- 1Laboratory of Neurogenetics, Pavlov Institute of Physiology Russian Academy of Sciences, Saint Petersburg, Russia
- 2Laboratory of Regulation of Brain Neuron Functions, Pavlov Institute of Physiology Russian Academy of Sciences, Saint Petersburg, Russia
- 3Department of Biochemistry, Faculty of Biology, Saint Petersburg State University, Saint Petersburg, Russia
- 4Department of Anatomy and Physiology of Humans and Animals, Faculty of Biology, Herzen State Pedagogical University of Russia, Saint Petersburg, Russia
Dysfunctions of kynurenine pathway of tryptophan metabolism (KPTM) are associated with multiple neuropathologies in vertebrates and invertebrates. Drosophila mutants with altered content of kynurenines are model objects for studying the molecular processes of neurodegeneration and senile dementia. The mutant cardinal (cd1) with accumulation of the redox stress inductor 3-hydroxykynurenine (3-HOK) shows age-dependent impairments of the courtship song and middle-term memory. The molecular mechanisms for 3-HOK accumulation in cd1 are still unknown. Here, we have studied age-dependent differences in spontaneous locomotor activity (SLA) for the wild type strain Canton-S (CS), cd1, and cinnabar (cn1) with an excess of neuroprotective kynurenic acid (KYNA). We have also estimated the level and distribution of protein-bound 3-HOK (PB-3-HOK) in Drosophila brains (Br) and head tissues. The middle-age cd1 show the higher running speed and lower run frequency compared to CS, for cn1 the situation is the opposite. There is a decrease in the index of activity for 40-day-old cd1 that seems to be an effect of the oxidative stress development. Surprisingly, PB-3-HOK level in Drosophila heads, brains, and head capsules (HC) is several times lower for cd1 compared to CS. This complements the traditional hypothesis that cd1 phenotype results from a mutation in phenoxazinone synthase (PHS) gene governing the brown eye pigment xanthommatin synthesis. In addition to 3-HOK dimerization, cd1 mutation affects protein modification by 3-HOK. The accumulation of free 3-HOK in cd1 may result from the impairment of 3-HOK conjugation with some proteins of the brain and head tissues.
Introduction
Kynurenine pathway of tryptophan metabolism (КPTM) is the major path of tryptophan catabolism in mammals (Badawy, 2017). Leading to nicotinamide adenine nucleotide (NAD+) production in cell, KPTM also plays an important role as the source of neuroactive compounds, collectively called kynurenines. The imbalance of kynurenines in nervous system has been observed during neurodegenerative disorders, such as Alzheimer’s, Parkinson’s, and Huntington’s diseases (Schwarcz et al., 2012). The multiple neurotropic effects of kynurenines were shown for both vertebrates and invertebrates (Lapin, 2004). A KPTM metabolite quinolinic acid (QUIN) is an agonist of ionotropic glutamate receptors (iGluR; El-Defrawy et al., 1986). Kynurenic acid (KYNA) is a non-specific iGluR (Kessler et al., 1989) and α7 nicotinic acetylcholine receptor (Hilmas et al., 2001) antagonist. It can interact with both mammalian and Drosophila iGluR receptors (Zhuravlev et al., 2012), ameliorating excitotoxicity. 3-hydroxykynurenine (3-НОК) and 3-hydroxyanthranilic acid (3-HAA) inhibit lipid peroxidation (Christen et al., 1990; Zhuravlev et al., 2016). At the same time, 3-HOK oxidative dimerization may cause overproduction of reactive oxygen species (ROS) and cell death (Okuda et al., 1996, 1998).
Neurotropic effects of kynurenines can be studied using simple model objects, such as Drosophila melanogaster mutants (Figure 1). In insects, KPTM is the source of the brown eye pigments ommochromes, including xanthommatin that is synthesized upon 3-HOK dimerization. 3-HOK also produces xanthurenic and cardinalic acids. In vermilion (v), KPTM is blocked with six-fold rise of tryptophan level. In cinnabar (cn), the inactivation of kynurenine monooxygenase (KMO) gene leads to two-fold increase in KYNA level. In cardinal (cd), there is a 2.9-fold rise of 3-HOK level, due to some defects in phenoxazinone synthase (PHS) gene. Xanthommatin can also be produced non-enzymatically due to the oxidative 3-HOK autodimerization. QUIN and nicotinic acid are not synthesized from 3-HAA in Drosophila and 3-HAA level is low (Linzen, 1974; Savvateeva et al., 2000; and references therein). Hence, cd can be used to study the specific neurotoxic effects of 3-HOK. Studying the behavioral characteristics of Drosophila KPTM mutants is of particular interest, as cn and cd alleles are marker genes in many transgenic fly strains, including those used in UAS-GAL4 system.
In many cases, 3-HOK and KYNA manifest themselves as antagonists. The increase in 3-HOK/KYNA level for Drosophila mutant htt, a model for Huntington’s disease, leads to neurodegeneration (Green et al., 2012). Inhibition of KMO increases 3-HOK/KYNA level, showing an advancement in neurodegeneration (Campesan et al., 2011). In aged cd, the middle-term memory is impaired and the calyx neuropathology is observed, while in aged cn the synaptic pathology is less (Savvateeva et al., 2000). On day 29, cd males demonstrate courtship song defects, such as high variance of interpulse interval (VIPI), while KYNA accumulation in cn seems to have neuroprotective effects, as their VIPI is lower compared to cd and the wild type Canton-S (CS; Savvateeva-Popova et al., 2003). For 5-day-old cd1, memory is impaired by heat shock and is restored by the application of a phenolic antioxidant (Nikitina et al., 2018). Heat shock induces apoptosis in cd1 brain (Br) on day 5 (Savvateeva-Popova et al., 2003), which may be due to acceleration of 3-HOK autoxidation leading to hydrogen peroxide production. The dual role of 3-HOK in oxidative stress development is manifested as total antioxidant capacity decrease in head tissues of 5–29-day-old cd1 compared to CS along with the tendency of lipid peroxidation level decrease in cd1 (Zhuravlev et al., 2018).
An important characteristic of Drosophila is spontaneous locomotor activity (SLA). Spontaneous activity can be defined as “the observable activity exhibited by an animal when not specifically activated by external stimuli” (Ewing, 1963). Previously, age-dependent SLA for Drosophila KPTM mutants was estimated as the percentage of moving females in a group recorded at time intervals (Kamyshev, 1980). However, this method did not consider specific SLA parameters, such as percentage of movement time (index of activity), running speed, total speed, etc. The evaluation of SLA parameters for Drosophila KPTM mutants was performed for the third instar larvae (Zakharov et al., 2012). Most of them decrease in cn1 with KYNA excess, while v1 and cd1 have slightly higher running speed compared to CS. In this study, we have evaluated the age-dependent changes in SLA parameters for the adult male CS, cd1, and cn1. We have also performed the measurement of 3-HOK protein-bound form (PB-3-HOK) in flies’ heads, as well as its distribution within CS and cd1 brains.
Materials and Methods
Materials
Drosophila Strains
The research was performed on D. melanogaster strains from Biocollection of Pavlov Institute of Physiology Russian Academy of Sciences, Saint Petersburg, Russia. The following strains were used:
1. Canton-S (CS), the wild type strain.
2. vermilion (v1) – a mutation in tryptophan 2,3-dioxygenase (TDO) gene; no kynurenines.
3. cinnabar (cn1) – a mutation in KMO gene; 3-HOK lack, KYNA excess.
4. cardinal (сd1; Bloomington Drosophila Stock Center #3052) – a mutation in PHS gene; 3-HOK excess.
All mutant strains were out-crossed to CS for nine generations. The strains were raised on standard yeast–raisin medium at 12:12 (8 a.m.–8 p.m.) daily illumination cycle at 25 ± 0.5°C; humidity was not controlled. Males of the studied strains were collected without narcotization within 1 day after eclosion and kept on the standard medium in small fruit fly vials at 22 ± 0.5°C until the experiment.
Methods
Spontaneous Locomotor Activity
Males were kept in small groups (4–5 males in a vial) before experiment. The same flies were tested at the different ages, with the exception of some dead or flying away. For each KPTM strain and the fly age, the experiment was carried out within a single day (mostly within 11 a.m.–3 p.m.; 18–24 flies per day) at 22 ± 1°C. SLA significantly varied from day to day, so CS flies of the same age served a control in each experiment. The procedure of analysis is described in (Zakharov et al., 2012), being adapted for imagoes. Flies were placed separately in flat round neon-lit chambers (diameter 20 mm, height 3 mm). Fly position on the chamber plane was represented by the weighted X and Y coordinates of all pixels brighter than the threshold level 60. Fly movement was then recorded for 1 h through the transparent сover using Logitech QuickCam web camera. Tracks were analyzed using Locotrack software (Zakharov, 2017). The full record was divided into 1 s quanta, and the mean speed of fly movement in each quantum was calculated. If the result was lower than 1 mm/s, the fly was considered to be in the rest (Colomb et al., 2012), otherwise it was considered to run. All the other settings were default for imagoes. The following SLA parameters were estimated: (1) index of activity (C.U.), (2) run frequency (N/100 s), (3) running speed (mm/s), (4) total speed (mm/s), and (5) run bout time (s). To reveal the differences between CS and cd1, large samplings were tested. For some tracks, the total fly trajectories were graphically visualized.
PB-3-НОК Level in Drosophila Homogenates
Flies heads or head parts were homogenized on ice in TE buffer (0.2 M tris-HCl, 1 mM EDTA, pH 7.4: 40–50 heads in 600 μl; six brains or head capsules (HC) in 30 μl). The homogenates were centrifuged at 4°C at 1,000 g for 10 min. Two microliters of supernatant was immobilized on a nitrocellulose membrane (Protran; Whatman). 3-HOK level was measured using dot blot technique according to the Abcam protocol (Abcam, Cambridge, UK). The membrane was incubated with anti-3-HOK monoclonal antibody (1:2000; Thermo Scientific, #MA1-16616), then with secondary HRP-conjugated antibody (1:1000, Thermo Scientific, #31431), and stained using Vector NovaRed Peroxidase Substrate Kit (Vector Laboratories). The signal level was estimated using Fiji software (Schindelin et al., 2012). Signal was normalized to β-actin (primary antibody: 1:2000; Sigma-Aldrich, A2066; secondary antibody: 1:1000; Abcam, ab97064) or to the total protein level measured by amido black staining (Sigma-Aldrich, 1.01167). In a control experiment, the homogenates were purified from proteins using Microcon Ultracel YM-3 columns (Millipore), and no signal was observed. Thus, the detected 3-HOK form is a protein-bound form (PB-3-HOK).
According to antibody manufacturer’s, BSA-3-HOK conjugates were used as standards. To perform conjugation, LC-SPDP crosslinker (Thermo Scientific, #21651) and L,D-3-HOK (Sigma-Aldrich, H1771) were mixed in PBS-EDTA buffer (pH 7.5), incubated for 60 min at room temperature and mixed with BSA (final concentrations: in 1 ml: 0.5 mM LC-SPDP, 0.95 mM (0.2 μg/μl) 3-HOK, 0.2% BSA). The mixture was incubated overnight at 4°C and centrifuged in spin columns to remove free 3-HOK. Conjugates were diluted in 100 μl PBS-EDTA (~2 μg/μl 3-HOK in conjugate). To make standard curve, serial dilution of standard was prepared, along with dilution of CS head capsule homogenate. To test antibody specificity, PB-3-HOK antibody was preincubated overnight with BSA-3-HOK (1:3 v/v ratio), and optical density (OD) difference with and without preincubation was measured.
To check the PB-3-HOK mass range, homogenates were mixed with Laemmly buffer (without 2-mercaptoethanol), separated by sodium dodecyl sulfate-polyacrylamide gel electrophoresis (SDS-PAGE), transferred to the PVDF membrane (Thermo Fisher Scientific, USA), and stained as it mentioned above.
PB-3-НОК Distribution in Drosophila Brains
The experiments were performed according to (Wu and Luo, 2006) with some modifications. After fixation in 4% paraformaldehyde solution in PBS buffer for 30 min, brains were incubated with primary antibody to 3-HOK (1:100 in PBT buffer) for 5 days and then with AlexaFluor 568 secondary antibody (Invitrogen, #11037; 1:100) for 1 day with shaking at 4°C. FITC-conjugated anti-horseradish peroxidase antibody (1:100; Jackson ImmunoResearch, 123-095-021) was used as a neuronal marker. Nuclei were stained with DAPI (1.2 μg/ml in PBS). The brains were scanned frontally by the confocal laser scanning microscope (LSM 710 Carl Zeiss; Confocal microscopy Resource Center; Pavlov Institute of Physiology Russian Academy of Sciences, Saint Petersburg, Russia) using X20 objective and analyzed with Fiji. For each brain, the average intensity of PB-3-HOK staining was measured in one hemisphere within the darkened area of pedunculus (Ped; x) and the area of the same size above it (y). x and y values were averaged for three optical slices with maximum Ped dimming. R = x/y – the relative decrease in PB-3-HOK for Ped area.
Statistical Analysis
Statistical analysis was performed with the help of Social Science Statistics resource1 using two-tailed Mann-Whitney U test (for SLA parameters) or two-tailed t-test (for PB-3-HOK level estimation).
Results
Spontaneous Locomotor Activity
The comparative analysis of SLA parameters has been performed for CS, cd1, and cn1 (Figure 2; Supplementary Figure S1). There are no interstrain differences on day 5. Index of activity is similar for all strains, except its decrease in cd1 compared to CS on day 40 (A). For cd1, run frequency is higher than for CS on days 21–40 (B). Total speed is the same for the both strains except its decrease in cd1 on day 40 (C), while running speed is higher in cd1 on days 13–29 (D) and run bout time is higher on day 29 (E). For cn1, the picture is actually the opposite: There is a pronounced increase in run frequency compared to CS during the studied period, along with decrease in total speed, running speed, and run bout time after day 5.
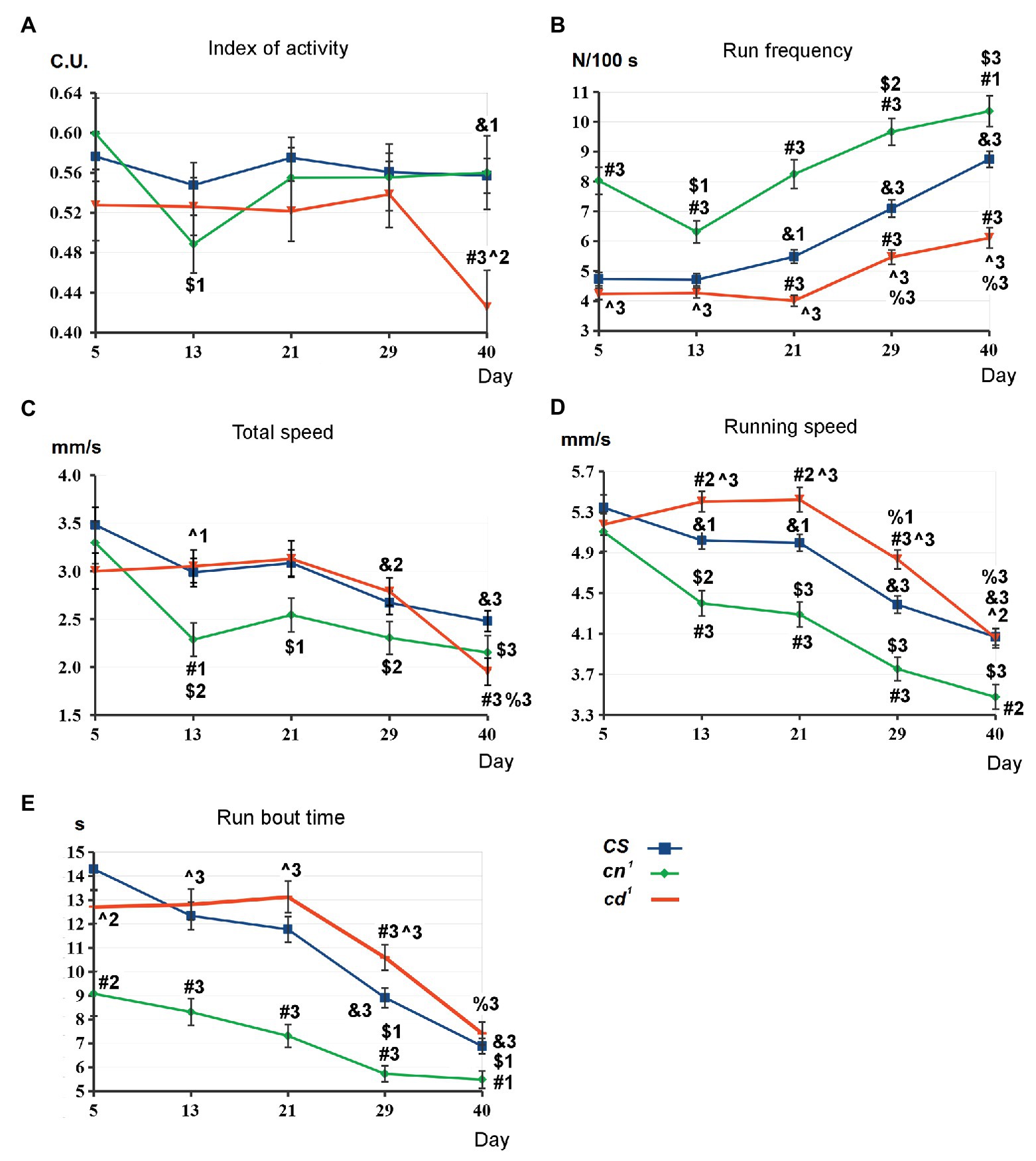
Figure 2. Spontaneous locomotor activity (SLA) parameters for kynurenine mutants. Statistical differences: # from Canton-S (CS), ^ from cinnabar (cn1), & from 5-day-old CS, $ from 5 day-old cn1, and % from 5 day-old cardinal (cd1). Method of analysis: two-tailed Mann-Whitney U-test, p < 0.05 (1), p < 0.01 (2), p < 0.001 (3); for CS: n = 163 (day 5)–143 (day 40), the average data of seven independent experiments; for cn1: n = 47 (day 5)–32 (day 40), the average data of two independent experiments; for cd1: n = 118 (day 5)–108 (day 40), the average data of five independent experiments. Here and below: mean values with standard error are shown (A-E): see in the text.
CS does not show the age-dependent changes in index of activity up to day 40. For all strains, run frequency rises with aging after day 13 that means the increase in the number of stops. Running speed and run bout time decrease for all strains on days 29–40 compared to day 5. For cn1, there is a slight decrease in several SLA parameters on day 13. However, we also observed a similar decrease in the control CS group, so it is possibly explained by the local change of flies’ activity due to some external factors. The pronounced decrease in index of activity for 40-day-old cd1 compared to CS and 5-day-old cd1 seems to be the effect of the neurotoxic processes.
cn1 index of activity does not differ from CS on day 40. Hence, it is not affected by the lack of the eye pigments ommochromes in cn1. The form of tracks shows centrophobia for all strains (Supplementary Figure S2A), as flies prefer to move along the walls of the chamber and avoid the central space, in agreement with previous data (Colomb et al., 2012). Most of the tracks form a complete circle demarcating the chamber edge, as flies mostly move along the side wall of the chamber. To test the influence of the pigment synthesis defect on flies’ locomotion, we analyzed their SLA under the red light on day 1 (Supplementary Figure S2B). Centrophobia is more expressed here, and some tracks are incomplete. Though both 1-day-old cn1 and cd1 lack xanthommatin (bright red eyes), a significant difference in their running speed was observed, indicating it to be the most specific parameter that depends solely on 3-HOK/KYNA level for each strain but not on their visual abilities.
Protein-Bound 3-HOK Level and Distribution
Non-specific lens proteins modification by 3-HOK seems to be involved in cataract formation (Korlimbinis and Truscott, 2006). To check possible changes of 3-HOK-protein conjugation in Drosophila, PB-3-HOK levels were measured in fly heads, as well as in brains and the head capsules separately. For heads of 5-day-old Drosophila KPTM mutants, PB-3-HOK decreases in the following order: CS > cd1 > v1 > cn1 (Figure 3). The decrease in PB-3HOK for cd1 compared to CS throughout the studied period of life is the most unexpected fact, as the level of free 3-HOK is known to be higher in cd1. There are no significant age-dependent changes in CS PB-3-HOK level; for cd1, it slightly decreases on days 13–21. This effect occurs simultaneously with the increase of cd1 running speed (see Figure 2). PB-3-HOK level decreases both in brains and in the head capsules of cd1. Its level in the head capsules is higher compared to brains, except for 5-day-old CS. For the studied range of concentrations, there is a linear dependence of PB-3HOK level in homogenate and OD of immunohistochemical staining (Supplementary Figure S3). In the head capsules, 3-HOK is mainly associated with 40–55 kDa proteins, for which nature and biological functions remain unknown. In brains, PB-3-HOK level is too low to be able to detect clear bands.
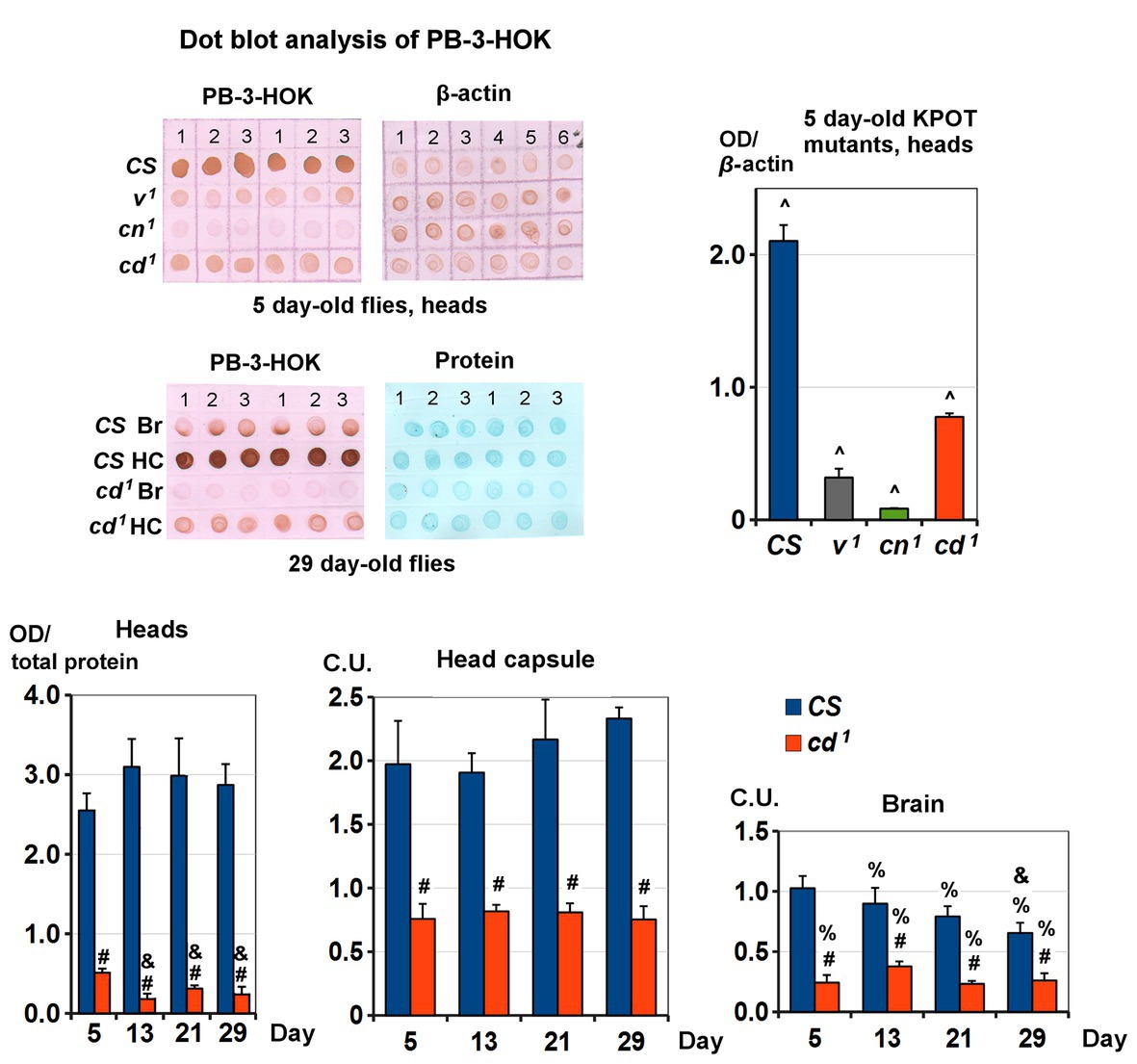
Figure 3. Protein-bound 3-HOK (PB-3-HOK) level in kynurenine mutants heads. Five-day-old CS and KPOT mutants: expressed as PB-3-HOK optical density (OD) normalized to beta-actin OD. All interstrain differences (^) are statistically significant (two-tailed t-test; p < 0.05, n = 3). CS and cd1 heads: expressed as OD normalized to total protein OD. Statistical differences: # from CS (p < 0.01), & from 5-day-old flies of the same strain (p < 0.05; two-tailed t-test; n = 5). CS and cd1 brains (Br) and head capsules (HC): expressed as OD normalized to total protein OD and to the mean of the all values for a given age (C.U.). Statistical differences: # from CS, & from 5-day-old flies of the same strain, and % from head capsules (two-tailed t-test, p < 0.05; n = 3).
PB-3-HOK distribution in Drosophila brains is nearly uniform. However, in 5-day-old CS, we can see the decline in PB-3-HOK level within a small round area just below the calyx of the mushroom body, where Ped begins (Figure 4). R value shows the normalized PB-3-HOK level within this area. On day 5, R is higher for cd1 and the area of decline is not observed so clearly as in CS. On day 29, R significantly increases for CS compared to day 5, and for cd1 the darkened area is virtually absent (R → 1). In some brains, the darkened area is closer to the antennal lobe; it also can vary in size and shape. Possibly, its lower contrast for cd1 is due to the lower total brain PB-3-HOK level for this strain.
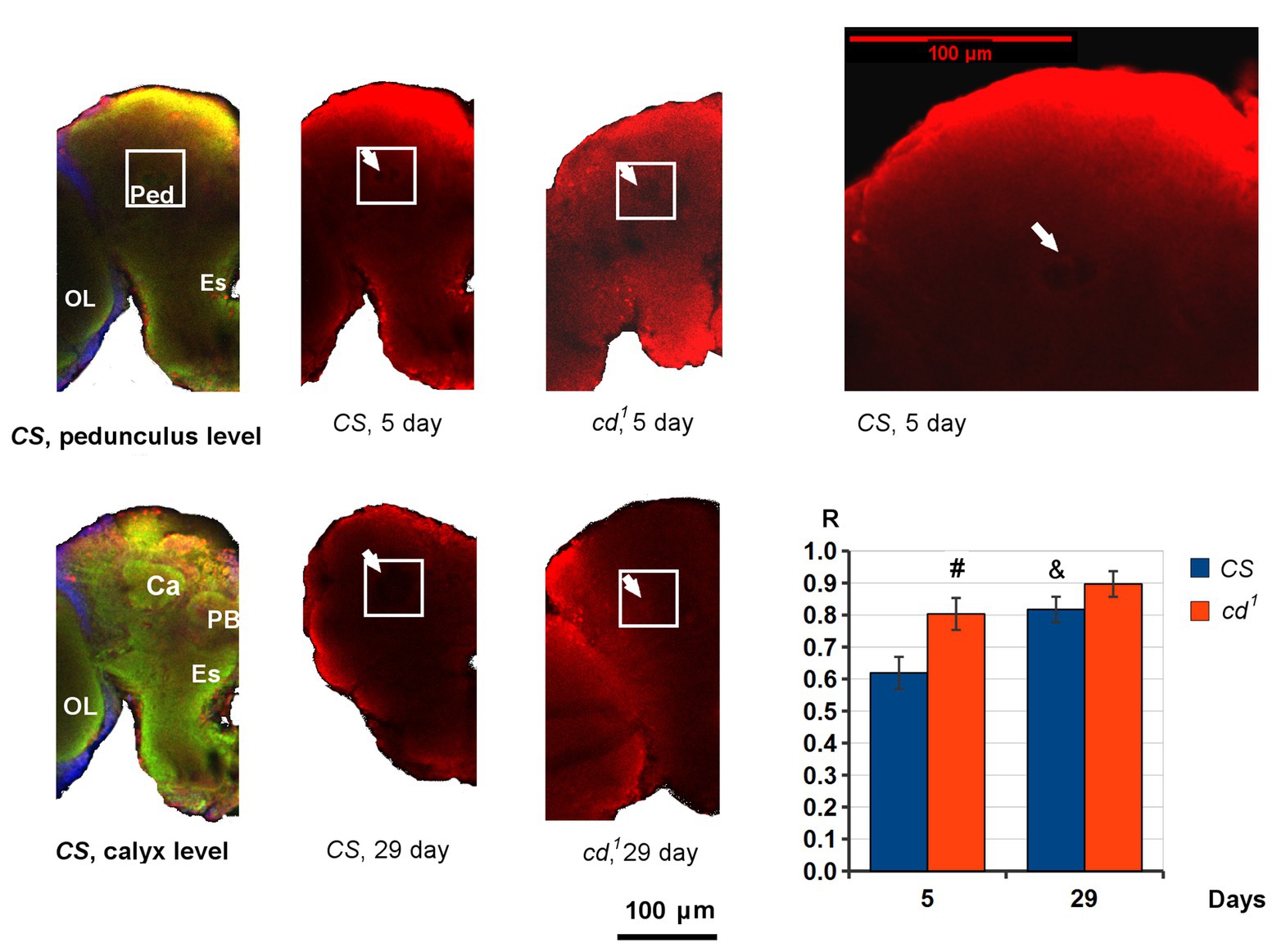
Figure 4. PB-3-HOK distribution in CS and cd1 brain. The part of the left hemisphere is shown frontally. The area of interest (white square) is shown on pedunculus (Ped) level. The area of PB-3-HOK decrease in Ped is indicated with a white arrow. Statistical differences: # cd1 from CS (p < 0.05), and & CS day 29 from CS day 5 (p < 0.01); two-sided t-test; n = 8 (CS day 5), 9 (CS day 29), 11 (cd1 day 5), 5 (cd1 day 29). Color scheme: green – neuropile, red – PB-3-HOK, blue – nuclei. Ca, calyx; Es, esophagus; OL, optic lobe; PB, protocerebral bridge; Ped, pedunculus.
Discussion
Assessment of specific SLA parameters for Drosophila mutants unravels genes controlling the motor functions (Fedotov et al., 2014) and helps to understand the molecular mechanisms of kynurenines’ neurotropic activity (Zakharov et al., 2012). As we have shown here for 13–29-day-old cd1, running speed is higher and run frequency is lower compared to CS. Stimulating effect of 3-HOK excess on the running speed may be caused by the rise in ROS level, as total antioxidant capacity decreases in cd1 heads (Zhuravlev et al., 2018). Decreased level of mitochondrial hydrogen peroxide in transgenic Drosophila leads to decrease in walking speed, as well as lifespan, indicating the importance of free radicals production at moderate level for the life processes (Bayne et al., 2005). Total SLA decrease in 40-day-old cd1 may reflect the deferred neurotoxic action of 3-HOK on the fly nervous system, leading to calyx synaptic pathology and memory defects in aged cd1 (Savvateeva et al., 2000). On the contrary, cn1 running speed decreases and run frequency rises relative to CS, which may be the inhibitory effects of KYNA on glutamate or acetylcholine neurotransmission.
For 5-day-old flies, running speed is about 5 mm/s, being close to previous data obtained by the same method (Fedotov et al., 2014), but much less than the median speed 15 mm/s in Buridan’s paradigm (Colomb et al., 2012) and similar values published in (Bayne et al., 2005). The differences may be explained by much less space of movement in our experiments, as flies regulate their walking speed, slowing when some objects are nearby (Creamer et al., 2018).
cd1 third instar larvae also show the increase in running speed, while cn1 demonstrated the total decrease in SLA parameters (Zakharov et al., 2012). As the larvae lack compound eyes, the observed effects are not due to defects in xanthommatin synthesis. Male courtship indices are equal for CS, cn1, and cd1, remaining high during the whole life, indicating that their response to the courtship-regulating pheromones and visual cues from a female remains unchanged. Three-hour memory in conditioned courtship suppression paradigm (CCSP) is intact in old CS and cn1, for cd1 it decreases since day 13 (Savvateeva et al., 2000). All these mutants differ in most SLA parameters on days 13–29, so we do not observe any correlation between their SLA and cognitive abilities in CCSP. Though the observed changes in running speed seem not to depend on the visual system, SLA investigation for kynurenine mutants in the darkness would clarify its effect on flies’ orientation abilities.
Previously, the percentage of 8–20-day-old cd running females was shown to be less than for CS. Running speed was not estimated there. cn was less active than CS after day 3, but there was no clear difference between cn and cd relative to CS observed by us. Both cn and cd had low life expectancy (~42 and 43 days vs. 48 days for CS), while v with KPTM block had the maximum lifespan (~64 days; Kamyshev, 1980).
The rise of 3HOK/KYNA index, which can be achieved by inhibiting TDO or KMO, correlates with neurodegeneration in htt mutant (Campesan et al., 2011; Green et al., 2012). For the scarlet mutant with a defect in 3-HOK transport to pigment cells, age-dependent progressive loss of dopaminergic neurons belonging to Protocerebral Posterial Lateral 1 (PPL1) cluster was shown, along with increase in ROS level, shortened lifespan, and decreased climbing index. cn1 also showed lower climbing index and shortened lifespan, but no loss of PPL1 neurons, which seem to be specifically susceptible to 3-HOK-induced neurodegeneration (Cunningham et al., 2018). As we investigated SLA on the horizontal plane, the negative geotaxis did not affect the flies’ activity. Study of PPL1 functioning for the old cd1, normal and with antioxidants preventing ROS formation, is a task for future.
Run frequency and running speed are independently controlled by Drosophila nervous system (Colomb et al., 2012). Flies’ walking is accompanied by global increase in activity of dopamine neurons within the brain (Aimon et al., 2019), that is in agreement with role of PPL1 neurons in movement control (Cunningham et al., 2018). Neural network responsible for generation of insect motor pulses is localized in thoracic segments (Bassler, 1983). SLA decreases after disruption of a network interconnecting the parts of the Drosophila central complex (CX; Martin et al., 1999). The decrease in running speed correlates with defects of the protocerebral bridge and some other parts of CX (Strauss and Heisenberg, 1993). At the same time, CX performs the complex sensory integration and pre-motor processing, regulating fly posture, turning, navigation, selection of motor actions, and inducing sleep. The mushroom bodies also control locomotion activity, mostly suppressing it (Emanuel et al., 2020).
Lack of PB-3-HOK in v1 heads is obviously due to KPMT blockade, and its minimal level in cn1 seems to be an effect of the specific 3-HOK synthesis impairment. As cd1 accumulates 3-HOK, in the case of protein non-enzymatic modification by 3-HOK, we would expect an increase in cd1 PB-3-HOK level. The reverse effect lets us to suppose a novel type of enzymatic modification of Drosophila proteins by 3-HOK, possibly associated with PHS. This explains the simultaneous decrease in 3-HOK enzymatic dimerization and its conjugation to proteins. Most of PB-3-HOK is located in the head capsules. As we have shown, it is also present in brains. An obvious PB-3-HOK decrease within CS Ped proves the specificity of staining. Ped contains the axons of Kenyon cells extending into the lobes of the mushroom body. This structure is known to be crucial for Drosophila olfactory learning and memory, but its role in 3-HOK distribution remains enigmatic.
The function of PB-3-HOK in Drosophila is unclear. 3-HOK and its derivatives can serve as UV-filters protecting retina (Korlimbinis and Truscott, 2006). Possibly, they play a similar role in the fly tissues. In some insects, xanthommatin forms a complex with an eye protein (Ajami and Riddiford, 1971). In Drosophila, the eye pigments are contained in a specific structure within pigment cells called ommochromasome, where PHS is localized (Figon and Casas, 2019). Thus, PB-3-HOK may be an intermediate of some protein-pigments complex in Drosophila eye.
Another role of 3-HOK conjugation to proteins may be its withdrawal from the active circulation. 3-HOK level is extremely high in Drosophila, reaching 396 μg/g fresh weight for cd1 tissues (Howells et al., 1977) or about 1.7 mM. This is much more than 3-HOK concentration used in vitro to induce apoptosis in neuronal cell culture (Okuda et al., 1996). Such a high 3-HOK level is necessary for Drosophila to synthesize eye pigments, but it’s excess would be toxic in the absence of a system of 3-HOK deactivation/deposition. Some specific protein carriers or membrane organelles, such as ommochromasomes, may perform this function.
In summary, 3-HOK accumulation seems to have a dual effect on cd1 SLA, enhancing it for the middle-aged flies and lowering it for the old flies. cd1 mutation also shows a dual effect on 3-HOK metabolism, disrupting xanthommatin synthesis, and 3-HOK conjugation to some proteins. In both cases, free 3-HOK should accumulate in cd1 brains leading to neurotoxicity development. Further studies, including PHS gene sequencing and checking the putative PHS involvement in PB-3-HOK formation, are necessary to elucidate the molecular mechanisms of 3-HOK metabolism in cd1.
Data Availability Statement
All datasets generated for this study are included in the article/Supplementary Material.
Author Contributions
AZ designed the study. AZ and PI performed the analysis of spontaneous locomotor activity. AZ and OV performed the analysis of PB-3-HOK level. AZ performed the analysis of PB-3-HOK distribution in Drosophila brains. AZ and ES-P wrote sections of the manuscript. All authors contributed to manuscript revision, read, and approved the submitted version.
Funding
The reported study was funded by Russian Foundation for Basic Research (RFBR) according to the research project N 18-34-00761.
Conflict of Interest
The authors declare that the research was conducted in the absence of any commercial or financial relationships that could be construed as a potential conflict of interest.
Acknowledgments
We thank A.A. Ilyinych (Herzen State Pedagogical University of Russia) for participating in SLA analysis and Dr. A.V. Medvedeva (Pavlov Institute of Physiology) for the help in making sample preparations for confocal microscopy. We also thank the Research park of Saint Petersburg State University, Resource Center for Ecological Safety Observatory for the opportunity to perform a study.
Supplementary Material
The Supplementary Material for this article can be found online at: https://www.frontiersin.org/articles/10.3389/fphys.2020.00971/full#supplementary-material.
Footnotes
References
Aimon, S., Katsuki, T., Jia, T., Grosenick, L., Broxton, M., Deisseroth, K., et al. (2019). Fast near-whole-brain imaging in adult Drosophila during responses to stimuli and behavior. PLoS Biol. 17:e2006732. doi: 10.1371/journal.pbio.2006732
Ajami, A. M., and Riddiford, L. M. (1971). Purification and characterization of an ommochrome-protein from the eyes of saturniid moths. Biochemistry 10, 1455–1460. doi: 10.1021/bi00784a029
Badawy, A. A. (2017). Kynurenine pathway of tryptophan metabolism: regulatory and functional aspects. Int. J. Tryptophan Res. 10, 1–20. doi: 10.1177/1178646917691938
Bayne, A. C., Mockett, R. J., Orr, W. C., and Sohal, R. S. (2005). Enhanced catabolism of mitochondrial superoxide/hydrogen peroxide and aging in transgenic Drosophila. Biochem. J. 391, 277–284. doi: 10.1042/BJ20041872
Campesan, S., Green, E. W., Breda, C., Sathyasaikumar, K. V., Muchowski, P. J., Schwarcz, R., et al. (2011). The kynurenine pathway modulates neurodegeneration in a Drosophila model of Huntington’s disease. Curr. Biol. 21, 961–966. doi: 10.1016/j.cub.2011.04.028
Christen, S., Peterhans, E., and Stocker, R. (1990). Antioxidant activities of some tryptophan metabolites: possible implication for inflammatory diseases. Proc. Natl. Acad. Sci. U. S. A. 87, 2506–2510. doi: 10.1073/pnas.87.7.2506
Colomb, J., Reiter, L., Blaszkiewicz, J., Wessnitzer, J., and Brembs, B. (2012). Open source tracking and analysis of adult Drosophila locomotion in Buridan’s paradigm with and without visual targets. PLoS One 7:e42247. doi: 10.1371/journal.pone.0042247
Creamer, M. S., Mano, O., and Clark, D. A. (2018). Visual control of walking speed in Drosophila. Neuron 100, 1460–1473.e6. doi: 10.1016/j.neuron.2018.10.028
Cunningham, P. C., Waldeck, K., Ganetzky, B., and Babcock, D. T. (2018). Neurodegeneration and locomotor dysfunction in Drosophila scarlet mutants. J. Cell Sci. 131:jcs216697. doi: 10.1242/jcs.216697
El-Defrawy, S. R., Boegman, R. J., Jhamandas, K., and Beninger, R. J. (1986). The neurotoxic actions of quinolinic acid in the central nervous system. Can. J. Physiol. Pharmacol. 64, 369–375. doi: 10.1139/y86-060
Emanuel, S., Kaiser, M., Pflueger, H., and Libersat, F. (2020). On the role of the head ganglia in posture and walking in insects. Front. Physiol. 11:135. doi: 10.3389/fphys.2020.00135
Ewing, A. W. (1963). Attempts to select for spontaneous activity in Drosophila melanogaster. Anim. Behav. 11, 369–378. doi: 10.1016/S0003-3472(63)80128-1
Fedotov, S. A., Bragina, J. V., Besedina, N. G., Danilenkova, L. V., Kamysheva, E. A., Panova, A. A., et al. (2014). The effect of neurospecific knockdown of candidate genes for locomotor behavior and sound production in Drosophila melanogaster. Fly 8, 176–187. doi: 10.4161/19336934.2014.983389
Figon, F., and Casas, J. (2019). Ommochromes in invertebrates: biochemistry and cell biology. Biol. Rev. Camb. Philos. Soc. 94, 156–183. doi: 10.1111/brv.12441
Green, E. W., Campesan, S., Breda, C., Sathyasaikumar, K. V., Muchowski, P. J., Schwarcz, R., et al. (2012). Drosophila eye color mutants as therapeutic tools for Huntington disease. Fly 6, 117–120. doi: 10.4161/fly.19999
Hilmas, C., Pereira, E. F., Alkondon, M., Rassoulpour, A., Schwarcz, R., and Albuquerque, E. X. (2001). The brain metabolite kynurenic acid inhibits alpha7 nicotinic receptor activity and increases non-alpha7 nicotinic receptor expression: physiopathological implications. J. Neurosci. 21, 7463–7473. doi: 10.1523/JNEUROSCI.21-19-07463.2001
Howells, A. J., Summers, K. M., and Ryall, R. L. (1977). Developmental patterns of 3-hydroxykynurenine accumulation in white and various other color mutants of Drosophila melanogaster. Biochem. Genet. 15, 1049–1059. doi: 10.1007/BF00484496
Kamyshev, N. G. (1980). Longetivity and its relation to the locomotor activity in Drosophila mutants with the defective tryptophan–xanthommatin metabolic pathway. Dokl. Akad. Nauk SSSR 253, 1476–1480.
Kessler, M., Terramani, T., Lynch, G., and Baudry, M. (1989). A glycine site associated with N-methyl-D-aspartic acid receptors: characterization and identification of a new class of antagonists. J. Neurochem. 52, 1319–1328. doi: 10.1111/j.1471-4159.1989.tb01881.x
Korlimbinis, A., and Truscott, R. J. (2006). Identification of 3-hydroxykynurenine bound to proteins in the human lens: a possible role in age-related nuclear cataract. Biochemistry 45, 1950–1960. doi: 10.1021/bi051744y
Lapin, I. P. (2004). Stress, Anxiety, Depression, Alcoholism, Epilepsy (the neuroactivities of kynurenines and new approaches to treatment). Saint Petersburg: DEAN.
Linzen, B. (1974). The tryptophan to ommochrome pathway in insects. Adv. Insect Physiol. 10, 117–246. doi: 10.1016/S0065-2806(08)60130-7
Martin, J. R., Raabe, T., and Heisenberg, M. (1999). Central complex substructures are required for the maintenance of locomotor activity in Drosophila melanogaster. J. Comp. Physiol. A. 185, 277–288. doi: 10.1007/s003590050387
Nikitina, E. A., Chernikova, D. A., Vasilyeva, O. V., Zhuravlev, A. V., Medvedeva, A. V., Domnina, N. S., et al. (2018). Effect of antioxidants on medium-term memory formation in Drosophila melanogaster cardinal mutant. Biotekhnologiya 34, 67–77. doi: 10.21519/0234-2758-2018-34-3-67-77
Okuda, S., Nishiyama, N., Saito, H., and Katsuki, H. (1996). Hydrogen peroxide-mediated neuronal cell death induced by an endogenous neurotoxin, 3-hydroxykynurenine. Proc. Natl. Acad. Sci. U. S. A. 93, 12553–12558. doi: 10.1073/pnas.93.22.12553
Okuda, S., Nishiyama, N., Saito, H., and Katsuki, H. (1998). 3-Hydroxykynurenine, an endogenous oxidative stress generator, causes neuronal cell death with apoptotic features and region selectivity. J. Neurochem. 70, 299–307. doi: 10.1046/j.1471-4159.1998.70010299.x
Savvateeva, E., Popov, A., Kamyshev, N., Bragina, J., Heisenberg, M., Senitz, D., et al. (2000). Age-dependent memory loss, synaptic pathology and altered brain plasticity in the Drosophila mutant cardinal accumulating 3-hydroxykynurenine. J. Neural Transm. 107, 581–601. doi: 10.1007/s007020070080
Savvateeva-Popova, E. V., Popov, A. V., Heinemann, T., and Riederer, P. (2003). Drosophila mutants of the kynurenine pathway as a model for ageing studies. Adv. Exp. Med. Biol. 527, 713–722. doi: 10.1007/978-1-4615-0135-0_84
Schindelin, J., Arganda-Carreras, I., Frise, E., Kaynig, V., Longair, M., Pietzsch, T., et al. (2012). Fiji: an open-source platform for biological-image analysis. Nat. Methods 9, 676–682. doi: 10.1038/nmeth.2019
Schwarcz, R., Bruno, J. P., Muchowski, P. J., and Wu, H. Q. (2012). Kynurenines in the mammalian brain: when physiology meets pathology. Nat. Rev. Neurosci. 13, 465–477. doi: 10.1038/nrn3257
Strauss, R., and Heisenberg, M. (1993). A higher control center of locomotor behavior in the Drosophila brain. J. Neurosci. 13, 1852–1861. doi: 10.1523/JNEUROSCI.13-05-01852.1993
Wu, J. S., and Luo, L. (2006). A protocol for dissecting Drosophila melanogaster brains for live imaging or immunostaining. Nat. protoc. 1, 2110–2115. doi: 10.1038/nprot.2006.336
Zakharov, G. (2017). Locotrack software [Internet]. Available at: https://github.com/GennadiyZakharov/locotrack (Accessed February 16, 2017).
Zakharov, G. A., Zhuravlev, A. V., Payalina, T. L., Kamyshov, N. G., and Savvateeva-Popova, E. V. (2012). The effect of mutations of the kynurenine pathway of tryptophan metabolism on locomotor behavior and gene expression in glutamatergic and cholinergic systems of D. melanogaster. Russ. J. Genet. Appl. Res. 2, 197–204. doi: 10.1134/S2079059712020141
Zhuravlev, A. V., Vetrovoy, O., and Savvateeva-Popova, E. V. (2018). Enzymatic and non-enzymatic pathways of kynurenines’ dimerization: the molecular factors for oxidative stress development kynyrenines’ dimerization and its impact in oxidative stress. PLoS Comput. Biol. 14:e1006672. doi: 10.1371/journal.pcbi.1006672
Zhuravlev, A. V., Zakharov, G. A., Shchegolev, B. F., and Savvateeva-Popova, E. V. (2012). Stacking interaction and its role in kynurenic acid binding to glutamate ionotropic receptors. J. Mol. Model. 18, 1755–1766. doi: 10.1007/s00894-011-1206-1
Keywords: Drosophila, kynurenine pathway, oxidative stress, spontaneous locomotor activity, 3-hydroxykynurenine, cardinal, protein modification by 3-hydroxykynurenine
Citation: Zhuravlev AV, Vetrovoy OV, Ivanova PN and Savvateeva-Popova EV (2020) 3-Hydroxykynurenine in Regulation of Drosophila Behavior: The Novel Mechanisms for Cardinal Phenotype Manifestations. Front. Physiol. 11:971. doi: 10.3389/fphys.2020.00971
Edited by:
Monique Gauthier, Université Toulouse III Paul Sabatier, FranceReviewed by:
Aram Megighian, University of Padua, ItalyAbraham Rosas-Arellano, National Autonomous University of Mexico, Mexico
Copyright © 2020 Zhuravlev, Vetrovoy, Ivanova and Savvateeva-Popova. This is an open-access article distributed under the terms of the Creative Commons Attribution License (CC BY). The use, distribution or reproduction in other forums is permitted, provided the original author(s) and the copyright owner(s) are credited and that the original publication in this journal is cited, in accordance with accepted academic practice. No use, distribution or reproduction is permitted which does not comply with these terms.
*Correspondence: Aleksandr V. Zhuravlev, YmVuZW9yQG1haWwucnU=
†Present address: Polina N. Ivanova, Laboratory of Epigenetics, Bioinformatics and Molecular Modeling, Saint Petersburg Institute of Bioregulation and Gerontology, Saint Petersburg, Russia