- 1Deanery of Biomedical Sciences, Edinburgh Medical School, Edinburgh University, Edinburgh, United Kingtom
- 2Centre for Inflammation Research, Deanery of Clinical Sciences, Edinburgh Medical School, Edinburgh, United Kingtom
- 3Istituto di Biofisica, Consiglio Nazionale delle Ricerche- CNR, Genoa, Italy
- 4Department of Life and Environmental Science, Faculty of Science and Technology, Bournemouth University, Poole, United Kingtom
Throughout its lifetime the heart is buffeted continuously by dynamic mechanical forces resulting from contraction of the heart muscle itself and fluctuations in haemodynamic load and pressure. These forces are in flux on a beat-by-beat basis, resulting from changes in posture, physical activity or emotional state, and over longer timescales due to altered physiology (e.g. pregnancy) or as a consequence of ageing or disease (e.g. hypertension). It has been known for over a century of the heart’s ability to sense differences in haemodynamic load and adjust contractile force accordingly (Frank, Z. biology, 1895, 32, 370–447; Anrep, J. Physiol., 1912, 45 (5), 307–317; Patterson and Starling, J. Physiol., 1914, 48 (5), 357–79; Starling, The law of the heart (Linacre Lecture, given at Cambridge, 1915), 1918). These adaptive behaviours are important for cardiovascular homeostasis, but the mechanism(s) underpinning them are incompletely understood. Here we present evidence that the mechanically-activated ion channel, Piezo, is an important component of the Drosophila heart’s ability to adapt to mechanical force. We find Piezo is a sarcoplasmic reticulum (SR)-resident channel and is part of a mechanism that regulates Ca2+ handling in cardiomyocytes in response to mechanical stress. Our data support a simple model in which Drosophila Piezo transduces mechanical force such as stretch into a Ca2+ signal, originating from the SR, that modulates cardiomyocyte contraction. We show that Piezo mutant hearts fail to buffer mechanical stress, have altered Ca2+ handling, become prone to arrhythmias and undergo pathological remodelling.
1 Introduction
In most animals over a certain size a heart is necessary to provide the propulsive force for the transport and distribution of materials around the body. The work required of the heart varies in response to bodily demands brought about by changes in activity, emotion, physiology, or by alterations associated with disease. Fluctuations in pressure and haemodynamic load, operating over different timescales require that the heart is able to adapt its performance.
As contraction-relaxation cycles are controlled ultimately by the cycling of Calcium ions (Ca2+) within cardiomyocytes, any change to the Ca2+cycle will affect heart performance. Upon cell membrane depolarization, extracellular Ca2+ enters the cardiomyocyte via sarcolemmal L-type voltage-gated Ca2+ channels, activating further Ca2+ release from the sarcoplasmic reticulum (SR) through the ryanodine receptor type 2 (RyR) in a process known as calcium induced calcium release (Fabiato, 1983). The increase in cytoplasmic Ca2+ activates the myofilaments and leads to contraction of the cell. This conversion of electrical activity into mechanical energy is known as excitation-contraction coupling (EC coupling). There is strong evidence that EC coupling is widely conserved in animal hearts, including invertebrate hearts such as Drosophila (Gu and Singh, 1995; Sullivan et al., 2000; Wolf et al., 2006; Ocorr et al., 2007; Lin et al., 2011; Cammarato et al., 2015; Limpitikul et al., 2018; Pineda et al., 2021). Any shift in the delicate balance in Ca2+ handling will cause a net change in cellular Ca2+ and influence EC coupling. Indeed, mechanisms that modulate EC coupling contribute to the repertoire of adaptive behaviour that exist in cardiac tissue (Frank, 1895; Patterson and Starling, 1914; Allen and Kurihara, 1982).
The Frank-Starling law of the heart is one example (Frank, 1895; Patterson and Starling, 1914). It describes the ability of the heart to sense differences in haemodynamic load and adjust contractile force accordingly. The Frank-Starling mechanism is intrinsic to cardiac tissue as it is maintained in de-innervated and in transplanted mammalian hearts (Pope et al., 1980). Even isolated cardiomyocytes are able to fine-tune contractility in response to mechanical load, revealing that the Frank-Starling mechanism is a cell-intrinsic property (White et al., 1995). Despite having been described for over a century, the mechanistic basis of how cardiomyocytes sense changes in load and transduce these to modulate contractile force is not fully understood. It is known that stretch-induced increase in force production is biphasic: an abrupt increase in force coincides with stretch, which is then followed by a slower response developing over a period of seconds to minutes (the slow force response or SFR) also known as the Anrep effect (Parmley and Chuck, 1973; Allen and Kurihara, 1982; Tucci et al., 1984; White et al., 1995). In comparison to the mechanism underpinning the rapid response—which is relatively well described (Allen and Blinks, 1978; Konhilas et al., 2002; Shiels and White, 2008; Sequeira and van der Velden, 2015)—the SFR mechanism is not well characterized. It is known that SFR is correlated with an increase in magnitude of the systolic Ca2+ transient (Allen and Kurihara, 1982; Allen et al., 1988; Le Guennec et al., 1991), and mechanically-activated ion channels have been invoked as part of the mechanism (Gannier et al., 1994; Calaghan and White, 2004; Ward et al., 2008).
The mechanically-activated ion channels of the Piezo family are possible candidates. Piezo ion channels belong to a class of mechanically-activated non-selective cationic channels conserved widely in animals, plants and other eukaryotic species (Coste et al., 2010). Piezo channels are known to have important mechanosensory functions in broad-ranging developmental and physiological contexts (Kim et al., 2012; Li et al., 2014; Maksimovic et al., 2014; Ranade et al., 2014; Woo et al., 2014; Retailleau et al., 2015; Nonomura et al., 2017; Zeng et al., 2018; Marshall et al., 2020). As Piezo channels have a conductance preference for Ca2+ we considered the simple hypothesis that in the heart Piezo might act to transduce mechanical force into a change in cytoplasmic Ca2+ concentration to modulate contractility (Coste et al., 2010) in response to stretch. Here, we use a Drosophila model to assess the role of Piezo in cardiac mechanotransduction and Ca2+ handling, and discuss its contribution to the SFR of the Frank-Starling law of the heart.
2 Results
2.1 Piezo is a sarcoplasmic reticulum ion channel in cardiac cells
The Drosophila heart is a simple tube-shaped vessel with a little over a hundred cells. It is divided into two regions: a posterior chamber, connected to a narrower region, the aorta, to the anterior (Figure 1A). The heart contains a small number of intracardiac valves and several inflow openings or ostia through which haemolymph flows (Figure 1A) (Rotstein and Paululat, 2016). The major cell-type is the cardiomyocyte, numbering 104 cells in the embryo/larva, which are reduced to 84 cells in the adult due to remodeling during metamorphosis (Rotstein and Paululat, 2016). We find that Piezo is expressed in the heart from late embryonic stages (Figure 1B, in situ hybridization), and maintained throughout the life of the larva and adult (Figures 1C,D, Piezo-Gal4) (Kim et al., 2012; Nagarkar-Jaiswal et al., 2015). Piezo is expressed in all cardiomyocytes and valve cells but not ostia (Figures 1E,F).
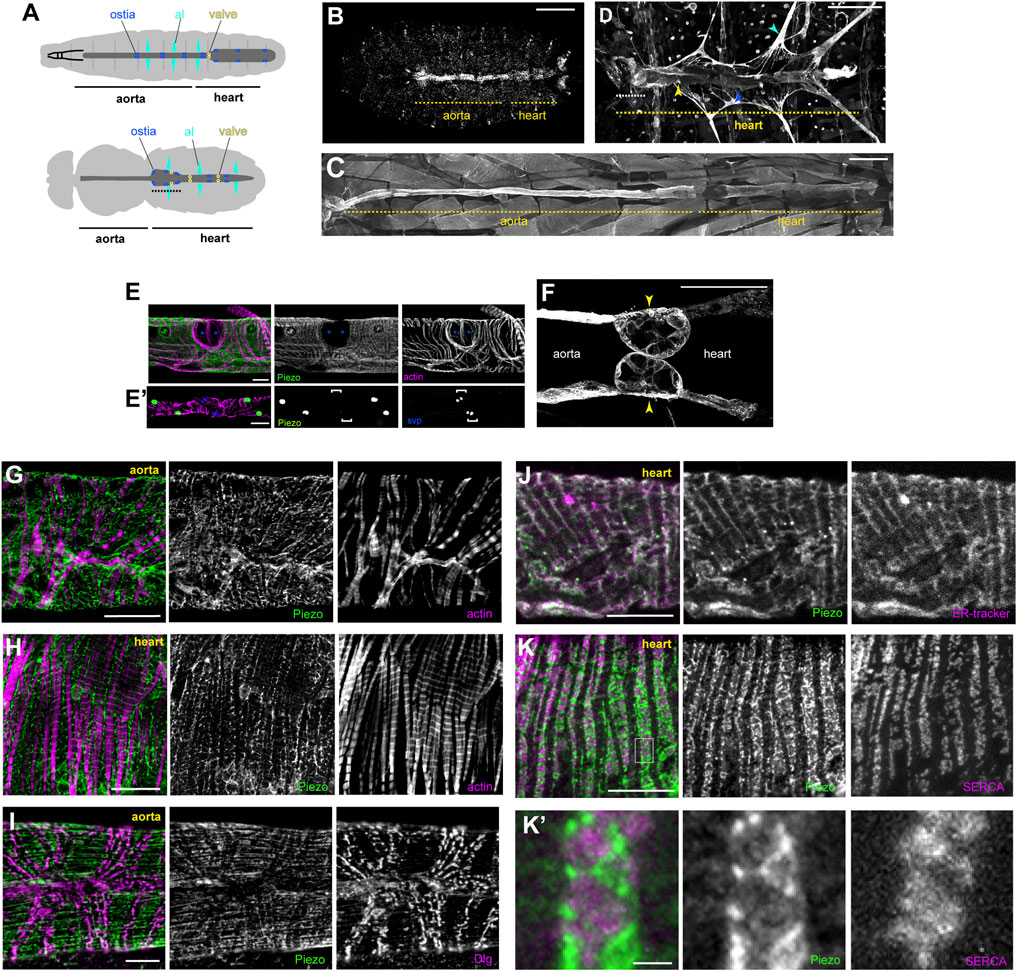
FIGURE 1. Piezo expression in the Drosophila heart. (A) Schematic figure of a Drosophila larva (upper) and adult (lower) depicting the heart, aorta and associated cell types. Cardiomyocytes (dark grey), ostia (blue), intracardiac valves (yellow), and alary muscles (al, cyan) are shown. Conical chamber is indicated with dashed line. (B) Piezo expression in a late stage Drosophila embryo revealed by in situ hybridization chain reaction. (C) Piezo expression in a larva revealed by Piezo-Gal4>UAS-Piezo::GFP. (D) Piezo expression in an adult revealed by Piezo-Gal4>UAS-Piezo::GFP. Conical chamber is indicated with dashed line. (E) Piezo-Gal4>UAS-nRFP (green), seven up-LacZ (blue, bracket in E′) and actin (magenta) reveal Piezo expression in cardiomyocytes but not ostia. (F) Piezo expression in larval intracardiac valves (yellow arrowheads) revealed by Piezo-Gal4>UAS-Piezo::GFP. (G,H) Piezo subcellular localisation revealed by Piezo-Gal4>UAS-Piezo::GFP (green) in the aorta (G) and heart (H) in relation to actin filaments (magenta). (I) Piezo subcellular localisation revealed by Piezo-Gal4>UAS-Piezo::GFP (green) in the aorta relative to T-tubule marker anti-Discs large (Dlg, magenta). (J) Piezo subcellular localisation revealed by Piezo-Gal4>UAS-Piezo::GFP (green) in the heart relative to the SR marker (ER-tracker, magenta). (K) Piezo subcellular localisation revealed by Piezo-Gal4>UAS-Piezo::GFP (green) in the heart relative to the SR marker anti-Serca (magenta). (K′) shows a higher magnification view of the box marked in (K). Scale bars = 100 µm (B, E′), 200 µm (C,D), 50 µm (E,F), 20 μm, (G,H), 10 µm (I–K), 2 µm (K′). For all images anterior is to the left.
To determine Piezo subcellular localisation we used UAS-Piezo:GFP (Kim et al., 2012) and a Piezo-MiMIC line (Nagarkar-Jaiswal et al., 2015) and stained with anti-GFP. Piezo exhibits an intricate net-like pattern that weaves around the myofibrils in cardiomyocytes (Figures 1G,H). Interestingly, Piezo does not localise to the cell surface plasma membrane as expected based on observations made for other cell types (Coste et al., 2010). To pinpoint the subcellular localisation of Piezo we explored the possibility that it localises to the T-tubule network or internal membranes such as the sarcoplasmic reticulum (SR) by carrying out staining for Piezo:GFP along with markers to show T-tubules and SR. T-tubules, revealed by anti-Discs large (Dlg), are arranged in transverse and occasional longitudinal stripes criss-crossing the heart in a pattern which is very distinct from Piezo (Figure 1I), suggesting Piezo does not localise to the T-tubule network. We marked the SR using ER-tracker and an antibody directed against Sarcoendoplasmic Reticulum Calcium Transport ATPase (anti-SERCA). Piezo:GFP overlaps extensively with ER-tracker suggesting SR localisation (Figure 1J). In support of this Piezo:GFP staining pattern closely aligns with the SR marker SERCA (Figure 1K). However it is notable—when viewed at high magnification—that whilst Piezo:GFP closely aligns with SERCA it shows very little direct overlap (Pearson’s coefficient = 0.03, Figure 1K’). Together these data suggest Piezo is an SR-resident channel that exists in an SR-compartment distinct from SERCA. This finding is initially surprising, as previous studies have identified Piezo as a plasma membrane channel (Coste et al., 2010). Indeed, using the same UAS-Piezo:GFP and Piezo-MiMIC lines, we find that Piezo localises to the plasma membrane in salivary glands (Supplementary Figure S1). Although localisation to internal membranes was unexpected, it is not completely without precedent, as the human orthologue (Piezo1/Fam38A) is found on endoplasmic reticular (ER) membranes in HeLa cells and localises to ER when transfected into CHO-K1 cells (McHugh et al., 2010); the rat orthologue (Mib) localises to ER when transfected into C6 glial cells (K et al., 2006); and Piezo has been shown to shuttle between subcellular compartments in MDCK cells, including the ER, in a cell density-dependent manner (Gudipaty et al., 2017). However, the findings presented here are the first to report Piezo localisation to SR membranes in vivo and the first evidence to show Piezo localises to the SR membrane in cardiomyocytes.
2.2 PiezoKO hearts fail to buffer mechanical stress
PiezoKO hearts function well under normal conditions. End diastolic diameter (EDD), end systolic diameter (ESD), length of the cardiac cycle and beats per minute are not significantly different in PiezoKO hearts when compared to controls (Supplementary Figure S2).
As mechanically-activated ion channels are suggested to contribute to cardiac adaptive behaviours, such as the Frank-Starling law, we decided to investigate whether Piezo is involved in adapting to fluctuations in mechanical force. To do this we first exposed the hearts to hypotonic stress to induce osmotic swelling, thereby stretching cell membranes and structures anchored to them (a commonly used method to assess mechanical stretch (Gomis et al., 2008; Jung et al., 2008; Fanchaouy et al., 2009)), and compared responses between PiezoKO and control hearts. For control hearts exposed to hypotonic stress, the diastolic interval lengthens but the majority of hearts continue to beat and maintain synchrony (Figures 2A,B; Supplementary Movie S1). In contrast, 75% of PiezoKO and 60% Piezo-RNAi knockdown hearts undergo diastolic cardiac arrest (Figures 2A,B; Supplementary Movie S2). PiezoKO hearts recover when returned to isotonic solution (measured after 5 min), revealing the phenotype to be one of acute response to hypotonic stress rather than one affecting general tissue viability. As the majority of control hearts are able to sustain heart function under conditions of hypotonic stress, whereas the majority of PiezoKO hearts are not, it suggests the existence of an adaptive mechanism to modulate heart function in the face of mechanical stress, and that this mechanism is dependent on Piezo.
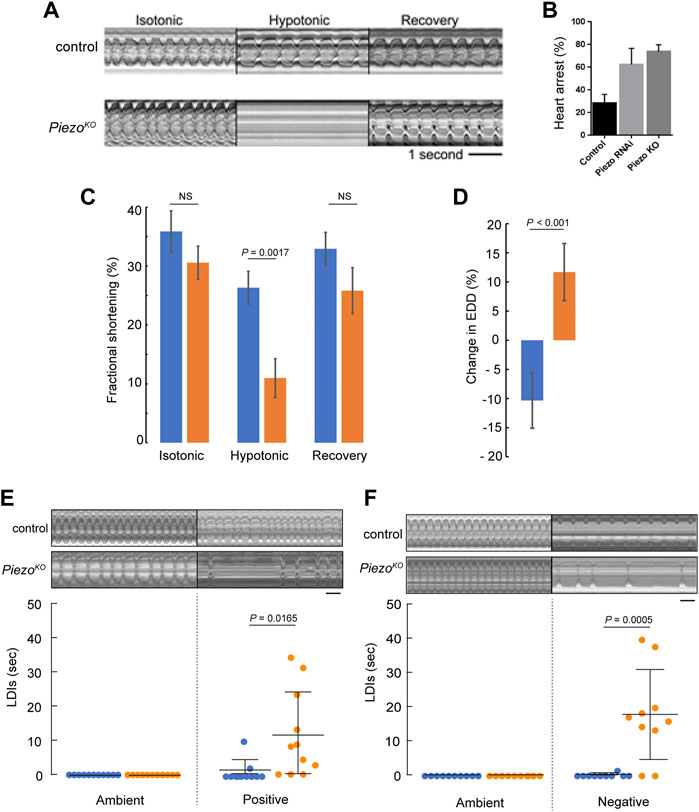
FIGURE 2. Piezo hearts fail to buffer mechanical stress. (A) M-mode kymograph traces for control (upper) and PiezoKO (lower) adult hearts in isotonic, hypotonic and isotonic (recovery) solutions. (B) Percentage of cardiac arrest during hypotonic stretch in control, Piezo-RNAi and PiezoKO animals. (n = 28, 23, and 24). (C) Fractional shortening in the conical chamber under isotonic (left), hypotonic (middle) and isotonic (recovery) conditions for control (blue, n = 12) and PiezoKO (orange, n = 12) hearts. There is no statistical difference in FS for control hearts in isotonic versus hypotonic solution (p = 0.19, n = 12, one-way ANOVA) (D) Response of the hearts to hypotonic stress. Percentage change in end diastolic diameter (EDD) for control (blue) and PiezoKO (orange) hearts measured at the conical chamber (n = 10 and 10). (E) Graph shows quantification of long diastolic intervals (LDIs) for control (blue, n = 11) and PiezoKO (orange, n = 11) hearts under conditions of ambient and positive (+220 mmHg) pressure. Each data point represents an individual heart preparation; the y-axis shows additive time in LDI over a 60 s period for each individual preparation. Typical M-mode kymograph trace for control and PiezoKO adult hearts are shown (upper panel). (F) Graph shows quantification of LDIs for control (blue, n = 10) and PiezoKO (orange, n = 10) hearts under conditions of ambient and negative (−482 mmHg) pressure. Each data point represents an individual heart preparation; the y-axis shows additive time in LDI over a 60 s period for each individual preparation. Typical M-mode kymograph trace for control and PiezoKO adult hearts are shown (upper panel). p-values, unpaired t-test. NS = not significant. Scale bar in A, E, F = 1 s.
To characterise contractile behavior of the heart under conditions of mechanical stress we measured fractional shortening (FS, a measure of contractility describing the fraction of diastolic dimension lost in systole) in the conical chamber (the anterior-most region of the adult heart, Figures 1A,D) in isotonic versus hypotonic conditions. Under isotonic conditions FS is comparable between genotypes (trending towards being reduced in PiezoKO but not reaching statistical significance, 35.9% ± 3.5% versus 30.6% ± 2.8% for control and PiezoKO hearts respectively; p = 0.32, n = 12, Figure 2C). When subjected to hypotonic stress FS in control hearts was not statistically different from isotonic conditions. In contrast, under hypotonic stress FS is significantly decreased in PiezoKO hearts (26.3 ± 2.8% for control versus 11.0 ± 3.2% for PiezoKO hearts respectively; p < 0.01, n = 12, Figure 2C). When returned to isotonic solution PiezoKO hearts recover. In addition, the impairment of FS under hypotonic stress in the PiezoKO hearts is associated with a qualitatively different response of the conical chamber, in that PiezoKO conical chambers relax beyond the baseline EDD seen in isotonic conditions (wider by 11.7 ± 4.9%), whereas control hearts are more contracted under hypotonic conditions relative to the EDD in isotonic conditions (narrower by 10.3 ± 4.8%; p < 0.001, n = 12) (Figure 2D). In summary these data indicate that PiezoKO hearts function almost normally under isotonic conditions but their response to hypotonicity is a widening at the end of diastole and reduced contraction during systole. This feature of widening at the end of diastole is typical of hearts treated with EDTA (Madan et al., 2020) and combined with impaired contraction as well as the subcellular location of Piezo, the data are consistent with the PiezoKO cardiomyocytes struggling to modulate Ca2+ release from internal stores as a response to hypotonic stress.
The effects of modulating ambient pressure were also assessed by exposing semi-intact adult heart preparations to either an increase (+29 KPa) or decrease (−64 KPa) in atmospheric pressure for a 10-min period and recording the number/duration of abnormal heart pauses (long diastolic intervals or LDIs) in 1 minute. (LDIs are defined as diastolic pauses greater than one second—a value approximately three times longer than the normal diastolic interval (Fink et al., 2009)). Raising ambient pressure induces only a small number of LDIs in control hearts (Figure 2E). In contrast, raising ambient pressure induces many LDIs in PiezoKO hearts (Figure 2E). A similar result was found for reducing ambient pressure (Figure 2F).
Together, these data provide compelling evidence that Piezo is part of a mechanism that modulates cardiac contractility to buffer the heart against mechanical stress.
2.3 Pharmacological inhibition of Piezo causes heart arrest under mechanical stress
As an alternative strategy to perturb Piezo activity we tested the effects of the spider venom peptide GsMTx4 on heart function. GsMTx4 is a potent inhibitor of cationic mechanosensitive ion channels, including mammalian Piezo1 and 2 (Bae et al., 2011; Lee et al., 2014; Alcaino et al., 2017). Application of GsMTx4 to the bathing medium does not induce heart arrest at ambient or negative pressures (Figure 3A). This is in line with previous studies showing that GsMTx4 had little effect either on stretch induced Ca2+ spark rate in rat ventricular cardiomyocytes (Iribe et al., 2009) or on heart rate in Drosophila larval hearts (de Castro et al., 2019). However, these results are consistent with our finding that Piezo localises to cardiomyocyte SR membranes, and would therefore be inaccessible to exogenous peptide. Therefore, to target GsMTx4 intracellularly we generated three Gal4 inducible GsMTx4 transgenic variants: a full-length (containing a signal peptide and therefore likely to be secreted, UAS-GsMTx4-FL), a non-secreted variant consisting of the active peptide alone (UAS-GsMTx4-AP) and an SR-targeted (UAS-GsMTx4-SR) (Beqja et al., 2020). We expressed each variant in the heart (using Piezo-Gal4 or Tin-Gal4) and analysed cardiac function at ambient and negative pressure. The phenotype when driving intracellular GsMTx4 mimics the PiezoKO phenotype (Figures 3B,C). All three variants, driven by either Piezo-Gal4 or Tin-Gal4 behave in a similar manner: they generally induce LDIs at ambient pressure, which are exacerbated further under negative pressure (Figures 3B,C). Of the three, the SR-targeted variant (UAS-GsMTx4-SR) produces the most potent phenotype, inducing long periods of diastolic arrest at both ambient and negative pressures. These data provide additional support for the existence of a Piezo-dependent mechanosensitive mechanism centred on the cardiomyocyte SR. Further, as Tin-Gal4 is cardiac-specific they also show Piezo activity is required autonomously in the heart. Interestingly, the GsMTx4-induced phenotypes are stronger than PiezoKO (Figure 2F), which indicates other mechanosensitive ion channels (also targeted by GsMTx4) might operate alongside Piezo.
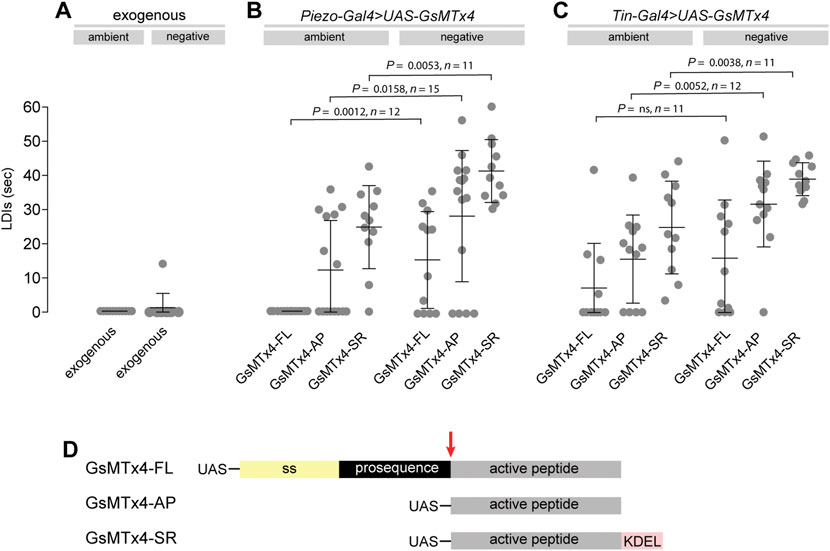
FIGURE 3. Pharmacological inhibition of Piezo causes heart arrest under mechanical stress. (A–C) Quantification of LDIs with GsMTx-4 applied exogenously (A, 5mM; n = 12), or when expressed from a transgenic construct using Piezo-Gal4 (B) or Tin-Gal4 (C), under conditions of ambient and negative (−482 mmHg) pressure. Each data point represents an individual heart preparation; the y-axis shows additive time in LDI over a 60 s period for each individual preparation. p-values, unpaired t-test. (D) Schematic drawing of the GsMTx-4 transgenic constructs used in the study: secreted (GsMTx4-FL); non-secreted (GsMTx4-AP); SR-targeted (GsMTx4-SR). UAS = Upstream Activation Sequence; ss = signal sequence; KDEL = ER/SR translocation signal; red arrow = mature peptide cleavage site.
2.4 Elevated systolic Ca2+ transients in response to hypotonic stress require Piezo
Our data indicate that Piezo is part of a mechanism to modulate cardiac contractility in response to mechanical stress. Given that Piezo is a Ca2+ permeable channel and is a SR-resident channel in cardiomyocytes, we explored the hypothesis that Piezo acts to transduce mechanical force into biochemical signal (change in cytoplasmic Ca2+ concentration) to modulate contractility in response to stretch. This hypothesis is in line with the increase in cytoplasmic Ca2+ in mammalian cardiomyocytes that occurs in response to mechanical stretch, including hypotonic stretch (Allen and Kurihara, 1982; Allen et al., 1988; Sadoshima et al., 1996; Brette et al., 2000). To test whether modulation of Ca2+ handling is involved in the Piezo-dependent response to stretch in Drosophila cardiomyocytes we compared systolic Ca2+ transients before (i.e. in isotonic solution) and during hypotonic stress in control and PiezoKO hearts. In control hearts there is a large and significant increase in the peak amplitude of the Ca2+ transient in response to hypotonic stress. The systolic calcium transient amplitude is 67 ± 10% (p < 0.0001, n = 18) larger than the pre-hypotonic (isotonic) value. In contrast, there is no change in the level of the Ca2+ transient in response to hypotonic stress in PiezoKO cardiomyocytes. The systolic calcium transient amplitude is 3 ± 4% (p = 0.648, n = 19) smaller compared to the pre-hypotonic (isotonic) value (Figure 4A). Figure 4B shows representative traces of Ca2+ transients for control (upper) and PiezoKO (lower) cardiomyocytes before and during hypotonic stress. During the change in solution the cardiac cycle pauses. When the cycle resumes, the Ca2+ transients in control cardiomyocytes (now stretched due to hypotonic stress) are significantly higher than they were in isotonic solution. This elevation in the Ca2+ transient is abolished in PiezoKO cardiomyocytes (Figure 4). This is not explained by a smaller SR Ca2+ content ([Ca2+]SR) in PiezoKO cardiomyocytes, as [Ca2+]SR in PiezoKO is significantly higher than control cardiomyocytes (Supplementary Figure S4). Rather, it points to a defect in the ability to mobilise Ca2+ from the SR. These data reveal the existence of a Piezo-dependent mechanism that modulates Ca2+ handling in response to hypotonic stress. They support the hypothesis that Piezo is the mechanotransducer that transduces mechanical force into a Ca2+ signal, which then acts on the myofilaments to fine-tune cardiac contraction.
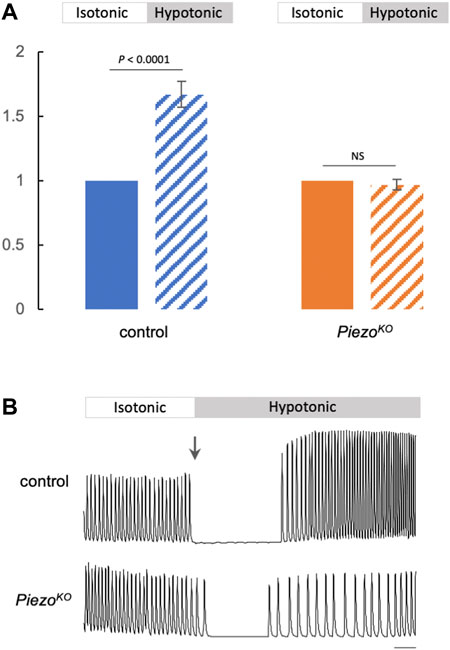
FIGURE 4. Elevated Ca2+ transients in response to hypotonic stretch require Piezo. (A) Summary data comparing Ca2+ transient amplitude in response to hypotonic stretch in control (blue) or PiezoKO (orange) cardiomyocytes (block colour = isotonic, stippling = hypotonic). Approximately 20 Ca2+ transients were measured in isotonic media to calculate mean amplitude per cardiomyocyte. The amplitudes of first 10 transients in hypotonic media were averaged. The resulting value was normalised to the isotonic value to allow comparison between genotypes. n = 18 (control) and 19 (PiezoKO) individual cardiomyocytes from independent heart preparations. (B) Representative Ca2+ traces from control (upper) and PiezoKO (lower) cardiomyocytes in isotonic/hypotonic solution (arrow marks the change from isotonic to hypotonic solution). Time is on x-axis, fluorescence (arbitrary units) on y-axis, scale bar = 1 s. p values, unpaired t-test. NS = not significant.
2.5 Dilated cardiac lumen in PiezoKO
In order to determine whether defects in mechanotransduction resulting from loss of Piezo affect cardiac structure we compared heart morphology in control and PiezoKO animals by measuring heart length and luminal cross-sectional area (Figure 5A). PiezoKO hearts are equivalent in length to control hearts at all life stages (Figure 5B). In contrast, lumen diameter in PiezoKO adult hearts is significantly enlarged compared to controls (Figures 5C,D). This difference is not apparent in first instar larvae—indicating that the phenotype emerges over time (Figures 5C,D). This increase in lumen size could stem from hypertrophic growth of PiezoKO hearts. Cardiac hypertrophy in both mammals and flies is associated with an increase in myocyte ploidy due to elevated endoreplication (Sandritter and Scomazzoni, 1964; Adler and Friedburg, 1986; Adler et al., 1996; Laflamme and Murry, 2011; Yu et al., 2013; Yu et al., 2015). To assess ploidy we measured nuclear cross-sectional area but do not find a difference between PiezoKO and controls (Figure 5E), indicating that the increase in lumen size is not a consequence of hypertrophic growth. Next, we set out to determine the effect of chronic high pressure on heart morphology in PiezoKO animals. We elevated haemocoel pressure by inducing renal failure; this results in defective osmoregulation, gross edema (Figure 5F), culminating in the Drosophila equivalent of ‘chronic hypertension’ (Saxena et al., 2014). Lumen size is significantly larger in hypertensive compared to normotensive flies for both control and PiezoKO (Figures 5C,D). This can be explained, at least in part, by cardiomyocyte hypertrophy; control and PiezoKO cardiomyocyte ploidy increase significantly under hypertensive compared to normotensive conditions (Figure 5E). This indicates hypertrophic growth is an adaptive response to a chronic increase in pressure, and reveals this growth is independent of Piezo. Strikingly however, when comparing flies under conditions of hypertension, the size of the lumen in PiezoKO hearts is grossly enlarged compared to controls (Figures 5C,D). This difference in heart size is not a result of additional hypertrophic growth as cardiomyocyte ploidy is equivalent in PiezoKO and controls under hypertensive conditions (Figure 5E). This corresponds to the overly relaxed conical chambers seen in hypotonic conditions (Figure 2D), a sign that PiezoKO hearts are not buffering Ca2+ properly to cope with the hypertensive conditions. Taken together, these data suggest that defects in mechanotransduction resulting from a lack of Piezo lead to pathological remodelling of heart tissue, culminating in dilation of the heart lumen under both normotensive and hypertensive conditions.
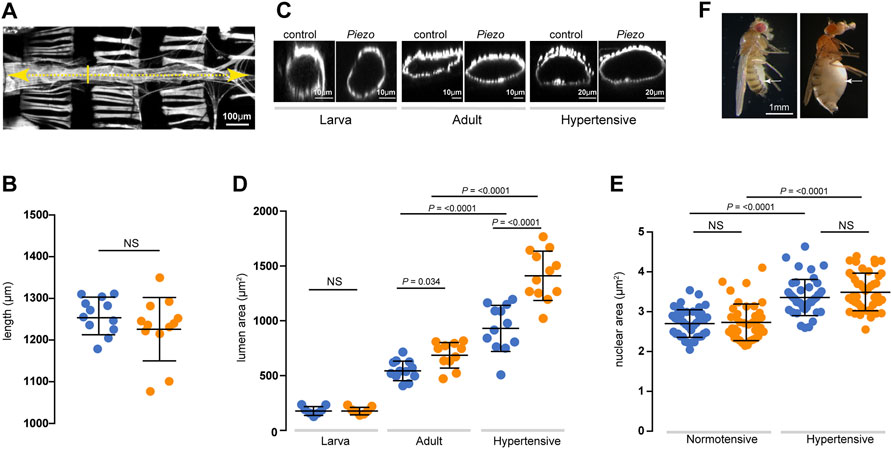
FIGURE 5. Dilation of the heart lumen in PiezoKO. (A) Adult heart stained with phalloidin to reveal morphology. Heart length (yellow dashed line) and lumen cross-sectional area at the position of the 2nd abdominal segment (yellow solid line) were measured. Anterior is to left. (B) Heart length of control (blue) and PiezoKO (orange) adult hearts (n = 12; n = 12). (C) Optical x-z projection of control or PiezoKO hearts stained with phalloidin from first instar larva (left), 3-day old adult (middle) and 10-day old hypertensive adult (right). (D) Lumenal area in control (blue) and PiezoKO (orange) hearts for first instar larvae (n = 7; n = 7), normotensive adults (n = 12; n = 11) and hypertensive adults (n = 12; n = 12). (E) Cardiomyocyte nuclear area in control (blue) and PiezoKO (orange) adult hearts under normotensive (n = 42; n = 45) and hypertensive conditions (n = 43; n = 41) n = number of cardiomyocytes from at least 3 individual hearts. (F) Control (left) and 10-day old hypertensive adult (right); in the hypertensive fly the heart is exposed to high blood pressure in the grossly swollen haemolymph-filled abdomen (white arrow indicates swollen abdomen). p-values, unpaired t-test.
3 Discussion
This work reveals that Piezo is an SR-resident channel with an important role in buffering mechanical stress in the Drosophila heart. We show the Drosophila heart responds to mechanical stretch with an increase in cytoplasmic Ca2+, consistent with studies in mammalian cardiomyocytes (Allen and Kurihara, 1982), and provide evidence that Piezo is part of the mechanism triggering this release. Our data support a simple model in which Piezo transduces mechanical force, such as stretch, into a Ca2+ signal originating from the SR that acts to modulate cardiomyocyte contraction (Figure 6). In the absence of this mechanism the heart is unable to buffer mechanical stress leading to arrhythmic behaviour and cardiac arrest. It has been known for over a century that the heart is able to adapt its behaviour in response to changes in its mechanical environment (Frank, 1895; Anrep, 1912; Patterson and Starling, 1914; Starling, 1918). Despite intense study the mechanistic basis of these adaptive responses is incompletely understood. We suggest Piezo is integral to this response.
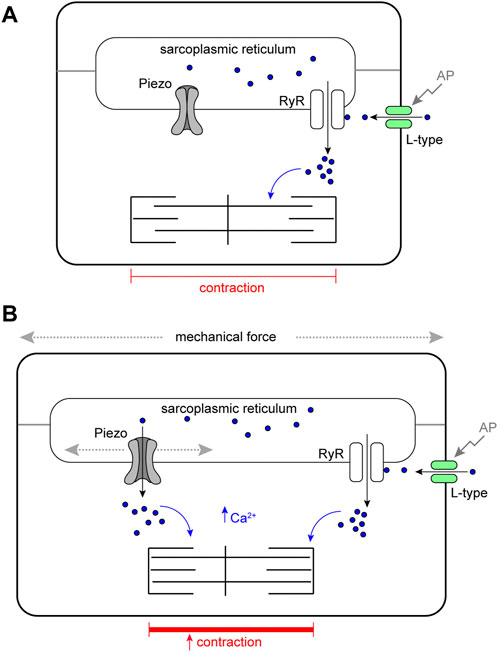
FIGURE 6. Schematic model of Piezo in the Drosophila cardiomyocyte. (A) Cardiomyocyte contraction is controlled by cytoplasmic Ca2+. Depolarisation of the membrane by an action potential (AP) opens sarcolemmal L-type voltage-gated Ca2+ channels activating further Ca2+ release from the sarcoplasmic reticulum (SR) through Ryanodine Receptors (RyR). Cytoplasmic Ca2+ activates the myofilaments and leads to contraction of the cell. In circumstances where mechanical force is within normal range, SR localised Piezo channels are closed. (B) In circumstances where mechanical force (e.g. stretch or compression). exceeds the normal range SR, localised Piezo channels open, additional Ca2+ is released from the SR elevating the cytoplasmic Ca2+ pool, this leads to a stronger contraction.
A novel finding of this work is that Piezo localises to the SR in cardiomyocytes; the major intracellular Ca2+ store. If mechanical force gates the open probability of Piezo, it would imply Piezo acts as an analogue transducer to fine-tune muscle contraction as a function of mechanical force exerted on the cardiomyocyte. An implication of this model is that mechanical force be transmitted to the SR membrane. The most relevant forces in the heart are likely to be stretch and compression, and there is evidence these types of force can be sensed directly by internal mechanosensors (Chen-Izu and Izu, 2017). It is possible that forces could also be transmitted from the sarcolemma to SR membranes through the cytoskeleton. It is notable in this regard that the microtubule cytoskeleton is closely apposed with the SR and required for the transmission of force in rat ventricular cardiomyocytes (Iribe et al., 2009). Further, it is known that mitochondria undergo considerable deformation in cardiomyocytes during the cardiac cycle, showing that force is readily transmitted to internal organelles (Yaniv et al., 2011; Rog-Zielinska et al., 2019). In contrast to the present work, Piezo has been shown (for example (Coste et al., 2010)) or assumed to localise to the plasma membrane. Whilst the work presented here is the first to show that Piezo localises to the SR in cardiomyocytes, other studies have revealed that Piezo localises to ER membranes, or have demonstrated mobility of Piezo between plasma and internal membranes as a function of mechanical force (McHugh et al., 2010; K et al., 2006; Gudipaty et al., 2017). Indeed, whilst a plasma membrane localisation was emphasised in the original characterisation of the Piezos, the majority of Piezo1 was found inside the cell (Coste et al., 2010), consistent with enrichment to organelle membranes. In the future it will be interesting to define the domain(s) and/or post-translational modifications that target Piezo to specific membranes and to determine how this is regulated in different cell types and under different mechanical conditions.
In some of our experiments we have used osmotic swelling as a method to induce stretch. This is a very different provocation to other forms of mechanical deformation such as axial stretch and will culminate in several changes to the cell including, for example, dilution of intracellular contents. We interpret the results of these experiments as part of a mechanism that links cell stretch to contractility (Figure 6) because different mechanical provocations induce defects in PiezoKO hearts (for example Figures 2E,F and Figure 3). However, the results of the osmotic swelling experiments are compatible with an involvement of Piezo in other, distinct functions in the cardiomyocyte such as a direct response to cell swelling or to the sensing of cell volume. It is notable in this regard that rat ventricular myocytes exhibit a modulation of contractility and intracellular Ca2+ concentration after hypoosmotic challenge, including an initial positive inotropic effect coupled to an increase in Ca2+ concentration (Brette et al., 2000), compatible with the data described here. It will be interesting to explore these possible further functions of Piezo in the future.
Although there is evidence Piezo is able to act in isolation (Coste et al., 2012), it is conceivable that it functions together with other channels or other proteins that modulate its activity depending on physiological context. An important question raised by our work is: does Piezo act alone in cardiomyocytes? Interestingly, the cardiac phenotypes reported here for GsMTx4 are stronger than PiezoKO. This is in line with a prior study showing that gadolinium (Gd3+), which is known to block a variety of mechanosensitive ion channels, strongly depressed heart rate in Drosophila (de Castro et al., 2019). This suggests the involvement of additional GsMTx4-sensitive mechanosensitive ion channels. The strongest phenotypes for GsMTx4 reported here are those where the toxin is targeted to the SR, suggesting the focus for other channels would also be the SR. TRP channels are known to be targeted by GsMTx4 (Spassova et al., 2006). It is known the TRPA channel painless is required for mechanotransduction in the Drosophila heart (Senatore et al., 2010), and strong genetic interaction between painless and Piezo in the context of detection of mechanical nociception in Drosophila sensory neurons (Kim et al., 2012) hint that they might also be part of the same pathway in the Drosophila heart. A recent paper showing that TRPC1 localises to the SR in rat cardiomyocytes lends additional support to this hypothesis (Hu et al., 2020). A further candidate is the recently described Piezo-like channel (Hu et al., 2019), however it is not known whether Piezo-like is expressed in Drosophila cardiomyocytes. Future studies analysing combinations of mutations in the fly heart could help define the full repertoire of mechanotransductive mechanisms at work in this tissue.
We also find PiezoKO hearts undergo pathological remodelling culminating in dilated cardiomyopathy. These phenotypes are not the result of defects in heart development in the embryo/early larva as they emerge over time. It is conceivable these defects are also part of a compensatory adaptive response of cardiac tissue emerging in response to a failure of mechanotransduction.
Is Piezo the key mechanotransducer in the heart, and what implications does this have for the Frank-Starling law of the heart? The existence of mechanically-activated ion channel activity in the vertebrate heart has long been suggested based on experimental data and mathematical modelling (Le Guennec et al., 1991; Sigurdson et al., 1992; Ruknudin et al., 1993; Tavi et al., 1996; Tavi et al., 1998; Reed et al., 2014) yet the identity of the channel(s) responsible has not been fully resolved. There are indications that TRP channels might be involved, e.g. TRPC1, TRPC3 and TRPC6 are both expressed at high levels in the heart, with evidence that TRPC3 and TRPC6 contribute to stretch-induced SFR (Ward et al., 2008; Seo et al., 2014; Yamaguchi et al., 2017; Yamaguchi et al., 2018; Hu et al., 2020). Piezo has also been considered a candidate as Piezo1 is found in the vertebrate cardiovascular system and has been shown to be upregulated upon myocardial infarction (Coste et al., 2010; Liang et al., 2017; Rode et al., 2017; Jiang et al., 2021). The data presented here, along with a recent paper by Jiang and others (Jiang et al., 2021), indicate a key role for Piezo as part of the key mechanotransduction mechanism. The data in Jiang et al. on the role of Piezo1 in the mouse heart also show Piezo is important to transduce mechanical force into a Ca2+ signal as part of the heart’s adaptive behaviour to mechanical stress (Jiang et al., 2021). However, in contrast to this work, they find Piezo localised to the T-tubule sarcolemma. Based on this result, along with data showing a requirement for Piezo in reactive oxygen species (ROS) signalling, they propose a hypothesis whereby Piezo acts as an indirect transducer of Ca2+ acting via ROS. Our data support a more direct mechanism of transduction (Figure 6). Whether these discrepancies reveal a difference between species, or provide an example of how Piezo can shuttle between different membranes depending on mechanical conditions remain to be investigated. Given the high degree of physiological conservation between fly and vertebrate hearts (Fink et al., 2009; Wolf and Rockman, 2011; Ocorr et al., 2014; Ahmad, 2017), we suggest that Piezo is a good candidate to modulate cardiac performance in response to mechanical stresses and strains, and might in part contribute to the SFR of the Frank-Starling mechanism.
4 Materials and methods
4.1 Fly strains
Flies were reared on standard media at 25°C. The amorphic allele PiezoKO (Kim et al., 2012) was used for the majority of experiments; this was backcrossed for 6 generations into the w1118 (control) genetic background prior to conducting experiments. w1118 was used as control for all experiments. The Piezo RNAi line used was P{KK101815}VIE-260B. For analysing Piezo expression and localisation the following lines were used: P{Piezo-Gal4.1.0}IIA, P{UAS-Piezo.GFP}IIA-2, P{UAS-Piezo.GFP}IIIA (Kim et al., 2012) and Mi{PT-GFSTF.0}PiezoMI04189-GFSTF.0 (Nagarkar-Jaiswal et al., 2015). To monitor Ca2+: P{20XUAS-IVS-GCaMP6f}attP40 (Chen et al., 2013). Inhibition of Piezo was achieved by crossing Piezo-Gal4 (above) or Tin-Gal4, P{tinC-Gal4. Δ4} to P{UAS-GsMTx4-FL, P{UAS-GsMTx4-AP or P{UAS-GsMTx4-SR (Beqja et al., 2020). Hypertensive flies were generated by inducing renal failure by crossing CtB-Gal4 (Sudarsan et al., 2002) to UAS-λtop4.4 (gift from Trudi Schüpbach). Experiments on hypertensive flies were carried out at 10 days to allow the haemolymph pressure to build in the body cavity (Figure 5F).
4.2 Immunohistochemistry and in situ hybridisation
Immunohistochemistry on heart preparations were performed using established protocols (Alayari et al., 2009). Hearts were incubated in “relaxing buffer” (Hanks Balanced Salt Solution +10 mM EGTA) prior to fixation so that hearts were in the same point (diastole) in the contraction cycle. Primary antibodies used: anti-GFP (goat, 1:500, ab6673, from Abcam), anti-SERCA (rabbit, 1:1000 (Sanyal et al., 2005), anti-Dlg (mouse, 1:40, DSHB). Appropriate biotinylated (Vector Laboratories) or fluorescent (AlexaFluor®488, 568, 633 or Cy3, Jackson ImmunoResearch) secondary antibodies were used at 1:200. Where necessary, the ABC biotin amplification kit was used (Vector Laboratories). Actin was stained using AlexaFluor®488 or 568 phalloidin (1:100, Molecular probes), DNA was stained using DAPI (1:1000, Molecular probes), SR membranes using ER-trackerTM Red (BODIPY™ TR Glibenclamide, Invitrogen). In some preparations fluorescently labelled Wheat Germ Agglutinin (AlexaFluor®633-WGA, 5ug/ml, Invitrogen) was used as a counter-stain; in such cases WGA was incubated with live tissue for 20 min at room temperature, followed by fixation in 4% formaldehyde. Heart preparations were mounted in Vectasheild (Vector Laboratories) and imaged on a Nikon A1R FLIM confocal microscope. Maximum intensity projection images were generated using Fiji. In situ hybridisation was carried out using the hybridisation chain reaction v3.0 (HCR v3.0) technique using established protocols (Choi et al., 2018) with a probe set size of 20 (Molecular Instruments).
4.3 Analysis of heart function
Acquisition and analysis of heart function were based on established protocols from a preparation of semi-intact 3-day old adult flies (Fink et al., 2009; Vogler and Ocorr, 2009; Ocorr et al., 2014). Heart preparations were conducted in Hank’s Balanced Salt Solution (Sigma, H6648) with the addition of 4 mM MgCl2 and 2 mM CaCl2 (referred to as HBSS below). Briefly, high frame rate (200 fps) movies recording movement of the heart wall were captured on a Zeiss Axioplan microscope with x40 water dipping objective connected to a Prime 95B sCMOS camera (Photometrics) running from Micro-Manager 1.4 software. M-mode kymographs were generated by excising a single pixel horizontal slice from each frame (encompassing the heart walls at the level of the 2nd abdominal segment or the conical chamber) and aligned horizontally using the “make montage” function in Fiji to provide an edge trace displaying heart wall movement in the y-axis and time along the x-axis.
4.4 Calcium visualisation
Tin-Gal4 was used to drive the genetically encoded Ca2+ sensor UAS-GCaMP6F ['F' = “fast variant” with the fastest decay kinetics of the GCaMP6 species (Chen et al., 2013)]. The GCaMP imaging rig included a Zeiss Axio Examiner microscope with a x40 dipping objective, fitted with GFP filters and LED light source (Carl Zeiss Ltd.) and connected to a Prime 95B sCMOS camera (Photometrics) running from Micro-Manager 1.4 software. Ca2+ transients were recorded from a single valve cardiomyocyte (as these produced the most reliable and robust Ca2+ signal) in intact hearts. Imaging was carried out at 200 fps, for ∼15 s. All experiments were carried out with 10-day old flies (as the Ca2+ signal was more robust at 10 compared to 3 days) at room temperature (∼22°C). Ca2+ transients were quantified using Fiji. Briefly, mean fluorescence in a 100 × 100 pixel region of interest (ROI) in a region outside the heart was used to establish background fluorescence. Ca2+ transients were measured in a 200 × 200 pixel ROI centered on the valve cardiomyocyte using the Plot Z-axis tool in Fiji.
4.5 Induction of mechanical stress
4.5.1 Hypotonic stress
Hypotonic solution was made by diluting HBSS 50:50 in dH2O, whilst maintaining concentration of CaCl2 (2 mM) and MgCl2 (4 mM). Isotonic solution (i.e. HBSS) = 311 mOsm, hypotonic solution = 172 mOsm. For experiments in Figure 2 isotonic solution was replaced with hypotonic solution 5 min prior to analysis, then replaced with isotonic to assess recovery. For experiments in Figure 4 isotonic solution was replaced with hypotonic solution during recording to capture the first Ca2+ transient after hypotonic stretch.
4.5.2 Modulation of ambient pressure
Two devices were constructed consisting of a specimen chamber (25 cm2 cell culture flask) in which the heart preparation was placed/viewed, connected to either 1) a syringe and one-way valve, to decrease air pressure in the chamber or 2) a bulb pump to increase air pressure in the chamber. Both devices were fitted with a pressure gauge to monitor pressure levels (Supplementary Figure S2). The chamber was viewed on a Leica DMRE Upright microscope with x10 objective, connected to a QImaging Retiga 2000R camera running from QCapture Pro0.6 software. Heart preparations were first imaged at ambient pressure (30 fps for 60 s), subjected to high/low pressure for 10 min prior to imaging again (30 fps for 60 s) at the high/low pressure.
4.6 GsMTx4 inhibition of piezo
For pharmacological inhibition of Piezo 5µM GsMTx4 (Alomone Labs) was added to HBSS. The genetically encoded GsMTx4 strains are described above; these were crossed to either Tin-Gal4 or Piezo-Gal4 and experiments were carried out on 3-day old adults.
4.7 Analysis of SR Ca2+ content
Ca2+ transients were imaged as described above. Imaging was carried out continuously whilst changing solutions. Heart preparations were imaged for ∼10 s in HBSS to record normal systolic Ca2+ transients, the solution was then replaced rapidly with HBSS +20 mM caffeine to record caffeine-induced Ca2+ transients. Caffeine induces Ca2+ release from the SR, resulting in a caffeine-induced Ca2+ transient larger than the normal systolic Ca2+ transient, which decayed back to resting levels within 10–20 s (Diaz et al., 2004). The amplitude of this caffeine-induced Ca2+ transient was estimated by subtracting the peak from the resting level. The obtained value was taken as relative estimate of the SR Ca2+ content between genotypes.
4.8 Image/data analysis
Image analysis was conducted in Fiji and data analysis in Prism. Details of the statistical tests used are provided in figure legends.
Data availability statement
The original contributions presented in the study are included in the article/Supplementary Material, further inquiries can be directed to the corresponding author.
Author contributions
BD designed and conceptualised the study. LZ, JC-B, JW, RB, OM, PH, MD, and BD performed experiments and analyzed the data. LZ and BD developed methodology. MD, PH, and BD supervised the study. BD, PH, and MD produced the figures and wrote the initial draft. All authors reviewed and edited the manuscript. BD acquired funding for, and administered the project.
Funding
This research was funded by a Wellcome Trust Seed Award (09910/Z/15/Z), a Royal Society Research Grant (RG170159), and an Institutional Strategic Support Fund Award (IS3-R51) to BD.
Acknowledgments
We thank members of the Denholm lab for comments on a draft of the manuscript. For the purpose of open access, the author has applied a CC BY public copyright licence to any Author Accepted Manuscript version arising from this submission.
Conflict of interest
The authors declare that the research was conducted in the absence of any commercial or financial relationships that could be construed as a potential conflict of interest.
Publisher’s note
All claims expressed in this article are solely those of the authors and do not necessarily represent those of their affiliated organizations, or those of the publisher, the editors and the reviewers. Any product that may be evaluated in this article, or claim that may be made by its manufacturer, is not guaranteed or endorsed by the publisher.
Supplementary material
The Supplementary Material for this article can be found online at: https://www.frontiersin.org/articles/10.3389/fphys.2022.1003999/full#supplementary-material
References
Adler C. P., Friedburg H., Herget G. W., Neuburger M., ScHwalb H. (1996). Variability of cardiomyocyte DNA content, ploidy level and nuclear number in mammalian hearts. Virchows Arch. 429 (2-3), 159–164. doi:10.1007/BF00192438
Adler C. P., Friedburg H. (1986). Myocardial DNA content, ploidy level and cell number in geriatric hearts: Post-mortem examinations of human myocardium in old age. J. Mol. Cell. Cardiol. 18 (1), 39–53. doi:10.1016/s0022-2828(86)80981-6
Ahmad S. M. (2017). Conserved signaling mechanisms in Drosophila heart development. Dev. Dyn. 246 (9), 641–656. doi:10.1002/dvdy.24530
Alayari N. N., Vogler G., Taghli-Lamallem O., Ocorr K., Bodmer R., Cammarato A. (2009). Fluorescent labeling of Drosophila heart structures. J. Vis. Exp. 32, 1423. doi:10.3791/1423
Alcaino C., Knutson K., Gottlieb P. A., Farrugia G., Beyder A. (2017). Mechanosensitive ion channel Piezo2 is inhibited by D-GsMTx4. Channels (Austin) 11 (3), 245–253. doi:10.1080/19336950.2017.1279370
Allen D. G., Blinks J. R. (1978). Calcium transients in aequorin-injected frog cardiac muscle. Nature 273 (5663), 509–513. doi:10.1038/273509a0
Allen D. G., Kurihara S. (1982). The effects of muscle length on intracellular calcium transients in mammalian cardiac muscle. J. Physiol. 327, 79–94. doi:10.1113/jphysiol.1982.sp014221
Allen D. G., Nichols C. G., Smith G. L. (1988). The effects of changes in muscle length during diastole on the calcium transient in ferret ventricular muscle. J. Physiol. 406, 359–370. doi:10.1113/jphysiol.1988.sp017385
Anrep V. G. (1912). On the part played by the suprarenals in the normal vascular reactions of the body. J. Physiol. 45 (5), 307–317. doi:10.1113/jphysiol.1912.sp001553
Bae C., Sachs F., Gottlieb P. A. (2011). The mechanosensitive ion channel Piezo1 is inhibited by the peptide GsMTx4. Biochemistry 50 (29), 6295–6300. doi:10.1021/bi200770q
Beqja D., Haidar S., Nikolaev M., Shen Y., Denholm B. (2020). Transgenic tarantula toxin: A novel tool to study mechanosensitive ion channels in Drosophila. J. Insect Physiol. 127, 104116. doi:10.1016/j.jinsphys.2020.104116
Brette F., Calaghan S. C., Lappin S., WhitE E., Colyer J., Le Guennec J. Y. (2000). Biphasic effects of hyposmotic challenge on excitation-contraction coupling in rat ventricular myocytes. Am. J. Physiol. Heart Circ. Physiol. 279 (4), H1963–H1971. doi:10.1152/ajpheart.2000.279.4.H1963
Calaghan S., White E. (2004). Activation of Na+-H+ exchange and stretch-activated channels underlies the slow inotropic response to stretch in myocytes and muscle from the rat heart. J. Physiol. 559 (1), 205–214. doi:10.1113/jphysiol.2004.069021
Cammarato A., Ocorr S., Ocorr K. (2015). Enhanced assessment of contractile dynamics in Drosophila hearts. Biotechniques 58 (2), 77–80. doi:10.2144/000114255
Chen T. W., Wardill T. J., Sun Y., Pulver S. R., Renninger S. L., Baohan A., et al. (2013). Ultrasensitive fluorescent proteins for imaging neuronal activity. Nature 499 (7458), 295–300. doi:10.1038/nature12354
Chen-Izu Y., Izu L. T. (2017). Mechano-chemo-transduction in cardiac myocytes. J. Physiol. 595 (12), 3949–3958. doi:10.1113/JP273101
Choi H. M. T., Schwarzkopf M., Fornace M. E., Acharya A., Artavanis G., Stegmaier J., et al. (2018). Third-generation in situ hybridization chain reaction: Multiplexed, quantitative, sensitive, versatile, robust. Development 145 (12), dev165753. doi:10.1242/dev.165753
Coste B., Mathur J., Schmidt M., Earley T. J., Ranade S., Petrus M. J., et al. (2010). Piezo1 and Piezo2 are essential components of distinct mechanically activated cation channels. Science 330 (6000), 55–60. doi:10.1126/science.1193270
Coste B., Xiao B., Santos J. S., Syeda R., Grandl J., Spencer K. S., et al. (2012). Piezo proteins are pore-forming subunits of mechanically activated channels. Nature 483 (7388), 176–181. doi:10.1038/nature10812
de Castro C., Titlow J., Majeed Z. R., Malloy C., King K. E., Cooper R. L. (2019). Mechanical and chemical factors required for maintaining cardiac rhythm in Drosophila melanogaster larva. J. Entomol. 16, 62–73. doi:10.3923/je.2019.62.73
Diaz M. E., O'Neill S. C., Eisner D. A. (2004). Sarcoplasmic reticulum calcium content fluctuation is the key to cardiac alternans. Circ. Res. 94 (5), 650–656. doi:10.1161/01.RES.0000119923.64774.72
Fabiato A. (1983). Calcium-induced release of calcium from the cardiac sarcoplasmic reticulum. Am. J. Physiol. 245 (1), C1–C14. doi:10.1152/ajpcell.1983.245.1.C1
Fanchaouy M., Polakova E., Jung C., Ogrodnik J., ShirokovaN. , Niggli E. (2009). Pathways of abnormal stress-induced Ca2+ influx into dystrophic mdx cardiomyocytes. Cell Calcium 46 (2), 114–121. doi:10.1016/j.ceca.2009.06.002
Fink M., Callol-Massot C., Chu A., Ruiz-Lozano P., Izpisua Belmonte J. C., Giles W., et al. (2009). A new method for detection and quantification of heartbeat parameters in Drosophila, zebrafish, and embryonic mouse hearts. Biotechniques 46 (2), 101–113. doi:10.2144/000113078
Gannier F., WhitE E., LAcAmpAgne A., Garnier D., Le Guennec J. Y. (1994). Streptomycin reverses a large stretch induced increases in [Ca2+]i in isolated Guinea pig ventricular myocytes. Cardiovasc. Res. 28 (8), 1193–1198. doi:10.1093/cvr/28.8.1193
Gomis A., Soriano S., Belmonte C., Viana F. (2008). Hypoosmotic- and pressure-induced membrane stretch activate TRPC5 channels. J. Physiol. 586 (23), 5633–5649. doi:10.1113/jphysiol.2008.161257
Gu G. G., Singh S. (1995). Pharmacological analysis of heartbeat in Drosophila. J. Neurobiol. 28 (3), 269–280. doi:10.1002/neu.480280302
Gudipaty S. A., Lindblom J., Loftus P. D., Redd M. J., Edes K., Davey C. F., et al. (2017). Mechanical stretch triggers rapid epithelial cell division through Piezo1. Nature 543 (7643), 118–121. doi:10.1038/nature21407
Hu Q., Ahmad A. A., Seidel T., Hunter C., Streiff M., Nikolova L., et al. (2020). Location and function of transient receptor potential canonical channel 1 in ventricular myocytes. J. Mol. Cell. Cardiol. 139, 113–123. doi:10.1016/j.yjmcc.2020.01.008
Hu Y., Wang Z., Liu T., Zhang W. (2019). Piezo-like gene regulates locomotion in Drosophila larvae. Cell Rep. 26 (6), 1369–1377. doi:10.1016/j.celrep.2019.01.055
Iribe G., Ward C. W., Camelliti P., Bollensdorff C., Mason F., Burton R. A. B., et al. (2009). Axial stretch of rat single ventricular cardiomyocytes causes an acute and transient increase in Ca2+ spark rate. Circ. Res. 104 (6), 787–795. doi:10.1161/CIRCRESAHA.108.193334
Jiang F., Yin K., Wu K., Zhang M., Wang S., Cheng H., et al. (2021). The mechanosensitive Piezo1 channel mediates heart mechano-chemo transduction. Nat. Commun. 12 (1), 869. doi:10.1038/s41467-021-21178-4
Jung C., Martins A. S., Niggli E., Shirokova N. (2008). Dystrophic cardiomyopathy: Amplification of cellular damage by Ca2+ signalling and reactive oxygen species-generating pathways. Cardiovasc. Res. 77 (4), 766–773. doi:10.1093/cvr/cvm089
K S., Hata M., Takahara S., Tsuzaki H., Yokota H., Akatsu H., et al. (2006). A novel membrane protein, encoded by the gene covering KIAA0233, is transcriptionally induced in senile plaque-associated astrocytes. Brain Res. 1108 (1), 19–27. doi:10.1016/j.brainres.2006.06.050
Kim S. E., Coste B., Chadha A., Cook B., Patapoutian A. (2012). The role of Drosophila Piezo in mechanical nociception. Nature 483 (7388), 209–212. doi:10.1038/nature10801
Konhilas J. P., Irving T. C., de Tombe P. P. (2002). Frank-Starling law of the heart and the cellular mechanisms of length-dependent activation. Pflugers Arch. 445 (3), 305–310. doi:10.1007/s00424-002-0902-1
Laflamme M. A., Murry C. E. (2011). Heart regeneration. Nature 473 (7347), 326–335. doi:10.1038/nature10147
Le Guennec J. Y., WhitE E., GannierF. , Argibay J. A., Garnier D. (1991). Stretch-induced increase of resting intracellular calcium concentration in single Guinea-pig ventricular myocytes. Exp. Physiol. 76 (6), 975–978. doi:10.1113/expphysiol.1991.sp003560
Lee W., Leddy H. A., Chen Y., Lee S. H., Zelenski N. A., McNulty A. L., et al. (2014). Synergy between Piezo1 and Piezo2 channels confers high-strain mechanosensitivity to articular cartilage. Proc. Natl. Acad. Sci. U. S. A. 111 (47), E5114–E5122. doi:10.1073/pnas.1414298111
Li J., Hou B., Tumova S., Muraki K., Bruns A., Ludlow M. J., et al. (2014). Piezo1 integration of vascular architecture with physiological force. Nature 515 (7526), 279–282. doi:10.1038/nature13701
Liang J., Huang B., Yuan G., Chen Y., Liang F., Zeng H., et al. (2017). Stretch-activated channel Piezo1 is up-regulated in failure heart and cardiomyocyte stimulated by AngII. Am. J. Transl. Res. 9 (6), 2945–2955.
Limpitikul W. B., Viswanathan M. C., O'Rourke B., Yue D. T., Cammarato A. (2018). Conservation of cardiac L-type Ca(2+) channels and their regulation in Drosophila: A novel genetically-pliable channelopathic model. J. Mol. Cell. Cardiol. 119, 64–74. doi:10.1016/j.yjmcc.2018.04.010
Lin N., Badie N., Yu L., Abraham D., Cheng H., Bursac N., et al. (2011). A method to measure myocardial calcium handling in adult Drosophila. Circ. Res. 108 (11), 1306–1315. doi:10.1161/CIRCRESAHA.110.238105
Madan A., Viswanathan M. C., Woulfe K. C., Schmidt W., Sidor A., Liu T., et al. (2020). TNNT2 mutations in the tropomyosin binding region of TNT1 disrupt its role in contractile inhibition and stimulate cardiac dysfunction. Proc. Natl. Acad. Sci. U. S. A. 117 (31), 18822–18831. doi:10.1073/pnas.2001692117
Maksimovic S., Nakatani M., Baba Y., Nelson A. M., Marshall K. L., Wellnitz S. A., et al. (2014). Epidermal Merkel cells are mechanosensory cells that tune mammalian touch receptors. Nature 509 (7502), 617–621. doi:10.1038/nature13250
Marshall K. L., Saade D., Ghitani N., Coombs A. M., Szczot M., Keller J., et al. (2020). PIEZO2 in sensory neurons and urothelial cells coordinates urination. Nature 588 (7837), 290–295. doi:10.1038/s41586-020-2830-7
McHugh B. J., Buttery R., Lad Y., Banks S., Haslett C., Sethi T. (2010). Integrin activation by Fam38A uses a novel mechanism of R-Ras targeting to the endoplasmic reticulum. J. Cell Sci. 123 (1), 51–61. doi:10.1242/jcs.056424
Nagarkar-Jaiswal S., DeLuca S. Z., Lee P. T., Lin W. W., Pan H., Zuo Z., et al. (2015). A genetic toolkit for tagging intronic MiMIC containing genes. Elife 4, e08469. doi:10.7554/eLife.08469
Nonomura K., Woo S. H., Chang R. B., Gillich A., Qiu Z., Francisco A. G., et al. (2017). Piezo2 senses airway stretch and mediates lung inflation-induced apnoea. Nature 541, 176–181. doi:10.1038/nature20793
Ocorr K., Reeves N. L., Wessells R. J., Fink M., Chen H. S. V., Akasaka T., et al. (2007). KCNQ potassium channel mutations cause cardiac arrhythmias in Drosophila that mimic the effects of aging. Proc. Natl. Acad. Sci. U. S. A. 104 (10), 3943–3948. doi:10.1073/pnas.0609278104
Ocorr K., Vogler G., Bodmer R. (2014). Methods to assess Drosophila heart development, function and aging. Methods 68 (1), 265–272. doi:10.1016/j.ymeth.2014.03.031
Parmley W. W., Chuck L. (1973). Length-dependent changes in myocardial contractile state. Am. J. Physiol. 224 (5), 1195–1199. doi:10.1152/ajplegacy.1973.224.5.1195
Patterson S. W., Starling E. H. (1914). On the mechanical factors which determine the output of the ventricles. J. Physiol. 48 (5), 357–379. doi:10.1113/jphysiol.1914.sp001669
Pineda S., Nikolova-Krstevski V., Leimena C., Atkinson A. J., Altekoester A. K., Cox C. D., et al. (2021). Conserved role of the large conductance calcium-activated potassium channel, KCa1.1, in sinus node function and arrhythmia risk. Circ. Genom. Precis. Med. 14 (2), e003144. doi:10.1161/CIRCGEN.120.003144
Pope S. E., Stinson E. B., Daughters G. T., Schroeder J. S., Ingels N. B., Alderman E. L. (1980). Exercise response of the denervated heart in long-term cardiac transplant recipients. Am. J. Cardiol. 46 (2), 213–218. doi:10.1016/0002-9149(80)90060-0
Ranade S. S., Qiu Z., Woo S. H., Hur S. S., Murthy S. E., Cahalan S. M., et al. (2014). Piezo1, a mechanically activated ion channel, is required for vascular development in mice. Proc. Natl. Acad. Sci. U. S. A. 111 (28), 10347–10352. doi:10.1073/pnas.1409233111
Reed A., Kohl P., Peyronnet R. (2014). Molecular candidates for cardiac stretch-activated ion channels. Glob. Cardiol. Sci. Pract. 2014 (2), 9–25. doi:10.5339/gcsp.2014.19
Retailleau K., Duprat F., Arhatte M., Ranade S. S., Peyronnet R., Martins J. R., et al. (2015). Piezo1 in smooth muscle cells is involved in hypertension-dependent arterial remodeling. Cell Rep. 13 (6), 1161–1171. doi:10.1016/j.celrep.2015.09.072
Rode B., Shi J., Endesh N., Drinkhill M. J., Webster P. J., Lotteau S. J., et al. (2017). Piezo1 channels sense whole body physical activity to reset cardiovascular homeostasis and enhance performance. Nat. Commun. 8 (1), 350. doi:10.1038/s41467-017-00429-3
Rog-Zielinska E. A., O'Toole E. T., Hoenger A., Kohl P. (2019). Mitochondrial deformation during the cardiac mechanical cycle. Anat. Rec. 302 (1), 146–152. doi:10.1002/ar.23917
Rotstein B., Paululat A. (2016). On the morphology of the Drosophila heart. J. Cardiovasc. Dev. Dis. 3 (2), E15. doi:10.3390/jcdd3020015
Ruknudin A., Sachs F., Bustamante J. O. (1993). Stretch-activated ion channels in tissue-cultured chick heart. Am. J. Physiol. 264 (3), H960–H972. doi:10.1152/ajpheart.1993.264.3.H960
Sadoshima J., Qiu Z., Morgan J. P., Izumo S. (1996). Tyrosine kinase activation is an immediate and essential step in hypotonic cell swelling-induced ERK activation and c-fos gene expression in cardiac myocytes. EMBO J. 15 (20), 5535–5546. doi:10.1002/j.1460-2075.1996.tb00938.x
Sandritter W., Scomazzoni G. (1964). Deoxyribonucleic acid content (feulgen photometry) and dry weight (interference microscopy) of normal and hypertrophic heart muscle fibers. Nature 202, 100–101. doi:10.1038/202100a0
Sanyal S., Consoulas C., Kuromi H., BAsole A., Mukai L., Kidokoro Y., et al. (2005). Analysis of conditional paralytic mutants in Drosophila sarco-endoplasmic reticulum calcium ATPase reveals novel mechanisms for regulating membrane excitability. Genetics 169 (2), 737–750. doi:10.1534/genetics.104.031930
Saxena A., Denholm B., Bunt S., Bischoff M., VijayRaghavan K., Skaer H. (2014). Epidermal growth factor signalling controls myosin II planar polarity to orchestrate convergent extension movements during Drosophila tubulogenesis. PLoS Biol. 12 (12), e1002013. doi:10.1371/journal.pbio.1002013
Senatore S., Rami Reddy V., Semeriva M., Perrin L., Lalevee N. (2010). Response to mechanical stress is mediated by the TRPA channel painless in the Drosophila heart. PLoS Genet. 6 (9), e1001088. doi:10.1371/journal.pgen.1001088
Seo K., Rainer P. P., Lee D. I., Hao S., Bedja D., Birnbaumer L., et al. (2014). Hyperactive adverse mechanical stress responses in dystrophic heart are coupled to transient receptor potential canonical 6 and blocked by cGMP-protein kinase G modulation. Circ. Res. 114 (5), 823–832. doi:10.1161/CIRCRESAHA.114.302614
Sequeira V., van der Velden J. (2015). Historical perspective on heart function: The frank-starling law. Biophys. Rev. 7 (4), 421–447. doi:10.1007/s12551-015-0184-4
Shiels H. A., White E. (2008). The Frank-Starling mechanism in vertebrate cardiac myocytes. J. Exp. Biol. 211 (13), 2005–2013. doi:10.1242/jeb.003145
Sigurdson W., Ruknudin A., Sachs F. (1992). Calcium imaging of mechanically induced fluxes in tissue-cultured chick heart: Role of stretch-activated ion channels. Am. J. Physiol. 262 (4), H1110–H1115. doi:10.1152/ajpheart.1992.262.4.H1110
Spassova M. A., Hewavitharana T., Xu W., Soboloff J., Gill D. L. (2006). A common mechanism underlies stretch activation and receptor activation of TRPC6 channels. Proc. Natl. Acad. Sci. U. S. A. 103 (44), 16586–16591. doi:10.1073/pnas.0606894103
Starling E. (1918). The law of the heart (Linacre Lecture, given at Cambridge, 1915). London: Longmans Green & Co.
Sudarsan V., Pasalodos-Sanchez S., Wan S., Gampel A., Skaer H. (2002). A genetic hierarchy establishes mitogenic signalling and mitotic competence in the renal tubules of Drosophila. Development 129 (4), 935–944. doi:10.1242/dev.129.4.935
Sullivan K. M. C., Scott K., Zuker C. S., Rubin G. M. (2000). The ryanodine receptor is essential for larval development in Drosophila melanogaster. Proc. Natl. Acad. Sci. U. S. A. 97 (11), 5942–5947. doi:10.1073/pnas.110145997
Tavi P., Han C., Weckstrom M. (1998). Mechanisms of stretch-induced changes in [Ca2+]i in rat atrial myocytes: Role of increased troponin C affinity and stretch-activated ion channels. Circ. Res. 83 (11), 1165–1177. doi:10.1161/01.res.83.11.1165
Tavi P., Laine M., Weckstrom M. (1996). Effect of gadolinium on stretch-induced changes in contraction and intracellularly recorded action- and afterpotentials of rat isolated atrium. Br. J. Pharmacol. 118 (2), 407–413. doi:10.1111/j.1476-5381.1996.tb15417.x
Tucci P. J., Bregagnollo E. A., Spadaro J., Cicogna A. C., Ribeiro M. C. (1984). Length dependence of activation studied in the isovolumic blood-perfused dog heart. Circ. Res. 55 (1), 59–66. doi:10.1161/01.res.55.1.59
Vogler G., Ocorr K. (2009). Visualizing the beating heart in Drosophila. J. Vis. Exp. (31), 1425. doi:10.3791/1425
Ward M.-L., Williams I. A., Chu Y., Cooper P. J., Ju Y. K., Allen D. G. (2008). Stretch-activated channels in the heart: Contributions to length-dependence and to cardiomyopathy. Prog. Biophys. Mol. Biol. 97 (2), 232–249. doi:10.1016/j.pbiomolbio.2008.02.009
White E., Boyett M. R., Orchard C. H. (1995). The effects of mechanical loading and changes of length on single Guinea-pig ventricular myocytes. J. Physiol. 482 (1), 93–107. doi:10.1113/jphysiol.1995.sp020502
Wolf M. J., Amrein H., Izatt J. A., Choma M. A., Reedy M. C., Rockman H. A. (2006). Drosophila as a model for the identification of genes causing adult human heart disease. Proc. Natl. Acad. Sci. U. S. A. 103 (5), 1394–1399. doi:10.1073/pnas.0507359103
Wolf M. J., Rockman H. A. (2011). Drosophila, genetic screens, and cardiac function. Circ. Res. 109 (7), 794–806. doi:10.1161/CIRCRESAHA.111.244897
Woo S. H., Ranade S., Weyer A. D., Dubin A. E., Baba Y., Qiu Z., et al. (2014). Piezo2 is required for Merkel-cell mechanotransduction. Nature 509 (7502), 622–626. doi:10.1038/nature13251
Yamaguchi Y., Iribe G., Kaneko T., Takahashi K., Numaga-Tomita T., Nishida M., et al. (2018). TRPC3 participates in angiotensin II type 1 receptor-dependent stress-induced slow increase in intracellular Ca(2+) concentration in mouse cardiomyocytes. J. Physiol. Sci. 68 (2), 153–164. doi:10.1007/s12576-016-0519-3
Yamaguchi Y., Iribe G., Nishida M., Naruse K. (2017). Role of TRPC3 and TRPC6 channels in the myocardial response to stretch: Linking physiology and pathophysiology. Prog. Biophys. Mol. Biol. 130, 264–272. doi:10.1016/j.pbiomolbio.2017.06.010
Yaniv Y., Juhaszova M., Wang S., Fishbein K. W., Zorov D. B., Sollott S. J. (2011). Analysis of mitochondrial 3D-deformation in cardiomyocytes during active contraction reveals passive structural anisotropy of orthogonal short axes. PLoS One 6 (7), e21985. doi:10.1371/journal.pone.0021985
Yu L., Daniels J., Glaser A. E., Wolf M. J. (2013). Raf-mediated cardiac hypertrophy in adult Drosophila. Dis. Model. Mech. 6 (4), 964–976. doi:10.1242/dmm.011361
Yu L., Daniels J. P., Wu H., Wolf M. J. (2015). Cardiac hypertrophy induced by active Raf depends on Yorkie-mediated transcription. Sci. Signal. 8 (362), ra13. doi:10.1126/scisignal.2005719
Keywords: Piezo, mechanotransduction, Frank-Starling, Drosophila, heart, calcium, mechanosensitive ion channel, sacroplasmic recticulum
Citation: Zechini L, Camilleri-Brennan J, Walsh J, Beaven R, Moran O, Hartley PS, Diaz M and Denholm B (2022) Piezo buffers mechanical stress via modulation of intracellular Ca2+ handling in the Drosophila heart. Front. Physiol. 13:1003999. doi: 10.3389/fphys.2022.1003999
Received: 26 July 2022; Accepted: 26 August 2022;
Published: 14 September 2022.
Edited by:
Fabien Brette, Institut National de la Santé et de la Recherche Médicale (INSERM), FranceReviewed by:
Jean-Yves Le Guennec, Université de Montpellier, FranceRobin Lewis Cooper, University of Kentucky, United States
Copyright © 2022 Zechini, Camilleri-Brennan, Walsh, Beaven, Moran, Hartley, Diaz and Denholm. This is an open-access article distributed under the terms of the Creative Commons Attribution License (CC BY). The use, distribution or reproduction in other forums is permitted, provided the original author(s) and the copyright owner(s) are credited and that the original publication in this journal is cited, in accordance with accepted academic practice. No use, distribution or reproduction is permitted which does not comply with these terms.
*Correspondence: Barry Denholm, QmFycnkuRGVuaG9sbUBlZC5hYy51aw==