- 1Interdisciplinary Center for the Study of Human Performance (CIPER), Faculdade de Motricidade Humana, Universidade de Lisboa, Lisbon, Portugal
- 2Faculdade de Motricidade Humana, Universidade de Lisboa, Lisbon, Portugal
- 3Department of Physical Education, São Paulo State University (UNESP), Bauru, Brazil
- 4Institute of Bioscience, Graduate Program in Human Development and Technology, São Paulo State University (UNESP), Rio Claro, Brazil
- 5Department of Science and Technology, Polytechnic Institute of Setúbal, Setúbal, Portugal
- 6Quality of Life Research Center, Santarém, Portugal
This study analyzed whether 100- and 200-m interval training (IT) in swimming differed regarding temporal, perceptual, and physiological responses. The IT was performed at maximal aerobic velocity (MAV) until exhaustion and time spent near to maximalVO2 peak oxygen uptake (⩒O2peak), total time limit (tLim), peak blood lactate [La−] peak, ⩒O2 kinetics (⩒O2K), and rate of perceived exertion (RPE) were compared between protocols. Twelve swimmers (seven males 16.1 ± 1.1 and five females 14.2 ± 1 years) completed a discontinuous incremental step test for the second ventilatory threshold (VT2), ⩒O2peak, and MAV assessment. The swimmers subsequently completed two IT protocols at MAV with 100- and 200-m bouts to determine the maximal ⩒O2 (peak-⩒O2) and time spent ≥VT2, 90, and 95% of ⩒O2peak for the entire protocols (IT100 and IT200) and during the first 800-m of each protocol (IT8x100 and IT4x200). A portable apparatus (K4b2) sampled gas exchange through a snorkel and an underwater led signal controlled the velocity. RPE was also recorded. The Peak-⩒O2 attained during IT8x100 and IT4x200 (57.3 ± 4.9 vs. 57.2 ± 4.6 ml·kg−1·min−1) were not different between protocols (p = 0.98) nor to ⩒O2peak (59.2 ± 4.2 ml·kg−1·min−1, p = 0.37). The time constant of ⩒O2K (24.9 ± 8.4 vs. 25.1 ± 6.3-s, p = 0.67) and [La−] peak (7.9 ± 3.4 and 8.7 ± 1.5 mmol·L−1, p = 0.15) also did not differ between IT100 and IT200. The time spent ≥VT2, 90, and 95%⩒O2peak were also not different between IT8x100 and IT4x200 (p = 0.93, 0.63, and 1.00, respectively). The RPE for IT8x100 was lower than that for IT4x200 (7.62 ± 2 vs. 9.5 ± 0.7, p = 0.01). Both protocols are considered suitable for aerobic power enhancement, since ⩒O2peak was attained with similar ⩒O2K and sustained with no differences in tLim. However, the fact that only the RPE differed between the IT protocols suggested that coaches should consider that nx100-m/15-s is perceived as less difficult to perform compared with nx200-m/30-s for the first 800-m when managing the best strategy to be implemented for aerobic power training.
Introduction
Interval training (IT) has been considered an effective exercise plan to improve endurance performance and maximal aerobic velocity (MAV, i.e., the velocity corresponding to the peak oxygen uptake, ⩒O2peak; Billat and Koralsztein, 1996; Billat et al., 2000; Dalamitros et al., 2016), and, therefore, has been proposed as a successful way to enhance cardiovascular and muscle adjustments needed to optimize performance during middle-distance racing in different sports, e.g., running and swimming (Billat, 2001; Libicz et al., 2005; Reis et al., 2012a,b; Espada et al., 2015, 2021). The time sustained with ⩒O2 responses closer to the maximal rates (90–100% of ⩒O2peak) is considered an important factor to maximize aerobic training benefits (⩒O2peak, O2 transport, and mitochondrial density) and avoid high oxygen deficits and fast metabolite accumulation, which can contribute to an increase in endurance capacity and tolerance at severe and maximal intensities (Billat and Koralsztein, 1996; Millet et al., 2003a,b; Bentley et al., 2005; Sousa et al., 2017).
The IT planning requires the organization of several parameters, such as work intensity, distance and duration, rest mode (active or passive) and duration, number of bouts to be performed (n repetitions), number of sets, and the duration of recovery between sets (Buchheit and Laursen, 2013a,b). When IT is planned to increase the time limit at MAV or/and to ensure an increase in time exercising closer to ⩒O2peak response, workouts have been designed with repeated bouts lasting 2–4 min, which is characterized as long-term work intervals (Buchheit and Laursen, 2013b; Wen et al., 2019). However, performing short-duration work intervals (<60 s) could allow the athlete to complete longer IT sessions with greater oxidative demands and lower anaerobic glycolytic contribution than long work intervals, despite the similarities between short and long work intervals regarding the time spent at ⩒O2peak (Zuniga et al., 2011; Rønnestad and Hansen, 2016) and the effectiveness for improving ⩒O2peak (Wen et al., 2019). Hence, the planning of work interval duration must consider the energetic balance that matches the specificity of the race to be performed.
When performing continuous exercise at MAV, the time limit approximates to ~5 min for different exercise modes (running, cycling, swimming, and paddling; Billat et al., 1996a). However, IT has been reported to increase significantly the time limit and time spent at high ⩒O2 when designed either with short- or long-distance work intervals at 1:1 ratio (30:30 or 120:120 s) but with higher blood lactate accumulation (>3 mmol·L−1) and oxygen deficit (>~5 ml·kg−1) when using the latter (Billat, 2001; Zuniga et al., 2011; Buchheit and Laursen, 2013b). In swimming, short-distance work intervals (n × 100-m) performed at submaximal or maximal velocities (≤95 or 100% MAV) have been shown to induce higher (absolute) time limit and time spent at submaximal or maximal ⩒O2 (>90 or 100% ⩒O2peak) than a single trial performed at same velocities (Bentley et al., 2005; Libicz et al., 2005; Sousa et al., 2017). Although the literature is not extensive, the temporal and ⩒O2 responses during IT in swimming, seems to point out that using 60–120-s work intervals at velocities ≥95% of MAV is recommended to stimulate improvements in aerobic power and endurance in high swimming intensity (Dalamitros et al., 2016; Sousa et al., 2017).
However, there are still doubts on how to define the IT to provide the best combination of aerobic and anaerobic responses in swimming, especially considering the requirements for successful performance in middle-distance events, as proposed for running and cycling (Billat, 2001; Spencer and Gastin, 2001; Buchheit and Laursen, 2013b). In swimming, performing IT at MAV with 1:1 or 1:1/2 ratios for work:rest elicits only moderate blood lactate accumulation, clearly lower values than those reported for running and cycling (Billat et al., 2000; Zuniga et al., 2011), which is probably attributed to the clearance mechanism during long rest periods (Bentley et al., 2005; Libicz et al., 2005). Therefore, we could expect that longer work intervals or decreases in the rest periods would lead to higher anaerobic glycolic energy release (Buchheit and Laursen, 2013b). However, this has not been studied in swimming.
⩒O2 kinetics (⩒O2K) has been associated with endurance performance (Jones and Burnley, 2009; Reis et al., 2012b; Espada et al., 2015; Almeida et al., 2020) and time spent at ⩒O2max (Millet et al., 2003a,b; Sousa et al., 2018), since faster kinetics can represent an accelerated oxidative rate. It has been reported that athletes with faster ⩒O2K can reach ⩒O2peak faster and present lower oxygen deficits (Millet et al., 2003b). However, Bentley et al. (2005) did not find any influence of faster kinetics with the time spent near ⩒O2 maximal values on swimmers when performing IT with 400-m bouts. Furthermore, Sousa et al. (2015) reported that swimmers seem to have slower ⩒O2K than runners and cyclists, which can indicate that IT in swimming could require longer work intervals to induce near maximal ⩒O2 responses.
Considering that different combinations of the IT parameters truly induce different acute physiological responses (time spent closer to maximal ⩒O2), it is crucial to investigate different types of IT. Therefore, to understand whether different combinations of IT produce different but high aerobic and anaerobic responses, while exercising at MAV, this study compared the ⩒O2, blood lactate accumulation, oxygen deficit, and rate of perceived exertion (RPE) responses during two different ITs, designed with 100- (IT100) and 200-m (IT200) swimming bouts, until exhaustion. The first 800 m of each IT session was also considered for analysis in order to allow a direct comparison between training sets (IT8x100 vs. IT4x200). We chose this format for IT in an attempt to represent the usual intermittent bouts in the daily training routine and therefore expected an analysis that is more ecological.
We hypothesize that both ITs will elicit the achievement of ⩒O2peak; however, IT100 will present longer times to exhaustion and consequently longer times spent near swimmers ⩒O2 maximal values, and swimmers with faster ⩒O2K will also present longer times to exhaustion and times spent near ⩒O2peak.
Materials and Methods
Experimental Design
To analyze the physiological and temporal responses during two different intermittent swimming (IT) protocols, the peak oxygen uptake (⩒O2peak), second ventilatory threshold (VT₂), and MAV were assessed by a discontinuous incremental step test performed until a maximal 200-m pace or to volitional exhaustion. In a randomized order, the swimmers performed two different IT protocols until exhaustion at MAV, consisting of 100 or 200-m repetitions, to compare the ventilatory and physiological responses between the two IT formats. All the swimmers performed the three testing protocols in front crawl swimming with in-water starts and open turns without underwater gliding (in a 25-m swimming pool), with gas exchange analysis recorded by a portable gas analyzer (K4b2, Cosmed®, Rome, Italy) connected to the swimmers by a respiratory snorkel and a valve system (new-AquaTrainer®, Cosmed, Rome, Italy). The transportation of this system along the swimming pool can be watching in the Supplementary Material.
The participants were instructed to report to the swimming pool well hydrated, fed, and to abstain from caffeine, alcohol, and strenuous exercise in the 24 h preceding the testing protocols. The same environmental conditions (time of day ± 2 h, water temperature ~28°C, and relative humidity ~50%) and same pre-test warm up protocol were ensured for all tests in order to minimize the effects of circadian rhythms and differences in prior exercise. The sessions were performed in the beginning of the preparatory period of the second macrocycle of competitive season of the swimmers, after a period of 2 weeks for training adaptation and were separated by at least 48 h.
Participants
Twelve well-trained young swimmers (seven males and five females) were informed about the procedures and experimental risks of the study and signed a written informed consent (and their legal guardians when under 18 years old). All the swimmers were fully familiarized with the equipment and procedures before the beginning of the tests. The recruited swimmers had to be regularly competing in state or national championships for a minimum of 3 years prior to the beginning of the study, as a criterion to participate in this study. This study was approved by the local University Ethical Committee (CEFMH: 39/2015) and was conducted in accordance with the 1964 Declaration of Helsinki (Harriss et al., 2017). The descriptive characteristics of the swimmers are presented in Table 1.
Incremental Step Test and Interval Training Protocol
The discontinuous incremental step test was structured with 6 × 250 and 1 × 200-m steps performed with 30-s rest for blood lactate sampling (Espada et al., 2015; Almeida et al., 2020). The velocity started at 50% of the velocity at 200-m (v200-m) maximal performance, which was performed 48 h before the execution of the incremental step test and ensured the similar swimming mode (in water starting, open turns and no underwater gliding). The following steps were incremented at 55, 60, 70, 80, 90, and 100% rates of v200-m, aiming to ensure a narrow rest-to-work transition for the three initial steps and therefore enabling ideal warming with no metabolism compromise (premature acidosis and glycogen depletion) for the remaining steps (Espada et al., 2015; Almeida et al., 2020).
In a randomized order, the swimmers performed two different IT swimming protocols at MAV 48 h after the incremental test and 48 h apart from each other. The IT was performed until voluntary exhaustion, following the protocols: (1) n repetitions of 100-m interspersed by 15-s of rest (IT100), and (2) n repetitions of 200-m interspersed by 30-s of rest (IT200). The comparison between each IT protocol considered the first 800 m (IT8x100 and IT4x200, respectively for the IT100 and IT200), as well as the entire IT100 and IT200 protocols, analyzing temporal, perceptual, and physiological responses.
For the control of swimming velocity during each step of the incremental test and during each n repetition of IT100 and IT200, an underwater visual pacer was employed, which was designed with 26 led lights that subsequently signaled the pacing (Pacer2Swim®, KulzerTEC, Santa Maria da Feira, Portugal), and was used to provide the swimmers an accurate notion of the correct velocity for each test. Figure 1 depicts an overall view of the IT protocols.
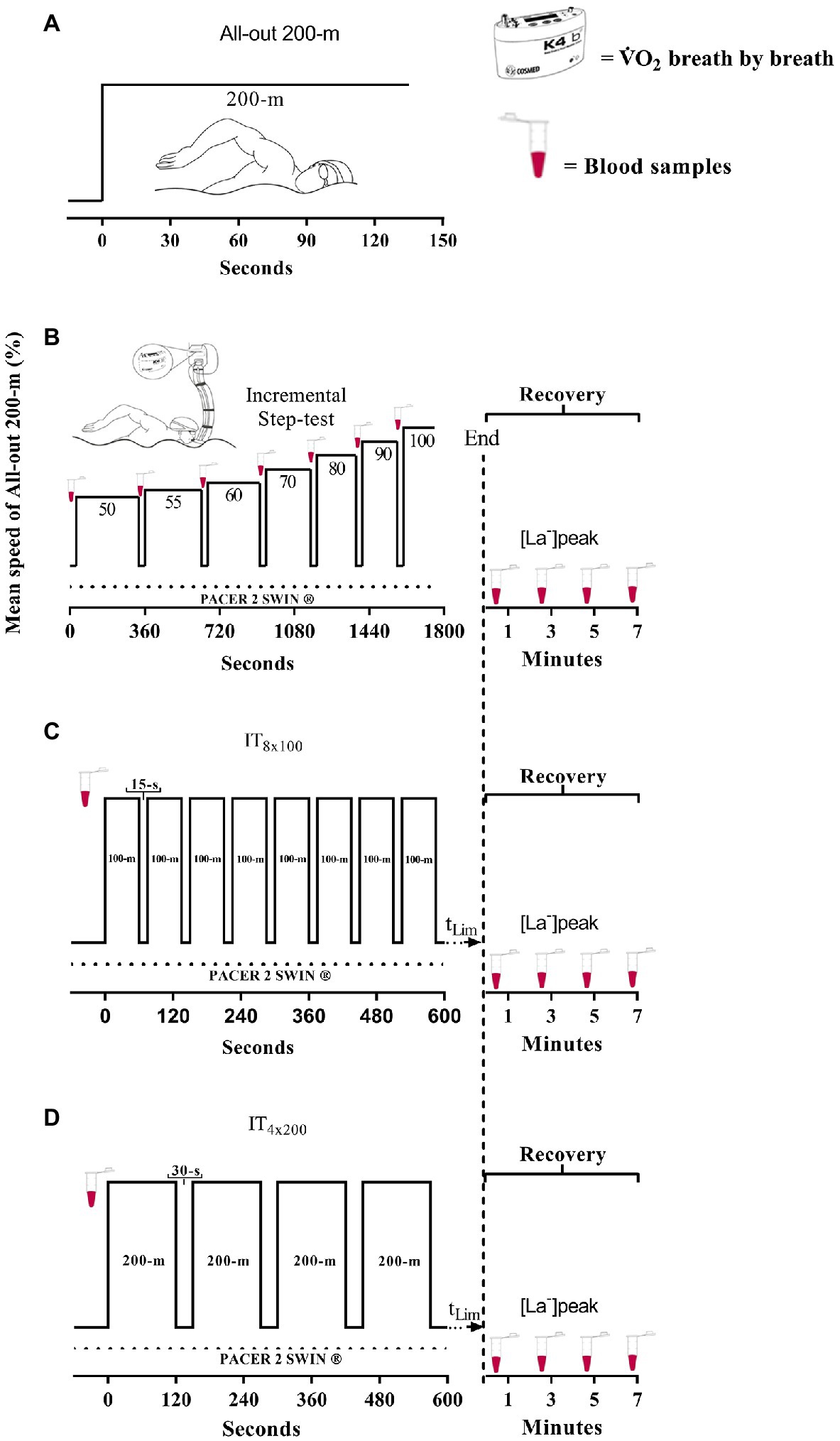
Figure 1. Overview of experimental design for 200-m performance (A), discontinuous incremental step test (B), IT8x100 (C), and IT4x200 (D). The total time limit (tLim) indicates n repetitions until exhaustion during IT100 and IT200, respectively (A,B).
Measurements and ⩒O2 Kinetics
For the gas exchange analysis, a telemetric portable gas analyzer (K4b2, Cosmed®, Rome, Italy) was connected to the swimmers by a respiratory snorkel and a valve system (new-AquaTrainer®, Cosmed, Rome, Italy), allowing breath-by-breath pulmonary gas collection (Reis et al., 2010; Baldari et al., 2013). The system was moved alongside the swimmers by a member of the research team. Before the start (10 min of resting), during, and after each protocol (at 1, 3, 5, and 7-min in the recovery phase) capillary blood samples (25 μl) were collected from the earlobe (carefully dried before each sampling) for blood lactate [La−] analysis (YSI, 2300 STAT®, Yellow Springs, Ohio). Exceptionally during the IT protocols, the blood samples were collected before (at rest) and after (at recovery) only. The peak of [La−] concentration ([La−]peak) was measured in the recovery phase after the incremental step test and each IT protocol. The RPE was recorded through the CR-10 scale of Borg (1990).
During the incremental step test, the ⩒O2peak was measured as the highest 30-s (moving) averaged ⩒O2 in each step, and MAV was considered as the velocity corresponding to the step where ⩒O2peak occurred (Billat and Koralsztein, 1996). VT₂ was determined by gas analysis in the incremental test according to the recommendations of Filho et al. (2012), and was examined visually using the responses from the V̇E/V̇CO2, V̇E/⩒O2, PETCO2, and PETO2 parameters. The criterion was the continuous increase in V̇E/⩒O2 and V̇E/V̇CO2 ratio curves related to the reduction in PETCO2. The point of VT2 localization was observed by two independent experts. Swimming velocity at VT2 corresponded to the incremental testing step at which VT2 occurred. Maximal exertion during the incremental step test was ensured by analyzing secondary criteria, as [La−]peak (≥8 mmol·l−1) and respiratory exchange ratio (RER > 1; Baldari et al., 2013). The maximal 30-s (moving) averaged ⩒O2 attained during each IT protocol was considered the Peak-⩒O2, and the MPeak-⩒O2 was the average of the maximal ⩒O2 (30-s moving average) attained during each bout of the IT protocols. Both Peak-⩒O2 and MPeak-⩒O2 were calculated in IT8x100 and IT4x200, as well as for the entire IT100 and IT200.
The time spent (in seconds) with ⩒O2 above the VT₂(t@VT2), 90% (t@90%), and 95% (t@95%) of ⩒O2peak and the corresponding percentage (%) for the total duration of each IT were determined, as well as the time to exhaustion (tLim) and distance performed by each swimmer.
For the ⩒O2K analysis, the outliers (exclusion of values lying over three SDs from the local mean) were previously excluded from the analysis, and the breath-by-breath data were interpolated into 1-s values. Only the first bout of each IT protocol (100 and 200-m) was used for the determination of the ⩒O2K parameters [time delay (TD), time constant (τ), and amplitude (A)]. The cardiodynamic phase of the ⩒O2 response at the onset of the exercise was discharged by removing the first 20 s of the ⩒O2 response (Filho et al., 2012). As described by Reis et al. (2010), an individual “snorkel delay” (ISD) that corresponded to the difference between the onset of exercise and the time when the following breaths summed up a tidal volume superior to the outlet tube volume was calculated for each test. The ISD was adapted to the specific characteristic of the snorkel device used in this study and then integrated into the time delay of the ⩒O2 response. The ⩒O2 vs. time mono-exponential adjustments were analyzed through an iterative procedure by minimizing the sum of the mean squares of the differences between the modeled and the measured ⩒O2 values, according to the following equation:
where ⩒O2(t) represents the relative ⩒O2 at a given time; ⩒O2base represents the ⩒O2 at rest, which was calculated as the average of the first 30-s of the last minute before the start of the exercise (after 10-min of passive rest); TD, τ, and A represent the time delay, time constant, and amplitude of the primary phase of the ⩒O2response, respectively (Rodríguez et al., 2003; Sousa et al., 2013; Almeida et al., 2020). The oxygen deficit (O2Def) at the onset of the first 100 and 200-m of each IT protocol was measured as the product between mean response time (MRT) and A, where the MRT is TD × τ (Whipp et al., 2005).
Statistical Analysis
Initially, normality and homogeneity of data were accessed by the Shapiro–Wilk and Levene tests. The comparison of the temporal, perceptual, and physiological responses between the two IT protocols was performed considering all the samples with the t-test for unpaired samples, or with the Mann–Whitney test when the assumptions for parametric tests were not met. The Kruskal–Wallis test compared ⩒O2peak and [La−]peak responses during the incremental step test vs. Peak-⩒O2 during IT8x100 and IT4x200, and vs. Peak-⩒O2 during the entire IT100 and IT200 protocols. The Spearman coefficient (ro) tested the rank-order correlation between physiological, perceptual, and temporal responses during the IT protocols. The effect sizes (ES) were calculated by Cliff’s δ, considering the n and p values for the differences analyzed by the Mann–Whitney test, which was interpreted as 0.2 weak, 0.36 medium, 0.52 strong, and 0.76 very strong (Sheskin, 2011). The ro was interpreted as <0.2 (trivial), 0.2–0.49 (small), 0.5–0.8 (medium), and >0.8 (large; Ferguson, 2009). All statistical analyses were performed with the Statistical Package for the Social Sciences (version 25.0; SPSS®, Chicago, IL, United States), and statistical significance was accepted at p ≤ 0.05.
Results
The physiological responses of the swimmers during the incremental step test are shown in Table 2. The [La−]peak and RER reached values corresponding to the maximal exertion, and the entire sample of participants (male and female) exhibited no large variance for maximal and submaximal indexes of aerobic conditioning level [coefficient of variation (CV) < 10% for ⩒O2peak and VT2]. An individual response of ⩒O2 increasing during the incremental step test and the profile of gas-exchange variable (⩒E, ⩒CO2, ⩒E/VO2, and ⩒E/⩒CO2) matching VT2 criteria are illustrated in Figure 2.
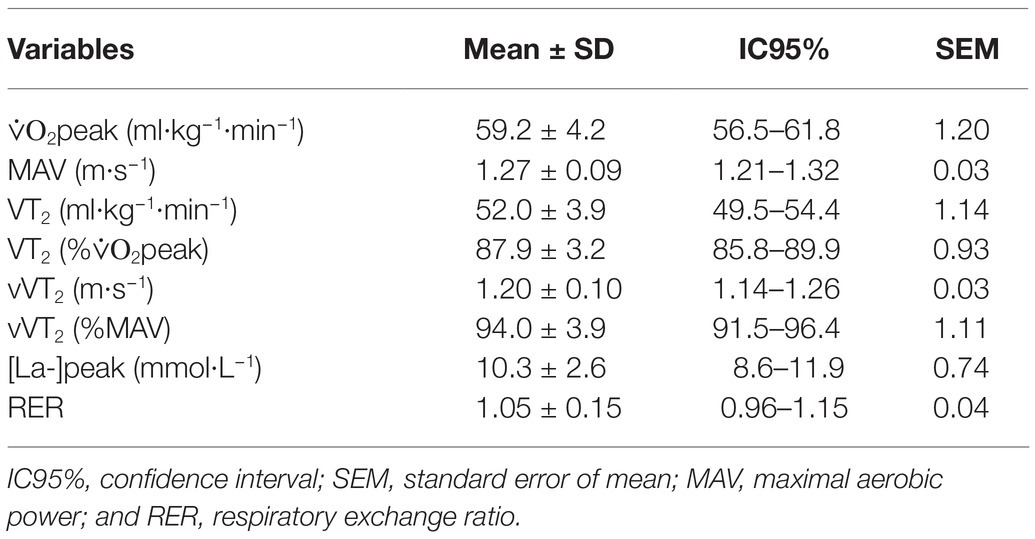
Table 2. Measurements (mean ± SD) during the incremental step test for the entire groups of participants (N = 12).
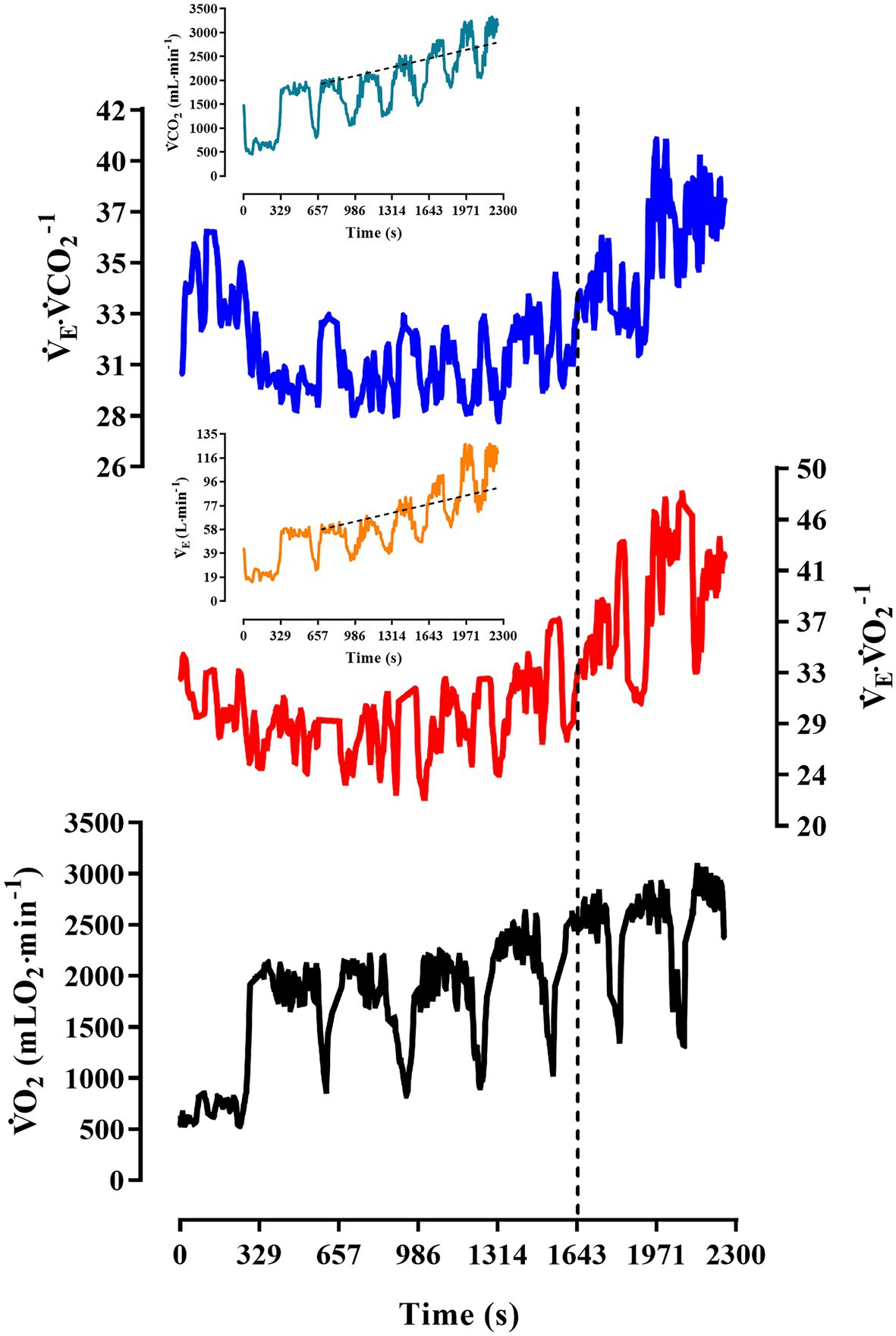
Figure 2. Pulmonary ⩒O2 and other gas-exchange responses during the incremental step test for a male participant. The vertical dashed line indicates VT2 occurrence and the inclined dashed line illustrates isocapnic disturbance and ventilatory compensation. The progression for this male swimmer ranged from 67 to 100%⩒O2peak, and time performance range was 265–161-s, respectively, from the first to the seventh step.
The physiological and perceptual responses during the IT protocols and ⩒O2K analysis are shown in Table 3. Typical responses of ⩒O2 in IT8x100 and IT4x200 are illustrated in Figure 3 for the male (panels A and B) and female (panels C and D) swimmers. The velocities while performing IT100 and IT200 did not differ from MAV (p = 0.89 and p = 0.39, respectively) or between each other (p = 0.44). When comparing ⩒O2peak vs. Peak-⩒O2 (IT8x100) vs. Peak-⩒O2 (IT4x200), no significant differences were observed (p = 0.37). However, differences were observed for the comparison of ⩒O2peak vs. MPeak-⩒O2 (IT8x100) vs. MPeak-⩒O2 (IT4x200; p = 0.01). Similar results were observed when comparing the IT100 and IT200 protocols. Therefore, there were no significant differences for ⩒O2peak vs. Peak-⩒O2 (IT100) vs. Peak-⩒O2 (IT200; p = 0.32), but significant differences were observed for ⩒O2peak vs. MPeak-⩒O2 (IT100) vs. MPeak-⩒O2 (IT200; p < 0.01). Additionally, no significant differences were observed for [La−]peak responses after the incremental step test vs. IT100 vs. IT200 (p = 0.15). Regarding the RPE, differences were observed when comparing IT8x100 vs. IT4x200 (p = 0.012; δ = 0.36) but no differences for IT100 vs. IT200 (p = 0.55). The measurements of A1 (p = 0.38), TD (p = 0.89), τ (p = 0.67), and O2Def (p = 0.98) did not differ between IT100 and IT200.
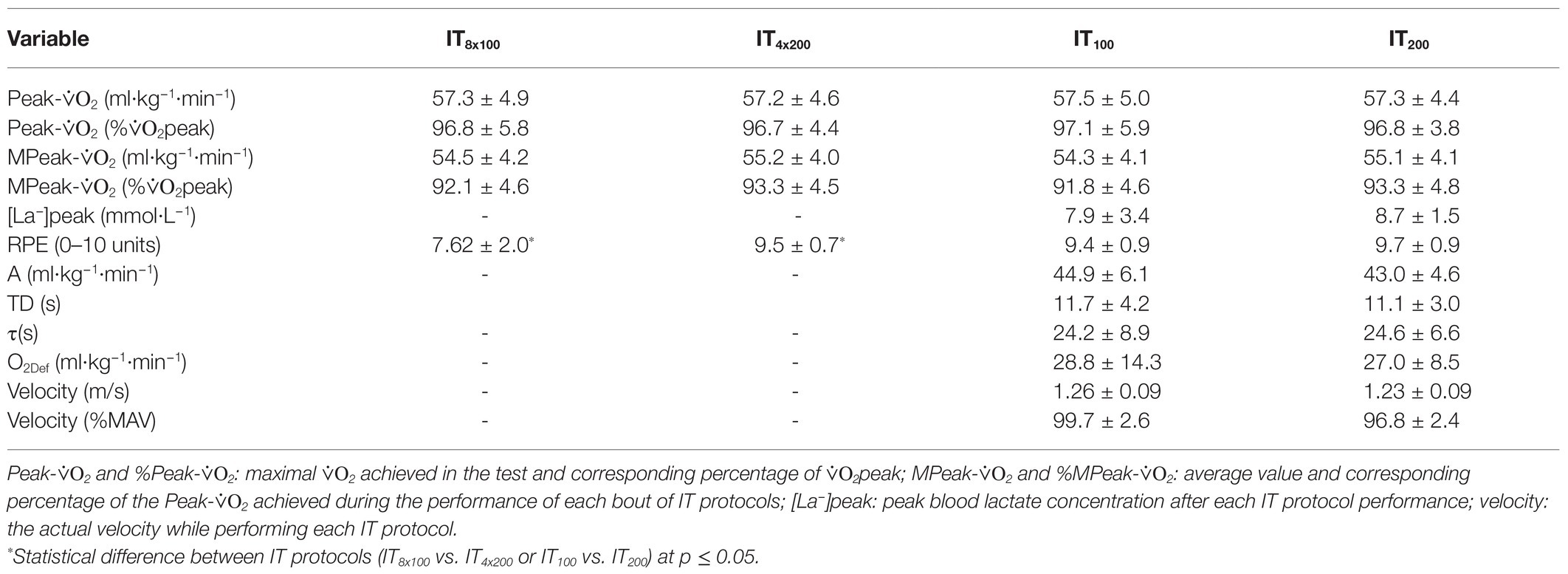
Table 3. Mean ± SD of the physiological and perceptual responses during the IT protocols, and ⩒O2 kinetics (⩒O2K) during the first bout of IT100 and IT200 (N = 12).
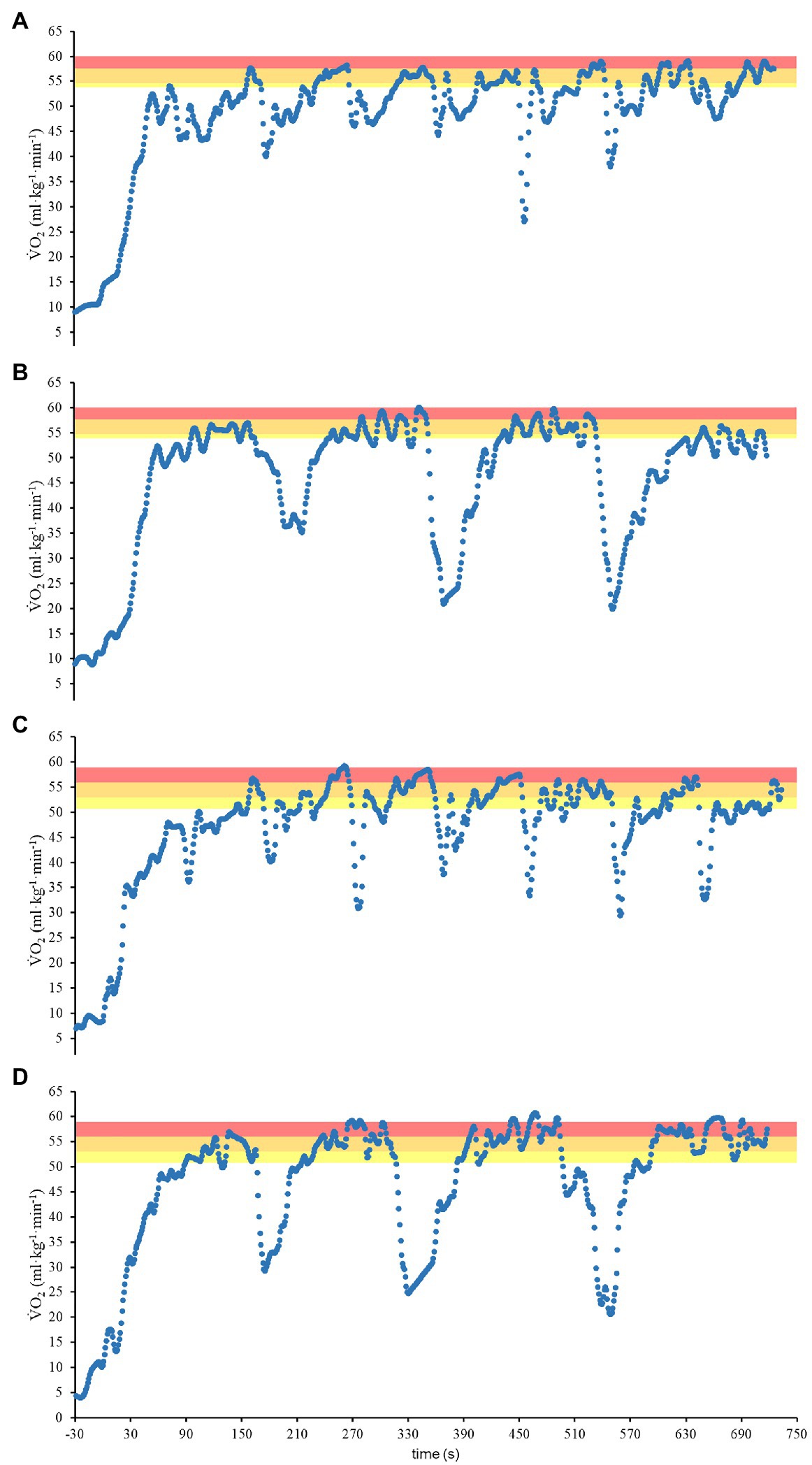
Figure 3. ⩒O2 response during IT8x100 (A,C) and IT4x200 (B,D) for male (A,B) and female (C,D) swimmers. The yellow, orange, and red shadow areas highlight the t@VT2, t@90%, and t@95% of ⩒O2peak, respectively.
The values of distance, time limit, and time spent above VT₂, 90, and 95% of ⩒O2peak during the IT protocols are shown in Table 4. The comparison between the percentage of t@VT₂, t@90%, and t@95% of ⩒O2peak during each IT protocol are illustrated in Figure 4. There were no significant differences in distance (p = 0.09) and tLim (p = 0.16) between IT100 and IT200. No significant differences were observed when comparing IT8x100 vs. IT4x200 with regard to t@VT₂ (p = 0.72), t@90% (p = 0.63), and t@95% (p = 1). Similarly, IT100 vs. IT200 did not differ regarding t@VT₂ (p = 0.22), t@90% (p = 0.29), and t@95% (p = 0.16). However, t@VT2 was higher than t@95% during IT8x100 (p < 0.01) and IT4x200 (p < 0.01).
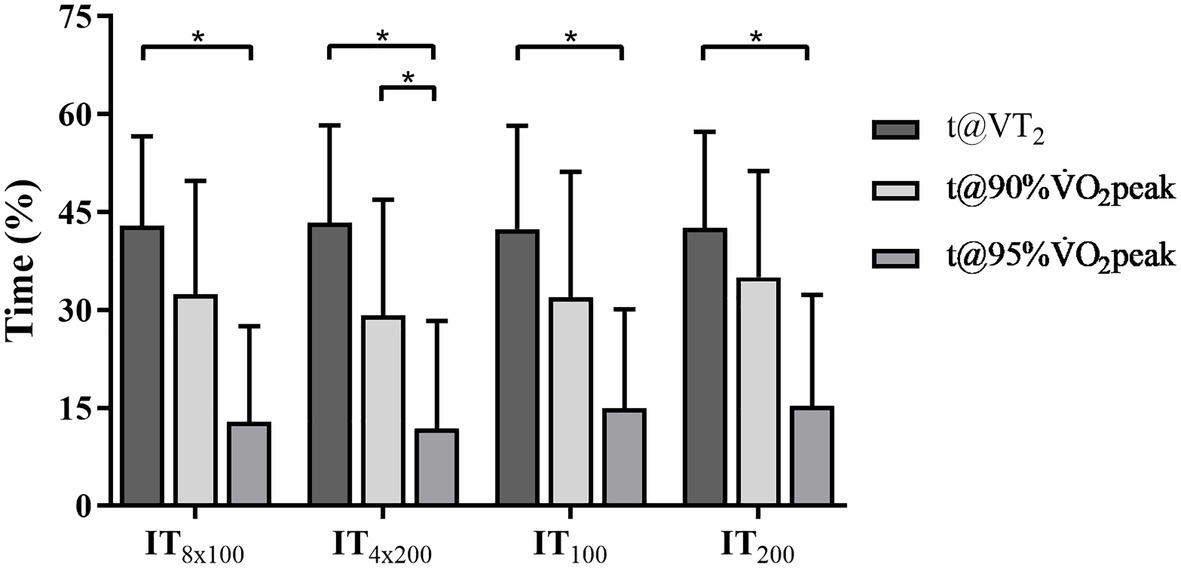
Figure 4. The percentage of t@VT2, t@90%⩒O2peak, and t@95%⩒O2peak at IT8x100, IT4x200, IT100, and IT200. *Statistical difference intra interval training (IT) protocols (t@VT2 vs. t@90%⩒O2peak vs. t@95%⩒O2peak) at p ≤ 0.05. No differences were observed between the IT protocols (IT8x100 vs. IT4x200 or IT100 vs. IT200).
The correlation between the IT protocols with regard to the temporal, physiological, and perceptual responses is shown in Table 5. The total distance and tLim attained during IT100 and IT200 did not associate the protocols with each other (p = 0.11 and p = 0.27) regarding performance. However, the [La−]peaks after IT100 and IT200 are positively correlated (p < 0.01), as well as τ and O2Def for ⩒O2K during the first bout of IT100 and IT200 (p = 0.03 and p = 0.05), despite Peak-⩒O2 not being associated when analyzing IT100 vs. IT200 (p = 0.53) and IT8x100 vs. IT4x200 (p = 0.6). Therefore, the IT protocols are associated with each other only with regard to some features of anaerobic contribution and initial ⩒O2 response. The tLim at IT100 and IT200 correlated with RPE at the strong (p < 0.01) and poor (p = 0.08) levels, respectively, but the distance swam at IT100 and IT200 correlated both with RPE (p < 0.01 and p = 0.04). The [La−]peak after IT100 showed no correlation with tLim in IT100 (p = 0.09), and negative correlation with total distance in IT100 (p = 0.03). Additionally, the [La−]peak after IT200 positively correlated with t@90%, t@95%, and O2Def during IT4x200 (p = 0.03; p = 0.05; p < 0.01). ⩒O2peak and MAV correlated negatively with [La−]peak after IT100 [ro = −0.8, p < 0.01, (medium); ro = −0.71, p = 0.01, (medium)]. MAV correlated negatively with t@95% during IT200 (ro = −0.59, p = 0.04), while ⩒O2peak had a positive correlation with total distance in IT100 (ro = 0.55, p = 0.07), as well as ⩒O2peak correlating significantly only with Peak-⩒O2 during IT4x200 [ro = 0.64, p = 0.02, (medium)] but showed a tendency with Peak-⩒O2 during IT8x100 (ro = 0.52, p = 0.09).
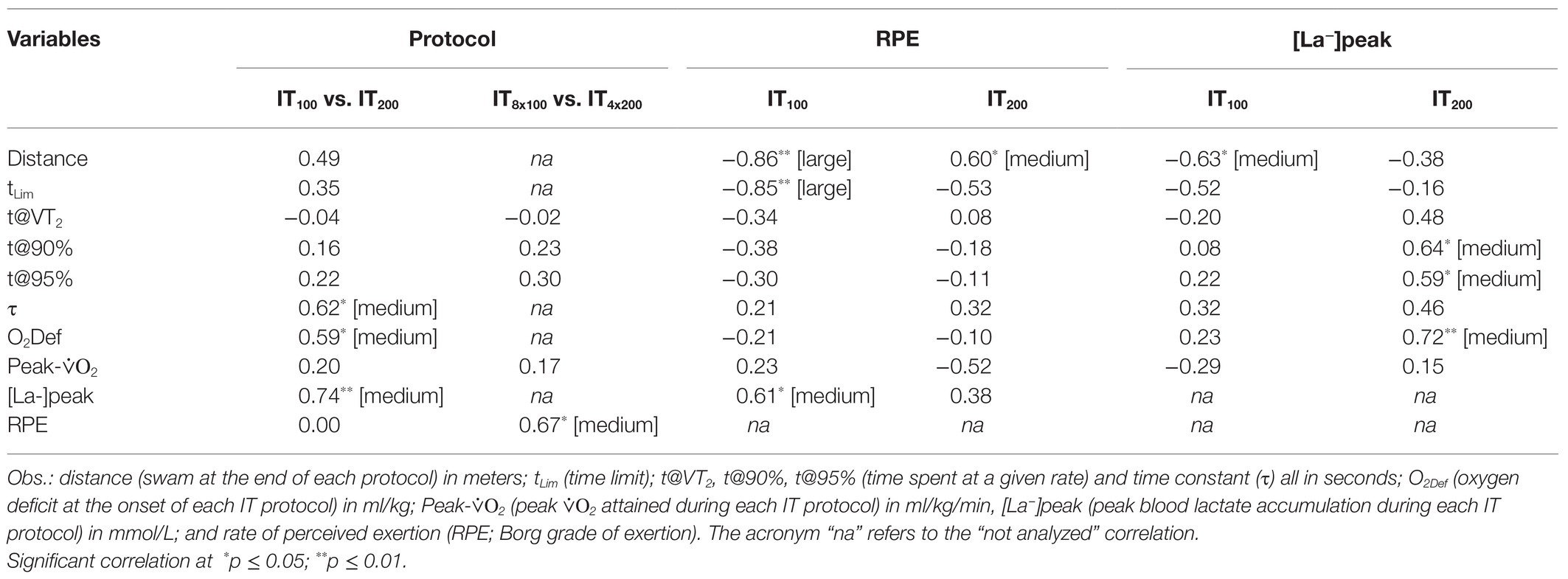
Table 5. Spearman rank-order coefficients for the responses of temporal, physiological, and perceptual variables between the IT protocols.
Discussion
This study analyzed the ⩒O2 response, as well as the blood lactate concentration and oxygen deficit induced by different intermittent training protocols. The findings support that the two IT formats studied (100-m/15-s and 200-m/30-s performed at MAV) were able to stimulate the exertion level close to maximal ⩒O2, as well as moderate to high anaerobic stimulation when considering blood lactate accumulation and oxygen deficit. Therefore, both training protocols showed to elicit physiological responses that were typical of middle-distance swimming performance. Moreover, the analysis of the first 800 m allowed the comparison between both training protocols (IT8x100 vs. IT4x200), highlighting that the perception of exertion level differed, while performing each IT, with a significantly higher RPE at IT4x200.
Other important findings to be highlighted are (i) the Peak-⩒O2 attained during both IT protocols did not differ from the ⩒O2peak attained during the incremental step test, which suggests that independently of the IT protocol (nx100-m/15-s and nx200-m/30-s) a maximal demand upon aerobic contribution was imposed; and (ii) the features of the stimulus upon [La−]peak and O2Def denoted a moderate to high reliance on anaerobic contribution, which was similar between the IT protocols and therefore reproduce the energetics required in middle-distance events. In perceptual terms, these IT protocols differed regarding the sensation of exertion, with IT8x100 perceived as a less exhaustive exercise, despite no physiological difference between the IT protocols.
To the best of the knowledge of the authors, only two studies analyzed the ⩒O2 response in swimming during IT protocols. Libicz et al. (2005) reported that well-trained triathletes spent double the time above 95% of ⩒O2max (~145 vs. ~69-s) in 8 × 100-m/30-s than in 16 × 50-m/15-s repetitions, despite the large variability observed in the ⩒O2 data constraining the level of statistical significance between each IT. Another study measured time sustained closer to ⩒O2max (>90% ⩒O2max) during two different IT protocols (16 × 100 vs. 4 × 400 m) performed at submaximal intensity (Δ25%LT-⩒O2max; Bentley et al., 2005). Similar to this study, the authors reported no influence of the work interval duration on time sustained above 90% of ⩒O2max (~564 vs. 341-s) nor on the maximal ⩒O2 (~93 vs. 92%) reached during each IT, as well as reporting no correlation between faster ⩒O2K (τ ~17-s during 400-m) and longer times spent closer to ⩒O2max. The lack of significance for the differences was attributed to the large variability, which is therefore corroborated to the current data for either total time-limit or time spent at a high ⩒O2 in swimming, which was higher than 30% and even larger at higher exercise intensity (90 and 95% ⩒O2peak) for both IT.
Notwithstanding, the variability in time-limit performance sounds not to be a matter of sex-related differences in the sample, since other studies including only males or combining male and female participants also reported large temporal variability (Billat et al., 1999; Zuniga et al., 2011), despite sex differences regarding the time limit in intermittent swimming performance remaining to be investigated. For continuous performance in swimming at paces demanding high ⩒O2, there are no differences in time limit between male and female swimmers, regardless of conditioning level (Fernandes et al., 2006). Indeed, the exercise tolerance (the endurance performance) during ⩒O2 sustained closer to ⩒O2peak is determined by the ability of the muscle system to attenuate the reliance on anaerobic sources at the onset of exercise, as well as the accumulation of metabolites, which are processes often analyzed through oxygen deficit, blood lactate accumulation, and ⩒O2 on-kinetics (Murgatroyd et al., 2011), with responses to specific exercise but not constrained to sex differences (Billat et al., 1996b; Carter et al., 2006; Reis et al., 2017).
Therefore, the decrease or increase in anaerobic reliance during intermittent exercise relies on the modification of the ratio of work and rest intervals (Billat, 2001; Buchheit and Laursen, 2013b). Interval training has been proposed to increase the time exercising with high ⩒O2 demand, which is not attained without a high stimulus on anaerobic glycolysis metabolism (Billat et al., 2000). However, according to Zuniga et al. (2011) and Rønnestad and Hansen (2016), short work intervals (30-s) compared with longer ones (3-min) may allow athletes to complete longer IT exercise sessions with greater metabolic demands and lower [La−]. Despite the study of Libicz et al. (2005), which argued that short work interval IT in swimming fails to induce longer time spent near ⩒O2max, while inducing an excessive muscular fatigue or acidosis for an effective improvement in endurance and middle-distance performance, this study was the first to evidence this combination.
With regard to the ability of the IT protocols to elicit maximal ⩒O2, this study observed that t@VT2 (exercise in a severe domain, encompassing time spent at or above VT2) is higher than 80% of tLim to perform either the first 800-m or the entire IT100 and IT200 protocols, while t@90% comprised 40–50% of time for the first 800-m or tLim for the entire protocols. Despite that the VT2 was attained ~88%⩒O2peak in this study, swimming at or above VT2 leads to an appreciable increase in VO2 (Pessôa Filho, 2012). Hence, the protocols studied enabled the increase in the time spent closer to ⩒O2peak, when compared with the findings reported by Sousa et al. (2017) for continuous swimming performance at or above 90%⩒O2peak at 90 and 100% of MAV (~78 and ~72%). Moreover, even considering time at or above 90% ⩒O2peak for this study in absolute terms (~300–450-s), it was longer than those reported by Sousa et al. (2017; ~268-s). However, in the study of Libicz et al. (2005), the time spent above 95% of maximal ⩒O2 was ~22% of total time during IT planned with 8 × 100-m/30-s, which percentage is higher than the ~12–15% of total time observed for t@95% during both IT8x100 and IT4x200 in the present study. It is likely that the mode of performance (continuous vs. intermittent) and rest interval between 100-m bouts (30 vs. 15-s) accounts for the differences between these studies and this study. In spite of the fact that this study only analyzed the effect of velocity at 100% of MAV on ⩒O2 demand, the ⩒O2 elicited during IT100 and IT200 has satisfactory high similarity to those reported for continuous or intermittent efforts in swimming (Libicz et al., 2005) and other sports modalities (Billat, 2001; Buchheit and Laursen, 2013b).
Nevertheless, total distance swam and tLim are not correlated between IT protocols, as well as t@VT2, t@90%, and t@95%, which means that the IT protocols are independent in those measures. Also, the protocols did not correlate regarding the peak of ⩒O2 reached during the performance of each IT protocol, although temporal ⩒O2K and anaerobiosis stimulus (O2Def and [La−]peak) correlated with each other between protocols. Therefore, both the protocols are suitable to match middle-distance specificity regarding energetic contribution, which approaches ~25–26 ml·kg−1 and ~12 mmol·L−1 for swimming (200- and 400-m; Campos et al., 2017). Indeed, the values observed for O2Def and [La−]peak in this study are also quite similar to the values reported for IT performed at 100%v⩒O2max in running and cycling (~20–31 ml·kg−1; ~5–7 mmol·L−1; Billat, 2001; Scott, 2006; Panissa et al., 2018).
However, the IT protocols showed particular correlations with anaerobic variables as follows: (i) negative coefficients between [La−]peak vs. ⩒O2peak, MAV, tLim, and total distance for IT100; and (ii) positive coefficients between [La−]peak vs. t@90%, t@95%, and O2Def for IT200. These results suggest that swimmers with the highest ⩒O2peak and MAV had the tendency to perform IT100 with low [La−]peak and, hence, tolerate more distance at MAV, which seems to account for the influence of lower perceived exertion reported (less uncomfortable) by those swimmers. In contrast, swimmers with higher MAV had spent less time at or above 90%⩒O2peak during IT200, suggesting that the improvements of the time at high rates of ⩒O2 are related to high ⩒O2peak (wide range to ⩒O2 adjustments) and anaerobic capacity (enable to support high O2Def and [La−]peak), and, therefore, perceived as harder to perform. Hence, the performance during IT200 exhibits a typical inverse relationship between MAV and tLim at rates closer to ⩒O2max (Billat et al., 1996a). Additionally, the combination of long exercise bouts (>2 min), high intensity (100% MAV), and short rest intervals (≤30 s), as designed for IT200, are difficult to manage with no acidosis because of the reduction in phosphocreatine stores replacement and the increasing reliance on the anaerobic glycolytic contribution (Billat, 2001; Buchheit and Laursen, 2013b).
These dynamics between phosphocreatine nadir and greater glycogen utilization can be more relevant to explain tLim than microvascular blood flow and muscle oxygen extraction (temporal parameters of ⩒O2K). The assumption that tLim is related to ⩒O2K considers that fast VO2 response until the targeted muscle O2 requirements would reduce O2 deficit and metabolite accumulation and, therefore, attenuate phosphocreatine and glycogen stores depletion (Millet et al., 2003b; Bailey et al., 2009). For example, the increase in O2 availability induced by prior heavy exercise could be higher for subjects with a slower time constant, improving the time spent above 90%⩒O2max when performing at 100 or 105% of MAV (Millet et al., 2003b). For Bailey et al. (2009), the analysis of ⩒O2K has the potential to demonstrate the enhancement of exercise tolerance after interval training through a substantial increase in oxidative energy contribution and a reduced reliance on anaerobiosis stimulus. Despite these authors not finding a correlation between the magnitude of changes in tolerance with time constant of ⩒O2K and aerobic conditioning indexes, this could be further explored in future studies trying to gather information on what adjustments in ⩒O2K ensure aerobic capacity enhancement, while training with the protocols proposed in this study.
Additionally, the better explanation for the tLim in IT200 is that superior performance was obtained by swimmers with high ⩒O2peak, which would delay the attainment of maximal ⩒O2 and thus a tendency to reduce the accumulation of metabolites, whereas during IT100 the short exercise interval attenuates anaerobic stimulus with no impairment on ⩒O2 response. This is in agreement with Zuniga et al. (2011) who reported that short work intervals (30-s) compared with longer ones (3-min) may allow athletes to complete a longer IT session with greater metabolic demands and lower [La−] accumulations. The findings of this study might be useful for coaches to decide on the work interval (100- or 200-m bouts) that match the needs for aerobic power of the team. In this study, the inclusion of male and female swimmers is a limitation when considering differences in muscle mass and blood perfusion in the upper limbs (Koons et al., 2019), but how sex differed with regard to ⩒O2 increase and tolerance during different work:rest ratio interval training still remains to be answered. Furthermore, swimming with a snorkel and open turns may be a constraint to free swimming training.
Conclusion
This study concluded that both the IT protocols performed at MAV showed similar physiological and temporal responses whatever the distance (100 or 200-m) utilized for exercise bouts. Additionally, the protocols can be considered suitable to improve middle-distance swimming performance, since both stimulated the exertion level close to maximal ⩒O2, as well as moderate to high blood lactate concentrations and oxygen deficit, which is the finding to be highlighted for IT in swimming, as first demonstrated in this study. The fact that only the perceived exertion level differed between the IT protocols suggested that coaches should consider that nx100-m/15-s is perceived as less difficult to perform than nx200-m/30-s for the first 800-m when managing the best strategy to be implemented for aerobic power enhancement. Finally, the ⩒O2K parameters (time constant and amplitude) were not associated to tolerance in each IT protocol, suggesting that tLim during IT is not related to the parameters of ⩒O2K that characterize oxidative contribution and anaerobiosis reliance, but this analysis should be considered to evaluate the potential of aerobic power enhancement with IT.
Data Availability Statement
The raw data supporting the conclusions of this article are fully available without restriction when required to the authors.
Ethics Statement
This study was approved by the local University Ethical Committee of the Faculdade de Motricidade Humana (UL-CEFMH: 39/2015). Written informed consent to participate in this study was provided by the participants’ legal guardian/next of kin.
Author Contributions
TA, DP, and FA conceived and designed the study. TA, DP, ME, JR, AS, DM, and FS conducted the experiments and analyzed the data. TA, DP, ME, JR, and FA wrote the manuscript. All authors contributed to the article and approved the submitted version.
Funding
The Foundation for Science and Technology (Portugal, SFRH/BD/73022/2010), and Coordenação de Aperfeiçoamento de Pessoal de Nível Superior – Brazil (CAPES – Finance Code 001), participated with a fellowship award. The São Paulo Research Foundation (FAPESP, PROCESS 2016/04544-3) participated with partial financial support for the study development. The Foundation for Science and Technology, I.P., (Grant/Award Number UIDB/04748/2020) participated with partial financial support for the study development.
Conflict of Interest
The authors declare that the research was conducted in the absence of any commercial or financial relationships that could be construed as a potential conflict of interest.
Acknowledgments
The authors would like to express their gratitude to the swimmers for their time and effort, and to the swimming teams for making both their infrastructures and staff available for the study.
Supplementary Material
The Supplementary Material for this article can be found online at: https://www.frontiersin.org/articles/10.3389/fphys.2021.662029/full#supplementary-material
Supplementary Material | Transportation of gas analyzer system and sampling unit at the side of the pool.
References
Almeida, T. A. F., Pessôa Filho, D. M., Espada, M. A. C., Reis, J. F., Simionato, A. R., Siqueira, L. O. C., et al. (2020). ⩒O2 kinetics and energy contribution in simulated maximal performance during short and middle distance-trials in swimming. Eur. J. Appl. Physiol. 120, 1097–1109. doi: 10.1007/s00421-020-04348-y
Bailey, S. J., Wilkerson, D. P., DiMenna, F. J., and Jones, A. M. (2009). Influence of repeated sprint training on pulmonary O2 uptake and muscle deoxygenation kinetics in humans. J. Appl. Physiol. 106, 1875–1887. doi: 10.1152/japplphysiol.00144.2009
Baldari, C., Fernandes, R. J., Meucci, M., Ribeiro, J., Vilas-Boas, J. P., and Guidetti, L. (2013). Is the new aquatrainer® snorkel valid for ⩒O2 assessment in swimming? Int. J. Sports Med. 34, 336–344. doi: 10.1055/s-0032-1321804
Bentley, D. J., Roels, B., Hellard, P., Fauquet, C., Libicz, S., and Millet, G. P. (2005). Physiological responses during submaximal interval swimming training: effects of interval duration. J. Sci. Med. Sport 8, 392–402. doi: 10.1016/S1440-2440(05)80054-4
Billat, L. V. (2001). Interval training for performance: a scientific and empirical practice. Special recommendations for middle- and long-distance running. Part I: aerobic interval training. Sports Med. 31, 13–31. doi: 10.2165/00007256-200131010-00002
Billat, V., Faina, M., Sardella, F., Marini, C., Fanton, F., Lupo, S., et al. (1996a). A comparison of time to exhaustion at ⩒O2max in elite cyclists, kayak paddlers, swimmers and runners. Ergonomics 39, 267–277. doi: 10.1080/00140139608964457
Billat, V., Beillot, J., Jan, J., Rochcongar, P., and Carre, F. (1996b). Gender effect on the relationship of time limit at 100% ⩒O2max with other bioenergetic characteristics. Med. Sci. Sports Exerc. 28, 1049–1055. doi: 10.1097/00005768-199608000-00016
Billat, L. V., Blondel, N., and Berthoin, S. (1999). Determination of the velocity associated with the longest time to exhaustion at maximal oxygen uptake. Eur. J. Appl. Physiol. Occup. Physiol. 80, 159–161. doi: 10.1007/s004210050573
Billat, L. V., and Koralsztein, J. P. (1996). Significance of the velocity at ⩒O2max and time to exhaustion at this velocity. Sports Med. 22, 90–108. doi: 10.2165/00007256-199622020-00004
Billat, V. L., Slawinski, J., Bocquet, V., Demarle, A., Lafitte, L., Chassaing, P., et al. (2000). Intermittent runs at the velocity associated with maximal oxygen uptake enables subjects to remain at maximal oxygen uptake for a longer time than intense but submaximal runs. Eur. J. Appl. Physiol. 81, 188–196. doi: 10.1007/s004210050029
Borg, G. (1990). Psychophysical scaling with applications in physical work and the perception of exertion. Scand. J. Work Environ. Health 16, 55–58. doi: 10.5271/sjweh.1815
Buchheit, M., and Laursen, P. B. (2013a). High-intensity interval training, solutions to the programming puzzle part I: cardiopulmonary emphasis. Sports Med. 43, 313–338. doi: 10.1007/s40279-013-0029-x
Buchheit, M., and Laursen, P. B. (2013b). High-intensity interval training, solutions to the programming puzzle part II: anaerobic energy, neuromuscular load and practical applications. Sports Med. 43, 927–954. doi: 10.1007/s40279-013-0066-5
Campos, E. Z., Kalva-Filho, C. A., Gobbi, R. B., Barbieri, R. A., Almeida, N. P., and Papoti, M. (2017). Anaerobic contribution determined in swimming distances: relation with performance. Front. Physiol. 8:755. doi: 10.3389/fphys.2017.00755
Carter, H., Pringle, J. S. M., Barstow, T. J., and Doust, J. H. (2006). Oxygen uptake kinetics during supra VO2max treadmill running in humans. Int. J. Sports Med. 27, 149–157. doi: 10.1055/s-2005-873076
Dalamitros, A. A., Zafeiridis, A. S., Toubekis, A. G., Tsalis, G. A., Pelarigo, J. G., Manou, V., et al. (2016). Effects of short-interval and long-interval swimming protocols on performance, aerobic adaptations, and technical parameters. J. Strength Cond. Res. 30, 2871–2879. doi: 10.1519/JSC.0000000000001369
Espada, M. C., Alves, F. B., Curto, D., Ferreira, C. C., Santos, F. J., Pessôa Filho, D. M., et al. (2021). Can an incremental step test be used for maximal lactate steady state determination in swimming? Clues for practice. Int. J. Environ. Res. Public Health 18:477. doi: 10.3390/ijerph18020477
Espada, M. C., Reis, J. F., Almeida, T. F., Bruno, P. M., Vleck, V. E., and Alves, F. B. (2015). Ventilatory and physiological responses in swimmers below and above their maximal lactate steady state. J. Strength Cond. Res. 29, 2836–2843. doi: 10.1519/JSC.0000000000000504
Ferguson, C. J. (2009). An effect size primer: a guide for clinicians and researchers. Prof. Psychol. Res. Pract. 40, 532–538. doi: 10.1037/a0015808
Fernandes, R. J., Billat, V. L., Cruz, A. C., Colaço, P. J., Cardoso, C. S., and Vilas-Boas, J. P. (2006). Does net energy cost of swimming affect time to exhaustion at the individual’s maximal oxygen consumption velocity? J. Sports Med. Phys. Fitness 46, 373–380.
Harriss, D. J., Macsween, A., and Atkinson, G. (2017). Standards for ethics in sport and exercise science research: 2018 update. Int. J. Sports Med. 38, 1126–1131. doi: 10.1055/s-0043-124001
Jones, A. M., and Burnley, M. (2009). Oxygen uptake kinetics: an underappreciated determinant of exercise performance. Int. J. Sports Physiol. Perform. 4, 524–532. doi: 10.1123/ijspp.4.4.524
Koons, N. J., Suresh, M. R., Schlotman, T. E., and Convertino, V. A. (2019). Interrelationship between sex, age, blood volume, and ⩒O2max. Aerosp. Med. Hum. Perform. 90, 362–368. doi: 10.3357/AMHP.5255.2019
Libicz, S., Roels, B., and Millet, G. P. (2005). ⩒O2 responses to intermittent swimming sets at velocity associated with ⩒O2max. Can. J. Appl. Physiol. 30, 543–553. doi: 10.1139/h05-140
Millet, G. P., Candau, R., Fattori, P., Bignet, F., and Varray, A. (2003a). ⩒O2 responses to different intermittent runs at velocity associated with ⩒O2max. Can. J. Appl. Physiol. 28, 410–423. doi: 10.1139/h03-030
Millet, G. P., Libicz, S., Borrani, F., Fattori, P., Bignet, F., and Candau, R. (2003b). Effects of increased intensity of intermittent training in runners with differing ⩒O2 kinetics. Eur. J. Appl. Physiol. 90, 50–57. doi: 10.1007/s00421-003-0844-0
Murgatroyd, S. R., Ferguson, C., Ward, S. A., Whipp, B. J., and Rossiter, H. B. (2011). Pulmonary O2 uptake kinetics as a determinant of high-intensity exercise tolerance in humans. J. Appl. Physiol. 110, 1598–1606. doi: 10.1152/japplphysiol.01092.2010
Panissa, V. L., Fukuda, D. H., Caldeira, R. S., Gerosa-Neto, J., Lira, F. S., Zagatto, A. M., et al. (2018). Is oxygen uptake measurement enough to estimate energy expenditure during high-intensity intermittent exercise? Quantification of anaerobic contribution by different methods. Front. Physiol. 9:868. doi: 10.3389/fphys.2018.00868
Pessôa Filho, D. M., Alves, F. B., Reis, J. F., Greco, C. C., and Denadai, B. S. (2012). ⩒O2 kinetics during heavy and severe exercise in swimming. Int. J. Sports Med. 33, 744–748. doi: 10.1055/s-0031-1299753
Reis, J. F., Alves, F. B., Bruno, P. M., Vleck, V., and Millet, G. P. (2012a). Effects of aerobic fitness on oxygen uptake kinetics in heavy intensity swimming. Eur. J. Appl. Physiol. 112, 1689–1697. doi: 10.1007/s00421-011-2126-6
Reis, J. F., Alves, F. B., Bruno, P. M., Vleck, V., and Millet, G. P. (2012b). Oxygen uptake kinetics and middle distance swimming performance. J. Sci. Med. Sport 15, 58–63. doi: 10.1016/j.jsams.2011.05.012
Reis, J. F., Millet, G. P., Bruno, P. M., Vleck, V., and Alves, F. B. (2017). Sex and Exercise Intensity Do Not Influence Oxygen Uptake Kinetics in Submaximal Swimming. Front. Physiol. 8. doi: 10.3389/fphys.2017.00072
Reis, J. F., Millet, G. P., Malatesta, D., Roles, B., Borrani, F., Vleck, V. E., et al. (2010). Are oxygen uptake kinetics modified when using a respiratory snorkel? Int. J. Sports Physiol. Perform. 5, 292–300. doi: 10.1123/ijspp.5.3.292
Rodríguez, F. A., Keskinen, K. L., Malvela, M. T., and Keskinen, O. P. (2003). “Oxygen uptake kinetics during free swimming: A pilot study,” in Biomechanics and Medicine in Swimming IX. ed. J. C. Chatard (Saint-Étienne: Publications de l’Université de Saint-Étienne), 379–384.
Rønnestad, B. R., and Hansen, J. (2016). Optimizing interval training at power output associated with peak oxygen uptake in well-trained cyclists. J. Strength Cond. Res. 30, 999–1006. doi: 10.1519/JSC.0b013e3182a73e8a
Scott, C. B. (2006). Estimating energy expenditure for brief bouts of exercise with acute recovery. Appl. Physiol. Nutr. Metab. 31, 144–149. doi: 10.1139/h05-013
Sheskin, D. J. (2011). Handbook of Parametric and Nonparametric Statistical Procedures. Boca Raton: Chapman and Hall/CRC, 1886.
Sousa, A., de Jesus, K., Figueiredo, P., Vilas-Boas, J. P., and Fernandes, R. J. (2013). Oxygen uptake kinetics at moderate and extreme swimming intensities. Rev. Bras. Med. Esporte 19, 186–190. doi: 10.1590/S1517-86922013000300008
Sousa, A. C., Fernandes, R. J., Vilas-Boas, J. P., and Figueiredo, P. (2018). High-intensity interval training in different exercise modes: lessons from time to exhaustion. Int. J. Sports Med. 39, 668–673. doi: 10.1055/a-0631-2682
Sousa, A., Figueiredo, P., Zamparo, P., Pyne, D. B., Vilas-Boas, J. P., and Fernandes, R. J. (2015). Exercise modality effect on bioenergetical performance at ⩒O2max intensity. Med. Sci. Sports Exerc. 47, 1705–1713. doi: 10.1249/MSS.0000000000000580
Sousa, A., Vilas-Boas, J. P., Fernandes, R. J., and Figueiredo, P. (2017). ⩒O2 at maximal and supramaximal intensities: lessons to high-intensity interval training in swimming. Int. J. Sports Physiol. Perform. 12, 872–877. doi: 10.1123/ijspp.2016-0475
Spencer, M. R., and Gastin, P. B. (2001). Energy system contribution during 200- to 1500-m running in highly trained athletes. Med. Sci. Sports Exerc. 33, 157–162. doi: 10.1097/00005768-200101000-00024
Wen, D., Utesch, T., Wu, J., Robertson, S., Liu, J., and Hu, G. (2019). Effects of different protocols of high intensity interval training for ⩒O2max improvements in adults: a meta-analysis of randomised controlled trials. J. Sci. Med. Sport 22, 941–947. doi: 10.1016/j.jsams.2019.01.013
Whipp, B. J., Ward, S. A., and Rossiter, H. B. (2005). Pulmonary O2 uptake during exercise: conflating muscular and cardiovascular responses. Med. Sci. Sports Exerc. 37, 1574–1585. doi: 10.1249/01.mss.0000177476.63356.22
Keywords: interval training, oxygen uptake kinetics, work-interval, performance, swimming
Citation: Almeida TAF, Pessôa Filho DM, Espada MC, Reis JF, Sancassani A, Massini DA, Santos FJ and Alves FB (2021) Physiological Responses During High-Intensity Interval Training in Young Swimmers. Front. Physiol. 12:662029. doi: 10.3389/fphys.2021.662029
Edited by:
François Billaut, Laval University, CanadaReviewed by:
Argyris G. Toubekis, National and Kapodistrian University of Athens, GreeceHugo A. Kerhervé, University of Rennes 2 – Upper Brittany, France
Copyright © 2021 Almeida, Pessôa Filho, Espada, Reis, Sancassani, Massini, Santos and Alves. This is an open-access article distributed under the terms of the Creative Commons Attribution License (CC BY). The use, distribution or reproduction in other forums is permitted, provided the original author(s) and the copyright owner(s) are credited and that the original publication in this journal is cited, in accordance with accepted academic practice. No use, distribution or reproduction is permitted which does not comply with these terms.
*Correspondence: Dalton Müller Pessôa Filho, ZGFsdG9uLnBlc3NvYS1maWxob0B1bmVzcC5icg==