Pathobiology of the Klotho Antiaging Protein and Therapeutic Considerations
- 1Department of Laboratory Medicine and Pathobiology, University of Toronto, Toronto, ON, Canada
- 2Department of Laboratory Medicine, Keenan Research Centre for Biomedical Science, Unity Health Toronto, Toronto, ON, Canada
- 3Department of Endocrinology and Metabolism, Huashan Hospital, Shanghai Medical School, Fudan University, Shanghai, China
- 4Shanghai Yinuo Pharmaceutical Co., Ltd., Shanghai, China
The α-Klotho protein (henceforth denoted Klotho) has antiaging properties, as first observed in mice homozygous for a hypomorphic Klotho gene (kl/kl). These mice have a shortened lifespan, stunted growth, renal disease, hyperphosphatemia, hypercalcemia, vascular calcification, cardiac hypertrophy, hypertension, pulmonary disease, cognitive impairment, multi-organ atrophy and fibrosis. Overexpression of Klotho has opposite effects, extending lifespan. In humans, Klotho levels decline with age, chronic kidney disease, diabetes, Alzheimer’s disease and other conditions. Low Klotho levels correlate with an increase in the death rate from all causes. Klotho acts either as an obligate coreceptor for fibroblast growth factor 23 (FGF23), or as a soluble pleiotropic endocrine hormone (s-Klotho). It is mainly produced in the kidneys, but also in the brain, pancreas and other tissues. On renal tubular-cell membranes, it associates with FGF receptors to bind FGF23. Produced in bones, FGF23 regulates renal excretion of phosphate (phosphaturic effect) and vitamin D metabolism. Lack of Klotho or FGF23 results in hyperphosphatemia and hypervitaminosis D. With age, human renal function often deteriorates, lowering Klotho levels. This appears to promote age-related pathology. Remarkably, Klotho inhibits four pathways that have been linked to aging in various ways: Transforming growth factor β (TGF-β), insulin-like growth factor 1 (IGF-1), Wnt and NF-κB. These can induce cellular senescence, apoptosis, inflammation, immune dysfunction, fibrosis and neoplasia. Furthermore, Klotho increases cell-protective antioxidant enzymes through Nrf2 and FoxO. In accord, preclinical Klotho therapy ameliorated renal, cardiovascular, diabetes-related and neurodegenerative diseases, as well as cancer. s-Klotho protein injection was effective, but requires further investigation. Several drugs enhance circulating Klotho levels, and some cross the blood-brain barrier to potentially act in the brain. In clinical trials, increased Klotho was noted with renin-angiotensin system inhibitors (losartan, valsartan), a statin (fluvastatin), mTOR inhibitors (rapamycin, everolimus), vitamin D and pentoxifylline. In preclinical work, antidiabetic drugs (metformin, GLP-1-based, GABA, PPAR-γ agonists) also enhanced Klotho. Several traditional medicines and/or nutraceuticals increased Klotho in rodents, including astaxanthin, curcumin, ginseng, ligustilide and resveratrol. Notably, exercise and sport activity increased Klotho. This review addresses molecular, physiological and therapeutic aspects of Klotho.
1 Introduction
Aging is associated with changes in almost all tissues and organs of the body, resulting eventually in debilitating and/or fatal conditions such as cardiovascular disease, chronic organ failure, neurodegeneration and cancer. Aging occurs at varying speed in different species, suggesting some poorly defined biological clock. The molecular mechanisms underlying aging are intensely studied, but still not well understood. However, the pathogenesis of age-associated diseases is likely multi-factorial (Franceschi et al., 2018). In humans, a limited number of genes have been clearly linked to accelerated aging as seen in progeria (a rare condition) (Coppede 2021) or, conversely, associated with longevity (Javidnia et al., 2022). The subject of this review is Klotho (kl), which is an antiaging gene, and the corresponding protein α-Klotho (henceforth denoted Klotho or KL). The gene was first identified in mice in 1997 (Kuro-o et al., 1997). Deficiency of the protein results in a syndrome that has several features of aging, as observed in mutant mice with either a hypomorphic Klotho allele (Klkl/kl) (Kuro-o, et al., 1997) or full knockout of the Klotho gene (Kl−/−) (Tsujikawa et al., 2003). Klotho-deficient mice exhibit stunted growth, renal disease, hyperphosphatemia, hypercalcemia, vascular calcification, cardiac hypertrophy, hypertension, organ fibrosis, multi-organ atrophy, osteopenia, pulmonary disease, cognitive impairment and short lifespan (Bian et al., 2015; Erben and Andrukhova, 2017; Kinoshita and Kawai, 2016; Kuro-o et al., 1997, 2010, 2019, 2021). Overexpression of the gene has the opposite effects, lengthening survival (Kurosu et al., 2005).
Klotho insufficiency appears to play a role in human aging and, specifically, in many of the diseases that are associated with aging. Klotho expression declines with age, renal failure, diabetes and neurodegenerative disease. The age-related decline in serum levels appears to be similar in men and women; and reference values have recently been reported (Espuch-Oliver et al., 2022). Notably, a recent study of American adults showed that low serum Klotho levels correlate with an increased all-cause death rate (Kresovich and Bulka, 2021).
Klotho can exist as a membrane-bound coreceptor for fibroblast growth factor 23 (FGF23) (Urakawa et al., 2006), or a soluble endocrine mediator with many functions (Figures 1, 2) (Dalton et al., 2017; Kuro-o, 2017). Age-related deterioration of renal function results in Klotho insufficiency, and hyperphosphatemia that contributes greatly to the aging phenotype (Kuro-o 2010, 2018, 2021). Klotho protects the kidney and promotes phosphate elimination (phosphaturic effect). Remarkably, independent of FGF23, it inhibits at least four pathways that have been linked to aging in various ways. Klotho blocks or inhibits transforming growth factor β (TGF-β), insulin-like growth factor 1 (IGF-1), nuclear factor κB (NF-κB), and Wnt/β-catenin (Figure 3). Consequently, as will be presented in this review, Klotho exerts major effects on several biological processes relevant to aging and disease (Figure 4):
1) FGF23-dependent phosphate, calcium and vitamin D regulation.
2) Antioxidant and anti-inflammatory activities.
3) Prevention of chronic fibrosis.
4) Protective effects against cardiovascular disease.
5) Anti-cancer (tumor suppressor) activities.
6) Metabolic regulatory functions relevant to diabetes.
7) Anti-apoptotic and anti-senescence functions; stem cell preservation.
8) Protection against neurodegenerative disease (Alzheimer’s and other).
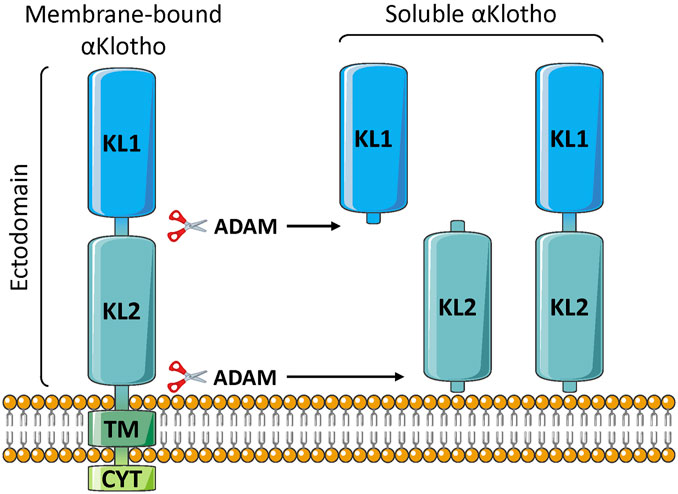
FIGURE 1. Klotho structure. The membrane-bound form is single-pass, and consists of two extracellular domains (KL1 and KL2), a transmembrane segment (TM), and a short non-signaling cytoplasmic tail (CYT). The soluble form is generated by proteolytic cleavage, usually by either ADAM10 or ADAM17 enzymes, to release the large soluble form (s-Klotho). This is the major form found in the circulation. It can be further cleaved to generate independent KL1 and KL2 fragments, but these are either minor forms or undetectable in the plasma.
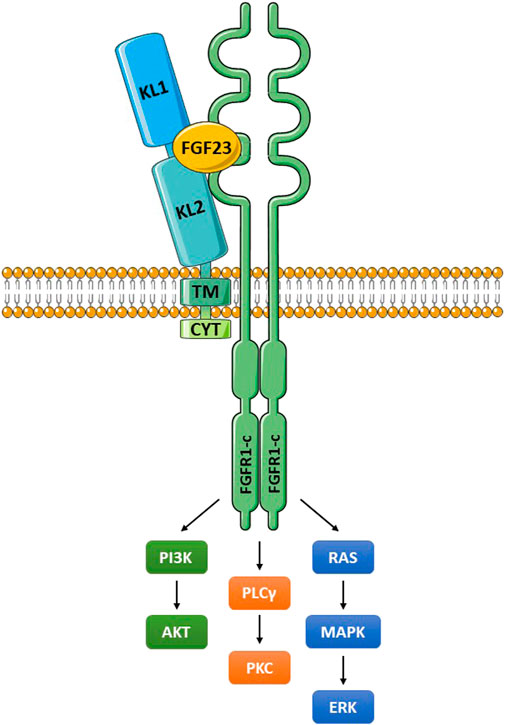
FIGURE 2. The Klotho/FGFR/FGF23 signaling complex. Klotho interacts with a FGFR (frequently FGFR1c) through an extension of its KL2 domain. FGF23 fits into a groove formed by components of KL1, KL2 and the FGFR. The membrane-bound and soluble Klotho forms (KL1/KL2 domains) can both bind to FGFR1c and function as coreceptors (Chen G, et al., 2018). These molecular interactions create a high affinity binding site for FGF23. The activated FGFR signals through multiple pathways as illustrated, and outlined in the text. Abbreviations: ERK, extracellular signal-regulated kinase; FGF23, fibroblast growth factor 23; FGFR1-c, FGF receptor 1c; MAPK, mitogen-activated protein kinase; PI3K, phosphoinositide 3-kinase; PKC, protein kinase C; PLCγ, phospholipase Cγ.
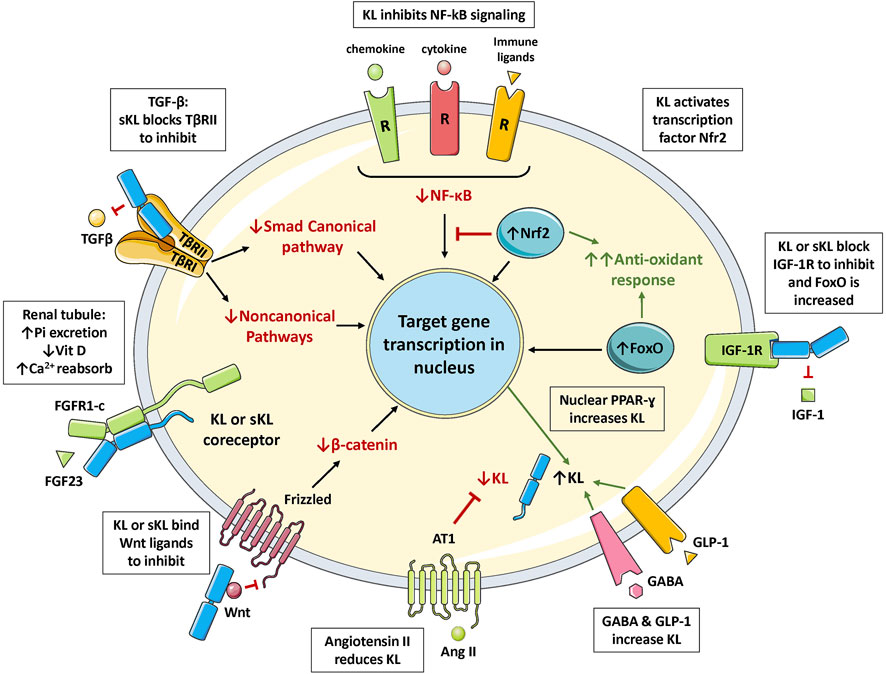
FIGURE 3. The multiple functions of Klotho. FGF23 binds to Klotho/FGFR1c receptor in the renal tubule to increase excretion of phosphate (phosphaturic effect), regulate vitamin D metabolism, and increase calcium reabsorption. Klotho binds to the TGF-β receptor (TβRII component) to block TGF-β action. Klotho inhibits activation of the inflammatory NF-κB pathway by preventing nuclear translocation of the active form. Klotho increases signaling in the Nrf2 pathway; inducing multiple antioxidant enzymes and inhibiting NF-κB. Klotho blocks IGF-1 receptor signaling; this increases activation of FoxO and antioxidant responses. Klotho also blocks activation of the Wnt pathway by binding to soluble Wnt ligands. Klotho expression is increased by PPAR-γ activation, and also by GLP-1 and GABA stimulation; but is suppressed by angiotensin II activation of the AT1 receptor. Abbreviations: Ang II, angiotensin II; Ca2+, calcium ion; FoxO, forkhead boxprotein O; GABA, γ-aminobutyric acid; GLP-1, glucagon-like peptide 1; IGF-1, insulin-like growth factor 1; IGFR, IGF-1 receptor; KL, membrane-bound αKlotho; NF-κB, nuclear factor κB; Nrf2, nuclear factor-erythroid 2-related factor 2; Pi, inorganic phosphate; PPAR-γ, peroxisome proliferator-activated receptors γ; R, receptor; sKL, soluble αKlotho; TGF-β, transforming growth factor β; TβRII, TGF-β receptor type II; Vit D, vitamin D.
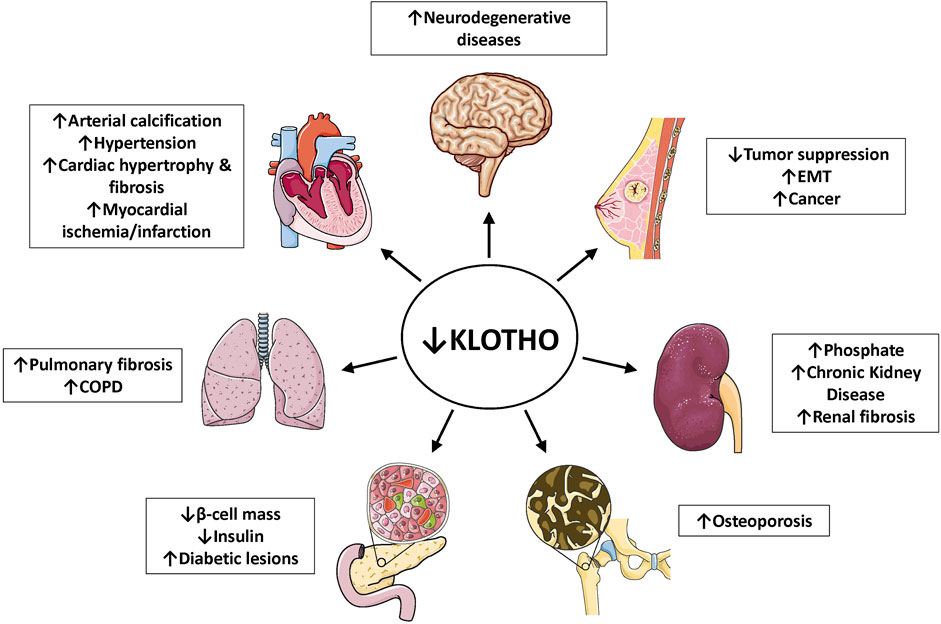
FIGURE 4. Klotho deficiency associates with multiple age-related diseases. As outlined in the captions, depressed Klotho levels are linked to hyperphosphatemia, chronic kidney diseases, multiple cardiovascular conditions, neurodegenerative diseases, several types of cancer, pulmonary fibrosis, COPD, bone disease and diabetes (reduced β-cell mass in the pancreas). References are listed in Table 1. Abbreviations: COPD, chronic obstructive pulmonary disease; EMT, epithelial-mesenchymal transition.
In addition to these topics, we will report on several factors that increase Klotho, including commonly prescribed drugs, recombinant proteins, gene therapies, traditional medicines, nutraceuticals, and exercise.
2 Sites of Klotho Production
Klotho is primarily produced in the kidney (renal tubules), but is also found in the brain (choroid plexus, CSF and neurons), pancreatic β cells, blood vessels and skin (Lim K, et al., 2015). Recent work also documents expression in peripheral blood circulating cells (Martin-Nunez et al., 2022). It is also excreted into the urine. Studies in nephrectomized rodents (Hu et al., 2016), and mice with kidney-specific genetic deletion of Klotho (Lindberg et al., 2014), have revealed the kidney is the principal source of circulating Klotho. It is produced by the proximal and distal convoluted renal tubules, perhaps more in the distal tubules (Lim K, et al., 2015). However, some authors report similar amounts of the membrane-bound form at both locations (Andrukhova et al., 2012; Erben and Andrukhova, 2017). Interestingly, Klotho has different functions in the proximal and distal tubules (Erben and Andrukhova, 2017). In the proximal tubule, Klotho promotes a phosphaturic effect and inhibits vitamin D production, whereas in the distal tubule it enhances Ca2+ reabsorption. Conditional gene knockout of Klotho in the proximal tubule reproduced many of the features of systemic gene knockout, confirming the importance of proximal tubular functions (Takeshita et al., 2017).
3 Molecular Features and Regulation
3.1 Membrane-Bound and Soluble Forms
Klotho consists of a single-pass membrane protein of 1,012 aa (130 kDa), in humans, with a very short intracytoplasmic segment (10 aa) (Xu and Sun 2015; Chen G, et al., 2018). The extracellular component consists of two domains, KL1 and KL2 (Figure 1). The extracellular portion can be cleaved by membrane proteases, primarily ADAM10 and ADAM17 (α-secretases), and this soluble α-Klotho form (s-Klotho) is released into body fluids where it acts as an endocrine hormone (Xu and Sun, 2015; Dalton et al., 2017). Additional cleavage might release smaller KL1 or KL2 fragments, although to our knowledge they have not been detected in the circulation. There is possibly another soluble form of Klotho; thought to be generated by alternative splicing, consisting of KL1 only and often denoted secreted Klotho. However, the sequence contains premature stop codons, and the mRNA is degraded (Mencke, et al., 2017a). Thus, it appears that the vast majority, or perhaps all, soluble Klotho consists of the KL1/KL2 form derived by proteolytic cleaving of the membrane-bound protein (shedding). Indeed, in rodents, administration of inhibitors of secretases mediating this proteolytic cleavage drastically reduce s-Klotho levels in the circulation (Hu et al., 2016).
The regulation of Klotho shedding is not fully elucidated, but the role of the α-secretase ADAM10 and ADAM17 is well documented, especially in renal tubular epithelial cells (Van Loon et al., 2015). The expression level of these enzymes might be increased in various ways, for example through the action of insulin, growth factors and cytokines (Bzowska et al., 2004; Chen CD, et al., 2007; Maretzky et al., 2008; Murphy, 2009). In contrast, tissue inhibitors of metalloproteinases (TIMPs) block the action of ADAM proteins (Chen CD, et al., 2007; Murphy, 2009). Some drugs might increase the proteolytic cleavage of Klotho. There are few published examples; however, ligustilide is a notable case. This natural compound increased the expression of ADAM10 and s-Klotho, and was protective in a mouse model of Alzheimer’s disease (see section 10.4) (Kuang et al., 2014). It is important to note that the ADAM secretase proteins have a very large number of substrates, and can modify many biological processes. Therefore, drugs targeting these enzymes are likely to lack specificity and be subject to toxicity.
The crystal structure of Klotho and its binding sites to FGF receptors (especially FGFR1c) and FGF23 have been reported (Chen G, et al., 2018). No specific receptor for s-Klotho has been identified. However, it binds to some receptors that are widely distributed, on many cell types; notably FGFRs and the TGF-β receptor.
3.2 Regulation of Klotho Expression
Several factors regulate Klotho expression, as reviewed (Xu and Sun, 2015). This may differ considerably in various tissues, and it is only partially understood. In the kidney, which is the main site of production, various factors can alter Klotho expression under physiological and pathological conditions, such as circulatory stress, hypertension, oxidative stress and diabetes. Klotho is generally depressed in inflammatory disorders, and is a potentially useful biomarker of inflammation (Wu and Chen, 2022).
Multiple transcription factors regulate Klotho gene expression, including those binding at promoter sites E-box, Ap-2, PAX4, Sp1 and Oct-1 (Xu and Sun, 2015). PAX4 is a positive regulator. There are PPAR-γ response elements in the 5′ flanking region of the gene that increase transcription. PAX4 and PPAR-γ are relevant to diabetes, as discussed in other sections. Vitamin D binds to vitamin D response elements in the promoter, to upregulate Klotho transcription. In contrast, NF-κB binds to the Klotho promoter but is inhibitory (Moreno et al., 2011; Lin et al., 2021). This might explain, at least in part, the lowered Klotho levels in inflammatory conditions. Notably, angiotensin II is a major inhibitor of Klotho transcription (Figure 3), and this has been attributed to the suppression of the positive regulator Sp1 (Zhou Q, et al., 2010). HMG-CoA reductase is also inhibitory and this can be reversed by statin drugs, which are inhibitors of this enzyme (Narumiya et al., 2004; Kuwahara et al., 2008) (Table 2). Statins upregulate Klotho mRNA, possibly by inactivating RhoA and/or diminishing the angiotensin II response (Narumiya et al., 2004). Interestingly, FGF23 is a strong negative regulator of Klotho transcription (Marsell et al., 2008). As a result, events that increase FGF23 production will reduce Klotho, such as chronic renal disease (Kuro-o, 2019). The Klotho gene is often turned off in cancer cells, possibly through a number of mechanisms (Rubinek and Wolf, 2016). In particular, the Klotho promoter is GC-rich and subject to DNA methylation, and in tumors it can be turned off by DNA hypermethylation. Klotho is also regulated by miRNAs, and this appears to be of importance in cancer (Abolghasemi et al., 2019).
4 Klotho Interactions With FGF23 and FGFRs
The extracellular domain of Klotho binds to either FGFR1c, -3c, or -4 (all receptor tyrosine kinases) to form a high affinity receptor for FGF23 (Figure 2) (Chen G, et al., 2018; Urakawa et al., 2006). In terms of nomenclature, FGF19, FGF21 and FGF23 are of often denoted endocrine FGFs (eFGFs), because they are soluble, enter the circulation and can act at a distance (Phan et al., 2021). FGF23 is an eFGF produced in bone that regulates renal phosphate homeostasis, together with vitamin D and parathyroid hormone (PTH) (Neyra et al., 2021; Rausch and Föller, 2022). The membrane-bound and soluble forms of Klotho can both function as coreceptors for FGF23 (Chen G, et al., 2018). Without Klotho the affinity of FGF23 for the receptor is very low. The activated FGFR signals through PI3K/Akt, phospholipase Cγ (PLCγ) and Ras/MAPK/ERK. The renal physiological functions of Klotho have been extensively reviewed (Erben and Andrukhova, 2017; Kuro-o 2017; Zou et al., 2018; Kuro-o 2019; Kuro-o 2021; Neyra et al., 2021; Saar-Kovrov et al., 2021), and are only briefly described here. Activation of FGFR1c regulates phosphate and calcium exchange in the kidney. This occurs through inhibition of the sodium-phosphate transporters NPT-2a and NPT-2c in the proximal renal tubule, thereby reducing inorganic phosphate (Pi) reabsorption (phosphaturic effect) (Erben, 2018). In the distal convoluted tubule, Ca2+ resorption through TRPV5 channels is enhanced. There is also in the distal tubule increased Na+ reabsorption, through enhanced expression of Na+:Cl− cotransporter (NCC). Of major physiological significance, FGF23/Klotho/FGFR1c suppresses the expression of 1α-hydroxylase in the proximal renal tubule, which inhibits synthesis of active vitamin D, denoted 1,25(OH)2D3 (calcitriol). Among other effects, vitamin D promotes phosphate and calcium absorption in the gut, and this will be reduced. Furthermore, in a Klotho-independent manner, FGF23 reduces secretion of parathyroid hormone (PTH), which additionally influences phosphate calcium balance (Ben-Dov et al., 2007).
A deficiency of either Klotho or FGF23 in mice results in excessive 1α-hydroxylase activity, overproduction of active vitamin D, and associated hyperphosphatemia and hypercalcemia. In Klotho-deficient mice, hypervitaminosis D and hyperphosphatemia both appear to contribute to the accelerated aging phenotype (Kuro-o 2010, 2018, 2019, 2021). However, a low phosphate diet ameliorates disease, even though vitamin D further increases, suggesting that the hyperphosphatemia is more important than vitamin D toxicity in the aging syndrome. Nevertheless, ablation of vitamin D responses attenuated the lesions of Klotho-deficient mice (Anour et al., 2012). It has been proposed that hypersaturation of phosphate and calcium ions in the blood leads to the formation of calciprotein particles (CPPs) (Kuro-o, 2021). The CPPs precipitate in tissues, and are thought to accelerate the pathologic changes associated with aging.
The most common clinical cause of Klotho deficiency is kidney failure, which can result from many acute or chronic conditions (Neyra et al., 2021). Rare human mutations causing a severe deficiency of either Klotho or FGF23 are associated with hyperphosphatemia beginning at an early age (Ito and Fukumoto, 2021). These are autosomal recessive diseases. The subjects develop massive calcification in soft tissues, blood vessels, and in multiple organs and anatomic sites throughout the body. There are bone and dental lesions. There is also evidence of systemic inflammation. Treatment includes low phosphate diet, phosphaturic drugs, anti-inflammatory drugs, and phosphate binding agents. This severe congenital deficiency of either functional Klotho or FGF23 is not comparable to the much less reduced Klotho expression seen in chronic diseases described in this review. Indeed, these are not associated with the massive calcification observed in congenital hyperphosphatemia.
5 β-Klotho
The Klotho homologue denoted β-Klotho (KLB) is an obligatory coreceptor for FGF19 and FGF21, which are both eFGFs (Kilkenny and Rocheleau., 2016). KLB, unlike Klotho, does not appear to have endocrine functions on its own. FGF19 plays a major role in bile acid homeostasis and liver metabolism (Dolegowska et al., 2019; Guthrie et al., 2022). FGF21 is involved in regulating lipid metabolism, insulin secretion and glucose homeostasis (Andersen et al., 2015; Bondurant and Potthoff, 2018; Dolegowska et al., 2019). FGF21 is produced in the liver, pancreatic islets, exocrine pancreas, and other tissues. The liver appears responsible for most of the circulating FGF21. The major metabolic functions of FGF21 have received considerable attention, and it has been examined in clinical trials for the treatment of obesity and diabetes.
6 Klotho Functions Independent of FGF23
6.1 Klotho Inhibits TGF-β
A key protective function of Klotho is the prevention of fibrosis (e.g., pulmonary, renal or cardiac fibrosis), which has been attributed in large part to its ability to block TGF-β, although other pathways contribute (Huang Q, et al., 2020; Mencke et al., 2017b). TGF-β is a pleiotropic cytokine that has been linked to aging by its promotion of cellular senescence, stem cell decline, immunologic impairment, fibrosis and several other age-related pathologies (Mikuła-Pietrasik et al., 2022; Pratsinis et al., 2017; Prud’homme 2007, et al., 2017b; Tominaga and Suzuki, 2019). This is of particular interest, because Klotho binds to the type II TGF-β receptor (TβRII), which blocks TGF-β binding and receptor signaling (Doi et al., 2011) (Figure 3). In the kidneys of aging mice, Klotho levels decline, whereas TGF-β and its signaling molecules increase (Oishi et al., 2021). Administration of s-Klotho protein inhibits TGF-β signaling and protects against renal fibrosis (Doi et al., 2011). In a more specific way, a Klotho-derived peptide (30 amino acids) that inhibits TGF-β by binding to its receptor also protected against renal fibrosis (Yuan et al., 2022). These experiments provide evidence that Klotho can suppress TGF-β activities in vivo.
Practically all cell types express TGF-β receptors, and its importance is considerable. TGF-β signaling and its regulation are complex (Mullen and Wrana, 2017; Derynck and Budi, 2019; Aashaq et al., 2022), and only a brief outline is provided here. TGF-β is secreted in latent form, and is activated by interaction with integrins and other mechanisms. Three isoforms of TGF-β exist (TGF-β1 is the most abundant), which all bind to the same signaling receptor (Groppe et al., 2008; Huang T, et al., 2011; Aashaq et al., 2022). This receptor consists of TβRI (also denoted ALK5) and TβRII. TGF-β binds to TβRII and TβRI forming a serine/threonine kinase complex. TβRII phosphorylates TβRI, which then phosphorylates Smad2 and Smad3 (canonical pathway). Smad2 and 3 form a complex with Smad4 (the common Smad), which translocates into the nucleus. There, it binds to DNA and regulates transcriptional events. Multiple molecules interact with the TGF-β receptor complex, and can alter the binding of TGF-β, or receptor signaling, in a positive or negative fashion (Pawlak and Blobe, 2022; Prud’homme et al., 2017b). For instance, either betaglycan (also denoted TβRIII), neuropilin-1 (Nrp1) or endoglin can associate with the TGF-β receptor, acting as coreceptors, and characteristically enhance TGF-β responses. Klotho also binds to this receptor, but it has the opposite effect.
The activation of several non-canonical (non-Smad2/3 dependent) pathways adds more complexity (Derynck and Budi, 2019; Aashaq et al., 2022; Baba et al., 2022). In some cells, especially endothelial cells, signaling can occur through the alternative ALK1/Smad1,5,8 pathway. Smad4 (common Smad) then participates as it does in the Smad2/3 pathway. The signaling TGF-β receptor can activate ERK, JNK, p38 MAPK, PI3K/Akt, Rho-like GTPases, NF-κB and other pathways. Furthermore, through canonical or non-canonical signaling, TGF-β can crosstalk with bone morphogenetic proteins (BMPs), Wnt, Hedgehog, Notch, Hippo (TAZ/YAP), JAK/STAT; as well as growth factors such as hepatocyte growth factor (HGF) and epidermal growth factor (EGF) (Luo 2017; Mullen and Wrana, 2017; Derynck and Budi, 2019). Thus, TGF-β can impact on a vast number of biologic processes. In the immune system, it is produced by regulatory T cells (Treg), and other cell types, and suppresses or regulates immune responses involving macrophages, dendritic cells, B cells, effector T cells, NK cells and neutrophils (de Streel and Lucas, 2021; Maizels 2021; Prud’homme 2007). Thus, it is a key regulator of the immune system.
The studies of Klotho inhibition of TGF-β mentioned above pertain primarily to renal disease, but are likely applicable to fibrosis elsewhere such as pulmonary fibrosis, and several other age-related conditions. This would be consistent with the ubiquitous expression and multiple functions of TGF-β and its receptors. TGF-β is involved in many of the lesion observed in diabetes. The role of TGF-β in neoplasia is complex and context dependent. At early stages of neoplasia it acts as a tumor suppressor, but it aggravates cancer in the late phases, and contributes to metastatic disease (de Streel and Lucas 2021; Baba et al., 2022). Therefore, its inhibition by Klotho is relevant to cancer therapy.
6.2 Klotho Inhibits NF-κB
Inflammation is thought to be a major contributor to aging, in a process sometimes referred to as inflammaging (de Almeida et al., 2020; Fulop et al., 2021). Chronic, low-grade inflammation can lead to permanent tissue damage. For example, inflammatory changes of various types have been linked to atherosclerosis, chronic renal disease, diabetes-related organ injury, and Alzheimer’s disease. Klotho exerts anti-inflammatory activities that appear to be independent of FGF23. Importantly, Klotho suppresses activation of the inflammatory NF-κB pathway (Buendia et al., 2015, 2016). This pathway plays a key role in initiating immune and/or inflammatory responses mediated by B cells, T cells, macrophages and polymorphonuclear leukocytes (PMLs) (Haga and Okada, 2022; Roberti et al., 2022). It also inhibits apoptosis of immune cells and other cell types, while promoting proliferation. It is activated by T-cell and B-cell costimulatory receptors, several inflammatory cytokines, chemokines, toll-like receptors (TLR), NOD-like receptors (NLR), stimulator of interferon genes (STING), and other factors that promote immunity against infectious agents (Roberti et al., 2022; Haga and Okada, 2022; Zhang T, et al., 2021). However, NF-κB is also implicated in detrimental inflammation contributing to aging, chronic inflammatory conditions and autoimmune diseases. NF-κB is active in many non-immune cell types, such as endothelial cells and some epithelial cells. It contributes to vascular lesions (e.g., atherosclerosis and vasculitis) and, importantly, it plays a detrimental role in cancer (Zhang T, et al., 2021). Thus, NF-κB can contribute the age-related diseases in many ways.
The NF-κB family includes NF-κB1 (p50), NF-κB2 (p52), RelA (p65), and RelB, and the NF-κB activation pathways have been recently reviewed (Roberti et al., 2022; Haga and Okada, 2022; Zhang T, et al., 2021). These proteins all have a Rel homology domain for sequence-specific DNA binding, as well as homo- and hetero-dimerization. There are two routes for signaling, denoted the canonical and non-canocical (alternative) pathways. Early signaling events in the NF-κB pathways involve the activation of kinases, such as TAK1 and the NEMO complex. In the canonical pathway, in the quiescent state, the inhibitor of κB (IκB) is bound to the NF-κB (p50/RelA) and this prevents its activation. In response to stimulatory action, the IκB protein is phosphorylated, ubiquitinated, and subjected to proteasomal proteolysis. This allows NF-κB to migrate into the nucleus and bind to DNA, and induce transcription of a large number of genes. In the non-canonical pathway, RelB is bound to a protein denoted p100. The activation of NF-κB inducing kinase (NIK) leads to phosphorylation of the p100 protein, and its subsequent degradation to the p52 protein. Then, a p52/RelB complex translocates into the nucleus and activates the transcription of the target genes. Interestingly, there is evidence that the two pathways interact, and might not be completely independent.
Major inhibitory functions of Klotho derive from its ability to block NF-κB signaling, as demonstrated in several studies. In this case, Klotho appears to prevent the nuclear translocation of NF-κB, although other mechanisms may also apply, as described below. For instance, Klotho suppressed TNF-α-induced activation of NF-κB in endothelial cells (Maekawa et al., 2009). Uremia is associated with circulating toxins that cause oxidative stress to endothelial cells and induce their senescence. These endothelial changes were attributed to NF-κB activation, and this was relieved by Klotho (Buendia et al., 2015, 2016). The authors concluded that Klotho prevents nuclear translocation of NF-κB, which is an essential step in the activation pathway. Other investigators reported similar findings (Yang, et al., 2012). In a diabetic cardiomyopathy model, Klotho inhibited NF-κB in vitro and in vivo (Guo et al., 2018). This suppression appeared to be due to an increase in nuclear factor-erythroid 2-related factor 2 (Nrf2) activation, which counteracts NF-κB (see below). In diabetic db/db mice, Klotho expression was reduced in the kidney, a finding linked to increased to NF-κB activation (Zhao Y, et al., 2011). This was reversed by the application of Klotho, with a consequent reduction in inflammatory cytokines. Klotho was also found to inhibit NF-κB activation and translocation in clonal β cells (Prud’homme et al., 2017a). In that case, Klotho knockdown with siRNA resulted in spontaneous nuclear translocation of NF-κB p65. This is consistent with Klotho preventing the degradation of the IκB protein, as suggested by others studying alveolar macrophages (Li L, et al., 2015). Interestingly, s-Klotho added to cultures reverses the effects of Klotho knockdown, and prevents NF-κB activation (Li L, et al., 2015; Prud’homme et al., 2017a). The mechanisms of NF-κB inhibition in these various studies may differ, and are not fully elucidated (Typiak and Piwkowska, 2021). Furthermore, once NF-κB is activated and has translocated into the nucleus it can bind to the Klotho promoter and inhibit expression, as previously noted (Moreno et al., 2011; Lin et al., 2021). Thus, under strong acute or chronic inflammatory conditions NF-κB activation might be dominant and, as a result, Klotho expression depressed.
6.3 Klotho Activates the Nrf2 Antioxidant Pathway
Klotho activated Nrf2 in renal, cardiovascular and neurological preclinical disease models (Maltese et al., 2017; Zhu et al., 2017; Xing et al., 2021; Xiang et al., 2022). Nrf2 is a transcription factor that controls responses to oxidative stress and toxins (Dinkova-Kostova et al., 2018; Qu et al., 2020; Panda et al., 2022). Its Neh2 domains interact with a negative regulator denoted Kelch-like ECH-associated protein 1 (Keap1) (Panda et al., 2022). Keap1 binds to Nrf2, promoting its ubiquitination and proteasomal degradation. Under the influence of oxidative stress (or other activators), the thiol groups on cysteine residues in Keap1 are modified and its function is negated, resulting in the release of Nrf2. Then, Nrf2 translocates into the nucleus and forms heterodimers with other proteins, and binds to an enhancer sequence termed antioxidant response element (ARE). ARE is involved in the expression of cellular defense genes that encode, for example, several antioxidant proteins and glutathione-conjugated coenzyme There is also an alternative pathway of Nrf2 regulation not requiring Keap1. Nrf2 protects against oxidative injury and, importantly, inhibits the inflammatory NF-κB pathway (Gao W, et al., 2022). The activation Nrf2 by Klotho appears to be an important factor in the protection against renal, vascular and other diseases.
6.4 Inhibition of the IGF-1 Pathway
The insulin/IGF-1 receptor signaling pathway has long been linked to aging, and it is sensitive to nutrients (Mathew et al., 2017; Johnson 2018). When nutrients are abundant, the downstream mediator denoted mechanistic target of rapamycin (mTOR; a protein kinase) is activated, and it is involved in several age-related disorders. Calorie restriction (CR) can mitigate this effect, and prolong life in some species (Kapahi et al., 2017; von Frieling and Roeder, 2020). Thus, it is of considerable importance that Klotho inhibits the IGF-1/PI3K/Akt/mTOR pathway (Kurosu et al., 2005:; Xie et al., 2013). This is one likely mechanism by which Klotho exerts its antiaging action, and protection against degenerative diseases (Fung et al., 2022). In this case, s-Klotho blocks insulin/IGF-1 receptor activation, preventing downstream signaling events including phosphorylation of insulin receptor substrates (IRS) and PI3K/Akt/mTOR signaling. The mTOR inhibitory drug rapamycin delays age-related disease such as vascular calcification in mice and, interestingly, it increases Klotho expression (Zhao Y, et al., 2015). The insulin/IGF-1 pathway also plays a role in cancer progression, and its inhibition by Klotho is tumor suppressive.
The insulin/IGF-1 pathway connects with antioxidant mechanisms through the FoxO forkhead transcription factors (FOXOs). Blockade of insulin/IGF-1 pathways releases inhibition of the FOXOs, resulting in their nuclear migration and the expression of several genes encoding antioxidant enzymes, such as manganese superoxide dismutase (Yamamoto et al., 2005). In accord with this, FOXO activation is depressed in Klotho-deficient mice, but augmented in Klotho-overexpressing mice.
6.5 Inhibition of the Wnt Pathway
Wnt is another major pathway blocked by Klotho (Liu H, et al., 2007). Wnt is a signaling cascade involved in embryogenesis, stem cell biology, cell fate specification, polarity, mitosis and migration, as recently reviewed (Hayat et al., 2022; Rim et al., 2022). Wnt dysregulation results in anomalies of development, various degenerative conditions and cancer. There is a canonical β-catenin dependent pathway, and a non-canonical (β-catenin independent) pathway (Hayat et al., 2022). Non-canonical has two subtypes, i.e., the planar cell polarity and the Wnt/Ca2+ pathways. In canonical signaling, multiple Wnt ligands are able to bind to the cognate receptor, denoted Frizzled, to initiate a response. In the absence of a Wnt ligand the pathway is inactive. This is due to the constant phosphorylation of cytoplasmic β-catenin, as mediated by a protein complex, which results in its elimination by proteasomal degradation. However, following the binding of a Wnt ligand to Frizzle and coreceptor LRP, the degradation of β-catenin is terminated, and it can migrate into the nucleus to activate target genes through interactions with the T-cell factor/lymphoid enhancer-binding factor (TCF/LEF) transcription factors. This canonical pathway regulates mainly cell proliferation, involving cyclin D1, c-myc and many other proteins; whereas the non-canonical pathways regulate cell polarity and motility (Hayat et al., 2022). The proliferative aspects are particularly relevant to cancer, such as colon cancer and hepatocellular carcinoma.
Klotho blocks Wnt activation by binding to several Wnt ligands, including Wnt1, Wnt3, Wnt4, and Wnt5a (Liu H, et al., 2007; Wang and Sun, 2009). In Klotho-deficient mice, excess Wnt activation promotes cell senescence, and has a negative impact on stem cell survival (Bian et al., 2015). In the kidney, Wnt overexpression is associated with fibrosis (Li X, et al., 2021), and it collaborates with TGF-β in this process. In this respect, Wnt can also cooperate with TGF-β to induce epithelial-mesenchymal transition (EMT), which is a precursor to fibrosis (Li X, et al., 2021). Moreover, EMT has also been linked to cancer stem cell (CSC) differentiation (Prud’homme, 2012; Prud’homme, 2017b). CSCs are highly tumorigenic, promote metastasis and resist chemotherapy.
7 Diabetes and Obesity Relevance
Interestingly, Klotho is depleted in the islets of diabetic patients (Lin and Sun, 2015a). Several investigators have reported depressed circulating Klotho levels in subjects with type 1 diabetes (T1D) (Keles et al., 2016; Tarhani et al., 2020; Zubkiewicz-Kucharska, 2021); and type 2 diabetes (T2D) especially when there is advanced disease (Nie et al., 2017; Fountoulakis et al., 2018; Zhang and Liu, 2018). Circulating levels of s-Klotho are depressed in db/db mice (T2D) (Takenaka et al., 2011) and diabetic NOD mice (T1D) (Prud’homme et al., 2020). There is evidence that Klotho plays an important role in glucose and lipid metabolism, as reviewed (Razzaque, 2012; Donate-Correa et al., 2016; Wan Q, et al., 2017; Landry et al., 2021a). Klotho-deficient mice display islet atrophy and reduced insulin production (Razzaque, 2012). Klotho increases β-cell expression of TRPV2 and enhances Ca2+ entry and the glucose-induced response (Lin and Sun, 2012).
FGFR1c is expressed by β cells, and its attenuation results in diabetes and reduced numbers of β cells (Hart et al., 2000). Both Klotho and KLB are expressed by islet cells, and this suggests these cells can respond to FGF23 (Klotho coreceptor) and FGF21 (KLB coreceptor). FGFR1c promotes signaling through several key pathways for β cells (Figure 2), e.g., PI3K/Akt (promotes cell survival), RAS-MAPK (promotes mitogenic response), PLC-PPAR-adiponectin (regulates glucose and lipid metabolism). In accord with this, Klotho administered by gene transfer in mice demonstrated major protective effects on β cells in T1D or T2D models (Lin and Sun, 2015a,b). Importantly, Klotho reduced β-cell apoptosis and increased the proliferation of these cells. A key pathologic finding in NOD mice is the development of insulitis. This first appears as a peri-islet mononuclear cell infiltrate (including T cells), followed by focal invasion of immune cells into the islets, and subsequently severe infiltration and β-cell loss. Klotho gene transfer reduced insulitis, suggesting an immunosuppressive and/or anti-inflammatory effect. In addition, recombinant Klotho improved renal disease and hypertension in db/db mice (Takenaka et al., 2019). The authors concluded that Klotho inhibited TGF-β and tumor necrosis factor (TNF) signaling, to decrease renal fibrosis.
Systemic γ-aminobutyric acid (GABA) treatment protects against type 1 diabetes (T1D) in mice (Tian et al., 2004; Soltani et al., 2011; Wan Y, et al., 2015; Wang et al., 2019). It induces mouse and human β-cell replication/regeneration, while reducing the apoptosis of these cells (Liu W, et al., 2017, 2021; Purwana et al., 2014; Sarnobat et al., 2022; Soltani et al., 2011; Tian et al., 2013; Untereiner et al., 2019). In terms of mechanisms, we found that GABA increases the production of Klotho, in a multiple low-dose streptozotocin (STZ) model of T1D (Prud’homme et al., 2017a). GABA therapy increased Klotho in the pancreatic β cells, kidneys and plasma. Similarly, in the case of human islets transplanted into immunodeficient mice, we observed that oral GABA treatment increased β-cell Klotho expression and replication, and diminished apoptosis (Liu W, et al., 2021). These effects were ameliorated by co-administration of a dipeptidyl peptidase 4 (DPP-4) inhibitor.
In autoimmune diabetes-prone NOD mice, the injection of s-Klotho protein increased pancreatic β-cell replication and the β-cell mass (Prud’homme et al., 2020). It also reduced insulitis, whereas a Klotho blocking antibody had the opposite effect. These findings were similar to those of others who employed gene transfer methods (Lin and Sun, 2015a,b). In vitro, Klotho stimulated human β-cell survival, replication and insulin secretion (Prud’homme et al., 2017a). It also inhibited NF-κB activation, which could explain the anti-apoptotic effect. Klotho might also protect β cells through antioxidant mechanisms that can be induced, for instance, by Nrf2 and FoxO (Figure 3). Note that although GABA has only been applied in preclinical diabetes, there are numerous GABAergic drugs in clinical use (Palma et al., 2017), to treat epilepsy and other diseases. Unlike systemically applied GABA that is blocked by the BBB, many of these drugs cross this barrier and this can produce multiple adverse effects. Other than GABA, it is unknown whether GABAergic drugs increase Klotho levels.
Paradoxically, Klotho deficient mice (KLkl/kl) are hypoglycemic, despite having low insulin levels (Razzaque 2012). This likely results from the fact that Klotho depresses insulin sensitivity and, therefore, the cells of Klotho-negative mice are more responsive to insulin. This raises the question of whether Klotho is an appropriate agent to treat diabetes. We hypothesize that Klotho therapy of diabetes will be helpful when normal plasma levels are restored. Interestingly, overexpression of Klotho in transgenic mice produced slight insulin resistance, and extended lifespan (Kurosu et al., 2005). In experimental T1D and T2D, as outlined in this review, Klotho therapy was beneficial. Whether humans will respond similarly is unknown. In our work, Klotho protein was administered at a low dose, every 48 h (Prud’homme et al., 2020). It has a half-life of only 7 h (Hu et al., 2016), which is relevant to efficacy and potential adverse effects.
One key issue regarding antidiabetic drugs such as GABA and GLP-1 is whether their general antidiabetic effects (Wang Q, et al., 2019; Drucker, 2018) enhance Klotho indirectly, or there is a more direct effect on Klotho gene expression. This has not been extensively studied, but we hypothesize this is due to a combination of mechanisms. In STZ-induced diabetes, STZ is toxic to the kidney (Cheng MF et al., 2010), not just pancreatic β cells, and it reduces Klotho in the circulation and kidneys (Prud’homme et al., 2017a). This was almost completely reversed by oral GABA administration. Studies of others have shown that GABA treatment protects the kidneys against tubular fibrosis and atrophy, in the subtotal nephrectomy model (Sasaki et al., 2007), and ischemia-reperfusion injury (Kobuchi et al., 2009). This is consistent with the fact that renal tubular cells express GABA receptors (Amenta et al., 1988; Sarang et al., 2008; Takano et al., 2014). Thus, GABA may be protecting renal tubular cells against injury, such that they continue producing Klotho at near-normal levels. However, GABA and/or GLP-1RA directly induced Klotho expression in pancreatic β cells. Of interest, the Klotho promoter has a transcription binding site for PAX4, which is a transcription factor essential for the differentiation of β cells in the pancreas (Sosa-Pineda et al., 1997; Xu and Sun, 2015). GLP-1 induces the expression of PAX4 in human islets (Brun et al., 2008), and therefore, this may (along with other factors) induce the expression of Klotho.
In addition to diabetes, Klotho has been found to have role in obesity. In humans, CSF Klotho levels are depressed in obese subjects (Landry et al., 2021a,b). Intracerebroventricular injection of Klotho in diabetes-prone mice reduced food intake, ameliorated glucose homeostasis and reduced body weight (Landry et al., 2020). This was related to FGFR signaling in neurons. Studies by these authors showed that Klotho acts on the arcuate nucleus of the hypothalamus (ARC) to regulate metabolism (Landry et al., 2021b). Neurons and astrocytes were both targeted, and FGFR signaling through PI3K (neurons) or ERK (astrocytes) was documented. Interestingly, they found no correlation between systemic and CSF concentrations of Klotho, and the mechanism of Klotho regulation in the CSF remains unclear. Circulating Klotho also appears to play and important role in metabolism. Systemic Klotho treatment in mice reduced adiposity and lipid accumulation in the liver; and increased lean mass and energy expenditure (Rao Z, et al., 2019). With Klotho treatment, quantitative PCR revealed lower expression of lipogenic genes.
8 Klotho as a Tumor Suppressor
There is considerable evidence that Klotho is a tumor suppressor molecule, as reviewed elsewhere (Rubinek and Wolf, 2016; Abolghasemi et al., 2019; Sachdeva et al., 2020; Ewendt et al., 2021). This is consistent with its capacity to inhibit pathways linked to cancer, including Wnt, IGF-1, TGF-β and NF-κB, as outlined previously (Figure 3; Table 1). The expression of Klotho is depressed or silenced in almost all types of cancer examined. Klotho-positive tumors generally have a better prognosis. The suppression of Klotho appears to occur by the same mechanism as other tumor suppressor genes. For instance, this involves DNA hypermethylation at promoter sites, histone modifications and miRNAs (Rubinek and Wolf, 2016; Abolghasemi et al., 2019). In experimental tumor models, Klotho has anti-tumor effects in vivo and/or in vitro. This was demonstrated in breast cancer (Wolf et al., 2008; Ligumsky et al., 2015), pancreatic cancer (Abramovitz et al., 2011), and other cancers including colon cancer, gastric cancer, and hepatocellular carcinoma (HCC). For instance, in pancreatic cancer, Klotho was poorly expressed in the tumor (Abramovitz et al., 2011). Overexpression of Klotho, or therapy with s-Klotho, inhibited the growth of pancreatic cancer cells. Indeed, multiple studies have shown that Klotho inhibits cancer-cell proliferation, colony formation and invasion; and promotes apoptosis and autophagy (Sachdeva et al., 2020). Similarly, in vivo, forced tumor overexpression of Klotho, or systemic administration of s-Klotho, inhibited the growth of several types of tumors. Importantly, this includes human tumors xenografted into mice. The membrane-bound and soluble forms of Klotho both have tumor suppressor effects.
9 Klotho and Neurodegenerative Diseases
Low Klotho levels correlate with neurodegenerative disease and cognitive impairment (Vo et al., 2018; Hanson et al., 2021), as well as frailty (Veronesi et al., 2021). For example, in nursing home residents, low serum Klotho was associated with poor cognition, frailty, dependence, and frequent falls (Sanz et al., 2021). The role of Klotho in the brain is not well understood, but there is evidence it is neuroprotective. In the central nervous system, Klotho is produced by the choroid plexus, and is found in the CSF. It is also expressed widely throughout the brain, predominantly in grey matter areas including the hippocampus (Clinton et al., 2013; Cararo-Lopes et al., 2017). It is produced by both neurons and oligodendrocytes. Klotho expression in the brain is decreased in aging subjects and early Alzheimer’s disease (Fung et al., 2022). In mouse models of Alzheimer’s disease, Klotho overexpression is beneficial.
Morphologically, in Alzheimer’s disease, the brain is characterized by an excess of β-amyloid (Aβ) plaques, as well as neuronal aggregation of Tau protein forming neurofibrillary tangles (NFTs) (Thal et al., 2013). It has been proposed that these substances are toxic to neurons. Klotho confers neuronal resistance to oxidative and endoplasmic reticulum stress, and is thought to protect against the toxicity of Aβ and NFTs (Zeldich et al., 2014; Fung et al., 2022). Aβ is generated from the proteolytic cleavage of amyloid precursor protein (APP) by secretases (Lichtenthaler et al., 2022). APP is a type 1 transmembrane protein, and its cleavage by an α-secretase results in the release (shedding) of a large extracellular segment, which does not form amyloid. This cleavage process is accomplished primarily by ADAM10, and is similar to the generation of s-Klotho. However, processing of APP by β-secretase and γ-secretase generates small secreted Aβ peptides that form amyloid. Interestingly, α-secretase cleavage prevents the subsequent generation of amyloidogenic peptides (Lichtenthaler et al., 2022). Therefore, increasing α-secretase activity, or decreasing β- and γ-secretase activity, might be of benefit.
One potential mechanism of disease involves deficient autophagy, which is a process that normally degrades and removes proteins and other substances, preventing their toxic accumulation in the cell. Klotho ameliorates autophagy, and this might protect against neurodegenerative conditions like Alzheimer’s (Fung et al., 2022). Klotho also appears to contribute to oligodendrocytic myelin production, and the maintenance of white matter integrity. There is evidence of an inflammatory component to Alzheimer’s disease, as well as some other neurodegenerative diseases, possibly involving NF-κB activation (Ju wang et al., 2019). Thus, as in other sites, Klotho may exert beneficial anti-inflammatory and anti-oxidative actions in the brain. Klotho might promote differentiation of microglia to the anti-inflammatory type (M2), instead of the inflammatory type (M1) (Fung et al., 2022).
The hippocampus plays a major role in memory. It is one of the few regions of the brain that sustains neurogenesis in adulthood, and there is loss of neurons in that area in Alzheimer’s disease (Rao YL, et al., 2022). Remarkably, recent work indicates that Klotho stimulates neurogenesis in the hippocampus. This was evident, for example, when comparing mice with Klotho deficiency vs. those overexpressing Klotho (Laszczyk et al., 2017). In accord with neuronal loss, Klotho deficient mice demonstrate severe cognitive defects. Recently, intermittent fasting in mice was shown to increase Klotho expression and neurogenesis in the hippocampus (Dias et al., 2021). Intermittent fasting proved superior to caloric restriction, and it was associated with improvement of long-term memory. Klotho-deficient mice did not respond to intermittent fasting. It is possible that drug therapy can achieve similar results. For instance, in a rat model of metabolic syndrome, the DPP-4 inhibitor vildagliptin (increases GLP-1) augmented hippocampal Klotho, concurrently decreased inflammatory and apoptotic biomarkers at that site, and prevented neurodegenerative changes (Yossef et al., 2020). This improved memory in treated rats. Note that most DPP-4 inhibitors, including vildagliptin, do not cross the blood-brain barrier (BBB) but they enhance circulating GLP-1 (Deacon, 2019), which does cross. In a rat model of STZ-induced cognitive impairment, simvastatin increased hippocampal Klotho and improved cognitive function (Adeli et al., 2017). Similarly, others found that rosiglitazone treatment improved cerebral Klotho expression (Chen LJ, et al., 2014). In the latter study, intracerebroventricular infusion of recombinant Klotho was also beneficial. Alternatively, some authors have delivered Klotho by gene transfer (Table 2), such as intracerebroventricular injection of a lentiviral vector encoding Klotho (Zeng et al., 2019). The findings outlined above regarding Klotho in neurodegenerative pathologies have all been obtained in rodents, and it remains to be determined whether they are applicable to humans.
To our knowledge, Klotho-based therapy has not been applied in humans with neurodegenerative diseases. Nevertheless, compared to subjects with low levels of Klotho, those with high levels (CSF or plasma) have superior cognitive functions, and a lower incidence of dementia (Semba et al., 2014; Shardell et al., 2016). It has been thought that Klotho levels in the blood and CSF are independent. Contrary to that view, some investigators have recently reported that serum and CSF levels correlate strongly, and high levels predict better cognitive function (Kundu et al., 2022). This is of major interest for future clinical studies, especially concerning drugs or other treatments that increase Klotho. A caveat is that the reasons for discrepancies between studies are not clear, concerning Klotho levels in the CSF or the circulation. There has been much concern about the specificity of some anti-Klotho antibodies used in ELISA and other assays (Kuro-o, 2019). Some authors recently examined this question in human serum samples from patients with a wide range of kidney function (Neyra et al., 2020). This was done with a widely used commercial ELISA, as well as immunoprecipitation-immunoblotting (IP-IB) with a highly specific Klotho antibody. The IP-IB Klotho results correlated with kidney function much better than the ELISA. Freeze-thaw cycles impaired Klotho detection. These findings suggest that relying solely on an ELISA assay could be misleading, and sample handling is important. Another relevant observation is the wide variability in Klotho levels between individuals. For instance, in a 18–35 age group approximately half of individuals had low levels, comparable to aged subjects, whereas the other half had high levels (Espuch-Oliver et al., 2022). In the 55–85 age group, however, levels were more consistently low. CSF levels are also quite variable (Kundu et al., 2022). In view of this, results obtained from small groups should be interpreted with caution. The biological significance of this variability is unclear, and long-term studies are required.
A Klotho gene variant denoted KL-VS (2 amino acid substitutions in the protein) appears to provide some protection against Alzheimer’s disease, but only in the heterozygous state (Driscoll et al., 2021; Nietzel et al., 2021). Beneficial effects were only observed in some subgroups that were studied. The mode of action of this variant is unclear although, interestingly, a reduction of Tau accumulation has been reported.
9.1 Summary of References for Disease Relevance
See also Table 1. Kidneys: Cheng et al., 2010; Kuro-o, 2010, 2019, 2021; Maquigussa et al., 2018; Oishi et al., 2021; Prud’homme et al., 2017a, 2020; Saar-Kovrov et al., 2021; Takenaka et al., 2017; Xing et al., 2021; Zou et al., 2018. Cardiovascular: Chen K, et al., 2021; Freunlich et al., 2021; Manrique et al., 2016; Myung et al., 2022; Martin-Nunez et al., 2022; Olejnik et al., 2018, 2020; Tyrenkov et al., 2021; Wang F and Zheng, 2022; Yang et al., 2015. Brain: Dias et al., 2021; Driscoll et al., 2021; Cararo-Lopes et al., 2017; Fung et al., 2022; Hanson et al., 2021; Kundu et al., 2022; Laszczyk et al., 2017; Mytych, 2022; Nietzel et al., 2021; Sanz et al., 2021. Cancer: Doi et al., 201; Ewendt et al., 2021; Rubinek and Wolf, 2016; Sachdeva et al., 2020. Lungs: Huang Q. et al., 2020; Gao et al., 2015. Bones: Kuro-o, 2017. 2019, 2021; Tasnim et al., 2021; Metabolism and diabetes: Keles et al., 2016; Landry et al., 2021a,b; Lin and Sun, 2012, 2015a, 2015b; Nie et al., 2017; Prud’homme et al., 2017a, 2020; Razzaque, 2012; Takenaka et al., 2019.
10 Approaches to Klotho-Based Therapy
10.1 Drugs in Clinical Use for Various Conditions
In view of the benefits of Klotho against aging and multiple diseases, as reported here in preclinical models (Figure 4; Table 1), it is of much interest of find applications in people. In humans, it is well established that plasma Klotho levels decrease with age and in common maladies, such as chronic renal diseases, diabetes and neurodegenerative diseases. In view of this, an obvious and safe goal would be to re-establish normal levels. Some drugs have already been mentioned for their potential application in specific diseases. In many of the studies reported (Table 2 and 3) the main effect was an increase in Klotho levels approaching or equal to the normal, rather than above normal. Based on studies in mice, overexpression might possibly extend lifespan, but the safety is less certain because other factors (perhaps insulin resistance) could become important. A caveat is that practically all the information available concerning Klotho-based treatment has been obtained in rodents.
A surprisingly large number of therapies increase Klotho levels in experimental models (Tables 2 and 3), either in the circulation or specific organs. Some of the compounds involved have received considerable attention in the literature as geroprotective molecules (Soo et al., 2020), but usually with scant examination of their Klotho-inducing capacity. This includes several drugs that are currently in clinical use for various conditions (Table 2).
10.1.1 RAAS Inhibitors
RAAS inhibitors are the best documented Klotho-enhancing clinical drugs; especially losartan and valsartan that block the angiotensin II receptor (AT1). Indeed, these are some of the few drugs that have been shown to enhance plasma Klotho in clinical trials (Karalliedde et al., 2013; Lim SC et al., 2014; Janic et al., 2019). For instance, losartan increased Klotho levels by 23% in diabetic patients (Lim SC et al., 2014). This is consistent with the ability of angiotensin II to suppress the expression of Klotho (Yoon HE, 2011). Whether Klotho augmentation contributes to the clinical effectiveness of these drugs is unknown. Of note, these results were obtained in diabetic subjects, and it remains to be determined whether the drugs will act similarly in other diseases, or healthy subjects.
10.1.2 Statins
Statins are another type of frequently applied drugs that increase Klotho (Table 2); however, there is limited evidence from clinical studies. Of major interest, the administration of a low-dose combination of fluvastatin and valsartan enhanced the expression of Klotho and SIRT1, which have both been linked to increased longevity (Janic et al., 2019). In contrast, mTOR and NF-κB were not increased. Statins and RAAS inhibitors are a very common drug combination in medicine, and this type of treatment might have an antiaging effect. Because these drugs have major effects on blood pressure, lipids, renal function, cardiovascular disease and other factors, it will be difficult to establish the specific contribution of Klotho.
10.1.3 Pentoxifylline
Pentoxifylline has been shown to increase Klotho in a clinical trial (Navarro-Gonzalez et al., 2018). This drug is usually applied for the treatment of ischemic peripheral vascular disease, but there are other indications. In diabetic patients (type 2), serum and urinary Klotho were increased, but the change in the serum levels was relatively modest (∼6%). The patients had advanced renal disease, and this may explain the low response. In clinical trials, a major factor to consider is the health of the kidney. Renal atrophy (typically nephrosclerosis) can be severe in patients with diabetes or hypertension, and because most circulating Klotho originates from the kidney this can lower levels. Thus, low Klotho levels are likely to be a reflection of renal disease, and if the renal mass is very low then any drug treatment might fail to increase Klotho.
10.1.4 Vitamin D
Vitamin D supplementation also increases Klotho, as shown in children with chronic kidney disease (Lerch et al., 2018). Children with mild to moderate kidney disease had decreased serum Klotho levels at the beginning of the study, and this was normalized by Vitamin D treatment. In contrast, those with severe renal disease had unchanged Klotho levels. This represents the limited data available from clinical work. In rodents, however, there is clear evidence of Klotho enhancement by vitamin D (Table2). To explain this therapeutic effect, the complex interactions underling FGF23/Klotho/vitamin D homeostasis must be considered, as recently reviewed (Haussler et al., 2020; Neyra et al., 2021).
10.1.5 Rapamycin and Everolimus
Rapamycin (sirolimus) and everolimus are inhibitors of mTOR in clinical use, which have both been shown to increase Klotho (Table 2). Rapamycin has long been proposed as a geroprotective drug. Clinically, rapamycin is applied primarily as an immunosuppressive drug to prevent transplanted organ rejection. The treatment of renal transplant recipients with rapamycin produced hypophosphatemia and insulin resistance (Tataranni et al., 2011). Treatment of proximal renal tubular cells with rapamycin in vitro induced the expression of Klotho. The authors proposed that Klotho was mediating the rapamycin-related clinical alterations. In a renal transplantation clinical trial, everolimus significantly increased Klotho serum levels (Mizusaki et al., 2019). These investigators measured Klotho levels before transplantation and 1 year after. They also compared patients that were treated with everolimus versus those that were not. In both cases, Klotho levels were boosted by this drug. It is of interest that renal transplantation itself increased Klotho, in accord with nephrectomy decreasing Klotho, and other evidence that the kidney is the main source of circulating Klotho (Hu et al., 2016). The increased Klotho levels in the everolimus-treated patients did not correlate significantly with improvements in renal function, including serum creatinine, blood urea nitrogen, eGFR, calcium and phosphorus. This study was small and other immunosuppressive agents were co-administered, which limits the interpretation. In rats with chronic renal failure, oral rapamycin treatment also increased klotho, and this improved vascular calcification (Zhao et al., 2015). The benefits were attributed to Klotho and inhibition of mTOR based on a number of pharmacological and genetic methods. Notably, rapamycin could not prevent the vascular disease when Klotho expression was suppressed with siRNA, or in Klotho knockout mice. These studies point to rapamycin and everolimus as effective drugs for the enhancement of Klotho levels. These drugs, however, can have considerable adverse effects, including depressed immunity against infectious agents, and cell toxicity in some organs. For instance, rapamycin is toxic to pancreatic β cells (Prud’homme et al., 2013).
10.1.6 Antidiabetic Drugs
Several clinical anti-diabetic drugs have been shown to increase Klotho expression in mice, e.g., GLP-1RAs, DPP-4 inhibitors, metformin and peroxisome proliferator-activated receptors γ (PPAR-γ) agonists (Table 2). Some were mentioned previously. Metformin, which is probably the most prescribed antidiabetic drug for T2D, increased Klotho in the circulation, kidneys and urine (Xue et al., 2019). It decreased the levels of mTOR, and this effect was reversed by Klotho suppression. Metformin has long been proposed as a geroprotective drug, although there are contradictory findings (Mohammed et al., 2021). In the case of diabetes treatment, a caveat is that metformin increases insulin sensitivity whereas Klotho has the opposite effect.
PPAR-γ is a transcription factor regulating insulin sensitivity and adipogenesis, and agonistic drugs find applications in diabetes and lipid disorders. PPAR-γ agonists are another class of drugs that enhance Klotho expression (Table 2). There is a non-canonical PPAR-responsive element in the 5′-flanking region of the human Klotho gene (Zhang H, et al., 2008), which likely explains this activity. These authors showed that in vitro and/or in vivo, troglitazone, ciglitazone and rosiglitazone increased Klotho mRNA expression. They observed increased Klotho protein in the kidneys. A PPAR-γ antagonist had the opposite effects.
10.1.6.1 Summary of References for Clinical Drugs That Increase Klotho
See also Table 2. Renin-angiotensin-aldosterone inhibitors: Janic et al., 2019; Li X and Zhou, 2010; Lim SC, et al., 2014; Yoon et al., 2011. Statins: Adeli et al., 2017; Janic et al., 2019; Kuwahara et al., 2008; Narumiya et al., 2004; Yoon et al., 2012. PPAR-γ agonists: Chen LJ, et al., 2014; Cheng et al., 2017; Huang KC, et al., 2014; Maquigussa et al., 2018; Shen et al., 2018; Zhang H, et al., 2008. mTOR inhibitors: Mizusaki et al., 2019; Tabibzadeh, 2021; Tataranni et al., 2011; Zhao Y, et al., 2015. Vitamin D: Haussler et al., 2020; Kuro-o, 2019; Lerch et al., 2018; Tsujikawa al., 2003. Antidiabetic drugs: Manrique et al., 2016; Liu W, et al., 2017, 2021; Son et al., 2019; Wang Q, et al., 2019; Yossef et al., 2020; Xue et al., 2019. Pentoxifylline: Navarro-Gonzalez et al., 2018. Other: Zhou et al., 2022; Kang and Xu, 2016.
10.2 Drugs in Preclinical Development
Numerous drugs, recombinant proteins (or peptides) and gene therapies have been tested in preclinical disease models for their ability to boost Klotho levels (Table 3). Specific examples of the administration of recombinant s-Klotho or peptides were discussed before. Gene therapy has been performed with viral vectors or plasmids, and cell therapy also appears feasible (Franco et al., 2021). In some cases, such as recombinant protein and gene therapies, the feasibility, potential adverse effects, accessibility, and high cost are likely to be limiting factors.
Recombinant Klotho protein therapy has been successfully applied to the treatment of renal, cardiovascular and neurodegenerative disease, as well as diabetes and cancer (Table 3). Generally, these have been short-term study, with injection of s-Klotho, the KL1 domain, or relevant peptides. Low doses are usually effective. Recombinant protein has been delivered systemically, or in the ventricles of the brain for neurological diseases.
Common food/diet components (e.g., astaxanthin, curcumin and resveratrol), and traditional medicines and/or their extracted compounds (e.g., baicalin, cordycepin, ginseng and ligustilide), have been examined and successfully induced Klotho (Table 3). Some of these are frequently incorporated into over-the-counter nutraceuticals. In general, these compounds ameliorated or restored Klotho levels in rodent disease models where they are depressed. This work was performed in animal models, and it is largely unknown whether these substances can increase Klotho in humans.
10.3 Exercise and Fitness
Of major interest, physical exercise and sports are simple ways to increase circulating Klotho, as reviewed by others (Amaro-Gahete et al., 2018). A single bout of exercise transiently increases circulating Klotho (Tan et al., 2018; Morishima and Ochi, 2021). The FIT-AGEING study examined the association between physical activity and fitness and plasma levels of s-Klotho in middle-aged sedentary adult people (Amaro-Gahete et al., 2019a,b). The authors reported that higher activity and fitness associated with increased s-Klotho levels. They assessed the effects of different courses of exercise over a 12-week period, and found that all types increased Klotho, as compared to no exercise. They report that higher s-Klotho associates with decreased fat mass and increased lean mass.
10.3.1 Summary of References for Drugs or Treatments in Development
See also Table 3. GABA receptor agonist: Liu W, et al., 2021; Prud’homme et al., 2017a; Son et al., 2019; Wang Q, et al., 2019. Recombinant Klotho protein or peptides: Ali et al., 2020; Chen TH, et al., 2013; Doi et al., 2011; Gupta et al., 2022; Hu et al., 2017; Leon et al., 2017; Maltese et al., 2017; Mencke et al., 2017; Myung et al., 2022; Oh et al., 2018; Prud’homme et al., 2020; Rao Z, et al., 2019; Takenaka et al., 2018, 2019, 2020; Yang et al., 2015; Yuan et al., 2022; Zhong et al., 2020. Gene therapy: Arbel Rubinstein et al., 2021; Franco et al., 2021; Lin & Sun, 2015a, b; Ni et al., 2021; Mencke et al., 2017; Shin et al., 2019; Xiang et al., 2021; Zeng et al., 2019. Traditional medicines, nutraceuticals and supplements: Dehghani et al., 2019; Feng and Huang, 2022; Hsu et al., 2014; Kamel et al., 2022; Long et al., 2018; Li SS, et al., 2018; Lim SW, et al., 2019; Long et al., 2018; Ostojic and Engeset, 2021; Zhang P, et al., 2016; Zhang XT, et al., 2020; Zhou et al., 2015. Exercise and sports: Amaro-Gahete et al., 2018, 2019a,b; Morishima and Ochi, 2021; Tan et al., 2018.
10.4 The BBB and Treatment of Neurodegenerative Diseases
The BBB (blood-brain barrier) blocks entry of the majority of drugs into the brain, and represents a challenge for the therapy of neurodegenerative conditions, as reviewed (Partridge, 2012; Nilles et al., 2022). Some small lipid-soluble drugs cross by lipid-mediated diffusion.
Other drugs may be shuttled by specific transporters. Large drugs (usually proteins) generally do not cross, or transfer very inefficiently, but transfer can be improved by engineering constructs for receptor-mediated transport. In addition, neuropilin-1 appears to facilitate entry into the brain of some cell-penetrating peptides, larger molecules, or viruses (Balistreri et al., 2021; Prud’homme and Glinka, 2012; Ruoslahti, 2017; Zhao L, et al., 2021).
RAAS blocking drugs represent an interesting example, because they can be divided into BBB-crossing and non-crossing types. For example, the AT1-receptor blockers losartan, irbesartan, olemesartan and eprosartan do not penetrate through the BBB, whereas valsartan, telmisartan and candesartan do cross (Ho et al., 2017). Memory is improved in older adults treated with AT1 blockers, and users of the BBB-crossing drugs performed better than those taking non-crossing drugs (Ho et al., 2017). The subjects receiving BBB-crossing drugs also had significantly fewer white matter hyperintensities (WMH) upon imaging. Other investigators (Ohrui et al., 2004) reported similar findings in patients receiving BBB-crossing angiotensin-converting enzyme (ACE) inhibitors. Those treated with the BBB-crossing drugs (captopril, perindopril) had a significantly lower risk of developing Alzheimer’s disease, compared to those taking non-crossing drugs (imidapril, enalapril). The protective mechanism is unclear. Can the BBB-crossing RAAS inhibitory drugs increase Klotho in the brain or CSF? To our knowledge, there is no data in humans. In the cerebrum of spontaneously hypertensive rats, however, valsartan treatment reduced cell damage, as determined by ultrastructural changes such as apoptotic body formation (Li and Zhou, 2010). Valsartan treatment was associated with increased intra-cerebral expression of Klotho, as determined by RT-PCR, immunohistochemistry, and Western blotting. This provides some evidence, albeit limited, that valsartan can increase Klotho expression in the brain. Statins are thought to have at least some neuroprotective effects. They can also be divided in BBB-crossing and non-crossing. The lipophylic statins (e.g., atorvastatin, lovastatin, simvastatin) cross, and the hydrophilic ones do not (Wood et al., 2010). In this case, simvastatin increased Klotho in the hippocampus of rats (Adeli et al., 2017). It is thus conceivable, but not yet certain, that both RAAS inhibitors and statins can enhance Klotho expression in the human brain, and this might protect against neurodegenerative diseases.
Some antidiabetic drugs (or at least members of these drug families) that enhance circulating Klotho levels in rodents can cross the BBB. This includes some GLP-1RAs (Pujadas and Drucker, 2016), metformin (Moreira, 2014), and at least one DPP-4 inhibitor (omariglipin) (Ayoub et al., 2018). DPP-4 inhibitors increase GLP-1 (Deacon, 2019), the natural GLP-1RA, which crosses the BBB. These antidiabetic drugs possibly ameliorate neurodegenerative disease in some patients (Mehan et al., 2022), but a potential contribution of Klotho, to our knowledge, has not been examined.
As shown in Table 3, several compounds derived from traditional medicines, or other sources, have been shown to increase Klotho expression in rodents. Interestingly, some of these compounds have been reported to cross the BBB, such as astaxanthin, baicalin, cordycepin, ligustilide and resveratrol. Curcumin (Reddy et al., 2018) as well as a component of ginseng, ginsenoside RG1 (Zhao YN, et al., 2018), also appear to cross the BBB at least to some extent. Ligustilide is of special interest, because it readily crossed the BBB and was protective in mice against ischemic brain injury and Alzheimer-like disease. In the ischemic brain, NF-κB and inflammatory changes were reduced (Long et al., 2018). In the Alzheimer’s disease model, IGF-1/Akt/mTOR signaling was inhibited (Kuang et al., 2014). Ligustilide increased Klotho in the choroid plexus. Downregulation of Klotho reduced the protective effects. The neuroprotection appears to depend at least in part on an enhancement of ADAM10 activity. As noted previously, α-secretase cutting of APP by ADAM10 produces shedding of a large non-pathogenic segment, and prevents the generation of small Aβ-forming peptides (Lichtenthaler et al., 2022). Ligustilide treatment enhanced ADAM10 activity resulting in the higher production of both s-Klotho and non-amiloidogenic APP. Aβ plaque formation was reduced in the ligustilide-treated mice. The authors (Kuang et al., 2014) concluded the enhancement of s-Klotho and α-processing of APP both contributed to the amelioration of the neurodegenerative disease.
In some cases, drugs that do not cross the BBB can ameliorate Alzheimer’s disease, or other neurodegenerative conditions, by indirect mechanisms. The improvement of hypertension, hyperlipidemia, diabetes or renal function can all have a major impact on the brain. In young and aged mice, systemic delivery of a Klotho-derived peptide that does not cross the BBB improved cognition and reduced neurodegenerative pathology (Leon et al., 2017). Others showed that systemic delivery of either s-Klotho or only the KL1 domain also improved cognition (Gupta et al., 2022). It is unclear how these beneficial Klotho-based effects are mediated, but penetrance into the brain does not appear to be required, and this merits further investigation.
11 Conclusion and Future Directions
In this manuscript we explored the many facets of Klotho biology. The best characterized aspects relate to renal physiology, and especially phosphate and calcium homeostasis. The importance of the FGF23/Klotho/FGFR interaction was evident in Klotho deficient mice. The major outcomes were hyperphosphatemia and hypervitaminosis D. The accelerated aging syndrome of these mice was greatly ameliorated by a low phosphate diet. Rare mutations of either Klotho or FGF23 in humans result in a hyperphosphatemic syndrome, characterized by massive tissue calcification and systemic inflammation. In chronic renal disease of various etiology, as well as diabetes, Klotho production is decreased. It has been proposed that declining renal function, and associated low Klotho, accelerate or aggravate many of the pathologies found in old age. This may involve, for example, the precipitation of CPPs in tissue (Kuro-o, 2021).
It seems unlikely that all the manifestations of Klotho insufficiency are related to FGF23-induced signaling. Indeed, as outlined in several sections, Klotho blocks major pathways that play a role in aging. This refers specifically to inhibition of TGF-β, Wnt, IGF-1 and NF-κB. These are complex pathways that impact on a vast number of biological processes. Thus, regardless of other mechanisms that may drive aging, the overactivation of these pathways is likely to be important. This is most evident in fibrosis, inflammation and cancer, which are all countered by Klotho. Furthermore, Klotho activates antioxidant pathways, such as Nrf2 and FOXOs, which are thought to mediate antiaging effects.
In terms of future directions, there are several important avenues. Klotho is described as an antiaging molecule, although there is no direct evidence that it delays aging in humans. This is one of the most important questions to address, but it will require very long-term studies. Klotho can be a marker of disease (kidney, brain or other), or a therapeutic molecule. In particular, the function(s) of Klotho in the brain are not well defined, but neuroprotective effects are apparent. Klotho levels in the circulation or CSF could indicate susceptibility to disease. Multiple drugs appear to increase Klotho, but studies in humans are scant. Does Klotho contribute to the therapeutic effects of some drugs used to treat hypertension, hyperlipidemia, diabetes or transplant rejection? Could some of these drugs ameliorate neurodegenerative diseases by enhancing Klotho? This is all unknown. Indeed, there have been practically no clinical investigations of Klotho-related treatment, outside of preclinical work. Another important aspect is the role of Klotho as a tumor suppressor molecule. This is well documented in animals models, but needs to be developed in the clinical context. The slim clinical applications of Klotho research can perhaps be explained by the limited understanding of its mechanisms of action, especially outside the kidney. However, knowledge of the molecular and physiological aspects of Klotho has progressed considerably in recent years, as outlined in this review.
For therapy, several approaches are feasible, as described in this manuscript. Clinical research focused on any of these approaches would be an important contribution to the field. The most direct approach is the administration of recombinant Klotho or peptides. In view of the relatively short half-life of these products, there is a need to produce long-acting constructs, or slow-release formulations. There is little evidence from preclinical work that Klotho administration is toxic, at least when restoring normal levels. However, the long-term effects of supra-normal levels are not known. Gene therapy is also feasible, but much more challenging. In addition to some clinical prescription drugs, there are also commonly available over-the-counter medicines, supplements, or nutraceuticals that increase Klotho. This is based on animal work, and it would be of much interest to examine the possibility of Klotho enhancement in humans. In the case of neurodegenerative diseases it is relevant that some drugs, including some traditional medicines, cross the BBB and might increase Klotho in the brain. Finally, exercise and the long-term maintenance of fitness are simple methods to increase Klotho. In conclusion, most of the studies on Klotho have been performed in animal disease models, and a considerable amount of work has to be performed to introduce Klotho therapy into the clinic. This review provides a framework of mechanisms and findings that favor the clinical investigation of Klotho-based treatments.
Author Contributions
GP wrote the manuscript, MK performed Klotho-related experiments and prepared figures for this manuscript, QW supervised some of the studies reported and edited the manuscript. All authors reviewed and approved the content of this manuscript.
Funding
The work performed by the authors in this manuscript was funded by the Juvenile Diabetes Research Foundation International (grant numbers 2-SRA-2015-64-Q-R and 2-SRA-2018-497-A-B), the St. Michael’s Hospital Foundation (Toronto, Canada), the Keenan Research Centre for Biomedical Science (Toronto, Canada), the Canadian Diabetes Association (grant number OG-3-13-4066), and the National Science Foundation of China (grant numbers 81570518, 81630020, 81800751).
Conflict of Interest
Author QW was employed by the company Shanghai Yinuo Pharmaceutical Co., Ltd. QW holds GLP-1 related patents.
The remaining authors declare that the research was conducted in the absence of any commercial or financial relationships that could be construed as a potential conflict of interest.
Publisher’s Note
All claims expressed in this article are solely those of the authors and do not necessarily represent those of their affiliated organizations, or those of the publisher, the editors and the reviewers. Any product that may be evaluated in this article, or claim that may be made by its manufacturer, is not guaranteed or endorsed by the publisher.
Acknowledgments
The authors thank Dr. Yelena Glinka, Dr. Anna Toulina, Dr. Wenjuan Liu and Dr. Dong Ok Son for their expert work and advice in performing experiments from our laboratories. Some images in the figures were from Servier Medical Art image (smart.servier.com), obtained under the Creative Commons Attribution 3.0 Unported License.
References
Aashaq, S., Batool, A., Mir, S. A., Beigh, M. A., Andrabi, K. I., and Shah, Z. A. (2022). TGF‐β Signaling: A Recap of SMAD‐independent and SMAD‐dependent Pathways. J. Cell. Physiology 237 (1), 59–85. doi:10.1002/jcp.30529
Abolghasemi, M., Yousefi, T., Maniati, M., and Qujeq, D. (2019). The Interplay of Klotho with Signaling Pathway and microRNAs in Cancers. J Cell. Biochem. 120 (9), 14306–14317. doi:10.1002/jcb.29022
Abramovitz, L., Rubinek, T., Ligumsky, H., Bose, S., Barshack, I., Avivi, C., et al. (2011). KL1 Internal Repeat Mediates Klotho Tumor Suppressor Activities and Inhibits bFGF and IGF-I Signaling in Pancreatic Cancer. Clin. Cancer Res. 17 (13), 4254–4266. doi:10.1158/1078-0432.CCR-10-2749
Adeli, S., Zahmatkesh, M., Tavoosidana, G., Karimian, M., and Hassanzadeh, G. (2017). Simvastatin Enhances the Hippocampal Klotho in a Rat Model of Streptozotocin-Induced Cognitive Decline. Prog. Neuro-Psychopharmacology Biol. Psychiatry 72, 87–94. doi:10.1016/j.pnpbp.2016.09.009
Ali, M. F., Venkatarayappa, S. K. B., Benny, M., Rojas, C., Yousefi, K., Shehadeh, L. A., et al. (2020). Effects of Klotho Supplementation on Hyperoxia-Induced Renal Injury in a Rodent Model of Postnatal Nephrogenesis. Pediatr. Res. 88, 565–570. doi:10.1038/s41390-020-0803-z
Amaro-Gahete, F. J., De-la-O, A., Jurado-Fasoli, L., Espuch-Oliver, A., de Haro, T., Gutierrez, A., et al. (2019b2019b). Exercise training increases the S-Klotho plasma levels in sedentary middle-aged adults: A randomised controlled trial. The FIT-AGEING study. J. Sports Sci. 37 (19), 2175–2183. doi:10.1080/02640414.2019.1626048
Amaro-Gahete, F. J., de-la-O, A., Jurado-Fasoli, L., Gutiérrez, Á., Ruiz, J. R., Castillo, M. J., et al. (2019a). Association of physical activity and fitness with S-Klotho plasma levels in middle-aged sedentary adults: The FIT-AGEING study. Maturitas 123, 25–31. doi:10.1016/j.maturitas.2019.02.001
Amaro-Gahete, F. J., de-la-O, A., Jurado-Fasoli, L., Ruiz, J. R., Castillo, M. J., and Gutiérrez, Á. (2019a2018). Role of Exercise on S-Klotho Protein Regulation: A Systematic Review. Cas 11 (2), 100–107. doi:10.2174/1874609811666180702101338
Amenta, F., Cavallotti, C., Iacopino, L., and Erdö, S. a. n. L. (1988). Autoradiographic Localization of the GABAA Receptor Agonist [3H]-Muscimol within Rat Kidney. Pharmacology 36 (6), 390–395. doi:10.1159/000138327
Andersen, B., Omar, B. A., Rakipovski, G., Raun, K., and Ahrén, B. (2015). Fibroblast growth factor 21 prevents glycemic deterioration in insulin deficient mouse models of diabetes. Eur. J. Pharmacol. 764, 189–194. doi:10.1016/j.ejphar.2015.07.003
Andrukhova, O., Zeitz, U., Goetz, R., Mohammadi, M., Lanske, B., and Erben, R. G. (2012). FGF23 Acts Directly on Renal Proximal Tubules to Induce Phosphaturia Through Activation of the ERK1/2-SGK1 Signaling Pathway. Bone 51 (3), 621–628. doi:10.1016/j.bone.2012.05.015
Anour, R., Andrukhova, O., Ritter, E., Zeitz, U., and Erben, R. G. (2012). Klotho lacks a vitamin D independent physiological role in glucose homeostasis, bone turnover, and steady-state PTH secretion In Vivo. PLoS One 7 (2), e31376. doi:10.1371/journal.pone.0031376
Arbel Rubinstein, T., Reuveni, I., Hesin, A., Klein-Goldberg, A., Olauson, H., Larsson, T. E., et al. (2021). A Transgenic Model Reveals the Role of Klotho in Pancreatic Cancer Development and Paves the Way for New Klotho-Based Therapy. Cancers 13 (24), 6297. doi:10.3390/cancers13246297
Ayoub, B. M., Mowaka, S., Safar, M. M., Ashoush, N., Arafa, M. G., Michel, H. E., et al. (2018). Repositioning of Omarigliptin as a once-weekly intranasal Anti-parkinsonian Agent. Sci. Rep. 8 (1), 8959. doi:10.1038/s41598-018-27395-0
Baba, A. B., Rah, B., Bhat, G. R., Mushtaq, I., Parveen, S., Hassan, R., et al. (2022). Transforming Growth Factor-Beta (TGF-β) Signaling in Cancer-A Betrayal Within. Front. Pharmacol. 13, 791272. doi:10.3389/fphar.2022.791272
Balistreri, G., Yamauchi, Y., and Teesalu, T. (2021). A widespread viral entry mechanism: The C-end Rule motif-neuropilin receptor interaction. Proc. Natl. Acad. Sci. U.S.A. 118 (49), e2112457118. doi:10.1073/pnas.2112457118
Ben-Dov, I. Z., Galitzer, H., Lavi-Moshayoff, V., Goetz, R., Kuro-o, M., Mohammadi, M., et al. (2007). The parathyroid is a target organ for FGF23 in rats. J. Clin. Investig. 117 (12), 4003–4008. doi:10.1172/JCI32409
BonDurant, L. D., and Potthoff, M. J. (2018). Fibroblast Growth Factor 21: A Versatile Regulator of Metabolic Homeostasis. Annu. Rev. Nutr. 38, 173–196. doi:10.1146/annurev-nutr-071816-064800
Brun, T., He, K. H. H., Lupi, R., Boehm, B., Wojtusciszyn, A., Sauter, N., et al. (2008). The diabetes-linked transcription factor Pax4 is expressed in human pancreatic islets and is activated by mitogens and GLP-1. Hum. Mol. Genet. 17 (4), 478–489. doi:10.1093/hmg/ddm325
Buchanan, S., Combet, E., Stenvinkel, P., and Shiels, P. G. (2020). Klotho, Aging, and the Failing Kidney. Front. Endocrinol. (Lausanne) 11, 560. doi:10.3389/fendo.2020.00560
Buendía, P., Carracedo, J., Soriano, S., Madueño, J. A., Ortiz, A., Martín-Malo, A., et al. (2015). Klotho Prevents NFκB Translocation and Protects Endothelial Cell From Senescence Induced by Uremia. Gerona 70 (10), 1198–1209. doi:10.1093/gerona/glu170
Buendía, P., Ramírez, R., Aljama, P., and Carracedo, J. (2016). Klotho Prevents Translocation of NFκB. Vitam. Horm. 101, 119–150. doi:10.1016/bs.vh.2016.02.005
Bzowska, M., Jura, N., Lassak, A., Black, R. A., and Bereta, J. (2004). Tumour necrosis factor-α stimulates expression of TNF-α converting enzyme in endothelial cells. Eur. J. Biochem. 271 (13), 2808–2820. doi:10.1111/j.1432-1033.2004.04215.x
Cararo-Lopes, M. M., Mazucanti, C. H. Y., Scavone, C., Kawamoto, E. M., and Berwick, D. C. (2017). The relevance of α-KLOTHO to the central nervous system: Some key questions. Ageing Res. Rev. 36, 137–148. doi:10.1016/j.arr.2017.03.003
Chen, C.-D., Podvin, S., Gillespie, E., Leeman, S. E., and Abraham, C. R. (2007). Insulin stimulates the cleavage and release of the extracellular domain of Klotho by ADAM10 and ADAM17. Proc. Natl. Acad. Sci. U.S.A. 104 (50), 19796–19801. doi:10.1073/pnas.0709805104
Chen, G., Liu, Y., Goetz, R., Fu, L., Jayaraman, S., Hu, M.-C., et al. (2018). α-Klotho is a non-enzymatic molecular scaffold for FGF23 hormone signalling. Nature 553 (7689), 461–466. doi:10.1038/nature25451
Chen, K., Wang, S., Sun, Q. W., Zhang, B., Ullah, M., and Sun, Z. (2021). Klotho Deficiency Causes Heart Aging via Impairing the Nrf2-GR Pathway. Circ. Res. 128 (4), 492–507. doi:10.1161/CIRCRESAHA.120.317348
Chen, L.-J., Cheng, M.-F., Ku, P.-M., and Lin, J.-W. (2014). Rosiglitazone Increases CerebralKlothoExpression to Reverse Baroreflex in Type 1-Like Diabetic Rats. BioMed Res. Int. 2014, 1–9. doi:10.1155/2014/309151
Chen, T.-H., Kuro-o, M., Chen, C.-H., Sue, Y.-M., Chen, Y.-C., Wu, H.-H., et al. (2013). The secreted Klotho protein restores phosphate retention and suppresses accelerated aging in Klotho mutant mice. Eur. J. Pharmacol. 698 (1-3), 67–73. doi:10.1016/j.ejphar.2012.09.032
Cheng, L., Zhang, L., Yang, J., and Hao, L. (2017). Activation of peroxisome proliferator-activated receptor γ inhibits vascular calcification by upregulating Klotho. Exp. Ther. Med. 13 (2), 467–474. doi:10.3892/etm.2016.3996
Cheng, M.-F., Chen, L.-J., and Cheng, J.-T. (2010). Decrease of Klotho in the kidney of streptozotocin-induced diabetic rats. J. Biomed. Biotechnol. 2010, 1–7. doi:10.1155/2010/513853
Clinton, S. M., Glover, M. E., Maltare, A., Laszczyk, A. M., Mehi, S. J., Simmons, R. K., et al. (2013). Expression of klotho mRNA and protein in rat brain parenchyma from early postnatal development into adulthood. Brain Res. 1527, 1–14. doi:10.1016/j.brainres.2013.06.044
Coppede, F. (2021). Mutations Involved in Premature-Ageing Syndromes. Tacg Vol. 14, 279–295. doi:10.2147/TACG.S273525
Dalton, G. D., Xie, J., An, S.-W., and Huang, C.-L. (2017). New Insights into the Mechanism of Action of Soluble Klotho. Front. Endocrinol. 8, 323. doi:10.3389/fendo.2017.00323
de Almeida, A. J. P. O., de Almeida Rezende, M. S., Dantas, S. H., de Lima Silva, S., de Oliveira, J. C. P. L., de Lourdes Assunção Araújo de Azevedo, F., et al. (2020). Unveiling the Role of Inflammation and Oxidative Stress on Age-Related Cardiovascular Diseases. Oxidative Med. Cell. Longev. 2020, 1–20. doi:10.1155/2020/1954398
de Streel, G., and Lucas, S. (2021). Targeting immunosuppression by TGF-β1 for cancer immunotherapy. Biochem. Pharmacol. 192, 114697. doi:10.1016/j.bcp.2021.114697
Deacon, C. F. (2019). Physiology and Pharmacology of DPP-4 in Glucose Homeostasis and the Treatment of Type 2 Diabetes. Front. Endocrinol. 10, 80. doi:10.3389/fendo.2019.00080
Dehghani, A., Hafizibarjin, Z., Najjari, R., Kaseb, F., and Safari, F. (2019). Resveratrol and 1,25-dihydroxyvitamin D co-administration protects the heart against D-galactose-induced aging in rats: evaluation of serum and cardiac levels of klotho. Aging Clin. Exp. Res. 31 (9), 1195–1205. doi:10.1007/s40520-018-1075-x
Derynck, R., and Budi, E. H. (2019). Specificity, versatility, and control of TGF-β family signaling. Sci. Signal. 12 (570), eaav5183. doi:10.1126/scisignal.aav5183
Dias, G. P., Murphy, T., Stangl, D., Ahmet, S., Morisse, B., Nix, A., et al. (2021). Intermittent fasting enhances long-term memory consolidation, adult hippocampal neurogenesis, and expression of longevity gene Klotho. Mol. Psychiatry 26 (11), 6365–6379. doi:10.1038/s41380-021-01102-4
Dinkova-Kostova, A. T., Kostov, R. V., and Kazantsev, A. G. (2018). The role of Nrf2 signaling in counteracting neurodegenerative diseases. FEBS J. 285 (19), 3576–3590. doi:10.1111/febs.14379
Doi, S., Zou, Y., Togao, O., Pastor, J. V., John, G. B., Wang, L., et al. (2011). Klotho Inhibits Transforming Growth Factor-β1 (TGF-β1) Signaling and Suppresses Renal Fibrosis and Cancer Metastasis in Mice. J. Biol. Chem. 286 (10), 8655–8665. doi:10.1074/jbc.M110.174037
Dolegowska, K., Marchelek-Mysliwiec, M., Nowosiad-Magda, M., Slawinski, M., and Dolegowska, B. (2019). FGF19 subfamily members: FGF19 and FGF21. J. Physiol. Biochem. 75 (2), 229–240. doi:10.1007/s13105-019-00675-7
Donate-Correa, J., Martín-Núñez, E., Delgado, N. P., de Fuentes, M. M., Arduan, A. O., Mora-Fernández, C., et al. (2016). Implications of Fibroblast Growth Factor/α-Klotho System in Glucose Metabolism and Diabetes. Cytokine Growth Factor Rev. 28, 71–77. doi:10.1016/j.cytogfr.2015.12.003
Driscoll, I., Ma, Y., Gallagher, C. L., Johnson, S. C., Asthana, S., Hermann, B. P., et al. (2021). Age-Related Tau Burden and Cognitive Deficits Are Attenuated in KLOTHO KL-VS Heterozygotes. Jad 82 (3), 1369–1370. PMID: 34250957]. doi:10.3233/JAD-219006
Drucker, D. J. (20182018). Mechanisms of Action and Therapeutic Application of Glucagon-like Peptide-1. Cell. Metab. 27 (4), 740–756. doi:10.1016/j.cmet.2018.03.001
Erben, R. G., and Andrukhova, O. (2017). FGF23-Klotho signaling axis in the kidney. Bone 100, 62–68. doi:10.1016/j.bone.2016.09.010
Erben, R. G. (2018). Physiological Actions of Fibroblast Growth Factor-23. Front. Endocrinol. 9, 267. doi:10.3389/fendo.2018.00267
Espuch-Oliver, A., Vázquez-Lorente, H., Jurado-Fasoli, L., de Haro-Muñoz, T., Díaz-Alberola, I., López-Velez, M. d. S., et al. (2022). References Values of Soluble α-Klotho Serum Levels Using an Enzyme-Linked Immunosorbent Assay in Healthy Adults Aged 18-85 Years. Jcm 11 (9), 2415. doi:10.3390/jcm11092415
Ewendt, F., Feger, M., and Föller, M. (2021). Role of Fibroblast Growth Factor 23 (FGF23) and αKlotho in Cancer. Front. Cell. Dev. Biol. 8, 601006. doi:10.3389/fcell.2020.601006
Feng, Y., and Huang, Q. (2022)., 22. PubMed, 433–440. doi:10.1111/ggi.14376Protective effects of cordycepin against d ‐galactose‐induced aging in rats: A view from the heartGeriatr. Gerontol. Int.
Fountoulakis, N., Maltese, G., Gnudi, L., and Karalliedde, J. (2018). Reduced Levels of Anti-Ageing Hormone Klotho Predict Renal Function Decline in Type 2 Diabetes. J. Clin. Endocrinol. Metab. 103 (5), 2026–2032. doi:10.1210/jc.2018-00004
Franceschi, C., Garagnani, P., Morsiani, C., Conte, M., Santoro, A., Grignolio, A., et al. (2018). The Continuum of Aging and Age-Related Diseases: Common Mechanisms but Different Rates. Front. Med. 5, 61. doi:10.3389/fmed.2018.00061
Franco, M. L., Beyerstedt, S., and Rangel, É. B. (2021). Klotho and Mesenchymal Stem Cells: A Review on Cell and Gene Therapy for Chronic Kidney Disease and Acute Kidney Disease. Pharmaceutics 14 (1), 11. doi:10.3390/pharmaceutics14010011
Freundlich, M., Gamba, G., and Rodriguez-Iturbe, B. (2021). Fibroblast growth factor 23-Klotho and hypertension: experimental and clinical mechanisms. Pediatr. Nephrol. 36 (10), 3007–3022. doi:10.1007/s00467-020-04843-6
Frieling, J., and Roeder, T. (2020). Factors that affect the translation of dietary restriction into a longer life. IUBMB Life 72 (5), 814–824. doi:10.1002/iub.2224
Fulop, T., Larbi, A., Pawelec, G., Khalil, A., Cohen, A. A., Hirokawa, K., et al. (2021). Immunology of Aging: the Birth of Inflammaging. Clin. Rev. Allerg. Immunol., 1–14. doi:10.1007/s12016-021-08899-6
Fung, T. Y., Iyaswamy, A., Sreenivasmurthy, S. G., Krishnamoorthi, S., Guan, X.-J., Zhu, Z., et al. (2022). Klotho an Autophagy Stimulator as a Potential Therapeutic Target for Alzheimer's Disease: A Review. Biomedicines 10 (3), 705. doi:10.3390/biomedicines10030705
Gao, W., Guo, L., Yang, Y., Wang, Y., Xia, S., Gong, H., et al. (2022). Dissecting the Crosstalk Between Nrf2 and NF-κB Response Pathways in Drug-Induced Toxicity. Front. Cell. Dev. Biol. 9, 809952. doi:10.3389/fcell.2021.809952
Gao, W., Yuan, C., Zhang, J., Li, L., Yu, L., Wiegman, C. H., et al. (2015). Klotho expression is reduced in COPD airway epithelial cells: effects on inflammation and oxidant injury. Clin. Sci. (Lond) 129 (12), 1011–1023. doi:10.1042/CS20150273
Groppe, J., Hinck, C. S., Samavarchi-Tehrani, P., Zubieta, C., Schuermann, J. P., Taylor, A. B., et al. (2008). Cooperative Assembly of TGF-β Superfamily Signaling Complexes Is Mediated by Two Disparate Mechanisms and Distinct Modes of Receptor Binding. Mol. Cell. 29 (2), 157–168. doi:10.1016/j.molcel.2007.11.039
Guo, Y., Zhuang, X., Huang, Z., Zou, J., Yang, D., Hu, X., et al. (2018). Klotho protects the heart from hyperglycemia-induced injury by inactivating ROS and NF-κB-mediated inflammation Both In Vitro and In Vivo. Biochimica Biophysica Acta (BBA) - Mol. Basis Dis. 1864 (1), 238–251. doi:10.1016/j.bbadis.2017.09.029
Gupta, S., Moreno, A. J., Wang, D., Leon, J., Chen, C., Hahn, O., et al. (2022). Pubmed, JN–RM. JN-RM-2458-21. doi:10.1523/JNEUROSCI.2458-21.2022KL1 domain of longevity factor klotho mimics the metabolome of cognitive stimulation and enhances cognition in young and aging miceJ. Neurosci.
Guthrie, G., Vonderohe, C., and Burrin, D. (2022). Fibroblast growth factor 15/19 expression, regulation, and function: An overview. Mol. Cell. Endocrinol. 548, 111617. doi:10.1016/j.mce.2022.111617
Haga, M., and Okada, M. (2022). Systems approaches to investigate the role of NF-κB signaling in aging. Biochem. J. 479 (2), 161–183. doi:10.1042/BCJ20210547
Hanson, K., Fisher, K., and Hooper, N. M. (2021). Exploiting the neuroprotective effects of α-klotho to tackle ageing- and neurodegeneration-related cognitive dysfunction. Neuronal Signal 5 (2), NS20200101. doi:10.1042/NS20200101
Hart, A. W., Baeza, N., Apelqvist, Å., and Edlund, H. (20002000). Attenuation of FGF signalling in mouse β-cells leads to diabetes. Nature 408 (6814), 864–868. doi:10.1038/35048589
Haussler, M. R., Livingston, S., Sabir, Z. L., Haussler, C. A., and Jurutka, P. W. (2020). Vitamin D Receptor Mediates a Myriad of Biological Actions Dependent on Its 1,25‐ Dihydroxyvitamin D Ligand: Distinct Regulatory Themes Revealed by Induction of Klotho and Fibroblast Growth Factor‐23. JBMR Plus 5 (1), e10432. doi:10.1002/jbm4.10432
Hayat, R., Manzoor, M., and Hussain, A. (2022)., 46. PubMed, 863–877. doi:10.1002/cbin.11797Wnt signaling pathway: A comprehensive reviewCell. Biol. Int.
Ho, J. K., Nation, D. A., and Nation, D. A.Alzheimer’s Disease Neuroimaging Initiative (2017). Memory is preserved in older adults taking AT1 receptor blockers. Alz Res. Ther. 9 (1), 33. doi:10.1186/s13195-017-0255-9
Hsu, S.-C., Huang, S.-M., Chen, A., Sun, C.-Y., Lin, S.-H., Chen, J.-S., et al. (2014). Resveratrol increases anti-aging Klotho gene expression via the activating transcription factor 3/c-Jun complex-mediated signaling pathway. Int. J. Biochem. Cell. Biol. 53, 361–371. doi:10.1016/j.biocel.2014.06.002
Hu, M. C., Bian, A., Neyra, J., and Zhan, M. (2015). Klotho, stem cells, and aging. Cia 10, 1233–1243. [. doi:10.2147/CIA.S84978
Hu, M. C., Kuro-o, M., and Moe, O. W. (2013). Klotho and chronic kidney disease. Contrib. Nephrol. 180, 47–63. doi:10.1159/000346778
Hu, M. C., Shi, M., Gillings, N., Flores, B., Takahashi, M., Kuro-o, M., et al. (2017). Recombinant α-Klotho may be prophylactic and therapeutic for acute to chronic kidney disease progression and uremic cardiomyopathy. Kidney Int. 91 (5), 1104–1114. doi:10.1016/j.kint.2016.10.034
Hu, M. C., Shi, M., Zhang, J., Addo, T., Cho, H. J., Barker, S. L., et al. (2016). Renal Production, Uptake, and Handling of Circulating αKlotho. J Am Soc Nephrol 27 (1), 79–90. doi:10.1681/ASN.2014101030
Huang, K.-C., Cherng, Y.-G., Chen, L.-J., Hsu, C.-T., and Cheng, J.-T. (2014). Rosiglitazone is effective to improve renal damage in type-1-like diabetic rats. Horm. Metab. Res. 46 (4), 240–244. doi:10.1055/s-0033-1357161
Huang, Q., Chen, Y., Shen, S., Wang, Y., Liu, L., Wu, S., et al. (2020). Klotho antagonizes pulmonary fibrosis through suppressing pulmonary fibroblasts activation, migration, and extracellular matrix production: a therapeutic implication for idiopathic pulmonary fibrosis. Aging 12 (7), 5812–5831. doi:10.18632/aging.102978
Huang, T., David, L., Mendoza, V., Yang, Y., Villarreal, M., De, K., et al. (2011). TGF-β signalling is mediated by two autonomously functioning TβRI:TβRII pairs. EMBO J. 30 (7), 1263–1276. doi:10.1038/emboj.2011.54
Ito, N., and Fukumoto, S. (2021). Congenital Hyperphosphatemic Conditions Caused by the Deficient Activity of FGF23. Calcif. Tissue Int. 108 (1), 104–115. doi:10.1007/s00223-020-00659-6
Janić, M., Lunder, M., Novaković, S., Škerl, P., and Šabovič, M. (2019). Expression of Longevity Genes Induced by a Low-Dose Fluvastatin and Valsartan Combination with the Potential to Prevent/Treat "Aging-Related Disorders". Ijms 20 (8), 1844. doi:10.3390/ijms20081844
Javidnia, S., Cranwell, S., Mueller, S. H., Selman, C., Tullet, J. M. A., Kuchenbaecker, K., et al. (2022). Mendelian randomization analyses implicate biogenesis of translation machinery in human aging. Genome Res. 32 (2), 258–265. doi:10.1101/gr.275636.121
Johnson, S. C. (2018). Nutrient Sensing, Signaling and Ageing: The Role of IGF-1 and mTOR in Ageing and Age-Related Disease. Subcell. Biochem. 90, 49–97. doi:10.1007/978-981-13-2835-0_3
Ju Hwang, C., Choi, D.-Y., Park, M. H., and Hong, J. T. (2019). NF-κB as a Key Mediator of Brain Inflammation in Alzheimer's Disease. Cnsnddt 18 (1), 3–10. doi:10.2174/1871527316666170807130011
Kamel, S. S., Baky, N. A. A., Karkeet, R. M., Osman, A. M. M., Sayed‐Ahmed, M. M., and Fouad, M. A. (2022). Astaxanthin extenuates the inhibition of aldehyde dehydrogenase and Klotho protein expression in cyclophosphamide‐induced acute cardiomyopathic rat model. Clin. Exp. Pharma Physio 49 (2), 291–301. doi:10.1111/1440-1681.13598
Kang, W.-L., and Xu, G.-S. (2016). Atrasentan increased the expression of klotho by mediating miR-199b-5p and prevented renal tubular injury in diabetic nephropathy. Sci. Rep. 6, 19979. doi:10.1038/srep19979
Kapahi, P., Kaeberlein, M., and Hansen, M. (2017). Dietary restriction and lifespan: Lessons from invertebrate models. Ageing Res. Rev. 39, 3–14. [PMID: 28007498]. doi:10.1016/j.arr.2016.12.005
Karalliedde, J., Maltese, G., Hill, B., Viberti, G., and Gnudi, L. (2013). Effect of renin-angiotensin system blockade on soluble Klotho in patients with type 2 diabetes, systolic hypertension, and albuminuria. Clin J Am Soc Nephrol 8 (11), 1899–1905. doi:10.2215/CJN.02700313
Keles, N., Dogan, B., Kalcik, M., Caliskan, M., Keles, N. N., Aksu, F., et al. (2016). Is serum Klotho protective against atherosclerosis in patients with type 1 diabetes mellitus? J. Diabetes its Complicat. 30 (1), 126–132. doi:10.1016/j.jdiacomp.2015.09.013
Kilkenny, D. M., and Rocheleau, J. V. (2016). The FGF21 Receptor Signaling Complex. Vitam. Horm. 101, 17–58. doi:10.1016/bs.vh.2016.02.008
Kinoshita, S., and Kawai, M. (2016). The FGF23/KLOTHO Regulatory Network and Its Roles in Human Disorders. Vitam. Horm. 101, 151–174. doi:10.1016/bs.vh.2016.02.001
Kobuchi, S., Shintani, T., Sugiura, T., Tanaka, R., Suzuki, R., Tsutsui, H., et al. (2009). Renoprotective effects of γ-aminobutyric acid on ischemia/reperfusion-induced renal injury in rats. Eur. J. Pharmacol. 623 (1-3), 113–118. doi:10.1016/j.ejphar.2009.09.023
Kresovich, J. K., and Bulka, C. M. (2021).Low serum klotho associated with all-cause mortality among a nationally representative sample of American adults. J. Gerontol. A Biol. Sci. Med. Sci. 77, glab308452–456. doi:10.1093/gerona/glab308
Kuang, X., Chen, Y.-S., Wang, L.-F., Li, Y.-J., Liu, K., Zhang, M.-X., et al. (2014). Klotho upregulation contributes to the neuroprotection of ligustilide in an Alzheimer's disease mouse model. Neurobiol. Aging 35 (1), 169–178. doi:10.1016/j.neurobiolaging.2013.07.019
Kundu, P., Zimmerman, B., Quinn, J. F., Kaye, J., Mattek, N., Westaway, S. K., et al. (2022)., 86. PubMed, 1471–1481. doi:10.3233/JAD-215719Serum Levels of α-Klotho Are Correlated with Cerebrospinal Fluid Levels and Predict Measures of Cognitive FunctionJad
Kuro-O, M. (2018). Molecular Mechanisms Underlying Accelerated Aging by Defects in the FGF23-Klotho System. Int. J. Nephrol. 2018, 9679841. doi:10.1155/2018/9679841
Kuro-o, M. (2010). A potential link between phosphate and aging-Lessons from Klotho-deficient mice. Mech. Ageing Dev. 131 (4), 270–275. doi:10.1016/j.mad.2010.02.008
Kuro-o, M. (2021). Klotho and calciprotein particles as therapeutic targets against accelerated ageing. Clin. Sci. (Lond) 135 (15), 1915–1927. doi:10.1042/CS20201453
Kuro-o, M. (2012). Klotho in health and disease. Curr. Opin. Nephrol. Hypertens. 21 (4), 362–368. doi:10.1097/MNH.0b013e32835422ad
Kuro-o, M., Matsumura, Y., Aizawa, H., Kawaguchi, H., Suga, T., Utsugi, T., et al. (1997). Mutation of the mouse klotho gene leads to a syndrome resembling ageing. Nature 390 (6655), 45–51. doi:10.1038/36285
Kuro-o, M. (2017). The FGF23 and Klotho system beyond mineral metabolism. Clin. Exp. Nephrol. 21 (Suppl. 1), 64–69. doi:10.1007/s10157-016-1357-6
Kuro-o, M. (2019). The Klotho proteins in health and disease. Nat. Rev. Nephrol. 15 (1), 27–44. doi:10.1038/s41581-018-0078-3
Kurosu, H., Yamamoto, M., Clark, J. D., Pastor, J. V., Nandi, A., Gurnani, P., et al. (2005). Suppression of aging in mice by the hormone Klotho. Science 309 (5742), 1829–1833. doi:10.1126/science.1112766
Kuwahara, N., Sasaki, S., Kobara, M., Nakata, T., Tatsumi, T., Irie, H., et al. (2008). HMG-CoA reductase inhibition improves anti-aging klotho protein expression and arteriosclerosis in rats with chronic inhibition of nitric oxide synthesis. Int. J. Cardiol. 123 (2), 84–90. PMID: 17434618]. doi:10.1016/j.ijcard.2007.02.029
Landry, T., Laing, B. T., Li, P., Bunner, W., Rao, Z., Prete, A., et al. (2020). Central α-Klotho Suppresses NPY/AgRP Neuron Activity and Regulates Metabolism in Mice. Diabetes 69 (7), 1368–1381. doi:10.2337/db19-0941
Landry, T., Li, P., Shookster, D., Jiang, Z., Li, H., Laing, B. T., et al. (2021b). Centrally circulating α-klotho inversely correlates with human obesity and modulates arcuate cell populations in mice. Mol. Metab. 44, 101136. doi:10.1016/j.molmet.2020.101136
Landry, T., Shookster, D., and Huang, H. (2021a). Circulating α-klotho regulates metabolism via distinct central and peripheral mechanisms. Metabolism 121, 154819. doi:10.1016/j.metabol.2021.154819
Laszczyk, A. M., Fox-Quick, S., Vo, H. T., Nettles, D., Pugh, P. C., Overstreet-Wadiche, L., et al. (2017). Klotho regulates postnatal neurogenesis and protects against age-related spatial memory loss. Neurobiol. Aging 59, 41–54. doi:10.1016/j.neurobiolaging.2017.07.008
Leon, J., Moreno, A. J., Garay, B. I., Chalkley, R. J., Burlingame, A. L., Wang, D., et al. (2017). Peripheral Elevation of a Klotho Fragment Enhances Brain Function and Resilience in Young, Aging, and α-Synuclein Transgenic Mice. Cell. Rep. 20 (6), 1360–1371. doi:10.1016/j.celrep.2017.07.024
Lerch, C., Shroff, R., Wan, M., Rees, L., Aitkenhead, H., Kaplan Bulut, I., et al. (2018). Effects of nutritional vitamin D supplementation on markers of bone and mineral metabolism in children with chronic kidney disease. Nephrol. Dial. Transpl. 33 (12), 2208–2217. doi:10.1093/ndt/gfy012
Li, L., Wang, Y., Gao, W., Yuan, C., Zhang, S., Zhou, H., et al. (2015). Klotho Reduction in Alveolar Macrophages Contributes to Cigarette Smoke Extract-induced Inflammation in Chronic Obstructive Pulmonary Disease. J. Biol. Chem. 290 (46), 27890–27900. doi:10.1074/jbc.M115.655431
Li, S.-s., He, A.-l., Deng, Z.-y., and Liu, Q.-f. (2018). Ginsenoside-Rg1 Protects against Renal Fibrosis by Regulating the Klotho/TGF-β1/Smad Signaling Pathway in Rats with Obstructive Nephropathy. Biol. Pharm. Bull. 41 (4), 585–591. doi:10.1248/bpb.b17-00934
Li, X., and Zhou, Q. (2010). Effect of valsartan on ultrastructure in the brain tissue and expression of Klotho gene in spontaneously hypertensive rats. Zhong Nan Da Xue Xue Bao Yi Xue Ban. 35 (3), 254–261. doi:10.3969/j.issn.1672-7347.2010.03.011
Li, X., Lu, P., Shao, X.-F., Jiang, T., Liu, F., and Li, G. (2021). Klotho Regulates Epithelial-to-Mesenchymal Transition In Vitrovia Wnt/β-Catenin Pathway and Attenuates Chronic Allograft Dysfunction in a Rat Renal Transplant Model. Ann. Transpl. 26, e930066. doi:10.12659/AOT.930066
Lichtenthaler, S. F., Tschirner, S. K., and Steiner, H. (2022). Secretases in Alzheimer's disease: Novel insights into proteolysis of APP and TREM2. Curr. Opin. Neurobiol. 72, 101–110. doi:10.1016/j.conb.2021.09.003
Ligumsky, H., Rubinek, T., Merenbakh-Lamin, K., Yeheskel, A., Sertchook, R., Shahmoon, S., et al. (2015). Tumor Suppressor Activity of Klotho in Breast Cancer Is Revealed by Structure-Function Analysis. Mol. Cancer Res. 13 (10), 1398–1407. doi:10.1158/1541-7786.MCR-15-0141
Lim, K., Groen, A., Molostvov, G., Lu, T., Lilley, K. S., Snead, D., et al. (2015). α-Klotho Expression in Human Tissues. J. Clin. Endocrinol. Metabolism 100 (10), E1308–E1318. doi:10.1210/jc.2015-1800
Lim, S. C., Liu, J.-J., Subramaniam, T., and Sum, C. F. (2014). Elevated circulating alpha-klotho by angiotensin II receptor blocker losartan is associated with reduction of albuminuria in type 2 diabetic patients. J. Renin Angiotensin Aldosterone Syst. 15 (4), 487–490. doi:10.1177/1470320313475905
Lim, S. W., Shin, Y. J., Luo, K., Quan, Y., Cui, S., Ko, E. J., et al. (2019). Ginseng increases Klotho expression by FoxO3-mediated manganese superoxide dismutase in a mouse model of tacrolimus-induced renal injury. Aging 11 (15), 5548–5569. doi:10.18632/aging.102137
Lin, W., Wu, X., Wen, J., Fei, Y., Wu, J., Li, X., et al. (2021). Nicotinamide retains Klotho expression and ameliorates rhabdomyolysis-induced acute kidney injury. Nutrition 91-92, 111376. doi:10.1016/j.nut.2021.111376
Lin, Y., and Sun, Z. (2015b2015). Antiaging Gene Klotho Attenuates Pancreatic β-Cell Apoptosis in Type 1 Diabetes. Diabetes 64 (12), 4298–4311. doi:10.2337/db15-0066
Lin, Y., and Sun, Z. (2012). Antiaging Gene Klotho Enhances Glucose-Induced Insulin Secretion by Up-Regulating Plasma Membrane Levels of TRPV2 in MIN6 β-Cells. Endocrinology 153 (7), 3029–3039. doi:10.1210/en.2012-1091
Lin, Y., and Sun, Z. (2015a2015). In Vivo Pancreatic β-Cell-Specific Expression of Antiaging Gene Klotho: A Novel Approach for Preserving β-Cells in Type 2 Diabetes. Diabetes 64 (4), 1444–1458. doi:10.2337/db14-0632
Lindberg, K., Amin, R., Moe, O. W., Hu, M.-C., Erben, R. G., Östman Wernerson, A., et al. (2014). The kidney is the principal organ mediating klotho effects. Clin J Am Soc Nephrol 25 (10), 2169–2175. doi:10.1681/ASN.2013111209
Liu, H., Fergusson, M. M., Castilho, R. M., Liu, J., Cao, L., Chen, J., et al. (2007). Augmented Wnt signaling in a mammalian model of accelerated aging. Science 317 (5839), 803–806. doi:10.1126/science.1143578
Liu, W., Lau, H. K., Son, D. O., Jin, T., Yang, Y., Zhang, Z., et al. (2021). Combined use of GABA and sitagliptin promotes human β-cell proliferation and reduces apoptosis. J. Endocrinol. 248 (2), 133–143. doi:10.1530/JOE-20-0315
Liu, W., Son, D. O., Lau, H. K., Zhou, Y., Prud’homme, G. J., Jin, T., et al. (2017). Combined Oral Administration of GABA and DPP-4 Inhibitor Prevents Beta Cell Damage and Promotes Beta Cell Regeneration in Mice. Front. Pharmacol. 8, 362. doi:10.3389/fphar.2017.00362
Long, F.-Y., Shi, M.-Q., Zhou, H.-J., Liu, D.-L., Sang, N., and Du, J.-R. (2018). Klotho upregulation contributes to the neuroprotection of ligustilide against cerebral ischemic injury in mice. Eur. J. Pharmacol. 820, 198–205. doi:10.1016/j.ejphar.2017.12.019
Luo, K. (2017). Signaling Cross Talk between TGF-β/Smad and Other Signaling Pathways. Cold Spring Harb. Perspect. Biol. 9 (1), a022137. doi:10.1101/cshperspect.a022137
Maekawa, Y., Ishikawa, K., Yasuda, O., Oguro, R., Hanasaki, H., Kida, I., et al. (2009). Klotho suppresses TNF-α-induced expression of adhesion molecules in the endothelium and attenuates NF-κB activation. Endocr 35 (3), 341–346. doi:10.1007/s12020-009-9181-3
Maizels, R. M. (2021). The multi-faceted roles of TGF-β in regulation of immunity to infection. Adv. Immunol. 150, 1–42. doi:10.1016/bs.ai.2021.05.001
Maltese, G., Psefteli, P.-M., Rizzo, B., Srivastava, S., Gnudi, L., Mann, G. E., et al. (2017). The anti-ageing hormone klotho induces Nrf2-mediated antioxidant defences in human aortic smooth muscle cells. J. Cell. Mol. Med. 21 (3), 621–627. doi:10.1111/jcmm.12996
Manrique, C., Habibi, J., Aroor, A. R., Sowers, J. R., Jia, G., Hayden, M. R., et al. (2016). Dipeptidyl peptidase-4 inhibition with linagliptin prevents western diet-induced vascular abnormalities in female mice. Cardiovasc Diabetol. 15, 94. doi:10.1186/s12933-016-0414-5
Maquigussa, E., Paterno, J. C., de Oliveira Pokorny, G. H., da Silva Perez, M., Varela, V. A., da Silva Novaes, A., et al. (2018). Klotho and PPAR Gamma Activation Mediate the Renoprotective Effect of Losartan in the 5/6 Nephrectomy Model. Front. Physiol. 9, 1033. doi:10.3389/fphys.2018.01033
Maretzky, T., Scholz, F., Köten, B., Proksch, E., Saftig, P., and Reiss, K. (2008). ADAM10-mediated E-cadherin release is regulated by proinflammatory cytokines and modulates keratinocyte cohesion in eczematous dermatitis. J. Investigative Dermatology 128 (7), 1737–1746. doi:10.1038/sj.jid.5701242
Marsell, R., Krajisnik, T., Goransson, H., Ohlsson, C., Ljunggren, O., Larsson, T. E., et al. (2008). Gene expression analysis of kidneys from transgenic mice expressing fibroblast growth factor-23. Nephrol. Dial. Transplant. 23 (3), 827–833. doi:10.1093/ndt/gfm672
Martín-Núñez, E., Pérez-Castro, A., Tagua, V. G., Hernández-Carballo, C., Ferri, C., Pérez-Delgado, N., et al. (2022). Klotho expression in peripheral blood circulating cells is associated with vascular and systemic inflammation in atherosclerotic vascular disease. Sci. Rep. 12 (1), 8422. doi:10.1038/s41598-022-12548-z
Mathew, R., Pal Bhadra, M., and Bhadra, U. (2017). Insulin/insulin-like growth factor-1 signalling (IIS) based regulation of lifespan across species. Biogerontology 18 (1), 35–53. doi:10.1007/s10522-016-9670-8
Mehan, S., Bhalla, S., Siddiqui, E. M., Sharma, N., Shandilya, A., and Khan, A. (2022). Potential Roles of Glucagon-Like Peptide-1 and Its Analogues in Dementia Targeting Impaired Insulin Secretion and Neurodegeneration. Dnnd Vol. 12, 31–59. doi:10.2147/DNND.S247153
Mencke, R., Harms, G., Moser, J., van Meurs, M., Diepstra, A., Leuvenink, H. G., et al. (2017a). Human alternative Klotho mRNA is a nonsense-mediated mRNA decay target inefficiently spliced in renal disease. JCI Insight 2 (20), e94375. doi:10.1172/jci.insight.94375
Mencke, R., Olauson, H., and Hillebrands, J.-L. (2017b2017b). Effects of Klotho on fibrosis and cancer: A renal focus on mechanisms and therapeutic strategies. Adv. Drug Deliv. Rev. 121, 85–100. doi:10.1016/j.addr.2017.07.009
Mikuła-Pietrasik, J., Rutecki, S., and Książek, K. (2022). The functional multipotency of transforming growth factor β signaling at the intersection of senescence and cancer. Cell. Mol. Life Sci. 79 (4), 196. doi:10.1007/s00018-022-04236-y
Mizusaki, K., Hasuike, Y., Kimura, T., Nagasawa, Y., Kuragano, T., Yamada, Y., et al. (2019). Inhibition of the Mammalian Target of Rapamycin May Augment the Increase in Soluble Klotho Levels in Renal Transplantation Recipients. Blood Purif. 47 (Suppl. 2), 12–18. doi:10.1159/000496630
Mohammed, I., Hollenberg, M. D., Ding, H., and Triggle, C. R. (2021). A Critical Review of the Evidence That Metformin Is a Putative Anti-Aging Drug That Enhances Healthspan and Extends Lifespan. Front. Endocrinol. 12, 718942. doi:10.3389/fendo.2021.718942
Moreira, P. I. (2014). Metformin in the diabetic brain: friend or foe? Ann. Transl. Med. 2 (6), 54. doi:10.3978/j.issn.2305-5839.2014.06.10
Moreno, J. A., Izquierdo, M. C., Sanchez-Niño, M. D., Suárez-Alvarez, B., Lopez-Larrea, C., Jakubowski, A., et al. (2011). The Inflammatory Cytokines TWEAK and TNFα Reduce Renal Klotho Expression through NFκB. J Am Soc Nephrol 22 (7), 1315–1325. doi:10.1681/ASN.2010101073
Morishima, T., and Ochi, E. (2021). Impact of a single bout of resistance exercise on serum Klotho in healthy young men. Physiol. Rep. 9 (21), e15087. doi:10.14814/phy2.15087
Mullen, A. C., and Wrana, J. L. (2017). TGF-β Family Signaling in Embryonic and Somatic Stem-Cell Renewal and Differentiation. Cold Spring Harb. Perspect. Biol. 9 (7), a022186. doi:10.1101/cshperspect.a022186
Murphy, G. (2009). Regulation of the proteolytic disintegrin metalloproteinases, the 'Sheddases'. Seminars Cell. & Dev. Biol. 20 (2), 138–145. doi:10.1016/j.semcdb.2008.09.004
Mytych, J. (2022). Actions of Klotho on hippocampal neuronal cells. Vitam. Horm. 118, 223–246. doi:10.1016/bs.vh.2021.12.001
Myung, J., Beom, J.-H., Kim, J.-H., Woo, J.-S., Park, I., Chung, S.-P., et al. (2022). Recombinant Klotho Protein Ameliorates Myocardial Ischemia/Reperfusion Injury by Attenuating Sterile Inflammation. Biomedicines 10 (4), 894. doi:10.3390/biomedicines10040894
Narumiya, H., Sasaki, S., Kuwahara, N., Irie, H., Kusaba, T., Kameyama, H., et al. (2004). HMG-CoA reductase inhibitors up-regulate anti-aging klotho mRNA via RhoA inactivation in IMCD3 cells. Cardiovasc. Res. 64 (2), 331–336. doi:10.1016/j.cardiores.2004.07.011
Navarro-González, J. F., Sánchez-Niño, M. D., Donate-Correa, J., Martín-Núñez, E., Ferri, C., Pérez-Delgado, N., et al. (2018). Effects of Pentoxifylline on Soluble Klotho Concentrations and Renal Tubular Cell Expression in Diabetic Kidney Disease. Diabetes Care 41 (8), 1817–1820. doi:10.2337/dc18-0078
Neitzel, J., Franzmeier, N., Rubinski, A., Dichgans, M., Brendel, M., au, fnm, et al. (2021). KL-VS heterozygosity is associated with lower amyloid-dependent tau accumulation and memory impairment in Alzheimer's disease. Nat. Commun. 12 (1), 3825. doi:10.1038/s41467-021-23755-z
Neyra, J. A., Hu, M. C., and Moe, O. W. (2021). Klotho in Clinical Nephrology. J Am Soc Nephrol 16 (1), 162–176. doi:10.2215/CJN.02840320
Neyra, J. A., Moe, O. W., Pastor, J., Gianella, F., Sidhu, S. S., Sarnak, M. J., et al. (2020). Performance of soluble Klotho assays in clinical samples of kidney disease. Clin. Kidney J. 13 (2), 235–244. doi:10.1093/ckj/sfz085
Ni, W., Zhang, Y., and Yin, Z. (2021). The protective mechanism of Klotho gene-modified bone marrow mesenchymal stem cells on acute kidney injury induced by rhabdomyolysis. Regen. Ther. 18, 255–267. doi:10.1016/j.reth.2021.07.003
Nie, F., Wu, D., Du, H., Yang, X., Yang, M., Pang, X., et al. (2017). Serum klotho protein levels and their correlations with the progression of type 2 diabetes mellitus. J. Diabetes its Complicat. 31 (3), 594–598. doi:10.1016/j.jdiacomp.2016.11.008
Nilles, K. L., Williams, E. I., Betterton, R. D., Davis, T. P., and Ronaldson, P. T. (2022). Blood-Brain Barrier Transporters: Opportunities for Therapeutic Development in Ischemic Stroke. Ijms 23 (3), 1898. doi:10.3390/ijms23031898
Oh, H. J., Nam, B. Y., Wu, M., Kim, S., Park, J., Kang, S., et al. (2018). Klotho plays a protective role against glomerular hypertrophy in a cell cycle-dependent manner in diabetic nephropathy. Am. J. Physiology-Renal Physiology 315 (4), F791–F805. doi:10.1152/ajprenal.00462.2017
Ohrui, T., Matsui, T., Yamaya, M., Arai, H., Ebihara, S., Maruyama, M., et al. (2004). Angiotensin-converting enzyme inhibitors and incidence of Alzheimer's disease in Japan. J. Am. Geriatr. Soc. 52 (4), 649–650. doi:10.1111/j.1532-5415.2004.52178_7.x
Oishi, H., Doi, S., Nakashima, A., Ike, T., Maeoka, Y., Sasaki, K., et al. (2021). Klotho overexpression protects against renal aging along with suppression of transforming growth factor-β1 signaling pathways. Am. J. Physiology-Renal Physiology 321 (6), F799–F811. doi:10.1152/ajprenal.00609.2020
Olejnik, A., Franczak, A., Krzywonos-Zawadzka, A., Kałużna-Oleksy, M., and Bil-Lula, I. (2018). The Biological Role of Klotho Protein in the Development of Cardiovascular Diseases. BioMed Res. Int. 2018, 1–17. doi:10.1155/2018/5171945
Olejnik, A., Krzywonos‐Zawadzka, A., Banaszkiewicz, M., and Bil‐Lula, I. (2020). Klotho protein contributes to cardioprotection during ischaemia/reperfusion injury. J. Cell. Mol. Medi 24 (11), 6448–6458. doi:10.1111/jcmm.15293
Ostojic, S. M., and Engeset, D. (2021). Improving Brain Creatine Uptake by Klotho Protein Stimulation: Can Diet Hit the Big Time? Front. Nutr. 8, 795599. doi:10.3389/fnut.2021.795599
Palma, E., Ruffolo, G., Cifelli, P., Roseti, C., Vliet, E. A. v., and Aronica, E. (2018). Modulation of GABAA Receptors in the Treatment of Epilepsy. Cpd 23 (37), 5563–5568. doi:10.2174/1381612823666170809100230
Panda, H., Wen, H., Suzuki, M., and Yamamoto, M. (2022). Multifaceted Roles of the KEAP1-NRF2 System in Cancer and Inflammatory Disease Milieu. Antioxidants 11 (3), 538. doi:10.3390/antiox11030538
Pardridge, W. M. (2012). Drug transport across the blood-brain barrier. J. Cereb. Blood Flow. Metab. 32 (11), 1959–1972. doi:10.1038/jcbfm.2012.126
Pawlak, J. B., and Blobe, G. C. (2022). TGF ‐β superfamily co‐receptors in cancer. Dev. Dyn. 251 (1), 117–143. doi:10.1002/dvdy.338
Phan, P., Saikia, B. B., Sonnaila, S., Agrawal, S., Alraawi, Z., Kumar, T. K. S., et al. (2021). The Saga of Endocrine FGFs. Cells 10 (9), 2418. doi:10.3390/cells10092418
Pratsinis, H., Mavrogonatou, E., and Kletsas, D. (2017). “TGF-β in Development and Ageing,” in Hormones in Ageing and Longevity. Gewerbestrasse 11, 6330. Editor S. I. S. Rattan (Cham, Switzerland: Springer International Publishing AG), 127–148. doi:10.1007/978-3-319-63001-4_7
Prud’homme, G. J. (2012). Cancer stem cells and novel targets for antitumor strategies. Cpd 18 (19), 2838–2849. doi:10.2174/138161212800626120
Prud'homme, G. J., Glinka, Y., Kurt, M., Liu, W., and Wang, Q. (2017a). The anti-aging protein Klotho is induced by GABA therapy and exerts protective and stimulatory effects on pancreatic beta cells. Biochem. Biophysical Res. Commun. 493 (4), 1542–1547. doi:10.1016/j.bbrc.2017.10.029
Prud'homme, G. J. (2007). Pathobiology of transforming growth factor β in cancer, fibrosis and immunologic disease, and therapeutic considerations. Lab. Investig. 87 (11), 1077–1091. doi:10.1038/labinvest.3700669
Prud’homme, G. J., Glinka, Y., Hasilo, C., Paraskevas, S., Li, X., and Wang, Q. (2013). GABA protects human islet cells against the deleterious effects of immunosuppressive drugs and exerts immunoinhibitory effects alone. Transplantation 96 (7), 616–623. doi:10.1097/TP.0b013e31829c24be
Prud’homme, G. J., Glinka, Y., Kurt, M., Liu, W., and Wang, Q. (2020). Systemic Klotho therapy protects against insulitis and enhances beta-cell mass in NOD mice. Biochem. Biophysical Res. Commun. 525 (3), 693–698. doi:10.1016/j.bbrc.2020.02.123
Prud’homme, G. J., Glinka, Y., Matkar, P. N., and Leong-Poi, H. (2017b). “The Role of Neuropilins in TGF-β Signaling and Cancer Biology,” in The Neuropilins: Role and Function in Health and Disease. Editors G. Neufeld, and O. Kessler (Cham: Sprinter International Publishing AG), 187–212. doi:10.1007/978-3-319-48824-0_11
Prud’homme, G. J., and Glinka, Y. (2012). Neuropilins are multifunctional coreceptors involved in tumor initiation, growth, metastasis and immunity. Oncotarget 3 (9), 921–939. doi:10.18632/oncotarget.626
Pujadas, G., and Drucker, D. J. (2016). Vascular Biology of Glucagon Receptor Superfamily Peptides: Mechanistic and Clinical Relevance. Endocr. Rev. 37 (6), 554–583. doi:10.1210/er.2016-1078
Purwana, I., Zheng, J., Li, X., Deurloo, M., Son, D. O., Zhang, Z., et al. (2014). GABA Promotes Human β-Cell Proliferation and Modulates Glucose Homeostasis. Diabetes 63 (12), 4197–4205. doi:10.2337/db14-0153
Qu, Z., Sun, J., Zhang, W., Yu, J., and Zhuang, C. (2020). Transcription factor NRF2 as a promising therapeutic target for Alzheimer's disease. Free Radic. Biol. Med. 159, 87–102. doi:10.1016/j.freeradbiomed.2020.06.028
Rao, Y. L., Ganaraja, B., Murlimanju, B. V., Joy, T., Krishnamurthy, A., and Agrawal, A. (2022). Hippocampus and its involvement in Alzheimer's disease: a review. 3 Biotech. 12 (2), 55. doi:10.1007/s13205-022-03123-4
Rao, Z., Landry, T., Li, P., Bunner, W., Laing, B. T., Yuan, Y., et al. (2019). Administration of alpha klotho reduces liver and adipose lipid accumulation in obese mice. Heliyon 5 (4), e01494. doi:10.1016/j.heliyon.2019.e01494
Rausch, S., and Föller, M. (2022). The regulation of FGF23 under physiological and pathophysiological conditions. Pflugers Arch. - Eur. J. Physiol. 474 (3), 281–292. doi:10.1007/s00424-022-02668-w
Razzaque, M. S. (2012). The role of Klotho in energy metabolism. Nat. Rev. Endocrinol. 8 (10), 579–587. doi:10.1038/nrendo.2012.75
Reddy, P. H., Manczak, M., Yin, X., Grady, M. C., Mitchell, A., Tonk, S., et al. (2018). Protective Effects of Indian Spice Curcumin Against Amyloid-β in Alzheimer's Disease. Jad 61 (3), 843–866. doi:10.3233/JAD-170512
Rim, E. Y., Clevers, H., and Nusse, R. (2022). The Wnt Pathway: From Signaling Mechanisms to Synthetic Modulators. Annu. Rev. Biochem. 91. doi:10.1146/annurev-biochem-040320-103615
Roberti, A., Chaffey, L. E., and Greaves, D. R. (2022). NF-κB Signaling and Inflammation-Drug Repurposing to Treat Inflammatory Disorders? Biology 11 (3), 372. doi:10.3390/biology11030372
Rubinek, T., and Wolf, I. (2016). The Role of Alpha-Klotho as a Universal Tumor Suppressor. Vitam. Horm. 101, 197–214. doi:10.1016/bs.vh.2016.03.001
Ruoslahti, E. (2017). Tumor penetrating peptides for improved drug delivery. Adv. Drug Deliv. Rev. 110-111, 3–12. doi:10.1016/j.addr.2016.03.008
Saar-Kovrov, V., Donners, M. M. P. C., and van der Vorst, E. P. C. (2021). Shedding of Klotho: Functional Implications in Chronic Kidney Disease and Associated Vascular Disease. Front. Cardiovasc. Med. 7, 617842. doi:10.3389/fcvm.2020.617842
Sachdeva, A., Gouge, J., Kontovounisios, C., Nikolaou, S., Ashworth, A., Lim, K., et al. (2020). Klotho and the Treatment of Human Malignancies. Cancers 12 (6), 1665. doi:10.3390/cancers12061665
Sanz, B., Arrieta, H., Rezola-Pardo, C., Fernández-Atutxa, A., Garin-Balerdi, J., Arizaga, N., et al. (2021). Low serum klotho concentration is associated with worse cognition, psychological components of frailty, dependence, and falls in nursing home residents. Sci. Rep. 11 (1), 9098. doi:10.1038/s41598-021-88455-6
Sarang, S. S., Lukyanova, S. M., Brown, D. D., Cummings, B. S., Gullans, S. R., and Schnellmann, R. G. (2008). Identification, Coassembly, and Activity of γ-Aminobutyric Acid Receptor Subunits in Renal Proximal Tubular Cells. J. Pharmacol. Exp. Ther. 324 (1), 376–382. doi:10.1124/jpet.107.129957
Sarnobat, D., Charlotte Moffett, R., Flatt, P. R., Irwin, N., and Tarasov, A. I. (2022). GABA and insulin but Not nicotinamide augment α- to β-cell transdifferentiation in insulin-deficient diabetic mice. Biochem. Pharmacol. 199, 115019. doi:10.1016/j.bcp.2022.115019
Sasaki, S., Tohda, C., Kim, M., and Yokozawa, T. (2007). .GAMMA.-Aminobutyric Acid Specifically Inhibits Progression of Tubular Fibrosis and Atrophy in Nephrectomized Rats. Biol. Pharm. Bull. 30 (4), 687–691. doi:10.1248/bpb.30.687
Semba, R. D., Moghekar, A. R., Hu, J., Sun, K., Turner, R., Ferrucci, L., et al. (2014). Klotho in the cerebrospinal fluid of adults with and without Alzheimer's disease. Neurosci. Lett. 558, 37–40. doi:10.1016/j.neulet.2013.10.058
Shardell, M., Semba, R. D., Rosano, C., Kalyani, R. R., Bandinelli, S., Chia, C. W., et al. (2016). Plasma Klotho and Cognitive Decline in Older Adults: Findings From the InCHIANTI Study. Gerona 71 (5), 677–682. doi:10.1093/gerona/glv140
Shen, D., Li, H., Zhou, R., Liu, M.-j., Yu, H., and Wu, D.-F. (2018). Pioglitazone attenuates aging-related disorders in aged apolipoprotein E deficient mice. Exp. Gerontol. 102, 101–108. doi:10.1016/j.exger.2017.12.002
Shin, Y. J., Luo, K., Quan, Y., Ko, E. J., Chung, B. H., Lim, S. W., et al. (2019). Therapeutic Challenge of Minicircle Vector Encoding Klotho in Animal Model. Am. J. Nephrol. 49 (5), 413–424. doi:10.1159/000499863
Soltani, N., Qiu, H., Aleksic, M., Glinka, Y., Zhao, F., Liu, R., et al. (2011). GABA exerts protective and regenerative effects on islet beta cells and reverses diabetes. Proc. Natl. Acad. Sci. U.S.A. 108 (28), 11692–11697. doi:10.1073/pnas.1102715108
Son, D. O., Liu, W., Li, X., Prud'homme, G. J., and Wang, Q. (2019). Combined effect of GABA and glucagon‐like peptide‐1 receptor agonist on cytokine‐induced apoptosis in pancreatic β‐cell line and isolated human islets. J. Diabetes 11 (7), 563–572. doi:10.1111/1753-0407.12881
Soo, S. K., Rudich, P. D., Traa, A., Harris-Gauthier, N., Shields, H. J., and Van Raamsdonk, J. M. (2020). Compounds that extend longevity are protective in neurodegenerative diseases and provide a novel treatment strategy for these devastating disorders. Mech. Ageing Dev. 190, 111297. doi:10.1016/j.mad.2020.111297
Sosa-Pineda, B., Chowdhury, K., Torres, M., Oliver, G., and Gruss, P. (1997). The Pax4 gene is essential for differentiation of insulin-producing β cells in the mammalian pancreas. Nature 386 (6623), 399–402. doi:10.1038/386399a0
Tabibzadeh, S. (2021). Signaling pathways and effectors of aging. Front. Biosci. 26 (1), 50–96. doi:10.2741/4889
Takano, K., Yatabe, M. S., Abe, A., Suzuki, Y., Sanada, H., Watanabe, T., et al. (2014). Characteristic expressions of GABA receptors and GABA producing/transporting molecules in rat kidney. PLoS One 9 (9), e105835. doi:10.1371/journal.pone.0105835
Takenaka, T., Inoue, T., Miyazaki, T., Kobori, H., Nishiyama, A., Ishii, N., et al. (2018). Klotho Ameliorates Medullary Fibrosis and Pressure Natriuresis in Hypertensive Rat Kidneys. Hypertension 72 (5), 1151–1159. doi:10.1161/HYPERTENSIONAHA.118.11176
Takenaka, T., Inoue, T., Miyazaki, T., Kobori, H., Nishiyama, A., Ishii, N., et al. (2017). Klotho suppresses the renin-angiotensin system in adriamycin nephropathy. Nephrol. Dial. Transpl. 32 (5), gfw340–800. doi:10.1093/ndt/gfw340
Takenaka, T., Kobori, H., Inoue, T., Miyazaki, T., Suzuki, H., Nishiyama, A., et al. (2020). Klotho supplementation ameliorates blood pressure and renal function in DBA/2-pcy mice, a model of polycystic kidney disease. Am. J. Physiology-Renal Physiology 318 (3), F557–F564. doi:10.1152/ajprenal.00299.2019
Takenaka, T., Kobori, H., Miyazaki, T., Suzuki, H., Nishiyama, A., Ishii, N., et al. (2019). Klotho protein supplementation reduces blood pressure and renal hypertrophy in db/db mice, a model of type 2 diabetes. Acta Physiol. 225 (2), e13190. doi:10.1111/apha.13190
Takeshita, A., Kawakami, K., Furushima, K., Miyajima, M., and Sakaguchi, K. (2018). Central role of the proximal tubular αKlotho/FGF receptor complex in FGF23-regulated phosphate and vitamin D metabolism. Sci. Rep. 8 (1), 6917. doi:10.1038/s41598-018-25087-3
Tan, S.-J., Chu, M. M., Toussaint, N. D., Cai, M. M., Hewitson, T. D., and Holt, S. G. (2018). High-intensity physical exercise increases serum α-klotho levels in healthy volunteers. J. Circulating Biomarkers 7, 184945441879458. doi:10.1177/1849454418794582
Tarhani, F., Heidari, G., and Nezami, A. (2020). Evaluation of α-klotho level in insulin dependent diabetes mellitus (IDDM) children. J. Pediatr. Endocrinol. Metab. 33 (6), 761–765. doi:10.1515/jpem-2019-0591
Tasnim, N., Dutta, P., Nayeem, J., Masud, P., Ferdousi, A., Ghosh, A. S., et al. (2021). Osteoporosis, an Inevitable Circumstance of Chronic Kidney Disease: A Systematic Review. Cureus 13 (10), e18488. doi:10.7759/cureus.18488
Tataranni, T., Biondi, G., Cariello, M., Mangino, M., Colucci, G., Rutigliano, M., et al. (2011). Rapamycin-induced hypophosphatemia and insulin resistance are associated with mTORC2 activation and Klotho expression. Am. J. Transpl. 11 (8), 1656–1664. doi:10.1111/j.1600-6143.2011.03590.x
Thal, D. R., von Arnim, C., Griffin, W. S. T., Yamaguchi, H., Mrak, R. E., Attems, J., et al. (2013). Pathology of clinical and preclinical Alzheimer's disease. Eur. Arch. Psychiatry Clin. Neurosci. 263 (Suppl. 2), 137–145. doi:10.1007/s00406-013-0449-5
Tian, J., Dang, H., Chen, Z., Guan, A., Jin, Y., Atkinson, M. A., et al. (2013). γ-Aminobutyric Acid Regulates Both the Survival and Replication of Human β-Cells. Diabetes 62 (11), 3760–3765. doi:10.2337/db13-0931
Tian, J., Lu, Y., Zhang, H., Chau, C. H., Dang, H. N., and Kaufman, D. L. (2004). γ-Aminobutyric Acid Inhibits T Cell Autoimmunity and the Development of Inflammatory Responses in a Mouse Type 1 Diabetes Model. J. Immunol. 173 (8), 5298–5304. doi:10.4049/jimmunol.173.8.5298
Tominaga, K., and Suzuki, H. I. (2019). TGF-β Signaling in Cellular Senescence and Aging-Related Pathology. Ijms 20 (20), 5002. doi:10.3390/ijms20205002
Tsujikawa, H., Kurotaki, Y., Fujimori, T., Fukuda, K., and Nabeshima, Y.-I. (2003). Klotho, a gene related to a syndrome resembling human premature aging, functions in a negative regulatory circuit of vitamin D endocrine system. Mol. Endocrinol. 17 (12), 2393–2403. doi:10.1210/me.2003-0048
Typiak, M., and Piwkowska, A. (2021). Antiinflammatory Actions of Klotho: Implications for Therapy of Diabetic Nephropathy. Ijms 22 (2), 956. doi:10.3390/ijms22020956
Tyurenkov, I. N., Perfilova, V. N., Nesterova, A. A., and Glinka, Y. (2021). Klotho Protein and Cardio-Vascular System. Biochem. Mosc. 86 (2), 132–145. doi:10.1134/S0006297921020024
Untereiner, A., Abdo, S., Bhattacharjee, A., Gohil, H., Pourasgari, F., Ibeh, N., et al. (2019). GABA promotes β‐cell proliferation, but does Not overcome impaired glucose homeostasis associated with diet‐induced obesity. FASEB J. 33 (3), 3968–3984. doi:10.1096/fj.201801397R
Urakawa, I., Yamazaki, Y., Shimada, T., Iijima, K., Hasegawa, H., Okawa, K., et al. (2006). Klotho converts canonical FGF receptor into a specific receptor for FGF23. Nature 444 (7120), 770–774. doi:10.1038/nature05315
van Loon, E. P. M., Pulskens, W. P., van der Hagen, E. A. E., Lavrijsen, M., Vervloet, M. G., van Goor, H., et al. (2015). Shedding of klotho by ADAMs in the kidney. Am. J. Physiology-Renal Physiology 309 (4), F359–F368. doi:10.1152/ajprenal.00240.2014
Veronesi, F., Borsari, V., Cherubini, A., and Fini, M. (2021). Association of Klotho with physical performance and frailty in middle-aged and older adults: A systematic review. Exp. Gerontol. 154, 111518. doi:10.1016/j.exger.2021.111518
Vo, H. T., Laszczyk, A. M., and King, G. D. (2018). Klotho, the Key to Healthy Brain Aging? Bpl 3 (2), 183–194. doi:10.3233/BPL-170057
Wan, Q., He, Y., and Yuan, M. (2017). Klotho in diabetes and diabetic nephropathy: a brief update review. Int. J. Clin. Exp. Med. 10 (3), 4342–4349. Available at: www.ijcem.com/ISSN:1940-5901/IJCEM0044613.
Wang, F., and Zheng, J. (2022). Association between serum alpha-Klotho and severe abdominal aortic calcification among civilians in the United States. Nutr. Metabolism Cardiovasc. Dis. 32 (22), 00106–01492. doi:10.1016/j.numecd.2022.02.017
Wang, Q., Prud'homme, G., and Wan, Y. (2015). GABAergic system in the endocrine pancreas: a new target for diabetes treatment. Dmso 8, 79–87. doi:10.2147/DMSO.S50642
Wang, Q., Ren, L., Wan, Y., and Prud'homme, G. J. (2019). GABAergic regulation of pancreatic islet cells: Physiology and antidiabetic effects. J. Cell. Physiology 234 (9), 14432–14444. doi:10.1002/jcp.28214
Wang, Y., and Sun, Z. (2009). Current understanding of klotho. Ageing Res. Rev. 8 (1), 43–51. doi:10.1016/j.arr.2008.10.002
Wolf, I., Levanon-Cohen, S., Bose, S., Ligumsky, H., Sredni, B., Kanety, H., et al. (2008). Klotho: A Tumor Suppressor and a Modulator of the IGF-1 and FGF Pathways in Human Breast Cancer. Oncogene 27 (56), 7094–7105. doi:10.1038/onc.2008.292
Wood, W. G., Eckert, G. P., Igbavboa, U., and Müller, W. E. (2010). Statins and Neuroprotection: A Prescription to Move the Field Forward. Ann. N. Y. Acad. Sci. 1199, 69–76. doi:10.1111/j.1749-6632.2009.05359.x
Wu, S.-E., and Chen, W.-L. (2022). Soluble klotho as an effective biomarker to characterize inflammatory states. Ann. Med. 54 (1), 1520–1529. doi:10.1080/07853890.2022.2077428
Xiang, T., Luo, X., Ye, L., Huang, H., and Wu, Y. (2022). Klotho alleviates NLRP3 inflammasome-mediated neuroinflammation in a temporal lobe epilepsy rat model by activating the Nrf2 signaling pathway. Epilepsy & Behav. 128, 108509. doi:10.1016/j.yebeh.2021.108509
Xiang, T., Luo, X., Zeng, C., Li, S., Ma, M., and Wu, Y. (2021). Klotho ameliorated cognitive deficits in a temporal lobe epilepsy rat model by inhibiting ferroptosis. Brain Res. 1772, 147668. doi:10.1016/j.brainres.2021.147668
Xie, B., Zhou, J., Shu, G., Liu, D.-c., Zhou, J., Chen, J., et al. (2013). Restoration of klotho gene expression induces apoptosis and autophagy in gastric cancer cells: tumor suppressive role of klotho in gastric cancer. Cancer Cell. Int. 13 (1), 18. doi:10.1186/1475-2867-13-18
Xing, L., Guo, H., Meng, S., Zhu, B., Fang, J., Huang, J., et al. (2021). Klotho ameliorates diabetic nephropathy by activating Nrf2 signaling pathway in podocytes. Biochem. Biophysical Res. Commun. 534, 450–456. doi:10.1016/j.bbrc.2020.11.061
Xu, Y., and Sun, Z. (2015). Molecular basis of Klotho: from gene to function in aging. Endocr. Rev. 36 (2), 174–193. doi:10.1210/er.2013-1079
Xue, J., Wang, L., Sun, Z., and Xing, C. (2019). Basic Research in Diabetic Nephropathy Health Care: A study of the Renoprotective Mechanism of Metformin. J. Med. Syst. 43 (8), 266. doi:10.1007/s10916-019-1412-4
Yamamoto, M., Clark, J. D., Pastor, J. V., Gurnani, P., Nandi, A., Kurosu, H., et al. (2005). Regulation of oxidative stress by the anti-aging hormone klotho. J. Biol. Chem. 280 (45), 38029–38034. doi:10.1074/jbc.M509039200
Yang, K., Nie, L., Huang, Y., Zhang, J., Xiao, T., Guan, X., et al. (2012). Amelioration of uremic toxin indoxyl sulfate-induced endothelial cell dysfunction by Klotho protein. Toxicol. Lett. 215 (2), 77–83. doi:10.1016/j.toxlet.2012.10.004
Yang, K., Wang, C., Nie, L., Zhao, X., Gu, J., Guan, X., et al. (2015). Zhao J Klotho Protects Against Indoxyl Sulphate-Induced Myocardial Hypertrophy. J. Am. Soc. Nephrol. 26 (10), 2434–2446. doi:10.1681/ASN.2014060543
Yoon, H. E., Ghee, J. Y., Piao, S., Song, J. H., Han, D. H., Kim, S., et al. (2011). Angiotensin II blockade upregulates the expression of Klotho, the anti-ageing gene, in an experimental model of chronic cyclosporine nephropathy. Nephrol. Dial. Transpl. 26 (3), 800–813. doi:10.1093/ndt/gfq537
Yossef, R. R., Al-Yamany, M. F., Saad, M. A., and El-Sahar, A. E. (2020). Neuroprotective Effects of Vildagliptin on Drug Induced Alzheimer’s Disease in Rats With Metabolic Syndrome: Role of Hippocampal Klotho and AKT Signaling Pathways. Eur. J. Pharmacol. 889, 173612. doi:10.1016/j.ejphar.2020.173612
Yoon, H. E., Lim, S. W., Piao, S. G., Song, J. H., Kim, J., and Yang, C. W. (2012). Statin upregulates the expression of klotho, an anti-aging gene, in experimental cyclosporine nephropathy. Nephron Exp. Nephrol. 120 (4), e123–33. doi:10.1159/000342117
Yuan, Q., Ren, Q., Li, L., Tan, H., Lu, M., Tian, Y., et al. (2022). A Klotho-derived peptide protects against kidney fibrosis by targeting TGF-beta signaling. Nat. Commun. 13 (1), 438. doi:10.1038/s41467-022-28096-z
Zeldich, E., Chen, C. D., Colvin, T. A., Bove-Fenderson, E. A., Liang, J., Tucker Zhou, T. B., et al. (2014). The neuroprotective effect of Klotho is mediated via regulation of members of the redox system. J. Biol. Chem. 289 (35), 24700–24715. [. doi:10.1074/jbc.M114.567321
Zeng, C. Y., Yang, T. T., Zhou, H. J., Zhao, Y., Kuang, X., Duan, W., et al. (2019). Lentiviral vector-mediated overexpression of Klotho in the brain improves Alzheimer's disease-like pathology and cognitive deficits in mice. Neurobiol. Aging 78, 18–28. doi:10.1016/j.neurobiolaging.2019.02.003
Zhang, H., Li, Y., Fan, Y., Wu, J., Zhao, B., Guan, Y., et al. (2008). Klotho is a target gene of PPAR-gamma. Kidney Int. 74 (6), 732–739. doi:10.1038/ki.2008.244
Zhang, L., and Liu, T. (2018). Clinical implication of alterations in serum Klotho levels in patients with type 2 diabetes mellitus and its associated complications. J. Diabetes Complicat. 32 (10), 922–930. doi:10.1016/j.jdiacomp.2018.06.002
Zhang, P., Li, Y., Du, Y., Li, G., Wang, L., and Zhou, F. (2016). Resveratrol Ameliorated Vascular Calcification by Regulating Sirt-1 and Nrf2. Transpl. Proc. 48 (10), 3378–3386. doi:10.1016/j.transproceed.2016.10.023
Zhang, T., Ma, C., Zhang, Z., Zhang, H., and Hu, H. (2020). NF-kappaB signaling in inflammation and cancer. MedComm 2 (4), 618–653. doi:10.1002/mco2.104
Zhang, X. T., Wang, G., Ye, L. F., Pu, Y., Li, R. T., Liang, J., et al. (2020). Baicalin reversal of DNA hypermethylation-associated Klotho suppression ameliorates renal injury in type 1 diabetic mouse model. Cell. Cycle 19 (23), 3329–3347. doi:10.1080/15384101.2020.1843815
Zhao, L., Chen, H., Lu, L., Zhao, C., Malichewe, C. V., Wang, L., et al. (2021). Design and screening of a novel neuropilin-1 targeted penetrating peptide for anti-angiogenic therapy in glioma. Life Sci. 270, 119113. doi:10.1016/j.lfs.2021.119113
Zhao, Y., Banerjee, S., Dey, N., LeJeune, W. S., Sarkar, P. S., Brobey, R., et al. (2011). Klotho depletion contributes to increased inflammation in kidney of the db/db mouse model of diabetes via RelA (serine)536 phosphorylation. Diabetes 60 (7), 1907–1916. doi:10.2337/db10-1262
Zhao, Y., Zhao, M. M., Cai, Y., Zheng, M. F., Sun, W. L., Zhang, S. Y., et al. (2015). Mammalian target of rapamycin signaling inhibition ameliorates vascular calcification via Klotho upregulation. Kidney Int. 88 (4), 711–721. doi:10.1038/ki.2015.160
Zhao, Y. N., Shao, X., Ouyang, L. F., Chen, L., and Gu, L. (2018). Qualitative detection of ginsenosides in brain tissues after oral administration of high-purity ginseng total saponins by using polyclonal antibody against ginsenosides. Chin. J. Nat. Med. 16 (3), 175–183. doi:10.1016/S1875-5364(18)30045-1
Zhong, X., Jagarlapudi, S., Weng, Y., Ly, M., Rouse, J. C., McClure, K., et al. (2020). Structure-function relationships of the soluble form of the antiaging protein Klotho have therapeutic implications for managing kidney disease. J. Biol. Chem. 295 (10), 3115–3133. doi:10.1074/jbc.RA119.012144
Zhou, Q., Lin, S., Tang, R., Veeraragoo, P., Peng, W., and Wu, R. (2010). Role of Fosinopril and Valsartan on Klotho Gene Expression Induced by Angiotensin II in Rat Renal Tubular Epithelial Cells. Kidney Blood Press Res. 33 (3), 186–192. doi:10.1159/000316703
Zhou, W., Chen, M. M., Liu, H. L., Si, Z. L., Wu, W. H., Jiang, H., et al. (2022). Dihydroartemisinin suppresses renal fibrosis in mice by inhibiting DNA-methyltransferase 1 and increasing Klotho. Acta Pharmacol. Sin. doi:10.1038/s41401-022-00898-3
Zhou, X., Yang, Q., Xie, Y., Sun, J., Hu, J., Qiu, P., et al. (2015). Tetrahydroxystilbene glucoside extends mouse life span via upregulating neural klotho and downregulating neural insulin or insulin-like growth factor 1. Neurobiol. Aging 36 (3), 1462–1470. doi:10.1016/j.neurobiolaging.2014.11.002
Zhu, H., Gao, Y., Zhu, S., Cui, Q., and Du, J. (2017). Klotho Improves Cardiac Function by Suppressing Reactive Oxygen Species (ROS) Mediated Apoptosis by Modulating Mapks/Nrf2 Signaling in Doxorubicin-Induced Cardiotoxicity. Med. Sci. Monit. 23, 5283–5293. doi:10.12659/msm.907449
Zou, D., Wu, W., He, Y., Ma, S., and Gao, J. (2018). The role of klotho in chronic kidney disease. BMC Nephrol. 19 (1), 285. doi:10.1186/s12882-018-1094-z
Keywords: aging, FGF23, hyperphosphatemia, klotho, IGF-1, NF-KappaB, TGF-beta, Wnt
Citation: Prud’homme GJ, Kurt M and Wang Q (2022) Pathobiology of the Klotho Antiaging Protein and Therapeutic Considerations. Front. Aging 3:931331. doi: 10.3389/fragi.2022.931331
Received: 28 April 2022; Accepted: 06 June 2022;
Published: 12 July 2022.
Edited by:
Cátia F. Lourenço, University of Coimbra, PortugalReviewed by:
Mujib Ullah, Stanford University, United StatesTaylor Landry, East Carolina University, United States
Copyright © 2022 Prud’homme, Kurt and Wang. This is an open-access article distributed under the terms of the Creative Commons Attribution License (CC BY). The use, distribution or reproduction in other forums is permitted, provided the original author(s) and the copyright owner(s) are credited and that the original publication in this journal is cited, in accordance with accepted academic practice. No use, distribution or reproduction is permitted which does not comply with these terms.
*Correspondence: Gérald J. Prud’homme, prudhommegj@gmail.com