- Intramural Research Program, National Institute on Aging, NIH, Baltimore, MD, United States
The current dogma about the heartbeat origin is based on “the pacemaker cell,” a specialized cell residing in the sinoatrial node (SAN) that exhibits spontaneous diastolic depolarization triggering rhythmic action potentials (APs). Recent high-resolution imaging, however, demonstrated that Ca signals and APs in the SAN are heterogeneous, with many cells generating APs of different rates and rhythms or even remaining non-firing (dormant cells), i.e., generating only subthreshold signals. Here we numerically tested a hypothesis that a community of dormant cells can generate normal automaticity, i.e., “the pacemaker cell” is not required to initiate rhythmic cardiac impulses. Our model includes 1) non-excitable cells generating oscillatory local Ca releases and 2) an excitable cell lacking automaticity. While each cell in isolation was not “the pacemaker cell”, the cell system generated rhythmic APs: The subthreshold signals of non-excitable cells were transformed into respective membrane potential oscillations via electrogenic Na/Ca exchange and further transferred and integrated (computed) by the excitable cells to reach its AP threshold, generating rhythmic pacemaking. Cardiac impulse is an emergent property of the SAN cellular network and can be initiated by cells lacking intrinsic automaticity. Cell heterogeneity, weak coupling, subthreshold signals, and their summation are critical properties of the new pacemaker mechanism, i.e., cardiac pacemaker can operate via a signaling process basically similar to that of “temporal summation” happening in a neuron with input from multiple presynaptic cells. The new mechanism, however, does not refute the classical pacemaker cell-based mechanism: both mechanisms can co-exist and interact within SAN tissue.
Introduction
During a normal life span the human heart executes more than two billion rhythmic contraction cycles, pumping blood to all organs commensurate with body demand at any given condition. Our understanding of the heartbeat origin has been evolving with the advances in technology, experimental techniques, ideas, and computation power. A major milestone in 1907 was the discovery of the sinoatrial node (SAN), a small piece of specialized heart tissue that paces heart contractions (Keith and Flack, 1907). In 1937, a slow potential change (i.e., the diastolic depolarization in current terminology) preceding the discharge of cardiac impulses was discovered by Arvanitaki working on the snail heart (Arvanitaki et al., 1937). Based on Hodgkin-Huxley formalism, Noble (Noble, 1962) explained the origin of the diastolic depolarization of “the pacemaker cell” as an interplay of kinetics of membrane ion currents. Further studies proposed a critical contribution of intracellular Ca cycling in addition to the membrane ion channels, giving rise a modern theory of the coupled-clock system or ignition process via positive feedback mechanisms among local Ca releases (LCRs), Na/Ca exchanger (NCX) and L-type Ca channels (Lyashkov et al., 2018).
At the SAN tissue level, it was initially thought that the pacemakecoupled-clock system or ignition process via positive feedback mechanisms among local Ca releases (LCRs), Na/Ca exchanger (NCX) and L-type Ca channelsr cell or a leading pacemaker center dictates the excitation rate and rhythm of other SAN cells (Sano et al., 1978; Bleeker et al., 1980). Subsequent studies suggested, however, that individual SAN cells mutually entrain each other to fire action potentials (APs) with a common period but different phases (dubbed “democratic” process) resulting in “apparent” propagation (Jalife, 1984; Michaels et al., 1987). Electrical mapping and imaging of SAN tissue demonstrated concentric excitation propagation from a leading center to SAN periphery (Efimov et al., 2004; Lang et al., 2011; Li et al., 2017). Thus, regardless of the propagation is real or apparent, the present dogma is that the pacemaker cell (possessing intrinsic automaticity) or a group of such cells with the fastest spontaneous diastolic depolarization rate within the SAN tissue paces or entrain other SAN cells and the entire heart.
While the spontaneously beating pacemaker cell(s) is thought to initiate cardiac impulse, only 10%–30% of isolated cells actually contract spontaneously (Nakayama et al., 1984). The remaining non-firing cells could not be ascribed as the true pacemaker cells and were not studied. Also, there has been always an uncertainty whether these cells were damaged by the enzymatic isolation procedure. Recent studies, however, demonstrated that this major population of non-firing cells (dubbed dormant cells) is not “dead,” but generates subthreshold oscillatory signals and many dormant cells can be reversibly “awakened” to generate normal automaticity in the presence of β-adrenergic receptor stimulation (Kim et al., 2018; Tsutsui et al., 2018; Tsutsui et al., 2021; Louradour et al., 2022). Dormant cells isolated from superior or inferior regions of SAN demonstrated different properties of noise of Ca signals and membrane potential (Vm) subthreshold oscillations (Grainger et al., 2021), indicating that the role of these cells differs in different SAN locations.
While earlier low-resolution imaging of SAN tissue showed concentric continuous spread of excitation propagation, recent studies performed at a higher (individual cell) resolution level discovered a major fraction of non-firing cells (Bychkov et al., 2020; Fenske et al., 2020), and the tissue excitation within the SAN center appeared to be discontinuous, consisting of functional cell communities (presumably functional modules) with different firing patterns (Bychkov et al., 2020). Ca signals and APs in the communities are highly heterogeneous, with many cells generating APs of different rates and rhythms or remaining non-firing, generating subthreshold signals (Supplementary Video S1). Thus, a possible functional role of non-firing cells and their subthreshold signals represents an open problem in cardiac biology.
One idea is that synchronized APs at the SAN exits emerge from heterogeneous subcellular subthreshold Ca signals, similar to multiscale complex processes of impulse generation within clusters of neurons in neuronal networks (Bychkov et al., 2020; Bychkov et al., 2022). Possible specific mechanisms of signal processing within the network also include stochastic resonance (Clancy and Santana, 2020; Grainger et al., 2021), a phase transition (Weiss and Qu, 2020; Maltsev et al., 2022a), and electrotonic interactions of firing cells with non-firing cells (Fenske et al., 2020).
Here we tested numerically a hypothesis that a fully functional pacemaker module generating rhythmic APs can be constructed exclusively from loosely connected dormant non-firing cells. Specifically, such module can be constructed with non-excitable cells (Ca oscillators) generating synchronized oscillatory local Ca releases driving rhythmic AP firing of an excitable dormant cell lacking intrinsic automaticity. Thus, cardiac impulse can be seen as an emergent property of the SAN cellular network/meshwork and it can be initiated by a community of dormant cells, i.e., without “the pacemaker cell” in its classical definition.
Materials and methods
We used our previously published three-dimensional SAN cell model operating normally as a coupled-clock system (Stern et al., 2014): 1) a Ca clock featuring individual stochastically operating ryanodine receptors (RyRs) embedded in the cell-distributed Ca store (sarcoplasmic reticulum) equipped with Ca pump and 2) a membrane clock featuring the full set of electrophysiology equations describing Vm and 10 different ion currents (Maltsev et al., 2022b). The clocks are coupled via electrogenic NCX and also via individual L-type Ca channels of two types, Cav1.2 and Cav1.3 differing in their activation voltages by 16 mV, stochastically operating and interacting with RyRs in couplons. We modelled a fully functional pacemaker module as a system of five loosely coupled dormant cells (Figure 1A). Each dormant cell model was a modification of our original model to exclude spontaneous AP firing. Four cells in the pacemaker module were modelled as pure Ca oscillators (CaOsc cells), having relatively high sarcoplasmic reticulum Ca pumping rate Pup and Na/Ca exchange rate, but lacking ICaL to prevent AP generation rendering cells non-excitable and non-firing, but generating subthreshold signals. These four CaOsc cells were connected to one cell that was modelled as a highly excitable dormant cell (AP cell) with large density of Cav1.2 but lacking automaticity in the absence of the low voltage activated Cav1.3. The funny current (If) was excluded from cell models. Our freedom in choosing If and ICaL was based on the experimental data that showed substantial cell-to-cell variations of these currents (Supplementary Figure S1). The idea for such functional module was inspired by our recent experimental observation in intact SAN tissue (Bychkov et al., 2022) that some SAN cells generate only oscillatory LCRs that precede AP-induced Ca transients in adjacent cells (Supplementary Video S2). Thus, in our model the oscillatory subthreshold signals from the four CaOsc cells would be transferred via a low conductance connection to the membrane of the AP cell, with its membrane acting as an integrator (a computer) of the oscillatory signals that depolarize the cell membrane towards the activation ICaL threshold, triggering an AP.
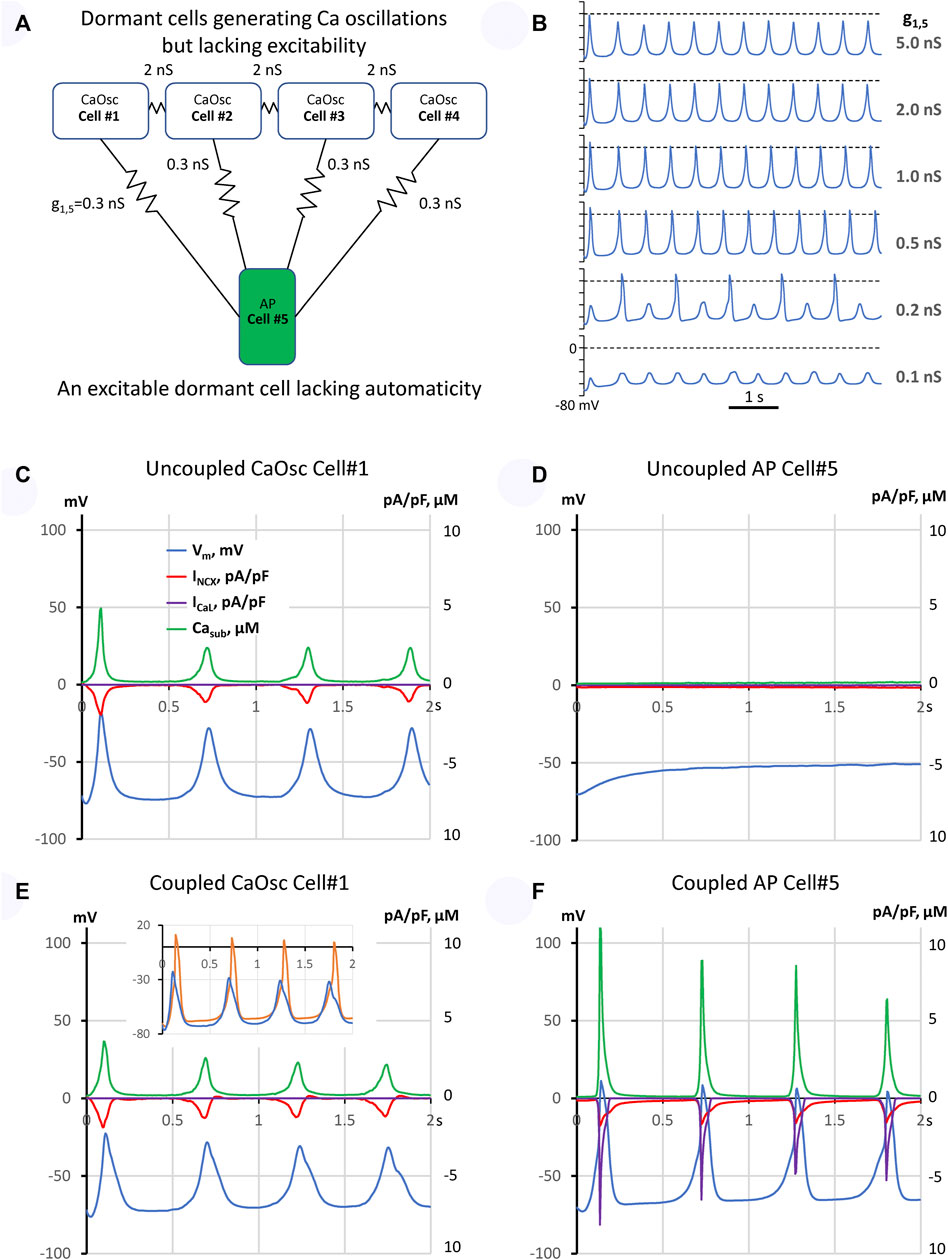
FIGURE 1. A model of emergent pacemaking in a network of five dormant cells (lacking intrinsic automaticity): (A) A diagram of the network: cells #1–4 operating as Ca oscillators (CaOsc) and an excitable Cell #5 (i.e., AP capable cell, labelled as APC) are loosely connected (zigzag lines with conductance values). (B) Vm time course of Cell #5 at various coupling with CaOsc cells (g1,5). (C–F): Time series of INCX, ICaL, Vm, and mean submembrane Ca (Casub) simulated for CaOsc cell #1 and APC cell #5 in separation (C,D) and when cells were coupled (E,F) as shown in panel (A). Inset in (E) shows overlapped Vm in coupled cells #1 (blue) and #5 (orange). Vm rise in cell #1 always preceded each AP upstroke in cell #5. See also related Supplementary Video S3.
Results
First, we simulated each cell model behavior in separation (uncoupled cells). CaOsc Cell#1 generated oscillations of submembrane [Ca], NCX current (INCX), and Vm (Figures 1C,D). Importantly, the Vm oscillations were driven by respective INCX oscillations and had a relatively small amplitude (vs. an AP). At the same time, AP cell #5 alone failed to generate APs, with Vm fluctuating at a −50 mV level. Then we performed simulation for the coupled-cell system (Figures 1E,F). While the CaOsc cells #1–4 continued to generate subthreshold Vm oscillations, the AP cell #5 generated rhythmic APs and AP-induced Ca transients paced by cells #1–4. Importantly, the pacing signals always preceded each AP generation (inset in Figure 1E, Supplementary Video S3). Next, we performed a parameter sensitivity analysis varying the coupling conductance between CaOsc cells and excitable AP cell (Figure 1B). The model generated rhythmic APs in a wide range of coupling conductance from 0.3 to 1 nS. Finally, we performed simulations for a more complex network configuration with more cells (Figure 2A) which demonstrated that CaOsc cells can drive more than just one AP cell (Cells #5 and #6) and the emergent automaticity can spread within further layers of dormant excitable cells (cells #7–9) (Figure 2B).
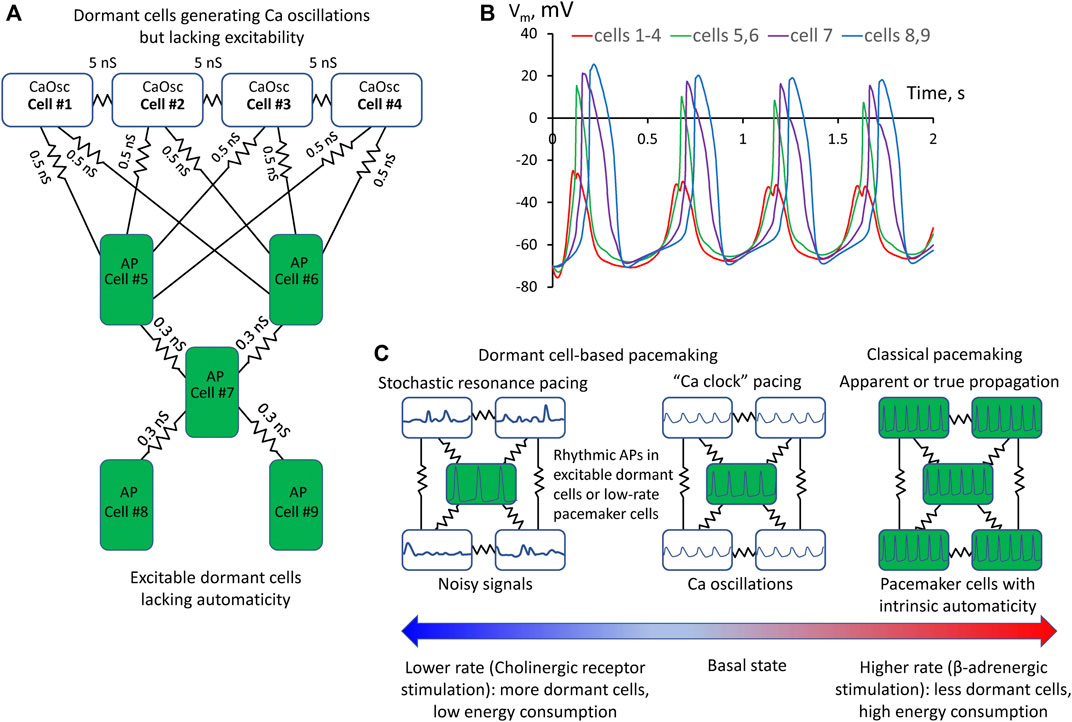
FIGURE 2. A more complex model of pacemaker network of nine dormant cells demonstrating that Ca oscillator cells can drive more than one excitable cell and the emergent automaticity can spread within further layers of dormant excitable cells. (A) A diagram of the network: cells #1–4 operating as Ca oscillators (CaOsc) and excitable Cells #5–9 (i.e., AP capable cells, labelled as APC) are loosely connected (zigzag lines with conductance values). (B) Vm time course in the cells of the network. Vm rise in cell #1 always preceded AP upstrokes in excitable cells. APs in the three layers of excitable cells appear with respective delays reflecting interactions withing excitable cell layers. (C) Prevalence of proposed pacemaking modes with respect to AP firing rates and autonomic modulation state: Stochastic resonance pacing prevails at low rates and cholinergic receptor stimulation, but Ca clock pacing and pacemaker cell pacing prevail in basal state and during β-adrenergic stimulation.
Discussion
Here we demonstrate for the first time that rhythmic cardiac pacemaker impulses can be generated by an ensemble of heterogeneous (specialized) dormant SAN cells, i.e., cells lacking intrinsic automaticity. Such dormant cells have been identified in isolation (Kim et al., 2018; Tsutsui et al., 2018; Grainger et al., 2021; Tsutsui et al., 2021; Louradour et al., 2022) and in intact SAN tissue (Bychkov et al., 2020; Fenske et al., 2020). While function of dormant cells remains unknown, it has been speculated that: 1) dormant cells stay “in reserve” and are “awaken” to function by β-adrenergic stimulation in the fight-or-flight response (Kim et al., 2018; Louradour et al., 2022); 2) non-firing cells can influence the frequency of AP firing cells via electrotonic interactions (Fenske et al., 2020). Experimental studies, however, have shown that dormant cells are not static or neutral: they generate subthreshold signals (Kim et al., 2018; Bychkov et al., 2020; Grainger et al., 2021). One pattern of Ca signaling observed in experimental recordings in intact SAN tissue was that “some SAN cells generate only LCRs that precede AP-induced Ca transients in adjacent cells” (Supplementary Video S2). It was further speculated that such subthreshold signals can somehow spatiotemporally summate and prompt initiation of full-scale signals (i.e., APs) within SAN tissue, e.g., via a stochastic resonance (Clancy and Santana, 2020; Grainger et al., 2021; Guarina et al., 2022), or a phase transition-like process each cycle (Weiss and Qu, 2020; Maltsev et al., 2022a). Thus, following this logic, in addition to the tonic influence or simple “wake-up” function under stress, dormant cells can be in fact functionally active generating important subthreshold signals. Here we suggest a simple model of multi-cellular pacemaker that can indeed operate via synchronization and integration of the subthreshold signals in a community of heterogeneous dormant cells that can reach a threshold each cycle to generate rhythmic APs and the excitation can spread in subsequent layers of dormant cells (Figures 1, 2).
The requirement of heterogeneity for such synergistic pacemaker system in our model is in line with that SAN tissue indeed represents an extremely heterogenous structure (Boyett et al., 2000; Lyashkov et al., 2007). For example, major membrane cell conductances such as of ICaL and If vary by an order of magnitude (Honjo et al., 1996; Monfredi et al., 2018) (Supplementary Figure S1). Dormant cells represent a major cell population (>50%) isolated from SAN (Nakayama et al., 1984; Kim et al., 2018; Grainger et al., 2021; Tsutsui et al., 2021; Louradour et al., 2022). Thus, the SAN tissue seems to be perfectly poised to such operation in the basal state and especially under parasympathetic stimulation, i.e., when the dormant cells become even more abundant in the SAN (Fenske et al., 2020).
Our model is robust: It generates rhythmic APs in a wide range of CaOsc-AP cell coupling conductance from 0.3 to 1 nS (Figures 1B,F). Weaker coupling generates alternations or subthreshold oscillations because the integrated impact of CaOsc cells is insufficient to bring Vm of AP cell to its AP threshold. Stronger coupling does bring Vm to the AP threshold, but AP cell cannot generate a full-scale AP because its electrotonic load of neighboring cells increases (Figure 1B). Thus, another requirement of our model is weak cell coupling that allows cells to function independently, i.e., specialize as Ca clock or AP generator. This requirement is met in the SAN center which lacks high conductance CX43 gap junctions (Boyett et al., 2006; Bychkov et al., 2020).
How would the new pacemaker mechanism work in real SAN tissue? The real SAN tissue exhibits complex network configurations with substantial heterogeneity of the cell coupling strength and parameters of the interacting cells which, in turn, are controlled by autonomic modulation. In addition to pure dormant cell-based pacemaking and classical pacemaker cell-based pacemaking, we can envision the existence of a transitional (mixed) type of network peacemaking, in which high-rate Ca oscillators of dormant cells would pace cells featuring low-rate intrinsic automaticity. On the other hand, in some dormant cells Ca releases can be noisy and dysrhythmic (Kim et al., 2018; Grainger et al., 2021), i.e., not that large and rhythmic as in the model presented here. However, the concept of dormant cell-driving pacemaking proposed here may also work for such cells. An interesting possibility (to be tested in the future) is that the overlapping Vm fluctuations of these “noisy” cells can prompt AP generation in the neighboring cells (dormant cells or low-rate pacemaker cells) when they are ready to fire after a refractory period, or, in other terms, via stochastic resonance (Clancy and Santana, 2020; Grainger et al., 2021), when the noise components resonate with intrinsic frequency of AP generators. The 4–17 mV amplitude of noise reported in dormant SAN cells isolated form mouse (Grainger et al., 2021) was smaller than ∼40 mV in our rabbit CaOsc cell model (Figure 1). Vm oscillation amplitudes can differ in dormant cells of different species and under different physiological and pathological conditions. For example, a wide range of Vm oscillation amplitudes (including those close to 40 mV in our model) was reported in isolated human and rabbit dormant SAN cells in their transition to AP firing state in response to β-adrenergic stimulation (Tsutsui et al., 2018; Tsutsui et al., 2021).
Hypothetical contribution of different pacing modes with respect to heart rate and its autonomic control is shown in Figure 2C. Previous studies showed that various degrees of LCR synchronization are achieved (and controlled) by respective various degrees of CaMKII and PKA-dependent phosphorylation of Ca cycling proteins (Sirenko et al., 2013). At the basal state and especially during β-adrenergic response, higher levels of cAMP and PKA-dependent phosphorylation increase LCR synchronization (Vinogradova et al., 2006) that would favor Ca clock- and classical pacemaker cell- based pacemaking among cells in local neighborhoods in SAN tissue. The role of dormant cell-based pacemaking (and especially its stochastic resonance subtype) is expected to increase at low rates as cholinergic receptor stimulation increases the number of dormant cells (Fenske et al., 2020) and decreases LCR synchronization (Ca clock disintegrates, LCRs become noisy and smaller) due to lower cAMP production and attendant decrease in PKA-dependent phosphorylation of Ca cycling proteins (Lyashkov et al., 2009).
In addition to Ca clock (i.e., LCR synchronization), we also want to emphasize the roles of NCX and ICaL that appear to be important not only for intrinsic automaticity of individual SAN cells (Maltsev and Lakatta, 2009; Lyashkov et al., 2018; Yue et al., 2020), but also in SAN tissue pacemaking: subthreshold Ca signals of synchronized LCRs are translated to respective Vm oscillations via NCX and further summated (i.e., computed) by the membrane of the AP generating cells in the neighborhood to bring their Vm to the threshold of ICaL activation and generate AP upstroke (Figure 1). Therefore, the AP generating cell lacking automaticity in our model must have a substantial contribution of high-threshold activation Cav1.2 in order to be highly excitable but, at the same time, remain dormant. Increasing contribution of low-threshold activation isoform Cav1.3 initiates ignition process that drives automaticity (Torrente et al., 2016; Lyashkov et al., 2018; Maltsev et al., 2022b). Thus, the amplitude of ICaL and its fraction of Cav1.2/Cav1.3 components may also regulate the occurrence and contribution of different modes of pacemaking in cell communities.
What are potential roles and benefits of the new pacemaker mechanism? It can keep the system alive at the edge of criticality in heterogenous SAN tissue (Maltsev et al., 2022a; Campana et al., 2022), e.g., in aging and disease. Indeed, major pacemaker components (ICaL, If, and LCRs) of intrinsic automaticity deteriorate with aging (Jones et al., 2007; Larson et al., 2013; Liu et al., 2014). Thus, in the absence of classical pacemaker cells, the dormant cell-based pacemaker mechanism might serve as the last resort keeping the heart ticking. Further, it can save energy. Indeed, dormant cells, generating subthreshold signals, do not generate high energy-demanding APs. Dormant excitable cells lacking Ca clock do not need to keep high rates of cAMP production and Ca pumping. During fight-or-flight response (critical for survival) the dormant cells “awake” (Kim et al., 2018; Tsutsui et al., 2018; Tsutsui et al., 2021; Louradour et al., 2022) and system operation can shift the gear from its energy-saving mode to classical paradigm of pacemaking driven by classical pacemaker cells at higher rates (Figure 2C).
In more general context, the shift of paradigm from pacemaker-cell based to network-based type of pacing is not unique: a similar paradigm shift has happened recently in studies of respiratory rhythm: the respiratory oscillations arise from complex and dynamic molecular, synaptic and neuronal interactions within a diverse neural microcircuit, and “pacemaker properties are not necessary for respiratory rhythmogenesis” (Feldman and Kam, 2015). However, it is important to emphasize that the new dormant cell-based pacemaker mechanism does not refute or substitute the classical pacemaker cell-based mechanism: both mechanisms can co-exist and synergistically interact in the form of different functional modules within the central SAN area (Bychkov et al., 2020; Norris and Maltsev, 2022) with different prevalence in different conditions (Figure 2C). Rather, our results challenge the exclusive role of the fully automatic pacemaker cells in heart pacemaker function, because a fully functional pacemaker can emerge from subthreshold signal integration (computation) within a dormant cell community.
Elucidation of the role of the new mechanism will need more experimental and theoretical studies. It remains to be determined how different mechanisms of network automaticity would shift and operate in the presence of various densities of ion currents (If, Cav1.2 and Cav1.3, ICaT, IKr, INCX, IKACh, etc.) and Ca clock activities (SR Ca pump and RyRs) in tissue models with larger cell numbers featuring more complex (realistic) cell network structure, coupling strength, and autonomic modulation state, i.e., various levels of cAMP and CaMKII- and PKA-dependent phosphorylation of both Ca- and membrane- clock proteins.
The numerical prototype of dormant cell-based pacemaker presented here is based on our previous single cell model (Stern et al., 2014) that simulates transitions of each RyR and L-type Ca channel molecule and diffusion among numerous voxels in the entire network SR and cytosol (including natural buffers) in three dimensions. While this specific cell model generates detailed local Ca dynamics, including oscillatory synchronized LCRs critical for the emergent pacemaking (Supplementary Video S3), it has a high computational demand. Future studies of SAN pacemaker mechanisms (including dormant-cell based pacemaking) in larger tissue models will likely use simpler cell models in the spirit of multi-scale modeling approach (Qu et al., 2011), such as common pool models featuring coupled-clock function (Maltsev et al., 2022a; Campana et al., 2022) or CRU-based models, generating LCRs (Maltsev et al., 2022b).
Data availability statement
The raw data supporting the conclusion of this article will be made available by the authors, without undue reservation.
Author contributions
MS wrote model computer program code and performed model simulations. VM wrote data visualization computer code, performed data analysis, and wrote the first draft of the manuscript. All authors contributed to conception, design of the study, manuscript revision, read, and approved the submitted version.
Funding
This research was supported by the Intramural Research Program of the National Institutes of Health, National Institute on Aging.
Acknowledgments
This study utilized the high-performance computational capabilities of the Biowulf Linux cluster at the National Institutes of Health, Bethesda, MD, United States (https://hpc.nih.gov/).
Conflict of interest
The authors declare that the research was conducted in the absence of any commercial or financial relationships that could be construed as a potential conflict of interest.
Publisher’s note
All claims expressed in this article are solely those of the authors and do not necessarily represent those of their affiliated organizations, or those of the publisher, the editors and the reviewers. Any product that may be evaluated in this article, or claim that may be made by its manufacturer, is not guaranteed or endorsed by the publisher.
Supplementary material
The Supplementary Material for this article can be found online at: https://www.frontiersin.org/articles/10.3389/fphys.2022.1090162/full#supplementary-material
SUPPLEMENTARY VIDEO S1 | An example of heterogeneous Ca signaling in central mouse SAN that includes rhythmic generation of AP-induced Ca transients in some cells and local subthreshold signals (LCRs) in other cells. Ca recording from (Bychkov et al., 2020).
SUPPLEMENTARY VIDEO S2 | An example of a local Ca signaling module in central mouse SAN in which some SAN cells generate only LCRs that precede AP-induced Ca transients in adjacent cells. Ca recording from (Bychkov et al., 2020). For exact anatomical location within intact SAN and detailed analysis of this video, see Figure 6 in (Bychkov et al., 2020).
SUPPLEMENTARY VIDEO S3 | Simulation results of our new pacemaker model comprised of dormant cells (Figure 1). Upper panel: Oscillatory synchronized LCRs under cell membrane in non-excitable Cell #1 (Ca oscillator). Lower panel: AP-induced Ca transients in excitable Cell #5, lacking intrinsic automaticity but paced by Ca oscillators in cells #1-4. Simulation duration is 2 s (see related time course of Vm, Figures 2C,D). Note: Ca oscillations in cell #1 always precede AP-induced Ca transients (global Ca spike) in cell #5. The panels are the cell cylinder surfaces unwrapping to squares with distributions of RyR clusters under the cell membrane shown by cyan shades reflecting Ca loading of the junctional sarcoplasmic reticulum in which the clusters are embedded: 0 is pure black and >600 μM is pure cyan. Local Ca dynamics is shown by red shades: 0.1 μM is pure black (resting Ca level) and >5 μM is pure red. Simulations were performed using our modified model of SAN cell in 3 dimensions with individual RyRs and L-type Ca channels (Stern et al., 2014).
References
Arvanitaki A., Fersard A., Kruta A., Kruta V. (1937). Reactions electroniques du myocarde en function de sur tonus initial. Compt. Rend. Sot. Biol. 124, 165–167.
Bleeker W. K., Mackaay A. J., Masson-Pevet M., Bouman L. N., Becker A. E. (1980). Functional and morphological organization of the rabbit sinus node. Circ. Res. 46, 11–22. doi:10.1161/01.res.46.1.11
Boyett M. R., Honjo H., Kodama I. (2000). The sinoatrial node, a heterogeneous pacemaker structure. Cardiovasc. Res. 47, 658–687. doi:10.1016/s0008-6363(00)00135-8
Boyett M. R., Inada S., Yoo S., Li J., Liu J., Tellez J., et al. (2006). Connexins in the sinoatrial and atrioventricular nodes. Adv. Cardiol. 42, 175–197. doi:10.1159/000092569
Bychkov R., Juhaszova M., Calvo-Rubio Barrera M., Donald L. a. H., Coletta C., Shumaker C., et al. (2022). The heart's pacemaker mimics brain cytoarchitecture and function: Novel interstitial cells expose complexity of the SAN. JACC. Clin. Electrophysiol. 8, 1191–1215. doi:10.1016/j.jacep.2022.07.003
Bychkov R., Juhaszova M., Tsutsui K., Coletta C., Stern M. D., Maltsev V. A., et al. (2020). Synchronized cardiac impulses emerge from heterogeneous local calcium signals within and among cells of pacemaker tissue. JACC. Clin. Electrophysiol. 6, 907–931. doi:10.1016/j.jacep.2020.06.022
Campana C. A., Ricci E., Bartolucci C., Severi S., Sobie E. A. (2022). Coupling and heterogeneity modulate pacemaking capability in healthy and diseased two-dimensional sinoatrial node tissue models. BioRxiv. (preprint). doi:10.1101/2022.04.14.488275
Clancy C. E., Santana L. F. (2020). Evolving discovery of the origin of the heartbeat: A new perspective on sinus rhythm. JACC. Clin. Electrophysiol. 6, 932–934. doi:10.1016/j.jacep.2020.07.002
Efimov I. R., Nikolski V. P., Salama G. (2004). Optical imaging of the heart. Circ. Res. 95, 21–33. doi:10.1161/01.RES.0000130529.18016.35
Feldman J. L., Kam K. (2015). Facing the challenge of mammalian neural microcircuits: Taking a few breaths may help. J. Physiol. 593, 3–23. doi:10.1113/jphysiol.2014.277632
Fenske S., Hennis K., Rotzer R. D., Brox V. F., Becirovic E., Scharr A., et al. (2020). cAMP-dependent regulation of HCN4 controls the tonic entrainment process in sinoatrial node pacemaker cells. Nat. Commun. 11, 5555. doi:10.1038/s41467-020-19304-9
Grainger N., Guarina L., Cudmore R. H., Santana L. F. (2021). The organization of the sinoatrial node microvasculature varies regionally to match local myocyte excitability. Funct. (Oxf) 2, zqab031. zqab031. doi:10.1093/function/zqab031
Guarina L., Moghbel A. N., Pourhosseinzadeh M. S., Cudmore R. H., Sato D., Clancy C. E., et al. (2022). Biological noise is a key determinant of the reproducibility and adaptability of cardiac pacemaking and EC coupling. J. Gen. Physiol. 154, e202012613. doi:10.1085/jgp.202012613
Honjo H., Boyett M. R., Kodama I., Toyama J. (1996). Correlation between electrical activity and the size of rabbit sino-atrial node cells. J. Physiol. 496 (3), 795–808. doi:10.1113/jphysiol.1996.sp021728
Jalife J. (1984). Mutual entrainment and electrical coupling as mechanisms for synchronous firing of rabbit sino-atrial pace-maker cells. J. Physiol. 356, 221–243. doi:10.1113/jphysiol.1984.sp015461
Jones S. A., Boyett M. R., Lancaster M. K. (2007). Declining into failure: The age-dependent loss of the L-type calcium channel within the sinoatrial node. Circulation 115, 1183–1190. doi:10.1161/CIRCULATIONAHA.106.663070
Keith A., Flack M. (1907). The form and nature of the muscular connections between the primary divisions of the vertebrate heart. J. Anat. Physiol. 41, 172–189.
Kim M. S., Maltsev A. V., Monfredi O., Maltseva L. A., Wirth A., Florio M. C., et al. (2018). Heterogeneity of calcium clock functions in dormant, dysrhythmically and rhythmically firing single pacemaker cells isolated from SA node. Cell Calcium 74, 168–179. doi:10.1016/j.ceca.2018.07.002
Lang D., Petrov V., Lou Q., Osipov G., Efimov I. R. (2011). Spatiotemporal control of heart rate in a rabbit heart. J. Electrocardiol. 44, 626–634. doi:10.1016/j.jelectrocard.2011.08.010
Larson E. D., St Clair J. R., Sumner W. A., Bannister R. A., Proenza C. (2013). Depressed pacemaker activity of sinoatrial node myocytes contributes to the age-dependent decline in maximum heart rate. Proc. Natl. Acad. Sci. U. S. A. 110, 18011–18016. doi:10.1073/pnas.1308477110
Li N., Hansen B. J., Csepe T. A., Zhao J., Ignozzi A. J., Sul L. V., et al. (2017). Redundant and diverse intranodal pacemakers and conduction pathways protect the human sinoatrial node from failure. Sci. Transl. Med. 9, eaam5607. doi:10.1126/scitranslmed.aam5607
Liu J., Sirenko S., Juhaszova M., Sollott S. J., Shukla S., Yaniv Y., et al. (2014). Age-associated abnormalities of intrinsic automaticity of sinoatrial nodal cells are linked to deficient cAMP-PKA-Ca2+ signaling. Am. J. Physiol. Heart Circ. Physiol. 306, H1385–H1397. doi:10.1152/ajpheart.00088.2014
Louradour J., Bortolotti O., Torre E., Bidaud I., Lamb N., Fernandez A., et al. (2022). L-type Cav1.3 calcium channels are required for beta-adrenergic triggered automaticity in dormant mouse sinoatrial pacemaker cells. Cells 11. doi:10.3390/cells11071114
Lyashkov A. E., Behar J., Lakatta E. G., Yaniv Y., Maltsev V. A. (2018). Positive feedback mechanisms among local Ca releases, NCX, and ICaL ignite pacemaker action potentials. Biophys. J. 114, 2024–1189. doi:10.1016/j.bpj.2018.03.024
Lyashkov A. E., Juhaszova M., Dobrzynski H., Vinogradova T. M., Maltsev V. A., Juhasz O., et al. (2007). Calcium cycling protein density and functional importance to automaticity of isolated sinoatrial nodal cells are independent of cell size. Circ. Res. 100, 1723–1731. doi:10.1161/CIRCRESAHA.107.153676
Lyashkov A. E., Vinogradova T. M., Zahanich I., Li Y., Younes A., Nuss H. B., et al. (2009). Cholinergic receptor signaling modulates spontaneous firing of sinoatrial nodal cells via integrated effects on PKA-dependent Ca2+ cycling and IKACh. Am. J. Physiol. Heart Circ. Physiol. 297, H949–H959. doi:10.1152/ajpheart.01340.2008
Maltsev A. V., Stern M. D., Lakatta E. G., Maltsev V. A. (2022a). Functional heterogeneity of cell populations increases robustness of pacemaker function in a numerical model of the sinoatrial node tissue. Front. Physiol. 13, 845634. doi:10.3389/fphys.2022.845634
Maltsev A. V., Stern M. D., Maltsev V. A. (2022b). Disorder in Ca2+ release unit locations confers robustness but cuts flexibility of heart pacemaking. J. Gen. Physiol. 154, e202113061. doi:10.1085/jgp.202113061
Maltsev V. A., Lakatta E. G. (2009). Synergism of coupled subsarcolemmal Ca2+ clocks and sarcolemmal voltage clocks confers robust and flexible pacemaker function in a novel pacemaker cell model. Am. J. Physiol. Heart Circ. Physiol. 296, H594–H615. doi:10.1152/ajpheart.01118.2008
Michaels D. C., Matyas E. P., Jalife J. (1987). Mechanisms of sinoatrial pacemaker synchronization: A new hypothesis. Circ. Res. 61, 704–714. doi:10.1161/01.res.61.5.704
Monfredi O., Tsutsui K., Ziman B., Stern M. D., Lakatta E. G., Maltsev V. A. (2018). Electrophysiological heterogeneity of pacemaker cells in the rabbit intercaval region, including the SA node: Insights from recording multiple ion currents in each cell. Am. J. Physiol. Heart Circ. Physiol. 314, H403–H414. doi:10.1152/ajpheart.00253.2016
Nakayama T., Kurachi Y., Noma A., Irisawa H. (1984). Action potential and membrane currents of single pacemaker cells of the rabbit heart. Pflugers Arch. 402, 248–257. doi:10.1007/BF00585507
Noble D. (1962). A modification of the Hodgkin-Huxley equations applicable to Purkinje fibre action and pace-maker potentials. J. Physiol. 160, 317–352. doi:10.1113/jphysiol.1962.sp006849
Norris C. F., Maltsev A. V. (2022). Meaningful local signaling in sinoatrial node identified by random matrix theory. BioRxiv. (preprint). doi:10.1101/2022.02.25.482007
Qu Z., Garfinkel A., Weiss J. N., Nivala M. (2011). Multi-scale modeling in biology: How to bridge the gaps between scales? Prog. Biophys. Mol. Biol. 107, 21–31. doi:10.1016/j.pbiomolbio.2011.06.004
Sano T., Sawanobori T., Adaniya H. (1978). Mechanism of rhythm determination among pacemaker cells of the mammalian sinus node. Am. J. Physiol. 235, H379–H384. doi:10.1152/ajpheart.1978.235.4.H379
Sirenko S., Yang D., Li Y., Lyashkov A. E., Lukyanenko Y. O., Lakatta E. G., et al. (2013). Ca2+-dependent phosphorylation of Ca2+ cycling proteins generates robust rhythmic local Ca2+ releases in cardiac pacemaker cells. Sci. Signal. 6, ra6. doi:10.1126/scisignal.2003391
Stern M. D., Maltseva L. A., Juhaszova M., Sollott S. J., Lakatta E. G., Maltsev V. A. (2014). Hierarchical clustering of ryanodine receptors enables emergence of a calcium clock in sinoatrial node cells. J. Gen. Physiol. 143, 577–604. doi:10.1085/jgp.201311123
Torrente A. G., Mesirca P., Neco P., Rizzetto R., Dubel S., Barrere C., et al. (2016). L-type Cav1.3 channels regulate ryanodine receptor-dependent Ca2+ release during sino-atrial node pacemaker activity. Cardiovasc. Res. 109, 451–461. doi:10.1093/cvr/cvw006
Tsutsui K., Florio M. C., Yang A., Wirth A. N., Yang D., Kim M. S., et al. (2021). cAMP-dependent signaling restores AP firing in dormant SA node cells via enhancement of surface membrane currents and calcium coupling. Front. Physiol. 12, 596832. doi:10.3389/fphys.2021.596832
Tsutsui K., Monfredi O., Sirenko-Tagirova S. G., Maltseva L. A., Bychkov R., Kim M. S., et al. (2018). A coupled-clock system drives the automaticity of human sinoatrial nodal pacemaker cells. Sci. Signal. 11, eaap7608. doi:10.1126/scisignal.aap7608
Vinogradova T. M., Lyashkov A. E., Zhu W., Ruknudin A. M., Sirenko S., Yang D., et al. (2006). High basal protein kinase A-dependent phosphorylation drives rhythmic internal Ca2+ store oscillations and spontaneous beating of cardiac pacemaker cells. Circ. Res. 98, 505–514. doi:10.1161/01.RES.0000204575.94040.d1
Weiss J. N., Qu Z. (2020). The sinus node: Still mysterious after all these years. JACC. Clin. Electrophysiol. 6, 1841–1843. doi:10.1016/j.jacep.2020.09.017
Keywords: heartbeat, pacemaker mechanism, diastolic depolarization, local calcium release, sinoatrial node
Citation: Maltsev VA and Stern MD (2022) The paradigm shift: Heartbeat initiation without “the pacemaker cell”. Front. Physiol. 13:1090162. doi: 10.3389/fphys.2022.1090162
Received: 05 November 2022; Accepted: 23 November 2022;
Published: 09 December 2022.
Edited by:
Daisuke Sato, University of California, Davis, United StatesReviewed by:
Seth H. Weinberg, The Ohio State University, United StatesMartin Falcke, Max Delbrück Center for Molecular Medicine (HZ), Germany
Copyright © 2022 Maltsev and Stern. This is an open-access article distributed under the terms of the Creative Commons Attribution License (CC BY). The use, distribution or reproduction in other forums is permitted, provided the original author(s) and the copyright owner(s) are credited and that the original publication in this journal is cited, in accordance with accepted academic practice. No use, distribution or reproduction is permitted which does not comply with these terms.
*Correspondence: Michael D. Stern, U3Rlcm5NaUBtYWlsLm5paC5nb3Y=