- 1LIBM, Inter-university Laboratory of Human Movement Science, University Savoie Mont Blanc, Chambéry, France
- 2ISSUL, Faculty of Biology and Medicine, Institute of Sport Sciences, University of Lausanne, Lausanne, Switzerland
Purpose: Hypoxia is one major environmental factor, supposed to mediate central motor command as well as afferent feedbacks at rest and during exercise. By using a comparison of normobaric (NH) and hypobaric (HH) hypoxia with the same ambient pressure in oxygen, we examined the potential differences on the cerebrovascular and muscular regulation interplay during a self-paced aerobic exercise.
Methods: Sixteen healthy subjects performed three cycling time-trials (250 kJ) in three conditions: HH, NH and normobaric normoxia (NN) after 24 h of exposure. Cerebral and muscular oxygenation were assessed by near-infrared spectroscopy, cerebral blood flow by Doppler ultrasound system. Gas exchanges, peripheral oxygen saturation, power output and associated pacing strategies were also continuously assessed.
Results: The cerebral oxygen delivery was lower in hypoxia than in NN but decreased similarly in both hypoxic conditions. Overall performance and pacing were significantly more down-regulated in HH versus NH, in conjunction with more impaired systemic (e.g. saturation and cerebral blood flow) and prefrontal cortex oxygenation during exercise.
Conclusions: The difference in pacing was likely the consequence of a complex interplay between systemic alterations and cerebral oxygenation observed in HH compared to NH, aiming to maintain an equivalent cerebral oxygen delivery despite higher adaptive cost (lower absolute power output for the same relative exercise intensity) in HH compared to NH.
1 Introduction
Hypoxia is one major environmental factor of interest when considering training and performance at altitude since it mediates cerebral oxygen supply and central motor command as well as afferent feedbacks at rest and during exercise (Amann et al., 2007; Subudhi et al., 2009; Subudhi, Panerai and Roach, 2010; Vogiatzis et al., 2011; Verges et al., 2012; Goodall, Twomey and Amann, 2014; Marillier et al., 2021). Only a few studies investigated self-paced exercise when arterial oxygenation is manipulated, suggesting marginal differences (Clark et al., 2007; Beidleman et al., 2014) or similar trends (Amann et al., 2006; Périard and Racinais, 2016) in pacing between normoxia and hypoxia, despite reduced average power output in the latter. A major concern regarding these studies is that subjects were exposed to simulated altitude for only 5–40 min before time-trials. Oxygen delivery to the tissues (e.g. muscle, brain) progressively evolves in the first hours of exposure to hypoxia (e.g. time delay in changes between arterial and cerebral oxygenation (Rupp and Leti, 2013), and from a practical point of view, exposure time preceding training/competing at altitude is usually longer and prone to influence how the practitioner may feel (Luks, 2015). Altogether, pacing strategies adopted after a more prolonged exposure period (e.g. 24-h) are relevant and may strongly differ from what has been observed with short-term acute exposure (Tucker, 2009). Discrepancies in the literature regarding altered pacing in hypoxia may also result from the type of hypoxia (normobaric hypoxia NH versus hypobaric hypoxia HH) used in these models. Indeed, numerous disparities have been recently found in subjects resting and exercising at a given pressure of inspired oxygen (PiO2), but among NH or HH conditions (Faiss et al., 2013). For instance, a combination of a higher tidal volume and lower respiratory frequency leading to a higher minute ventilation in NH compared to HH (Conkin and Wessel, 2008) has been reported and markers of oxidative stress have recently been shown lower in NH compared to HH (Faiss et al., 2013; Ribon et al., 2016). The mechanisms underlying these slight physiological differences are not so clear yet. Nevertheless, we have just shown that such differences are associated with impaired global performance in HH compared to NH on a 250-kJ cycling time-trial at 3,450 m (Saugy et al., 2016). However, the extent to which underlying physiological responses driving pacing strategies would be differentially affected in HH versus NH remains unknown.
We therefore examined if there are differences in cerebrovascular regulation and muscular activation in relation to oxygen consumption, arterial saturation, cerebral and muscle hemodynamics and deoxygenation, subjective discomfort, RPE and how it would influence or be influenced by different pacing strategies during a 250-kJ cycling time-trial conducted after 24 h: 1) in normobaric hypoxia (NH) at a simulated altitude of 3,450 m, 2) in hypobaric hypoxia (HH) at a terrestrial altitude of 3,450 m and, 3) in normobaric normoxia (NN) as a control condition. We hypothesized that there might be subtle differences in the regulation of oxygen delivery to the brain between NH and HH, inducing different pacing strategies and consequently, the supposed better endurance performance in NH, when compared to HH. It was also anticipated that SpO2 and cerebral hemodynamics would be more affected in HH, compared to NH, leading to suboptimal pace management and a greater performance decrement. By comparing cerebrovascular and brain versus muscle deoxygenation time-course during self-paced endurance exercise in NH versus HH, we aim to better understand how exercise is regulated in hypoxia and to explore new mechanisms on the interplay between cerebrovascular and muscular regulation in humans.
2 Methods
Experimental design and partial parts of the methods have already been presented in a previous paper (Saugy et al., 2016) focusing on global exercise performance in HH versus NH but with no mention to the present interests (i.e., exercise intensity regulation and underlying cerebrovascular and muscular regulation). For the convenience of the reader, key methodological information is redefined in the present paper.
2.1 Subjects
Sixteen healthy, trained male subjects volunteered to participate to this study (mean ± SD; age 34.7 ± 9.5 years, body weight 75.2 ± 7.2 kg, height 180 ± 6 cm, maximal oxygen consumption VO2max 60.2 ± 9.9 ml kg−1 min−1). Participants were all experienced (recreational or competitive but not elite) cyclists. Written informed consent was obtained from each participant before participation. Subjects were non-smokers, and neither acclimatized nor recently exposed to altitude. All procedures conformed to the standards set by the Declaration of Helsinki and the study was approved by a Medical Ethics Committee (Commission Cantonale Valaisanne d’Ethique Médicale, CCVEM; Agreement 051/09).
2.2 Experimental design
The experimental design consisted in a preliminary visit and three testing sessions. During the first meeting to the laboratory, subjects completed the baseline anthropological measurements and filled the consent form. Participants then performed 1) a maximal incremental exercise test (FiO2: 0.21; 60 W + 30 W min−1) to determine VO2max and peak workload on a braked cycle ergometer including a Powertap sensor (Cycleops IC 400 Pro, Madison, Wisconsin, United States); and 2) a familiarization test with the 250-kJ time-trial on the same ergocycle.
The experimental design was then composed of three different sessions in a randomized order separated by at least 12 days. One session was performed in a hypobaric hypoxia (HH) environment at the Altitude Research Station in Jungfraujoch (3,450 m, FiO2 of 20.9%, BP of 481.8 ± 4.7 mmHg, PiO2 of 90.9 ± 1.0 mmHg, temperature of 21.3 ± 0.6°C, humidity of 45.1 ± 8.3%). Two sessions were conducted in a hypoxic chamber (ATS Altitude, Sydney, Australia) built in our laboratory (Sion, 485 m, Switzerland). Temperature inside the chamber was maintained constant by an internal air conditioning system. One of the two sessions completed in the chamber was performed in normobaric hypoxia (NH) with a FiO2 of 13.6% (BP of 715.8 ± 3.8 mm Hg, PiO2 of 91.0 ± 0.6 mmHg, a temperature of 22.7 ± 0.8°C, and a humidity of 41.0 ± 4.8%) corresponding to a simulated altitude of 3,450 m. The other session was performed in normobaric normoxia (NN) with a FiO2 of 20.9% (BP: 718.1 ± 3.3 mmHg, PiO2 of 140.5 ± 0.6 mmHg, a temperature of 23 ± 1°C, and a humidity of 42.8 ± 4.4%.). These parameters were controlled regularly with an electronic device (GOX 100 oximeter, Greisinger, Regenstauf, Germany). In order to blind subjects to altitude, the system was also running normoxic airflow into the chamber during the NN sessions.
Each session consisted of 26 h of exposure in each condition (NN, NH, HH) in a randomized blinded order. After 24 h of exposure, subjects completed a self-paced 250 kJ time-trial, being free to increase/decrease the resistance to adjust the workload as they became familiar with it in the preliminary session (up/down electronic shifter at the handlebar). All trials were preceded by a rest period of 5 min, followed by 3 min warm-up period at 70 W. Setting (bike dimensions and braking resistance) was individualized but strictly identical between the three conditions for each subject. The participants were instructed to complete the 250 kJ as rapidly as possible. Work progressively completed from 0 to 250 kJ was the only information (visually) provided to subjects during the time-trial. With regard of the participants expertise in cycling and their VO2max, it was expected that the self-paced exercise would last 15–20 min in normoxia. In order to prevent excessive thermal stress, a fan providing a high wind speed was placed directly in front (∼80 cm) of the subjects during the trials.
Particular attention was given to the standardization and the control of the conditions. The participants were asked to maintain their usual training and physical activities during the whole experimental protocol to avoid fitness changes between sessions. Similar standardized meals were provided at the same time under each condition. The bedding was similar among conditions and sleep quality was not different between HH and NH (Saugy et al., 2016). The daily schedule and activities were exactly the same for all the conditions and the time trial was performed at the same time of the day in each condition.
2.3 Measurements
2.3.1 Gas exchanges, heart rate, SpO2 and perceptual variables
Breath-by-breath pulmonary gas exchange (oxygen uptake, VO2; carbon dioxide production and end-tidal carbon dioxide partial pressure, PETCO2), minute ventilation (
Subjects were regularly asked to qualify their rate of perceived exertion (RPE, Borg Scale, 6–20) during the time-trial. Legs and breathing feelings were also assessed with visual analog scales (VAS, 0–10), ranging from “no difficulty” to “extremely difficult”.
2.3.2 Power, workload and cadence
Power output, speed, cadence and total workload were continuously recorded during the time-trial by the cycle ergometer (Cycleops IC 400 Pro, Madison, United States).
2.3.3 Electromyographic recordings
Quadriceps electromyography (EMG) was continuously recorded from the right vastus lateralis (VL) using bipolar silver chloride surface electrodes of 10-mm diameter (Kendall Meditrace 100). Electrodes were taped lengthwise on the skin over the muscle belly following SENIAM recommendations, with an interelectrode distance of 20 mm. Positions of the electrodes were marked on the skin to ensure precise replacement in other sessions. Reference electrode was attached on the patella. Low impedance (<10 kΩ) at the skin-electrode was obtained by shaving and abrading the skin with an abrasive sponge and cleaning with alcohol. EMG data were recorded at 2 kHz with Biopac system (MP150, Biopac System, Goleta, United States) and amplified with a bandwidth frequency ranging from 10 to 500 Hz. For data analysis, the integral of the EMG activity was calculated over 10-kJ time-periods throughout time-trial using the formula:
where m is the raw EMG signal.
2.3.4 Cerebrovascular variables
Mean middle cerebral artery blood flow velocities (MCAv) were measured bilaterally using a 2-MHz pulsed Doppler ultrasound system (ST3, Spencer technology, Seattle, United States). The Doppler ultrasound probes were positioned over right and left temporal windows and held firmly in place with an adjustable headband (Marc 600 Headframe, Spencer technology). The signals were at depths ranging from 44 to 58 mm. Signal quality was optimized using an M-mode screen shot and insonation depth, probes and headband locations were marked to ensure within-subject repeatability. Bilateral MCAv were averaged to represent an index of global cerebral blood flow at rest and during exercise. Cerebral O2 delivery (cDO2) before and during exercise was calculated using the equation: cDO2 = mean MCAv x CaO2, where CaO2 refers to the oxygen content of the arterial blood estimated as follows: CaO2 = [hemoglobin concentration assessed in each condition after 20 h of exposure x 1.36 x current SpO2/100], oxygen dissolved in plasma being neglected. cDO2 was then expressed as a percentage of the resting normoxic (NN) pre-exercise value.
2.3.5 Near-infrared Spectroscopy measurements
Cerebral oxygenation in the left prefrontal (PFC) and motor (MC) cortex was assessed by monitoring changes in oxy- and deoxy-hemoglobin (O2Hb and HHb, respectively) obtained with spatially resolved, continuous wave near-infrared spectroscopy (NIRS) (Oxymon MkIII, Artinis, Zetten, Netherlands). Theoretical and performance details of NIRS have been previously described (Perrey, 2008). PFC NIRS probes were centered between Fp1 and F3 locations according to the international 10–20 EEG system, with 3.5-cm interoptode distance. MC NIRS data were expressed as the average of a 4-channel square setting (3-cm interoptode distance) fixed with headbands between Cz and C3 locations. Muscle oxygenation was assessed from the right vastus lateralis (at mid thigh) using a 4-cm interoptode distance. For PFC and muscle, probe holders were secured to the skin using double-sided adhesive tape to minimize any change in its relative position and all optodes were covered with black sweatbands for them to be shield from ambient light. Total hemoglobin changes (THb = O2Hb + HHb) were calculated to reflect the changes in tissue blood volume within the illuminated areas and difference in hemoglobin (HbDiff = HbO2 - HHb) was calculated as a reliable estimator of change in tissue (de-) oxygenation status (Rooks et al., 2010). NIRS data were recorded at 10 Hz, filtered with a 2-s moving Gaussian window smoothing algorithm and expressed as relative changes (∆μmol) from the stable baseline preceding each time-trial.
2.4 Statistics
Data are reported as means and standard deviations with 95% confidence intervals. Data were tested for equality of variance (Fisher-Snedecor F-test) and for normality (Shapiro-Wilk test). To investigate the pacing strategies, we divided the time-trial in 25 slices of 10 kJ (increments of 4% of the total work completed). One-way ANOVA were used to determine if systemic (SpO2, VO2) and cerebrovascular (MCAv, cDO2) variables were different between conditions before the time-trial (i.e., at baseline, BL). When a significant main effect was found, Bonferroni post-hoc tests were used to localize differences between conditions (NN, NH, HH). Two-way ANOVA (condition x time) with repeated measures were used for each parameter during the time-trial. When significant main or interaction effects were found, Bonferroni post-hoc tests were used to localize differences between conditions (NN, NH, HH) and/or time (each 10 kJ slice from 0 to 250 kJ). Null hypothesis was rejected at p < 0.05. All analyses were made using Sigmaplot 11.0 software (Systat Software, San Jose, United States).
3 Results
3.1 Time-trial performance and pacing
Time-trial performance data are summarized in Table 1. Compared to NN (i.e., 1,041 ± 151 [955.9–1,126.9] s), the mean time required to complete 250 kJ was 24.1 ± 9.6 [18.7–29.5] % and 33.2 ± 12.4 [26.2–40.2] % higher for NH and HH, respectively (both p < 0.001, cf. Supplementary Material). The mean time was 7.5 ± 7.5 [3.2–11.7] % higher in HH than in NH (p < 0.01). Compared to NN the whole time-trial pace was reduced for both hypoxic conditions (Figure 1A, p < 0.001). The HH power output was significantly reduced compared to NH from 140 to 220 kJ (p < 0.05). When expressed as a function of the average power sustained in each respective condition (Figure 1B), an interaction effect was observed (p < 0.05) showing inverse trends between NN and both NH and HH, despite a comparable range of variation (∼30%, ∼21 and ∼23%, for NN, NH and HH, respectively). In HH, normalized power output followed a similar pattern to NH. Between 85% and the end of the time-trial (so called “final burst” or “end-spurt”), the relative increase in power output was 9, 18 and 21% in NH, HH and NN, respectively.
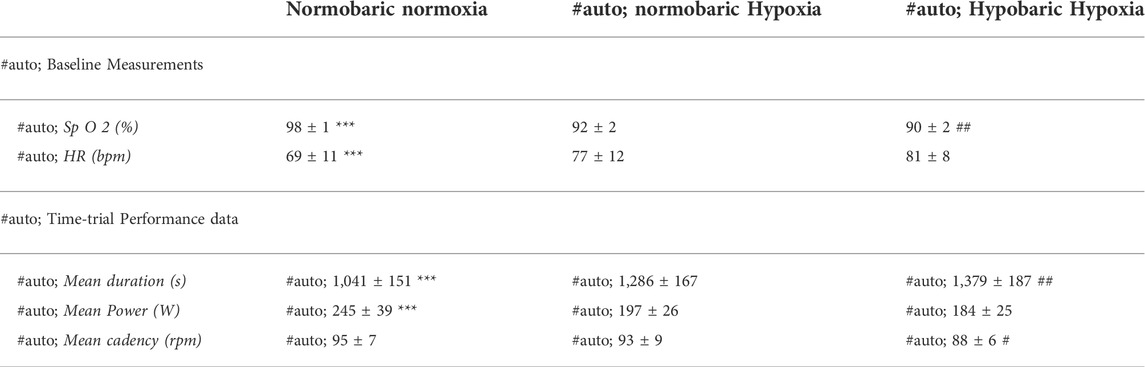
TABLE 1. Baseline physiological measurements before exercise and performance results during the self-paced 250-kj time-trial in normobaric normoxia (NN), normobaric hypoxia (NH) and hypobaric hypoxia (HH). ***p < 0.001 for difference between NN and both hypoxic conditions; ##p < 0.01 for difference between NH and HH.
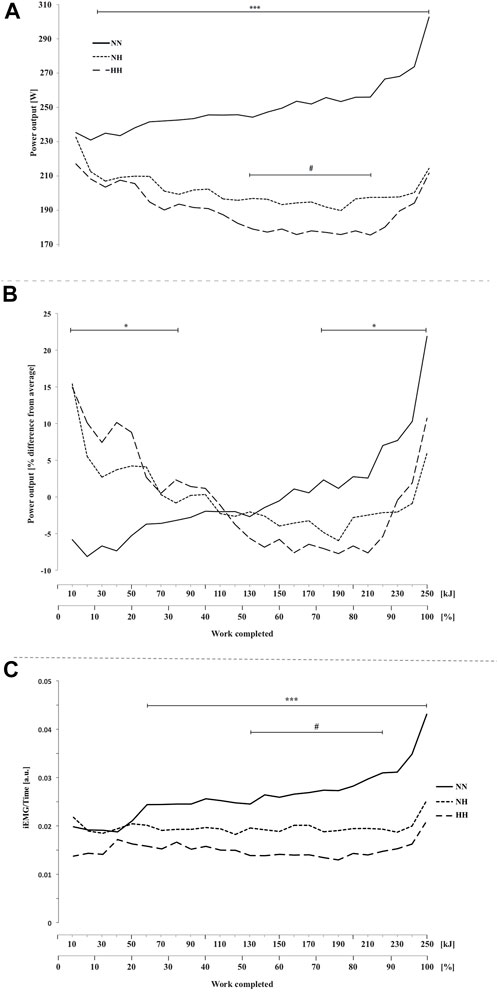
FIGURE 1. Power output (A), normalized power output (B) and electromyographic activity (iEMG) measured for the vastus lateralis (C) during the self-paced 250-kJ time-trial in normobaric normoxia (NN), normobaric hypoxia (NH) and hypobaric hypoxia (HH). *p < 0.05, ***p < 0.001 for difference between NN and both hypoxic conditions; #p < 0.05 for difference between NH and HH.
3.2 Pulse oxygen saturation
As presented in Figure 2A, SpO2 was significantly higher at baseline (97.7 ± 1.2 [97.1–98.4] %; p < 0.001) and during cycling in NN compared to both hypoxic conditions (0–250 kJ; p < 0.001) and SpO2 was significantly lower in HH compared to NH at baseline (92.2 ± 2.1 [91.1–93.3] vs 89.9 ± 1.9 [88.9–91.1] %; p < 0.01) and during the first half of the time-trial (0–140 kJ; p < 0.05).
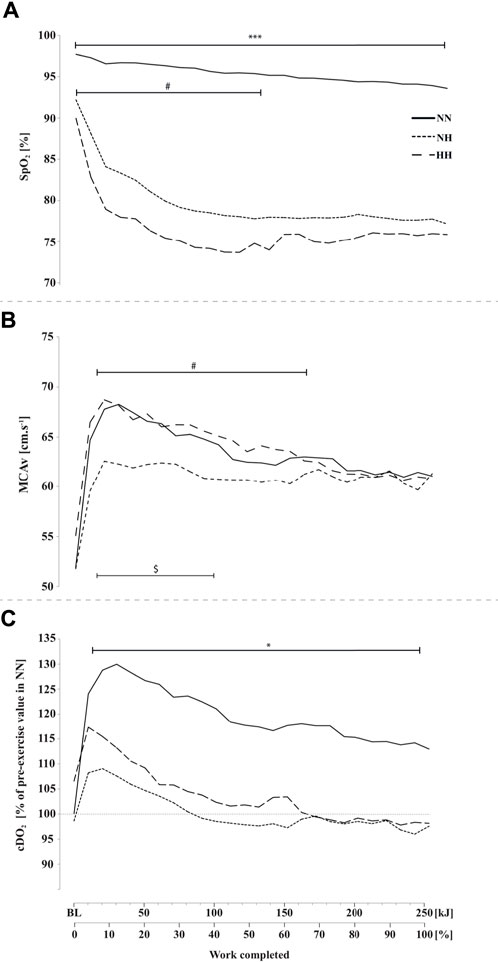
FIGURE 2. Peripheral oxygen saturation (SpO2, panel (A), middle cerebral artery blood flow mean velocity (MCAv, panel (B) and cerebral oxygen delivery cDO2, panel (C) during the self-paced 250-kJ time-trial in normobaric normoxia (NN), normobaric hypoxia (NH) and hypobaric hypoxia (HH). *p < 0.05, ***p < 0.001 for difference between NN and both hypoxic conditions; $p < 0.05 for difference between NN and NH; #p < 0.05 for difference between NH and HH.
3.3 Cerebrovascular variables
MCAv at baseline was not different between conditions. MCAv was increased in the first quarter of the time-trial in both NN, NH and HH (+31%, +15% and +25%, respectively), the increase being lower in NH compared to HH (from 10 to 150 kJ, p < 0.05) and NN (from 10 to 100 kJ, p < 0.05) while no difference was found along time-trial between HH and NN (Figure 2B), where MCAv decreased from 40 kJ onward. Baseline cDO2 values were not different between conditions (Figure 2C). In contrast, during time-trial cDO2 was lower in both NH and HH (on average, by 17%) compared to NN (p < 0.05), with no significant difference between NH and HH. In the latter conditions cDO2 decreased to near baseline values at ∼100 kJ while it remained elevated in NN (p < 0.05).
3.4 Cardio-respiratory parameters
VO2 was higher for NN compared to both hypoxic conditions (from 70 to 250 kJ; p < 0.001) and trend to be lower for HH compared to NH before the end-spurt (from 140 to 210 kJ; p = 0.08) (Figure 3A). No difference was observed between conditions in VO2 relative to VO2max (Figure 3B). Heart rate was higher at baseline for both hypoxic conditions (78 ± 12 [71–84] and 81 ± 8 [76–85] bpm for NH and HH, respectively) compared to NN (69 ± 11 [62–75] bpm) but no difference was observed during the time-trial (Figure 3C). PETCO2 at baseline was lower for NH (26.8 ± 2.9 [25.1–28.4] mmHg) than NN (30.6 ± 3.3 [28.7–32.5] mmHg; p < 0.05) and HH (29.9 ± 2.5 [28.5–31.4] mmHg; p < 0.05), but no statistical difference was reached during the time-trial (Figure 3D).
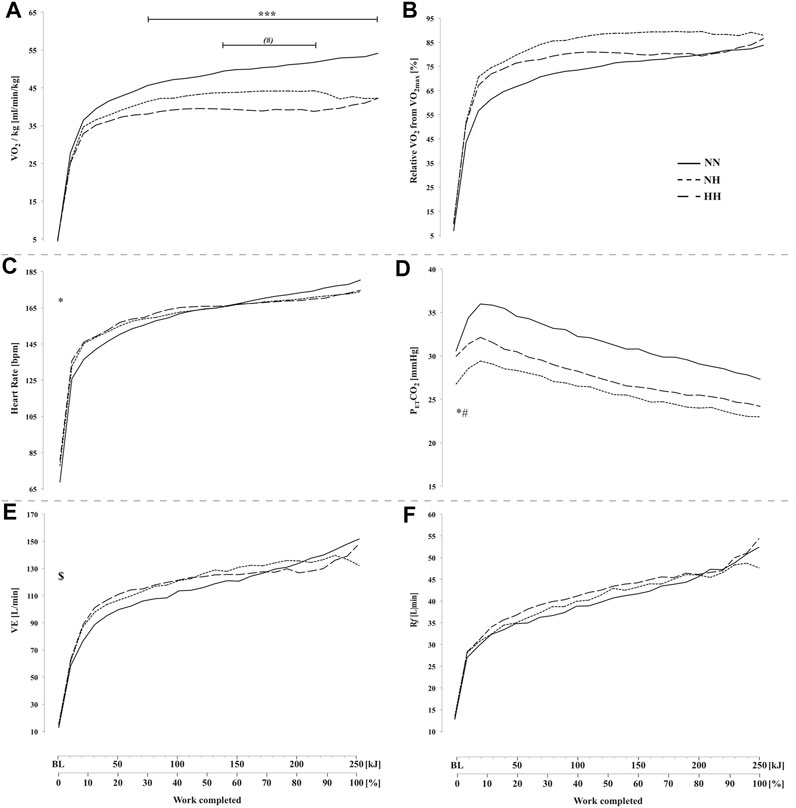
FIGURE 3. Absolute oxygen uptake VO2, panel (A), oxygen uptake relative to VO2max panel (B), heart rate (panel (C), end-tidal CO2 pressure (PETCO2, panel (D), minute ventilation (
3.5 Cerebral oxygenation
These results are presented in Figures 4A–F. PFC and MC HbDiff were higher for NN than for the two hypoxic sessions throughout the time-trial (p < 0.001, Figures 4A,B). Lower values were found in HH compared to NH in PFC HbDiff at the beginning of the time-trial (20–120 kJ, p < 0.05, Figure 4A), while higher values were observed in HH compared to NH in MC HbDiff in the second part of the time-trial (160–240 kJ, p < 0.05, Figure 4B). Exercise induced an increase in cerebral HHb that was lower for NN than the two hypoxic conditions in both PFC and MC (p < 0.001, Figures 4C,D). This increase was particularly limited during the first half of the time-trial in NN. During the second half of the time-trial, both MC HbDiff and HHb tended to “plateau”. MC HHb was also slightly lower for HH compared to NH in this last part of exercise (p < 0.05). Increase in cerebral THb along exercise was similar in the three conditions in PFC (Figure 4E) but was higher for NN in MC during the second half of the time-trial (from 100 kJ onward, p < 0.001, Figure 4F) compared to the increase observed in both hypoxic conditions.
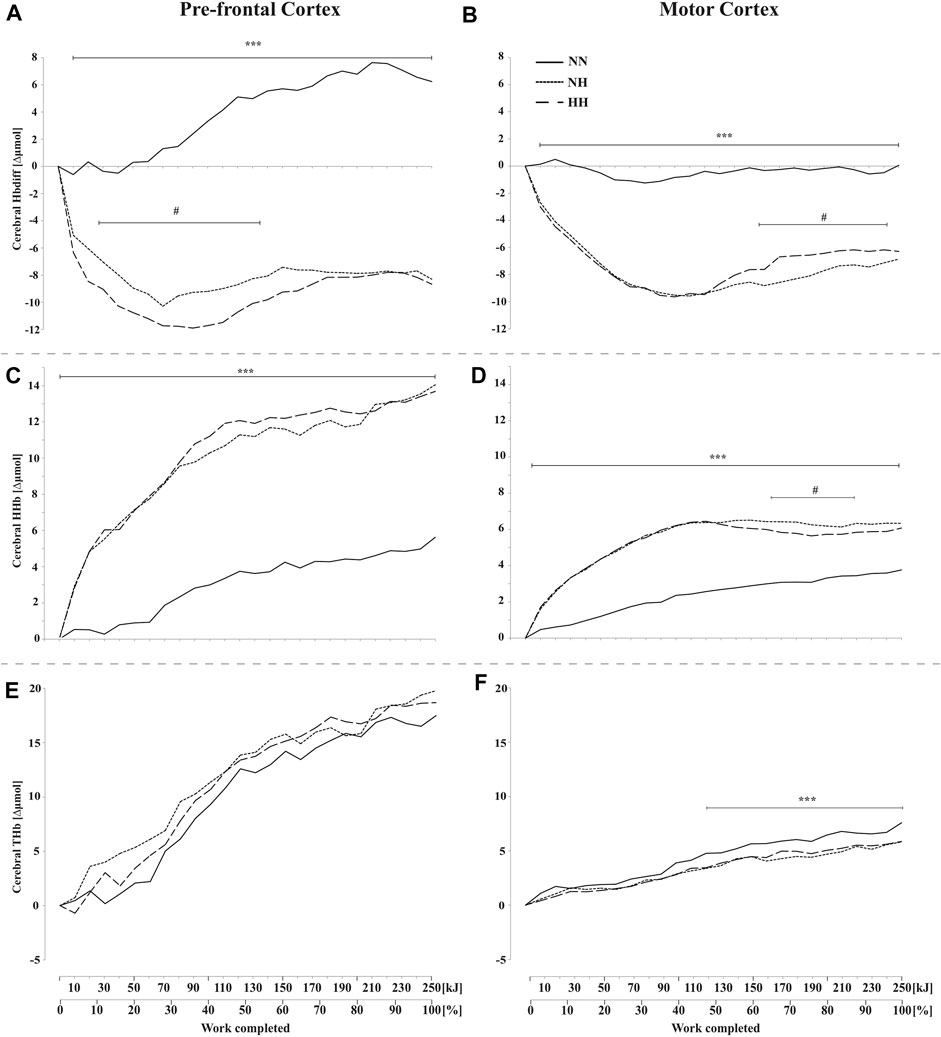
FIGURE 4. Mean changes in cerebral hemoglobin difference (HbDiff = O2Hb-HHb), deoxy-(HHb), and total-hemoglobin (THb), during the self-paced 250-kJ time-trial in normobaric normoxia (NN), normobaric hypoxia (NH) and hypobaric hypoxia (HH). Data are shown for the prefrontal cortex (PFC, panels (A,C,E)) and for the motor cortex (MC, panels (B,D,F)). ***p < 0.001 for difference between NN and both hypoxic conditions; #p < 0.05 for difference between NH and HH.
3.6 Muscle oxygenation
Muscle HbDiff was lower for NH compared to NN from 20 to 210 kJ (p < 0.05) and lower for NN compared to HH from 190 to 240 kJ (p < 0.05) (Figure 5A). HbDiff was also lower in NH compared to HH from 50 kJ onward (p < 0.05). No difference has been found in muscle HHb increase during time-trials between conditions (Figure 5B). Muscle THb was higher in HH compared to both NN and NH conditions from 90 kJ onward (p < 0.05) (Figure 5C), with no difference between NN and NH.
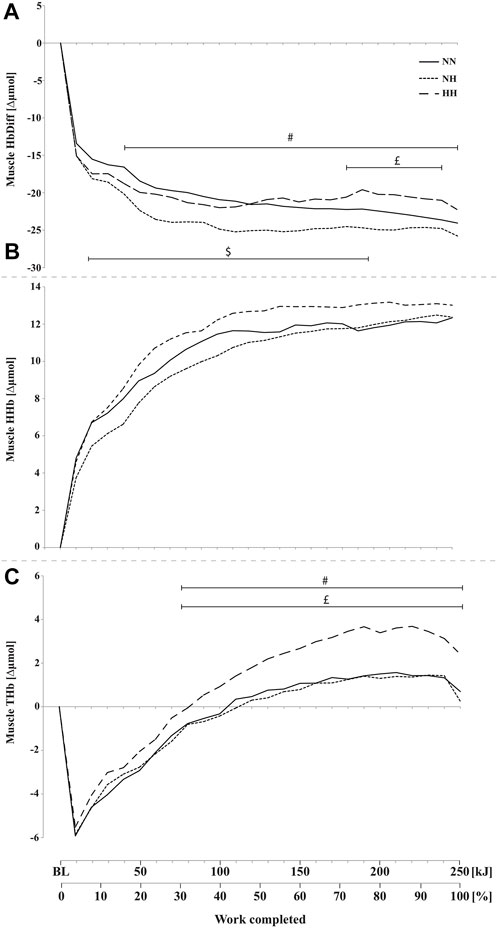
FIGURE 5. Mean changes in muscle hemoglobin difference (HbDiff = O2Hb-HHb, panel (A), deoxy-(HHb, panel (B), and total-hemoglobin (THb, panel (C), during the self-paced 250-kJ time-trial in normobaric normoxia (NN), normobaric hypoxia (NH) and hypobaric hypoxia (HH) for the vastus lateralis. $p < 0.05 for difference between NN and NH; £p < 0.05 for difference between NN and HH; #p < 0.05 for difference between NH and HH.
3.7 Electromyographic activity
EMG activity was higher for NN compared to both hypoxic conditions from 60 kJ onward (Figure 1C, p < 0.001) and it was lower for HH compared to NH from 130 to 210 kJ (p < 0.05).
3.8 Subjective feelings
Perceived exertion (Figure 6A) and legs discomfort (Figure 6B) were not different between conditions along cycling. However, breathing discomfort (Figure 6C) was higher for both hypoxic conditions from the beginning to the end of the time-trial (p < 0.001, with no difference between NH and HH. In addition, a biphasic evolution from 0 to 70 kJ (i.e. improvement or plateau) and then from 70 to 250 kJ (i.e. worsening) was observed with a time effect on both legs and breathing discomfort (both p < 0.001, Figures 6B,C).
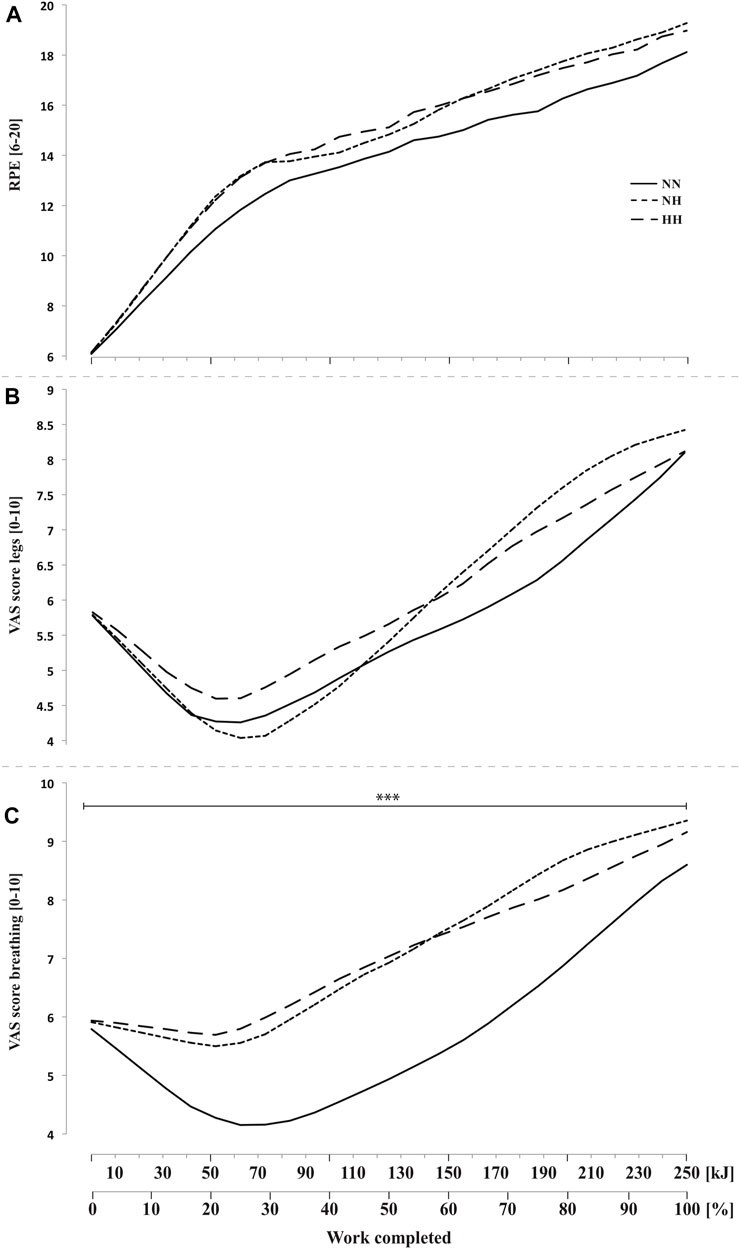
FIGURE 6. Rate of perceived exertion (RPE, panel (A) and visual analog scale (VAS) scores for legs feelings (panel (B) and breathing discomfort (panel (C) during the self-paced 250-kJ time-trial in normobaric normoxia (NN), normobaric hypoxia (NH) and hypobaric hypoxia (HH). ***p < 0.001 for difference between NN and both hypoxic conditions.
4 Discussion
This study was designed to investigate time-trial performance in conjunction with systemic, muscle and cerebrovascular variables after a 24-h exposure to hypoxia. The major findings were that, for the same pressure in oxygen, there were subtle differences in cerebrovascular regulation during an endurance exercise performed in normobaric versus hypobaric hypoxia likely resulting in differences in pacing and total performance. Moreover, there was an inverse and more variable pacing pattern in hypoxia compared to normoxia, in conjunction with depressed cerebrovascular function for a same relative intensity of exercise (i.e., %VO2max, HR,
Pacing in hypoxia versus normoxia
Hypoxia-induced impairments of aerobic performance have been extensively studied by the past but scarce studies have described how pacing strategy (i.e., self-selected distribution of power output or energetic reserves) may help to understand why we fatigue when oxygen availability becomes challenging.
Due to the experimental design, participants started exercise at the same workload (∼226 W) in normoxia and hypoxia but acutely reduced power output until end-spurt in hypoxia whereas a slight positive trend was seen in normoxia over the same period. Variation in power outputs (as a function of mean power during the trial) from the start to 85% of time-trial was twice higher in hypoxia compared to normoxia (∼22 and ∼11%, respectively).
Previous reports examined exclusively time-trials performed after very short exposure to hypoxia, ranging from only 3–40 min (Clark et al., 2007; Fan and Kayser, 2013; Périard and Racinais, 2016) up to 2 h (Beidleman et al., 2014). Sudden and acute hypoxic exposure provides an interesting model that stresses oxygen transport systems but in real-world training/competing (particularly at terrestrial altitude), exposure time preceding exercise is usually longer and is prone to deeply influence how pace would be regulated (Tucker, 2009). We previously showed that the first hours at altitude progressively affect motor cortical excitability (Rupp et al., 2013a) or muscle and cerebral oxygenation (Rupp and Leti, 2013) for instance. In the present experiment, the prooxidant/antioxidant balance became impaired from 10 h in hypoxia (Ribon et al., 2016), hematologic parameters were affected after 20 h in hypoxia (Saugy et al., 2016) and sleep quality was significantly disturbed in hypoxia the night before time-trial (Heinzer et al., 2016). This might, at least partly explain why available evidence suggests that the acute reduction in both maximal aerobic power and endurance performance is maximal within 16–24 h of exposure (Schuler et al., 2007), and resorbs progressively thereafter.
Initial phase. The intensity set at the start of the time-trial in hypoxia led to a rapid decrease in power output over that period (from start to kJ 70), with a 15% decrease in normalized power output compared to a +3% variation only in normoxia (Figure 1A). Interestingly, similar rate of increase in RPE were observed in all conditions in the present study in the first 30% of completed work (0–70 kJ). These RPE were however generated from a very different balance between central drive and afferent feedbacks (physiological adaptations) in normoxia and hypoxia. A wide range of variables can influence pacing but recently, tactical adaptations in the chosen pacing strategies have been incredibly manipulated with the use of central nervous system drugs and selective block of the central projection of ascending sensory pathways (e.g., fentanyl, opioid analgesic) (Amann et al., 2009), so that the understanding of the role of neurophysiological processes has grown.
Cortical representation of muscle involved in cycling is served dominantly by MCA (Jorgensen, Perko and Secher, 1992). Transcranial Doppler can provide quantitative information on cerebral hemodynamic changes at the macrovascular level (i.e., cerebral arteries) but is unable to assess directly the qualitative repercussions of such changes for the tissue at the microvascular level. How much reductions in SpO2 and cDO2 translate into changes in cerebral tissue oxygenation when self-paced exercise is performed in hypoxia remains largely unknown. Hence, NIRS is increasingly used to measure the (mis)balance between oxygen supply and utilization directly in tissue micro-vessels (venules, arterioles and capillaries), with a predominant venous contribution (70–80%) (Hamaoka et al., 2007).
We found that the cerebrovascular responses in NN over the first part of exercise appeared appropriate (e.g., cDO2 maintained at ∼125% from baseline value, preserved/increased brain oxygenation), while it is likely that the brain function was threatened in hypoxia over that period from a rapid decrease in SpO2, cDO2 (Figures 2A,C) and cerebral oxygenation towards low levels (Figures 3A–D).
Main phase. From 30 to 85% of the time-trial mean power output difference between NN and both hypoxic conditions continued to grow (Figure 1A), still without any difference in RPE (Figure 6) and relative exercise intensity as expressed from %VO2max (Figure 3B) HR (Figure 3C) or ventilation parameters (Figures 3E,F). The lower work-rate in hypoxia was achieved by the alteration in the degree of skeletal muscle recruitment (i.e., lower iEMG) (Figure 1C) in accordance with Peltonen et al. (Peltonen et al., 1997). This is corroborated for the first time by a concomitant significant cerebral hypoperfusion at the micro-vascular level (from THb with NIRS, Figure 4F) over the motor cortex, which directly drives the muscles.
Synthesizing information from a wide range of brain systems and exerting control over cognitive and executive behavior (e.g. sensory information integration, decision-making, movement planning, pacing strategies and motivation), PFC associative areas play a central role in the orchestration of thoughts and actions in accordance with internal goals (Ramnani and Owen, 2004) (of particular interest in our study), but the central motor drive is ultimately conducted from the premotor and primary motor areas, which have never been investigated during self-paced exercise before. From the study of Subudhi et al. (Subudhi et al., 2009) it has been often argued that there is a good correlation between prefrontal, premotor and motor cortices oxygenation measurements during exercise. However, this has been shown during a short, maximal incremental exercise, where pacing strategy was minimal as power output was compelled throughout the test. We recently demonstrated that PFC and MC oxygenation profiles can differ during submaximal fatiguing exercise (Rupp et al., 2013b) and to our knowledge the present study is the first to present simultaneous macro-circulation in MCA and both PFC and MC micro-hemodynamics and oxygenation during a self-paced exercise.
Here, PFC and MC oxygenation were both markedly depressed in hypoxia from the start of the time-trial (Figures 4A–D), confirming what has been reported before almost exclusively during progressive maximal exercises in hypoxia and in the PFC (Subudhi et al., 2009; Vogiatzis et al., 2011; Marillier et al., 2021). Presumably, the traditionally-observed exercise-induced increase in cerebral cortex oxygenation reflects progressive increase in oxygen metabolic demand with increased neuronal networks activation. Despite lower central drive and muscle activity during time-trial in hypoxia, it is likely that the challenging environmental conditions (cf., low FiO2) blunted the ability of the neurovascular coupling to increase or even maintain cerebral oxygenation to habitual levels aiming at preserving a positive balance between oxygen supply and consumption. An hypothesis might be that power output is rapidly then steadily decreased in hypoxia to prevent the body to be exposed to unacceptable levels of SpO2, cDO2 and cerebral oxygenation or, at least, to values that would be considered as incompatible with the remaining expected time before exercise completion. The diminished power output certainly allowed cDO2 and cerebral hemodynamics not to decrease further (e.g. under baseline values for cDO2). Of interest is that both PFC and MC HbDiff plateaued in hypoxia during the second half of the time-trial while the rate of HHb increase was lower. On the other hand it is important to stress that PFC and MC oxygenation would be only one of many afferent signals influencing complex regulation of motor drive during self-paced exercise (St Clair Gibson and Noakes, 2004).
In the present study, CBF declined in both NN and HH despite increased versus decreased power output, respectively. This adaptation mirrors the PETCO2 decrease over the same period (Figure 3D) and might thus be triggered by hyperventilation-induced hypocapnia (cf. Figures 3E,F). This assumption is corroborated by previous results who observed a similar decrease in MCAv during a 750-kJ time-trial in normoxia (Périard and Racinais, 2016). Conversely, other results demonstrated a maintain MCAv (in normoxia) or an increased MCAv (and almost maintained cDO2) in hypoxia during a 15-km time-trial (Fan and Kayser, 2013). To explain these results, authors underlined a higher RPE during the time-trial in hypoxia (5,000 m) and suggested a greater sensorimotor activation compared to normoxia.
End-spurt. In accordance with well-known field observations and as previously described in the literature (Roelands et al., 2009) our results indicated a characteristic end-spurt phenomenon in the last 10–15% of the time-trial. This end-spurt was seen whatever the condition (Figure 1B). Our results confirm that the subjects have the drive and/or motivation to augment power output when approaching the end-point in normoxia but also in hypoxia (what is not the case anymore after administration of a serotonin reuptake inhibitor in normoxia) (Roelands et al., 2009).
Normobaric versus hypobaric hypoxia
When comparing HH and NH, it should be noted that power output at the immediate onset of the time-trial (0–20 kJ, 8%) was similar and also similar to NN, suggesting that the initial selection of work rate was more likely based on previous experience and expectations of exercise duration, rather than on an instantaneous (baseline) afferent input from hypoxemic or disturbed organ/tissue homeostasis (e.g., modified baseline HR, SpO2, PETCO2). Indeed, even the knowledge of the condition (no possibility to blind HH) had no influence on the initial power output, and we recall participants had no feedback on their power output throughout the time-trials. However, the tendency towards lower power output in HH in the first part of the time-trial appeared significant in the second part (from ∼30% of total work onward). Trends and normalized variation in power output (Figure 1A) were similar in HH and NH throughout the time-trial, but for the first time we were able to identify subtle differences in underlying physiological adaptations (i.e., cerebrovascular responses) with a potential explanation of the lower performance in HH.
Part of the explanation may arise from distinct baseline status in HH and NH after the 24-h exposure; time-window of particular interest with regards to the early signs of AMS for instance (Subudhi, Panerai and Roach, 2010), which are known to be correlated to the degree of hypoxemia (Fulco et al., 2011). Accordingly, baseline SpO2 before the time-trial was significantly lower in HH despite similar PiO2 (Figure 2A). This might have impacted subjective feelings (e.g. RPE, leg and ventilation discomforts) during exercise so that power output had to be further reduced in HH compared to NH to match an equivalent relative exercise intensity (%VO2max, HR, …). Besides, underlying mechanisms associated with the same relative exercise intensity showed differences, likely explaining lower power output in HH in the second half (140–220 kJ, corresponding to 55–85%) of the time-trial duration.
Mass oxygen delivery to the brain is dependent on cerebral blood flow (cDO2 assessed from MCAv in the present study) and on arterial content in oxygen, which is a function of hemoglobin concentration and arterial saturation in oxygen. It seems consistent from the literature to report that the rate of cDO2 is an important information integrated by the central nervous system to regulate central drive. We demonstrated that cDO2 was extremely reduced in both HH and NH compared to NN, already in the first part of the time-trial. It is particularly interesting to see how the progressive impairments in power output mirrored the decrease and plateau in cDO2, conceivably preventing any further decrease under dramatically low values in hypoxia. This was observed in a very similar extent in both HH and NH but we show here for the first time that the comparable cDO2 levels achieved, resulted from distinct mechanisms in NH and HH (cf. SpO2-MCAv profiles) and were seen in conjunction with significantly different cerebral oxygenation states in both conditions.
First SpO2 was significantly lower at rest and during the first half of the time-trial in HH compared to NH, what may explain 1) the higher hemoconcentration measured before exercise in HH (+6%, (Saugy et al., 2016)) as a compensatory mechanism and, 2) the higher MCAv values in HH (+7.5% over 10–150 kJ) throughout exercise likely due to a higher hypoxia-induced cerebral vasodilation (Casey and Joyner, 2012). At the same time, exercise-induced decrease in PETCO2 was approximately the same in NN, NH and HH (-8 mmHg on average over 20–250 kJ with similar
However, impaired cDO2 is only part of the equation (i.e., reduction in the ability of CNS to voluntarily activate skeletal muscle, (Goodall, Twomey and Amann, 2014)) and explains mainly the decreased performance in both NH and HH compared to NN. Cerebral oxygenation kinetics described in the present study also help to understand how and why pacing might be more down-regulated in HH compared to NH. From our data, one may speculate that a comparable central drive was produced in HH and NH in the initial part of the time-trial (e.g., same MC deoxygenation, iEMG, power output, muscle deoxygenation, absolute VO2). However, PFC deoxygenation was significantly higher in HH (Figure 4A) during that period. As cDO2 was the same in HH and NH along the time-trial, the lower PFC oxygenation may have resulted from higher extraction rate of oxygen (i.e., higher neuronal activity) in this part of the brain. Whatever the explanation (e.g., greater integrative process, higher planning activity), the fact is that lowering power output in HH allowed PFC HbDiff to progressively “restore” to NH levels. Finally, these observations explain similar PFC activity in the second part of the time-trial for lower power output in HH than in NH. Consistently (from 55 to 85% of the total duration, prior end-spurt), the lower power output in HH vs. NH was seen in conjunction with a lower degree of muscle recruitment (i.e., lower MC HHb, lower muscle deoxygenation and blood perfusion, lower iEMG).
Methodological considerations
Some limitations inherent to NIRS measurement should be noted. Part of the detected NIR light may be affected by the changes in optical properties of superficial tissue layers between the optode and the investigated tissue (e.g. scalp and skull for the brain; skin and fat for the muscle) (Takahashi et al., 2011). We sought to minimize the effects of near-surface blood flow in the observed chromophore concentration changes by controlling room air temperature, by giving attention to ensure NIRS setup to be non-compressive and by using enlarged inter-optode distances to reach the maximal light path providing a sufficient signal-to-noise ratio of the optical density measurements (Rolfe, 2000). Moreover, CBF may be heterogeneously distributed at exercise and under hypoxia (Pagani et al., 2011), we investigated MCA macrocirculation plus multiple sites of interest by NIRS and we acknowledge that observed tissue oxygenation cannot be generalized to whole brain (or to other muscles from NIRS on vastus lateralis). Finally, we contend that MCAv is a reliable index of changes in global cerebral blood flow during exercise in normoxia and hypoxia, as the cross-sectional area of the MCA has been shown unchanged within a wide range of changes in PETCO2 (Valdueza et al., 1997) and in comparable hypoxic situations (<5,000 m) (Poulin and Robbins, 1996).
Conclusion
This study showed that pacing strategy during a cycling time-trial is impaired after 24 h in hypoxia (different trend and higher variability) compared to normoxia and this is likely the result from a compromised ability of the central nervous system to voluntarily activate skeletal muscles, owing to inadequate oxygen delivery to the brain. With simultaneous multi-systemic parameters, cerebrovascular function, muscle activity and subjective feelings, light is shed on tissue-specific adaptations and new insights are provided into the mechanisms underlying the higher pacing down-regulation in hypobaric versus normobaric hypoxia. Despite equivalent PiO2, HH is a more stressful stimulus than NH (e.g., lower SpO2 and higher PFC deoxygenation for a given power output during the first half) and suggests a higher “adaptive cost” (same RPE, leg and ventilation discomforts for a lower power output during the second half). As a consequence, aiming to maintain an equivalent oxygen delivery to the brain, the system likely adopts a more “protective” strategy, leading to a further impaired performance in HH. In addition, same relative exercise intensity and physiological disturbances were achieved in HH from the production of lower absolute power when compared to NH, emphasizing once more time that exercise at terrestrial and simulated altitude cannot be carelessly interchanged.
Data availability statement
The raw data supporting the conclusions of this article will be made available by the authors, without undue reservation.
Ethics statement
The studies involving human participants were reviewed and approved by Commission Cantonale Valaisanne d'Ethique Medicale, CCVEM; Agreement 051/09. The patients/participants provided their written informed consent to participate in this study.
Author contributions
TR, JS and GM designed the protocol. TR, JS and NB collected the data. TR and JS performed the data analysis with close support from NB. TR, JS and GM prepared the figures and wrote the manuscript. TR, JS, NB and GM reviewed the manuscript, approved the final version of the manuscript and agree to be accountable for all aspects of the work. All persons designated as authors qualify for authorship, and all those who qualify for authorship are listed.
Funding
This study was funded by grants from Bundes Amt für Sport (BASPO; Switzerland). The authors have no conflict of interest, source of funding or financial ties to disclose and no current or past relationship with companies or manufacturers who could benefit from the results of the present study.
Acknowledgments
The authors thank Alexandre Lamon and Rémi Favre for their technical support. The authors declare that the results of the study are presented clearly, honestly, and without fabrication, falsification, or inappropriate data manipulation.
Conflict of interest
The authors declare that the research was conducted in the absence of any commercial or financial relationships that could be construed as a potential conflict of interest.
Publisher’s note
All claims expressed in this article are solely those of the authors and do not necessarily represent those of their affiliated organizations, or those of the publisher, the editors and the reviewers. Any product that may be evaluated in this article, or claim that may be made by its manufacturer, is not guaranteed or endorsed by the publisher.
Supplementary material
The Supplementary Material for this article can be found online at: https://www.frontiersin.org/articles/10.3389/fphys.2022.893872/full#supplementary-material
References
Amann M., Proctor L. T., Sebranek J. J., Pegelow D. F., Dempsey J. A. (2009). Opioid-mediated muscle afferents inhibit central motor drive and limit peripheral muscle fatigue development in humans. J. Physiol. 587 (1), 271–283. doi:10.1113/jphysiol.2008.163303
Amann M., Romer L. M., Pegelow D. F., Jacques A. J., Hess C. J., Dempsey J. A. (2006). Effects of arterial oxygen content on peripheral locomotor muscle fatigue. J. Appl. Physiol. (1985). 101 (1), 119–127. doi:10.1152/japplphysiol.01596.2005
Amann M., Romer L. M., Subudhi A. W., Pegelow D. F., Dempsey J. A. (2007). Severity of arterial hypoxaemia affects the relative contributions of peripheral muscle fatigue to exercise performance in healthy humans. J. Physiology 581, 389–403. doi:10.1113/jphysiol.2007.129700
Beidleman B. A., Fulco C. S., Staab J. E., Andrew S. P., Muza S. R. (2014). Cycling performance decrement is greater in hypobaric versus normobaric hypoxia. Extrem. Physiol. Med. 3, 8. doi:10.1186/2046-7648-3-8
Casey D. P., Joyner M. J. (2012). Compensatory vasodilatation during hypoxic exercise: Mechanisms responsible for matching oxygen supply to demand. J. Physiol. 590 (24), 6321–6326. doi:10.1113/jphysiol.2012.242396
Clark S. A., Bourdon P. C., Schmidt W., Singh B., Cable G., Onus K. J., et al. (2007). The effect of acute simulated moderate altitude on power, performance and pacing strategies in well-trained cyclists. Eur. J. Appl. physiology 102 (1), 45–55. doi:10.1007/s00421-007-0554-0
Conkin J., Wessel J. H. (2008). Critique of the equivalent air altitude model. Aviat. Space Environ. Med. 79 (10), 975–982. doi:10.3357/asem.2331.2008
Faiss R., Pialoux V., Sartori C., Faes C., Deriaz O., Millet G. P. (2013). Ventilation, oxidative stress, and nitric oxide in hypobaric versus normobaric hypoxia. Med. Sci. Sports Exerc. 45 (2), 253–260. doi:10.1249/MSS.0b013e31826d5aa2
Fan J.-L., Kayser B. (2013). The effect of adding CO2 to hypoxic inspired gas on cerebral blood flow velocity and breathing during incremental exercise. PloS one 8 (11), e81130. doi:10.1371/journal.pone.0081130
Fulco C. S., Muza S. R., Beidleman B. A., Demes R., Staab J. E., Jones J. E., et al. (2011). Effect of repeated normobaric hypoxia exposures during sleep on acute mountain sickness, exercise performance, and sleep during exposure to terrestrial altitude. Am. J. Physiol. Regul. Integr. Comp. Physiol. 300 (2), R428–R436. doi:10.1152/ajpregu.00633.2010
Goodall S., Twomey R., Amann M. (2014). Acute and chronic hypoxia: Implications for cerebral function and exercise tolerance. Fatigue. 2 (2), 73–92. doi:10.1080/21641846.2014.909963
Hamaoka T., McCully K. K., Quaresima V., Yamamoto K., Chance B. (2007). Near-infrared spectroscopy/imaging for monitoring muscle oxygenation and oxidative metabolism in healthy and diseased humans. J. Biomed. Opt. 12 (6), 062105. doi:10.1117/1.2805437
Heinzer R., Saugy J. J., Rupp T., Tobback N., Faiss R., Bourdillon N., et al. (2016). Comparison of sleep disorders between real and simulated 3, 450-m altitude. Comp. Sleep Disord. between Real SimulatedSLEEP 339 (08), 4501517–4501523. doi:10.5665/sleep.6010
Jorgensen L. G., Perko G., Secher N. H. (1992). Regional cerebral artery mean flow velocity and blood flow during dynamic exercise in humans. J. Appl. Physiol. 73 (5), 1825–1830. doi:10.1152/jappl.1992.73.5.1825
Luks A. M. (2015). Physiology in medicine: A physiologic approach to prevention and treatment of acute high-altitude illnesses. J. Appl. Physiol. 118 (5), 509–519. doi:10.1152/japplphysiol.00955.2014
Marillier M., Rupp T., Bouzat P., Walther G., Baillieul S., Millet G. Y., et al. (2021). Cerebral haemodynamics and oxygenation during whole-body exercise over 5 days at high altitude. Exp. Physiol. 106 (1), 65–75. doi:10.1113/EP088354
Pagani M., Salmaso D., Sidiras G. G., Jonsson C., Jacobsson H., Larsson S. A., et al. (2011). Impact of acute hypobaric hypoxia on blood flow distribution in brain. Acta Physiol. 202 (2), 203–209. doi:10.1111/j.1748-1716.2011.02264.x
Peltonen J. E., Rusko H. K., Rantamaki J., Sweins K., Niittymaki S., Viitasalo J. T. (1997). Effects of oxygen fraction in inspired air on force production and electromyogram activity during ergometer rowing. Eur. J. Appl. Physiol. 76 (6), 495–503. doi:10.1007/s004210050281
Périard J. D., Racinais S. (2016). Performance and pacing during cycle exercise in hyperthermic and hypoxic conditions. Med. Sci. Sports Exerc. 48 (5), 845–853. doi:10.1249/MSS.0000000000000839
Perrey S. (2008). Non-invasive NIR spectroscopy of human brain function during exercise. Methods (San Diego, Calif.) 45 (4), 289–299. doi:10.1016/j.ymeth.2008.04.005
Poulin M. J., Robbins P. A. (1996). Indexes of flow and cross-sectional area of the middle cerebral artery using Doppler ultrasound during hypoxia and hypercapnia in humans. Stroke 27 (12), 2244–2250. doi:10.1161/01.str.27.12.2244
Ramnani N., Owen A. M. (2004). Anterior prefrontal cortex: Insights into function from anatomy and neuroimaging. Nat. Rev. Neurosci. 5 (3), 184–194. doi:10.1038/nrn1343
Ribon A., Saugy J. J., Rupp T., Faiss R., Debevec T., Pialoux V., et al. (2016). Exposure to hypobaric hypoxia results in higher oxidative stress compared to normobaric hypoxia. Respir. Physiol. Neurobiol. 223, 23–27. doi:10.1016/j.resp.2015.12.008
Roelands B., Goekint M., Buyse L., Pauwels F., De Schutter G., Piacentini F., et al. (2009). Time trial performance in normal and high ambient temperature: Is there a role for 5-HT? Eur. J. Appl. Physiol. 107 (1), 119–126. doi:10.1007/s00421-009-1109-3
Rolfe P. (2000). In vivo near-infrared spectroscopy. Annu. Rev. Biomed. Eng. 2 (1), 715–754. doi:10.1146/annurev.bioeng.2.1.715
Rooks C. R., Thom N. J., McCully K. K., Dishman R. K. (2010). Effects of incremental exercise on cerebral oxygenation measured by near-infrared spectroscopy: A systematic review. Prog. Neurobiol. 92 (2), 134–150. doi:10.1016/j.pneurobio.2010.06.002
Rupp T., Jubeau M., Millet G. Y., Perrey S., Esteve F., Wuyam B., et al. (2013). The effect of hypoxemia and exercise on acute mountain sickness symptoms. J. Appl. Physiol. 114 (2), 180–185. doi:10.1152/japplphysiol.00769.2012
Rupp T., Jubeau M., Millet G. Y., Wuyam B., Levy P., Verges S., et al. (2013). Muscle, prefrontal, and motor cortex oxygenation profiles during prolonged fatiguing exercise. Adv. Exp. Med. Biol. 789, 149–155. doi:10.1007/978-1-4614-7411-1_21
Rupp T., Leti T., Jubeau M., Millet G. Y., Bricout V. A., Levy P., et al. (2013). Tissue deoxygenation kinetics induced by prolonged hypoxic exposure in healthy humans at rest. J. Biomed. Opt. 18 (9), 095002. doi:10.1117/1.JBO.18.9.095002
Saugy J. J., Rupp T., Faiss R., Lamon A., Bourdillon N., Millet G. P. (2016). Cycling time trial is more altered in hypobaric than normobaric hypoxia. Med. Sci. Sports Exerc. 48 (4), 680–688. doi:10.1249/MSS.0000000000000810
Schuler B., Thomsen J. J., GassMannM. , Lundby C. (2007). Timing the arrival at 2340 m altitude for aerobic performance. Scand. J. Med. Sci. Sports 17 (5), 588–594. doi:10.1111/j.1600-0838.2006.00611.x
St Clair Gibson A., Noakes T. D., St ClAir Gibson A. (2004). Evidence for complex system integration and dynamic neural regulation of skeletal muscle recruitment during exercise in humans. Br. J. Sports Med. 38 (6), 797–806. doi:10.1136/bjsm.2003.009852
Subudhi A. W., Miramon B. R., Granger M. E., Roach R. C. (2009). Frontal and motor cortex oxygenation during maximal exercise in normoxia and hypoxia. J. Appl. Physiol. 106 (4), 1153–1158. doi:10.1152/japplphysiol.91475.2008
Subudhi A. W., Panerai R. B., Roach R. C. (2010). Effects of hypobaric hypoxia on cerebral autoregulation. Stroke 41 (4), 641–646. doi:10.1161/STROKEAHA.109.574749
Takahashi T., Takikawa Y., Kawagoe R., Shibuya S., Iwano T., Kitazawa S. (2011). Influence of skin blood flow on near-infrared spectroscopy signals measured on the forehead during a verbal fluency task. NeuroImage 57 (3), 991–1002. doi:10.1016/j.neuroimage.2011.05.012
Tucker R. (2009). The anticipatory regulation of performance: The physiological basis for pacing strategies and the development of a perception-based model for exercise performance. Br. J. Sports Med. 43 (6), 392–400. doi:10.1136/bjsm.2008.050799
Valdueza J. M., Balzer J. O., Villringer A., Vogl T. J., KutteR R., Einhaupl K. M. (1997). Changes in blood flow velocity and diameter of the middle cerebral artery during hyperventilation: Assessment with MR and transcranial Doppler sonography. AJNR. Am. J. Neuroradiol. 18 (10), 1929–1934.
Verges S., Rupp T., Jubeau M., Wuyam B., Esteve F., Levy P., et al. (2012). Invited review: Cerebral perturbations during exercise in hypoxia. Am. J. Physiol. Regul. Integr. Comp. Physiol. 302 (8), R903–R916. doi:10.1152/ajpregu.00555.2011
Vogiatzis I., Louvaris Z., Habazettl H., Athanasopoulos D., Andrianopoulos V., Cherouveim E., et al. (2011). Frontal cerebral cortex blood flow, oxygen delivery and oxygenation during normoxic and hypoxic exercise in athletes. J. Physiol. 589 (16), 4027–4039. doi:10.1113/jphysiol.2011.210880
Keywords: altitude, cerebral oxygen delivery, near-infrared spectroscopy, pacing strategies, performance, time-trial exercise
Citation: Rupp T, Saugy JJ, Bourdillon N and Millet GP (2022) Brain-muscle interplay during endurance self-paced exercise in normobaric and hypobaric hypoxia. Front. Physiol. 13:893872. doi: 10.3389/fphys.2022.893872
Received: 10 March 2022; Accepted: 27 July 2022;
Published: 25 August 2022.
Edited by:
Xu Yan, Victoria University, AustraliaReviewed by:
Hannes Gatterer, Eurac Research, ItalyHayden Gerhart, Grove City College, United States
Peter Rasmussen, University of Zurich, Switzerland
Copyright © 2022 Rupp, Saugy, Bourdillon and Millet. This is an open-access article distributed under the terms of the Creative Commons Attribution License (CC BY). The use, distribution or reproduction in other forums is permitted, provided the original author(s) and the copyright owner(s) are credited and that the original publication in this journal is cited, in accordance with accepted academic practice. No use, distribution or reproduction is permitted which does not comply with these terms.
*Correspondence: Grégoire P. Millet, Z3JlZ29pcmUubWlsbGV0QHVuaWwuY2g=
†These authors have contributed equally to this work and share first authorship