- Department of Entomology, Iowa State University, Ames, IA, United States
Characterizing the physiological changes that accompany malaria parasite infection of the mosquito host is crucial to our understanding of vectorial capacity in Anopheles mosquitoes, yet has not fully been explored. In this study, we examine the role of uric acid metabolism in the mosquito, Anopheles gambiae, following malaria parasite infection. We demonstrate that levels of uric acid are significantly decreased in the excreta and the mosquito at 24 and 48 h post-Plasmodium infection when compared to controls fed on naïve mouse blood. When we examine the expression of well-known enzymes responsible for uric acid metabolism, we see a significant increase in both urate oxidase (UO) and allatoicase (ALLC) expression following Plasmodium infection. Targeting the essential first step in uric acid metabolism by silencing UO resulted in elevated levels of uric acid, enhancing malaria parasite survival. With implications from other insect systems that bacteria can modulate UO expression, we examined the possibility that the mosquito microbiota and its expansion following blood-feeding may contribute to increased UO levels. However, there was no difference in uric acid metabolism between septic and aseptic mosquitoes, indicating that the mosquito microbiome is not associated with the manipulation of UO expression. Together, our study provides new evidence that Plasmodium infection causes the mosquito host to actively metabolize uric acid by increasing UO expression to limit Plasmodium oocyst survival, suggesting that nitrogen metabolism is an essential pathway in defining mosquito vector competence.
Introduction
Mosquito blood-feeding provides essential resources for egg production and enables mosquitoes to serve as efficient vectors to acquire and transmit several mosquito-borne pathogens. Most notably, Plasmodium parasites cause the devastating public health and socioeconomic impacts of malaria throughout the world (World Health Organization, 2019).
Independent of the presence of a pathogen, blood-feeding also represents a major physiological event. Digestion of the protein-rich blood meal and its conversion into nutritional resources represents a major metabolic event for the mosquito, stimulating several physiological processes, including vitellogenesis (Attardo et al., 2005), immune induction (Upton et al., 2015; Reynolds et al., 2020b), and epithelial turnover (Taracena et al., 2018). In addition, enzymes that promote blood digestion result in the release of heme, which can lead to an increase in levels of reactive oxygen species (ROS) and potential cytotoxic effects (Whiten et al., 2018). To overcome this oxidative stress, mosquitoes maintain physiological homeostasis by detoxifying the accumulated reactive molecules via peroxidases and catalase activities (Molina-Cruz et al., 2008; Champion and Xu, 2017). In addition, excessive amino acids are catabolized into uric acid (Von Dungern and Briegel, 2001; Scaraffia et al., 2008), providing additional antioxidants to maintain physiological homeostasis (Molina-Cruz et al., 2008), or are converted by the enzymes urate oxidase (UO), allantoinase (ALLN), allantoicase (ALLC) into urea through the uricolytic pathway as nitrogenous waste (Scaraffia et al., 2008; Isoe and Scaraffia, 2013).
Several studies provide evidence that this regulation of oxidative homeostasis is integral to mosquito vector competence, where increased levels of ROS and subsequent nitration in the mosquito host have been shown to limit malaria parasite survival (Luckhart et al., 1998; Kumar et al., 2003; Peterson et al., 2007; Molina-Cruz et al., 2008; Eleftherianos et al., 2021). Reciprocally, when increased levels of antioxidants are provided (as either uric acid/urate or ascorbic acid) to Plasmodium infected mosquitoes, parasite survival is increased (Peterson et al., 2007; Molina-Cruz et al., 2008). Similarly, in other insect systems, uric acid promotes the success of Trypanosoma brucei brucei survival in tsetse flies (MacLeod et al., 2007), and it has been suggested that Drosophila actively reduce uric acid levels to limit bacterial growth (Chambers et al., 2012; Lang et al., 2019). Therefore, the physiological and immunological mechanisms of uric acid synthesis and metabolism are essential to our understanding of mosquito vector competence.
Herein, we describe differences in the regulation of uric acid metabolism between blood-fed (naïve) and Plasmodium-infected Anopheles gambiae, demonstrating that malaria parasite infection promotes increased levels of urate oxidase (UO) activity in the mosquito host. Driving the metabolism of uric acid into urea, we demonstrate that silencing UO results in increased uric acid levels, enhancing parasite survival. Taken together, these observations suggest that the mosquito host manipulates uric acid metabolism in response to infection to limit pathogen survival.
Materials and Methods
Mosquito Rearing and Plasmodium Infection
Anopheles gambiae (Keele strain) were reared at 27°C and 80% humidity, with a 14/10-h day/night cycle. Larvae were fed on fish flakes (Tetramin, Tetra), and adult mosquitoes were maintained on 10% sucrose solution.
Female Swiss Webster mice were infected with a mCherry strain of Plasmodium berghei as previously described (Kwon et al., 2017; Kwon and Smith, 2019). Parasite infection was quantified at 8 days post-infection by counting oocyst numbers from individual midgut samples using a fluorescent microscope (Nikon Eclipse 50i, Nikon).
Uric Acid Quantification
Anopheles gambiae mosquitoes were challenged by blood-feeding with either a naïve or P. berghei-infected mouse. Within 30 min after challenge, fully engorged mosquitoes were collected and placed in a glass vial (20 × 70 mm) containing a small cotton ball applied with 200 μl of 10% sucrose for the first 24 h, and transferred to a new glass vial and kept for additional 24 h. Mosquitoes were maintained at 19°C with 80% relative humidity and a 14/10-h light/dark cycle. Uric acid levels were measured in excreta from individual mosquitoes by the addition of 0.5 ml of 1% lithium carbonate in distilled water to each glass vial to dissolve the solid excreta. To each tube, 2.5 ml of uric acid reagent consisting of a 10:1 mixture of Solution I (377 mM sodium carbonate, 213 mM glycine, 3.1 mM cupric sulfate in distilled water) and Solution II (19 mM neocuproine hydrochloride monohydrate in distilled water) were, respectively added to the resuspended lithium carbonate. The mixture was incubated for 5 min at room temperature to maximize (∼95%) color development as previously described (Handel, 1975; Van Handel and Klowden, 1996). A standard curve was generated using serial dilutions of uric acid in 1% lithium carbonate solution (ranges from 50 μg to 0.1 ug/ml) and was used to determine uric acid concentrations in samples when measured at 450 nm using VERSAmax™ Tunable Microplate Reader (Molecular Devices). Individual mosquito samples at 48 h post-challenge were homogenized in 0.5 ml of 1% lithium carbonate solution to extract uric acid, heated at 80°C for 3 min, and centrifuged at 12,000 × g for 10 min (Scaraffia et al., 2008). The supernatant (0.5 ml) was mixed with the uric acid reagent mixture (2.5 ml) to measure uric acid levels as described above.
Gene Expression Analysis by qRT-PCR
To determine whether differences in uric acid production between blood-fed and P. berghei infected mosquitoes display changes in uricolytic pathway gene expression, mosquitoes challenged with either a naïve or P. berghei-infected blood meal were collected at 3, 24, and 48 h post-challenge. Mosquitoes (n = 15 per treatment at each time point) were used for total RNA isolation using TRIzol (Thermo Fisher Scientific). Total RNA (2 μg) was treated with DNase I (New England Biolabs) and used for cDNA synthesis using the RevertAid First Strand cDNA Synthesis kit (Thermo Fisher Scientific). qRT-PCR analysis was performed using cDNA (1:5 dilution), 500 nM of gene-specific primers and PowerUp™SYBR®Green Master Mix (Thermo Fisher Scientific) with the following cycling conditions: 95°C for 10 min, 40 cycles with 95°C for 15 s and 65°C for 60 s. Ribosomal protein S7 transcript levels were used as an internal reference as described previously (Kwon et al., 2017; Kwon and Smith, 2019). A comparative CT (2–ΔΔCt) method was employed to determine relative transcript abundance for each transcript (Livak and Schmittgen, 2001). Primers used for qRT-PCR are listed in Supplementary Table 1.
Gene-Silencing via RNAi
Since mosquitoes challenged with P. berghei exhibited increases of urate oxidase expression and lower amounts of uric acid production as compared to naive blood-fed mosquitoes, the potential role of UO in Plasmodium survival was investigated through gene silencing. RNAi experiments were performed as previously described (Kwon et al., 2017; Kwon and Smith, 2019). T7 DNA template was amplified using cDNA prepared from whole mosquitoes collected 24 h post-P. berghei infection and T7 primers designed using the E-RNAi web application.1 Primers are listed in Supplementary Table 1. Amplified PCR products were gel purified using the Gel DNA Recovery kit (Zymo Research) and dsRNA was prepared using the MEGAscript RNAi kit (Life Technologies) according to the manufacturer’s instructions. After ethanol precipitation, dsRNA was resuspended in nuclease free water to a final concentration of 3 μg/μl. Three to four-day old mosquitoes were cold anesthetized and injected intrathoracically with 69 nl (∼200 ng) of dsRNA per mosquito using a Nanoject III injection system (Drummond Scientific). Silencing efficiency was evaluated 2 days post-injection of dsRNA in whole mosquito samples (15 mosquitoes per treatment). Total RNA isolation, cDNA synthesis and qRT-PCR analysis were performed as described above. To evaluate the effect of gene-silencing on uric acid metabolism and Plasmodium survival, mosquitoes at 2 days post-injection of dsRNA were challenged with P. berghei. Engorged individual mosquitoes were transferred to a glass vial and kept for 24 h, then transferred to a new glass vial for an additional 24 h to measure uric acid in excreta, as well as in the whole mosquito body at 48 h-post-infection. Samples for the uric acid measurement were prepared as described above. Oocyst survival was determined by counting oocyst numbers at 8 days post-infection.
Contribution of Midgut Bacteria to Uric Acid Metabolism
To determine whether the expression of UO is altered by bacterial abundance in mosquitoes, mosquitoes (n = 10 per treatment at each time point) were challenged with either a naive or an infected blood meal and collected at 3 h, 24 h and 48 h post-challenge. Mosquitoes were surface-sterilized in 75% ethanol for 5 min, then washed twice with sterile 1xPBS before used for total RNA isolation and cDNA synthesis as described above. Universal bacterial 16s rRNA primers (Supplementary Table 1) were used for the quantification of bacteria as previously described (Blumberg et al., 2013; Smith et al., 2015; Reynolds et al., 2020a). Newly emerged female mosquitoes (0 to 1-day-old) were maintained on 10% sucrose or a 10% sterile sucrose solution containing antibiotics (100 units/mL of penicillin and 100 μg/mL of streptomycin) for 3 days. The reduction of bacterial 16s rRNA in aseptic mosquitoes (n = 10) was determined by qRT-PCR at 3 days post-treatment using the universal bacterial primers. The mosquitoes were challenged with P. berghei infection to quantify uric acid in excreta and whole body from individual mosquitoes at 24 h and 48 h post-infection as described above.
Results
Uric Acid Levels Differ Between Blood-Fed and Plasmodium-Infected Mosquitoes
With previous results supporting the role of uric acid as a key antioxidant that supports malaria parasite survival (Peterson et al., 2007; Molina-Cruz et al., 2008), we wanted to examine if uric acid production varied between naïve and infected blood meals. To approach this question, we challenged mosquitoes with a naïve or P. berghei-infected mouse and examined levels of uric acid in the excreta of individual mosquitoes at 24 h and 48 h post-challenge. Compared to mosquitoes fed on a naïve blood meal, infected mosquitoes excreted significantly less uric acid at both 24 h and 48 h post-infection (Figure 1A). However, since this only examined excreta, there is the potential that uric acid was instead being retained in the mosquito host. To address this, we examined levels of uric acid in whole mosquitoes, demonstrating that uric acid levels were also similarly reduced in P. berghei-infected mosquitoes at 48 h post-challenge (Figure 1B). Together, these observations suggest that uric acid metabolism in the mosquito is altered as a result of Plasmodium infection.
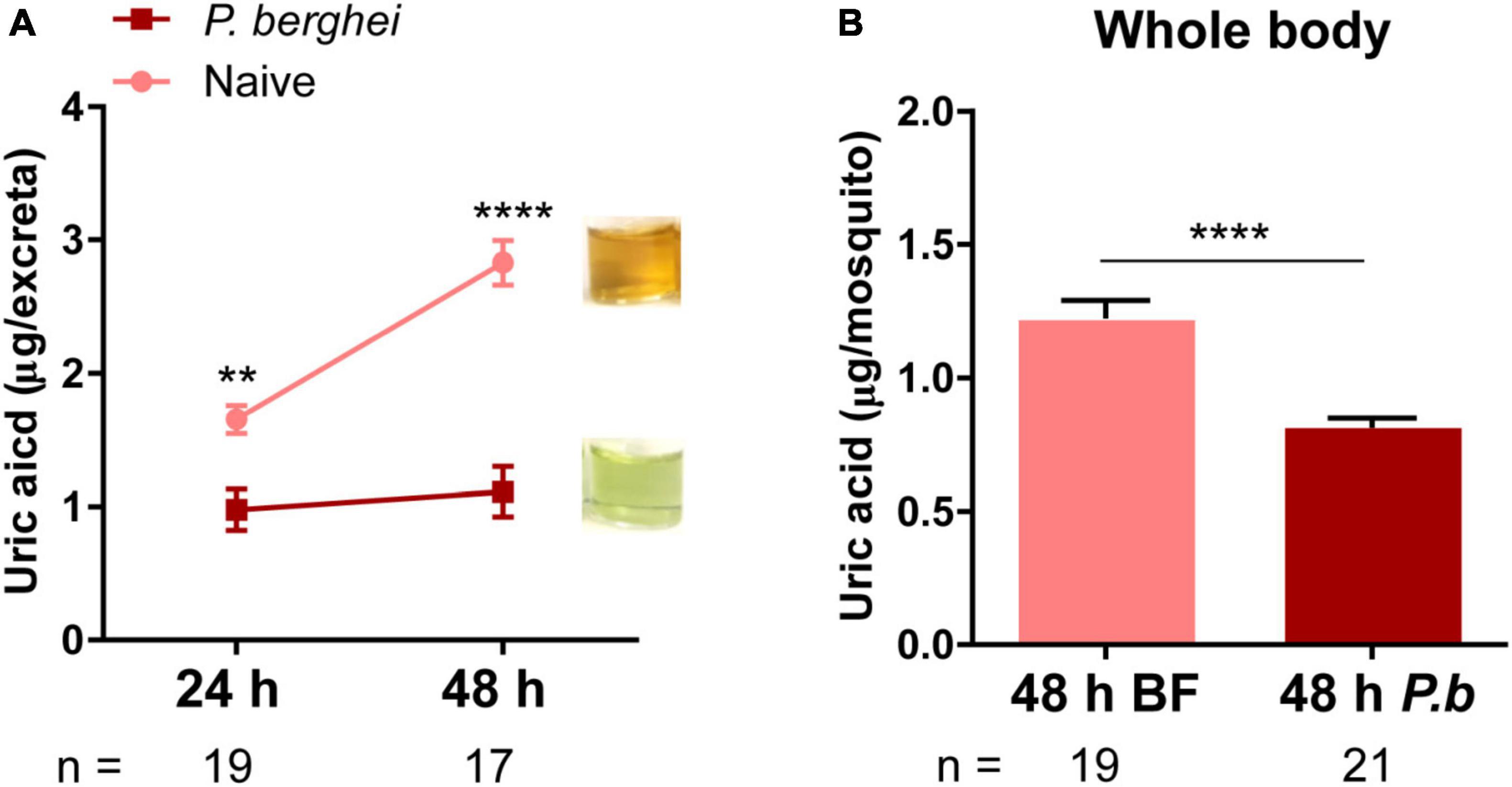
Figure 1. Uric acid levels are reduced in excreta and in whole mosquitoes following Plasmodium infection. Uric acid in the excreta of individual mosquitoes was measured at both 24 and 48 h after blood-feeding (naïve) or P. berghei infection. Plasmodium infection significantly reduced uric acid concentration in excreta when compared to mosquitoes that fed on a naïve blood meal. Inset images display representative images of differences in the intensity of uric acid observed in excreta samples at 48 h-post challenge (A). Data from excreta samples were analyzed using a two-way repeated-measures ANOVA followed by Sidak’s multiple comparisons. Uric acid levels were similarly measured in whole mosquito samples 48 h after blood-feeding (naïve) or P. berghei infection (B), where Plasmodium-infected mosquitoes retained less uric acid than those provided with a naïve blood meal. Data were analyzed by an unpaired t-test. Bar graphs represent mean ± SEM of three independent experiments. Asterisks denote significance (**P < 0.01, ****P < 0.0001). n, number of individual mosquitoes examined. Data analysis was performed using GraphPad Prism 7.
Key Enzymes in Uric Acid Metabolism Are Influenced by Plasmodium Infection
Previous studies have closely examined the metabolism of nitrogenous compounds in the uricolytic pathway following mosquito blood-feeding (Scaraffia et al., 2008; Isoe and Scaraffia, 2013), defining key enzymes involved in the production of uric acid and its eventual breakdown into urea (Figure 2A). Given the differences in uric acid levels between blood-fed and infected mosquitoes in Figure 1, we examined how infection may regulate the expression of key enzymes in the uricolytic pathway (Figure 2A). Relative expression levels of xanthine dehydrogenase (XDH), urate oxidase (UO), allatoinase (ALLN) and allatoicase (ALLC) were analyzed in mosquitoes challenged with a naïve or Plasmodium-infected blood meal at 3, 24, and 48 h post-challenge, with time points representing pre-ookinete invasion, ookinete invasion, and post-ookinete invasion, respectively. UO and ALLC expression was significantly increased in Plasmodium-infected mosquitoes 48 h post-infection when compared to mosquitoes fed on naïve blood (Figure 2B), suggesting that ookinete invasion and/or the formation of early oocysts are responsible for these physiological changes. In contrast, there was no difference in the relative expression of XDH and ALLN (Figure 2B). Since UO is required for the initial step in uric acid metabolism (Figure 2A), its increased expression may account for the reduced uric acid levels following Plasmodium infection (Figure 1).
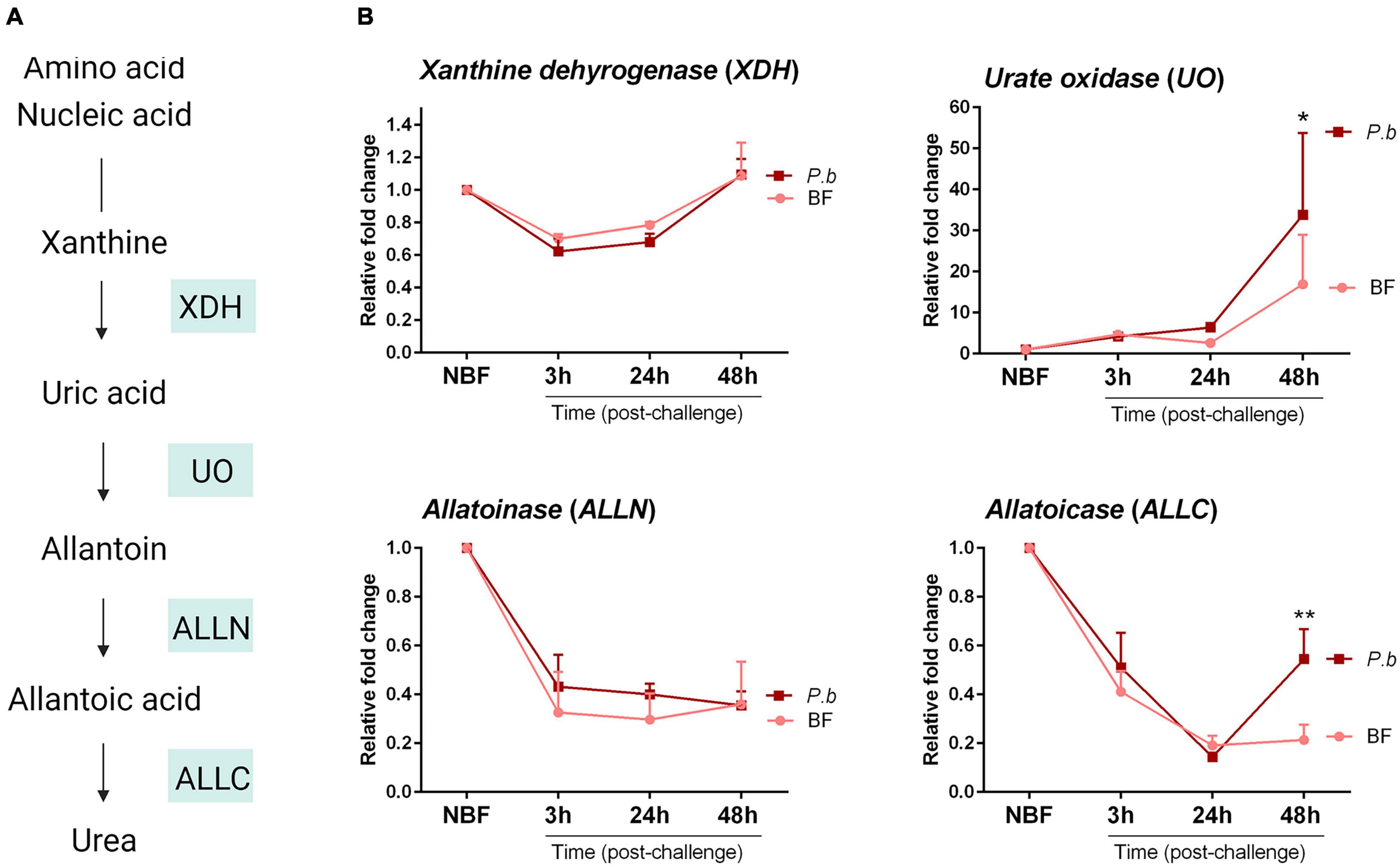
Figure 2. Gene expression analysis of uricolytic pathway enzymes following blood-feeding and P. berghei infection. (A) Overview of the intermediates and enzymes involved in nitrogen metabolism of amino acids and nucleic acids into urea. Genes encoding key enzymes: xanthine dehydrogenase (XDH), urate oxidase (UO), allatoinase (ALLN), and allatoicase (ALLC), are shown in shaded boxes. (B) Gene expression analysis of XDH, UO, ALLN, and ALLC under sugar-fed (NBF) conditions or 3, 24, and 48 h after feeding on a naïve or P. berghei-infected blood meal. Data from three independent experiments are displayed as the mean ± SEM and were analyzed using a two-way repeated-measures ANOVA followed by Sidak’s multiple comparison to determine significant differences. Asterisks denote significance (*P < 0.05, **P < 0.01). Data analysis was performed using GraphPad Prism 7.
Urate oxidase-Silencing Increases Levels of Uric Acid and Enhances Plasmodium Survival
To more closely examine the role of UO on uric acid metabolism and its effects on Plasmodium infection, we performed RNAi to silence UO expression. Following the injection of dsRNA, UO expression was significantly reduced 2 days post-injection (Figure 3A). To confirm the role of UO in uric acid metabolism, we demonstrate that UO-silencing results in increased uric acid levels in excreta at 48 h post-infection (Figure 3B) as well as the accumulation of uric acid in UO-silenced mosquitoes (Figure 3C). When UO-silenced mosquitoes were challenged with P. berghei infection, there were significantly higher oocyst numbers (Figure 3D), suggesting that the higher accumulation of uric acid in UO-silenced mosquitoes promoted increased oocyst survival similar to previous experiments when uric acid was administered through sugar feeding (Molina-Cruz et al., 2008). Therefore, these findings indicate that mosquito host manipulation of the oxidative environment following infection via UO regulation is a novel defense mechanism to decrease Plasmodium survival.
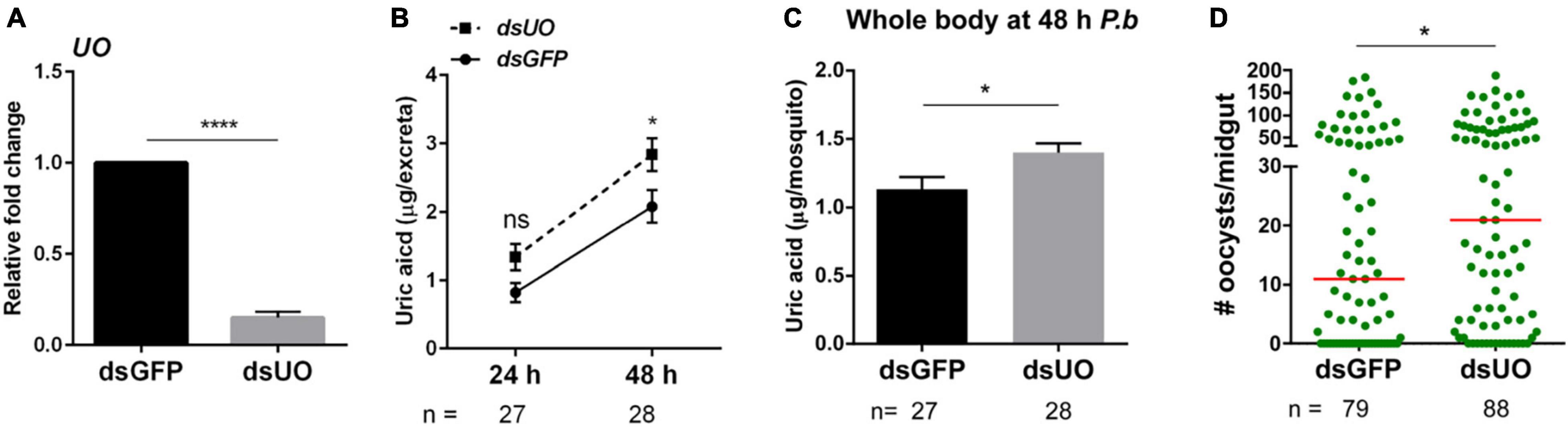
Figure 3. Urate oxidase (UO) silencing increases uric acid levels and Plasmodium oocyst survival. To determine whether accumulation of uric acid in the mosquito promotes the success of oocyst survival, Urate oxidase (UO) silencing was validated by qRT-PCR 2 days post-injection of dsRNA (A), resulting in the increase in uric acid measured in excreta (B) and whole mosquitoes (C) as compared to dsGFP controls. When UO-silenced mosquitoes were challenged with P. berghei, we observed a significant increase in oocyst survival when compared to GFP controls at 8 days post-infection (D). qRT-PCR and whole mosquito uric acid data were analyzed using an unpaired t-test, while uric acid excreta data were analyzed using a two-way repeated-measures ANOVA followed by Sidak’s multiple comparison test. Data are displayed as the mean ± SEM of three independent experiments. Differences in oocyst survival were analyzed using Mann–Whitney analysis, with median oocyst numbers represented by the horizontal red line. Asterisks denote significance (*P < 0.05, ****P < 0.0001). n, number of individual mosquitoes examined. Data analysis was performed using GraphPad Prism 7.
The Mosquito Microbiota Does Not Influence Uric Acid Metabolism
Previous studies have argued that bacteria present in the midgut microbiota may contribute to immune responses initiated in the mosquito following Plasmodium infection (Dong et al., 2009; Rodrigues et al., 2010; Barletta et al., 2019), supporting that bacteria may contribute to the increase in UO expression following Plasmodium infection. This is further supported by data showing that bacterial infections upregulate UO expression in Drosophila (Chambers et al., 2012). Therefore, we wanted to explore whether the microbiota contributed to the differences in uric acid metabolism following infection (Figure 1). To approach this question, we first examined 16s rRNA expression, which serves as a proxy to assess bacteria numbers (Blumberg et al., 2013; Smith et al., 2015; Reynolds et al., 2020a), in naïve blood-fed and Plasmodium-infected blood-fed mosquitoes. Both blood-fed and infected mosquitoes displayed increased bacterial proliferation when compared to sugar-fed (NBF) mosquitoes, although infection status had no effect on bacteria numbers (Figure 4A). We next examined whether aseptic mosquitoes treated with antibiotics displayed any differences in uric acid metabolism. While bacteria numbers were significantly reduced following antibiotic treatment (Figure 4B), aseptic mosquitoes displayed no difference in UO expression (Figure 4C), or uric acid production (Figure 4D). Therefore, our results suggest that the midgut microbiota have no influence on uric acid metabolism, arguing that Plasmodium infection is solely responsible for the changes to mosquito physiology that result in the reduction of uric acid.
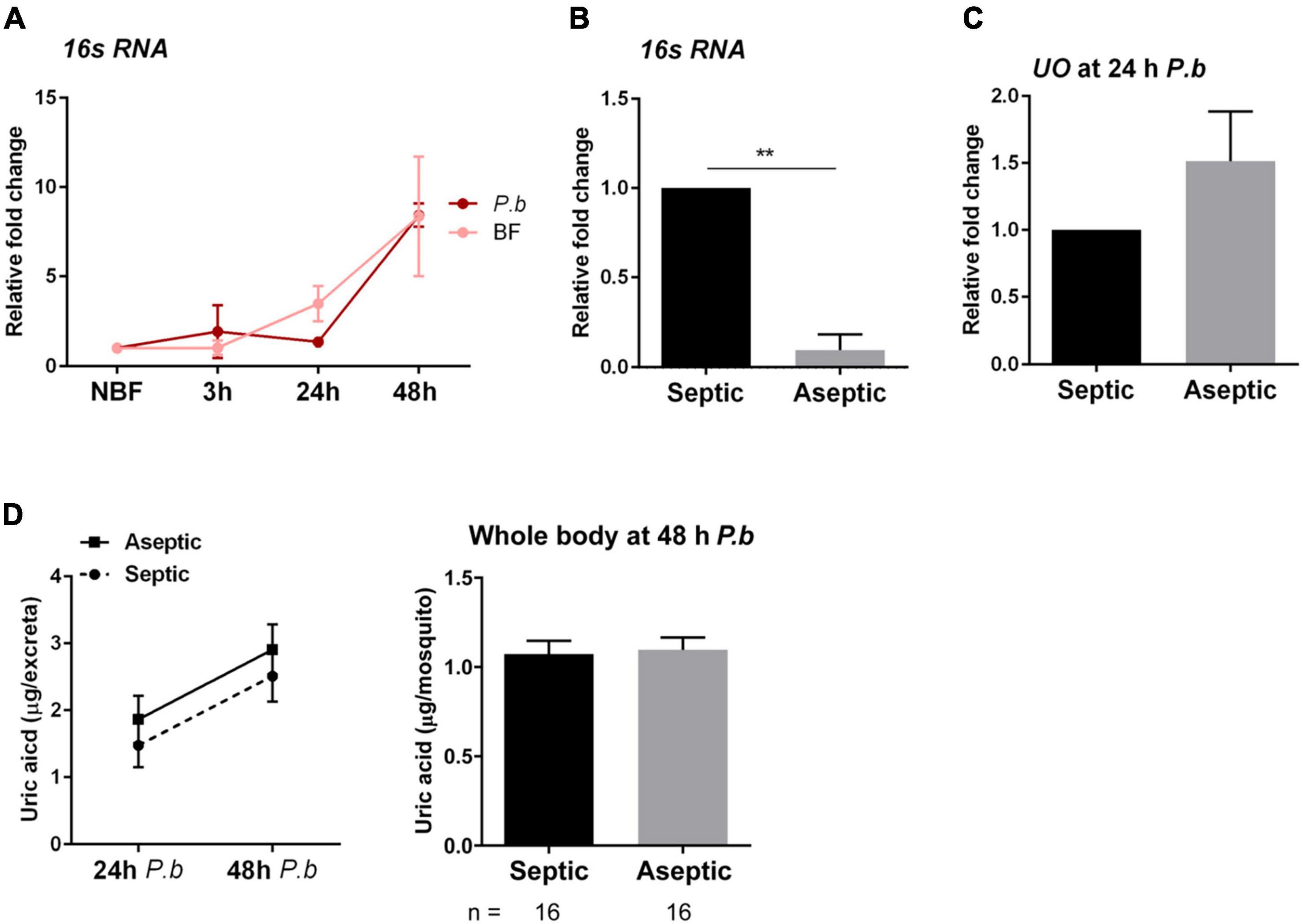
Figure 4. The mosquito microbiota does not influence uric acid production. The impacts of blood-feeding and P. berghei infection on the mosquito microbiome were analyzed in whole mosquitoes by the relative quantification of bacterial 16s rRNA via qRT-PCR (A). Mosquitoes were examined from sugar-fed (NBF) conditions or 3, 24, and 48 h after feeding on a naïve or P. berghei-infected blood meal. To determine the contribution of the mosquito microbiota to the metabolism of uric acid, mosquitoes were treated with antibiotics in 10% sucrose for 3 days (aseptic), and compared to mosquitoes maintained on 10% sucrose alone (septic) through the relative quantification of bacterial 16s rRNA (B). The reduction of microbiota levels did not influence urate oxidase (UO) expression (C), or the production of uric acid (D), measured in both the excreta and the whole mosquito. For all experiments, data were from three independent experiments and displayed as the mean ± SEM. Data examining the 16s rRNA levels (A) and uric acid in excreta (D) were analyzed using a two-way repeated-measures ANOVA followed by a Sidak’s multiple comparison test, while an unpaired t-test was used for all other experiments to determine significance. Asterisks denote significance (**P < 0.01). n, number of individual mosquitoes examined. Data analysis was performed using GraphPad Prism 7.
Discussion
Following blood-feeding, the conversion of blood-derived amino acids into lipids and carbohydrates is an essential physiological process needed for egg production. During this process, amino acids are deaminated, resulting in the production of toxic nitrogenous waste that must be excreted by the mosquito vector as uric acid, urea, or as free ammonia (Scaraffia, 2016). While uric acid can be excreted without further modification, uric acid can also be metabolized into allantoin, allantoic acid, and ultimately to urea through several enzymes involved in a uricolytic pathway (Scaraffia et al., 2008; Isoe and Scaraffia, 2013).
Here, we demonstrate that An. gambiae modulate uric acid levels through the regulation of UO expression in response to P. berghei infection. Although the mechanism is currently unknown, our data suggest that the mosquito host responds to parasite infection by upregulating UO expression, the initial enzyme responsible for uric acid degradation (Scaraffia et al., 2008; Isoe and Scaraffia, 2013). As a result, uric acid levels are decreased, creating an unfavorable environment for Plasmodium survival in the mosquito host (summarized in Figure 5). Similar physiological mechanisms have also been described in Drosophila, where uric acid levels were decreased following the induction of UO expression in response to bacterial challenge (Lang et al., 2019), suggesting that the manipulation of uric acid metabolism is an important defense mechanism of insects against pathogen infection.
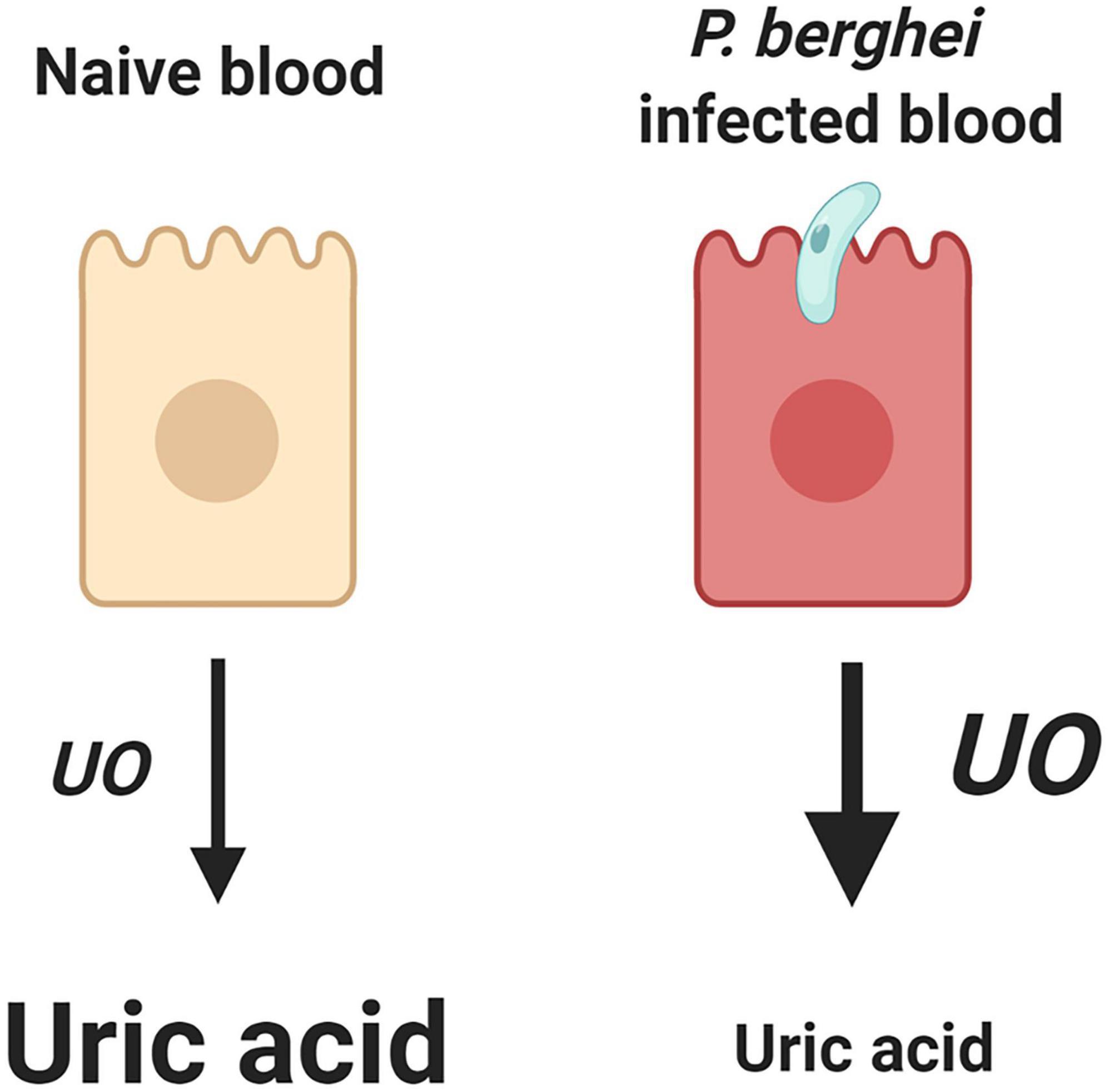
Figure 5. Summary of uric acid metabolism in response to a naïve or P. berghei-infected blood meal. We demonstrate that the mosquito host modulates levels of uric acid through the regulation of urate oxidase (UO), where upon infection UO expression is increased, resulting in less uric acid, which consequently limits malaria parasite survival. Created with BioRender.com.
Uric acid has previously been described as a potent antioxidant across species, scavenging free radicals to maintain redox homeostasis (Ames et al., 1981; Hilliker et al., 1992). With the production of reactive oxygen and nitrogen metabolites in mosquitoes following blood meal digestion (Sadrzadeh et al., 1984; DeJong et al., 2007; Bottino-Rojas et al., 2015) or in response to Plasmodium infection (Luckhart et al., 1998; Kumar et al., 2003; Molina-Cruz et al., 2008), it is not surprising that mosquitoes are able to modify levels of uric acid and other detoxifying enzymes (such as superoxide dismutase, peroxidases, and catalase) to maintain physiological homeostasis. Previous studies have demonstrated that the supplementation of uric acid can improve Plasmodium oocyst survival (Peterson et al., 2007; Molina-Cruz et al., 2008), similar to our results in which UO, the enzyme responsible for the degradation of uric acid, was silenced via RNAi.
Previous studies have suggested that increased oxidative stress can negatively influence mosquito fecundity (DeJong et al., 2007; Champion and Xu, 2018). Although we did not examine the influence of UO-silencing on mosquito fecundity in this study, the reduction in uric acid levels following malaria parasite infection may contribute to the lower fecundity of mosquitoes infected with P. berghei (Hogg and Hurd, 1995, 1997; Ahmed et al., 2002). This is supported by a recent study in which reduced uric acid levels impaired egg production in kissing bugs (Gandara et al., 2021). As a result, further experiments are required to more closely examine the influence of uric acid levels on mosquito fecundity. In addition, potential differences between P. berghei and P. falciparum infection may have different impacts on mosquito physiology, thus warranting further exploration.
While previous studies in Drosophila (Chambers et al., 2012) and Anopheles coluzzii (Chabanol et al., 2020) have, respectively suggested uric acid production and nitrogen metabolism are influenced by bacteria, our experiments using the administration of antibiotics to deplete the microbiota did not alter UO expression or uric acid metabolism. These results suggest that the changes in uric acid production following Plasmodium infection are independent of the microbiota and are produced by yet unknown mechanisms in response to malaria parasite challenge.
Additional components of the uricolytic pathway may also influence mosquito physiology and pathogen infection, yet have not been fully explored. Previous studies in An. stephensi demonstrate that allantoin, a downstream product of uric acid metabolism, does not influence malaria parasite infection (Peterson et al., 2007). However, the role of allantoic acid on parasite survival has not previously been examined. Our data demonstrating the increased expression of ALLC following P. berghei challenge suggests that allantoic acid may also influence parasite infection and is a target of future work.
In summary, we demonstrate that the mosquito host modulates uric acid metabolism in response to malaria parasite infection through the regulation of urate oxidase (UO) activity, limiting P. berghei survival. These new insights connect previous observations of the role of uric acid as a determinant of malaria parasite infection, highlighting the importance of uric acid metabolism in defining mosquito vector competence to Plasmodium infection.
Data Availability Statement
The original contributions presented in the study are included in the article/Supplementary Material, further inquiries can be directed to the corresponding authors.
Ethics Statement
The animal study was reviewed and approved by the Animal Care and Use Committee at Iowa State University.
Author Contributions
HK performed the experiments. HK and RS conceived the experiments, analyzed the data, and wrote the manuscript. Both authors contributed to the article and approved the submitted version.
Funding
This work was supported by a Postdoctoral Association Seed Grant Award from Iowa State University (to HK) and R21AI44705 (to RS) from the National Institutes of Health, National Institute of Allergy and Infectious Diseases.
Conflict of Interest
The authors declare that the research was conducted in the absence of any commercial or financial relationships that could be construed as a potential conflict of interest.
Publisher’s Note
All claims expressed in this article are solely those of the authors and do not necessarily represent those of their affiliated organizations, or those of the publisher, the editors and the reviewers. Any product that may be evaluated in this article, or claim that may be made by its manufacturer, is not guaranteed or endorsed by the publisher.
Supplementary Material
The Supplementary Material for this article can be found online at: https://www.frontiersin.org/articles/10.3389/fphys.2021.821869/full#supplementary-material
Footnotes
References
Ahmed, A. M., Baggott, S. L., Maingon, R., and Hurd, H. (2002). The costs of mounting an immune response are reflected in the reproductive fitness of the mosquito Anopheles gambiae. Oikos 97, 371–377.
Ames, B. N., Cathcart, R., Schwiers, E., and Hochstein, P. (1981). Uric acid provides an antioxidant defense in humans against oxidant- and radical-caused aging and cancer: A hypothesis. Proc. Natl. Acad. Sci. U. S. A. 78, 6858–6862. doi: 10.1073/pnas.78.11.6858
Attardo, G. M., Hansen, I. A., and Raikhel, A. S. (2005). Nutritional regulation of vitellogenesis in mosquitoes: Implications for anautogeny. Insect Biochem. Mole. Biol. 2005, 661–675. doi: 10.1016/j.ibmb.2005.02.013
Barletta, A. B. F., Trisnadi, N., Ramirez, J. L., and Barillas-Mury, C. (2019). Mosquito midgut prostaglandin release establishes systemic immune priming. iScience 19, 54–62. doi: 10.1016/j.isci.2019.07.012
Blumberg, B. J., Trop, S., Das, S., and Dimopoulos, G. (2013). Bacteria- and IMD pathway-independent immune defenses against Plasmodium falciparum in Anopheles gambiae. PLoS One 8:e0072130. doi: 10.1371/journal.pone.0072130
Bottino-Rojas, V., Talyuli, O. A. C., Jupatanakul, N., Sim, S., Dimopoulos, G., Venancio, T. M., et al. (2015). Heme signaling impacts global gene expression, immunity and dengue virus infectivity in Aedes aegypti. PLoS One 10:e0135985. doi: 10.1371/journal.pone.0135985
Chabanol, E., Behrends, V., Prévot, G., Christophides, G. K., and Gendrin, M. (2020). Antibiotic treatment in Anopheles coluzzii affects carbon and nitrogen metabolism. Pathogens 9:679. doi: 10.3390/pathogens9090679
Chambers, M. C., Song, K. H., and Schneider, D. S. (2012). Listeria monocytogenes infection causes metabolic shifts in Drosophila melanogaster. PLoS One 7:e0050679. doi: 10.1371/journal.pone.0050679
Champion, C. J., and Xu, J. (2017). The impact of metagenomic interplay on the mosquito redox homeostasis. Free Radic. Biol. Med. 105, 79–85. doi: 10.1016/j.freeradbiomed.2016.11.031
Champion, C. J., and Xu, J. (2018). Redox state affects fecundity and insecticide susceptibility in Anopheles gambiae. Sci. Rep. 8:13054. doi: 10.1038/s41598-018-31360-2
DeJong, R. J., Miller, L. M., Molina-Cruz, A., Gupta, L., Kumar, S., and Barillas-Mury, C. (2007). Reactive oxygen species detoxification by catalase is a major determinant of fecundity in the mosquito Anopheles gambiae. Proc. Natl. Acad. Sci. U. S. A. 104, 2121–2126. doi: 10.1073/pnas.0608407104
Dong, Y., Manfredini, F., and Dimopoulos, G. (2009). Implication of the mosquito midgut microbiota in the defense against malaria parasites. PLoS Pathog. 5:e1000423. doi: 10.1371/journal.ppat.1000423
Eleftherianos, I., Heryanto, C., Bassal, T., Zhang, W., Tettamanti, G., and Mohamed, A. (2021). Haemocyte-mediated immunity in insects: Cells, processes and associated components in the fight against pathogens and parasites. Immunology 164, 401–432. doi: 10.1111/imm.13390
Gandara, A. C. P., Dias, F. A., de Lemos, P. C., Stiebler, R., Bombaça, A. C. S., Menna-Barreto, R., et al. (2021). Urate and NOX5 control blood digestion in the hematophagous insect Rhodnius prolixus. Front. Physiol. 12:633093. doi: 10.3389/fphys.2021.633093
Handel, E. (1975). Direct determination of uric acid in fecal material. Biochem. Med. 12, 92–93. doi: 10.1016/0006-2944(75)90099-x
Hilliker, A. J., Duyf, B., Evans, D., and Phillips, J. P. (1992). Urate-null rosy mutants of Drosophila melanogaster are hypersensitive to oxygen stress. Proc. Natl. Acad. Sci. U. S. A. 89, 4343–4347. doi: 10.1073/pnas.89.10.4343
Hogg, J. C., and Hurd, H. (1995). Malaria-induced reduction of fecundity during the first gonotrophic cycle of Anopheles stephensi mosquitoes. Med. Vet. Entomol. 9, 176–180. doi: 10.1111/j.1365-2915.1995.tb00175.x
Hogg, J. C., and Hurd, H. (1997). The effects of natural Plasmodium falciparum infection on the fecundity and mortality of Anopheles gambiae s.l. in north east Tanzania. Parasitology 114, 325–331. doi: 10.1017/s0031182096008542
Isoe, J., and Scaraffia, P. Y. (2013). Urea synthesis and excretion in Aedes aegypti mosquitoes are regulated by a unique cross-talk mechanism. PLoS One 8:e0065393. doi: 10.1371/journal.pone.0065393
Kumar, S., Christophides, G. K., Cantera, R., Charles, B., Han, Y. S., Meister, S., et al. (2003). The role of reactive oxygen species on Plasmodium melanotic encapsulation in Anopheles gambiae. Proc. Natl. Acad. Sci. U. S. A. 100, 14139–14144. doi: 10.1073/pnas.2036262100
Kwon, H., Arends, B. R., and Smith, R. C. (2017). Late-phase immune responses limiting oocyst survival are independent of TEP1 function yet display strain specific differences in Anopheles gambiae. Parasit. Vectors 10:369. doi: 10.1186/s13071-017-2308-0
Kwon, H., and Smith, R. C. (2019). Chemical depletion of phagocytic immune cells in Anopheles gambiae reveals dual roles of mosquito hemocytes in anti- Plasmodium immunity. Proc. Natl. Acad. Sci. 116, 14119–14128. doi: 10.1073/pnas.1900147116
Lang, S., Hilsabeck, T. A., Wilson, K. A., Sharma, A., Bose, N., Brackman, D. J., et al. (2019). A conserved role of the insulin-like signaling pathway in diet-dependent uric acid pathologies in Drosophila melanogaster. PLoS Genet. 15:e1008318. doi: 10.1371/journal.pgen.1008318
Livak, K. J., and Schmittgen, T. D. (2001). Analysis of relative gene expression data using real-time quantitative PCR and. Methods 25, 402–408. doi: 10.1006/meth.2001.1262
Luckhart, S. H. L., Odovotz, Y. O. V., Ui, L. I. C., Osenberg, R. O. R., Vodovotz, Y., Cui, L., et al. (1998). The mosquito Anopheles stephensi limits malaria parasite development with inducible synthesis of nitric oxide. Proc. Natl. Acad. Sci. U. S. A. 95, 5700–5705. doi: 10.1073/pnas.95.10.5700
MacLeod, E. T., Maudlin, I., Darby, A. C., and Welburn, S. C. (2007). Antioxidants promote establishment of trypanosome infections in tsetse. Parasitology 134, 827–831. doi: 10.1017/S0031182007002247
Molina-Cruz, A., DeJong, R. J., Charles, B., Gupta, L., Kumar, S., Jaramillo-Gutierrez, G., et al. (2008). Reactive oxygen species modulate Anopheles gambiae immunity against bacteria and Plasmodium. J. Biol. Chem. 283, 3217–3223. doi: 10.1074/jbc.M705873200
Peterson, T. M. L., Gow, A. J., and Luckhart, S. (2007). Nitric oxide metabolites induced in Anopheles stephensi control malaria parasite infection. Free Radic. Biol. Med. 42, 132–142. doi: 10.1016/j.freeradbiomed.2006.10.037
Reynolds, R. A., Kwon, H., Alves e Silva, T. L., Olivas, J., Vega-Rodriguez, J., and Smith, R. C. (2020a). The 20-hydroxyecdysone agonist, halofenozide, promotes anti-Plasmodium immunity in Anopheles gambiae via the ecdysone receptor. Sci. Rep. 10, 21084. doi: 10.1038/s41598-020-78280-8
Reynolds, R. A., Kwon, H., and Smith, R. C. (2020b). 20-Hydroxyecdysone primes innate immune responses that limit bacterial and malarial parasite survival in Anopheles gambiae. mSphere 5, e983–e919. doi: 10.1128/mSphere.00983-19
Rodrigues, J., Brayner, F. A., Alves, L. C., Dixit, R., and Barillas-Mury, C. (2010). Hemocyte differentiation mediates innate immune memory in Anopheles gambiae mosquitoes. Science 329, 1353–1356. doi: 10.1126/science.1190689
Sadrzadeh, S. M. H., Graf, E., Panter, S. S., Hallaway, P. E., and Eaton, J. W. (1984). Hemoglobin. A biologic Fenton reagent. J. Biol. Chem. 259, 14354–14356.
Scaraffia, P. Y. (2016). Disruption of Mosquito Blood Meal Protein Metabolism. Genetic Control of Malaria and Dengue. Acad. 2016, 253–275.
Scaraffia, P. Y., Tan, G., Isoe, J., Wysocki, V. H., Wells, M. A., and Miesfeld, R. L. (2008). Discovery of an alternate metabolic pathway for urea synthesis in adult Aedes aegypti mosquitoes. Proc. Natl. Acad. Sci. U. S. A. 105, 518–523. doi: 10.1073/pnas.0708098105
Smith, R. C., Barillas-Mury, C., and Jacobs-Lorena, M. (2015). Hemocyte differentiation mediates the mosquito late-phase immune response against Plasmodium in Anopheles gambiae. Proc. Natl. Acad. Sci. 112, E3412–E3420. doi: 10.1073/pnas.1420078112
Taracena, M. L., Bottino-rojas, V., Talyuli, O. A. C., Oliveira, H. M., Anglero, Y. I., Walter-nuno, B., et al. (2018). Regulation of midgut cell proliferation impacts Aedes aegypti susceptibility to dengue virus. PLoS Negl. Trop. Dis. 12:e0006498. doi: 10.1371/journal.pntd.0006498
Upton, L. M., Povelones, M., and Christophides, G. K. (2015). Anopheles gambiae blood feeding initiates an anticipatory defense response to Plasmodium berghei. J. Innate Immun. 7, 74–86. doi: 10.1159/000365331
Van Handel, E., and Klowden, M. J. (1996). Defecation by the mosquito, Aedes aegypti, is controlled by the terminal abdominal ganglion. J. Insect Physiol. 42, 139–142. doi: 10.1016/0022-1910(95)00089-5
Von Dungern, P., and Briegel, H. (2001). Enzymatic analysis of uricotelic protein catabolism in the mosquito Aedes aegypti. J. Insect Physiol. 47, 73–82. doi: 10.1016/s0022-1910(00)00095-0
Whiten, S. R., Eggleston, H., and Adelman, Z. N. (2018). Ironing out the details: Exploring the role of iron and heme in blood-sucking arthropods. Front. Physiol. 8:1134. doi: 10.3389/fphys.2017.01134
Keywords: mosquito physiology, host-pathogen interactions, uric acid, urate oxidase, malaria, Anopheles gambiae, Plasmodium berghei
Citation: Kwon H and Smith R (2022) Anopheles gambiae Actively Metabolizes Uric Acid Following Plasmodium Infection to Limit Malaria Parasite Survival. Front. Physiol. 12:821869. doi: 10.3389/fphys.2021.821869
Received: 24 November 2021; Accepted: 31 December 2021;
Published: 24 January 2022.
Edited by:
Yong Hun J. O., Chonnam National University, South KoreaReviewed by:
Bharat Bhusan Patnaik, Fakir Mohan University, IndiaAmr A. Mohamed, Cairo University, Egypt
Xiaoli Bing, Nanjing Agricultural University, China
Chelsea T. Smartt, University of Florida, United States
Copyright © 2022 Kwon and Smith. This is an open-access article distributed under the terms of the Creative Commons Attribution License (CC BY). The use, distribution or reproduction in other forums is permitted, provided the original author(s) and the copyright owner(s) are credited and that the original publication in this journal is cited, in accordance with accepted academic practice. No use, distribution or reproduction is permitted which does not comply with these terms.
*Correspondence: Hyeogsun Kwon, aHNrd29uQGlhc3RhdGUuZWR1; Ryan Smith, c21pdGhyQGlhc3RhdGUuZWR1