- 1Physiology and Biomedical Engineering, Mayo Clinic College of Medicine and Science, Rochester, MN, United States
- 2Renal Division, Brigham and Womens Hospital and Harvard Medical School, Boston, MA, United States
- 3Biomedical Engineering, Boston University, Boston, MA, United States
- 4Renal Division, West Roxbury VA Medical Center, West Roxbury, MA, United States
- 5Nephrology and Hypertension, Mayo Clinic College of Medicine and Science, Rochester, MN, United States
Introduction: Nephrolithiasis is a painful and costly healthcare complication. The most common kidney stones are composed of calcium oxalate and thus renal handling of oxalate is an important facet of understanding the pathogenesis of nephrolithiasis. Recently, the Drosophila melanogaster Malpighian tubule (MT) has emerged as a robust model of trans-epithelial ion transport and nephrolithiasis as MTs readily form luminal calcium-oxalate crystals in the presence of oxalate. Drosophila Prestin (dPrestin, Slc26a6) transports oxalate across the apical surface of the MT into the lumen but a full model of the trans-epithelial movement of oxalate (Ox2−) in the Drosophila MT has been lacking as the basolateral oxalate transporter has remained uncharacterized.
Methods: The objective of this work was to identify and characterize the Drosophila basolateral Ox2− transporter through ex vivo real-time quantification of intracellular pH (pHi) and Xenopus oocyte transport assays.
Results: A putative basolateral oxalate transporter CG5002 (“Neat”) was identified through sequence homology and displayed robust Cl−-independent Ox2− transport and electroneutral Ox2− transport in Xenopus oocytes. pHi in extracted fly MTs was monitored by using the GAL4/UAS system to selectively express pHerry, a pseudo-ratiometric genetically-encoded pH indicator (GEpHI) in the cytosol of the principal cells of the MT. Basolateral perfusion of MTs in CO2/HCO3−-buffered solution produced a large acidification followed by rapid recovery in the transitional segment of the anterior MT. Recovery was interrupted by basolateral application of 1 mM Ox2− or 1 mM SO42. Tissue specific knock-down of Neat with interference RNA (RNAi) reduced the rate of acid-loading in the transitional segment of the MT with regard to Ox2− and SO42−. Knockdown of Neat in the MT also significantly reduced luminal calcium oxalate crystal formation in a fly ex vivo model of calcium oxalate nephrolithiasis.
Discussion: These data indicate Neat is a significant Drosophila basolateral MT oxalate transporter and the basolateral movement of oxalate is functionally coupled to movement of acid equivalents, potentially as Ox2−/HCO3− exchange, Ox2−/OH− exchange, or Ox2−:H+ co-transport.
1 Introduction
Nephrolithiasis is a worldwide disease (∼10%) with an increasing prevalence and significant morbidity, which is affected by both environmental and genetic factors (Wang and Wang, 2024). It is characterized by the formation of various crystals within the kidneys or urinary tract. The most common form of nephrolithiasis results from calcium oxalate (CaOx) deposition. The mechanism of CaOx formation in nephrolithiasis involves multiple factors, among which the imbalance between inhibitors and promoters of Ca2+ and oxalate (Ox2-) transepithelial transport and crystallization is of potential importance. Understanding the mechanisms of those anion movements is crucial for developing strategies to prevent and treat CaOx-related kidney stones. Therefore, an assessable model of transepithelial Ox2- movement is desirable.
The adult Drosophila melanogaster (fruit fly) Malpighian renal tubule (MT) is a highly active excretory organ lined with a single epithelial layer of metabolically active principal cells. It has been identified as a site of rapid calcium excretion (Dube et al., 2000; MacPherson et al., 2005; Southall et al., 2006; Terhzaz et al., 1999) and Ox2− transport (Chen et al., 2011; Hirata et al., 2012a; Hirata et al., 2010), similar to the functions of the human kidney. Drosophila reliably develop CaOx crystals upon dietary exposure to high (10 mM) Na2Ox supplementation and the growth of microliths in MT can be viewed in real time. Cellular assays of ion transport physiology can be combined with genetic binary expression systems to target fluorescent reporter and RNA interference (RNAi) constructs to individual cells, thus providing a robust system for studies of solute transport at the molecular, cellular, and organ scales. Therefore, our lab and others developed a Drosophila model of CaOx nephrolithiasis which can be used for more precisely investigating ion and solute movements (Chen et al., 2011; Hirata et al., 2012a; Hirata et al., 2010). Using this model, we have identified Drosophila prestin (dPrestin), a key apical transporter which mediates Ox2− and SO42− export into MT lumen. Formation of CaOx crystals quantitatively decreased in dPrestin-knockdown animals (Hirata et al., 2012a; Hirata et al., 2012b). These data, as well as studies of oxalate transport via dPrestin in a Xenopus oocyte expression system, indicate dPrestin is functionally the Diptera ortholog of SLC26A6 and mouse Slc26a6 (Landry et al., 2016). These proteins play important roles in the transporting multiple anions, e.g., Cl−, HCO3−, Ox2−, SO42−, and formate− (Landry et al., 2016; Ohana et al., 2013; Shimshilashvili et al., 2020; Yang et al., 2021).
Prior results indicate that dPrestin functions as an apical Ox2− transporter in fly MTs, but how oxalate is moved from the hemolymph into the MT to complete the model of transepithelial Ox2− transport has not been determined. In mammals, a sulfate anion transporter (Sat1, Slc26a1) was cloned by functional expression from rat liver as a Na+ independent sulfate transporter (Bissig et al., 1994). Subsequent work demonstrated oxalate and HCO3− transport via Slc26a1 (Karniski et al., 1998; Xie et al., 2002). Oocyte expression experiments illustrated pH-dependent sulfate uptake (Xie et al., 2002). Based on their expression work, Krick and associates hypothesized that in the proximal tubule under physiological conditions, Slc26a1 (Sat1) exchanges intracellular sulfate for extracellular HCO3− which in turn is recycled by Slc4a4 (NBCe1) to contribute to kidney oxalate secretion in hyperoxalemic patients (Krick et al., 2009). SO42− uptake was competitively inhibited by Ox2− (Ki of 63.5 µM), a value close to the oxalate concentration detected in the serum of patients with hyperoxaluria or end-stage renal disease, whereas oxalate uptake showed saturation kinetics with a Km of 53.5 µM, a value close to that obtained for the Ki for competitive inhibition of SO42− uptake by Ox2− (63.5 µM), indicating an interaction at the same site of SLC26A1 (Krick et al., 200). Genetic knock-out experiments in mice revealed that sat1 (Slc26a1)-loss is associated with hyposulfatemia, hypersulfaturia, calcium oxalate urolithiasis, and nephrocalcinosis (Dawson et al., 2010; Markovich, 2011). Human SAT1 (SLC26A1) mutations are associated with calcium oxalate nephrolithiasis (Gee et al., 2016). Thus, in mammals, both apical Slc26a6 and basolateral Slc26a1 affect the CaOx kidney stone formation. This makes it important to understand how these apical and basolateral transporters work together to regulate transepithelial oxalate transport and subsequent CaOx stone formation.
Similarly, for transepithelial Ox2− transport in the Drosophila Malpighian tubule (MT), there should be another Ox2− transporter. This yet unknown transporter should ideally be at the basolateral membrane to move Ox2− from the hemolymph into the MT, so that dPrestin might then move Ox2− from the MT cell to the MT lumen to then be excreted. The aim of the current study was to identify another Drosophila oxalate transporter, elucidate its function, and determine if it impacts calcium oxalate (CaOx) crystallization in this model.
In a functional simplified in vivo model (Drosophila), knowing both apical and basilateral transporters will facilitate understanding differences and similarities of CaOx crystallization-stone formation (Figure 1). Here, we have characterized a Drosophila Ox2− transporter (CG5002; “Neat”; named such as knockdown produces MTs “without rocks”) as a candidate for the other oxalate transporter. These studies use Xenopus oocyte expression to test Ox2− transport by Drosophila Slc26 member, and then the GAL4/UAS system (Brand and Perrimon, 1993) to selectively express pHerry (Rossano et al., 2013), a genetically-encoded pH indicator (GEpHI), with and without RNA interference (RNAi)-mediated knockdown of Neat while also following MT crystal formation. Together these data support the hypothesis that Neat is likely a Drosophila oxalate uptake system working in concert with dPrestin, is bicarbonate coupled, and is a functional equivalent of mammalian basolateral Ox2− transporter Slc26a1.
2 Methods
2.1 Ethical approval - animal health and welfare
Xenopus laevis were housed and cared for in accordance and approval of the Institutional Care and Use Committees of the Mayo Clinic College of Medicine and Brigham and Women’s Hospital.
2.2 RNA and oocyte care
Constructs containing coding regions of indicated transporters were subcloned into the pGEMHE Xenopus expression vector and capped cRNA synthesized. Oocytes were injected with 50 nL cRNA (0.2 μg/μL, 10 ng/oocyte) or water as previously (Hirata et al., 2010; Romero et al., 2000) for other transporters and incubated at 16°C in OR3 media. Oocytes were studied 3–10 days after injection. Amount of cRNA injected was constant through all experiments and transporter expression was assumed to be constant as well.
2.3 Oocyte oxalate uptake assay
All uptake experiments using Xenopus laevis oocytes were performed as described previously (Mandal et al., 2017; Mandal and Mount, 2019). Briefly, for [14C]-oxalate uptake experiments in oocytes, each oocyte was microinjected using a fine-tipped micropipette fitted in a microinjector (World Precision Instruments Inc. Sarasota, FL) with equal amount (∼25 ng/50 nL) of in vitro synthesized intact appropriate cRNA (checked by 1% formaldehyde-agarose gel electrophoresis in MOPS buffer, pH 8.0) and incubated in isotonic ND96 medium (96 mM NaCl, 2.0 mM KCl, 1.8 mM CaCl2, 1.0 mM MgCl2 and 5 mM HEPES, pH 7.4) containing 3 mM pyruvate and gentamycin (10 μg/mL at 16°C–18°C for approximately 48 h for the expression of respective protein from injected cRNA) in chloride-free medium (Cl− was substituted by equimolar gluconate ion), pH 7.4 at 25°C. Oocytes were then washed four times with isotonic ND96 medium without pyruvate and gentamycin. After 1 h of incubation in the indicated chloride-free uptake medium (1 mL/well) containing [14C]-oxalate (20 μM; 51 μCi/μmol; Moravek Inc. Brea, CA) in 12-well plates at room temperature [25°C] in a horizontal shaker-incubator, oocytes (15–20 in each group) were washed three times with the ice-cold uptake medium to remove external radioisotope. Radioisotope content of each individual oocyte was measured by scintillation counter (LS 6500 multi-Purpose Scintillation Counter, Beckman Coulter, Brea, CA) after solubilization in 0.3 mL of 10% (v/v) SDS and addition of 2.5 mL of scintillation fluid. All uptake experiments included at least 15–20 oocytes in each experimental group; statistical significance was defined as p ≤ 0.05 by one-way ANOVA with Bonferonni’s multiple comparisons tests and results were reported as mean ± SD. All of the uptake experiments were performed more than three times for confirmation with appropriate controls; data shown for each figure are from a single representative experiment (units are pmol/oocyte/h).
2.4 Oocyte electrophysiology
Electrophysiology protocols were performed as we previously reported (Romero et al., 2000). All solutions were either ND96 (96 mM NaCl, 2 mM KCl, 1.8 mM CaCl2, 1 mM MgCl2, and 5 mM HEPES, pH 7.5) with additional 1 mM Na2SO4 or 300 µM Na-oxalate as indicated. HCO3− solutions were continuously bubbled with 5% CO2/33 mM HCO3− (pH 7.5). For unclamped Vm measurements, data were recorded with an OC-725C voltage clamp amplifier (Warner Instruments, Hamden, CT), filtered at 2–5 kHz, digitized at 10 kHz. For voltage clamp experiments, oocytes were clamped to −60 mV and then voltage rapidly shifted to −160 mV to +60 mV in 20 mV steps (70 ms sweeps, filtered at 1 kHz; HEKA Elektronik, Harvard Bioscience Inc.; https://www.heka.com/). The resulting linear IV-plots are then expressed as “slope conductance” in the appropriate non-CO2/HCO3− solution (Chen et al., 2024). Full solution composition appears in Table 1. For ion-selective microelectrodes to monitor pHi, we used a liquid-ion-exchange (lix) resin (H+ ionophore I, mixture B; Fluka Chemical, Ronkonkoma, NY, United States). Intracellular pH microelectrodes had slopes of at least −54 mV/pH unit (Romero et al., 2000). Whenever possible, the slope of pH changes (pH-electrode and pH-sensor) within an individual trace was calculated from regions with linear change and overlapping range of absolute pH to minimize the effects of intrinsic and HCO3−-derived buffering on perceived rate of pH changes (Chesler, 1990; Rossano and Romero, 2017; Vaughan-Jones and Wu, 1990).
2.5 Drosophila husbandry
Flies were kept on standard medium in vials at 22°C, 12:12-h photoperiod, and 40% relative humidity. All lines were backcrossed into a w1118 (Bonini) background and this line served as controls.
2.6 Cell type-specific knockdown of CG5002 (Neat)
This was performed as described previously (Reynolds et al., 2024). To specifically knock down CG5002, we utilized the CapaR-GAL4 driver (Terhzaz et al., 2012) to restrict expression to the MT principal cells and crossed it VDRC lines KK100600 or GD10058 (Vienna, Vienna BioCenter Core Facility) containing a hairpin dsRNA sequence directed against CG5002.
2.7 Quantitative RT-PCR
Quantitative RT-PCR validation was performed as described elsewhere (Reynolds et al., 2024). mRNA was prepared from 7-day-old W1118 or experimental tubules using Qiagen RNAeasy column. Superscript II and an oligo-dT were used for reverse transcription. For each sample, 500 ng of cDNA were added to 12.5 μL of 2 μM SYBR green reaction mix (Finnzymes, GRI, Essex, United Kingdom) and 1 μL of 6.6 μM forward and reverse primers. An Opticon 2 thermocycler was set as follows: 95°C for 15 min followed by 35 cycles of 95°C for 30 s, 58°C for 30 s, and 72°C for 30 s. The ribosomal protein L32 (RPL32) gene was used as a standard in all experiments. For each condition, we used three independent samples; each PCR was repeated three independent times to verify results. Full primer sequences are listed in Table 2.
2.8 Ex vivo cellular pH imaging of Drosophila MTs with a genetically-encoded pH indicator
Live epifluorescence imaging of pHerry (Rossano et al., 2017), a pseudo-ratiometric GEpHI consisting of a pH-sensitive superecliptic pHluorin (Miesenbock et al., 1998) (SepH; 488/510 nm ex./em.) and pH-insensitive mCherry (Shaner et al., 2004) (565/620 nm ex./em.) joined by a short peptide linker, was performed as previously described (Rossano and Romero, 2017). Briefly, UAS-pHerry-myr (encoding pHerry with an additional N-terminus myristylation sequence to aid in near-membrane targeting and nuclear exclusion) flies were crossed to CapaR-GAL4 flies to drive construct expression in the principal cells of the MT. Anterior MTs were extracted from 7d female flies and transferred to poly-l-lysine-coated slides. Preparations were moved to an inverted wide-field epifluorescent microscope with GFP (SEpH) and RFP (mCherry) filter sets (470/40 nm ex., 515 nm longpass em; 500 nm dichroic and 546/10 nm ex, 590 nm longpass em, 565 nm dichroic), a ×10/0.45 air objective, a monochromatic camera for live-image capture, and an in-line computer-controlled perfusion system. SEpH and mCherry images were collected in an interleaved fashion with 300 ms exposure for each channel and an additional 400 ms to allow for channel switching, yielding a total imaging rate of 1 Hz. MTs were continuously bathed in insect saline consisting of 121.5 mM NaCl, 20 mM KCl, 20 mM glucose, 8.6 mM HEPES, and 10.24 mM NaHCO3 with pH 6.8 set by HCl and NaOH. In NH4Cl pulse experiments NH4Cl was provided in equimolar exchange for NaCl. pHi calibration was performed as previously described (Rossano et al., 2013; Rossano et al., 2017; Thomas et al., 1979) using modified solution consisting of 130 mM KCl, 20 mM Glucose, and 8.6 mM MES, HEPES, or TAPS as appropriate for desired pH range. pH was adjusted with HCl and NMDG and solution was supplemented with 10 μM nigericin in DMSO. Additional point calibrations were used at the end of some imaging experiments. When possible, control and experimental tubules were image simultaneously with the MTs positioned side-by-side to minimize the effects of variable perfusion rates between experiments. Rates of change in pHi were compared in segments of traces within the same pHi to minimize the influence of the pH-dependence of intracellular buffering (Chesler, 1990; Rossano and Romero, 2017; Vaughan-Jones and Wu, 1990). Imaging stacks were acquired in Intelligent Imaging Innovations Slidebook software, background corrected and exported for fluorescence quantification in ImageJ. Full compositions of solutions are described in Table 3.
2.9 Ex vivo CaOx birefringence experiments
Adult flies (7d) were allowed to feed on standard food. Malpighian tubule (MT) from female flies were dissected in Schneider’s medium and transferred immediately to poly-l-lysine-coated slides with insect saline. MTs were allowed to settle for 10 min, at which point solution was swapped for saline supplemented with 10 mM Na-oxalate (equimolar exchange for NaCl).
2.10 CaOx crystal quantification
CaOx crystals were quantified using ImageJ software as previously (Landry et al., 2016). Images were taken at ×10 magnification with an air objective and an inline polarizer. 600 μm was measured from the terminal end of the respective MT. Once measured, brightness and contrast were adjusted, resulting in only birefringent crystals in the designated 600 μm of tubule being visible, and the remaining tubule artifacts were blacked out. A threshold was then set, resulting in binary coloration so that crystals could be counted. Crystals were analyzed using the Analyze Particles feature in ImageJ with the minimum desired size of stone detection specified at 2 μm2. Data generated included an accurate and specific crystal count. Data were then analyzed via one-way ANOVA with Bonferroni post hoc test (P < 0.05) or Student’s t-test (P < 0.05) using GraphPad Prism.
2.11 Data analysis and presentation
Data were compiled in GraphPad Prism and analyzed as indicated in individual data sets. Image analysis was performed in Intelligent Imaging Innovations Slidebook software as well as ImageJ. Curve fitting was performed in Microsoft Excel and GraphPad Prism. Representative micrographs were processed in Adobe Photoshop and figures were assembled with Adobe Illustrator.
2.12 Experimental solutions
Full Composition of all experimental solutions is described in Tables 1, 3.
2.13 Statistical methods
All statistical calculations were performed in GraphPad Prism. Comparison across two groups was performed by unpaired Student’s t-test. Comparisons more than two groups were performed by ANOVA with Bonferroni multiple comparisons correction. Sample sizes for experiments were determined by number of oocytes or number of extracted Malpighian tubules (one tubule per fly). Additional details are provided in descriptions of individual experiments.
3 Results
3.1 Which Drosophila Slc26 proteins transport oxalate in the Malpighian tubule ?
Previous work showed that apical protein dPrestin could transport oxalate into the Malpighian tubule lumen (Figure 1) (Hirata et al., 2012a). This dPrestin (Slc26a5/a6) is the only Drosophila Slc26 protein molecularly close to other known mammalian oxalate transporters (Hirata et al., 2012b) (Figures 2A,B). All others Drosophila Slc26 proteins, at the molecular sequence level, are most closely related to SLC26A11 (Figure 2C; Supplemental Figure S1), a Na+ independent SO42− transporter (Vincourt et al., 2003) and a Cl−/HCO3− exchanger (Xu et al., 2011). SLC26A11/Slc26a11 proteins have not been reported to transport Ox2−.
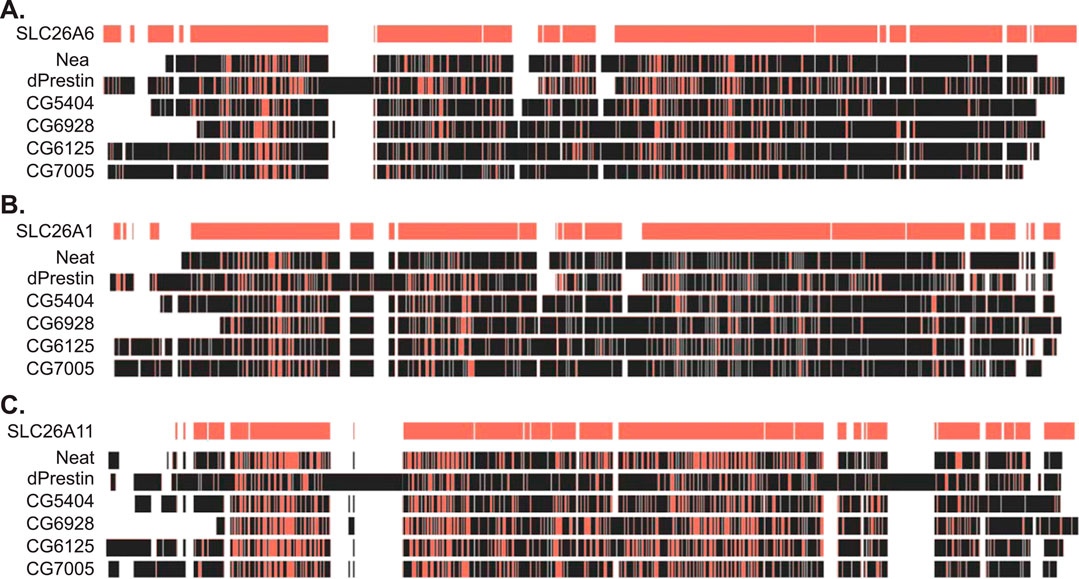
Figure 2. Amino acid sequence similarity between fly and human SLC26 proteins. (A) Amino acid sequence alignment of fly SLC26 proteins to human SLC26A6. (B) Amino acid sequence alignment of fly SLC26 proteins to human SLC26A1. (C) Amino acid sequence alignment of fly SLC26 proteins to human SLC26A11. Red indicates sequence match to human SLC26 sequences on the top line of each section. Alignment performed by Clustal Omega within the Benchling platform. Ascension numbers are as follows: SLC26A6, NM_134263; SLC26A1, AF297659; SLC26A11, AF345195; dPrestin, NM_140767; CG5002 (Neat), AY240021; CG5404, NM_142225; CG6125, AY240022; CG6928, AY240023; CG7005, NM_079766. The amino acid sequence alignment is in Supplementary Figure S1.
To first test for oxalate transport, we used 14C-oxalate uptake experiments with candidate transporters expressed in Xenopus oocytes (Figure 3A). Known human oxalate transporters (SLC26A6, SLC26A2/DTDST) and dPrestin were tested for oxalate uptake and compared to water-injected controls, SLC26A11, and Drosophila Slc26 clones [CG5002 (Neat), CG5404, CG6125, CG6928, CG7005]. As previously shown, SLC26A6 (114.60 ± 18.30 pmol/oocyte/h), DTDST (48.001 ± 8.66) and dPrestin (109.16 ± 26.80) all show significant oxalate uptake vs. control (16.63 ± 2.99, p < 0.00001) with SLC26A6 and dPrestin having the highest oxalate uptake without extracellular Cl− (Figure 3A). SLC26A11 does not show any statistically significant oxalate uptake compared to the control (17.15 ± 2.78, p = 1). Of the Drosophila Slc26 proteins, only Neat (53.43 ± 5.49, p < 0.00001) and CG5404 (32.74 ± 11.83, p < 0.05) showed significant oxalate uptake in Cl− free solution. CG6125 (27.69 ± 3.69, p = 0.98), CG6928 (19.27 ± 3.17, p = 1) and CG7005 (21.62 ± 4.00, p = 1) did not differ from controls (16.63 ± 2.99).
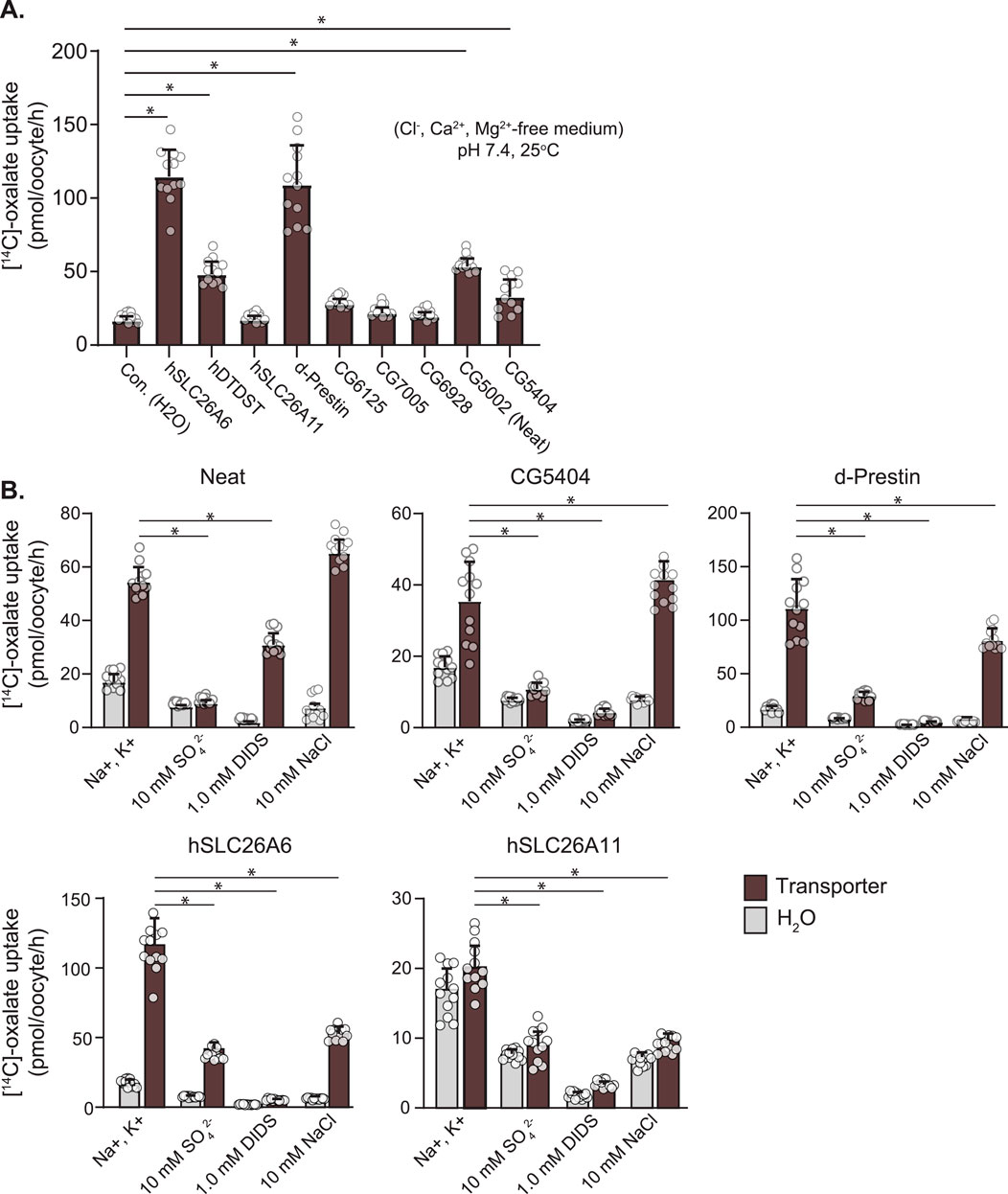
Figure 3. Ox2− Uptake by Drosophila Slc26-transporters in Xenopus Oocytes. (A) 14C-labeled oxalate uptake in Xenopus oocytes expressing oxalate transporters and water injected control. (B) Response of 14C-labeled oxalate uptake in Xenopus oocytes expressing oxalate transporters and water injected controls to control solution, 10 mM SO42−, 1 mM DIDS, and 10 mM NaCl. Circles indicate individual oocytes. Bars = mean + SD. N = 12 oocytes per group. * p < 0.05 by one-way ANOVA with Bonferroni multiple comparisons tests.
Within these same uptake experiments, cis-inhibition by 10 mM SO42− and 1 mM DIDS were evaluated (Figure 3B). As expected from the previously published activity of dPrestin (111.14 ± 27.28 pmol\oocyte/h), both DIDS (29.41 ± 3.64) and SO42− (4.69 ± 0.73) cis-inhibit dPrestin oxalate uptake to water injected control levels (16.94 ± 3.04; SO42−, 7.74 ± 0.65; DIDS, 1.91 ± 0,38). A similar cis-inhibition pattern was found comparing CG5404 to control Ox2− uptakes. For Neat (54.40 ± 5.59), cis-addition (bath) of 10 mM SO42− (9.03 ± 1.22) fully blocked Ox2− uptake (Figure 3B, left). However, 1 mM DIDS only reduced Ox2− uptake by half (30.92 ± 4.29).
Many SLC26 protein family members transport chloride, and thus we evaluated if cis application of Cl− in previously chloride-free solutions could inhibit Ox2− uptake within the same experiments (Figure 3B, right sides of panels). Both Neat (54.40 ± 5.59) and CG5404 (32.74 ± 11.83) displayed no significant cis inhibition of Ox2− uptake by Cl− (66.29 ± 5.18 and 37.91 ± 4.64 respectively). Chloride inhibited Ox2− uptake by dPrestin by approximately half (111.14 ± 27.28 to 68.52 ± 9.45). Similarly robust inhibition of oxalate uptake by cis application of Cl− was observed in SLC26A6 (114.60 ± 18.30 to 52.82 ± 5.07). Taken together, these data support our ability to detect the effects of extracellular Cl− on Ox2− uptake and reveal Neat does not function as an Ox2−/Cl− exchanger.
3.2 Which Drosophila Slc26 mRNAs exist in the MT?
The next consideration was which Drosophila Slc26 oxalate transporter mRNAs or proteins have expression in MTs. Searching FlyAtlas2 (flyatlas2.org), we found that Neat was 175 ± 21 FPKM (adult male) and 191 ± 27 FPKM (adult female) while CG5404 is 16 ± 2.5 FPKM (male) and 15 ± 4.0 FPKM (female). These data indicate that Neat mRNA expression is ∼10-fold higher than cg5404, and that oxalate uptake is ∼2-fold higher. The combined data indicate that Neat is the Drosophila Slc26 transporter of most interest for a candidate basolateral Ox2− transporter in the Malpighian tubule. CG5404 expression is increased relative to Neat in hindgut (140 vs 18 FPKM in adult females).
3.3 Electrophysiology of Neat function – Xenopus oocytes
With Neat identified as the likely oxalate transporter of interest we further characterized its transport of Ox2−, HCO3−, and SO42− in a Xenopus oocyte heterologous expression system (Figure 4). Neat demonstrated electroneutral HCO3− influx in the presence of HCO3−/CO2 buffered saline (dpH/dt 0.0094 ± 0.0047 pH units per minute as compared to 0.0013 ± 0.0003 in water injected control oocytes; Figures 4A–C). This influx of HCO3− was stopped by the addition of 300 µM Ox2− (ΔdpH/dt: −0.010 ± 0.004; Figure 4D) or 1 mM SO42− (ΔdpH/dt: −0.010 ± 0.004; Figure 4E). Each of the substrates produced significant changes in dpH/dt compared to those observed in water injected oocytes (p < 0.005 by Student’s unpaired t-test in all cases). Expression of Neat did not significantly alter resting pHi (Figure 4F). These electrophysiology data suggest Neat mediates electroneutral HCO3−/Ox2− exchange and HCO3−/SO42− exchange. Voltage clamp experiments revealed that dPrestin elicited conductance changes with 5 mM SO42− as previously reported (Hirata et al., 2012b). However, neither Ox2− nor SO42− elicited a conductance change from baseline in Neat. Additionally, the overall Neat-oocyte conductance (5.94 ± 2.03 µS) was about 25% of baseline dPrestin conductance (19.44 ± 4.25 µS; Figure 4G). These data further support the conclusion that ion transport via Neat is electroneutral.
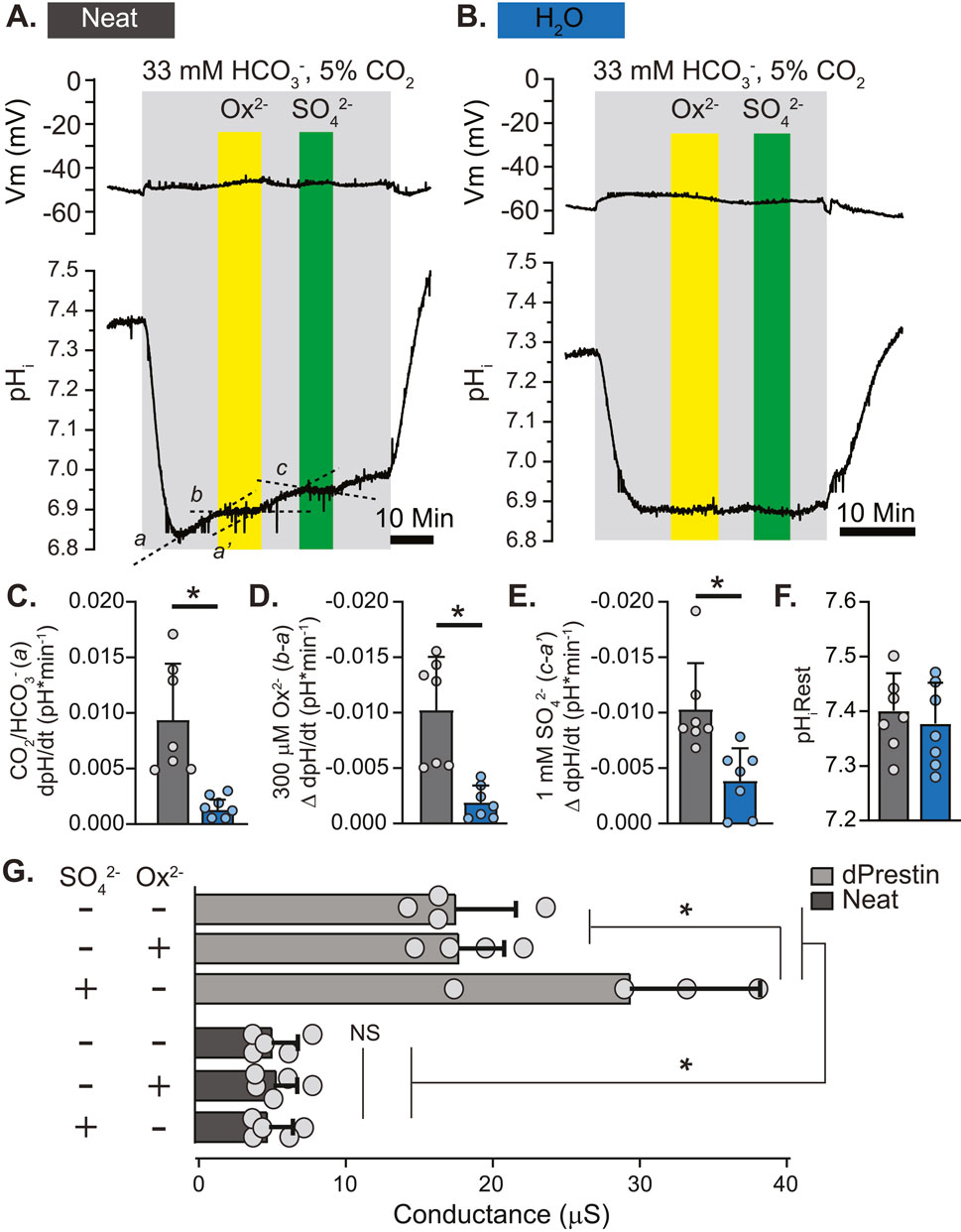
Figure 4. Putative Drosophila Ox2− Transporter “Neat” Displays Electroneutral HCO3−/Ox2− and HCO3−/SO42− Exchange in Xenopus Oocytes. (A, B) simultaneous un-clamped membrane voltage (Vm; voltage electrode) and pHi (intracellular pH-sensitive electrode) responses to extracellular Ox2− (300 μM) and SO42− (1 mM) in Xenopus oocytes expressing Neat (A) and control (H2O; (B). (C–E) Rate of change of pHi in response to CO2/HCO3− solution (C), Ox2− (D), and SO42− (E). Slopes derived from a, b, a’, and c in (A). The same regions were used to calculate slopes in control (H2O) oocytes, but labels are omitted from B for clarity. Circles = individual oocytes. Bars = Mean + SD. * = p ≤ 0.05 by two-way unpaired Student’s t-test. (F). Initial pHi prior to 5%CO2/33 mM HCO3− solution (pH 7.5). Circles = individual oocytes. Bars = Mean + SD. (G) Basal and oxalate/sulfate-induced conductance measurements obtained by voltage clamp in oocytes expressing dPrestin and Neat. Circles = individual oocytes. Bars = Mean + SD. *p < 0.01 by one-way ANOVA with Bonferroni post hoc test.
3.4 Neat function in Drosophila MTs
The fact that Neat mediates exchange of acid equivalents raised the possibility that we could investigate Neat activity in Drosophila MTs ex vivo through the use of genetically encoded pH indicators targeted to the cytosolic surface of the MT principal cell membrane. To this end, we used the GAL4/UAS binary expression system to express pHerry (Rossano et al., 2017), a pseudo-ratiometric genetically encoded pH indicator consisting of a pH-sensitive GFP variant (SEpH) and a pH insensitive RFP variant (mCherry) in the principal cells of adult Drosophila MTs and calibrated the biosensor to monitor pHi in real time through live epifluorescent imaging as previously described (Rossano and Romero, 2017). Both the GFP and RFP components of pHerry were easily visualized in extracted MTs (Figure 5A), and direct manipulation of pHi with NH4Cl pulses confirmed the expected pH sensitivity of the SEpH component and the pH insensitivity of the mCherry component (Figures 5B,C). We used the high potassium/nigericin technique (Thomas et al., 1979) to calibrate the GFP/RFP ratio as a function of pHi (Figures 5D,E). Boltzmann fit to these data yielded R2 = 0.95 and pKa = 7.24, which is in agreement with previously published ex vivo and in situ calibration of superecliptic pHluorin-based pH Indicators in Drosophila (Rossano et al., 2013; Rossano et al., 2017).
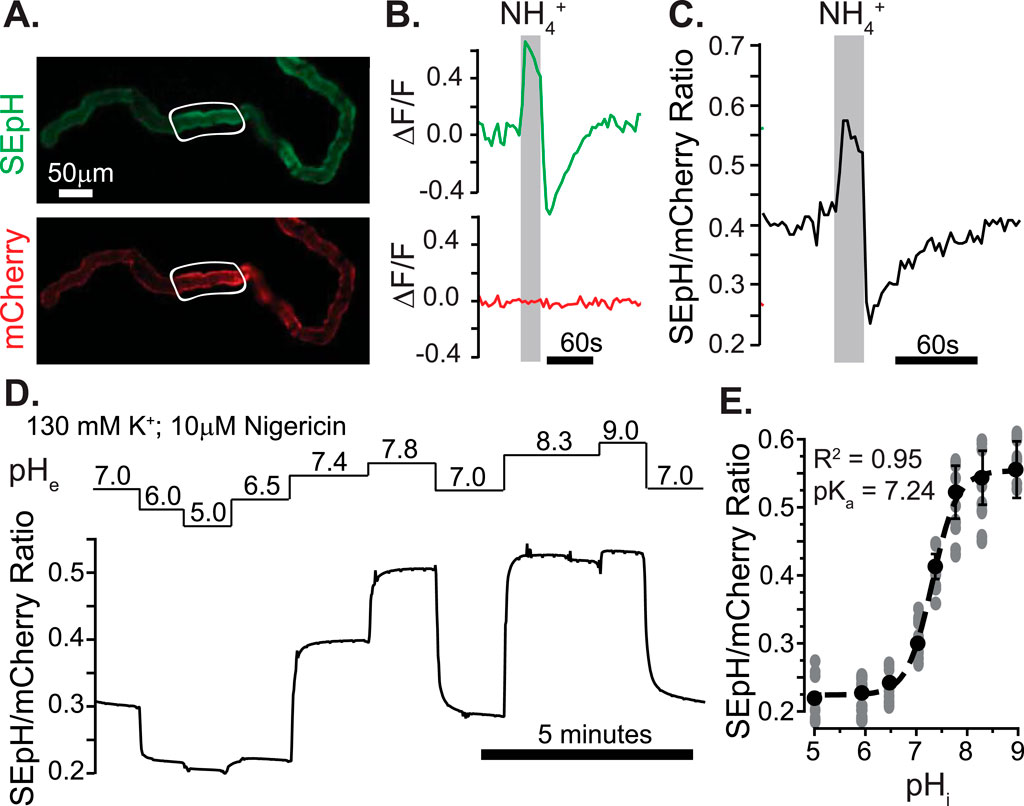
Figure 5. Ex vivo quantification of intracellular pH in transitional segment MT principal cells with a pseudo-ratiometric genetically-encoded pH Indicator. (A) Fluorescent micrograph of dissected MT from UAS-pHerry-myr; CapaR-GAL4 female fly displaying pH-sensitive (SEpH; 488 nm ex./510 nm em) and pH-insensitive (mCherry; 565 nm ex./630 nm em) components of pHerry. (B) Relative fluorescence changes in SEpH and mCherry in response to Intracellular alkalinization and acid loading by bath-applied 40 mM NH4Cl pulse. Traces derived from background corrected ROIs in (A) (white outlines). (C) Ratio of SepH to mCherry signal from (B). (D) Representative trace of normalized SEpH/mCherry ratio in response to high K+/Nigericin calibration with imposed extracellular pH (pHe) values. (E) Calibration curve of pHerry ratio derived from Boltzmann fit of data from (D). Circles = individual principal cells, data set derived from 8 MTs. In all cases SepH and mCherry frames were captured at 300 ms each with 400 ms allotted for filter switching, yielding an imaging rate of 1 Hz.
We next combined live imaging of pHi with the robust RNAi toolkit available in Drosophila to evaluate changes in pHi of MT principal cells attributable to HCO3−, SO42−, and Ox2− in the presence and absence of Neat knockdown with two separate RNAi constructs (KK100600 and GD10058 from the Vienna stock center). When CapaR-GAL4 was used to drive RNAi expression in MT principal cells quantitative PCR revealed knockdown of transcript level to 34% ± 0.5% and 40% ± 0.5% of control level in RNAi-1 (KK100600) and RNAi-2 (GD10058), respectively. Transcript levels were normalized to RPL32 in all cases and data were pooled across three runs from each genotype in RNA extracted from adult Drosophila MTs. Freshly extracted MTs expressing membrane-targeted pHerry were mounted on cover glass and perfused with insect saline buffered either with HEPES or HCO3−/CO2 with additional oxalate and sulfate to monitor changes in pHi in response to basolateral addition of HCO3−, Ox2−, and SO42− (Figures 6A,B). Switching to HCO3− buffered saline produced an initial fall in pHi followed by a gradual alkalinization mediated by HCO3− influx. This alkalinization was significantly slowed when Neat was knocked down with either RNAi construct as compared to that seen in control MTs (ΔdpH/dt: +0.14 ± 0.04 vs. +0.06 ± 0.04 in RNAi-1 experiments and +0.17 ± 0.06 vs. +0.07 ± 0.06 in RNAi-2 experiments, p < 0.01 in both cases by Students unpaired t-test; Figure 6C). This alkalinization was quickly reversed with the addition of 1 mM basolateral oxalate as well as 1 mM basolateral sulfate. In each case the change in dpH/dt with the addition of oxalate (−0.72 ± 0.18 vs. −0.41 ± 0.21 in RNAi-1 and -0.67 ± 0.10 vs. −0.28 ± 0.14 in RNAi-2) and sulfate (−0.19 ± 0.06 vs. −0.05 ± 0.05 in RNAi-1 and -0.15 ± 0.05 vs. −0.04 ± 0.03 in RNAi-2) was significantly reduced by knockdown of Neat (Figures 6D,E). Knockdown of Neat did not change resting pHi in the principal cells of the transitional segment of MTs (Figure 6F). Taken together with our prior oocyte expression experiments these data strongly support the conclusion that Neat mediates significant HCO3−/Ox2− and HCO3−/SO42− exchange on the basolateral membrane of Drosophila MT principal cells of the transitional segment.
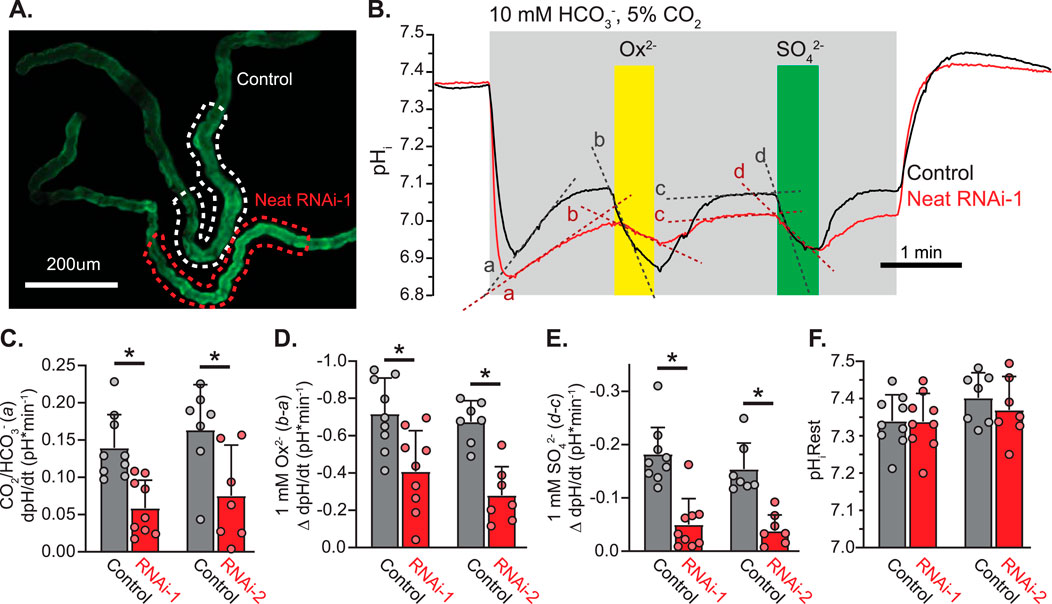
Figure 6. Drosophila CG5002 (Neat) contributes to basolateral HCO3−/Ox2− and HCO3−/SO42− exchange in transitional segment MTs ex vivo (A). Representative fluorescent micrograph of SEpH component of pHerry in MT principal cells from control (UAS-pHerry-myr; CapaR-GAL4) and Neat knockdown (UAS-pHerry-myr; UAS-RNAiKK100600; CapaR-GAL4) flies. (B) Representative traces of transitional segment principal cell pHi derived from calibrated pHerry fluorescent ratio demonstrating pH response to bath-applied CO2/HCO3−, Ox2−, and SO42− solutions. Traces correspond to dashed ROIs in A and represent the average of all principal cells within the transitional segment. Note recovery of pHi following an initial acidification in CO2/HCO3− solution is reversed by both oxalate and sulfate, although to a lesser extent when Neat is knocked down. (C–E) Change in the rate of pHi change in response to 5%CO2/10 mM HCO3− solution (pH 6.8) (C), Ox2− (D), and SO42− (E). RNAi-1 and RNAi-2 denote two separate RNA-i constructs targeting Neat (KK100600 and GD10058). Each experiment conducted with a control and Neat RNA-i MT in the same field of view as in (A). Slopes derived from a, b, c, and d in (B). Circles = single MTs. Bars = Mean + SD. * = p ≤ 0.05 by two-way unpaired Student’s t-test. (F) Initial pHi prior to 5%CO2/10 mM HCO3− solution (pH 6.8). Circles = individual MTs. Bars = Mean + SD.
3.5 Neat and CaOx crystallization in Drosophila MTs
We hypothesized that if Neat was a significant mediator of basolateral Ox2− transport in the Drosophila MT then knockdown of this transporter should significantly impair transepithelial transport of oxalate and formation of CaOx crystals in the MT lumen. Previous work has demonstrated that knockdown of the apical oxalate transporter in the MT significantly reduces luminal CaOx crystal formation when Ox2− solution is perfused across extracted Drosophila MTs (Landry et al., 2016). To test if this was the case for the putative basolateral Ox2− transporter, we assessed CaOx crystal burden in extracted MTs from control and Neat knockdown flies via polarizing birefringence. 60-min bath application of insect saline containing 10 mM Ox2− was sufficient to induce prominent crystal formation (Figure 7A). CaOx crystal count was significantly reduced when Neat was knocked down by both RNAi-1 and RNAi-2 constructs (12.64 ± 4.14 and 21.5 ± 9.39 for each construct respectively vs. 33.20 ± 8.67 in control, p < 0.0001 by one-way ANOVA with Bonferroni multiple comparisons correction; Figure 7B). These data suggest Neat significantly contributes to transepithelial Ox2− movement, and this contribution is of similar magnitude to that of the previously described apical Ox2− transporter dPrestin (Landry et al., 2016).
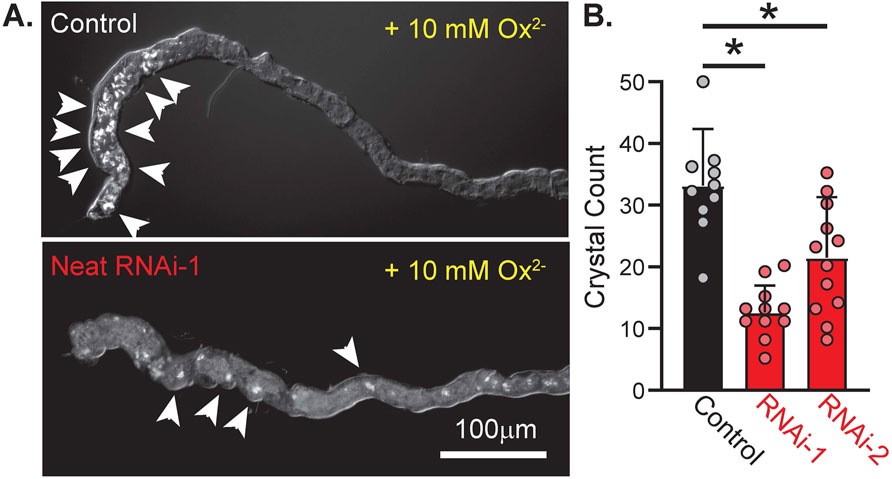
Figure 7. Knockdown of CG5002 (Neat) reduces accumulation of oxalate crystals in MT lumen during basolateral application of oxalate solution. (A) Representative polarized light images of ex vivo MTs from control (UAS-pHerry-myr; CapaR-GAL4) and Neat knockdown (UAS-pHerry-myr; UAS-RNAiKK100600; CapaR-GAL4) flies after 60 min exposure to bath-applied 10 mM Ox2− solution. Arrowheads denote prominent crystal formation. (B) Crystal count from lumen of MTs following 60-min Ox2− exposure. Circles indicate individual MTs. Bars = mean + SD. * = p ≤ 0.05 by one-way ANOVA with Bonferroni multiple comparisons tests.
4 Discussion
Drosophila melanogaster has been widely used as a credible model in exploring the pathophysiology of CaOx nephrolithiasis (Akouris et al., 2022; Al et al., 2020; Ali et al., 2018; Branco et al., 2021; Chen et al., 2012; Chen et al., 2011; Chi et al., 2015; Chung et al., 2016; El-Salam et al., 2018; El-Salam et al., 2017; Farkaš et al., 2016; Han et al., 2019; Hirata, et al., 2012a; Landry et al., 2019; Landry et al., 2016; Lu et al., 2024; Miller et al., 2013; Reynolds et al., 2024; Sun et al., 2022; Yang et al., 2018). Our previous experiments illustrated that Slc26a6 ortholog dPrestin mediated oxalate export on apical membrane of Drosophila renal tubule. To better understand how oxalate crosses into the MT lumen and affects CaOx crystallization in MTs, we undertook the present work to characterize another Drosophila Slc26 oxalate transporter. These studies focused on Drosophila GC5002 (Neat), and we found that Neat contributes to Ox2− transepithelial movement in MTs via electroneutral HCO3−/Ox2− and HCO3−/SO42− exchange. Sequence homology suggested several previously uncharacterized Drosophila oxalate transporters within the Slc26 family, but Ox2− uptake experiments only revealed significant oxalate transport via Neat and CG5404 (Figure 3A). Ox2− uptake by Neat was almost completely inhibited by SO42− and partly inhibited by DIDS in Cl− free solution (Figure 3B). Surprisingly, application of extracellular chloride did not inhibit Ox2− uptake by Neat, consistent with the interpretation that Neat does not function as an Ox2−/Cl− exchanger. Available expression data indicated that CG5404 was minimally expressed in the MT while Neat was highly expressed, suggesting it was the most likely candidate to function as the basolateral Ox2− transporter contributing to transepithelial Ox2− movement in concert with apical dPrestin.
Cis-application of SO42− blocked Ox2− uptake via Neat (Figure 3B), and electrophysiological recordings in Xenopus oocytes revealed both electroneutral HCO3−/Ox2− and HCO3−/SO42− exchange (Figures 4A,G). Taken together, these data suggest both Ox2− and SO42− share a binding site on Neat and ion exchange occurs via movement of base equivalents. An alternative explanation is that Neat engages in electroneutral exchange of HCO3− for another ion, and this activity is competitively inhibited by both Ox2− and SO42−. The observation that Neat does not reduce Ox2− uptake in response to Cl− (Figure 3B) makes this unlikely, but we cannot completely rule out this possibility as Neat mediates electroneutral HCO3− influx in both oocytes and MTs even in the absence of Ox2− and SO42− (slope “a” in Figures 3A,C and slope “a” in Figures 6B,C respectively). Future studies are needed to determine if this HCO3− influx is balanced by movement of Cl−, OH−, or metabolic substrates such as formate− (also transported by some Slc26 proteins).
We validated tools for measuring intracellular pH at a cellular scale within the principal cells that comprise the Drosophila MT (Rossano and Romero, 2017) (Figure 5). We combined these quantifiable live imaging tools with multiple RNAi constructs and tissue specific transgene expression to knockdown Neat in the principal cells of the MT while monitoring the effect on pHi changes elicited by basolateral perfusion of oxalate and sulfate in HCO3−/CO2 buffered saline (Figure 6). These experiments were in agreement with the prior heterologous expression systems data as they revealed HCO3−/Ox2− and HCO3−/SO42− exchange on the basolateral membrane of the MT. These transport modalities were significantly attributable to Neat as knockdown with two separate RNAi constructs, validated to reduce transcript levels to approximately 34% and 40% of control lines, significantly reduced base equivalent movement in the presence of both Ox2− and SO42− (Figures 6B–E). Although we cannot confirm the exact subcellular distribution of Neat within MT principal cells or the extent of Neat protein knockdown, the basolateral membrane is the most likely location as all of the changes in pHi were elicited by basolateral bath perfusion of substrates. Perfusion of the apical surface was likely minimal as the tubules are pinched shut when mounted on cover glass. Prior work (as well as data presented here; Figure 7) has demonstrated that genetic manipulation of Ox2− transport is sufficient to alter calcium oxalate crystal formation in the Malpighian tubule lumen (Landry et al., 2016). These results are inconsistent with persistent apical perfusion by bath application of solutions. Simultaneous availability of soluble oxalate to the apical and basolateral surfaces would make crystal formation a function of transepithelial Ca2+, not Ox2−, transport. Such transport has not been observed or hypothesized for either Neat or dPrestin.
Characterization of the basolateral Ox2− transporter within the MT is an important step in understanding Drosophila secretory biology, but we further characterized the role of Neat in contributing to a fly model of renal nephrolithiasis to demonstrate that modulation of Neat (and its mammalian functional orthologs) may present a novel target for therapeutics in oxalate kidney stone pathology. Knockdown of Neat reduced luminal CaOx crystal formation after bath application of 10 mM Na-oxalate (Figure 7). Mutations in human SLC26A1 which decrease transporter activity and impair membrane trafficking are associated with calcium oxalate nephrolithiasis. This is likely due to expression of SLC26A1 in the distal ileum, cecum, and proximal colon in humans, as disruption of basolateral Ox2− transporter in those organs leads to retention of oxalate, hyperoxaluria, and ultimately hyperoxaluria (Gee et al., 2016). The fly MT represents a much simpler and experimentally tractable system, and our experiments demonstrate that it recapitulates key aspects of oxalate transepithelial movement. Future studies will need to account for diverse expression patterns of transporters across different tissues. Additional studies in flies using dietary oxalate may resolve the contribution of Neat to whole animal oxalate processing, but such experiments were beyond the scope of this study as the full tissue expression profile and subcellular localization of Neat remains to be characterized.
5 Conclusion
In conclusion, Neat contributes to sulfate and oxalate transport at the Drosophila MT as it mediates transepithelial exchange of HCO3−/Ox2− and HCO3−/SO42−. Together with dPrestin on apical membrane, Neat forms the basolateral component of transepithelial oxalate movement (Figure 8). The studies presented here demonstrate the power of the fly MT in modeling the physiology of nephrolithiasis as we were able to characterize the transport modalities of Neat within an ex vivo cellular system using live imaging of GEpHis, cell-specific RNAi, and a whole organ functional assay of oxalate crystal formation. These data were in agreement with Neat activity in heterologous expression systems. This research not only fills a critical gap in our understanding of oxalate transport within the Drosophila MT but also lays the groundwork for further exploration into the genetic and molecular mechanisms underlying human nephrolithiasis and potential therapeutic targets.
Data availability statement
The raw data supporting the conclusions of this article will be made available by the authors, without undue reservation.
Ethics statement
The animal study was approved by Institutional Animal Care and Use Committee at Mayo Clinic and Brigham and Women’s Hospital. All experiments were carried out in accordance with these approved protocols (oocyte extraction). The study was conducted in accordance with the local legislation and institutional requirements.
Author contributions
AR: Conceptualization, Data curation, Formal Analysis, Funding acquisition, Investigation, Methodology, Resources, Validation, Visualization, Writing – original draft, Writing – review and editing. LZ: Funding acquisition, Investigation, Writing – original draft, Writing – review and editing. JA: Funding acquisition, Investigation, Writing – review and editing. HH: Investigation, Writing – review and editing, Data curation, Formal Analysis. AM: Formal Analysis, Investigation, Writing – review and editing. JD: Formal Analysis, Investigation, Writing – review and editing. DM: Writing – review and editing, Data curation, Funding acquisition. MR: Data curation, Funding acquisition, Writing – review and editing, Conceptualization, Formal Analysis, Investigation, Methodology, Project administration, Resources, Supervision, Validation, Visualization, Writing – original draft.
Funding
The author(s) declare that financial support was received for the research and/or publication of this article. The funding was from NIH: T32-DK007013 (AJR, LZ); R25-GM075148 (JBA); P01-DK070756, P50-AR060772 (DBM); DK092408, DK100227, DK128844, R25-DK101405 (MFR).
Acknowledgments
The authors would like to thank Gregory Macleod for the UAS-pHerry-myr flies and Julian A.T. Dow for the CapaR-GAL4 Drosophila stocks.
Conflict of interest
The authors declare that the research was conducted in the absence of any commercial or financial relationships that could be construed as a potential conflict of interest.
Publisher’s note
All claims expressed in this article are solely those of the authors and do not necessarily represent those of their affiliated organizations, or those of the publisher, the editors and the reviewers. Any product that may be evaluated in this article, or claim that may be made by its manufacturer, is not guaranteed or endorsed by the publisher.
Supplementary material
The Supplementary Material for this article can be found online at: https://www.frontiersin.org/articles/10.3389/fphys.2025.1468451/full#supplementary-material
References
Akouris P. P., Chmiel J. A., Stuivenberg G. A., Kiattiburut W., Bjazevic J., Razvi H., et al. (2022). Osteopontin phosphopeptide mitigates calcium oxalate stone formation in a Drosophila melanogaster model. Urolithiasis 51, 19. doi:10.1007/s00240-022-01395-2
Al K. F., Daisley B. A., Chanyi R. M., Bjazevic J., Razvi H., Reid G., et al. (2020). Oxalate-degrading Bacillus subtilis mitigates urolithiasis in a Drosophila melanogaster model. mSphere 5 (5), e00498-20. doi:10.1128/mSphere.00498-20
Ali S. N., Dayarathna T. K., Ali A. N., Osumah T., Ahmed M., Cooper T. T., et al. (2018). Drosophila melanogaster as a function-based high-throughput screening model for antinephrolithiasis agents in kidney stone patients. Dis. Model Mech. 11 (11), dmm035873. doi:10.1242/dmm.035873
Bissig M., Hagenbuch B., Stieger B., Koller T., Meier P. J. (1994). Functional expression cloning of the canalicular sulfate transport system of rat hepatocytes. J. Biol. Chem. 269, 3017–3021. doi:10.1016/s0021-9258(17)42040-0
Branco A. J., Vattamparambil A. S., Landry G. M. (2021). Lead (Pb(2+))-induced calcium oxalate crystallization ex vivo is ameliorated via inositol 1,4,5-trisphosphate receptor (InsP(3)R) knockdown in a Drosophila melanogaster model of nephrolithiasis. Environ. Toxicol. Pharmacol. 87, 103695. doi:10.1016/j.etap.2021.103695
Brand A. H., Perrimon N. (1993). Targeted gene expression as a means of altering cell fates and generating dominant phenotypes. Development 118, 401–415. doi:10.1242/dev.118.2.401
Chen A. P., Holmes H., Decker J. W., Chang M. H., Romero M. F. (2024). Disorder within order: identification of the disordered loop of STAS domain as the inhibitory domain in SLC26A9 chloride channel. J. Biol. Chem. 26 (301), 108145. doi:10.1016/j.jbc.2024.108145
Chen W. C., Lin W. Y., Chen H. Y., Chang C. H., Tsai F. J., Man K. M., et al. (2012). Melamine-induced urolithiasis in a Drosophila model. J. Agric. Food Chem. 60, 2753–2757. doi:10.1021/jf204647p
Chen Y. H., Liu H. P., Chen H. Y., Tsai F. J., Chang C. H., Lee Y. J., et al. (2011). Ethylene glycol induces calcium oxalate crystal deposition in Malpighian tubules: a Drosophila model for nephrolithiasis/urolithiasis. Kidney Int. 80, 369–377. doi:10.1038/ki.2011.80
Chesler M. (1990). The regulation and modulation of pH in the nervous system. Prog. Neurobiol. 34, 401–427. doi:10.1016/0301-0082(90)90034-e
Chi T., Kim M. S., Lang S., Bose N., Kahn A., Flechner L., et al. (2015). A Drosophila model identifies a critical role for zinc in mineralization for kidney stone disease. PLoS One 10, e0124150. doi:10.1371/journal.pone.0124150
Chung V. Y., Konietzny R., Charles P., Kessler B., Fischer R., Turney B. W. (2016). Proteomic changes in response to crystal formation in Drosophila Malpighian tubules. Fly. (Austin) 10, 91–100. doi:10.1080/19336934.2016.1171947
Dawson P. A., Russell C. S., Lee S., McLeay S. C., van Dongen J. M., Cowley D. M., et al. (2010). Urolithiasis and hepatotoxicity are linked to the anion transporter Sat1 in mice. J. Clin. Invest. 120, 706–712. doi:10.1172/JCI31474
Dube K., McDonald D. G., O’Donnell M. J. (2000). Calcium transport by isolated anterior and posterior Malpighian tubules of Drosophila melanogaster: roles of sequestration and secretion. J. Insect Physiol. 46, 1449–1460. doi:10.1016/s0022-1910(00)00069-x
El-Salam M. A., Bastos J., Han J. J., Previdi D., Coelho E. B., Donate P., et al. (2018). The synthesized plant metabolite 3,4,5-tri-O-galloyl quinic acid methyl ester inhibits calcium oxalate crystal growth in a Drosophila model, downregulates renal cell surface Annexin A1 expression, and decreases crystal adhesion to cells. J. Med. Chem. 61, 1609–1621. doi:10.1021/acs.jmedchem.7b01566
El-Salam M. A. A., Bastos J., Han J. J., Previdi D., Donate P., Romero M. F., Lieske J. C., et al. (2017). The plant 3,4,5-tri-O-galloyl quinic acid methyl ester inhibits calcium oxalate crystals growth in a Drosophila model and decreases renal cell annexin A1 surface expression and crystal adhesion. J. Am. Soc. Nephrol. 28 (10S), 278. doi:10.1681/ASN.20172810S11
Farkaš R., Pečeňová L., Mentelová L., Beňo M., Beňová-Liszeková D., Mahmoodová S., et al. (2016). Massive excretion of calcium oxalate from late prepupal salivary glands of Drosophila melanogaster demonstrates active nephridial-like anion transport. Dev. Growth Differ. 58, 562–574. doi:10.1111/dgd.12300
Gee H. Y., Jun I., Braun D. A., Lawson J. A., Halbritter J., Shril S., et al. (2016). Mutations in SLC26A1 cause nephrolithiasis. Am. J. Hum. Genet. 98, 1228–1234. doi:10.1016/j.ajhg.2016.03.026
Han S., Zhao C., Pokhrel G., Sun X., Chen Z., Xu H. (2019). Hydroxycitric acid tripotassium inhibits calcium oxalate crystal formation in the Drosophila melanogaster model of hyperoxaluria. Med. Sci. Monit. 25, 3662–3667. doi:10.12659/MSM.913637
Hirata T., Cabrero P., Bondeson D. P., Berkholz D. S., Thompson J. R., Ritman E., et al. (2012). In vivo Drosophilia genetic model for calcium oxalate nephrolithiasis. Am. J. Physiol. Ren. Physiol. 303 (303), F1555–F1562. doi:10.1152/ajprenal.00074.2012
Hirata T., Cabrero P., Dow J. A. T., Romero M. F. (2010). Drosophila Prestin provides an in vivo model for oxalate kidney stone formation. J. Am. Soc. Nephrol. 21 (11S), 486A. doi:10.1681/ASN.20102111S11
Hirata T., Czapar A., Brin L., Haritonova A., Bondeson D. P., Linser P., et al. (2012). Ion and solute transport by Prestin in Drosophila and Anopheles. J. Insect Physiol. 58, 563–569. doi:10.1016/j.jinsphys.2012.01.009
Karniski L. P., Lotscher M., Fucentese M., Hilfiker H., Biber J., Murer H. (1998). Immunolocalization of sat-1 sulfate/oxalate/bicarbonate anion exchanger in the rat kidney. Am. J. Physiol. 275, F79–F87. doi:10.1152/ajprenal.1998.275.1.F79
Krick W., Schnedler N., Burckhardt G., Burckhardt B. C. (2009). Ability of sat-1 to transport sulfate, bicarbonate, or oxalate under physiological conditions. Am. J. Physiol. Ren. Physiol. 297, F145–F154. doi:10.1152/ajprenal.90401.2008
Landry G. M., Furrow E., Holmes H. L., Hirata T., Kato A., Williams P., et al. (2019). Cloning, function, and localization of human, canine and Drosophila ZIP10 (SLC39A10), a Zn2+ transporter. Am. J. Physiol. Ren. Physiol. 316, F263–F273. doi:10.1152/ajprenal.00573.2017
Landry G. M., Hirata T., Anderson J. B., Cabrero P., Gallo C. J. R., Dow J. A. T., et al. (2016). Sulfate and thiosulfate inhibit oxalate transport via a dPrestin (Slc26a6)-dependent mechanism in an insect model of calcium oxalate nephrolithiasis. Am. J. Physiol. Ren. Physiol. 310, F152–F159. doi:10.1152/ajprenal.00406.2015
Lu Y., Wu Z., Du Z., Lin X., Tian E., Zhang F., et al. (2024). The anti-urolithiasis activity and safety of strangury-relieving herbs: a comparative study based on fruit fly kidney stone model. J. Ethnopharmacol. 326, 117968. doi:10.1016/j.jep.2024.117968
MacPherson M. R., Pollock V. P., Kean L., Southall T. D., Giannakou M. E., Broderick K. E., et al. (2005). Transient receptor potential-like channels are essential for calcium signaling and fluid transport in a Drosophila epithelium. Genetics 169, 1541–1552. doi:10.1534/genetics.104.035139
Mandal A. K., Mercado A., Foster A., Zandi-Nejad K., Mount D. B. (2017). Uricosuric targets of tranilast. Pharmacol. Res. Perspect. 5, e00291. doi:10.1002/prp2.291
Mandal A. K., Mount D. B. (2019). Interaction between ITM2B and GLUT9 links urate transport to neurodegenerative disorders. Front. Physiol. 10, 1323. doi:10.3389/fphys.2019.01323
Markovich D. (2011). Physiological roles of renal anion transporters NaS1 and Sat1. Am. J. Physiol. Ren. Physiol. 300, F1267–F1270. doi:10.1152/ajprenal.00061.2011
Miesenbock G., De Angelis D. A., Rothman J. E. (1998). Visualizing secretion and synaptic transmission with pH-sensitive green fluorescent proteins. Nature. 394 (394), 192–195. doi:10.1038/28190
Miller J., Chi T., Kapahi P., Kahn A. J., Kim M. S., Hirata T., et al. (2013). Drosophila melanogaster as an emerging translational model of human nephrolithiasis. J. Urol. 190, 1648–1656. doi:10.1016/j.juro.2013.03.010
Ohana E., Shcheynikov N., Moe O. W., Muallem S. (2013). SLC26A6 and NaDC-1 transporters interact to regulate oxalate and citrate homeostasis. J. Am. Soc. Nephrol. 24, 1617–1626. doi:10.1681/ASN.2013010080
Reynolds C. J., Gillen C. M., Burke R., Tsering Y., Loucks E., Judd-Mole S., et al. (2024). Drosophila ClC-c is a homolog of human ClC-5 and a new model for Dent disease type 1. Kidney360. 5, 414–426. doi:10.34067/KID.0000000000000352
Romero M. F., Henry D., Nelson S., Harte P. J., Dillon A. K., Sciortino C. M. (2000). Cloning and characterization of a Na+ driven anion exchanger (NDAE1): a new bicarbonate transporter. J. Biol. Chem. 275, 24552–24559. doi:10.1074/jbc.M003476200
Rossano A. J., Chouhan A. K., Macleod G. T. (2013). Genetically encoded pH-indicators reveal activity-dependent cytosolic acidification of Drosophila motor nerve termini in vivo. J. Physiol. 591, 1691–1706. doi:10.1113/jphysiol.2012.248377
Rossano A. J., Kato A., Minard K., Romero M. F., Macleod G. T. (2017). Na+/H+ exchange via the Drosophila vesicular glutamate transporter (DVGLUT) mediates activity-induced acid efflux from presynaptic terminals. J. Physiol. 595, 805–824. doi:10.1113/JP273105
Rossano A. J., Chouhan A. K., Macleod G. T. (2013). Genetically encoded pH-indicators reveal activity-dependent cytosolic acidification of Drosophila motor nerve termini in vivo. J. Physio. 21, 1691–1706. doi:10.1113/jphysiol.2012.248377
Rossano A. J., Romero M. F. (2017). Optical quantification of intracellular pH in Drosophila melanogaster malpighian tubule epithelia with a fluorescent genetically-encoded pH indicator. J. Vis. Exp. 126, e55698. doi:10.3791/55698
Shaner N. C., Campbell R. E., Steinbach P. A., Giepmans B. N. G., Palmer A. E., Tsien R. Y. (2004). Improved monomeric red, orange and yellow fluorescent proteins derived from Discosoma sp red fluorescent protein. Nat. Biotechnol. 22, 1567–1572. doi:10.1038/nbt1037
Shimshilashvili L., Aharon S., Moe O. W., Ohana E. (2020). Novel human polymorphisms define a key role for the SLC26A6-STAS domain in protection from Ca(2+)-oxalate lithogenesis. Front. Pharmacol. 11, 405. doi:10.3389/fphar.2020.00405
Southall T. D., Terhzaz S., Cabrero P., Chintapalli V. R., Evans J. M., Dow J. A., et al. (2006). Novel subcellular locations and functions for secretory pathway Ca2+/Mn2+-ATPases. Physiol. genomics 26, 35–45. doi:10.1152/physiolgenomics.00038.2006
Sun P., Liao S. G., Yang R. Q., Lu C. L., Ji K. L., Cao D. H., et al. (2022). Aspidopterys obcordata vine inulin fructan affects urolithiasis by modifying calcium oxalate crystallization. Carbohydr. Polym. 15 (294), 119777. doi:10.1016/j.carbpol.2022.119777
Terhzaz S., Cabrero P., Robben J. H., Radford J. C., Hudson B. D., Milligan G., et al. (2012). Mechanism and function of Drosophila capa GPCR: a desiccation stress-responsive receptor with functional homology to human neuromedinU receptor. PLoS One 7, e29897. doi:10.1371/journal.pone.0029897
Terhzaz S., O’Connell F. C., Pollock V. P., Kean L., Davies S. A., Veenstra J. A., et al. (1999). Isolation and characterization of a leucokinin-like peptide of Drosophila melanogaster. J. Exp. Biol. 202 (24), 3667–3676. doi:10.1242/jeb.202.24.3667
Thomas J. A., Buchsbaum R. N., Zimniak A., Racker E. (1979). Intracellular pH measurements in Ehrlich ascites tumor cells utilizing spectroscopic probes generated in situ. Biochemistry 18 (11), 2210–2218. doi:10.1021/bi00578a012
Vaughan-Jones R. D., Wu M. L. (1990). pH dependence of intrinsic H+ buffering power in the sheep cardiac Purkinje fibre. J. Physiol. 425, 429–448. doi:10.1113/jphysiol.1990.sp018112
Vincourt J. B., Jullien D., Amalric F., Girard J. P. (2003). Molecular and functional characterization of SLC26A11, a sodium-independent sulfate transporter from high endothelial venules. Faseb J. 17, 890–892. doi:10.1096/fj.02-0787fje
Wang X., Wang Q. (2024). Current dietary and medical prevention of renal calcium oxalate stones. Int. J. Gen. Med. 17, 1635–1649. doi:10.2147/ijgm.S459155
Xie Q., Welch R., Mercado A., Romero M. F., Mount D. B. (2002). Molecular characterization of the murine Slc26a6 anion exchanger: functional comparison with Slc26a1. Am. J. Physiol. Ren. Physiol. 283, F826–F838. doi:10.1152/ajprenal.00079.2002
Xu J., Barone S., Li H., Holiday S., Zahedi K., Soleimani M. (2011). Slc26a11, a chloride transporter, localizes with the vacuolar H(+)-ATPase of A-intercalated cells of the kidney. Kidney Int. 80, 926–937. doi:10.1038/ki.2011.196
Yang H., Male M., Li Y., Wang N., Zhao C., Jin S., et al. (2018). Efficacy of hydroxy-L-proline (HYP) analogs in the treatment of primary hyperoxaluria in Drosophila melanogaster. BMC Nephrol. 19 (19), 167. doi:10.1186/s12882-018-0980-8
Keywords: oxalate transport, Drosophila Slc26, bicarbonate transport, genetically encoded pH-sensor, renal epithelia, intracellular pH, Malpighian tubule
Citation: Rossano AJ, Zhang L, Anderson JB, Holmes HL, Mandal AK, Decker JW, Mount DB and Romero MF (2025) Ex vivo quantification of intracellular pH in Drosophila Malpighian tubule reveals basolateral HCO3−/oxalate exchange through a novel oxalate transporter “Neat”. Front. Physiol. 16:1468451. doi: 10.3389/fphys.2025.1468451
Received: 22 July 2024; Accepted: 04 April 2025;
Published: 28 April 2025.
Edited by:
Michael L. Jennings, University of Arkansas for Medical Sciences, United StatesReviewed by:
Kazuaki Homma, Northwestern University, United StatesZhifei Wang, St. John’s University, United States
Copyright © 2025 Rossano, Zhang, Anderson, Holmes, Mandal, Decker, Mount and Romero. This is an open-access article distributed under the terms of the Creative Commons Attribution License (CC BY). The use, distribution or reproduction in other forums is permitted, provided the original author(s) and the copyright owner(s) are credited and that the original publication in this journal is cited, in accordance with accepted academic practice. No use, distribution or reproduction is permitted which does not comply with these terms.
*Correspondence: Michael F. Romero, cm9tZXJvLm1pY2hhZWxAbWF5by5lZHU=
†ORCID: Adam J. Rossano, orcid.org/0000-0001-8959-106X; Lili Zhang, orcid.org/0000-0001-9007-5281; Jacob B. Anderson, orcid.org/0000-0003-1343-3908; Heather L. Holmes, orcid.org/0009-0003-0047-2980; Asim K. Mandal, orcid.org/0000-0001-9044-9095; James W. Decker, orcid.org/0009-0004-6134-4015; David B. Mount, orcid.org/0000-0003-0310-2304; Michael F. Romero, orcid.org/0000-0002-1555-7835