- 1Physical Effort Laboratory, Sports Center, Federal University of Santa Catarina, Florianopolis, Brazil
- 2Leonardo da Vinci University/Uniasselvi, Indaial, Brazil
- 3School of Sport and Service Management, University of Brighton, Eastbourne, United Kingdom
- 4Exercise Physiology Laboratory, Department of Medicine, Università Degli Studi di Udine, Udine, Italy
- 5Human Performance Laboratory, São Paulo State University, Rio Claro, Brazil
A link between muscle fatigue, decreased efficiency and the slow component of oxygen uptake (VO2sc) has been suggested. However, a cause-effect relationship remains to be elucidated. Although alterations in VO2 kinetics after elevated baseline work rate have previously been reported, to date no study has observed the effect on muscle force production (MFP) behavior considering physiological differences between male and female subjects. This study investigated the effect of elevated baseline work rate on the VO2 kinetics and MFP in 10 male and 10 female healthy subjects. Subjects performed 4 transitions of very-heavy (VH) intensity cycling in a randomized order after unloaded (U-VH) or moderate (M-VH) exercise. Maximal isokinetic efforts (MIE) were performed before and after each condition at two different cadences (60 or 120 rpm). Whereas baseline VO2 and time constant (τ) were significantly higher in M-VH compared to U-VH, the fundamental amplitude and the VO2 slow component (VO2sc) were significantly lower in M-VH (p < 0.05) in both sexes. Blood lactate concentration ([La]) and rate of perceived exertion (RPE) were not influenced by condition or sex (p > 0.05). The MFP post-exercise was not significantly influenced by condition in both sexes and cadences (Δtorque for males: at 60 rpm in U-VH = 13 ± 10 Nm, in M-VH = 13 ± 9 Nm; at 120 rpm in U-VH = 22 ± 14 Nm, in M-VH = 21 ± 12 Nm; for females: at 120 rpm in U-VH = 10 ± 9 Nm, in M-VH = 12 ± 8 Nm; p > 0.05), with the exception that female subjects presented smaller decreases in M-UH at 60 rpm compared to U-VH (11 ± 13 vs. 18 ± 14 Nm, respectively, p < 0.05). There was no correlation between the decrease in torque production and VO2 kinetics parameters (p > 0.05). The alterations in VO2 kinetics which have been suggested to be linked to changes in motor unit recruitment after elevated baseline work rate did not reflect alterations in MFP and fatigue in both sexes.
Introduction
Over the last decades, numerous studies have aimed to understand the physiological mechanisms underlying a loss of work efficiency or an increase in the O2 cost per unit of work during constant-load exercise above the gas exchange threshold (GET), i.e., the slow component of O2 uptake kinetics (VO2SC). Ever since, Poole et al. (1988, 1991) demonstrated that the mechanism explaining the VO2sc is likely within the exercising muscle. Thereafter, previous literature investigated motor unit recruitment and/or muscle fatigue without taking the lactate metabolism or the O2 consuming process outside the exercising limbs as a dominant mediator into consideration (Jones et al., 2011). Cannon et al. (2011) described muscle fatigue as an increased ATP cost of already recruited motor units, instead of the recruitment of less efficient muscle fibers, as the primary mechanisms explaining the VO2SC. Notwithstanding, Keir et al. (2016) showed a relationship between the VO2SC and the time course of peripheral muscle fatigue (muscle torque production in response to electrically stimulated contractions) during high-intensity exercise. Moreover, Temesi et al. (2017) suggested that subjects with slower VO2 kinetics (i.e., higher τ-values) experienced more peripheral fatigue during very-heavy (VH) cycling exercise. However, this relationship between VO2 kinetics and muscle fatigue remains to be elucidated. Hopker et al. (2016) analyzed the effect of muscle damage on VO2 kinetics and suggested that locomotor muscle fatigue does not influence the kinetic response, i.e., τ or the VO2SC. Furthermore, de Souza et al. (2016) showed differences in the VO2SC and τ despite a similar magnitude of muscle fatigue during VH cycling exercise. do Nascimento Salvador et al. (2018) demonstrated that prior cycling exercise decreased the VO2SC behavior, but did not modify the time-course of muscle torque production in a subsequent VH cycling bout.
Previous literature showed alterations in VO2 dynamic when high-intensity exercise was immediately preceded by an elevated baseline (elevated VO2-values and/or elevated work rate) (Hughson and Morrissey, 1982; Wilkerson and Jones, 2006; Jones et al., 2008; Da Boit et al., 2014; Wüst et al., 2014). Lower amplitudes of the fundamental component and VO2sc and a slower time response (i.e., higher τ-values) can be found during high-intensity exercise when preceded by an elevated baseline compared to unloaded pedaling. It has been suggested that these changes may represent adjustments in the muscle O2 delivery and/or the muscle recruitment of motor units which are characterized by less mitochondrial content, lower metabolic efficiency and are positioned higher in the muscle recruitment hierarchy (i.e., type II fibers) (Bearden and Moffatt, 2001; Wilkerson and Jones, 2006). Dimenna et al. (2010) stated that it is not the elevated baseline VO2 per se that explains a slower VO2 kinetics, but the proportionally greater contribution of higher-order fibers to power production during transitions from an elevated baseline work rate. This greater contribution of type II fibers could alter the interaction between muscle efficiency and VO2 kinetics. Notably, the effect of elevated baseline on muscle force production (MFP) and its association with VO2 kinetics remains to be established.
To the best of our knowledge, no study investigated the relationship between VO2 kinetics and muscle fatigue with respect to the differences in pulmonary and neuromuscular capacities between male and female subjects. Females present smaller lung volumes, lower resting lung diffusion capacities and differences in O2 delivery, O2 extraction and blood flow (Mitchell et al., 1992; Harms, 2006; Murias et al., 2013; Dominelli et al., 2015). Lower muscle mass in females could be accompanied by a lower oxygen delivery and utilization. Reis et al. (2017) stated that the lower cardiac and respiratory capacities during exercise could reduce O2 delivery and utilization to the muscle, and consequently, lead to slower VO2 kinetics in females. Up to date, surprisingly little is known about the gender differences in VO2 kinetics (see Reis et al., 2017, for more details). Hunter (2014) indicated that females exhibit less fatigue (loss of maximal torque) than males for dynamic fatiguing contractions when the velocity of contraction was controlled. Moreover, there are differences between sexes in the neuromuscular activation pattern of the quadriceps muscle (Clark et al., 2005), in muscle mass activated (greater in males) (Enoka and Duchateau, 2008; Hunter, 2014), muscle fiber type (greater proportional of type II in men) and gene expression and interactions with sex-specific hormones (Maher et al., 2009; Liu et al., 2010). Thus, it is reasonable to state that differences in sex could influence the MFP behavior and consequently, VO2 kinetics during cycling exercise. If the relationship between muscle fatigue and VO2 kinetics is “cause and effect,” then this relation should be sex-specific. Regarding to these differences in muscle fiber type and fatigue in men and women, investigating muscle fatigue at different cadences might provide further mechanistic insight. It is already known that proportionally more type II fibers are activated at 120 rpm and therefore, isokinetic cycling at 120 rpm reflects predominantly type II fiber fatigue in contrast to cycling at 60 rpm which fatigues predominantly type I fibers (Sargeant, 2007; Cannon et al., 2011). Moreover, understanding the mechanistic bases of the VO2sc will be crucial when designing interventions to enhance performance (Jones et al., 2011). A better understanding of strategies to speed-up the phase II τ or reduce the VO2sc and muscle inefficiency associated with it has the potential to increase exercise tolerance in both, male and female subjects.
Thus, the main aim of the present study was to investigate whether changes in VO2 kinetics after elevated baseline work rate reflect alterations in MFP behavior during maximal isokinetic efforts at different cadences (60 and 120 rpm). Besides, to study whether differences between sexes could influence these changes. In a case of a cause-effect relationship between VO2 kinetics and muscle fatigue it was expected a “mirror image” between MFP behavior and VO2 kinetics. We hypothesized that (1) the work-to-work transitions lead to a lower VO2sc amplitude and a slower time constant in both sexes; (2) female subjects present a lower amplitude of fundamental and slow phases compared to male counterparts; (3) changes in VO2sc and τ after elevated baseline work rate are accompanied by alterations in MFP, for both velocities and both sexes.
Materials and Methods
Ethics Statement
The present work was approved by the Research Ethics Committee of the Federal University of Santa Catarina and was conducted in accordance with the Declaration of Helsinki. After being fully informed of the risks and stresses associated with the study, the participants gave their written informed consent to participate.
Participants
Twenty healthy participants (10 females: age 28 ± 6 years; mass 58 ± 7 kg; height 161 ± 5 cm; 10 males: age 26 ± 5 years; mass 75 ± 7 kg; height 177 ± 5 cm) volunteered to participate in the study. Subjects undertook exercise at a recreational level (3–4 sessions per week; 150–300 min per week), and were familiar with laboratory exercise testing procedures. Women performed the constant work-rate tests in the follicular phase being performed 2–4 days after the menses.
Overview of Study Design
Subjects were required to visit the laboratory on 5 occasions. On the first visit, each subject performed a maximal ramp test for the determination of the GET, VO2peak and peak power output (Ppeak). On subsequent visits, subjects performed bouts of very heavy-intensity exercise immediately following unloaded (20 W – U-VH) or moderate (95% GET – M-VH) baseline to verify the effects of work-to-work transitions on VO2 kinetics and muscle force behavior (Figure 1). A maximal isokinetic effort (MIE; constant pedal cadence at 60 or 120 rpm) was performed following a standardized warm-up and immediately following the constant work-rate cycling bout to quantify reductions in peak torque. Subjects were instructed to avoid any intake of caffeine for 3 h, or alcohol and strenuous exercise in the 24 h preceding the test sessions and to arrive at the laboratory in a rested and fully hydrated state, at least 2 h post-prandial. All tests were performed at the same time of day in a controlled environmental laboratory condition (19–22°C; 50–60% RH) to minimize the effects of diurnal biological variation. Subjects performed only one test on any given day, and each test was separated by at least 48 h but completed within a period of 2 weeks.
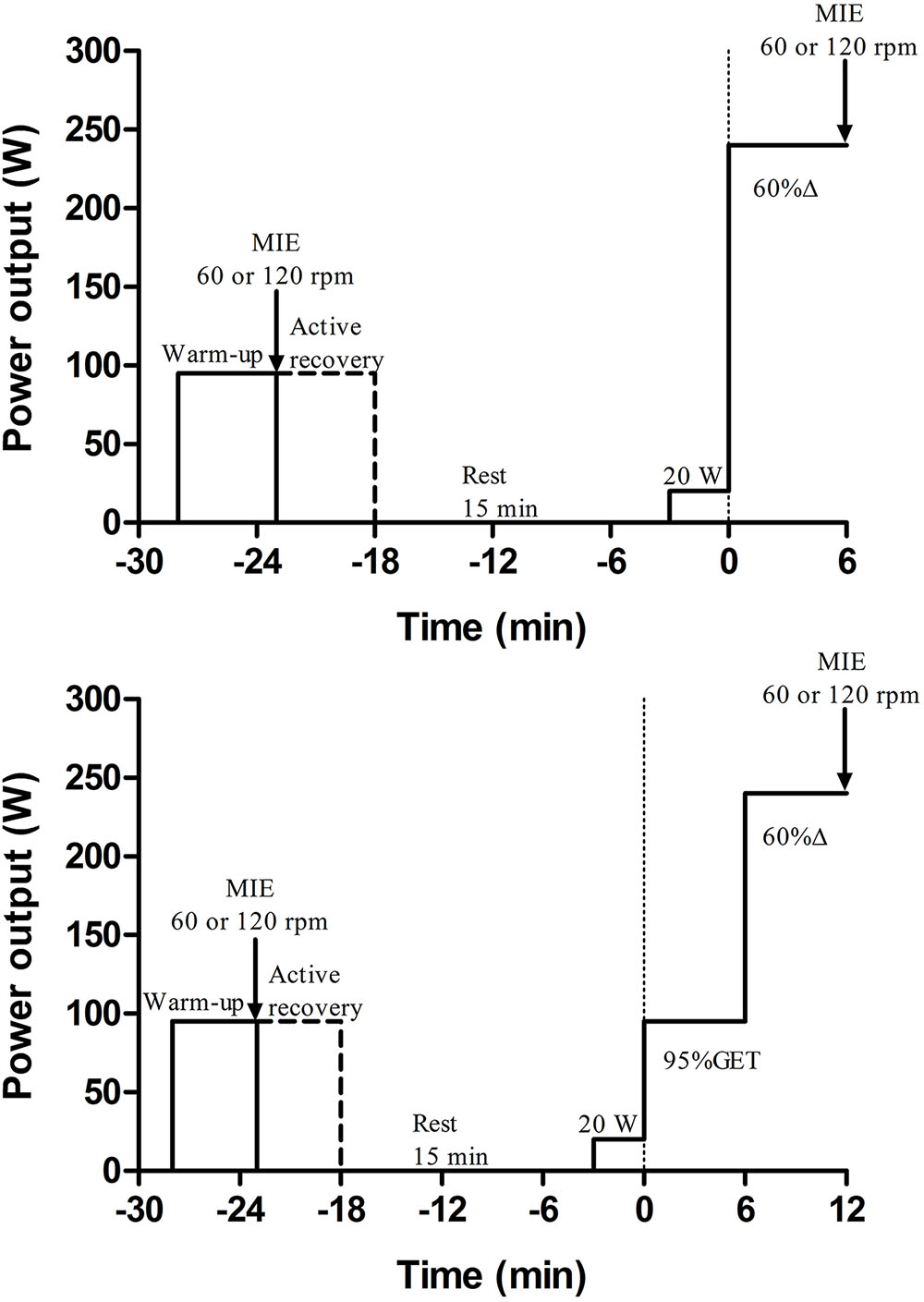
Figure 1. Experimental design for a representative participant. Top control condition (unloaded to very-heavy intensity exercise – U-VH); bottom experimental condition (moderate to very-heavy intensity exercise M-VH). Warm-up and active recovery were performed at 95% of the gas exchange threshold (GET). After a passive recovery period (15 min) participants started to cycling during 3 min of baseline (20 W) followed immediately by increases in the power output to 60% of the difference between the work-rate at the GET and VO2peak (60% Δ; U-VH) or to 95%GET plus 60% Δ (M-VH). MIE, maximal isokinetic effort. Please note that M-VH was 6 min longer than U-VH (moderate + VH exercise).
Equipment
All tests were performed on an electromagnetically braked cycle ergometer (Excalibur Sport PFM, Lode BV, Groningen, Netherlands). Respiratory and pulmonary gas exchange variables were measured using a breath-by-breath analyzer (Quark PFTergo, Cosmed, Rome, Italy). Before each test, the O2 and CO2 analysis systems were calibrated using ambient air (20.94% O2 and 0.03% CO2) and a gas of a known O2 and CO2 concentration (16.00% O2 and 5.00% CO2) according to the manufacturer’s instructions. Likewise, the turbine flow meter was calibrated before each test using a 3 L syringe (Quark PFTergo, Cosmed, Rome, Italy). A monitor connected to the gas analyzer was used to measure heart rate (HR). Capillary blood samples (25 μl) were obtained from the earlobe of each subject and the blood lactate concentration ([La]) was measured using an electrochemical analyzer (YSI 2700 STAT, Yellow Springs, OH, United States). The cycle ergometer, the breath-by-breath analyzer and the electrochemical analyzer were calibrated in accordance with specific manufacturer’s recommended procedures.
Determination of GET and VO2peak
On the first laboratory visit, 15 min after the isokinetic sprint familiarization subjects performed an incremental ramp test for the determination of the GET, VO2peak, and Ppeak. After a 4 min period of cycling at 20 W (baseline), an incremental ramp test to exhaustion was undertaken with power output increasing by a rate of 30 W.min-1 from the baseline. Subjects were instructed to maintain their preferred cadence (female 77 ± 6 rpm; male 82 ± 6 rpm) throughout the test. The preferred cadence along with saddle and handle bar height and configuration was recorded and replicated in subsequent tests. Each subject was verbally encouraged to undertake maximal effort. The test was terminated when the cadence fell by more than 10 rpm below the preferred cadence for more than 5 s despite strong verbal encouragement (Black et al., 2015). Breath-by-breath pulmonary gas exchange and HR data were measured continuously during the test and averaged over 15 s periods. VO2peak was defined as the highest value obtained in a 15 s interval, or if a VO2 plateau observed, it was considered as the average of the final minute of exercise (Day et al., 2003). The attainment of VO2peak was defined using the criteria proposed by Bassett and Howley (2000). The Ppeak was considered as the highest power output attained during the test. The GET was determined using a cluster of measurements as the V-slope method and the ventilatory equivalent method (Beaver et al., 1986). The data from the ramp test were used to calculate the work rate corresponding to 60% Δ (i.e., GET plus 60% of the difference between the work-rate at the GET and VO2peak). The lag in VO2 during incremental exercise taken into account by a deduction of two-thirds of the ramp rate from the work-rate at the GET (Burnley et al., 2011).
Maximal Isokinetic Effort Measurement
The cycle ergometer was instrumented with pedal force measurements (Lode PFM, Groningen, Netherlands) to quantify muscle fatigue during the MIE. A switch from the hyperbolic mode to the isokinetic mode happened instantaneously when required. This protocol was similar to previously used protocols (Cannon et al., 2011; de Souza et al., 2016; Hopker et al., 2016; do Nascimento Salvador et al., 2018) and considered as reliable (do Nascimento Salvador et al., 2018) (no differences were observed for both peak torque – intraclass correlation coefficient = 0.99, typical error = 3.7% and, peak power output – intraclass correlation coefficient = 0.99; typical error = 4.2% obtained during the pre-exercise assessments). Peak torque was assessed for each subject by a 5 s cycling sprint test in the isokinetic mode at 60 or 120 rpm. In the pre-exercise muscle function assessment, subjects performed a 5 min warm-up at 95% GET immediately followed by the 5 s MIE. After this, subjects performed 5 min of active recovery at 95% GET and a period of 15 min rest before the main exercise bouts. The MIE was repeated immediately after the exercise in U-VH and M-VH (see Figure 1). Subjects were given an auditory cue to begin the all-out effort in the seated position and strong verbal encouragement was given throughout the 5 s. The torque and power data were recorded continuously during the MIE. As described by Altenburg et al. (2007), the peak torque in each crank arm was determined as the average of the four consecutive highest torque values (2 s). Thus, the peak torque during the MIE was then considered as the average of the peak values of both left and right crank arms.
Data Analysis
Breath-by-breath data for each test were initially examined to exclude outlier values caused by sighs, swallowing and coughs (Lamarra et al., 1987). After that, for each exercise transition, the breath-by-breath data was processed and analyzed as described previously (do Nascimento et al., 2015; de Souza et al., 2016; do Nascimento Salvador et al., 2018). Non-linear regression techniques were used to fit the data after the onset of a fundamental phase with an exponential function (OriginPro 8; OriginLab). An iterative process ensured that the sum of squared errors was minimized. Due to large inter-individual differences in the duration of the exponential region (Murgatroyd et al., 2011), the identification of the VO2sc during the VH intensity exercise was performed individually. The fundamental VO2 kinetics (phase II) was isolated following the iterative method to identify the exponential region (Rossiter et al., 2001, 2002; Murgatroyd et al., 2011). The identification at the end of the fundamental phase (i.e., TDs) was performed by fitting a window from the start of the fundamental phase (i.e., after 20 s cardio-dynamic phase) initially set at 60 s. The window was lengthened iteratively until the exponential model fit demonstrated a discernible and consistent departure from the measured VO2-values by considering the criteria proposed in literature (Rossiter et al., 2001, 2002; Murgatroyd et al., 2011). Thus, the fitting window was constrained to this time point and a single-exponential fitting was performed only on the fundamental phase to identify the kinetics parameters. The model was constrained in VO2baseline to aid in the identification of the key parameters according to the following equation:
where: VO2(t) represents the value of VO2 at a given time (t); VO2baseline is the average value over the last minute of baseline cycling; A is the asymptotic amplitude for the exponential term describing changes in VO2 from baseline to its asymptote; τ is the time constant; and the TD is the time delay. The VO2SC was calculated according to the Equation (2):
Where: VO2end is the average VO2 value over the last 20 s of a 6 min exercise bout.
Statistical Analysis
Descriptive statistics are expressed as mean ± standard deviation. The Shapiro–Wilk test was applied to ensure a Gaussian distribution of the data (n < 50). A two-way mixed-model ANOVA was used to analyze the interaction over time and condition. Assumptions of sphericity were assessed using the Mauchly test, and any violation was corrected using the Greenhouse-Geisser correction factor. The Shapiro–Wilk test was used to verify the normality of residuals. When significant effects were observed the Bonferroni post hoc test was used for pairwise comparisons. Analyzes were performed using the Statistical Package for Social Sciences Windows (SPSS Inc. version 17.0; Chicago, IL, United States). The level of significance adopted was set at p < 0.05.
Results
HRmax, VO2peak, and Ppeak were 183 ± 10 bpm, 46.8 ± 8.2 ml.kg-1.min-1 and 351 ± 51 W for males and; 181 ± 7 bpm, 39.2 ± 8.3 ml.kg-1.min-1, and 235 ± 26 W for females, respectively (Table 1). The [La] at the beginning and at the end of the ramp test were 1.4 ± 0.3 and 11.0 ± 2.5 mmol.L-1 for males and; 1.7 ± 0.6 and 9.1 ± 1.9 mmol.L-1 for females, respectively. The 60% Δ was performed at 240 ± 33 W and 157 ± 23 W and represented 82 ± 3 and 85 ± 3% of VO2peak for male and female subjects, respectively.
Square-Wave Exercise Bouts
During M-VH, the VO2baseline was significantly higher, A, TD, and VO2sc lower and τ and TDs slower compared to U-VH for both sexes (p < 0.05). There were no significant differences for Atotal (i.e., A + VO2baseline) and VO2end between conditions for both sexes (p > 0.05; Table 2). Female subjects presented lower amplitudes, VO2sc and VO2end than male counterparts (p > 0.05; Figure 2). The [La] post-exercise was not significantly different between conditions or sexes (p > 0.05; Figure 3). There was no difference between conditions (p = 0.23) in both sexes (p = 0.31) for rate of perceived exertion (RPE), thus, male and female subjects perceived the effort in a similar way after the exercise in both conditions (Figure 4).
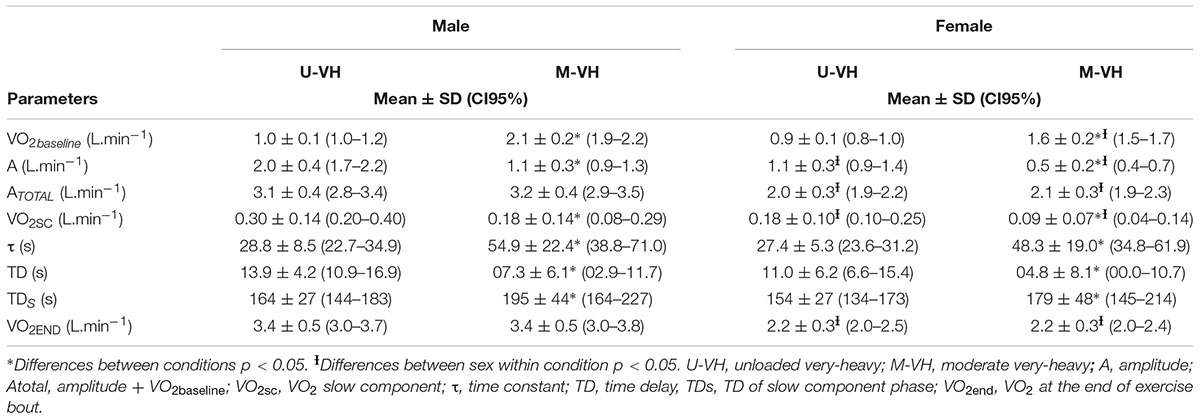
Table 2. VO2 kinetics responses during rest-to-work and work-to-work exercise in male and female subjects.
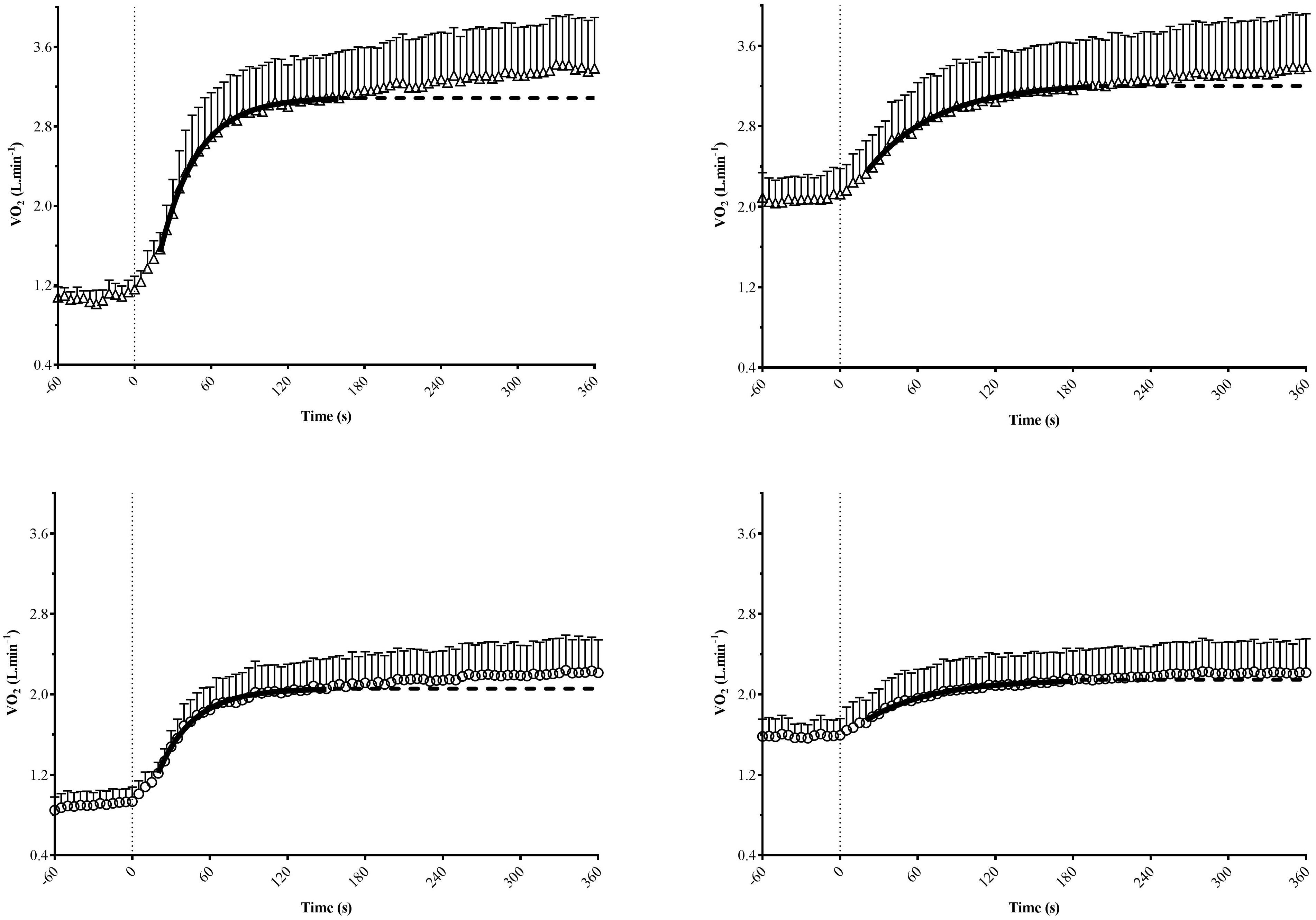
Figure 2. Mean group values of oxygen uptake (VO2) kinetics for male (top) and female (bottom) subjects during transitions from unloading (left) or moderate (right) exercise. Non-linear least-squares regression modeling (continuous black line), with the fit extrapolated (dashed line) to the end of exercise were showed. Standard deviation values were shown just on the upper side of mean symbols for clarity.
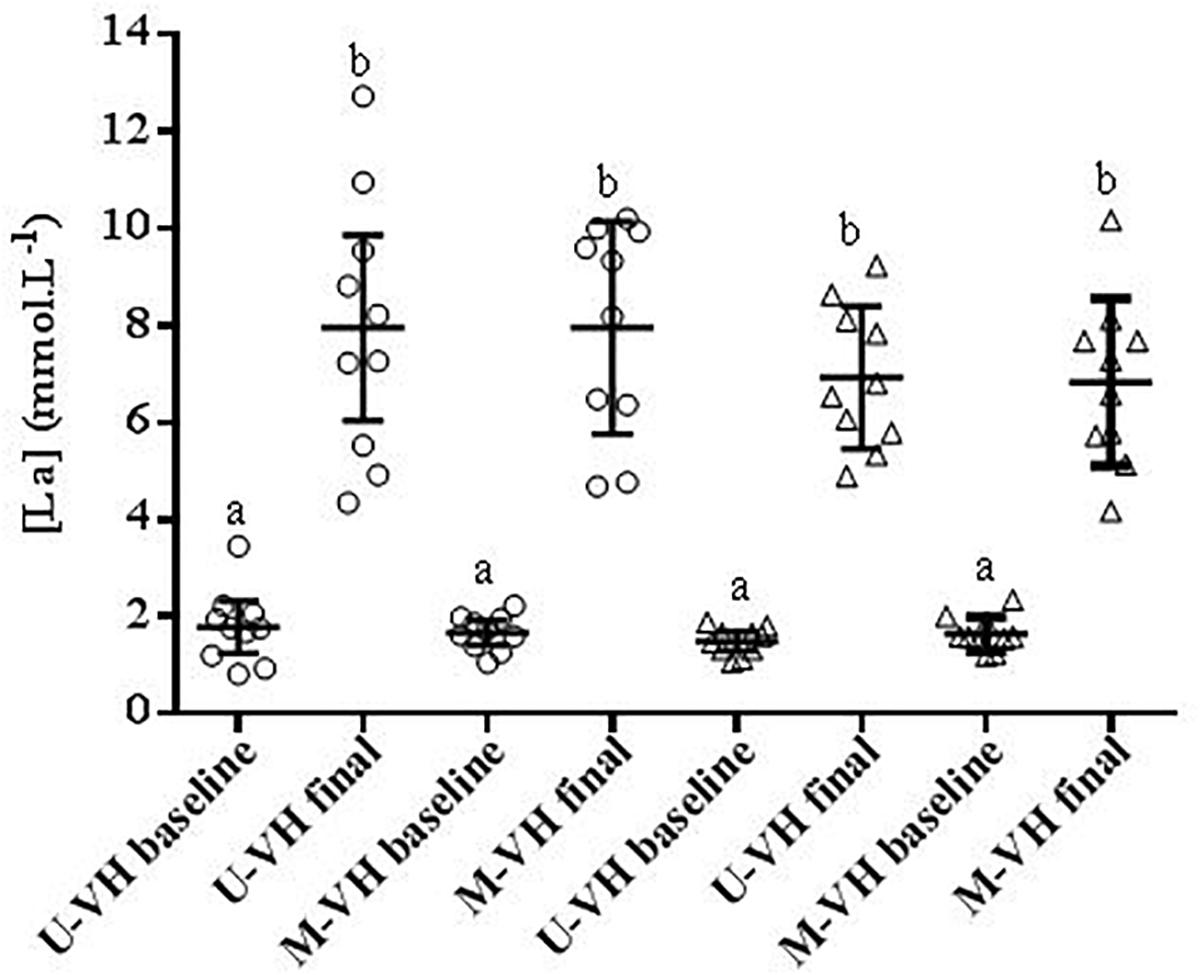
Figure 3. [La] = blood lactate concentration. Circle symbols show the female values. Triangle symbols show male values. U-VH, unloaded to very heavy intensity exercise; M-VH, moderate to very heavy intensity exercise. Different letters showed significant differences p < 0.05.
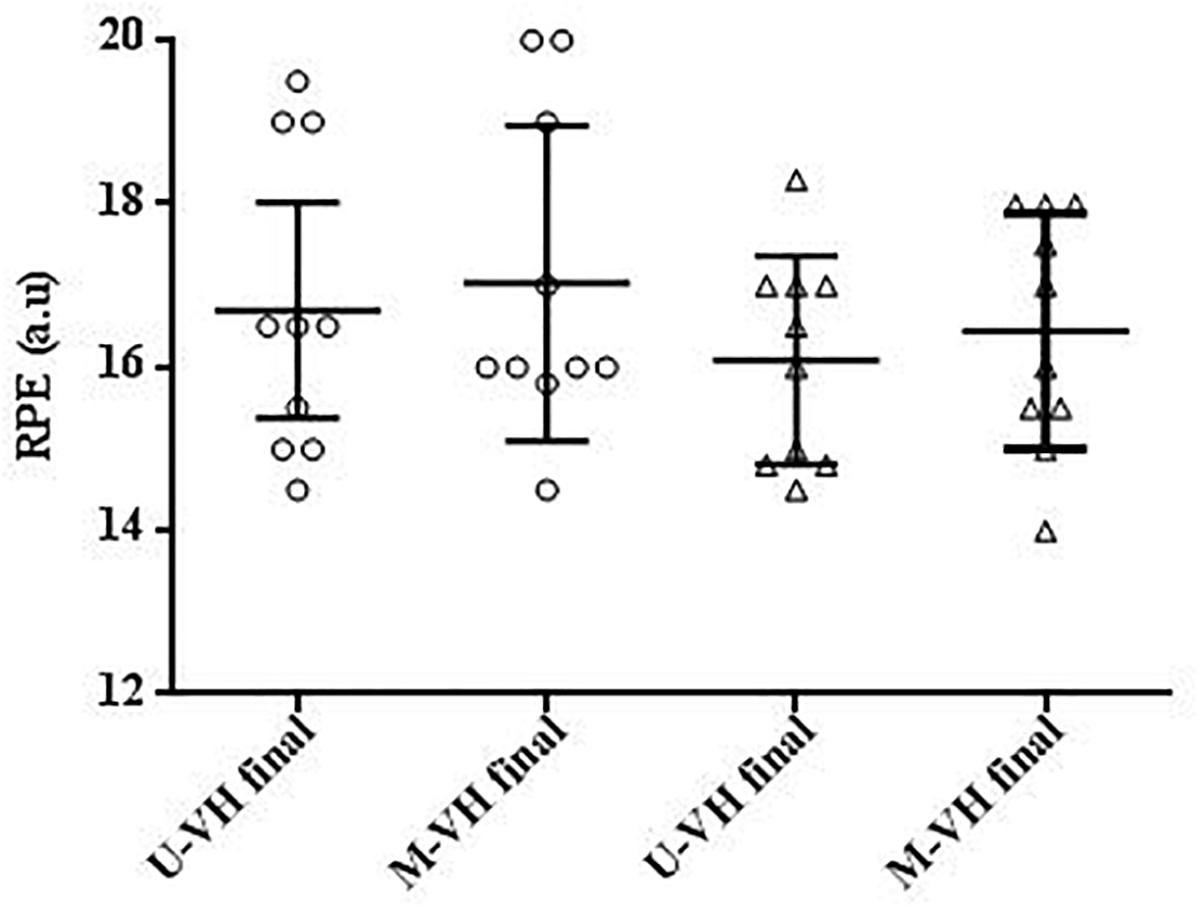
Figure 4. RPE = rating of perceived exertion. Circle symbols show female values. Triangle symbols show male values. U-VH, unloaded to very heavy intensity exercise; M-VH, moderate to very heavy intensity exercise.
Torque Production Behavior
There were no significant differences in MFP between conditions (U-VH vs. M-VH) for men in both velocities (60 rp main effect condition vs. time, F = 0.09; p = 0.77; 120 rp main effect condition vs. time, F = 0.48; p = 0.50). Female subjects showed no significant differences in MFP between conditions at 120 rpm (main effect condition vs. time, F = 0.36; p = 0.56), but smaller decreases in torque production were observed following M-VH at 60 rpm compared to U-VH (main effect condition vs. time, F = 18.2; p = 0.01). The torque decrement at 120 rpm was lower for female than male subjects (p < 0.05, Table 3 and Figure 5). There were no significant correlation between Δ torque and VO2SC or τ in the different conditions or velocities in both sexes.
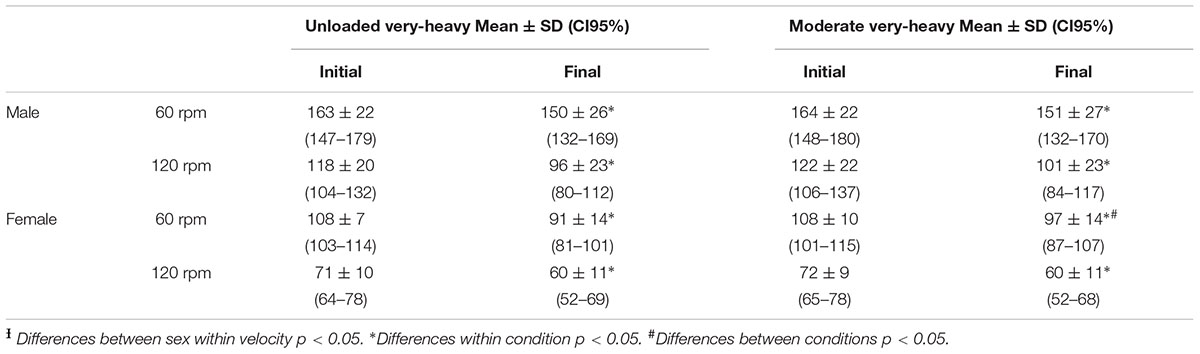
Table 3. Torque production (Nm) behavior before and after rest-to-work and work-to-work exercise in male and female subjects.
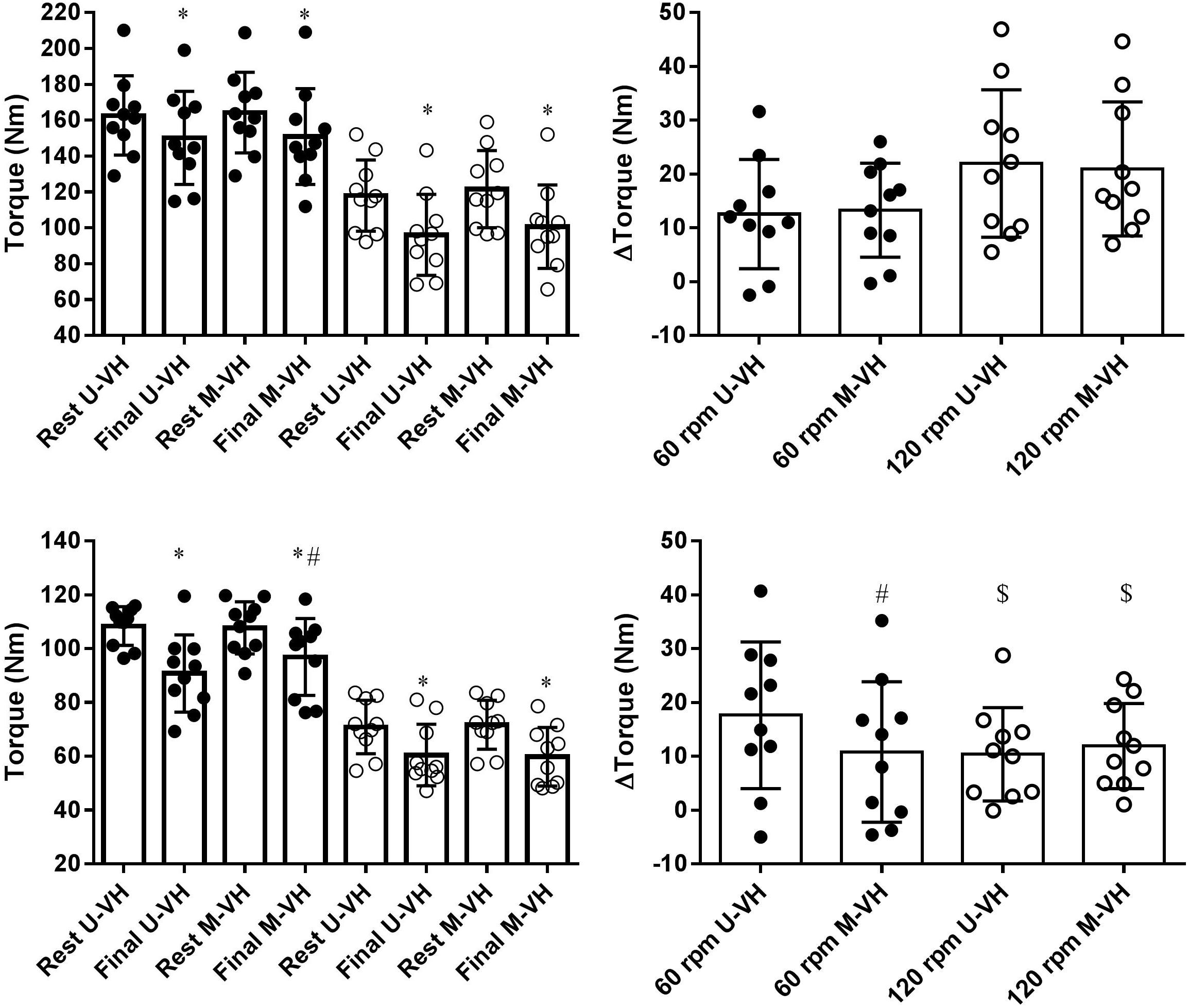
Figure 5. Torque production behavior during maximal isokinetic efforts at 60 (black circles) or 120 (open circles) rpm for males (top panels) or females (bottom panels) subjects. Right panels show Δtorque values. U-VH, unloaded to very heavy intensity exercise; M-VH, moderate to very heavy intensity exercise. $Differences between sex within velocity p < 0.05. ∗Differences within condition p < 0.05. #Differences between conditions p < 0.05.
Discussion
The main finding of this study was that alterations in VO2 kinetics induced by preceding elevated baseline work rate did not reflect alterations in MFP for both healthy males and females. Thus, this work has demonstrated experimentally that isolated decreases in VO2sc and fundamental phase amplitudes or changes in TDs and τ are not linked to MFP during high-intensity cycling exercise in healthy male and female subjects despite the differences between sexes. Considering that there were no differences between conditions (U-VH vs. M-VH) or sexes in RPE and [La], it is suggested that the effects of work-to-work transitions on the VO2 kinetics can be dissociated from differences in blood acidosis and the perception of effort. We hypothesized that elevated baseline work rate would lead to lower amplitudes of the VO2SC and slower values for τ for both sexes despite of the differences between male and female counterparts. Our findings confirm these hypotheses. However, the hypothesis stating that changes in VO2 kinetics would be accompanied by alterations in MFP for both velocities and both sexes was not confirmed.
The Effects of Elevated Baseline Work Rate on VO2 Kinetics
The putative mechanism likely explaining the alterations in VO2 dynamics preceded by elevated baseline VO2 may be represented by the balance between the parasympathetic and sympathetic control of the HR. Elevated work rates seem to alter parasympathetic withdrawal leaving the slower sympathetic control to mediate increases in HR (Hughson and Morrissey, 1982; Bearden and Moffatt, 2001; DiMenna et al., 2010). It has been suggested that a slowing of the HR kinetics may limit the O2 delivery to cellular respiration (Hughson and Morrissey, 1982; DiMenna et al., 2010). Alternatively, cellular respiration might adjust more slowly in muscle fibers that are already active and/or recruitment of motor units that are believed to possess slower VO2 kinetics and a higher VO2 cost of tension (Poole et al., 2008). Likewise, the fundamental PCr τ is lengthened and the fall in PCr is greater compared to U-VH transitions (Jones et al., 2008). These mechanisms are consistent with a greater proportional involvement of muscle fibers that are positioned higher in the recruitment hierarchy (e.g., type II muscle fibers) (Henneman et al., 1965; Jones et al., 2008; Dimenna et al., 2010). Although the present study does not allow to distinguish between the effect of elevated VO2 and elevated work-rate (Please, see the Supplementary Material about this discussion), our results extend the effects of elevated baseline work rate from previous literature for female subjects (Hughson and Morrissey, 1982; Wilkerson and Jones, 2006; Jones et al., 2008; Dimenna et al., 2010; Da Boit et al., 2014; Wüst et al., 2014).
It is important to acknowledge that training status largely influences O2 kinetics, presenting higher phase II τ-values in untrained vs. trained subjects (Koppo et al., 2004). Our phase II τ-values are similar compared with participants from similar training status (recreationally active) at similar intensities (60% Δ) (DiMenna et al., 2010; Cannon et al., 2011; Da Boit et al., 2014; Keir et al., 2016). Despite the differences in the amplitudes of the fundamental and slow phases between sexes in both U-VH and M-VH, τ was not significantly different between sexes despite an elevated baseline. These results are in contrast to previous findings reported for τ in adolescents (Fawkner and Armstrong, 2003; Lai et al., 2016), but in agreement in relation to VO2SC. Besides, we extend these results to a healthy population during cycling compared to the results of Reis et al. (2017) which showed no differences in τ between women and men trained swimmers during heavy-intensity swimming. Moreover, our results are in accordance to findings reported for middle aged subjects during moderate cycling (O’Connor et al., 2012). To date, no study has investigated the effect of work-to-work transition on the VO2 kinetics and MFP comparing sexes. Although males present higher fundamental amplitudes and similar τ-values compared to females, the gross rate of increase of oxygen uptake per second is higher in men, suggesting a quicker onset (Reis et al., 2017). According to Reis et al. (2017) this could be due to the higher VO2peak and anatomic differences (e.g., muscle mass) presented by males. It has been reported that females could present lower O2 delivery, O2 extraction and blood flow due to smaller hearts, smaller lung volumes and lower diffusion capacities and cardiac outputs (Harms, 2006; Dominelli et al., 2015). The present study found significant lower values for VO2peak, power at GET and VE for females compared to their male counterparts. This could be a result of the anatomic differences in the cardiorespiratory system. According to Murias et al. (2013) females were less effective in matching O2 delivery and O2 utilization, which may be due to a lower tonic sympathetic activity in women leading to systemic differences in blood flow distribution. However, a lower oxygen delivery to the muscles seems not to influence the VO2 kinetics in high-intensity exercise (Reis et al., 2017). Olfert et al. (2004) affirmed that female subjects did not experience greater O2 diffusion limitations during exercise, which may emphasize the importance of absolute lung size or aerobic fitness in determining susceptibility to gas exchange impairment rather than sex per se.
VO2 Kinetics vs. Muscle Force Production
Previous literature demonstrated a relation between VO2 kinetics parameters (i.e., VO2SC, τ) and the loss in torque/force production during high-intensity exercise (Cannon et al., 2011; Keir et al., 2016; Temesi et al., 2017). Cannon et al. (2011) proposed that greater levels of muscle fatigue are reflected by a larger amplitude of the VO2SC or vice-versa. The mechanisms contributing to peripheral muscle fatigue has been suggested to contribute to an increased O2 cost of exercise (Keir et al., 2016). Moreover, Temesi et al. (2017) suggested that subjects with slower VO2 kinetics (higher τ-values) experience a greater level of peripheral muscle fatigue, specifically, more excitation-contraction coupling failure. Thus, it was expected that alterations of MFP behavior would be displayed in the VO2 kinetics. In a case of cause-effect relationship, it was expected that higher τ-values would be related with a greater muscle force loss. Or in other hand, lower VO2SC would be related with a lower muscle force decrement. However, we could not confirm this hypothesis. The decrease in torque production in both conditions and for both sexes were not related or linked with the VO2SC or τ. Our results are in accordance with Hopker et al. (2016) who showed that exercise-induced muscle damage led to significant locomotor muscle fatigue, but did not alter the VO2SC or τ during subsequent high-intensity cycling. Hopker et al. (2016) also affirmed that the results from Cannon et al. (2011) could be misleading because the relationship presented by these authors was considering different intensity domains. Deley et al. (2006) demonstrated an inverse relation between the level of muscle fatigue in type II muscle fibers induced by an electromyostimulation protocol and the VO2SC during subsequent high-intensity cycling. Additionally, τ was not altered by this intervention. Moreover, Thistlethwaite et al. (2008) investigated two types of prior exercise (knee extension vs. cycling) which caused different activation patterns/levels of additional motor units (∼38% in knee extension vs. 21% in cycling) and found similar VO2 responses (VO2SC or τ) during the subsequent bout of heavy cycling exercise. The authors concluded that muscle fatigue is neither the primary determinant of the VO2SC nor does it affect the τ of the fundamental phase. Recently, do Nascimento Salvador et al. (2018) showed that prior VH cycling changed the VO2SC and the trajectory of the VO2SC in a subsequent VH cycling exercise, but did not alter MFP behavior. The present study is in line with these findings and challenges a “cause-effect” relationship between VO2SC and muscle fatigue.
The present study observed no differences in Δtorque at 60 and 120 rpm following M-VH and U-VH for males and at 120 rpm (Figure 5) for females despite the differences in the VO2 kinetics. The differences in VO2 fundamental and slow amplitudes as well as in muscle torque production in absolute values could indicate a possible link between muscle force and VO2 kinetics and this hypothesis was not discarded. However, no correlation between Δtorque and VO2SC or τ was found in any condition or velocity in both sexes. These results do not support previous literature proposing a causative relation between muscle fatigue and VO2 kinetics (Cannon et al., 2011; Keir et al., 2016; Temesi et al., 2017). However, female participants showed a smaller decrement in torque production in M-VH at MIE 60 rpm. This may be explained by a smaller percentage of type II fibers and a greater fatigue resistance in females compared to males (Maher et al., 2009; Liu et al., 2010; Hunter, 2014). Further, a reduced proportion of type II muscle fibers may explain the smaller decrements in torque production by females observed at 120 rpm at which the reliance on type II muscle fibers increases. Considering the higher percentage of type II muscle fibers in men (Maher et al., 2009), it may be suggested that proportionally more type II fibers were activated at 120 rpm (Sargeant, 2007) and consequently, led to a greater level of muscle fatigue compared to 60 rpm and compared to women. When exercise is preceded by an elevated baseline work rate, it may be suggested that the recruitment of additional motor units which are characterized by a smaller mitochondrial content and a higher VO2 cost per unit of force (i.e., type II fibers) becomes inevitable in order to maintain the exercise intensity (Wüst et al., 2014). Thus, female subjects may have shown a lower Δtorque in M-VH at 60 rpm because of a lesser reliance on type II fibers.
It is noteworthy that Temesi et al. (2017) did not find a relationship between changes in maximal voluntary activation and τ-values. They stated a lack of relationship between τ-values and central fatigue. According to Hopker et al. (2016), the RPE measured indirectly supports the assumption that a level of central motor command was required in order to produce the power output. This study found that RPE immediately post-exercise was neither different between conditions (U-VH vs. M-VH) nor sexes. Based on these considerations, a dissociation between the changes in VO2 kinetics (i.e., slower τ and the lower VO2SC) and a decrease in central motor drive may be suggested. The performance of a maximal isokinetic cycling effort taken immediately post-exercise is a “global” but ecologically valid protocol to quantify the decrease in MFP in cycling. However, the identification of the origin of fatigue (i.e., central or peripheral) is not possible. Beelen et al. (1995) suggest that fatigue in this type of dynamic isokinetic exercise may be due to changes in the muscle itself and not due to failure of central drive. Considering that muscle fatigue measured by MIE recovers back to baseline values within 1–3 min (Beelen et al., 1995; Coelho et al., 2015; do Nascimento Salvador et al., 2018), it represents a tendency to indicate more the peripheral fatigue.
Conclusion
In summary, this investigation has demonstrated that the fundamental and the VO2SC amplitude were smaller and the time constant and time delay of the slow phase were longer during high-intensity cycling exercise from elevated baseline work rate despite of the differences in both sexes. These alterations were dissociated from the changes in blood lactate concentration and from the perception of exertion. The same RPE for both sexes in both conditions may indicate a similar level of fatigue in central motor drive. Further, MFP decreased following exercise to a similar magnitude for both conditions (U-VH and M-VH) in males and females, with the exception that females demonstrated a smaller decrease following M-VH during isokinetic efforts at 60 rpm compared to U-VH. This pattern in torque production could be related to the activation pattern and distribution of muscle fiber type in men and women. The decrease in muscle force was not associated with VO2 kinetics parameters. Thus, isolated alterations in VO2 kinetics after work-to-work transitions, which may be linked to changes in motor unit recruitment, do not reflect alterations in MFP and fatigue in healthy male and female subjects. These results challenge a “cause-effect” relationship between VO2sc or τ and muscle fatigue.
Ethics Statement
The present work was approved by the Research Ethics Committee of the Federal University of Santa Catarina and was conducted in accordance with the Declaration of Helsinki. After being fully informed of the risks and stresses associated with the study, the participants gave their written informed consent to participate.
Author Contributions
BD and PdNS conceived and designed the work. PdNS, BG, and BD acquired, analyzed, and interpreted the data for the work. PdNS, LS, BG, LG, and BD drafted the work or revised it critically for important intellectual content. All authors have approved the final version of the manuscript and agreed to be accountable for all aspects of the work. All persons designated as authors qualify for authorship, and all those who qualify for authorship are listed.
Funding
This study was supported by grants from Coordenacão de Aperfeiçoamento de Pessoal de Nível Superior (CAPES) and CNPq, Conselho Nacional de Desenvolvimento Científico e Tecnológico – Brasil (154191/2018-3).
Conflict of Interest Statement
The authors declare that the research was conducted in the absence of any commercial or financial relationships that could be construed as a potential conflict of interest.
Acknowledgments
We express our gratitude to all participants involved in this study, as well as, all the laboratory staff (LAEF – UFSC) whom participated in data collection. We also acknowledge Angela Sabrine do Nascimento Salvador for their help in proof reading the manuscript.
Supplementary Material
The Supplementary Material for this article can be found online at: https://www.frontiersin.org/articles/10.3389/fphys.2019.00471/full#supplementary-material
References
Altenburg, T. M., Degens, H., van Mechelen, W., Sargeant, A. J., and de Haan, A. (2007). Recruitment of single muscle fibers during submaximal cycling exercise. J. Appl. Physiol. 103, 1752–1756. doi: 10.1152/japplphysiol.00496.2007
Bassett, D., and Howley, E. (2000). Limiting factors for maximum oxygen uptake and determinants of endurance performance. Med. Sci. Sports Exerc. 32, 70–84.
Bearden, S. E., and Moffatt, R. J. (2001). VO2 and heart rate kinetics in cycling: transitions from an elevated baseline. J. Appl. Physiol. 90, 2081–2087. doi: 10.1152/jappl.2001.90.6.2081
Beaver, W., Wasserman, K., and Whipp, B. (1986). A new method for detecting anaerobic threshold by gas exchange. J. Appl. Physiol. 60, 2020–2027. doi: 10.1152/jappl.1986.60.6.2020
Beelen, A., Sargeant, A. J., Jones, D. A., and de Ruiter, C. J. (1995). Fatigue and recovery of voluntary and electrically elicited dynamic force in humans. J. Physiol. 484(Pt 1), 227–235. doi: 10.1113/jphysiol.1995.sp020660
Black, M. I., Jones, A. M., Bailey, S. J., and Vanhatalo, A. (2015). Self-pacing increases critical power and improves performance during severe-intensity exercise. Appl. Physiol. Nutr. Metab. 9, 1–9. doi: 10.1139/apnm-2014-0442
Burnley, M., Davison, G., and Baker, J. R. (2011). Effects of priming exercise on VO 2 kinetics and the power-duration relationship. Med. Sci. Sports Exerc. 43, 2171–2179. doi: 10.1249/MSS.0b013e31821ff26d
Cannon, D. T., White, A. C., Andriano, M. F., Kolkhorst, F. W., and Rossiter, H. B. (2011). Skeletal muscle fatigue precedes the slow component of oxygen uptake kinetics during exercise in humans. J. Physiol. 589, 727–739. doi: 10.1113/jphysiol.2010.197723
Clark, B. C., Collier, S. R., Manini, T. M., and Ploutz-Snyder, L. L. (2005). Sex differences in muscle fatigability and activation patterns of the human quadriceps femoris. Eur. J. Appl. Physiol. 94, 196–206. doi: 10.1007/s00421-004-1293-0
Coelho, A. C., Cannon, D. T., Cao, R., Porszasz, J., Casaburi, R., Knorst, M. M., et al. (2015). Instantaneous quantification of skeletal muscle activation, power production, and fatigue during cycle ergometry. J. Appl. Physiol. 118, 646–654. doi: 10.1152/japplphysiol.00948.2014
Da Boit, M., Bailey, S. J., Callow, S., Dimenna, F. J., and Jones, A. M. (2014). Effects of interval and continuous training on O2 uptake kinetics during severe-intensity exercise initiated from an elevated metabolic baseline. J. Appl. Physiol. 116, 1068–1077. doi: 10.1152/japplphysiol.01365.2013
Day, J. R., Rossiter, H. B., Coats, E. M., Skasick, A., and Whipp, B. J. (2003). The maximally attainable VO2 during exercise in humans: the peak vs. maximum issue. J. Appl. Physiol. 95, 1901–1907. doi: 10.1152/japplphysiol.00024.2003
de Souza, K. M., Dekerle, J., do Nascimento Salvador, P. C., de Lucas, R. D., Guglielmo, L. G. A., Greco, C. C., et al. (2016). Rate of utilisation of a given fraction of W’ does not affect fatigue during severe-intensity exercise. Exp. Physiol. 58, 7250–7257. doi: 10.1113/EP085451
Deley, G., Millet, G. Y., Borrani, F., Lattier, G., and Brondel, L. (2006). Effects of two types of fatigue on the VO(2) slow component. Int. J. Sports Med. 27, 475–482. doi: 10.1055/s-2005-865837
DiMenna, F. J., Bailey, S. J., Vanhatalo, A., Chidnok, W., and Jones, A. M. (2010). Elevated baseline VO2 per se does not slow O2 uptake kinetics during work-to-work exercise transitions. J. Appl. Physiol. 109, 1148–1154. doi: 10.1152/japplphysiol.00550.2010
Dimenna, F. J., Fulford, J., Bailey, S. J., Vanhatalo, A., Wilkerson, D. P., and Jones, A. M. (2010). Influence of priming exercise on muscle [PCr] and pulmonary O2 uptake dynamics during “work-to-work” knee-extension exercise. Respir. Physiol. Neurobiol. 172, 15–23. doi: 10.1016/j.resp.2010.04.017
do Nascimento, P. C., de Lucas, R. D., Souza, K. M., De Aguiar, R. A., Denadai, B. S., and Guglielmo, L. G. A. (2015). The effect of prior exercise intensity on oxygen uptake kinetics during high - intensity running exercise in trained subjects. Eur. J. Appl. Physiol. 115, 147–156. doi: 10.1007/s00421-014-3000-0
do Nascimento, Salvador, P. C., Souza, K. M., De Lucas, R. D., Guglielmo, L. G. A., and Denadai, B. S. (2018). The effects of priming exercise on the VO2 slow component and the time-course of muscle fatigue during very heavy intensity exercise in humans. Appl. Physiol. Nutr. Metab. 43, 909–919. doi: 10.1139/apnm-2017-0769
Dominelli, P. B., Render, J. N., Molgat-Seon, Y., Foster, G. E., Romer, L. M., and Sheel, A. W. (2015). Oxygen cost of exercise hyperpnoea is greater in women compared with men. J. Physiol. 593, 1965–1979. doi: 10.1113/jphysiol.2014.285965
Enoka, R. M., and Duchateau, J. (2008). Muscle fatigue: what, why and how it influences muscle function. J. Physiol. 5861, 11–23. doi: 10.1113/jphysiol.2007.139477
Fawkner, S. G., and Armstrong, N. (2003). Oxygen uptake kinetic response to exercise in children. Sport. Med. 33, 651–669. doi: 10.2165/00007256-200333090-00002
Harms, C. A. (2006). Does gender affect pulmonary function and exercise capacity? Respir. Physiol. Neurobiol. 151, 124–131. doi: 10.1016/j.resp.2005.10.010
Henneman, E., Somjen, G., and Carpenter, D. O. (1965). Functional significance of cell size in spinal motoneurons. J. Neurophysiol. 28, 560–580. doi: 10.1586/ern.11.57
Hopker, J. G., Caporaso, G., Azzalin, A., Carpenter, R., and Marcora, S. M. (2016). Locomotor muscle fatigue does not alter oxygen uptake kinetics during high-intensity exercise. Front. Physiol. 7:463. doi: 10.3389/fphys.2016.00463
Hughson, R. L., and Morrissey, M. (1982). Delayed kinetics of respiratory gas exchange in the transition from prior exercise. J. Appl. Physiol. 52, 921–929. doi: 10.1152/jappl.1982.52.4.921
Hunter, S. K. (2014). Sex differences in human fatigability: mechanisms and insight to physiological responses. Acta Physiol. 210, 768–789. doi: 10.1111/apha.12234
Jones, A., Grassi, B., Christensen, P., Krustrup, P., Bangsbo, J., and Poole, D. (2011). Slow component of VO2 kinetics: mechanistic bases and practical applications. Med. Sci. Sport Exerc. 43, 2046–2062. doi: 10.1249/MSS.0b013e31821fcfc1
Jones, A. M., Wilkerson, D. P., and Fulford, J. (2008). Muscle [phosphocreatine]dynamics following the onset of exercise in humans: the influence of baseline work-rate. J. Physiol. 586, 889–898. doi: 10.1113/jphysiol.2007.142026
Keir, D. A., Copithorne, D. B., Hodgson, M. D., Pogliaghi, S., Rice, C. L., and Kowalchuk, J. M. (2016). The slow component of pulmonary O2 uptake accompanies peripheral muscle fatigue during high intensity exercise. J. Appl. Physiol. 7, ja00249.2016. doi: 10.1152/japplphysiol.00249.2016
Koppo, K., Bouckaert, J., and Jones, A. M. (2004). Effects of training status and exercise intensity on phase II VO2 kinetics. Med. Sci. Sports Exerc. 36, 225–232. doi: 10.1249/01.MSS.0000113473.48220.20
Lai, N., Martis, A., Belfiori, A., Tolentino-silva, F., Nasca, M. M., Strainic, J., et al. (2016). Gender differences in VO 2 and HR kinetics at the onset of moderate and heavy exercise intensity in adolescents. Physiol. Rep. 4, 1–12. doi: 10.14814/phy2.12970
Lamarra, N., Whipp, B. J., Ward, S. A., and Wasserman, K. (1987). Effect of interbreath fluctuations on characterizing exercise gas exchange kinetics. J. Appl. Physiol. 62, 2003–2012. doi: 10.1152/jappl.1987.62.5.2003
Liu, D., Sartor, M. A., Nader, G. A., Gutmann, L., Treutelaar, M. K., Pistilli, E. E., et al. (2010). Skeletal muscle gene expression in response to resistance exercise: sex specific regulation. BMC Genomics 11:659. doi: 10.1186/1471-2164-11-659
Maher, A. C., Fu, M. H., Isfort, R. J., Varbanov, A. R., Qu, X. A., and Tarnopolsky, M. A. (2009). Sex differences in global mRNA content of human skeletal muscle. PLoS One 4:e0006335. doi: 10.1371/journal.pone.0006335
Mitchell, J. H., Tate, C., Raven, P., Cobb, F., Kraus, W., Moreadith, R., et al. (1992). Acute response and chronic adaptation to exercise in women. Med. Sci. Sports Exerc. 24, S258–S265.
Murgatroyd, S. R., Ferguson, C., Ward, S. A., Whipp, B. J., and Rossiter, H. B. (2011). Pulmonary O2 uptake kinetics as a determinant of high-intensity exercise tolerance in humans. J. Appl. Physiol. 110, 1598–1606. doi: 10.1152/japplphysiol.01092.2010
Murias, J. M., Keir, D. A., Spencer, M. D., and Paterson, D. H. (2013). Sex-related differences in muscle deoxygenation during ramp incremental exercise. Respir. Physiol. Neurobiol. 189, 530–536. doi: 10.1016/j.resp.2013.08.011
O’Connor, E., Kiely, C., O’Shea, D., Green, S., and Egana, M. (2012). Similar level of impairment in exercise performance and oxygen uptake kinetics in middle-aged men and women with type 2 diabetes. AJP Regul. Integr. Comp. Physiol. 303, R70–R76. doi: 10.1152/ajpregu.00012.2012
Olfert, I. M., Balouch, J., Kleinsasser, A., Knapp, A., Wagner, H., Wagner, P. D., et al. (2004). Does gender affect human pulmonary gas exchange during exercise? J. Physiol. 557, 529–541. doi: 10.1113/jphysiol.2003.056887
Poole, D. C., Barstow, T. J., McDonough, P., and Jones, A. M. (2008). Control of oxygen uptake during exercise. Med. Sci. Sports Exerc. 40, 462–474. doi: 10.1249/MSS.0b013e31815ef29b
Poole, D. C., Schaffartzik, W., Knight, D. R., Derion, T., Kennedy, B., Guy, H. J., et al. (1991). Contribution of exercising legs to the slow component of oxygen uptake kinetics in humans. J. Appl. Physiol. 71, 1245–1260. doi: 10.1152/jappl.1991.71.4.1245
Poole, D. C., Ward, S. A., Gardner, G. W., and Whipp, B. J. (1988). Metabolic and respiratory profile of the upper limit for prolonged exercise in man. Ergonomics 31, 1265–1279. doi: 10.1080/00140138808966766
Reis, J. F., Millet, G. P., Bruno, P. M., Vleck, V., and Alves, F. B. (2017). Sex and exercise intensity do not influence oxygen uptake kinetics in submaximal swimming. Front. Physiol. 8:72. doi: 10.3389/fphys.2017.00072
Rossiter, H. B., Ward, S. A., Kowalchuk, J. M., Howe, F. A., Griffiths, J. R., and Whipp, B. J. (2001). Effects of prior exercise on oxygen uptake and phosphocreatine kinetics during high-intensity knee-extension exercise in humans. J. Physiol. 537, 291–303. doi: 10.1111/j.1469-7793.2001.0291k.x
Rossiter, H. B., Ward, S. A., Kowalchuk, J. M., Howe, F. A., Griffiths, J. R., and Whipp, B. J. (2002). Dynamic asymmetry of phosphocreatine concentration and O(2) uptake between the on- and off-transients of moderate- and high-intensity exercise in humans. J. Physiol. 541, 991–1002. doi: 10.1113/jphysiol.2001.012910
Sargeant, A. J. (2007). Structural and functional determinants of human muscle power. Exp. Physiol. 92, 323–331. doi: 10.1113/expphysiol.2006.034322
Temesi, J., Mattioni Maturana, F., Peyrard, A., Piucco, T., Murias, J. M., and Millet, G. Y. (2017). The relationship between oxygen uptake kinetics and neuromuscular fatigue in high-intensity cycling exercise. Eur. J. Appl. Physiol. 117, 969–978. doi: 10.1007/s00421-017-3585-1
Thistlethwaite, J. R., Thompson, B. C., Gonzales, J. U., and Scheuermann, B. W. (2008). Prior heavy knee extension exercise does not affect VO2 kinetics during subsequent heavy cycling exercise. Eur. J. Appl. Physiol. 102, 481–491. doi: 10.1007/s00421-007-0614-5
Wilkerson, D. P., and Jones, A. M. (2006). Influence of initial metabolic rate on pulmonary O2 uptake on-kinetics during severe intensity exercise. Respir. Physiol. Neurobiol. 152, 204–219. doi: 10.1016/j.resp.2005.10.001
Keywords: motor unit recruitment, muscle fatigue, O2 delivery, oxidative phosphorylation, elevated baseline, VO2 kinetics
Citation: do Nascimento Salvador PC, Schäfer L, Grassi B, Guglielmo LGA and Denadai BS (2019) Changes in VO2 Kinetics After Elevated Baseline Do Not Necessarily Reflect Alterations in Muscle Force Production in Both Sexes. Front. Physiol. 10:471. doi: 10.3389/fphys.2019.00471
Received: 29 October 2018; Accepted: 04 April 2019;
Published: 25 April 2019.
Edited by:
Kevin I. Watt, The University of Melbourne, AustraliaReviewed by:
Stephan Van Der Zwaard, Leiden Institute of Advanced Computer Science, NetherlandsAntonio Longo, Università degli Studi di Catania, Italy
Copyright © 2019 do Nascimento Salvador, Schäfer, Grassi, Guglielmo and Denadai. This is an open-access article distributed under the terms of the Creative Commons Attribution License (CC BY). The use, distribution or reproduction in other forums is permitted, provided the original author(s) and the copyright owner(s) are credited and that the original publication in this journal is cited, in accordance with accepted academic practice. No use, distribution or reproduction is permitted which does not comply with these terms.
*Correspondence: Paulo Cesar do Nascimento Salvador, bmFzY2ltZW50b3NhbHZhZG9ycGNAZ21haWwuY29t orcid.org/0000-0001-8228-5115 Benedito Sérgio Denadai, YmRlbmFkYWlAcmMudW5lc3AuYnI=