- 1Department of Plant Medicals, Andong National University, Andong, South Korea
- 2Department of Horticulture and Breeding, Andong National University, Andong, South Korea
- 3Department of Horticultural Science, Kyungpook National University, Daegu, South Korea
Salicylic acid is a plant hormone that can mediate various plant physiological processes. Salicylic acid can bind to human high mobility group box 1 (HMGB1) and interrupt its role in mediating immune responses. Dorsal switch protein 1 (DSP1) is an insect homolog of HMGB1. In this study, a DSP1 (Se-DSP1) encoded in Spodoptera exigua, a phytophagous insect, was characterized, and its potential role in immune response was explored. Upon bacterial challenge, Se-DSP1 was localized in the nucleus and released into the hemolymph. The released Se-DSP1 could mediate both cellular and humoral immune responses by activating eicosanoid biosynthesis. Salicylic acid could bind to Se-DSP1 with a high affinity. The immune responses of S. exigua were significantly interrupted by SA feeding. Larvae reared on tomatoes with high endogenous SA levels became more susceptible to entomopathogens. Taken together, these results suggest a tritrophic defensive role of plant SA against phytophagous insects.
Introduction
High-mobility group proteins are non-histone DNA-binding proteins in eukaryotes. These high-mobility group (HMG) proteins include at least three unrelated protein groups: HMGA, HMGB, and HMGN (Reeves, 2003). High-mobility group A proteins bind to A/T-rich DNA sequences and promote the binding of other transcription factors, while HMGN proteins bind to nucleosomes and promote chromatin decondensation (Bustin, 2001). On the other hand, HMGB proteins are characterized by a DNA-binding box with an acidic tail that can non-specifically bind to DNA and induce sharp bends, allowing the easy accession of transcription factors and chromatin remodeling complexes (Thomas and Travers, 2001). In mammals, four HMGB proteins (HMGB1–4) are known. They are ubiquitously expressed during embryogenesis, although they show different spatial expression patterns in the adult stage (Stros, 2010).
Human high mobility group box 1 is a ubiquitously expressed and highly conserved nuclear protein that plays important role in the chromatin organization and transcriptional regulation in mammals (Bianchi et al., 2017). It was first discovered in the calf thymus as a chromatin-associated protein with high acidic and basic amino acid contents (Goodwin and Johns, 1977). Furthermore, HMGB1 consists of two HMG boxes (boxes A and B) and a long acidic tail (Stros et al., 2007). The two HMG boxes have different functions and properties: box A recognizes pre-bent and linear DNA (Muller et al., 2001), while box B binds to mini-circular DNA or bending linear DNA (Webb et al., 2001). Additional motifs have been found in HMGB1. For example, a coiled coil region can mediate protein–protein interactions for the transcriptional control of target genes through nuclear receptors (Parry et al., 2008). A low complexity region contains repeats of single or short amino acid repeat motifs. It plays various functional roles in modulating protein–protein interactions, protein–nucleic acid interactions, and protein subcellular localization (Salichs et al., 2009).
High mobility group box 1 can be released under stress either passively from dead cells (Bianchi et al., 2017) or actively secreted from activated immune cells, enterocytes, hepatocytes, and, possibly, several other cellular types (Tsung et al., 2007). Released HMGB1 can act as a damage-associated molecular pattern (DAMP) and activate the innate immune system by interacting with pattern recognition receptors (Jong and Dong, 2018).
The dorsal switch protein 1 of Drosophila melanogaster is a homolog of HMGB1 first identified in insects (Mosrin-Huaman et al., 1998). In addition to HMGB1 signature motifs, dorsal switch protein 1 (DSP1) possesses two glutamine-rich domains at its N-terminus (Canaple et al., 1997). The null mutants of DSP1 exhibit homeotic transformations associated with the downregulation of Sex combs-reduced expression (Decoville et al., 2001). Furthermore, DSP1 acts as a transcription factor and a chromatin remodeling factor by enhancing other transcriptional factors such as the Hox protein (Zappavigna et al., 1996), p53 (Jayaraman et al., 1998), and steroid hormone receptor (Boonyaratanakornkit et al., 1998). Another HMGB1 homolog has been found in a mosquito, Aedes aegypti. It shows high homologies with HMGB1 and Drosophila DSP1 (Ribeiro et al., 2012). This mosquito HMGB1 can facilitate the transcriptional factor Rel to bind to the nuclear factor kappa B (NF-kB) promoter to express antiviral genes against a dengue viral infection (de Mendonça Amarante et al., 2017). In addition to dipteran insects, a lepidopteran insect (Plodia interpunctella) also possesses HMGB1-like proteins (Aleporou-Marinou et al., 2003). Thus, HMGB1 might be highly conserved in insects as in mammals and play crucial roles in the immune responses of insects by contributing to chromatin organization and transcriptional regulation. However, little is known about the role of HMGB1 as a DAMP and in subsequent immunity-related responses in insects.
Salicylic acid is a plant hormone that can mediate plant defense against microbial pathogens. It can also regulate plant growth and development (Koo et al., 2020). In particular, the salicylic acid (SA) signal is linked to a resistance to biotrophic microbial pathogens by inducing the expression of pathogenesis-related genes that encode antimicrobial or other defensive proteins and by upregulating reactive oxygen species (Ton et al., 2006). With respect to insect resistance, plants can use the SA signal against phloem-feeding insects, although the efficacy of SA is controversial (Zarate et al., 2007). Little information exists about the SA signal in interactions between plants and chewing insects such as lepidopterans (Wu and Baldwin, 2009). Meanwhile, HMGB1 can bind to SA and lose its proinflammatory activity (Choi et al., 2015). The fact that SA is a metabolite of aspirin (= acetylated SA) in humans suggests a novel non-steroidal anti-inflammatory drug (NSAID) action of aspirin, which is known to inhibit cyclooxygenase 2 (COX-2) to shutdown inflammatory prostaglandin (PG) production (Choi et al., 2015). Cyclooxygenase inhibitors such as aspirin are effective in inhibiting insect immune responses (Stanley and Kim, 2011). We hypothesized that plant SA could participate in plant defense against phytophagous insects by interacting with insect HMGB1 homologs. Thus, it might lead to the suppression of insect immune responses against microbial entomopathogens. Thus, the objective of this study was to perform a functional characterization of DSP1 (Se-DSP1) in the lepidopteran insect Spodoptera exigua and subsequent analyses of Se-DSP1 to determine its functional association with plant SA. Our results propose a novel plant defense mechanism utilizing SA against insect pests so that such insects could be susceptible to microbial entomopathogens by interfering with their immune systems.
Materials and Methods
Insect Rearing and Bacteria Culture
The larvae of S. exigua were reared using the method of Goh et al. (1990) at 25 ± 1°C, with which the larvae underwent five instars (“L1–L5”). For the immune challenge, Escherichia coli Top10 (Invitrogen, Carlsbad, CA, United States) were cultured with the method described previously (Hasan et al., 2019). Bacterial cells were resuspended in distilled water to have 4.5 × 104 cells/μl for treatment. For a pathogenicity test, Bacillus thuringiensis subsp. aizawai (Bt) and Xenorhabdus hominickii ANU101 (Xh) were cultured with the method described previously (Mollah et al., 2020a).
Chemicals
An anticoagulant buffer (ACB) was prepared with 186 mM of NaCl, 17 mM of Na2EDTA, and 41 of mM citric acid, and then the pH was adjusted to 4.5 with HCl. Phosphate-buffered saline (PBS) was prepared with 100 mM of phosphoric acid, and the pH was adjusted to 7.4. The transfection reagent Metafectene Pro was purchased from Biontex (Plannegg, Germany). Salicylic acid (2-hydroxybenzoic acid: SA, ≥99%) was obtained from Sigma-Aldrich (Seoul, Korea) and dissolved in dimethylsulfoxide.
Bioinformatics and Sequence Analysis
A sequence (Se-DSP1) of S. exigua homologous to human HMGB1 (GenBank accession number: CAG33144.1) was obtained from a transcriptome (GenBank accession number: GAFU01017850.1). Its open reading frame (ORF) was deposited at GenBank (accession number: MK737894). Phylogenetic and domain analyses were performed using the MEGA6 and Clustal W programs from the European Molecular Biology Laboratory's European Bioinformatics Institute (EMBL-EBI) (www.ebi.ac.uk). Bootstrapping values were obtained with 1,000 repetitions to support branches. Protein domains were predicted using the SMART (http://smart.embl-heidelberg.de/) search program.
RNA Extraction and RT-qPCR
Total RNA extraction, complementary DNA (cDNA) preparation, and quantitative reverse transcription (RT-q)PCR were performed following the methods described previously (Mollah et al., 2020a,b). The synthesized first-stranded cDNA was used as a template for PCR amplification with 35 cycles of 94°C for 30 s, 58.8°C for 30 s, and 72°C for 30 s after an initial heat treatment at 94°C for 2 min with the gene-specific primers listed in Supplementary Table S2.
RNA Interference (RNAi) of Se-DSP1 Expression
The DNA template was amplified with the gene-specific primers of Se-DSP1 (Supplementary Table S2) containing a T7 promoter sequence (5′-TAATACGACTCACTATAGGGAGA-3′) at the 5′ end. Double-stranded RNA (dsRNA) was prepared following the method described previously (Mollah et al., 2020b). A viral gene, CpBV302, was used as a control dsRNA (“dsCON”).
Analyses of Immune Responses
For a cellular immune assay, hemocytic nodules were assessed with the method described previously (Mollah et al., 2020a). For humoral immune response, the expression levels of six antimicrobial peptide (AMP) genes (Supplementary Table S2) were assessed with the RT-qPCR method as described above.
Purification of Recombinant Se-DSP1 and SA Binding Affinity
The ORF of Se-DSP1 was cloned into a pET expression vector (Invitrogen, Carlsbad, CA, United States). After overexpression with isopropyl β-D-thiogalactopyranoside, the recombinant protein containing a 6×His tag was purified with the method described previously (Mollah et al., 2021). Resulting proteins contained partial degraded fragments, which were removed by gel filtration chromatography with Sephadex G-100 resin (Sigma-Aldrich, Seoul, Korea). To detect the binding of Se-DSP1 to SA, a thermal shift assay (Simeonov, 2013) was performed with a Protein Thermal Shift (Applied Biosystem, Foster City, CA, United States) dye kit according to the instructions of the manufacturer. Briefly, a reaction mixture consisted of 5 μl of a protein thermal shift buffer, 2.5 μl of protein thermal shift dye (8×, prepared by kit), 10 μl (500 ng) of purified protein (Se-DSP1), and 2.5 μl of ligands at different final concentrations (0, 5, 10, 15, and 20 μM). A melting curve experiment type was set up using the StepOne Real Time PCR System (Applied Biosystems, Foster City, CA, United States) with a continuous ramping mode (2.96°C/min), at which two thermal extremes were 25°C for 2 min at the initial step and 99°C for 2 min at the final step. Melting temperatures resulting from the experiment were plotted with SigmaPlot 10.0 (Systat Software, San Jose, CA, United States). A dissociation constant (Kd) was calculated using the ligand binding equation category.
Phospholipase A2 (PLA2) Enzyme Activity, Western Blotting, and Immunofluorescence Staining
Phospholipase A2 enzyme activity was measured with a PLA2 Assay Kit (Cayman Chemical, Ann Arbor, MI, United States) using diheptanoyl thio-phosphatidyl choline for the secretory phospholipase A2 (sPLA2) substrate and arachidonyl thio-phosphatidyl choline for the cellular PLA2 (cPLA2) substrate after sample preparation as described previously (Vatanparast et al., 2018). Briefly, the L5 larvae of S. exigua were injected with dsDSP1 and dsCON (1 μg/larva) using a microsyringe (Hamilton, Reno, NV, United States), incubated at room temperature for 24 h, and then challenged with E. coli (104 cells/larva). At 8 h post injection of the bacteria, fat body was collected. The reaction volume consisted of 10 μl of fat body extract, 10 μl of 5,5-dithio-bis-(2-nitrobenzoic acid), 5 μl of the assay buffer, and 200 μl of the substrate. The change in absorbance at 405 nm was monitored with three biologically independent replications.
For the Western blot, hemolymph samples were collected from the L5 larvae of S. exigua. After centrifugation with 800 × g for 3 min at 4°C, the supernatant plasma samples were obtained and used for the analysis. A polyclonal antibody against rat HMGB1 (NB100-2322; Abcam, Waltham, MA, United States) was used after 5,000 times of dilution. α-Tubulin was used as a reference protein and detected by a polyclonal antibody (GTX112141; GeneTex, Irvine, CA, United States) after 1,000 times of dilution. The primary antibodies were detected with an anti-rabbit immunoglobulin G (IgG)–alkaline phosphatase secondary antibody (WP20007; Sigma-Aldrich, Seoul, Korea) after incubation with a substrate (BICP/NBT; Sigma-Aldrich, Seoul, Korea). This antibody showed a specific cross-reactivity with Se-DSP1 (Supplementary Figure S1).
An immunofluorescence assay (IFA) was performed according to the method described previously (Mollah et al., 2021). Briefly, hemocytes and fat body tissues were collected from the L5 larvae of S. exigua. Furthermore, Se-DSP1 was observed with the HMGB1 antibody. The cytoplasm and nucleus were stained with Alexa Fluor 555 phalloidin and 4′,6-diamidino-2-phenylindole (DAPI, 1 μg/ml) (Thermo Fisher Scientific, Rockford, IL, United States), respectively. Subsequently, cells were observed under a fluorescence microscope (DM2500; Leica, Wetzlar, Germany) at 400× magnification under microscope parameter conditions (Supplementary Table S3).
Bioassay for Bacterial Pathogens
To determine Bt virulence, the L4 larvae were subjected to feeding assays using a leaf-dipping method. Briefly, a piece of cabbage leaf (3 × 3 cm) was soaked in 250 ppm of a Bt suspension containing different concentrations of SA for 5 min. The treated leaves were then provided to larvae to allow feeding for 24 h. To determine Xh virulence, the L4 larvae were subjected to injecting assays. Each larva was injected with 100 live bacterial cells containing different concentrations of SA using a microsyringe (Hamilton, Reno, NV, United States). The treated larvae were then incubated for another 4 days under rearing conditions, and mortality was monitored. Each replication consisted of 10 larvae. Each treatment was replicated three times.
Effect of Tomato Plants on Susceptibility of S. exigua to Bacterial Pathogens
Two lines of Solanum esculantum, “M82” and “IL2-2,” were used. Seeds were obtained from the Tomato Genetics Resource Center at the University of California (Davis CA, United States) (http://tgrc.ucdavis.edu/). For the bioassays, the L2 larvae of S. exigua were reared on 4-week-old tomato plants for 7 days with a 16-h light/8-h dark period at a temperature of 25 ± 2°C. The larvae were then used to assess immune responses indicated by nodule formation and AMP expression, as described above. The larvae were also used to determine bacterial virulence, as described above.
Quantification of SA in Tomato Leaves
Approximately 300 mg of leaf tissues were collected from each line of tomato plants, and then extracted and analyzed for SA levels, as described previously (Bowling et al., 1994). The SA concentration was determined by high-performance liquid chromatography (HPLC) with an ARH-601 organic acid column (100 × 6.5 mm; Transgenomic Inc., Omaha, NE, United States) run at 45°C in.01 N of H2SO4 and a flow rate of 0.6 ml/min.
Results
Prediction of a DSP1 Homolog (Se-DSP1) From S. exigua
By interrogation with a D. melanogaster DSP1 sequence (GenBank accession number: NP_727960.1) as a query, Se-DSP1 was predicted from a transcriptome (GenBank accession number: GAFU 01017850.1). Its ORF consisted of 1,518 bp encoding 505 amino acids. Se-DSP1 shared 95.1, 77.1, 73, and 52.1% amino acid sequence similarities with the DSP genes of Helicoverpa armigera, Leptinotarsa decemlineata, D. melanogaster, and the HMGB1 of Homo sapiens, respectively. A phylogenetic analysis showed that Se-DSP1 was closely related to other lepidopterans within an insect cluster (Supplementary Figure S2A). Like mammalian HMGB1, Se-DSP1 comprised HMG boxes A and B and an acidic tail (Supplementary Figure S2B). Phosphorylation and disulfide linkage sites are frequently located in box A compared with other domains. In the two HMG boxes, human HMGB1 and Se-DSP1 exhibited higher sequence identities (69.9%) in box A than in box B (39.4%). Unlike mammalian HMGB1s, insect DSP1s possess additional N-terminal domains such as a coiled coil, low complexity, and RNase.
Expression Profile of Se-DSP1
In all developmental stages, Se-DSP1 was expressed showing high expression levels at the L5 and adult stages. It had low expression levels at the pupal stage (Supplementary Figure S3A). At the L5 larval stage, Se-DSP1 was expressed in all the tested tissues such as hemocytes, fat body, gut, and epidermis (Supplementary Figure S3B). The basal expression levels of Se-DSP1 were significantly upregulated following the immune challenge with E. coli in hemocytes and the fat body (Supplementary Figure S3C). Such an upregulation was more acute in hemocytes than in the fat body.
Translocation of Se-DSP1 Upon Immune Challenge
In naïve larvae, Se-DSP1 was localized in the nuclei of the hemocytes (Figure 1A). Upon bacterial challenge, some Se-DSP1 proteins migrated to the cytosol. Similarly, Se-DSP1 was localized in nuclei of the fat body and isolated from naïve larvae, although some of them were detected in the cytosol after the immune challenge (Figure 1B). Interestingly, Se-DSP1 was detected in the plasma of the immune-challenged larvae, although it was slightly detected in the plasma collected from the naïve larvae (Figure 1C).
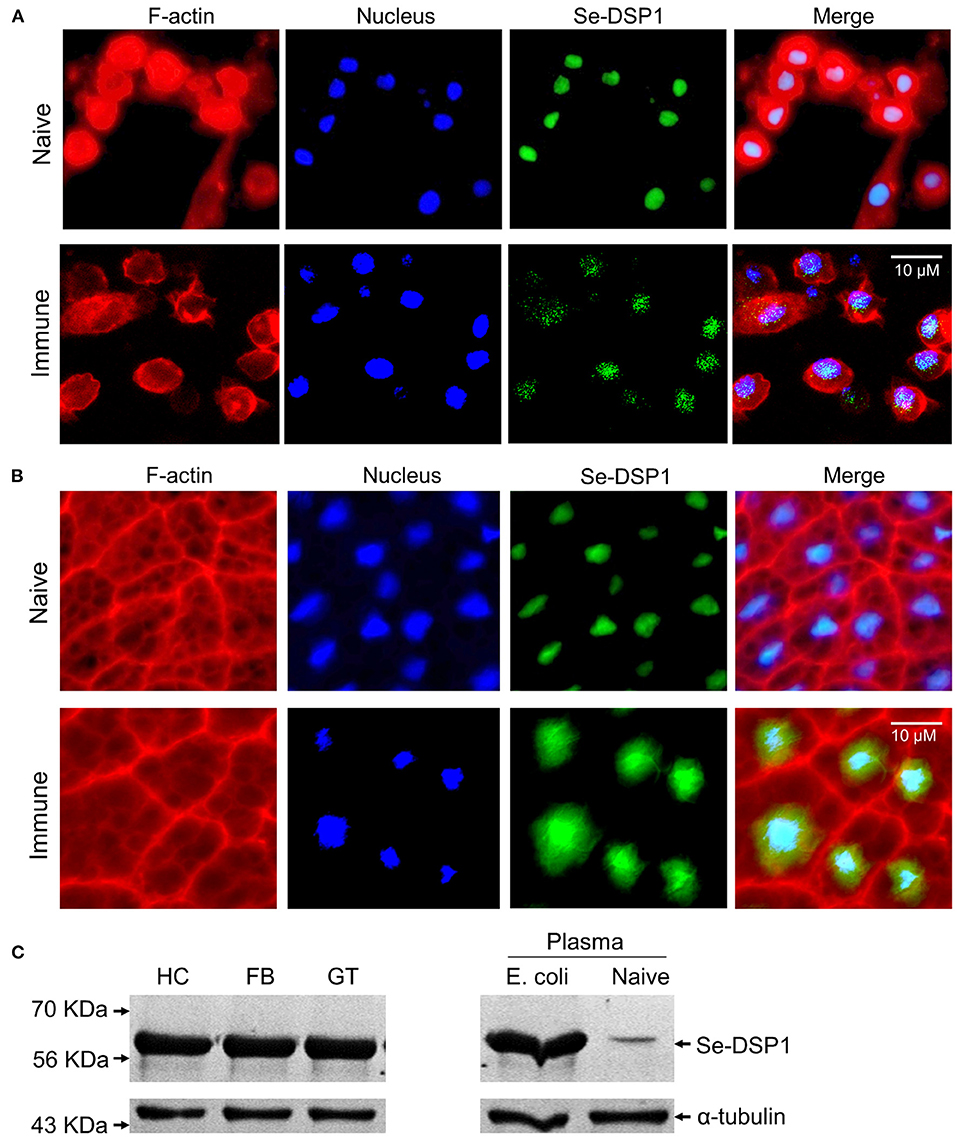
Figure 1. The release of dorsal switch protein 1 (DSP1) Spodoptera exigua (Se-DSP1) proteins from nuclei in response to an immune challenge. (A) The mobilization of Se-DSP1 in hemocytes. (B) The mobilization of Se-DSP1 in the fat body. Cytosol and nuclei were stained with phalloidin against F-actin and 4′,6-diamidino-2-phenylindole (DAPI) against nuclear DNA. Se-DSP1 was detected with a polyclonal antibody raised against mammalian high mobility group box 1 (HMGB1). Supplementary Table S3 describes the setting values of the fluorescence microscope to get the immunofluorescence images. (C) Western blotting against different tissue proteins of hemocyte (“HC”), fat body (“FB”), and midgut (“GT”). For the immune challenge, each of the L5 larvae were injected with 4.5 × 104 heat-killed (HK) Escherichia coli cells. Plasma samples were collected 6 h post injection (PI). A homogenous immunoblotting intensity against α-tubulin indicates the equal amount of protein sample loading in each lane.
RNAi of Se-DSP1 Expression Results in Immunosuppression
To analyze the role of Se-DSP1 in association with immune responses, its expression was manipulated by RNAi with gene-specific dsRNA (Figure 2A). Such an RNAi treatment significantly reduced Se-DSP1 expression levels by more than 90% in the hemocytes and fat body at 24 h. The reduced mRNA levels were confirmed by weaker bands in Western blotting against Se-DSP1. At such a reduced expression level of DSP1, cellular immune response was measured by counting hemocytic nodules (= a cellular immune response sequestering small pathogens with hemocytes and subsequent melanization) after the bacterial challenge (Figure 2B). The RNAi-treated larvae exhibited a significant (P < 0.05) impairment in cellular immune response based on nodule formation. Furthermore, the bacterial challenge upregulated the gene expression levels of six AMPs in the control larvae (Figure 2C). The reduction of AMP gene expression in the RNAi-treated larvae was significant (F = 997; df = 1, 144; P < 0.0001) compared with that in the control larvae.
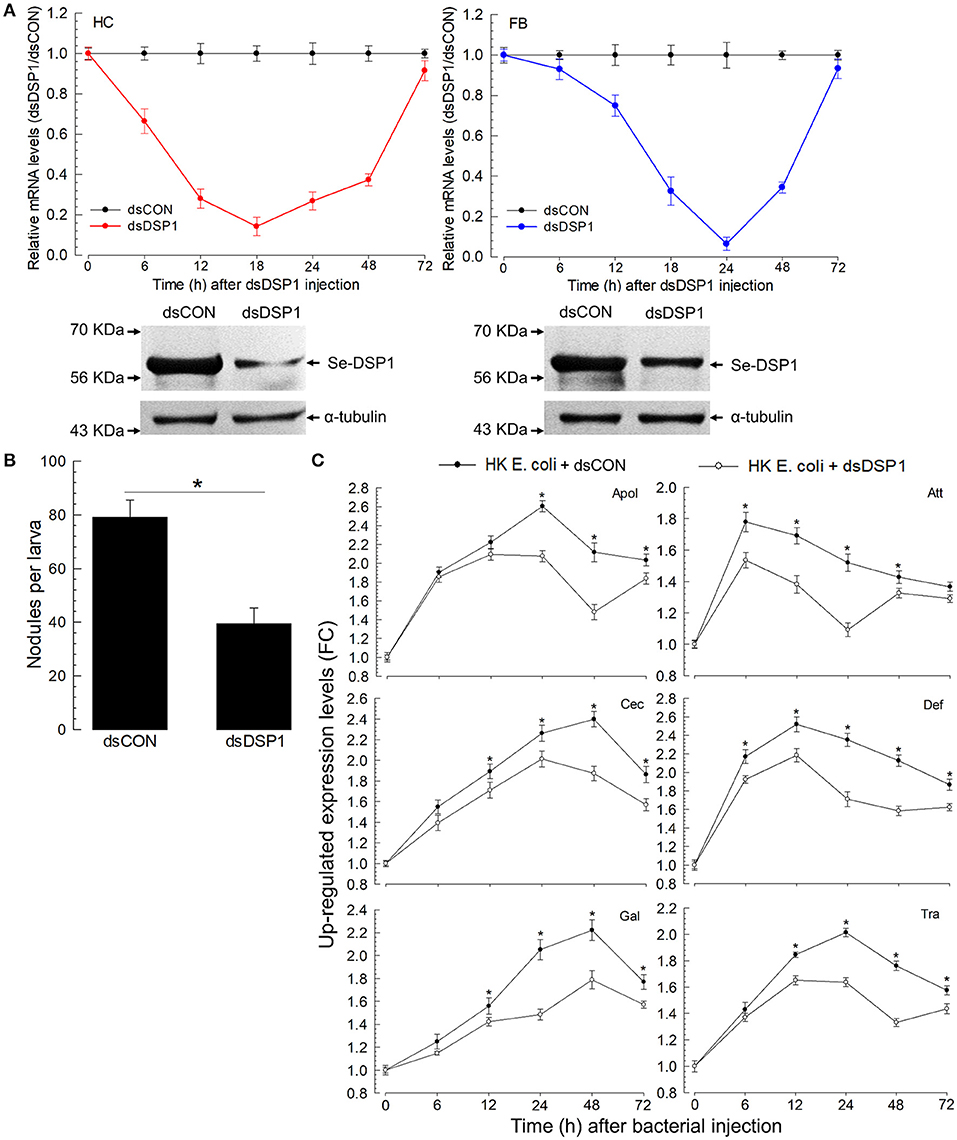
Figure 2. Effects of ribonucleic acid interference (RNAi) on Se-DSP1 expression and immune responses of S. exigua. (A) The temporal change of Se-DSP1 expression level after the treatment of L5 larvae with double-stranded (ds)RNA specific to Se-DSP1 (1 μg per larva). A viral gene, CpBV302, was used as a control dsRNA (“dsCON”). The lower panels indicate Western blotting against HC and FB extracts. A homogenous immunoblotting intensity against α-tubulin indicates the equal amount of protein sample loading in each lane. (B) The suppression of hemocyte nodule formation. For the bacterial challenge, HK E. coli (4.5 × 104 cells per larva) were injected into each larva 24 h after dsRNA treatment. At 8 h PI, the number of nodules was assessed. (C) The suppression of six AMP gene expressions after RNAi treatment. A ribosomal protein, RL32, was used as an internal control. Relative expression levels were determined by normalization compared with the levels of the control gene expression followed by the calculation of relative ratios compared with the lowest level. Each treatment was replicated three times. Asterisks above SD bars indicate a significant difference among means at Type I error = 0.05 (least significant difference, LSD, test).
Se-DSP1 Activates Eicosanoid Biosynthesis to Activate Immune Responses
Eicosanoids mediate various insect immune responses (Stanley and Kim, 2011). To understand the immune mediation of Se-DSP1, its activation of eicosanoid immune signaling was tested by measuring PLA2 activities, catalyzing the committed step for eicosanoid biosynthesis, after RNAi treatment against Se-DSP1 (Figure 3). Two substrates, arachidonate phospholipid and non-arachidonate phospholipid, were used to measure PLA2 enzyme activities. In particular, the PLA2 activity obtained from the non-arachidonate phospholipid was designated as sPLA2 activity. In contrast, cPLA2 activity was obtained from PLA2 activity using the arachidonate phospholipid. The immune challenge significantly (P < 0.05) increased both PLA2 activities (Figures 3A,B). However, the larvae treated with RNAi specific to Se-DSP1 expression failed to induce PLA2 activities. Such suppressed immune responses by RNAi treatment were significantly (P < 0.05) rescued by arachidonic acid (AA) or prostaglandin E2 (PGE2) in cellular (Figure 3C) or humoral immunity (Figure 3D).
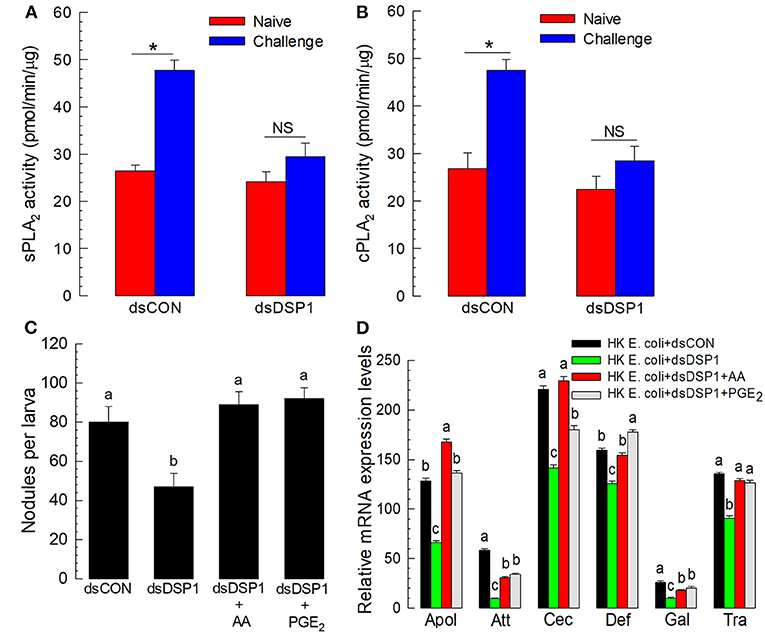
Figure 3. Se-DSP1 activates eicosanoid biosynthesis to mediate the cellular and humoral immune responses of S. exigua. The suppression of bacteria-induced (A) secretory phospholipase A2 (sPLA2) and (B) cellular PLA2 (cPLA2) activities by RNAi specific to Se-DSP1 expression. A viral gene, CpBV302, was used as a control dsRNA (“dsCON”). For the immune challenge, each of the L5 larvae was injected with 4.5 × 104 HK E. coli cells 24 h after an injection with dsRNA specific to Se-DSP1 (dsDSP1) (1 μg per larva). At 8 h after the immune challenge, fat bodies were collected and used for enzyme assays. An asterisk on SD bars indicates a significant difference among means at Type I error = 0.05 (LSD test). “NS” stands for no significant difference. (C) the rescue effect of eicosanoids on immune responses suppressed by RNAi treatment in hemocytic nodule formation. (D) Antimicrobial peptide (AMP) gene expression. Arachidonic acid (AA) or prostaglandin E2 (PGE2) at a dose of 10 μg per larva was injected along with HK E. coli (4.5 × 104 cells per larva) 24 h post dsRNA injection,. Nodule formation was assessed, and fat body tissues were collected and used to analyze AMP gene expression 8 h after E. coli injection. A ribosomal protein, RL32, was used as an internal control. Each treatment was replicated three times. Different letters above standard deviation bars indicate significant difference among means at Type I error = 0.05 (LSD test).
Se-DSP1 Alone Induces PLA2 Activity
A recombinant protein of Se-DSP1 was generated using a bacterial expression system. The recombinant protein was then purified using an affinity chromatography system containing nickel-nitrilotriacetic acid (Ni-NTA) resin and further cleared with a gel filtration chromatography system containing Sephadex G-100 (Sigma-Aldrich, Seoul, Korea) (Figure 4A). During the purification of the full-length Se-DSP1, a partial protein was also purified and confirmed by a Western analysis against a V5 epitope (Figure 4B). The truncated protein was assessed by liquid chromatography with tandem mass spectrometry (LC-MS/MS) and showed its partial structure deleting the N-terminal extension domain (Supplementary Figure S4). The injection of Se-DSP1 significantly increased the PLA2 activity of the naïve larvae (Figure 4C). However, the partial Se-DSP1 failed to induce enzyme activity. The enzyme induction with full-length Se-DSP1 was prevented by the addition of an HMGB1 antibody recognizing Se-DSP1 (Figure 4C).
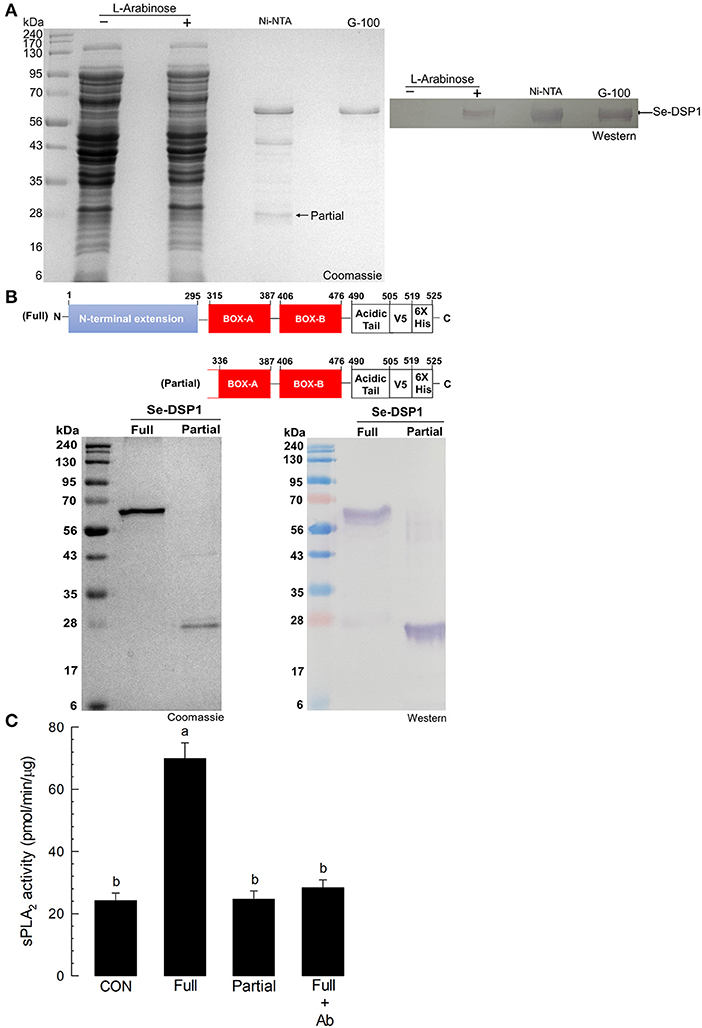
Figure 4. The activation of fat body PLA2 enzyme activity by Se-DSP1. (A) The purification of recombinant Se-DSP1 by affinity chromatography (“Ni-NTA”) and size-exclusion chromatography (“G-100”). The purified protein was confirmed with a monoclonal antibody specific to the V5 tag of the recombinant Se-DSP1 by Western blotting. (B) the purification of partial Se-DSP1. A comparison of protein domains between full and partial Se-DSP1. The partial protein was confirmed in its sequences by liquid chromatography with tandem mass spectrometry (LC-MS/MS) (Supplementary Figure S4). (C) The upregulation of the sPLA2 activity of S. exigua larvae by injecting a full length of Se-DSP1 (1 μg/larva). The enzyme activity in the fat body was measured 8 h after the injection. Partial Se-DSP1 was injected in the same amount. Control (“CON”) represented the phosphate-buffered saline (PBS) injection. An antibody (“Ab”) addition (2 μl/larva) used a commercial polyclonal antibody that recognized Se-DSP1. Each treatment was replicated three times. Different letters above SD bars indicate a significant difference among means at Type I error = 0.05 (LSD test).
Salicylic Acid (SA), a Plant Hormone, Antagonizes Mediating Effects of Se-DSP1 in Insect Immune Responses
Salicylic acid is known to bind to human HMGB1 and interrupt immune mediation (Choi et al., 2015). This suggests that it might antagonize the immune-mediating role of Se-DSP1, which is an insect homolog of human HMGB1. To test this hypothesis, different doses of SA were injected to larvae. The immune responses of the larvae were then assessed (Figure 5). Six AMP genes expressed in response to the bacterial challenge exhibited significant (P < 0.05) reductions in their expression levels after RNAi treatment using dsRNA specifically targeting the Se-DSP1 expression (Figure 5A). Among the six AMPs regulated by Se-DPS1, SA significantly (P < 0.05) prevented the upregulation of the expression of the AMP genes, except for attacin 1 (Att).
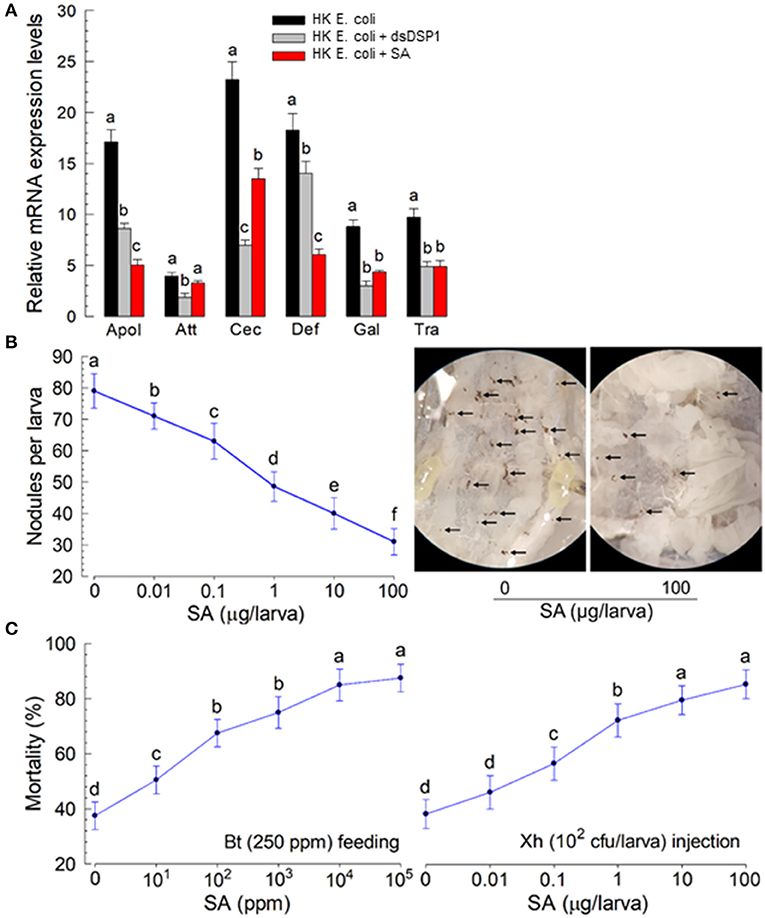
Figure 5. Insect immunosuppression induced by plant hormone salicylic acid (SA) in S. exigua. (A) The suppression of AMP gene expression by SA in response to bacterial infection. The effect of SA was compared to that of RNAi specific to Se-DSP1 expression. The RNAi treatment was performed by injecting dsRNA specific to Se-DSP1 (dsDSP1) (1 μg per larva). SA was injected to the L5 larvae at a dose of 10 μg per larva along with bacterial injection, 24 h after a dsDSP1 injection. For the bacterial challenge, HK E. coli (5.5 × 104 cells per larva) were injected into the larvae. After 6 h, fat bodies were collected and used to assess the expression levels of six AMP genes: apolipophorin III (“Apol”), attacin I (“Att”), cecropin (“Cec”), defensin I (“Def”), gallerimycin (“Gal”), and transferrin I (“Tra”). A ribosomal protein, RL32, was used as an internal control. Each treatment was replicated three times. A bacterial challenge to RNAi-treated larvae was performed 24 h after a dsDSP1 injection. (B) The suppression of cellular immune response by SA treatment. Hemocyte nodule formation was assessed by injecting HK E. coli at 4.5 × 104 cells per larva. Nodules (see photos taken at ×50 magnification) were counted 8 h after an E. coli injection. (C) Enhanced bacterial pathogenicity by SA treatment. The pathogenic test used two bacterial pathogens: Bacillus thuringiensis subsp. aizawai (Bt) by feeding and Xenorhabdus hominickii (Xh) by injection. Each treatment was replicated three times. For bioassays, 10 larvae were used per replication. Different letters above SD bars indicate a significant difference among means at Type 1 error = 0.05 (LSD test).
The immunosuppressive role of SA in immune responses was supported further by a mixture treatment of SA and entomopathogens. Nodule formation in response to the bacterial (X. hominickii) challenge was suppressed by SA injection in a dose-dependent manner (Figure 5B). The addition of SA to X. hominickii also enhanced bacterial pathogenicity by a hemocoelic injection assay (Figure 5C). The suppressive effect of SA was also observed after SA-feeding treatment. The addition of SA to Bacillus thuringiensis treatment significantly enhanced the bacterial pathogenicity after oral administration.
The immunosuppressive role of SA suggested that it might bind to Se-DSP1 and inhibit its immune mediation. In addition, Se-DSP1-like human HMGB1 (Choi et al., 2015) possesses highly conserved residues Arg and Lys to interact with SA (Supplementary Figure S5). To test the direct binding hypothesis, the purified Se-DSP1 protein was assessed for its binding affinity to SA via thermal stability assay (Figure 6A). When the concentration of SA was increased, the melting temperature (median dissociation temperature between SA and Se-DSP1 following denaturation) increased (see inset “full” figure in Figure 6A). In contrast, the partial Se-DSP1 did not show a thermal shift of the melting points in different SA concentrations (see inset “partial” figure in Figure 6A). The thermal shift curve of the full-length Se-DSP1 was used to estimate its dissociation constant (Kd) with SA: 1.28 ±.05 μM. The binding of SA to Se-DSP1 resulted in the inhibition of PLA2 induction (Figure 6B). In addition, the inhibition of PLA2 activity by SA treatment led to the suppression of cellular immune response assessed by nodulation (Figure 6C). The effect of the SA treatment was similar to that of the RNAi treatment against Se-DSP1 expression. Because SA inhibited Se-DSP1 and subsequent PLA2 activity (see the diagram), the addition of AA significantly (P < 0.05) rescued the cellular immune response.
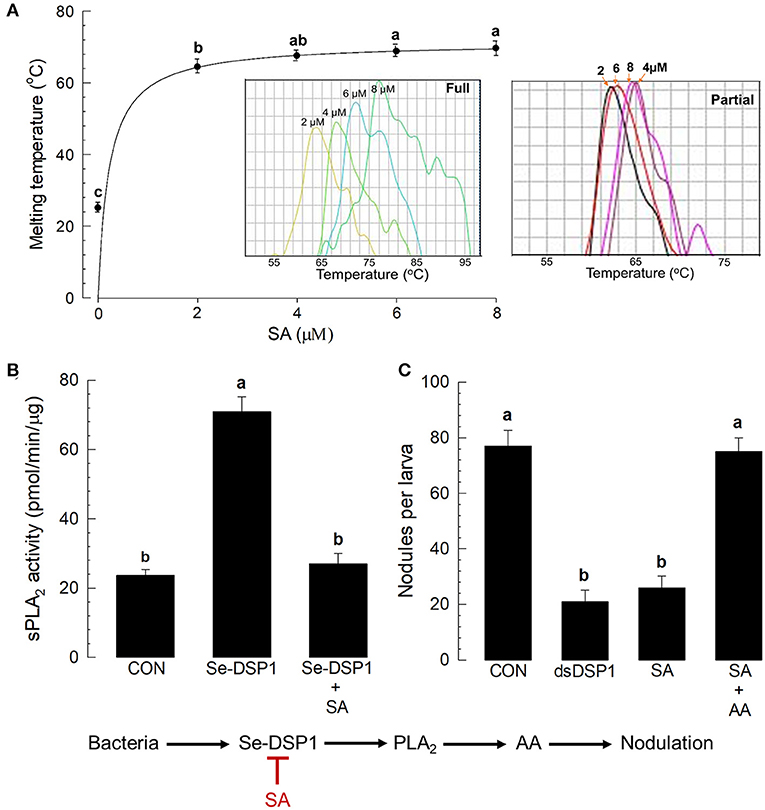
Figure 6. Salicylic acid binding to Se-DSP1 and the suppression of immune mediation. (A) The binding affinity of SA to Se-DSP1 by a thermal shifting assay (inset figures of full and partial Se-DSP1). (B) The suppression of sPLA2 activity induced by Se-DSP1 (1 μg/larva). The enzyme activity in the fat body was measured 8 h after the injection. SA was injected to the L5 larvae at a dose of 10 μg per larva. Control (“CON”) represented the PBS injection. (C) The rescue of SA-suppressed nodulation by the addition of AA (10 μg per larva). For the nodulation assay, HK E. coli were injected at a dose of 4.5 × 104 cells per larva. At 8 h after the bacterial injection, nodule formation was assessed. Each treatment was replicated three times. Different letters above SD bars indicate a significant difference among means at Type I error = 0.05 (LSD test).
SA Content in Tomato Plants Increases Insect Susceptibility to Entomopathogens
The immunosuppressive effect of SA on insect immunity suggested that plants might use SA to suppress the immune responses of phytophagous insects, leading the insects to be susceptible to entomopathogens. Before we tested the efficacy of SA in a plant host, an artificial diet containing SA was fed to S. exigua larvae and showed significant suppression in a cellular immune response (Figure 7A). Then, to test the role of SA in plant defense response against insects, an isogenic tomato line and its parental line were selected because of their different SA contents (Figure 7B). In particular, IL2-2 is an isogenic line generated by introgressing a segment of chromosome 2 from Solanum pennellii “LA716” to the S. lycopersicum “M82” variety by successive backcrosses (Eshed and Zamir, 1995). Furthermore, IL2-2 possesses a significantly higher amount of SA amount M82.
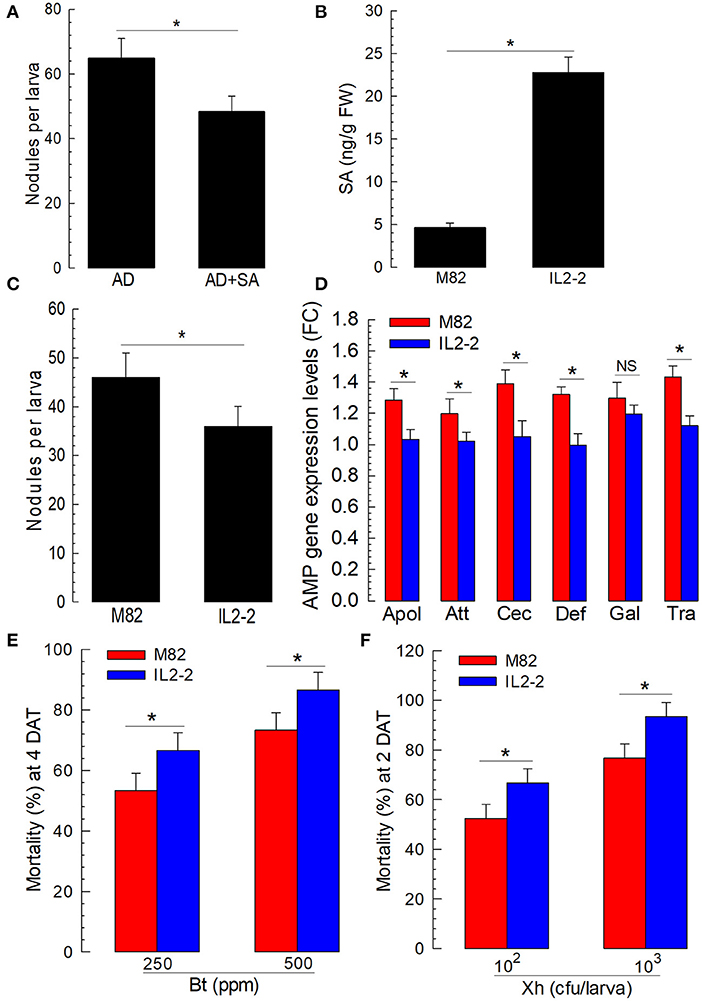
Figure 7. Influence of different tomato varieties on the immune responses and susceptibilities of S. exigua larvae to entomopathogens. (A) The suppression of cellular immune response by the addition of SA to artificial diet (“AD + SA”). AD was dipped in 1,000 ppm of the SA solution and used to feed L5 larvae for 3 days. The control diet (AD) was treated with dimethyl sulfoxide (DMSO). To assess cellular immune response, hemocyte nodule formation was determined after injecting HK E. coli at 4.5 × 104 cells per larva. Nodule formation was assessed 8 h after the E. coli injection. (B) SA contents in M82 and IL2-2 (n = 5). (C) The suppression of nodulation with a high SA line, IL2-2. (D) The suppression of humoral immune response with a high SA line, IL2-2. For the assessment of humoral immune response, HK E. coli (4.5 × 104 cells per larva) were injected. After 6 h, fat bodies were collected and used to assess the expression levels of six AMP genes: apolipophorin III (“Apol”), attacin 1 (“Att”), cecropin (“Cec”), defensin I (“Def”), gallerimycin (“Gal”), and transferrin I (“Tra”). A ribosomal protein, RL32, was used as an internal control. Each treatment was replicated three times. (E,F) The influence of M82 and IL2-2 on the susceptibility of S. exigua larvae to entomopathogens of Bt and Xh. To determine pathogenicity, a feeding assay was performed for Bt, while a hemocoelic injection was performed for Xh. Each treatment was replicated three times. For each replication, 10 L2 larvae were used. Asterisks indicate a significant difference between control and treatment at Type I error = 0.05 (LSD test).
The larvae of S. exigua were fed with tomato leaves. Their immune responses were then assessed after a bacterial challenge. There was a significant (P < 0.05) difference in cellular immunity based on nodule formation between the larvae fed with M82 and those fed with IL2-2 (Figure 7C). The expression levels of the six AMP genes regulated by Se-DSP1 were then compared among the larvae fed with two different lines of tomato. The expression levels of the AMPs, except for gallerimycin, exhibited a significant (P < 0.05) reduction after the bacterial challenge when the larvae were fed with IL2-2 (Figure 7D).
To explore the role of SA in plant defense response by suppressing the insect immune system, the S. exigua larvae fed with either M82 or IL2-2 were treated with entomopathogens (Figures 7E,F). Both entomopathogenic bacteria (B. thuringiensis and X. hominickii) showed significantly different virulence levels against the larvae fed with either M82 or IL2-2. The larvae fed with IL2-2 were more susceptible to both bacterial pathogens compared with larvae fed with M82, indicating that endogenous SA in plants could effectively suppress insect immune defenses to two different entomopathogenic bacteria.
Discussion
Mammalian HMGB1, a major player in chromatin organization and transcription regulation, can activate innate immune responses by acting as a DAMP. Although HMGB1-like proteins, also known as DSP1s, have been discovered and studied in diverse insects, their contribution to innate immune response has yet to be well-understood. This study characterized the immunological role of Se-DSP1 by acting as a DAMP and its significance in the co-evolutionary context of host plants.
Molecular characterization via phylogenetic analysis and domain analysis showed that insect DSP1s, such as Se-DSP1, were different from other HMGB1s. Insect DSP1s were separately clustered in the phylogenetic analysis. They possessed additional unique domains in addition to domains (two HMG boxes and a C-terminal acidic tail) commonly shared by HMGB1. The extended N-terminal tail in DSP1s contains a coiled coil, low complexity, and RNase PH domains. The longest HMGB1-like molecule in this analysis was Bm-DSP1 (625 amino acid residues) compared to human HMGB1 (215 residues). Another difference is the length of the C-terminal acidic tail. Human HMGB1 has a longer acidic tail with 30 residues, compared with the acidic tail of Bm-DSP1 and Se-DSP1, which only has 15 and 18 residues, respectively. Despite structural variations, Dm-DSP1 exhibited similar DNA-binding properties like mammalian HMGB (Zappavigna et al., 1996; Boonyaratanakornkit et al., 1998; Jayaraman et al., 1998). Furthermore, a mutant DSP1 lacking additional N-terminal domains and wild-type Dm-DSP1 have been found to have similar DNA-binding and subsequent bending activities (Janke et al., 2003). This suggests that the additional domains might have physiological functions other than chromatin organization. Our current study showed that the deletion of the N-terminal extension domain led to the impairment of the immune mediation of Se-DSP1. This suggests that the N-terminal domain plays a crucial role in activating PLA2 catalytic activity to mediate immune responses in insects.
Upon the immune challenge, Se-DSP1 expression was highly inducible. This protein was then released into the hemolymph. Furthermore, Se-DSP1 was expressed in all the developmental stages of S. exigua and the four larvae tissues tested in this study. This is because Se-DSP1, like other HMGB1s, might be a non-histone component of chromatin in the nucleus (Andersson and Tracey, 2011). Its basal expression was highly upregulated by the bacterial challenge in S. exigua larvae in fat body and hemocytes (see Supplementary Figure S3). In addition, the immune challenge induced the translocation of Se-DSP1 from the nucleus to extracellular hemocoel. In the naïve larvae of S. exigua, Se-DSP1 proteins were localized in the nucleus. However, the bacterial challenge stimulated the translocation of this protein to the cytoplasm and extracellular milieu. This suggests that Se-DSP1 is secreted from cells that recognize an infection-associated signal. In mammals, signals that can induce HMGB1 secretion include pathogen infection, damage, and other stresses such as hypoxia (Andrassy et al., 2008), drug treatment (Ditsworth et al., 2007), and lethal irradiation (Apetoh et al., 2007). Secretion requires the translocation of HMGB1 from the nucleus to the cytoplasm. For such a translocation, a vesicle type of transportation is used for HMGB1 secretion (Wang, 1999; Bianchi et al., 2017). In addition, the exosome appears to be another secretion route for HMGB1 in macrophages (Liu et al., 2006) or platelets (Goetzl et al., 2016). These suggest that, upon immune challenge, Se-DSP1 may translocate from the nucleus to the cytoplasm, where translocated proteins are included in vesicles to be associated with exosomes for secretion to mediate the immune responses of S. exigua.
By activating eicosanoid biosynthesis, Se-DSP1 could mediate cellular and humoral immune responses. The nodule formation of hemocytes is a cellular immune response in insects that effectively gets rid of microbial pathogens. Different hemocytes cooperate to make black nodules by enclosing infecting microbes with granulocytes and plasmatocytes. The subsequent melanization by phenoloxidase originates from oenocytoids (Lavine and Strand, 2002; Shrestha and Kim, 2008). The larvae treated with RNAi specifically targeting the Se-DSP1 expression were significantly impaired in nodule formation. In addition, the RNAi-treated larvae failed to effectively induce AMP gene expression. These findings support that Se-DSP1 has an immune-associated function. Such an immune mediation function of Se-DSP1 might be executed by activating eicosanoid biosynthesis, because the RNAi-treated larvae exhibited a significant reduction in PLA2 activities. Furthermore, PLA2 catalyzes the committed step for eicosanoid biosynthesis by releasing AA from phospholipids (Kim et al., 2018; Mouchlis and Dennis, 2019). Eicosanoids then mediate cellular and humoral immune responses against various microbial pathogens, such as bacteria, fungi, and viruses (Stanley and Kim, 2020). Thus, the activation of PLA2 by Se-DSP1 could upregulate the levels of eicosanoids to mediate immune responses. The role of DSP1 in mediating immune responses via eicosanoids was demonstrated in a coleopteran insect, Tenebrio molitor (Mollah and Kim, 2021). However, it remains unclear how Se-DSP1 activates PLA2 in S. exigua. In mammals, extracellular HMGB1 is associated with multiple receptors, such as Toll-like receptors (TLRs), to activate NF-kB (Andersson and Tracey, 2011). Toll immune signaling pathways are conserved in S. exigua (Hwang et al., 2013). In toll signaling, a Pelle adaptor is required to activate NF-kB and PLA2 in S. exigua (Hwang et al., 2013), suggesting that the Se-DSP1 released in the hemolymph can bind to a toll receptor to activate Pelle, a signal component. Pelle then activates PLA2, probably by phosphorylation. This suggests that Se-DSP1 may bind to a toll receptor to activate PLA2 activity.
Recombinant Se-DSP1 exhibits a relatively high affinity (Kd = 1.28 μM) to SA based on a thermal shift assay. However, binding affinity estimates appear to be different depending on binding assay methods. For example, SA exhibited a binding affinity in the nanomolar range to a reduced form of human HMGB1 by a surface plasmon resonance assay, although other titration method indicated a much lower affinity in the millimolar range (Choi et al., 2015). Choi et al. (2015) have also shown that the binding site of SA is located in boxes A and B of HMGB1, where amino acid residues in these two boxes involved in binding to SA are well-conserved in Se-DSP1, especially in Arg and Lys (see Supplementary Figure S5). Salicylic acid binding to Se-DSP1 had physiological significance, because SA treatment significantly reduced the immune responses of insects. Furthermore, SA has long been used to reduce inflammation (Volt et al., 2009). Aspirin, an SA derivative, has been used for pain relief. It is an anti-inflammatory drug that, by irreversible inhibition via the acetylation of COX-2, suppresses PG levels (Ekinci, 2011). Salicylic acid is a metabolite of aspirin in the human body and may increase the anti-inflammatory effect of aspirin (Choi et al., 2015). Immunosuppression was induced by either injecting or feeding SA to the S. exigua larvae. As mentioned earlier, ingested SA might bind to extracellular Se-DSP1, but fails to activate PLA2 activity upon an immune challenge. Under immunosuppressive conditions, the SA-treated larvae increased their susceptibility to entomopathogenic microbes. Indeed, the SA-treated larvae exhibited increased susceptibility to entomopathogens. This was supported further by assays using larvae fed with endogenous SA in planta. Larvae fed with tomato leaves with higher SA levels became more susceptible to entomopathogenic microbes than control larvae that were not fed with such tomato leaves. This suggests that plant hormone SA might have an indirect role in defending phytophagous insects by eliciting tri-trophic interactions. Endogenous plant SA might make insects become more vulnerable to their bacterial pathogens (B. thuringiensis or X. hominickii), leading to an effective strategy for plants to ward off phytophagous insects. Since the first report showing that SA can induce tobacco mosaic virus resistance in tobacco, it has been found that SA can act as both a phytohormone-mediating plant defense activator and a growth regulator in various plants (Koo et al., 2020). Especially, SA can induce the resistance of plants against different microbial pathogens, such as viruses, bacteria, fungi, and oomycetes. A positive correlation between endogenous levels of SA and resistance responses against biotrophic and hemibiotrophic pathogens has been well-demonstrated (Glazebrook, 2005). This study introduced a novel tri-trophic interaction orchestrated by SA among plant-insect-entomopathogenic microbes. High levels of plant endogenous SA consumed by an herbivore insect (S. exigua) caused significant immunosuppression of the insect. Such an effect was mediated by the interaction between SA and Se-DSP1, making the insect vulnerable to entomopathogenic bacteria. Increasing SA content could be a novel crop breeding strategy for insect resistance.
Data Availability Statement
The datasets presented in this study can be found in online repositories. The names of the repository/repositories and accession number(s) can be found in the article/Supplementary Material.
Author Contributions
MM and YK carried out the experiment and wrote the manuscript with support from HC, IY, and JL. YK conceived the original idea. YK, IY, HC, and JL supervised the project. All the authors contributed to the article and approved the submitted version.
Funding
The authors have received funding from the following: a grant (No. 2017R1A2133009815) from the National Research Foundation (NRF) funded by the Ministry of Science, ICT and Future Planning, Republic of Korea.
Conflict of Interest
The authors declare that the research was conducted in the absence of any commercial or financial relationships that could be construed as a potential conflict of interest.
Publisher's Note
All claims expressed in this article are solely those of the authors and do not necessarily represent those of their affiliated organizations, or those of the publisher, the editors and the reviewers. Any product that may be evaluated in this article, or claim that may be made by its manufacturer, is not guaranteed or endorsed by the publisher.
Supplementary Material
The Supplementary Material for this article can be found online at: https://www.frontiersin.org/articles/10.3389/fphys.2021.744272/full#supplementary-material
References
Aleporou-Marinou, V., Drosos, Y., Ninios, Y., Agelopoulou, B., and Patargias, T. (2003). High mobility group-like proteins of the insect Plodia interpunctella. Biochem. Genet. 41, 39–46. doi: 10.1023/A:1020922512440
Andersson, U., and Tracey, K. J. (2011). HMGB1 is a therapeutic target for sterile Inflammation and infection. Annu. Rev. Immunol. 29, 139–162. doi: 10.1146/annurev-immunol-030409-101323
Andrassy, M., Volz, H. C., Igwe, J. C., Funke, B., Eichberger, S. N., Kaya, Z., et al. (2008). High-mobility group box-1 in ischemia-reperfusion injury of the heart. Circulation 117, 3216–3226. doi: 10.1161/CIRCULATIONAHA.108.769331
Apetoh, L., Ghiringhelli, F., Tesniere, A., Obeid, M., Ortiz, C., Criollo, A., et al. (2007). Toll-like receptor 4-dependent contribution of the immune system to anticancer chemotherapy and radiotherapy. Nat. Med. 13, 1050–1059. doi: 10.1038/nm1622
Bianchi, M. E., Crippa, M. P., Manfredi, A. A., Mezzapelle, R., Querini, P. R., and Venereau, E. (2017). High-mobility group box 1 protein orchestrates responses to tissue damage via inflammation, innate and adaptive immunity, and tissue repair. Immunol. Rev. 280, 74–82. doi: 10.1111/imr.12601
Boonyaratanakornkit, V., Melvin, V., Prendergast, P., Altmann, M., Ronfani, L., Bianchi, M. E., et al. (1998). High mobility group chromatin proteins 1 and 2 functionally interact with steroid hormone receptors to enhance their DNA binding in vitro and transcriptional activity in mammalian cells. Mol. Cell Biol. 18, 4471–4487. doi: 10.1128/MCB.18.8.4471
Bowling, S. A., Guo, A., Cao, H., Gordon, A. S., Klessig, D. F., and Dong, X. (1994). A mutation in Arabidopsis that leads to constitutive expression of systemic acquired resistance. Plant Cell 6, 1845–1857. doi: 10.1105/tpc.6.12.1845
Bustin, M. (2001). Chromatin unfolding and activation by HMGN chromosomal proteins. Trends Biochem. Sci. 6, 431–437. doi: 10.1016/S0968-0004(01)01855-2
Canaple, L., Decoville, M., Leng, M., and Locker, D. (1997). The Drosophila DSP1 gene encoding an HMG 1-like protein: genomic organization, evolutionary conservation and expression. Gene 184, 285–290. doi: 10.1016/S0378-1119(96)00616-6
Choi, H. W., Tian, M., Song, F., Venereau, E., Preti, A., Park, S. W., et al. (2015). Aspirin's active metabolite salicylic acid targets high mobility group box 1 to modulate inflammatory responses. Mol. Med. 21, 526–535. doi: 10.2119/molmed.2015.00148
de Mendonça Amarante, A., Jupatanakul, N., de Abreu da Silva, I. C., Carneiro, V. C., Vicentino, A. R. R., Dimopolous, G., et al. (2017). The DNA chaperone HMGB1 potentiates the transcriptional activity of Rel1A in the mosquito Aedes aegypti. Insect Biochem. Mol. Biol. 80, 32–41. doi: 10.1016/j.ibmb.2016.11.006
Decoville, M., Giacomello, E., Leng, M., and Locker, D. (2001). DSP1, an HMGB1-like protein, is involved in the regulation of homeotic genes. Genetics 157, 237–244. doi: 10.1093/genetics/157.1.237
Ditsworth, D., Zong, W. X., and Thompson, C. B. (2007). Activation of poly (ADP)-ribose polymerase (PARP-1) induces release of the proinflammatory mediator HMGB1 from the nucleus. J. Biol. Chem. 282, 17845–17854. doi: 10.1074/jbc.M701465200
Ekinci, D. (2011). Salicylic acid derivatives: synthesis, features and usage as therapeutic tools. Expert Opin. Ther. Pat. 21, 1831–1841. doi: 10.1517/13543776.2011.636354
Eshed, Y., and Zamir, D. (1995). An introgression line population of Lycopersicon pennellii in the cultivated tomato enables the identification and fine mapping of yield-associated QTL. Genetics 141, 1147–1162. doi: 10.1093/genetics/141.3.1147
Glazebrook, J. (2005). Contrasting mechanisms of defense against biotrophic and necrotrophic pathogens. Annu. Rev. Phytopathol. 43, 205–227. doi: 10.1146/annurev.phyto.43.040204.135923
Goetzl, E. J., Goetzl, L., Karliner, J. S., Tang, N., and Pulliam, L. (2016). Human plasma platelet-derived exosomes: effects of aspirin. FASEB J. 30, 2058–2063. doi: 10.1096/fj.201500150R
Goh, H. G., Lee, S. G., Lee, B. P., Choi, K. M., and Kim, J. H. (1990). Simple mass-rearing of beet armyworm, Spodoptera exigua (Hübner) (Lepidoptera: Noctuidae), on an artificial diet. Korean J. Appl. Entomol. 29, 180–183.
Goodwin, G. H., and Johns, E. W. (1977). The isolation and purification of the high mobility group (HMG) nonhistone chromosomal proteins. Methods Cell Biol. 16, 257–267. doi: 10.1016/S0091-679X(08)60104-1
Hasan, M. A., Ahmed, S., and Kim, Y. (2019). Biosynthetic pathway of arachidonic acid in Spodoptera exigua in response to bacterial challenge. Insect Biochem. Mol. Biol. 111, 1–15. doi: 10.1016/j.ibmb.2019.103179
Hwang, J., Park, Y., Kim, Y., and Lee, D. (2013). An entomopathogenic bacterium, Xenorhabdus nematophila, suppresses expression of antimicrobial peptides controlled by Toll and Imd pathways by blocking eicosanoid biosynthesis. Arch. Insect Biochem. Physiol. 83, 151–169. doi: 10.1002/arch.21103
Janke, C., Martin, D., Giraud-Panis, M. J., Decoville, M., and Locker, D. (2003). Drosophila DSP1 and rat HMGB1 have equivalent DNA binding properties and share a similar secondary fold. J. Biochem. 133, 533–539. doi: 10.1093/jb/mvg063
Jayaraman, L., Moorthy, N. C., Murthy, K. G. K., Manley, J. L., Bustin, M., and Prives, C. (1998). High mobility group protein-1 (HMGB1) is a unique activator of p53. Genes Dev. 12, 462–472. doi: 10.1101/gad.12.4.462
Jong, S. R., and Dong, H. S. (2018). Damage-associated molecular patterns in inflammatory diseases. Immune Netw. 18, 1–14. doi: 10.4110/in.2018.18.e27
Kim, Y., Ahmed, S., Stanley, D., and An, C. (2018). Eicosanoid-mediated immunity in insects. Dev. Comp. Immunol. 83, 130–143. doi: 10.1016/j.dci.2017.12.005
Koo, Y. M., Heo, A. Y., and Choi, H. W. (2020). Salicylic acid as a safe plant protector and growth regulator. Plant Pathol. J. 36, 1–10. doi: 10.5423/PPJ.RW.12.2019.0295
Lavine, M. D., and Strand, M. R. (2002). Insect hemocytes and their role in immunity. Insect Biochem. Mol. Biol. 32, 1295–1309. doi: 10.1016/S0965-1748(02)00092-9
Liu, S., Stolz, D. B., Sappington, P. L., Macias, C. A., Killeen, M. E., Tenhunen, J. J., et al. (2006). HMGB1 is secreted by immunostimulated enterocytes and contributes to cytomix-induced hyperpermeability of Caco-2 monolayers. Am. J. Physiol. Cell Physiol. 290, C990–C999. doi: 10.1152/ajpcell.00308.2005
Mollah, M. M. I., Ahmed, S., and Kim, Y. (2021). Immune mediation of HMG-like DSP1 via Toll-Spatzle pathway and its specific inhibition by salicylic acid analogs. PLoS Pathog. 17:e1009467. doi: 10.1371/journal.ppat.1009467
Mollah, M. M. I., Dekebo, A., and Kim, Y. (2020a). Immunosuppressive activities of novel PLA2 inhibitors from Xenorhabdus hominickii, an entomopathogenic bacterium. Insects 11:505. doi: 10.3390/insects11080505
Mollah, M. M. I., and Kim, Y. (2021). HMGB1-like dorsal switch protein 1 of the mealworm, Tenebrio molitor, acts as a damage-associated molecular pattern. Arch. Insect Biochem. Physiol. 107:e21795. doi: 10.1002/arch.21795
Mollah, M. M. I., Roy, M. C., Choi, D. Y., Hasan, M. A., Baki, M. A. A., Yeom, H. S., et al. (2020b). Variations of Indole metabolites and NRPS-PKS loci in two different virulent strains of Xenorhabdus hominickii. Front. Microbiol. 11:583594. doi: 10.3389/fmicb.2020.583594
Mosrin-Huaman, C., Canaple, L., Locker, D., and Decoville, M. (1998). DSP1 gene of Drosophila melanogaster encodes an HMG-domain protein that plays roles in development. Dev. Genet. 23, 324–334. doi: 10.1002/(SICI)1520-6408(1998)23:4<324::AID-DVG7>3.0.CO;2-T
Mouchlis, V. D., and Dennis, E. A. (2019). Phospholipase A2 catalysis and lipid mediator lipidomics. Biochim. Biophys. Acta 1864, 766–771. doi: 10.1016/j.bbalip.2018.08.010
Muller, S., Bianchi, M. E., and Knapp, S. (2001). Thermodynamics of HMGB1 interaction with duplex DNA. Biochemistry 40, 10254–10261. doi: 10.1021/bi0100900
Parry, D. A., Fraser, R. D., and Squire, J. M. (2008). Fifty years of coiled-coils α-helical bundles: a close relationship between sequence and structure. J. Struct. Biol. 163, 258–269. doi: 10.1016/j.jsb.2008.01.016
Reeves, R. (2003). HMGA proteins: flexibility finds a nuclear niche? Biochem. Cell. Biol. 81, 185–195. doi: 10.1139/o03-044
Ribeiro, F. S., de Abreu da Silva, I. C., Carneiro, V. C., dos Santos Belgrano, F., Mohana-Borges, R., de Andrade Rosa, I., et al. (2012). The dengue vector Aedes aegypti contains a functional high mobility group box 1 (HMGB1) protein with a unique regulatory C-terminus. PLoS ONE 7:e40192. doi: 10.1371/journal.pone.0040192
Salichs, E., Ledda, A., Mularoni, L., Alba, M. M., and de la Luna, S. (2009). Genome-wide analysis of histidine repeats reveals their role in the localization of human proteins to the nuclear speckles compartment. PLoS Genet. 5:e1000397. doi: 10.1371/journal.pgen.1000397
Shrestha, S., and Kim, Y. (2008). Eicosanoid mediate prophenoloxidase release from oenocytoids in the beet armyworm, Spodoptera exigua. Insect Biochem. Mol. Biol. 38, 99–112. doi: 10.1016/j.ibmb.2007.09.013
Simeonov, A. (2013). Recent developments in the use of differential scanning fluorometry in protein and small molecule discovery and characterization. Expert Opin. Drug. Discov. 8, 1071–1082. doi: 10.1517/17460441.2013.806479
Stanley, D., and Kim, Y. (2011). Prostaglandins and their receptors in insect biology. Front. Endocrinol. 2:105. doi: 10.3389/fendo.2011.00105
Stanley, D., and Kim, Y. (2020). Why most insects have very low proportions of C20 polyunsaturated fatty acids: the oxidative stress hypothesis. Arch. Insect Biochem. Physiol. 103:e21622. doi: 10.1002/arch.21622
Stros, M. (2010). HMGB proteins: interactions with DNA and chromatin. Biochim. Biophys. Acta 1799, 101–113. doi: 10.1016/j.bbagrm.2009.09.008
Stros, M., Launholt, D., and Grasser, K. D. (2007). The HMG-box: a versatile protein domain occurring in a wide variety of DNA-binding proteins. Cell. Mol. Life Sci. 64, 2590–2606. doi: 10.1007/s00018-007-7162-3
Thomas, J. O., and Travers, A. A. (2001). HMG1 and 2, and related ‘architectural’ DNA-binding proteins. Trends Biochem. Sci. 26, 167–174. doi: 10.1016/S0968-0004(01)01801-1
Ton, J., Pieterse, C. M. J., and van Loon, L. C. (2006). “The relationship between basal and induced resistance in Arabidopsis,” in Multigenic and Induced Systemic Resistance in Plants, eds S. Tuzun and E. Bent (New York, NY: Springer), 197–224. doi: 10.1007/0-387-23266-4_9
Tsung, A., Klune, J. R., Zhang, X., Jeyabalan, G., Cao, Z., Peng, X., et al. (2007). HMGB1 release induced by liver ischemia involves Toll-like receptor 4–dependent reactive oxygen species production and calcium-mediated signaling. J. Exp. Med. 204, 2913–2923. doi: 10.1084/jem.20070247
Vatanparast, M., Ahmed, S., Herrero, S., and Kim, Y. (2018). A non-venomous sPLA2 of a lepidoptera insect: its physiological functions in development and immunity. Dev. Comp. Immunol. 89, 83–92. doi: 10.1016/j.dci.2018.08.008
Volt, A. C., Dempsey, D. A., and Klessig, D. F. (2009). Salicylic acid, a multifaceted hormone to combat disease. Annu. Rev. Phytopathol. 47, 177–206. doi: 10.1146/annurev.phyto.050908.135202
Wang, H. (1999). HMG-1 as a late mediator of endotoxin lethality in mice. Science 285, 248–251. doi: 10.1126/science.285.5425.248
Webb, M., Payet, D., Lee, K. B., Travers, A. A., and Thomas, J. O. (2001). Structural requirements for cooperative binding of HMG1 to DNA minicircles. J. Mol. Biol. 309, 79–88. doi: 10.1006/jmbi.2001.4667
Wu, J., and Baldwin, I. T. (2009). Herbivory-induced signaling in plants: perception and action. Plant Cell Environ. 32, 1161–1174. doi: 10.1111/j.1365-3040.2009.01943.x
Zappavigna, V., Falciola, L., Citterich, M. H., Mavilio, F., and Bianchi, M. E. (1996). HMGB1 interacts with HOX proteins and enhances their DNA binding and transcriptional activation. EMBO J. 15, 4981–4991. doi: 10.1002/j.1460-2075.1996.tb00878.x
Keywords: salicylic acid, eicosanoid, HMGB1, immunity, insect
Citation: Mollah MMI, Choi HW, Yeam I, Lee JM and Kim Y (2021) Salicylic Acid, a Plant Hormone, Suppresses Phytophagous Insect Immune Response by Interrupting HMG-Like DSP1. Front. Physiol. 12:744272. doi: 10.3389/fphys.2021.744272
Received: 12 August 2021; Accepted: 06 September 2021;
Published: 04 October 2021.
Edited by:
Natraj Krishnan, Mississippi State University, United StatesReviewed by:
Seung-Joon Ahn, Mississippi State University, United StatesWen Liu, Huazhong Agricultural University, China
Copyright © 2021 Mollah, Choi, Yeam, Lee and Kim. This is an open-access article distributed under the terms of the Creative Commons Attribution License (CC BY). The use, distribution or reproduction in other forums is permitted, provided the original author(s) and the copyright owner(s) are credited and that the original publication in this journal is cited, in accordance with accepted academic practice. No use, distribution or reproduction is permitted which does not comply with these terms.
*Correspondence: Yonggyun Kim, aG9zYW5uYUBhbnUuYWMua3I=