Corrigendum: Lactate and Myocardiac Energy Metabolism
- 1Department of General Surgery, Qilu Hospital of Shandong University, Jinan, China
- 2Department of Colorectal and Anal Surgery, Feicheng Hospital Affiliated to Shandong First Medical University, Feicheng, China
- 3Department of General Surgery, The First Affiliated Hospital of Shandong First Medical University, Jinan, China
- 4Adelaide Medical School and Centre of Research Excellence in Translating Nutritional Science to Good Health, The University of Adelaide, Adelaide, SA, Australia
- 5Endocrine and Metabolic Unit, Royal Adelaide Hospital, Adelaide, SA, Australia
The myocardium is capable of utilizing different energy substrates, which is referred to as “metabolic flexibility.” This process assures ATP production from fatty acids, glucose, lactate, amino acids, and ketones, in the face of varying metabolic contexts. In the normal physiological state, the oxidation of fatty acids contributes to approximately 60% of energy required, and the oxidation of other substrates provides the rest. The accumulation of lactate in ischemic and hypoxic tissues has traditionally be considered as a by-product, and of little utility. However, recent evidence suggests that lactate may represent an important fuel for the myocardium during exercise or myocadiac stress. This new paradigm drives increasing interest in understanding its role in cardiac metabolism under both physiological and pathological conditions. In recent years, blood lactate has been regarded as a signal of stress in cardiac disease, linking to prognosis in patients with myocardial ischemia or heart failure. In this review, we discuss the importance of lactate as an energy source and its relevance to the progression and management of heart diseases.
Introduction
The heart is an efficient bio-pump of high energy demand. Adenosine triphosphate (ATP) is the direct source of energy that supports the contraction and relaxation of the myocardium. ATP can be derived through the processes of oxidation and fermentation, during which the intermediate pyruvate is transformed into lactate. The latter has long been considered to be a by-product of glucose metabolism. Over the last several decades, amounting research has demonstrated that lactate is a major energy substrate for skeletal muscle, heart and brain (Gertz et al., 1988; Bergman et al., 1999b; Glenn et al., 2015), as well as a main gluconeogenesis precursor (Bergman et al., 2000; Meyer et al., 2002a,b; Emhoff et al., 2013b) and a signaling molecule (Hashimoto et al., 2007). Increased levels of blood lactate are also associated with poor outcomes in critical systemic diseases, including severe trauma, hypoxemia, septic shock and so forth (Cerovic et al., 2003; Nguyen et al., 2004; Khosravani et al., 2009). However, the biochemical and clinical significance of lactate within the field of myocadiac metabolism remains under-appreciated, reflecting an incomplete understanding of its production, transport, metabolism and biological functions (Garcia-Alvarez et al., 2014). Herein, we discuss myocardial energy metabolism, with an emphasis on the role of lactate metabolism and its relevance to the progression and management of heart diseases, including acute myocardial ischemia, and heart failure, and on diabetic state.
Historical View on Myocardial Energy Metabolism
The initial understanding of cardiac function was first described by a Greek philosopher Aristotle. According to the latter, the heart produces necessary heat to maintain life, and cessation of the heartbeat means absence of life (Beloukas et al., 2013). This philosophy led to the recognition of energy metabolism being central to cardiac function and the capacity of cardiomyocyte to utilize various substrates to provide energy.
Oxygen was identified as a basic element of cardiac metabolism and function as early as in the 18th century (Gh, 2006). Subsequently, carbohydrate was described as an energy substrate during cardiac contraction (Locke and Rosenheim, 1907), but contributed no more than a third to the total cardiac energy demand (Evans, 1914). This observation stimulated the search of other energy substrates for myocadiac energy metabolism, which had been advanced rapidly since the introduction of coronary sinus catheterization technique (Bing et al., 1947). In this way, the oxygen extraction ratios can be computed to reflect the aerobic catabolism of different substrates. In 1954, Bing and his colleagues assessed the utilization of glucose, lactate, pyruvate, fatty acids, ketones and amino acids of the heart in vivo (Bing et al., 1947; Bing, 1954), and showed that fatty acids were the major energy substrate of the human heart, accounting for 67% of the total usage of oxygen. Surprisingly, lactate, formerly known as a metabolic waste product and fatigue agent, contributed 16.5% to the total usage of oxygen, in a comparable degree to glucose (17.9%) (Bing, 1954).
Subsequent studies concentrated on energy substrate usage of the heart under different circumstances. Keul et al. (1965a, b) found that, in humans during moderate intensity exercise, the contribution of fatty acids fell from 34 to 21%, while the contribution of lactate increased from 29 to 62%. In anesthetized dogs, the contribution of lactate to cardiac oxidative energy production increased to 87% when the arterial lactate concentration exceeded 4.5 mmol/L (Drake et al., 1980). These studies suggest that lactate competes with fatty acids for cardiac oxygen consumption. In recent years, the emergence of dual carbon-labeled carbohydrate isotope technique has allowed myocardial substrate utilization to be quantified precisely in humans. Based on this technique, it was observed that the myocardial isotopic lactate uptake increased from 34.9 μmol/min at rest to 120.4 μmol/min at 5 min of moderate intensity exercise in healthy male subjects (Gertz et al., 1988). This result further confirmed lactate as an important energy substrate for the heart, particularly under stress. It is now widely accepted that the heart uses various energy substrates to generate ATP, which is key to the maintenance of normal cardiac function under different circumstances.
Myocardial Lactate Metabolism
As discussed, in addition to fatty acids, carbon sources, including glucose and lactate, are important energy substrates of the myocardium. Traditionally, it has been thought that full oxidation of glucose to CO2 provides most cells energy in human body, and lactate is only a product of incomplete oxidation in the face of urgent energy demands. However, if this was the case, whole-body glucose consumption would dominate over lactate consumption, and lactate production would be equivalent to its clearance (as a precursor of hepatic and renal gluconeogenesis). Rather, lactate has a circulatory turnover flux approximately twice that of glucose on a molar basis during fasting (Dunn et al., 1976; Katz et al., 1981; Stanley et al., 1986; Wolfe, 1990). Modern studies have proved that lactate could be produced continuously under aerobic conditions, and be used as an important energy source for the heart (Brooks, 2018). Accordingly, “lactate shuttle theory” was proposed to describe the transport and function of lactate within the body: lactate could act as the vehicle linking glycolysis and oxidative metabolism, and the linkages between lactate “producer” and “consumer” exist within and among cells, tissues, and organs (Brooks, 2018). In this section we will discuss the development of lactate shuttle theory in the context of myocardial energy metabolism.
Tissue-Tissue and Cell-Cell Lactate Transport
Benefiting from the development of differential arterio-venous metabolite analysis and radiotracer techniques, the whole process of circulating lactate production and disposal was characterized in several animal studies (Brooks et al., 1984). In rats, intravenous injection of 14C-lactate resulted in exhaled breath gas containing 14CO2, providing early evidence for the concept of circulating lactate in contributing to energy metabolism (Brooks et al., 1973; Brooks and Gaesser, 1980). To better explore the production and disposal of circulating lactate across diverse physiological states, Donovan CM and Brooks GA subsequently recorded rates of lactate disposal in rodent models both at rest and during exercise with radiotracer techniques (Brooks and White, 1978; Brooks and Donovan, 1983; Donovan and Brooks, 1983). By measuring the quantity and activity of O2, CO2, circulating carbon sources (glucose and lactate) and other metabolites during resting and exercise (Brooks et al., 1977), the authors observed in rats that the rates of lactate flux in circulating peripheral blood were unanticipatedly high in the resting state, although to a lesser extent when compared to glucose flux. However, during exercise, the rates of lactate flux in circulating blood were observed to increase above those of glucose flux.
These phenomena are likely to have reflected the breakdown of glycogen and an increase in the production of lactate during exercise. However, the latter was not necessarily accompanied by increased blood lactate concentrations (Brooks and Donovan, 1983; Donovan and Brooks, 1983), which can be attributed to the markedly increased lactate metabolic clearance rate during exercise. Hence, relatively stable blood lactate concentration during exercise can be explained by a greater lactate metabolic clearance rate due to increased lactate oxidation and gluconeogenesis. Such adaption can avoid metabolic acidosis during exercise. As technology improves, non-invasive and non-radiating methods are available for evaluation of glucose and lactate metabolism in the human body, and the aforementioned effects of exercise on glucose and lactate flux rates have also been consistently replicated (Stanley et al., 1985; Mazzeo et al., 1986; Bergman et al., 1999a,b, 2000; Emhoff et al., 2013a,b). Fluctuations of lactate in the blood indicate that lactate transport between different tissues via the circulatory system. However, the main producers and consumers of blood lactate underlying these changes remain unknown.
In the following research, investigators attempted to assess tissue specificity of lactate metabolism (Katz et al., 1981; Stanley et al., 1986; Gertz et al., 1988; Bergman et al., 1999a,b). Two earlier studies in the 1970s found that lactate concentrations in red skeletal muscles in motion were lower than white skeletal muscles and their blood supplies (Mole et al., 1973; Hooker and Baldwin, 1979). Although these phenomena seemed unexplained at that time, this provided early evidence to suggest working skeletal muscles as a source of the circulating lactate. In 1988, the utilization of lactate released from working muscles by heart as a carbon source was observed (Gertz et al., 1988), which marked an important milestone for research in lactate metabolism. Subsequently, lactate transport between white and red skeletal muscles (Stanley et al., 1986; Bergman et al., 1999a), and between working skeletal muscles and heart were gradually identified (Katz et al., 1981; Gertz et al., 1988; Bergman et al., 2009). It is now well-documented that the beating heart takes up, and oxidizes lactate as a consumer in the process of the lactate transport.
The potential for lactate to transport across neighboring cells and tissues has been reported over two decades. It was initially found to occur in astrocytes-neurons in brain and fibroblasts-cancer cells in tumors (Lisanti et al., 2013; Magistretti and Allaman, 2015). Recently, mouse cardiomyocytes and fibroblasts co-culture models have shown the fibroblasts-cardiomyocytes lactate transport (Wisniewski et al., 2015; Gizak et al., 2020), supporting the concept that cardiomyocytes and fibroblasts form metabolic syncytia to share energy substrates, including lactate, and exchange molecular signals. Neighboring lactate transport improves energy metabolism efficiency and orchestrates substrate utilization in tissues. Such a metabolic architecture enables metabolic adaptability and plasticity. Nevertheless, accurate mechanisms of lactate transport between cardiomyocytes and fibroblasts are still unclear.
Transmembrane and Intracellular Lactate Transport
The increasing recognition of tissue-tissue and cell-cell lactate transport has provided a strong impetus to understand the metabolic fate of lactate transport and utilization within the cell. The discovery of membrane lactate transporters provided a plausible explanation of the transmembrane lactate transport. Lactate oxidation rates comply with Michaelis-Menten kinetics, suggesting that cellular uptake and release of lactate are facilitated by membrane transporters. The membrane lactate transporter was first observed on rat sarcolemmal vesicles in 1990 (Roth and Brooks, 1990), and subsequently named as monocarboxylate transport (MCT) (Garcia et al., 1994).
Hitherto, various isoforms of MCT have been identified to account for intracellular lactate transport, but how is lactate utilized in the cell, and the site at which the intracellular lactate utilization takes place remains debated. Previous studies on humans and various mammals demonstrated that intracellular lactate metabolism consumes oxygen (Depocas et al., 1969; Donovan and Brooks, 1983; Stanley et al., 1985; Mazzeo et al., 1986). Lactate oxidation for energy supply especially prominent when heart or muscle is in load condition (Gertz et al., 1981, 1988; Stanley et al., 1986; Bergman et al., 1999b). With this in mind, issues arose about exactly where lactate oxidation happened within a working cardiomyocyte. Given that lactate dehydrogenase (LDH), a key enzyme to catalyze the inter-conversion of lactate and pyruvate, is widely present in the cytosol, lactate oxidation was first considered to take place in the cytosol. However, the results from some studies were inconsistent (Laughlin et al., 1993; Chatham et al., 2001). In working muscles-beating heart lactate syncytium, increased rates of lactate flux were found to accompany by augmented blood flow and oxygen consumption. In addition, when 13C-pyruvate was injected into myocardial blood circulation, the peaks of cytosolic 13C-pyruvate and 13C-lactate were observed. However, when 13C-lactate was injected directly, 13C-pyruvate was not detected in the cytosol (Chatham et al., 2001).
If not in the cytosol, where does intracellular lactate oxidation occur? Another candidate location for intracellular lactate oxidation is the mitochondria, the pivotal organelle for energy metabolism. Lactate oxidation was observed in several mitochondrial preparations (Kline et al., 1986; Brandt et al., 1987; De Bari et al., 2004; Passarella et al., 2014), of which mitochondrial preparations from human skeletal muscles provided the strongest evidence (Jacobs et al., 2013). Lactate oxidative capacity of muscle mitochondria was subsequently confirmed by magnetic resonance spectroscopy imaging (MRSI) (Park et al., 2015; Chen et al., 2016). However, some studies using the same experimental settings showed inconsistent results, challenging the lactate oxidative capacity in the mitochondria (Popinigis et al., 1991; Sahlin et al., 2002). This discrepancy might be related to the separation process of mitochondria (Kirkwood et al., 1986; Glancy et al., 2017), which is highly dependent on the isolated system and susceptible to be contamination and/or disturbance. Therefore, confirmation by more reliable experiments is still required due to its conceptual importance. More recently, identification of the mitochondrial lactate oxidation complex (mLOC) with techniques of organelle purification and magnetic resonance spectroscopy imaging has provided solid evidence on mitochondrial transmembrane transport of lactate mitochondria as a key site of intracellular lactate oxidation (Figure 1; Park et al., 2015; Chen et al., 2016). Components of the mLOC include the MCT1, CD147, LDH, terminal electron transport chain element cytochrome oxidase (COX), pyruvate dehydrogenase (PDH), Krebs cycle related-enzymes, and mitochondrial respiratory chain (De Bari et al., 2004; Hashimoto et al., 2006, 2008; Atlante et al., 2007; Sonveaux et al., 2008; Passarella et al., 2014). Mitochondrial MCT1 is located in the inner membrane and is responsible for mitochondrial transmembrane transport of lactate, and CD147 is an indispensable chaperone protein of MCT1 (Park et al., 2015; Chen et al., 2016). Mitochondrial LDH was initially discovered in the sperm, but is now revealed to also exist in the liver, kidney and heart (Kline et al., 1986; Brandt et al., 1987; Tempia et al., 1988). Lactate oxidative capacity is strongly associated with the expression of mitochondrial LDH (Tempia et al., 1988), and is also associated with COX (Hashimoto et al., 2006). Finally, lactate is transformed to the end product (CO2) by PDH, Krebs cycle related-enzymes, and mitochondrial respiratory chain catalysis. Future research in order to better define the mLOC is eagerly anticipated.
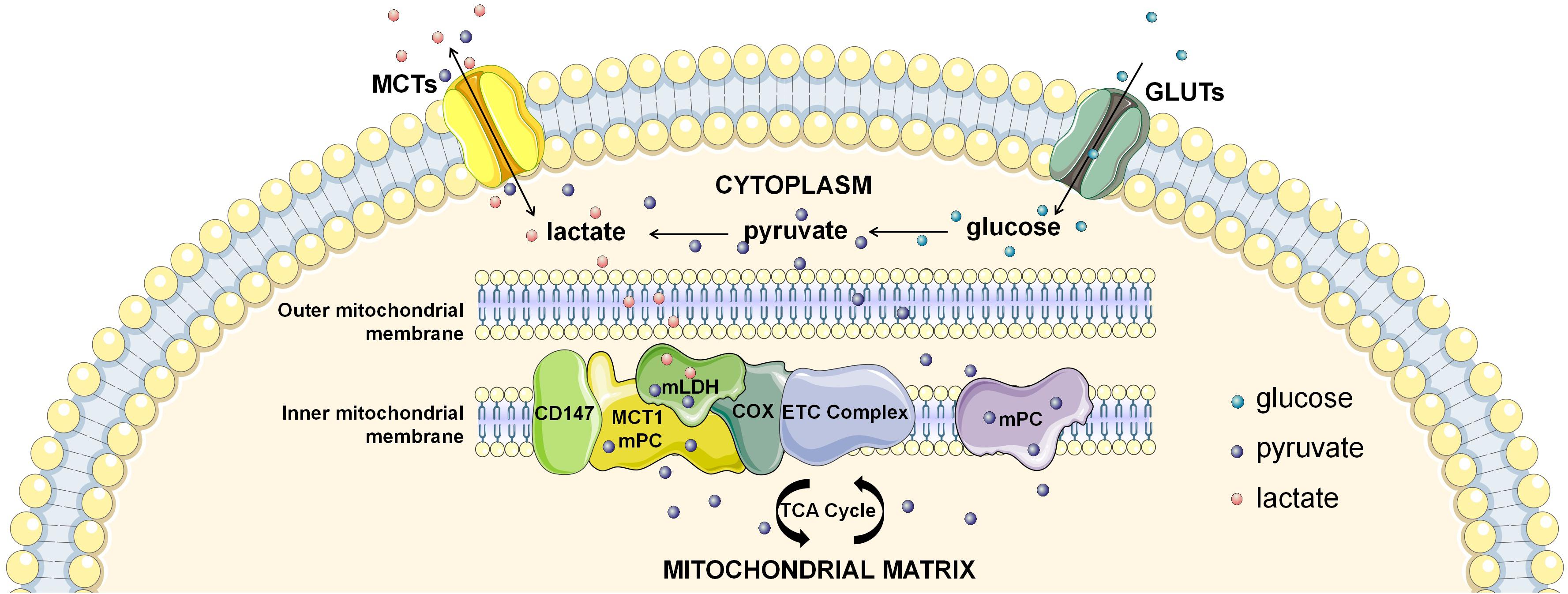
Figure 1. The intracellular lactate shuttle. Both extracellular uptake and glycolytic production make up the cytosolic lactate pool. Intracellular lactate is shuttled from the cytoplasm to mitochondria for subsequent oxidization, facilitated by the mitochondrial lactate oxidation complex (mLOC). Monocarboxylate transporter 1 (MCT1) is inserted into the inner mitochondrial membrane, and CD147 is an indispensable chaperone protein of MCT1. Mitochondrial LDH (mLDH) is distributed on the surface of the inner mitochondrial membrane, and oxidizes lactate to pyruvate. In addition, terminal electron transport chain element cytochrome oxidase (COX) is responsible for the endergonic lactate oxidation. Mitochondrial pyruvate carrier (mPC) is distributed in the inner mitochondrial membrane, which is responsible for the mitochondrial transmembrane transport of pyruvate.
Another issue regarding intracellular lactate metabolism is why cells generate and consume lactate at the same time. For example, cardiomyocytes release glycolytically derived lactate and take up extracellular lactate simultaneously (Goodwin et al., 1998; Bartelds et al., 1999; Emhoff et al., 2013a). Although some studies indicated that glycolytically derived pyruvate is preferentially shifted to lactate rather than to acetyl-CoA, pyruvate derived from exogenous lactate tends to form acetyl-CoA (Barnard et al., 1971; Chatham et al., 1999). To account for these observations, one plausible explanation is that pathways of glycolytic lactate production and oxidation of exogenous lactate are functionally separate in the cardiomyocyte, i.e., compartmentation of intracellular lactate metabolism (Barnard et al., 1971; Mowbray and Ottaway, 1973; Sinniah, 1978; Lewandowski, 1992). LDH reversibly catalyzed the conversion of pyruvate to lactate or lactate to pyruvate. Given that the equilibrium constant for LDH is far in the direction of lactate and the change in free energy is large, glycolytically derived pyruvate can easily shift to lactate, rather than enter the TCA cycle (Barnard et al., 1971; Rabinowitz and Enerback, 2020). In addition, myocardial mitochondrial abundance is greatest at the subsarcolemmal surface (Palmer et al., 1977). Therefore, when exogenous lactate enters cardiomyocytes, it is expected to be readily transported into the mitochondria and enters the TCA cycle. This explanation reconciles in vivo and in vitro observations relating to myocardial lactate generation and consumption (Wisneski et al., 1985; Gertz et al., 1988; Mazer et al., 1990; Goodwin et al., 1998; Bartelds et al., 1999), and is in keeping with the lactate shuttle theory (Brooks et al., 1999; Brooks, 2000). It is recently proposed a hypothesis that characteristics of intracellular lactate metabolism enables the uncoupling of mitochondrial energy generation from glycolysis, and confers cells with increased metabolic flexibility (Rabinowitz and Enerback, 2020). Future studies of intracellular lactate metabolism are needed to investigate the regulation of lactate uptake and efflux and assess the exact value of the compartmentation of lactate metabolism.
Lactate Metabolism in Myocardial Diseases
In order to be compatible with complicated and volatile physiological and pathological states, the heart has evolved into an “omnivore” to consume different energy substrates in varying proportions. In myocardial diseases, there are universal disorders in energy substrate utilization and metabolic flexibility. While ATP production in the myocardium is often impaired in different pathological states, there is less consensus as to what actual switches in energy substrate preference occur. Traditionally, lactate has been regarded as an undesirable metabolite and has, accordingly, been used to as a biomarker of myocardial injury. However, later studies suggests that lactate is of greater relevance than other metabolic substrates, to the maintenance of metabolic flexibility in the metabolically unhealthy heart (Hutter et al., 1984; Lopaschuk et al., 2010; Garcia-Alvarez et al., 2014), raising a fundamental question: is lactate in heart disease a savior or a devil? This section will discuss lactate oxidation in the context of acute myocardial ischemia, heart failure and diabetes.
Acute Myocardial Ischemia
Acute myocardial ischemia is a common feature of acute critical cardiac events including acute coronary syndrome, cardiogenic shock, and cardiac arrest. There is usually a clear etiology and the course of disease is often brief. Sudden heart attacks lead to drastic changes of cardiac metabolic environment within a short period of time. Myocardium death is mainly caused by deprivation of oxygen and energy substrates in acute myocardial ischemia (Ong et al., 2010). While fatty acids remain the main energy substrate in the ischemic myocardium (Stanley, 2001), respiration and oxidative phosphorylation functions of mitochondria are markedly impaired during ischemia. There is evidence that the number of mitochondria is augmented in acute myocardial ischemia (Ide et al., 2001), which might represent a compensatory response for acute ischemia and hypoxia. It has been proved that the most striking metabolic switch event in the ischemic myocardium relates to increased glycolysis (Trueblood et al., 2000; Askenasy, 2001; Russell et al., 2004; Carvajal et al., 2007). However, the raw materials for glycolysis in acute myocardial ischemia are in association with the extent of ischemia. During low-flow conditions, glucose uptake and lactate release may be maintained in ischemic myocardium, and increased glycolysis depends on higher influx of glucose and increased activity of glycolytic enzymes (King and Opie, 1998a,b; Horman et al., 2012; Herzig and Shaw, 2018). When blood flow is completely interrupted, glucose is replaced by glycogenolysis (Smeele et al., 2011; Wu et al., 2011). Despite profound differences in the sources of glycolytic substrates, activating or prolonging glycolysis has been shown to be beneficial for myocardial salvage in both conditions (Vanoverschelde et al., 1994; Lochner et al., 1996; Fiolet and Baartscheer, 2000; Trueblood et al., 2000; Askenasy, 2001; Russell et al., 2004; Carvajal et al., 2007; Kim et al., 2011; Smeele et al., 2011; Wu et al., 2011; Timmermans et al., 2014; Vanoverschelde et al., 1994; Lochner et al., 1996; King and Opie, 1998a,b; Fiolet and Baartscheer, 2000; Trueblood et al., 2000; Askenasy, 2001; Russell et al., 2004; Carvajal et al., 2007; Kim et al., 2011; Smeele et al., 2011; Wu et al., 2011; Horman et al., 2012; Timmermans et al., 2014; Herzig and Shaw, 2018).
The accumulation of lactate in the ischemic myocardium provides an important source of energy; both uptake and use of lactate by the myocardium increase significantly in the acute ischemic heart (Hutter et al., 1984; Lopaschuk et al., 2010). In animal shock models, lactate deprivation is related to increased mortality, while exogenous lactate infusion is associated with myocardial salvage (Barbee et al., 2000; Revelly et al., 2005; Levy et al., 2007).
Several observational studies have demonstrated that hyperlactatemia is associated with a poor prognosis in patients with acute coronary syndrome (Lazzeri et al., 2010; Vermeulen et al., 2010; Kossaify et al., 2013). In patients who received percutaneous coronary intervention, plasma lactate measured after percutaneous coronary intervention is a reliable predictor for mortality (Lazzeri et al., 2009; Valente et al., 2012). In cardiogenic shock, the prognostic value of lactate has been controversial. Some studies identified increased lactate as an independently prognostic factor (Weil and Afifi, 1970; Chiolero et al., 2000; Koreny et al., 2002; Attana et al., 2013b), however, others did not (Geppert et al., 2006). Moreover, elevated lactate levels are positively associated with mortality in cardiac arrest (Donnino et al., 2007; Nolan et al., 2008; Cocchi et al., 2011; Andersen et al., 2013). Accordingly, a large body of research is in support of lactate as a prognostic factor in acute myocardial ischemia. It is regrettable that there is yet no consensus about the cut-off values for lactate that would be associated with worse outcome. In some recent observational studies, serial measurements of lactate have been shown to be more efficient than a single measurement for outcome prediction in acute myocardial ischemia (Attana et al., 2013a).
Based upon the evidence to date, it appears that lactate has the potential to act both as an “energy substrate” and a “prognostic factor.” Numerous studies have shown benefits in preserving the function of ischemic myocardium by modifying cardiac energy substrates and increasing ATP production (Dyck et al., 2004, 2006; Ussher and Lopaschuk, 2008; Lionetti et al., 2011). Both promoting the utilization of glucose and reducing the β-oxidation of fatty acids have been proposed as anti-ischemic strategies (Stanley et al., 2005; Dyck et al., 2006). However, there is a lack of clinical evidence to support treatment with lactate supplementation or deprivation in patients with acute cardiac events. It should also be noted that observational studies do not allow to conclude on the causal relationship between lactate and clinical outcomes.
Heart Failure
Heart failure is a complex disease which represents the end-stage outcome for many cardiac and systemic diseases (Savarese and Lund, 2017). Despite the heterogeneity of etiology (Jessup and Brozena, 2003), heart failure is associated with a marked reduction in the production of energy (Hearse, 1979; Neubauer et al., 1997; Neubauer, 2007). Impaired mitochondrial structure and oxidative function have been reported in the failing heart (Casademont and Miro, 2002; Neubauer, 2007; Aubert et al., 2013; Fukushima et al., 2015). Along with these changes, alterations of energy substrates were also detected (Krahe et al., 1993; Collins-Nakai et al., 1994; Lopaschuk et al., 2010). For example, decreasing ratio of fatty acids oxidation was observed in pressure overload-induced rat failing heart as well as mouse gene-knockout failing heart models (Allard et al., 1994; Casademont and Miro, 2002; Neubauer, 2007; Bugger et al., 2010), and ketone body was reported to be an alternative fuel in advanced human heart failure (Aubert et al., 2016; Bedi, Snyder et al., 2016). Although the myocardial glucose uptake is augmented, glucose oxidation and its contribution to ATP production is markedly decreased (Paolisso et al., 1994; Moravec et al., 1996; Funada et al., 2009; Mori et al., 2013; Zhabyeyev et al., 2013; Zhang et al., 2013). Where does the glucose go? In both humans and animals, there is increased flux of glycolysis (Allard et al., 1994; Degens et al., 2006; Akki et al., 2008; Symons and Abel, 2013) and plasma concentrations of lactate during heart failure (Diakos et al., 2016; Fillmore et al., 2018). It is therefore apparent that mitochondrial oxidation shifts to glycolysis as a major metabolic reprogramming event in the failing heart.
Increased glycolysis is linked to augmented production of endogenous lactate. In addition, the intracellular lactate shuttle process is inhibited by impaired activity of mLOC, manifested by the progressive impairment of PDH (Funada et al., 2009; Zhabyeyev et al., 2013; Zhang et al., 2013; Dodd et al., 2014; Diakos et al., 2016; Fillmore et al., 2018) and low expression of MCT (Gupte et al., 2014). Taken together, these events would give rise to the accumulation of intracellular lactate in the myocardium. This however, does not allow to conclude whether high level of intracellular lactate is good or bad! On the one hand, it has been indicated that intracellular lactate overload is able to trigger the influx of Na+ and Ca2+, which can induce a decrease in the systolic function of myocardium (Fiolet and Baartscheer, 2000; Jaswal et al., 2011). On the other hand, both basic science and clinical evidence have pointed toward an important role of lactate as a key energy substrate in the failing heart (Schurr et al., 1997; Chiolero et al., 2000; Luptak et al., 2005). A recent study described heart metabolomics profiling of the uptake and release of metabolites in patients with or without heart failure. This study showed that the failing heart nearly doubled lactate consumption compared to the normal heart (Murashige et al., 2020). The mRNA expression of MCT4 (which mediates the transmembrane transport of lactate) increased 2.5–3.5 times higher of its original level during myocardial injury (Zhu et al., 2013; Gabriel-Costa et al., 2015). Promoting the lactate transport from cytoplasm to mitochondria improved energy deficiency in heart failure (Wilson et al., 1998; McClelland and Brooks, 2002; Zhu et al., 2013). Furthermore, inhibition of MCT4 and hence lactate export in a cell model of heart failure led to further accumulation of intracellular lactate and increased lactate transport for mitochondrial oxidization (Cluntun et al., 2021).
Hyperlactatemia and lactic acidosis reflect an unbalanced state of lactate production and disposal. In long-term clinical practice, lactate is regarded as a risk factor of heart failure. In patients who suffer from heart failure and have elevated blood lactate, many clinicians may empirically consider pharmacotherapeutic interventions with agents that may modulate lactate metabolism, and vasodilators or positive inotropic drugs. However, systemic lactate deprivation is disadvantageous to myocardial energy supply in pathological conditions (Levy et al., 2007). Given that lactate concentrations in capillary, arterial, and venous blood are insufficient to distinguish excess lactate production from impaired lactate clearance, it would be imprudent to treat abnormal blood lactate levels in patients with heart failure.
Lactate Metabolism in Diabetic State
Adaptations to long-term diabetic state induce changes in cardiac energy substrate preference. Rising circulating fatty acids (Reaven et al., 1988; Young et al., 2002; Atkinson et al., 2003), high myocardial uptake of fatty acids (Avogaro et al., 1990; Sampson et al., 2003; Bonen et al., 2004; Peterson et al., 2008), and increased myocardial fatty acid β-oxidation (Belke et al., 2000; Aasum et al., 2003; Carley and Severson, 2005; How et al., 2007; Bugger and Abel, 2014; Riehle and Abel, 2016; Kenny and Abel, 2019) are important metabolic characteristics in diabetes. In addition, there are studies reporting hyperketonemia and high myocardial ketone body utilization in poorly controlled diabetes (Lommi et al., 1996, 1997; Kodde et al., 2007). The so-called “ketone body metabolic pathway” doesn’t really exist in the cell, because ketone bodies can cross the mitochondrial membrane and the cell membrane directly through the MCTs and enter the TCA cycle (Halestrap, 2013; Felmlee et al., 2020). The flow of ketone bodies into the TCA cycle is expected to inhibit mitochondrial oxidation of glucose and lactate in cardiomyocytes. Compared to studies on fatty acid and ketone body metabolism in heart, very few studies have assessed myocardial glucose and lactate utilization in the diabetic state. It is generally accepted that acceleration of fatty acid β-oxidation and insulin resistance are related to the reduction of glucose oxidation (Randle et al., 1963; Randle, 1995, 1998; Peterson et al., 2004). With regard to myocardial lactate metabolism, several studies observed the rate of lactate efflux was greater than lactate uptake under diabetic condition (Chatham et al., 2001). Given that MCT expression in cardiomyocytes is not influenced by diabetes in rat models (Chatham et al., 1999), decreased lactate uptake might be related to the increased cytosolic NADH/NAD+ ratio in the diabetic state (Puckett and Reddy, 1979; Ramasamy et al., 1997; Trueblood and Ramasamy, 1998; Chatham et al., 1999). Taken together, myocardial glucose and lactate metabolism are impaired on diabetic state. Impaired insulin signaling was reported to impel the deterioration of cardiac function (Abel et al., 1999; Peterson et al., 2004; Zhang et al., 2013; Byrne et al., 2016). Patients with diabetes exhibited an increased risk of heart failure, and cardiac hypertrophy is the main pathologic change of the myocardial remodeling. As discussed, substrate switch of heart failure generally appeared as a decreased fatty acid oxidation and an increased uptake of glucose and lactate (Neubauer, 2007; Casademont and Miro; 2002, Allard et al., 1994; Krishnan et al., 2009; Bugger et al., 2010). By contrast, diabetic heart failure exhibits different substrate switch—an increase in fatty acid metabolism and a decrease in glucose and lactate metabolism (Bugger and Abel, 2014; Riehle and Abel, 2016; Kenny and Abel, 2019). It is also intriguing that these divergent cardiac energy substrate preferences result in similar myocardial remodeling.
Metformin is the most widely used oral agent for the management of type 2 diabetes. In the landmark UKPDS study, the use of metformin was reported to reduce the risk of myocardial infarction by 39% (No Authors listed., 1998). Metformin is associated with a modest increase plasma lactate levels, but the risk of lactate acidosis is rare (Abbasi et al., 2000; Liu et al., 2009; Shen et al., 2012; Koren et al., 2017). Metformin does not appear to affect myocardial lactate utilization, but has been shown to reduce the intracellular lactate shuttle and increase lactate accumulation (Madiraju et al., 2014; Duca et al., 2015; Lu et al., 2017). Given that metformin is associated with increased lactate production, and tissue hypoxia is always present during heart failure. Metformin has previously been believed to increase the risk of lactic acidosis in heart failure patients. However, in recent years, metformin has been exhibited to be safe and effective in patients with heart failure in several large retrospective studies (Misbin et al., 1998; Aguilar et al., 2011). Based on these studies, metformin has been recommended for patients with diabetes mellitus and chronic heart failure (Eurich et al., 2005, 2013). Taken together, the effects of metformin on lactate metabolism do not outweigh its the benefits in diabetic cardiomyopathy. But we have to pay more attention to metformin-associated lactic acidosis (MALA), a symptom that may occur in the clinic. The exact mechanism of MALA is still unknown, but it would be necessary to elevated blood metformin concentration and secondary obstacles of lactate production and clearance (Lucis, 1983; Owen et al., 2000; Almirall et al., 2008; Frid et al., 2010; Bridges et al., 2014). As these secondary events may be unpredictable and heterogeneous, current clinical application of metformin may be too conservative. Given that fatal consequence of MALA, metformin must be used with caution, particularly in patients with circulatory dysfunction (Buse et al., 2016).
Conclusion
In the pursuit of an understanding of myocardial metabolism, lactate was once considered as a metabolic waste, and the metamorphosis from “ugly duckling” to “white swan” was full of frustration and ordeals. Up to time now, it is apparent that lactate has multiple identities in myocardial metabolism, including energy substrate, metabolite, signal molecule, and prognostic factor. Cardiac lactate metabolism is a dynamic process that can rapidly shift to adapt to alterations in cardiac energy requirements or changing environment. Lactate is also very important as an energy substrate in acute myocardial ischemia, heart failure, and diabetic state, and abnormal myocardial lactate metabolism is closely related to diseases. Characterization of the lactate metabolic profile of myocardium in physiological and pathological conditions may help direct future pharmacological therapies to harmonize the metabolic flexibility of the heart.
Author Contributions
SD, LQ, ZC, CC, KW, and XZ were involved in writing of the manuscript. SH, XZ, and TW involved in the conception. XZ and TW was involved in the conception, design, and the coordination of the study. SD, LQ, and ZC were involved in the picture drawing. SD, LQ, ZC, CC, KW, SH, XZ, and TW critically reviewed the manuscript and have approved the publication of this final version of the manuscript. XZ and TW are the guarantors of this work. All authors contributed to the article and approved the submitted version.
Conflict of Interest
The authors declare that the research was conducted in the absence of any commercial or financial relationships that could be construed as a potential conflict of interest.
Publisher’s Note
All claims expressed in this article are solely those of the authors and do not necessarily represent those of their affiliated organizations, or those of the publisher, the editors and the reviewers. Any product that may be evaluated in this article, or claim that may be made by its manufacturer, is not guaranteed or endorsed by the publisher.
Acknowledgments
The authors would like to thank all of the research participants, scholars, and funding agencies who have contributed to the research cited in this manuscript.
References
Aasum, E., Hafstad, A. D., Severson, D. L., and Larsen, T. S. (2003). Age-dependent changes in metabolism, contractile function, and ischemic sensitivity in hearts from db/db mice. Diabetes 52, 434–441. doi: 10.2337/diabetes.52.2.434
Abbasi, A. A., Kasmikha, R., and Sotingeanu, D. G. (2000). Metformin-induced lacticacidemia in patients with type 2 diabetes mellitus. Endocr. Pract. 6, 442–446. doi: 10.4158/EP.6.6.442
Abel, E. D., Kaulbach, H. C., Tian, R., Hopkins, J. C., Duffy, J., Doetschman, T., et al. (1999). Cardiac hypertrophy with preserved contractile function after selective deletion of GLUT4 from the heart. J. Clin. Invest. 104, 1703–1714. doi: 10.1172/JCI7605
Aguilar, D., Chan, W., Bozkurt, B., Ramasubbu, K., and Deswal, A. (2011). Metformin use and mortality in ambulatory patients with diabetes and heart failure. Circ. Heart Fail. 4, 53–58. doi: 10.1161/CIRCHEARTFAILURE.110.952556
Akki, A., Smith, K., and Seymour, A. M. (2008). Compensated cardiac hypertrophy is characterised by a decline in palmitate oxidation. Mol. Cell. Biochem. 311, 215–224. doi: 10.1007/s11010-008-9711-y
Allard, M. F., Schonekess, B. O., Henning, S. L., English, D. R., and Lopaschuk, G. D. (1994). Contribution of oxidative metabolism and glycolysis to ATP production in hypertrophied hearts. Am. J. Physiol. 267, H742–H750. doi: 10.1152/ajpheart.1994.267.2.H742
Almirall, J., Briculle, M., and Gonzalez-Clemente, J. M. (2008). Metformin-associated lactic acidosis in type 2 diabetes mellitus: incidence and presentation in common clinical practice. Nephrol. Dial. Transplant. 23, 2436–2438. doi: 10.1093/ndt/gfn152
Andersen, L. W., Mackenhauer, J., Roberts, J. C., Berg, K. M., Cocchi, M. N., and Donnino, M. W. (2013). Etiology and therapeutic approach to elevated lactate levels. Mayo Clin. Proc. 88, 1127–1140. doi: 10.1016/j.mayocp.2013.06.012
Askenasy, N. (2001). Glycolysis protects sarcolemmal membrane integrity during total ischemia in the rat heart. Basic Res. Cardiol. 96, 612–622. doi: 10.1007/s003950170013
Atkinson, L. L., Kozak, R., Kelly, S. E., Onay Besikci, A., Russell, J. C., and Lopaschuk, G. D. (2003). Potential mechanisms and consequences of cardiac triacylglycerol accumulation in insulin-resistant rats. Am. J. Physiol. Endocrinol. Metab. 284, E923–E930. doi: 10.1152/ajpendo.00360.2002
Atlante, A., de Bari, L., Bobba, A., Marra, E., and Passarella, S. (2007). Transport and metabolism of L-lactate occur in mitochondria from cerebellar granule cells and are modified in cells undergoing low potassium dependent apoptosis. Biochim. Biophys. Acta 1767, 1285–1299. doi: 10.1016/j.bbabio.2007.08.003
Attana, P., Lazzeri, C., Chiostri, M., Gensini, G. F., and Valente, S. (2013a). Dynamic behavior of lactate values in venous-arterial extracorporeal membrane oxygenation for refractory cardiac arrest. Resuscitation 84, e145–6. doi: 10.1016/j.resuscitation.2013.07.007
Attana, P., Lazzeri, C., Chiostri, M., Picariello, C., Gensini, G. F., and Valente, S. (2013b). Strong-ion gap approach in patients with cardiogenic shock following ST-elevation myocardial infarction. Acute Card. Care 15, 58–62. doi: 10.3109/17482941.2013.776691
Aubert, G., Martin, O. J., Horton, J. L., Lai, L., Vega, R. B., Leone, T. C., et al. (2016). The Failing Heart Relies on Ketone Bodies as a Fuel. Circulation 133, 698–705. doi: 10.1161/CIRCULATIONAHA.115.017355
Aubert, G., Vega, R. B., and Kelly, D. P. (2013). Perturbations in the gene regulatory pathways controlling mitochondrial energy production in the failing heart. Biochim. Biophys. Acta 1833, 840–847. doi: 10.1016/j.bbamcr.2012.08.015
Avogaro, A., Nosadini, R., Doria, A., Fioretto, P., Velussi, M., Vigorito, C., et al. (1990). Myocardial metabolism in insulin-deficient diabetic humans without coronary artery disease. Am. J. Physiol. 258, E606–E618. doi: 10.1152/ajpendo.1990.258.4.E606
Barbee, R. W., Kline, J. A., and Watts, J. A. (2000). Depletion of lactate by dichloroacetate reduces cardiac efficiency after hemorrhagic shock. Shock 14, 208–214. doi: 10.1097/00024382-200014020-00022
Barnard, R. J., Edgerton, V. R., Furukawa, T., and Peter, J. B. (1971). Histochemical, biochemical, and contractile properties of red, white, and intermediate fibers. Am. J. Physiol. 220, 410–414. doi: 10.1152/ajplegacy.1971.220.2.410
Bartelds, B., Knoester, H., Beaufort-Krol, G. C., Smid, G. B., Takens, J., Zijlstra, W. G., et al. (1999). Myocardial lactate metabolism in fetal and newborn lambs. Circulation 99, 1892–1897. doi: 10.1161/01.cir.99.14.1892
Bedi, K. C. Jr., Snyder, N. W., Brandimarto, J., Aziz, M., Mesaros, C., Worth, A. J., et al. (2016). Evidence for Intramyocardial Disruption of Lipid Metabolism and Increased Myocardial Ketone Utilization in Advanced Human Heart Failure. Circulation 133, 706–716. doi: 10.1161/CIRCULATIONAHA.115.017545
Belke, D. D., Larsen, T. S., Gibbs, E. M., and Severson, D. L. (2000). Altered metabolism causes cardiac dysfunction in perfused hearts from diabetic (db/db) mice. Am. J. Physiol. Endocrinol. Metab. 279, E1104–E1113. doi: 10.1152/ajpendo.2000.279.5.E1104
Beloukas, A. I., Magiorkinis, E., Tsoumakas, T. L., Kosma, A. G., and Diamantis, A. (2013). Milestones in the history of research on cardiac energy metabolism. Can. J. Cardiol. 29, 1504–1511. doi: 10.1016/j.cjca.2012.10.008
Bergman, B. C., Butterfield, G. E., Wolfel, E. E., Lopaschuk, G. D., Casazza, G. A., Horning, M. A., et al. (1999a). Muscle net glucose uptake and glucose kinetics after endurance training in men. Am. J. Physiol. 277, E81–E92. doi: 10.1152/ajpendo.1999.277.1.E81
Bergman, B. C., Horning, M. A., Casazza, G. A., Wolfel, E. E., Butterfield, G. E., and Brooks, G. A. (2000). Endurance training increases gluconeogenesis during rest and exercise in men. Am. J. Physiol. Endocrinol. Metab. 278, E244–E251. doi: 10.1152/ajpendo.2000.278.2.E244
Bergman, B. C., Tsvetkova, T., Lowes, B., and Wolfel, E. E. (2009). Myocardial glucose and lactate metabolism during rest and atrial pacing in humans. J. Physiol. 587, 2087–2099. doi: 10.1113/jphysiol.2008.168286
Bergman, B. C., Wolfel, E. E., Butterfield, G. E., Lopaschuk, G. D., Casazza, G. A., Horning, M. A., et al. (1999b). Active muscle and whole body lactate kinetics after endurance training in men. J. Appl. Physiol. 87, 1684–1696. doi: 10.1152/jappl.1999.87.5.1684
Bing, R. J., Vandam, L. D., Gregoire, F., Handelsman, J. C., Goodale, W. T., Eckenhoff, J. E., et al. (1947). Catheterization of the coronary sinus and the middle cardiac vein in man. Proc. Soc. Exp. Biol. Med. 66:239. doi: 10.3181/00379727-66-16049p
Bonen, A., Parolin, M. L., Steinberg, G. R., Calles-Escandon, J., Tandon, N. N., Glatz, J. F., et al. (2004). Triacylglycerol accumulation in human obesity and type 2 diabetes is associated with increased rates of skeletal muscle fatty acid transport and increased sarcolemmal FAT/CD36. FASEB J. 18, 1144–1146. doi: 10.1096/fj.03-1065fje
Brandt, R. B., Laux, J. E., Spainhour, S. E., and Kline, E. S. (1987). Lactate dehydrogenase in rat mitochondria. Arch. Biochem. Biophys. 259, 412–422. doi: 10.1016/0003-9861(87)90507-8
Bridges, H. R., Jones, A. J., Pollak, M. N., and Hirst, J. (2014). Effects of metformin and other biguanides on oxidative phosphorylation in mitochondria. Biochem. J. 462, 475–487. doi: 10.1042/BJ20140620
Brooks, G. A. (2000). Intra- and extra-cellular lactate shuttles. Med. Sci. Sports Exerc. 32, 790–799. doi: 10.1097/00005768-200004000-00011
Brooks, G. A. (2018). The Science and Translation of Lactate Shuttle Theory. Cell. Metab. 27, 757–785. doi: 10.1016/j.cmet.2018.03.008
Brooks, G. A., and Donovan, C. M. (1983). Effect of endurance training on glucose kinetics during exercise. Am. J. Physiol. 244, E505–E512. doi: 10.1152/ajpendo.1983.244.5.E505
Brooks, G. A., and Gaesser, G. A. (1980). End points of lactate and glucose metabolism after exhausting exercise. J. Appl. Physiol. Respir. Environ. Exerc. Physiol. 49, 1057–1069. doi: 10.1152/jappl.1980.49.6.1057
Brooks, G. A., and White, T. P. (1978). Determination of metabolic and heart rate responses of rats to treadmill exercise. J. Appl. Physiol. Respir. Environ. Exerc. Physiol. 45, 1009–1015. doi: 10.1152/jappl.1978.45.6.1009
Brooks, G. A., Bissell, M. J., and Bassham, J. A. (1977). Ion-retardation desalting of blood and other animal tissues for separation of soluble metabolites by two-dimensional chromatography. Anal. Biochem. 83, 580–588. doi: 10.1016/0003-2697(77)90061-6
Brooks, G. A., Brauner, K. E., and Cassens, R. G. (1973). Glycogen synthesis and metabolism of lactic acid after exercise. Am. J. Physiol. 224, 1162–1166. doi: 10.1152/ajplegacy.1973.224.5.1162
Brooks, G. A., Donovan, C. M., and White, T. P. (1984). Estimation of anaerobic energy production and efficiency in rats during exercise. J. Appl. Physiol. Respir. Environ. Exerc. Physiol. 56, 520–525. doi: 10.1152/jappl.1984.56.2.520
Brooks, G. A., Dubouchaud, H., Brown, M., Sicurello, J. P., and Butz, C. E. (1999). Role of mitochondrial lactate dehydrogenase and lactate oxidation in the intracellular lactate shuttle. Proc. Natl. Acad. Sci. U. S. A. 96, 1129–1134. doi: 10.1073/pnas.96.3.1129
Bugger, H., and Abel, E. D. (2014). Molecular mechanisms of diabetic cardiomyopathy. Diabetologia 57, 660–671. doi: 10.1007/s00125-014-3171-6
Bugger, H., Schwarzer, M., Chen, D., Schrepper, A., Amorim, P. A., Schoepe, M., et al. (2010). Proteomic remodelling of mitochondrial oxidative pathways in pressure overload-induced heart failure. Cardiovasc. Res. 85, 376–384. doi: 10.1093/cvr/cvp344
Buse, J. B., DeFronzo, R. A., Rosenstock, J., Kim, T., Burns, C., Skare, S., et al. (2016). The Primary Glucose-Lowering Effect of Metformin Resides in the Gut, Not the Circulation: results From Short-term Pharmacokinetic and 12-Week Dose-Ranging Studies. Diabetes Care 39, 198–205. doi: 10.2337/dc15-0488
Byrne, N. J., Levasseur, J., Sung, M. M., Masson, G., Boisvenue, J., Young, M. E., et al. (2016). Normalization of cardiac substrate utilization and left ventricular hypertrophy precede functional recovery in heart failure regression. Cardiovasc. Res. 110, 249–257. doi: 10.1093/cvr/cvw051
Carley, A. N., and Severson, D. L. (2005). Fatty acid metabolism is enhanced in type 2 diabetic hearts. Biochim. Biophys. Acta 1734, 112–126. doi: 10.1016/j.bbalip.2005.03.005
Carvajal, K., Zarrinpashneh, E., Szarszoi, O., Joubert, F., Athea, Y., Mateo, P., et al. (2007). Dual cardiac contractile effects of the alpha2-AMPK deletion in low-flow ischemia and reperfusion. Am. J. Physiol. Heart Circ. Physiol. 292, H3136–H3147. doi: 10.1152/ajpheart.00683.2006
Casademont, J., and Miro, O. (2002). Electron transport chain defects in heart failure. Heart Fail. Rev. 7, 131–139. doi: 10.1023/a:1015372407647
Cerovic, O., Golubovic, V., Spec-Marn, A., Kremzar, B., and Vidmar, G. (2003). Relationship between injury severity and lactate levels in severely injured patients. Intensive Care Med. 29, 1300–1305. doi: 10.1007/s00134-003-1753-8
Chatham, J. C., Des Rosiers, C., and Forder, J. R. (2001). Evidence of separate pathways for lactate uptake and release by the perfused rat heart. Am. J. Physiol. Endocrinol. Metab. 281, E794–E802. doi: 10.1152/ajpendo.2001.281.4.E794
Chatham, J. C., Gao, Z. P., Bonen, A., and Forder, J. R. (1999). Preferential inhibition of lactate oxidation relative to glucose oxidation in the rat heart following diabetes. Cardiovasc. Res. 43, 96–106. doi: 10.1016/s0008-6363(99)00056-5
Chen, Y. J., Mahieu, N. G., Huang, X., Singh, M., Crawford, P. A., Johnson, S. L., et al. (2016). Lactate metabolism is associated with mammalian mitochondria. Nat. Chem. Biol. 12, 937–943. doi: 10.1038/nchembio.2172
Chiolero, R. L., Revelly, J. P., Leverve, X., Gersbach, P., Cayeux, M. C., Berger, M. M., et al. (2000). Effects of cardiogenic shock on lactate and glucose metabolism after heart surgery. Crit. Care Med. 28, 3784–3791. doi: 10.1097/00003246-200012000-00002
Cluntun, A. A., Badolia, R., Lettlova, S., Parnell, K. M., Shankar, T. S., Diakos, N. A., et al. (2021). The pyruvate-lactate axis modulates cardiac hypertrophy and heart failure. Cell Metab. 33, 629–648.e10. doi: 10.1016/j.cmet.2020.12.003
Cocchi, M. N., Miller, J., Hunziker, S., Carney, E., Salciccioli, J., Farris, S., et al. (2011). The association of lactate and vasopressor need for mortality prediction in survivors of cardiac arrest. Minerva. Anestesiol. 77, 1063–1071.
Collins-Nakai, R. L., Noseworthy, D., and Lopaschuk, G. D. (1994). Epinephrine increases ATP production in hearts by preferentially increasing glucose metabolism. Am. J. Physiol. 267, H1862–H1871. doi: 10.1152/ajpheart.1994.267.5.H1862
De Bari, L., Atlante, A., Valenti, D., and Passarella, S. (2004). Partial reconstruction of in vitro gluconeogenesis arising from mitochondrial l-lactate uptake/metabolism and oxaloacetate export via novel L-lactate translocators. Biochem. J. 380, 231–242. doi: 10.1042/BJ20031981
Degens, H., de Brouwer, K. F., Gilde, A. J., Lindhout, M., Willemsen, P. H., Janssen, B. J., et al. (2006). Cardiac fatty acid metabolism is preserved in the compensated hypertrophic rat heart. Basic Res. Cardiol. 101, 17–26. doi: 10.1007/s00395-005-0549-0
Depocas, F., Minaire, Y., and Chatonnet, J. (1969). Rates of formation and oxidation of lactic acid in dogs at rest and during moderate exercise. Can. J. Physiol. Pharmacol. 47, 603–610. doi: 10.1139/y69-106
Diakos, N. A., Navankasattusas, S., Abel, E. D., Rutter, J., McCreath, L., Ferrin, P., et al. (2016). Evidence of Glycolysis Up-Regulation and Pyruvate Mitochondrial Oxidation Mismatch During Mechanical Unloading of the Failing Human Heart: implications for Cardiac Reloading and Conditioning. JACC Basic Transl. Sci. 1, 432–444. doi: 10.1016/j.jacbts.2016.06.009
Dodd, M. S., Atherton, H. J., Carr, C. A., Stuckey, D. J., West, J. A., Griffin, J. L., et al. (2014). Impaired in vivo mitochondrial Krebs cycle activity after myocardial infarction assessed using hyperpolarized magnetic resonance spectroscopy. Circ. Cardiovasc. Imaging 7, 895–904. doi: 10.1161/CIRCIMAGING.114.001857
Donnino, M. W., Miller, J., Goyal, N., Loomba, M., Sankey, S. S., Dolcourt, B., et al. (2007). Effective lactate clearance is associated with improved outcome in post-cardiac arrest patients. Resuscitation 75, 229–234. doi: 10.1016/j.resuscitation.2007.03.021
Donovan, C. M., and Brooks, G. A. (1983). Endurance training affects lactate clearance, not lactate production. Am. J. Physiol. 244, E83–E92. doi: 10.1152/ajpendo.1983.244.1.E83
Drake, A. J., Haines, J. R., and Noble, M. I. (1980). Preferential uptake of lactate by the normal myocardium in dogs. Cardiovasc. Res. 14, 65–72. doi: 10.1093/cvr/14.2.65
Duca, F. A., Cote, C. D., Rasmussen, B. A., Zadeh-Tahmasebi, M., Rutter, G. A., Filippi, B. M., et al. (2015). Metformin activates a duodenal Ampk-dependent pathway to lower hepatic glucose production in rats. Nat. Med. 21, 506–511. doi: 10.1038/nm.3787
Dunn, A., Katz, J., Golden, S., and Chenoweth, M. (1976). Estimation of glucose turnover and recycling in rabbits using various [3H, 14C]glucose labels. Am. J. Physiol. 230, 1159–1162. doi: 10.1152/ajplegacy.1976.230.4.1159
Dyck, J. R., Cheng, J. F., Stanley, W. C., Barr, R., Chandler, M. P., Brown, S., et al. (2004). Malonyl coenzyme a decarboxylase inhibition protects the ischemic heart by inhibiting fatty acid oxidation and stimulating glucose oxidation. Circ. Res. 94, e78–84. doi: 10.1161/01.RES.0000129255.19569.8f
Dyck, J. R., Hopkins, T. A., Bonnet, S., Michelakis, E. D., Young, M. E., Watanabe, M., et al. (2006). Absence of malonyl coenzyme A decarboxylase in mice increases cardiac glucose oxidation and protects the heart from ischemic injury. Circulation 114, 1721–1728. doi: 10.1161/CIRCULATIONAHA.106.642009
Emhoff, C. A., Messonnier, L. A., Horning, M. A., Fattor, J. A., Carlson, T. J., and Brooks, G. A. (2013b). Gluconeogenesis and hepatic glycogenolysis during exercise at the lactate threshold. J. Appl. Physiol. 114, 297–306. doi: 10.1152/japplphysiol.01202.2012
Emhoff, C. A., Messonnier, L. A., Horning, M. A., Fattor, J. A., Carlson, T. J., and Brooks, G. A. (2013a). Direct and indirect lactate oxidation in trained and untrained men. J. Appl. Physiol. 115, 829–838. doi: 10.1152/japplphysiol.00538.2013
Eurich, D. T., Majumdar, S. R., McAlister, F. A., Tsuyuki, R. T., and Johnson, J. A. (2005). Improved clinical outcomes associated with metformin in patients with diabetes and heart failure. Diabetes Care 28, 2345–2351. doi: 10.2337/diacare.28.10.2345
Eurich, D. T., Weir, D. L., Majumdar, S. R., Tsuyuki, R. T., Johnson, J. A., Tjosvold, L., et al. (2013). Comparative safety and effectiveness of metformin in patients with diabetes mellitus and heart failure: systematic review of observational studies involving 34,000 patients. Circ. Heart Fail. 6, 395–402. doi: 10.1161/CIRCHEARTFAILURE.112.000162
Evans, C. L. (1914). The effect of glucose on the gaseous metabolism of the isolated mammalian heart. J. Physiol. 47, 407–418. doi: 10.1113/jphysiol.1914.sp001633
Felmlee, M. A., Jones, R. S., Rodriguez-Cruz, V., Follman, K. E., and Morris, M. E. (2020). Monocarboxylate Transporters (SLC16): function, Regulation, and Role in Health and Disease. Pharmacol. Rev. 72, 466–485. doi: 10.1124/pr.119.018762
Fillmore, N., Levasseur, J. L., Fukushima, A., Wagg, C. S., Wang, W., Dyck, J. R. B., et al. (2018). Uncoupling of glycolysis from glucose oxidation accompanies the development of heart failure with preserved ejection fraction. Mol. Med. 24:3. doi: 10.1186/s10020-018-0005-x
Fiolet, J. W., and Baartscheer, A. (2000). Cellular calcium homeostasis during ischemia; a thermodynamic approach. Cardiovasc. Res. 45, 100–106. doi: 10.1016/s0008-6363(99)00294-1
Frid, A., Sterner, G. N., Londahl, M., Wiklander, C., Cato, A., Vinge, E., et al. (2010). Novel assay of metformin levels in patients with type 2 diabetes and varying levels of renal function: clinical recommendations. Diabetes Care 33, 1291–1293. doi: 10.2337/dc09-1284
Fukushima, A., Milner, K., Gupta, A., and Lopaschuk, G. D. (2015). Myocardial Energy Substrate Metabolism in Heart Failure : from Pathways to Therapeutic Targets. Curr. Pharm. Des. 21, 3654–3664. doi: 10.2174/1381612821666150710150445
Funada, J., Betts, T. R., Hodson, L., Humphreys, S. M., Timperley, J., Frayn, K. N., et al. (2009). Substrate utilization by the failing human heart by direct quantification using arterio-venous blood sampling. PLoS One 4:e7533. doi: 10.1371/journal.pone.0007533
Gabriel-Costa, D., da Cunha, T. F., Bechara, L. R., Fortunato, R. S., Bozi, L. H., Coelho Mde, A., et al. (2015). Lactate up-regulates the expression of lactate oxidation complex-related genes in left ventricular cardiac tissue of rats. PLoS One 10:e0127843. doi: 10.1371/journal.pone.0127843
Garcia, C. K., Goldstein, J. L., Pathak, R. K., Anderson, R. G., and Brown, M. S. (1994). Molecular characterization of a membrane transporter for lactate, pyruvate, and other monocarboxylates: implications for the Cori cycle. Cell 76, 865–873. doi: 10.1016/0092-8674(94)90361-1
Garcia-Alvarez, M., Marik, P., and Bellomo, R. (2014). Stress hyperlactataemia: present understanding and controversy. Lancet Diabetes Endocrinol. 2, 339–347. doi: 10.1016/S2213-8587(13)70154-2
Geppert, A., Dorninger, A., Delle-Karth, G., Zorn, G., Heinz, G., and Huber, K. (2006). Plasma concentrations of interleukin-6, organ failure, vasopressor support, and successful coronary revascularization in predicting 30-day mortality of patients with cardiogenic shock complicating acute myocardial infarction. Crit. Care Med. 34, 2035–2042. doi: 10.1097/01.CCM.0000228919.33620.D9
Gertz, E. W., Wisneski, J. A., Neese, R., Bristow, J. D., Searle, G. L., and Hanlon, J. T. (1981). Myocardial lactate metabolism: evidence of lactate release during net chemical extraction in man. Circulation 63, 1273–1279. doi: 10.1161/01.cir.63.6.1273
Gertz, E. W., Wisneski, J. A., Stanley, W. C., and Neese, R. A. (1988). Myocardial substrate utilization during exercise in humans. Dual carbon-labeled carbohydrate isotope experiments. J. Clin. Invest. 82, 2017–2025. doi: 10.1172/JCI113822
Gh, V. K. (2006). [The 100(th) anniversary of “The Conduction System of the Mammalian Heart” by Sunao Tawara]. Herzschrittmacherther. Elektrophysiol. 17, 140–145. doi: 10.1007/s00399-006-0525-x
Gizak, A., McCubrey, J. A., and Rakus, D. (2020). Cell-to-cell lactate shuttle operates in heart and is important in age-related heart failure. Aging 12, 3388–3406. doi: 10.18632/aging.102818
Glancy, B., Hartnell, L. M., Combs, C. A., Femnou, A., Sun, J., Murphy, E., et al. (2017). Power Grid Protection of the Muscle Mitochondrial Reticulum. Cell. Rep. 19, 487–496. doi: 10.1016/j.celrep.2017.03.063
Glenn, T. C., Martin, N. A., Horning, M. A., McArthur, D. L., Hovda, D. A., Vespa, P., et al. (2015). Lactate: brain fuel in human traumatic brain injury: a comparison with normal healthy control subjects. J. Neurotrauma. 32, 820–832. doi: 10.1089/neu.2014.3483
Goodwin, G. W., Taylor, C. S., and Taegtmeyer, H. (1998). Regulation of energy metabolism of the heart during acute increase in heart work. J. Biol. Chem. 273, 29530–29539. doi: 10.1074/jbc.273.45.29530
Gupte, A. A., Hamilton, D. J., Cordero-Reyes, A. M., Youker, K. A., Yin, Z., Estep, J. D., et al. (2014). Mechanical unloading promotes myocardial energy recovery in human heart failure. Circ. Cardiovasc. Genet. 7, 266–276. doi: 10.1161/CIRCGENETICS.113.000404
Halestrap, A. P. (2013). Monocarboxylic acid transport. Compr. Physiol. 3, 1611–1643. doi: 10.1002/cphy.c130008
Hashimoto, T., Hussien, R., and Brooks, G. A. (2006). Colocalization of MCT1, CD147, and LDH in mitochondrial inner membrane of L6 muscle cells: evidence of a mitochondrial lactate oxidation complex. Am. J. Physiol. Endocrinol. Metab. 290, E1237–E1244. doi: 10.1152/ajpendo.00594.2005
Hashimoto, T., Hussien, R., Cho, H. S., Kaufer, D., and Brooks, G. A. (2008). Evidence for the mitochondrial lactate oxidation complex in rat neurons: demonstration of an essential component of brain lactate shuttles. PLoS One 3:e2915. doi: 10.1371/journal.pone.0002915
Hashimoto, T., Hussien, R., Oommen, S., Gohil, K., and Brooks, G. A. (2007). Lactate sensitive transcription factor network in L6 cells: activation of MCT1 and mitochondrial biogenesis. FASEB J. 21, 2602–2612. doi: 10.1096/fj.07-8174com
Hearse, D. J. (1979). Oxygen deprivation and early myocardial contractile failure: a reassessment of the possible role of adenosine triphosphate. Am. J. Cardiol. 44, 1115–1121. doi: 10.1016/0002-9149(79)90177-2
Herzig, S., and Shaw, R. J. (2018). AMPK: guardian of metabolism and mitochondrial homeostasis. Nat. Rev. Mol. Cell. Biol. 19, 121–135. doi: 10.1038/nrm.2017.95
Hooker, A. M., and Baldwin, K. M. (1979). Substrate oxidation specificity in different types of mammalian muscle. Am. J. Physiol. 236, C66–C69. doi: 10.1152/ajpcell.1979.236.1.C66
Horman, S., Beauloye, C., Vanoverschelde, J. L., and Bertrand, L. (2012). AMP-activated protein kinase in the control of cardiac metabolism and remodeling. Curr. Heart Fail. Rep. 9, 164–173. doi: 10.1007/s11897-012-0102-z
How, O. J., Larsen, T. S., Hafstad, A. D., Khalid, A., Myhre, E. S., Murray, A. J., et al. (2007). Rosiglitazone treatment improves cardiac efficiency in hearts from diabetic mice. Arch. Physiol. Biochem. 113, 211–220. doi: 10.1080/13813450701783281
Hutter, J. F., Schweickhardt, C., Piper, H. M., and Spieckermann, P. G. (1984). Inhibition of fatty acid oxidation and decrease of oxygen consumption of working rat heart by 4-bromocrotonic acid. J. Mol. Cell. Cardiol. 16, 105–108. doi: 10.1016/s0022-2828(84)80718-x
Ide, T., Tsutsui, H., Hayashidani, S., Kang, D., Suematsu, N., Nakamura, K., et al. (2001). Mitochondrial DNA damage and dysfunction associated with oxidative stress in failing hearts after myocardial infarction. Circ. Res. 88, 529–535. doi: 10.1161/01.res.88.5.529
Jacobs, R. A., Meinild, A. K., Nordsborg, N. B., and Lundby, C. (2013). Lactate oxidation in human skeletal muscle mitochondria. Am. J. Physiol. Endocrinol. Metab. 304, E686–E694. doi: 10.1152/ajpendo.00476.2012
Jaswal, J. S., Keung, W., Wang, W., Ussher, J. R., and Lopaschuk, G. D. (2011). Targeting fatty acid and carbohydrate oxidation–a novel therapeutic intervention in the ischemic and failing heart. Biochim. Biophys. Acta 1813, 1333–1350. doi: 10.1016/j.bbamcr.2011.01.015
Jessup, M., and Brozena, S. (2003). Heart failure. N. Engl. J. Med. 348, 2007–2018. doi: 10.1056/NEJMra021498
Katz, J., Okajima, F., Chenoweth, M., and Dunn, A. (1981). The determination of lactate turnover in vivo with 3H- and 14C-labelled lactate. The significance of sites of tracer administration and sampling. Biochem. J. 194, 513–524. doi: 10.1042/bj1940513
Kenny, H. C., and Abel, E. D. (2019). Heart Failure in Type 2 Diabetes Mellitus. Circ. Res. 124, 121–141. doi: 10.1161/CIRCRESAHA.118.311371
Keul, J., Doll, E., Steim, H., Fleer, U., and Reindell, H. (1965a). [on Metabolism of the Human Heart. 3. Oxidative Metabolism of the Human Heart in Different Work Conditions]. Pflugers Arch. Gesamte, Physiol. Menschen. Tiere. 282, 43–53.
Keul, J., Doll, E., Steim, H., Homburger, H., Kern, H., and Reindell, H. (1965b). [on Metabolism of the Human Heart. I. Substrate Supply of the Healthy Human Heart during Rest, during and after Physical Work]. Pflugers Arch. Gesamte. Physiol. Menschen. Tiere. 282, 1–27.
Khosravani, H., Shahpori, R., Stelfox, H. T., Kirkpatrick, A. W., and Laupland, K. B. (2009). Occurrence and adverse effect on outcome of hyperlactatemia in the critically ill. Crit. Care 13:R90. doi: 10.1186/cc7918
Kim, A. S., Miller, E. J., Wright, T. M., Li, J., Qi, D., Atsina, K., et al. (2011). A small molecule AMPK activator protects the heart against ischemia-reperfusion injury. J. Mol. Cell. Cardiol. 51, 24–32. doi: 10.1016/j.yjmcc.2011.03.003
King, L. M., and Opie, L. H. (1998a). Glucose and glycogen utilisation in myocardial ischemia–changes in metabolism and consequences for the myocyte. Mol. Cell. Biochem. 180, 3–26. doi: 10.1007/978-1-4615-5687-9_1
King, L. M., and Opie, L. H. (1998b). Glucose delivery is a major determinant of glucose utilisation in the ischemic myocardium with a residual coronary flow. Cardiovasc. Res. 39, 381–392. doi: 10.1016/s0008-6363(98)00100-x
Kirkwood, S. P., Munn, E. A., and Brooks, G. A. (1986). Mitochondrial reticulum in limb skeletal muscle. Am. J. Physiol. 251, C395–C402. doi: 10.1152/ajpcell.1986.251.3.C395
Kline, E. S., Brandt, R. B., Laux, J. E., Spainhour, S. E., Higgins, E. S., Rogers, K. S., et al. (1986). Localization of L-lactate dehydrogenase in mitochondria. Arch. Biochem. Biophys. 246, 673–680. doi: 10.1016/0003-9861(86)90323-1
Kodde, I. F., van der Stok, J., Smolenski, R. T., and de Jong, J. W. (2007). Metabolic and genetic regulation of cardiac energy substrate preference. Comp. Biochem. Physiol. A Mol. Integr. Physiol. 146, 26–39. doi: 10.1016/j.cbpa.2006.09.014
Koren, S., Zilberman-Itskovich, S., Koren, R., Doenyas-Barak, K., and Golik, A. (2017). Metformin Does Not Induce Hyperlactatemia in Patients Admitted to Internal Medicine Ward. Isr. Med. Assoc. J. 19, 300–303.
Koreny, M., Karth, G. D., Geppert, A., Neunteufl, T., Priglinger, U., Heinz, G., et al. (2002). Prognosis of patients who develop acute renal failure during the first 24 hours of cardiogenic shock after myocardial infarction. Am. J. Med. 112, 115–119. doi: 10.1016/s0002-9343(01)01070-1
Kossaify, A., Garcia, A., Succar, S., Ibrahim, A., Moussallem, N., Kossaify, M., et al. (2013). Perspectives on the value of biomarkers in acute cardiac care and implications for strategic management. Biomark Insights 8, 115–126. doi: 10.4137/BMI.S12703
Krahe, T., Schindler, R., Neubauer, S., Ertl, G., Horn, M., and Lackner, K. (1993). [31P-cardio-MR-spectroscopy in myocardial insufficiency]. Rofo 159, 64–70. doi: 10.1055/s-2008-1032723
Krishnan, J., Suter, M., Windak, R., Krebs, T., Felley, A., Montessuit, C., et al. (2009). Activation of a HIF1alpha-PPARgamma axis underlies the integration of glycolytic and lipid anabolic pathways in pathologic cardiac hypertrophy. Cell. Metab. 9, 512–524. doi: 10.1016/j.cmet.2009.05.005
Laughlin, M. R., Taylor, J., Chesnick, A. S., DeGroot, M., and Balaban, R. S. (1993). Pyruvate and lactate metabolism in the in vivo dog heart. Am. J. Physiol. 264, H2068–H2079. doi: 10.1152/ajpheart.1993.264.6.H2068
Lazzeri, C., Sori, A., Chiostri, M., Gensini, G. F., and Valente, S. (2009). Prognostic role of insulin resistance as assessed by homeostatic model assessment index in the acute phase of myocardial infarction in nondiabetic patients submitted to percutaneous coronary intervention. Eur. J. Anaesthesiol. 26, 856–862. doi: 10.1097/EJA.0b013e32832a235c
Lazzeri, C., Valente, S., Chiostri, M., Picariello, C., and Gensini, G. F. (2010). Evaluation of acid-base balance in ST-elevation myocardial infarction in the early phase: a prognostic tool? Coron. Artery Dis. 21, 266–272. doi: 10.1097/mca.0b013e32833b20c6
Levy, B., Mansart, A., Montemont, C., Gibot, S., Mallie, J. P., Regnault, V., et al. (2007). Myocardial lactate deprivation is associated with decreased cardiovascular performance, decreased myocardial energetics, and early death in endotoxic shock. Intensive Care Med. 33, 495–502. doi: 10.1007/s00134-006-0523-9
Lewandowski, E. D. (1992). Metabolic heterogeneity of carbon substrate utilization in mammalian heart: nMR determinations of mitochondrial versus cytosolic compartmentation. Biochemistry 31, 8916–8923. doi: 10.1021/bi00152a031
Lionetti, V., Stanley, W. C., and Recchia, F. A. (2011). Modulating fatty acid oxidation in heart failure. Cardiovasc. Res. 90, 202–209. doi: 10.1093/cvr/cvr038
Lisanti, M. P., Martinez-Outschoorn, U. E., and Sotgia, F. (2013). Oncogenes induce the cancer-associated fibroblast phenotype: metabolic symbiosis and “fibroblast addiction” are new therapeutic targets for drug discovery. Cell Cycle 12, 2723–2732. doi: 10.4161/cc.25695
Liu, F., Lu, J. X., Tang, J. L., Li, L., Lu, H. J., Hou, X. H., et al. (2009). Relationship of plasma creatinine and lactic acid in type 2 diabetic patients without renal dysfunction. Chin. Med. J. 122, 2547–2553.
Lochner, A., Pentz, A., Williams, K., Tromp, E., and Harper, I. S. (1996). Substrate effects on sarcolemmal permeability in the normoxic and hypoxic perfused rat heart. Basic Res. Cardiol. 91, 64–78. doi: 10.1007/BF00788867
Locke, F. S., and Rosenheim, O. (1907). Contributions to the physiology of the isolated heart: the consumption of dextrose by mammalian cardiac muscle. J. Physiol. 36, 205–220. doi: 10.1113/jphysiol.1907.sp001229
Lommi, J., Koskinen, P., Naveri, H., Harkonen, M., and Kupari, M. (1997). Heart failure ketosis. J. Intern. Med. 242, 231–238. doi: 10.1046/j.1365-2796.1997.00187.x
Lommi, J., Kupari, M., Koskinen, P., Naveri, H., Leinonen, H., Pulkki, K., et al. (1996). Blood ketone bodies in congestive heart failure. J. Am. Coll. Cardiol. 28, 665–672. doi: 10.1016/0735-1097(96)00214-8
Lopaschuk, G. D., Ussher, J. R., Folmes, C. D., Jaswal, J. S., and Stanley, W. C. (2010). Myocardial fatty acid metabolism in health and disease. Physiol. Rev. 90, 207–258. doi: 10.1152/physrev.00015.2009
Lu, L., Ye, S., Scalzo, R. L., Reusch, J. E. B., Greyson, C. R., and Schwartz, G. G. (2017). Metformin prevents ischaemic ventricular fibrillation in metabolically normal pigs. Diabetologia 60, 1550–1558. doi: 10.1007/s00125-017-4287-2
Luptak, I., Balschi, J. A., Xing, Y., Leone, T. C., Kelly, D. P., and Tian, R. (2005). Decreased contractile and metabolic reserve in peroxisome proliferator-activated receptor-alpha-null hearts can be rescued by increasing glucose transport and utilization. Circulation 112, 2339–2346. doi: 10.1161/CIRCULATIONAHA.105.534594
Madiraju, A. K., Erion, D. M., Rahimi, Y., Zhang, X. M., Braddock, D. T., Albright, R. A., et al. (2014). Metformin suppresses gluconeogenesis by inhibiting mitochondrial glycerophosphate dehydrogenase. Nature 510, 542–546. doi: 10.1038/nature13270
Magistretti, P. J., and Allaman, I. (2015). A cellular perspective on brain energy metabolism and functional imaging. Neuron 86, 883–901. doi: 10.1016/j.neuron.2015.03.035
Mazer, C. D., Stanley, W. C., Hickey, R. F., Neese, R. A., Cason, B. A., Demas, K. A., et al. (1990). Myocardial metabolism during hypoxia: maintained lactate oxidation during increased glycolysis. Metabolism 39, 913–918. doi: 10.1016/0026-0495(90)90300-2
Mazzeo, R. S., Brooks, G. A., Schoeller, D. A., and Budinger, T. F. (1986). Disposal of blood [1-13C]lactate in humans during rest and exercise. J. Appl. Physiol. 60, 232–241. doi: 10.1152/jappl.1986.60.1.232
McClelland, G. B., and Brooks, G. A. (2002). Changes in MCT 1, MCT 4, and LDH expression are tissue specific in rats after long-term hypobaric hypoxia. J. Appl. Physiol. 92, 1573–1584. doi: 10.1152/japplphysiol.01069.2001
Meyer, C., Dostou, J. M., Welle, S. L., and Gerich, J. E. (2002a). Role of human liver, kidney, and skeletal muscle in postprandial glucose homeostasis. Am. J. Physiol. Endocrinol. Metab. 282, E419–E427. doi: 10.1152/ajpendo.00032.2001
Meyer, C., Stumvoll, M., Dostou, J., Welle, S., Haymond, M., and Gerich, J. (2002b). Renal substrate exchange and gluconeogenesis in normal postabsorptive humans. Am. J. Physiol. Endocrinol. Metab. 282, E428–E434. doi: 10.1152/ajpendo.00116.2001
Misbin, R. I., Green, L., Stadel, B. V., Gueriguian, J. L., Gubbi, A., and Fleming, G. A. (1998). Lactic acidosis in patients with diabetes treated with metformin. N. Engl. J. Med. 338, 265–266. doi: 10.1056/NEJM199801223380415
Mole, P. A., Baldwin, K. M., Terjung, R. L., and Holloszy, J. O. (1973). Enzymatic pathways of pyruvate metabolism in skeletal muscle: adaptations to exercise. Am. J. Physiol. 224, 50–54. doi: 10.1152/ajplegacy.1973.224.1.50
Moravec, J., El, Alaoui-Talibi Z, Moravec, M., and Guendouz, A. (1996). Control of oxidative metabolism in volume-overloaded rat hearts: effect of pretreatment with propionyl-L-carnitine. Adv. Exp. Med. Biol. 388, 205–212. doi: 10.1007/978-1-4613-0333-6_25
Mori, J., Alrob, O. A., Wagg, C. S., Harris, R. A., Lopaschuk, G. D., and Oudit, G. Y. (2013). ANG II causes insulin resistance and induces cardiac metabolic switch and inefficiency: a critical role of PDK4. Am. J. Physiol. Heart Circ. Physiol. 304, H1103–H1113. doi: 10.1152/ajpheart.00636.2012
Mowbray, J., and Ottaway, J. H. (1973). The effect of insulin and growth hormone on the flux of tracer from labelled lactate in perfused rat heart. Eur. J. Biochem. 36, 369–379. doi: 10.1111/j.1432-1033.1973.tb02921.x
Murashige, D., Jang, C., Neinast, M., Edwards, J. J., Cowan, A., Hyman, M. C., et al. (2020). Comprehensive quantification of fuel use by the failing and nonfailing human heart. Science 370, 364–368. doi: 10.1126/science.abc8861
Neubauer, S. (2007). The failing heart–an engine out of fuel. N. Engl. J. Med. 356, 1140–1151. doi: 10.1056/NEJMra063052
Neubauer, S., Horn, M., Cramer, M., Harre, K., Newell, J. B., Peters, W., et al. (1997). Myocardial phosphocreatine-to-ATP ratio is a predictor of mortality in patients with dilated cardiomyopathy. Circulation 96, 2190–2196. doi: 10.1161/01.cir.96.7.2190
Nguyen, H. B., Rivers, E. P., Knoblich, B. P., Jacobsen, G., Muzzin, A., Ressler, J. A., et al. (2004). Early lactate clearance is associated with improved outcome in severe sepsis and septic shock. Crit. Care Med. 32, 1637–1642. doi: 10.1097/01.ccm.0000132904.35713.a7
No Authors listed. (1998). Intensive blood-glucose control with sulphonylureas or insulin compared with conventional treatment and risk of complications in patients with type 2 diabetes (UKPDS 33). UK Prospective Diabetes Study (UKPDS) Group. Lancet 352, 837–853. doi: 10.1016/s0140-6736(98)07019-6
Nolan, J. P., Neumar, R. W., Adrie, C., Aibiki, M., Berg, R. A., Bottiger, B. W., et al. (2008). Post-cardiac arrest syndrome: epidemiology, pathophysiology, treatment, and prognostication. A Scientific Statement from the International Liaison Committee on Resuscitation; the American Heart Association Emergency Cardiovascular Care Committee; the Council on Cardiovascular Surgery and Anesthesia; the Council on Cardiopulmonary, Perioperative, and Critical Care; the Council on Clinical Cardiology; the Council on Stroke. Resuscitation 79, 350–379. doi: 10.1016/j.resuscitation.2008.09.017
Ong, S. B., Subrayan, S., Lim, S. Y., Yellon, D. M., Davidson, S. M., and Hausenloy, D. J. (2010). Inhibiting mitochondrial fission protects the heart against ischemia/reperfusion injury. Circulation 121, 2012–2022. doi: 10.1161/CIRCULATIONAHA.109.906610
Owen, M. R., Doran, E., and Halestrap, A. P. (2000). Evidence that metformin exerts its anti-diabetic effects through inhibition of complex 1 of the mitochondrial respiratory chain. Biochem. J. 348, 607–614. doi: 10.1042/0264-6021:3480607
Palmer, J. W., Tandler, B., and Hoppel, C. L. (1977). Biochemical properties of subsarcolemmal and interfibrillar mitochondria isolated from rat cardiac muscle. J. Biol. Chem. 252, 8731–8739. doi: 10.1016/s0021-9258(19)75283-1
Paolisso, G., Gambardella, A., Galzerano, D., D’Amore, A., Rubino, P., Verza, M., et al. (1994). Total-body and myocardial substrate oxidation in congestive heart failure. Metabolism 43, 174–179. doi: 10.1016/0026-0495(94)90241-0
Park, J. M., Josan, S., Mayer, D., Hurd, R. E., Chung, Y., Bendahan, D., et al. (2015). Hyperpolarized 13C NMR observation of lactate kinetics in skeletal muscle. J. Exp. Biol. 218, 3308–3318. doi: 10.1242/jeb.123141
Passarella, S., Paventi, G., and Pizzuto, R. (2014). The mitochondrial L-lactate dehydrogenase affair. Front. Neurosci. 8:407. doi: 10.3389/fnins.2014.00407
Peterson, L. R., Herrero, P., McGill, J., Schechtman, K. B., Kisrieva-Ware, Z., Lesniak, D., et al. (2008). Fatty acids and insulin modulate myocardial substrate metabolism in humans with type 1 diabetes. Diabetes 57, 32–40. doi: 10.2337/db07-1199
Peterson, L. R., Herrero, P., Schechtman, K. B., Racette, S. B., Waggoner, A. D., Kisrieva-Ware, Z., et al. (2004). Effect of obesity and insulin resistance on myocardial substrate metabolism and efficiency in young women. Circulation 109, 2191–2196. doi: 10.1161/01.CIR.0000127959.28627.F8
Popinigis, J., Antosiewicz, J., Crimi, M., Lenaz, G., and Wakabayashi, T. (1991). Human skeletal muscle: participation of different metabolic activities in oxidation of L-lactate. Acta Biochim. Pol. 38, 169–175.
Puckett, S. W., and Reddy, W. J. (1979). A decrease in the malate–aspartate shuttle and glutamate translocase activity in heart mitochondria from alloxan-diabetic rats. J. Mol. Cell. Cardiol. 11, 173–187. doi: 10.1016/0022-2828(79)90462-0
Rabinowitz, J. D., and Enerback, S. (2020). Lactate: the ugly duckling of energy metabolism. Nat. Metab. 2, 566–571. doi: 10.1038/s42255-020-0243-4
Ramasamy, R., Oates, P. J., and Schaefer, S. (1997). Aldose reductase inhibition protects diabetic and nondiabetic rat hearts from ischemic injury. Diabetes 46, 292–300. doi: 10.2337/diab.46.2.292
Randle, P. J. (1995). Metabolic fuel selection: general integration at the whole-body level. Proc. Nutr. Soc. 54, 317–327. doi: 10.1079/pns19950057
Randle, P. J. (1998). Regulatory interactions between lipids and carbohydrates: the glucose fatty acid cycle after 35 years. Diabetes Metab. Rev. 14, 263–283. doi: 10.1002/(sici)1099-0895(199812)14:4<263::aid-dmr233<3.0.co;2-c
Randle, P. J., Garland, P. B., Hales, C. N., and Newsholme, E. A. (1963). The glucose fatty-acid cycle. Its role in insulin sensitivity and the metabolic disturbances of diabetes mellitus. Lancet 1, 785–789. doi: 10.1016/s0140-6736(63)91500-9
Reaven, G. M., Hollenbeck, C., Jeng, C. Y., Wu, M. S., and Chen, Y. D. (1988). Measurement of plasma glucose, free fatty acid, lactate, and insulin for 24 h in patients with NIDDM. Diabetes 37, 1020–1024. doi: 10.2337/diab.37.8.1020
Revelly, J. P., Tappy, L., Martinez, A., Bollmann, M., Cayeux, M. C., Berger, M. M., et al. (2005). Lactate and glucose metabolism in severe sepsis and cardiogenic shock. Crit. Care Med. 33, 2235–2240. doi: 10.1097/01.ccm.0000181525.99295.8f
Riehle, C., and Abel, E. D. (2016). Insulin Signaling and Heart Failure. Circ. Res. 118, 1151–1169. doi: 10.1161/CIRCRESAHA.116.306206
Roth, D. A., and Brooks, G. A. (1990). Lactate and pyruvate transport is dominated by a pH gradient-sensitive carrier in rat skeletal muscle sarcolemmal vesicles. Arch. Biochem. Biophys. 279, 386–394. doi: 10.1016/0003-9861(90)90506-t
Russell, R. R. III, Li, J., Coven, D. L., Pypaert, M., Zechner, C., Palmeri, M., et al. (2004). AMP-activated protein kinase mediates ischemic glucose uptake and prevents postischemic cardiac dysfunction, apoptosis, and injury. J. Clin. Invest. 114, 495–503. doi: 10.1172/JCI19297
Sahlin, K., Fernstrom, M., Svensson, M., and Tonkonogi, M. (2002). No evidence of an intracellular lactate shuttle in rat skeletal muscle. J. Physiol. 541, 569–574. doi: 10.1113/jphysiol.2002.016683
Sampson, M. J., Davies, I. R., Braschi, S., Ivory, K., and Hughes, D. A. (2003). Increased expression of a scavenger receptor (CD36) in monocytes from subjects with Type 2 diabetes. Atherosclerosis 167, 129–134. doi: 10.1016/s0021-9150(02)00421-5
Savarese, G., and Lund, L. H. (2017). Global Public Health Burden of Heart Failure. Card. Fail. Rev. 3, 7–11. doi: 10.15420/cfr.2016:25:2
Schurr, A., Payne, R. S., Miller, J. J., and Rigor, B. M. (1997). Brain lactate is an obligatory aerobic energy substrate for functional recovery after hypoxia: further in vitro validation. J. Neurochem. 69, 423–426. doi: 10.1046/j.1471-4159.1997.69010423.x
Shen, Y., Liu, F., Li, Q., Tang, J., Zheng, T., Lu, F., et al. (2012). The gonadal hormone regulates the plasma lactate levels in type 2 diabetes treated with and without metformin. Diabetes Technol. Ther. 14, 469–474. doi: 10.1089/dia.2011.0275
Sinniah, B. (1978). Hymenolepis diminuta infection in a Malaysian oil palm estate worker–first case from Malaysia. Southeast Asian J. Trop. Med. Public Health 9, 453–454.
Smeele, K. M., Ter, Horst LH, Koeman, A., Heikkinen, S., Laakso, M., and Weber, N. C. (2011). The effect of standard chow and reduced hexokinase II on growth, cardiac and skeletal muscle hexokinase and low-flow cardiac ischaemia-reperfusion injury. Lab. Anim. 45, 160–166. doi: 10.1258/la.2011.010096
Sonveaux, P., Vegran, F., Schroeder, T., Wergin, M. C., Verrax, J., Rabbani, Z. N., et al. (2008). Targeting lactate-fueled respiration selectively kills hypoxic tumor cells in mice. J. Clin. Invest. 118, 3930–3942. doi: 10.1172/JCI36843
Stanley, W. C. (2001). Cardiac energetics during ischaemia and the rationale for metabolic interventions. Coron. Artery Dis. 12, S3–S7.
Stanley, W. C., Gertz, E. W., Wisneski, J. A., Morris, D. L., Neese, R. A., and Brooks, G. A. (1985). Systemic lactate kinetics during graded exercise in man. Am. J. Physiol. 249, E595–E602. doi: 10.1152/ajpendo.1985.249.6.E595
Stanley, W. C., Gertz, E. W., Wisneski, J. A., Neese, R. A., Morris, D. L., and Brooks, G. A. (1986). Lactate extraction during net lactate release in legs of humans during exercise. J. Appl. Physiol. 60, 1116–1120. doi: 10.1152/jappl.1986.60.4.1116
Stanley, W. C., Morgan, E. E., Huang, H., McElfresh, T. A., Sterk, J. P., Okere, I. C., et al. (2005). Malonyl-CoA decarboxylase inhibition suppresses fatty acid oxidation and reduces lactate production during demand-induced ischemia. Am. J. Physiol. Heart Circ. Physiol. 289, H2304–H2309. doi: 10.1152/ajpheart.00599.2005
Symons, J. D., and Abel, E. D. (2013). Lipotoxicity contributes to endothelial dysfunction: a focus on the contribution from ceramide. Rev. Endocr. Metab. Disord. 14, 59–68. doi: 10.1007/s11154-012-9235-3
Tempia, A., Fiore, G., Berardino, M., Aimone-Secat, M., Ballaris, M. A., and Pattono, R. (1988). [Use of 3 different anesthetic mixtures in peridural anesthesia for extracorporeal shockwave lithotripsy (ESWL)]. Minerva. Anestesiol. 54, 315–320.
Timmermans, A. D., Balteau, M., Gelinas, R., Renguet, E., Ginion, A., de Meester, C., et al. (2014). A-769662 potentiates the effect of other AMP-activated protein kinase activators on cardiac glucose uptake. Am. J. Physiol. Heart Circ. Physiol. 306, H1619–H1630. doi: 10.1152/ajpheart.00965.2013
Trueblood, N. A., Ramasamy, R., Wang, L. F., and Schaefer, S. (2000). Niacin protects the isolated heart from ischemia-reperfusion injury. Am. J. Physiol. Heart Circ. Physiol. 279, H764–H771. doi: 10.1152/ajpheart.2000.279.2.H764
Trueblood, N., and Ramasamy, R. (1998). Aldose reductase inhibition improves altered glucose metabolism of isolated diabetic rat hearts. Am. J. Physiol. 275, H75–H83. doi: 10.1152/ajpheart.1998.275.1.H75
Ussher, J. R., and Lopaschuk, G. D. (2008). The malonyl CoA axis as a potential target for treating ischaemic heart disease. Cardiovasc. Res. 79, 259–268. doi: 10.1093/cvr/cvn130
Valente, S., Lazzeri, C., Crudeli, E., Chiostri, M., Giglioli, C., Bernardo, P., et al. (2012). Intraaortic balloon pump: incidence and predictors of complications in the Florence registry. Clin. Cardiol. 35, 200–204. doi: 10.1002/clc.20975
Vanoverschelde, J. L., Janier, M. F., Bakke, J. E., Marshall, D. R., and Bergmann, S. R. (1994). Rate of glycolysis during ischemia determines extent of ischemic injury and functional recovery after reperfusion. Am. J. Physiol. 267, H1785–H1794. doi: 10.1152/ajpheart.1994.267.5.H1785
Vermeulen, R. P., Hoekstra, M., Nijsten, M. W., van der Horst, I. C., van Pelt, L. J., Jessurun, G. A., et al. (2010). Clinical correlates of arterial lactate levels in patients with ST-segment elevation myocardial infarction at admission: a descriptive study. Crit. Care 14, R164. doi: 10.1186/cc9253
Weil, M. H., and Afifi, A. A. (1970). Experimental and clinical studies on lactate and pyruvate as indicators of the severity of acute circulatory failure (shock). Circulation 41, 989–1001. doi: 10.1161/01.cir.41.6.989
Wilson, M. C., Jackson, V. N., Heddle, C., Price, N. T., Pilegaard, H., Juel, C., et al. (1998). Lactic acid efflux from white skeletal muscle is catalyzed by the monocarboxylate transporter isoform MCT3. J. Biol. Chem. 273, 15920–15926. doi: 10.1074/jbc.273.26.15920
Wisneski, J. A., Gertz, E. W., Neese, R. A., Gruenke, L. D., Morris, D. L., and Craig, J. C. (1985). Metabolic fate of extracted glucose in normal human myocardium. J. Clin. Invest. 76, 1819–1827. doi: 10.1172/JCI112174
Wisniewski, J. R., Gizak, A., and Rakus, D. (2015). Integrating Proteomics and Enzyme Kinetics Reveals Tissue-Specific Types of the Glycolytic and Gluconeogenic Pathways. J. Proteome. Res. 14, 3263–3273. doi: 10.1021/acs.jproteome.5b00276
Wolfe, R. R. (1990). Isotopic measurement of glucose and lactate kinetics. Ann. Med. 22, 163–170. doi: 10.3109/07853899009147263
Wu, R., Smeele, K. M., Wyatt, E., Ichikawa, Y., Eerbeek, O., Sun, L., et al. (2011). Reduction in hexokinase II levels results in decreased cardiac function and altered remodeling after ischemia/reperfusion injury. Circ. Res. 108, 60–69. doi: 10.1161/CIRCRESAHA.110.223115
Young, M. E., Guthrie, P. H., Razeghi, P., Leighton, B., Abbasi, S., Patil, S., et al. (2002). Impaired long-chain fatty acid oxidation and contractile dysfunction in the obese Zucker rat heart. Diabetes 51, 2587–2595. doi: 10.2337/diabetes.51.8.2587
Zhabyeyev, P., Gandhi, M., Mori, J., Basu, R., Kassiri, Z., Clanachan, A., et al. (2013). Pressure-overload-induced heart failure induces a selective reduction in glucose oxidation at physiological afterload. Cardiovasc. Res. 97, 676–685. doi: 10.1093/cvr/cvs424
Zhang, L., Jaswal, J. S., Ussher, J. R., Sankaralingam, S., Wagg, C., Zaugg, M., et al. (2013). Cardiac insulin-resistance and decreased mitochondrial energy production precede the development of systolic heart failure after pressure-overload hypertrophy. Circ. Heart Fail. 6, 1039–1048. doi: 10.1161/CIRCHEARTFAILURE.112.000228
Keywords: myocardium, cardiac metabolism, energy substrate, lactate, lactate shuttle theory, myocardial ischemia, heart failure, diabetic cardiomyopathy
Citation: Dong S, Qian L, Cheng Z, Chen C, Wang K, Hu S, Zhang X and Wu T (2021) Lactate and Myocardiac Energy Metabolism. Front. Physiol. 12:715081. doi: 10.3389/fphys.2021.715081
Received: 26 May 2021; Accepted: 29 July 2021;
Published: 17 August 2021.
Edited by:
Alex Rafacho, Federal University of Santa Catarina, BrazilReviewed by:
Alexandra Latini, Federal University of Santa Catarina, BrazilHenver Simionato Brunetta, State University of Campinas, Brazil
Copyright © 2021 Dong, Qian, Cheng, Chen, Wang, Hu, Zhang and Wu. This is an open-access article distributed under the terms of the Creative Commons Attribution License (CC BY). The use, distribution or reproduction in other forums is permitted, provided the original author(s) and the copyright owner(s) are credited and that the original publication in this journal is cited, in accordance with accepted academic practice. No use, distribution or reproduction is permitted which does not comply with these terms.
*Correspondence: Xiang Zhang, xiang.zhang02@hotmail.com