- 1Center for Infectious Disease Control, National Institute for Public Health and the Environment, Bilthoven, Netherlands
- 2Center for Translational Immunology, University Medical Center Utrecht, Utrecht, Netherlands
- 3Theoretical Biology and Bioinformatics, Utrecht University, Utrecht, Netherlands
CD8+ T cells play an important role in protection against viral infections. With age, changes in the T-cell pool occur, leading to diminished responses against both new and recurring infections in older adults. This is thought to be due to a decrease in both T-cell numbers and T-cell receptor (TCR) diversity. Latent infection with cytomegalovirus (CMV) is assumed to contribute to this age-associated decline of the immune system. The observation that the level of TCR diversity in the total memory T-cell pool stays relatively stable during aging is remarkable in light of the constant input of new antigen-specific memory T cells. What happens with the diversity of the individual antigen-specific T-cell repertoires in the memory pool remains largely unknown. Here we studied the effect of aging on the phenotype and repertoire diversity of CMV-specific and Epstein-Barr virus (EBV)-specific CD8+ T cells, as well as the separate effects of aging and CMV-infection on the EBV-specific T-cell repertoire. Antigen-specific T cells against both persistent viruses showed an age-related increase in the expression of markers associated with a more differentiated phenotype, including KLRG-1, an increase in the fraction of terminally differentiated T cells, and a decrease in the diversity of the T-cell repertoire. Not only age, but also CMV infection was associated with a decreased diversity of the EBV-specific T-cell repertoire. This suggests that both CMV infection and age can impact the T-cell repertoire against other antigens.
Introduction
CD8+ T cells play an important role in the control and clearance of viral infections. One of the key components of a protective T-cell response is the recognition of viral epitopes via the T-cell receptor (TCR). T-cell receptors are formed via the random process of somatic V(D)J-recombination, leading to a large collection of TCRs with different specificities (Market and Papavasiliou, 2003). It is generally assumed that the diversity of the T-cell receptor repertoire is positively correlated with the level of protection against infectious diseases (Turner et al., 2009). The diversity of the total CD8+ T-cell repertoire decreases with age (Britanova et al., 2014, 2016; Yoshida et al., 2017), which is mainly caused by a decrease in naive T-cell numbers (Britanova et al., 2014) as well as a decreased TCR diversity within the naive T-cell pool (Qi et al., 2014; Egorov et al., 2018). Together with less efficient priming of T cells (Briceno et al., 2016), this may explain why both CD8+ T-cell protection against viral infections and vaccine efficacy decrease with age (Goronzy et al., 2001; Deng et al., 2004).
Although the level of TCR diversity within the total CD8+ T-cell memory pool seems to remain stable with age (Qi et al., 2014), the composition of the memory T-cell pool keeps changing at the antigen-specific level. Exposure to new antigens during life leads to recruitment of new T-cell specificities into the memory pool, and existing memory T-cell clones may expand or contract. The diversity of T cells that are already present in the memory T-cell pool may be affected by the arrival of new memory T-cell specificities, due to competition for T-cell growth and survival factors. The relative stability of the diversity of the total CD8+ T-cell memory pool therefore does not imply that individual antigen-specific T-cell repertoires are stably maintained with age. To study such changes in the memory T-cell pool, we investigated how the diversity of the antigen-specific TCR repertoires against cytomegalovirus (CMV) and Epstein Barr virus (EBV) changes with age.
Both CMV and EBV cannot be cleared from the body and repeatedly challenge the immune system, leading to high, and therefore easily detectable, frequencies of antigen-specific CD8+ T cells over all ages in the majority of individuals (Khan et al., 2004; Sukdolak et al., 2013). Previous longitudinal studies focusing on the effect of aging on the antigen-specific repertoire have suggested that the T-cell repertoires against EBV and CMV remain relatively stable, at least during the first few years after primary infection, as the same T-cell clones were identified at different timepoints (Annels et al., 2000; Hadrup et al., 2006; Iancu et al., 2009; Klarenbeek et al., 2012). Consistent with this, several cross-sectional studies into the CMV and EBV-specific T-cell repertoires reported similar Vβ-skewing in young and older adults, and even identical TCR sequences between individuals of different age groups (Khan et al., 2002; Schwanninger et al., 2008; Cardenas Sierra et al., 2014). Although these studies have led to the view that antigen-specific T-cell repertoires are rather stable with age, it remains unknown, if the diversity of antigen-specific T-cell repertoires is maintained (Lanfermeijer et al., 2020).
The cellular immune response against CMV is even more pronounced than the response against EBV, and can reach up to 40% of the CD8+ T-cell pool in the blood (Khan et al., 2002; Remmerswaal et al., 2015). Furthermore, CMV-infection leads to changes in the CD8+ T-cell pool similar to those observed with aging, including the presence of large fractions of terminally differentiated cells (Almanzar et al., 2005; Chidrawar et al., 2009) and a more skewed and less diverse TCR repertoire (Khan et al., 2002; Nikolich-Zugich, 2008). It has been suggested that the large numbers of CMV-specific T cells can compete with non-CMV-specific T cells (Pawelec et al., 2005; Derhovanessian et al., 2009; Tu and Rao, 2016), leading to memory attrition (Sad and Krishnan, 2003). Studies on the effect of CMV-infection on non-CMV-specific T cells are not unambiguous, however. Several studies have shown that mice infected with murine CMV (MCMV) have impaired responses to heterologous infections (Cicin-Sain et al., 2012; Mekker et al., 2012; Smithey et al., 2012; Redeker et al., 2017). In contrast, another study observed a positive effect of MCMV infection on the diversity of the T-cell repertoire specific for a heterologous infection (Smithey et al., 2018). Studies in humans showed similarly contradicting results: while one study showed that CMV+ individuals had lower absolute numbers of EBV-specific T cells than CMV− individuals (Khan et al., 2004), another study found that the diversity of the non-CMV-specific memory T-cell repertoire was comparable in CMV− and CMV+ individuals, thereby suggesting that the memory T-cell pool simply expands to accommodate the large frequencies of CMV-specific T cells (Lindau et al., 2019). Thus, the effect of CMV infection on non-CMV-specific T-cell responses and their repertoire diversity, and how this is linked to aging, remains poorly understood (Lanfermeijer et al., 2020).
To gain insight into the maintenance of the repertoire of antigen-specific T cells, we studied the effect of aging on the phenotype and TCR repertoire composition of T cells specific for two immune-dominant CMV and EBV epitopes. In addition, we investigated how the EBV-specific T-cell repertoire is influenced by CMV-infection and how this is linked to aging. We observed that the richness of the CMV-specific and EBV-specific T-cell repertoire declines with age, independent of CMV serostatus. CMV infection led to a further decrease in diversity of the EBV-specific T-cell repertoire. This suggests that CMV infection and age both play an important role in the diversity of the antigen-specific T-cell repertoires.
Methods and Materials
Study Design
Samples of healthy individuals covering a broad age range were combined from two cohorts. Samples of young adults (n = 34) between 18 and 52 years of age, from a cohort of unvaccinated controls or pre-vaccination participants, were used from a study carried out in 2009–2011 (the Pandemic influenza vaccination trial, Netherlands Trial Register NL1952) (Rosendahl Huber et al., 2018). The study was approved by the Central Committee on Research Involving Human Subjects of the Netherlands. Samples of older adults (n = 57), ≥60 years of age, were control samples from a study carried out in 2014–2015 (Influenza-like-illness-3, NL4666) (Kaaijk et al., submitted). This study was approved by the acknowledged ethical committee, METC Noord Holland. Both studies were carried out in accordance with the recommendations of Good Clinical Practice with written informed consent from all subjects, in accordance with the Declaration of Helsinki. See Supplementary Figure 1 for a flowchart of the selection criteria of the donors used for the analysis.
Cytomegalovirus (CMV)-Specific and Epstein Barr Virus (EBV)-Specific Antibodies
For healthy young adults, CMV-specific antibody levels were measured using a commercial ELISA kit (IBL international GMBH) according to manufacturer's instructions. Participants with a CMV antibody level of ≥12 U/ml or higher were considered CMV+, those with a level of ≤8 U/ml were considered CMV−. None of the participants included in this study scored between the 8 and 12 U/ml range. For older healthy adults, CMV-specific antibody levels and EBV-specific antibody levels were simultaneously measured in serum by an in-house-developed multiplex immunoassay (Tcherniaeva et al., 2018). Individuals with a CMV-specific antibody level of ≤4 RU (relative units)/ml were considered to be CMV− and individuals with an antibody level >7.5 RU/ml were considered CMV+. None of the participants included in this study had a CMV-specific antibody level between 4 and 7.5 RU/ml. Individuals were considered EBV- with an antibody level of ≤16 RU/ml, whereas those with an antibody level of >30 RU/ml were considered EBV+. None of the older participants included in this study had an EBV−specific antibody level between 16 and 30 RU/ml. Note that the EBV-status of the younger individuals remained unknown, therefore only individuals with high EBVA2−GLC-specific T-cell frequencies were used in our analysis.
PBMC and Serum Isolation
Peripheral blood mononuclear cells were obtained by Lymphoprep (Progen) density gradient centrifugation from heparinized blood, according to the manufacturer's instructions. PBMCs were frozen in 90% fetal calf serum and 10% dimethyl sulfoxide at −135°C until further use. Serum was isolated out of tubes with clot-activation factor and stored at −80°C until further use.
Antigen-Specific T Cells by Flow Cytometry
HLA-A2 positive healthy individuals were selected for subsequent EBV-specific and CMV-specific T cell analysis, by staining PBMCs for expression of HLA-A2 with the HLA-A2(BB7.2)-V450 antibody (BD Bioscience). From the HLA-A2 positive individuals, ±4 million PBMCs were stained using the HLA-class I dextramer containing the GLCTLVAML epitope of the BMLF1 protein of EBV (A*0201/GLCTLVAML-APC, Immudex) or the NLVPMVATV epitope of the pp65 protein of CMV (A*0201/NLVPMVATV-APC, Immudex), for 20 min at room temperature to assess their virus-specific T-cell frequencies.
Surface staining was performed for 30 min at 4°C with the following antibodies: Fixable Viability Staining-780 (BD bioscience), CD3 (SK7)-AF700(BD bioscience), CD8(RPA-T8)-BrilliantViolet510, CD45RO(UCHL1)-BrilliantViolet711, CD27(O323)-BrilliantViolet786, CCR7(150503)-BrilliantUV395 (BD bioscience), KLRG-1(13F12F2)-PE-Cy7 (eBioscience), PD-1(EH12.2H7)-PerCP Cy5.5, CD95(DX2)-BrilliantViolet421 (BD Biosciences), CD127(A019D5)-BrilliantViolet650, CD57(HCD57)-PE, and CXCR3(G025H7)-PE-Dazzle. All antibodies were purchased from Biolegend, unless stated otherwise. Acquisition was performed on a LSRFortessaX20 and data analysis was performed using FlowJo (Treestar). tSNE-analyses were performed using Cytobank (www.cytobank.org) (Kotecha et al., 2010) on 30 randomly selected dextramer-positive CD8+ T cells per sample and labeled with epitope-specificity, age, and CMV-serostatus. The tSNE clustering was performed on all these data combined (including both antigen-specificities). Perplexity of the clustering was set at 100. Cofactors for ArcSinH transformation were calculated using the flowVS package for R (https://www.bioconductor.org/packages/release/bioc/html/flowVS.html). Both packages were slightly adapted to allow for FlowCytometric data analysis and integrated in an in-house developed pipeline.
Isolation of Antigen-Specific T Cells for T-Cell Receptor Analysis
CD8+ T cells were isolated from PBMCs using a negative selection microbeads kit (Miltenyi Biotec) according to the manufacturer's protocol. Next, CD8+ T cells were labeled at room temperature for 20 min with the A*0201/GLCTLVAML-APC dextramer and with the A*0201/NLVPMVATV-APC dextramer for CMV+ individuals. Subsequently surface staining was performed using the following mAbs: CD3(UCHT1)-PerCP (Biolegend), CD4(OKT4)-BV510 (Biolegend), and CD8(RPA-T8)-FITC (Biolegend). CD3+CD4−CD8+dextramer+ cells were then sorted by FACS Melody (BD) directly into RNAlater (Ambion Inc. Applied Biosystems) and stored at −80°C for subsequent TCRβ clonotype analysis.
Preparing TCRβ cDNA Libraries for Sequencing
TCRβ analysis was performed as described previously (Shugay et al., 2014), with minor modifications. Briefly, mRNA was isolated with the RNA microkit (Qiagen) according to the manufacturer's protocol. Isolated mRNA was used for cDNA synthesis with 5′RACE template switch technology to introduce a universal primer binding site, and unique molecular identifiers (UMIs) were added at the 5′ end of the cDNA molecules using the SMARTScribe Reverse Transcriptase (TaKaRa). cDNA synthesis was followed by an AMPure XP bead-based clean-up (Beckman Coulter). Purified cDNA molecules were amplified in two subsequent PCR steps (25 cycles in PCR1 and 20 cycles in PCR2) using the Q5® High-Fidelity DNA Polymerase (New England BioLabs), with an AMPure XP bead-based clean-up in between. PCR products were size-selected on gel and purified using the Nucleospin PCR clean-up kit (Machery-Nagel). The PCR products were sequenced via Illumina MiSeq paired end 2x250 nucleotide (nt) sequencing.
TCRβ Clonotype Analysis
Raw sequencing data were processed using the 12nt UMIs to correct for amplification biases and error-correction of reads. RTCR (Gerritsen et al., 2016) was used to identify both the UMI sequence and clonotype information from the reads. Because of the relatively small number of cells per sample, additional filtering steps were followed to minimize cross-sample contamination and biases introduced by errors in the UMI sequence. Sequences were only accepted if their UMI was observed in at least 40 sequencing reads. Sequences with identical UMIs in multiple samples were removed if they did not occur in at least 1,000 sequencing reads or if their absolute frequency was lower than 10% of the maximum frequency in the other samples. UMIs were clustered within each sample if they were within a Hamming distance of 3. More detailed information about the processing and filtering of reads is provided in the Supplementary Material.
Clonotypes were defined by their CDR3 amino acid sequence and V and J segment. Our sequencing reads do not always allow to distinguish between very similar V-segments, e.g., TRBV12-3 and TRBV12-4, which are annotated as V12-3/4 in Figure 3, Supplementary Figures 4, 5, and Supplementary Tables 1, 2A,B.
For measuring diversity, the richness (defined as number of distinct clonotypes) of each sample was determined using normalized sample sizes (i.e., by iteratively sampling, without replacement, a given number of UMIs from the full set of UMIs that were identified in the sample). This approach accounts for the fact that the number of RNA molecules sampled may differ between cells. Diversity was calculated using the previously described Simpson's diversity index (Venturi et al., 2007). This index ranges between 0 and 1, with 0 representing minimal diversity and 1 representing maximal diversity. Sequence generation probabilities (CDR3+ V and J segments) were calculated using the default recombination model of OLGA (Sethna et al., 2019). Known antigen specificity of sequences was assessed using the VDJdb (Shugay et al., 2017; retrieved on 29 October 2020). Sequences from CMVA2−NLV-and EBVA2−GLC-specific samples were counted as a match if their V gene + CDR3 amino acid sequence + J gene was listed as a human TCRbeta sequence specific for the NLVPMVATV or GLCTLVAML epitope, respectively.
Statistical Analysis of Flow Cytometry Data
Differences between the groups (for example CMV− compared to CMV+) were assessed using Mann-Whitney U-tests. Correlations were tested with Spearman's rank correlation coefficient. For all analyses, p-values < 0.05 were considered statistically significant. Data were analyzed using GraphPad Prism 8.3 and SPSS statistics 22 for Windows (SPSS Inc., Chicago, IL, USA).
Results
Characteristics of the Study Population
Healthy HLA-A2 positive individuals were on average 57.8 ± 19.0 years old (n = 91, range 21–82 years) and 57.1% of these individuals were CMV+. Samples were obtained from two different cohorts, one containing young adults (21–52 years old) (n = 34), and one containing older adults (≥60 years old) (n = 57), of whom respectively 55.9 and 57.9% were CMV+ (Table 1). No significant differences in age or sex were observed between CMV− and CMV+ individuals. Supplementary Figure 1 gives a flowchart of the selection criteria of the donors used for the analysis.
Changes in the Phenotype of Antigen-Specific CD8+ T Cells With Age
To study the association between age and the antigen-specific CD8+ T-cell frequencies against EBV and CMV, we performed a dextramer-staining with one immuno-dominant epitope of CMV (NLVPMVATV) derived from the pp65 protein and one immune-dominant epitope of EBV (GLCTLVAML) derived from the BMLF-1 protein, both presented on the HLA-A2 molecule. We investigated the T-cell frequency against these two epitopes at different ages. The frequencies of CMVA2−NLV-specific T cells tended to be slightly higher than the frequencies of EBVA2−GLC-specific T cells [median of 0.220% vs. median of 0.120%; P = 0.0990 (ns)] (Figure 1A). We observed less inter-individual heterogeneity in the CMVA2−NLV-specific T-cell frequencies of younger compared to older adults; in the older adults, in whom these frequencies ranged from very low (from 0.01%) to very high (up to 17%) (Figure 1B, left panel). EBVA2−GLC-specific T-cell frequencies were not significantly associated with the age of the individuals (Figure 1B, right panel).
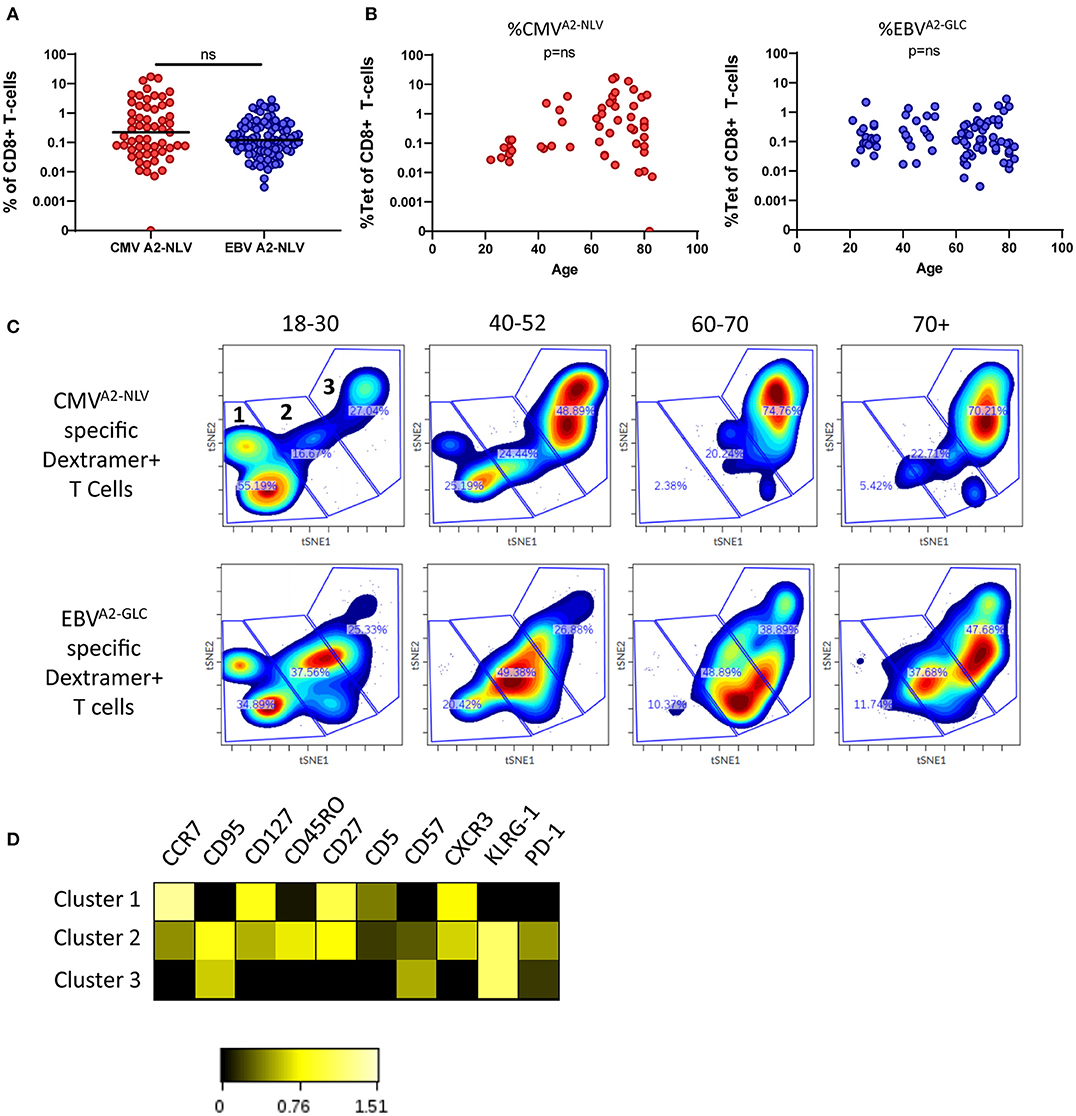
Figure 1. Changes in the phenotype of antigen-specific CD8+ T cells with age. (A) Percentage of CMVA2−NLV-specific CD8+ T cells (red, n = 56) and EBVA2−GLC-specific CD8+ T cells (blue, n = 99). Horizontal lines represent group median. (B) Percentage of CMVA2−NLV (left) and EBVA2−GLC-specific (right) CD8+ T cells as a function of age. (C) t-SNE analysis of CMVA2−NLV-specificand EBVA2−GLC-specific CD8+ T cells of donors (n = 47 and n = 77, respectively) categorized in four age groups. Clustering is based on MFI of CD5, PD-1, CD57, KLRG-1, CXCR3, CCR7, CD45RO, CD95, CD27, and CD127 of both epitopes. From each sample 30 cells were used. Three large clusters were manually identified. (D) Heatmap of expression of markers of the three t-SNE clusters. Clustering of t-SNE based on both CMVA2−NLV and EBVA2−GLC-specific CD8+ T cells. Heatmap was based on the Log10 ratio of the median expression of the markers, normalized per marker to its column's minimum. Difference between epitopes was compared by Mann Whitney U-test. Correlations were tested with Spearman's rank correlation coefficient, ns stands for a non-significant p-value.
To assess the association between age and the phenotype of CMVA2−NLV and EBVA2−GLC-specific T cells, we performed a cluster analysis (tSNE) based on the expression of the memory T-cell markers CD27, CCR7, CD95, CD45RO, and CXCR3, on CD57 and KLRG-1, which are associated with a more differentiated phenotype and on the inhibitory receptor PD-1, and CD5, which plays a role in TCR signaling (Voisinne et al., 2018). For the tSNE analysis the same amount of antigen-specific T cells (i.e., 30) per sample was used. The very same clustering was applied on the samples in the four age groups and per epitope-specificity. We observed clear differences in the subset distribution between these groups for both CMVA2−NLV and EBVA2−GLC-specific T cells (Figure 1C). We identified 3 large clusters (1–3, Figure 1C, upper left panel), in which cluster 1 contains Central memory type markers CCR7high, CD27high, KLRG-1low, CD57low cells, while cluster 3 contains the more differentiated cells, expressing CCR7low, CD27low, and KLRG-1high. Cluster 2 forms an intermediate cluster based on the expression of these markers (Figure 1D). Despite relatively large inter-individual variation (Supplementary Figure 2A), our data suggest a shift from cluster 1 to cluster 3 for both CMVA2−NLV and EBVA2−GLC-specific T cells with age (Figure 1C, Supplementary Figure 2A). This shift in clusters occurs earlier and becomes more pronounced with age for CMVA2−NLV-specific T cells than for EBVA2−GLC-specific T cells.
Phenotypic Changes of CMV-Specific and EBV-Specific T Cells Are Differently Associated With Age
We next explored how these phenotypic changes associated with age by quantifying the expression of various markers in the individual samples of CMVA2−NLV-specific and EBVA2−GLC-specific T cells. This allowed us to use the expression data of all the dextramer+ T cells. The composition of the memory population for the different ages based on conventional gating supported our observations of the cluster analysis. The memory subsets were defined based on the expression of CD27 and CD45RO in which the CD27−CD45RO− subset is referred to as Temra cells. We found a positive association between age and the percentage of Temra cells for both CMVA2−NLV-specific T cells [p = 0.0032, r = 0.4167, slope of 0.75%/year (p = 0.0006)] and, albeit to a lesser extent, EBVA2−GLC-specific T cells [p = 0.0010, r = 0.0.3796, slope of 0.25%/year (p = 0.1045)] (Figure 2A). The fraction of Temra cells correlated positively with the frequency of CMVA2−NLV-specific T cells, but not with the percentage of EBVA2−GLC-specific T cells (Supplementary Figure 3A). For EBVA2−GLC-specific T cells, the proportion of effector memory cells (Tem, CD27−CD45RO+) increased significantly with age (p < 0.0001, r = 0.5001) (Supplementary Figure 3B, right panel). The cluster analysis based on MFI from Figure 1 also showed a gradual decrease in CCR7 expression with age, which was confirmed by plotting the geometric mean of the fluorescence intensity (gMFI) of CCR7 on a continuous scale for both CMVA2−NLV-specific (p < 0.0001, r = 0.0.4411) and EBVA2−GLC-specific T cells (p = 0.0020, r = 0.3604) (Figure 2B).
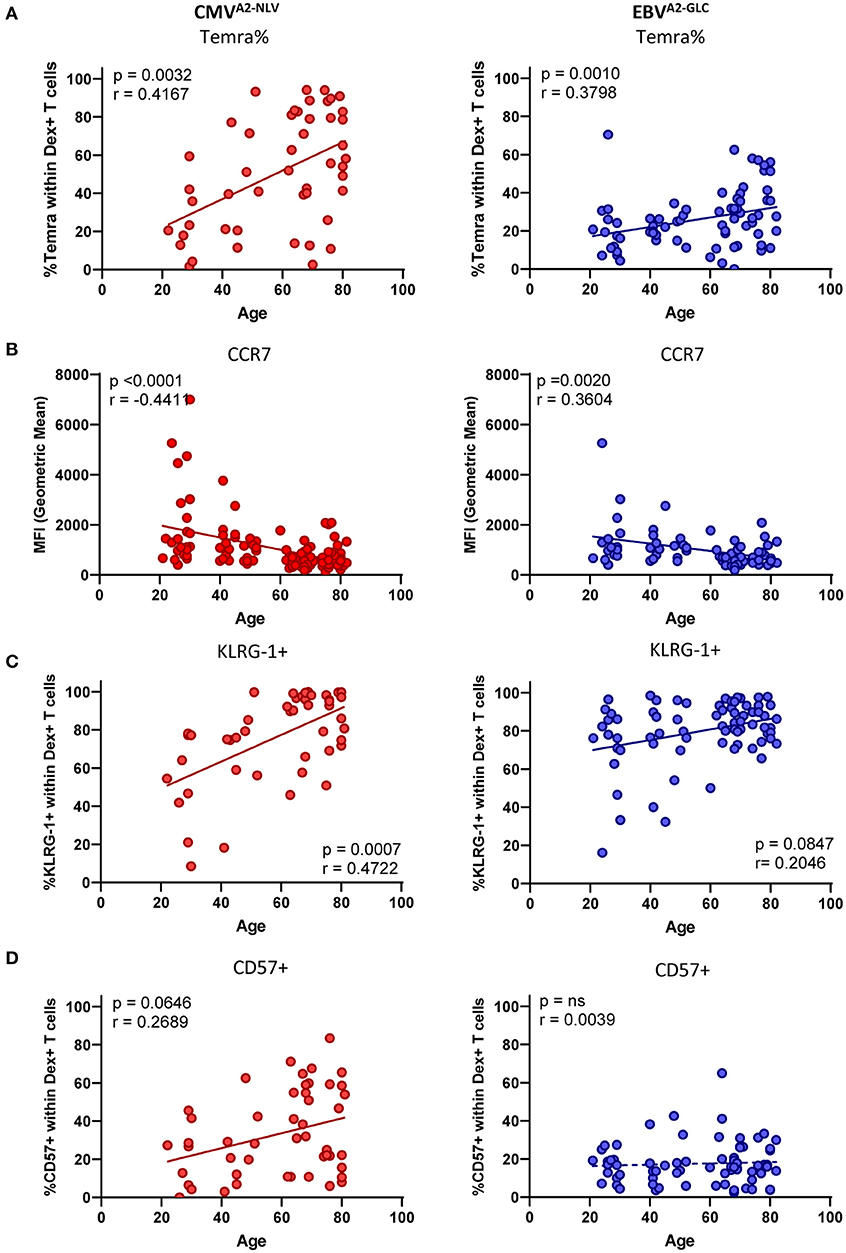
Figure 2. The phenotype of CMV-specific and EBV-specific T cells is affected differently by age. (A–D) Phenotype analysis of both CMVA2−NLV-specific (red) and EBVA2−GLC-specific CD8+ T cells (blue) plotted against age. (A) Percentage of Temra cells (CD27–, CD45RO–). (B) Geometric Mean of the fluorescent intensity of CCR7. Percentage of KLRG-1+ (C) and CD57+ (D) within Dextramer+ CD8+ T cells. Solid lines indicate a slope significantly (p < 0.05) different from a slope of 0, whereas a dotted line indicates no significant difference. Only donors with a sufficient T-cell response (at least 25 cells) were used for the phenotypical staining. Correlations were tested with Spearman's rank correlation coefficient.
Next, we analyzed the expression of markers associated with a more differentiated phenotype and exhaustion-associated markers on the antigen-specific T cells more closely. Both CMVA2−NLV- and EBVA2−GLC-specific T cells are associated with high expression of the inhibitory marker KLRG-1. CD57 is a senescence marker known to be specifically highly expressed by CMV-specific T cells, compared to T cells against other chronic viruses (Hoji et al., 2007; van den Berg et al., 2019). We indeed found high percentages of KLRG-1+ cells for both CMVA2−NLV-specific (mean of 76.6%) and EBVA2−GLC-specific T cells (mean of 79.8%), and the percentage of KLRG-1+ cells was positively associated with age, both CMVA2−NLV-specific (p = 0.0007, r = 0.4722) and EBVA2−GLC-specific T cells (p = 0.0847, r = 0.2046) (Figure 2C). The percentage of CD57+ T cells showed a positive trend with age for CMVA2−NLV-specific T cells (p = 0.0645, r = 0.2689), while it was low across all ages for EBVA2−GLC- specific T cells (Figure 2D). We observed a significant positive association between the frequency of CMVA2−NLV-specific T cells and the percentage of KLRG-1+, CD57+ cells in the CMVA2−NLV-specific T-cell population (Supplementary Figure 3A, upper panels). The frequency of EBVA2−GLC-specific T cells, in contrast, was only positively associated with the percentage of KLRG-1+ EBVA2−GLC-specific T cells (Supplementary Figure 3A, lower panels), and not with the percentage of CD57+ EBVA2−GLC-specific T cells.
We also investigated the percentage of CMVA2−NLV-specific and EBVA2−GLC-specific T cells expressing PD-1, which in the context of chronic (active) infection is often used as an exhaustion marker (Jubel et al., 2020). We found no significant correlation between the percentage of PD-1+ cells in the antigen-specific T-cell pool and age, both for EBV-specific and for CMV-specific T cells (Supplementary Figure 3C).
The CMVA2-NLV-Specific Repertoire Is Less Diverse Than the EBVA2-GLC-Specific Repertoire
We then investigated the TCR repertoire of CMVA2−NLV-specific and EBVA2−GLC-specific T cells in our samples by sequencing the TCRβ-chain. We used unique molecular identifiers (UMIs) to correct for sequencing errors and unequal PCR amplification, and performed an additional filtering procedure to exclude sequences that were likely due to contamination between samples or mutation in the UMI sequence (see Supplementary Material for more details). We proceeded with the samples in which at least 10 UMI-TCR pairs remained after filtering. The distribution of the TCRβ sequences per individual are shown in Figure 3 (see Supplementary Figure 4 for samples with <10 UMI-TCR pairs, all identified TCR sequences are provided in Supplementary Tables 1, 2A,B), with colors indicating the TCRβ sequences that are shared between individuals. We observed two different TCR sequences that were shared between individuals in the CMVA2−NLV-specific repertoire samples, and eight in the EBVA2−GLC-specific repertoire samples (Supplementary Figures 5A,B). A substantial fraction of the observed TCR sequences were also present in the VDJ database (VDJdb) of reported antigen-specific TCR sequences (Shugay et al., 2017) (Supplementary Figure 5C). We found that the sequences that were shared between individuals within our study or between an individual of our study and the VDJdb had an over 16-fold higher generation probability than those that were not shared (Supplementary Figure 5D). This supports the idea that the likelihood of TCR generation plays an important role in the presence, abundance and sharing of antigen-specific TCR sequences (Venturi et al., 2006, 2008; Elhanati et al., 2018).
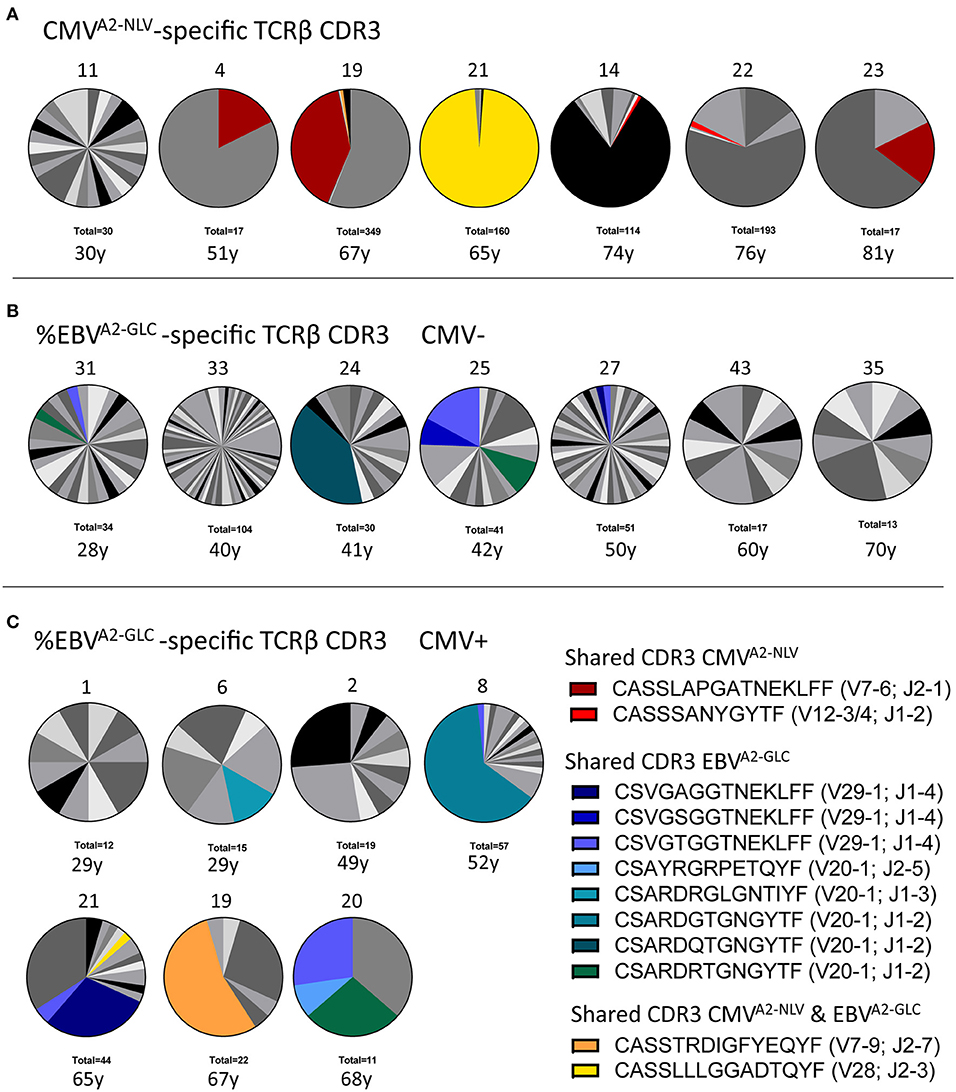
Figure 3. Characterization of the antigen-specific TCRβ repertoire. (A) Distribution of TCRβ sequences in samples of CMVA2−NLV-specific CD8+ T cells (n = 7). (B,C) T-cell repertoire of EBVA2−GLC-specific CD8+ T cells of both CMV− (B) and CMV+ individuals (C). Each pie depicts the repertoire of a different sample, with its Donor ID on top and the total number of UMI-TCR pairs identified at the bottom, as well as the individual's age. Colors represent shared TCRβ sequences between donors. Gray scales depict unique TCRβ sequences. Note two shared sequences between the CMVA2−NLV and EBVA2−GLC sample of two single individuals (yellow and orange). As this sharing was limited to these single donors and involved a very abundant TCRβ in either one of the samples, we expect that this overlap occurred during the sorting of the cells, probably due to unspecific binding of the dextramer.
To investigate the diversity of the antigen-specific T-cell repertoire, we used several measures of TCR diversity. The absolute richness, i.e., the total number of distinct clonotypes observed in a sample, was significantly higher for the EBVA2−GLC-specific T cells than for the CMVA2−NLV-specific T cells (p = 0.0289) (Figure 4A), even though the frequency of EBVA2−GLC-specific T cells was lower than that of CMVA2−NLV-specific T cells. However, the absolute richness is largely influenced by the total number of UMI-TCR pairs in a sample, i.e., the number of cDNA molecules that were sequenced. To overcome this potential bias, we therefore also used several alternative measures of TCR diversity. First, we calculated the unique clonotype ratio, by dividing the number of unique clonotypes by the total number of TCR sequences in each sample. Next, we calculated a normalized richness by counting the number of distinct TCR sequences in equally sized subsamples of the actual samples (by taking the mean richness of 10 randomly chosen subsamples). We also calculated the Simpson's diversity index as a sample size-independent measure of the TCR diversity in each sample (Venturi et al., 2007). While richness quantifies the variety of different TCRs, Simpson's diversity index quantifies the evenness of the frequency distribution across the TCRs. Even after excluding differential sample sizes as a confounding factor, we found a higher TCR diversity in the EBVA2−GLC-specific compared to the CMVA2−NLV-specific T-cell repertoire (Figure 4B). We repeated these analyses on the subset of samples that contained at least 20 UMI-TCR pairs, which did not change the results qualitatively.
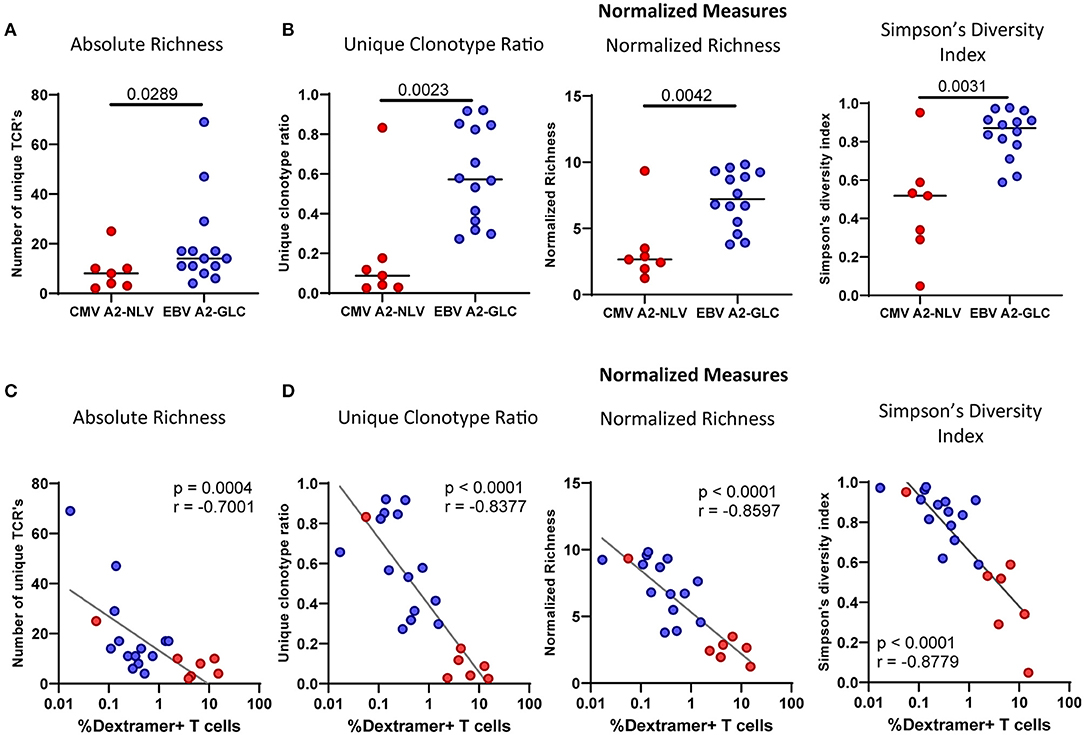
Figure 4. The CMVA2−NLV-specific TCRβ repertoire is less diverse than the and EBVA2−GLC-specific TCRβ repertoire. Repertoire diversity calculations of the CMVA2−NLV and EBVA2−GLC-specific CD8+ T-cell repertoire. (A) Richness calculations based on the number of different TCRβ sequences. (B) Measures normalized for differential sample sizes: Unique Clonotype Ratio (left), normalized richness (middle), and Simpson's Diversity Index (right panel). (C,D) Correlation plot of the repertoire richness (C) and normalized diversity (D) and the frequency of the CMVA2−NLV-specific (red) and EBVA2−GLC-specific (blue) T cells. Horizontal lines in (A,B) show group medians. The unique clonotype ratio was calculated by dividing the number of unique clonotypes by the total number of TCR sequences. Normalized richness was calculated by counting the number of distinct TCR sequences in subsamples of 10 sequences. The mean of 1,000 subsampling iterations is shown. Differences between epitopes were compared by Mann Whitney. Correlations were tested with Spearman's rank correlation coefficient.
To understand what explains the higher diversity in some of the samples, we investigated the correlation between T-cell frequencies and the diversity of the repertoire for the CMVA2−NLV-specific and EBVA2−GLC-specific T-cell data combined. We found a negative correlation between all diversity measures and the frequency of antigen-specific T cells (Figures 4C,D). This suggests that individuals with high frequencies of antigen-specific T cells had large clonal expansions, leading to a decrease in TCR repertoire diversity.
Both Age and CMV-Infection Are Associated With a Lower Diversity of the EBVA2-GLC-Specific T-Cell Repertoire
To investigate whether age is associated with the diversity of the antigen-specific T-cell repertoire, we analyzed the normalized richness and the Simpson's diversity index of the repertoire of both CMVA2−NLV-specific and EBVA2−GLC-specific T cells in relation to the age of the individuals. The normalized richness of the antigen-specific T-cell repertoires against both CMVA2−NLV and EBVA2−GLC showed a negative trend with age (p = ns, r = 0.1786 and p = 0.0645, r = −0.5105, respectively), although the decrease with age observed for the CMVA2−NLV-specific samples was largely based on the datapoint of one young adult (Figure 5A). The Simpson's diversity index showed the same negative trend, although this was also not significant (p = ns for CMVA2−NLV and p = 0.0925 for EBVA2−GLC) (Figure 5A). This suggests that the diversity of the CMVA2−NLV-specific and EBVA2−GLC-specific T-cell repertoire decrease with age.
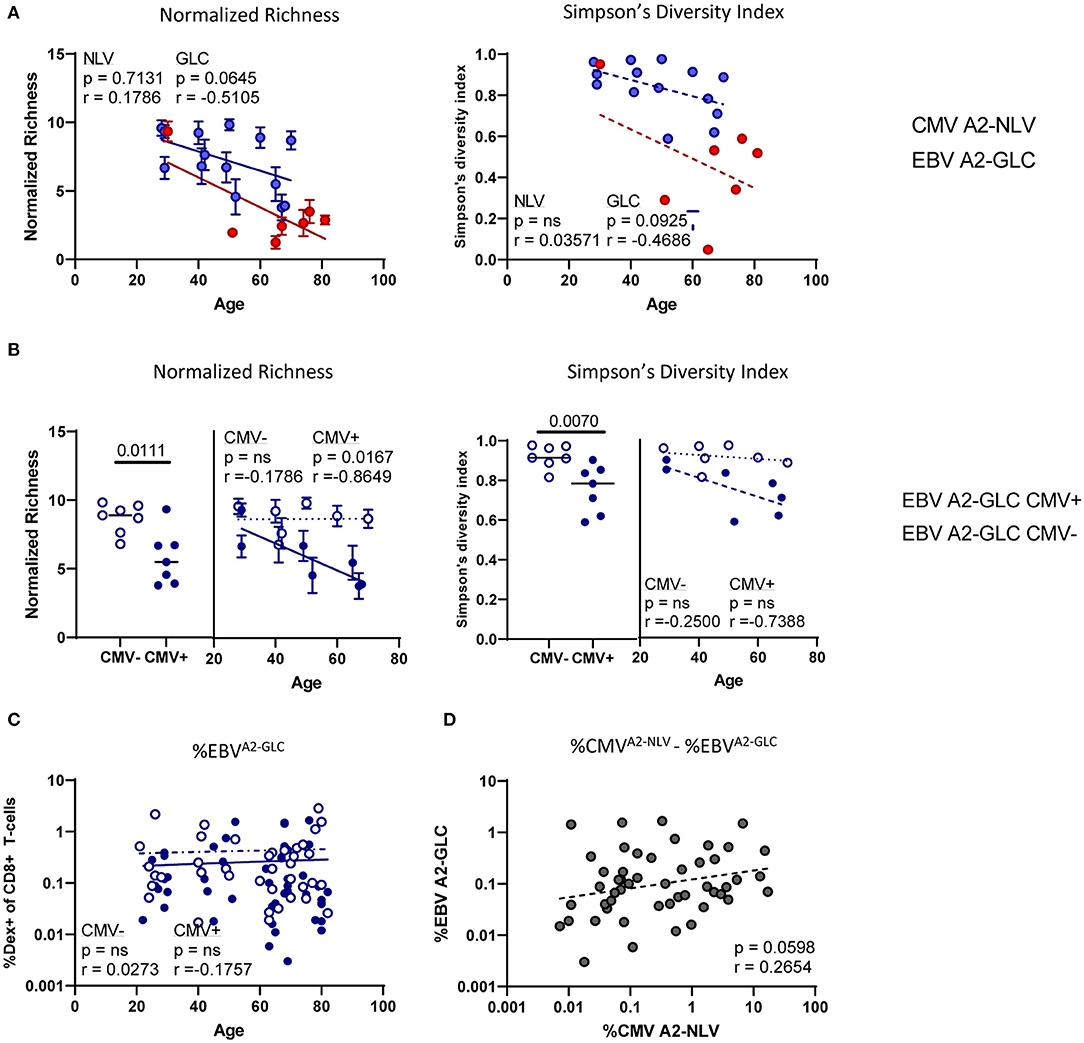
Figure 5. The CMVA2−NLV-specific TCRβ repertoire and the EBVA2−GLC-specific TCRβ repertoire decrease with age, whereas the diversity of the EBVA2−GLC-specific TCRβ repertoire is lower in the CMV+ individuals than in the CMV− individuals. (A) Correlation plots of normalized richness score (left) and Simpson's diversity index (right panels) of CMVA2−NLV-specific and EBVA2−GLC-specific T-cell repertoires and age. Whiskers denote standard deviations over 1,000 subsampling iterations. (B) Normalized richness score and Simpson's diversity index of the EBVA2−GLC-specific T-cell repertoire divided in CMV− (blue, open circles) and CMV+ individuals (blue, filled circles). (C) Percentage of EBVA2−GLC-specific CD8+ T cells in all CMV− (blue open circles) and CMV+ individuals (blue filled circles). (D) Correlation of CMVA2−NLV and EBVA2−GLC-specific CD8+ T-cell frequencies within donors. Frequencies are log transformed for statistical analysis. Solid lines indicate a slope significantly (p < 0.05) different from a slope of 0, whereas a dotted line indicates no significant difference. All correlations were tested by Spearman's Rank correlation coefficient.
As there are indications in mice that CMV-infection can affect the T-cell response against heterologous virus infections (Cicin-Sain et al., 2012; Mekker et al., 2012; Redeker et al., 2017), we then stratified the EBVA2−GLC-specific T-cell data according to the individuals' CMV status. We found a higher diversity of the EBVA2−GLC-specific T-cell repertoire in CMV− compared to CMV+ individuals based on both normalized richness (p = 0.0111) and Simpson's diversity index (p = 0.0070) (Figure 5B). Linear regression analysis of these data suggests a lower diversity of the EBVA2−GLC-specific T-cell repertoire in the presence of CMV over the entire observed age range (Figure 5B). A significant decrease in diversity of the EBVA2−GLC-specific T-cell repertoire with age was only observed in CMV+ individuals.
A likely explanation for the decreased diversity of the EBVA2−GLC-specific T-cell repertoire in CMV+ individuals could be memory attrition, i.e., large frequencies of CMVA2−NLV-specific T cells outcompeting non-CMV-specific T cells. If there is indeed a role for memory attrition, one would expect that (1) the percentage of EBVA2−GLC-specific T cells is lower in CMV+ compared to CMV− individuals, and (2) that the percentage of EBVA2−GLC-specific T cells correlates negatively with the percentage of CMVA2−NLV-specific T cells. CMV+ individuals indeed had significantly lower percentages of EBVA2−GLC-specific CD8+ T cells compared to CMV− individuals (p = 0.0300) (Supplementary Figure 6A). The lower fraction of EBVA2−GLC-specific T cells was seen at all ages (Figure 5C). However, the percentage of EBV-specific T cells was not negatively associated with the percentage of CMVA2−NLV-specific T cells. If anything, there was a trend toward a positive correlation between both antigen-specific T-cell frequencies (p = 0.0598, r2 = 0.2654) (Figure 5D). Thus, the decreased frequency and diversity of EBVA2−GLC-specific T cells in CMV+ individuals does not seem to be due to memory attrition.
Based on the same t-SNE cluster analysis as shown in Figure 1B, we compared the EBVA2−GLC-specific CD8+ T-cell population of CMV− (n = 32) and CMV+ (n = 40) individuals (Supplementary Figure 6B). For this analysis, data from donors of all ages were pooled. For none of the three earlier identified clusters did we find any significant differences between CMV+ and CMV− individuals (Supplementary Figure 6B). As the t-SNE gates were rather rough, we wondered whether there would be any differences in the phenotype of EBVA2−GLC-specific T cells when analyzing the data in more detail. Based on conventional gating, we observed no substantial CMV-related differences based on for example the percentage of CD57+ or KLRG-1+ cells. Only the percentage of PD-1+ expressing EBVA2−GLC-specific T cells was significantly higher in CMV− as compared to CMV+ individuals (p = 0.0255), while the EBVA2−GLC-specific T-cell population in CMV+ individuals had a significantly higher percentage of effector memory T cells than in CMV− individuals (p = 0.0106) (Supplementary Figure 6C). Thus, CMV-infection may induce subtle changes in non-CMV-specific T cell populations, like those specific for EBV.
Discussion
In this study, we investigated the effect of age and CMV-infection on the phenotype and diversity of the antigen-specific T-cell repertoire. We focused on CMVA2−NLV-specific and EBVA2−GLC-specific T cells, as these antigen-specific T cells are readily detectable in the T-cell pool at all ages. The antigen-specific T cells against both persistent viruses showed an age-related increase in the expression of several markers associated with a more differentiated phenotype, including KLRG-1, an increase in the fraction of terminally differentiated T cells and a decrease in the diversity of the antigen-specific T-cell repertoire. CMV-infection has also been proposed to reduce the diversity of the total memory T-cell pool (Khan et al., 2002; Emerson et al., 2017). However, the effect of CMV on the diversity of other antigen-specific T-cell repertoires remains poorly understood. Here we show that CMV infection is associated with a lower diversity of the EBVA2−GLC-specific T-cell repertoire. Although the exact mechanism behind this association remains unknown, our data suggest that the decreased diversity of the EBVA2−GLC-specific T-cell repertoire in CMV+ individuals is not due to memory attrition. We found that antigen-specific T cells against CMVA2−NLV and EBVA2−GLC are different at the phenotypic level; CMVA2−NLV-specific T cells have higher percentages of Temra cells, as defined by CD27- and CD45RO-, and higher expression of CD57 than EBVA2−GLC-specific T cells. These findings are in line with other studies, showing that EBVA2−GLC-specific T cells are predominantly CD45RO+ (Kuijpers et al., 2003) and more often express CD27 (Appay et al., 2002). The presence of terminally differentiated CMVA2−NLV-specific T cells is probably explained by the presence of large clonal expansions, which are typical for CMV infection (van den Berg et al., 2019).
The relatively high expression of markers associated with a more differentiated phenotype and the relatively low TCR repertoire diversity of the CMVA2−NLV-specific T-cell population becomes even more pronounced in individuals at older age. Based on the high frequencies of CMVA2−NLV-specific T cells in older adults, this age-effect is probably also linked to the presence of large clonal expansions consisting of terminally differentiated cells. It has been suggested that the increase in CMV-specific T-cell numbers with age is due to periodical infectious reactivation (van Boven et al., 2017). Although EBVA2−GLC-specific T cells also show changes associated with age, these are less pronounced than for CMVA2−NLV-specific T cells. This may be related to differences in cellular tropism between the two herpes viruses (Shenk, 2008; Hatton et al., 2014), or to possible differences in viral reactivation frequencies (Scheinberg et al., 2007; Thomasini et al., 2017).
It is tempting to speculate that the features of the T-cell responses against CMVA2−NLV and EBVA2−GLC that we observed are characteristic for the immune response to these two viruses in general. Although these two epitopes tend to be immunodominant, and thereby represent a fair share of the T-cell response in many individuals, it was recently shown that different combinations of HLA-alleles can influence the immunodominance of an epitope (Maleeva et al., 2019). This may also explain the large variation in antigen-specific T-cell frequencies that we observed in the four age groups. It remains to be investigated whether our results also apply to other epitopes for these two viruses, and to individuals in which these responses are less dominant.
Previous studies on the effect of aging on the antigen-specific T-cell repertoire have mostly focused on the maintenance of TCR sequences that are shared between individuals or that occur at different timepoints (Annels et al., 2000; Klarenbeek et al., 2012) or on a biased usage of Vβ-segments (Schwanninger et al., 2008). As these studies observed dominant T-cell clones both in a longitudinal setting and in a cross-sectional setting across different ages, they led to the view that the antigen-specific T-cell repertoires against CMV and EBV are relatively stable over time. Even though the T-cell repertoire analyses were performed on a relatively small number of samples, our direct assessment of the TCR diversity of EBVA2−GLC-specific T cells, and to a lesser extent of CMVA2−NLV-specific T cells, showed a clear decrease in diversity with age, suggesting that the antigen-specific T-cell repertoires against these viruses are not as stable as previously thought. These seemingly contradicting conclusions may be due to the process of convergent contraction of the T cell repertoire, in which lower frequency clonotypes are lost over time, while only few T cells persist (Smith et al., 2020). Observations of these persisting T-cell clones over time would suggest that the antigen-specific T-cell repertoire is relatively stable, even though the richness and diversity of the repertoire may decrease with age. To study whether convergent contraction of the T-cell repertoire is indeed happening, a longitudinal study should be performed, focusing on the richness and diversity of the antigen-specific T-cell repertoire.
It remains unknown why the diversity of antigen-specific T-cell repertoires decreases with age and whether age is the real driver of the decrease in T-cell diversity or whether other factors play an important role. We cannot exclude the possibility that the older individuals of the study population had been infected at an older age, possibly leading to an antigen-specific T-cell repertoire of lower diversity because the diversity of the naive (precursor) pool is known to decrease with age (Britanova et al., 2014; Egorov et al., 2018). However, the recent finding that only a very small percentage of individuals seroconvert for CMV at later age (Samson et al., 2020), as well as the finding that more than 90% of the population is infected with EBV during adolescence (Balfour et al., 2013; Winter et al., 2020), makes this explanation unlikely. In our view, a more likely explanation for the reduced diversity in the antigen-specific T-cell repertoire of older individuals would be that older individuals have been infected for a longer time, and have lost more T-cell clones over time, for example due to exhaustion after restimulation (Lanfermeijer et al., 2020).
Our results indicate that CMV-infection is associated with a lower diversity of the EBVA2−GLC-specific T-cell repertoire. As it is generally thought that T-cell receptor diversity is positively correlated with the level of protection against infectious diseases, one would expect that this decreased diversity would lead to a decreased EBV-specific T-cell response in CMV+ individuals. If our findings also hold true for other antigens, this would suggest that CMV+ individuals are less protected against other infections. This is in line with most mouse studies, which show a negative effect of CMV-infection on the T-cell efficacy against heterologous infections (Cicin-Sain et al., 2012; Mekker et al., 2012; Smithey et al., 2012; Redeker et al., 2017). How CMV-infection would lead to this lower diversity remains unknown. Although memory attrition has often been suggested to play a role, our data do not support this idea, as we observed a positive correlation between the frequencies of EBVA2−GLC- and CMVA2−NLV-specific T cells. The explanation for this positive correlation might be that some individuals are better T-cell responders than others. The observation that CMV-infection leads to lower frequencies of antigen-specific T cells is not surprising, as it is a relative measure, which is easily skewed by the high percentages of CMV-specific T cells. Likewise, in another study of our group it was shown that influenza-specific T-cell frequencies in older individuals were lower in CMV+ compared to CMV− individuals. Importantly, however, this did not result in lower influenza-specific IFNγ responses in CMV+ individuals (van den Berg et al., in press), suggesting that CMV did not negatively impact the influenza-specific T-cell response; although sample sizes were unfortunately too small to confirm this based on the diversity of the influenza-specific TCR repertoire. It remains puzzling why these different results are observed between studies, and it would be interesting to understand whether e.g., the acute or chronic nature of a pathogen plays a role.
T cells isolated from blood represent only a small fraction of the total T-cell pool in the body, as it has been estimated that blood contains only 2 percent of all the T cells in the body (Westermann, 1990). Although many T cells travel through different compartments in the body, it remains to be investigated whether antigen-specific T-cell characteristics, like their phenotype and repertoire diversity, that are observed in the blood can be extrapolated to T cells in other sites of the body. The presence of tissue-resident T cells, which hardly circulate through the blood, complicates this even further. In the case of CMV and EBV, however, the blood may in fact be the most informative site to follow the antigen-specific T-cell response. It has previously been suggested that most CMV-specific T cells are present in the blood (Gordon et al., 2017). In line with this, a recent human study showed that most terminally differentiated memory CD8+ T cells, including CMV-specific T cells, are confined to the intravascular circulation and do not circulate through the thoracic duct lymph (Buggert et al., 2020). Also for EBV, a bloodborne virus infecting B cells, the blood may in fact be the most representative compartment to study the antigen-specific T-cell response. It remains to be investigated whether the changes we observed in the antigen-specific T-cell repertoire in the blood also apply to antigen-specific T cells in other sites of the body. So far, studies have shown both a minimal overlap in the naïve T-cell pool between spleen and lymph nodes (Thome et al., 2016), as well as high degrees of overlap when focusing on the memory T-cell pool between blood and the thoracic duct lymph (Buggert et al., 2020), between peripheral blood and lymph nodes (Remmerswaal et al., 2015) and between spleen and lymph nodes (Thome et al., 2014).
In contrast to the commonly-held view that the antigen-specific T-cell repertoires against CMV and EBV are relatively stable with age, we here show that they both clearly decrease with age. Our data suggest that not only age but also CMV-infection is associated with the diversity of the EBVA2−GLC-specific T-cell repertoire. Insights into how antigen-specific T-cell repertoires evolve with age and under the influence of other infections, like latent CMV, are important for the development of novel vaccination strategies to protect older adults against infectious diseases. One of the proposed strategies to prevent older adults is to induce protective immune responses through vaccination at a younger age. This would require stability of the induced immune response in order to provide protection later in life. Our data suggest that the antigen-specific T-cell repertoire is not as stable as previously thought. Unfortunately, this implies that vaccination may also come too early. Ideally, one would like to vaccinate before the age-associated decline in naive T-cell repertoire diversity, but late enough to ensure that a substantial level of protection is maintained until later in life. Further research is needed to investigate why some cells are maintained while others are not, and to define the optimal moment of vaccination to protect the elderly.
Data Availability Statement
The raw data supporting the conclusions of this article will be made available by the authors, without undue reservation.
Ethics Statement
The studies involving human participants were reviewed and approved by Central Committee on Research Involving Human Subjects of the Netherlands and ethical committee, METC Noord Holland. The patients/participants provided their written informed consent to participate in this study.
Author Contributions
The original idea for this study was from DvB and JAMB. JL performed the majority of experiments, gathered data, and analysis. PdG performed data analysis. MH and MV performed experiments. JvB designed the original studies of the individuals. DvB and JAMB supervised the project. JL and PdG prepared figures and wrote manuscript with contributions and review from DvB, JAMB, and JvB. All authors contributed to the article and approved the submitted version.
Funding
This work was supported by The Netherlands Organization for Scientific Research (NWO) Graduate Program 22.005.023 (to PdG).
Conflict of Interest
The authors declare that the research was conducted in the absence of any commercial or financial relationships that could be construed as a potential conflict of interest.
Acknowledgments
We authors are grateful to Dr. Eric Spierings and the HLA laboratory of the UMC Utrecht for the sequencing. The authors thank Dr. T Guichelaar for critically reading the manuscript and for his useful comments.
Supplementary Material
The Supplementary Material for this article can be found online at: https://www.frontiersin.org/articles/10.3389/fragi.2021.665637/full#supplementary-material
References
Almanzar, G., Schwaiger, S., Jenewein, B., Keller, M., Herndler-Brandstetter, D., Wurzner, R., et al. (2005). Long-term cytomegalovirus infection leads to significant changes in the composition of the CD8+ T-cell repertoire, which may be the basis for an imbalance in the cytokine production profile in elderly persons. J. Virol. 79, 3675–3683. doi: 10.1128/JVI.79.6.3675-3683.2005
Annels, N. E., Callan, M. F. C., Tan, L., and Rickinson, A. B. (2000). Changing patterns of dominant tcr usage with maturation of an EBV-specific cytotoxic T cell response. J. Immunol. 165, 4831–4841. doi: 10.4049/jimmunol.165.9.4831
Appay, V., Dunbar, P. R., Callan, M., Klenerman, P., Gillespie, G. M. A., Papagno, L., et al. (2002). Memory CD8+ T cells vary in differentiation phenotype in different persistent virus infections. Nat. Med. 8, 379–385. doi: 10.1038/nm0402-379
Balfour, H. H. Jr, Sifakis, F., Sliman, J. A., Knight, J. A., Schmeling, D. O., and Thomas, W. (2013). Age-specific prevalence of Epstein-Barr virus infection among individuals aged 6-19 years in the United States and factors affecting its acquisition. J. Infect. Dis. 208, 1286–1293. doi: 10.1093/infdis/jit321
Briceno, O., Lissina, A., Wanke, K., Afonso, G., von Braun, A., Ragon, K., et al. (2016). Reduced naive CD8(+) T-cell priming efficacy in elderly adults. Aging Cell 15, 14–21. doi: 10.1111/acel.12384
Britanova, O. V., Putintseva, E. V., Shugay, M., Merzlyak, E. M., Turchaninova, M. A., Staroverov, D. B., et al. (2014). Age-related decrease in TCR repertoire diversity measured with deep and normalized sequence profiling. J. Immunol. 192, 2689–2698. doi: 10.4049/jimmunol.1302064
Britanova, O. V., Shugay, M., Merzlyak, E. M., Staroverov, D. B., Putintseva, E. V., Turchaninova, M. A., et al. (2016). Dynamics of individual T cell repertoires: from cord blood to centenarians. J. Immunol. 196, 5005–5013. doi: 10.4049/jimmunol.1600005
Buggert, M., Vella, L. A., Nguyen, S., Wu, V. H., Chen, Z., Sekine, T., et al. (2020). The identity of human tissue-emigrant CD8(+) T cells. Cell 183, 1946–1961. doi: 10.1101/2020.08.11.236372
Cardenas Sierra, D., Velez Colmenares, G., Orfao de Matos, A., Fiorentino Gomez, S., and Quijano Gomez, S. M. (2014). Age-associated Epstein-Barr virus-specific T cell responses in seropositive healthy adults. Clin. Exp. Immunol. 177, 320–332. doi: 10.1111/cei.12337
Chidrawar, S., Khan, N., Wei, W., McLarnon, A., Smith, N., Nayak, L., et al. (2009). Cytomegalovirus-seropositivity has a profound influence on the magnitude of major lymphoid subsets within healthy individuals. Clin. Exp. Immunol. 155, 423–432. doi: 10.1111/j.1365-2249.2008.03785.x
Cicin-Sain, L., Brien, J. D., Uhrlaub, J. L., Drabig, A., Marandu, T. F., and Nikolich-Zugich, J. (2012). Cytomegalovirus infection impairs immune responses and accentuates T-cell pool changes observed in mice with aging. PLoS Pathog. 8:e1002849. doi: 10.1371/journal.ppat.1002849
Deng, Y., Jing, Y., Campbell, A. E., and Gravenstein, S. (2004). Age-related impaired type 1 T cell responses to influenza: reduced activation ex vivo, decreased expansion in CTL culture in vitro, and blunted response to influenza vaccination in vivo in the elderly. J. Immunol. 172, 3437–3446. doi: 10.4049/jimmunol.172.6.3437
Derhovanessian, E., Larbi, A., and Pawelec, G. (2009). Biomarkers of human immunosenescence: impact of Cytomegalovirus infection. Curr. Opin. Immunol. 21, 440–445. doi: 10.1016/j.coi.2009.05.012
Egorov, E. S., Kasatskaya, S. A., Zubov, V. N., Izraelson, M., Nakonechnaya, T. O., Staroverov, D. B., et al. (2018). The changing landscape of naive T cell receptor repertoire with human aging. Front. Immunol. 9:1618. doi: 10.3389/fimmu.2018.01618
Elhanati, Y., Sethna, Z., Callan, C. G. Jr., Mora, T., and Walczak, A. M. (2018). Predicting the spectrum of TCR repertoire sharing with a data-driven model of recombination. Immunol. Rev. 284, 167–179. doi: 10.1111/imr.12665
Emerson, R. O., DeWitt, W. S., Vignali, M., Gravley, J., Hu, J. K., Osborne, E. J., et al. (2017). Immunosequencing identifies signatures of cytomegalovirus exposure history and HLA-mediated effects on the T cell repertoire. Nat. Genet. 49, 659–665. doi: 10.1038/ng.3822
Gerritsen, B., Pandit, A., Andeweg, A. C., and de Boer, R. J. (2016). RTCR: a pipeline for complete and accurate recovery of T cell repertoires from high throughput sequencing data. Bioinformatics 32, 3098–3106. doi: 10.1093/bioinformatics/btw339
Gordon, C. L., Miron, M., Thome, J. J., Matsuoka, N., Weiner, J., Rak, M. A., et al. (2017). Tissue reservoirs of antiviral T cell immunity in persistent human CMV infection. J. Exp. Med. 214, 651–667. doi: 10.1084/jem.20160758
Goronzy, J. J., Fulbright, J. W., Crowson, C. S., Poland, G. A., O'Fallon, W. M., and Weyand, C. M. (2001). Value of immunological markers in predicting responsiveness to influenza vaccination in elderly individuals. J. Virol. 75, 12182–12187. doi: 10.1128/JVI.75.24.12182-12187.2001
Hadrup, S. R., Strindhall, J., Kollgaard, T., Seremet, T., Johansson, B., Pawelec, G., et al. (2006). Longitudinal studies of clonally expanded CD8 T cells reveal a repertoire shrinkage predicting mortality and an increased number of dysfunctional cytomegalovirus-Specific T cells in the very elderly. J. Immunol. 176, 2645–2653. doi: 10.4049/jimmunol.176.4.2645
Hatton, O. L., Harris-Arnold, A., Schaffert, S., Krams, S. M., and Martinez, O. M. (2014). The interplay between Epstein-Barr virus and B lymphocytes: implications for infection, immunity, and disease. Immunol. Res. 58, 268–276. doi: 10.1007/s12026-014-8496-1
Hoji, A., Connolly, N. C., Buchanan, W. G., and Rinaldo, C. R. Jr. (2007). CD27 and CD57 expression reveals atypical differentiation of human immunodeficiency virus type 1-specific memory CD8+ T cells. Clin. Vaccine Immunol. 14, 74–80. doi: 10.1128/CVI.00250-06
Iancu, E. M., Corthesy, P., Baumgaertner, P., Devevre, E., Voelter, V., Romero, P., et al. (2009). Clonotype selection and composition of human CD8 T cells specific for persistent herpes viruses varies with differentiation but is stable over time. J. Immunol. 183, 319–331. doi: 10.4049/jimmunol.0803647
Jubel, J. M., Barbati, Z. R., Burger, C., Wirtz, D. C., and Schildberg, F. A. (2020). The role of PD-1 in acute and chronic infection. Front. Immunol. 11:487. doi: 10.3389/fimmu.2020.00487
Khan, N., Hislop, A., Gudgeon, N., Cobbold, M., Khanna, R., Nayak, L., et al. (2004). Herpesvirus-specific CD8 T cell immunity in old age: cytomegalovirus impairs the response to a coresident EBV infection. J. Immunol. 173, 7481–7489. doi: 10.4049/jimmunol.173.12.7481
Khan, N., Shariff, N., Cobbold, M., Bruton, R., Ainsworth, J. A., Sinclair, A. J., et al. (2002). Cytomegalovirus seropositivity drives the CD8 t cell repertoire toward greater clonality in healthy elderly individuals. J. Immunol. 169, 1984–1992. doi: 10.4049/jimmunol.169.4.1984
Klarenbeek, P. L., Remmerswaal, E. B., ten Berge, I. J., Doorenspleet, M. E., van Schaik, B. D., Esveldt, R. E., et al. (2012). Deep sequencing of antiviral T-cell responses to HCMV and EBV in humans reveals a stable repertoire that is maintained for many years. PLoS Pathog. 8:e1002889. doi: 10.1371/journal.ppat.1002889
Kotecha, N., Krutzik, P. O., and Irish, J. M. (2010). Web-based analysis and publication of flow cytometry experiments. Curr. Protoc. Cytom. 10:17. doi: 10.1002/0471142956.cy1017s53
Kuijpers, T. W., Vossen, M. T., Gent, M. R., Davin, J. C., Roos, M. T., Wertheim-van Dillen, P. M., et al. (2003). Frequencies of circulating cytolytic, CD45RA+CD27-, CD8+ T lymphocytes depend on infection with CMV. J. Immunol. 170, 4342–4348. doi: 10.4049/jimmunol.170.8.4342
Lanfermeijer, J., Borghans, J. A. M., and van Baarle, D. (2020). How age and infection history shape the antigen-specific CD8(+) T-cell repertoire: implications for vaccination strategies in older adults. Aging Cell 19:e13262. doi: 10.1111/acel.13262
Lindau, P., Mukherjee, R., Gutschow, M. V., Vignali, M., Warren, E. H., Riddell, S. R., et al. (2019). Cytomegalovirus exposure in the elderly does not reduce CD8 T cell repertoire diversity. J. Immunol. 202, 476–483. doi: 10.4049/jimmunol.1800217
Maleeva, A. V., Shmarov, V. A., Kiryukhin, D. O., Efimov, G. A., and Savchenko, V. G. (2019). Repertoire of cytomegalovirus-specific T cells is focused on the immunodominant epitopes in fixed hierarchy dependent on HLA genotype of the donor. Blood 134:2327. doi: 10.1182/blood-2019-130241
Market, E., and Papavasiliou, F. N. (2003). V(D)J recombination and the evolution of the adaptive immune system. PLoS Biol. 1:E16. doi: 10.1371/journal.pbio.0000016
Mekker, A., Tchang, V. S., Haeberli, L., Oxenius, A., Trkola, A., and Karrer, U. (2012). Immune senescence: relative contributions of age and cytomegalovirus infection. PLoS Pathog. 8:e1002850. doi: 10.1371/journal.ppat.1002850
Nikolich-Zugich, J. (2008). Ageing and life-long maintenance of T-cell subsets in the face of latent persistent infections. Nat. Rev. Immunol. 8, 512–522. doi: 10.1038/nri2318
Pawelec, G., Akbar, A., Caruso, C., Grubeck-Loebenstein, B., Solana, R., and Wikby, A. (2005). Human immunosenescence: is it infectious? Immunol. Rev. 205, 257–268. doi: 10.1111/j.0105-2896.2005.00271.x
Qi, Q., Liu, Y., Cheng, Y., Glanville, J., Zhang, D., Lee, J. Y., et al. (2014). Diversity and clonal selection in the human T-cell repertoire. Proc. Natl. Acad. Sci. U. S. A. 111, 13139–13144. doi: 10.1073/pnas.1409155111
Redeker, A., Remmerswaal, E. B. M., van der Gracht, E. T. I., Welten, S. P. M., Hollt, T., Koning, F., et al. (2017). The contribution of cytomegalovirus infection to immune senescence is set by the infectious dose. Front. Immunol. 8:1953. doi: 10.3389/fimmu.2017.01953
Remmerswaal, E. B., Klarenbeek, P. L., Alves, N. L., Doorenspleet, M. E., van Schaik, B. D., Esveldt, R. E., et al. (2015). Clonal evolution of CD8+ T cell responses against latent viruses: relationship among phenotype, localization, and function. J. Virol. 89, 568–580. doi: 10.1128/JVI.02003-14
Rosendahl Huber, S. K., Hendriks, M., Jacobi, R. H. J., van de Kassteele, J., Mandersloot-Oskam, J. C., van Boxtel, R. A. J., et al. (2018). Immunogenicity of influenza vaccines: evidence for differential effect of secondary vaccination on humoral and cellular immunity. Front. Immunol. 9:3103. doi: 10.3389/fimmu.2018.03103
Sad, S., and Krishnan, L. (2003). Maintenance and attrition of T-cell memory. Crit. Rev. Immunol. 23, 129–147. doi: 10.1615/CritRevImmunol.v23.i12.70
Samson, L. D., van den Berg, S. P., Engelfriet, P., Boots, A. M., Hendriks, M., de Rond, L. G., et al. (2020). Limited effect of duration of CMV infection on adaptive immunity and frailty: insights from a 27-year-long longitudinal study. Clin. Transl. Immunol. 9:e1193. doi: 10.1002/cti2.1193
Scheinberg, P., Fischer, S. H., Li, L., Nunez, O., Wu, C. O., Sloand, E. M., et al. (2007). Distinct EBV and CMV reactivation patterns following antibody-based immunosuppressive regimens in patients with severe aplastic anemia. Blood 109, 3219–3224. doi: 10.1182/blood-2006-09-045625
Schwanninger, A., Weinberger, B., Weiskopf, D., Herndler-Brandstetter, D., Reitinger, S., Gassner, C., et al. (2008). Age-related appearance of a CMV-specific high-avidity CD8+ T cell clonotype which does not occur in young adults. Immun. Ageing 5:14. doi: 10.1186/1742-4933-5-14
Sethna, Z., Elhanati, Y., Callan, C. G., Walczak, A. M., and Mora, T. (2019). OLGA: fast computation of generation probabilities of B- and T-cell receptor amino acid sequences and motifs. Bioinformatics 35, 2974–2981. doi: 10.1093/bioinformatics/btz035
Shenk, T. E. S. M. F. (2008). Human cytomegalovirus. Curr. Top. Microbiol. Immunol. 325, 297–313. doi: 10.1007/978-3-540-77349-8
Shugay, M., Bagaev, D. V., Zvyagin, I. V., Vroomans, R. M., Crawford, J. C., Dolton, G., et al. (2017). VDJdb: a curated database of T-cell receptor sequences with known antigen specificity. Nucleic Acids Res. 46, 419–427. doi: 10.1093/nar/gkx760
Shugay, M., Britanova, O. V., Merzlyak, E. M., Turchaninova, M. A., Mamedov, I. Z., Tuganbaev, T. R., et al. (2014). Towards error-free profiling of immune repertoires. Nat. Methods 11, 653–655. doi: 10.1038/nmeth.2960
Smith, C. J., Venturi, V., Quigley, M. F., Turula, H., Gostick, E., Ladell, K., et al. (2020). Stochastic expansions maintain the clonal stability of CD8(+) T cell populations undergoing memory inflation driven by murine cytomegalovirus. J. Immunol. 204, 112–121. doi: 10.4049/jimmunol.1900455
Smithey, M. J., Li, G., Venturi, V., Davenport, M. P., and Nikolich-Zugich, J. (2012). Lifelong persistent viral infection alters the naive T cell pool, impairing CD8 T cell immunity in late life. J. Immunol. 189, 5356–5366. doi: 10.4049/jimmunol.1201867
Smithey, M. J., Venturi, V., Davenport, M. P., Buntzman, A. S., Vincent, B. G., Frelinger, J. A., et al. (2018). Lifelong CMV infection improves immune defense in old mice by broadening the mobilized TCR repertoire against third-party infection. Proc. Natl. Acad. Sci. U. S. A. 115, E6817–E6825. doi: 10.1073/pnas.1719451115
Sukdolak, C., Tischer, S., Dieks, D., Figueiredo, C., Goudeva, L., Heuft, H. G., et al. (2013). CMV-, EBV- and ADV-specific T cell immunity: screening and monitoring of potential third-party donors to improve post-transplantation outcome. Biol. Blood Marrow Transplant 19, 1480–1492. doi: 10.1016/j.bbmt.2013.07.015
Tcherniaeva, I., den Hartog, G., Berbers, G., and van der Klis, F. (2018). The development of a bead-based multiplex immunoassay for the detection of IgG antibodies to CMV and EBV. J. Immunol. Methods 462, 1–8. doi: 10.1016/j.jim.2018.07.003
Thomasini, R. L., Pereira, D. S., Pereira, F. S. M., Mateo, E. C., Mota, T. N., Guimaraes, G. G., et al. (2017). Aged-associated cytomegalovirus and Epstein-Barr virus reactivation and cytomegalovirus relationship with the frailty syndrome in older women. PLoS ONE 12:e0180841. doi: 10.1371/journal.pone.0180841
Thome, J. J., Grinshpun, B., Kumar, B. V., Kubota, M., Ohmura, Y., Lerner, H., et al. (2016). Longterm maintenance of human naive T cells through in situ homeostasis in lymphoid tissue sites. Sci Immunol. 1:6506. doi: 10.1126/sciimmunol.aah6506
Thome, J. J., Yudanin, N., Ohmura, Y., Kubota, M., Grinshpun, B., Sathaliyawala, T., et al. (2014). Spatial map of human T cell compartmentalization and maintenance over decades of life. Cell 159, 814–828. doi: 10.1016/j.cell.2014.10.026
Tu, W., and Rao, S. (2016). Mechanisms underlying T cell immunosenescence: aging and cytomegalovirus infection. Front. Microbiol. 7:2111. doi: 10.3389/fmicb.2016.02111
Turner, S. J., La Gruta, N. L., Kedzierska, K., Thomas, P. G., and Doherty, P. C. (2009). Functional implications of T cell receptor diversity. Curr. Opin. Immunol. 21, 286–290. doi: 10.1016/j.coi.2009.05.004
van Boven, M., van de Kassteele, J., Korndewal, M. J., van Dorp, C. H., Kretzschmar, M., van der Klis, F., et al. (2017). Infectious reactivation of cytomegalovirus explaining age- and sex-specific patterns of seroprevalence. PLoS Comput. Biol. 13:e1005719. doi: 10.1371/journal.pcbi.1005719
van den Berg, S. P. H., Lanfermeijer, J., Jacobi, R. H. J., Hendriks, M., Vos, M., and van Schuijlenburg, R., et al. (in press). Latent CMV infection is associated with lower influenza virus-specific memory T-cell frequencies, but not with an impaired T-cell response to acute influenza virus infection. Front. Immunol. doi: 10.3389/fimmu.2021.663664.
van den Berg, S. P. H., Pardieck, I. N., Lanfermeijer, J., Sauce, D., Klenerman, P., van Baarle, D., et al. (2019). The hallmarks of CMV-specific CD8 T-cell differentiation. Med. Microbiol. Immunol. 208, 365–373. doi: 10.1007/s00430-019-00608-7
Venturi, V., Kedzierska, K., Price, D. A., Doherty, P. C., Douek, D. C., Turner, S. J., et al. (2006). Sharing of T cell receptors in antigen-specific responses is driven by convergent recombination. PNAS 103, 18691–189696. doi: 10.1073/pnas.0608907103
Venturi, V., Kedzierska, K., Turner, S. J., Doherty, P. C., and Davenport, M. P. (2007). Methods for comparing the diversity of samples of the T cell receptor repertoire. J. Immunol. Methods. 321, 182–195. doi: 10.1016/j.jim.2007.01.019
Venturi, V., Price, D. A., Douek, D. C., and Davenport, M. P. (2008). The molecular basis for public Tcell responses? Nat. Rev. 8:2260. doi: 10.1038/nri2260
Voisinne, G., Gonzalez de Peredo, A., and Roncagalli, R. (2018). CD5, an undercover regulator of TCR signaling. Front. Immunol. 9:2900. doi: 10.3389/fimmu.2018.02900
Westermann, J. P. R. (1990). Lymphocyte subsets in the blood: a diagnostic window on the lymphoid system. Immunol. Today 11, 406–410. doi: 10.1016/0167-5699(90)90160-B
Winter, J. R., Jackson, C., Lewis, J. E., Taylor, G. S., Thomas, O. G., and Stagg, H. R. (2020). Predictors of Epstein-Barr virus serostatus and implications for vaccine policy: a systematic review of the literature. J. Glob. Health 10:010404. doi: 10.7189/jogh.10.010404
Keywords: aging, cytomegalovirus, Epstein-Barr virus, T-cell repertoire, T cell
Citation: Lanfermeijer J, de Greef PC, Hendriks M, Vos M, van Beek J, Borghans JAM and van Baarle D (2021) Age and CMV-Infection Jointly Affect the EBV-Specific CD8+ T-Cell Repertoire. Front. Aging 2:665637. doi: 10.3389/fragi.2021.665637
Received: 08 February 2021; Accepted: 31 March 2021;
Published: 29 April 2021.
Edited by:
Anshu Agrawal, University of California, Irvine, United StatesReviewed by:
Mladen Jergovic, University of Arizona, United StatesVanessa Venturi, University of New South Wales, Australia
Copyright © 2021 Lanfermeijer, de Greef, Hendriks, Vos, van Beek, Borghans and van Baarle. This is an open-access article distributed under the terms of the Creative Commons Attribution License (CC BY). The use, distribution or reproduction in other forums is permitted, provided the original author(s) and the copyright owner(s) are credited and that the original publication in this journal is cited, in accordance with accepted academic practice. No use, distribution or reproduction is permitted which does not comply with these terms.
*Correspondence: Debbie van Baarle, ZC52YW4uYmFhcmxlQHVtY2cubmw=
†Present address: Debbie van Baarle, Virology and Immunology Research, Department of Medical Microbiology and Infection Prevention, University Medical Center Groningen, Groningen, Netherlands
‡These authors have contributed equally to this work