- 1Julius Maximilian University of Würzburg, Würzburg, Germany
- 2Neurobiology and Genetics, Theodor-Boveri-Institute, Biocenter, University of Würzburg, Würzburg, Würzburg, Germany
- 3Graduate School of Natural Science and Technology, Okayama University, Okayama, Japan
Drosophila’s dorsal clock neurons (DNs) consist of four clusters (DN1as, DN1ps, DN2s, and DN3s) that largely differ in size. While the DN1as and the DN2s encompass only two neurons, the DN1ps consist of ∼15 neurons, and the DN3s comprise ∼40 neurons per brain hemisphere. In comparison to the well-characterized lateral clock neurons (LNs), the neuroanatomy and function of the DNs are still not clear. Over the past decade, numerous studies have addressed their role in the fly’s circadian system, leading to several sometimes divergent results. Nonetheless, these studies agreed that the DNs are important to fine-tune activity under light and temperature cycles and play essential roles in linking the output from the LNs to downstream neurons that control sleep and metabolism. Here, we used the Flybow system, specific split-GAL4 lines, trans-Tango, and the recently published fly connectome (called hemibrain) to describe the morphology of the DNs in greater detail, including their synaptic connections to other clock and non-clock neurons. We show that some DN groups are largely heterogenous. While certain DNs are strongly connected with the LNs, others are mainly output neurons that signal to circuits downstream of the clock. Among the latter are mushroom body neurons, central complex neurons, tubercle bulb neurons, neurosecretory cells in the pars intercerebralis, and other still unidentified partners. This heterogeneity of the DNs may explain some of the conflicting results previously found about their functionality. Most importantly, we identify two putative novel communication centers of the clock network: one fiber bundle in the superior lateral protocerebrum running toward the anterior optic tubercle and one fiber hub in the posterior lateral protocerebrum. Both are invaded by several DNs and LNs and might play an instrumental role in the clock network.
Introduction
Circadian clocks anticipate the 24-h rhythms on Earth. In animals, a master clock is located in the brain that tunes physiology, metabolism, and behavior to their respective temporal niche. In mammals and insects, this circadian master clock consists of tightly interacting neurons that may fulfill different roles in the clock network based on their neurochemistry, morphology and physiology (Beckwith and Ceriani, 2015; Stengl and Arendt, 2016; Helfrich-Förster, 2017; Herzog et al., 2017; Top and Young, 2018; Rojas et al., 2019; King and Sehgal, 2020; Michel and Meijer, 2020; Mieda, 2020; Ahmad et al., 2021).
The clock network of Drosophila melanogaster consists of only about 150 clock neurons which can be divided according to the location of their cell bodies into five lateral and four dorsal groups. Some of them overlap with their projections in the ventrolateral brain in the so-called accessory medulla (AME) and in the dorsomedial brain, important communication centers of the clock network (Figure 1; Guo et al., 2016, 2018; Chatterjee et al., 2018; Fujiwara et al., 2018; Goda et al., 2018; Schubert et al., 2018; Díaz et al., 2019; Reinhard et al., 2022). So far, most attention has been focused on the lateral neurons in the anterior brain (s-LNvs, l-LNvs, fifth LN, and LNds) because some of them appear most important for driving rhythmic behavior under constant environmental conditions, in the absence of all Zeitgebers (Ewer et al., 1992; Frisch et al., 1994; Helfrich-Förster et al., 1998; Renn et al., 1999; Grima et al., 2004). More recently, the lateral neurons in the posterior brain (LPNs) have also been studied in detail and found to be involved in the control of sleep and activity patterns in the presence of light-dark or temperature cycles but not under constant environmental conditions (Chen et al., 2016; Díaz et al., 2019; Ni et al., 2019; Reinhard et al., 2022).
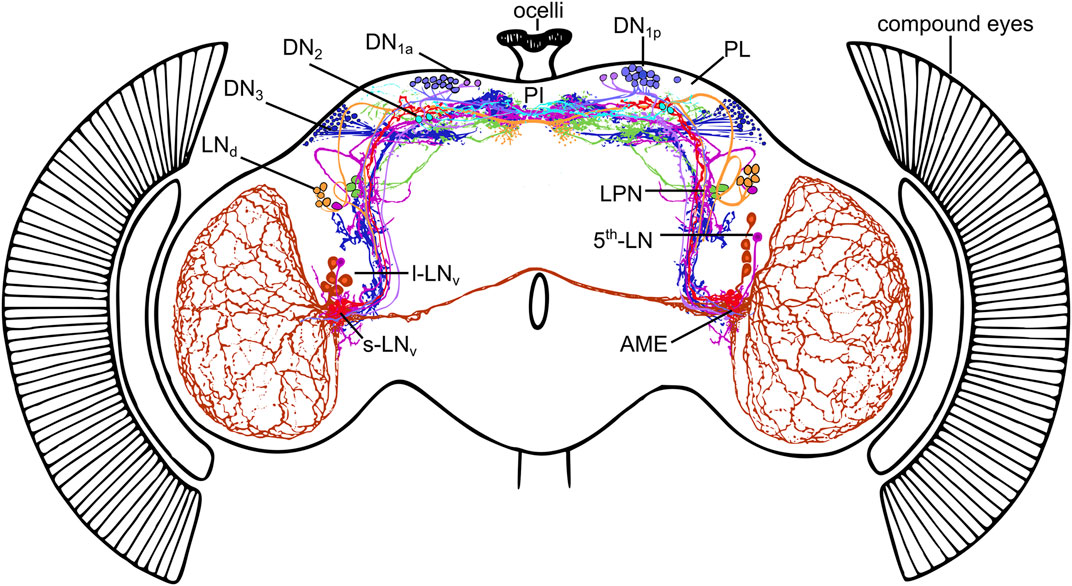
FIGURE 1. The circadian clock neurons in the brain of Drosophila melanogaster. The clock network consists of Lateral Neurons (s-LNvs, 5th-LN, l-LNvs, LNds, and LPNs) and Dorsal Neurons (DN1as, DN1ps, DN2s, DN3s), the neurites of which are highly connected. Many of them send fibers into the dorsal protocerebrum (including the neurosecretory centers in the pars intercerebralis (PI) and pars lateralis (PL)) as well as into the accessory medulla (AME) of both hemispheres, small neuropils at the base of the medulla. The AME can be regarded as the communication center of the clock neurons. Modified from Helfrich-Förster et al., 2007a with data from Schubert et al., 2018 and Reinhard et al., 2022 and this study.
The four groups of dorsal neurons (DN1as, DN1ps, DN2s, and DN3s) are the least well characterized. Like the LPNs, the DNs appear to be less important in controlling rhythmic behavior under constant environmental conditions. Nevertheless, they appear to fine-tune behavioral rhythmicity under light and temperature cycles (Miyasako et al., 2007; Picot et al., 2009; Yoshii et al., 2009; Zhang L. et al., 2010, Zhang et al., 2010 Y.; Harper et al., 2016; Chatterjee et al., 2018; Yadlapalli et al., 2018; Goda et al., 2019; Ni et al., 2019; Lamaze and Stanewsky, 2020). Furthermore, these clock neurons play essential roles in the output from the core clock network to downstream neurons. For example, they participate in the control of sleep and metabolism (Cavanaugh et al., 2014; Kunst et al., 2014; Barber et al., 2016; Guo et al., 2016, 2018; Ni et al., 2019)
Here, we used a combination of GAL4, GAL80, and newly generated split-GAL4 transgenic lines (Sekiguchi et al., 2020) to address specific groups of dorsal clock neurons that were not accessible so far. These drivers combined with the Flybow system (Hadjieconomou et al., 2011; Shimosako et al., 2014; Schubert et al., 2018) and with other tools, including trans-Tango (Talay et al., 2017), and electron microscopic data from the hemibrain (Scheffer et al., 2020) allowed us to characterize the anatomy and the synaptic connections of some DNs in greater detail. We will discuss our results in the light of recent physiological and behavioral studies that provide insights into the functions of the DNs.
Materials and Methods
Fly Strains, Husbandry, and Crossings
Unless stated otherwise, fly strains used in this study were reared on standard cornmeal medium with yeast at 25 ± 0.2°C and 60 ± 5% relative humidity under light-dark cycles (LD) of 12:12 h. All fly lines used are described in Supplementary Table S1 (including references).
To reveal the anatomy of specific clock neurons, GAL4 or split-GAL4 lines were crossed to a membrane-bound GFP reporter (10xUAS-myr::GFP) or to a cytoplasmic myc-tagged GFP reporter (20UAS-6xGFP, Supplementary Table S1). They were co-stained with antibodies against the clock protein Period (PER) and different antibodies against several neuropeptides to verify their neurochemistry (see immunohistochemical procedure below). To reveal the post- and presynaptic sites of the neurons of interest, we crossed the GAL4-drivers to UAS-DenMark::mCherry and UAS-nSyb::EGFP, respectively (Supplementary Table S1).
A driver stock for usage with the Flybow system (see below) was built by balancing the GAL4-lines listed in Table 1 and crossing them to y w;hs-mFlp5MH12/CyO; TM2/TM6B or to y w; GlaBc/CyO;hs-mFlp5MH3/TM6B depending on which chromosome the GAL4 insertion was located. Experimental flies were obtained by crossing the balanced GAL4/hs-mFlp5 lines to either hs-Flp1;+;FB2.0B49b or to hs-Flp1;FB2.0B260b;+ (Supplementary Table S1).
Flybow
For labeling individual clock neurons we used the revised multicolor Flybow system with the Flybow2.0B reporter construct, which carries an FRT-site flanked stop-codon upstream of the fluorescent protein sequences (Shimosako et al., 2014). The stop codon can be removed by Flp-recombination, induced by a heat shock-promotor controlled hs-Flp DNA-recombinase. Experimental flies were kept at 18°C (18 ± 0.2°C and 60 ± 5% relative humidity with a LD cycle of 12:12) to prevent uncontrolled Flippase recombination events. Three heat shocks (37°C) of 45–60 min were applied on three consecutive days to induce Flippase-recombinase activity at different larval and pupal stages until the clock network was fully developed, which appears to be the case in late pupal stages (Helfrich-Förster et al., 2007a). Adult flies (4–5 days after eclosion) were dissected and the brains were immunolabeled with anti-GFP, anti-mCherry, and anti-nc82 antibody-solution, as described in Schubert et al. (2018).
Trans-Tango
To reveal putative post-synaptic partners of selected clock neurons, we applied the trans-Tango technique (Talay et al., 2017) in combination with new clock driver lines (Sekiguchi et al., 2020). The crosses were reared at 25°C and the progeny transferred at 18°C after eclosion for several days, to obtain an accumulation of the post-synaptic signal. In general, we sampled flies after 17, 28, 30, 34, and 53 days at 18°C and found that the post-synaptic signal strength increases significantly with time. Presynaptic neurons were revealed by anti-GFP and postsynaptic neurons by anti-Hemagglutinin (HA) antibody staining (see below and Supplementary Table S2).
Immunocytochemistry
Fluorescent immunocytochemistry was performed according to the protocol described in Schubert et al. (2018). In brief, male flies were fixed as whole animals for 2–3 h in 4% paraformaldehyde after they have been entrained to LD12:12 cycles for 4–5 days after eclosion. For anti-PER staining, fixation was performed 1 h before lights-on (ZT23), since PER accumulation is maximal at this time (Zerr et al., 1990). For the other antibody staining, flies were fixed around ZT3. For staining against Cryptochrome (CRY), the flies were kept several days under constant darkness to allow CRY to accumulate. After dissecting the brains in phosphate-buffered saline (pH 7.4) they were incubated in 5% normal goat serum in PBT 0.5% overnight and then incubated in the primary antibody solution for ∼2 days. Fluorescent tagged secondary antibodies were applied for at least 3 h. All used antibodies are listed in Supplementary Table S2. The immunostained brains were aligned on a specimen slide, embedded in Vectashield 1000 mounting medium (Vector Laboratories, Burlingame, CA, United States), and stored at 4°C until scanning. If not otherwise stated at least 10 different brains were stained for each antibody or antibody combination.
Confocal Microscopy and Image Processing
Fluorescence protein expression and antibody staining were visualized and scanned with a Leica TCS SP8 or a Leica SPE confocal microscope (Leica Microsystems, Wetzlar, Germany), Leica SP8 was equipped with hybrid detectors, a photon multiplier tube, and a white light laser for excitation, using the laser and detector settings as described in Shimosako et al. (2014). Leica SPE was equipped with a photomultiplier tube and 488, 532, and 635 nm solid-state lasers for excitation.
We used a 20-fold glycerol immersion objective (HC PL APO, Leica Microsystems, Wetzlar Germany) for whole-mount scans and obtained confocal stacks with 2 µm z-step size and 1024 × 1024 pixels. For a more detailed view, we used a 63-fold glycerol objective (HC PL APO, Leica Microsystems, Wetzlar Germany) and scanned the brains with a resolution of 2048 × 2048 pixels and a z-resolution of 1 µm. All focal planes were scanned three to four times and the frames were averaged to reduce background noise. The hybrid detectors of SP8 were used with photon counting mode and each focal plane was scanned and accumulated four times. The obtained confocal stacks were maximum projected and analyzed with Fiji ImageJ (Schindelin et al., 2012). Besides contrast and brightness, no further manipulations were done to the confocal images. For displaying the depth information in maximum projections we used the plug-in “Z-stack Depth Color Code” (v.0.0.2) for Fiji. For the reconstruction of the DN2s, we used the simple neurite tracer plug-in (Arshadi et al., 2021). The reconstructions were visualized using the natverse libraries (v 0.2.4, Bates et al., 2020) for RStudio (v 1.3.1093). The anti-nc82 staining (anti-bruchpilot) was used to align our stacks to the Janelia Farm Research Campus standard brain (JFRC2) by using the computational morphometry tool kit graphical user interface plug-In for Fiji (CMTK GUI, Rohlfing and Maurer, 2003; Jefferis et al., 2007). We compared our anatomical results with the results gained in the hemibrain of a single female fly (Scheffer et al., 2020) and the reconstructions of the DN1as by Marin et al. (2020) available for the full adult female brain (FAFB, Zheng et al., 2018).
Comparison With Electron Microscopic Reconstruction Data
For a comparison of our findings with the reconstructed neurons of the electron microscopic data set from the hemibrain and the FAFB, we used the natverse libraries (v 0.2.4, Bates et al., 2020) and hemibrainr package (v.0.5.0, Bates and Jefferis, 2022) for R (v. 4.0.5) via RStudio (v 1.3.1093). The neuron-specific information was obtained from the hemibrain dataset (Scheffer et al., 2020, v 1.2.1) of the neuprint server (neuprint.janelia.org). For the FAFB dataset (Zheng et al., 2018) the reconstructions were obtained from the Virtual Fly Brain CATMAID server (fafb.catmaid.virtualflybrain.org). The annotation of the neurons was used as a reference and the identity of the neurons was verified by their morphology and the orientation in the brain if possible. For morphological comparisons, the neurons and brain regions of the hemibrain were transformed into the JRC 2018F template space (Bogovic et al., 2020) using xform_brain (nat.templatebrains v 1.0). When different neuron types were shown in the same brain, the neurons were additionally mirrored to the other hemisphere. Connectivity data was visualized using the ggplot2 package (v3.3.5, Wickham, 2016).
Results
To facilitate understanding, we describe the morphology and synaptic connectivity of the different dorsal neurons in the context of the existing literature and, where appropriate, discuss the reasons for differences between studies.
The DN1as
The DN1as express PER from the first larval instar onward (Kaneko et al., 1997). They are glutamatergic (Hamasaka et al., 2007; Collins et al., 2012) and in adult flies, they express additionally the neuropeptides IPNamide (Shafer et al., 2006) and CChamide1 (Fujiwara et al., 2018). Furthermore, they express the intracellular blue-light receptor Cryptochrome (CRY) (Yoshii et al., 2008). We addressed the two DN1as with Flybow using the R16C05-GAL4 driver (Pfeiffer et al., 2008). Using this method, we were able to trace individual DN1as in 30 brains, whereas in 11 brains only a single DN1a was labeled and could be analyzed at the single-cell level. In addition, the two DN1as were stained together in the DN1a-specific split-GAL4 line R43D05-p65.AD; R93B11-DBD (Sekiguchi et al., 2020). In the following, we will describe the morphology of the DN1as according to the results of both staining methods compared to the reconstructions at the electron microscopic level (Supplementary Video S1).
The cell bodies of both DN1as are located in the anterior superior cell body rind dorsal to the superior lateral protocerebrum (SLP) (Shafer et al., 2006). Initially, the DN1as project ventrally along the surface of the SLP into the posterior-most part of the SLP. The projections of both cells bifurcate at the level of the posterior boundary between the lateral horn (LH) and the SLP and invade the posterior-most part of the LH. Here, the neurites of both DN1as branch extensively and innervate the ventromedial region of the posterior LH (Figure 2C), together with the s-LNv terminals. Both neurons send projections medially that terminate dorsally to the Kenyon cells of the mushroom bodies in the lateral and dorsal accessory calyx (CA, Figures 2A,C, arrow). Further projections run ventrally to the accessory medulla (AME, Figure 2, open arrowhead). Concerning the shape and extent of arborizations in the posterior SLP and LH, the two neurons differed slightly. The varicose arborizations of one DN1a appeared slightly more restricted around the CA whereas the other DN1a showed less dense projections, extending slightly more medially in the SLP as well as more ventrally in the posterior LH. The biggest difference between the two DN1as represented the single neurite of the neuron with the slightly broader arborization pattern, reaching from the dense ramifications around the CA in the dorsal SLP (Figure 2 double arrowhead). In roughly one-third of the DN1as analyzed with Flybow the projections to the AME were not visible. However, the DN1a-specific split-GAL4 line revealed that both DN1as project ventrally (Figure 2C open arrowhead). This projection ran parallel to the neurites of the Pigment Dispersing Factor (PDF)-positive s-LNvs until it reached and joined the posterior optic commissure (Figures 2A,C, open arrow). Along with this major fiber bundle, the ventral DN1a projections invaded the ipsilateral AME and often ran onto the surface or along the anteromedial edge of the medulla (Figures 2A,C). Due to the vast overlap of their arborizations in most parts, distinguishing the two neurons in the polarity staining was difficult. We observed signals of the post- and presynaptic markers in the posterior ventromedial LH, probably originating from both DN1as. The presynaptic vesicles in the projection to the AME were consistently and strongly labeled (nSyb::EGFP), whereas only a faint DenMark (TLN::mCherry) signal (postsynaptic marker) was found in these neurites (Figures 2D,E).
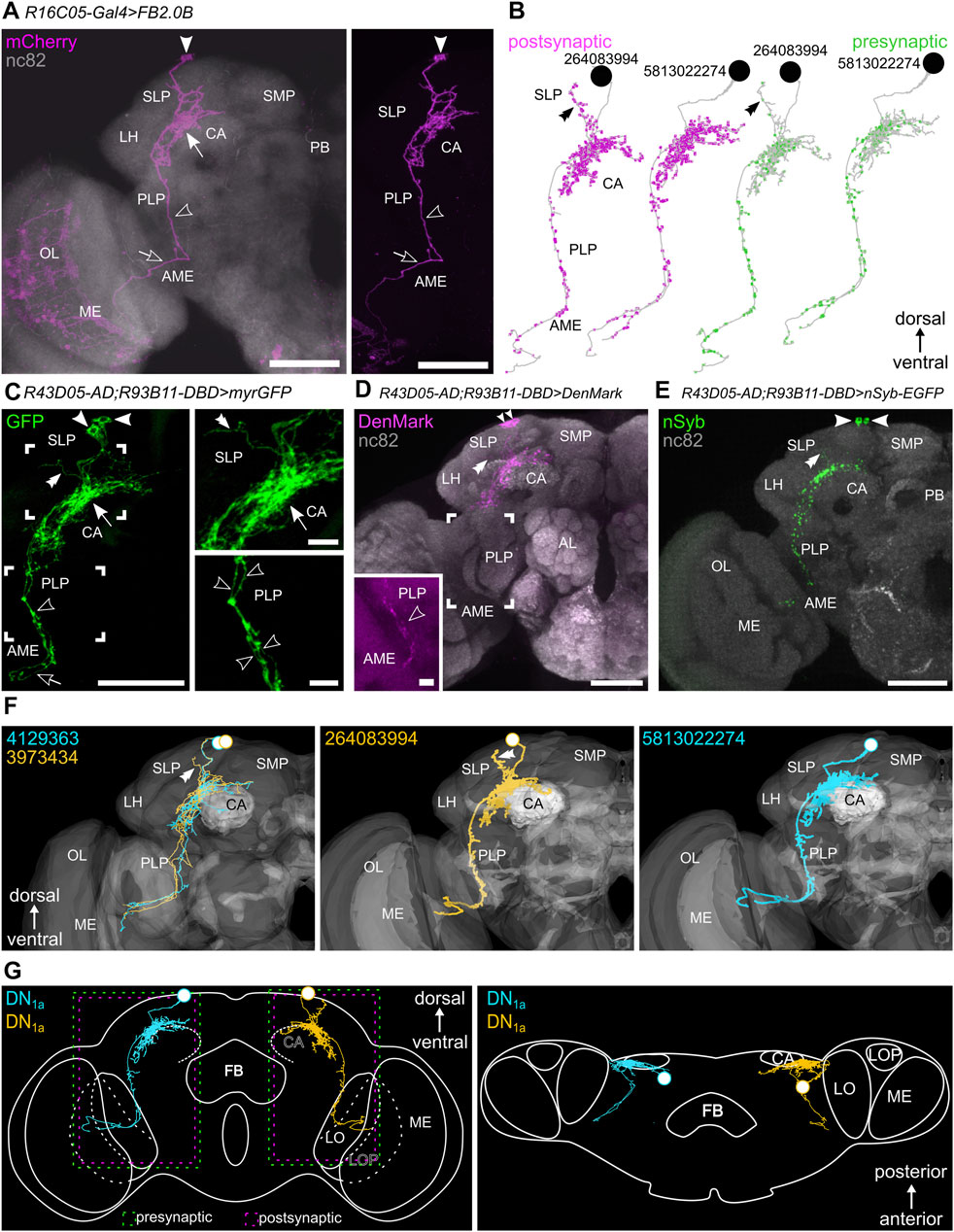
FIGURE 2. The morphology of the two DN1as. (A) Flybow-reporter expression (mCherrry, magenta) driven by R16C05-GAL4 and anti-nc82 neuropil staining (gray). Only one of the two DN1as is labeled together with several small cells in the optic lobe. A single fiber of this neuron projects from the SLP through the ventromedial lateral horn (LH) and the posterior lateral protocerebrum (PLP) into the accessory medulla (AME) and further toward the surface of the medulla (ME) (open arrow). The arrow points to its dense arborizations around the mushroom body calyx (CA). (B) Postsynaptic (magenta) and presynaptic (green) sites of the two DN1as (revealed in the hemibrain) are distributed over the entire neurons. (C) Morphology of both DN1as visualized in the highly DN1a specific split-GAL4 line with membrane-bound GFP (maximal projection of 47 confocal planes). Both DN1as project to the AME (open arrow and arrowhead) and only differ by a faint anteriorly projecting fiber in the SLP (double arrowhead, see also inset). The insets show magnifications of the arborizations close to the CA and the PLP. (D) Staining against the DenMark reporter (mCherry) which visualizes the dendritic fibers of the DN1as. The inset shows a magnification of the ventrally running fibers towards the AME with a maximal projection of only 15 confocal planes for clarification. (E) GFP staining of the nSyb-EGFP fusion protein that visualizes the axonal terminals of the DN1as. (F) Reconstructions of the two DN1as on the electron microscopic level derived from the FAFB (Marin et al., 2020) and the hemibrain (Scheffer et al., 2020) The double arrowhead highlights the anterior running arm of one of the two DN1as (yellow). (G) Schematic overview of the DN1a morphology from a frontal (left) and dorsal (right) view. The rectangles with dashed lines in the left picture represent post- and presynaptic sites as revealed by DenMark and nSyb staining, respectively. LOP, lobula plate; LO, lobula; CA, mushroom body calyx; SLP, superior lateral protocerebrum; SMP, superior medial protocerebrum; LH, lateral horn; AL, antennal lobes; PB, protocerebral bridge; OL, optic lobes; FB, fan-shaped body; ME, medulla; Scale bars represent 50 and 10 µm in detail views.
The reconstructions of the hemibrain largely support our observations and the two annotated DN1as (#264083994 and #5813022274) have the same characteristic arborization pattern as described above (Figure 2F). The reconstructions by Marin et al. (2020) using the FAFB dataset (Figure 2F, neuron #4129363 and #3973434) support additionally our observation that the DN1as differ in the neurite reaching in the dorsal SLP (Figure 2F double arrowhead). Output synapses (presynaptic terminals) were present throughout the neurites, but less densely in the one fiber of DN1a #264083994 that projects dorsally into the SLP. This dorsally projecting fiber carried mainly input synapses (postsynaptic terminals) (Figure 2B double arrowhead).
Synaptic Partners of the DN1as
Both DN1as appear strongly linked to the s-LNvs. The neurites of both neuron types have been shown to largely overlap with each other (Fujiwara et al., 2018), an observation that we confirm with our study. The DN1as overlap with the terminals of the s-LNvs in the SLP and with the dendrites of the s-LNvs in the AME. Also functionally, the DN1as and the s-LNvs are connected as was shown by previous work: the DN1as signal via glutamate and the neuropeptide CCHamide1 to the s-LNvs (Hamasaka et al., 2007; Collins et al., 2012; Fujiwara et al., 2018; Song et al., 2021) while the s-LNvs signal via glycine and PDF to the DN1as (Shafer et al., 2008; Yoshii et al., 2009; Frenkel et al., 2017). To further investigate this mutual synaptic connection and to identify synaptic outputs of the DN1as to other downstream neurons we performed trans-Tango and visualized the results by anti-HA in flies of different ages (17, 28, 30 days).
When driving trans-Tango in the DN1as, several clock neurons (PER-positive) were co-labeled by the HA-antibody (Figures 3A,B): one to two DN3s, the Ion transport peptide (ITP)-positive LNd and fifth LN, one to four s-LNvs (Figures 3A,B, white arrows) and sometimes weakly one to four l-LNvs as already shown by Song et al. (2021). Also, the DN1as themselves were labeled in some brains. In addition, one characteristic neuron of the dorsal fan-shaped body (dFB) was marked by anti-HA. Its cell body was located in the posterior brain (Figure 3A magenta arrowheads) with neurites running into the eighth layer of the FB and laterally toward a dense fiber hub close to the LPNs, in which several clock neurons arborize and the DN1as contribute few fibers (Figure 3A, magenta arrows; see also later). Further non-clock neurons were labeled in the lateral, dorsolateral and posterior dorsal brain. These were located close to the LNds, DN3s, and DN1ps, respectively, but were not double-labeled by anti-PER (Figures 3A,B, open magenta arrowheads). While the DN3s and most of the LNs were already stained in young flies, the neuron projecting to the dFB was only marked in older flies.
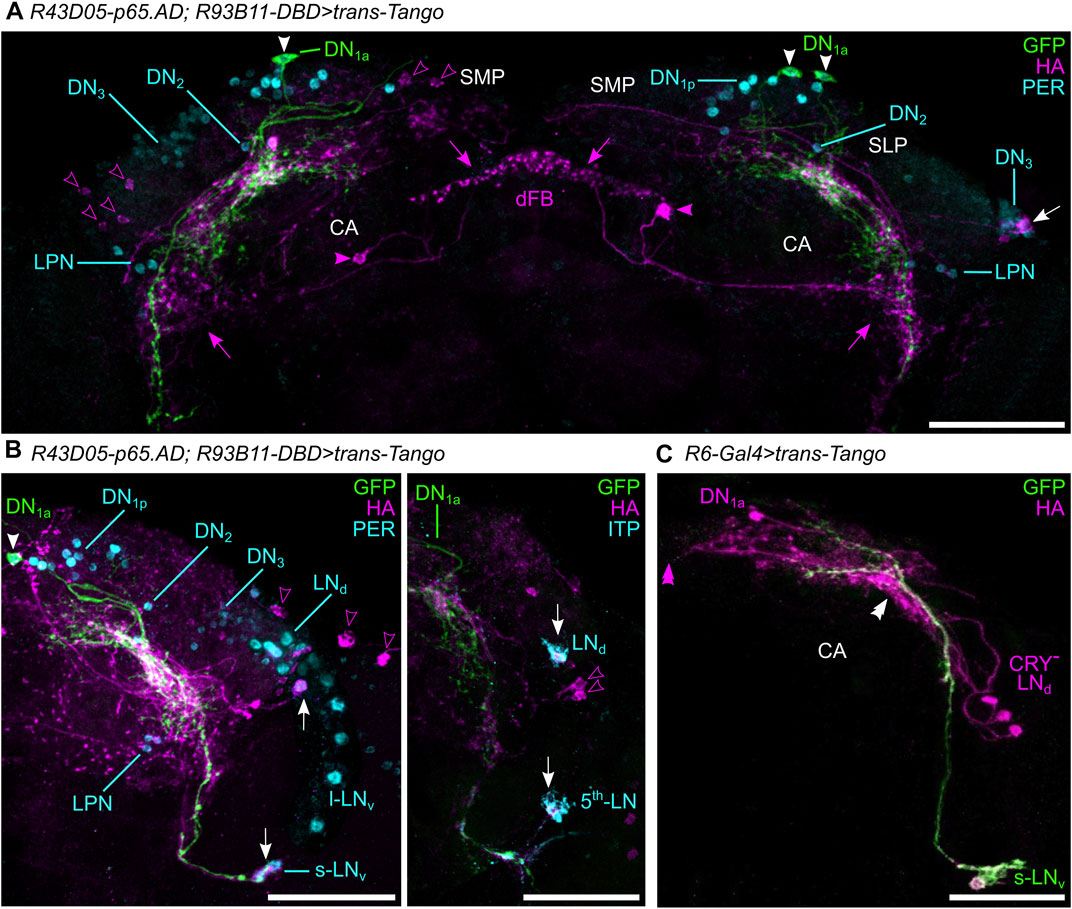
FIGURE 3. Neurons pre- and postsynaptic to the DN1as revealed by trans-Tango. (A) Maximal projections of 30 confocal planes depicting a frontal view of the anterior part of the dorsal protocerebrum with the presynaptic DN1as marked in green (green fluorescent protein, GFP), their postsynaptic partners in magenta (hemagglutinin, HA), and other clock neurons marked in cyan (labeled with anti-PER). One to two DN3 clock neurons were labeled by HA and are consequently postsynaptic to the DN1as (white arrow). HA additionally labeled unknown postsynaptic neurons (open magenta arrowheads) in the superior medial (SMP) and the superior lateral protocerebrum (SLP). Further, a neuron of the eighth layer of the fan-shaped body (FB) was labeled as postsynaptic to the DN1as (magenta arrowheads). It innervated not only the dorsal FB (dFB) but also the posterior lateral protocerebrum at about the height of the LPNs (magenta arrows). In this region, a network of fibers postsynaptic of the DN1as is found that is adjacent to the dense varicose fibers of the DN1as. (B) Maximal projection of 40 confocal planes depicting frontal views of the right lateral protocerebrum with the presynaptic DN1as labeled in green and their postsynaptic partners in magenta. In the left picture, all clock neurons are labeled by anti-PER in cyan, whereas in the right picture the ITP-positive clock neurons (LNd and fifth LN) are labeled in cyan. White arrows point to clock neurons that are postsynaptic to the DN1as, Two s-LNvs and one LNd (left) and the ITP-positive fifth LN and LNd (right). Note that in the brain shown to the left the l-LNvs and the fifth LNd are located quite dorsally and cannot be distinguished from each other. The LNds are also located more dorsally than usually and overlap with the DN3s. (C) Frontal view of the lateral protocerebrum with the presynaptic s-LNvs marked by GFP and their postsynaptic partners in magenta (maximal projection of 30 confocal planes). The s-LNv appear to have synaptic contacts with at least one DN1a and the three CRY-negative LNds. Since the CRY-negative LNds do not project contralaterally to the other hemisphere (Schubert et al., 2018) the midline of the brain (magenta double arrowhead) is free of marked fibers. The white double arrowhead marks the putative area of synaptic contacts between the s-LNv and the DN1a/LNd. CA, calyx of the mushroom bodies. The scale bars represent 50 µm.
When driving trans-Tango in the s-LNvs, we found that at least one DN1a is postsynaptic to the s-LNvs (Figure 3C). In addition, the three CRY-negative LNds were marked, indicating that they are also postsynaptic to the s-LNvs.
The trans-Tango staining was rather consistent with the presynaptic (output) sites of the DN1as revealed on the ultrastructural level in the hemibrain (Figure 4A). Most output synapses were found in the superior protocerebrum (SLP and SMP) to the ITP- and CRY-positive LNd and the fifth LN, but no outputs were found to the s-LNvs (Figure 4A) and very few to DN3-like neurons (see below). The virtual lack of output to the DN3s is understandable because these neurons are not unequivocally annotated in the hemibrain, but it is less clear why the s-LNvs are not among the listed postsynaptic partners of the DN1as. Most probably, they form only a small amount of synapses with these clock cells that have been overlooked. The same might be true for the input synapses from the s-LNvs that we detected in our trans-Tango staining. The s-LNvs are not listed as input partners of the DN1as in the hemibrain (Figure 4B). Nevertheless, input from the s-LNvs to the DN1as was also revealed in a recent functional study, strongly indicating that our observation is valid (Song et al., 2021).
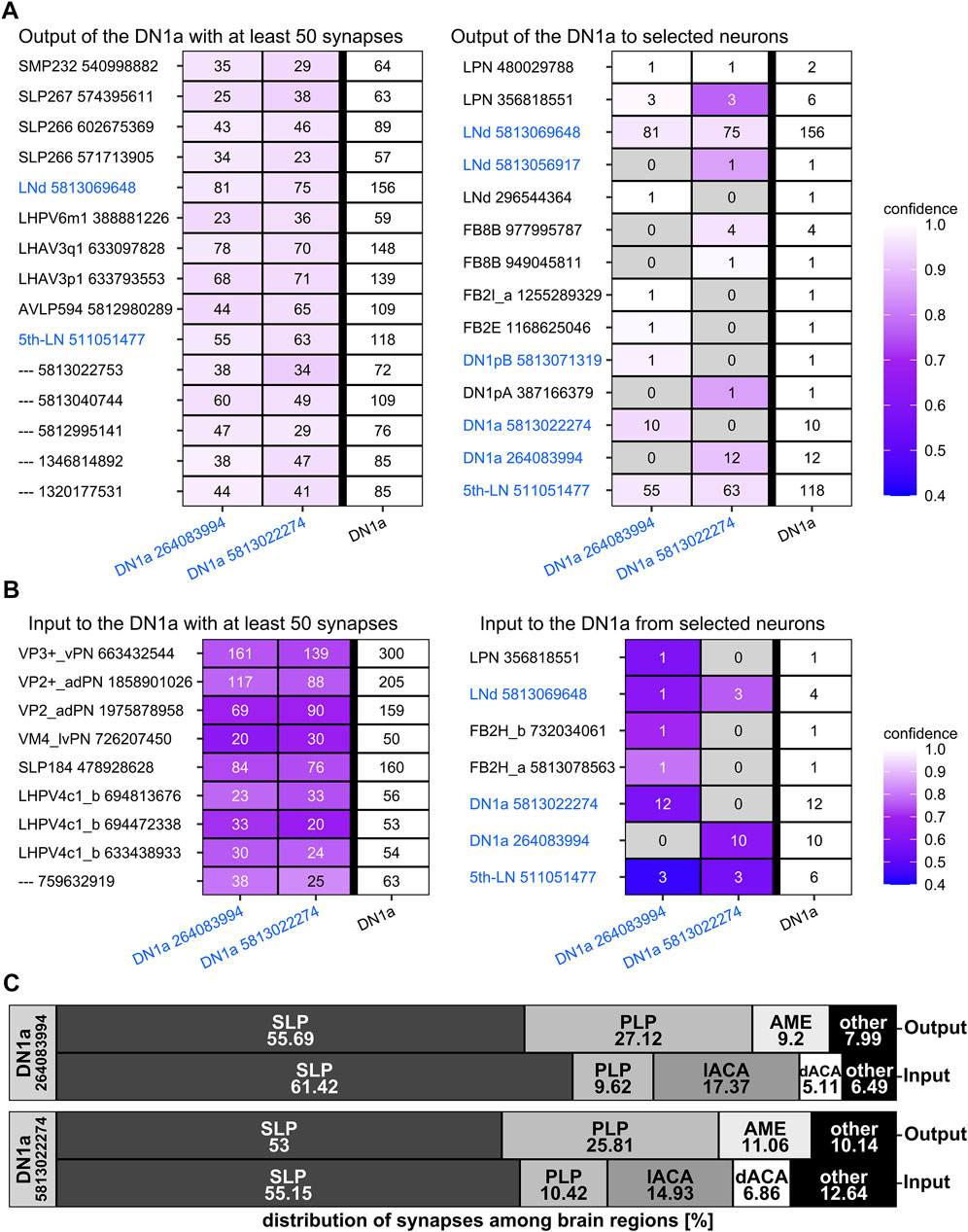
FIGURE 4. Synaptic contacts of the two DN1as revealed from the hemibrain. (A) Postsynaptic partners of the two DN1as with at least 50 synapses (left panel) and output connections to selected partners such as the clock neurons and the fan-shaped body (FB) neurons (right panel). CRY-positive clock neurons are highlighted in blue. The DN1as give strong output to neurons with cell bodies located in the superior lateral protocerebrum (SLP) or the lateral horn (LH). Among these are the ITP positive lateral neurons (LNd and fifth LN). In addition, the two DN1as form synapses with each other, but they have relatively few output synapses to the other clock neurons, including a neuron arborizing in the eighth layer of the FB. (B) Presynaptic partners of the two DN1as with at least 50 synapses and selected presynaptic partners. The DN1as get most input from temperature sensing projection neurons of the antennal lobes (VP3+_vPN, VP2+_adPN, VP2_adPN) and considerable input from neurons with cell bodies in the LH and SLP. Only little input comes from the clock neurons. (C) Synapse distribution of the two DN1as among the brain regions. The DN1as form about 50% of their input and output synapses in the SLP. In the second place are output synapses in the PLP, an area from which they also get input, although to a lower amount. Furthermore, they give output to the AME but do not receive input worth mentioning from there. Instead, they receive considerable input from the lateral and dorsal accessory calyx (lACA, dACA). As can be expected from their similar morphology, the two DN1as largely coincide in synapse distribution and synaptic partners.
As expected from the slightly different morphology of the two DN1as, DN1a #264083994 with the predominantly dendritic fiber in the SLP received slightly more input from neurons located in this brain region. Otherwise, the two DN1as show large similarities in their synaptic connections (Figure 4C) and seem to signal to each other (Figure 4B).
While the main DN1a input and output synapses are formed in the SLP and posterior lateral protocerebrum (PLP), their neurites in the AME appear mainly presynaptic. The DN1as get especially strong input (20% of their input synapses) in the dorsal and lateral accessory calyces that are located adjacent to the SLP (Figure 4C). Most of this input appears to come from temperature sensing neurons in the antennal lobes (VP3+_vPN/VP2+_adPN as well as VP2_adPN, Figure 4B) as was also revealed by Marin et al. (2020).
The DN1ps
The DN1ps are a demonstrably heterogeneous group in respect to their neurochemistry and morphology. About half of the approximately 15 cells per brain hemisphere express CRY and the PDF receptor (PDFR) (Shafer et al., 2008; Yoshii et al., 2008; Im and Taghert, 2010). Five to six of the CRY-positive DN1ps are positive for glutamate (Hamasaka et al., 2007; Guo et al., 2016) and the Diuretic Hormone 31 (DH31, Kunst et al., 2014; Goda et al., 2016) and at least four of these CRY-/DH31-positive neurons express additionally Allatostatin C (AstC, Díaz et al., 2019; Zhang et al., 2021) and CNMamide (Jung et al., 2014; Abruzzi et al., 2017; Jin et al., 2021; Zhang et al., 2021). As recently shown, four AstC-positive DN1ps additionally express the receptors for PDF and DH31 (Goda et al., 2018; Lin et al., 2022). The neurochemistry of the CRY-/PDFR-negative DN1ps is so far unknown.
It is not surprising that the DN1ps differ also in their morphology. The latter has been described in detail in three studies coming from different labs (Chatterjee et al., 2018; Guo et al., 2018; Lamaze et al., 2018). These studies used combinations of different GAL4, LexA, GAL80, and split-GAL4 lines to address different subgroups of the DN1ps and stochastic flip outs to label single neurons. The most important line, which we used also in our study, is the Clk4.1M-GAL4 line. It targets about 10 out of the 15 DN1ps comprising the majority (∼6 out of 7) of the CRY-positive neurons plus ∼4 CRY-negative cells (Figure 2; Zhang Y. et al., 2010, Zhang et al., 2010 L.; Chatterjee et al., 2018). The R18H11-GAL4 line from the FlyLight library collection (Pfeiffer et al., 2008) originates from the gene coding for PDFR and targets only the five to six CRY-/PDFR-positive neurons that are additionally glutamate- and DH31-positive (Kunst et al., 2014; Goda et al., 2016; Guo et al., 2016, 2018; Chatterjee et al., 2018). In contrast, the R18H11-LexA line additionally drives in few CRY-/PDFR-negative DN1ps (Chatterjee et al., 2018; Guo et al., 2018), presumably because PDFR expression is not completely absent in the PDFR-negative characterized neurons, but only largely reduced, so that it is still revealed by strong drivers. Nevertheless, none of the used lines targets all DN1ps, so additional types of DN1ps may exist (especially CRY-negative ones) that have not yet been characterized.
Previous studies have principally identified two main types of DN1ps: One type remaining ipsilaterally and projecting to the anterior optic tubercle (AOTU) (the so-called anterior projecting dorsal neurons), while the other type crosses the midline of the brain through the pars intercerebralis but lacks projections to the AOTU (Chatterjee et al., 2018; Lamaze et al., 2018). According to Chatterjee et al. (2018), the DN1ps projecting to the AOTU express CRY, whereas it is unclear which DN1ps of the other type are CRY-positive.
We analyzed the DN1ps of 22 Clk4.1M-GAL4 brains in combination with the Flybow-reporter to reveal potential differences in the morphology of the DN1ps. As it turned out, the modified Flp-recombinase had not been activated sufficiently, and only four brains showed individually labeled neurons. Nevertheless, we were able to identify three different types of DN1p subgroups. All DN1p cell bodies were located in the dorsal posterior cell body rind innervating the posterior superior medial protocerebrum (SMP) and SLP, as well as the posterior ventromedial LH and dorsal parts of the PLP, where they followed the s-LNv projections in ventral direction, although no fibers reached the AME (Figure 5).
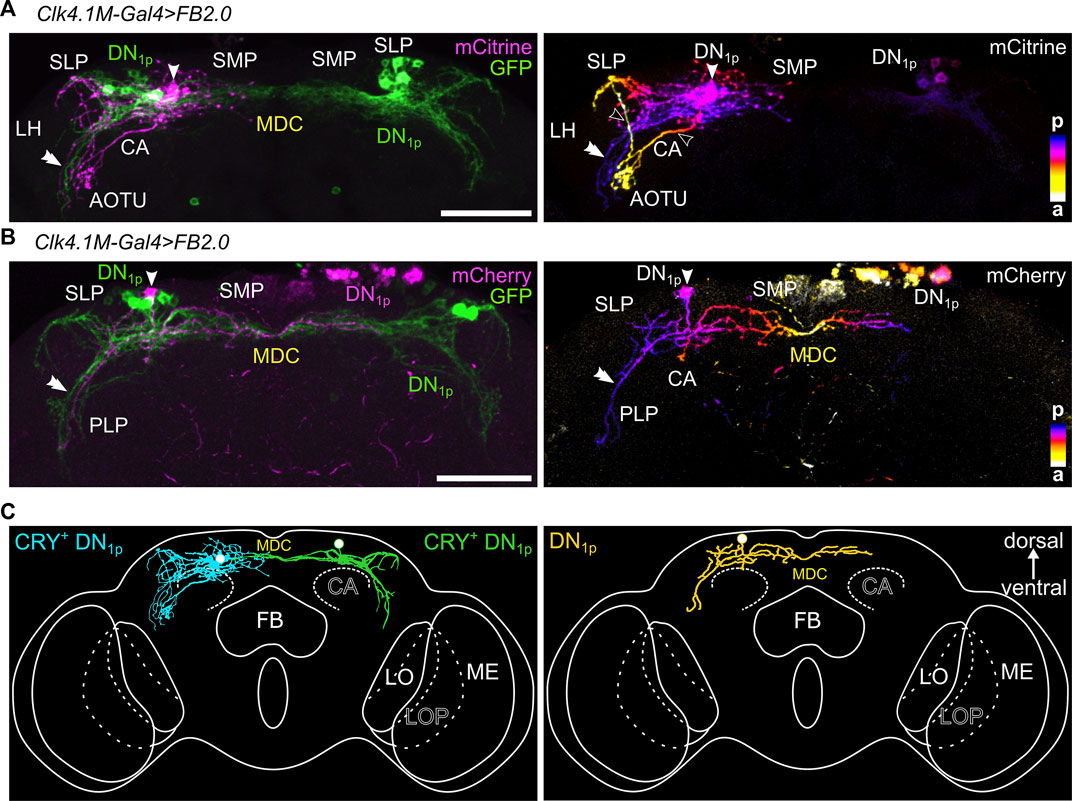
FIGURE 5. Different morphological subtypes within the DN1ps as revealed by Flybow staining. (A,B) Clk4.1M-GAL4 driven Flybow-reporter expression in different DN1ps. The left panels show the original Flybow-reporter expression in which an individually labeled neuron (magenta, arrowhead) expresses mCitrine or mCherry, while several other DN1ps express the default GFP reporter (green). The right panels depict the single DN1ps that are marked in magenta in the left panels with color-coded depth information (anterior (a) in yellow colors, posterior (p) in blue colors as shown in the color code bars in the right bottom of each figure). (A) mCitrine marks a single neuron with arborizations in the superior lateral protocerebrum (SLP). Two fibers (open arrowheads) run anterior to the lateral anterior optic tubercle (AOTU), where they arborize prominently. Another fiber (double arrowhead) remains in the posterior brain and runs ventrally following the s-LNv projections. (B) Single DN1p expressing mCherry (magenta) among four other GFP expressing DN1ps. The single DN1p has a prominent fiber following the path of the s-LNvs in the ipsilateral posterior lateral protocerebrum (PLP) and crosses the dorsoventral midline of the brain through the middle dorsal commissure (MDC). No arborizations in the anterior SLP are visible. (C) Schematic depiction of the three DN1p subtypes identified by Flybow. SMP, superior medial protocerebrum; LH, lateral horn; PLP, posterior lateral protocerebrum; ME, medulla; LO, lobula; LOP, lobula plate; FB, fan-shaped body. Scale bars represent 50 μm.
A single labeled DN1p largely resembled the previously described CRY-positive neurons that invade the AOTU and form pronounced varicosities in this region (Figure 5A, called a-DN1p in Lamaze et al., 2018). The fibers of this neuron formed two loops running from posterior to anterior and terminated in the AOTU (open arrowheads). One loop ran around the SLP and the second one ventrally on the surface of the superior clamp (SCL) and the inferior clamp until both converged shortly before the AOTU. In addition, fibers from this neuron remained posterior and followed the s-LNv projections in the PLP (double arrowhead) for a short distance toward the AME. Most significantly, all neurites of this neuron remained ipsilaterally and did not cross the midline of the brain in the middle dorsal commissure (MDC). A similar neuron is described in Figures 1C,D of Lamaze et al. (2018) and called anteriorly projecting DN1p (a-DN1p).
The other labeled DN1ps appeared to belong to the second type of DN1ps described by Lamaze et al. (2018), Chatterjee et al. (2018), and Guo et al. (2018). These neurons were called vc-DN1p_neurons by Lamaze et al. (2018) and sent fibers through the MDC to the contralateral SMP and on the ipsilateral side, their projections in the PLP appeared to reach slightly further toward the AME than that of the first DN1p type (Figure 5B). We could identify two different subtypes with this projection pattern. One neuron of the first subtype was individually labeled (Figure 5B right and yellow neuron in Figure 5C), whereas the presence of the second subtype (Figure 5C green) was concluded from the samples with varying numbers of visible cells. Fibers of the second subtype started to form a loop around the SLP as was typical for the two CRY-positive DN1ps described above, but they did not complete this loop and did not reach the AOTU (green neuron in Figure 5C). A very similar neuron (called vc-DN1p) is described in Figure 1C of Lamaze et al. (2018). Furthermore, similar DN1ps were found in Jin et al. (2021; Figure 5E in Jin et al.) and Zhang et al. (2021; Figure 3C in Zhang et al.) among the CNMamide-positive neurons. Since the latter are CRY-positive, we assume that this type of DN1p is CRY-positive. In case of the yellow colored neuron in Figure 5C, we do not know about its CRY expression. Therefore, no statement about CRY is made. The same applies for all further statements regarding the DN1ps.
Seven DN1ps are annotated in the hemibrain, which were again divided into two types (called A and B in the hemibrain) (Figures 6–8 and Supplementary Video S2). The two type B neurons (cyan in Figure 6) resemble CRY-positive neurons described above, which remain ipsilateral and project into the AOTU. The five type A neurons all project contralaterally and do not enter the AOTU (green and magenta in Figure 6). Among these, the DN1p depicted in green closely resembles the just described CRY-/CNMamide-positive DN1ps that send fibers into the anterior SLP and follow the s-LNv projections in the PLP several micrometers (green in Figure 6). The other four type A neurons do not show any fibers following the s-LNv projections in the PLP, while the fibers extending toward the anterior SLP were generally present (magenta in Figure 6). The DN1p neuron type that is not annotated in the hemibrain is depicted in Figure 5C (yellow). This neuron completely lacks the anterior running fibers but shows prominent neurites following the s-LNv projections in the PLP. A similar neuron is described by Lamaze et al. (2018; vc-DN1p neuron in Figure 1D) making us confident that this is a true subtype.
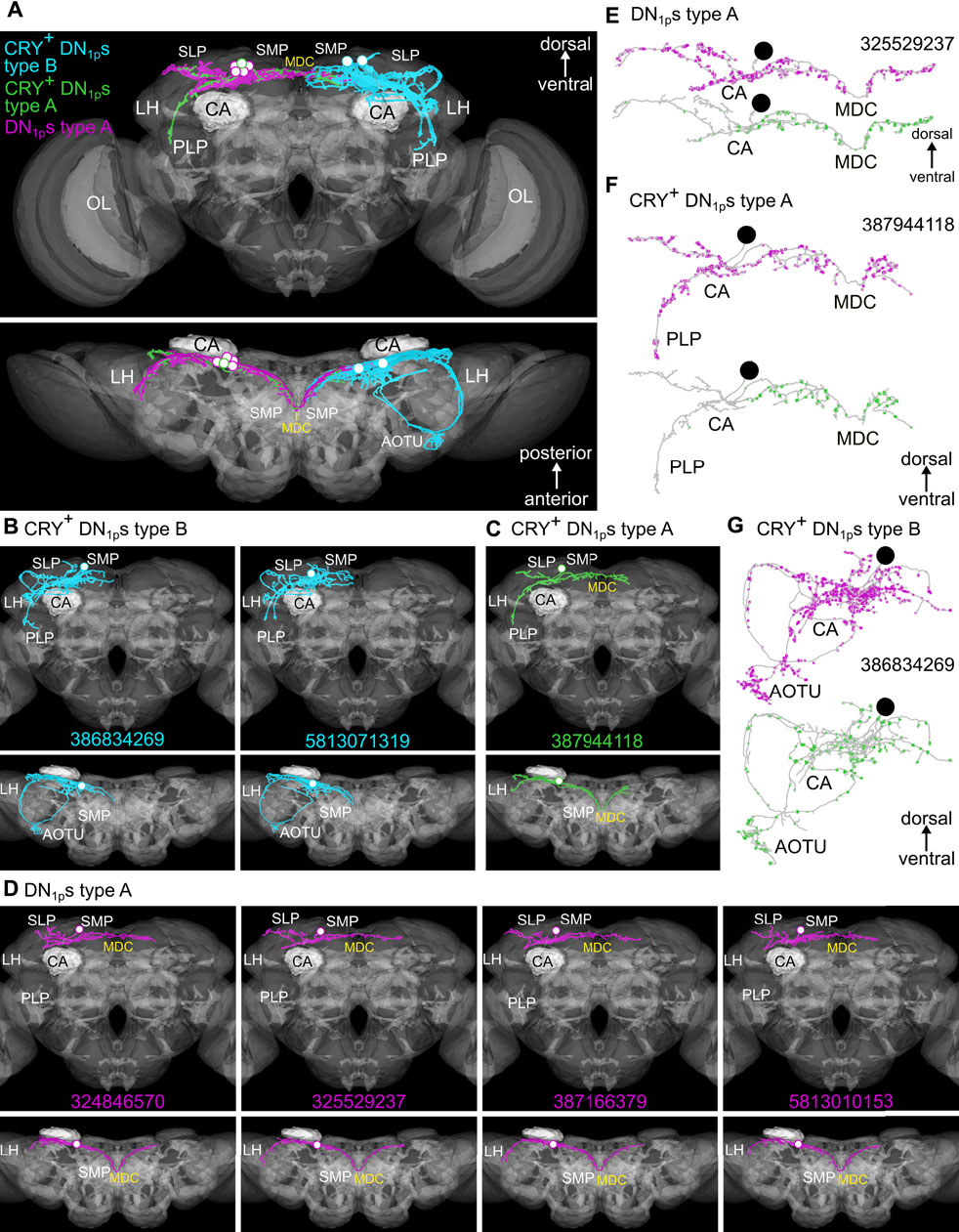
FIGURE 6. The different morphologies of the seven DN1ps annotated in the hemibrain. (A) Standard brain (JRC 2018F) showing the three different morphological subtypes among the seven DN1ps annotated in the hemibrain. The presumably CRY-positive neurons are depicted in cyan and green, while the still undefined DN1ps are shown in magenta. (B) Detailed representation of the two Type B CRY-positive DN1ps in ventral-dorsal (top) and anterior-posterior (bottom) orientation. Both neurons arborize in the superior lateral protocerebrum (SLP), the superior medial protocerebrum (SMP), and project ventrally to the posterior lateral protocerebrum (PLP). Additionally, they send two fibers to the anterior optic tubercle (AOTU) each of them forming a loop that runs on the dorsal and ventral surface of the SLP, respectively (compare with G). (C) Representation of the type A CRY-positive DN1p (#387944118). The projections of this neuron remain mostly posterior. In the SLP, this DN1p also sends one fiber toward the AOTU but does not reach it. In further contrast to the two Type B CRY-positive DN1ps neurons, it projects via the middle dorsal commissure (MDC) to the contralateral superior medial protocerebrum (SMP). (D) Representation of four type A DN1ps with still undefined nature. These neurons are morphologically similar to the type A CRY-positive DN1p and cross the midline in the MDC, but they remain completely in the SLP and SMP and do not send fibers to the PLP. (E–G) postsynaptic (input, magenta) and presynaptic (output, green) sites of the three different DN1p subtypes. (E,F) Type A DN1ps receive input all over their neurites but restrict output to the ipsilateral and contralateral SMP. (G) Type B DN1ps receive input and give output all over their neurites. CA, mushroom body calyx; LH, lateral horn; OL, optic lobes.
Synapses and Putative Synaptic Partners of the DN1ps
Previous studies used diverse GAL4 lines to perform syt-GFP (Guo et al., 2016, 2018; Lamaze et al., 2018) and DenMark staining (Chatterjee et al., 2018; Guo et al., 2018; Lamaze et al., 2018) and revealed putative presynaptic output sites of the DN1ps in the SMP close to the pars intercerebralis and in the AOTU, to which the CRY-positive DN1ps project (Guo et al., 2018; Lamaze et al., 2018). Chatterjee et al. (2018) found that the characteristic anterior projecting loops of the CRY-positive DN1ps around the SLP are not only presynaptic but also dendritic.
We used the connectome data of the hemibrain (Scheffer et al., 2020) to reveal the input and output sites of the DN1ps and could confirm the results of Chatterjee et al. (2018) and specify the results of the other studies. While the input and output sites of the CRY-positive DN1ps (type B neurons) are equally distributed across the entire surface of the neurons, the type A DN1ps show output sites mainly in the SMP, while they receive input along the whole neuron (Figures 6E–G, Figure 8, Figure 9). This is true for all type A neurons independent of the subtype (Figure 8).
Trans-Tango staining by Guo et al. (2018) revealed some LNds and tubercle bulb neurons as possible postsynaptic partners of the DN1ps, as well as weak signals in some DN3s. Here, we used again the connectivity data from the hemibrain (Scheffer et al., 2020) to investigate the pre-and postsynaptic partners of the DN1ps in more detail. Since we found a strong difference between the CRY-positive type B and the more heterogenous type A DN1ps, we analyzed the two groups separately (Figures 7–9). The type A DN1ps show mainly output to CRY-positive clock neurons with the strongest synaptic output to the ITP and CRY-positive LNd and fifth LN (Figure 7A). Other clock neurons are only contacted with very few synapses and likewise the output to non-clock neurons is generally weak. The connection to the ITP and CRY-positive LNd and the fifth LN appears reciprocal since type A DN1ps also receive strong input from these two neurons (Figure 7B). Otherwise, the type A DN1ps get little input from the clock network (Figure 7B).
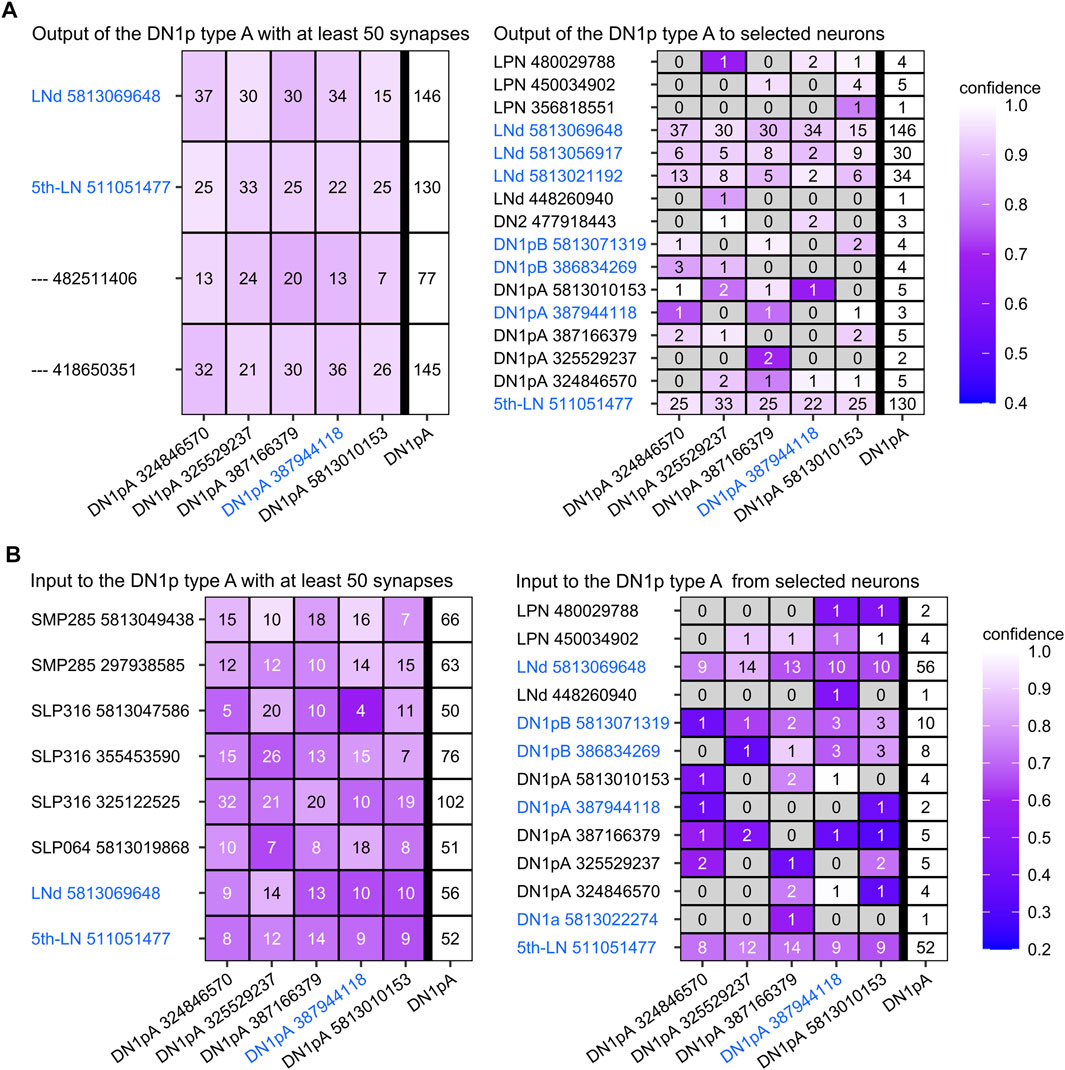
FIGURE 7. Synaptic partners of the five annotated Type A DN1ps from the hemibrain. (A) Postsynaptic partners of the Type A DN1ps with at least 50 synapses (left panel) and clock neurons as postsynaptic partners (right panel). CRY-positive clock neurons are in blue letters. The Type A DN1ps have relatively few postsynaptic partners with more than 50 synapses. Most interestingly the ITP positive lateral neurons (LNd and fifth LN) are among these few neurons. More generally, the type A DN1ps are signaling to all three CRY-positive LNds although the synaptic strength of the connections is weaker for the ITP negative ones. Only very few output synapses are established with the other clock neurons. (B) Presynaptic partners of the type A DN1ps with at least 50 synapses (left panel) and selected clock neurons as presynaptic partners (right panel). The type A DN1ps receive most input from neurons with their cell body located in the superior lateral protocerebrum (SLP) as well as in the superior medial protocerebrum (SMP). Among these neurons are again the ITP positive lateral neurons (LNd and fifth LN). Input from other clock neurons is minor, just two of the type A DN1ps get weak input from the CRY-positive DN1ps. The only for sure CRY-positive Type A DN1p (#387944118, green in Figure 6) is among these two neurons.
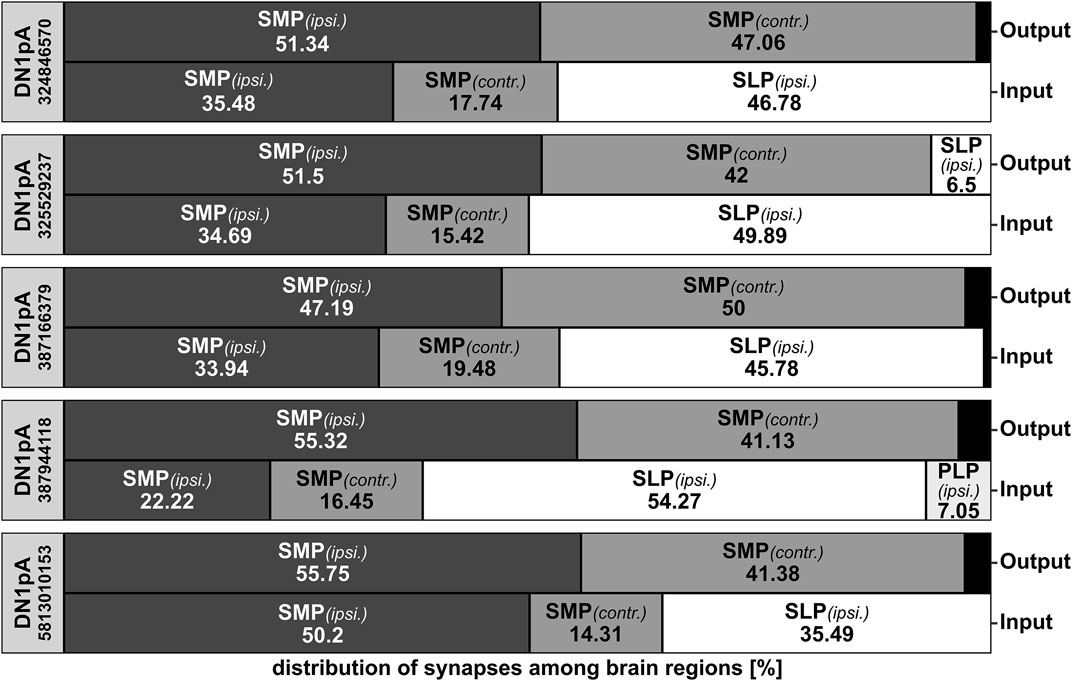
FIGURE 8. Distribution of the synapses of the five annotated hemibrain type A DN1ps among the brain regions. The type A DN1ps restrict their output mainly to the ipsilateral (ipsi.) and contralateral (contr.) superior medial protocerebrum (SMP) while they get about 50% of their input additionally from the ipsilateral superior lateral protocerebrum (SLP). The CRY-positive DN1p of type A (#387944118, green in Figure 6) that sends fibers to the posterior lateral protocerebrum (PLP) is the only neuron that receives input from there. In addition, this neuron receives most input from the SLP, to which it sends a dendritic fiber following the loop around the SLP toward the AOTU.
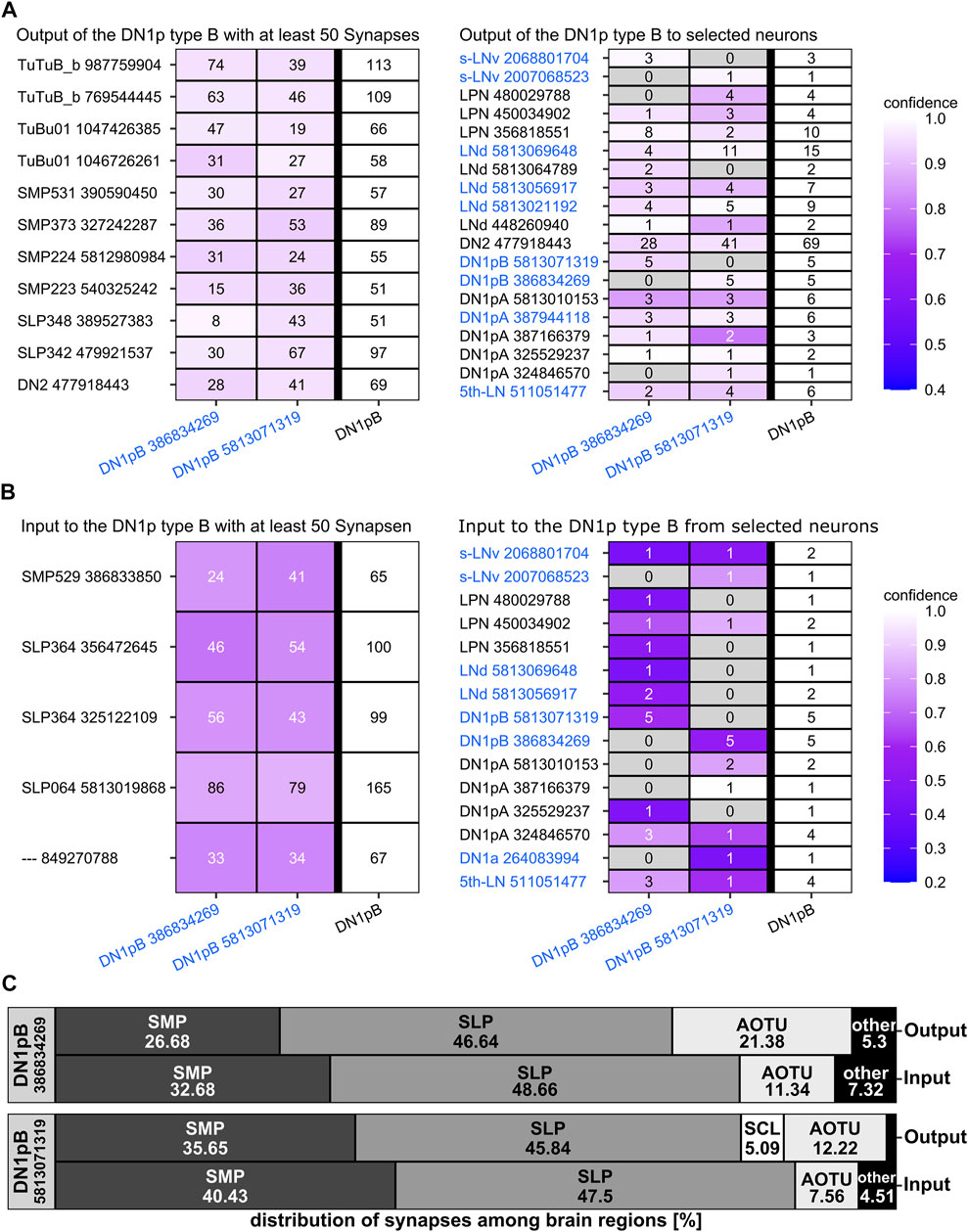
FIGURE 9. Synaptic partners of the two annotated CRY-positive type B DN1ps from the hemibrain. (A) Postsynaptic partners of the CRY-positive type B DN1ps with at least 50 synapses and postsynaptic clock neurons. As expected, the DN1ps project on many tubercle bulb neurons (TuBu) of the anterior optic tubercle (AOTU) and to tubercle-tubercle neurons (TuTuB) which are connecting the AOTUs of both hemispheres. Additionally, neurons with their cell bodies in the superior lateral protocerebrum (SLP) as well as in the superior medial protocerebrum (SMP) get strong input by the CRY-positive type B DN1ps. Further, they give strong input to one DN2 as the only clock neuron which is getting strong input from the type B CRY-positive DN1ps. Other clock neurons like the LPN and the type A DN1ps get only weak input from the CRY-positive DN1ps. (B) As the CRY-positive DN1ps give output to neurons of the SMP and SLP they also do get strong input by neurons from these brain regions. Interestingly they do not seem to receive synaptic input from the clock network. (C) Distribution of the input and output synapses of the CRY-positive DN1ps among the brain regions. The neurons form most of their synapses in the SMP, AOTU, and SLP with most synapses in the latter. The number of input and output synapses are similar in the different brain regions, but the two DN1ps slightly differ in the percentage of output synapses in the SMP and AOTU. DN1p #5813071319 has more input and output in the SMP and less input and output in the AOTU than DN1p #386834269. In addition, DN1p #5813071319 is the only so far annotated DN1p that has output in the superior clamp (SCL). Note that the two Type B DN1ps do not receive significant input from the PLP, although they send fibers into this region.
As already shown by Guo et al. (2018), the CRY-positive type B DN1ps signal to tubercle bulb neurons as well as to tubercle-tubercle neurons which connect both AOTUs (Figure 9A). Additionally, we found that they signal strongly to at least one DN2 (Figure 9A), whereas their synaptic contact with other clock neurons is rather weak. Their weak contact within the clock network also concerns the ITP- and CRY-positive LNd and fifth LN, which have strong reciprocal synaptic connections with the Type A DN1ps (Figure 9A). There is also very little synaptic input from the clock network to the CRY-positive type B DN1ps (Figure 9B).
The DN2s
The DN2s consist of only two neurons that have been described for the first time 25 years ago (Kaneko et al., 1997). Their importance in the clock network is highlighted by their early appearance during larval development, together with the s-LNvs, the fifth LN, and the DN1as, but their function and fine anatomy are yet unclear. The somata of the DN2s are located near the dorsal terminals of the s-LNvs and do not seem to express CRY (Benito et al., 2008; Yoshii et al., 2008). The arborizations of both DN2s appear to remain in the dorsal protocerebrum and cross the midline of the brain through the pars intercerebralis, to terminate close to the cell bodies of their contralateral counterparts (Helfrich-Förster, 2005). However, due to the wide expression of the clock drivers available (i.e. Clk856-GAL4, Gummadova et al., 2009) and the vast overlap of the fibers of other clock neurons in the SLP, it was impossible to finely trace the neurites of the DN2s so far.
We used the Clk206-GAL4 driver in combination with cry-GAL80 to restrict the expression of Flybow exclusively to the CRY-negative clock neurons, which comprise the DN2s. Unfortunately, GAL80 was not sufficient to repress GAL4 expression in the CRY-positive s-LNvs and DN1as, except for the fifth LN. Consequently, none of the 17 brains analyzed contained individually labeled DN2s. Nonetheless, since all additionally marked neurons have been described at single-cell resolution in our previous study (Schubert et al., 2018), we were able to specifically assign certain projections to known clock neuronal morphologies and therefore to characterize the unique pattern of the DN2s. We found two main projections of the DN2s - one toward the medial brain passing to the contralateral hemisphere, the other projection running laterally. The latter runs dorsolaterally around the SLP and invades the anterior-lateral part of the AOTU. The loop around the SLP reminds strongly of the projections of the CRY-positive DN1ps described above. Since the staining with the Clk206-GAL4;cry-GAL80 line was only partly satisfying, we looked for split-Gal4 lines that label specifically the DN2s.
Indeed, the just described DN2 projection pattern was confirmed by using a split-GAL4 line that specifically labels one DN2 and none of the other clock neurons (R43D03-AD; VT003234; Sekiguchi et al., 2020, Supplementary Video S3) and by the Clk9M-GAL4 line combined with Pdf-GAL80, which has been reported to label one or both DN2 (Kaneko et al., 2012; Goda et al., 2016). The new split-GAL4 DN2 driver was considerably weaker than the Clk206-GAL4 and the Clk9M-GAL4 driver, but we got satisfying labeling with a cytosolic GFP reporter that accumulates GFP stronger than the membrane-bound GFP does (Supplementary Table S1). In the case of Clk9M-GAL4; Pdf-GAL80, membrane-bound GFP was sufficient for labeling the DN2s. As expected, the novel split-GAL4 line marked only one DN2 and this cell was always CRY-negative (Figure 10A). With the Clk9M-GAL4; Pdf-GAL80, we could see two DN2s in only one brain (out of 16, Figure 10B). In all other brains, again only one DN2 was labeled, and in about half of the cases, the labeled DN2 was weakly CRY-positive (Figure 10B). This suggests that the two DN2s can be distinguished by their CRY expression level, and that Clk9M-GAL4 drives GFP either in the CRY-negative or in a weakly CRY-positive DN2. Nevertheless, we did not see any difference in morphology between the different individually labeled cells, suggesting that the two DN2s are very similar. In the following, we will describe the typical morphology of the DN2s in more detail.
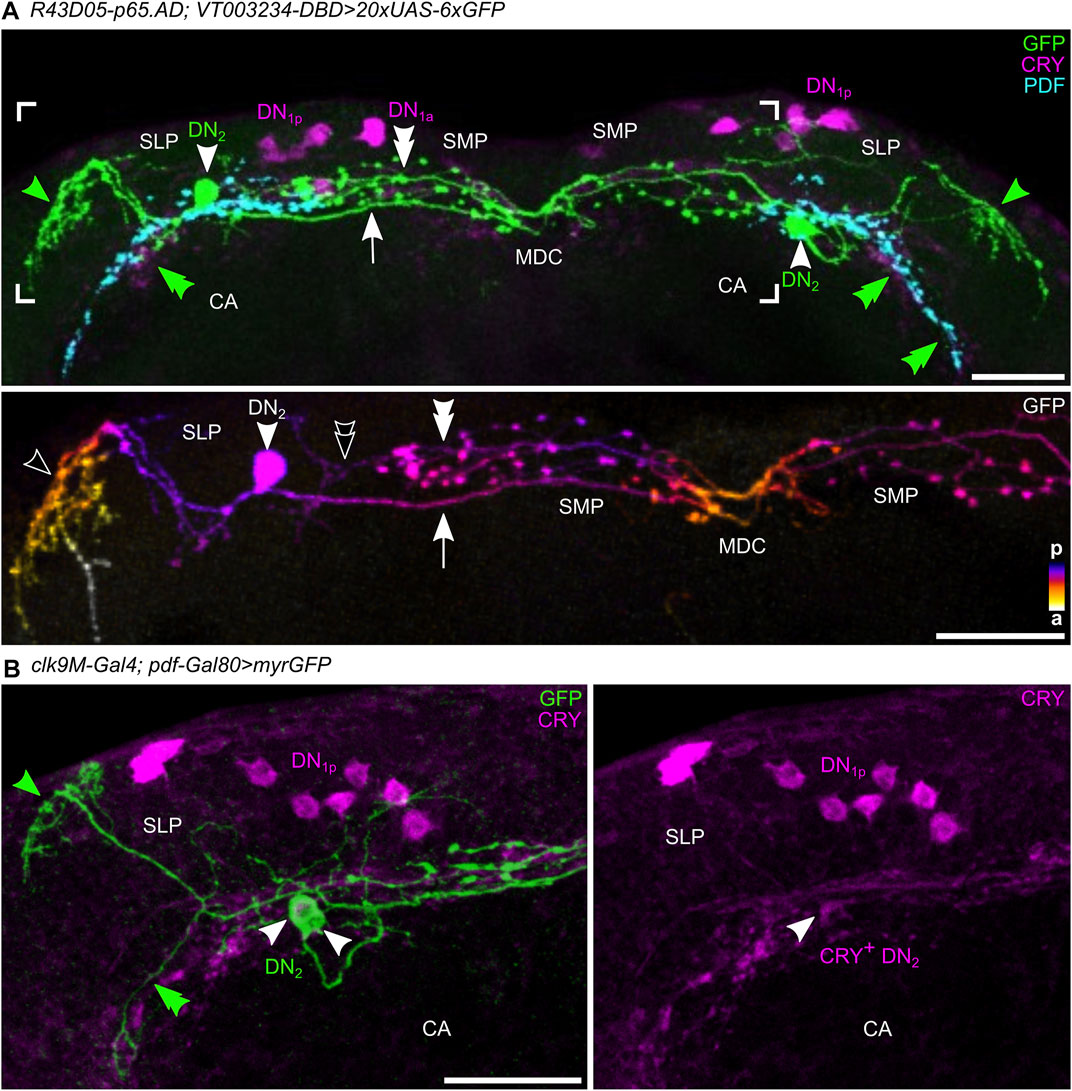
FIGURE 10. The morphology of the DN2s. (A) Maximal projection of 20 confocal planes showing a frontal view of the dorsal protocerebrum, in which one DN2 is marked with cytosolic GFP using the split-GAL4 line R43D03-AD; VT003234 with the 20xUAS-6xGFP reporter) and counter-stained with anti-CRY (magenta) and anti-PDF (cyan). The lower panel shows the GFP labeling of the area marked above in greater detail in color-coded depth information. Fibers in the posterior (p) brain are marked blue and fibers further anterior (a) are marked in yellow/white. (B) Maximal projection of 26 confocal planes showing a frontal view of the left dorsolateral protocerebrum, in which two DN2s are marked with membrane-bound GFP (using Clk9M-GAL4; Pdf-GAL80 with the UAS-myrGFP reporter) and counter-stained with anti-CRY (magenta). Note that one of the two DN2s is weakly CRY-positive (CRY+ DN2, right). Green arrowheads mark the DN2 fibers projecting to the anterior optic tubercle (AOTU), green double arrowheads mark fibers that follow the PDF terminals for several micrometers ventrally, green arrows point to the DN2 fiber running contralaterally, and thick green arrows to the varicose terminals on the contralateral side. From these, in some cases, weak, nonvaricose fibers continue toward the contralateral soma of DN2. MDC, middle dorsal commissure; CA calyx of the mushroom bodies. Scale bars represent 50 µm.
Shortly after leaving the soma, the DN2 neurites bifurcate into two main branches. As found by Flybow, one runs to the contralateral brain hemisphere (arrow in Figure 10A), the other runs laterally (green arrowhead). The laterally running branch projects into the dorsal SLP and then runs further anteriorly forming the characteristic loop around the SLP toward the AOTU, but the DN2 fibers do not reach it (Figures 10–12). Often this branch splits into two prominent fibers (Figure 11A). Before the split-off of the lateral branch(es), some less intensively stained fibers turn ventrally and follow the PDF-positive s-LNv terminals for several micrometers (Figure 10, green double arrowheads). Generally, the ipsilateral remaining DN2 fibers were in close vicinity to the s-LNv terminals, suggesting an interaction between the two clock neuron types (see also below). In one case (out of 15 brains), fibers of the s-LNv terminals even followed the dorsolaterally projecting DN2 fibers (magenta arrowhead in Figure 11A). The contralaterally projecting fibers of the DN2s showed pronounced varicosities and terminated in the superior protocerebrum slightly before they reached the contralateral DN2 somata (white double arrowheads in Figures 10A, 11A). Sometimes we saw fine non-varicose fibers extending from the varicose fibers further laterally (open double arrowhead, Figure 10A).
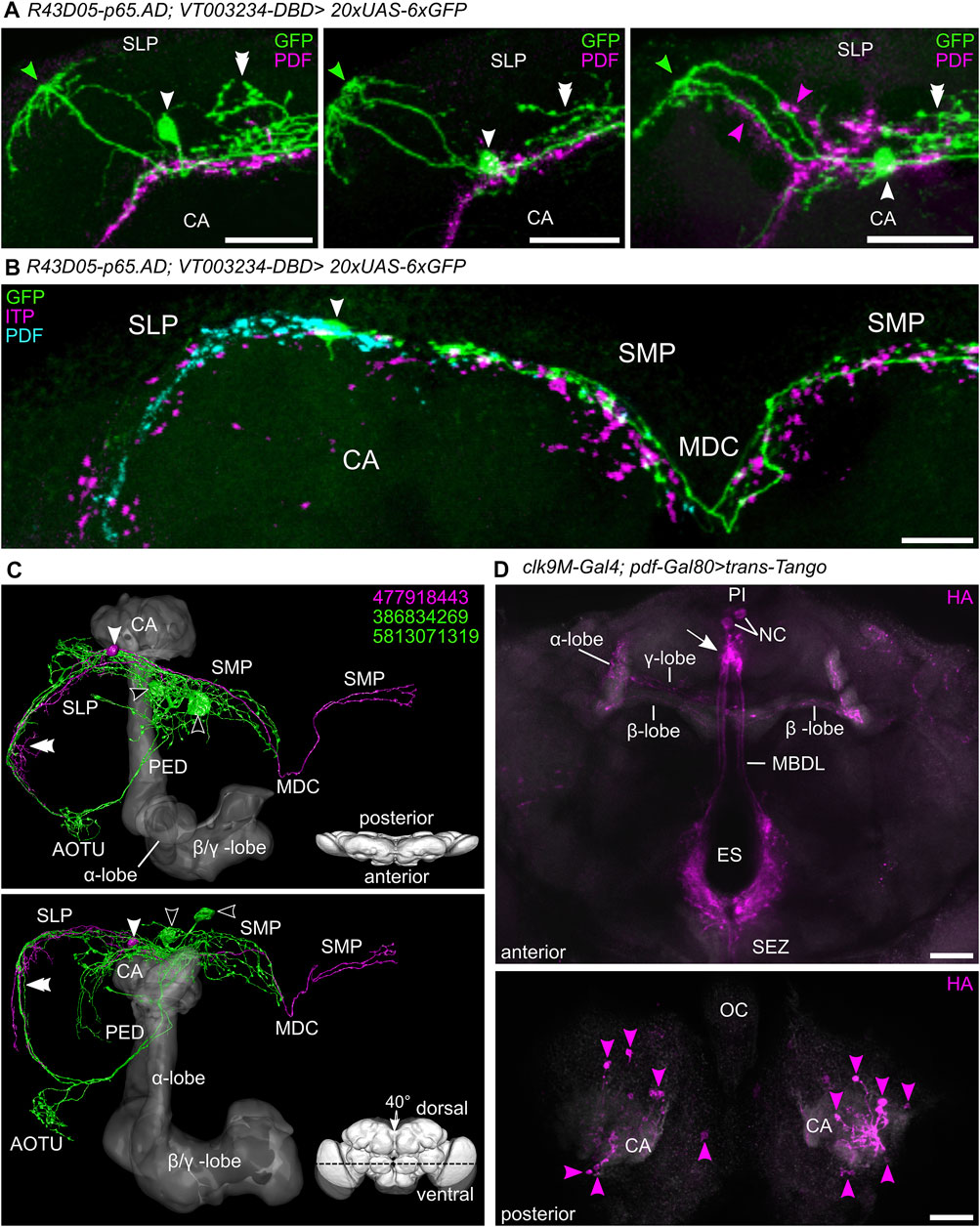
FIGURE 11. Vicinity of the DN2s to other clock neurons and putative downstream synaptic partners. (A) Confocal images (overlay of 27, 43, and 21 stacks, respectively) demonstrating the close vicinity of the DN2s (green) to the PDF-positive terminals of the s-LNvs (magenta). Labeling as in Figure 9. (B) Maximal projection of 18 confocal planes showing the close vicinity of the DN2s (green) to the ITP-positive LNd and fifth LN (magenta) and the PDF-positive terminals of the s-LNvs (cyan). White arrows mark the overlap of ITP-positive fibers with the contralateral projecting fibers of the DN2s in the superior median protocerebrum (SMP) and the middle dorsal commissure (MDC). (C) Overlay between DN2 and CRY-positive DN1p fibers running around the SLP to the anterior optic tubercle (AOTU). (D) Neurons postsynaptic to the DN2s revealed by trans-Tango. Top: maximal projection of eight confocal planes from the anterior brain depicting postsynaptic HA staining in neurosecretory cells (NC) running in the median bundle (MBDL) and around the esophagus (ES) to the subesophageal zone (SEZ) as well as in the α- and β-lobes of the mushroom bodies. The white arrow points to a dense HA-positive fiber net that lies exactly in the midline of the brain where the DN2s cross in the MDC (compare with B). Bottom: maximal projection of four confocal planes from the posterior brain showing postsynaptic staining in Kenyon cells (small magenta arrows) of the mushroom body as well as in the calyces (CA). OC, ocellar tract. Scale bars represent 25 µm.
The arborizations of the DN2s did not only largely overlap with the PDF-positive fibers of the s-LNvs but also with those of the ITP-positive fibers from the fifth LN and LNd (Figure 11B). This was especially true in the SMP to SLP, in which the arborizations of the ipsilateral DN2s were less varicose than those running to the contralateral hemisphere. As for ITP-positive clock neurons, the close vicinity to the DN2s was maintained throughout the SMP where both clock neuron types cross the midline of the brain in the MDC (Figure 11B).
Synapses and Putative Synaptic Partners of the DN2sSo far, the neuromessengers used by the DN2s are unknown. The prominent varicosities in the contralaterally projecting terminals of the DN2s suggest modulatory neuropeptides that are released via volume transmission, but the DN2s may additionally utilize fast neurotransmitters operating via synapses. Single-cell transcriptome data (Ma et al., 2021) proposed glutamate as a neurotransmitter of the DN2s, but glutamate was not detected in the DN2s by immunohistochemistry (Hamasaka et al., 2007). Our polarity assessment by Synaptobrevin::EGFP and Denmark shows that the DN2s are strongly polarized (Figures 12B,C,E). They receive input in the ipsilateral brain hemisphere and have output synapses at the contralateral hemisphere. A very similar distribution of synapses was also found in neuron #477918443 of the hemibrain (Figures 12E, 13B). This neuron morphologically largely resembles the two DN2s (Figure 12D and Supplementary Video S3). As revealed by our polarity staining most input synapses are found on the DN2 fibers projecting anteriorly around the SLP toward the AOTU. These DN2 fibers largely intertwine with presynaptic fibers from the CRY-positive DN1ps (Figure 11C, double arrowhead), suggesting that the latter signal to the DN2s, an assumption that is strongly supported by the high number of output synapses from the CRY-positive DN1ps to the DN2s (Figures 9A, 13A). The only other clock neurons giving input to the DN2s are the s-LNvs (Figure 13A). This supports the findings of Tang et al. (2017) who could find putative synaptic contact between the s-LNvs and the DN2s using GFP Reconstitution Across Synaptic Partner (GRASP) (Tang et al., 2017).
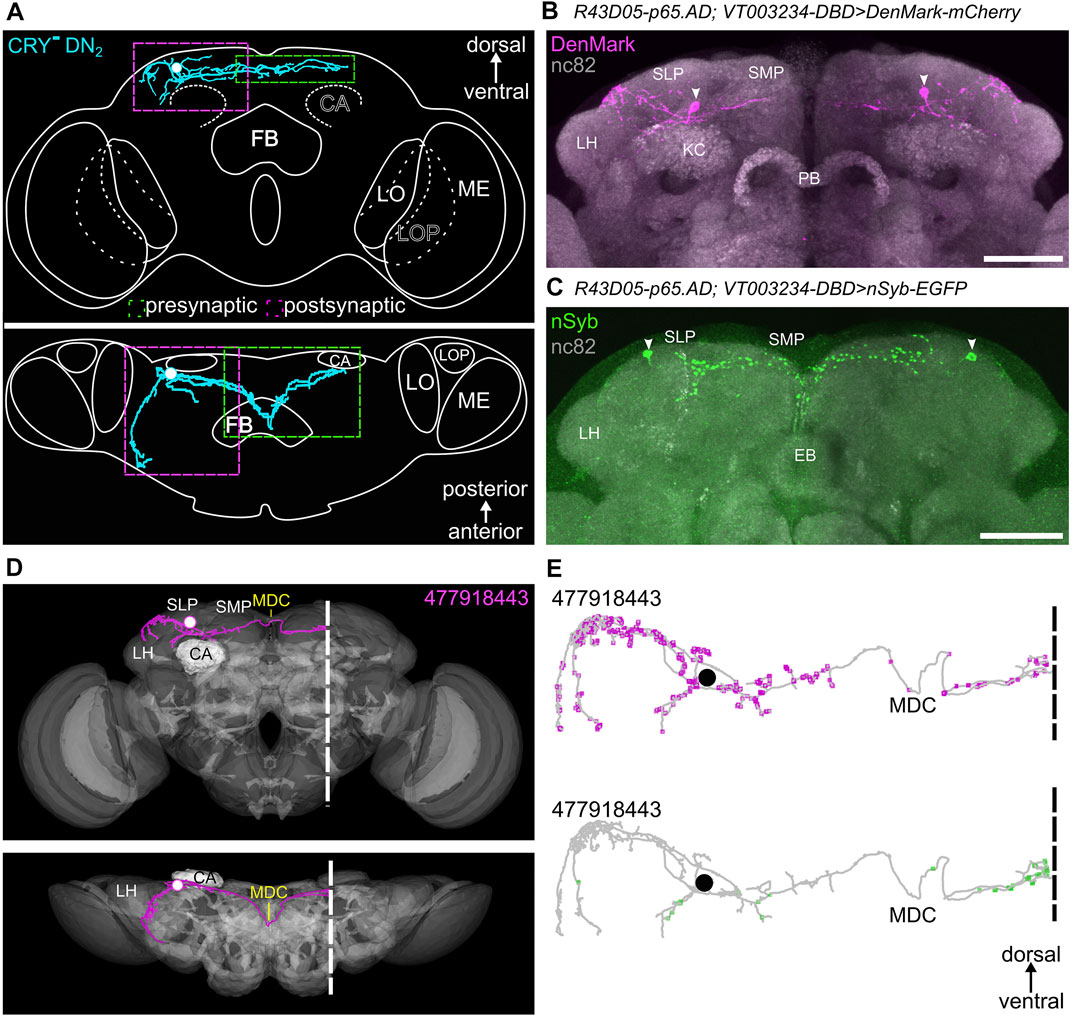
FIGURE 12. Morphology of DN2 #477918443 found in the hemibrain and its input and output sites. (A) Schematic summary of the morphology and the input (magenta) and output (green) sites of DN2 #477918443. (B) Staining against mCherry the fluorescent marker of DenMark which shows the dendritic sites of the DN2 addressed by the split-GAL4 line. While the dendritic signal is strong in the arborizations branching in the superior lateral protocerebrum (SLP) and the fibers running anterior at the border between the SLP and the lateral horn (LH), no or only weak signal could be recognized in the superior medial protocerebrum (SMP). (C) Staining against GFP to reveal the axonal endings marked by the enhanced GFP-(EGFP) tagged neuronal Synaptobrevin (nSyb). In contrast to the DenMark staining, the signal is mostly restricted to the SMP and only some or no signal could be recognized in the lateral brain. (D) Reconstruction of DN2 #477918443 on electron microscopic level found in the hemibrain. The DN2 shows only a few arborizations in the ipsilateral SMP but sends fibers through the middle dorsal commissure (MDC) to the contralateral SMP. Additionally, fibers are running and branching further on the ipsilateral border of SLP and LH anterior in the direction of the anterior optic tubercle (AOTU) without reaching it. The white dashed line shows the border of the hemibrain. (E) Postsynaptic (magenta, input) and presynaptic (green, output) sites of the DN2. The input sites of the DN2 are mainly restricted to the SLP as shown in the DenMark staining, while the output sites are largely absent since they are located in the contralateral SMP. ME, medulla; LO, lobula; LOP, lobula plate; FB, fan-shaped body; EB, ellipsoid body; CA, mushroom body calyx; PB, protocerebral bridge. Scale bars represent 50 μm.
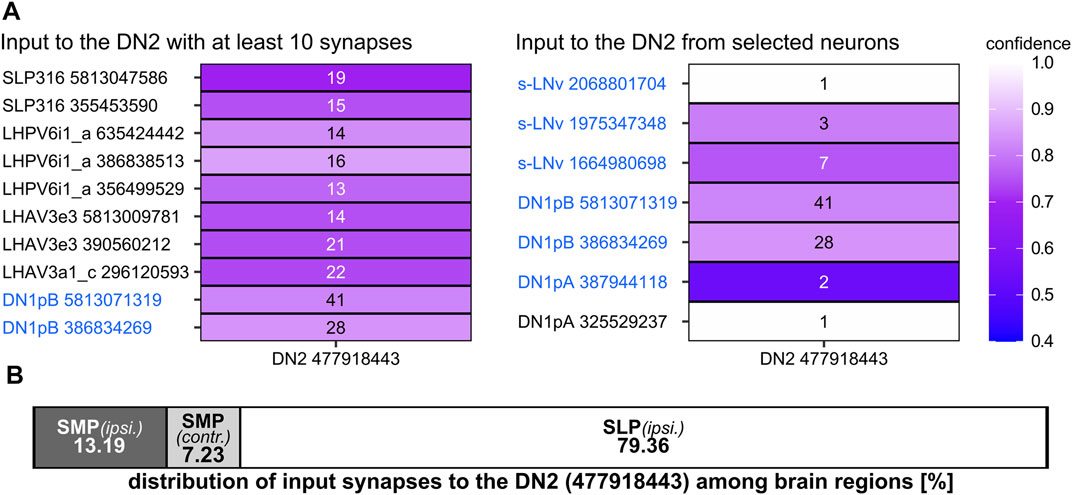
FIGURE 13. Presynaptic partners of DN2 #477918443 and the distribution of postsynaptic synapses among the brain regions. (A) Presynaptic partners of the DN2 with at least 10 synapses and selected clock neurons as presynaptic partners. The DN2 gets input from neurons with their cell bodies located in the superior lateral protocerebrum (SLP) as well as in the lateral horn (LH). Additionally, the DN2 gets input from the CRY-positive DN1ps as already visible in the postsynaptic partners of the CRY-positive DN1ps. Further, it gets weak input from some s-LNvs. (B) Distribution of the DN2 postsynaptic synapses (input) among the brain regions. The DN2 gets around 80% of the input in the ipsilateral SLP and only around 20% of the input in the ipsilateral (ipsi.) or contralateral (contr.) SMP.
Trans-Tango staining with the split-GAL4 and GAL4/GAL80 DN2 lines turned out to be more equivocal than for the other clock neurons. This was the case because the R43D03-AD; VT003234 split-GAL4 line was such a weak driver that we could not detect the DN2s in the presynaptic GFP channel, making it therefore difficult to trust the postsynaptic staining. The Clk9M-GAL4; Pdf-GAL80 line, on the other hand, showed sometimes expression in the DN1as that made us doubt that we can rely on all postsynaptic labeling we saw. Nevertheless, both drivers led consistently to HA-expression in Kenyon cells of the mushroom bodies and all their lobes (Figure 11D). Since the DN1as do not signal onto the mushroom bodies and the DN2s neurites project close to the calyces of the mushroom bodies, it is quite likely that they indeed form synapses with specific Kenyon cells. In addition, in the stronger Clk9M-GAL4; Pdf-GAL80 line, we found always postsynaptic labeling in the pars intercerebralis. In several brains, few neurosecretory cells of the pars intercerebralis were labeled, projecting through the median bundle down to the esophageal foramen and the subesophageal zone (Figure 11D). Since the neurites of the DN2s cross the midline of the brain at the exact location where we see dense HA labeling (arrow in Figure 11D), this staining pattern is likely true and the DN2s do indeed form synapses with neurosecretory cells of the pars intercerebralis.
It is also important to note that we did not see any postsynaptic staining in any of the clock neurons, suggesting that the DN2s might not form any output synapses with other clock neurons. In the hemibrain, no output synapses of the DN2 (#477918443) were annotated, because the latter are found on the contralateral hemisphere. Input to the DN2s came mainly from the ipsilateral SLP and here mainly from the CRY-positive DN1ps (Figure 13).
The DN3s
Comprising approximately 40 cells, the DN3s are by far the largest clock neuron cluster in the brain of the adult fly. Several studies have already revealed the presence of larger and smaller DN3 cell bodies, and that some of them are situated more anteriorly in the brain compared to the others (Helfrich-Förster, 2003; Shafer et al., 2006; Helfrich-Förster et al., 2007b). This is in line with our observations made on 43 brains, in which the Flybow-reporters were expressed under the control of the pan-clock-neuronal Clk856-GAL4 driver. The somata of approximately 15 DN3s are located posterior to the LH, whereas the remaining cells reside more anteriorly in the dorsal and lateral cell body rind of the LH, respectively. The majority of DN3s possess rather small cell bodies and only about four to five neurons have larger somata. Half of the larger cells are found among the small posterior DN3s, while the other two are found among the more anteriorly located neurons of this group. Interestingly, the projections of the large DN3s strongly resemble the arborization pattern of the ITP- and CRY-positive LNs (fifth LN and LNd) (Schubert et al., 2018). Their projections initially run along the posterior boundary between the LH and the SLP (Figures 14A,B open arrowhead). The first branching occurred on the posterior surface of the brain at the level of the trijunction between the LH, SLP, and SCL (Figures 14A,B, arrows). Here, some of the fibers from the large DN3 projected anteriorly to the AOTU via three paths. The fiber bundle running along the first path, projects around the surface of the SLP. This is the same path (or better loop) used by the CRY-positive DN1ps and the DN2s projecting towards the AOTU. The second path towards the AOTU coincides with the second loop of the CRY-positive DN1ps that runs along the trijunction of SLP, SCL, and superior intermediate protocerebrum. The third path originates in the lateral PLP and runs along the surface of the anterior ventrolateral protocerebrum. These different fiber bundles running in the different paths are hard to identify in Figure 14 (anterior projecting fibers in yellow) and are best seen in Supplementary Video S4.
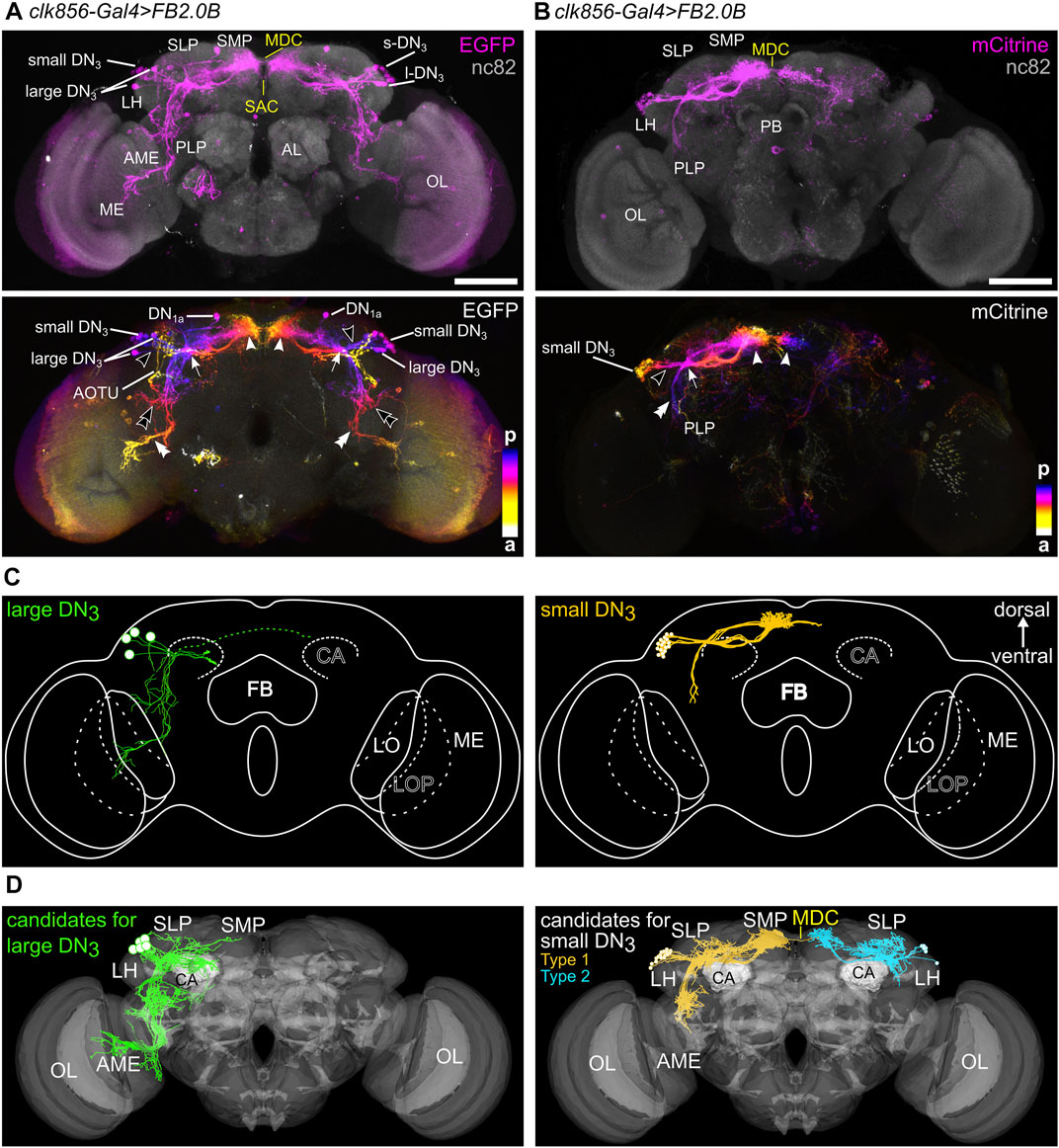
FIGURE 14. The morphology of the DN3 subclasses. (A,B) Two exemplary brains, which express the Flybow-reporters in different subsets of DN3 (maximal projections of 57 (A) and 32 (B) confocal planes). The upper panel shows an overlay with neuropil counterstaining (nc82), the lower panel shows the neurons with color-coded depth information. Fibers in the posterior (p) brain are marked blue and fibers further anterior (a) are marked in yellow/white. (A) Fibers in the accessory medulla (AME) could only be observed in brains, in which the larger DN3s were labeled. Likewise, the projections through the superior arch commissure (SAC) are absent in brains, in which the large DN3s are not labeled (B). (B) Arborization pattern of the small DN3s. The projections of these cells in the posterior lateral protocerebrum (PLP) do not invade the AME. The fibers run through the middle dorsal commissure (MDC), but not via the SAC into the contralateral hemisphere. (C) Estimated projection pattern of the large and small DN3s. All DN3s have arborizations in the dorsal brain, but the contribution to the SAC is unique to the larger cells. The overlap with projections from other neurons (mainly small DN3s) made further analysis of the DN3 arborization pattern in the dorsal brain impossible (indicated by dashed line). (D) Reconstruction of potential DN3 candidates from the hemibrain. According to their morphology, the putative DN3s can be divided into three subtypes (green, yellow, cyan). While the green neurons look similar to the above-described morphology of the large DN3s the yellow neurons resemble the described morphology of the small DN3s (compare with C). The cyan depicted neurons are not matching the described morphologies based on the Flybow staining. They possess the arborization pattern of the small DN3s in the superior lateral protocerebrum (SLP) but lack the fibers projecting ventrally to the posterior lateral protocerebrum (PLP). These neurons could be a further subtype of the DN3s, which was not detected by the Flybow staining. Candidates for the large DN3: #510602264, #5813021221, #356140100, #5813057153, #5813020750; candidates for (DN3) small DN3 type 1: #357949102, #5813010377, #358285424, #358976515, #450371612, #478937243, #510680957, #571364144, #664447502, #5813026780, #5813032640, #451424866, #574062996 (DN3_A). Candidates for small DN3 type 2: #326465302, #356835277, #386847799, #417536905, #417882515, #479260921, #358631450, #328273806 (DN3_B). LH, lateral horn; ME, medulla; LO, lobula; LOP, lobula plate; FB, fan-shaped body; EB, ellipsoid body; CA, mushroom body calyx; PB, protocerebral bridge; AL, antennal lobe. Scale bars represent 50 μm.
Other fibers of the DN3s remained initially posterior (blue colors in Figure 14A) and passed ventrally through the PLP until they reached the ipsilateral AME (Figure 14A white double arrowhead). On their way to the AME, numerous side-branches separated from the main bundle and formed a kind of fiber hub (Figure 14A double open arrowhead) where they overlap with the ITP- and CRY-positive LNs (see Supplementary Video S5).
Most fibers of the DN3 projected medially forming a dense varicose fiber network in the SMP (Figures 14A,B, arrowheads). From there, a portion of the projections crossed the midline of the brain via the MDC and superior arch commissures (SAC) reaching into the contralateral hemisphere (Figures 14A,B).
We observed a different arborization pattern in brains in which only the small DN3s were labeled compared to brains in which both, the large and small DN3s were stained (Figure 14B). In these brains, the two hemispheres were connected exclusively via the MDC, with no additional projections through the SAC (Figure 14B). However, there are even more apparent differences between the small and large DN3s: the small DN3s lack the fiber bundle towards the AOTU and the projections ending in the AME. The fibers of the small DN3s, that pass through the PLP toward the AME terminate in the fiber hub of the PLP halfway to the AME described above (Figure 14B double arrowheads). Thus, only the four to five large DN3s send fibers into the AOTU and AME (Figure 14C).
No DN3s could be annotated with certainty in the hemibrain dataset until now. However, there are possible candidates available of which three subtypes can be distinguished. While two subtypes comprise only five (named DN3) and six (named DN3B) neurons, respectively, the third subtype comprises 15 neurons (named DN3A) (Supplementary Video S4). Comparing the morphology of these DN3 candidates with our findings, we are fairly confident that the subtype with five neurons comprises the large DN3s, as their projection pattern is consistent with our descriptions (Figure 14D). The only difference is that there are no fibers reported running through the SAC in the hemibrain that we observed in our Flybow staining, but this might be caused by a general lack of contralateral projections in the hemibrain, which we discussed previously (Reinhard et al., 2022).
The second group of DN3s candidates from the hemibrain that matches our description is the DN3A subtype comprising 15 neurons. These neurons largely resemble the small DN3s. As we found in our staining they project halfway down to the AME and terminate in the PLP fiber hub.
The third subtype of DN3 candidates in the hemibrain completely lacks the projections extending ventrally towards the AME in the PLP. Otherwise, it looks similar to the small DN3s. We did not see this subtype in our staining, but most likely, the DN3s comprise more subtypes than we and others could identify so far.
The small DN3s were also labeled by Guo et al. (2018) as common neurons between the Clk856-GAL4 and the R77H08-LexA driver lines (Guo et al., 2018 Supplementary Figure S1A). Furthermore, Meiselman et al. (2022) recently found the large (and several small DN3s) labeled by a specific split-GAL4 line. These authors report the same arborization pattern that we describe here. They also found a faint commissure in the superior brain, but unfortunately, we could not distinguish between the SAC and MDC according to their figures (Figure 2K in Meiselman et al., 2022).
Due to the lack of specific driver lines, we have not yet been able to perform a polarity analysis of the DN3s. It will be most promising to do so in the future with the recently published split-GAL4 line. In addition, we assume that the hemibrain DN3 candidates will be certainly annotated as DN3s as soon as further experiments allow a more accurate comparison in respect of their polarity and synaptic partners.
Discussion
This study aimed to unravel the morphology and connectivity of Drosophila’s dorsal clock neurons. Throughout the results, we have compared our findings with those of previous studies, which we largely confirm. Nevertheless, we found new details that reveal a so far unknown degree of heterogeneity within certain clock neuron groups that might impact their function. Only the two DN1as and two DN2s appear to have similar morphology. This observation is supported by single-cell RNA sequencing analysis that found no qualitative differences within the neurons of these groups (Ma et al., 2021). At the same time, the DN1as and DN2s (together with the four s-LNvs and the fifth LN) are functional from the first larval instar onward making them basic clock neurons that might be of particular functional importance (Kaneko et al., 1997). In the following, we will discuss our findings in the light of numerous functional studies that have attributed specific roles to the clock neuron groups, with a special emphasis on the DN1as and DN2s.
Putative Functional Impact of the DN1as
The DN1as express two neuropeptides (CCHamide1 and IPNamide) (Shafer et al., 2006; Fujiwara et al., 2018) and one neurotransmitter (glutamate) (Hamasaka et al., 2007). They are functionally strongly interconnected with the s-LNvs via neuropeptide signaling. While the s-LNvs signal to the DN1as via PDF (Shafer et al., 2008; Im et al., 2011), the DN1as signal to the s-LNvs via CCHamide1 (Fujiwara et al., 2018). Here, we show by trans-Tango that the two neurons are additionally interconnected via regular synapses. This fits with previous trans-Tango studies (Song et al., 2021) as well as immunohistochemical results demonstrating that the s-LNvs express metabotropic glutamate receptors that can be activated by glutamate from the DN1as (Hamasaka et al., 2007). Vice versa, the DN1as might express glycine receptors that can be activated by glycine released from the s-LNvs, although this has not yet been proven (Frenkel et al., 2017). Manipulations of the reciprocal peptidergic connections between the DN1as and the s-LNvs affect the flies’ free-running period, their morning and evening activity as well as their siesta under light-dark conditions (Fujiwara et al., 2018). The synaptic connections between the two neuron groups have not been specifically manipulated, but the knockdown of glutamate receptors in the s-LNvs increases the free-running period and the nocturnal activity under light-dark conditions (Hamasaka et al., 2007). Furthermore, downregulation of glutamate synthetase in all dorsal clock neurons increases the rhythmicity of flies under constant light conditions (de Azevedo et al., 2020), indicating that glutamate signaling from the DNs is involved in the general light sensitivity of the clock. Although it is unlikely that this effect is mediated exclusively by the DN1as (some DN1ps and DN3s are also glutamatergic as shown by Hamasaka et al. (2007) and Collins et al. (2012)), the DN1as appear to contribute strongly to the light detection. At night, their silencing reduces light-induced startle responses, whereas during the day, PDFR-dependent signaling to the DN1as limits light-induced startle responses (Song et al., 2021). As we show here, the DN1as get strong input from the dorsal and lateral accessory calyces. The dorsal accessory calyces transfer visual information from the lobula (Li et al., 2020). Thus, DN1as may directly receive light information from the optic lobe.
On the other hand, the lateral accessory calyces convey thermosensory information from neurons in the antennal lobes (Marin et al., 2020). While about half of the clock neurons are intrinsically light-sensitive (via CRY), none of them is per se temperature-sensitive (Sehadova et al., 2009) but they rely on temperature input from peripheral sensory neurons (the aristae on the antennae, the chordotonal organs and the antennal AC neurons (Tang et al., 2017; reviewed in; George and Stanewsky, 2021)). Indeed, the DN1as get strong synaptic input from several temperature sensing projection neurons from the antennal lobes. Alpert et al. (2020) showed that temperatures below the preferred temperature for flies (∼25°C) silence the DN1as via GABAergic synapses from such thermosensory projection neurons. This silencing leads to reduced activity and increased sleep in the morning and evening, which can only be overwritten by light and PDF signaling. Indeed, we found that the DN1as signal not only to the morning neurons (the s-LNvs) but even stronger to the evening neurons, namely the CRY- and ITP-positive fifth LN and LNd. In addition, the DN1as have few output synapses to tangential neurons arborizing in the second and eighth layer of the dFB, which overlap with the fibers of several clock neurons in the PLP. The dFB is known to be involved in the control of sleep (reviewed in Bertolini and Helfrich-Förster, 2021).
Altogether these results demonstrate that the DN1as play a central role in light- and temperature integration of the circadian clock and can prominently influence the morning and evening neurons as well as the sleep of flies.
Putative Functional Impact of the DN1ps
We could show that the DN1ps consist of more than the originally described two types of CRY-positive and CRY-negative neurons. In the hemibrain, seven of the ∼15 DN1ps are annotated. The seven neurons are classified into type A and type B neurons, but the type A neurons appear heterogeneous. At least one type A neuron looks different from the others (green neuron in Figure 6). We found the same neuron type by Flybow and identified an additional one that was not yet annotated in the hemibrain. Lamaze et al. (2018) depict a further DN1p type in their supplement that was neither identified in the hemibrain nor in the present study. These two previously undescribed DN1ps project contralaterally like the type A neurons but differ in their projections toward the AOTU and PLP. The DN1p identified here lacks the anterior projections to the AOTU but shows prominent fibers to the PLP (Figure 6D right panel), whereas the DN1p identified by Lamaze projects anteriorly to the AOTU and lacks fibers in the PLP (Supplementary Figure S1A in Lamaze and Stanewsky, 2020). These findings demonstrate that the DN1ps encompass many neurons with different morphology and most likely also different neurochemistry. Single-cell RNA sequencing data underline this conclusion: according to their gene expression, the DN1ps comprise five to six distinct clusters (Ma et al., 2021). This heterogeneity could explain the different and partly contradicting findings regarding their function(s) (reviewed in Lamaze and Stanewsky, 2020). We will not repeat these details here but instead, focus on the main findings adding new insights that derive from the present study.
The DN1ps appear to comprise morning and evening neurons (Chatterjee et al., 2018), which is strongly supported by our findings. Type A DN1ps receive strong synaptic input from the evening neurons (ITP positive fifth LN and LNd) and signal even stronger back to them. Thus, there exists a prominent mutual connection between the type A DN1ps and the evening neurons, suggesting that the five annotated type A DN1ps are by themselves evening neurons. Nevertheless, the seven neurons are heterogeneous and at least one of them (green neuron in Figures 5, 6) gets synaptic input in the PLP, a region where many clock neurons arborize. This DN1p may additionally receive input from morning neurons (s-LNvs) via PDF or from the LPNs via DH31 (Reinhard et al., 2022). So far this is the only annotated type A DN1p neuron that is most likely CRY-positive, expresses the PDF receptor and additionally receives synaptic input in the PLP. It is tempting to speculate that all DN1ps that overlap with the s-LNv projections in the PLP are CRY-positive, but this has to be proven by future studies. The type B DN1ps are all CRY-positive, largely overlap with projections from the s-LNvs, and express the PDFR. Thus, they are connected via PDF with the morning neurons and can contribute to the morning activity of the flies.
At the same time, DN1ps consist of sleep- and activity-promoting neurons (Guo et al., 2018; Lamaze et al., 2018). Through their fibers projecting to the pars intercerebralis, they affect wakefulness by signaling to neurosecretory cells expressing the Drosophila homolog of the mammalian corticotropin-releasing factor (Cavanaugh et al., 2014; Kunst et al., 2014). In contrast, the anterior projections of the DN1ps to the AOTU, appear to play a prominent role in sleep control because in the AOTU, CRY-positive type B DN1ps signal on sleep-promoting ring neurons of the ellipsoid body, influencing their neuronal activity (Lamaze et al., 2018). Here, we could confirm the intimate connection of the DN1ps to the AOTU. Moreover, we could show that the projections of the DN1ps toward the AOTU largely overlap with fibers from the DN2s and large DN3s. The DN1ps strongly signal via these projections to the DN2s (see below).
DN1ps are also known to promote the siesta of the flies (Guo et al., 2016) and to inhibit activity at low temperatures (Yadlapalli et al., 2018). They respond to cool temperatures (∼16°C) with a strong increase in firing rate, while they are inactive at warm temperatures (>25°C) (Yadlapalli et al., 2018). In contrast to the DN1as, the DN1ps do not arborize in the lateral accessory lobes, but they seem to receive temperature information from thermoreceptors in the aristae of the antennae and chordotonal organs in the body via signals from anterior cells (AC) which are known to be involved in temperature sensing pathways (Hamada et al., 2008; Yadlapalli et al., 2018; Jin et al., 2021). Thus, the DN1ps appear to be a major gateway for temperature sensing into the circadian neural network, which continuously integrates temperature changes to coordinate the timing of sleep and activity. Most likely, the DN1ps pass this information to other clock neurons, which do not receive temperature input directly but are similarly involved in temperature-related behavior (e.g. DN2, Kaneko et al., 2012; Goda et al., 2016).
It is worth noting that the so far annotated type A DN1ps are quite polar and have their synaptic output sites in the contralateral SMP. The same is true for the DN2s, which we will discuss in the following. This feature might be important for integrating information from both hemispheres.
Putative Functional Impact of the DN2s
The two DN2s, similarly to the DN1as, show a special association with the s-LNvs. All three clock neuron groups express PER cyclically already during the larval stages, whereas PER in the larval DN2s cycles in antiphase to the other clock neurons (Kaneko et al., 1997). This anti-phase cycling depends on PDF signaling from the s-LNvs to the DN2s (Picot et al., 2009) suggesting that the DN2s are PDFR positive, although they are reported to be CRY-negative in adults (Benito et al., 2008; Yoshii et al., 2008). Here, we report weak CRY-immunostaining in one of the DN2s, strongly suggesting that the PDFR is also present since they are always co-expressed (Im et al., 2011).
The DN2s are important for temperature entrainment in larvae (Picot et al., 2009) and temperature preference in adults (Kaneko et al., 2012). In contrast to the DN1as, the DN2s do not arborize in the lateral accessory calyces and we could not detect any direct input from temperature sensing neurons of the antennal lobes. Nevertheless, the DN2s could get information indirectly via other clock neurons. The most promising candidates are the type B CRY-positive DN1ps that get input from thermosensory AC neurons and that strongly contact the DN2s. Furthermore, Tang et al. (2017) suggested that AC neurons project also directly to the DN2s. In addition to synaptic input, the DN2s may receive neuropeptidergic input from the DN1ps via DH31 (Goda et al., 2016).
The DN2s themselves do barely signal to other clock neurons, suggesting that they are pure output neurons of the clock network. As mentioned, they are highly polar as they receive synaptic input in the ipsilateral SLP and have output synapses in the contralateral SMP. As we show here by trans-Tango, their main output sites are the mushroom bodies and neurosecretory cells in the pars intercerebralis. These two sites are highly involved in the regulation of sleep, arousal, and metabolism (see above and discussion in Reinhard et al. (2022)). Consequently, DN2s can impact both processes and adapt them to different environmental temperatures.
Putative Functional Impact of the DN3s
The DN3s are the largest and, until very recently, also the less well-characterized group of the dorsal neurons. They are heterogeneous, not only regarding their size and morphology but also in respect to their gene expression, which divides them into two clusters (Ma et al., 2021). Here, we confirm previous findings that the DN3s consist of neurons with large and small somata with different morphologies, but they may be composed of further subgroups, as suggested by data from the hemibrain.
Some (∼4) of the DN3s express a homolog of the vertebrate circadian gene nocturnin, coding for a deadenylase involved in mRNA decay (Nagoshi et al., 2010), and up to 17 DN3s express the gene quasimodo, coding for a Zona pellucida domain protein (Chen et al., 2011; Buhl et al., 2016). Both proteins are involved in the light perception of the clock. Other observations suggest an additional role of the DN3s in the sensory integration of temperature cues into the circadian system (Harper et al., 2016). Consistent with this observation, up to 10 DN3s neurons express the DH31 receptor, and together with the DN2s cells, they appear necessary for regulating the temperature preference of the flies at the beginning of the night (Goda et al., 2016). The large DN3s are additionally responsive to PDF (Shafer et al., 2008). Furthermore, some DN3s express glutamate, and the majority of them express the neuropeptide AstC (Guo et al., 2018; Díaz et al., 2019; Meiselman et al., 2022). Díaz et al. (2019) suggest that the AstC-positive DN3s signal to at least one LNd and in this way influence the evening activity of the flies. Guo et al. (2018) demonstrate a sleep-promoting role of the DN3s, especially of the small DN3s. Meiselman et al. (2022) show that the AstC-positive DN3s regulate the cold-induced dormancy of the flies, supporting the role of the DN3s in temperature-dependent behavior.
All these different roles are supported by morphological data. Especially the arborizations of the large DN3s reach the entire clock network. They form arborizations in all four fiber hubs of the clock network (AME, SMP, ventral PLP and the loop in the SLP). In the AME they might not only receive light input (Li et al., 2018) but also communicate with all lateral neurons. In the SMP, they overlap with all clock neurons besides the LNvs. In the ventral PLP hub (double open arrowhead in Figure 14A) their fibers largely overlap with the cell bodies of the LPNs and with fibers from the evening neurons (ITP-positive fifth LN and LNd) and the small DN3s. In the SLP loop, they are again in close contact with the evening neurons as well as with many DNs. Consequently, they may communicate with all these neurons. From our trans-Tango data, we already know that one to two larger DN3s get input from the DN1as. Connectivity data from the hemibrain suggest weak synaptic input from the type B CRY-positive DN1ps to some of the small DN3s. The large DN3 even appear to have dense arborizations in the lateral accessory calyces, which would predispose them to receive information from the thermosensory neurons of the antennal lobes. Moreover, they cross the midline of the brain in two commissures, meaning that they can integrate information from both brain hemispheres. The small DN3s form a prominent fiber network in the SLP and SMP and run ventrally in the PLP where most of them appear to terminate in the fiber hub formed by the arborizations of the large DN3s, fifth LN, and LNd. Thus, they contribute to the wide connectivity of the large DN3s within the clock network.
Conclusion
Here, we show that the DNs are an integral part of the clock network. Functionally, they appear to integrate light and temperature information into the clock network and contribute to the control of many behavioral outputs reaching from sleep and activity to the control of metabolism via the neurosecretory system of the fly (sumarized in Figure 15). Anatomically, we show that the fibers of the DNs intermingle with those of the LNs (Supplementary Video S5). Besides the already known AME and the SMP (often referred to simply as the dorsal protocerebrum), we identify two areas in the brain, in which the interplay between all clock neurons appears especially intimate. The first area is the SLP, where several clock neurons join a fiber tract, which forms a loop around the SLP and terminates in the AOTU. These are the CRY-positive type B DN1ps, the DN2s, the large DN3s, the LPNs, and the ITP- and CRY-positive fifth LN and LNd. The second area lies in the PLP very close to the cell bodies of the LPNs, where the s-LNvs pass through and the large and small DN3s, the DN1as, and the ITP- and CRY-positive fifth LN and LNd contribute to a fiber hub. These two brain areas may serve as additional communications centers for the clock neurons. They may also serve as additional input and output centers of the clock. Here, we show that the clock network signals to certain dFB neurons via the PLP hub and Kind et al. (2021) have recently demonstrated connections from the visual system to the AME and the PLP hub as well as the AOTU close to the loop in the SLP. It will be most promising to unravel these connections in further detail.
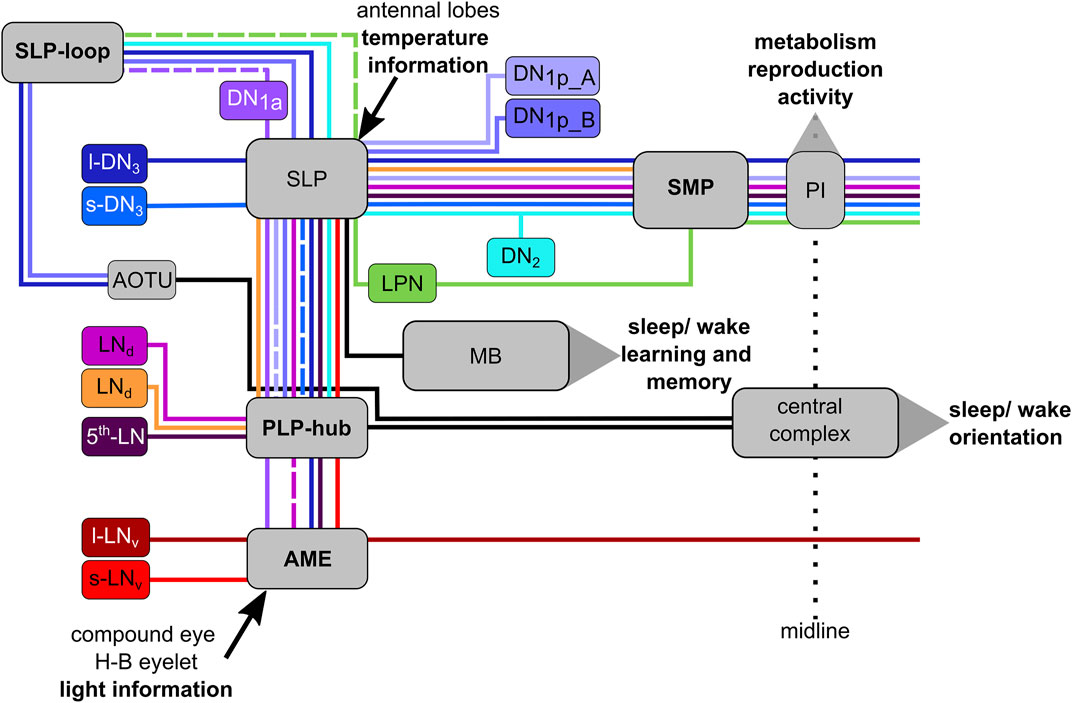
FIGURE 15. Schematic overview of the Drosophila clock network in the left hemisphere including input and output sites. The major groups of clock neurons and their corresponding projections are depicted in color, while the brain regions they invade are shown in grey. Bold letters highlight major hubs and tracts, in which the fibers of several clock neurons intermingle and contact each other. These are the “accessory medulla” (AME), the newly discovered “posterior lateral protocerebrum hub” (PLP-hub), the “superior lateral protocerebrum loop” (SLP-loop) and the “superior medial protocerebrum” (SMP). Dashed colored lines indicate that not all neurons of the relevant group show the drawn projections. Besides the intrinsical light-sensitivity of some clock neurons (via CRY), the clock network receives light input from the compound eyes and the Hofbauer-Buchner (H-B) eyelets in the AME, while it receives temperature information solely from peripheral sensory organs in the chordotonal organs antennae and antennal lobes in the “superior lateral protocerebrum” (SLP). Output from the clock network to control rhythms in metabolism, reproduction and activity occurs in the “pars intercerebralis” (PI), while the rhythmic control of the sleep/wake cycle occurs via connections to the mushroom bodies (MB) and the central complex. The MBs fulfill additional functions such as learning and memory that are also modulated by the clock network. The central complex consists of the protocerebral bridge, the fan-shaped body, the ellipsoid body and the noduli and has additional important roles in orientation and navigation. Flying straight for several hours needs the input from the clock to compensate for the movements of the sun across the sky. This input may come via the DN1p_B (also called anteriorly projecting DN1p) and the DN3 with large somata (l-DN3) that run to the anterior optic tubercle (AOTU). The anterior tubercle neurons are connected with the ring neurons of the central complex of which some are involved in orientation and others in sleep. Certain neurons of the fan-shaped body have synaptic contact with clock neurons in the PLP hub and by this way may carry rhythmic information into the central complex.
Data Availability Statement
The raw data supporting the conclusions of this article will be made available by the authors, without undue reservation.
Author Contributions
FS performed and analyzed experiments for the anatomy studies with the help of NH. NR performed and analyzed experiments for the anatomy and connectivity studies. EB, DR and GM acquired data for the connectivity studies, MS generated the DN2 split-GAL4 line. NR analyzed the data, compiled the figures, and wrote the first version of the manuscript together with FS and CH-F. CH-F improved the manuscript and wrote the abstract and discussion with contributions from NR, EB, DR and TY. CH-F, DR and TY conceived the project, supervised the study, and contributed to funding.
Funding
This research was funded by the Deutsche Forschungsgemeinschaft (DFG, German Research Foundation) (grants FO 207/16-1, RI 2411/1-1 and for the Leica SP8: 251610680, INST 93/809-1 FUGG) and the JSPS KAKENHI (19H03265). Publication was supported by the Open Access Publication Fund of the University of Würzburg. We thank Heinrich Dircksen for ITP antisera, Ralf Stanewsky for PER antisera and Takeshi Todo for CRY antisera, Jan Veenstra for antibodies against AstC, and DH31 as well as Fumika Hamada for the Clk9M-Gal4; pdf-Gal80 fly line, and Patrick Greguletz for help with preparing and immunostaining. Furthermore, we are grateful to Maria Fernandez and Orie Shafer for communicating results prior to publication.
Conflict of Interest
The authors declare that the research was conducted in the absence of any commercial or financial relationships that could be construed as a potential conflict of interest.
Publisher’s Note
All claims expressed in this article are solely those of the authors and do not necessarily represent those of their affiliated organizations, or those of the publisher, the editors and the reviewers. Any product that may be evaluated in this article, or claim that may be made by its manufacturer, is not guaranteed or endorsed by the publisher.
Supplementary Material
The Supplementary Material for this article can be found online at: https://www.frontiersin.org/articles/10.3389/fphys.2022.886432/full#supplementary-material
References
Abruzzi K. C., Zadina A., Luo W., Wiyanto E., Rahman R., Guo F., et al. (2017). RNA-seq Analysis of Drosophila Clock and Non-clock Neurons Reveals Neuron-specific Cycling and Novel Candidate Neuropeptides. Plos Genet. 13, e1006613. doi:10.1371/journal.pgen.1006613
Ahmad M., Li W., Top D. (2021). Integration of Circadian Clock Information in the Drosophila Circadian Neuronal Network. J. Biol. Rhythms 36, 203–220. doi:10.1177/0748730421993953
Alpert M. H., Frank D. D., Kaspi E., Flourakis M., Zaharieva E. E., Allada R., et al. (2020). A Circuit Encoding Absolute Cold Temperature in Drosophila. Curr. Biol. 30, 2275–2288. e5. doi:10.1016/j.cub.2020.04.038
Arshadi C., Günther U., Eddison M., Harrington K. I. S., Ferreira T. A. (2021). SNT: a Unifying Toolbox for Quantification of Neuronal Anatomy. Nat. Methods 18, 374–377. doi:10.1038/s41592-021-01105-7
Azevedo R. V. D. M. d., Hansen C., Chen K.-F., Rosato E., Kyriacou C. P. (2020). Disrupted Glutamate Signaling in Drosophila Generates Locomotor Rhythms in Constant Light. Front. Physiol. 11, 145. doi:10.3389/fphys.2020.00145
Barber A. F., Erion R., Holmes T. C., Sehgal A. (2016). Circadian and Feeding Cues Integrate to Drive Rhythms of Physiology in Drosophila Insulin-Producing Cells. Genes Dev. 30, 2596–2606. doi:10.1101/gad.288258.116
Bates A. S., Jefferis G. S. X. E. (2022). Hemibrainr: Code for Working with Data from Janelia FlyEM's Hemibrain Project and the Flywire Data Sets. Available at: https://github.com/flyconnectome/hemibrainr.
Bates A. S., Manton J. D., Jagannathan S. R., Costa M., Schlegel P., Rohlfing T., et al. (2020). The Natverse, a Versatile Toolbox for Combining and Analysing Neuroanatomical Data. eLife 9, e53350. doi:10.7554/eLife.53350
Beckwith E. J., Ceriani M. F. (2015). Communication between Circadian Clusters: The Key to a Plastic Network. FEBS Lett. 589, 3336–3342. doi:10.1016/j.febslet.2015.08.017
Benito J., Houl J. H., Roman G. W., Hardin P. E. (2008). The Blue-Light Photoreceptor CRYPTOCHROME Is Expressed in a Subset of Circadian Oscillator Neurons in the Drosophila CNS. J. Biol. Rhythms 23, 296–307. doi:10.1177/0748730408318588
Bertolini E., Helfrich-Förster C. (2021). “-Sleep and the Circadian Clock in Insects,” in Reference Module in Neuroscience and Biobehavioral Psychology (Amsterdam, Netherlands: Elsevier). doi:10.1016/B978-0-12-822963-7.00037-2
Bogovic J. A., Otsuna H., Heinrich L., Ito M., Jeter J., Meissner G., et al. (2020). An Unbiased Template of the Drosophila Brain and Ventral Nerve Cord. PLOS ONE 15, e0236495. doi:10.1371/journal.pone.0236495
Buhl E., Bradlaugh A., Ogueta M., Chen K.-F., Stanewsky R., Hodge J. J. L. (2016). Quasimodo Mediates Daily and Acute Light Effects on Drosophila Clock Neuron Excitability. Proc. Natl. Acad. Sci. U.S.A. 113, 13486–13491. doi:10.1073/pnas.1606547113
Cavanaugh D. J., Geratowski J. D., Wooltorton J. R. A., Spaethling J. M., Hector C. E., Zheng X., et al. (2014). Identification of a Circadian Output Circuit for Rest:activity Rhythms in Drosophila. Cell 157, 689–701. doi:10.1016/j.cell.2014.02.024
Chatterjee A., Lamaze A., De J., Mena W., Chélot E., Martin B., et al. (2018). Reconfiguration of a Multi-Oscillator Network by Light in the Drosophila Circadian Clock. Curr. Biol. 28, 2007–2017. e4. doi:10.1016/j.cub.2018.04.064
Chen J., Reiher W., Hermann-Luibl C., Sellami A., Cognigni P., Kondo S., et al. (2016). Allatostatin A Signalling in Drosophila Regulates Feeding and Sleep and Is Modulated by PDF. Plos Genet. 12, e1006346. doi:10.1371/journal.pgen.1006346
Chen K. F., Peschel N., Zavodska R., Sehadova H., Stanewsky R. (2011). QUASIMODO, a Novel GPI-Anchored Zona Pellucida Protein Involved in Light Input to the Drosophila Circadian Clock. Curr. Biol. 21, 719–729. doi:10.1016/j.cub.2011.03.049
Collins B., Kane E. A., Reeves D. C., Akabas M. H., Blau J. (2012). Balance of Activity between LNvs and Glutamatergic Dorsal Clock Neurons Promotes Robust Circadian Rhythms in Drosophila. Neuron 74, 706–718. doi:10.1016/j.neuron.2012.02.034
Díaz M. M., Schlichting M., Abruzzi K. C., Long X., Rosbash M. (2019). Allatostatin-C/AstC-R2 Is a Novel Pathway to Modulate the Circadian Activity Pattern in Drosophila. Curr. Biol. 29, 13–22. e3. doi:10.1016/j.cub.2018.11.005
Ewer J., Frisch B., Hamblen-Coyle M., Rosbash M., Hall J. (1992). Expression of the Period Clock Gene within Different Cell Types in the Brain of Drosophila Adults and Mosaic Analysis of These Cells' Influence on Circadian Behavioral Rhythms. J. Neurosci. 12, 3321–3349. doi:10.1523/JNEUROSCI.12-09-03321.1992
Frenkel L., Muraro N. I., Beltrán González A. N., Marcora M. S., Bernabó G., Hermann-Luibl C., et al. (2017). Organization of Circadian Behavior Relies on Glycinergic Transmission. Cel Rep. 19, 72–85. doi:10.1016/j.celrep.2017.03.034
Frisch B., Hardin P. E., Hamblen-Coyle M. J., Rosbash M., Hall J. C. (1994). A Promoterless Period Gene Mediates Behavioral Rhythmicity and Cyclical Per Expression in a Restricted Subset of the drosophila Nervous System. Neuron 12, 555–570. doi:10.1016/0896-6273(94)90212-7
Fujiwara Y., Hermann-Luibl C., Katsura M., Sekiguchi M., Ida T., Helfrich-Förster C., et al. (2018). The CCHamide1 Neuropeptide Expressed in the Anterior Dorsal Neuron 1 Conveys a Circadian Signal to the Ventral Lateral Neurons in Drosophila melanogaster. Front. Physiol. 9, 1276. doi:10.3389/fphys.2018.01276
George R., Stanewsky R. (2021). Peripheral Sensory Organs Contribute to Temperature Synchronization of the Circadian Clock in Drosophila melanogaster. Front. Physiol. 12. doi:10.3389/fphys.2021.622545
Goda T., Doi M., Umezaki Y., Murai I., Shimatani H., Chu M. L., et al. (2018). Calcitonin Receptors Are Ancient Modulators for Rhythms of Preferential Temperature in Insects and Body Temperature in Mammals. Genes Dev. 32, 140–155. doi:10.1101/gad.307884.117
Goda T., Tang X., Umezaki Y., Chu M. L., Kunst M., Nitabach M. N., et al. (2016). Drosophila DH31 Neuropeptide and PDF Receptor Regulate Night-Onset Temperature Preference. J. Neurosci. 36, 11739–11754. doi:10.1523/JNEUROSCI.0964-16.2016
Goda T., Umezaki Y., Alwattari F., Seo H. W., Hamada F. N. (2019). Neuropeptides PDF and DH31 Hierarchically Regulate Free-Running Rhythmicity in Drosophila Circadian Locomotor Activity. Sci. Rep. 9. doi:10.1038/s41598-018-37107-3
Grima B., Chélot E., Xia R., Rouyer F. (2004). Morning and Evening Peaks of Activity Rely on Different Clock Neurons of the Drosophila Brain. Nature 431, 869–873. doi:10.1038/nature02935
Gummadova J. O., Coutts G. A., Glossop N. R. J. (2009). Analysis of theDrosophila ClockPromoter Reveals Heterogeneity in Expression between Subgroups of Central Oscillator Cells and Identifies a Novel Enhancer Region. J. Biol. Rhythms 24, 353–367. doi:10.1177/0748730409343890
Guo F., Holla M., Díaz M. M., Rosbash M. (2018). A Circadian Output Circuit Controls Sleep-Wake Arousal in Drosophila. Neuron 100, 624–635. e4. doi:10.1016/j.neuron.2018.09.002
Guo F., Yu J., Jung H. J., Abruzzi K. C., Luo W., Griffith L. C., et al. (2016). Circadian Neuron Feedback Controls the Drosophila Sleep-Activity Profile. Nature 536, 292–297. doi:10.1038/nature19097
Hadjieconomou D., Rotkopf S., Alexandre C., Bell D. M., Dickson B. J., Salecker I. (2011). Flybow: Genetic Multicolor Cell Labeling for Neural Circuit Analysis in Drosophila melanogaster. Nat. Methods 8, 260–266. doi:10.1038/nmeth.1567
Hamada F. N., Rosenzweig M., Kang K., Pulver S. R., Ghezzi A., Jegla T. J., et al. (2008). An Internal Thermal Sensor Controlling Temperature Preference in Drosophila. Nature 454, 217–220. doi:10.1038/nature07001
Hamasaka Y., Rieger D., Parmentier M.-L., Grau Y., Helfrich-Förster C., Nässel D. R. (2007). Glutamate and its Metabotropic Receptor inDrosophilaclock Neuron Circuits. J. Comp. Neurol. 505, 32–45. doi:10.1002/cne.21471
Harper R. E. F., Dayan P., Albert J. T., Stanewsky R. (2016). Sensory Conflict Disrupts Activity of the Drosophila Circadian Network. Cel Rep. 17, 1711–1718. doi:10.1016/j.celrep.2016.10.029
Helfrich-Förster C. (2005). Neurobiology of the Fruit Fly's Circadian Clock. Genes, Brain Behav. 4, 65–76. doi:10.1111/j.1601-183X.2004.00092.x
Helfrich-Förster C., Shafer O. T., Wülbeck C., Grieshaber E., Rieger D., Taghert P. (2007a). Development and Morphology of the Clock-Gene-Expressing Lateral Neurons ofDrosophila Melanogaster. J. Comp. Neurol. 500, 47–70. doi:10.1002/cne.21146
Helfrich-förster C., Stengl M., Homberg U. (1998). Organization of the Circadian System in Insects. Chronobiology Int. 15, 567–594. doi:10.3109/07420529808993195
Helfrich-Förster C. (2017). “The Drosophila Clock System,” in Biological Timekeeping: Clocks, Rhythms and Behaviour. Editor V. Kumar (New Delhi: Springer India), 133–176. doi:10.1007/978-81-322-3688-7_6
Helfrich-Förster C. (2003). The Neuroarchitecture of the Circadian Clock in the Brain ofDrosophila Melanogaster. Microsc. Res. Tech. 62, 94–102. doi:10.1002/jemt.10357
Helfrich-Förster C., Yoshii T., Wülbeck C., Grieshaber E., Rieger D., Bachleitner W., et al. (2007b). The Lateral and Dorsal Neurons ofDrosophila melanogaster:New Insights about Their Morphology and Function. Cold Spring Harbor Symposia Quantitative Biol. 72, 517–525. doi:10.1101/sqb.2007.72.063
Herzog E. D., Hermanstyne T., Smyllie N. J., Hastings M. H. (2017). Regulating the Suprachiasmatic Nucleus (SCN) Circadian Clockwork: Interplay between Cell-Autonomous and Circuit-Level Mechanisms. Cold Spring Harb Perspect. Biol. 9, a027706. doi:10.1101/cshperspect.a027706
Im S. H., Li W., Taghert P. H. (2011). PDFR and CRY Signaling Converge in a Subset of Clock Neurons to Modulate the Amplitude and Phase of Circadian Behavior in Drosophila. PLOS ONE 6, e18974. doi:10.1371/journal.pone.0018974
Im S. H., Taghert P. H. (2010). PDF Receptor Expression Reveals Direct Interactions between Circadian Oscillators Indrosophila. J. Comp. Neurol. 518, 1925–1945. doi:10.1002/cne.22311
Jefferis G. S. X. E., Potter C. J., Chan A. M., Marin E. C., Rohlfing T., Maurer C. R., et al. (2007). Comprehensive Maps of Drosophila Higher Olfactory Centers: Spatially Segregated Fruit and Pheromone Representation. Cell 128, 1187–1203. doi:10.1016/j.cell.2007.01.040
Jin X., Tian Y., Zhang Z. C., Gu P., Liu C., Han J. (2021). A Subset of DN1p Neurons Integrates Thermosensory Inputs to Promote Wakefulness via CNMa Signaling. Curr. Biol. 31, 2075–2087. e6. doi:10.1016/j.cub.2021.02.048
Jung S.-H., Lee J.-H., Chae H.-S., Seong J. Y., Park Y., Park Z.-Y., et al. (2014). Identification of a Novel Insect Neuropeptide, CNMa and its Receptor. FEBS Lett. 588, 2037–2041. doi:10.1016/j.febslet.2014.04.028
Kaneko H., Head L. M., Ling J., Tang X., Liu Y., Hardin P. E., et al. (2012). Circadian Rhythm of Temperature Preference and its Neural Control in Drosophila. Curr. Biol. 22, 1851–1857. doi:10.1016/j.cub.2012.08.006
Kaneko M., Helfrich-Förster C., Hall J. C. (1997). Spatial and Temporal Expression of theperiodandtimelessGenes in the Developing Nervous System ofDrosophila: Newly Identified Pacemaker Candidates and Novel Features of Clock Gene Product Cycling. J. Neurosci. 17, 6745–6760. doi:10.1523/JNEUROSCI.17-17-06745.1997
Kind E., Longden K. D., Nern A., Zhao A., Sancer G., Flynn M. A., et al. (2021). Synaptic Targets of Photoreceptors Specialized to Detect Color and Skylight Polarization in Drosophila. eLife 10, e71858. doi:10.7554/eLife.71858
King A. N., Sehgal A. (2020). Molecular and Circuit Mechanisms Mediating Circadian Clock Output in the Drosophila Brain. Eur. J. Neurosci. 51, 268–281. doi:10.1111/ejn.14092
Kunst M., Hughes M. E., Raccuglia D., Felix M., Li M., Barnett G., et al. (2014). Calcitonin Gene-Related Peptide Neurons Mediate Sleep-specific Circadian Output in Drosophila. Curr. Biol. 24, 2652–2664. doi:10.1016/j.cub.2014.09.077
Lamaze A., Krätschmer P., Chen K.-F., Lowe S., Jepson J. E. C. (2018). A Wake-Promoting Circadian Output Circuit in Drosophila. Curr. Biol. 28, 3098–3105. e3. doi:10.1016/j.cub.2018.07.024
Lamaze A., Stanewsky R. (2020). DN1p or the “Fluffy” Cerberus of Clock Outputs. Front. Physiol. 10. doi:10.3389/fphys.2019.01540
Li J., Mahoney B. D., Jacob M. S., Caron S. J. C. (2020). Visual Input into the Drosophila melanogaster Mushroom Body. Cel Rep. 32, 108138. doi:10.1016/j.celrep.2020.108138
Li M.-T., Cao L.-H., Xiao N., Tang M., Deng B., Yang T., et al. (2018). Hub-organized Parallel Circuits of central Circadian Pacemaker Neurons for Visual Photoentrainment in Drosophila. Nat. Commun. 9, 4247. doi:10.1038/s41467-018-06506-5
Lin H.-H., Kuang M. C., Hossain I., Xuan Y., Beebe L., Shepherd A. K., et al. (2022). A Nutrient-specific Gut Hormone Arbitrates between Courtship and Feeding. Nature 602, 632–638. doi:10.1038/s41586-022-04408-7
Ma D., Przybylski D., Abruzzi K. C., Schlichting M., Li Q., Long X., et al. (2021). A Transcriptomic Taxonomy of Drosophila Circadian Neurons Around the Clock. eLife 10, e63056. doi:10.7554/eLife.63056
Marin E. C., Büld L., Theiss M., Sarkissian T., Roberts R. J. V., Turnbull R., et al. (2020). Connectomics Analysis Reveals First-, Second-, and Third-Order Thermosensory and Hygrosensory Neurons in the Adult Drosophila Brain. Curr. Biol. 30, 3167–3182. e4. doi:10.1016/j.cub.2020.06.028
Meiselman M. R., Alpert M. H., Cui X., Shea J., Gregg I., Gallio M., et al. (2022). Recovery from Cold-Induced Reproductive Dormancy Is Regulated by Temperature-dependent AstC Signaling. Curr. Biol. 32, 1362–1375. doi:10.1016/j.cub.2022.01.061
Michel S., Meijer J. H. (2020). From Clock to Functional Pacemaker. Eur. J. Neurosci. 51, 482–493. doi:10.1111/ejn.14388
Mieda M. (2020). The central Circadian Clock of the Suprachiasmatic Nucleus as an Ensemble of Multiple Oscillatory Neurons. Neurosci. Res. 156, 24–31. doi:10.1016/j.neures.2019.08.003
Miyasako Y., Umezaki Y., Tomioka K. (2007). Separate Sets of Cerebral Clock Neurons Are Responsible for Light and Temperature Entrainment of Drosophila Circadian Locomotor Rhythms. J. Biol. Rhythms 22, 115–126. doi:10.1177/0748730407299344
Nagoshi E., Sugino K., Kula E., Okazaki E., Tachibana T., Nelson S., et al. (2010). Dissecting Differential Gene Expression within the Circadian Neuronal Circuit of Drosophila. Nat. Neurosci. 13, 60–68. doi:10.1038/nn.2451
Ni J. D., Gurav A. S., Liu W., Ogunmowo T. H., Hackbart H., Elsheikh A., et al. (2019). Differential Regulation of the Drosophila Sleep Homeostat by Circadian and Arousal Inputs. eLife 8, e40487. doi:10.7554/eLife.40487
Nicolaï L. J. J., Ramaekers A., Raemaekers T., Drozdzecki A., Mauss A. S., Yan J., et al. (2010). Genetically Encoded Dendritic Marker Sheds Light on Neuronal Connectivity in Drosophila. Proc. Natl. Acad. Sci. U.S.A. 107, 20553–20558. doi:10.1073/pnas.1010198107
Pfeiffer B. D., Jenett A., Hammonds A. S., Ngo T.-T. B., Misra S., Murphy C., et al. (2008). Tools for Neuroanatomy and Neurogenetics in Drosophila. Proc. Natl. Acad. Sci. U.S.A. 105, 9715–9720. doi:10.1073/pnas.0803697105
Pfeiffer B. D., Ngo T.-T. B., Hibbard K. L., Murphy C., Jenett A., Truman J. W., et al. (2010). Refinement of Tools for Targeted Gene Expression in Drosophila. Genetics 186, 735–755. doi:10.1534/genetics.110.119917
Picot M., Klarsfeld A., Chélot E., Malpel S., Rouyer F. (2009). A Role for Blind DN2 Clock Neurons in Temperature Entrainment of the Drosophila Larval Brain. J. Neurosci. 29, 8312–8320. doi:10.1523/JNEUROSCI.0279-08.2009
Reinhard N., Bertolini E., Saito A., Sekiguchi M., Yoshii T., Rieger D., et al. (2022). The Lateral Posterior Clock Neurons of Drosophila melanogaster Express Three Neuropeptides and Have Multiple Connections within the Circadian Clock Network and beyond. J. Comp. Neurol. doi:10.1002/cne.25294
Renn S. C. P., Park J. H., Rosbash M., Hall J. C., Taghert P. H. (1999). A Pdf Neuropeptide Gene Mutation and Ablation of PDF Neurons Each Cause Severe Abnormalities of Behavioral Circadian Rhythms in Drosophila. Cell 99, 791–802. doi:10.1016/S0092-8674(00)81676-1
Rohlfing T., Maurer C. R. (2003). Nonrigid Image Registration in Shared-Memory Multiprocessor Environments with Application to Brains, Breasts, and Bees. IEEE Trans. Inform. Technol. Biomed. 7, 16–25. doi:10.1109/TITB.2003.808506
Rojas P., Plath J. A., Gestrich J., Ananthasubramaniam B., Garcia M. E., Herzel H., et al. (2019). Beyond Spikes: Multiscale Computational Analysis of In Vivo Long-Term Recordings in the Cockroach Circadian Clock. Netw. Neurosci. 3, 944–968. doi:10.1162/netn_a_00106
Scheffer L. K., Xu C. S., Januszewski M., Lu Z., Takemura S. Y., Hayworth K. J., et al. (2020). A Connectome and Analysis of the Adult Drosophila central Brain. eLife 9, e57443. doi:10.7554/eLife.57443
Schindelin J., Arganda-Carreras I., Frise E., Kaynig V., Longair M., Pietzsch T., et al. (2012). Fiji: an Open-Source Platform for Biological-Image Analysis. Nat. Methods 9, 676–682. doi:10.1038/nmeth.2019
Schubert F. K., Hagedorn N., Yoshii T., Helfrich-Förster C., Rieger D. (2018). Neuroanatomical Details of the Lateral Neurons ofDrosophila Melanogastersupport Their Functional Role in the Circadian System. J. Comp. Neurol. 526, 1209–1231. doi:10.1002/cne.24406
Sehadova H., Glaser F. T., Gentile C., Simoni A., Giesecke A., Albert J. T., et al. (2009). Temperature Entrainment of Drosophila's Circadian Clock Involves the Gene Nocte and Signaling from Peripheral Sensory Tissues to the Brain. Neuron 64, 251–266. doi:10.1016/j.neuron.2009.08.026
Sekiguchi M., Inoue K., Yang T., Luo D.-G., Yoshii T. (2020). A Catalog of GAL4 Drivers for Labeling and Manipulating Circadian Clock Neurons in Drosophila melanogaster. J. Biol. Rhythms 35, 207–213. doi:10.1177/0748730419895154
Shafer O. T., Helfrich-Förster C., Renn S. C. P., Taghert P. H. (2006). Reevaluation ofDrosophila Melanogaster's Neuronal Circadian Pacemakers Reveals New Neuronal Classes. J. Comp. Neurol. 498, 180–193. doi:10.1002/cne.21021
Shafer O. T., Kim D. J., Dunbar-Yaffe R., Nikolaev V. O., Lohse M. J., Taghert P. H. (2008). Widespread Receptivity to Neuropeptide PDF throughout the Neuronal Circadian Clock Network of Drosophila Revealed by Real-Time Cyclic AMP Imaging. Neuron 58, 223–237. doi:10.1016/j.neuron.2008.02.018
Shimosako N., Hadjieconomou D., Salecker I. (2014). Flybow to Dissect Circuit Assembly in the Drosophila Brain. Methods Mol. Biol. 1082, 57–69. doi:10.1007/978-1-62703-655-9_4
Song B. J., Sharp S. J., Rogulja D. (2021). Daily Rewiring of a Neural Circuit Generates a Predictive Model of Environmental Light. Sci. Adv. 7, eabe4284. doi:10.1126/sciadv.abe4284
Stengl M., Arendt A. (2016). Peptidergic Circadian Clock Circuits in the Madeira Cockroach. Curr. Opin. Neurobiol. 41, 44–52. doi:10.1016/j.conb.2016.07.010
Talay M., Richman E. B., Snell N. J., Hartmann G. G., Fisher J. D., Sorkaç A., et al. (2017). Transsynaptic Mapping of Second-Order Taste Neurons in Flies by Trans-tango. Neuron 96, 783–795. e4. doi:10.1016/j.neuron.2017.10.011
Tang X., Roessingh S., Hayley S. E., Chu M. L., Tanaka N. K., Wolfgang W., et al. (2017). The Role of PDF Neurons in Setting the Preferred Temperature before Dawn in Drosophila. eLife 6, e23206. doi:10.7554/eLife.23206
Top D., Young M. W. (2018). Coordination between Differentially Regulated Circadian Clocks Generates Rhythmic Behavior. Cold Spring Harb Perspect. Biol. 10, a033589. doi:10.1101/cshperspect.a033589
Wickham H. (2016). ggplot2: Elegant Graphics for Data Analysis. 2nd ed. Berlin, Germany: Springer International Publishing. doi:10.1007/978-3-319-24277-4
Yadlapalli S., Jiang C., Bahle A., Reddy P., Meyhofer E., Shafer O. T. (2018). Circadian Clock Neurons Constantly Monitor Environmental Temperature to Set Sleep Timing. Nature 555, 98–102. doi:10.1038/nature25740
Yoshii T., Todo T., Wülbeck C., Stanewsky R., Helfrich-Förster C. (2008). Cryptochrome Is Present in the Compound Eyes and a Subset ofDrosophila's Clock Neurons. J. Comp. Neurol. 508, 952–966. doi:10.1002/cne.21702
Yoshii T., Vanin S., Costa R., Helfrich-Förster C. (2009). Synergic Entrainment of Drosophila's Circadian Clock by Light and Temperature. J. Biol. Rhythms 24, 452–464. doi:10.1177/0748730409348551
Zerr D., Hall J., Rosbash M., Siwicki K. (1990). Circadian Fluctuations of Period Protein Immunoreactivity in the CNS and the Visual System of Drosophila. J. Neurosci. 10, 2749–2762. doi:10.1016/j.cub.2013.02.05710.1523/jneurosci.10-08-02749.1990
Zhang C., Daubnerova I., Jang Y.-H., Kondo S., Žitňan D., Kim Y.-J. (2021). The Neuropeptide Allatostatin C from Clock-Associated DN1p Neurons Generates the Circadian Rhythm for Oogenesis. Proc. Natl. Acad. Sci. U.S.A. 118, e2016878118. doi:10.1073/pnas.2016878118
Zhang L., Chung B. Y., Lear B. C., Kilman V. L., Liu Y., Mahesh G., et al. (2010a). DN1p Circadian Neurons Coordinate Acute Light and PDF Inputs to Produce Robust Daily Behavior in Drosophila. Curr. Biol. 20, 591–599. doi:10.1016/j.cub.2010.02.056
Zhang Y., Liu Y., Bilodeau-Wentworth D., Hardin P. E., Emery P. (2010b). Light and Temperature Control the Contribution of Specific DN1 Neurons to Drosophila Circadian Behavior. Curr. Biol. 20, 600–605. doi:10.1016/j.cub.2010.02.044
Zhang Y. Q., Rodesch C. K., Broadie K. (2002). Living Synaptic Vesicle Marker: Synaptotagmin-GFP. Genesis 34, 142–145. doi:10.1002/gene.10144
Zheng Z., Lauritzen J. S., Perlman E., Robinson C. G., Nichols M., Milkie D., et al. (2018). A Complete Electron Microscopy Volume of the Brain of Adult Drosophila melanogaster. Cell 174, 730–743. e22. doi:10.1016/j.cell.2018.06.019
Glossary
AME accessory medulla
AOTU anterior optic tubercle
AstC Allatostatin C
CA mushroom body calyx
contr. contralateral
CRY Cryptochrome
DenMark dendritic marker
dFB dorsal fan-shaped body
DH31 diuretic hormone 31
DN dorsal neurons
DN1a anterior dorsal neurons group 1
DN1p posterior dorsal neurons group 1
DN1p_A posterior remaining subtype of DN1p (hemibrain nomenclature), also known as vc-DN1p ()
DN1p_B anterior projecting subtype of DN1p (hemibrain nomenclature), also known as a-DN1p ()
DN2 dorsal neurons group 2
DN3 dorsal neurons group 3
EGFP enhanced green fluorescent protein
FAFB full adult female brain (electron microscopic dataset)
FB fan-shaped body of the central complex
FB2.0B UAS-Flybow2.0B; refers to the Flybow system
GFP green fluorescent protein
HA hemagglutinin
hs-Flp heat shock FLP recombinase
ICL inferior clamp
ipsi. ipsilateral
ITP ion transport peptide
LD light-dark
l-DN3 large dorsal neurons 3
LH lateral horn
l-LNv large ventrolateral neurons
5th LN 5th lateral neuron
LN lateral neurons
LNd dorsolateral neurons
LO lobula
LPN lateral posterior neurons
MDC middle dorsal commissure
ME medulla
myrGFP myristoylated GFP
nSyb neuronal Synaptobrevin
OL optic lobe
PDF Pigment dispersing factor
PDFR PDF receptor
PER Period
PI pars intercerebralis
PL pars lateralis
PLP posterior lateral protocerebrum
SAC superior arch commissure
SCL superior clamp
s-DN3 small dorsal neurons 3
SEZ subesophageal zone
s-LNv small ventrolateral neurons
SLP superior lateral protocerebrum
SMP superior medial protocerebrum
UAS upstream activating sequence
ZT Zeitgeber time
Keywords: circadian clock, Drosophila melanogaster, dorsal clock neurons, trans-tango, flybow, neuroanatomy, hemibrain, clock network
Citation: Reinhard N, Schubert FK, Bertolini E, Hagedorn N, Manoli G, Sekiguchi M, Yoshii T, Rieger D and Helfrich-Förster C (2022) The Neuronal Circuit of the Dorsal Circadian Clock Neurons in Drosophila melanogaster. Front. Physiol. 13:886432. doi: 10.3389/fphys.2022.886432
Received: 28 February 2022; Accepted: 28 March 2022;
Published: 29 April 2022.
Edited by:
Ezio Rosato, University of Leicester, United KingdomReviewed by:
Ralf Stanewsky, University of Münster, GermanySheyum Syed, University of Miami, United States
Copyright © 2022 Reinhard, Schubert, Bertolini, Hagedorn, Manoli, Sekiguchi, Yoshii, Rieger and Helfrich-Förster. This is an open-access article distributed under the terms of the Creative Commons Attribution License (CC BY). The use, distribution or reproduction in other forums is permitted, provided the original author(s) and the copyright owner(s) are credited and that the original publication in this journal is cited, in accordance with accepted academic practice. No use, distribution or reproduction is permitted which does not comply with these terms.
*Correspondence: Charlotte Helfrich-Förster, Y2hhcmxvdHRlLmZvZXJzdGVyQGJpb3plbnRydW0udW5pLXd1ZXJ6YnVyZy5kZQ==
†These authors have contributed equally to this work and share first authorship
‡Present addresses: Enrico Bertolini, Center for Integrative Genomics, Faculty of Biology and Medicine, University of Lausanne, Lausanne, Switzerland