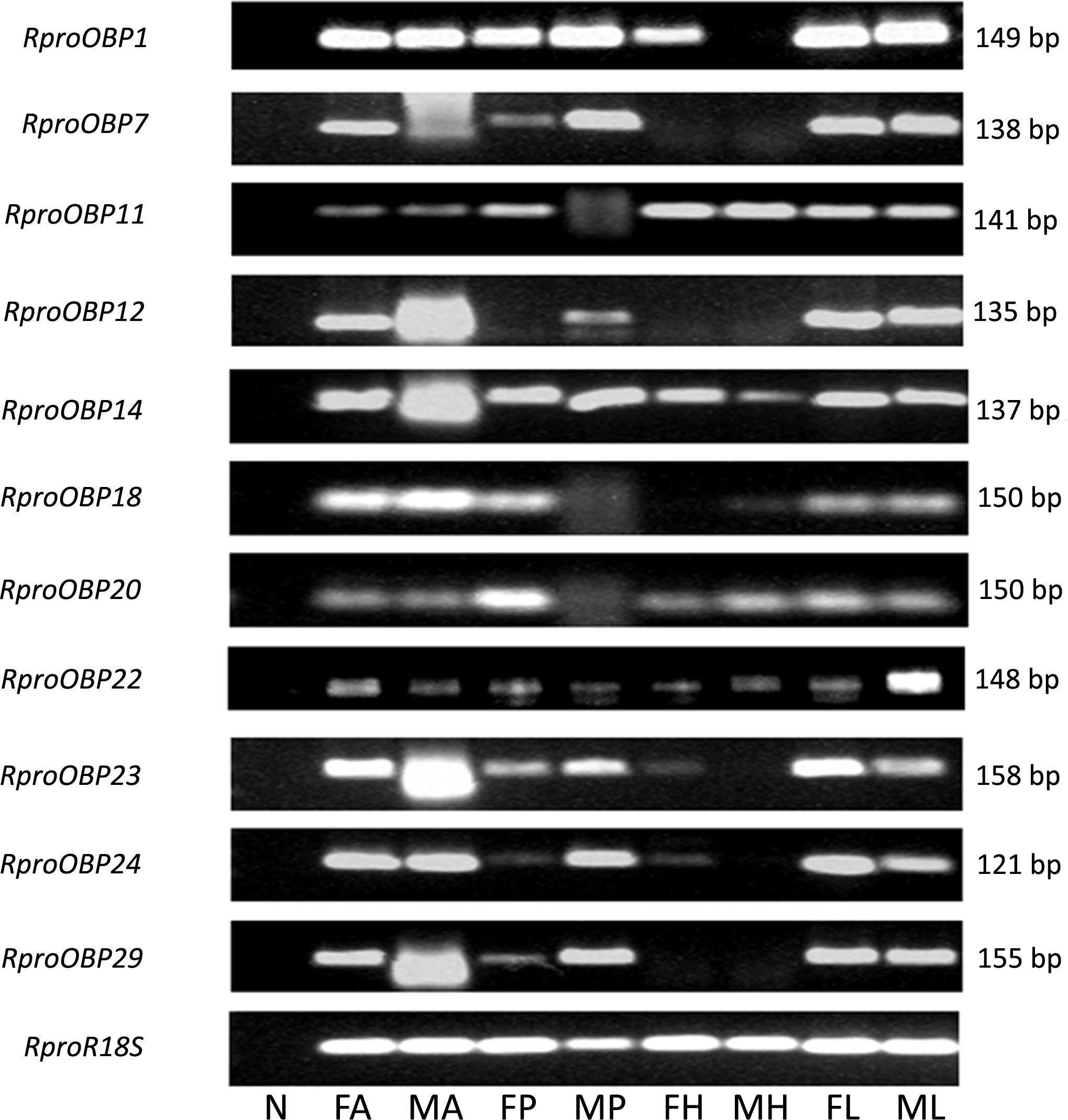
FIGURE 1. Expression profile of RproOBP1, RproOBP7, RproOBP11, RproOBP12, RproOBP14, RproOBP18, RproOBP20, RproOBP22, RproOBP23, RproOBP24, and RproOBP29 in different Rhodnius prolixus tissues evaluated by conventional PCR. N, negative control; FA, female antennae; MA, male antennae; FP, female proboscis; MP, male proboscis; FH, female head; MH, male head; FL, female legs; ML, male legs. RproR18S was used as an endogenous control. The amplicon size (bp) is indicated on the right. Heads were used without antennae and proboscis.
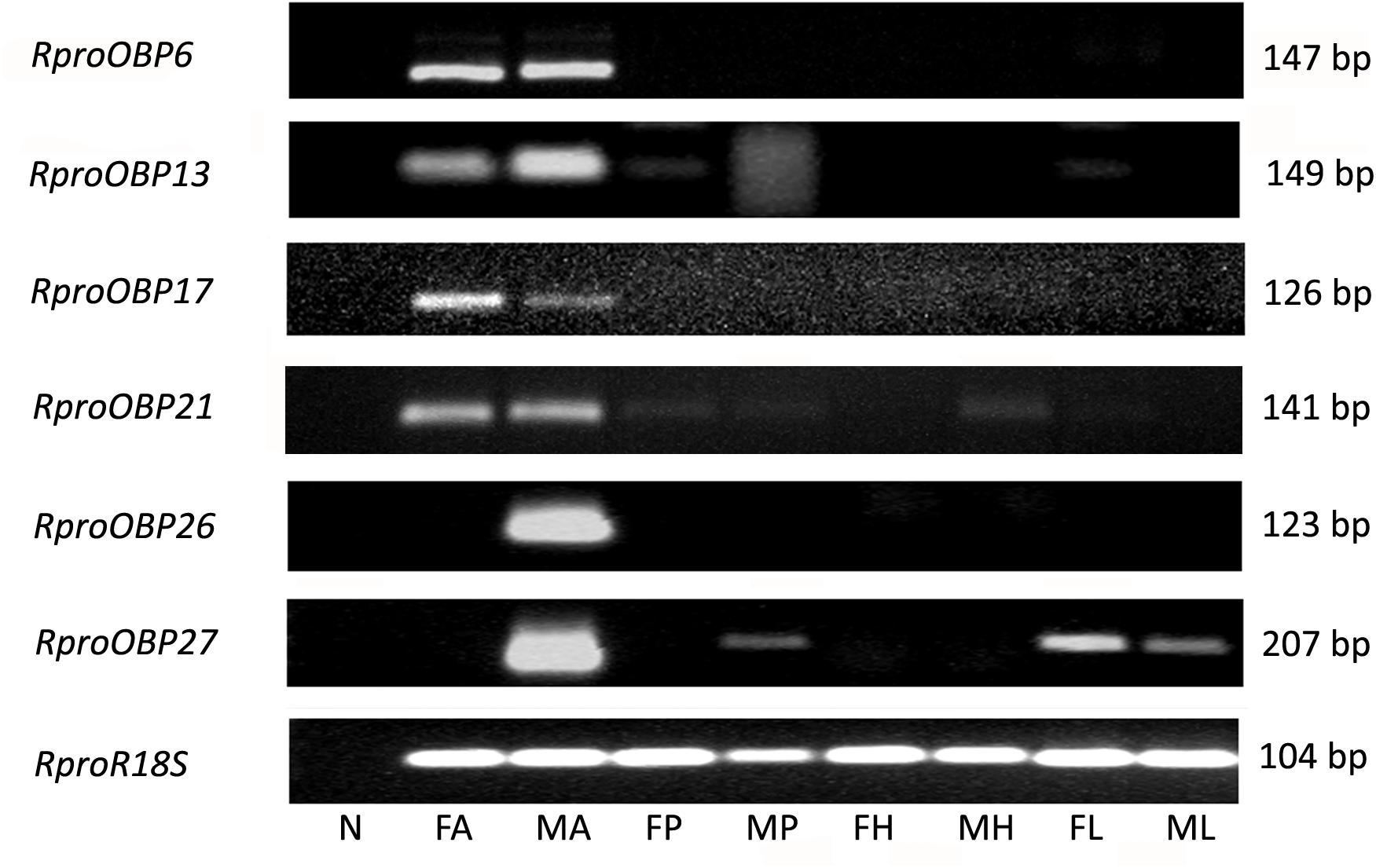
FIGURE 2. Expression profile of RproOBP6, RproOBP13, RproOBP17, RproOBP21, RproOBP26, and RproOBP27 in different R. prolixus tissues evaluated by conventional PCR. N, negative control; FA, female antennae; MA, male antennae; FP, female proboscis; MP, male proboscis; FH, female head; MH, male head; FL, female legs; ML, male legs. RproR18S was used as an endogenous control. The amplicon size (bp) is indicated on the right. Heads were used without antennae and proboscis.
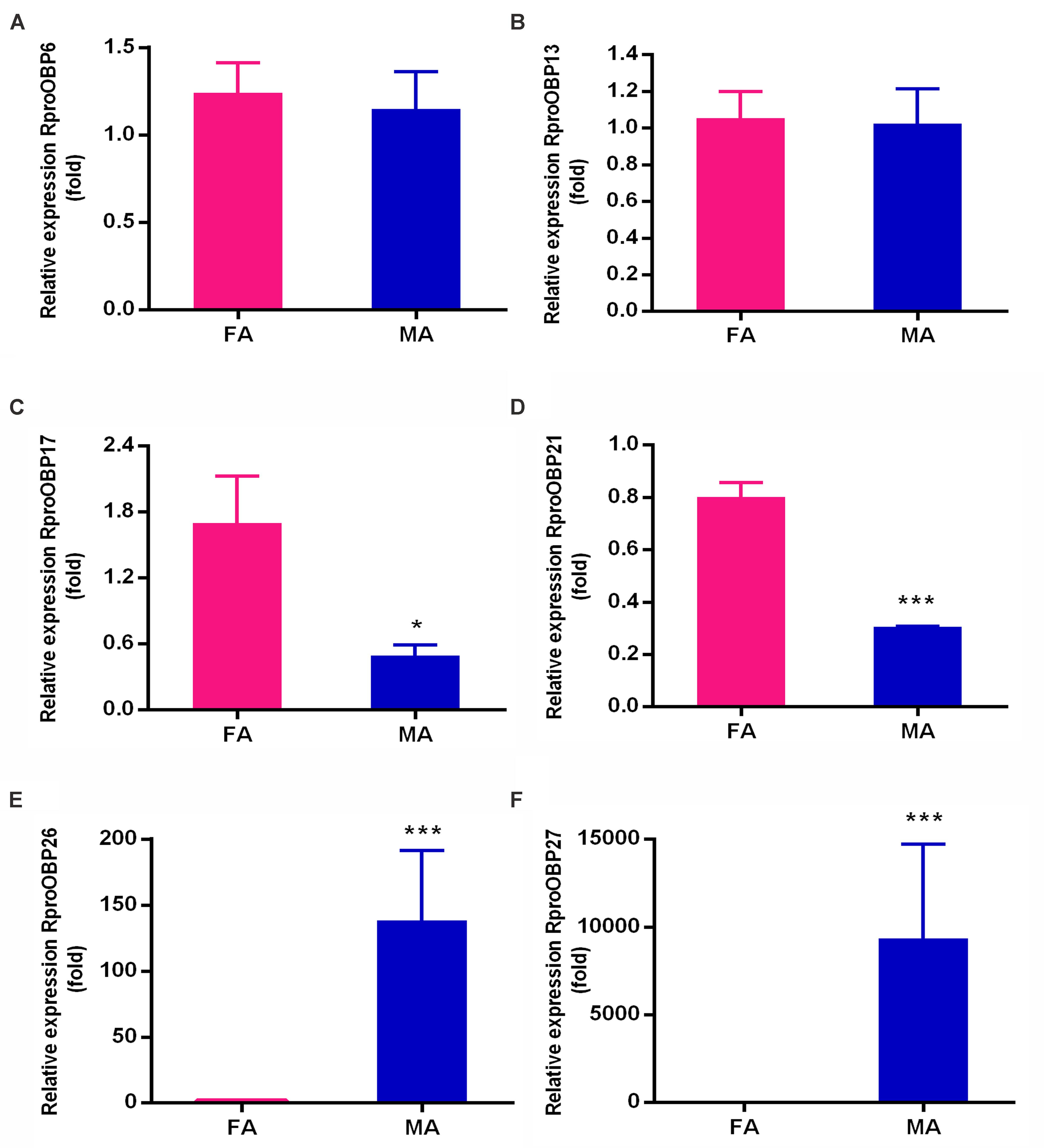
FIGURE 3. Relative transcript levels of (A) RproOBP6, (B) RproOBP13, (C) RproOBP17, (D) RproOBP21, (E) RproOBP26, and (F) RproOBP27 genes in female and male R. prolixus antennae, determined by qPCR. The relative expression levels of gene transcripts were compared to the female antennae. Error bars represent standard deviation (SD) of the means of three biological replicates. Statistical analysis was performed using t-test followed by the Mann–Whitney test. RproR18S was used as an endogenous control. Asterisks represent a significant difference between males and females (P < 0.05). FA, female antennae; MA, male antennae.
Role of RproOBP27 on Male Behavior
Silencing of RproOBP27
Next, we reduced the expression of RproOBP27 using RNAi and evaluated the behavior of the male phenotype. Transcript levels of RproOBP27 were compared to control dsβ-gal. RproR18S was utilized as a reference gene to calculate relative expression. dsOBP27 injected-group exhibited a significant reduction in RproOBP27 expression (8x) when compared to control groups (Figure 4A). In fact, the dsβ-gal fold change mean was 1.06, while dsOBP27 was 0.13, which indicates an 88% expression decrease.
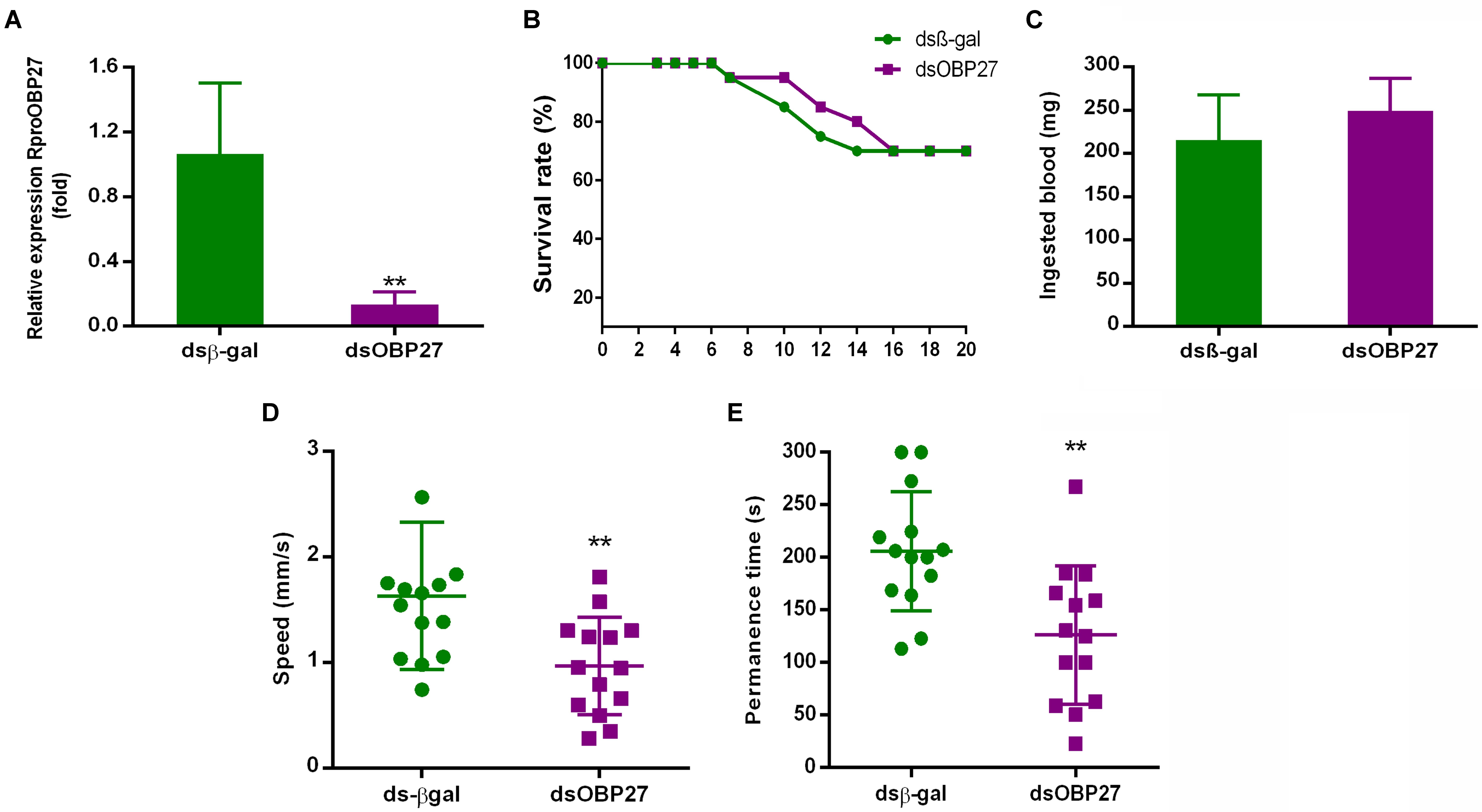
FIGURE 4. (A) qPCR analysis of RproOBP27 gene in antennae of dsRNA treated adult male. Insects were injected with control dsβ-gal (not related gene) or with dsOBP27. The relative expression levels of gene transcripts were compared to the dsβ-gal. RproR18S was used as an endogenous control. (B) Survival rate of starved N5 males treated with dsRNA (N = 20 for dsOBP27 and N = 20 for dsβ-gal). The survival of the insects was monitored from the 3rd to the 20th day after dsRNA injection. (C) Blood feeding of insects injected with dsOBP27 and dsβ-gal. The treated-dsOBP27 and dsβ-gal N5s were weighed 2 h before and 2 h after blood intake. The ingested mass (mg) was calculated by the weight difference after and before the feed. (D) Speed of dsRNA treated male to access female. After molt, the adults injected with dsOBP27 and dsβ-gal were blood-fed. After 7 days fed insects were individually tested using a polystyrene tube. The time spent by males to arrive close to a caged female was recorded during for up to 300 s. (E) Time spent by dsRNA treated male close to female. After molt, the adult injected with dsOBP27 and dsβ-gal was blood-fed. After 7 days fed insects were individually tested using a polystyrene tube. Time spent by males close to female was recorded for up to 300 s. Error bars represent standard deviation of the means of three biological and technical replicate. Statistical analysis was performed using t-test followed by the Mann–Whitney test. Asterisks represent a significant difference (P < 0.05).
Effects of Reduction in the Expression of RproOBP27 on Male Physiology
Insect survival was monitored from 3 to 20 days before molting. The survival index ranged from 70 to 95%, which showed that the injection of dsβ-gal and dsOBP27 did not affect the insect’s lifespan (Figure 4B). Another important aspect of male physiology, which was not affected by dsRNA treatment, was blood feeding. The reduction of RproOBP27 expression did not affect the ability of male adults to take a blood meal (Figure 4C). There was no significant difference (P = 0.4206) in blood intake by dsOBP27-treated (249.1 ± 16.85 mg/blood, N = 5) and dsβ-gal-treated (215.8 ± 23.14 mg/blood, N = 5) insects.
Behavioral Response of dsOBP27-Treated Male
The time spent by the male to move across the tube and reach next to the female was recorded for a period of 300 s. dsOBP27-treated insects accessed the FZ (female zone) with a speed of 0.96 ± 0.12 mm/s, whereas dsβ-gal-treated insects responded significantly faster (1.63 ± 0.19 mm/s, N = 14, P = 0.0065) (Figure 4D). dsOBP27 insect-groups stayed close to females for a significantly (P = 0.002) shorter period of time (126.1 ± 17.6 s) than dsβ-gal-treated insects (205.9 ± 15.2 s) (Figure 4E). Additionally, we observed that, as opposed to treated insects, control males attempted to copulate with females through the mesh separating them in the arena.
3D Model Prediction and in silico Forecasting of RproOBP27 Function
Using Phyre2, 12 3D models were obtained, including Antheraea polyphemus PBP1 [PDB#2JPO; confidence (C) = 99.4; % i.d. = 18]; Amylois transitella PBP1 (PDB#4INW; C = 99.4; % i.d. = 18); Bombyx mori PBP1 (PDB#1DQE; C = 99.3; % i.d. = 15), OBP2 (PDB#2WCL; C = 99.3; % i.d. = 22), Leucophaea maderae PBP (PDB#1OW4; C = 99.1; % i.d. = 10); Apis melifera OBP5 (PDB#3R72; C = 99.1; % i.d. = 12); An. gambiae OBP4 (PDB#3Q8I; C = 99.0; % i.d. = 17); A. melifera OBP14 (PDB#3S0B; C = 98.9; % i.d. = 10), OBP (PDB#1R5R; C = 98.9; % i.d. = 14), Phormia regina OBP56a (PDB#5DIC; C = 98.9; % i.d. = 14); An. gambiae OBP20 (PDB#3BV1; C = 98.8; % i.d. = 16), and Locusta migratoria OBP1 (PDB#4PT1; C = 98.8; % i.d. = 16). Then, PROCHECK and Verify 3D were used to find a model for RproOBP27. The best model for RproOBP27 (Figure 5A) was obtained using the crystal structure of OBP20 from An. gambiae (AgamOBP20, PDB#3VB1) as template and used in docking studies. This model was the one which best satisfied the criteria required by PROCHECK and Verify 3D in order to validate as a good model (Supplementary Figures S6, S7). Binding affinities of RproOBP27 were tested against 2-methyl-3-buten-2-ol, (2S)-pentanol, (3E)-2-methyl-3-penten-2-ol and (2R/2S)-4-methyl-3-penten-2-ol. Thermodynamically, ligands tightly bind the active site of a protein when the free binding energy of the process is low. Therefore, such parameter was used to estimate binding affinities of 2-methyl-3-buten-2-ol, (2S)-pentanol, (3E)-2-methyl-3-penten-2-ol and (2R/2S)-4-methyl-3-penten-2-ol to RproOBP27. Negative values suggested favorable interactions with all tested ligands (Figure 5B). However, since using a cut-off value of -4.00 results still indicate that RproOBP27 is able to bind (3E)-2-methyl-3-penten-2-ol and (2R/2S)-4-methyl-3-penten-2-ol, both compounds already described as being involved in flight orientation modulated by female-emitted volatiles, a male-specific behavior. The 3D model of RproOBP27 docked with MG volatiles (3E)-2-methyl-3-penten-2-ol and (2R/2S)-4-methyl-3-penten-2-ol appears in Supplementary Figure S8. The amino acid sequence of RproOBP27 is displayed in Supplementary Figure S9.
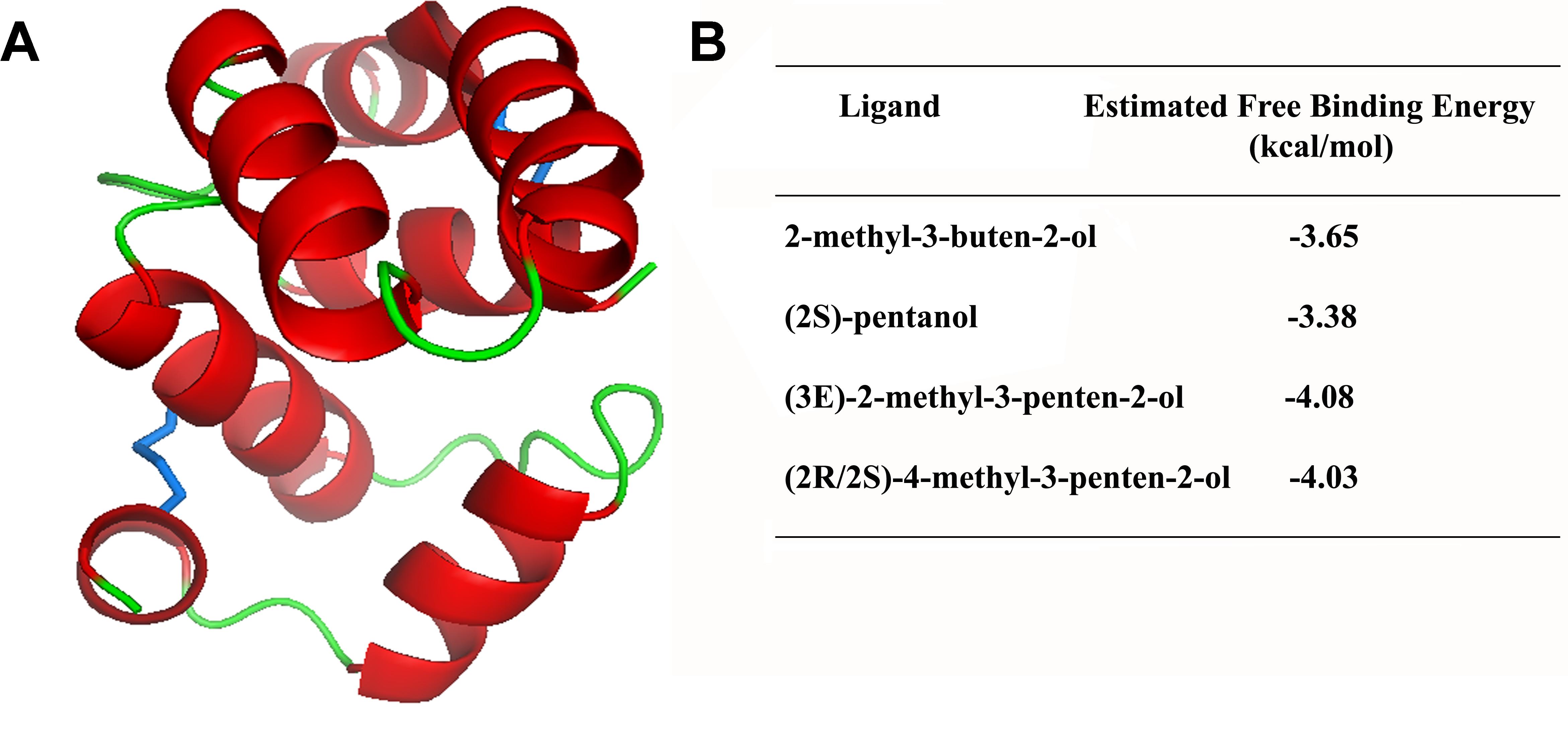
FIGURE 5. 3D model of RproOBP27. α-Helices are shown in red, loops in green and disulfide linkages are highlighted in blue. 3D modeling was developed using the crystal structure of OBP20 from Anopheles gambiae (PDB Code: 3VB1) as template by the online program PHYRE2 and validated using PROCHECK and Verify3D.
Discussion
Chemical communication is one of the oldest forms of communication used from worms to mammals (Wyatt, 2014; Tomberlin et al., 2016). Insects have a refined olfactory system for the detection of chemical signals from the environment. Chemical signals evoke specific behaviors which allows insects to obtain food, find mates and shelter, and run away from predators. The first contact between the external environment and the internal olfactory machinery occurs when odorants penetrate through the sensillum pores in antennae and reach soluble OBPs found in the sensillar lymph (Brito et al., 2016). Subsequently ORs, IRs, and odorant degrading enzymes are involved. The processing of these semiochemicals ultimately leads to a behavioral response which is essential for insect survival (Leal, 2012). Thus, blocking the first step of the process could be a key factor for controlling insect populations. Research regarding olfactory mechanisms of R. prolixus for such purpose only became possible after genome release, when many genes related to chemosensory detection were identified (Mesquita et al., 2015). Here, we present a compilation of data that strongly suggests the role of RproOBP27 in R. prolixus behavior.
Profile of Odorant Binding Protein Genes
Although the genome predicts 27 genes which encode OBPs (Mesquita et al., 2015), only 17 OBPs were found to be expressed in adult antennae (Oliveira et al., 2017), which suggests that several genes belonging to the OBP family may not be directly involved in odor transport, as observed in other insects (Pelosi et al., 2018). Moreover, amongst the 11 OBP transcripts identified in antenna, leg, proboscis, and head from adults (Figure 1), four had already been described in the midgut transcriptome: RproOBP1, RproOBP11, RproOBP14, and RproOBP24 (Ribeiro et al., 2014). Such evidence favors the assumption that these proteins might be involved in transporting general molecules, not necessarily related to odorant reception. In fact, RproOBP11, known as Rhodnius heme-binding protein (RHBP), is responsible for the transport of heme radicals generated from blood digestion, shielding cells from oxidative stress (Dansa-Petretski et al., 1995). Some OBPs, for instance, are important in nutrition as lipids solubilizers and other components of the insect diet (Sanchez-Gracia et al., 2009). Therefore, it was not entirely surprising to find transcripts for OBPs distributed in non-olfactory tissues.
Using conventional PCR, six OBPs transcripts were specifically expressed in antennae: RproOBP6, RproOBP13, RproOBP17, RproOBP21, RproOBP26, and RproOBP27 (Figure 2), which suggests these OBPs may, in fact, be associated with odorant transport as it has been reported for other insects (Leal, 2012; Schultze et al., 2012; Sun et al., 2014). Of note, no clear differences were observed in transcript levels of antenna specific OBPs between male and female using conventional PCR (Figure 2).
Given that qPCR data showed RproOBP6 and RproOBP13 were expressed in male and female antennae (Figures 3A,B), it is conceivable that these OBPs are involved in the detection of odorants eliciting common adult behaviors (e.g., host finding). R. prolixus belongs to the Reduviidae family, where adults are hematophagous (Guerenstein and Lazzari, 2009; Sant’Anna et al., 2017), therefore, adults need to accurately detect host specific volatiles to acquire their blood meal (Otalora-Luna et al., 2004). Thus, we propose that RproOBP6 and RproOBP13 might transport host emanations.
Transcripts for RproOBP17 and RproOBP21 were enriched in female antennae (Figures 3C,D), indicating these proteins might be involved in female-specific behaviors. This hypothesis is supported by the finding that in the mosquito Culex quinquefasciatus, another hematophagous insect, some OBPs expressed in the female antenna are specifically related to the detection of oviposition odorants. OBP2 is postulated to carry the oviposition attractant skatole, whereas OBP1 and OBP5 were implicated in the transport of a mosquito oviposition pheromone (MOP), which induced oviposition behavior in females (Pelletier et al., 2010; Yin et al., 2015).
Lastly, transcripts for RproOBP26 and RproOBP27 were found to be significantly expressed in the male antenna (Figures 3E,F). These results strongly suggest that these proteins could play a role in male-specific behaviors, such as sex pheromone detection. In the mosquito Aedes aegypti, OBP10 is enriched in antennae and wings of adult male and it expression pattern has been suggested to correspond to proteins that may play a role on male chemosensory behavior such as pheromone detection (Bohbot and Vogt, 2005). Although RproOBP26 was highly expressed in antennae (Figure 3E), it was also reported to be overexpressed in the midgut of R. prolixus (called RP-3726) (Ribeiro et al., 2014). Here we showed that transcripts for RproOBP26 were significantly more expressed in male than female antennae (Figure 3E). However, proteome studies have found soluble RproOBP26 in both male and the female antennae (Oliveira et al., 2017). Thus, we cannot rule out the possibility that RproOBP26 might be involved in the transport of non-sensorial molecules in the gut, as well as semiochemicals in antennae. Although, only one gene for RproOBP26 has been annotated in the genome (Mesquita et al., 2015), we could not exclude the possibility of RproOBP26 has alternative splicing, as previously observed for other insect species (Forêt and Maleszka, 2006; Hull et al., 2014).
Role of OBP27 in R. prolixus Behavior
Previously, we have demonstrated a direct correlation between an olfactory protein (Orco) and R. prolixus behavior by RNAi (Franco et al., 2016). We then surmised that silencing OBPs might lead to behavioral changes in the phenotype. After all, gene silencing has already been successfully applied to investigate functions of OBPs in other insects (Chen et al., 2008; Pelletier et al., 2010; Rebijith et al., 2016). Of the two OBPs specific to male antennae, we selected RproOBP27 for these studies. We envisioned that this protein might generate a clearer picture than RproOBP26 given the possible dual role (or multiple roles) played by RproOBP26 in R. prolixus physiology.
Adult males treated with dsOBP27 had a reduction of 88% in RproOBP27 expression (Figure 4A), representing a drastic decrease in the amount of protein circulating in antennae. However, this reduction in gene expression did not interfere with survival or blood-intake, since both groups (control- and dsOBP27-insects) ingested almost the same amount of blood (Figures 4B,C). Differently, a reduction in expression of odorant coreceptor Orco in R. prolixus antenna affected directly the ability of insect to take a blood meal (Franco et al., 2016). Thus, we can suggest that RproOBP27 is not involved in the host-seeking or blood-intake behavior. Next, we tested whether RNAi treatment would affect male ability to detect females. Insects injected with a control gene were able to detected females and run in their direction faster than dsOBP27-treated males (Figure 4D). Further, dsOBP27-males spent almost 40% less time nearby the female when compared to control insects (Figure 4E). In addition, while males from control groups tried to stay close to females, dsOBP27-treated insects kept running around the tube, indicating they were not able to detect a female. Based on this clear behavioral difference, we hypothesize that RproOBP27 may be involved in the reception of semiochemicals related to mating finding. This hypothesis is further supported by in silico analysis.
Volatile compounds emitted by R. prolixus female MGs are known to modulate male orientation and to increase copulation attempts (Pontes et al., 2014). Of the 12 compounds identified in MGs, four are considered putative sex pheromones, namely, 2-methyl-3-buten-2-ol, (2S)-pentanol, (3E)-2-methyl-3-penten-2-ol, and (2R/2S)-4-methyl-3-penten-2-ol (Pontes et al., 2008). Docking results (Figure 5) indicate favorable interactions with all four tested ligands due to negative values calculated for free binding energy. Even when a more restricted analysis, based on previous studies for predicting behaviorally active compounds (Jayanthi et al., 2014), is used to estimate binding potential, 2-methyl-3-penten-2-ol and (2R/2S)-4-methyl-3-penten-2-ol still meet the criteria for high binding affinity to RproOBP27. These results further support our hypothesis that RproOBP27 is a carrier of female-derived semiochemicals.
In the Lucerne plant bug, Adelphocoris lineolatus, expression of OBP1 is 1.91 times higher in male than in female antennae and this protein was shown to exhibit high binding affinity with two putative pheromone components (Gu et al., 2011b). Recent study suggested that OBP expression could be regulated by nutritional state. In A. lineolatus starvation significantly increased expression of AlinOBP13 in male and female antenna (Sun et al., 2014). Likewise, starved R. prolixus males did not express RproOBP27 (Supplementary Figure S4B), which was found only in the antennae of fed males. This dataset is consistent with the findings that unfed males from this species do not respond to sexual signals (Baldwin et al., 1971). Taking together, the evidence presented here strongly suggests that RporoOBP27 is likely involved in the reception of sex pheromone(s).
Author Contributions
AM and WL designed the project and experiments. DO, NB, and TF performed the experiments. DO, NB, TF, MM, WL, and AM analyzed the data. DO, NB, WL, and AM wrote the paper. DO, NB, TF, MM, WL, and AM revised the paper.
Funding
This work was supported by grants from Fundação Carlos Chagas Filho de Amparo à Pesquisa do Estado do Rio de Janeiro (FAPERJ), Conselho Nacional de Desenvolvimento Científico e Tecnológico (CNPq), Instituto Nacional de Ciência e Tecnologia em Entomologia Molecular (Brazil) (INCT-EM/CNPq), and Coordenação de Aperfeiçoamento de Pessoal de Nível Superior (CAPES). Work at the University of California, Davis was supported in part by National Institute of Allergy and Infectious Diseases of the National Institutes of Health (NIH) (Grant No. R01AI095514).
Conflict of Interest Statement
The authors declare that the research was conducted in the absence of any commercial or financial relationships that could be construed as a potential conflict of interest.
Acknowledgments
The authors are grateful to Lauriene D. Severino, Yasmin de Paule Gutierrez Simão, and Desenir Adriano Pedro for their excellent technical assistance.
Supplementary Material
The Supplementary Material for this article can be found online at: https://www.frontiersin.org/articles/10.3389/fphys.2018.01175/full#supplementary-material
FIGURE S1 | Expression profile of (A) RproOBP1 and RproOBP24; (B) RproOBP26; (C) RproOBP11 and RproOBP13; (D) RproOBP12 and RproOBP7 in different R. prolixus tissues evaluated by conventional PCR. Original 1% agarose gel stained with GelRedTM. M, molecular weight; N, negative control; FA, female antennae; MA, male antennae; FP, female proboscis; MP, male proboscis; FH, female head; MH, male head; FL, female legs; ML, male legs.
FIGURE S2 | Expression profile of (A) RproOBP14 and RproOBP6; (B) RproOBP17; (C) RproOBP18 and RproOBP20; (D) RproOBP21 in different R. prolixus tissues evaluated by conventional PCR. Original 1% agarose gel stained with GelRedTM. M, molecular weight; N, negative control; FA, female antennae; MA, male antennae; FP, female proboscis; MP, male proboscis; FH, female head; MH, male head; FL, female legs; ML, male legs.
FIGURE S3 | Expression profile of (A) RproOBP22; (B) RproOBP22 and RproOBP23; (C) RproOBP22 and RproOBP26; (D) RproOBP29 and RproOBP27 in different R. prolixus tissues evaluated by conventional PCR. Original 1% agarose gel stained with GelRedTM. M, molecular weight; N, negative control; FA, female antennae; MA, male antennae; FP, female proboscis; MP, male proboscis; FH, female head; MH, male head; FL, female legs; ML, male legs.
FIGURE S4 | Expression profile of (A) RproR18S; (B) RproOBP27, RproOBP26, and RproOBP21 in different R. prolixus tissues evaluated by conventional PCR. Original 1% agarose gel stained with GelRedTM. M, molecular weight; N, negative control; FA, female antennae; MA, male antennae; FP, female proboscis; MP, male proboscis; FH, female head; MH, male head; FL, female legs; ML, male legs.
FIGURE S5 | Device used in female recognition bioassay. A polystyrene tube (10 × 2 cm) divided into three zones: female zone (FZ), intermediate zone (IZ), and male release zone (MZ). A gate divides the MZ from IZ. A protective mesh was used to separate MZ and IZ from FZ. An adult female was placed in front of the protection mesh attached by a tape on the tube. Then a male was placed in the MZ and the gate was opened. Adapted from Zermoglio et al. (2015).
FIGURE S6 | PROCHECK results from predicted 3D model of RproOBP27.
FIGURE S7 | Verify3D results from predicted 3D model of RproOBP27.
FIGURE S8 | 3D model of RproOBP27 docked with metasternal gland volatile compounds (putative sex pheromones). (A) RproOBP27 docked with (3E)-2-methyl-3-penten-2-ol. (B) RproOBP27 docked with (2R/2S)-4-methyl-3-penten-2-ol.
FIGURE S9 | RproOBP27 sequence. The signal peptide is highlighted in red.
TABLE S1 | Oligonucleotides used in the PCR, qPCR and dsRNA synthesis reactions.
Footnotes
References
Baldwin, W. F., Knight, A. G., and Lynn, K. R. (1971). A sex pheromone in the insect Rhodnius prolixus (Hemiptera: Reduviidae). Can. Entomol. 103, 18–22. doi: 10.4039/Ent10318-1
Benton, R. (2006). On the ORigin of smell: odorant receptors in insects. Cell Mol. Life Sci. 63, 1579–1585. doi: 10.1007/s00018-006-6130-7
Benton, R., Vannice, K. S., Gomez-Diaz, C., and Vosshall, L. B. (2009). Variant ionotropic glutamate receptors as chemosensory receptors in Drosophila. Cell 136, 149–162. doi: 10.1016/j.cell.2008.12.001
Biessmann, H., Andronopoulou, E., Biessmann, M. R., Douris, V., Dimitratos, S. D., Eliopoulos, E., et al. (2010). The Anopheles gambiae odorant binding protein 1 (AgamOBP1) mediates indole recognition in the antennae of female mosquitoes. PLoS One 5:e9471. doi: 10.1371/journal.pone.0009471
Bikadi, Z., and Hazai, E. (2009). Application of the PM6 semi-empirical method to modeling proteins enhances docking accuracy of AutoDock. J. Cheminform. 1:15. doi: 10.1186/1758-2946-1-15
Bohbot, J., and Vogt, R. G. (2005). Antennal expressed genes of the yellow fever mosquito (Aedes aegypti L.); characterization of odorant-binding protein 10 and takeout. Insect Biochem. Mol. Biol. 35, 961–979. doi: 10.1016/j.ibmb.2005.03.010
Brito, N. F., Moreira, M. F., and Melo, A. C. (2016). A look inside odorant-binding proteins in insect chemoreception. J. Insect Physiol. 95, 51–65. doi: 10.1016/j.jinsphys.2016.09.008
Chen, X. G., Mathur, G., and James, A. A. (2008). Gene expression studies in mosquitoes. Adv. Genet. 64, 19–50. doi: 10.1016/S0065-2660(08)00802-X
Cruz-Lopez, L., Malo, E. A., Rojas, J. C., and Morgan, E. D. (2001). Chemical ecology of triatomine bugs: vectors of Chagas disease. Med. Vet. Entomol. 15, 351–357. doi: 10.1046/j.0269-283x.2001.00340.x
Dansa-Petretski, M., Ribeiro, J. M., Atella, G. C., Masuda, H., and Oliveira, P. L. (1995). Antioxidant role of Rhodnius prolixus heme-binding protein. Protection against heme-induced lipid peroxidation. J. Biol. Chem. 270, 10893–10896. doi: 10.1074/jbc.270.18.10893
de Bruyne, M., and Baker, T. C. (2008). Odor detection in insects: volatile codes. J. Chem. Ecol. 34, 882–897. doi: 10.1007/s10886-008-9485-4
Deng, Y., Yan, H., Gu, J., Xu, J., Wu, K., Tu, Z., et al. (2013). Molecular and functional characterization of odorant-binding protein genes in an invasive vector mosquito, Aedes albopictus. PLoS One 8:e68836. doi: 10.1371/journal.pone.0068836
Diaz-Albiter, H. M., Ferreira, T. N., Costa, S. G., Rivas, G. B., Gumiel, M., Cavalcante, D. R., et al. (2016). Everybody loves sugar: first report of plant feeding in triatomines. Parasit. Vectors 9:114. doi: 10.1186/s13071-016-1401-0
Dickens, J. C., Callahan, F. E., Wergin, W. P., Murphy, C. A., and Vogt, R. G. (1998). Intergeneric distribution and immunolocalization of a putative odorant-binding protein in true bugs (Hemiptera, Heteroptera). J. Exp. Biol. 201(Pt 1), 33–41.
Du, X., Li, Y., Xia, Y. L., Ai, S. M., Liang, J., Sang, P., et al. (2016). Insights into protein-ligand interactions: mechanisms, models, and methods. Int. J. Mol. Sci. 17:E144. doi: 10.3390/ijms17020144
Eisenberg, D., Luthy, R., and Bowie, J. U. (1997). VERIFY3D: assessment of protein models with three-dimensional profiles. Methods Enzymol. 277, 396–404. doi: 10.1016/S0076-6879(97)77022-8
Fan, J., Francis, F., Liu, Y., Chen, J. L., and Cheng, D. F. (2011). An overview of odorant-binding protein functions in insect peripheral olfactory reception. Genet. Mol. Res. 10, 3056–3069. doi: 10.4238/2011.December.8.2
Forêt, S., and Maleszka, R. (2006). Function and evolution of a gene family encoding odorant binding-like proteins in a social insect, the honey bee (Apis mellifera). Genome Res. 16, 1404–1413. doi: 10.1101/gr.5075706
Franco, T. A., Oliveira, D. S., Moreira, M. F., Leal, W. S., and Melo, A. C. (2016). Silencing the odorant receptor co-receptor RproOrco affects the physiology and behavior of the Chagas disease vector Rhodnius prolixus. Insect Biochem. Mol. Biol. 69, 82–90. doi: 10.1016/j.ibmb.2015.02.012
Gaillard, I., Rouquier, S., and Giorgi, D. (2004). Olfactory receptors. Cell. Mol. Life Sci. 61, 456–469. doi: 10.1007/s00018-003-3273-7
Gu, S. H., Wang, S. P., Zhang, X. Y., Wu, K. M., Guo, Y. Y., Zhou, J. J., et al. (2011a). Identification and tissue distribution of odorant binding protein genes in the lucerne plant bug Adelphocoris lineolatus (Goeze). Insect Biochem. Mol. Biol. 41, 254–263. doi: 10.1016/j.ibmb.2011.01.002
Gu, S. H., Wang, W. X., Wang, G. R., Zhang, X. Y., Guo, Y. Y., Zhang, Z., et al. (2011b). Functional characterization and immunolocalization of odorant binding protein 1 in the lucerne plant bug, Adelphocoris lineolatus (GOEZE). Arch. Insect Biochem. Physiol. 77, 81–99. doi: 10.1002/arch.20427
Guerenstein, P. G., and Lazzari, C. R. (2009). Host-seeking: how triatomines acquire and make use of information to find blood. Acta Trop. 110, 148–158. doi: 10.1016/j.actatropica.2008.09.019
He, P., Zhang, J., Liu, N.-Y., Zhang, Y.-N., Yang, K., and Dong, S.-L. (2011). Distinct expression profiles and different functions of odorant binding proteins in Nilaparvata lugens Sta°l. PLoS One 6:e28921. doi: 10.1371/journal.pone.0028921
Hull, J. J., Perera, O. P., and Snodgrass, G. L. (2014). Cloning and expression profiling of odorant-binding proteins in the tarnished plant bug, Lygus lineolaris. Insect Mol. Biol. 23, 78–97. doi: 10.1111/imb.12064
Jayanthi, K. P., Kempraj, V., Aurade, R. M., Roy, T. K., Shivashankara, K. S., and Verghese, A. (2014). Computational reverse chemical ecology: virtual screening and predicting behaviorally active semiochemicals for Bactrocera dorsalis. BMC Genomics 15:209. doi: 10.1186/1471-2164-15-209
Kelley, L. A., Mezulis, S., Yates, C. M., Wass, M. N., and Sternberg, M. J. (2015). The Phyre2 web portal for protein modeling, prediction and analysis. Nat. Protoc. 10, 845–858. doi: 10.1038/nprot.2015.053
Kim, S., Thiessen, P. A., Bolton, E. E., Chen, J., Fu, G., Gindulyte, A., et al. (2016). PubChem substance and compound databases. Nucleic Acids Res. 4, D1202–D1213. doi: 10.1093/nar/gkv951
Laskowski, R. A., Rullmannn, J. A., MacArthur, M. W., Kaptein, R., and Thornton, J. M. (1996). AQUA and PROCHECK-NMR: programs for checking the quality of protein structures solved by NMR. J. Biomol. NMR 8, 477–486. doi: 10.1007/BF00228148
Leal, W. S. (2012). Odorant reception in insects: roles of receptors, binding proteins, and degrading enzymes. Annu. Rev. Entomol. 58, 373–391. doi: 10.1146/annurev-ento-120811-153635
Li, J., Zhang, L., and Wang, X. (2016). An odorant-binding protein involved in perception of host plant odorants in locust Locusta migratoria. Arch. Insect Biochem. Physiol. 91, 221–229. doi: 10.1002/arch.21319
Lin, X., Jiang, Y., Zhang, L., and Cai, Y. (2017). Effects of insecticides chlorpyrifos, emamectin benzoate and fipronil on Spodoptera litura might be mediated by OBPs and CSPs. Bull. Entomol. Res. 4, 1–9. doi: 10.1017/S0007485317001195
Liu, R., Lehane, S., He, X., Lehane, M., Hertz-Fowler, C., Berriman, M., et al. (2010). Characterisations of odorant-binding proteins in the tsetse fly Glossina morsitans morsitans. Cell. Mol. Life Sci. 67, 919–929. doi: 10.1007/s00018-009-0221-1
Livak, K. J., and Schmittgen, T. D. (2001). Analysis of relative gene expression data using real-time quantitative PCR and the 2-ΔΔCT Method. Methods 25, 402–408. doi: 10.1006/meth.2001.1262
Majerowicz, D., Alves-Bezerra, M., Logullo, R., Fonseca-de-Souza, A. L., Meyer-Fernandes, J. R., Braz, G. R., et al. (2011). Looking for reference genes for real-time quantitative PCR experiments in Rhodnius prolixus (Hemiptera: Reduviidae). Insect Mol. Biol. 20, 713–722. doi: 10.1111/j.1365-2583.2011.01101.x
Mastrobuoni, G., Qiao, H., Iovinella, I., Sagona, S., Niccolini, A., Boscaro, F., et al. (2013). A proteomic investigation of soluble olfactory proteins in Anopheles gambiae. PLoS One 8:e75162. doi: 10.1371/journal.pone.0075162
Mesquita, R. D., Vionette-Amaral, R. J., Lowenberger, C., Rivera-Pomar, R., Monteiro, F. A., Minx, P., et al. (2015). Genome of Rhodnius prolixus, an insect vector of Chagas disease, reveals unique adaptations to hematophagy and parasite infection. Proc. Natl. Acad. Sci. U.S.A. 112, 14936–14941. doi: 10.1073/pnas.1506226112
Oliveira, D. S., Brito, N. F., Nogueira, F. C. S., Moreira, M. F., Leal, W. S., Soares, M. R., et al. (2017). Proteomic analysis of the kissing bug Rhodnius prolixus antenna. J. Insect Physiol. 100, 108–118. doi: 10.1016/j.jinsphys.2017.06.004
Otalora-Luna, F., Perret, J. L., and Guerin, P. M. (2004). Appetence behaviours of the triatomine bug Rhodnius prolixus on a servosphere in response to the host metabolites carbon dioxide and ammonia. J. Comp. Physiol. A Neuroethol. Sens. Neural Behav. Physiol. 190, 847–854. doi: 10.1007/s00359-004-0540-5
Pelletier, J., Guidolin, A., Syed, Z., Cornel, A. J., and Leal, W. S. (2010). Knockdown of a mosquito odorant-binding protein involved in the sensitive detection of oviposition attractants. J. Chem. Ecol. 36, 245–248. doi: 10.1007/s10886-010-9762-x
Pelletier, J., and Leal, W. S. (2009). Genome analysis and expression patterns of odorant-binding proteins from the Southern house mosquito Culex pipiens quinquefasciatus. PLoS One 4:e6237. doi: 10.1371/journal.pone.0006237
Pelosi, P., Iovinella, I., Zhu, J., Wang, G., and Dani, F. R. (2018). Beyond chemoreception: diverse tasks of soluble olfactory proteins in insects. Biol. Rev. Camb. Philos. Soc. 93, 184–200. doi: 10.1111/brv.12339
Pitts, R. J., Mozuraitis, R., Gauvin-Bialecki, A., and Lemperiere, G. (2014). The roles of kairomones, synomones and pheromones in the chemically-mediated behaviour of male mosquitoes. Acta Trop. 132(Suppl.), S26–S34. doi: 10.1016/j.actatropica.2013.09.005
Pontes, G., Zacharias, C. A., Manrique, G., and Lorenzo, M. G. (2014). Female odours promote the activation of sheltered kissing bug Rhodnius prolixus males and modulate their orientation. Med. Vet. Entomol. 28, 257–263. doi: 10.1111/mve.12040
Pontes, G. B., Bohman, B., Unelius, C. R., and Lorenzo, M. G. (2008). Metasternal gland volatiles and sexual communication in the triatomine bug. Rhodnius prolixus. J. Chem. Ecol. 34, 450–457. doi: 10.1007/s10886-008-9431-5
Pontes, G. B., and Lorenzo, M. G. (2012). Female metasternal gland odours mediate male aggregation in Rhodnius prolixus, a triatomid bug. Med. Vet. Entomol. 26, 33–36. doi: 10.1111/j.1365-2915.2011.00983.x
Rebijith, K. B., Asokan, R., Hande, H. R., Kumar, N. K., Krishna, V., Vinutha, J., et al. (2016). RNA interference of odorant-binding protein 2 (OBP2) of the cotton aphid, Aphis gossypii (Glover), resulted in altered electrophysiological responses. Appl. Biochem. Biotechnol. 178, 251–266. doi: 10.1007/s12010-015-1869-7
Ribeiro, J. M., Genta, F. A., Sorgine, M. H., Logullo, R., Mesquita, R. D., Paiva-Silva, G. O., et al. (2014). An insight into the transcriptome of the digestive tract of the bloodsucking bug, Rhodnius prolixus. PLoS Negl. Trop. Dis. 8:e2594. doi: 10.1371/journal.pntd.0002594
Rolandi, C., and Schilman, P. E. (2017). Aggregated oviposition in Rhodnius prolixus, sensory cues and physiological consequences. J. Insect Physiol. 98, 74–82. doi: 10.1016/j.jinsphys.2016.12.001
Sanchez-Gracia, A., Vieira, F. G., and Rozas, J. (2009). Molecular evolution of the major chemosensory gene families in insects. Heredity 103, 208–216. doi: 10.1038/hdy.2009.55
Sant’Anna, M. R., Soares, A. C., Araujo, R. N., Gontijo, N. F., and Pereira, M. H. (2017). Triatomines (Hemiptera, Reduviidae) blood intake: physical constraints and biological adaptations. J. Insect Physiol. 97, 20–26. doi: 10.1016/j.jinsphys.2016.08.004
Schultze, A., Schymura, D., Forstner, M., and Krieger, J. (2012). Expression pattern of a ‘Plus-C’ class odorant binding protein in the antenna of the malaria vector Anopheles gambiae. Insect Mol. Biol. 21, 187–195. doi: 10.1111/j.1365-2583.2011.01125.x
Shorter, J. R., Dembeck, L. M., Everett, L. J., Morozova, T. V., Arya, G. H., Turlapati, L., et al. (2016). Obp56h modulates mating behavior in Drosophila melanogaster. G3 13, 3335–3342. doi: 10.1534/g3.116.034595
Sun, L., Xiao, H. J., Gu, S. H., Zhou, J. J., Guo, Y. Y., Liu, Z. W., et al. (2014). The antenna-specific odorant-binding protein AlinOBP13 of the alfalfa plant bug Adelphocoris lineolatus is expressed specifically in basiconic sensilla and has high binding affinity to terpenoids. Insect Mol. Biol. 23, 417–434. doi: 10.1111/imb.12089
Swarup, S., Williams, T. I., and Anholt, R. R. (2011). Functional dissection of odorant binding protein genes in Drosophila melanogaster. Genes Brain Behav. 10, 648–657. doi: 10.1111/j.1601-183X.2011.00704.x
Syed, Z., and Leal, W. S. (2009). Acute olfactory response of Culex mosquitoes to a human- and bird-derived attractant. Proc. Natl. Acad. Sci. U.S.A. 106, 18803–18808. doi: 10.1073/pnas.0906932106
Tomberlin, J. K., Crippen, T. L., Wu, G., Griffin, A. S., Wood, T. K., and Kilner, R. M. (2016). Indole: an evolutionarily conserved influencer of behavior across kingdoms. Bioessays 39:1600203. doi: 10.1002/bies.201600203
Vitta, A. C., Bohman, B., Unelius, C. R., and Lorenzo, M. G. (2009). Behavioral and electrophysiological responses of Triatoma brasiliensis males to volatiles produced in the metasternal glands of females. J. Chem. Ecol. 35, 1212–1221. doi: 10.1007/s10886-009-9709-2
Vogt, R. G. (2002). Odorant binding protein homologues of the malaria mosquito Anopheles gambiae; possible orthologues of the OS-E and OS-F OBPs OF Drosophila melanogaster. J. Chem. Ecol. 28, 2371–2376. doi: 10.1023/A:1021009311977
Vogt, R. G., Callahan, F. E., Rogers, M. E., and Dickens, J. C. (1999). Odorant binding protein diversity and distribution among the insect orders, as indicated by LAP, an OBP-related protein of the true bug Lygus lineolaris (Hemiptera, Heteroptera). Chem. Senses 24, 481–495. doi: 10.1093/chemse/24.5.481
WHO (2015). Chagas disease in Latin America: an epidemiological update based on 2010 estimates. Wkly Epidemiol. Rec. 90, 33–43.
Wojtasek, H., and Leal, W. S. (1999). Conformational change in the pheromone-binding protein from Bombyx mori induced by pH and by interaction with membranes. J. Biol. Chem. 274, 30950–30956. doi: 10.1074/jbc.274.43.30950
Wyatt, T. D. (2014). “Introduction to chemical signaling in vertebrates and invertebrates,” in Neurobiology of Chemical Communication, ed. C. Mucignat-Caretta (Baton Rouge: CRC Press).
Xu, P., Choo, Y. M., De La Rosa, A., and Leal, W. S. (2014). Mosquito odorant receptor for DEET and methyl jasmonate. Proc. Natl. Acad. Sci. U.S.A. 111, 16592–16597. doi: 10.1073/pnas.1417244111
Yin, J., Choo, Y. M., Duan, H., and Leal, W. S. (2015). Selectivity of odorant-binding proteins from the southern house mosquito tested against physiologically relevant ligands. Front. Physiol. 6:56. doi: 10.3389/fphys.2015.00056
Zermoglio, P. F., Martin-Herrou, H., Bignon, Y., and Lazzari, C. R. (2015). Rhodnius prolixus smells repellents: behavioural evidence and test of present and potential compounds inducing repellency in Chagas disease vectors. J. Insect Physiol. 81, 137–144. doi: 10.1016/j.jinsphys.2015.07.012
Zhang, X. Y., Zhu, X. Q., Gu, S. H., Zhou, Y. L., Wang, S. Y., Zhang, Y. J., et al. (2016). Silencing of odorant binding protein gene AlinOBP4 by RNAi induces declining electrophysiological responses of Adelphocoris lineolatus to six semiochemicals. Insect Sci. 24, 789–797. doi: 10.1111/1744-7917.12365
Keywords: odorant binding proteins, Rhodnius prolixus, RNAi, sexual behavior, olfaction
Citation: Oliveira DS, Brito NF, Franco TA, Moreira MF, Leal WS and Melo ACA (2018) Functional Characterization of Odorant Binding Protein 27 (RproOBP27) From Rhodnius prolixus Antennae. Front. Physiol. 9:1175. doi: 10.3389/fphys.2018.01175
Received: 28 April 2018; Accepted: 06 August 2018;
Published: 23 August 2018.
Edited by:
Peng He, Guizhou University, ChinaReviewed by:
Guan-Heng Zhu, University of Kentucky, United StatesYa-Nan Zhang, Huaibei Normal University, China
Joe Hull, Agricultural Research Service (USDA), United States
George F. Obiero, Max-Planck-Institut für Chemische Ökologie, Germany
Copyright © 2018 Oliveira, Brito, Franco, Moreira, Leal and Melo. This is an open-access article distributed under the terms of the Creative Commons Attribution License (CC BY). The use, distribution or reproduction in other forums is permitted, provided the original author(s) and the copyright owner(s) are credited and that the original publication in this journal is cited, in accordance with accepted academic practice. No use, distribution or reproduction is permitted which does not comply with these terms.
*Correspondence: Ana C. A. Melo, YW5hbWVsb0BpcS51ZnJqLmJy